- 1Université Claude Bernard Lyon 1, CNRS, INRAE, VetAgro Sup, UMR5557 Ecologie Microbienne, Villeurbanne, France
- 2University of Belgrade, Faculty of Agriculture, Belgrade, Serbia
Fusarium species are cosmopolitan soil phytopathogens from the division Ascomycota, which produce mycotoxins and cause significant economic losses of crop plants. However, soils suppressive to Fusarium diseases are known to occur, and recent knowledge on microbial diversity in these soils has shed new lights on phytoprotection effects. In this review, we synthesize current knowledge on soils suppressive to Fusarium diseases and the role of their rhizosphere microbiota in phytoprotection. This is an important issue, as disease does not develop significantly in suppressive soils even though pathogenic Fusarium and susceptible host plant are present, and weather conditions are suitable for disease. Soils suppressive to Fusarium diseases are documented in different regions of the world. They contain biocontrol microorganisms, which act by inducing plants’ resistance to the pathogen, competing with or inhibiting the pathogen, or parasitizing the pathogen. In particular, some of the Bacillus, Pseudomonas, Paenibacillus and Streptomyces species are involved in plant protection from Fusarium diseases. Besides specific bacterial populations involved in disease suppression, next-generation sequencing and ecological networks have largely contributed to the understanding of microbial communities in soils suppressive or not to Fusarium diseases, revealing different microbial community patterns and differences for a notable number of taxa, according to the Fusarium pathosystem, the host plant and the origin of the soil. Agricultural practices can significantly influence soil suppressiveness to Fusarium diseases by influencing soil microbiota ecology. Research on microbial modes of action and diversity in suppressive soils should help guide the development of effective farming practices for Fusarium disease management in sustainable agriculture.
1 Introduction
The fungal genus Fusarium encompasses several plant-pathogenic species, which are among the most destructive phytopathogens world-wide, causing diseases on many agricultural crops (Burgess and Bryden, 2012). They are ubiquitous in parts of the world where cereals and other crops are grown and they produce a wide variety of mycotoxins, which may be present in feed and food products (Babadoost, 2018; Moretti et al., 2018; Chen et al., 2019). Consumption of products that are contaminated with mycotoxins may cause acute or chronic effects in both animals and humans, and could result in immune-suppressive or carcinogenic effects (Jard et al., 2011). By producing mycotoxins and by inducing necrosis and wilting in plants, Fusarium fungi are causing huge economic losses of cereal crops throughout the world (Khan et al., 2017). Their broad distribution has been attributed to their ability to develop on different substrates and plant species, and to produce spores that enable efficient propagation (Desjardins, 2006; Arie, 2019). They are typical soil-borne microorganisms, routinely found in plant-associated fungal communities (Reyes Gaige et al., 2020).
Efficient management of plant diseases caused by Fusarium is important to limit crop losses and to reduce mycotoxin production in alimentary products (Babadoost, 2018). Because mycotoxin synthesis can occur not only after harvesting but also before, one of the best ways to reduce its presence in food and feed products is to prevent its formation in the crop (Jard et al., 2011). Over the years, different methods, such as the use of resistant cultivars and chemical fungicides, have been undertaken in order to control or prevent crop diseases (Willocquet et al., 2021). In spite of that, Fusarium continues to cause huge crop losses, up to 70% in South America, 54% in the United States and 50% in Europe in the case of Fusarium head blight (FHB) disease of wheat (Scott et al., 2021).
Alternative control methods, based on plant-protection effects of beneficial microorganisms, have also been investigated (Janvier et al., 2007; Nguyen et al., 2018). Farming practices greatly influence these effects by shaping the rhizosphere microbial community (Campos et al., 2016), stimulating the activity of beneficial rhizosphere microorganisms and restricting the activity of soil-borne Fusarium pathogens (Janvier et al., 2007). Indeed, crop rotation, tillage and addition of organic amendments may provide some control of soil-borne pathogens, through different microbial direct and indirect mechanisms (Janvier et al., 2007). The effect of plant-protecting soil microbiota on plant health is of particular interest in the case of disease-suppressive soils, which were defined by Baker and Cook (1974) as “soils in which the pathogen does not establish or persist, establishes but causes little or no damage, or establishes and causes disease for a while but thereafter the disease is less important, although the pathogen may persist in the soil”. Suppressive soils represent a reservoir of beneficial microorganisms, which may confer effective plant protection against various soil-borne diseases (Gómez Expósito et al., 2017). This biocontrol potential of suppressive soils is of great importance when considering phytopathogens like Fusarium spp., which are causing increasing damage to crops in the on-going climate change context (Babadoost, 2018). Insight into the time and space microbial dynamics of soils suppressive to Fusarium diseases, together with the understanding of microbial modes of action and agricultural practices applied, is needed in order to develop safe, effective, and stable tools for disease management (Gómez Expósito et al., 2017).
By selecting their rhizosphere microbiome (Tkacz et al., 2015; Gruet et al., 2023), plants may contribute themselves to suppressiveness (Almario et al., 2014; Gómez Expósito et al., 2017). Soil represents the richest known reservoir of microbial biodiversity (Curtis et al., 2002; Wang et al., 2016) and displays several compartments, i.e. the bulk soil containing microorganisms that are not affected by the roots, the rhizosphere where soil microorganisms are under the influence of roots (and roots exudates), the rhizoplane with root-adhering microorganisms, and the endosphere for root tissues colonized by microorganisms (Sánchez-Cañizares et al., 2017). The rhizosphere and rhizoplane harbor an abundant community of bacteria, archaea, oomycetes and fungi, whose individual members can have beneficial, deleterious or neutral effects on the plant. The collective genome of this microbial community is larger than that of the plant itself, and is often referred to as the plant’s second genome (Berendsen et al., 2012). Thus, this alliance of the plant and its associated microorganisms represents a holobiont, which has interdependent, fine-tuned and complex functioning (Berendsen et al., 2012; Vandenkoornhuyse et al., 2015; Sánchez-Cañizares et al., 2017). In this system, the plant is a key player, as nearly 40% of all photosynthates are released directly by roots into the rhizosphere, serving as a fuel for microbial communities, thus recruiting and shaping this microbiome (Berendsen et al., 2012; Tkacz and Poole, 2015). These photosynthates are conditioned by the plant genotype, developmental stage, metabolism, immune system and its ability to exudate (Sánchez-Cañizares et al., 2017). In this context, suppressiveness will depend on microbiome diversity and functioning.
This review deals with recent knowledge on soils suppressive to Fusarium diseases, which sheds new lights on molecular and ecological mechanisms underpinning phytoprotection effects and highlights the importance of microbial diversity in the functioning of these suppressive soils. To this end, we summarize current knowledge on Fusarium taxonomy and ecology, and their mechanisms of plant infection. In addition, we review our understanding of biocontrol agents against Fusarium and their modes of action. Finally, we focus on soils suppressive to Fusarium diseases and the importance of farming and environmental factors modulating suppressiveness, with an emphasis on the particularities of the different Fusarium pathosystems.
2 Fusarium phytopathogens and plant diseases
2.1 Fusarium ecology
Fusarium species occur in soils, but they can also grow in and on living and dead plants (Laraba et al., 2021) and animals (Xia et al., 2019), with the ability to live as parasites or saprophytes (Smith, 2007; Summerell, 2019). Some can also be found in caves (Bastian et al., 2010) or in man-made water systems (Sautour et al., 2012). Fusarium species are mostly known as phytopathogens, but some of them have been evidenced as contaminants in industrial processes, indoor environments, or pharmaceutical and food products (Abdel-Azeem et al., 2019), whereas others behave as opportunistic human/animal pathogens (Al-Hatmi et al., 2019; da Silva Santos et al., 2020) or are fungicolous (Torbati et al., 2021).
Focusing on plant-interacting Fusarium species, their saprophytic potential enables them to survive the winter in the crop debris, in the form of mycelium or spores that serve as plant-infecting propagules in the spring (Figure 1A) (Leslie and Summerell, 2006). Fusarium species vary in reproduction strategies, and they produce sexual spores as well as three types of asexual spores, i.e. (i) microconidia, which are typically produced under all environmental conditions, (ii) macroconidia, which are often found on the surface of diseased plants, and (iii) chlamydospores (survival structures), which are thick walled and produced from macroconidia or older mycelium (Ajmal et al., 2023). More than 80% of Fusarium species propagate using asexual spores, but not all of them produce all three types of spores, while sexual reproduction can involve self-fertility or out-crossing (Rana et al., 2017). Additionally, some species produce sclerotia, which promote survival in soil (Leslie and Summerell, 2006).
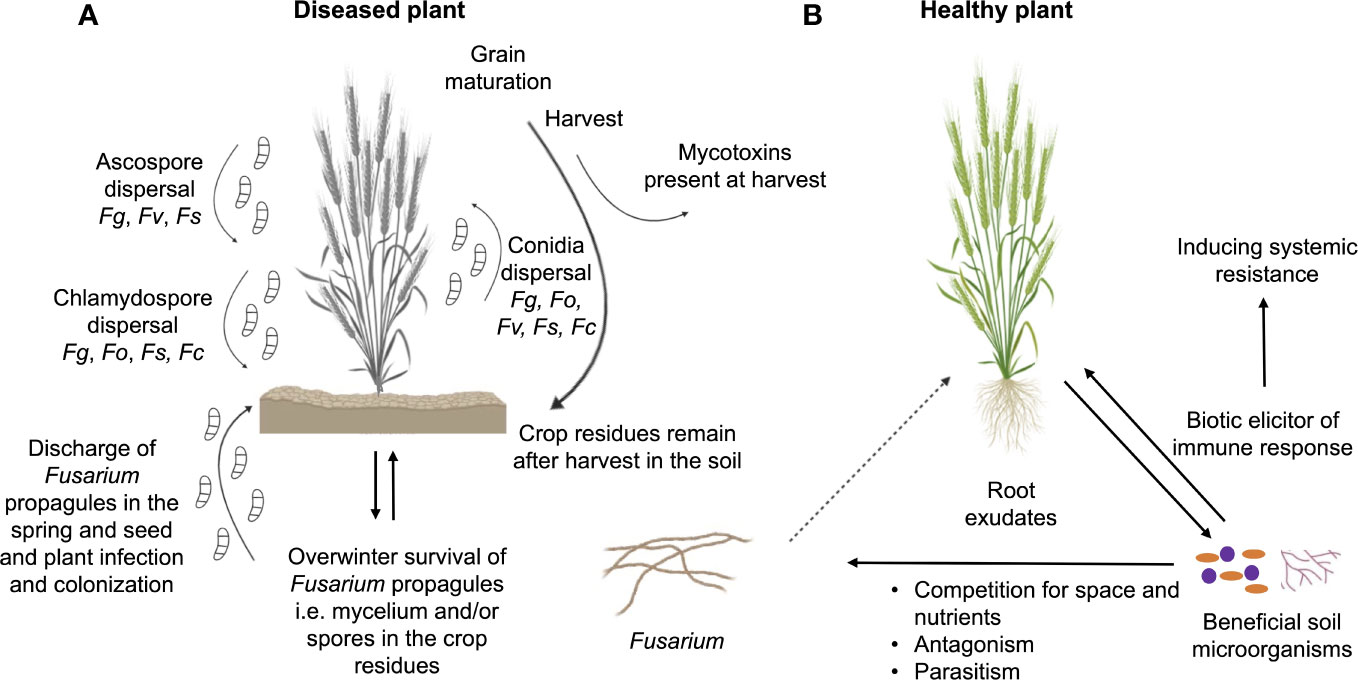
Figure 1 Interactions of Fusarium species with plant and other microbiota members. (A) Life cycle of Fusarium species and their mechanism of plant infection by producing three types of spores: ascospores, conidia and chlamydospores. Fg, F graminearum; Fo, F oxysporum; Fs, F solani; Fc, F culmorum; Fv, F verticillioides. (B) Dynamic interactions between beneficial soil microorganisms, plant and phytopathogenic Fusarium species.
Fusarium shows climatic preferences, as F. oxysporum, F. solani, F. verticillioides (formerly F. moniliforme), F. tricinctum, F. fujikuroi, F. pseudograminearum and F. graminearum are found worldwide, F. culmorum and F. avenaceum in temperate regions, whereas some species occur in tropical or cool regions (Backhouse and Burgess, 2002; Babadoost, 2018; Senatore et al., 2021). The growth of each Fusarium species is largely determined by abiotic environmental conditions, notably temperature and humidity (Table S1) (Xu, 2003; Crous et al., 2021). However, other environmental factors, such as soil characteristics, cropping systems, agricultural practices and other human activities may influence the diversity of Fusarium in soils (Abdel-Azeem et al., 2019; Pfordt et al., 2020; Wang et al., 2020; Du et al., 2022).
2.2 Taxonomy of Fusarium spp.
The Fusarium genus exhibits high level of variability in terms of morphological, physiological and ecological properties, which represents a difficulty in establishing a consistent taxonomy of these species (Burgess et al., 1996). An additional difficulty for classification is the existence of both asexual (anamorph) and sexual (teleomorph) phases in their life cycle (Summerell, 2019). Based on the most widely used classification, the anamorph state of the genus Fusarium is classified in the family Nectriaceae, order Hypocreales and division Ascomycota (Crous et al., 2021). Several teleomorphs have been related to Fusarium species, but not all Fusarium species have a known sexual state in their life cycle (Munkvold, 2017). Most of these teleomorphs are in the genus Gibberella, including the economically important pathogens, such as G. zeae (anamorph F. verticillioides) and G. moniliformis (anamorph F. verticillioides) (Keszthelyi et al., 2007). Other Fusarium teleomorphs are members of the genera Albonectria, Neocosmospora or Haematonectria. Teleomorphs are usually not observed in the field, but rather under lab conditions. The dual anamorph-teleomorph nomenclature for fungi has now been abolished, and the name Fusarium has been retained for these fungi (Geiser et al., 2013).
The genus Fusarium is currently composed of 23 species complexes and at least 69 well-individualized species. Fusarium species complexes are groups of closely-related species with the same morphology, which are strongly supported from a phylogenetic perspective (O’Donnell et al., 2013; O’Donnell et al., 2015; Summerell, 2019; Xia et al., 2019; Laraba et al., 2021; Senatore et al., 2021; Yilmaz et al., 2021), as shown in Figure 2. Within a given Fusarium species, certain strains may be pathogenic while others are not (Fuchs et al., 1997; De Lamo and Takken, 2020; Constantin et al., 2021). However, most phytopathogenic species belong to the F. fujikuroi, F. sambucinum, F. oxysporum, F. tricinctum or F. solani species complexes (O’Donnell et al., 2013; Senatore et al., 2021). Furthermore, Fusarium species capable of infecting a wide range of plants are classified into different formae speciales, based on the host plant they can infect (Edel-Hermann and Lecomte, 2019; Coleman, 2016). Currently, there are 106 well-described F. oxysporum formae speciales (Edel-Hermann and Lecomte, 2019) and 12 well-described F. solani formae speciales (Šišić et al., 2018).
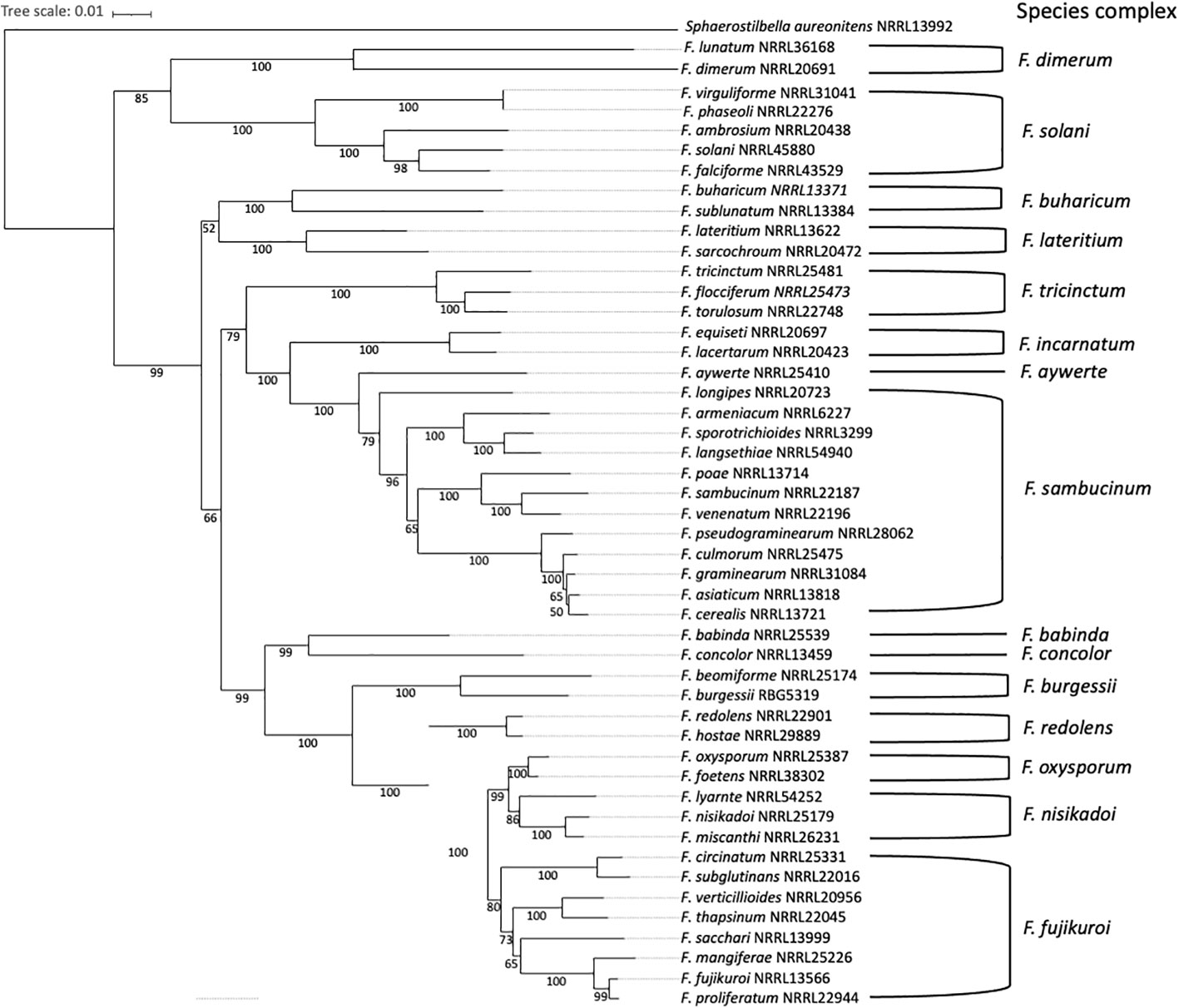
Figure 2 Phylogenetic relationship between pathogenic Fusarium species and 15 different species complexes. The distance-method tree (1000 bootstrap replicates) was inferred from the rpb1 (RNA Polymerase 1) data set, using the SeaView multiplatform (Gouy et al., 2010). The tree was visualized using iTol (Letunic and Bork, 2021). Sphaerostilbella aureonitens NRRL 13992 was used as an outgroup. Species complexes delimitation is based on the phylogeny published in Summerell (2019).
Over the past 100 years, the taxonomy of Fusarium has undergone many changes, but most classification procedures have been based on the size and shape of the macroconidia, the presence or absence of microconidia and chlamydospores, and the structure of the conidiophores (Ristić, 2012). Identification of Fusarium species based on morphological characteristics also included observations of colony pigmentation and type of aerial mycelium (Crous et al., 2021). The standard method now used to identify Fusarium isolates to a species level is to sequence one (or more) of the following genes: translocation elongation factor-1α (tef-1α), RNA polymerase 1 and 2 (rpb1 and rpb2), β-tubulin (tub), histone (his), ATP citrate lyase (acl1) or calmodulin (CaM) (Herron et al., 2015; Summerell, 2019; Crous et al., 2021; Laraba et al., 2021; Yilmaz et al., 2021). The tef-1α gene is a first-choice marker as it has good resolution power for the majority of Fusarium species, while sequencing the gene rpb2 allows differentiation of close species. The other genetic markers mentioned have variable resolution power and are often used together with tef-1α or rpb2 (Crous et al., 2021). The internal transcribed spacer regions of the ribosomal gene (ITS), which are common barcodes to identify fungi, are not recommended for Fusarium identification, as they are not sufficiently informative for a significant number of Fusarium species (Summerell, 2019).
2.3 Mechanisms of Fusarium infection, symptoms and etiology
Before infecting the host plant tissues, soil-borne pathogens may grow in the rhizosphere or on the host as saprophytes, managing to escape the rhizosphere battlefield (Raaijmakers et al., 2009). The outcome is directly influenced by host and microbial defense mechanisms, at the level of the holobiont (Berendsen et al., 2012; Vandenkoornhuyse et al., 2015). During their life cycle, plants are exposed to numerous phytopathogens, and they have developed different adaptive strategies. Upon pathogen attack, both composition and quantity of root metabolites may change (Rolfe et al., 2019), which can be useful for direct defense against the pathogens (Rizaludin et al., 2021), for signaling the impending threat to the neighboring plants (Pélissier et al., 2021), or for recruiting beneficial microorganisms with biocontrol capabilities. The latter phenomenon is referred to as the ‘a cry for help’ strategy (Rizaludin et al., 2021).
If the pathogen manages to escape from the rhizosphere battlefield, the infection cycle can proceed. Plant infection by Fusarium occurs in a few successive stages (Figure 1A), which differs according to Fusarium species. Seeds infected with Fusarium in the previous season can also serve as disease initiators (Jiménez-Díaz et al., 2015). F. graminearum grows saprophytically on crop debris, which is the overwintering reservoir of the pathogen (Brown et al., 2010). The fungus may infect roots and cause damage to the collar (Ares et al., 2004). During the crop anthesis and under warm and humid weather conditions, asexual conidia, sexual ascospores or chlamydospores are dispersed by rain or wind and reach the outer anthers and outer glumes of the plant. After spore germination, hyphae penetrate the host plant through the cracked anthers, followed by inter- and intracellular mycelial growth, resulting in damage to host tissues and especially head blight disease (Brown et al., 2010). Unlike F. graminearum, F. culmorum produces only asexual conidia and chlamydospores, which are also dispersed by rain and wind, reaching plant heads and infecting the ears during the anthesis. Subsequently, conidia germinate on the lemma and palea, followed by inter- and intracellular mycelial growth (Wagacha and Muthomi, 2007). In contrast, the infection cycle of F. oxysporum begins when mycelia, germinating asexual conidia or chlamydspores enter the healthy plant through the root tip, lateral roots or root wounds. The fungus progresses intracellularly, entering the xylem sap flow and being transported to the aerial parts of the plant where it forms infection structures. The infection structures that form close the vascular vessels, disrupt nutrient translocation, leading to stomatal closure, leaf wilting and plant death (Banerjee and Mittra, 2018; Redkar et al., 2022a; Redkar et al., 2022b). In the case of F. verticillioides, infection starts when mycelia, asexual conidia or sexual ascospores are carried inside the seed or on the seed surface and later develop inside the growing plant, moving from the roots up to the maize kernels (Oren et al., 2003; Gai et al., 2018). Sometimes, the fungus colonizes and grows along the veins of the plant root, while sometimes it manages to penetrate the plant cells and form internal hyphae, therefore causing damage (Lei et al., 2011; Blacutt et al., 2018). Finally, for F. solani, the attachment of mycelia, asexual conidia, sexual ascospores or chlamydospores to the susceptible host is the first step in disease development, after which the fungus enters the host through stomata or the epidermis. Following penetration, F. solani is able to spread through the xylem, ultimately causing wilting of the host plant (Coleman, 2016).
It is reported that mycotoxins play a key role in pathogenesis, and that the aggressiveness of Fusarium depends on its toxin-producing capacity (Mesterházy, 2002; Xia et al., 2019; Laraba et al., 2021; Senatore et al., 2021; Yilmaz et al., 2021). Several mycotoxins are produced by Fusarium species, including the trichothecenes deoxynivalenol (DON) and nivalenol (NIV), zearalenone (ZEA), the cyclodepsipeptides beauvericin (BEA) and enniatins (ENN), and fusaric acid (Wagacha and Muthomi, 2007; Munkvold et al., 2021). The biosynthesis of these toxins is encoded by the tri, pks, bea and fus genes, respectively (Dhanti et al., 2017). However, not every species has the ability of producing all of the abovementioned mycotoxins. For example, DON and NIV are commonly produced by F. graminearum and F. culmorum, while ZEA and fusaric acid are often produced by some members of the F. sambucinum species complex (i.e. F. graminearum, F. culmorum), the F. fujikuroi complex (F. verticillioides) and the F. incarnatum-equiseti complex (Nešić et al., 2014; Munkvold et al., 2021), and BEA and ENN are produced by certain F. oxysporum and members of the F. tricinctum species complex (Munkvold et al., 2021; Senatore et al., 2021). DON production by F. graminearum is reported to be essential for disease development in wheat spikes (Cuzick et al., 2008). Spikes treated with DON or NIV led to yield losses even in the absence of the pathogen, indicating a strong negative effect of these trichothecenes on wheat growth (Ittu et al., 1995). In addition to DON, fusaric acid is also a virulence factor involved in programmed cell death (López-Díaz et al., 2018). It was shown that alkaline pH and low nitrogen and iron availabilities lead to increased fusaric acid production in F. oxysporum (Palmieri et al., 2023). Besides mycotoxins, there are other metabolites produced by Fusarium species that play a role in disease pathogenesis. Deletion of the F. graminearum gene cluster responsible for the synthesis of fusaoctaxin A abolished the fungal ability to colonize wheat coleoptiles (Jia et al., 2019). Extracellular lipases secreted by F. graminearum affected the plant’s defense responses by inhibiting callose synthase activity (Blümke et al., 2014).
Diseases caused by Fusarium species include blights, wilts and rots of various crops in natural environments and in agroecosystems (Nelson et al., 1994; Ma et al., 2013). Fusarium Head Blight (FHB) or ‘scab’ is a disease caused primarily by the F. graminearum species complex. It is the fourth-ranked fungal phytopathogen in term of economic importance (Dean et al., 2012; Legrand et al., 2017), causing yield losses of 20% to 70% (Bai and Shaner, 1994). F. graminearum is responsible for kernel damage and mycotoxin production (Ma et al., 2013) in cereals like wheat, barley, rice and oats (Goswami and Kistler, 2004). Typical symptoms of FHB begin soon after flowering, as diseased spikelets gradually bleach, leading to bleaching of the entire head. After this stage, black spherical structures called perithecia may appear on the surface of diseased spikelets. Later, as the disease becomes more severe, the fungus begins to attack the kernels inside the head, causing them to wrinkle and shrink (Schmale and Bergstrom, 2003). FHB can also be caused by F. culmorum, which is dominant in cooler regions of Europe (Wagacha and Muthomi, 2007). Vascular wilt is responsible for severe losses in crops such as melon, tomato, cotton, bean and banana. It is caused by Fusarium oxysporum, the fifth most economically important fungal phytopathogen (Michielse and Rep, 2009; Dean et al., 2012; Husaini et al., 2018). Symptoms of vascular wilt are first observed on the older leaves, as they begin to droop, followed by defoliation and yellowing of the younger leaves and eventually, plant death (Britannica, 2017; Redkar et al., 2022a). Root, stem and foot rots of various non-grain host plants are often caused by Fusarium solani, and the disease symptoms depend on the host plant and the particular forma specialis (Voigt, 2002; Coleman, 2016). However, typical symptoms of root, stem and foot rots include brown lesions on the affected plant organs. Fusarium verticillioides causes ear and stalk rot in hosts such as maize, sorghum and rice (Murillo-Williams and Munkvold, 2008; Dastjerdi and Karlovsky, 2015), whereas F. graminearum is responsible for causing Fusarium ear and stalk rot in maize (Goswami and Kistler, 2004). Fusarium ear rot is characterized by discoloration of single or multiple kernels in different areas of the ear, while early signs of stalk rot include lodging and discoloration of the stem.
3 Biocontrol agents against Fusarium and their modes of action
Plant-beneficial microorganisms present in the rhizosphere may protect plants from Fusarium pathogens, through different modes of action including (i) induction of resistance in the plant, (ii) competition with the pathogens for space and nutrients, (iii) amensalism based on the production of different metabolites or lytic enzymes, or (iv) parasitism (Figure 1B) (Nguvo and Gao, 2019; Morimura et al., 2020). Some of them are also able to inhibit mycotoxin synthesis or to enhance their detoxification (Legrand et al., 2017; Morimura et al., 2020). Certain biocontrol microorganisms have multiple modes of action, which may be expressed simultaneously or sequentially (Legrand et al., 2017).
3.1 Induced systemic resistance
Induced Systemic Resistance (ISR) is the phenomenon whereby a plant, once appropriately stimulated by biological or chemical inducers, exhibits enhanced resistance when challenged by a pathogen (Walters et al., 2013). ISR involves (i) the plant perception of inducing signals, (ii) signal transduction by plant tissues, and (iii) expression of plant mechanisms inhibiting penetration of the pathogen into the host tissues (Magotra et al., 2016). A wide variety of microorganisms, including the bacteria Pseudomonas, Bacillus, Streptomyces and the fungi Trichoderma and non-pathogenic F. oxysporum can induce ISR (Fuchs et al., 1997; Choudhary et al., 2007; Zhao et al., 2014; Galletti et al., 2020) in plants against Fusarium (Table 1). ISR in the plant-Fusarium system is based on microbial induction of the activity of various defense-related enzymes in plants, such as chitinase (Amer et al., 2014), lipoxygenase (Aydi Ben Abdallah et al., 2017), polyphenol oxidase (Akram et al., 2013), peroxidase, phenylalanine ammonia-lyase (Zhao et al., 2012), β-1,3-glucanase, catalase (Sundaramoorthy et al., 2012), and also the accumulation of phytoalexins, defense metabolites against fungi (Kuć, 1995). Cyclic lipopeptide antibiotics, e.g. fusaricidin (Li and Chen, 2019) and external cell components, e.g. lipopolysaccharides (LPS) (Leeman et al., 1995) can also trigger ISR. Some biocontrol agents can lead to ISR in different plant species, while other biocontrol agents show plant species specificity, suggesting specific recognition between microorganisms and receptors on the root surface (Choudhary et al., 2007).
Bacillus amyloliquefaciens subsp. plantarum strain SV65 was assessed on tomato plants infected or not with F. oxysporum f. sp. lycopersici (FOL). The expression of genes coding for lipoxygenase or pathogenesis-related (PR) proteins, i.e. acidic protein PR-1 and PR-3 chitinases was induced by B. amyloliquefaciens subsp. plantarum SV65 in both FOL-inoculated and uninoculated plants, suggesting its ability to induce ISR (Aydi Ben Abdallah et al., 2017). Inoculation of chilli plants with Bacillus subtilis EPCO16 and EPC5 and P. protegens Pf1, separately or in combination, induced ISR, with enhanced phytoalexin activities, and protected plants against F. solani (Sundaramoorthy et al., 2012). Inoculation of chickpea plants with a combination of Bacillus sp., Brevibacillus brevis and Mesorhizobium ciceri lead to the accumulation of peroxidase, polyphenol oxidase, phenylalanine ammonia lyase and phenols in plants as well as resistance to F. oxysporum (Kumari and Khanna, 2019). Paenibacillus polymyxa WLY78 controls Fusarium wilt, caused by Fusarium oxysporum f. sp. cucumerinum, through the production of fusaricidin, which can induce ISR in cucumber via the salicylic acid pathway (Li and Chen, 2019). Tomato showed increased catalase and peroxidase activities when treated with either Streptomyces sp. IC10 and Y28, or with Y28 alone, respectively, outlining a strain-specific ISR in tomato against Fusarium wilt mediated by FOL (Abbasi et al., 2019). Streptomyces bikiniensis increased the activities of peroxidase, phenylalanine ammonia-lyase and β-1,3-glucanase in cucumber leaves (Zhao et al., 2012). Nonpathogenic Fusarium oxysporum Fo47 can triger induced resistance to FOL and protects tomato from Fusarium wilt (Fuchs et al., 1999). Trichoderma gamsii IMO5 increased transcript levels of ISR-marker genes ZmLOX10, ZmAOS and ZmHPL in maize leaves, thereby protecting the plant from the pink ear rot pathogen F. verticillioides (Galletti et al., 2020).
An important determinant of biocontrol efficacy is the population density of ISR-triggering microorganisms. For example, ~105 CFU of Pseudomonas defensor (ex fluorescens) WCS374 per g of root are required for significant suppression of Fusarium wilt of radish (Raaijmakers et al., 1995). Another important feature of ISR in plants is that its effects are not only expressed at the site of induction but also in plant parts that are distant from the site of induction (Pieterse et al., 2014). For example, root-colonizing Pseudomonas simiae (ex fluorescens) WCS417r induced resistance in carnation, with phytoalexin accumulation in stems, and protected shoots from F. oxysporum (Van Peer et al., 1991). Priming of barley heads with P. fluorescens MKB158 led to changes in the levels of 1203 transcripts (including some involved in host defense responses), upon inoculation with pathogenic F. culmorum (Petti et al., 2010).
3.2 Competition for space and nutrients
In the case of competition, biocontrol of pathogens occurs when another microorganism is able to colonize the environment faster and use nutrient sources more efficiently than the pathogen itself, especially under limited conditions (Maheshwari et al., 2013; Legrand et al., 2017). Bacteria and fungi have the ability to compete with Fusarium, but the underlying mechanism of competition is sometimes unclear. For example, competition between non-pathogenic F. oxysporum strains and pathogenic F. oxysporum has been described, reducing disease incidence (Eparvier and Alabouvette, 1994; Fuchs et al., 1999). Similarly, a non-aflatoxigenic Aspergillus flavus strain was found to outcompete a mycotoxin-producing F. verticillioides during colonization of maize (Reis et al., 2020). Competition may involve bacteria such as Pseudomonas capeferrum (ex putida) strain WCS358, which suppresses Fusarium wilt of radish (Lemanceau et al., 1993).
In some cases, traits involved in competition have been identified. In P. putida (Trevisan) Migula isolate Corvallis, competition for root colonization entails plant’s production of agglutinin, and P. putida mutants lacking the ability to agglutinate with this plant glycoprotein showed reduced levels of rhizosphere colonization and suppression of Fusarium wilt of cucumber (Tari and Anderson, 1988). P. capeferrum WCS358 suppresses Fusarium wilt of radish by competing for iron through the production of its pseudobactin siderophore (Lemanceau et al., 1993). In addition to bacteria, the fungus Trichoderma asperellum strain T34 can control the disease caused by F. oxysporum f. sp. lycopersici on tomato plants by competing for iron (Segarra et al., 2010).
3.3 Amensalism based on antibiosis or lytic enzymes
Another important microbial mechanism to suppress plant pathogens is the secretion of inhibitors by beneficial microorganisms. They include anti-fungal secondary metabolites, sometimes termed antibiotics (e.g. fengycin, iturin, surfactin (Chen et al., 2018), fusaricidin and polymyxin (Zalila-Kolsi et al., 2016)), as well as Volatile Organic Compounds (VOCs; Zaim et al., 2016; Legrand et al., 2017) (Table 2). Extracellular lytic enzymes such as cellulase, chitinase, pectinase, xylanase (Khan et al., 2018), protease and glucanase (Saravanakumar et al., 2017), can also interfere with Fusarium growth or activity.
Bacillota representatives (formerly Firmicutes), i.e. Bacillus and Brevibacillus species are highlighted in several studies as candidates for Fusarium biocontrol through production of anti-fungal metabolites (Palazzini et al., 2007; Zhao et al., 2014; Chen et al., 2018; Johnson et al., 2020). Bacillus subtilis SG6 has the ability to produce fengycins and surfactins acting against F. graminearum (Zhao et al., 2014), whereas Bacillus velezensis LM2303 exhibited strong inhibition of F. graminearum and significantly reduced FHB severity under field conditions (Chen et al., 2018). Genome mining of B. velezensis LM2303 identified 13 biosynthetic gene clusters encoding secondary metabolites and chemical analysis confirmed their presence. These metabolites included three antifungal metabolites (fengycin B, iturin A, and surfactin A) and eight antibacterial metabolites (surfactin A, butirosin, plantazolicin and hydrolyzed plantazolicin, kijanimicin, bacilysin, difficidin, bacillaene A and bacillaene B, 7-o-malonyl macrolactin A and 7-o-succinyl macrolactin A) (Chen et al., 2018). Brevibacillus fortis NRS-1210 produces edeine, a compound with antimicrobial activity, which inhibits chlamydospore germination and conidia growth in F. oxysporum f. sp. cepae (Johnson et al., 2020). Pseudomonadota representatives (formerly Proteobacteria) are also known for disturbing Fusarium growth or activity. Thin layer chromatography analysis showed that Gluconacetobacter diazotrophicus produces pyoluteorin, which is involved in the suppression of F. oxysporum (Logeshwarn et al., 2011), while Burkholderia sp. HQB-1 produces phenazine-1-carboxylic acid, which is efficient at controlling Fusarium wilt of banana, caused by F. oxysporum f. sp. cubense (Xu et al., 2020). Pseudomonas sp. EM85 was successful in suppressing disease caused by F. verticillioides and F. graminearum, by producing antifungal antibiotics and fluorescent pigments (Pal et al., 2001). Besides bacteria, Trichoderma fungi synthesize a number of secondary metabolites such as pyrones (which completely inhibit spore germination of F. oxysporum), koningins (which affect the growth of F. oxysporum) and viridin (which prevents the germination of spores of F. caeruleum) (Reino et al., 2008).
VOCs have recently received more attention, as they can enable interactions between organisms in the soil ecosystem through both water and air phases (De Boer et al., 2019). Paenibacillus polymyxa WR-2 produced VOCs when cultivated in the presence of organic fertilizer and root exudates. Among them, benzothiazole, benzaldehyde, undecanal, dodecanal, hexadecanal, 2-tridecanone and phenol inhibited mycelial growth and spore germination of F. oxysporum f. sp. niveum (Raza et al., 2015). Chryseobacterium sp. AD48 inhibited growth of F. solani through the production of VOCs (Tyc et al., 2015). VOCs produced by Lysobacter antibioticus HS124 enhanced mycelial development, but they also reduced sporulation and spore germination of F. graminearum at the same time (Kim et al., 2019). In addition, testing the antagonistic mechanisms of Aspergillus pseudocaelatus and T. gamsii revealed the presence of the VOCs 2,3,4-trimethoxyphenylethylamine, 3-methoxy-2-(1-methylethyl)-5-(2-methylpropyl) pyrazine, (Z)-9- octadecenamide, pyrrolo [1,2-a] pyrazine-1,4-dione, hexahydro-3-(2-methylpropyl)-, thieno [2,3-c] pyridine-3-carboxamide,4,5,6,7-tetrahydro-2-amino-6-methyl- and hexadecanamide, which have an inhibitory activity against F. solani (Zohair et al., 2018).
Regarding extracellular lytic enzymes, B. subtilis 30VD-1 inhibited FOL by producing cellulase, chitinase, pectinase, xylanase and protease (Khan et al., 2018), while Bacillus pumilus synthesized a chitinolytic enzyme that reduced severity of disease caused by F. oxysporum on buckwheat under gnotobiotic conditions (Agarwal et al., 2017). Brevibacillus reuszeri affected the growth of F. oxysporum by producing chitinolytic enzymes (Masri et al., 2021). Kosakonia arachidis EF1 produced different cell-wall degrading enzymes, such as chitinases, proteases, cellulases and endoglucanases, which inhibited growth of F. verticillioides and F. oxysporum f. sp. cubense. Scanning electron microscopy revealed broken fungal mycelia surface and hyphae fragmentation when pathogens were grown in the presence of K. arachidis EF1 (Singh et al., 2021).
3.4 Parasitism
Mycoparasitism is an ancient lifestyle, during which one fungus parasitizes another fungus (Kubicek et al., 2011). It involves direct physical contact with the host mycelium (Pal and McSpadden Gardener, 2006), secretion of cell wall-degrading enzymes and subsequent hyphal penetration (Viterbo et al., 2002). Mycoparasitic relationships can be biotrophic, where the host remains alive and the mycoparasitic fungus obtains nutrients from the mycelium of its partner, or necrotrophic, where the parasite contacts and penetrates the host, resulting in the death of the host and allowing the mycoparasite to use the remains of the host as a nutrient source (Jeffries, 1995). Several species of fungi are mycoparasitic, of which Trichoderma is the best described. Contact between the mycoparasitic fungi Gliocladium roseum, Penicillium frequentans, T. atroviride, T. longibrachiatum or T. harzianum and their phytopathogenic targets F. culmorum, F. graminearum and F. nivale triggers the formation of various mycoparasitic structures, such as hooks and pincers, which lead to cell disruption in the phytopathogens (Pisi et al., 2001). When T. asperellum and T. harzianum were grown in the presence of F. solani cell wall, they secreted several cell wall-degrading enzymes, such as β-1,3-glucanase, N-acetylglucosaminidases, chitinase, acid phosphatase, acid proteases and alginate lyase (Qualhato et al., 2013), and similarly, Clonostachys rosea produced chitinase and β-1,3-glucanase in the presence of F. oxysporum cell wall (Chatterton and Punja, 2009). Sphaerodes mycoparasitica is a biotrophic fungus that parasitizes F. avenaceum, F. oxysporum and F. graminearum hyphae and forms hooks as parasitic structures (Vujanović and Goh, 2009). However, the direct contribution of mycoparasitism to biological control is difficult to quantify as mycoparasitic fungi typically exhibit a number of different biocontrol mechanisms (Pal and McSpadden Gardener, 2006).
3.5 Inhibition and detoxification of mycotoxins
Biocontrol research often focuses on pathogen inhibition, and effects on mycotoxin synthesis or detoxification are often neglected (Pellan et al., 2020). It can be expected that Fusarium inhibition will diminish mycotoxin synthesis, but one comprehensive study found that B. amyloliquefaciens FZB42 inhibited F. graminearum but at the same time stimulated biosynthesis of DON toxin (Gu et al., 2017). Conversely, DON production of F. graminearum (on wheat kernels) was reduced by more than 80% with B. amyloliquefaciens WPS4-1 and WPP9 (Shi et al., 2014), and Paenibacillus polymyxa W1-14-3 and C1-8-b (He et al., 2009), whereas Pseudomonas strains MKB158 and MKB249 significantly reduced DON production in F. culmorum-infected wheat seeds (Khan and Doohan, 2009). Pseudomonas sp. MKB158 lowered expression of the gene coding for trichodiene synthase (an enzyme involved in the production of trichothecene mycotoxins in Fusarium) by 33%, in wheat treated with F. culmorum (Khan et al., 2006). DON production in both F. graminearum and F. verticillioides was also inhibited by the fungus T. asperellum TV1 and the oomycete Pythium oligandrum M1/ATCC (Pellan et al., 2020). Other mycotoxins may be targeted, as Trichoderma harzianum Q710613, T. atroviride Q710251 and T. asperellum Q710682 decreased ZEA production in a dual-culture assay with F. graminearum (Tian et al., 2018), and Streptomyces sp. XY006 lowered the synthesis of fusaric acid in Fusarium oxysporum f. sp. cubense (Wang et al., 2023).
4 Soils suppressive to Fusarium diseases
4.1 General suppressiveness
Soils that are suppressive to soil-borne diseases have been known for more than 70 years (Vasudeva and Roy, 1950), and disease suppression is associated primarily with the activity of beneficial microorganisms (Schlatter et al., 2017). These microorganisms interact with phytopathogens, thus affecting their survival, development or infection of the plant (Weller et al., 2002; Raaijmakers et al., 2009). Two types of soil suppressiveness have been described, i.e. general (microbial community-based) suppressiveness and specific (microbial population-based) suppressiveness (Schlatter et al., 2017). General suppressiveness is dependent on the entire soil microbial biomass, which causes pathogen inhibition through various mechanisms, especially competition and the microbial release of inhibitors (Garbeva et al., 2011; De Boer et al., 2019), and it cannot be transferred experimentally between the soils (Weller et al., 2002). Hence, all soils may present some level of general suppressiveness to soil-borne diseases, and this level depends on soil type, agricultural practices and total microbial activity (Janvier et al., 2007; Raaijmakers et al., 2009).
General suppressiveness typically results in the inability of the pathogen to survive and proliferate in soil, and is termed fungistasis in the case of fungal phytopathogens. Fungistasis can affect Fusarium pathogens (De Boer et al., 2019; Legrand et al., 2019), but its significance in relation to different Fusarium species or formae speciales needs clarification. Legrand et al. (2019) determined the soil fungistasis status of 31 wheat fields in the case of F. graminearum, highlighting higher bacterial diversity, a higher prevalence of Pseudomonas and Bacillus species and a denser network of co-occurring bacterial taxa in soils with fungistasis. It suggests the importance of cooperations within diversified bacterial communities (including with antagonistic taxa) to control F. graminearum in soil (Legrand et al., 2019). Accordingly, both bacterial and fungal communities differed between Fusarium wilt-diseased soils vs healthy (presumably suppressive) soils taken from from eight countries and grown with different crop plants (Yuan et al., 2020).
4.2 Specific suppressiveness to Fusarium diseases
Besides general suppressiveness, there is also specific suppression to certain diseases, which relies on the activity of a few plant-protecting microbial groups (Weller et al., 2007; Almario et al., 2014; Mousa and Raizada, 2016). Specific suppressiveness may be conferred to non-suppressive soils (i.e. conducive soils) by inoculating them with 0.1% - 10% of suppressive soil (Garbeva et al., 2004; Raaijmakers et al., 2009). Although abiotic factors, such as soil physicochemical properties, may contribute to the control of a given pathogen, specific suppressiveness is essentially a phenomenon mediated by beneficial soil microorganisms, since sterilization processes convert suppressive into conducive soils (Garbeva et al., 2004). It is expected that specific suppressiveness entails the contribution of a few plant-protecting microbial groups (Weller et al., 2007), but microbial community comparison of suppressive vs conducive soils may evidence significant differences for a large number of taxa (Kyselková et al., 2009; Legrand et al., 2019; Ossowicki et al., 2020; Yuan et al., 2020; Lv et al., 2023).
The phenomenon of disease suppressiveness has been described for many soil-borne fungal pathogens, including Gaeumannomyces graminis var. tritici (Shipton et al., 1973; Weller et al., 2007; Schlatter et al., 2017), Thievalopsis basicola (Stutz et al., 1986; Almario et al., 2014) and Rhizoctonia solani (Mendes et al., 2011; Schlatter et al., 2017). It is also well established in the case of several Fusarium pathogenic species (Table 3), such as F. culmorum on wheat (in the Netherlands and Germany; Ossowicki et al., 2020) and barley (in Denmark; Rasmussen et al., 2002), F. oxysporum f. sp. albedinis on palm tree (in Marocco; Rouxel and Sedra, 1989), F. oxysporum f. sp. batatas on sweet potato (in California; Smith and Snyder, 1971), F. oxysporum f. sp. cubense on banana (in India, Indonesia, China, Gran Canaria island and several Central America states; Stotzky and Torrence Martin, 1963; Domínguez et al., 1996; Shen et al., 2015b; Wang et al., 2019; Nisrina et al., 2021; Yadav et al., 2021; Fan et al., 2023), F. oxysporum f. sp. cucumerinum on cucumber (in California; Sneh et al., 1984) and cape gooseberry (in Colombia; Bautista et al., 2023), F. oxysporum f. sp. dianthi on carnation (in Italy; Garibaldi et al., 1983), F. oxysporum f. sp. fragariae on strawberry (in Korea; Cha et al., 2016), F. oxysporum f. sp. lini on flax (in Italy, California; Kloepper et al., 1980; Tamietti and Pramotton, 1990), F. oxysporum f. sp. lycopersici on tomato (in France, Italy; Tamietti and Alabouvette, 1986; Tamietti et al., 1993) and wheat (in Italy; Tamietti and Matta, 1984), F. oxysporum f. sp. melonis on melon (in France; Louvet et al., 1976), F. oxysporum f. sp. niveum on watermelon (in Florida; Larkin et al., 1993), F. oxysporum f. sp. radicis-cucumerinum on cucumber (in Israel; Klein et al., 2013), F. udum on pigeon-pea (in India; Vasudeva and Roy, 1950), and F. graminearum on wheat (in Serbia; Todorović et al., unpublished data). Therefore, unlike with other pathogenic taxa, suppressiveness is documented across a wide range of Fusarium pathosystems. It also appears that suppressiveness to Fusarium diseases occurs in numerous parts of the world (Figure 3).
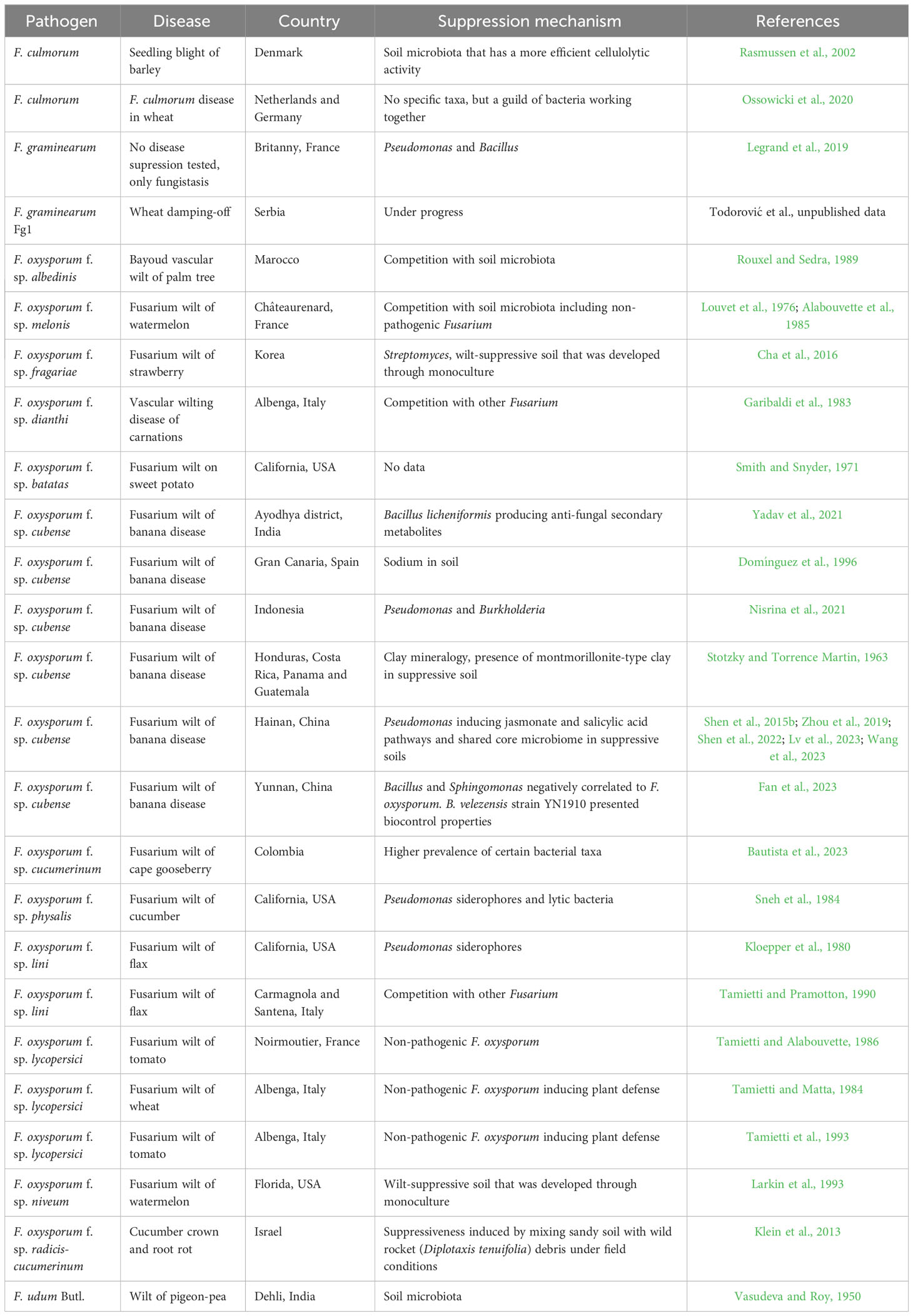
Table 3 List of locations with soils suppressive to Fusarium diseases known to date, with a pathosystem, disease and the underlying suppression mechanism.
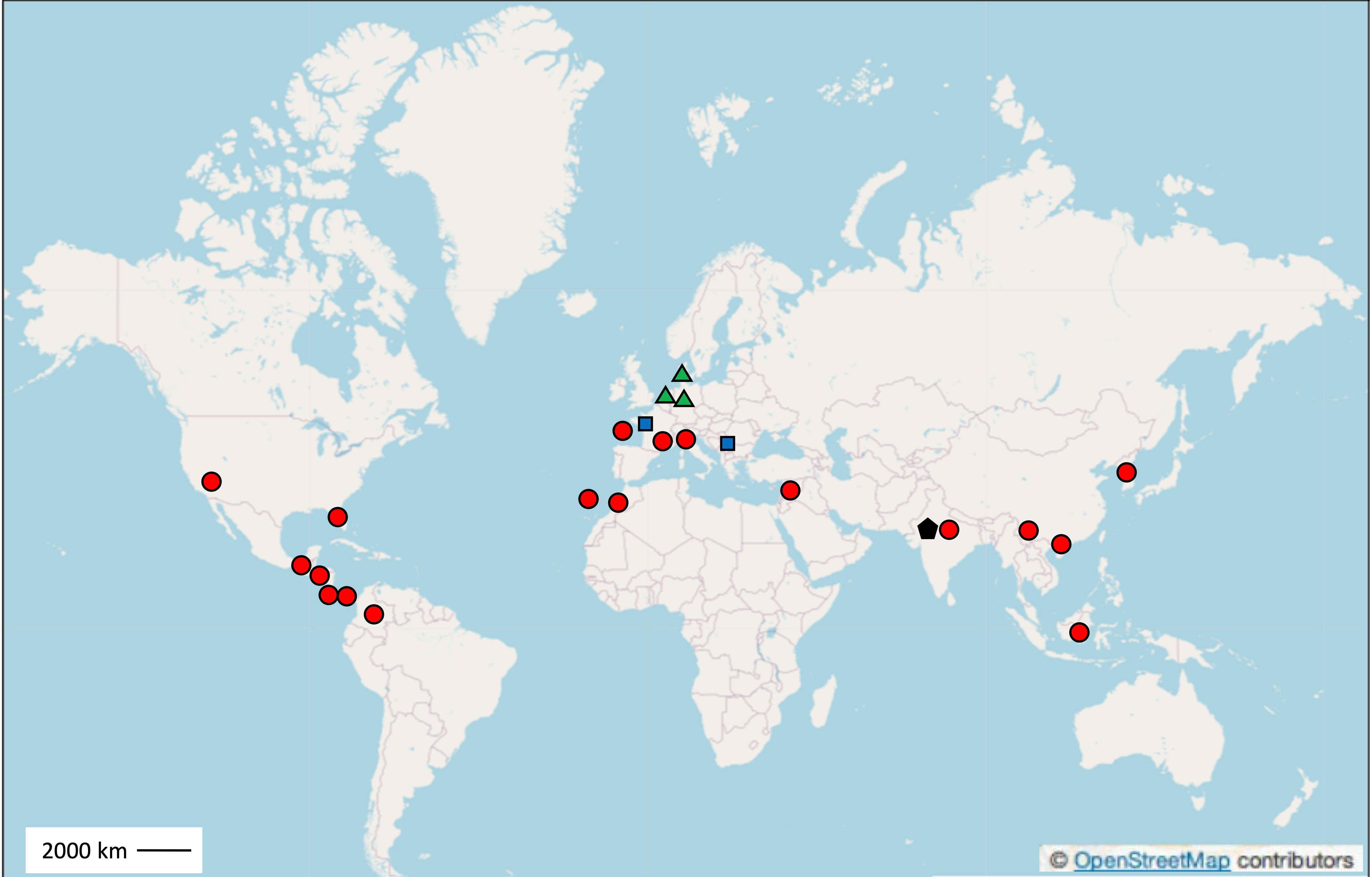
Figure 3 Geographic locations of the main field sites with soils documented to be suppressive to Fusarium diseases, in Europe including France (Noirmoutier Island, Châteaurenard in Southeast France, and Brittany), Denmark, The Netherlands, Germany, Italy (Albenga, Carmagnola, and Santena), Gran Canaria Island (Spain, located in the Atlantic Ocean), and Serbia, in North America (California and Florida), Central America (Honduras, Costa Rica, Panama, and Guatemala), South America (Colombia), Asia (Korea, China, India, Israel, and Indonesia), and Africa (Morocco). Each location is marked with the corresponding pathogen: F. oxysporum (indicated by a red dot), F. culmorum (green triangle), F. graminearum (blue square), and F. udum (black pentagon).
4.3 Natural and induced specific suppressiveness to Fusarium diseases
Specific suppressiveness is sometimes an intrinsic property of the soil and persists over years, despite changing ecological conditions related to crop rotation. This natural/long-term suppressiveness is well documented for several pathosystems, for instance in Swiss soils suppressive to tobacco black root rot (T. basicola) near Morens (Stutz et al., 1986). Suppressive and conducive soils may be located at small geographic distances in the landscape, and differences in plant disease incidence between neighbouring fields that share similar climatic conditions and agronomic practices are attributed by the differences in the resident microbiota in these soils (Almario et al., 2014). Natural suppressiveness has also been extensively studied in the case of Fusarium diseases, in particular with the Fusarium wilt suppressive soils of Salinas Valley (California) or Châteaurenard (France). In these soils, Fusarium wilt disease remains minor despite the long history of cultivation of different crops, and the introduction of small amount of these soils to sterilized suppressive soil or conducive soil significantly decreased Fusarium wilt disease incidence (Scher and Baker, 1980; Alabouvette, 1986). In both locations, the small level of disease in plants cannot be attributed to the absence of Fusarium in the soil, but rather to plant protection by the soil microbiota (Sneh et al., 1984; Alabouvette et al., 1985; Siegel-Hertz et al., 2018), as found in later investigations (Bautista et al., 2023).
Specific disease suppressiveness can also result from particular farming practices leading to the built-up of a plant-protecting microbiota. Often, this takes place following crop monoculture, typically after early disease outbreak, and is examplified by take-all decline of wheat (Weller et al., 2002; Sanguin et al., 2009) and barley (Schreiner et al., 2010). Induced suppressiveness is initiated and maintained by monoculture, in the presence of the pathogen Gaeumannomyces graminis var. tritici (Weller et al., 2002). Soil suppressiveness to Fusarium diseases is usually natural, but cases of induced suppressiveness are also documented. Thus, soils found in Hainan island (China) that were grown for years with banana in confontration with pathogenic F. oxysporum displayed rhizosphere enrichment in microbial taxa conferring protection from banana wilt (termed banana Panama disease) (Shen et al., 2022), watermelon monoculture in Florida induced suppressiveness to wilt caused by F. oxysporum f. sp. niveum (Larkin et al., 1993), and 15 years of strawberry monoculture in Korea triggered suppressiveness to wilt caused by F. oxysporum f. sp. fragariae (Cha et al., 2016). Soil addition of wild rocket residues resulted in suppressiveness to cucumber crown and root rot (F. oxysporum f. sp. radicis-cucumerinum) in Israel (Klein et al., 2013), whereas suppressiveness to Fusarium wilt can also be induced by microbial biofertilizer inoculants reshaping the soil microbiome (Xiong et al., 2017). Thus, organic fertilizer containing B. amyloliquefaciens W19 enhanced levels of indigenous Pseudomonas spp. and provided suppression of Fusarium wilt of banana (Tao et al., 2020). The combined action of B. amyloliquefaciens W19 and Pseudomonas spp. is thought to cause a decrease in Fusarium density in the root zone of banana. Organic fertilizers inoculated with Erythrobacter sp. YH-07 controlled Fusarium wilt in tomato, as a direct result of the bacteria and indirectly by altering the composition of the microbial community (Tang et al., 2023). Organic fertilizer amended with Bacillus and Trichoderma resulted in an increase in indigenous Lysobacter spp., thus indirectly inducing suppression of Fusarium wilt of vanilla (Xiong et al., 2017).
5 The microbiome of soils suppressive to Fusarium diseases
5.1 Biocontrol microorganisms in soils suppressive to Fusarium diseases
Many biocontrol strains originate from suppressive soils, and they were investigated as a mean to understand disease suppressiveness. In the case of Fusarium diseases, examples include Pseudomonas sp. Q2-87 (P. corrugata subgroup) (Weller et al., 2007), isolated from wheat in take-all decline soils but that protects tomato from F. oxysporum f. sp. radicis-lycopersici, Pseudomonas sp. C7 (P. corrugata subgroup) (Lemanceau and Alabouvette, 1991) isolated from soil suppressive to Fusarium wilt of tomato, and non-pathogenic F. oxysporum strains Fo47 (Fuchs et al., 1997; Duijff et al., 1998; Fuchs et al., 1999), CAV 255 (Sajeena et al., 2020) and Ro-3 (Bubici et al., 2019). Based on the biocontrol traits thus identified, the corresponding microbial functional groups have been characterized in suppressive vs conducive soils, using isolate collections, molecular fingerprints or sequencing. Fluorescent Pseudomonas bacteria, especially those producing the antifungal metabolite 2,4-diacetylphloroglucinol, have been extensively targeted in take-all-decline soils (Cook and Rovira, 1976; Weller et al., 2002; Weller et al., 2007) and soils suppressive to black root rot (Stutz et al., 1986; Laville et al., 1992; Kyselková and Moënne-Loccoz, 2012), whereas studies on soils suppressive to R. solani diseases have focused on Pseudomonas spp. producing antifungal lipopeptides (Mendes et al., 2011), Streptomyces spp. producing volatile metabolites (Cordovez et al., 2015) and Paraburkholderia graminis producing sulfurous volatile compounds (Carrión et al., 2018). In the case of soils suppressive to Fusarium diseases, competition with pathogenic Fusarium species is considered important, involving the entire soil microbiota or more specifically non-pathogenic Fusarium strains in Châteaurenard soils (Louvet et al., 1976; Alabouvette, 1986), or fluorescent Pseudomonas (iron competition; Scher and Baker, 1980; Sneh et al., 1984) in soils of Salinas Valley (California) or Châteaurenard (France). The role of extracellular lytic enzymes can be significant, as soil microbiota may protect barley from Fusarium culmorum via a more efficient cellulolytic activity than the pathogen, which consequently is outcompeted for nutrients (Rasmussen et al., 2002). Suppressiveness may result in part from chitinolytic effects of the soil microbiota against the pathogen, based on inhibition of Fusarium fungi by chitinases in vitro and effective protection of plant by chitinase-producing inoculants (Veliz et al., 2017). Other modes of action evidenced include the production of antifungal secondary metabolites in wilt-suppressive soils, such as a new thiopeptide by Streptomyces (Cha et al., 2016) and phenazines by Pseudomonas spp. (Mazurier et al., 2009), and immunity stimulation in banana (induction of the jasmonate and salicylic acid pathways) by fluorescent Pseudomonas (Lv et al., 2023).
5.2 Microbial diversity in soils suppressive to Fusarium diseases
Specific disease suppressiveness is attributed to the contribution of a few plant-benefical populations, but comparison of suppressive vs conducive soils has evidenced differences in the occurrence or prevalence of multiple taxa, in the case of suppressiveness to take all (Sanguin et al., 2009; Schreiner et al., 2010; Chng et al., 2015), black root rot (Kyselková et al., 2009), R. solani-mediated damping-off (Mendes et al., 2011), or potato common scab (Rosenzweig et al., 2012). Similar findings were made with soils suppressive to Fusarium diseases. No single phylum was uniquely associated with F. oxysporum wilt suppressiveness in Korean soils, even though Actinomycetota (formerly Actinobacteria) was identified as the most prevalent bacterial taxa colonizing strawberry in suppressive soils (Cha et al., 2016). Likewise, the bacterial genera Devosia, Flavobacterium and Pseudomonas were more abundant (and the pathogen less abundant) in Chinese soils suppressive to banana wilt than in conducive soils, and Pseudomonas inoculants isolated from suppressive could control the disease (Lv et al., 2023). Compared with conducive soil, Fusarium wilt suppressive soil from Châteaurenard displayed higher relative abundance of Adhaeribacter, Arthrobacter, Amycolatopsis, Geobacter, Massilia, Microvirga, Paenibacillus, Rhizobium, Rhizobacter, Rubrobacter and Stenotrophomonas (but not Pseudomonas) (Siegel-Hertz et al., 2018). However, differences were also found in the fungal community, with several fungal genera (Acremonium, Ceratobasidium, Chaetomium, Cladosporium, Clonostachys, Mortierella, Penicillium, Scytalidium, Verticillium, but also Fusarium) detected exclusively in the wilt suppressive soil (Siegel-Hertz et al., 2018). Data also pointed to a greater degree of microbial complexity in suppressive soils, with particular co-occurrence networks of taxa (Bakker et al., 2014; Lv et al., 2023). In German and Dutch soils, co-occurrence networks showed that the suppressive soil microbiota involves a guild of bacteria that probably function together, and in two of the suppressive soils this guild is dominated by Acidobacteriota (formerly Acidobacteria) (Ossowicki et al., 2020).
Many studies focused on a few, geographically-close soils, which does not provide a global view on the importance of microbial diversity. However, two studies have considered geographically diverse agricultural soils suppressive to Fusarium wilt. Various Chinese soils suppressive to banana wilt mediated by F. oxysporum were shown to share a common core microbiota, specific to suppressive soils, which included the genus Pseudomonas (Shen et al., 2022). In a wider range of soils from the Netherlands and Germany, soils suppressive to F. culmorum-mediated wilt of wheat did not display a specific bacterial species that correlated with suppressiveness (Ossowicki et al., 2020). There was no relation either with soil physicochemical composition (i.e. soil type, pH, contents in C, N, or bioavailable Fe, K, Mg, P) or field history, yet suppressiveness was microbial in nature, as sterilizing suppressive soils made them become conducive. This suggests that each suppressive soil may harbor its own set of phytobeneficial bacteria, supporting the notion of functional redundancy between microbiomes, meaning that different microbiomes may share common functionalities despite taxonomic differences in the microbial actors involved (Lemanceau et al., 2017). Taken together, this might be explained by the fact that protection of wheat from F. culmorum-mediated wilt corresponds to a case of natural suppressiveness (Ossowicki et al., 2020), where biogeographic patterns are probably important, whereas soils suppressive to Fusarium wilt of banana are induced by monoculture (Wang et al., 2019; Shen et al., 2022), with convergent effects resulting from similar banana recruitment across different soil types.
To go beyond individual analyses considered separately, we re-analyzed sequence data from five investigations comparing disease-suppressive and conducive soils of cultivated plants (flax, watermelon, bananas, and wheat) infected by different Fusarium species (F. oxysporum or F. culmorum). At the level of bacterial phyla, fluctuations among Châteaurenard (flax-F. oxysporum; Siegel-Hertz et al., 2018), Hainan (banana-F. oxysporum; Shen et al., 2022) (Figure S1A) and Dutch/German (wheat-F. culmorum; Ossowicki et al., 2020) (Figure S1B) suppressive soils were important, as were those among their conducive counterparts, and the comparison between suppressive and conducive soils at these locations was not fruitful. In another study, fluctuations among other Hainan (banana-F. oxysporum; Zhou et al., 2019) suppressive or conducive soils were of less magnitude, but again the comparison was not insightful (Figure S1B). In contrast, Jiangsu (watermelon-F. oxysporum; Wang et al., 2015) suppressive soils displayed a higher relative abundance of Acidobacteriota and Pseudomonadota than in conducive soils (Figure S1B), but this property was not relevant when considering the other locations/plant species/Fusarium species. Based on heatmap comparisons (Figure S2), the main finding was the lower prevalence of the Bacillota phylum in the Jiangsu (watermelon-F. oxysporum) suppressive vs conducive soils, which was restricted to the case of these soils.
At the level of bacterial genera, the comparison of Châteaurenard (flax-F. oxysporum), Hainan (banana-F. oxysporum) or Dutch/German (wheat-F. culmorum) soils did not lead to the identification of indicator taxa (Figures 4, S3), but at Jiangsu (watermelon-F. oxysporum) the genera Bacillus, Dongia, Rhodoplanes and Terrimonas were less prevalent and the genera Ferruginibacter, Flavobacterium, Pseudomonas and Sphingomonas more prevalent in suppressive soils than in conducive soils (Figure S3A). Therefore, the comparison between suppressive and conducive soils was sometimes meaningful at the local scale, but typically not when considering a wider range of geographic or biological (plant and Fusarium species) conditions together. In other words, the information available so far points that suppressiveness to Fusarium diseases relies on microbial selection processes by roots that depend on local conditions, i.e. probably related to microbial biogeography, soil type, plant species, Fusarium genotype and most likely other local factors as well.
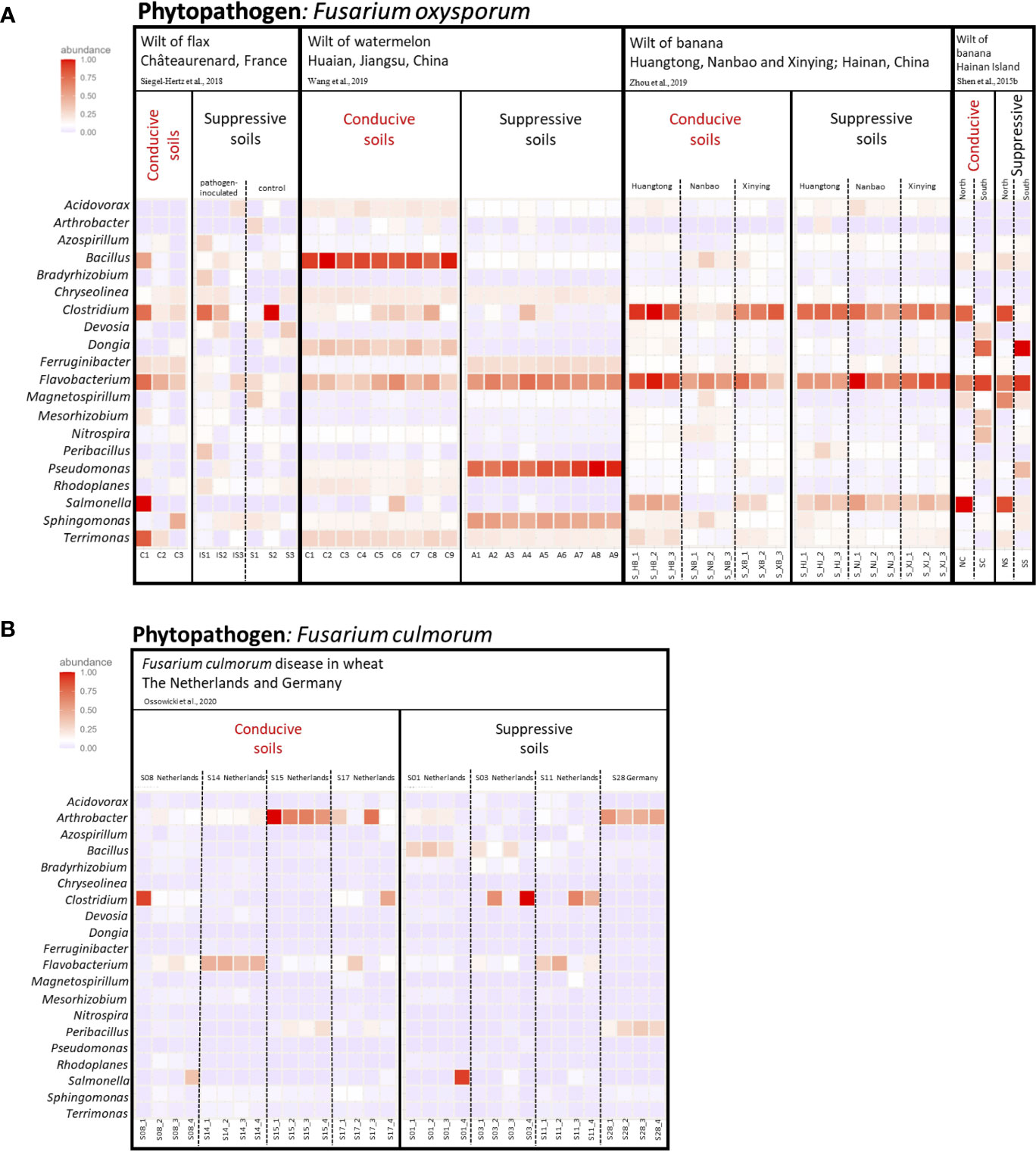
Figure 4 Heatmap of the major bacterial genera detected in the rhizosphere of plants grown in soils suppressive or conducive to different Fusarium diseases, based on analysis (File S1) of selected studies (Shen et al., 2015b; Siegel-Hertz et al., 2018; Wang et al., 2019; Zhou et al., 2019; Ossowicki et al., 2020). (A) The 20 most abundant genera in soils conducive or suppressive to diseases caused by Fusarium oxysporum. In Siegel-Hertz et al. (2018), suppressive soils were assessed after Fusarium inoculation or without. (B) The 20 most abundant genera in soils conducive or suppressive to diseases caused by Fusarium culmorum. The color intensity in each cell indicates the relative abundance (%) of a genus in each study for each plant type. When relevant, dotted lines are used to separate pathogen-inoculated samples from non-inoculated samples (in Châteaurenard) or samples from different fields. More details on individual conditions are available in Table S2.
6 Variability and management of soil suppressiveness to Fusarium diseases
6.1 Environmental factors influencing soil suppressiveness to Fusarium diseases
Environmental conditions in soil may influence Fusarium autecology, the composition and activity of the soil microbial community, the tripartite interactions between this microbiota, Fusarium pathogens and the plant, and ultimately the level of disease suppressiveness (Marshall and Alexander, 1960; Amir and Alabouvette, 1993; Mazzola, 2002; Czembor et al., 2015). Key environmental factors in this regard include soil physicochemical properties and weather conditions (Weber and Kita, 2010).
Early work on the suppressiveness of soils to vascular Fusarium diseases drew attention to the positive role of certain abiotic factors and, in particular, montmorillonite-type clays (Stover, 1956; Stotzky and Torrence Martin, 1963). In addition, higher clay contents may contribute to reduced infestation by Fusarium (Kurek and Jaroszuk-Ściseł, 2003; Deltour et al., 2017), by altering oxygen diffusion, pH buffering and nutrient availability (Orr and Nelson, 2018). Höper et al. (1995) showed that the level of suppressiveness to Fusarium wilt of flax increased in soils amended with montmorillonite, kaolinite or illite at pH 7. A negative correlation between soil pH and Fusarium disease severity was reported in experiments with flax (Senechkin et al., 2014), strawberry (Fang et al., 2012) and banana (Shen et al., 2015a). However, the correlation between pH and Fusarium wilt incidence was positive in studies on banana (Peng et al., 1999) and watermelon (Cao et al., 2016). Certain experiments acidified soil originally at pH 8.0 (Peng et al., 1999) or 7.4 (Cao et al., 2016), whereas others limed an acidic soil (Fang et al., 2012; Senechkin et al., 2014; Shen et al., 2015a). Inconsistencies may relate to the complexity of pH effects on Fusarium pathogens and diseases, and possible interactions with soil properties, Fusarium and plant genotypes, or other experimental conditions. In addition, soil suppressiveness to Fusarium wilt necessitates sufficient levels of nitrogen, as disease incidence negatively correlates with the NH4+ and NO3– contents in the soil (Li et al., 2016; Meng et al., 2019). Moreover, the addition of calcium to the soils suppressed Fusarium wilt in several soil type × plant conditions (Spiegel et al., 1987; Peng et al., 1999; Gatch and du Toit, 2017). In Brittany, F. graminearum growth positively correlated with manganese and iron contents in the soil (Legrand et al., 2019). A positive correlation was found between hemicellulose concentration and suppression of Fusarium wilt in tomato and carnation (Castaño et al., 2011), as well as cellulose concentration and suppression of Fusarium seedling blight of barley (Rasmussen et al., 2002). This is attributed to the activity of cellulolytic microorganisms that limit Fusarium growth, as lower organic matter content (following decomposition) would reduce resources supporting this microbiota and disease suppression (Orr and Nelson, 2018).
Climatic conditions, notably temperature and precipitation may strongly affect the incidence of Fusarium diseases (Orr and Nelson, 2018). Phytopathogenic species F. oxysporum, F. solani, F. verticillioides, F. graminearum and F. culmorum develop best under humid conditions, at water activity above 0.86 (Table S1) (Thrane, 2014). Severity of Fusarium wilt in lettuce (Scott et al., 2009; Ferrocino et al., 2013) and FHB in wheat was positively correlated with soil temperature (Xu et al., 2007; Nazari et al., 2018). For example, Fusarium wilt incidence significantly increased when lettuce was grown at 22-26°C instead of 18-22°C (Ferrocino et al., 2013). Similarly, in both conducive and suppressive soils, severity of Fusarium wilt of banana was significantly increased when temperature was raised from 24°C to 34°C (Peng et al., 1999).
6.2 Farming practices and the management of soil suppressiveness to Fusarium diseases
As many other soil-inhabiting pathogenic fungi, the Fusarium spp. can overwinter as mycelium in plant debris or dormant structures in the soil, which leads to cause the initial infection of plants in the following season (Nelson et al., 1994; Janvier et al., 2007; Leplat et al., 2013; Xu et al., 2021). Therefore, cultural practices removing the primary inoculum of the pathogen from overwintering soils are useful to prevent future infection (Voigt, 2002). However, farming practices also influence soil suppressiveness by shaping the rhizosphere microbial community (Campos et al., 2016) and stimulating the activity of beneficial rhizosphere microorganisms (Janvier et al., 2007). In this context, various agricultural practices, such as crop rotation/monocropping, tillage, organic amendments and fertilisers, are important to consider to develop suppressiveness-based control methods in farm fields (Janvier et al., 2007; Fu et al., 2016).
Except in the few cases where monoculture induces suppressiveness to Fusarium diseases (Larkin et al., 1993; Shen et al., 2022), cropping systems based on rotation of different plant species result in reduced survival of soil-borne pathogen propagules over the short term (Winter et al., 2014). Crop rotation may reduce severity and incidence of diseases caused by Fusarium spp. (Wang et al., 2015; Khemir et al., 2020). For example, compared with the tomato monoculture, soil management under wheat - tomato rotation changes soil microbial composition by increasing the abundance of microbial taxa such as Bacillus, Paenibacillus, Pseudomonas, Streptomyces, Aspergillus, Penicillium and Mortierella, which may control Fusarium wilt of tomato (De Corato et al., 2020). Reduced incidence of F. pseudograminearum and F. culmorum in the soils under cereal – legumes rotation management may be due to the non-host character of the legumes (Evans et al., 2010). However, not all crop rotations lead to reduced disease pressure (Ranzi et al., 2017). In the case of the FHB, it was advocated to rotate wheat and corn with crops like soybean, until it was shown that F. graminearum can also cause disease in soybean, as it has a wide range of hosts (Marburger et al., 2015). This suggests that there is no common rule regarding the relationship between crop rotation and Fusarium disease incidence.
Crop residues of high cellulose content promoted the activity of beneficial cellulolytic microorganisms and limited the development of Fusarium culmorum (Rasmussen et al., 2002), as organic amendments represent a favorable environment for beneficial microorganisms that are able to combat phytopathogenic Fusarium species (Maher et al., 2008; Cuesta et al., 2012). Accordingly, organic amendments like animal manure, solid wastes and different composts are often used to improve soil health by delivering nutrients to the soil and also by stimulating beneficial microbiota (Fu et al., 2016; Mousa and Raizada, 2016). Thus, soils with added organic amendments exhibited inhibitory effects against F. verticillioides by reducing the production of a fungal pigment and sporulation, consequently disabling fungal spread (Nguyen et al., 2018). Addition of vermicompost reduced tomato infection by F. oxysporum f. sp. lycopersici (Szczech, 1999) and mulched straw contributed to the suppression of seedling blight caused by F. culmorum (Knudsen et al., 1999). Soils supplemented with coffee residue compost or rapeseed meal exhibited suppressiveness to F. oxysporum-mediated wilt, and microorganisms isolated from supplemented soils inhibited F. oxysporum growth on agar plates (Mitsuboshi et al., 2018). Carbon addition to soil influenced the soil microbiome, enhancing Fusarium-inhibitory populations from the Streptomyces genus (Dundore-Arias et al., 2020). However, increasing organic matter content may promote Fusarium survival in certain (rare) cases. One study tested the effects of 18 composts (made from different mixtures of manure, domestic biowaste and green waste) on Fusarium wilt disease suppression, caused by F. oxysporum f. sp. lini, and it was shown that only one compost did not positively affect the disease suppression (Termorshuizen et al., 2006). The efficiency of organic amendments in controlling plant diseases is determined by the pathosystem, the application rate, the kind of amendment and the level of maturity of composts or disintegration phase of crop residues (Janvier et al., 2007).
Tillage, which is one factor influencing organic matter decomposition, appears to have contrasting effects on soil suppressiveness. Under conventional tillage, tillage depth appears to play a crucial role in soil survival of Fusarium, such that the deeper the tillage, the lower the abundance of Fusarium species (Steinkellner and Langer, 2004). This can be partly explained by the fact that the pathogen is displaced from its niche, reducing its ability to survive (Bailey and Lazarovits, 2003), and the rate of decomposition of buried residues is faster than at the soil surface (Leplat et al., 2013). The carbon released during these decomposition processes increases the activity of the soil microbiota, thereby improving the overall functioning of the soil (Bailey and Lazarovits, 2003). Under conservation tillage, surface residues persist and can act as a long-term source of inoculum for plant infection by F. verticillioides, F. proliferatum and F. subglutinans, as they can colonise crop residues and produce overwintering spores that often survive the period when plants are absent from the agrosystem (Bockus and Shroyer, 1998; Cotten and Munkvold, 1998; Pereyra et al., 2004). This is consistent with results suggesting that conservation tillage and leaving crop residues in situ increase Fusarium abundance (Govaerts et al., 2008; Wang et al., 2020). For example, spores of Fusarium species could be recovered from plant residues more than two years after harvest (Pereyra et al., 2004). In certain cases, lower occurrence of plant infection by F. culmorum, F. equiseti (Weber et al., 2001) and F. pseudograminearum (Theron et al., 2023) was found under conservation tillage compared with conventional tillage. These contrasting results might be due to differences in environmental factors, cropping patterns and soil types, which could modulate interactions between soil conditions, Fusarium ecology and plant physiology (Sturz and Carter, 1995). The use of simplified tillage practices was proposed to reduce F. culmorum abundance, by mixing crop residues with the topsoil layer to promote the growth of beneficial straw-decomposing microorganisms (Weber and Kita, 2010).
Different fertilizers have different effects on phytopathogenic Fusarium spp. On one hand, the development of FHB caused by F. culmorum and F. graminearum increased with inorganic nitrogen fertilization (Lemmens et al., 2004), and on the other hand, nitrite could reduce the population of F. oxysporum (Löffler et al., 1986). Besides, higher doses of nitrogen may contribute to higher accumulation of Fusarium mycotoxins (Podolska et al., 2017). The addition of phosphorus fertilizer, in the form of P2O5, significantly reduced Fusarium-caused wilting in chickpea, lentil and lupine, in both greenhouse and field conditions (Elhassan et al., 2010). Organic fertilizers can lead to an increase in indigenous microbial populations, thus contributing to suppression of Fusarium wilt disease (Montalba et al., 2010; Raza et al., 2015). When grown with the addition of organic N fertilizer, highbush blueberry exhibited increased tolerance to F. solani, in parallel to increased soil microbial activity and mycorrhizal colonization (Montalba et al., 2010).
7 Conclusion and outlook
Disease-suppressiveness of soils is a useful model to understand microbial phytoprotection and develop sustainable plant protection strategies for soils devoid of this property. In this review, we summarized the current knowledge on Fusarium phytopathogens, the available control methods and soils suppressive to Fusarium diseases, with the underlying mechanisms involved in the suppression. On one hand, extensive information is available on environmental and microbial properties responsible for suppressiveness to Fusarium diseases. One prominent feature is the diversity of Fusarium-based pathosystems for which suppressive soils are documented, in terms of Fusarium species (often F. oxysporum, but not only), host plants (both monocots and dicots), types of disease (often wilt, but not only), geographic locations of soil and farming conditions, and types of suppressiveness (i.e. natural suppressiveness to Fusarium diseases, but also monoculture-induced suppressiveness as well as fungistasis towards Fusarium pathogens). This diversity is paralleled by differences in microbiota composition and diversity associated with disease control in the different cases of suppressiveness. On the other hand, despite the fact that soils suppressive to Fusarium diseases have been studied for decades, they are still poorly understood in terms of microbiota functioning, and knowledge remains fragmented.
On this basis, additional research is needed to integrate further the scientific approaches used to decipher suppressiveness to Fusarium diseases. First, by combining complementary assessment methodology with current next-generation sequencing and ecological networks research, and incorporating experimental strategies to manipulate and transplant rhizosphere microbiome (or single microorganisms) of plants grown in suppressive soils to those in conducive soils to go beyond correlative work, as started recently (Ye et al., 2020; Jiang et al., 2022). Second, by extending the range of soil conditions investigated, and develop meta-analyses to estimate key microbiota differences between suppressive and conducive soils, as pioneered by Yuan et al. (2020). Third, by considering a wider range of biological actors, including beneficial fungi (often neglected), soil fauna (likely to influence microbial communities, Fusarium vectorisation, and plant health; e.g. Dita et al., 2018; Wagner et al., 2022). Fourth, by taking into account plant genetics, behavior and physiological responses to Fusarium pathogens (e.g. Liu et al., 2019). Therefore, there is a need for a more multidisciplinary approach to understand microbiota functioning in soils suppressive to Fusarium diseases.
Author contributions
All authors contributed to the writing of this review article and approved the submitted version.
Funding
IT was funded by a grant from the Ministry of Youth and Sports, Belgrade, Serbia (grant numbers 670-00-573/1/372/2019-04, 670-00-2590/1/304/2020-04, 670-00-2551/1/298/2021-04 and 670-00-1/1/317/2022-01) and grants from Campus France (grant numbers 964308G, 972203C and 103939T). This research was also funded through the 2018-2019 BiodivERsA joint call for research proposals, under the BiodivERsA3 ERA-Net COFUND programme, and with the funding organization ANR (Paris) (project SuppressSOIL ANR-19-EBI3-0007), as well as by The Ministry of Education, Science, and Technological Development of the Republic of Serbia (grant number 451‑03‑47/2023‑01/200116).
Acknowledgments
We are grateful to Danis Abrouk (iBio) for help with retrieving metabarcoding sequences from various articles and comparative analyses.
Conflict of interest
The authors declare that the research was conducted in the absence of any commercial or financial relationships that could be construed as a potential conflict of interest.
Publisher’s note
All claims expressed in this article are solely those of the authors and do not necessarily represent those of their affiliated organizations, or those of the publisher, the editors and the reviewers. Any product that may be evaluated in this article, or claim that may be made by its manufacturer, is not guaranteed or endorsed by the publisher.
Supplementary material
The Supplementary Material for this article can be found online at: https://www.frontiersin.org/articles/10.3389/fpls.2023.1228749/full#supplementary-material
Abbreviations
DON, Deoxynivalenol; NIV, Nivalenol; ZEA, Zearalenone; FHB, Fusarium Head Blight; ISR, Induced Systemic Resistance; LPS, Lipopolysaccharides; FOL, F. oxysporum f. sp. lycopersici; PR, Pathogenesis-Related; VOC, Volatile Organic Compound; BEA, beauvericin; ENN, enniatins.
References
Abbasi, S., Safaie, N., Sadeghi, A., Shamsbakhsh, M. (2019). Streptomyces strains induce resistance to Fusarium oxysporum f. sp. lycopersici Race 3 in tomato through different molecular mechanisms. Front. Microbiol. 10. doi: 10.3389/fmicb.2019.01505
Abdel-Azeem, A. M., Abdel-Azeem, M. A., Darwish, A. G., Nafady, N. A., Ibrahim, N. A. (2019). “Fusarium: Biodiversity, ecological significances, and industrial applications,” in Recent Advancement in White Biotechnology Through Fungi: Volume 1: Diversity and Enzymes Perspectives Fungal Biology. Eds. Yadav, A. N., Mishra, S., Singh, S., Gupta, A. (Cham: Springer International Publishing), 201–261. doi: 10.1007/978-3-030-10480-1_6
Abdelrahman, M., Abdel-Motaal, F., El-Sayed, M., Jogaiah, S., Shigyo, M., Ito, S., et al. (2016). Dissection of Trichoderma longibrachiatum - induced defense in onion (Allium cepa L.) against Fusarium oxysporum f. sp. cepa by target metabolite profiling. Plant Sci. 246, 128–138. doi: 10.1016/j.plantsci.2016.02.008
Abo-Elyousr, K. A. M., Saad, M. M., Al-Qurashi, A. D., Ibrahim, O. H. M., Mousa, M. A. A. (2022). Management of cumin wilt caused by Fusarium oxysporum using native endophytic bacteria. Agronomy 12, 1–14. doi: 10.3390/agronomy12102510
Adeniji, A. A., Aremu, O. S., Babalola, O. O. (2019). Selecting lipopeptide-producing, Fusarium-suppressing Bacillus spp.: Metabolomic and genomic probing of Bacillus velezensis NWUMFkBS10.5. MicrobiologyOpen 8 (6), e00742. doi: 10.1002/mbo3.742
Agarwal, M., Dheeman, S., Dubey, R. C., Kumar, P., Maheshwari, D. K., Bajpai, V. K. (2017). Differential antagonistic responses of Bacillus pumilus MSUA3 against Rhizoctonia solani and Fusarium oxysporum causing fungal diseases in Fagopyrum esculentum Moench. Microbiol. Res. 205, 40–47. doi: 10.1016/j.micres.2017.08.012
Ahmad, T., Bashir, A., Farooq, S., Riyaz-Ul-Hassan, S. (2022). Burkholderia gladioli E39CS3, an endophyte of Crocus sativus Linn., induces host resistance against corm-rot caused by Fusarium oxysporum. J. Appl. Microbiol. 132, 495–508. doi: 10.1111/jam.15190
Ajmal, M., Hussain, A., Ali, A., Chen, H., Lin, H. (2023). Strategies for controlling the sporulation in Fusarium spp. J. Fungi. 9, 10. doi: 10.3390/jof9010010
Akram, W., Anjum, T., Ali, B. (2015). Searching ISR determinant/s from Bacillus subtilis IAGS174 against Fusarium wilt of tomato. BioControl 60, 271–280. doi: 10.1007/s10526-014-9636-1
Akram, W., Mahboob, A., Javed, A. A. (2013). Bacillus thuringiensis strain 199 can induce systemic resistance in tomato against Fusarium wilt. Eur. J. Microbiol. Immunol. 3, 275–280. doi: 10.1556/EuJMI.3.2013.4.7
Alabouvette, C. (1986). Fusarium-wilt suppressive soils from the Châteaurenard region : review of a 10-year study. Agronomie 6 (3), 273–284. doi: 10.1051/agro:19860307
Alabouvette, C., Couteaudier, Y., Louvet, J., Bremeersch, P., Richard, P., Soulas, M.-L. (1985). Recherches sur la resistance des sols aux maladies. XI. Etude comparative du comportement des Fusarium spp. dans un sol résistant et un sol sensible aux fusarioses vasculaires enrichis en glucose. Agronomie 5, 63–68. doi: 10.1051/agro:19850109
Al-Hatmi, A. M., de Hoog, G. S., Meis, J. F. (2019). Multiresistant Fusarium pathogens on plants and humans: solutions in (from) the antifungal pipeline? Infect. Drug Resist. 12, 3727–3737. doi: 10.2147/IDR.S180912
Almario, J., Muller, D., Défago, G., Moënne-Loccoz, Y. (2014). Rhizosphere ecology and phytoprotection in soils naturally suppressive to Thielaviopsis black root rot of tobacco. Environ. Microbiol. 16, 1949–1960. doi: 10.1111/1462-2920.12459
Amer, M. A., El-Samra, I. A., Abou-El-Seoud, I. I., El-Abd, S. M., Shawertamimi, N. K. (2014). Induced systemic resistance in tomato plants against Fusarium wilt disease using biotic inducers. Middle East J. Agric. Res. 3 (4), 1090–1103.
Amir, H., Alabouvette, C. (1993). Involvement of soil abiotic factors in the mechanisms of soil suppressiveness to Fusarium wilts. Soil Biol. Biochem. 25, 157–164. doi: 10.1016/0038-0717(93)90022-4
Ares, J. L. A., Ferro, R. C. A., Ramirez, L. C., González, J. M. (2004). Fusarium graminearum Schwabe, a maize root and stalk rot pathogen isolated from lodged plants in Northwest Spain. Span. J. Agric. Res. 2, 249–252. doi: 10.5424/sjar/2004022-82
Arie, T. (2019). Fusarium diseases of cultivated plants, control, diagnosis, and molecular and genetic studies. J. Pestic. Sci. 44, 275–281. doi: 10.1584/jpestics.J19-03
Aydi Ben Abdallah, R., Stedel, C., Garagounis, C., Nefzi, A., Jabnoun-Khiareddine, H., Papadopoulou, K. K., et al. (2017). Involvement of lipopeptide antibiotics and chitinase genes and induction of host defense in suppression of Fusarium wilt by endophytic Bacillus spp. in tomato. Crop Prot. 99, 45–58. doi: 10.1016/j.cropro.2017.05.008
Babadoost, M. (2018). “Fusarium: Historical and continued importance,” in Fusarium—Plant Diseases, Pathogen Diversity, Genetic Diversity, Resistance and Molecular Markers. Ed. Askun, T. (London, UK: Intech Open), 13–24. doi: 10.5772/intechopen.74147
Backhouse, D., Burgess, L. W. (2002). Climatic analysis of the distribution of Fusarium graminearum, F. pseudograminearum and F. culmorum on cereals in Australia. Australas. Plant Pathol. 31, 321–327. doi: 10.1071/AP02026
Bai, G., Shaner, G. (1994). Scab of wheat: Prospects for control. Plant Dis. 78, 760–766. doi: 10.1094/PD-78-0760
Bailey, K. L., Lazarovits, G. (2003). Suppressing soil-borne diseases with residue management and organic amendments. Soil Tillage Res. 72, 169–180. doi: 10.1016/S0167-1987(03)00086-2
Baker, K. F., Cook, R. J. (1974). Biological control of plant pathogens. St. Paul: Am. Phytopathol. Soc 433.
Bakker, M. G., Schlatter, D. C., Otto-Hanson, L., Kinkel, L. L. (2014). Diffuse symbioses: roles of plant–plant, plant–microbe and microbe–microbe interactions in structuring the soil microbiome. Mol. Ecol. 23, 1571–1583. doi: 10.1111/mec.12571
Banerjee, A., Mittra, B. (2018). Morphological modification in wheat seedlings infected by Fusarium oxysporum. Eur. J. Plant Pathol. 152, 521–524. doi: 10.1007/s10658-018-1470-3
Bastian, F., Jurado, V., Nováková, A., Alabouvette, C., Saiz-Jimenez, C. (2010). The microbiology of Lascaux cave. Microbiol. Read. Engl. 156, 644–652. doi: 10.1099/mic.0.036160-0
Bautista, D., García, D., Dávila, L., Caro-Quintero, A., Cotes, A. M., González, A., et al. (2023). Studying the microbiome of suppressive soils against vascular wilt, caused by Fusarium oxysporum in cape gooseberry (Physalis Peruviana). Environ. Microbiol. Rep. 15, 1–12. doi: 10.1111/1758-2229.13195
Berendsen, R. L., Pieterse, C. M. J., Bakker, P. A. H. M. (2012). The rhizosphere microbiome and plant health. Trends Plant Sci. 17, 478–486. doi: 10.1016/j.tplants.2012.04.001
Blacutt, A. A., Gold, S. E., Voss, K. A., Gao, M., Glenn, A. E. (2018). Fusarium verticillioides: Advancements in understanding the toxicity, virulence, and niche adaptations of a model mycotoxigenic pathogen of maize. Phytopathology 108, 312–326. doi: 10.1094/PHYTO-06-17-0203-RVW
Blümke, A., Falter, C., Herrfurth, C., Sode, B., Bode, R., Schäfer, W., et al. (2014). Secreted fungal effector lipase releases free fatty acids to inhibit innate immunity-related callose formation during wheat head infection. Plant Physiol. 165, 346–358. doi: 10.1104/pp.114.236737
Bockus, W. W., Shroyer, J. P. (1998). The impact of reduced tillage on soilborne plant pathogens. Annu. Rev. Phytopathol. 36, 485–500. doi: 10.1146/annurev.phyto.36.1.485
Britannica, The Editors of Encyclopaedia (2017) Fusarium wilt. Available at: https://www.britannica.com/science/fusarium-wilt (Accessed June 15 2022).
Brown, N. A., Urban, M., van de Meene, A. M. L., Hammond-Kosack, K. E. (2010). The infection biology of Fusarium graminearum: Defining the pathways of spikelet to spikelet colonisation in wheat ears. Fungal Biol. 114, 555–571. doi: 10.1016/j.funbio.2010.04.006
Bubici, G., Kaushal, M., Prigigallo, M. I., Gómez-Lama Cabanás, C., Mercado-Blanco, J. (2019). Biological control agents against Fusarium wilt of banana. Front. Microbiol. 10, 616. doi: 10.3389/fmicb.2019.00616
Burgess, L. W., Bryden, W. L. (2012). Fusarium: A ubiquitous fungus of global importance. Microbiol. Aust. 33, 22–25. doi: 10.1071/MA12022
Burgess, L. W. B., Summerell, A., Backhouse, D., Benyon, F., Lević, J. (1996). Biodiversity and population studies in Fusarium. Sydowia 48, 1–11.
Campos, S. B., Lisboa, B. B., Camargo, F. A. O., Bayer, C., Sczyrba, A., Dirksen, P., et al. (2016). Soil suppressiveness and its relations with the microbial community in a Brazilian subtropical agroecosystem under different management systems. Soil Biol. Biochem. 96, 191–197. doi: 10.1016/j.soilbio.2016.02.010
Cao, Y., Wang, J., Wu, H., Yan, S., Guo, D., Wang, G., et al. (2016). Soil chemical and microbial responses to biogas slurry amendment and its effect on Fusarium wilt suppression. Appl. Soil Ecol. 107, 116–123. doi: 10.1016/j.apsoil.2016.05.010
Carrión, V. J., Cordovez, V., Tyc, O., Etalo, D. W., de Bruijn, I., de Jager, V. C. L., et al. (2018). Involvement of Burkholderiaceae and sulfurous volatiles in disease-suppressive soils. ISME J. 12, 2307–2321. doi: 10.1038/s41396-018-0186-x
Castaño, R., Borrero, C., Avilés, M. (2011). Organic matter fractions by SP-MAS 13C NMR and microbial communities involved in the suppression of Fusarium wilt in organic growth media. Biol. Control. 58, 286–293. doi: 10.1016/j.biocontrol.2011.05.011
Cha, J.-Y., Han, S., Hong, H.-J., Cho, H., Kim, D., Kwon, Y., et al. (2016). Microbial and biochemical basis of a Fusarium wilt-suppressive soil. ISME J. 10, 119–129. doi: 10.1038/ismej.2015.95
Chatterton, S., Punja, Z. K. (2009). Chitinase and beta-1,3-glucanase enzyme production by the mycoparasite Clonostachys rosea f. catenulata against fungal plant pathogens. Can. J. Microbiol. 55, 356–367. doi: 10.1139/w08-156
Chen, L., Heng, J., Qin, S., Bian, K. (2018). A comprehensive understanding of the biocontrol potential of Bacillus velezensis LM2303 against Fusarium head blight. PloS One 13 (6), e0198560. doi: 10.1371/journal.pone.0198560
Chen, Y., Kistler, H. C., Ma, Z. (2019). Fusarium graminearum trichothecene mycotoxins: Biosynthesis, regulation, and management. Annu. Rev. Phytopathol. 57, 15–39. doi: 10.1146/annurev-phyto-082718-100318
Chng, S., Cromey, M. G., Dodd, S. L., Stewart, A., Butler, R. C., Jaspers, M. V. (2015). Take-all decline in New Zealand wheat soils and the microorganisms associated with the potential mechanisms of disease suppression. Plant Soil. 397, 239–259. doi: 10.1007/s11104-015-2620-4
Choudhary, D. K., Prakash, A., Johri, B. N. (2007). Induced systemic resistance (ISR) in plants: mechanism of action. Indian J. Microbiol. 47, 289–297. doi: 10.1007/s12088-007-0054-2
Coleman, J. J. (2016). The Fusarium solani species complex: ubiquitous pathogens of agricultural importance. Mol. Plant Pathol. 17, 146–158. doi: 10.1111/mpp.12289
Constantin, M. E., Fokkens, L., de Sain, M., Takken, F. L., Rep, M. (2021). Number of candidate effector genes in accessory genomes differentiates pathogenic from endophytic Fusarium oxysporum strains. Front. Plant Sci. 12, 761740. doi: 10.3389/fpls.2021.761740
Cook, R. J., Rovira, A. D. (1976). The role of bacteria in the biological control of Gaeumannomyces graminis by suppressive soils. Soil Biol. Biochem. 8, 269–273. doi: 10.1016/0038-0717(76)90056-0
Cordovez, V., Carrion, V. J., Etalo, D. W., Mumm, R., Zhu, H., van Wezel, G. P., et al. (2015). Diversity and functions of volatile organic compounds produced by Streptomyces from a disease-suppressive soil. Front. Microbiol. 6. doi: 10.3389/fmicb.2015.01081
Cotten, T. K., Munkvold, G. P. (1998). ). Survival of Fusarium moniliforme, F. proliferatum, and F. subglutinans in maize stalk residue. Phytopathology 88, 550–555. doi: 10.1094/PHYTO.1998.88.6.550
Crous, P. W., Lombard, L., Sandoval-Denis, M., Seifert, K. A., Schroers, H. J., Chaverri, P., et al. (2021). Fusarium: more than a node or a foot-shaped basal cell. Stud. Mycol. 98, 100116. doi: 10.1016/j.simyco.2021.100116
Cuesta, G., García-de-la-Fuente, R., Abad, M., Fornes, F. (2012). Isolation and identification of actinomycetes from a compost-amended soil with potential as biocontrol agents. J. Environ. Manage. 95, S280–S284. doi: 10.1016/j.jenvman.2010.11.023
Curtis, T. P., Sloan, W. T., Scannell, J. W. (2002). Estimating prokaryotic diversity and its limits. Proc. Natl. Acad. Sci. U. S. A. 99, 10494–10499. doi: 10.1073/pnas.142680199
Cuzick, A., Urban, M., Hammond-Kosack, K. (2008). Fusarium graminearum gene deletion mutants map1 and tri5 reveal similarities and differences in the pathogenicity requirements to cause disease on Arabidopsis and wheat floral tissue. New Phytol. 177, 990–1000. doi: 10.1111/j.1469-8137.2007.02333.x
Czembor, E., Stępień, Ł., Waśkiewicz, A. (2015). Effect of environmental factors on Fusarium species and associated mycotoxins in maize grain grown in Poland. PloS One 10, e0133644. doi: 10.1371/journal.pone.0133644
da Silva Santos, A. C., Diniz, A. G., Tiago, P. V., de Oliveira, N. T. (2020). Entomopathogenic Fusarium species: a review of their potential for the biological control of insects, implications and prospects. Fungal Biol. Rev. 34, 41–57. doi: 10.1016/j.fbr.2019.12.002
Dastjerdi, R., Karlovsky, P. (2015). Systemic infection of maize, sorghum, rice, and beet seedlings with fumonisin-producing and non-producing Fusarium verticillioides strains. Plant Pathol. J. 31, 334–342. doi: 10.5423/PPJ.OA.05.2015.0088
Dean, R., Van Kan, J. A. L., Pretorius, Z. A., Hammond-Kosack, K. E., Di Pietro, A., Spanu, P. D., et al. (2012). The top 10 fungal pathogens in molecular plant pathology. Mol. Plant Pathol. 13 (4), 414–430. doi: 10.1111/j.1364-3703.2011.00783.x
De Boer, W., Li, X., Meisner, A., Garbeva, P. (2019). Pathogen suppression by microbial volatile organic compounds in soils. FEMS Microbiol. Ecol. 95 (8), fiz105. doi: 10.1093/femsec/fiz105
De Corato, U., Patruno, L., Avella, N., Salimbeni, R., Lacolla, G., Cucci, G., et al. (2020). Soil management under tomato-wheat rotation increases the suppressive response against Fusarium wilt and tomato shoot growth by changing the microbial composition and chemical parameters. Appl. Soil Ecol. 154, 103601. doi: 10.1016/j.apsoil.2020.103601
De Lamo, F. J., Takken, F. L. (2020). Biocontrol by Fusarium oxysporum using endophyte-mediated resistance. Front. Plant Sci. 11, 37. doi: 10.3389/fpls.2020.00037
Deltour, P., Franca, S. C., Pereira, O. L., Cardoso, I., De Neve, S., Debode, J., et al. (2017). Disease suppressiveness to Fusarium wilt of banana in an agroforestry system: influence of soil characteristics and plant community. Agric. Ecosyst. Environ. 239, 173–181. doi: 10.1016/j.agee.2017.01.018
Desjardins, A. E. (2006). Fusarium mycotoxins: chemistry, genetics, and biology (St Paul, MN, USA: APS Press).
Dhanti, K. R., Widiastuti, A., Joko, T. (2017). “Detection of mycotoxin-encoding genes in Fusarium spp. isolated from maize kernels in Indonesia,” in Proceeding of the 1st International Conference on Tropical Agriculture, Cham (Switzerland: Springer International Publishing). doi: 10.1007/978-3-319-60363-6_11
Dita, M., Barquero, M., Heck, D., Mizubuti, E. S. G., Staver, C. P. (2018). Fusarium wilt of banana: Current knowledge on epidemiology and research needs toward sustainable disease management. Front. Plant Sci. 9. doi: 10.3389/fpls.2018.01468
Domínguez, J., Negrín, M. A., Rodríguez, C. M. (1996). Soil chemical characteristics in relation to Fusarium wilts in banana crops of Gran Canaria Island (Spain). Commun. Soil Sci. Plant Anal. 27, 2649–2662. doi: 10.1080/00103629609369729
Du, S., Trivedi, P., Wei, Z., Feng, J., Hu, H. W., Bi, L., et al. (2022). The proportion of soil-borne fungal pathogens increases with elevated organic carbon in agricultural soils. mSystems 7, e01337–e01321. doi: 10.1128/msystems.01337-21
Duijff, B. J., Gianinazzi-Pearson, V., Lemanceau, P. (1997). Involvement of the outer membrane lipopolysaccharides in the endophytic colonization of tomato roots by biocontrol Pseudomonas fluorescens strain WCS417r. New Phytol. 135, 325–334. doi: 10.1046/j.1469-8137.1997.00646.x
Duijff, B. J., Pouhair, D., Olivain, C., Alabouvette, C., Lemanceau, P. (1998). Implication of systemic induced resistance in the suppression of Fusarium wilt of tomato by Pseudomonas fluorescens WCS417r and by nonpathogenic Fusarium oxysporum Fo47. Eur. J. Plant Pathol. 104, 903–910. doi: 10.1023/A:1008626212305
Dundore-Arias, J. P., Castle, S. C., Felice, L., Dill-Macky, R., Kinkel, L. L. (2020). Carbon amendments influence composition and functional capacities of indigenous soil microbiomes. Front. Mol. Biosci. 6. doi: 10.3389/fmolb.2019.00151
Edel-Hermann, V., Lecomte, C. (2019). Current status of Fusarium oxysporum formae speciales and races. Phytopathology 109 (4), 512–530. doi: 10.1094/PHYTO-08-18-0320-RVW
Elhassan, A., El-Tilib, A., H.S., I., Awadelkarim, A. (2010). Effect of phosphorus fertilizer treatments on incidence of Fusarium root-rot/wilt disease complex, and on yield components of lupine, chickpea and lentil crops. Arab Univ. J. Agric. Sci. 18, 193–202. doi: 10.21608/ajs.2010.14989
Eparvier, A., Alabouvette, C. (1994). Use of ELISA and GUS-transformed strains to study competition between pathogenic and non-pathogenic Fusarium oxysporum for root colonization. Biocontrol Sci. Technol. 4, 35–47. doi: 10.1080/09583159409355310
Evans, M. L., Hollaway, G. J., Dennis, J. I., Correll, R., Wallwork, H. (2010). Crop sequence as a tool for managing populations of Fusarium pseudograminearum and F. culmorum in South-Eastern Australia. Australas. Plant Pathol. 39, 376–382. doi: 10.1071/AP09092
Fan, H., He, P., Xu, S., Li, S., Wang, Y., Zhang, W., et al. (2023). Banana disease-suppressive soil drives Bacillus assembled to defense Fusarium wilt of banana. Front. Microbiol. 14, 1211301. doi: 10.3389/fmicb.2023.1211301
Fang, X., You, M. P., Barbetti, M. J. (2012). Reduced severity and impact of Fusarium wilt on strawberry by manipulation of soil pH, soil organic amendments and crop rotation. Eur. J. Plant Pathol. 134, 619–629. doi: 10.1007/s10658-012-0042-1
Fatima, S., Anjum, T. (2017). Identification of a potential ISR determinant from Pseudomonas aeruginosa PM12 against Fusarium wilt in tomato. Front. Plant Sci. 8. doi: 10.3389/fpls.2017.00848
Ferrocino, I., Chitarra, W., Pugliese, M., Gilardi, G., Gullino, M. L., Garibaldi, A. (2013). Effect of elevated atmospheric CO2 and temperature on disease severity of Fusarium oxysporum f.sp. lactucae on lettuce plants. Appl. Soil Ecol. 72, 1–6. doi: 10.1016/j.apsoil.2013.05.015
Fu, L., Ruan, Y., Tao, C., Li, R., Shen, Q. (2016). Continous application of bioorganic fertilizer induced resilient culturable bacteria community associated with banana Fusarium wilt suppression. Sci. Rep. 6, 27731. doi: 10.1038/srep27731
Fuchs, J.-G., Moënne-Loccoz, Y., Défago, G. (1997). Nonpathogenic Fusarium oxysporum strain Fo47 induces resistance to Fusarium wilt in tomato. Plant Dis. 81, 492–496. doi: 10.1094/PDIS.1997.81.5.492
Fuchs, J.-G., Moënne-Loccoz, Y., Défago, G. (1999). Ability of nonpathogenic Fusarium oxysporum Fo47 to protect tomato against Fusarium wilt. Biol. Control. 14, 105–110. doi: 10.1006/bcon.1998.0664
Gai, X., Dong, H., Wang, S., Liu, B., Zhang, Z., Li, X., et al. (2018). Infection cycle of maize stalk rot and ear rot caused by Fusarium verticillioides. PloS One 13, e0201588. doi: 10.1371/journal.pone.0201588
Galletti, S., Paris, R., Cianchetta, S. (2020). Selected isolates of Trichoderma gamsii induce different pathways of systemic resistance in maize upon Fusarium verticillioides challenge. Microbiol. Res. 233, 126406. doi: 10.1016/j.micres.2019.126406
Garbeva, P., Hol, W. H. G., Termorshuizen, A. J., Kowalchuk, G. A., de Boer, W. (2011). Fungistasis and general soil biostasis – A new synthesis. Soil Biol. Biochem. 43, 469–477. doi: 10.1016/j.soilbio.2010.11.020
Garbeva, P., van Veen, J. A., van Elsas, J. D. (2004). Microbial diversity in soil: selection microbial populations by plant and soil type and implications for disease suppressiveness. Annu. Rev. Phytopathol. 42, 243–270. doi: 10.1146/annurev.phyto.42.012604.135455
Garibaldi, A., Dalla, G. C., Della, G. C., D’Aquila, F. (1983). Osservazioni su terreni repressivi nei confronti di Fusarium oxysporum f. sp. dianthi (Prill. et Del.) Snyd. et Hans. Riv. Ortoflorofrutticoltura Ital. 67, 251–259.
Gatch, E. W., du Toit, L. J. (2017). Limestone-mediated suppression of Fusarium wilt in spinach seed crops. Plant Dis. 101, 81–94. doi: 10.1094/PDIS-04-16-0423-RE
Geiser, D. M., Aoki, T., Bacon, C. W., Baker, S. E., Bhattacharyya, M. K., Brandt, M. E., et al. (2013). One fungus, one name: Defining the genus Fusarium in a scientifically robust way that preserves longstanding use. Phytopathology 103, 400–408. doi: 10.1094/PHYTO-07-12-0150-LE
Gómez Expósito, R., de Bruijn, I., Postma, J., Raaijmakers, J. M. (2017). Current insights into the role of rhizosphere bacteria in disease suppressive soils. Front. Microbiol. 8. doi: 10.3389/fmicb.2017.02529
Goswami, R. S., Kistler, H. C. (2004). Heading for disaster: Fusarium graminearum on cereal crops. Mol. Plant Pathol. 5, 515–525. doi: 10.1111/j.1364-3703.2004.00252.x
Gouy, M., Guindon, S., Gascuel, O. (2010). SeaView version 4: A multiplatform graphical user interface for sequence alignment and phylogenetic tree building. Mol. Biol. Evol. 27, 221–224. doi: 10.1093/molbev/msp259
Govaerts, B., Mezzalama, M., Sayre, K. D., Crossa, J., Lichter, K., Troch, V., et al. (2008). Long-term consequences of tillage, residue management, and crop rotation on selected soil micro-flora groups in the subtropical highlands. Appl. Soil Ecol. 38, 197–210. doi: 10.1016/j.apsoil.2007.10.009
Gruet, C., Alaoui, M., Gerin, F., Prigent-Combaret, C., Börner, A., Muller, D., et al. (2023). Genomic content of wheat has a higher influence than plant domestication status on the ability to interact with Pseudomonas plant growth-promoting rhizobacteria. Plant Cell Environ. 46, 3933–3948. doi: 10.1111/pce.14698
Gu, Q., Yang, Y., Yuan, Q., Shi, G., Wu, L., Lou, Z., et al. (2017). Bacillomycin D produced by Bacillus amyloliquefaciens is involved in the antagonistic interaction with the plant-pathogenic fungus Fusarium graminearum. Appl. Environ. Microbiol. 83, e01075–e01017. doi: 10.1128/AEM.01075-17
He, J., Boland, G. J., Zhou, T. (2009). Concurrent selection for microbial suppression of Fusarium graminearum, Fusarium head blight and deoxynivalenol in wheat. J. Appl. Microbiol. 106, 1805–1817. doi: 10.1111/j.1365-2672.2009.04147.x
Herron, D. A., Wingfield, M. J., Wingfield, B. D., Rodas, C. A., Marincowitz, S., Steenkamp, E. T. (2015). Novel taxa in the Fusarium fujikuroi species complex from Pinus spp. Stud. Mycol. 80, 131–150. doi: 10.1016/j.simyco.2014.12.001
Höper, H., Steinberg, C., Alabouvette, C. (1995). Involvement of clay type and pH in the mechanisms of soil suppressiveness to Fusarium wilt of flax. Soil Biol. Biochem. 27, 955–967. doi: 10.1016/0038-0717(94)00238-V
Husaini, A. M., Sakina, A., Cambay, S. R. (2018). Host-pathogen interaction in Fusarium oxysporum infections: Where do we stand? Mol. Plant-Microbe Interact. 31, 889–898. doi: 10.1094/MPMI-12-17-0302-CR
Ittu, M., Hagima, I., Moraru, I., Raducanu, F. (1995). Reaction of some wheat and triticale genotypes to toxins, culture filtrates and cultures of Fusarium. In vivo screening and the relation between results obtained in vivo and in vitro. Theor. Appl. Genet. 27 (1), 1–13.
Janvier, C., Villeneuve, F., Alabouvette, C., Edel-Hermann, V., Mateille, T., Steinberg, C. (2007). Soil health through soil disease suppression: Which strategy from descriptors to indicators? Soil Biol. Biochem. 39, 1–23. doi: 10.1016/j.soilbio.2006.07.001
Jard, G., Liboz, T., Mathieu, F., Guyonvarch, A., Lebrihi, A. (2011). Review of mycotoxin reduction in food and feed: From prevention in the field to detoxification by adsorption or transformation. Food Addit. Contam. 28, 1590–1609. doi: 10.1080/19440049.2011.595377
Jeffries, P. (1995). Biology and ecology of mycoparasitism. Can. J. Bot. 73, 1284–1290. doi: 10.1139/b95-389
Jia, L.-J., Tang, H.-Y., Wang, W.-Q., Yuan, T.-L., Wei, W.-Q., Pang, B., et al. (2019). A linear nonribosomal octapeptide from Fusarium graminearum facilitates cell-to-cell invasion of wheat. Nat. Commun. 10, 922. doi: 10.1038/s41467-019-08726-9
Jiang, G., Zhang, Y., Gan, G., Li, W., Wan, W., Jiang, Y., et al. (2022). Exploring rhizo-microbiome transplants as a tool for protective plant-microbiome manipulation. ISME Commun. 2, 1–10. doi: 10.1038/s43705-022-00094-8
Jiménez-Díaz, R. M., Castillo, P., Jiménez-Gasco, M., del, M., Landa, B. B., Navas-Cortés, J. A. (2015). Fusarium wilt of chickpeas: Biology, ecology and management. Crop Prot. 73, 16–27. doi: 10.1016/j.cropro.2015.02.023
Johnson, E. T., Bowman, M. J., Dunlap, C. A. (2020). Brevibacillus fortis NRS-1210 produces edeines that inhibit the in vitro growth of conidia and chlamydospores of the onion pathogen Fusarium oxysporum f. sp. cepae. Antonie Van Leeuwenhoek. 113, 973–987. doi: 10.1007/s10482-020-01404-7
Keszthelyi, A., Jeney, A., Kerényi, Z., Mendes, O., Waalwijk, C., Hornok, L. (2007). Tagging target genes of the MAT1-2-1 transcription factor in Fusarium verticillioides (Gibberella fujikuroi MP-A). Antonie Van Leeuwenhoek 91, 373–391. doi: 10.1007/s10482-006-9123-5
Khan, M. R., Doohan, F. M. (2009). Bacterium-mediated control of Fusarium Head Blight disease of wheat and barley and associated mycotoxin contamination of grain. Biol. Control. 48, 42–47. doi: 10.1016/j.biocontrol.2008.08.015
Khan, M. R., Fischer, S., Egan, D., Doohan, F. M. (2006). Biological control of Fusarium seedling blight disease of wheat and barley. Phytopathology 96, 386–394. doi: 10.1094/PHYTO-96-0386
Khan, N., Martínez-Hidalgo, P., Ice, T. A., Maymon, M., Humm, E. A., Nejat, N., et al. (2018). Antifungal activity of Bacillus species against Fusarium and analysis of the potential mechanisms used in biocontrol. Front. Microbiol. 9, 2363. doi: 10.3389/fmicb.2018.02363
Khan, N., Maymon, M., Hirsch, A. (2017). Combating Fusarium infection using Bacillus-based antimicrobials. Microorganisms 5, 75. doi: 10.3390/microorganisms5040075
Khemir, E., Samira, C., Moretti, A., Gharbi, M. S., Allagui, M. B., Gargouri, S. (2020). Impacts of previous crops on inoculum of Fusarium culmorum in soil, and development of foot and root rot of durum wheat in Tunisia. Phytopathol. Mediterr. 59, 187–201. doi: 10.36253/phyto-10827
Kim, Y.-T., Monkhung, S., Lee, Y. S., Kim, K. Y. (2019). Effects of Lysobacter antibioticus HS124, an effective biocontrol agent against Fusarium graminearum, on crown rot disease and growth promotion of wheat. Can. J. Microbiol. 65, 904–912. doi: 10.1139/cjm-2019-0285
Klein, E., Ofek, M., Katan, J., Minz, D., Gamliel, A. (2013). Soil suppressiveness to Fusarium disease: Shifts in root microbiome associated with reduction of pathogen root colonization. Phytopathology 103, 23–33. doi: 10.1094/PHYTO-12-11-0349
Kloepper, J. W., Leong, J., Teintze, M., Schroth, M. N. (1980). Pseudomonas siderophores: A mechanism explaining disease-suppressive soils. Curr. Microbiol. 4, 317–320. doi: 10.1007/BF02602840
Knudsen, I. M. B., Debosz, K., Hockenhull, J., Jensen, D. F., Elmholt, S. (1999). Suppressiveness of organically and conventionally managed soils towards brown foot rot of barley. Appl. Soil Ecol. 12, 61–72. doi: 10.1016/s0929-1393(98)00156-5
Kubicek, C. P., Herrera-Estrella, A., Seidl-Seiboth, V., Martinez, D. A., Druzhinina, I. S., Thon, M., et al. (2011). Comparative genome sequence analysis underscores mycoparasitism as the ancestral life style of Trichoderma. Genome Biol. 12, 1–15. doi: 10.1186/gb-2011-12-4-r40
Kuć, J. (1995). “Induced systemic resistance — an overview,” in Induced Resistance to Disease in Plants. Eds. Hammerschmidt, R., Kuć, J. (Dordrecht: Springer Netherlands), 169–175. doi: 10.1007/978-94-015-8420-3_8
Kumari, P., Khanna, V. (2019). Seed bacterization stimulated resistance in chickpea against Fusarium oxysporum f. sp. ciceris. Indian Phytopathol. 72, 689–697. doi: 10.1007/s42360-019-00163-4
Kurek, E., Jaroszuk-Ściseł, J. (2003). Rye (Secale cereale) growth promotion by Pseudomonas fluorescens strains and their interactions with Fusarium culmorum under various soil conditions. Biol. Control. 26, 48–56. doi: 10.1016/S1049-9644(02)00115-9
Kyselková, M., Kopecký, J., Frapolli, M., Défago, G., Ságová-Marečková, M., Grundmann, G., et al. (2009). Comparison of rhizobacterial community composition in soil suppressive or conducive to tobacco black root rot disease. ISME J. 3, 1127–1138. doi: 10.1038/ismej.2009.61
Kyselková, M., Moënne-Loccoz, Y. (2012). “Pseudomonas and other microbes in disease-suppressive soils,” in Organic Fertilisation, Soil Quality and Human Health Sustainable Agriculture Reviews. Ed. Lichtfouse, E. (Dordrecht: Springer Netherlands), 93–140. doi: 10.1007/978-94-007-4113-3_5
Laraba, I., McCormick, S. P., Vaughan, M. M., Geiser, D. M., O’Donnell, K. (2021). Phylogenetic diversity, trichothecene potential, and pathogenicity within Fusarium sambucinum species complex. PloS One 16, e0245037. doi: 10.1371/journal.pone.0245037
Larkin, R. P., Hopkins, D. L., Martin, F. N. (1993). Ecology of Fusarium oxysporum f. sp. niveum in soils suppressive and conducive to Fusarium wilt of watermelon. Phytopathology 83, 1105–1116. doi: 10.1094/Phyto-83-1105
Laville, J., Voisard, C., Keel, C., Maurhofer, M., Défago, G., Haas, D. (1992). Global control in Pseudomonas fluorescens mediating antibiotic synthesis and suppression of black root rot of tobacco. Proc. Natl. Acad. Sci. U. S. A. 89, 1562–1566. doi: 10.1073/pnas.89.5.1562
Leeman, M., van Pelt, J. A., Den Ouden, F. M., Heinsbroek, M., Bakker, P. A. H. M., Schippers, B. (1995). Induction of systemic resistance against Fusarium wilt of radish by lipopolysaccharides of Pseudomonas fluorescens. Phytopathology 85, 1021–1027. doi: 10.1094/Phyto-85-1021
Legrand, F., Chen, W., Cobo-Díaz, J. F., Picot, A., Le Floch, G. (2019). Co-occurrence analysis reveal that biotic and abiotic factors influence soil fungistasis against Fusarium graminearum. FEMS Microbiol. Ecol. 95, fiz056. doi: 10.1093/femsec/fiz056
Legrand, F., Picot, A., Cobo-Díaz, J. F., Chen, W., Le Floch, G. (2017). Challenges facing the biological control strategies for the management of Fusarium Head Blight of cereals caused by F. graminearum. Biol. Control. 113, 26–38. doi: 10.1016/j.biocontrol.2017.06.011
Lei, W., Xiao-Ming, W., Rong-Qi, X., Hong-Jie, L. (2011). Root infection and systematic colonization of DsRed-labeled Fusarium verticillioides in maize. Acta Agron. Sin. 37, 793–802. doi: 10.1016/S1875-2780(11)60023-0
Lemanceau, P., Alabouvette, C. (1991). Biological control of Fusarium diseases by fluorescent Pseudomonas and non-pathogenic Fusarium. Crop Prot. 10, 279–286. doi: 10.1016/0261-2194(91)90006-D
Lemanceau, P., Bakker, P., de Kogel, W., Alabouvette, C., Schippers, B. (1993). Antagonistic effect of nonpathogenic Fusarium oxysporum Fo47 and pseudobactin 358 upon pathogenic Fusarium oxysporum f. sp. dianthi. Appl. Environ. Microbiol. 59, 74–82. doi: 10.1128/AEM.59.1.74-82.1993
Lemanceau, P., Blouin, M., Muller, D., Moënne-Loccoz, Y. (2017). Let the core microbiota be functional. Trends Plant Sci. 22, 583–595. doi: 10.1016/j.tplants.2017.04.008
Lemmens, M., Haim, K., Lew, H., Ruckenbauer, P. (2004). The effect of nitrogen fertilization on Fusarium head blight development and deoxynivalenol contamination in wheat. J. Phytopathol. 152, 1–8. doi: 10.1046/j.1439-0434.2003.00791.x
Leplat, J., Friberg, H., Abid, M., Steinberg, C. (2013). Survival of Fusarium graminearum, the causal agent of Fusarium head blight. A review. Agron. Sustain. Dev. 33, 97–111. doi: 10.1007/s13593-012-0098-5
Leslie, J. F., Summerell, B. A. (2006). The Fusarium laboratory manual (Ames: John Wiley and Sons). doi: 10.1002/9780470278376
Letunic, I., Bork, P. (2021). Interactive Tree Of Life (iTOL) v5: an online tool for phylogenetic tree display and annotation. Nucleic Acids Res. 49, W293–W296. doi: 10.1093/nar/gkab301
Li, Y., Chen, S. (2019). Fusaricidin produced by Paenibacillus polymyxa WLY78 induces systemic resistance against Fusarium wilt of cucumber. Int. J. Mol. Sci. 20, 1–19. doi: 10.3390/ijms20205240
Li, R., Shen, Z., Sun, L., Zhang, R., Fu, L., Deng, X., et al. (2016). Novel soil fumigation method for suppressing cucumber Fusarium wilt disease associated with soil microflora alterations. Appl. Soil Ecol. 101, 28–36. doi: 10.1016/j.apsoil.2016.01.004
Liu, Y., Zhu, A., Tan, H., Cao, L., Zhang, R. (2019). Engineering banana endosphere microbiome to improve Fusarium wilt resistance in banana. Microbiome 7, 74. doi: 10.1186/s40168-019-0690-x
Löffler, H. J. M., Cohen, E. B., Oolbekkink, G. T., Schippers, B. (1986). Nitrite as a factor in the decline of Fusarium oxysporum f. sp. dianthi in soil supplemented with urea or ammonium chloride. Neth. J. Plant Pathol. 92, 153–162. doi: 10.1007/BF01999797
Logeshwarn, P., Thangaraju, M., Kandaiah, R. (2011). Antagonistic potential of Gluconacetobacter diazotrophicus against Fusarium oxysporum in sweet potato (Ipomea batatus). Arch. Phytopathol. Plant Prot. 44, 216–223. doi: 10.1080/03235400902952707
López-Díaz, C., Rahjoo, V., Sulyok, M., Ghionna, V., Martín-Vicente, A., Capilla, J., et al. (2018). Fusaric acid contributes to virulence of Fusarium oxysporum on plant and mammalian hosts. Mol. Plant Pathol. 19, 440–453. doi: 10.1111/mpp.12536
Louvet, J., Rouxel, F., Alabouvette, C. (1976). Recherches sur la resistance des sols aux maladies. I. Mise en evidence de la nature microbiobiologique de la résistance d’un sol au developpement de la fusariose vasculaire du melon. Ann. Phytopathol. 8, 425–436.
Lv, N., Tao, C., Ou, Y., Wang, J., Deng, X., Liu, H., et al. (2023). Root-associated antagonistic Pseudomonas spp. contribute to soil suppressiveness against banana Fusarium wilt disease of banana. Microbiol. Spectr. 11 (2), e0352522. doi: 10.1128/spectrum.03525-22
Ma, L.-J., Geiser, D. M., Proctor, R. H., Rooney, A. P., O’Donnell, K., Trail, F., et al. (2013). Fusarium pathogenomics. Annu. Rev. Microbiol. 67, 399–416. doi: 10.1146/annurev-micro-092412-155650
Magotra, S., Trakroo, D., Ganjoo, S., Vakhlu, J. (2016). “Bacillus-mediated-Induced Systemic Resistance (ISR) against Fusarium corm rot,” in Microbial-mediated Induced Systemic Resistance in Plants. Eds. Choudhary, D. K., Varma, A. (Singapore: Springer International Publishing), 15–22. doi: 10.1007/978-981-10-0388-2_2
Maher, M., Prasad, M., Raviv, M. (2008). "Organic soilless media components", in Soilless culture, theory and practice. Ed. Raviv, L. (Amsterdam: Elsevier), 459–504. doi: 10.1016/B978-044452975-6.50013-7
Maheshwari, D. K., Saraf, M., Aeron, A. (2013). Bacteria in agrobiology: Disease management (Berlin: Springer International Publishing). doi: 10.1007/978-3-642-33639-3
Marburger, D. A., Venkateshwaran, M., Conley, S. P., Esker, P. D., Lauer, J. G., Ané, J. M. (2015). Crop rotation and management effect on Fusarium spp. populations. Crop Sci. 55, 365–376. doi: 10.2135/cropsci2014.03.0199
Marshall, K. C., Alexander, M. (1960). Competition between soil bacteria and Fusarium. Plant Soil. 12, 143–153. doi: 10.1007/BF01377367
Masri, M., Sukmawaty, E., Amir, A. A. (2021). Antifungal activity of chitinolytic bacteria Lysinibacillus fusiformis and Brevibacillus reuszeri against the fungal pathogens Rhizoctonia solani and Fusarium oxysporum. Microbiol. Indones. 15, 135–138. doi: 10.5454/mi.15.4.3
Mazurier, S., Corberand, T., Lemanceau, P., Raaijmakers, J. M. (2009). Phenazine antibiotics produced by fluorescent pseudomonads contribute to natural soil suppressiveness to Fusarium wilt. ISME J. 3, 977–991. doi: 10.1038/ismej.2009.33
Mazzola, M. (2002). Mechanisms of natural soil suppressiveness to soilborne diseases. Antonie Van Leeuwenhoek. 81, 557–564. doi: 10.1023/A:1020557523557
Mendes, R., Kruijt, M., de Bruijn, I., Dekkers, E., van der Voort, M., Schneider, J. H. M., et al. (2011). Deciphering the rhizosphere microbiome for disease-suppressive bacteria. Science 332, 1097–1100. doi: 10.1126/science.1203980
Meng, T., Wang, Q., Abbasi, P., Ma, Y. (2019). Deciphering differences in the chemical and microbial characteristics of healthy and Fusarium wilt-infected watermelon rhizosphere soils. Appl. Microbiol. Biotechnol. 103, 1497–1509. doi: 10.1007/s00253-018-9564-6
Mesterházy, Á. (2002). Role of deoxynivalenol in aggressiveness of Fusarium graminearum and F. culmorum and in resistance to Fusarium Head Blight. Eur. J. Plant Pathol. 108, 675–684. doi: 10.1023/A:1020631114063
Michielse, C. B., Rep, M. (2009). Pathogen profile update: Fusarium oxysporum. Mol. Plant Pathol. 10, 311–324. doi: 10.1111/j.1364-3703.2009.00538.x
Mitsuboshi, M., Kioka, Y., Noguchi, K., Asakawa, S. (2018). Evaluation of suppressiveness of soils exhibiting soil-borne disease suppression after long-term application of organic amendments by the co-cultivation method of pathogenic Fusarium oxysporum and indigenous soil microorganisms. Microbes Environ. 33, 58–65. doi: 10.1264/jsme2.ME17072
Montalba, R., Arriagada, C., Alvear, M., Zúñiga, G. E. (2010). Effects of conventional and organic nitrogen fertilizers on soil microbial activity, mycorrhizal colonization, leaf antioxidant content, and Fusarium wilt in highbush blueberry (Vaccinium corymbosum L.). Sci. Hortic. 125, 775–778. doi: 10.1016/j.scienta.2010.04.046
Moretti, A., Pascale, M., Logrieco, A. F. (2018). Mycotoxin risks under a climate change scenario in Europe. Trends Food Sci. Technol. 84, 38–40. doi: 10.1016/j.tifs.2018.03.008
Morimura, H., Ito, M., Yoshida, S., Koitabashi, M., Tsushima, S., Camagna, M., et al. (2020). In vitro assessment of biocontrol effects on Fusarium head blight and deoxynivalenol (DON) accumulation by DON-degrading bacteria. Toxins 12 (6), 399. doi: 10.3390/toxins12060399
Mousa, W. K., Raizada, M. N. (2016). Natural disease control in cereal grains. Encycl. Food Grains. 4, 257–263. doi: 10.1016/B978-0-12-394437-5.00206-0
Munkvold, G. P. (2017). “Fusarium species and their associated mycotoxins,” in Mycotoxigenic fungi. Eds. Moretti, A., Susca, A. (New York, NY: Humana Press), 51–106.
Munkvold, G. P., Proctor, R. H., Moretti, A. (2021). Mycotoxin production in Fusarium according to contemporary species concepts. Annu. Rev. Phytopathol. 59 373–402. doi: 10.1146/annurev-phyto-020620-102825
Murillo-Williams, A., Munkvold, G. P. (2008). Systemic infection by Fusarium verticillioides in maize plants grown under three temperature regimes. Plant Dis. 92, 1695–1700. doi: 10.1094/PDIS-92-12-1695
Nazari, L., Pattori, E., Manstretta, V., Terzi, V., Morcia, C., Somma, S., et al. (2018). Effect of temperature on growth, wheat head infection, and nivalenol production by Fusarium poae. Food Microbiol. 76, 83–90. doi: 10.1016/j.fm.2018.04.015
Nelson, P. E., Dignani, M. C., Anaissie, E. J. (1994). Taxonomy, biology, and clinical aspects of Fusarium species. Clin. Microbiol. Rev. 7, 479–504. doi: 10.1128/CMR.7.4.479
Nešić, K., Ivanović, S., Nešić, V. (2014). Fusarial toxins: secondary metabolites of Fusarium fungi. Rev. Environ. Contam. Toxicol. 228, 101–120. doi: 10.1007/978-3-319-01619-1_5
Nguvo, K. J., Gao, X. (2019). Weapons hidden underneath: biocontrol agents and their potentials to activate plant induced systemic resistance in controlling crop Fusarium diseases. J. Plant Dis. Prot. 126, 177–190. doi: 10.1007/s41348-019-00222-y
Nguyen, P.-A., Strub, C., Durand, N., Alter, P., Fontana, A., Schorr-Galindo, S. (2018). Biocontrol of Fusarium verticillioides using organic amendments and their actinomycete isolates. Biol. Control. 118, 55–66. doi: 10.1016/j.biocontrol.2017.12.006
Nisrina, L., Effendi, Y., Pancoro, A. (2021). Revealing the role of Plant Growth Promoting Rhizobacteria in suppressive soils against Fusarium oxysporum f.sp. cubense based on metagenomic analysis. Heliyon 7, e07636. doi: 10.1016/j.heliyon.2021.e07636
O’Donnell, K., Rooney, A. P., Proctor, R. H., Brown, D. W., McCormick, S. P., Ward, T. J., et al. (2013). Phylogenetic analyses of RPB1 and RPB2 support a middle Cretaceous origin for a clade comprising all agriculturally and medically important fusaria. Fungal Genet. Biol. 52, 20–31. doi: 10.1016/j.fgb.2012.12.004
O’Donnell, K., Ward, T. J., Robert, V. A. R. G., Crous, P. W., Geiser, D. M., Kang, S. (2015). DNA sequence-based identification of Fusarium: current status and future directions. Phytoparasitica 43, 583–595. doi: 10.1007/s12600-015-0484-z
Oren, L., Ezrati, S., Cohen, D., Sharon, A. (2003). Early Events in the Fusarium verticillioides - maize interaction characterized by using a green fluorescent protein-expressing transgenic isolate. Appl. Environ. Microbiol. 69, 1695–1701. doi: 10.1128/AEM.69.3.1695-1701.2003
Orr, R., Nelson, P. N. (2018). Impacts of soil abiotic attributes on Fusarium wilt, focusing on bananas. Appl. Soil Ecol. 132, 20–33. doi: 10.1016/j.apsoil.2018.06.019
Ossowicki, A., Tracanna, V., Petrus, M. L. C., van Wezel, G., Raaijmakers, J. M., Medema, M. H., et al. (2020). Microbial and volatile profiling of soils suppressive to Fusarium culmorum of wheat. Proc. Biol. Sci. 287, 20192527. doi: 10.1098/rspb.2019.2527
Pal, K. K., McSpadden Gardener, B. (2006). Biological control of plant pathogens. Plant Health Instruct. 2, 1117–1142. doi: 10.1094/PHI-A-2006-1117-02
Pal, K. K., Tilak, K. V. B. R., Saxena, A. K., Dey, R., Singh, C. S. (2001). Suppression of maize root diseases caused by Macrophomina phaseolina, Fusarium moniliforme and Fusarium graminearum by plant growth promoting rhizobacteria. Microbiol. Res. 156, 209–223. doi: 10.1078/0944-5013-00103
Palazzini, J. M., Ramirez, M. L., Torres, A. M., Chulze, S. N. (2007). Potential biocontrol agents for Fusarium head blight and deoxynivalenol production in wheat. Crop Prot. 26, 1702–1710. doi: 10.1016/j.cropro.2007.03.004
Palmieri, D., Segorbe, D., López-Berges, M. S., De Curtis, F., Lima, G., Di Pietro, A., et al. (2023). Alkaline pH, low iron availability, poor nitrogen sources and CWI MAPK signaling are associated with increased fusaric acid production in Fusarium oxysporum. Toxins 15 (1), 50. doi: 10.3390/toxins15010050
Pélissier, R., Violle, C., Morel, J.-B. (2021). Plant immunity: Good fences make good neighbors? Curr. Opin. Plant Biol. 62, 102045. doi: 10.1016/j.pbi.2021.102045
Pellan, L., Durand, N., Martinez, V., Fontana, A., Schorr-Galindo, S., Strub, C. (2020). Commercial biocontrol agents reveal contrasting comportments against two mycotoxigenic fungi in cereals: Fusarium graminearum and Fusarium verticillioides. Toxins 12, 152. doi: 10.3390/toxins12030152
Peng, H. X., Sivasithamparam, K., Turner, D. W. (1999). Chlamydospore germination and Fusarium wilt of banana plantlets in suppressive and conducive soils are affected by physical and chemical factors. Soil Biol. Biochem. 31, 1363–1374. doi: 10.1016/S0038-0717(99)00045-0
Pereyra, S. A., Dill-Macky, R., Sims, A. L. (2004). Survival and inoculum production of Gibberella zeae in wheat residue. Plant Dis. 88, 724–730. doi: 10.1094/PDIS.2004.88.7.724
Petti, C., Khan, M., Doohan, F. (2010). Lipid transfer proteins and protease inhibitors as key factors in the priming of barley responses to Fusarium head blight disease by a biocontrol strain of Pseudomonas fluorescens. Funct. Integr. Genomics 10, 619–627. doi: 10.1007/s10142-010-0177-0
Pfordt, A., Ramos Romero, L., Schiwek, S., Karlovsky, P., von Tiedemann, A. (2020). Impact of environmental conditions and agronomic practices on the prevalence of Fusarium species associated with ear-and stalk rot in maize. Pathogens 9, 236. doi: 10.3390/pathogens9030236
Pieterse, C. M. J., Zamioudis, C., Berendsen, R. L., Weller, D. M., Van Wees, S. C. M., Bakker, P. A. H. M. (2014). Induced systemic resistance by beneficial microbes. Annu. Rev. Phytopathol. 52, 347–375. doi: 10.1146/annurev-phyto-082712-102340
Pisi, A., Cesari, A., Zakrisson, E., Filippini, G., Roberti, R., Mantovani, W. (2001). SEM investigation about hyphal relationships between some antagonistic fungi against Fusarium spp. foot rot pathogen of wheat. Phytopathol. Mediterr. 40, 37–44. doi: 10.14601/PHYTOPATHOL_MEDITERR-1588
Podolska, G., Bryła, M., Sułek, A., Waśkiewicz, A., Szymczyk, K., Jędrzejczak, R. (2017). Influence of the cultivar and nitrogen fertilisation level on the mycotoxin contamination in winter wheat. Qual. Assur. Saf. Crops Foods. 9, 451–461. doi: 10.3920/QAS2016.1064
Qualhato, T. F., Lopes, F. A. C., Steindorff, A. S., Brandão, R. S., Jesuino, R. S. A., Ulhoa, C. J. (2013). Mycoparasitism studies of Trichoderma species against three phytopathogenic fungi: evaluation of antagonism and hydrolytic enzyme production. Biotechnol. Lett. 35, 1461–1468. doi: 10.1007/s10529-013-1225-3
Raaijmakers, J., Leeman, M., van Oorschot, M., van der Sluis, I., Schippers, B., Bakker, P. (1995). Dose-response relationships in biological control of Fusarium wilt of radish by Pseudomonas spp. Phytopathology 85, 1075–1081. doi: 10.1094/Phyto-85-1075
Raaijmakers, J. M., Paulitz, T. C., Steinberg, C., Alabouvette, C., Moënne-Loccoz, Y. (2009). The rhizosphere: a playground and battlefield for soilborne pathogens and beneficial microorganisms. Plant Soil. 321, 341–361. doi: 10.1007/s11104-008-9568-6
Rana, A., Sahgal, M., Johri, B. N. (2017). “Fusarium oxysporum: genomics, diversity and plant–host interaction,” in Developments in Fungal Biology and Applied Mycology. Eds. Satyanarayana, T., Deshmukh, S., Johri, B. (Singapore: Springer International Publishing), 159–199. doi: 10.1007/978-981-10-4768-8_10
Ranzi, C., Camera, J. N., Deuner, C. C. (2017). Influence of continuous cropping on corn and soybean pathogens. Summa Phytopathol. 43, 14–19. doi: 10.1590/0100-5405/2150
Rasmussen, P. H., Knudsen, I. M. B., Elmholt, S., Jensen, D. F. (2002). Relationship between soil cellulolytic activity and suppression of seedling blight of barley in arable soils. Appl. Soil Ecol. 19, 91–96. doi: 10.1016/S0929-1393(01)00177-9
Raza, W., Yuan, J., Ling, N., Huang, Q., Shen, Q. (2015). Production of volatile organic compounds by an antagonistic strain Paenibacillus polymyxa WR-2 in the presence of root exudates and organic fertilizer and their antifungal activity against Fusarium oxysporum f. sp. niveum. Biol. Control. 80, 89–95. doi: 10.1016/j.biocontrol.2014.09.004
Redkar, A., Gimenez Ibanez, S., Sabale, M., Zechmann, B., Solano, R., Di Pietro, A. (2022a). Marchantia polymorpha model reveals conserved infection mechanisms in the vascular wilt fungal pathogen Fusarium oxysporum. New Phytol. 234, 227–241. doi: 10.1111/nph.17909
Redkar, A., Sabale, M., Zuccaro, A., Di Pietro, A. (2022b). Determinants of endophytic and pathogenic lifestyle in root colonizing fungi. Curr. Opin. Plant Biol. 67, 102226. doi: 10.1016/j.pbi.2022.102226
Reino, J. L., Guerrero, R. F., Hernández-Galán, R., Collado, I. G. (2008). Secondary metabolites from species of the biocontrol agent Trichoderma. Phytochem. Rev. 7, 89–123. doi: 10.1007/s11101-006-9032-2
Reis, T. A., Oliveira, T. D., Zorzete, P., Faria, P., Corrêa, B. (2020). A non-toxigenic Aspergillus flavus strain prevents the spreading of Fusarium verticillioides and fumonisins in maize. Toxicon 181, 6–8. doi: 10.1016/j.toxicon.2020.04.091
Reyes Gaige, A., Todd, T., Stack, J. P. (2020). Interspecific competition for colonization of maize plants between Fusarium proliferatum and Fusarium verticillioides. Plant Dis. 104, 2102–2110. doi: 10.1094/PDIS-09-19-1964-RE
Ristić, D. (2012). Characterization of Fusarium species pathogen for sorghum [Sorghum bicolor (L.) Moench] in Serbia and genotype susceptibility. [dissertation] (Belgrade: University of Belgrade, Faculty of Agriculture). doi: 10.2298/BG20121008RISTIC
Rizaludin, M. S., Stopnisek, N., Raaijmakers, J. M., Garbeva, P. (2021). The chemistry of stress: Understanding the ‘cry for help’ of plant roots. Metabolites 11, 357. doi: 10.3390/metabo11060357
Rolfe, S. A., Griffiths, J., Ton, J. (2019). Crying out for help with root exudates: adaptive mechanisms by which stressed plants assemble health-promoting soil microbiomes. Curr. Opin. Microbiol. 49, 73–82. doi: 10.1016/j.mib.2019.10.003
Rosenzweig, N., Tiedje, J. M., Quensen, J. F., Meng, Q., Hao, J. J. (2012). Microbial communities associated with potato common scab-suppressive soil determined by pyrosequencing analyses. Plant Dis. 96, 718–725. doi: 10.1094/PDIS-07-11-0571
Rouxel, F., Sedra, H. (1989). Resistance des sols aux maladies. Mise en evidence de la resistance d’un sol de la palmeraie de Marrakech aux fusarioses vasculaires. Al Awamia. 66, 35–54.
Sajeena, A., Nair, D. S., Sreepavan, K. (2020). Non-pathogenic Fusarium oxysporum as a biocontrol agent. Indian Phytopathol. 73, 177–183. doi: 10.1007/s42360-020-00226-x
Sánchez-Cañizares, C., Jorrín, B., Poole, P. S., Tkacz, A. (2017). Understanding the holobiont: the interdependence of plants and their microbiome. Curr. Opin. Microbiol. 38, 188–196. doi: 10.1016/j.mib.2017.07.001
Sanguin, H., Sarniguet, A., Gazengel, K., Moënne-Loccoz, Y., Grundmann, G. L. (2009). Rhizosphere bacterial communities associated with disease suppressiveness stages of take-all decline in wheat monoculture. New Phytol. 184, 694–707. doi: 10.1111/j.1469-8137.2009.03010.x
Saravanakumar, K., Li, Y., Yu, C., Wang, Q.-Q., Wang, M., Sun, J., et al. (2017). Effect of Trichoderma harzianum on maize rhizosphere microbiome and biocontrol of Fusarium stalk rot. Sci. Rep. 7, 1771. doi: 10.1038/s41598-017-01680-w
Sautour, M., Edel-Hermann, V., Steinberg, C., Sixt, N., Laurent, J., Dalle, F., et al. (2012). Fusarium species recovered from the water distribution system of a French university hospital. Int. J. Hyg. Environ. Health 215, 286–292. doi: 10.1016/j.ijheh.2011.11.003
Scher, F. M., Baker, R. (1980). Mechanism of biological control in a Fusarium-suppressive soil. Phytopathology 70, 412–417. doi: 10.1094/Phyto-70-412
Schlatter, D., Kinkel, L., Thomashow, L., Weller, D., Paulitz, T. (2017). Disease suppressive soils: New insights from the soil microbiome. Phytopathology 107, 1284–1297. doi: 10.1094/PHYTO-03-17-0111-RVW
Schmale, D. G., Bergstrom, G. C. (2003). Fusarium head blight in wheat. Plant Health Instr. doi: 10.1094/PHI-I-2003-0612-01
Schreiner, K., Hagn, A., Kyselková, M., Moënne-Loccoz, Y., Welzl, G., Munch, J. C., et al. (2010). Comparison of barley succession and take-all disease as environmental factors shaping the rhizobacterial community during take-all decline. Appl. Environ. Microbiol. 76, 4703–4712. doi: 10.1128/AEM.00481-10
Scott, J. C., Gordon, T. R., Shaw, D. V., Koike, S. T. (2009). Effect of temperature on severity of Fusarium wilt of lettuce caused by Fusarium oxysporum f. sp. lactucae. Plant Dis. 94, 13–17. doi: 10.1094/PDIS-94-1-0013
Scott, P., Strange, R., Korsten, L., Gullino, M. L. (2021). Plant Diseases and Food Security in the 21st Century (The Netherlands: Springer International Publishing).
Segarra, G., Casanova, E., Avilés, M., Trillas, I. (2010). Trichoderma asperellum strain T34 controls Fusarium wilt disease in tomato plants in soilless culture through competition for iron. Microb. Ecol. 59, 141–149. doi: 10.1007/s00248-009-9545-5
Senatore, M. T., Ward, T. J., Cappelletti, E., Beccari, G., McCormick, S. P., Busman, M., et al. (2021). Species diversity and mycotoxin production by members of the Fusarium tricinctum species complex associated with Fusarium head blight of wheat and barley in Italy. J. Food Microbiol. 358, 109298. doi: 10.1016/j.ijfoodmicro.2021.109298
Senechkin, I. V., van Overbeek, L. S., van Bruggen, A. H. C. (2014). Greater Fusarium wilt suppression after complex than after simple organic amendments as affected by soil pH, total carbon and ammonia-oxidizing bacteria. Appl. Soil Ecol. 73, 148–155. doi: 10.1016/j.apsoil.2013.09.003
Shen, Z., Ruan, Y., Wang, B., Zhong, S., Su, L., Li, R., et al. (2015a). Effect of biofertilizer for suppressing Fusarium wilt disease of banana as well as enhancing microbial and chemical properties of soil under greenhouse trial. Appl. Soil Ecol. 93, 111–119. doi: 10.1016/j.apsoil.2015.04.013
Shen, Z., Ruan, Y., Xue, C., Zhong, S., Li, R., Shen, Q. (2015b). Soils naturally suppressive to banana Fusarium wilt disease harbor unique bacterial communities. Plant Soil. 393, 21–33. doi: 10.1007/s11104-015-2474-9
Shen, Z., Thomashow, L. S., Ou, Y., Tao, C., Wang, J., Xiong, W., et al. (2022). Shared core microbiome and functionality of key taxa suppressive to banana Fusarium wilt. Research 2022, 9818073. doi: 10.34133/2022/9818073
Shi, C., Yan, P., Li, J., Wu, H., Li, Q., Guan, S. (2014). Biocontrol of Fusarium graminearum growth and deoxynivalenol production in wheat kernels with bacterial antagonists. Int. J. Environ. Res. Public. Health 11, 1094–1105. doi: 10.3390/ijerph110101094
Shipton, P. J., Cook, R. J., Sitton, J. W. (1973). Occurrence and transfer of a biological factor in soil that suppresses take-all of wheat in Eastern Washington. Phytopathology 63, 511–517. doi: 10.1094/Phyto-63-511
Siegel-Hertz, K., Edel-Hermann, V., Chapelle, E., Terrat, S., Raaijmakers, J. M., Steinberg, C. (2018). Comparative microbiome analysis of a Fusarium wilt suppressive soil and a Fusarium wilt conducive soil from the Châteaurenard region. Front. Microbiol. 9, 568. doi: 10.3389/fmicb.2018.00568
Singh, R. K., Singh, P., Guo, D.-J., Sharma, A., Li, D.-P., Li, X., et al. (2021). Root-derived endophytic diazotrophic bacteria Pantoea cypripedii AF1 and Kosakonia arachidis EF1 promote nitrogen assimilation and growth in sugarcane. Front. Microbiol. 12. doi: 10.3389/fmicb.2021.774707
Šišić, A., Baćanović-Šišić, J., Al-Hatmi, A. M. S., Karlovsky, P., Ahmed, S. A., Maier, W., et al. (2018). The “forma specialis” issue in Fusarium: A case study in Fusarium solani f. sp. pisi. Sci. Rep. 8, 1252. doi: 10.1038/s41598-018-19779-z
Smith, S. N. (2007). An overview of ecological and habitat aspects in the genus Fusarium with special emphasis on the soil-borne pathogenic forms. Plant Pathol. Bull. 16, 97–120.
Smith, S. N., Snyder, W. C. (1971). Relationship of inoculum density and soil types to severity of Fusarium wilt of sweet potato. Phytopathology 61, 1049–1051. doi: 10.1094/Phyto-61-1049
Sneh, B., Dupler, M., Elad, Y., Baker, R. (1984). Chlamydospore germination of Fusarium oxysporum f. sp. cucumerinum as affected by fluorescent and lytic bacteria from a Fusarium-suppressive soil. Phytopathology 74 (9), 1115–1124. doi: 10.1094/PHYTO-74-1115
Spiegel, Y., Netzer, D., Kafkafi, U. (1987). The role of calcium nutrition on Fusarium-wilt syndrome in muskmelon. J. Phytopathol. 118, 220–226. doi: 10.1111/j.1439-0434.1987.tb00451.x
Steinkellner, S., Langer, I. (2004). Impact of tillage on the incidence of Fusarium spp. in soil. Plant Soil. 267, 13–22. doi: 10.1007/s11104-005-2574-z
Stotzky, G., Torrence Martin, R. (1963). Soil mineralogy in relation to the spread of Fusarium wilt of banana in Central America. Plant Soil. 18, 317–337. doi: 10.1007/BF01347232
Stover, R. H. (1956). Studies on Fusarium wilt of bananas: I. The behavior of F. oxysporum f. cubense in different soils. Can. J. Bot. 34, 927–942. doi: 10.1139/b56-073
Sturz, A. V., Carter, M. R. (1995). Conservation tillage systems, fungal complexes and disease development in soybean and barley rhizospheres in Prince Edward Island. Soil Tillage Res. 34, 225–238. doi: 10.1016/0167-1987(95)00469-9
Stutz, E. W., Défago, G., Kern, H. (1986). Naturally occurring fluorescent pseudomonads involved in suppression of black root rot of tobacco. Phytopathology 76, 181–185. doi: 10.1094/Phyto-76-181
Summerell, B. A. (2019). Resolving Fusarium: Current status of the genus. Annu. Rev. Phytopathol. 57, 323–339. doi: 10.1146/annurev-phyto-082718-100204
Sundaramoorthy, S., Raguchander, T., Ragupathi, N., Samiyappan, R. (2012). Combinatorial effect of endophytic and plant growth promoting rhizobacteria against wilt disease of Capsicum annum L. caused by Fusarium solani. Biol. Control. 60, 59–67. doi: 10.1016/j.biocontrol.2011.10.002
Szczech, M. M. (1999). Suppressiveness of vermicompost against Fusarium wilt of tomato. J. Phytopathol. 147, 155–161. doi: 10.1046/j.1439-0434.1999.147003155.x
Tamietti, G., Alabouvette, C. (1986). Résistance des sols aux maladies : XIII - Rôle des Fusarium oxysporum non pathogènes dans les mécanismes de résistance d’un sol de Noirmoutier aux fusarioses vasculaires. Agronomie 6, 541–548. doi: 10.1051/agro:19860606
Tamietti, G., Ferraris, L., Matta, A., Abbattista Gentile, I. (1993). Physiological responses of tomato plants grown in Fusarium suppressive soil. J. Phytopathol. 138, 66–76. doi: 10.1111/j.1439-0434.1993.tb01361.x
Tamietti, G., Matta, A. (1984). “Fusarium lycopersici-suppressive properties in the soil of Albenga (Italy): preliminary observations,” in Proc. 6th Congr. Union Phytopathol, Mediterr, Cairo. 129–131.
Tamietti, G., Pramotton, R. (1990). La réceptivité des sols aux fusarioses vasculaires : rapports entre résistance et microflore autochtone avec référence particulière aux Fusarium non pathogènes. Agronomie 10, 69–76. doi: 10.1051/agro:19900109
Tang, T., Sun, X., Liu, Q., Dong, Y., Zha, M. (2023). Treatment with organic manure inoculated with a biocontrol agent induces soil bacterial communities to inhibit tomato Fusarium wilt disease. Front. Microbiol. 13, 1006878. doi: 10.3389/fmicb.2022.1006878
Tao, C., Li, R., Xiong, W., Shen, Z., Liu, S., Wang, B., et al. (2020). Bio-organic fertilizers stimulate indigenous soil Pseudomonas populations to enhance plant disease suppression. Microbiome 8, 137. doi: 10.1186/s40168-020-00892-z
Tari, P. H., Anderson, A. J. (1988). Fusarium wilt suppression and agglutinability of Pseudomonas putida. Appl. Environ. Microbiol. 54, 2037–2041. doi: 10.1128/aem.54.8.2037-2041.1988
Termorshuizen, A. J., van Rijn, E., van der Gaag, D. J., Alabouvette, C., Chen, Y., Lagerlöf, J., et al. (2006). Suppressiveness of 18 composts against 7 pathosystems: Variability in pathogen response. Soil Biol. Biochem. 38, 2461–2477. doi: 10.1016/j.soilbio.2006.03.002
Theron, J. S., Johannes van Coller, G., Rose, L. J., Labuschagne, J., Swanepoel, P. A. (2023). The effect of crop rotation and tillage practice on Fusarium crown rot and agronomic parameters of wheat in South Africa. Crop Prot. 166, 106175. doi: 10.1016/j.cropro.2022.106175
Thrane, U. (2014). “Fusarium,” in Encyclopedia of Food Microbiology. Eds. Batt, C. A., Tortorello, M. L. (Amsterdam, The Netherlands: Elsevier), 76–81. doi: 10.1016/B978-0-12-384730-0.00141-5
Tian, Y., Tan, Y., Yan, Z., Liao, Y., Chen, J., de Boevre, M., et al. (2018). Antagonistic and detoxification potentials of Trichoderma isolates for control of zearalenone (ZEN) producing Fusarium graminearum. Front. Microbiol. 8. doi: 10.3389/fmicb.2017.02710
Tkacz, A., Cheema, J., Chandra, G., Grant, A., Poole, P. S. (2015). Stability and succession of the rhizosphere microbiota depends upon plant type and soil composition. ISME J. 9, 2349–2359. doi: 10.1038/ismej.2015.41
Tkacz, A., Poole, P. S. (2015). Role of root microbiota in plant productivity. J. Exp. Bot. 66, 2167–2175. doi: 10.1093/jxb/erv157
Torbati, M., Arzanlou, M., da Silva Santos, A. C. (2021). Fungicolous Fusarium species: Ecology, diversity, isolation, and identification. Curr. Microbiol. 78, 2850–2859. doi: 10.1007/s00284-021-02584-9
Tyc, O., Zweers, H., de Boer, W., Garbeva, P. (2015). Volatiles in inter-specific bacterial interactions. Front. Microbiol. 6, 1412. doi: 10.3389/fmicb.2015.01412
Vandenkoornhuyse, P., Quaiser, A., Duhamel, M., Le Van, A., Dufresne, A. (2015). The importance of the microbiome of the plant holobiont. New Phytol. 206, 1196–1206. doi: 10.1111/nph.13312
Van Peer, R., Niemann, G. J., Schippers, B. (1991). Induced resistance and phytoalexin accumulation in biological control of Fusarium wilt of carnation by Pseudomonas sp. strain WCS417r. Phytopathology 81, 728–734. doi: 10.1094/Phyto-81-728
Vasudeva, R. S., Roy, T. C. (1950). The effect of associated soil microflora on Fusarium udum Butl., the fungus causing wilt of pigeon-pea (Cajanus cajan (L.) Millsp.). Ann. Appl. Biol. 37, 169–178. doi: 10.1111/j.1744-7348.1950.tb01037.x
Veliz, E. A., Martínez-Hidalgo, P., Hirsch, A. M. (2017). Chitinase-producing bacteria and their role in biocontrol. AIMS Microbiol. 3, 689. doi: 10.3934/microbiol.2017.3.689
Viterbo, A., Ramot, O., Chernin, L., Chet, I. (2002). Significance of lytic enzymes from Trichoderma spp. in the biocontrol of fungal plant pathogens. Antonie Van Leeuwenhoek. 81, 549–556. doi: 10.1023/a:1020553421740
Voigt, K. (2002). “Management of Fusarium diseases,” in Agricultural Applications. Ed. Kempken, F. (Berlin, Heidelberg: Springer Berlin Heidelberg), 217–242. doi: 10.1007/978-3-662-03059-2_12
Vujanović, V., Goh, Y. K. (2009). Sphaerodes mycoparasitica sp. nov., a new biotrophic mycoparasite on Fusarium avenaceum, F. graminearum and F. oxysporum. Mycol. Res. 113, 1172–1180. doi: 10.1016/j.mycres.2009.07.018
Wagacha, J. M., Muthomi, J. W. (2007). Fusarium culmorum: Infection process, mechanisms of mycotoxin production and their role in pathogenesis in wheat. Crop Prot. 26, 877–885. doi: 10.1016/j.cropro.2006.09.003
Wagner, T. A., Duke, S. E., Davie, S. M., Magill, C., Liu, J. (2022). Interaction of Fusarium wilt race 4 with root-knot nematode increases disease severity in cotton. Plant Dis. 106, 2558–2562. doi: 10.1094/PDIS-12-21-2725-SC
Walters, D. R., Ratsep, J., Havis, N. D. (2013). Controlling crop diseases using induced resistance: challenges for the future. J. Exp. Bot. 64, 1263–1280. doi: 10.1093/jxb/ert026
Wang, X., Du, Z., Chen, C., Guo, S., Mao, Q., Wu, W., et al. (2023). Antifungal effects and biocontrol potential of lipopeptide-producing Streptomyces against banana Fusarium wilt fungus Fusarium oxysporum f. sp. cubense. Front. Microbiol. 14, 1177393. doi: 10.3389/fmicb.2023.1177393
Wang, T., Hao, Y., Zhu, M., Yu, S., Ran, W., Xue, C., et al. (2019). Characterizing differences in microbial community composition and function between Fusarium wilt diseased and healthy soils under watermelon cultivation. Plant Soil 438, 421–433. doi: 10.1007/s11104-019-04037-6
Wang, H., Li, X., Li, X., Wang, J., Li, X., Guo, Q., et al. (2020). Long-term no-tillage and different residue amounts alter soil microbial community composition and increase the risk of maize root rot in Northeast China. Soil Tillage Res. 196, 104452. doi: 10.1016/j.still.2019.104452
Wang, B., Li, R., Ruan, Y., Ou, Y., Zhao, Y., Shen, Q. (2015). Pineapple–banana rotation reduced the amount of Fusarium oxysporum more than maize–banana rotation mainly through modulating fungal communities. Soil Biol. Biochem. 86, 77–86. doi: 10.1016/j.soilbio.2015.02.021
Wang, J.-T., Zheng, Y.-M., Hu, H.-W., Li, J., Zhang, L.-M., Chen, B.-D., et al. (2016). Coupling of soil prokaryotic diversity and plant diversity across latitudinal forest ecosystems. Sci. Rep. 6 (1), 19561. doi: 10.1038/srep19561
Weber, R., Hrynczuk, B., Runowska-Hrynczuk, B., Kita, W. (2001). Influence of the mode of tillage on diseases of culm base in some winter wheat varieties, oats and spring wheat. J. Phytopathol. 149, 185–188. doi: 10.1046/j.1439-0434.2001.00592.x
Weber, R., Kita, W. (2010). Effects of tillage system and forecrop type on frequency of Fusarium culmorum and F. avenaceum occurrence on culm base of some winter wheat (Triticum aestivum L.) cultivars. Acta Agrobot. 63, 121–128. doi: 10.5586/aa.2010.014
Weller, D. M., Landa, B. B., Mavrodi, O. V., Schroeder, K. L., de la Fuente, L., Blouin Bankhead, S., et al. (2007). Role of 2,4-diacetylphloroglucinol-producing fluorescent Pseudomonas spp. in the defense of plant roots. Plant Biol. Stuttg. Ger. 9, 4–20. doi: 10.1055/s-2006-924473
Weller, D. M., Raaijmakers, J. M., McSpadden Gardener, B. B., Thomashow, L. S. (2002). Microbial populations responsible for specific soil suppressiveness to plant pathogens. Annu. Rev. Phytopathol. 40, 309–348. doi: 10.1146/annurev.phyto.40.030402.110010
Willocquet, L., Meza, W. R., Dumont, B., Klocke, B., Feike, T., Kersebaum, K. C., et al. (2021). An outlook on wheat health in Europe from a network of field experiments. Crop Prot. 139, 105335. doi: 10.1016/j.cropro.2020.105335
Winter, M., de Mol, F., von Tiedemann, A. (2014). Cropping systems with maize and oilseed rape for energy production may reduce the risk of stem base diseases in wheat. Field Crops Res. 156, 249–257. doi: 10.1016/j.fcr.2013.10.009
Xia, J. W., Sandoval-Denis, M., Crous, P. W., Zhang, X. G., Lombard, L. (2019). Numbers to names-restyling the Fusarium incarnatum-equiseti species complex. Persoonia 43, 186–221. doi: 10.3767/persoonia.2019.43.05
Xiong, W., Guo, S., Jousset, A., Zhao, Q., Wu, H., Li, R., et al. (2017). Bio-fertilizer application induces soil suppressiveness against Fusarium wilt disease by reshaping the soil microbiome. Soil Biol. Biochem. 114, 238–247. doi: 10.1016/j.soilbio.2017.07.016
Xu, X. (2003). Effects of environmental conditions on the development of Fusarium Ear Blight. Eur. J. Plant Pathol. 109, 683–689. doi: 10.1023/A:1026022223359
Xu, F., Liu, W., Song, Y., Zhou, Y., Xu, X., Yang, G., et al. (2021). The distribution of Fusarium graminearum and Fusarium asiaticum causing Fusarium head blight of wheat in relation to climate and cropping system. Plant Dis. 105, 2830–2835. doi: 10.1094/PDIS-01-21-0013-RE
Xu, X.-M., Monger, W., Ritieni, A., Nicholson, P. (2007). Effect of temperature and duration of wetness during initial infection periods on disease development, fungal biomass and mycotoxin concentrations on wheat inoculated with single, or combinations of Fusarium species. Plant Pathol. 56, 943–956. doi: 10.1111/j.1365-3059.2007.01650.x
Xu, Z., Wang, M., Du, J., Huang, T., Liu, J., Dong, T., et al. (2020). Isolation of Burkholderia sp. HQB-1, a promising biocontrol bacteria to protect banana against Fusarium wilt through phenazine-1-carboxylic acid secretion. Front. Microbiol. 11, 605152. doi: 10.3389/fmicb.2020.605152
Yadav, K., Damodaran, T., Dutt, K., Singh, A., Muthukumar, M., Rajan, S., et al. (2021). Effective biocontrol of banana Fusarium wilt tropical race 4 by a Bacillus rhizobacteria strain with antagonistic secondary metabolites. Rhizosphere 18, 100341. doi: 10.1016/j.rhisph.2021.100341
Ye, X., Li, Z., Luo, X., Wang, W., Li, Y., Li, R., et al. (2020). A predatory myxobacterium controls cucumber Fusarium wilt by regulating the soil microbial community. Microbiome 8 (49), 1–17. doi: 10.1186/s40168-020-00824-x
Yilmaz, N., Sandoval-Denis, M., Lombard, L., Visagie, C. M., Wingfield, B. D., Crous, P. W. (2021). Redefining species limits in the Fusarium fujikuroi species complex. Persoonia 46, 129–162. doi: 10.3767/persoonia.2021.46.05
Yuan, J., Wen, T., Zhang, H., Zhao, M., Penton, C. R., Thomashow, L. S., et al. (2020). Predicting disease occurrence with high accuracy based on soil macroecological patterns of Fusarium wilt. ISME J. 14 (12), 2936–2950. doi: 10.1038/s41396-020-0720-5
Zaim, S., Belabid, L., Bayaa, B., Bekkar, A. A. (2016). “Biological control of chickpea Fusarium wilts using rhizobacteria PGPR,” in Microbial-mediated Induced Systemic Resistance in Plants. Eds. Choudhary, D., Varma, A. (Singapore: Springer Nature), 147–162.
Zalila-Kolsi, I., Ben Mahmoud, A., Ali, H., Sellami, S., Nasfi, Z., Tounsi, S., et al. (2016). Antagonist effects of Bacillus spp. strains against Fusarium graminearum for protection of durum wheat (Triticum turgidum L. subsp. durum). Microbiol. Res. 192, 148–158. doi: 10.1016/j.micres.2016.06.012
Zhao, S., Du, C.-M., Tian, C.-Y. (2012). Suppression of Fusarium oxysporum and induced resistance of plants involved in the biocontrol of cucumber Fusarium wilt by Streptomyces bikiniensis HD-087. World J. Microbiol. Biotechnol. 28, 2919–2927. doi: 10.1007/s11274-012-1102-6
Zhao, Y., Selvaraj, J., Xing, F., Zhou, L., Wang, Y., Song, H., et al. (2014). Antagonistic action of Bacillus subtilis strain SG6 on Fusarium graminearum. PloS One 9, e92486. doi: 10.1371/journal.pone.0092486
Zhou, D., Jing, T., Chen, Y., Wang, F., Qi, D., Feng, R., et al. (2019). Deciphering microbial diversity associated with Fusarium wilt-diseased and disease-free banana rhizosphere soil. BMC Microbiol. 19, 1–13. doi: 10.1186/s12866-019-1531-6
Keywords: deoxynivalenol, nivalenol, zearalenone, Fusarium head blight, induced systemic resistance, lipopolysaccharides
Citation: Todorović I, Moënne-Loccoz Y, Raičević V, Jovičić-Petrović J and Muller D (2023) Microbial diversity in soils suppressive to Fusarium diseases. Front. Plant Sci. 14:1228749. doi: 10.3389/fpls.2023.1228749
Received: 25 May 2023; Accepted: 10 November 2023;
Published: 04 December 2023.
Edited by:
Inmaculada Larena, Instituto Nacional de Investigación y Tecnología Agraria y Alimentaria (INIA-CSIC), SpainReviewed by:
Belen Guijarro, Instituto Nacional de Investigación y Tecnología Agroalimentaria (INIA), SpainCarmen Gómez-Lama Cabanás, Spanish National Research Council (CSIC), Spain
Copyright © 2023 Todorović, Moënne-Loccoz, Raičević, Jovičić-Petrović and Muller. This is an open-access article distributed under the terms of the Creative Commons Attribution License (CC BY). The use, distribution or reproduction in other forums is permitted, provided the original author(s) and the copyright owner(s) are credited and that the original publication in this journal is cited, in accordance with accepted academic practice. No use, distribution or reproduction is permitted which does not comply with these terms.
*Correspondence: Daniel Muller, daniel.muller@univ-lyon1.fr
†ORCID: Irena Todorović, orcid.org/0000-0001-9119-8398
Yvan Moënne-Loccoz, orcid.org/0000-0002-9817-1953
Vera Raičević, orcid.org/0000-0001-9046-2951
Jelena Jovičić-Petrović, orcid.org/0000-0002-6458-8312
Daniel Muller, orcid.org/0000-0002-6619-4691