- 1Centre for Interdisciplinary Research in Space (CIRiS), NTNU Social Research, Trondheim, Norway
- 2Department of Agricultural Sciences, University of Naples Federico II, Portici, Italy
- 3EnginSoft Società per Azioni, Bergamo, Italy
In situ production of food, water and oxygen is essential for long-duration human space missions. Higher plants represent a key element in Bioregenerative Life Support Systems (BLSS), where crop cultivation can be based on water and nutrients recovered from waste and wastewater. Human urine exemplifies an important waste stream with potential to provide crops with nitrogen (N) and other nutrients. Dynamic waste composition and treatment processes may result in mineralized fractions with varying ammonium (NH4+) to nitrate (NO3-) ratios. In this study, lettuce was cultivated in the unique ESA MELiSSA Plant Characterization Unit, an advanced, gas-tight hydroponic research facility offering controlled environment and continuous monitoring of atmospheric gas composition. To evaluate biological and system effects of nutrient solution NH4+:NO3- ratio, two crop tests were run with different NH4+ to total N ratio (NH4+:N) and elevated concentrations of Na+ and Cl- in line with a urine recycling scenario. Plants cultivated at 0.5 mol·mol-1 NH4+:N (HiNH4+) achieved 50% lower shoot biomass compared to those cultivated at 0.1 mol·mol-1 NH4+:N (LoNH4+), accompanied by higher shoot dry weight content and lower harvest index. Analyses of projected leaf area over time indicated that the reduced biomass observed at harvest could be attributed to a lower specific growth rate during the close-to-exponential growth phase. The HiNH4+ crop produced 40% less O2 over the full cultivation period. However, normalization of the results indicated a marginal increase in O2 production per time and per projected leaf area for the HiNH4+ crop during the exponential growth phase, in line with a higher shoot chlorophyll content. Mineral analysis demonstrated that the biomass content of NH4+ and NO3- varied in line with the nutrient solution composition. The ratio of consumed NH4+ to consumed N was higher than the NH4+:N ratio of the nutrient solution for both crop tests, resulting in decreasing NH4+:N ratios in the nutrient solution over time. The results provide enhanced insight for design of waste processes and crop cultivation to optimize overall BLSS efficiency and hold valuable potential for improved resource utilization also in terrestrial food production systems.
1 Introduction
Human spaceflight missions to remote locations call for on-site food production and resource recirculation. In contrast to Low Earth Orbit, complete resupply of resources from Earth is challenged by increased mission duration and travel distances. Higher plants represent a key element for regeneration of air, water and food for astronauts (Wheeler et al., 2002; Pannico et al., 2022), either as part of stand-alone Bioregenerative Life Support Systems (BLSS), or in a combination with physicochemical methods. The MELiSSA project (Micro Ecological Life Support System Alternative) is an international collaboration led by the European Space Agency (ESA), emphasizing the development of a BLSS to support long-term space missions. In an integrated loop, waste streams from a crew compartment are to be processed by thermophilic, heterotrophic and nitrifying bacteria to provide input to photosynthetic compartments with higher plants and algae to regenerate mineralized nutrients and carbon dioxide (CO2), and produce food, pure water, and oxygen (O2) for the crew (Godia et al., 2002). An important objective of the MELiSSA project is to develop mathematical models that can predict critical processes such as plant growth, photosynthesis, and transpiration, based on parameters such as plant species, cultivation conditions, and the processed waste stream fed to the plants.
In a scenario of crop cultivation based on mineralized human waste, urine is a valuable resource due to its high nitrogen (N) content. N is an essential element and a critical input factor for crop cultivation, with profound effects on plant growth and development as a constituent of numerous vital compounds such as nucleic acids, chlorophyll, amino acids, proteins, ATP, phytohormones, auxin and cytokinins (Marschner and Marschner, 2012; Taiz, 2015; De Bang et al., 2021). The use of recycled organic waste for crop cultivation typically requires mineralization of organic compounds by physicochemical and/or microbial processes. N is primarily taken up by plant roots as inorganic ammonium (NH4+) and nitrate (NO3-) (Marschner and Marschner, 2012; Taiz, 2015), that may be derived from urine. While fresh urine is rich in urea-N (up to 12 g L-1), this compound is rapidly hydrolyzed upon storage in a non-sterile environment to NH4+/NH3 that may be further converted to NO3- by physicochemical and/or microbial processes (Udert et al., 2006; Larsen et al., 2021). As the result of this upstream treatment depends on the mineralization strategy and the process conditions, the resulting nutrient solution provided to the plants may vary in the absolute concentrations of NH4+ and NO3- and the ratio between them. This may in turn affect not only the plant uptake rates of these inorganic N species, but also other physiological and metabolic processes such as uptake of other nutrients, enzyme activity, photosynthesis and respiration, water balance, signaling pathways, leaf expansion, and root architecture - eventually influencing the overall plant growth and crop yield (Guo et al., 2007a; Guo et al., 2007c; Andrews et al., 2013; Liu and Von Wiren, 2017). A nutrient solution containing both NH4+ and NO3- is typically preferred to optimize plant growth and development rather than using either NO3- or NH4+ as the sole source of N, although the use of NH4+ as the dominant N source should be avoided to reduce risk of ammonium toxicity and reduced plant growth (Roosta and Schjoerring, 2008; Taiz, 2015; Song et al., 2021; Weil et al., 2021; Hameed et al., 2022). Beyond N, urine contains other plant macronutrients such as potassium, phosphorus, sulfur, and lower levels of calcium and magnesium (Udert et al., 2006; Larsen et al., 2021). On the other hand, feeding crops with processed urine introduces non-essential elements such as sodium (Na+) and chloride (Cl-). Plants naturally accumulate salts, and the presence of Na+ and Cl- in the nutrient solution may affect plant physiology and growth. At some concentrations, NaCl has been demonstrated to act as a eustressor with beneficial effects on crop quality (Kronzucker et al., 2013; Rouphael et al., 2018). However, high concentrations of salt is among the most limiting factors for plant growth and may cause injurious abiotic stress, altering morphological and physiological plant traits and ultimately reducing crop yield (Chinnusamy and Zhu, 2006; Acosta-Motos et al., 2017).
To make possible a new generation of scientific studies related to the higher plant compartment, one of the most complex compartments in the MELiSSA loop, a Plant Characterization Unit (PCU) was recently designed and assembled on the premises of Department of Agricultural Sciences of University of Naples Federico II in Italy as part of the ESA MELiSSA PaCMan (PlAnt Characterization unit for closed life support system – engineering, MANufacturing & testing) activity (Pannico et al., 2022). The PCU is a gas-tight cultivation chamber for characterization of higher plants, offering extensive monitoring and control of the cultivation environment, including its separated hydroponic and atmospheric loops.
Lettuce is a highly relevant species for bioregenerative life support systems with high harvest index, efficiency (per area, time, and volume), and potential yield (edible biomass, O2 and water), combined with relatively modest horticultural requirements (Berkovich et al., 2004). Lettuce has been successfully cultivated onboard the International Space Station (Khodadad et al., 2020) and represents a healthy and nutritious addition to the human diet, rich in vitamin C, antioxidants, polyphenols and dietary fiber (Khodadad et al., 2020). Furthermore, it is one of the most used vegetables in terrestrial hydroponic cultivation systems (Zhu et al., 2020), illustrating the benefits of a deeper understanding of plant responses to nutrient solutions derived from organic waste in commercial crop production also on Earth, with the potential to improve resource utilization and reduce environmental impact of industrial food production systems.
The underlying hypotheses and scenario of this study was that strategies and operating conditions of upstream waste processing will affect the composition of the mineralized nutrients fed to the plants in a life support system. As these strategies and conditions may be designed and controlled, but also give rise to fluctuations and dynamics, it is critical to understand and predict consequences of nutrient solution composition on crop cultivation. More specifically, urine ammonification and nitrification may result in nutrient solutions with varying concentrations of NH4+ and NO3-, including their relative ratio, which will impact downstream crop cultivation. Furthermore, beyond the incoming nutrient streams, nutrient utilization rates inside the plant compartment will dictate the evolution of the nutrient solution in a closed-loop scenario with complete recycling of water, nutrients and non-nutrients. This study aimed at investigating biological and system effects of high and low NH4+:N ratios on lettuce cultivated in a urine recycling scenario, exploiting the recently established PCU facility offering controlled hydroponic cultivation conditions and state of the art monitoring of critical parameters such as O2 production.
2 Materials and methods
2.1 Lettuce seedling production
About 100 lettuce seeds (Lactuca sativa L. cultivar ‘Grand Rapids’) were disinfected in 5% sodium hypochlorite solution for 15 minutes followed by three rinsing cycles in ultrapure water. The disinfected seeds were dispersed onto absorbent paper, moist with ultrapure water and incubated at room temperature under indirect lighting. After 48 hours, germinated seeds with about 1 cm radicle were transferred to Styrofoam trays with vermiculite soaked in nutrient solution prepared according to Peiro et al. (2020) at 0.5X strength, and incubated at 24°C in a dedicated nursery with 65% relative humidity (RH), a light/dark regime of 16/8 hours and a light intensity of 400 ± 50 μmol·m–2·s–1 (PPFD). Ten days after sowing, 18 equally developed seedlings (evaluated by visual inspection) were selected from the pool of 100 germinated seeds. Each seedling was installed into a bottomless 50 mL test tube (CLS430829, Corning, NY, USA) filled with rockwool (Delta 4G 42/40, Grodan, Roermond, The Netherlands) cut into a cylindrical shape and vertically split in two. The seedling’s root system was sandwiched between the two halves of the rockwool column and inserted into the 50 mL tube. The top surface of the rockwool was then covered with grafting mastic (Cortimax, Cisa Adriatica S.r.l., Pescara, Italy) to minimize gas exchange between the root and shoot zones of the cultivation chamber.
2.2 Cultivation chamber and cultivation conditions
For each of the two crop tests (designated HiNH4+ and LoNH4+), 18 lettuce plants were cultivated inside the PCU. Within one crop test, all plants shared the same nutrient solution (recirculated within the PCU’s hydroponic loop) and the same atmosphere (recirculated within the PCU’s atmospheric loop). The plants were cultivated for 28 days inside the PCU chamber to a total plant age of 38 days. The day/night cycle was 16/8 hours, with an average light intensity across the plant positions of 430 µmol·m-2·s-1 (PPFD represented by 23% blue, 20% green and 57% red light). PPFD and spectral composition were recorded by eighteen spectral scans (one per plant position, measured at a height of 3 cm above the medium surface) using a spectral radiometer (MSC15, Gigahertz-Optik, Türkenfeld, Germany). The largest deviation between this average light intensity and the intensity at any of the plant positions was 68 µmol·m-2·s-1 (PPFD), corresponding to 16% of the average intensity. The air temperature and RH were automatically controlled as described by Pannico et al. (2022), at 26°C and 50% during the day and 20°C and 70% during the night, respectively. The atmospheric CO2 concentration was controlled at 1000 ppmv (parts per million by volume) by automatic injection of pure CO2 during the day phase, while it was allowed to increase during the night phase due to respiration. Atmospheric O2 concentration started at ambient levels and increased based on plant photosynthesis. The atmospheric O2 concentration was not controlled beyond one chamber venting per crop test (Day 19) to avoid O2 levels rising above 25%.
2.3 Nutrient solution preparation, monitoring, and maintenance
The nutrient solutions were based on the recipe described by Peiro et al. (2020) with the exception of i) the NH4+:N ratio was either reduced (LoNH4+ crop test) or increased (HiNH4+ crop test) using SO42- as counter-ion for required charge balancing, and ii) Na+ and Cl- levels were increased (both crop tests). Identification of prioritized nutrient solutions for the two PCU crop tests was based on preliminary deep water crop cultivation experiments with various NH4+:N ratios (0.00, 0.11, 0.22, 0.44, 0.50 mol·mol-1), nutrient solution strengths (0.75, 1.00, 1.25 X) and NaCl concentrations (0, 5 mM). PCU crop cultivation conditions were selected aiming at the presence of NaCl (5 mM) to mimic a urine recycling scenario (see Results section), reduced nutrient solution strength (0.75 X) to minimize negative effects of elevated total ionic strength (maintaining same electrical conductivity for the LoNH4+ crop test as for the nutrient solution of Peiro et al. (2020), 1.9 mS/cm), and two NH4+:N ratios representing considerable differences (high and low) while still providing acceptable plant growth conditions and representing relevant degrees of nitrification in a BLSS perspective (see Results section). The nutrient solutions of the LoNH4+ and HiNH4+ crop tests were obtained by mixing the following A and B stock solutions made from reverse osmosis water: LoNH4+ Stock A consisted of 434 mM Ca(NO3)2, 90 mM CaCl2, 9.6 mM FeCl3 and 12 mM Na-EDTA. HiNH4+ Stock A consisted of 213 mM Ca(NO3)2, 311 mM CaCl2, 9.6 mM FeCl3 and 12 mM Na-EDTA. LoNH4+ Stock B consisted of 120 mM MgSO4, 420 mM KNO3, 180 mM KH2PO4, 207 mM NH4NO3, 187 mM NaNO3, 590 mM NaCl, and micronutrients. HiNH4+ Stock B consisted of 120 mM MgSO4, 420 mM KNO3, 415 mM (NH4)2SO4, 180 mM KH2PO4, 107 mM NH4NO3, 315 mM Na2SO4, 145 mM NaCl, and micronutrients. Micronutrients (part of Stock B) were 2.4 mM H3BO3, 0.42 mM ZnSO4, 96 µM CuSO3, 0.6 mM MnSO4, and 60 µM H2MoO4. For the LoNH4+ crop test, LoNH4+ A and B stock solutions were added at a 1:1 ratio to achieve an electrical conductivity of 1.9 mS·cm-1 at startup, similar to that of Peiro et al. (2020). This resulted in a total N concentration of 11 mM. For the HiNH4+ crop test, HiNH4+ A and B stock solutions were added at a 1:1 ratio to achieve the same total N concentration (11 mM). This resulted in an electrical conductivity of 2.2 mS·cm-1 at startup, due to higher concentrations of SO42- used for charge balancing. The measured ion concentrations, pH and EC of both nutrient solutions are summarized in Table 1. The total liquid volume in the hydroponic loop was 265 - 275 L throughout both crop tests.
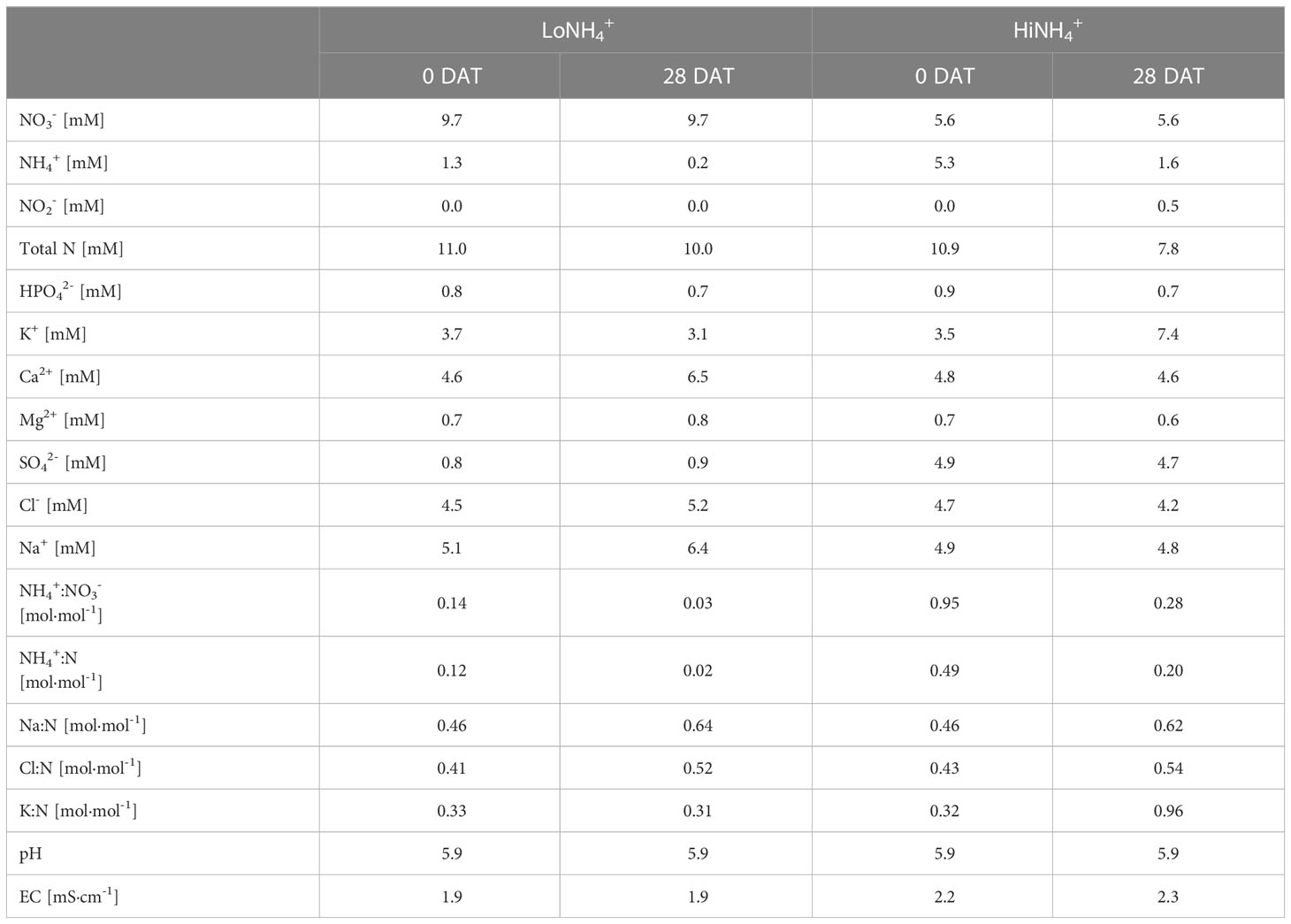
Table 1 Characterization of the LoNH4+ and HiNH4+ nutrient solutions at start (0 days after transplant (DAT)) and end (28 DAT) of each crop test.
Water quality of the recirculating nutrient solution was continuously monitored, including pH (2 x Endress+Hauser Orbisint CPS11D Memosens, Germany), electrical conductivity (EC; 2 x Endress+Hauser Indumax CLS50D, Germany), dissolved O2 (Mettler Toledo InPro 6860i, Switzerland), dissolved CO2 (Labolytic optical CO2 sensor 0-15 mg/L, Trondheim, Norway), and nitrate (Endress+Hauser Viomax CAS51D, Germany, installed in an at-line configuration with auto-sampling and auto-dilution of the nutrient solution). Nutrient solution temperature was continuously monitored and automatically controlled at 18 ± 0.5°C. For both crop tests, pH was adjusted to 5.9 at startup using 0.5 M H2SO4 and then maintained at 5.9 by automatic addition of 0.5 M KOH throughout both crop tests (no addition of acid necessary during the cultivation period). Throughout both crop tests, the actual pH of the nutrient solution typically deviated less than 0.05 pH units from the set-point, and never more than 0.2 pH units. During both crop tests, nutrient stock solutions A and B were automatically fed at a 1:1 ratio to maintain a constant NO3- concentration. The recirculating nutrient solution was mechanically filtered in three steps with reducing pore size from 1.6 to 0.45 µm, using filter cartridges (PP3 Sartopure - Sartobran, Sartorius, Goettingen, Germany). The filtration unit was implemented between the plant cultivation chamber and the stirred tank holding the nutrient solution that was continuously fed to the plants.
2.4 Data collection, sampling, and analysis
Atmospheric concentrations of O2 and CO2 inside the cultivation chamber were continuously measured by an O2/CO2 gas analyzer (California Analytical Instruments, 700 LX NDIR/O2, Orange, CA, USA). Additionally, the O2 and CO2 concentrations of the pressure compensation system’s gas tank were measured at crop test start and end, and before and after chamber venting. The amount of atmospheric gas inside the PCU was determined continuously based on ideal gas law, a total atmospheric volume of 4.9 m3, and continuously measured gas pressure and temperature. This allowed computation of daily atmospheric leak rates and plant O2 production (Pannico et al., 2022). To keep the air humidity constant, the water produced by the plant transpiration was condensed and collected (Pannico et al., 2022). During the crop tests presented in this study, the injection rates of CO2 and water into the atmospheric loop were inaccurate, unfortunately preventing accurate mass balances for CO2 and water and thereby also preventing calculation of consumed amounts of CO2 and produced amounts of water.
Top-view images of the 1.2 m × 1.5 m cultivation chamber floor were acquired every hour (FLIR Blackfly S BFS-U3-200S6C-C @ 70 dpi), and projected leaf area as observed from above was calculated for images acquired every second day based on image segmentation by color. Projected Leaf Area Index (PLAI) was calculated as the measured projected leaf area of all 18 plants (m2) per total cultivation area (m2) (Zheng and Moskal, 2009). SPAD index for indication of chlorophyll content (average of ten measurements per plant; SPAD-502, Minolta Corp. Ltd, Osaka, Japan), dark-adapted chlorophyll fluorescence for the maximum quantum yield of photosystem II (Fv/Fm) (average of two measurements per plant; Plant Stress Kit, Opti-Sciences), and leaf color index (average of three measurements per plant; Chroma Meter CR-400, Konika Minolta) were measured immediately after opening the cultivation chamber at day of harvest. Fresh weight of shoot and stem, and leaf number were determined for each plant by destructive sampling. Additionally, total leaf area of each plant was determined from top-view images of the plant’s individual leaves (Canon EOS 90D + Tamron SP 35 mm @ 59 dpi) based on image segmentation by color.
The lead taproot of each plant was isolated and imaged (Canon EOS 90D + Tamron SP 35 mm @ 294 dpi for LoNH4+ and 334 dpi for HiNH4+) in a tray with water. The lead taproot was defined as the medial root with the largest diameter in the basal zone, not considering the diameter of the laterals. Root length, diameter and number of links and forks were analyzed with WinRhizo (Pro 2021a, Regent Instruments Inc.). Following counting of leaf number and determination of shoot fresh weight (FW) of each plant, shoots and roots were oven-dried at 60°C until constant weight was reached for determination of dry weight (DW) per plant. Total plant DW was calculated as shoot DW + root DW, while harvest index was calculated as shoot DW per total plant DW. Dried samples of shoots and roots were analyzed for total carbon (C) and N content via combustion analysis by a Micro Elemental Analyser UNICUBE® (Elementar, Langenselbold Hesse, Germany) equipped with a thermal conductivity detector. Leaf soluble cations and anions were determined by liquid ion exchange chromatography (ICS-3000, Dionex, Sunnyvale, CA, USA) as described by Pannico et al. (2019).
Every two days, nutrient solution samples (25 mL) were extracted from the hydroponic loop for offline analysis. Nutrient ion concentrations were determined by ion exchange chromatography (ICS 3000 Dionex) and the results were smoothed by moving average to filter out noise from analysis uncertainty. N mass balance was calculated based on measured parameters at the start and end of each crop test, including concentrations of inorganic N in the nutrient solution (measured via optical sensor and ion chromatography methods described above), total liquid volume, total shoot and root biomass and their N content, in addition to the amount of nutrient stock solutions introduced and samples removed throughout the crop test.
Statistical analyses were performed with SPSS (IBM SPSS version 28.0.0.0), Anova with Tukey HSD post-test, tests of normality, Independent Samples t-test and Mann Whitney U test.
3 Results
Building on the background presented in the introduction, the results section first presents the experiment rationale with respect to nutrient solution composition and cultivation conditions. Representing the first scientific paper detailing crop cultivation results from the recently established PCU facility, plant growth and selected scientific datasets offered by this facility are then illustrated on a high level, before being explored in more detail to present effects of crop cultivation in low vs. high NH4+:N ratio.
3.1 Urine recycling scenario: Experiment rationale and nutrient solution design
The experiment rationale was built on the hypothesis presented in the introduction that both design and natural dynamics of upstream waste processing may result in mineralized waste streams with different NH4+:NO3- ratios, which in turn may affect the biology of a downstream plant compartment and thus the overall BLSS performance. Effects of different NH4+:NO3- ratios in the nutrient solution were explored in a mineralized urine scenario, using NH4+ and NO3- as the sole N sources. Two crop tests (two treatments), designated LoNH4+ and HiNH4+, were performed with NH4+ to total N ratios (NH4+:N) of 0.1 and 0.5 mol·mol-1, respectively. Relating to a foreseen upstream nitrification process, the LoNH4+ scenario (0.1 mol·mol-1 NH4+:N) represents nearly complete nitrification, while the HiNH4+ scenario (0.5 mol·mol-1 NH4+:N) represents nitrification without increased alkalinity and thus limited by redox, as reviewed by Larsen et al. (2021). At the same time, these scenarios represent considerable different, low and high NH4+:N ratios for lettuce cultivation (Zhu et al., 2020; Du et al., 2022; Hameed et al., 2022) while still avoiding the use of NH4+ as the dominant N source to limit the potential of ammonium toxicity and thereby allow acceptable growth (see Materials and methods). To allow direct comparison of results, total N concentration was identical at startup of both crop tests (11 mM). Capitalizing on a state-of-the-art nutrient monitoring and control system, the NO3- concentration was kept constant (9.7 mM in LoNH4+; 5.6 mM in HiNH4+) throughout each of the two crop tests to minimize effects of the absolute NO3- concentration itself. This allowed the NH4+:N ratio to change throughout the cultivation period depending on the total consumption of NH4+ and NO3- in the system, demonstrating the development of the NH4+:N ratio over time.
In line with a urine recycling scenario, the crop tests were performed with an elevated NaCl concentration of 5 mM, representing a Na:N concentration ratio of 0.46 mol·mol-1. This ratio was designed based on typical Na:N ratios of mineralized urine. While fresh urine is typically characterized by 0.17 mol·mol-1 Na:N and Cl:N (adapted from Udert et al., 2006), several reports illustrate ratios of approximately 0.25 mol·mol-1 in urine-based fertilizer solutions (adapted from Bonvin et al., 2015; Mauerer et al., 2018; Halbert-Howard et al., 2021). However, the final composition of the mineralized nutrient solutions depends on urine storage and ammonification and nitrification processes. As urine is prone to ammonia volatilization, resulting Na:N and Cl:N ratios may be considerably elevated - exemplified by the real stored urine fraction by Udert and Wachter (2012) with Na:N and Cl:N ratios of 0.5 mol·mol-1. Thus, the Na:N and Cl:N concentration ratios used in this study represent a urine-based nutrient solution, accounting for effects by urine storage and processing. However, effects of potential Na+ accumulation over time in a closed loop system are not accounted for in this study, as such predictions would benefit from more insight in Na uptake ratios as function of nutrient solution composition and cultivation conditions.
Beyond NH4+/NO3- and Na+/Cl-, the two crop tests were run with similar concentrations of macro- and micronutrients, except for SO42- used for charge balancing of NH4+/NO3-. The total strength of the nutrient solutions was designed so that the total electrical conductivity of the LoNH4+ nutrient solution at start was identical to that of Peiro et al. (2020) (1.9 mS·cm-1) used for similar MELiSSA studies of hydroponic lettuce cultivation (resulting in an electrical conductivity of 2.2 mS·cm-1 for the HiNH4+ nutrient solution due to the elevated requirement of SO42- for charge balancing). The resulting nutrient solutions based on the above design criteria are illustrated in Table 1.
3.2 Crop cultivation in the ESA MELiSSA Plant Characterization Unit
The results reported in this paper were obtained in the recently established ESA MELiSSA Plant Characterization Unit (PCU), in which 18 lettuce plants were hydroponically cultivated as deep-water culture for 28 days after transplant (DAT), to a total age of 38 days, across a 1.8 m2 plant cultivation area (Figure 1). Extensive monitoring and control systems provided stable cultivation conditions, including atmospheric temperature and RH, and nutrient solution temperature, pH and NO3- concentration (see Materials and methods, Table 1; Figure 3). The closed nature of the atmospheric loop combined with continuous monitoring of atmospheric O2 and CO2 concentrations represent the basis for determination of O2 production and CO2 consumption by the plants. Atmospheric leak rates in percent gas volume per hour were calculated daily and demonstrated a stable and high system tightness with an average leak rate of 0.082 ± 0.017%·h-1 and 0.095 ± 0.022%·h-1 for the LoNH4+ and HiNH4+ crop tests, respectively. The atmospheric O2 concentration increased during the day phase resulting from photosynthesis, while it decreased during the night phase due to respiration (Figure 2). As the O2 production during the day phase exceeds the O2 consumption during the night phase, atmospheric O2 gradually increased throughout the crop tests creating a need to vent the cultivation chamber to stay below 25% O2 for safety reasons (performed at Day 19 for both crop tests). Atmospheric CO2 was controlled at a minimum of 1000 ppm during the day phase, while it increased due to respiration during the night phase (up to 2140 ppm during the LoNH4+ crop test). As the PCU chamber is sealed and only opened for venting and reduction of atmospheric O2 concentration, sampling or physical inspection of the plants is not possible during a crop test. To demonstrate biomass development, Projected Leaf Area Index (PLAI) was calculated based on top-view images acquired by the PCU’s internal color camera (Figure 4). To further indicate differences in biomass development over time (Figure 4) despite the lack of true biomass measurements, PLAI was used as a basis for calculating a specific growth rate, here introduced as µPLAI, according to Equation 1 where t symbolizes time (in DAT).
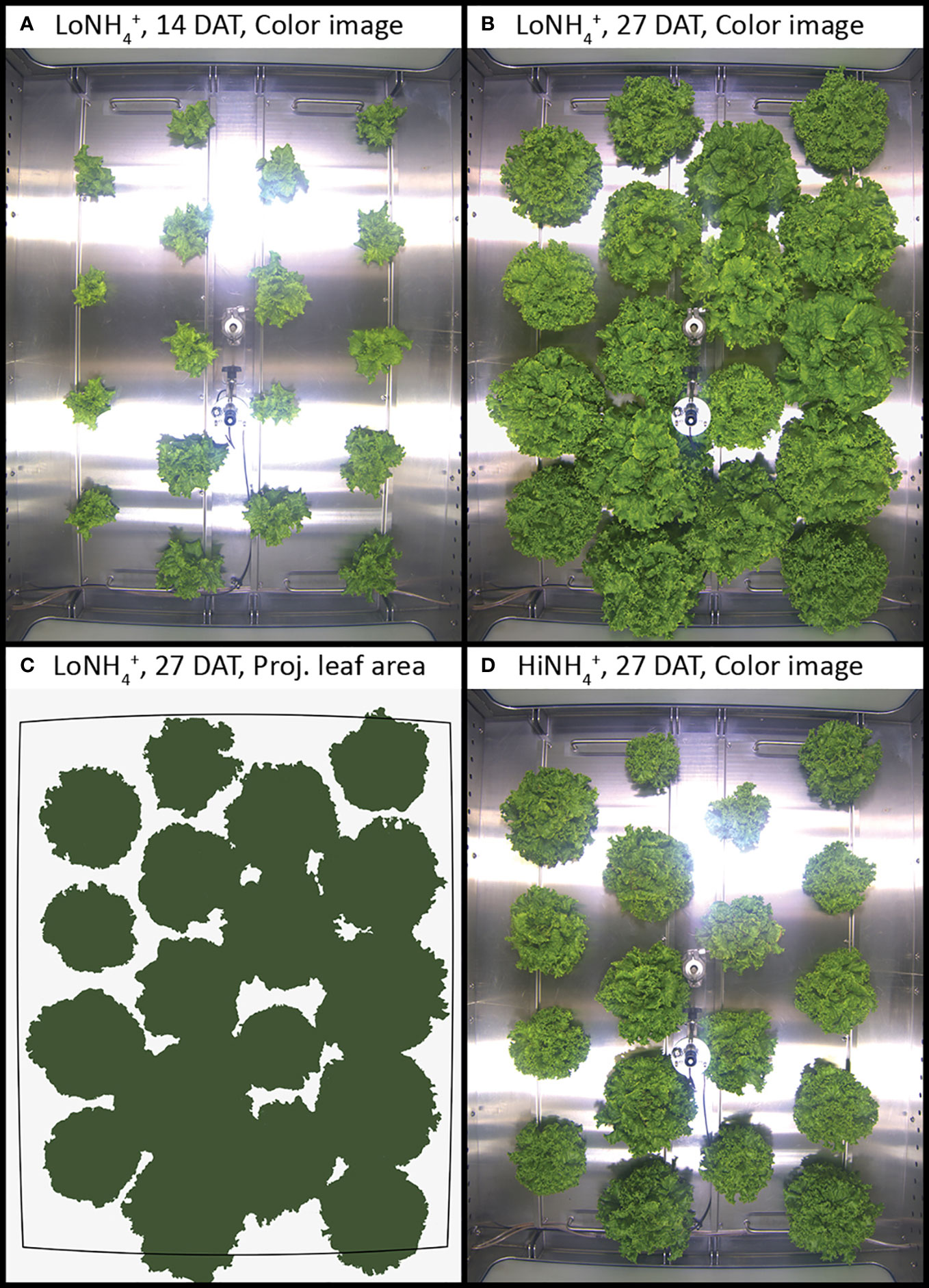
Figure 1 Top-view color images of lettuce inside the PCU cultivation chamber at 14 and 27 days after transplant (DAT), cultivated in LoNH4+ (A, B) and HiNH4+ nutrient solution (D). Example illustrating image segmentation for determination of projected leaf area (C).
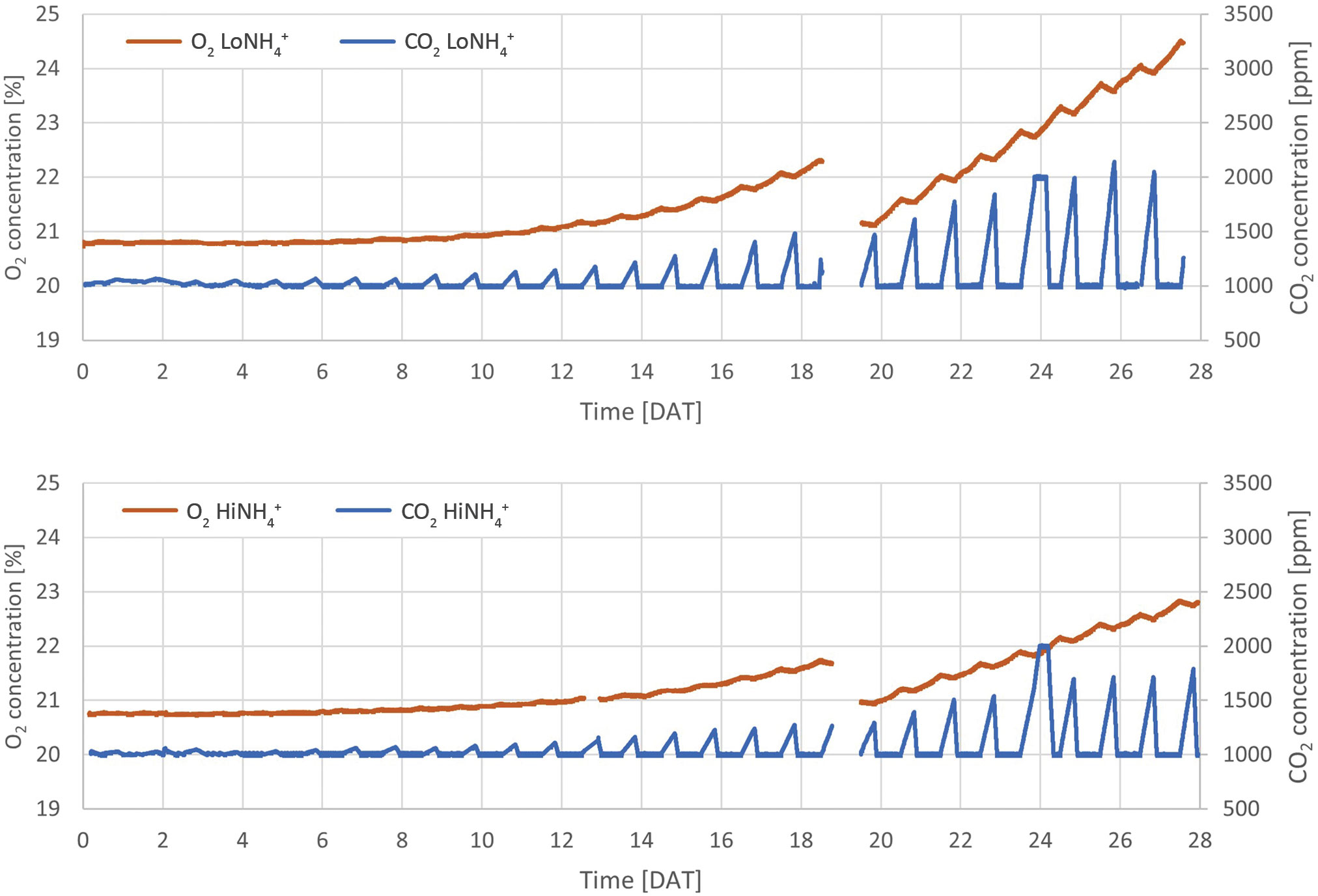
Figure 2 Absolute concentrations of atmospheric O2 and CO2 throughout the LoNH4+ (upper graph) and HiNH4+ (lower graph) crop tests. Missing data from 19 DAT represents a venting event in which the cultivation chamber was opened to reduce the O2 concentration.
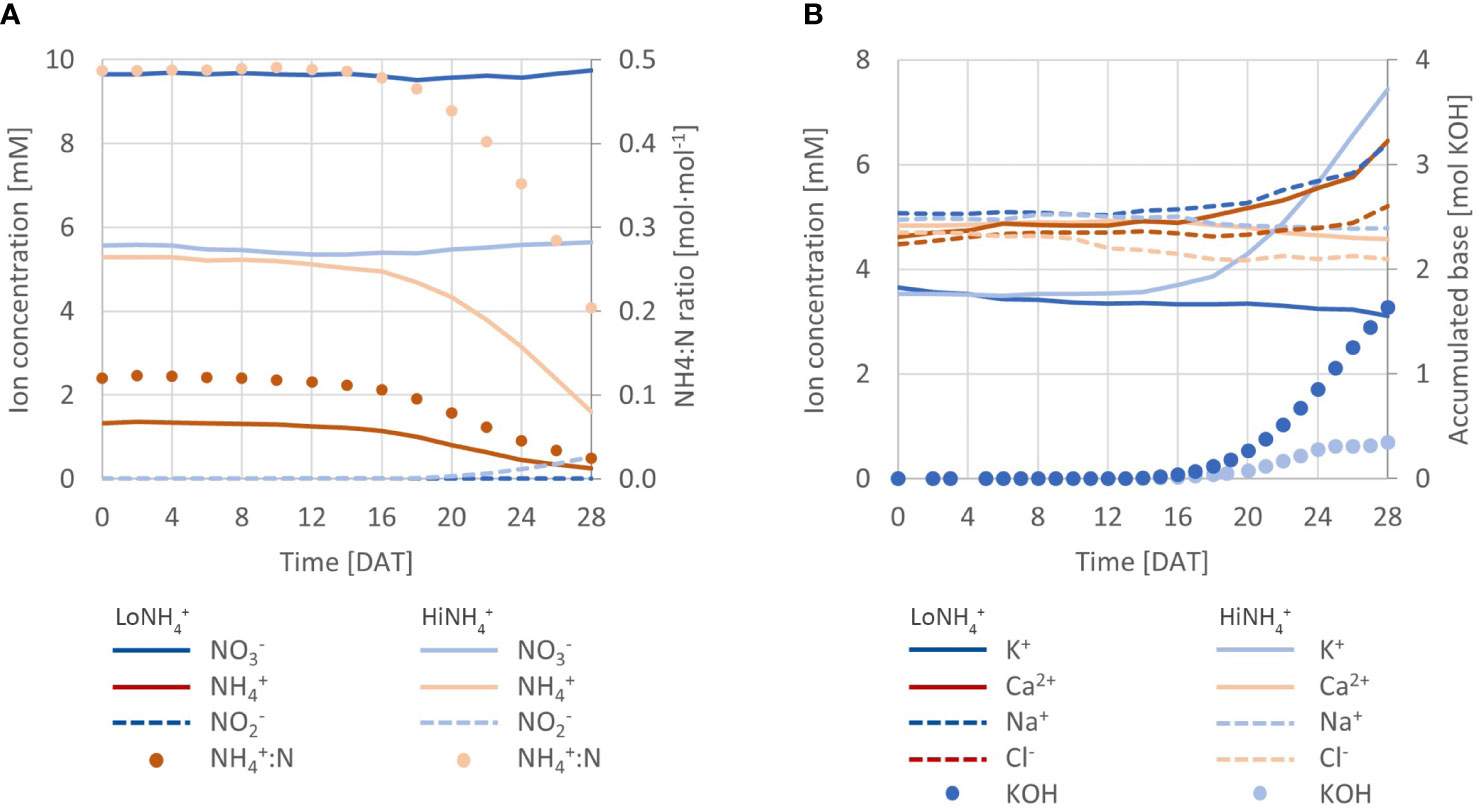
Figure 3 (A) Measured concentration of NO3-, NH4+, and NO2- throughout the LoNH4+ and HiNH4+ crop tests (lines; left Y-axis), in addition to the ratio of NH4+ to total N (NH4+:N; dots; right Y-axis). (B) Development of K+, Ca2+, Na+ and Cl- concentrations in the nutrient solutions of both crop test (lines; left Y-axis), in addition to accumulated addition of base (KOH; dots; right Y-axis).
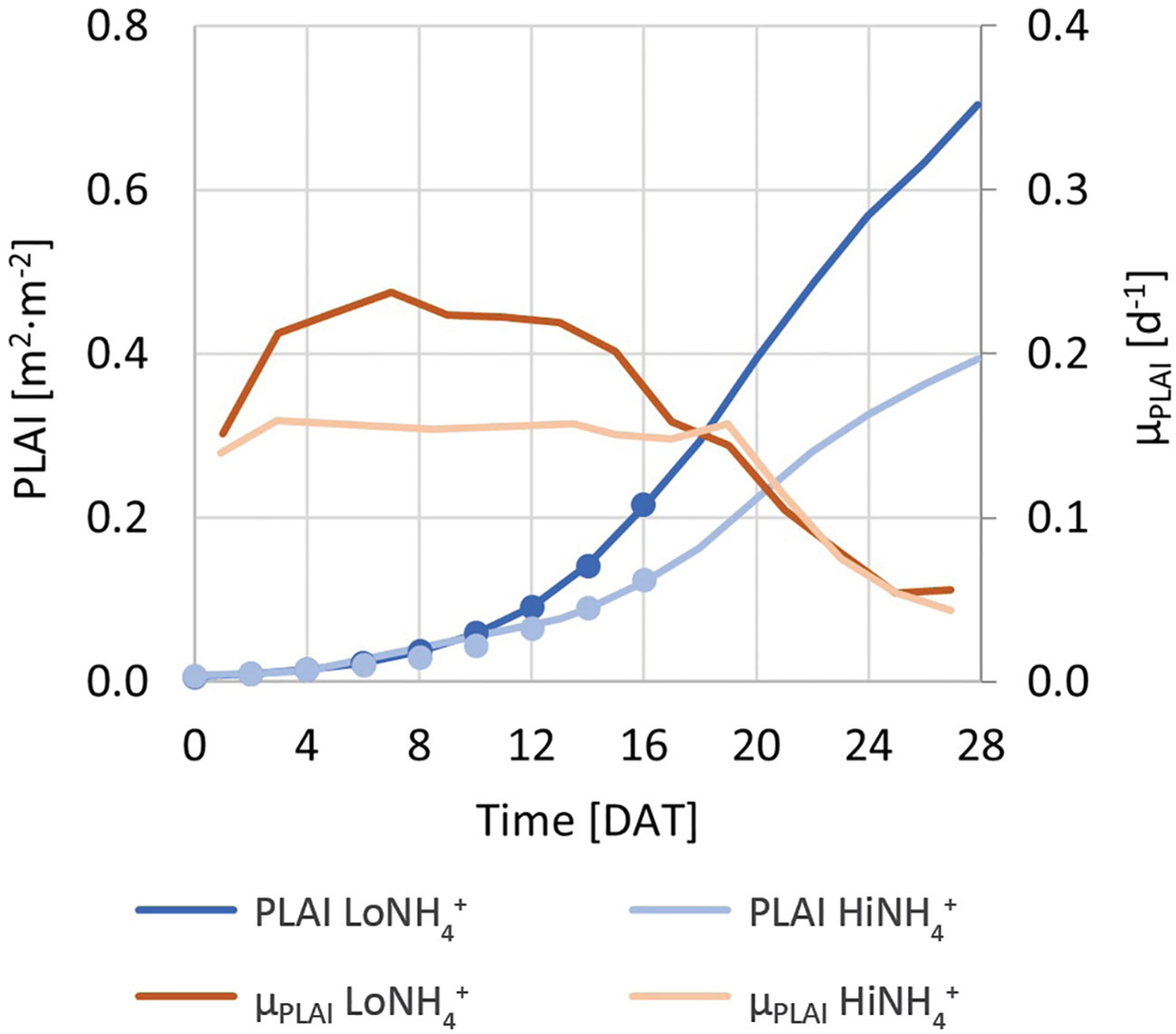
Figure 4 Blue solid lines (left Y-axis): PLAI (Projected Leaf Area Index) of the LoNH4+ and HiNH4+ crop tests. Blue circles (left Y-axis): Trendlines based on exponential regression of a subset of the PLAI dataset from 0 to 16 DAT (coinciding with the PLAI dataset in blue solid lines). Red lines (right Y-axis): PLAI-based specific growth rate, µPLAI, of the LoNH4+ and HiNH4+ plants.
3.3 Plant development and physiology at high and low NH4+:NO3- ratios
3.3.1 Shoot development over time as observed by non-destructive methods
A relatively stable µPLAI during the first 16 - 18 days indicates close to exponential growth for plants in both the HiNH4+ crop test and the LoNH4+ crop test in this period. From approximately 20 DAT, as the plants mature and start to overlap, the PLAI-based specific growth rates of both treatments coincide and decrease (Figure 4). Regression analysis of PLAI from 0 to 16 DAT was performed using exponential trendlines (Figure 4; R2 factors > 0.999), demonstrating a reduced µPLAI for plants growing in the HiNH4+ nutrient solution (0.18 per day) compared to that of LoNH4+ (0.22 per day). The initial PLAI of HiNH4+ and LoNH4+ plants were similar due to identical germination and seedling phases (both 0.010 m2·m-2 at 2 DAT). The final PLAI of the LoNH4+ crop test at 28 DAT reached 0.70 m2·m-2 corresponding to a projected leaf area of 1.3 m2, while corresponding values of HiNH4+ were 44% lower with 0.39 m2·m-2 PLAI and 0.71 m2 projected leaf area. Closer inspection of the PLAI of the two crops illustrate that their differences stayed below approximately 20% during the first 10 days in the PCU, before it increased gradually up to approximately 45% at 18 DAT and remained at this level throughout the rest of the cultivation period.
3.3.2 Root and shoot biomass and morphology at harvest
In line with the differences demonstrated by non-destructive PLAI analysis, detailed inspection of the plants after destructive sampling at 28 DAT illustrated a total leaf area of the HiNH4+ crop which was 50% lower than that of the LoNH4+ crop (3.5 m2 vs. 7.0 m2, respectively, for the 18 plants; Table 2). The results demonstrate that the shoot biomass was affected in a similar way, with the HiNH4+ plants having 58% lower shoot fresh weight and 40% lower shoot dry weight compared to the LoNH4+ plants. The difference in these numbers illustrate a higher shoot dry weight content of the HiNH4+ plants compared to the LoNH4+ plants (10% vs. 7%, respectively). Furthermore, the HiNH4+ plants developed a significantly lower number of leaves (35%) compared to the LoNH4+ group.
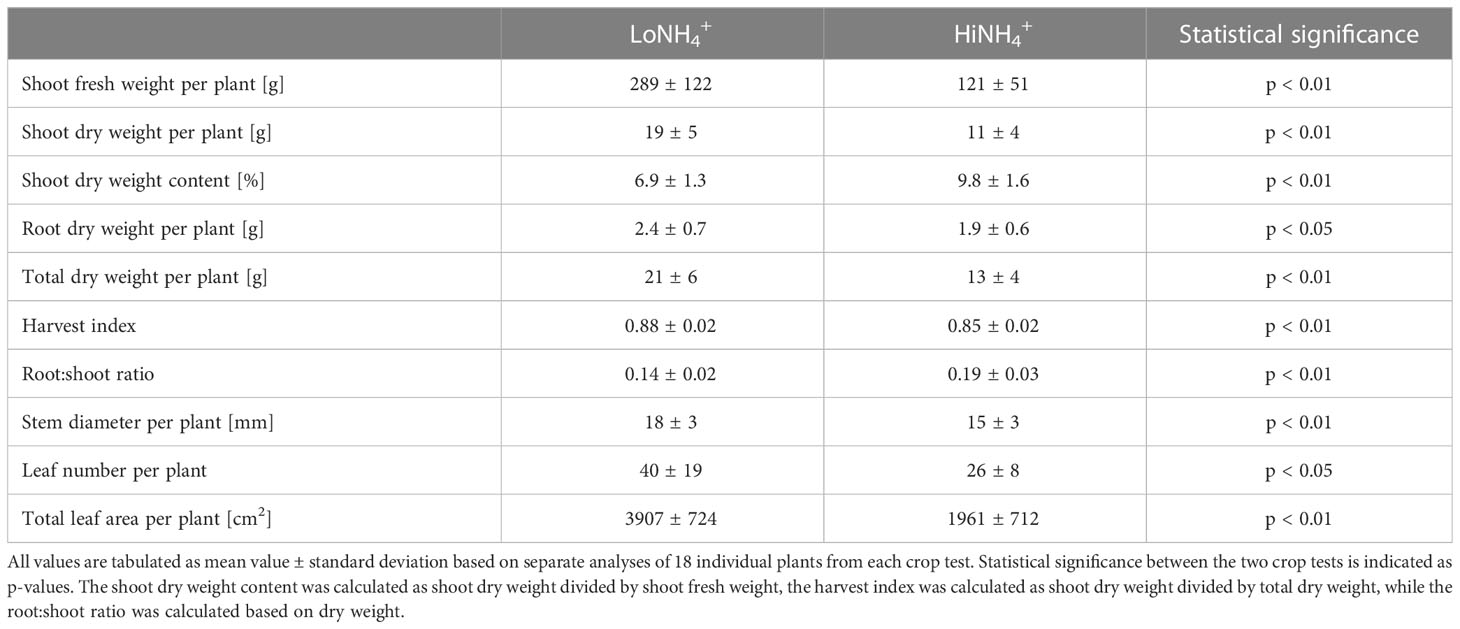
Table 2 Biomass characteristics of plants from the LoNH4+ and HiNH4+ crop tests, measured at harvest (28 DAT).
The dry root biomass difference between the two crops (19% lower for HiNH4+ plants) was smaller than the shoot dry biomass difference (40% lower for HiNH4+), illustrating a higher root:shoot ratio and a lower harvest index of the HiNH4+ plants compared to those of LoNH4+ (Table 2). Following harvest and destructive sampling, the lead taproot of each plant (see Materials and methods) was isolated, imaged, and analyzed. The taproots of the HiNH4+ and LoNH4+ plants demonstrated considerable differences, as qualitatively indicated in Figure 5. In average, the lead taproots of HiNH4+ were shorter (55% reduction in total length, including both mother root and lateral roots), thinner (15% reduction in average diameter) and less branched (57% less forks) than those of the LoNH4+ plants.
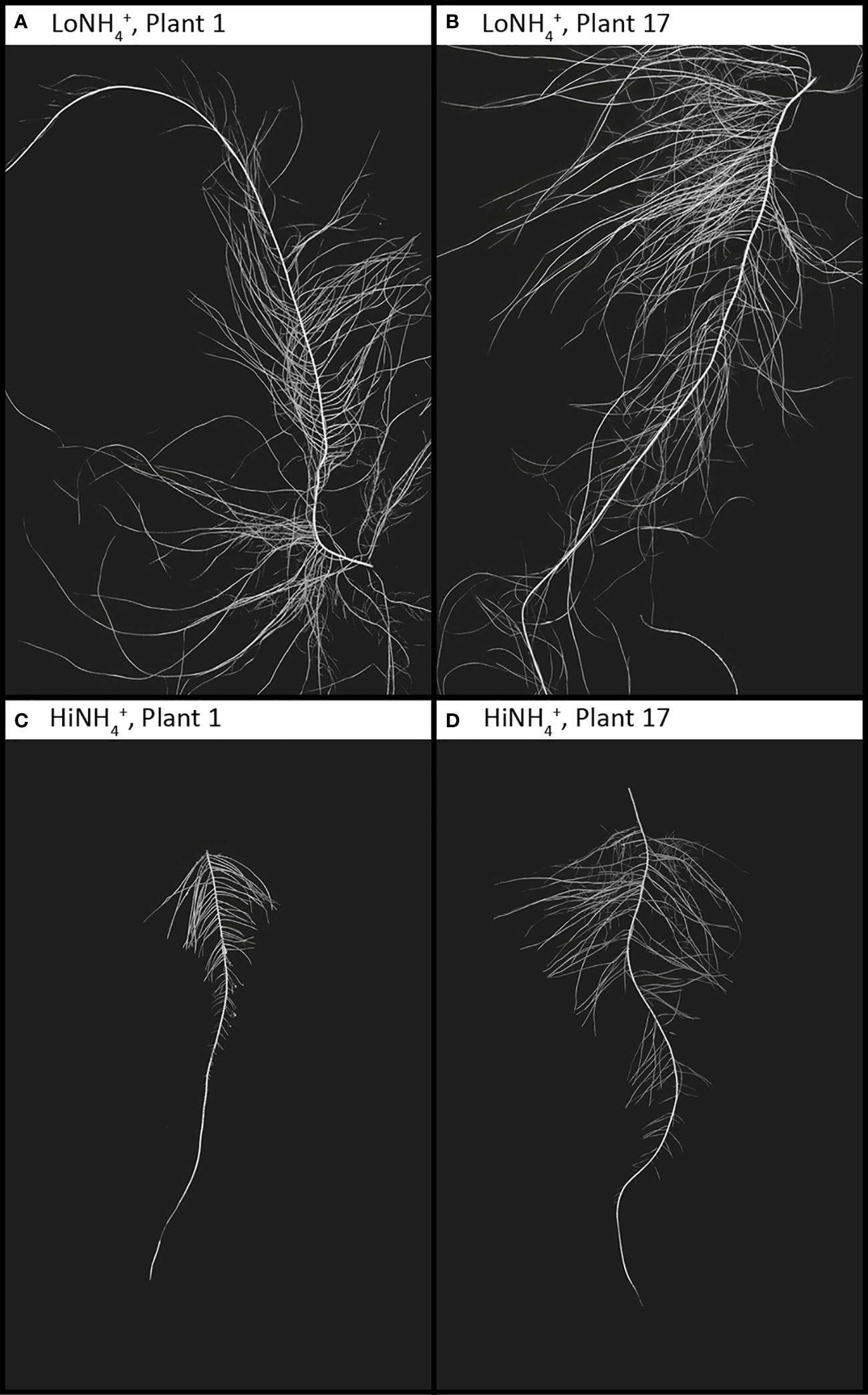
Figure 5 Lead taproots of two example LoNH4+ plants (A, B) and two HiNH4+ plants (C, D) from harvest at 28 DAT. All images are represented at the same resolution, covering a 20 x 30 cm area. Root and background contrast and color levels have been modified from the raw images to enhance root morphology.
3.3.3 Leaf chlorophyll content, color index and Fv/Fm at harvest
Dark-adapted chlorophyll fluorescence measurements of the maximum quantum yield of photosystem II (Fv/Fm) were identical for both treatments (Table 3), while an elevated SPAD index indicated a higher chlorophyll content of the leaves of the HiNH4+ plants. Color measurements illustrated significant differences as evaluated by the L*a*b color space, with the HiNH4+ leaf color shifted more towards green compared to the color of the LoNH4+ leaves.
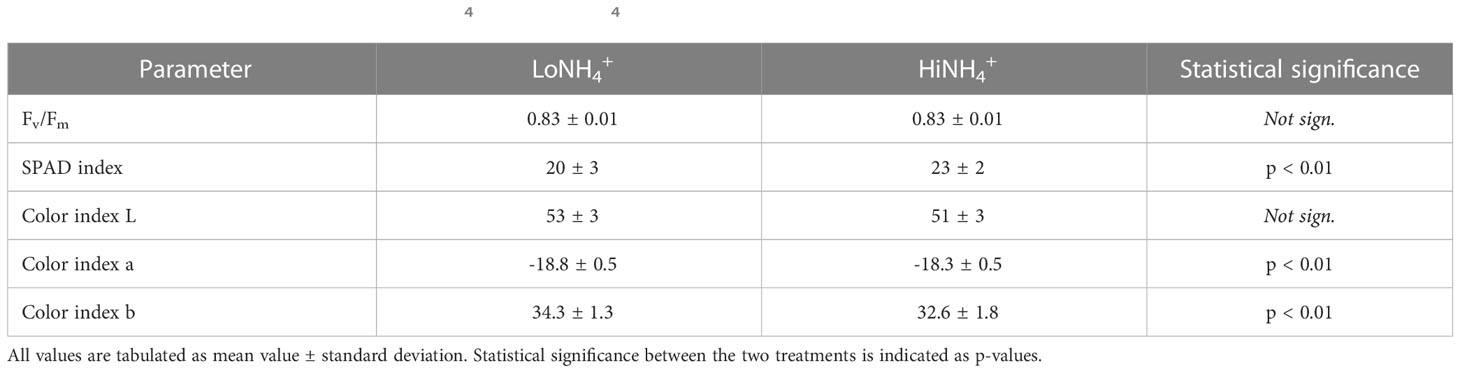
Table 3 Fv/Fm (average of two measurements per plant), SPAD (average of ten measurements per plant) and Color index (average of three measurements per plant) values of the 18 LoNH4+ and 18 HiNH4+ plants as measured at harvest (28 DAT).
3.3.4 Nutrient ion and element composition of shoots and roots at harvest
Nutrient ion and element analyses of lettuce shoot and root biomass harvested at 28 DAT demonstrated considerable differences between plants cultivated in the two different nutrient solutions (Table 4). The biomass content of NO3- and NH4+ varied in line with their concentrations and ratios in the nutrient solutions. The HiNH4+ plants exhibited an NH4+ root content nearly three times higher and a NO3- shoot and root content 7 – 8 times lower than that of the LoNH4+ plants. Considering total N, the most significant differences were observed for the roots, for which the total N content was 28% higher in the HiNH4+ plants. Additionally, the C content of HiNH4+ plants was higher than that of the LoNH4+ plants (9% higher in shoot content and 15% higher in root content). The content of other nutrient ions was generally lower in plants cultivated in HiNH4+ nutrient solution compared to those cultivated in LoNH4+. This includes the cations K+ (52% lower shoot content and 36% lower root content), Na+ (32% lower shoot content and 22% lower root content), and Mg2+ (47% lower shoot content). Ca2+ illustrated the same trend (55% lower shoot content and 17% lower root content in HiNH4+) although the differences were not statistically significant due to high variability in the results. Reduced nutrient ion content of HiNH4+ plants could also be observed for the anions HPO42- (44% lower shoot content) and Cl- (72% lower root content), while no statistically significant differences could be observed for SO42-.
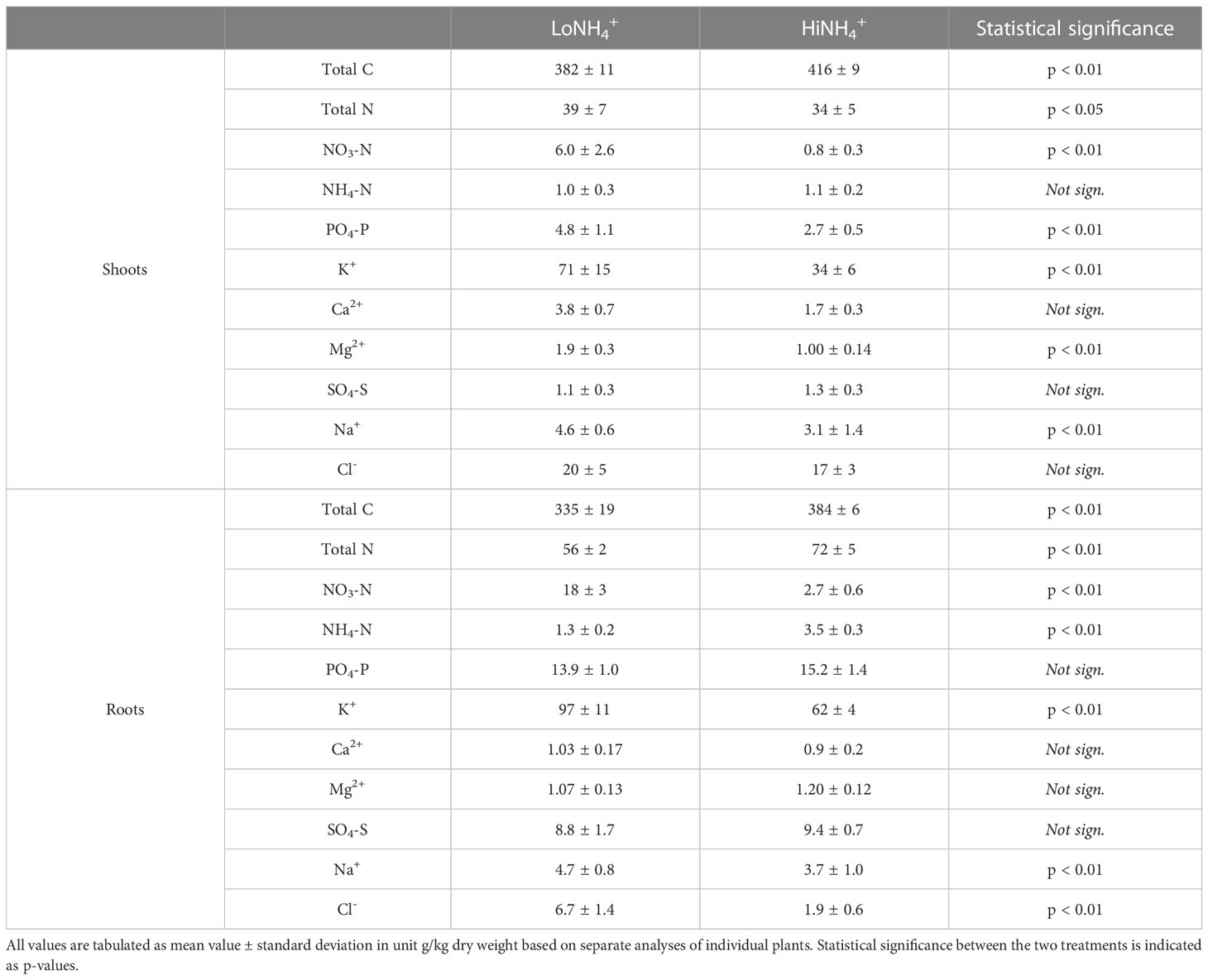
Table 4 Average shoot and root nutrient ion and element content of the 18 LoNH4+ and 18 HiNH4+ lettuce plants as measured at harvest (28 DAT).
3.4 Crop performance and system dynamics at high and low NH4+:NO3- ratios
3.4.1 O2 production
Continuous monitoring of the atmospheric O2 and CO2 concentrations inside the cultivation chamber illustrated O2 production during the day (when CO2 was controlled at 1000 ppm by automatic CO2 injection), and O2 consumption and CO2 production during the night (Figure 2). Detailed inspection of the datasets illustrates an immediate increase in atmospheric CO2 concentration and a corresponding decrease in O2 starting from the time at which the cultivation chamber LEDs were turned off, as illustrated in Figure 6A, detailing the first two days after the chamber venting. Reversely, an immediate decrease of CO2 concentration and increase of O2 was initiated when the LEDs were reactivated at the end of the night phase. During the 16-hour day phase of the 21st day (20 DAT), the O2 concentration of the cultivation chamber increased 0.48 vol-% in the LoNH4+ crop test, and 0.26 vol-% in the HiNH4+ crop test. During this day, the LoNH4+ plants covered a 0.71 m2 cultivation area, while the HiNH4 plants covered 0.40 m2 (as evaluated by projected leaf area). During the following 8-hour night phase, the CO2 concentration increased linearly to 1614 ppm (LoNH4+) and 1391 ppm (HiNH4+) (with no injection of CO2). After reactivating the LEDs, the atmospheric CO2 concentration returned to 1000 ppm after 1.5 hours (LoNH4+) and 1.6 hours (HiNH4+) of daylight. Corresponding analyses at the end of the crop tests demonstrated an increase in O2 concentration of 0.59 vol-% by the LoNH4+ plants (1.21 m2 projected leaf area), and 0.33 vol-% by the HiNH4+ plants (0.71 m2 projected leaf area) during the day phase of the 28th day. The total O2 amount inside the PCU atmospheric loop and its pressure compensation system was precisely determined at the time of cultivation chamber closing and opening (see Materials and methods). Compensating for the accurately determined chamber gas leaks, this allows detailed calculations of the O2 amount produced by the plants during the two phases of each crop test before (0 - 18 DAT) and after (20 - 28 DAT) chamber venting (totaling 26.6 days; Table 5). Considering the absolute O2 production, the HiNH4+ plants produced 34% less O2 during the first phase (0 - 18 DAT) and 43% less O2 during the second phase (20 - 28 DAT) compared to the LoNH4+ plants. However, accounting for the smaller projected leaf area (PLA) of the HiNH4+ plants, average O2 production per day and projected leaf area was similar for the LoNH4+ and HiNH4+ plants both during the first phase of the crop tests (1.2 vs 1.3 mol·d-1·m-2, respectively) and during the second phase (both 1.1 mol·d-1·m-2). Furthermore, relating the total O2 production to the total shoot DW at harvest also demonstrates similar values for the two crops, with 37 mol O2 produced (over 26.6 days) per kg shoot DW for both crops. Estimated daily O2 production throughout the crop tests was calculated based on the O2 concentration in the cultivation chamber, the leak rate, and estimated daily gas composition of the atmospheric pressure compensation system (see Materials and methods). As the latter introduces a modest uncertainty in the calculations of daily O2 production, the resulting datasets were smoothed by polynomial regression (Figure 6B). Evaluating the differences in daily O2 production at 10, 14, 18, 22 and 26 DAT based on these datasets, the HiNH4+ plants produced 26, 37, 40, 42 and 45% less compared to the LoNH4+ plants, respectively.
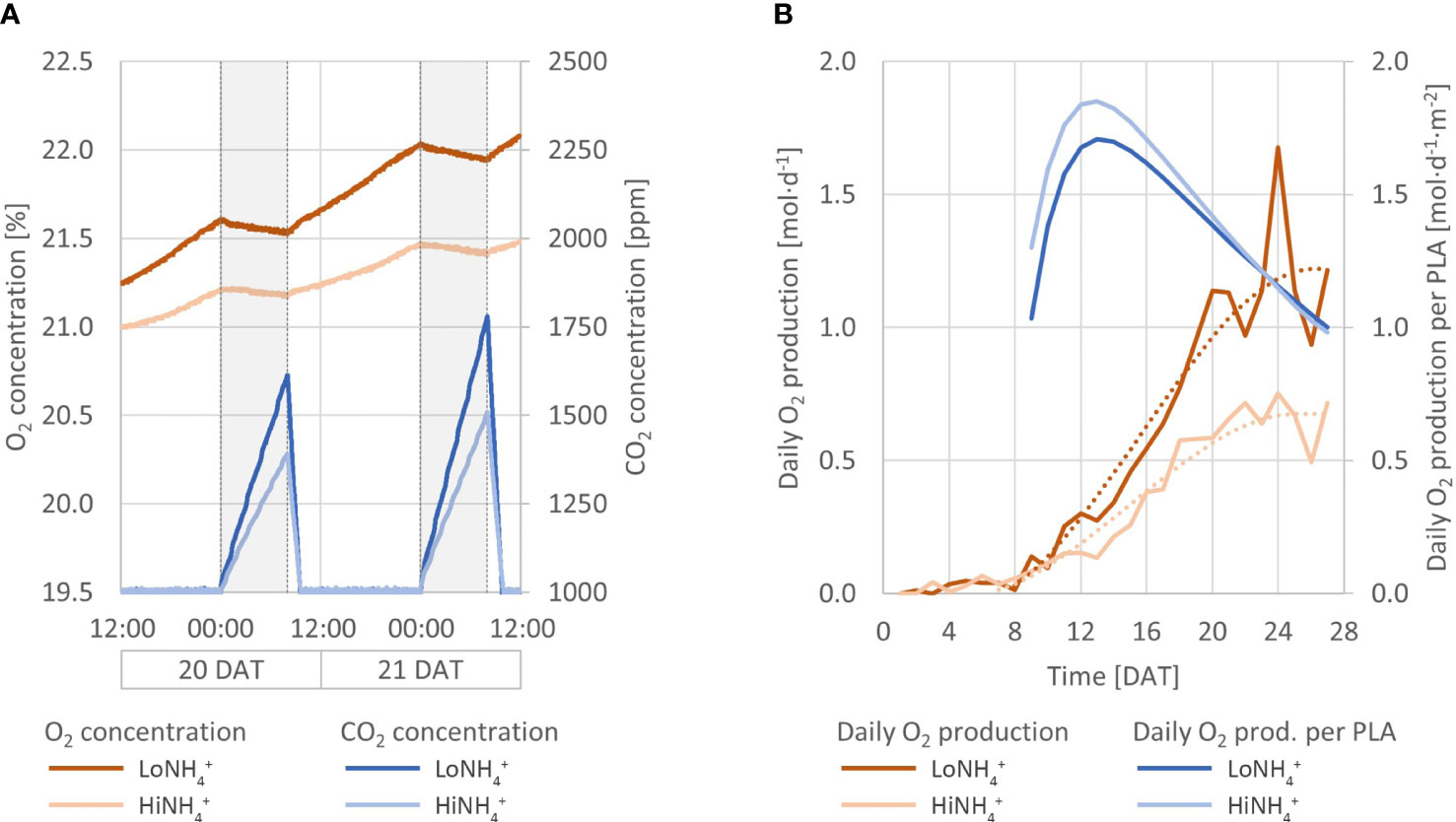
Figure 6 (A) Atmospheric O2 (red lines) and CO2 (blue lines) concentration inside the PCU cultivation chamber over 48 hours (20 - 21 DAT) for the LoNH4+ and HiNH4+ crop tests. Night phases are indicated with gray background. (B) Daily O2 production (by 18 plants; solid red lines) of the LoNH4+ and HiNH4+ crop tests, plotted together with smoothed datasets based on 3rd degree polynomial regression (dotted red lines). Additionally, daily O2 production per projected leaf area (PLA; blue lines).
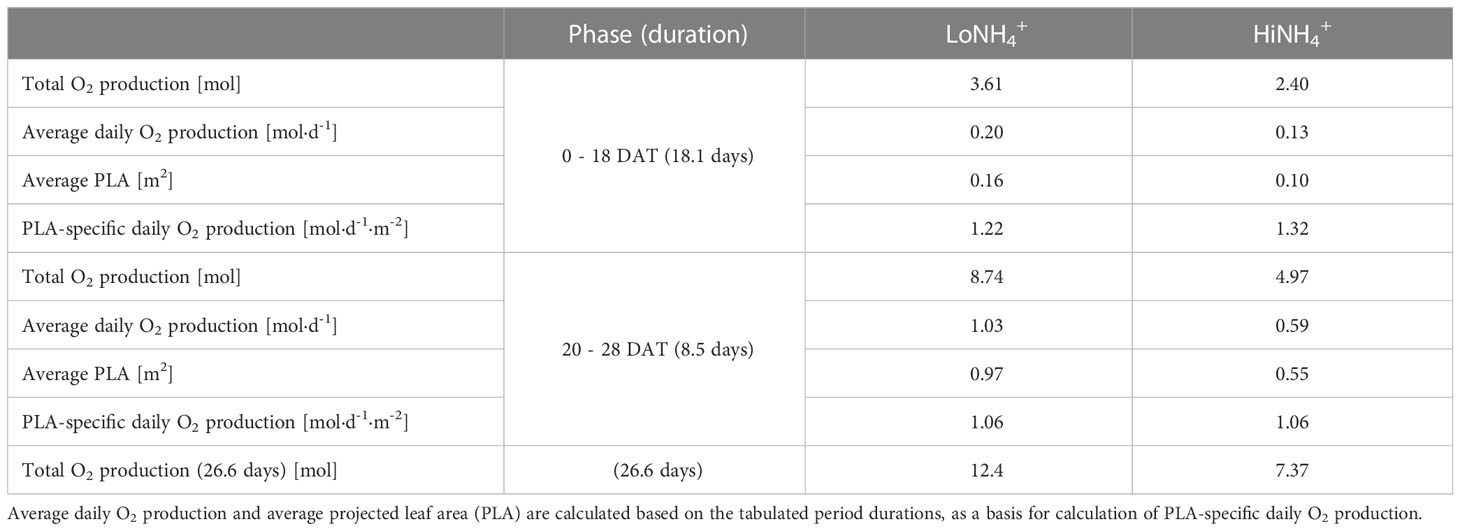
Table 5 Total O2 production of 18 plants during the LoNH4+ and HiNH4+ crop tests, tabulated for the cultivation periods before (18.1 days) and after (8.5 days) the chamber venting, in addition to the full crop test period (26.6 days, not accounting for the day of venting at 19 DAT).
To account for the considerable difference in biomass between the two treatments, the daily O2 production (smoothed dataset) was evaluated per projected leaf area (PLA), measured non-destructively throughout the crop tests. These results (Figure 6B), illustrate similar daily O2 productions per PLA for both treatments, with an indication of an increased O2 production per PLA for the HiNH4+ plants during the early phase of the crop tests (15, 7, and 4% increase at 10, 14 and 18 DAT, respectively, and no difference from 20 to 28 DAT). The highest specific O2 productivity (per time and PLA) estimated was 1.85 mol·d-1·m-2 for the HiNH4+ crop (13 DAT) and 1.71 mol·d-1·m-2 for the LoNH4+ crop (13 DAT).
3.4.2 Nutrient solution development
From start, the total N concentration was the same in both crop tests (11 mM; Table 1), while the NH4+:N ratios were different (0.1 mol·mol-1 for LoNH4+, 0.5 mol·mol-1 for HiNH4+). Based on an advanced PCU nutrient management system, automatic feeding of nutrient stock solutions (with the same NH4+:N ratios as from start, thus 0.1 or 0.5 mol·mol-1, see Materials and methods) successfully maintained a constant NO3- concentration throughout both crop tests (Figure 3A), at 9.7 mM for LoNH4+ and 5.6 mM for HiNH4+ (Table 1). While this control strategy required considerable feeding of nutrient stock solutions during the LoNH4+ crop test (0.5 L of each stock solution), practically no nutrient stock solution feeding was required during the HiNH4+ crop test (0.03 L of each), indicating a considerable NO3- consumption during the LoNH4+ crop test, and practically no NO3- consumption during the HiNH4+ crop test.
Without control of nutrient solution NH4+ concentration, NH4+ levels were allowed to vary based on N consumption. Throughout both crop tests, the NH4+ concentration was considerably reduced (Table 1), demonstrating a higher consumption of NH4+ relative to total N than the NH4+:N ratio of the nutrient solution. In consequence, the nutrient solution NH4+:N ratio during the LoNH4+ crop test was reduced from 0.12 to 0.02 mol·mol-1, while it was reduced from 0.49 to 0.20 mol·mol-1 during the HiNH4+ crop test.
Nitrite (NO2-) could not be detected (< 1 mg N L-1) throughout the full LoNH4+ crop test and throughout the close-to-exponential phase of the HiNH4+ crop test (up to 20 DAT). During the latter test, NO2- was detected during the last 6 days of cultivation (0.1 mM at 22 DAT, increasing to 0.5 mM at 28 DAT). To rule out major effects of nitrification on observed NH4+ consumption, operation of the cultivation facility was prolonged after removal of all plants from the system. Over the next 6 days, the NH4+ concentration decreased 0.5 mM in parallel with a further 0.4 mM increase of NO2-. Nutrient solution pH was kept constant by automatic pH control with KOH and H2SO4. No acid addition was required in any of the crop tests, while base addition was characterized by a considerably higher KOH consumption during the HiNH4+ crop test (1.6 mol OH-) than during the LoNH4+ crop test (0.3 mol OH-) (Figure 3B).
Despite no control of electrical conductivity (EC), the NO3–based feeding of nutrient stock solutions and the pH-based feeding of base resulted in a relatively constant EC of 1.9 mS/cm during the LoNH4+ crop test and 2.2 - 2.3 mS/cm during the HiNH4+ crop test (Table 1).
Development of macronutrient concentrations (Figure 2B) in the nutrient solution was most profound for K+, with a considerable increase from 3.5 to 7.4 mM during the HiNH4+ crop test (KOH was automatically added to maintain pH at setpoint). In contrast, K+ concentration decreased by 0.6 mM over the LoNH4+ crop test. Other ions demonstrating a concentration change of more than 0.2 mM over the course of a crop test (beyond K+ and N ion species) included Ca2+, Na+ and Cl-, demonstrating an increase during the LoNH4+ crop test (requiring considerable feeding of nutrient stock solutions) and a decrease during the HiNH4+ (conducted practically without feeding of nutrient stock solutions). For other macronutrients, including HPO42-, Mg2+, and SO42-, analyses throughout the crop tests demonstrated stable levels (Table 1).
3.4.3 N mass balance
With a considerable nutrient solution volume (270 L), a limited cultivation area (1.8 m2), and a crop with modest nutrient requirements (lettuce), mass balances are vulnerable to measurement inaccuracies especially with respect to ion concentrations in the nutrient solution. Nevertheless, a N mass balance was calculated (Table 6) based on the available datasets, demonstrating a mass balance closure of 94% (LoNH4+) and 89% (HiNH4+).
The total consumption of NO3- and NH4+ during the LoNH4+ crop test was 0.9 and 0.4 mol, respectively, illustrating an apparent NH4+:N consumption ratio of 0.31 mol·mol-1 during the LoNH4+ crop test. In contrast, with no observed consumption of NO3- during the HiNH4+ crop test, N consumption was apparently based on NH4+ only. Relative to the produced biomass (Table 2), the consumption of inorganic N (Table 6) was 3.1 mol·kgDW-1 for both crop tests.
3.4.4 Incorporation of C and N into edible biomass
To illustrate C and N recycling in a BLSS perspective, the amounts of C and N incorporated into edible biomass (18 plants per crop) was calculated for both crop tests (Figure 7) based on measured shoot DW at harvest (Table 2), and their composition (Table 4). The edible biomass of the HiNH4+ crop (202 g DW) was 40% lower than that of the LoNH4+ crop (337 g DW), while the relative shoot C content of the HiNH4+ crop (42%) was higher than that of the LoNH4+ crop (38%). In sum, the HiNH4+ crop incorporated 35% less C (84 g) into total edible biomass compared to the LoNH4+ crop (128 g). For N, the relative shoot content of the HiNH4+ crop (3.4%) was lower than that of the LoNH4+ crop (3.9%). In sum, the HiNH4+ crop incorporated 48% less N (7 g) into total edible biomass compared to the LoNH4+ crop (13 g).
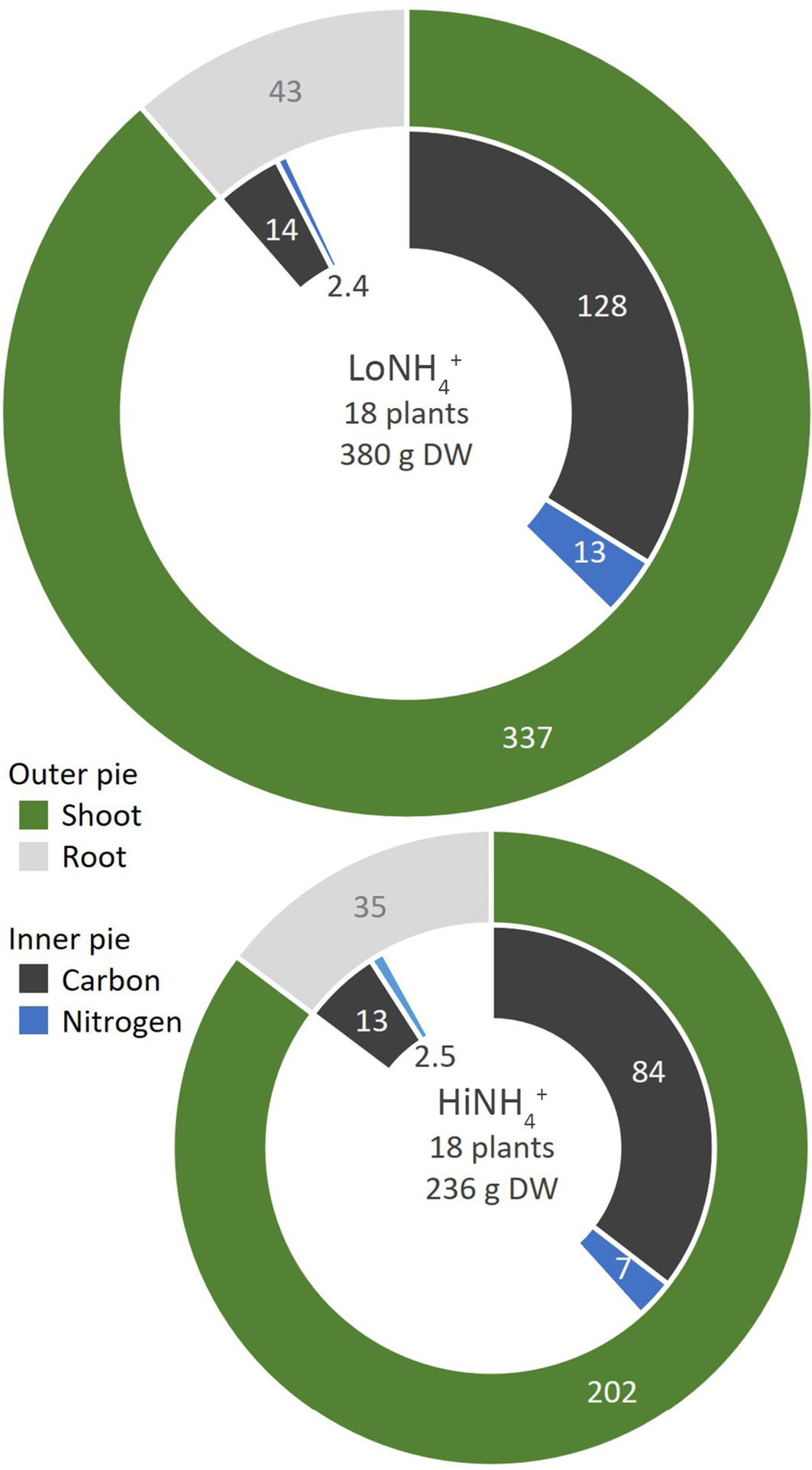
Figure 7 Incorporation of C (black) and N (blue) into shoots (green) and roots (gray) of the LoNH4+ (upper) and HiNH4+ (lower) crops. All numbers represent element or biomass dry weight in gram and relate to the full crop consisting of 18 plants. The relative difference in total pie area corresponds to the relative difference in total dry weight of the two crops.
4 Discussion
During long duration human space missions, bioregenerative life support systems may provide food, regenerated water, and O2 for the astronauts. Given the limited availability of nutrient resources for plant production in this scenario, recycling and reuse of nutrients such as N from human urine is important. Depending on the upstream mineralization process of urine, the nutrient solution may have various NH4+:NO3- ratios and elevated NaCl concentrations. To maximize production of food, O2 and clean water, crop cultivation conditions should be optimized. However, as a BLSS consists of multiple processes, the performance of the regenerative loop including ammonification and nitrification needs to be evaluated and optimized as a whole. This illustrates a possible trade-off between the overall BLSS efficiency and crop cultivation and yield, which should be considered in a long-term perspective with continuous operation. Increased knowledge on plant responses to various optimal and sub-optimal cultivation conditions and nutrient solutions will contribute to improved computer models, BLSS efficiency and improvement in design and control of integrated processes linking waste management and crop cultivation.
4.1 Biomass production and morphology
In this research campaign, considerable differences were identified between lettuce plants cultivated in nutrient solutions containing high and low NH4+:N ratios. In a BLSS perspective, the results demonstrate that both NH4+:N ratios, representing considerably different scenarios of upstream waste treatment, allow plant growth and development, and thus fixation of C and N into edible biomass. There is, however, a clear impact on biomass productivity (produced amount per time) and therefore on the total production over a given time. Under the conditions tested during the 28-day crop tests performed in this campaign, the HiNH4+ crop provided 40% lower edible biomass (shoot dry weight), resulting in the incorporation of 35% less C and 48% less N into edible biomass compared to the crop cultivated at low NH4+:N. These results are in line with previous research on lettuce, illustrating that increased ammonium concentrations strongly affect growth, resulting in decreased root and shoot biomass accumulation (Qiu et al., 2014; Wenceslau et al., 2021; Du et al., 2022; Hameed et al., 2022). Furthermore, the results are in line with general effects of ammonium toxicity on plant growth, demonstrating a biomass-reducing effect of high ammonium concentrations attributed to for example oxidative stress, nutrient imbalances and reduced photosynthesis (Barickman and Kopsell, 2016; Song et al., 2021; Weil et al., 2021). In line with the biomass results, the total leaf area per plant at time of harvest was reduced by a 2-factor for the HiNH4+ plants compared to the LoNH4+ plants. This effect is supported by other reports on hydroponically grown lettuce and other plants, demonstrating lower leaf areas with increasing ammonium concentrations (Guo et al., 2007a; Guo et al., 2007b; Urlic et al., 2017; Wenceslau et al., 2021). Beyond the NH4+:N ratio, other potential stress factors include the nutrient solution’s total ionic strength and the presence of Na+ and Cl- added to mimic a urine recycling scenario. While several studies indicate no or minor growth retardation of lettuce cultivated in the presence of seawater, elevated electrical conductivity and/or Na+ and Cl- concentrations similar to the cultivation conditions used in the presented work (Atzori et al., 2019 and references within), one should not rule out combined effects of salinity and NH4+:N ratio especially in a foreseen future closed-loop scenario with accumulating Na+ and Cl- concentrations and increasing total electrical conductivity.
Calculation of PLAI throughout the crop tests offered a method to evaluate plant growth and PLAI-based growth rate without opening the closed cultivation chamber. While noticeable differences in PLAI (and thus biomass) between the HiNH4+ and LoNH4+ crops could not easily be observed until after approximately 10 days of cultivation, calculation of µPLAI indicated a difference in specific growth rate between the two crops starting already in the early cultivation phase. This indicates that under the conditions tested, the elevated NH4+:N ratio introduced negative effects on plant growth also during the close-to-exponential cultivation period. At the same time, this indicates that the observed NO2- in the nutrient solution was not the main cause of the observed differences in biomass production (and thus O2 production, see below). While elevated NO2- concentrations in the nutrient solution may cause reduced growth (Hoque et al., 2008), NO2- was not observed until the late cultivation phase of the HiNH4+ crop, at a time which µPLAI of both crops had started to decrease and coincided. Coinciding µPLAI towards the end of the cultivation period may be explained by reduced specific growth rates as plants mature, and the fact that the presented growth rates are based on PLAI which, by definition, cannot exceed a factor of 1 due to a limited cultivation area and overlapping leaves.
Beyond the overall reduction in biomass production of the HiNH4+ crop, these plants displayed a significantly higher root:shoot ratio than the LoNH4+ plants, indicating a higher relative use of energy towards root growth. This is in line with previous research showing increasing root:shoot ratio with increasing NH4+:NO3- ratio in several species, including lettuce (Zhu et al., 2020), tobacco (Walch-Liu et al., 2000) and cucumber (Zhou et al., 2017). More specifically, alteration of biomass partitioning between root and shoot is a known plant response to nutritional stress (Maskova and Herben, 2018) and NH4+:K+ imbalance has been demonstrated to negatively influence carbohydrate accumulation, disturbing the root-to-shoot biomass partitioning (Zhao et al., 2020). In our study, NH4+ and K+ were among the nutrient ions that demonstrated the greatest differences between the biomass content of the HiNH4+ and LoNH4+ crops. Additionally, the two crops demonstrated differences in root size and morphology. The HiNH4+ plants exhibited shorter primary and secondary roots, along with a significantly lower number of forks and links illustrating less branching. This is likely to be caused by the nutrient availability (especially of NH4+ and NO3-) and plant nutritional status, which are known to strongly influence the development of the root system (Lima et al., 2010). NH4+ is known to affect root architecture by inhibition of primary root growth and elongation, while promoting lateral branching (Liu and Von Wiren, 2017; Liu et al., 2013). Conversely, NO3- has been shown to stimulate lateral root elongation (Lima et al., 2010; Marschner and Marschner, 2012). These observations represent valuable details for mathematical modelling of plant growth and development, as the root’s surface area is critical for mass and energy transfer.
4.2 O2 production
In total, the HiNH4+ crop produced 41% less O2 than the LoNH4+. However, as the growth of the HiNH4+ plants was impaired, the O2 production should be evaluated also in terms of specific production. When O2 production was normalized by shoot dry weight at harvest, the amount of O2 produced was 37 mol·kg-1 for both the LoNH4+ and the HiNH4+ crops. On a high level, O2 production following biomass production is in line with the general mechanism of photosynthesis, converting light energy to chemical energy utilized for the synthesis and accumulation of organic matter (Evert and Eichhorn, 2013; Taiz, 2015), and hence, the photosynthetic rate is determining for biomass productivity. Furthermore, measurements of chlorophyll fluorescence on dark-adapted leaves demonstrated that the Fv/Fm of both crops (0.83) was within a range indicating a healthy and well-functioning photosystem II (0.79 – 0.84) (Bjorkman and Demmig, 1987).
The total O2 production, when normalized per unit leaf area at harvest, was 1.8 and 2.1 mol·m-2 for the LoNH4+ and HiNH4+ crops, respectively. Similarly, O2 production per PLA demonstrates no reduction in specific O2 production of the HiNH4+ plants compared to the LoNH4+ plants. On the contrary, detailed examination of the data indicates a marginal increase of the specific O2 production per PLA by the HiNH4+ plants during the second and third week of the cultivation period. This indication is in line with a higher SPAD index of the leaves of the HiNH4+ plants, and a color index more towards green compared to the LoNH4+ plants. These findings are again in line with other investigations on both lettuce and species such as Arabidopsis and kohlrabi, demonstrating a higher chlorophyll concentration in leaves of plants cultivated with a higher proportion of NH4+ in the fertilizer (Blanke et al., 1996; Qiu et al., 2014; Hu et al., 2015). However, the scientific literature on chlorophyll content as a response to NH4+ concentration and NH4+:NO3- ratio demonstrate complex relationships and varying results. Song et al. (2021) demonstrated that lettuce and cabbage chlorophyll content was higher in plants exposed to an NH4+:NO3- ratio of 50:50, than both 0:100 and 100:0, while Zhu et al. (2020) reported a higher chlorophyll concentration in lettuce seedlings exposed to an NH4+:NO3- ratio 25:75 than both 100:0, 50:50 and 0:100. Studies of other species also show varying results, for example, an increase in chlorophyll content with increasing NH4+:NO3- ratios was not discovered in kale (Assimakopoulou et al., 2019). Different theories are proposed to explain an increased chlorophyll content as a response to high ammonium. The higher chlorophyll concentration may be caused by ammonium-stress inhibiting leaf expansion, resulting in a denser chlorophyll content rather than an actual total increase (Sanchez-Zabala et al., 2015). Furthermore, carbon skeletons in the leaves favor NH4+ assimilation. Based on this, it has been proposed that a higher chlorophyll content might be a strategy to increase photosynthetic CO2 assimilation to produce more carbon skeletons and thereby mitigate NH4+ accumulation (Sanchez-Zabala et al., 2015).
4.3 Biomass composition
The 3-fold increase of root NH4+ content of the HiNH4+ plants compared to the LoNH4+ plants is comparable to the difference between the two nutrient solutions, with 3 to 5-fold elevated NH4+ concentration (at start and end, respectively) and 3 to 7-fold elevated NH4+:N ratio in the HiNH4+ nutrient solution compared to the LoNH4+ nutrient solution. Such elevated root NH4+ content is consistent with the roots being the primary site for initial NH4+ accumulation, where it is incorporated into organic complexes, while N is typically transported to the shoots in other forms such as amino acids (Mengel and Kirkby, 2001; Marschner and Marschner, 2012). Additionally, the biomass NO3- content varied considerably between the crops, and in line with the nutrient solution composition. The shoots and roots of the HiNH4+ plants demonstrated an 87% and 85% reduction compared to those of the LoNH4+ plants, respectively, in line with the 42% lower NO3- concentration in the nutrient solution. A similar reduction of leaf NO3- content with increasing NH4+:N ratio in the nutrient solution was reported for example for rocket salad (Kim et al., 2006). The average nitrate content of the LoNH4+ and HiNH4+ edible biomass (shoots) was 6.0 and 0.8 g NO3-N per kg dry weight, respectively (Table 4). Considering the shoot dry weight content (Table 2), this corresponds to 1.8 g and 0.3 g NO3- per kg fresh weight, respectively, both below the maximum nitrate levels set by the European Union (Commission Regulation No 1258/2011).
Analyses of the shoot and root content of nutrient ions beyond N species demonstrated an overall trend of similar or lower content of both anions and cations in the HiNH4+ plants compared to the LoNH4+ plants. As demonstrated by the ionic concentration trend of the nutrient solution, all macronutrients beyond NH4+ were available at relatively stable concentrations throughout the crop tests, indicating that the differences between the two crops were not governed by nutrient availability, but rather by direct or indirect consequences of the difference in NH4+ concentration and/or NH4+:N ratio. This is in line with reports on reduced uptake of ions such as K+, Ca2+ and Mg2+ with increasing NH4+ concentration and NH4+:NO3- ratio in the nutrient solution (Britto and Kronzucker, 2002; Savvas et al., 2006; Roosta and Schjoerring, 2008; Fallovo et al., 2009). For example, Weil et al. (2021) reported that the concentration of both K+ and Ca2+ in lettuce shoots declined significantly with increasing nutrient solution NH4+:N ratio, concluding that the uptake of cationic nutrients and the plant growth were reduced when the NH4+:N ratio exceeded 0.50 mol·mol-1. The observed effects on nutrient ion content have been explained by antagonism (Lasa et al., 2001; Marschner and Marschner, 2012); that the NH4+ ion resembles the K+ ion in radius and hydration shell size and may therefore move through K+ channels (Marschner and Marschner, 2012), and that uptake of NO3- occurs simultaneously with the uptake of Ca2+ or K+ so that an increasing proportion of NO3- will increase the content of Ca2+ and K+ in leaves (Du et al., 2022).
The content of NaCl in the nutrient solutions, which was designed to mimic a urine recycling scenario, may have impacted plant nutrient uptake in both the high and low NH4+- scenarios. In general, excess Na+ uptake lowers the uptake of essential ions and disturbs the root-shoot transport. It has been widely observed that Na+, even at low levels, inhibits the transport systems for K+ uptake due to the chemical similarities between the two ions (Amini and Ehsanpour, 2005; Kronzucker et al., 2013). For some functions, Na+ may also replace potassium, such as for example for maintenance of vacuole osmotic potential (Wakeel et al., 2011). Na+ may cause osmotic stress, which decreases the passive uptake of calcium, resulting in a lower concentration of calcium in the plant. Another possible effect of NaCl in plant nutrition, is that Cl- may antagonize the uptake of nitrate, while it assists uptake of ammonium (Song et al., 2021).
The HiNH4+ lettuce crop demonstrated a higher carbon content in both leaves and roots compared to the LoNH4+ plants. During stress conditions that affect plant growth but still allow for photosynthesis, accumulation of sugars is commonly observed Peshev and Van Den Ende, 2013). Exposed to high NH4+ concentrations, plants have been shown to synthesize sugars to provide carbon skeletons to increase NH4+ assimilation (Raab and Terry, 1994). Additionally, plants exposed to abiotic stress often accumulate reactive oxygen species (ROS). Accumulation of soluble sugars can mitigate this toxic effect caused by plant stress by contributing to ROS scavenging (Keunen et al., 2013; Peshev and Van Den Ende, 2013). Sugars can also protect chloroplasts and thereby stabilize photosynthesis under stress conditions, and they may serve as osmoprotectants ( Peshev and Van Den Ende, 2013). Additionally, the uptake of NO3- against the electrochemical gradient requires more use of fixed carbon compared to acquisition of NH4+ (Britto and Kronzucker, 2013).
4.4 Nutrient consumption and nutrient solution evolution in a long-term perspective
Maximizing resource utilization limits the possibilities of flushing the hydroponic loop and restarting the system with a fresh and balanced nutrient solution. Thus, long term development of the nutrient solution needs to be understood, modelled, and ultimately designed and controlled in a trade off with the productivity of the plants and the design and performance of upstream processes. In light of the observed effects of the NH4+:N ratio, the development of this ratio over time should be considered. The results obtained under the conditions tested indicate a preference for NH4+ during the HiNH4+ crop test, in which N consumption apparently almost exclusively could be attributed to NH4+ consumption, although one cannot rule out that a modest nitrification activity could convert some NH4+ to NO3- and thus mask some NO3- consumption by the plants. Also, during the LoNH4+ crop test, the consumption of NH4+ relative to total N in the system was higher than the concentration of NH4+ relative to total N in the nutrient solution. In consequence, the nutrient solution NH4+:N ratio decreased over time during both crop tests (from 0.12 to 0.02 mol·mol-1 in the LoNH4+ crop test; from 0.49 to 0.20 in the HiNH+4 crop test). Even after a hypothetical addition of nutrient stock solutions to restore the total N concentration before an additional plant cultivation period, the initial NH4+:N ratio of the second period will be lower than that of the first (0.03 mol·mol-1 after topping up the remaining LoNH4+ nutrient solution to the initial 11 mM total N; 0.29 mol·mol-1 after topping up the remaining HiNH4+ nutrient solution to the initial 11 mM total N). These considerations illustrate a potential to combine high-NH4+:N stock solutions generated by upstream waste treatment processes with efficient long-term plant biomass and O2 productivity despite initially reduced productivity. In a modelling perspective, it illustrates the importance of understanding the relative NH4+:NO3- consumption in the higher plant compartment combined with the effects of the NH4+:NO3- ratio of the mineralized waste from upstream compartments. High NH4+ to NO3- preference has been observed in several other studies, including a study of N absorption by tomato, in which 50% of plant N was absorbed as NH4+ even though this ion represented only 10% of available N (the remaining 90% being NO3-) (Glass et al., 2002). N preference may be linked to energy considerations as uptake of NO3- requires energy while NH4+ can be directly absorbed, hence many plants prefer to take up NH4+ if both are available (Mengel and Kirkby, 2001; Marschner and Marschner, 2012; Du et al., 2022). Additionally, NO3- uptake is inhibited by ammonium, and lettuce appears to absorb ammonium faster than NO3- when the source contains both N-forms (Savvas et al., 2006; Britto and Kronzucker, 2013). However, plant preference for NH4+ or NO3- is known to vary across plant species, physiological phase and environmental conditions (Britto and Kronzucker, 2013), and thus observed N preference may only be valid for a given species, developmental stage, and cultivation conditions. An improved understanding of the NH4+ to NO3- preference, for example as function of cultivation conditions, should be further pursued, as this may hold potential to reduce ammonium toxicity even at conditions of high NH4+:N ratios.
During the LoNH4+ crop test which required a considerable amount of nutrient stock solution feeding, accumulation of Na+, Ca2+ and to a certain extent Cl- was observed together with a marginal reduction of K+. This illustrates imbalance between the system’s feed rates and consumption rates. Nutrient ion levels in the nutrient stock solutions may be tuned relative to for example total N as far as charge balancing allows in order to achieve a balanced nutrient solution. Accumulation of Na+ and Cl-, however, represents a potential general challenge for the utilization of mineralized human waste. Accumulation of Na+ in the nutrient solution may to a certain extent be mitigated by increased plant Na+ uptake rates with increasing Na+ concentrations in the nutrient solution (Neocleous and Savvas, 2017; Breś et al., 2022). Nevertheless, the extent and the implications of long-term accumulation of non-nutrients in crop cultivation based on human waste are important aspects that would benefit from further attention in a BLSS perspective.
The ratio between consumed NH4+ and consumed NO3- affects nutrient solution pH. NH4+ uptake causes release of protons and thereby reduced pH in the root zone, which again may impact nutrient uptake (Lasa et al., 2001; Marschner and Marschner, 2012; Weil et al., 2021). This effect was clearly demonstrated during the HiNH4+ crop test with a substantial amount of base added to maintain a constant pH. The HiNH4+ scenario, with an NH4+:N ratio of 0.5 mol·mol-1 is based on urine ammonification and partly nitrification without addition of alkalinity (Larsen et al., 2021). In a BLSS scenario, the results presented illustrate that no or low alkalinity addition during the nitrification step results in increased alkalinity requirement during the crop cultivation step instead. In this case, addition of OH- during crop cultivation should be carefully balanced with appropriate anions to avoid nutrient imbalance over time. In this context, it is interesting to notice that addition of macronutrient ions prone to be present at low concentrations in mineralized urine due to precipitation (such as Ca2+) could possibly serve a dual purpose of being a counter ion for OH- charge balancing and being a required nutrient solution supplement. Such feeding strategies must, however, be carefully tuned and consider factors such as salt availability and solubility.
4.5 Strategies to maximize food and O2 production in a BLSS perspective
In a Lunar greenhouse perspective, cultivation area will be limited and should be utilized in an efficient way. In a scenario of constant plant density throughout the cultivation period, plants should be cultivated to an adult stage, as the plant growth rate (g per day) and the O2 production rate (mol per day) are highest during the end of the cultivation period, thus increasing also the average growth rate and the average O2 production rate as evaluated over the full cultivation period. However, such a strategy comes at the cost of a low utilization rate of the available cultivation area (low PLAI), especially during the first part of the cultivation period. In a more optimized scenario, initial plant density can be high to achieve a high PLAI even with small plants. As the plants grow, the plant density can be reduced to give room for the expanding plants. In such an optimized scenario, specific production per time and PLA should be considered when aiming at optimizing food (biomass) and O2 production. Under the conditions tested, the specific O2 production per time and PLA reached a maximum around 13 DAT (Figure 6B), indicating that adolescent lettuce plants were more effective in O2 production per time and PLA than seedlings and adult plants. With O2 production linked to biomass production, this period with the highest specific O2 production per time and PLA coincides with the period of highest specific growth rate (here estimated as µPLAI; Figure 4). Thus, in an optimized scenario with dynamic plant density to continuously operate at a high PLAI, the results obtained under the conditions tested suggest that plants should be cultivated up to approximately 16 - 20 days after transplant (26 - 30 days after germination) to maintain a maximized specific biomass (food) production per time and PLA and a maximized specific O2 production per time and PLA. Furthermore, it is interesting to notice the indication of a higher O2 production per time and PLA of the HiNH4+ crop compared to that of the LoNH4+ crop (Figure 6B), illustrating that as long as PLAI is kept high, O2 productivity may be high even under cultivation conditions suboptimal for growth. Such an effect, that may be attributed to the plant’s complex physiological response to mitigate suboptimal cultivation conditions, is especially interesting considering the possible need to accept suboptimal conditions for a given BLSS step in order to maximize the total efficiency of the whole loop.
5 Conclusion
The results of this study demonstrate the importance of understanding and considering direct and indirect effects of upstream waste processing on crop cultivation in BLSS. The urine utilization scenarios of 0.1 and 0.5 mol·mol-1 NH4+:N demonstrated significant effects on plant development, plant nutrient composition and O2 production. Under the conditions tested, plants cultivated at the high NH4+:N ratio demonstrated a high preference for ammonium combined with a reduced specific growth rate and thus a reduced total biomass production. O2 production per time and projected leaf area reached a maximum during the adolescent plant phase, coinciding with exponential growth and indicating a strong relationship between biomass and O2 production. Interestingly, the specific O2 production per time and projected leaf area was marginally higher for the plants cultivated at high NH4+:N ratio, in line with a higher chlorophyll content. In a life support perspective, the results illustrate design concepts for crop cultivation strategies depending on the performance of upstream processes (e.g. urine mineralization and high/low NH4+:N) and downstream needs (food or O2). In addition, parameters such as plant density and cultivation duration may be adjusted or balanced against each other to optimize performance. Ultimately, the results may guide the design and control of both crop cultivation and other processes in a regenerative BLSS loop towards a common trade-off and to achieve a greater good rather than optimizing individual processes.
The unique features of the ESA MELiSSA Plant Cultivation Unit (PCU) with its gas-tight atmospheric and hydroponic loops with extensive monitoring and control enabled collection of comprehensive and detailed data on crop O2 production, plant growth via image-based analyses, and nutrient solution dynamics throughout the entire cultivation period. Together with future expanded capabilities of the PCU, such as analyses of CO2 consumption and water production, this will contribute to improved understanding of plant responses and further advancement of computer models for predictions of plant growth and production in various BLSS and cultivation scenarios.
This study represents a further advancement towards increased waste- and resource recycling in plant-based food production systems. A deeper understanding of these processes and their impacts is crucial for the production of food and for the regeneration of water and O2 in future human space exploration. Additionally, considering relevant terrestrial challenges such as depletion of mineral resources and pollution of ground waters due to nutrient runoff, nutrient recycling fuels sustainable agriculture also on Earth. Exploitation of waste streams for plant production illustrates synergies between space exploration and terrestrial food production, and knowledge on plant responses to different resource utilization scenarios improves the development and design of systems and processes for both Earth and space.
Data availability statement
The raw data supporting the conclusions of this article will be made available by the authors, without undue reservation.
Author contributions
ØJ, MS and A-IJ designed the experiment. MS, ØJ, AP and CQ executed the crop tests and performed analyses and calculations. All authors contributed to interpretation of results. MS and ØJ prepared the draft manuscript. All authors contributed to the article and submitted and approved the submitted section.
Funding
This research was funded by the European Space Agency (ESA) through the MELiSSA PaCMan 2 project (Plant characterization unit for closed life support system - engineering, manufacturing and testing), contract number 4000115852/15/NL/AT.
Acknowledgments
The authors would like to thank Luigi Duri (University of Naples Federico II) for his invaluable assistance during the laboratory work. Furthermore, the full PaCMan project team is acknowledged for the PCU development efforts, with special thanks for valuable technical and scientific discussions to Lorenzo Bucchieri (EnginSoft S.p.A.), Emmanuel Frossard, Geremia Pellegri and Icíar Giménez de Azcárate Bordóns (ETH Zürich), Youssef Rouphael and Stefania de Pascale (University of Naples Federico II), Adam Harper and Matt Whiteley (Hosokawa Micron Ltd.), Gionata Cimini (Odys S.r.l.) and Christel Paille (ESA/ESTEC).
Conflict of interest
CQ is employed by EnginSoft S.p.a.
The remaining authors declare that the research was conducted in the absence of any commercial or financial relationships that could be construed as a potential conflict of interest.
Publisher’s note
All claims expressed in this article are solely those of the authors and do not necessarily represent those of their affiliated organizations, or those of the publisher, the editors and the reviewers. Any product that may be evaluated in this article, or claim that may be made by its manufacturer, is not guaranteed or endorsed by the publisher.
References
Acosta-Motos, J. R., Ortuno, M. F., Bernal-Vicente, A., Diaz-Vivancos, P., Sanchez-Blanco, M. J., Hernandez, J. A. (2017). Plant responses to salt stress: adaptive mechanisms. Agronomy-Basel 7. doi: 10.3390/agronomy7010018
Amini, F., Ehsanpour, A. A. (2005). Soluble Proteins, Proline, Carbohydrates and Na+/K+ Changes in Two Tomato (Lycopersicon esculentum Mill.) Cultivars under in vitro Salt Stress. Am. J. Biochem. Biotechnol. 1, 212–216. doi: 10.3844/ajbbsp.2005.212.216
Andrews, M., Raven, J. A., Lea, P. J. (2013). Do plants need nitrate? The mechanisms by which nitrogen form affects plants. Ann. Appl. Biol. 163, 174–199. doi: 10.1111/aab.12045
Assimakopoulou, A., Salmas, I., Kounavis, N., Bastas, A. I., Michopoulou, V., Michail, E. (2019). The impact of ammonium to nitrate ratio on the growth and nutritional status of kale. Notulae Botanicae Horti Agrobotanici Cluj-Napoca 47, 848–859. doi: 10.15835/nbha47311466
Atzori, G., Mancuso, S., Masi, E. (2019). Seawater potential use in soilless culture: A review. Scientia Hortic. 249, 199–207. doi: 10.1016/j.scienta.2019.01.035
Barickman, T. C., Kopsell, D. A. (2016). Nitrogen form and ratio impact Swiss chard (Beta vulgaris subsp cicla) shoot tissue carotenoid and chlorophyll concentrations. Scientia Hortic. 204, 99–105. doi: 10.1016/j.scienta.2016.04.007
Berkovich, Y. A., Chetirkin, P. V., Wheeler, R. M., Sager, J. C. (2004). Evaluating and optimizing horticultural regimes in space plant growth facilities. Adv. Space Res. 34, 1612–1618. doi: 10.1016/j.asr.2003.08.080
Bjorkman, O., Demmig, B. (1987). Photon Yield of O-2 Evolution and Chlorophyll Fluorescence Characteristics at 77-K among Vascular Plants of Diverse Origins. Planta 170, 489–504. doi: 10.1007/BF00402983
Blanke, M. M., Bacher, W., Pring, R. J., Baker, E. A. (1996). Ammonium nutrition enhances chlorophyll and glaucousness in Kohlrabi. Annals of Botany 78, 599–604.
Bonvin, C., Etter, B., Udert, K. M., Frossard, E., Nanzer, S., Tamburini, F., et al. (2015). Plant uptake of phosphorus and nitrogen recycled from synthetic source-separated urine. Ambio 44 Suppl 2, S217–S227. doi: 10.1007/s13280-014-0616-6
Breś, W., Kleiber, T., Markiewicz, B., Mieloszyk, E., Mieloch, M. (2022). The effect of naCl stress on the response of lettuce (Lactuca sativa L.). Agronomy 12, 244. doi: 10.3390/agronomy12020244
Britto, D. T., Kronzucker, H. J. (2002). NH4+ toxicity in higher plants: a critical review. J. Plant Physiol. 159, 567–584. doi: 10.1078/0176-1617-0774
Britto, D. T., Kronzucker, H. J. (2013). Ecological significance and complexity of N-source preference in plants. Ann. Bot. 112, 957–963. doi: 10.1093/aob/mct157
Chinnusamy, V., Zhu, J., Zhu, J. K. (2006). Salt Stress Signaling and Mechanisms of Plant Salt Tolerance. In: Setlow, J.K. (eds) Genetic Engineering. Genetic Engineering: Principles and Methods (Boston, MA: Springer). 27, 141–177. doi: 10.1007/0-387-25856-6_9
De Bang, T. C., Husted, S., Laursen, K. H., Persson, D. P., Schjoerring, J. K. (2021). The molecular-physiological functions of mineral macronutrients and their consequences for deficiency symptoms in plants. New Phytol. 229, 2446–2469. doi: 10.1111/nph.17074
Du, K. K., Zhang, J. L., Han, Y. Y., Hao, J. H., Qin, X. X., Liu, C. J., et al. (2022). Effects of varying NO3-:NH4+ Ratios on lettuce (Lactuca sativa L.) nitrogen metabolism. Pakistan J. Bot. 54, 2081–2088. doi: 10.30848/PJB2022-6(37)
Evert, R. F., Eichhorn, S. E. (2013). Raven biology of plants (Peter Marshall, Macmillan Education).
Fallovo, C., Colla, G., Schreiner, M., Krumbein, A., Schwarz, D. (2009). Effect of nitrogen form and radiation on growth and mineral concentration of two Brassica species. Scientia Hortic. 123, 170–177. doi: 10.1016/j.scienta.2009.09.003
Glass, A. D. M., Britto, D. T., Kaiser, B. N., Kinghorn, J. R., Kronzucker, H. J., Kumar, A., et al. (2002). The regulation of nitrate and ammonium transport systems in plants. J. Exp. Bot. 53, 855–864. doi: 10.1093/jexbot/53.370.855
Godia, F., Albiol, J., Montesinos, J. L., Perez, J., Creus, N., Cabello, F., et al. (2002). MELISSA: a loop of interconnected bioreactors to develop life support in space. J. Biotechnol. 99, 319–330. doi: 10.1016/S0168-1656(02)00222-5
Guo, S. W., Chen, G., Zhou, Y., Shen, Q. R. (2007b). Ammonium nutrition increases photosynthesis rate under water stress at early development stage of rice (Oryza sativa L.). Plant Soil 296, 115–124. doi: 10.1007/s11104-007-9302-9
Guo, S. W., ZHOU, Y., GAO, Y. X., LI, Y., SHEN, Q. R. (2007c). New insights into the nitrogen form effect on photosynthesis and photorespiration. Pedosphere 17, 601–610. doi: 10.1016/S1002-0160(07)60071-X
Guo, S., Zhou, Y., Shen, Q., Zhang, F. (2007a). Effect of ammonium and nitrate nutrition on some physiological processes in higher plants - Growth, photosynthesis, photorespiration, and water relations. Plant Biol. 9, 21–29. doi: 10.1055/s-2006-924541
Halbert-Howard, A., Häfner, F., Karlowsky, S., Schwarz, D., Krause, A. (2021). Evaluating recycling fertilizers for tomato cultivation in hydroponics, and their impact on greenhouse gas emissions. Environ. Sci. pollut. Res. 28, 59284–59303. doi: 10.1007/s11356-020-10461-4
Hameed, M. K., Umar, W., Razzaq, A., Aziz, T., Maqsood, M. A., Wei, S. W., et al. (2022). Differential metabolic responses of lettuce grown in soil, substrate and hydroponic cultivation systems under NH4+/NO3- application. Metabolites 12. doi: 10.3390/metabo12050444
Hoque, M. M., Ajwa, H. A., Smith, R. (2008). Nitrite and ammonium toxicity on lettuce grown under hydroponics. Commun. Soil Sci. Plant Anal. 39, 207–216. doi: 10.1080/00103620701759194
Hu, L. L., Yu, J. H., Liao, W. B., Zhang, G. B., Xie, J. M., Lv, J., et al. (2015). Moderate ammonium:nitrate alleviates low light intensity stress in mini Chinese cabbage seedling by regulating root architecture and photosynthesis. Scientia Horticulturae 186, 143–153.
Keunen, E., Peshev, D., Vangronsveld, J., Van Den Ende, W., Cuypers, A. (2013). Plant sugars are crucial players in the oxidative challenge during abiotic stress: extending the traditional concept. Plant Cell Environ. 36, 1242–1255. doi: 10.1111/pce.12061
Khodadad, C. L. M., Hummerick, M. E., Spencer, L. E., Dixit, A. R., Richards, J. T., Romeyn, M. W., et al. (2020). Microbiological and nutritional analysis of lettuce crops grown on the international space station. Front. Plant Sci. 11. doi: 10.3389/fpls.2020.00199
Kim, S. J., Kawaharada, C., Ishii, G. (2006). Effect of ammonium: nitrate nutrient ratio on nitrate and glucosinolate contents of hydroponically-grown rocket salad (Eruca sativa Mill.). Soil Sci. Plant Nutr. 52, 387–393. doi: 10.1111/j.1747-0765.2006.00048.x
Kronzucker, H. J., Coskun, D., Schulze, L. M., Wong, J. R., Britto, D. T. (2013). Sodium as nutrient and toxicant. Plant Soil 369, 1–23. doi: 10.1007/s11104-013-1801-2
Larsen, T. A., Riechmann, M. E., Udert, K. M. (2021). State of the art of urine treatment technologies: A critical review. Water Research X, 13. doi: 10.1016/j.wroa.2021.100114
Lasa, B., Frechilla, S., Lamsfus, C., Aparicio-Tejo, P. M. (2001). The sensitivity to ammonium nutrition is related to nitrogen accumulation. Scientia Hortic. 91, 143–152. doi: 10.1016/S0304-4238(01)00239-4
Lima, J. E., Kojima, S., Takahashi, H., Von Wiren, N. (2010). Ammonium triggers lateral root branching in Arabidopsis in an AMMONIUM TRANSPORTER1;3-dependent manner. Plant Cell 22, 3621–3633. doi: 10.1105/tpc.110.076216
Liu, Y., Lai, N. W., Gao, K., Chen, F. J., Yuan, L. X., Mi, G. H. (2013). Ammonium inhibits primary root growth by reducing the length of meristem and elongation zone and decreasing elemental expansion rate in the root apex in arabidopsis thaliana. PloS One 8. doi: 10.1371/journal.pone.0061031
Liu, Y., Von Wiren, N. (2017). Ammonium as a signal for physiological and morphological responses in plants. J. Exp. Bot. 68, 2581–2592. doi: 10.1093/jxb/erx086
Marschner, H., Marschner, P. (2012). Marschner’s mineral nutrition of higher plants (London, UK: Elsevier).
Maskova, T., Herben, T. (2018). Root:shoot ratio in developing seedlings: How seedlings change their allocation in response to seed mass and ambient nutrient supply. Ecol. Evol. 8, 7143–7150. doi: 10.1002/ece3.4238
Mauerer, M., Rocksch, T., Dannehl, D., Schuch, I., Mewis, I., Förster, N., et al. (2018). Impact of different concentrations of nitrified urine in a recirculating nutrient solution on growth, yield and quality of lettuce. DGG Proc. 8, 1–5. doi: 10.5288/dgg-pr-mm-2018
Mengel, K., Kirkby, E. A. (2001). Principles of plant nutrition 5th edition (Dordrecht, Netherlands: Kluwer Academic Publishers).
Neocleous, D., Savvas, D. (2017). Simulating NaCl accumulation in a closed hydroponic crop of zucchini: Impact on macronutrient uptake, growth, yield, and photosynthesis. J. Plant Nutr. Soil Sci. 180, 283–293. doi: 10.1002/jpln.201600338
Pannico, A., Cimini, G., Quadri, C., Paradiso, R., Bucchieri, L., Rouphael, Y., et al. (2022). A plant characterization unit for closed life support. Front. Astronomy Space Sci. 9. doi: 10.3389/fspas.2022.820752
Pannico, A., El-Nakhel, C., Kyriacou, M. C., Giordano, M., Stazi, S. R., De Pascale, S., et al. (2019). Combating micronutrient deficiency and enhancing food functional quality through selenium fortification of select lettuce genotypes grown in a closed soilless system. Front. Plant Sci. 10. doi: 10.3389/fpls.2019.01495
Peiro, E., Pannico, A., Colleoni, S. G., Bucchieri, L., Rouphael, Y., De Pascale, S., et al. (2020). Air distribution in a fully-closed higher plant growth chamber impacts crop performance of hydroponically-grown lettuce. Front. Plant Sci. 11. doi: 10.3389/fpls.2020.00537
Peshev, D., Van Den Ende, W. (2013). “Sugars as antioxidants in plants,” in Crop improvement under adverse conditions. Eds. Tuteja, N., Gill, S. S. (London: Springer).
Qiu, Z. P., Yang, Q. C., Liu, W. K. (2014). Effects of nitrogen fertilizer on nutritional quality and root secretion accumulation of hydroponic lettuce. Int. Symposium New Technol. Environ. Control Energy-Saving Crop Production Greenhouse Plant Factory - Greensys 2013 1037, 679–685. doi: 10.17660/ActaHortic.2014.1037.87
Raab, T. K., Terry, N. (1994). Nitrogen-source regulation of growth and photosynthesis in beta-vulgaris L. Plant Physiol. 105, 1159–1166. doi: 10.1104/pp.105.4.1159
Roosta, H. R., Schjoerring, J. K. (2008). Effects of nitrate and potassium on ammonium toxicity in cucumber plants. J. Plant Nutr. 31, 1270–1283. doi: 10.1080/01904160802135050
Rouphael, Y., Petropoulos, S. A., Cardarelli, M., Colla, G. (2018). Salinity as eustressor for enhancing quality of vegetables. Scientia Hortic. 234, 361–369. doi: 10.1016/j.scienta.2018.02.048
Sanchez-Zabala, J., Gonzalez-Murua, C., Marino, D. (2015). Mild ammonium stress increases chlorophyll content in Arabidopsis thaliana. Plant Signaling Behav. 10. doi: 10.4161/15592324.2014.991596
Savvas, D., Passam, H. C., Olympios, C., Nasi, E., Moustaka, E., Mantzos, N., et al. (2006). Effects of ammonium nitrogen on lettuce grown on pumice in a closed hydroponic system. Hortscience 41, 1667–1673. doi: 10.21273/HORTSCI.41.7.1667
Song, J., Yang, J., Jeong, B. R. (2021). Growth, Quality, and Nitrogen Assimilation in Response to High Ammonium or Nitrate Supply in Cabbage (Brassica campestris L.) and Lettuce (Lactuca sativa L.). Agronomy-Basel 11. doi: 10.3390/agronomy11122556
Taiz, L. (2015). Plant Physiology and Development, sixth edition (Sunderland CT, USA: Sinauer Associates Inc).
Udert, K. M., Larsen, T. A., Gujer, W. (2006). Fate of major compounds in source-separated urine. Water Sci. Technol. 54, 413–420. doi: 10.2166/wst.2006.921
Udert, K. M., Wachter, M. (2012). Complete nutrient recovery from source-separated urine by nitrification and distillation. Water Res. 46, 453–464. doi: 10.1016/j.watres.2011.11.020
Urlic, B., Spika, M. J., Becker, C., Klaring, H. P., Krumbein, A., Ban, S. G., et al. (2017). Effect of NO3 and NH4 concentrations in nutrient solution on yield and nitrate concentration in seasonally grown leaf lettuce. Acta Agriculturae Scandinavica Section B-Soil Plant Sci. 67, 748–757. doi: 10.1080/09064710.2017.1347704
Wakeel, A., Farooq, M., Qadir, M., Schubert, S. (2011). Potassium substitution by sodium in plants. Crit. Rev. Plant Sci. 30, 401–413. doi: 10.1080/07352689.2011.587728
Walch-Liu, P., Neumann, G., Bangerth, F., Engels, C. (2000). Rapid effects of nitrogen form on leaf morphogenesis in tobacco. J. Exp. Bot. 51, 227–237. doi: 10.1093/jexbot/51.343.227
Weil, S., Barker, A. V., Zandvakili, O. R., Etemadi, F. (2021). Plant growth and calcium and potassium accumulation in lettuce under different nitrogen regimes of ammonium and nitrate nutrition. J. Plant Nutr. 44, 270–281. doi: 10.1080/01904167.2020.1806313
Wenceslau, D., Oliveira, D. F., Rabelo, H., Ferbonink, G. F., Gomes, L. A. A., Leonel, E. C. A., et al. (2021). Nitrate concentration and nitrate/ammonium ratio on lettuce grown in hydroponics in Southern Amazon. Afr. J. Agric. Res. 17, 862–868.
Wheeler, R. M., Stutte, G. W., Subbarao, G. V., Yorio, N. C. (2002). “Plant growth and human life support for space travel,” in Handbook of plant and crop physiology, 2 ed. Ed. Pessarakli, M. (New York: Marcel Dekker Inc).
Zhao, H. Y., Sun, S. M., Zhang, L. H., Yang, J. J., Wang, Z. Y., Ma, F. W., et al. (2020). Carbohydrate metabolism and transport in apple roots under nitrogen deficiency. Plant Physiol. Biochem. 155, 455–463. doi: 10.1016/j.plaphy.2020.07.037
Zheng, G., Moskal, L. M. (2009). Retrieving leaf area index (LAI) using remote sensing: theories, methods and sensors. Sensors 9, 2719–2745. doi: 10.3390/s90402719
Zhou, J. Y., Wang, M., Sun, Y. M., Gu, Z. C., Wang, R. R., Saydin, A., et al. (2017). Nitrate increased cucumber tolerance to fusarium wilt by regulating fungal toxin production and distribution. Toxins 9. doi: 10.3390/toxins9030100
Keywords: ammonium, nitrate, recycling, oxygen, food production, photosynthesis, life support system
Citation: Schiefloe M, Jakobsen ØM, Pannico A, Quadri C and Jost A-IK (2023) From urine to food and oxygen: effects of high and low NH4+:NO3- ratio on lettuce cultivated in a gas-tight hydroponic facility. Front. Plant Sci. 14:1229476. doi: 10.3389/fpls.2023.1229476
Received: 26 May 2023; Accepted: 13 July 2023;
Published: 31 July 2023.
Edited by:
Cyprien Verseux, University of Bremen, GermanyReviewed by:
Roberta Bulgari, University of Turin, ItalyByoung Ryong Jeong, Gyeongsang National University, Republic of Korea
Copyright © 2023 Schiefloe, Jakobsen, Pannico, Quadri and Jost. This is an open-access article distributed under the terms of the Creative Commons Attribution License (CC BY). The use, distribution or reproduction in other forums is permitted, provided the original author(s) and the copyright owner(s) are credited and that the original publication in this journal is cited, in accordance with accepted academic practice. No use, distribution or reproduction is permitted which does not comply with these terms.
*Correspondence: Mona Schiefloe, bW9uYS5zY2hpZWZsb2VAY2lyaXMubm8=; Øyvind Mejdell Jakobsen, b3l2aW5kLm0uamFrb2JzZW5AY2lyaXMubm8=
†These authors have contributed equally to this work and share first authorship