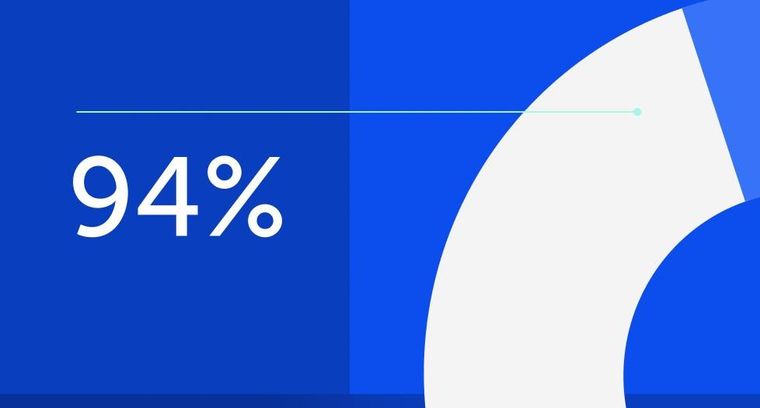
94% of researchers rate our articles as excellent or good
Learn more about the work of our research integrity team to safeguard the quality of each article we publish.
Find out more
ORIGINAL RESEARCH article
Front. Plant Sci., 21 July 2023
Sec. Plant Abiotic Stress
Volume 14 - 2023 | https://doi.org/10.3389/fpls.2023.1224334
This article is part of the Research TopicThe Impact of Abiotic Stresses on Agriculture: Mitigation through Climate Smart StrategiesView all 13 articles
A field experiment with wheat was conducted at Pusa (25.98°N, 85.67°E, 52 m amsl), Bihar (middle Gangetic plains of India), to assess the responses of phenology, yield attributes, and yield to growing season temperature and heat stress. For this purpose, wheat was planted on five dates (viz., 15 November, 25 November, 5 December, 15 December, and 25 December) for three consecutive years (viz., 2014–2015, 2015–2016, and 2016–2017) with three prominent cultivars of the region (viz., RAU-3711, HD-2824, and HD-2733). Five dates of sowing represent different wheat-growing micro-environments as imposed by varying sowing dates encompassing the entire sowing window. The study observed the significant effect of sowing dates on phenophase duration. In general, with progress in the date of sowing, tiller initiation was delayed, while the reverse trend was observed for later growth phases. Sowing environments significantly influenced the number of effective tillers m−2. Average numbers of effective tillers (ET) m−2 for the wheat sown during 15–25 November were almost 11.6% higher than those of the 25 December sown crop. Grain filling duration (GFD) showed a declining trend with the advancement of sowing dates due to increased thermal load on the crop during the reproductive period. 15 November planted crop exhibited the highest GFD (47 days), which was shortened significantly beyond 25 November, signifying agrometeorological non-suitability of wheat sowing beyond this window. Wheat sown on 25 November recorded the highest grain yield (3.21 Mg ha−1), 48.61% higher than the 25 December sown crop due to the congenial thermal regime. In this context, we have identified optimal and sub-optimal conditions to escape heat stress for higher wheat productivity. Moreover, the sum of deviation of temperature from optimum thresholds, computed for sensitive growth phases (50% flowering to physiological maturity), helped us to identify heat stress and its impact on wheat. Genotype-by-environment (GGE) biplot analysis revealed that RAU-3711 was found to be the most stable cultivar. A decrease in the yield of wheat by 4.9% to 12.0%, sown during November, and 33.8% to 42.4%, sown during December, is predicted in 2050-51 and 2080-81, respectively, under RCP 4.5.
Wheat is the most important staple food crop in the world. It also plays a vital role in food security for millions of people in India and occupies approximately 30.6 million hectares area with a total production of 98.5 million tonnes (FAO, 2017) contributing approximately 43% to the country’s granary (Bapuji Rao et al., 2015). India is the second largest producer of wheat after China with approximately 12% share in global wheat production. In recent years, rising temperature due to climate change has been a cause of concern for sustainable wheat production in India, more specifically over Indo-Gangetic Plains stretching a vast area from Punjab in the West and Bihar in the East. The concern is real regarding the negative effect of warming on the phenology and yield of crops (Xiao et al., 2012; Wang et al., 2013). Under such a situation, several adaptation measures, viz., wheat sowing by zero tillage, growing new heat-resistant cultivars, and changing planting schedules, are being taken up by policymakers and farmers. Wheat production in Indo-Gangetic Plains is vulnerable to short-term temperature extremes (Lobell et al., 2012). Prevalence of dry westerly wind and sudden rise of temperature during the fag end of the growing season tends to shorten the grain filling period (Garg et al., 2013; Sandhu et al., 2016; Vashisth et al., 2020) and negatively impact grain setting of wheat (Sattar and Srivastava, 2021). It significantly affects growth and photosynthetic efficiency (Wang et al., 2011) and consequently reduces biomass and productivity (Farooq et al., 2011). Grain number, which is determined from 30 days before flowering until shortly after flowering, and grain size, dependent on grain filling (Lobell et al., 2012), crop duration, and crop biomass, together tend to decide the final yield of the crop.
Phenology is an integral part of crop weather models, which is used to specify the appropriate time and rate of specific phasic development processes (Singh et al., 2001). In this context, studying crop phenology vis-à-vis thermal regime in the field and the integrated effect of weather on yield assumes significance to bring in proper resilience against the adverse impact of high temperature on crop growth. Varying dates of sowing expose the crop in a year to different temperatures during its growing period, which helps properly understand the response of phenology to ambient temperature (Vijaya et al., 2015). Xiao et al. (2013) showed that changes in the phenological phases of winter wheat are strongly related to temperature trends. Given the potential impacts of global warming on yield, the study of phenology assumes great importance due to its impacts on productivity and farming practices (Xiao et al., 2012; Wang et al., 2013). Temperature plays a great role in modifying the enzymatic functions of plants and causes a change in phenology, which is directly related to yield (Zhu et al., 2018).
Temperature-based agrometeorological indices, viz., growing degree day (GDD), helio-thermal unit (HTU), and photo-thermal units (PTU), have a direct relationship with the growth and yield of crops. Accordingly, these indices along with thermal efficiencies are important parts of understanding the responses of phenology and yield to growing season temperature. Heat stress manifested by the occurrence of significantly higher than normal temperatures for 15–25 days during the reproductive period of wheat in the rabi (winter) season of 2021–2022 caused a significant reduction of grain yield in India (Bal et al., 2022). Against the backdrop of the problem of terminal heat stress and variable wheat yield, we hypothesized that by optimizing sowing dates, wheat can be grown under a congenial thermal regime, thus offsetting the negative impacts.
Since temperature significantly influences wheat yield, it would be prudent to simulate future yield under a projected climatic scenario. Future wheat yield can be simulated by different crop simulation models. The CERES-Wheat model of Hoogenboom et al. (2019) is the most widely used crop growth model, and it is an effective tool to quantify the effects of cultivar, climate, soil, and management on wheat growth across the globe. It can be effectively used to simulate yield in the projected climate (Shen et al., 2022). Keeping in mind the above facts, an attempt has been made in this article to evaluate the responses of phenology and yield of wheat to growing season temperature and heat stress. A systematic study on this aspect for a region like the middle Gangetic Plains of eastern India appeared to be meager. In this context, the objectives of the investigation were set i) to quantify the impact of varying thermal regimes induced by varying sowing environments on crop phenology and yield ii) to optimize the exact sowing environment of wheat based on the response of phenology and yield to growing season thermal regime.
The field experiment was carried out at the University Farm of Dr. Rajendra Prasad Central Agricultural University, Pusa (25.98°N, 85.67°E, 52 m), Bihar, located in the middle Gangetic plains of India.
The region experiences a sub-humid subtropical monsoon climate. It has four major seasons, viz., summer (March–May), monsoon (June–September), post-monsoon (October–November), and winter (December–February). The average annual rainfall of the area is approximately 1,230 mm. Approximately 85% of rainfall occurs during the kharif (monsoon) season. May is the warmest summer month of the year with a daily maximum temperature of 37°C–41°C, while the coldest winter month is January with a daily minimum temperature of 5°C–8°C. December, January, and February are the main winter months in the region. Temperature decreases significantly from November, which becomes lowest in January. Increasing temperature from March onward heralds the commencement of the summer season. Locally, the period from 15 October to 15 March is called rabi season, in which important irrigated crops such as wheat, maize, potato, mustard, cauliflower, cabbage, and chickpea are grown.
The soil of the experimental field has sandy loam soil, which is the dominant soil textural class of the region. The physicochemical properties of the experimental soil are given in Table 1.
The experiment was conducted over three wheat growing seasons, viz., rabi (winter) seasons of 2014–2015, 2015–2016, and 2016–2017. The crop was planted on five dates every year (viz., 15 November (D1), 25 November (D2), 5 December (D3), 15 December (D4), and 25 December (D5)) with three prominent cultivars (viz., RAU-3711, HD-2824, and HD-2733) of the region in factorial randomized block design with three replications. The sowing was staggered to impose heat stress on the crop at critical growth phases. Recommended package of practices as followed by the farmers of the region was adopted. The crop was grown under irrigated conditions, and three irrigations were applied 21 days after sowing (DAS), 45 DAS, and 75 DAS. Nitrogen, phosphorus, and potassium at 120, 60, and 40 kg ha−1 were applied. All P and K and a half dose of N were applied at sowing as basal dose. The remaining half dose of N was top dressed in two equal splits at crown root initiation and boot stages. Hand weeding was carried out in the field to keep the field weed free. No infestation of insect pests was observed on the crop. Hence, no pesticide was applied during the period of the experiment. The occurrence of phenological events like tillering, booting, flowering, milk, dough, and physiological maturity in Julian Day was recorded from each plot, and average dates of these phases were calculated over the years and used for analysis. GDD, also known as heat unit (HU), was calculated at different phenological stages of the crop.
Growing degree-day is defined as the mean temperature above the base temperature. Mathematically, the GDD was computed by using the following equation (Nuttonson, 1955; Sastry and Chakravarty, 1982) at different phenological stages of the crop.
where GDD is the growing degree day (day °C), Tmax is the daily maximum temperature (°C), Tmin is the daily minimum temperature (°C), and Tb is the base temperature (°C); the base temperature was taken as 5°C (Nuttonson, 1955; Amrawat et al., 2013).
Helio-thermal unit was calculated by multiplying GDD and daily bright sunshine hours (BSS). BSS for a particular day as recorded by the sunshine recorder in the Agrometeorological Observatory was used in the estimation of HTU. The sum of HTU for the duration of each phenophase was calculated by using the following equation (Sastry and Chakravarty, 1982), and accumulated HTU at physiological maturity was calculated as follows:
where n is the actual duration of bright sunshine hours.
Accumulated PTUs at physiological maturity were calculated by multiplying GDD with the length of maximum possible sunshine hours (Nuttonson, 1955; Sastry and Chakravarty, 1982). It is mathematically expressed as PTU = GDD × N, where N is the maximum possible sunshine hours.
Heat use efficiency (HUE), helio-thermal use efficiency (HTUE), and photo-thermal use efficiency (PTUE) were calculated following Singh and Khushu (2012).
Daily weather data on maximum and minimum temperatures for the growing season were collected from the nearby Agrometeorological Observatory, Dr. Rajendra Prasad Central Agricultural University, Pusa, Bihar.
Observations on yield attributes, viz., effective tillers per m−2, number of grains per spike, and test weight (1,000-grain), were taken from the net plot area. To determine the test weight of grains, 1,000 seeds from each plot were counted and dried until a constant weight was obtained. The crop was harvested manually with the help of a sickle from the net plot area. After the removal of excess moisture from grains of each plot, the grain yield and straw yield (kg per plot) were recorded after taking weight by open pan electronic balance, which was later converted to Mg ha−1. The harvest index (%) was calculated by dividing economic yield (grain) by the biological yield (grain + straw), as follows:
Grain filling duration (GFD) was calculated by counting the number of calendar days from 50% flowering to physiological maturity. The grain filling rate (GFR) was calculated by dividing the grain yield (kg ha−1) by GFD (days).
Pearson’s correlation coefficients between grain yield, grain filling duration, and its rate, and yield attributes and weather parameters were computed (Gomez and Gomez, 1984). The sum of deviations of maximum and minimum temperatures from optimum thresholds was calculated at critical growth phases for each date of sowing, and based on the largest deviations, heat stress was identified for the crop sown on different sowing dates. In this study, the sum of deviation from optimum thresholds of maximum temperature and minimum temperature for the sensitive growth phases, viz., flowering to milking and flowering to maturity, was calculated by taking 25°C and 12°C as optimum threshold maximum and minimum temperature, respectively, for flowering to milking (F-Mlk) and 27°C and 14°C as threshold maximum and minimum temperatures for flowering to maturity (F-Mat) stages (Sattar et al., 2020 and Sattar and Srivastava, 2021).
The data on crop phenology, yield, and other parameters were subjected to appropriate statistical analysis through SPSS software (version 17.0), and the significance of mean values was compared using the least significant difference values (Gomez and Gomez, 1984).
Genotype-by-environment (GGE) biplot analysis was carried out using R software version 4.2.3 with the help of the GGE Biplots package to visualize and interpret the multi-environment data and performance of varieties (Saeidnia et al., 2023). Each sowing date was considered a test environment. Accordingly, five test environments were used for testing the varieties. Variety with higher yield and stability, and which-won-where pattern were visualized for studying the higher yield producing variety under different sowing environments.
We also used the CERES-Wheat model (DSSAT v. 4.75) to simulate wheat yields for the years 2050-51 and 2080-81 using the Intergovernmental Panel on Climate Change (IPCC)) AR5 scenario of Representative Concentration Pathway 4.5 (RCP 4.5). Soil data, crop management data, and weather data of the site were used as necessary inputs to run the CERES-Wheat model. For simulation purposes, the model required a set of genetic coefficients pertaining to the phenology and growth of wheat. The genetic coefficients of wheat cultivars, namely, RAU-3711, HD-2824, and HD-2733, were estimated by the genetic sub-model of the DSSAT with repeated interactions until a close match between simulated and observed parameters of phenology and yield was obtained (Table 2). With the use of RCP 4.5, the average projected yield based on these varieties for 2050-51 and 2080-81 is discussed in the article. The yield of wheat varieties grown during 2014–2015 was considered as a baseline for comparison with the projected yield.
The phenological development of a crop is the most important biological footprint of climate change and warming impact. The study of crop phenology has important implications to understand crop response and adaptation to climate change (Tao et al., 2022). In the present study, crop micro-environment as imposed by different dates of sowing significantly influenced thermal days required to achieve different phonological stages, viz., tiller initiation, booting, 50% flowering, milking, dough, and maturity (Tables 3, 4). It extended from 26 to 38, 61 to 79, 82 to 97, 81 to 104, 99 to 132, and 106 to 139 days to reach tiller initiation, booting, 50% flowering, milking, dough, and maturity, respectively, over the experimentation period (2014–2015 to 2016–2017). Many authors (Tao et al., 2012; Li et al., 2021; Tao et al., 2022) opined that the phenology of crops is intricately related to crop management, sowing dates, and cultivars. In the Indo-Gangetic Plains, forced maturity of wheat due to a sudden rise in ambient temperature is common during the post-heading phase of the crop (Ram et al., 2012). Hence, quantification of the exact duration of phenological stages in a particular crop-growing environment and their impact on the yield is very important (Amrawat et al., 2013). This is more pertinent concerning the effect of changing climate on crop phenology (Liu et al., 2018). In the present study, the crop planted on 15 November took 78, 91, 100, 130, and 138 days to achieve booting and 50% flowering, milking, dough, and maturity stages, respectively, which were significantly higher than the rest of the sowing dates. However, the highest days to reach tiller initiation (37) were associated with the crop planted on 25 December (D5), which was significantly higher than the rest of the sowing dates. Days to tiller initiation varied from 27 days in D2 to 37 days in D5 (Table 2). In general, with progress in the date of sowing, tiller initiation was delayed. However, a reverse trend was recorded for days before booting. Tillering stage of the crop sown on later dates encountered lower temperatures. There was a difference of 16 days to complete booting for D1 and D5. Similarly, days to 50% flowering showed a declining trend as that of the booting stage, and D1 took 91 days to attain 50% flowering, which was significantly higher than that of all other dates of sowing. There was a difference of 18 days to reach 50% flowering for D1 and D5. A similar trend was also observed for the milking, dough, and maturity stages. There was a difference of 18, 29, and 30 calendar days to reach the milking, dough, and maturity stages, respectively, between D1 and D5. D1 took 138 days to reach the maturity stage, while all other dates took significantly lower days to reach the maturity stage. The 3-year average data revealed that the duration of different phenophases differed significantly due to cultivars except for tiller initiation (Tables 3, 4). Systematic and accurate records of crop phenological data along with information on cultivars and management practices allow researchers to study the effects of weather on crop productivity based on actual weather data (Tao et al., 2012; Palosuo et al., 2015; Tao et al., 2017). This helps in better understanding the impact of adaptation mechanisms and studying the impact of future climate on crop production. Sattar et al. (2020) reported a reduced duration of crop phenology in response to elevated temperature. Late-sown crops had to encounter higher temperatures during critical phenophases. Out of three cultivars, HD-2733 and HD-2824 registered a similar number of days to complete different phenophases. In contrast, RAU-3711 availed significantly lesser days to attain booting (69), 50% flowering (79), milking (88), dough (114), and maturity (114) stages when compared to HD-2824 and HD-2733.
Grain filling duration and grain filling rate of wheat were significantly affected during 3 years of experimentation (Table 5). GFD, in general, showed a declining trend with the advancement of sowing dates. D1 had a grain filling duration of 47 days, which was significantly higher than all other dates of sowing. The minimum grain filling duration (34 days) was associated with D5. Delayed sowing beyond D3 significantly reduced the GFD across varieties except for RAU-3711, which gave statistically similar GFD when compared with the highest GFD for 2015–2016. Kheiri et al. (2021) reported that variations in the length of the grain filling period contributed to significant changes in the grain yield of wheat cultivars Sardari and Azar2 in Iran. A 5°C increase in temperature above 20°C increased the rate of grain filling and shortened the grain filling duration by 12 days in wheat (Yin et al., 2009). In this study, the highest GFR for 2014–2015, 2015–2016, and 2016–2017 was recorded with D2 (111 kg ha−1 day−1), D3 (55 kg ha−1 day−1), and D3 (63 kg ha−1 day−1), respectively. The pooled data indicated that the grain filling rate increased up to D3 and then started declining up to D5, implying that wheat yield decreased sharply beyond D3. This might be due to increased thermal load on the crop, which is manifested in terms of higher accumulation of GDD due to delayed sowing. Chen et al. (2018) reported a shortening of the reproductive period due to an increase in GDD and extreme temperature (34°C) degree days (EDD). The highest GFD was recorded with the cultivar RAU-3711 (Table 5), whereas HD-2824 recorded the highest GFR. Exposing the crop to higher temperatures at critical growth phases tends to significantly affect phenophase duration and crop yield (Parya et al., 2010). Poudel et al. (2021) reported that the optimum temperature during the anthesis and grain filling stage ranges from 12°C to 22°C. High temperatures greater than 22°C during anthesis to grain maturity reduced grain yield due to a decrease in grain filling duration (Joshi et al., 2007). Shortening of grain filling duration is a serious problem in wheat owing to higher average temperature during the post-heading period (Lobell et al., 2012; Garg et al., 2013).
The date of sowing produced a significant effect on the number of effective tillers per m2. D2 generated the highest number of effective tillers among all the dates of sowing (Table 6). The reduced number of tillers for the crop sown after 25 November might be due to the survival of less number of tillers under high-temperature conditions. Temperature decides the tiller initiation process in the axils of the basal leaves of wheat plants, and under unfavorable thermal regimes, the process of development of tillers either slows down or stops (Rahman et al., 2009). In our study, the impact of heat stress on the number of effective tillers was clearly visible. During 2014–2015, which did not experience any heat stress, effective tillers per m2 were observed to be the maximum (489.3), which was significantly higher as compared to that of the other two seasons. However, under the unfavorable thermal regime, the effective tillers per m2 reduced to 285.6 during 2015–2016 (Supplementary Table 1). The highest number of grains per spike was recorded with D3 for average data (Table 6). However, due to variations in temperature regime during the post-heading to grain filling period in different growing seasons, the maximum number of grains per spike was found to differ substantially from year to year. Considering test weight, it showed a declining trend with the advancement of sowing date, perhaps due to increased thermal load on the crop, causing grain shrinkage under the production of reactive oxygen species, reduced pollen tube development, increased pollen mortality, and grain abortion (Nawaz et al., 2013; Dubey et al., 2019). Among the years, the crop during 2014–2015 recorded the highest test weight (40.7 g), which was significantly higher than the other 2 years. The lowest test weight (30.4) was noted during 2015–2016, wherein the crop faced severe heat stress (Supplementary Table 1). In the case of cultivars, the highest number of effective tillers per m2 was associated with HD-2733 for all the years of experimentation, showing thermo-tolerant characteristics, and thus, this cultivar resisted the negative impact of seasonal temperature increase on active tillering. Similarly, cultivars caused significant variation in the number of grains per spike except for 2014. Poudel et al. (2020) recorded heat stress-induced reduction in tiller number and spikelets per spike in wheat, resulting in poor grain yield. Higher day length reduced the spikelet number by decreasing the initiation period (Masoni et al., 2001; Arduni et al., 2009). Owing to the differential thermal regime experienced by wheat planted under different sowing windows, grain yield was found to be significantly affected (Table 7). Grain yield increased from D1 to D2/D3 and then declined down to D5 over 3 years of experimentation. For a higher yield of wheat (>4.0 Mg ha−1) in the region, it is necessary that the period from flowering to dough stage must be completed by 15 March, beyond which yield decreases significantly (0.5 Mg ha−1 per week) due to high-temperature stress. The optimal and sub-optimal conditions for wheat growth based on yields, normal weather of congenial, and a heat stress year are given in Figure 1. The maximum grain yield was achieved with D2 for 2014–2015 and 2016–2017 (4.55 and 2.87 Mg ha−1, respectively), while D3 gave the highest grain yield for 2015–2016 (2.31 Mg ha−1). Average data of 3 years showed that D2 produced the highest grain yield (3.21 Mg ha−1), 48.61% higher than D5 (2.16 Mg ha−1). Grain yield obtained with D2 and D3 were statistically similar. Among the years, the highest grain yield was observed in 2014–2015 (4.03 Mg ha−1), which was significantly higher than the other 2 years (Supplementary Table 1). However, the lowest grain yield was observed in 2015–2016 (1.96 Mg ha−1). The crop during this year experienced the highest heat stress (Figure 2). Higher grain yield during 2014–2015 compared to the rest of the years could be ascribed to a congenial thermal regime that favored the production of higher effective tillers per m2 (489.3) and test weight (40.7 g). Dubey et al. (2019) reported yield loss of wheat at New Delhi by 70, 29, and 77 kg ha−1 per day due to delay in sowing beyond the first week of November in varieties, viz., HD-2932, WR-544 and HD-2967 respectively. In one study conducted by Poudel et al. (2021), the grain yield of wheat was reduced by 47.6% under heat-stress conditions.
Figure 1 Optimal and sub-optimal conditions during flowering to grain filling stage for higher yield of wheat.
Figure 2 Effect of sowing environment on sum of deviations from threshold maximum and minimum temperature from flowering to milking stage (A, B) and flowering to maturity stage (C, D) in wheat. tTmax, threshold maximum temperature; tTmin, threshold minimum temperature.
The interaction effect of sowing date and varieties were found significant for grain yield. Hence, genotype plus GGE biplot analysis shows the magnitude and pattern of the genotype–environment interaction effect among the genotypes in a graphical way. It revealed that the first two principal components (PCs) accounted for 70.37% and 28.56% variation of genotype + genotype–environment sum of squares, explaining a total of 98.93% variation (Figures 3, 4). The which-won-where pattern of the interaction between the date of sowing and varieties for grain yield (Figure 3) showed that variety RAU-3711 produced the highest grain yield when sown on 25 November (D2). Similarly, HD-2824 produced the highest grain yield when sown on 5 December (D3) and 15 December (D4). However, HD-2733 produced the highest grain yield when sown on 15 November (D1) and 25 December (D5). The average grain yield and stability performance of varieties are graphically depicted through the average environment coordination method (Figure 4), which helped in identifying the highest-yielding and most stable variety. The single-arrowed line that passes through the origin of the biplot and points toward higher mean values is the AEC abscissa, whereas the other line in the graph depicts the AEC ordinate. The variety farthest from the origin on the positive side of the AEC abscissa has the highest grain yield, and that farthest from the origin on the negative side of the AEC abscissa has the lowest grain yield. Therefore, the variety HD-2824 recorded the highest grain yield followed by HD-2733 and RAU-3711. However, the greater the absolute length of the projection of a variety, the less stable it is. As per Figure 4, the variety RAU-3711 was the most stable among the three varieties.
Figure 3 Polygon view of GGE biplot depicting varietal performance under various sowing dates. GGE, genotype by environment.
Figure 4 GGE biplot depicting the ranking of wheat varieties based on grain yield performance and stability. GGE, genotype by environment.
Straw yield followed a similar trend as grain yield for yearly data, but average data showed both D2 and D3 recorded the same straw yield (5.46 Mg ha−1), which was 30% higher than that of the crop planted on D5 (Table 7). Biological yield (BY) increased from D1 to D2/D3 and then declined to D5 over 3 years of experimentation. It followed a similar pattern as that of grain yield, perhaps due to the partitioning of photosynthates synchronized linearly with temperature variation. The highest BY for 2014, 2015, and 2016 and average data was recorded with D2, D3, D3, and D2, respectively. Average data showed that BY increased from D1 to D2 and then started declining. The highest BY was recorded with D2 (8.49 Mg ha−1), which was comparable with that of D3 (8.46 Mg ha−1) and D1 (8.18 Mg ha−1). D2 recorded 37.60% higher BY than D5. Delayed sowing shortens the crop growth duration and, consequently, the amount of radiation interception by the crop canopy. In response to this, biomass and yield tend to decrease significantly for the late-sown crop (Tao et al., 2015). The date of sowing significantly influenced the harvest index during 2014 and 2015 and for average data. For 2014–2015 and average data, it increased till D2, after which it started declining. However, for 2015–2016, it declined with the advancement of sowing dates. The highest harvest index for 2014–2015, 2015–2016, 2016–2017, and average data was recorded with D2, D1, D2, and D2, respectively. Varieties caused significant yield variation during 3 years of experimentation (Table 7). The highest grain yield for 2014–2015, 2015–2016, and 2016–2017 were obtained with HD-2733 (4.30 Mg ha−1), HD-2824 (2.09 Mg ha−1), and RAU-3711 (2.53 Mg ha−1), respectively. Similarly, the straw yield was significantly affected only during 2014–2015, where HD-2733 recorded a significantly higher straw yield (6.36 Mg ha−1) than the other two varieties. The average data showed that the effect of cultivar was non-significant for straw yield. The effect of varieties on BY was significant for 2014–2015, 2015–2016, and 2016–2017. However, for average data, it was non-significant. The highest BY for 2014–2015, 2015–2016, and 2016–2017 was observed with HD-2733, RAU-3711, and RAU-3711, respectively.
Varieties had a non-significant effect on harvest index (HI) except for the years 2014–2015 (Table 7). The highest HI for 2014–2015 was recorded with HD-2824 (40.66), which was comparable with HD-2733 (40.35). Similarly, HD-2824, HD-2733, and HD-2733 recorded the highest HI for 2015–2016, 2016–2017, and average data. When the sowing was delayed beyond 25 November, late-sown crops had to experience higher temperatures during the period of experimentation. HI decreased for later sown crops due to exposure to high temperatures. Dubey et al. (2019) linked the reduction of HI under the late sown condition of wheat with the greenness index and grain filling period under heat stress. High temperature during the grain filling period shrinks the size of grains in wheat apart from reduced grain filling duration (Asseng et al., 2011; Lobell et al., 2012; Tao et al., 2015). In wheat, the period from the onset of spike initiation to flowering is very sensitive to temperature acceleration, and it seems to be the main reason for the reduction in sink size under high-temperature conditions, resulting in poor grain yield (Poudel et al., 2021). However, a normal sowing window provides an opportunity to accumulate more biomass as compared to late sowing due to a longer growing period, which helps produce a higher grain yield (Singh and Pal, 2003; Dar Eajaz et al., 2018).
Air temperature modifies the enzymatic functions of plants and causes a change in phenology, which is directly related to yield (Zhu et al., 2018). The effect of temperature on crops can be effectively explained through the GDD concept. Hence, agrometeorological indices, viz., GDDs and photo-thermal index, have great practical significance in evaluating phenology and growth parameters (Streck et al., 2008; Kumar et al., 2010). Sub-optimal photo-thermal regimes during crop growing season have a profound impact on crop yield. Considering heat unit accumulation by wheat sown on different dates, it was observed that accumulated HU varied at 264°C–445°C, 640°C–917°C, 804°C–1,156°C, 926°C–1,269°C, 1,309°C–1,808°C, and 1,456°C–1,960°C days for tiller initiation, booting, 50% flowering, milking, dough, and maturity, respectively, over the experimentation period (Tables 8, 9). The average data showed that the D1 accumulated 408°C, 894°C, 1,056°C, 1,190°C, 1,709°C, and 1,860°C days for completion of tiller initiation, booting, 50% flowering, milking, dough, and maturity stages of wheat, respectively, which were significantly higher than rest of the sowing dates. Similarly, accumulated PTUs and HTU at physiological maturity were found to vary significantly with sowing environments and cultivars (Table 10). In order to harness the maximum benefits of the ambient thermal environment for higher yield, it is vital that sowing is completed at the right time. Inappropriate sowing dates cause varied weather conditions, especially in terms of the thermal requirement and radiation received by the crop canopy. In this context, an agrometeorological index such as GDD integrates phenological behavior with the thermal regime since it has a direct relation with the growth and development of crops (Mishra et al., 2007).
Table 8 Growing degree day (heat unit) (°C days) at different phenophases of wheat as influenced by sowing environments and cultivars.
Table 9 Growing degree day (heat unit) (°C days) at different phenophases of wheat as influenced by sowing environments and cultivars.
The date of sowing significantly affected HUE during all the years of experimentation (Table 11). HUE increased up to D2 and thereafter declined until D5 during 2014–2015 and 2016–2017, but for 2015–2016 and average data, it increased up to D3 and thereafter declined until D5. PTUE and HTUE were observed to be higher for the crop planted from 25 November to 5 December. Progressive delay in sowing corresponded to an increase in temperature, causing a shortening of the crop growing period leading to lower yield and higher accumulated HU. Dar Eajaz et al. (2018) linked lower thermal use efficiency of delayed sown wheat beyond the optimum window to lower yield under high moisture stress.
The sums of deviation from optimum thresholds of maximum temperature and minimum temperature for sensitive growth phases, viz., flowering to milking and flowering to maturity, were correlated with grain filling duration and grain yield to identify the degree of association and impact of heat stress. Data revealed that the sum of deviation from threshold maximum temperature (tTmax) for the F-Mlk period varied between 3.4°C in D1 in 2014–2015 and 59.5°C in D4 in 2015–2016 (Figure 2). The highest sum of deviation from tTmax for the F-Mlk period for 2014–2015, 2015–2016, and 2016–2017 was recorded with D5 (29.8°C), D4 (59.5°C), and D4 (45.8°C), respectively. Late sowing (D4 and D5) had a higher sum of deviation from tTmax during F-Mlk than the crop sown on D1 or D2 or D3 in general. The sum of deviation from threshold minimum temperature (tTmin) for the F-Mlk period varied between −12.1°C in D1 for 2016–2017 and 47.9°C in D5 for 2015–2016 (Figure 2). The highest sum of deviation from tTmin for the F-Mlk period for 2014, 2015, and 2016 was recorded with D3 (31.0°C), D5 (47.9°C), and D4 (18.6°C), respectively. The sum of deviation from tTmax for the F-Mat period varied from 47.6°C in D1 in 2014 to 232.5°C for D5 in 2015 (Figure 2). The highest sum of deviation from tTmax for the F-Mat period for 2014–2015, 2015–2016, and 2016–2017 was recorded with D5 (146.8°C), D5 (232.5°C), and D4 (162.2°C), respectively. Delaying the sowing operation increased the magnitude of the sum of deviation from tTmax for the F-Mat period. Late sowing (D4 and D5) had a higher sum of deviation from tTmax during F-Mat than the crop sown on D1 or D2 or D3. The sum of deviation from threshold minimum temperature (tTmin) for the F-Mat period varied between −49.9°C in D1 for 2016–2017 and 117.9°C in D5 for 2015–2016 (Figure 2). During the wheat growing season of 2008–2009, the accumulated sum of deviation from normal was negatively associated with the grain yield of wheat, and the number of tillers per m2 was reduced by 30%–35% due to high-temperature stress, lowering grain yield by 25%–30% (Anonymous, 2009). The highest sum of deviation from tTmin for the F-Mat period for 2014–2015, 2015–2016, and 2016–2017 was recorded with D5 (56.9°C, 117.9°C, and 95.4°C, respectively). Like tTmax during F-Mat, late sowing (D4 and D5) produced a higher sum of deviation from tTmin during F-Mat than sowing on D1, D2, or D3. The higher sum of deviation from thresholds due to the occurrence of high temperature during the post-heading period as a result of late sowing tends to accelerate the crop senescence and consequently shorten the duration (Dias and Lidon, 2009; Talukder et al., 2014). The greater the sum of deviation, the higher the heat stress experienced by the crop. This caused grain yield to reduce for later sown crops (beyond 25 November). At sensitive growth stages, such as flowering to milking, greater head load as manifested by the higher accumulation of sum of deviation (SD) enhanced canopy temperature (Siebert and Ewert, 2014), disrupted pollination, and caused pollen sterility, reducing the number of grains, consequently leading to reduced grain yield (Wheeler, 2012; Tao et al., 2015). The plots of maximum and minimum temperatures against the normal during three wheat growing seasons of the experimental period indicated that high temperatures during the reproductive and grain-filling period of wheat led to poor yield and yield attributes during 2015–2016 (Figure 5). For comparison with other years, maximum and minimum temperatures against the normal values are given in Supplementary Figure 1.
Figure 5 Variation of actual maximum temperature (Tmax) and minimum temperature (Tmin) during wheat growing seasons of 2015–2016 along with normal.
The correlation between grain yield, grain filling duration, and its rate and yield attributes with the sum of deviations from threshold maximum and minimum temperature from flowering to milking stage and flowering to maturity stage in wheat is presented in Figure 6. The correlation study indicated that grain yield was negatively and significantly affected by the sum of deviation (SD) from tTmax for F-Mat (SDTmaxF-Mat) (−0.804***) and the sum of deviation from tTmax for F-Mlk (SDTmaxF-Mlk) (−0.663***) followed by the sum of deviation from tTmin from F-Mat (SDTminF-Mat) (−0.593***). Sattar et al. (2020) observed that maximum temperature, minimum temperature, and bright sunshine hour occurring during 50% flowering to milking and 50% flowering to maturity phases of wheat demonstrated a negative correlation with grain yield.
Figure 6 Correlation between grain yield, grain filling duration, and its rate and yield attributes with sum of deviations from threshold maximum and minimum temperature from flowering to milking stage and flowering to maturity stage in wheat. * p< 0.05, ** p< 0.01, *** p< 0.001. GY, grain yield; GFR, grain filling rate; GD, grain filling duration; ET, number of effective tillers per m2; GPS, number of grains per spike; TW, test weight; tTmax, threshold maximum temperature; tTmin, threshold minimum temperature; SDTmaxF-Mlk, sum of deviation from tTmax for F-Mlk; SDTminF-Mlk, sum of deviation from tTmin from F-Mlk; SDTmaxF-Mat, sum of deviation from tTmax for F-Mat; SDTminF-Mat, sum of deviation from tTmin from F-Mat.
Similarly, SDTminF-Mat (−0.791***), the sum of deviation from tTmin from F-Mlk (SDTminF-Mlk) (0.592***), SDTmaxF-Mat (−0.436**), and SDTmaxF-Mlk (−0.359*) negatively and significantly impacted the grain filling duration. SDTmaxF-Mat (−0.672***), SDTmaxF-Mlk (−0.56***), and SDTminF-Mat (−0.372*) negatively and significantly impacted the grain filling rate. Liu et al. (2018) observed a significant negative correlation of the phase duration of wheat with mean temperature. Effective tillers per m2, which positively and significantly affected GY (0.843***), were negatively and significantly affected by SDTmaxF-Mat (−0.62***), SDTmaxF-Mlk (0.594***), and SDTminF-Mat (−0.382**). Test weight, which positively and significantly affected GY (0.822**), was negatively and significantly affected by SDTmaxF-Mat (−0.786***), SDTmaxF-Mlk (−0.723***), and SDTminF-Mat (−0.583***). However, the number of grains per spike did not vary significantly by these deviations SD from tTmax and tTmin, implying that this plant parameter is genetic and is not affected by temperature variation. Arduni et al. (2009) hypothesized that day length affects spikelet initiation and number. From the present study, it was revealed that SDTmaxF-Mat, SDTmaxF-Mlk, and SDTminF-Mat negatively impact the number of effective tillers per m2, which is one of the most important yield attributes of wheat. Similarly, SDTmaxF-Mat, SDTmaxF-Mlk, and SDTminF-Mat negatively impact test weight and grain filling rate. Similarly, SDTmaxF-Mat, SDTmaxF-Mlk, SDTminF-Mat, and SDTminF-Mlk negatively impact grain filling duration. The strategy should be such that grain filling duration is completed before the onset of critical temperature thresholds of 29°C–30°C (Dubey et al., 2019; Sattar and Srivastava, 2021). In a study conducted by Hatfield et al. (2011), cereal grain yield was found to decrease between 4.1% and 10% due to an increase in the seasonal average temperature by 1°C. In the present study, excess thermal load computed in terms of the cumulative sum of deviation from thresholds provided an important criterion for assessing the impact of thermal stress on crop yield. Tao et al. (2017) highlighted the importance of different impacts of maximum and minimum temperatures during different growth stages of winter wheat, as well as the importance of management (e.g., shift of sowing date) and cultivars’ shift in adapting to climate change in the major wheat growing region.
The projected yields of wheat for the year 2050-51 and 2080-81 simulated through the CERES-wheat DSSAT model is presented in Figure 7, which revealed that during 2050-51, the predicted wheat yield will vary from 3.07 to 3.88 Mg ha−1 across different sowing dates from 15 November to 25 December. Considering the projected yield for 2080-81, a significant decrease was observed, and it is predicted to range between 2.01 and 3.25 Mg ha−1. For the crop planted during November and December, a decrease in yield by 4.9% to 12.0% and 33.8% to 42.4% is predicted during 2050-51 and 2080-81, respectively (Figure 8). Chhabra and Haris (2014) also reported a decline in wheat yield in the region by 3.6%–13% in 2050 and 14.1%–40% in 2080. The grain yield of wheat was projected to decline in Pakistan by 7%–18% in 2050 and 9%–30% in 2090 under RCP 4.5 (Ishaque et al., 2023). In view of the significant decrease in wheat yield in the future, effective mitigation and adaptation measures will be required to sustain wheat production in the region.
Figure 7 Predicted yield of wheat for 2050-51 and 2080-81 on different sowing dates by CERES-Wheat model under RCP 4.5. D1, 15 November; D2, 25 November; D3, 5 December; D4, 15 December; D5, 25 December.
Figure 8 Decrease (%) in wheat yield in 2050-51 and 2080-81 on different sowing dates by CERES-Wheat model under RCP 4.5. D1, 15 November; D2, 25 November; D3, 5 December; D4, 15 December; D5, 25 December.
The responses of phenology, yield, and yield attributing characters of wheat to differential thermal regimes are critically evaluated in this article, and we found that the crop response did vary significantly across different sowing environments. Excess thermal load computed in terms of the cumulative sum of deviation from critical thresholds provided a useful criterion for assessing the effect of heat stress on wheat yield in association with various agrometeorological indices such as GDDs, HTU, and accumulated PTU. Differential sowing dates generated varied weather patterns especially in terms of the thermal requirement during the growing period of wheat. For a higher yield of wheat (>4.0 Mg ha−1) in the region, it is necessary that grain filling is completed by 15 March, beyond which yield decreases substantially (0.5 Mg ha−1 per week) due to high-temperature stress. The optimal and sub-optimal conditions for wheat growth stressed the importance of the manipulation of sowing dates. Hence, it is necessary that wheat is planted at appropriate times for higher productivity. Critical responses of wheat phenology, yield, and yield attributing characters to varying sowing environments indicated that tactical decisions by the wheat growers keeping heat stress in mind would form an important guiding factor for wheat farming. Heat stress during the post-heading period is a serious climatic constraint for successful wheat production in the region. Since wheat growth is very sensitive to temperature, farmers in the area would be advised to finish their wheat planting before 25 November. Accordingly, shifting the planting time from the window of 25 November–10 December, which is usually practiced by 80% of wheat growers of the region to the window as prescribed in this study (i.e., finishing wheat sowing before 25 November), would be an important adaptation option for realizing higher yield and mitigating the negative impact of terminal heat stress on wheat growth and productivity. GGE biplot analysis indicated that RAU-3711 performed better when sown on 25 November (D2), whereas HD-2824 and HD-2733 performed better under 5 December (D3) and 15 December (D4) and 15 November (D1) and 25 December (D5). Wheat yield is predicted to decline significantly in 2050-51 and 2080-81 under RCP 4.5 scenario. Further studies using different models with a range of cultivars and management practices are needed to evaluate the impact of future climate change on wheat yield in the region.
The original contributions presented in the study are included in the article/Supplementary Material. Further inquiries can be directed to the corresponding author.
Conceptualization: AS. Methodology: AS, GN, SKB. Data curation: AS, GN, GS, SKB. Original draft: AS, GN. Writing, review, and editing: AS, GN, RKJ, SKB, GS. Visualization: AS, GN. All authors contributed to the article and approved the submitted version.
The work has been carried out under the All India Coordinated Research Project on Agrometeorology (AICRPAM) funded by the Indian Council of Agricultural Research (ICAR), New Delhi. Sincere thanks are due to the authority of Dr. Rajendra Prasad Central Agricultural University for providing the necessary facilities for carrying out the research under the project.
The authors declare that the research was conducted in the absence of any commercial or financial relationships that could be construed as a potential conflict of interest.
All claims expressed in this article are solely those of the authors and do not necessarily represent those of their affiliated organizations, or those of the publisher, the editors and the reviewers. Any product that may be evaluated in this article, or claim that may be made by its manufacturer, is not guaranteed or endorsed by the publisher.
The Supplementary Material for this article can be found online at: https://www.frontiersin.org/articles/10.3389/fpls.2023.1224334/full#supplementary-material
Amrawat, T., Solanki, N. S., Sharma, S. K., Jajoria, D. K., Dotaniya, M. L. (2013). Phenology growth and yield of wheat in relation to agrometeorological indices under different sowing dates. Afr. J. Agricutural Res. 8 (49), 6366–6374. doi: 10.5897/AJAR2013.8019
Anonymous (2009). Annual Progress Report, All India Coordinated Research Project on Agrometeorology Vol. 52 (RAU, Pusa, Bihar: Indian Council of Agricultural Research).
Arduni, I., Ercoli, L., Mariotti, M., Masoni, A. (2009). Sowing Date Affect Spikelet Number and Grain Yield of Durum Wheat. Cereal Res. Commun. 37 (3), 469–478. doi: 10.1556/CRC.37.2009.3.16
Asseng, S., Foster, I., Turner, N. C. (2011). The impact of temperature variability on wheat yields. Global Change Biol. 17, 997–1012. doi: 10.1111/j.1365-2486.2010.02262.x
Bal, S. K., Prasad, J. V. N. S., Singh, V. K. (2022). Heat wave 2022 Causes, impacts and way forward for Indian Agriculture Vol. 50 (Hyderabad, Telangana, India: Technical Bulletin No. ICAR/CRIDA/ TB/01/2022, ICAR-Central Research Institute for Dryland Agriculture).
Bapuji Rao, B., Santhibhushan Chowdary, P., Sandeep, V. M., Pramod, V. P., Rao, V. U. M. (2015). Spatial analysis of the sensitivity of wheat yields to temperature in India. Agric. For. Meteorol. 200, 192–202. doi: 10.1016/j.agrformet.2014.09.023
Chen, Y., Zhang, Z., Tao, F., Palosuot, T., Rötter Reimund, P.. (2018). Impacts of heat stress on leaf area index and growth duration of winter wheat in the North China Plain. Field Crops Res. 222, 230–237. doi: 10.1016/j.fcr.2017.06.007
Chhabra, V., Haris, A. A. (2014). Temperature Trends and their impact on rabi crops in changing climatic scenario of Bihar. Sch. J. Agric. Vet. Sci. 1 (4A), 230–234.
Dar Eajaz, A., Brar, A. S., Yousuf, A. (2018). Growing degree days and heat use efficiency of wheat as influenced by thermal and moisture regimes, Jour. Agrometeorol. 20 (2), 168–170. doi: 10.54386/jam.v20i2.535
Dias, A. S., Lidon, F. C. (2009). Evaluation of grain filling rate and duration in bread and durum wheat, under heat stress after anthesis. J. Agron. Crop Sci. 195, 137–147. doi: 10.1111/j.1439-037X.2008.00347.x
Dubey, R., Pathak, H., Singh, S., Chakraborty, B., Thakur, A. K., Fagodia, R. K. (2019). Impact of Sowing Dates on Terminal Heat Tolerance of Different Wheat (Triticum aestivum L.) Cultivars. Natl. Acad. Sci. Lett. 42, 445–449. doi: 10.1007/s40009-019-0786-7
FAO. (2017). Food and Agriculture Organization of the United Nations. Available at: http://FAOSTAT.fao.org.
Farooq, M., Bramley, H., Palta, J. A., Siddique, K. H. (2011). Heat stress in wheat during reproductive and grain-filling phases. Crit. Rev. Plant Sci. 30 (6), 491–507. doi: 10.1080/07352689.2011.615687
Garg, D., Sareen, S., Dalal, S., Tiwari, R., Singh, R. (2013). Grain filling duration and temperature pattern influence on the performance of wheat genotypes under late planting. Cereal Res. Commun. 41, 500–507. doi: 10.1556/CRC.2013.0019
Gomez, K. A., Gomez, A. A. (1984). Statistical procedures for agricultural research (Hoboken: Wiley).
Hatfield, J. L., Boote, K. J., Kimball, B. A., Ziska, L. H., Izaurralde, R. C., Ort, D., et al. (2011). Climate impact on agriculture: implications for crop production. J. Agron. 103, 351–370. doi: 10.2134/agronj2010.0303
Hoogenboom, G., Porter, C. H., Shelia, V., Boote, K. J., Singh, U., White, J. W., et al. (2019). Decision Support System for Agrotechnology Transfer (DSSAT) Version 4.7.5 (Florida, USA: DSSAT Foundation, Gainesville). Available at: https://DSSAT.net.
Ishaque, W., Osman, R., Hafiza, B. S., Malghani, S., Zhao, B., Zu, M., et al. (2023). Quantifying the impacts of climate change on wheat phenology, yield, and evapotranspiration under irrigated and rainfed conditions. Agric. Water Manage. 275. doi: 10.1016/j.agwat.2022.108017
Joshi, A. K., Mishra, B., Chatrath, R., Ferrara, G. O., Singh, R. P. (2007). Wheat improvement in India: present status, emerging challenges and future prospects. Euphytica 157 (3), 431–446. doi: 10.1007/s10681-007-9385-7
Kheiri, M., Soufizadeh, S., Moghaddam, S. M., Ghaffari, A. (2021). Exploring the Impact of Weather Variability on Phenology, Length of Growing Period, and Yield of Contrast Dryland Wheat Cultivars. Agric. Res. 10 (4), 556–568. doi: 10.1007/s40003-020-00523-x
Kumar, R., Ramesh, K., Singh, R. D., Prasad, R. (2010). Modulation of wild marigold (Tagetes minuta L.) phenophases towards the varying temperature regimes - a field study. J. Agrometeorol. 12, 234–240. doi: 10.54386/jam.v12i2.1313
Li, L., Wang, B., Feng, P., Wangh, H., He, Q., Wang, Y., et al. (2021). Crop yield forecasting and associated optimum lead time analysis based on multi-source environmental data across China. Agric. For. Meteorol. 308-309, 108558. doi: 10.1016/j.agrformet.2021.108558
Liu, Y., Chen, Q., Ge, Q., Dai, J., Dou, Y. (2018). Effects of climate change and agronomic practice on changes in wheat phenology. Climate Change 150, 273–287. doi: 10.1007/s10584-018-2264-5
Lobell, D. B., Sibley, A., Ortiz-Monasterio, J. I. (2012). Extreme heat effects on wheat senescence in India. Nat. Clim. Change 2, 186–189. doi: 10.1038/nclimate1356
Masoni, A., Arduini, I., Mariotti, M., Ercoli, L., Bonari, E. (2001). Number of spikelets and leaf appearance rate in durum wheat as affected by temperature, daylength and nitrogen availability. Agricol. Medit. 131, 57–65.
Mishra, A. K., Tripathi, P., Mishra, S. R., Kumar, R. (2007). Heat uni requirement of wheat cultivars as affected by moisture application frequencies. Jour. Agrometeorol. 2, 295–298. doi: 10.54386/jam.v9i2.1147
Nawaz, A., Farooq, M., Cheema, S. ,. A., Wahid, A. (2013). Differential response of wheat cultivars to terminal heat stress. Int. J. Agric. Biol. 15, 1354–1358.
Nuttonson, M. Y. (1955). Wheat climate relationship and the use of phenology in ascerting the thermal and photo thermal requirement of wheat Vol. 388 (Washington DC, USA: American Institute of crop ecology).
Palosuo, T., Rötter, R. P., Salo, T., Peltonen-Sainio, P., Tao, F., Lehtonen, H. (2015). Effects of climate and historical adaptation measures on barley yield trends inFinland. Clim. Res. 65, 221–236. doi: 10.3354/cr01317
Parya, M., Nath, R., Mazumder, D., Chakraborty, P. K. (2010). Effect of thermal stress on wheat productivity in West Bengal, Jour. Agrometeorol. 12 (2), 217–220. doi: 10.54386/jam.v12i2.1309
Poudel, M. R., Ghimire, S., Pandey, M. P., Krishnahari, D., Bahadur, T. D., Paudel, H. K., et al. (2020). Evaluation of wheat genotypes under irrigated, heat stress and drought conditions. J. Biol. Today's World 9 (1), 1–3.
Poudel, P. B., Poudel, M. R., Puri, R. R. (2021). Evaluation of heat stress tolerance in spring wheat (Triticum aestivum L.) genotypes using stress tolerance indices in western region of Nepal. J. Agric. Food Res. 5, 100179. doi: 10.1016/j.jafr.2021.100179
Rahman, M. ,. A., Chikushi, J., Yoshida, S., Karim, A. J. M. S. (2009). Growth and yield components of wheat genotypes exposed to high temperature stress under control environment. Bangladesh J. Agric. Res. 34 (3), 360–372.
Ram, H., Singh, G., Mavi, G. S., Sohu, V. S. (2012). Accumulated heat unit requirement and yield of irrigated wheat (Triticum aestivum L.) varieties under different crop growing environment in central Punjab. J. Agrometeorol. 14, 147–153. doi: 10.54386/jam.v14i2.1414
Saeidnia, F., Taherian, M., Nazeri, S. M. (2023). Graphical analysis of multi-environmental trials for wheat grain yield based on GGE-biplot analysis under diverse sowing dates. BMC Plant Biol. 23, 198. doi: 10.1186/s12870-023-04197-9
Sandhu, S. S., Prabhjyot-Kaur, T. ,. P., Patel, S. R., Rajinder, P., Solanki, N. S., Ramesh., K., et al. (2016). Effect of intra-seasonal temperature on wheat at different locations of India: A study using CERES-Wheat model. J. Agrometeorol. 18 (2), 222–233. doi: 10.54386/jam.v18i2.939
Sastry, P. S. N., Chakravarty, N. K. (1982). Energy summation indices for wheat crop in India. Agric. Meteorol. 27 (1-2), 45–48. doi: 10.1016/0002-1571(82)90018-8
Sattar, A., Singh, G., Singh, S., Kumar, M., Kumar, P. V., Bal, S. K. (2020). Evaluating temperature thresholds and optimizing sowing dates of wheat in Bihar. J. Agrometeorol. 22 (2), 158–164. doi: 10.54386/jam.v22i2.156
Sattar, A., Srivastava, R. C. (2021). Modelling climate smart rice-wheat production system in the middle Gangetic plains of India. Theor. Appl. Climatology 144, 77–91. doi: 10.1007/s00704-020-03497-6
Shen, H., Wang, Yu., Jiang, K., Li, S., Huang, D., Wu, J., et al. (2022). Simulation modeling for effective management of irrigation water for winter wheat Agricultural. Water Manage 269. doi: 10.1016/j.agwat.2022.107720
Siebert, S., Ewert, F. (2014). Future crop production threatened by extreme heat. Environ. Res. Lett. 9, 041001. doi: 10.1088/1748-9326/9/4/041001
Singh, M., Khushu, M. K. (2012). Growth and yield prediction of wheat in relation to agroclimatic indices under irrigated and rainfed condition. J. Agrometeorol. 14 (1), 63–66. doi: 10.54386/jam.v14i1.1386
Singh, S., Pal, M. (2003). Growth, yield and phonological response of wheat cultivars to delayed sowing. Indian J. Plant Physiol. 8, 277–287.
Singh, A. K., Tripathi, P., Mishra, S. R. (2001). Phenology, growing degree-days and phasic development model of wheat under rice-wheat cropping system. Ind. J. Agril. Sci. 71 (6), 363–366.
Streck, N. A., Lago, I., Gabriel, L. F., Samboranha, F. K. (2008). Simulating maize phenology as a function of air temperature with a linear and a nonlinear model. Pesquisa Agropecuária Bras. 43, 449–455. doi: 10.1590/S0100-204X2008000400002
Talukder, A. S. M. H., Mcdonald, G. K., Gill, G. S. (2014). Effect of short-term heat stress prior to flowering and early grain set on the grain yield of wheat. Field Crop Res. 160, 54–63. doi: 10.1016/j.fcr.2014.01.013
Tao, F., Xiao, D., Zhang, S., Zhang, Z., Rotter, R. P. (2017). Wheat yield benefited from increases in minimum temperature in theHuang-Huai-Hai Plain of China in the past three decades. Agric. For. Meteorol. 239, 1–14. doi: 10.1016/j.agrformet.2017.02.033
Tao, F. L., Zhang, S., Zhang, Z. (2012). Spatiotemporal changes of wheat phenology in China under the effects of temperature, day length and cultivar thermalcharacteristics. Eur. J. Agron. 43, 201–212. doi: 10.1016/j.eja.2012.07.005
Tao, F., Zhang, L., Zhang, Z., Chen, Y. (2022). Climate warming outweighed agricultural managements in affecting wheat phenology across China during 1981-2018. Agric. For. Meteorol. 316, 108865. doi: 10.1016/j.agrformet.2022.108865
Tao, F., Zhao, Z., Shuai, Z., Reimund, R. (2015). Heat stress impacts on wheat growth and yield were reduced in theHuang-Huai-Hai Plain of China in the past three decades Europ. J. Agron. 71, 44–52. doi: 10.1016/j.eja.2015.08.003
Vashisth, A., Goyal, A., Krishnan, P. (2020). Effect of weather variability on growth and yield of wheat crop under semi-arid region of India. J. Agrometeorol. 22 (2), 124–131. doi: 10.54386/jam.v22i2.152
Vijaya, K. P., Rao, V. U. M., Bhavani, O., Dubey, A. P., Singh, C. B., Venkateswarlu, B. (2015). Sensitive growth stages and temperature thresholds in wheat (Triticum aestivum L.) for index-based crop insurance in the Indo-Gangetic Plains of India J. Agric. Sci., 154 (2), 321–333. doi: 10.1017/S0021859615000209
Wang, J., Wang, E., Feng, L., Yin, H., Yu, W. (2013). Phenological trends of winter wheat in response to varietal and temperature changes in the North China Plain. Field Crops Res. 144(2013), 135–144. doi: 10.1016/j.fcr.2012.12.020
Wang, J., Wang, E., Yang, X., Zhang, F., Yin, H. (2011). Increased yield potential of wheat–maize cropping system in the North China Plain by climate change adaptation. Climat Change 113, 825–840. doi: 10.1007/s10584-011-0385-1
Wheeler, T. (2012). Wheat crops feel the heat. Nat. Clim. Change 2, 152–153. doi: 10.1038/nclimate1425
Xiao, D., Tao, F., Liu, Y., Shi, W, Wang, M, Liu, F, et al. (2013). Observed changes in winter wheat phenology in the North China Plain for 1981–2009. Int. J. Biometeorol. 57 (2), 275–285. doi: 10.1007/s00484-012-0552-8
Xiao, D., Tao, F., Liu, Y., Shi, W., Wang, M., Liu, F, et al. (2012). Observed changes in winter wheat phenology in the North China Plain for 1981–2009. Int. J. Biometeorol 57, 275–285. doi: 10.1007/s00484-012-0552-8
Yin, X., Guo, W., Spiertz, J. H. (2009). Aquantitative approach to characterizesink—source relationships during grain filling in contrasting wheat genotypes. Field Crops Res. 114, 119–126. doi: 10.1016/j.fcr.2009.07.013
Keywords: wheat, phenology, yield, sowing window, heat stress, simulation, CERES-wheat
Citation: Sattar A, Nanda G, Singh G, Jha RK and Bal SK (2023) Responses of phenology, yield attributes, and yield of wheat varieties under different sowing times in Indo-Gangetic Plains. Front. Plant Sci. 14:1224334. doi: 10.3389/fpls.2023.1224334
Received: 17 May 2023; Accepted: 03 July 2023;
Published: 21 July 2023.
Edited by:
Debojyoti Moulick, Independent Researcher, Kolkata, IndiaReviewed by:
Sanjay Singh Rathore, Indian Agricultural Research Institute (ICAR), IndiaCopyright © 2023 Sattar, Nanda, Singh, Jha and Bal. This is an open-access article distributed under the terms of the Creative Commons Attribution License (CC BY). The use, distribution or reproduction in other forums is permitted, provided the original author(s) and the copyright owner(s) are credited and that the original publication in this journal is cited, in accordance with accepted academic practice. No use, distribution or reproduction is permitted which does not comply with these terms.
*Correspondence: Abdus Sattar, YXNhdHRhckBycGNhdS5hYy5pbg==
Disclaimer: All claims expressed in this article are solely those of the authors and do not necessarily represent those of their affiliated organizations, or those of the publisher, the editors and the reviewers. Any product that may be evaluated in this article or claim that may be made by its manufacturer is not guaranteed or endorsed by the publisher.
Research integrity at Frontiers
Learn more about the work of our research integrity team to safeguard the quality of each article we publish.