- 1Marine Biodiversity and Ecology Laboratory, Department of Zoology, Faculty of Science, The Maharaja Sayajirao University of Baroda, Vadodara, Gujarat, India
- 2University Grants Commission-Career Advancement Scheme (UGC-CAS) Department of Biosciences, Saurashtra University, Rajkot, Gujarat, India
- 3Department of Microbiology, Atmiya University, Rajkot, Gujarat, India
- 4Department of Life Sciences, Hemchandracharya North Gujarat University, Patan, Gujarat, India
- 5DNA Division, Directorate of Forensic Science, Gandhinagar, India
- 6School of Chemical Sciences, Swami Ramanand Teerth Marathwada University, Nanded, Maharashtra, India
- 7Department of Biosciences, Veer Narmad South Gujarat University, Surat, India
- 8Department of Veterinary Clinical Sciences, College of Veterinary Medicine, Iowa State University, Ames, IA, United States
Aedes mosquitoes are the major cause of several vector-borne diseases in tropical and subtropical regions. Synthetic pesticides against these mosquitoes have certain limitations; hence, natural, eco-friendly, and safe larvicides obtained from plant resources are used to overcome these. In the present study, the larvicidal efficiency of Commiphora wightii against the fourth instar stage of the dengue fever mosquito Aedes aegypti (Linnaeus, 1762) was studied. The gum resin of C. wightii was collected using the borehole tapping method, and hexane extracts in different concentrations were prepared. The fourth-instar larvae were exposed to the extracts, and percent mortality, as well as LC20, LC50, and LC90, was calculated. Volatile compounds of the hexane gum extract were analyzed by Headspace GC/MS, and the sequence of the acetylcholine, Gamma-aminobutyric acid (GABA) receptor, and octopamine receptor subunit of A. aegypti was obtained. It was found that the hexane gum extract was toxic and lethal for larvae at different concentrations. Minimum mortality was observed at 164 µg mL−1 (10%/h), while maximum mortality was at 276 µg mL−1 (50%/h). The lethal concentrations LC20, LC50, and LC90 were 197.38 µg mL−1, 294.13 µg mL−1, and 540.15 µg mL−1, respectively. The GC/MS analysis confirmed the presence of diterpenes, monoterpenes, monoterpene alcohol, and sesquiterpenes in the gum samples, which are lethal for larvae due to their inhibitory activity on the acetylcholinesterase enzyme, GABA receptor, and octopamine receptor subunit. The use of commonly occurring plant gum for the control of mosquitoes was explored, and it was found that the gum of C. wightii had larvicidal activities and could be potentially insecticidal.
Introduction
Mosquitoes are a well-known insect vector, transmitting various diseases that lead to millions of deaths every year (Acock et al., 2022; Kaavya et al., 2022). Globally, there is a documented presence of approximately 3,540 species (41 genera) of mosquitoes (Harbach and Besansky, 2014). Among these, Aedes mosquitoes are the main cause of viral vector-borne diseases such as chikungunya, Zika virus infection, yellow fever, hemorrhagic fever, and dengue fever in the tropical and subtropical regions (Yang et al., 2009; Kaavya et al., 2022). Out of these, dengue is a dominant and rapidly spreading disease caused by Aedes aegypti mosquitoes (Guzman and Harris, 2015). The transmission of dengue fever is facilitated by female A. aegypti mosquitoes, which deposit their eggs in stagnant water to raise the immature stages of their biphasic life cycle (Benelli and Mehlhorn, 2016).
Presently, the absence of efficacious dengue vaccines necessitates reliance on mosquito control as the sole preventive measure against the disease. This can be achieved through the wide use of different insecticides available. Synthetic larvicides are widely used in Aedes mosquitoes’ breeding places to control their vector-containing larva growth. However, deficiency in the control plans has resulted in the development of resistance by larvae against these synthetic larvicides (Braga et al., 2004; Medronho, 2008; Dusfour et al., 2011). In a study using WHO protocol to determine insecticide resistance and its mechanisms in the primary dengue vector in dengue-endemic districts of West Bengal, India, it was found that most Aedes populations were resistant to multiple synthetic insecticides (Bharati and Saha, 2018).
The development of insecticidal resistance has become a major issue in controlling vectors and, hence, vector-borne diseases. Such resistance can be induced due to changes in mosquitoes’ enzymatic system, leading to rapid detoxification and sequestration of exposed insecticide. Moreover, mutations in the target site can also prevent insecticide–target interaction (Hemingway et al., 2004). Synthetic larvicides also have an adverse effect on the environment.
In order to overcome these issues, many researchers have explored natural, eco-friendly, and safe larvicides obtained from plant resources. Various studies have documented biologically active plant compounds having efficient larvicidal and insecticidal effects on mosquitoes. It has been observed that these biologically active plant compounds are evolving and that they have no negative effects on the environment. They present the best alternative to synthetic pesticides, as they are environment-friendly, least toxic to human health, and convenient to use. Approximately 2,000 plant species are known for producing compounds that can be used for pest management, out of which 344 species are reported to be used against mosquitoes. Although these many species are known for mosquito control, phytochemical extracts of only a few plants have been known to act against mosquitoes in the form of growth regulators, repellents, and ovipositional barriers.
Guggulu is an oleo-gum resin that exudes from the bark of Commiphora wightii (Arnott) Bhandari or “Indian bdellium.” It has been used in Ayurveda since time immemorial to treat a variety of disorders, such as inflammation, gout, rheumatism, obesity, and lipid metabolism disorders (Sarup et al., 2015). Commiphora wightii resin incense was burned to repel mosquitoes traditionally by the tribes of Rajasthan, India (Kantheti and Padma, 2017).
The larvicidal activity of C. wightii gum resin and the effect of the identified volatile compounds on the development of A. aegypti larvae were analyzed. Moreover, the phytochemicals were screened against a homology model of the nervous system protein target acetylcholinesterase, the GABA receptor subunit, and the octopamine receptor of A. aegypti larvae.
Materials and methods
Collection of gum and preparation of hexane gum extract
The borehole tapping method was used to obtain gum resin from the bark of C. wightii. Pure hexane (Sigma Aldrich; purity: ≥99%; CAS Number: 592-41-6) was used to prepare the extract from the gum sample using the Soxhlet extraction procedure for 3 h (Vasishth and Guleria, 2017). Later, 200-mL samples were extracted and stored in a vacuum-tight glass reagent bottle at 4°C until further analysis.
Culturing of Aedes aegypti larva
Dengue fever mosquitoes (A. aegypti) were cultured in the small mosquito insectary under laboratory conditions at the botanical garden of the Department of Biosciences, Saurashtra University, Rajkot, Gujarat, India. The colony was maintained in breeding cages 40 cm × 40 cm × 40 cm with standard conditions like temperature (28°C ± 1°C), humidity (80% ± 5% RH), and photoperiod (14L:10D) (Kumar et al., 2010; Udayanga et al., 2019). The fourth instar stage of A. aegypti larvae was used for bioassays.
Larvicidal activity
The larvicidal activity was carried out at a laboratory scale. Different concentrations of the plant gum extracts in hexane were prepared to range from 164 µg mL−1 to 284 µg mL−1. For each hexane gum extract, 50 larvae of A. aegypti (fourth instar stage) were exposed for 60 min. The controls were exposed to the hexane solvent. The duration required for the mortality of half the number of larvae and all of the larvae in each concentration of hexane gum extract was recorded. Each bioassay was replicated in triplicates. The percent mortality of larvae was calculated using the following formula:
Statistical analysis
The percent mortality data were subjected to probit analysis for the calculation of LC20, LC50, and LC90. The regression analysis at the 95% confidence level and chi-square values were acculated by considering a p-value<0.05 to be statistically significant. The analyses were performed using Past software (4.03 version).
Headspace GC/MS analysis of gum samples
The volatile compounds present in each hexane gum extract were analyzed by Headspace GC/MS. Their total ion chromatograms were obtained using a GERSTEL DHS System (Germany) connected to an Agilent 7890A GC and 5975C MS equipped with an Inert MSD with Triple Axis Detector (Germany). Each gum sample (1 g) was placed in a 20-mL standard headspace vial, which was placed in a tray of a GERSTEL Multipurpose Sampler. Volatile compounds were injected automatically into a CP-WAX 52 GC column (60 m × 25 μm film thickness × 0.25 mm) inner diameter (INNOWax, Germany). In the analytical conditions, the flow rate of helium gas was 1.2 mL/min, inlet pressure was 25 kPa, linear velocity was 1 mL/min at 210°C, injector temperature was 250°C, and injection mode was splitless. In the MS scan conditions, the source temperature was 200°C, the interface temperature was 250°C, the electron energy was 70 eV, and the mass scan was in the range of 40–350 amu. The temperature program was from 40°C to 240°C at a rate of 5°C/min. The relative percentage of each identified compound was calculated from the GC peak area. The compounds in each sample were identified through a comparison of spectral mass patterns and linear retention indices based on the n-alkane standards from C8–C32 with data reported in the Wiley and NIST databases. The retention index was calculated as per the retention index system proposed by Van Den Dool and Kratz (1963) using the following formula for temperature-programmed retention index:
where Ix is the temperature-programmed retention index, and tn, tn+1, and tx are the retention time (in min) of the two n-alkanes containing n and n + 1 carbons and the compound of interest, respectively.
Homology modeling
The acetylcholinesterase and GABA receptor subunit protein and octopamine receptor sequences of A. aegypti were retrieved in FASTA format from the National Center for Biotechnology Information (https://www.ncbi.nlm.nih.gov/protein) with accession number AAB35001.1, AAA68961.1, and XP021692997.1, respectively. The protein’s three-dimensional structure was computationally generated with the homology modeling technique as described by the developer (Waterhouse et al., 2018).
Molecular docking study
All molecular structures of phytochemicals reported in various fractions during LC-MS analysis were acquired from the PubChem database (https://pubchem.ncbi.nlm.nih.gov). The ligand library included natural substrates and established inhibitors. The protein structures and ligands were transformed to pdbqt format using Openbabel software, and the energy was minimized by implementing the Autodock Vina Tool’s Python script. Autodock Vina 1.2.0 was used for docking following the developers’ default parameters (Eberhardt et al., 2021). The software iGEMDOCK version 2.1 was employed to investigate postdocking ligand interactions (Hsu et al., 2011).
To model the target sequence, ProMod3 3.2.1 was used, and the SWISS-MODEL template library (SMTL version 2023-04-05, PDB release 2023-03-31) was searched for evolution-related structures that matched the target sequence.
The 6huj, 6xyy, and 2z73 PDB structures were used as a template for acetylcholinesterase, GABA receptor subunit, and octopamine receptor, respectively. The obtained model had 94.74%, 93.29%, and 79.88% amino acid residue in the Ramachandran plot favored region.
Results
Larvicidal properties against A. aegypti
The larvicidal activity was examined in the range of 164–284 µg mL−1 (Figure 1). Minimum mortality was observed at the concentration of 164 µg mL−1 (10%/h), while maximum mortality was observed at the concentration of 276 µg mL−1 (50%/h). The lethal concentrations LC20, LC50, and LC90 were 197.38 µg mL−1, 294.13 µg mL−1, and 540.15 µg mL−1, respectively (χ2 = 0.905, p< 0.001) (Table 1). There was no significant mortality observed in the control group treated with only hexane solvent (Figure 1).
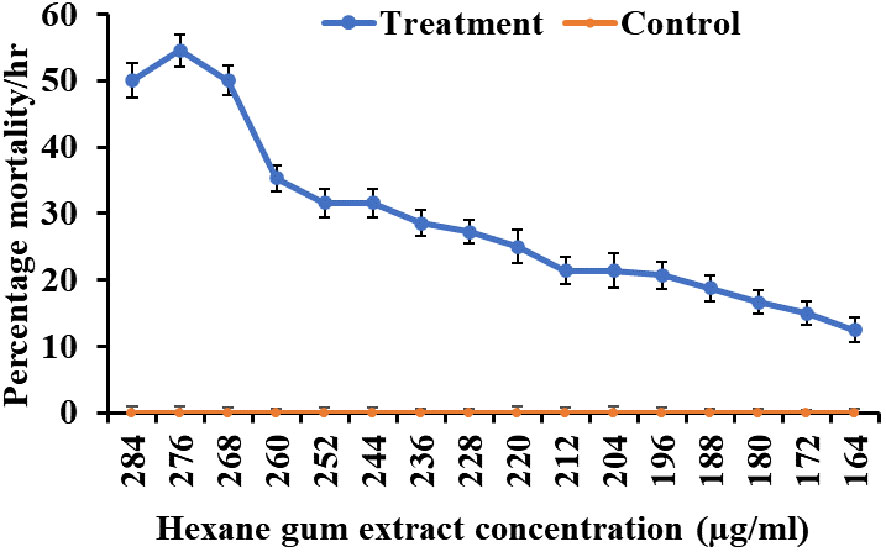
Figure 1 Mortality at different concentrations of Commiphora wightii gum hexane extracts against the fourth instar stage of Aedes aegypti larvae.

Table 1 Mosquito larvicidal effectiveness of hexane gum extract against Aedes aegypti (95% confidence interval) (***p< 0.001).
Identification of volatile compounds from plant gum extracts prepared in hexane
Headspace GC/MS analysis was conducted for the hexane gum extract of C. wightii, which revealed the following types of volatile compounds: monoterpenes [α-Pinene (11.725%), 2-Norpinene 3,6,6-trimethyl- (10.607%), α-Ocimene (2.309%), α-Myrcene (5.289%), Limonene (5.324%), α-Terpineol (1.056%), β-Acoradiene (2.262%), and Sesquisabinene (1.140%)], sesquiterpenes [Caryophyllene (6.128%), β-Elemene (2.381%), α-Curcumene (3.961%), Sesquithujene (1.693%), and α-Bisabolene (1.033%)], and diterpenes [Cembrene (1.757%) and Neocembrene (9.950%)] (Supplementary Figure S1; Table 2). The refraction index of all identified compounds was calculated as per the retention index system proposed by Van Den Dool and Kratz (1963). The calculated retention indices of each identified compound were compared with the retention indices available in the NIST Chemistry WebBook, SRD 69 (Table 2). Previous studies have reported the presence of phytoconstituent groups such as monoterpenoids, sesquiterpenoids, diterpenoids, and triterpenoids; steroids; flavonoids; guggultetrols; lignans; sugars; and amino acids in the resin of C. wightii. Specific phytochemicals, viz., monoterpene (Limonene, α-Terpineol, α-Pinene, and α-Myrcene) and diterpene (Cembrene), have been reported in the gum resin of C. wightii (Sarup et al., 2015; Chandran et al., 2020).
Molecular docking study
Acetylcholinesterase molecular docking
Acetylcholinesterase was docked against 15 unique phytochemicals, including the substrate acetylcholine and the inhibitor carbamate insecticide propoxur. The docking binding energy for phytochemicals ranged between -5.4 and -8.2. The binding energy of the substrate acetylcholine and the inhibitor carbamate insecticide propoxur was -4.5 and -7.8, respectively. The least binding energy was obtained for α-Bisabolene (-8.2), Caryophyllene (-8.1), and β-Acoradiene (-7.9), followed by other compounds (Figure 2). Acetylcholinesterase and docked phytochemical interaction analysis displayed diverse interaction profiles into five clusters. Acetylcholine and the inhibitor propoxur have been classified into different clusters. The three highest ranking α-Bisabolene, Caryophyllene, and β-Acoradiene had similar interaction profiles and shared a common bond pattern with substrate acetylcholine and inhibitor propoxur, indicating the potential of inhibition activity at the molecular level along with the obtained best docking score (Figure 3).
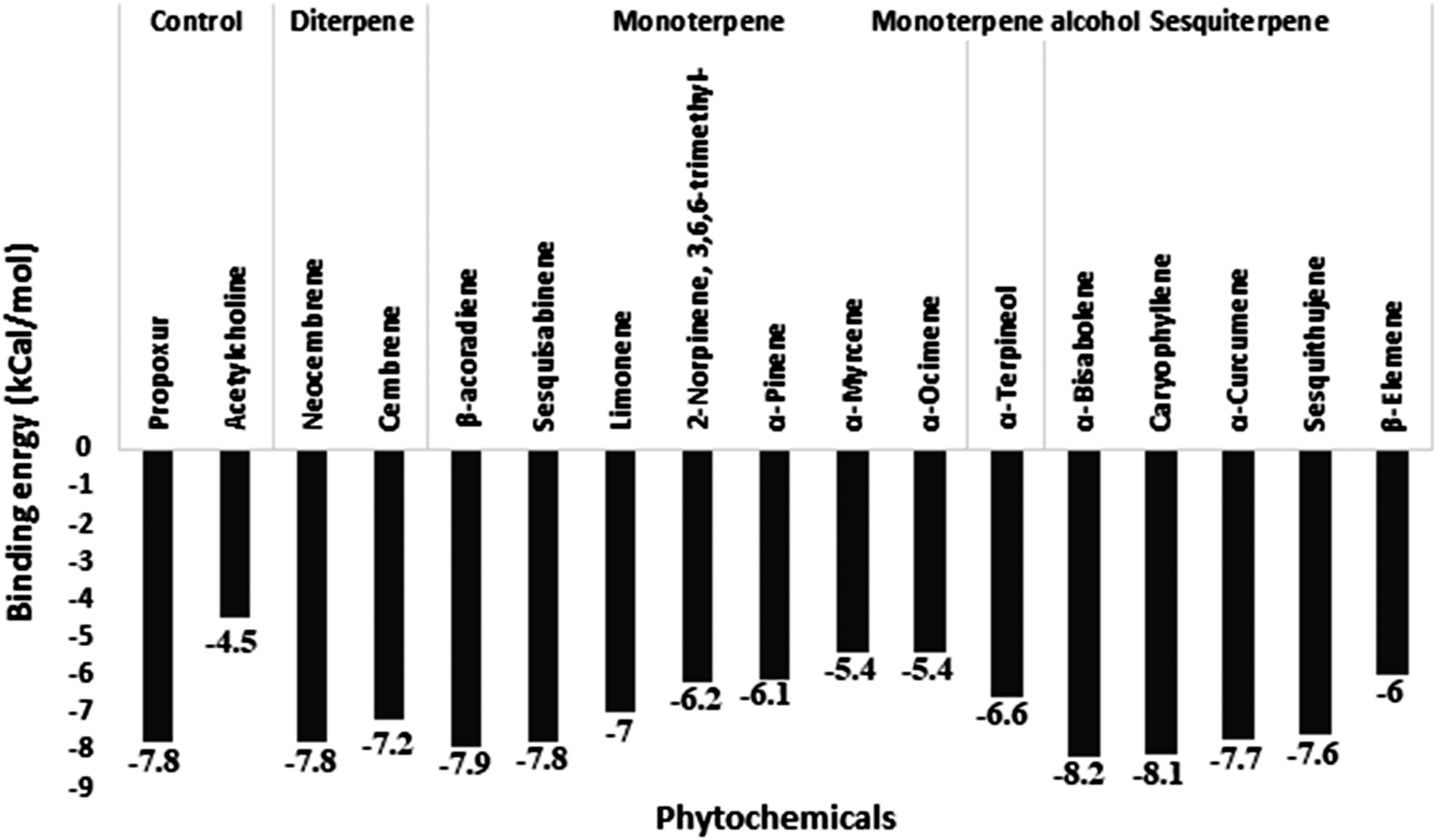
Figure 2 Molecular docking binding energy of Commiphora wightii phytochemicals against the acetylcholinesterase receptor.
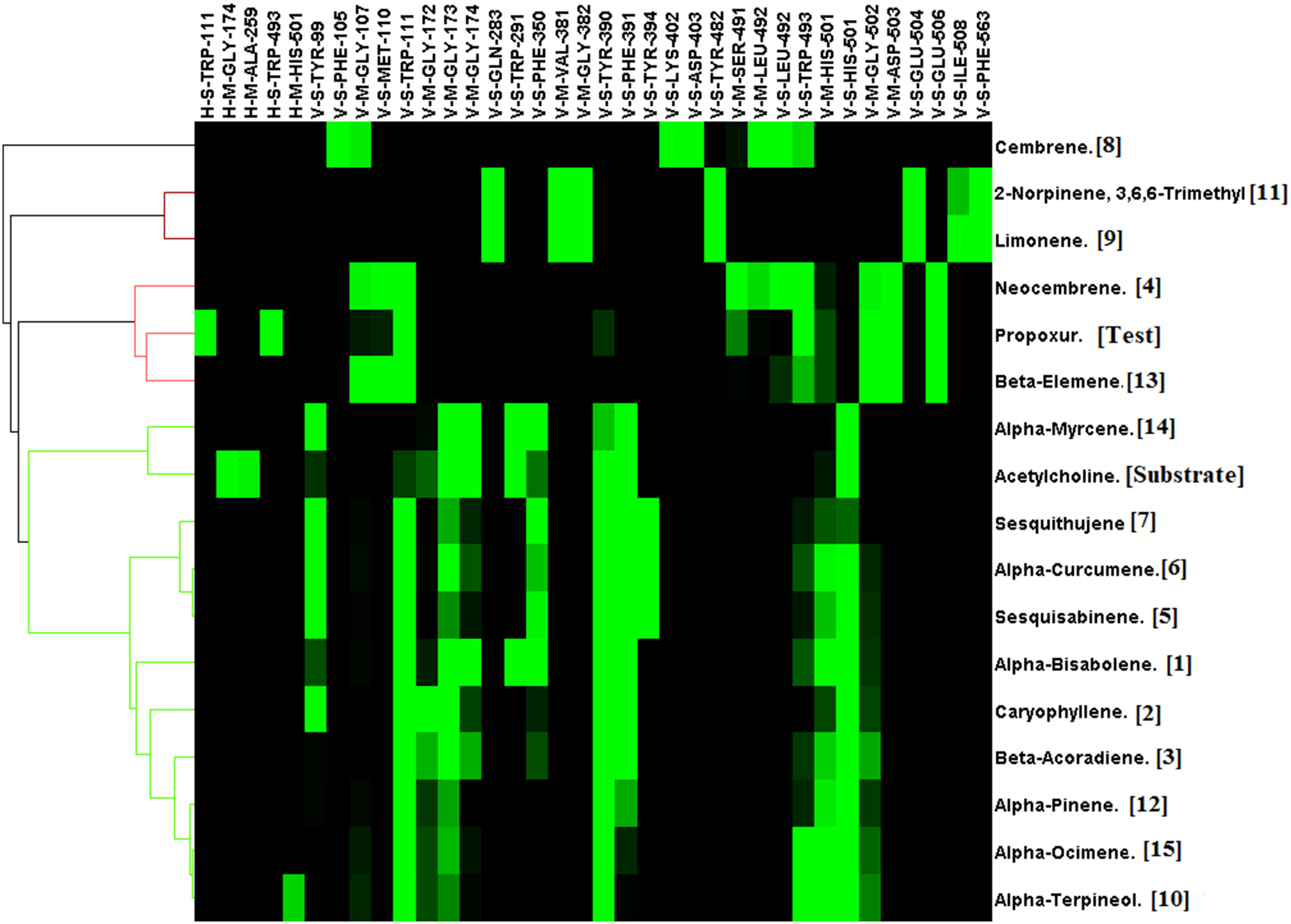
Figure 3 Interaction profile of Commiphora wightii phytochemicals best pose against the acetylcholinesterase receptor. Numbers in the bracket indicate the docking rank, black color indicates zero value, and green color indicates negative energy.
GABA receptor molecular docking
In addition to 15 phytochemicals for GABA receptors, natural substrate Gamma-Aminobutyric Acid and organochlorine insecticide endosulfan were also identified. The docking binding energy for phytochemicals ranged between -2.5 and -7.4. The minimum docking binding energy was obtained for Cembrene (-7.4), Bisabolene (-7.2), and Neocembrene (-7.2), followed by other compounds (Figure 4). The interaction analysis for GABA receptor molecular docking against phytochemicals revealed four distinct clusters (Figure 5). Substrate Gamma-Aminobutyric Acid was grouped in a unique cluster with a single member, and the inhibitor endosulfan was grouped in a different cluster with three other phytochemicals. Cembrene, the top-ranking compound, was also classified in a unique cluster with only one member, but it shared a few binding interactions with the inhibitor endosulfan. Conversely, Neocembrene, which is ranked second, pertains to the endosulfan cluster and exhibits a comparable binding interaction. On the contrary, the third compound with the highest rank, Bisabolene, exhibited a binding interaction that was comparable to that of Gamma-Aminobutyric Acid and was also found to be in close proximity (Figure 5).
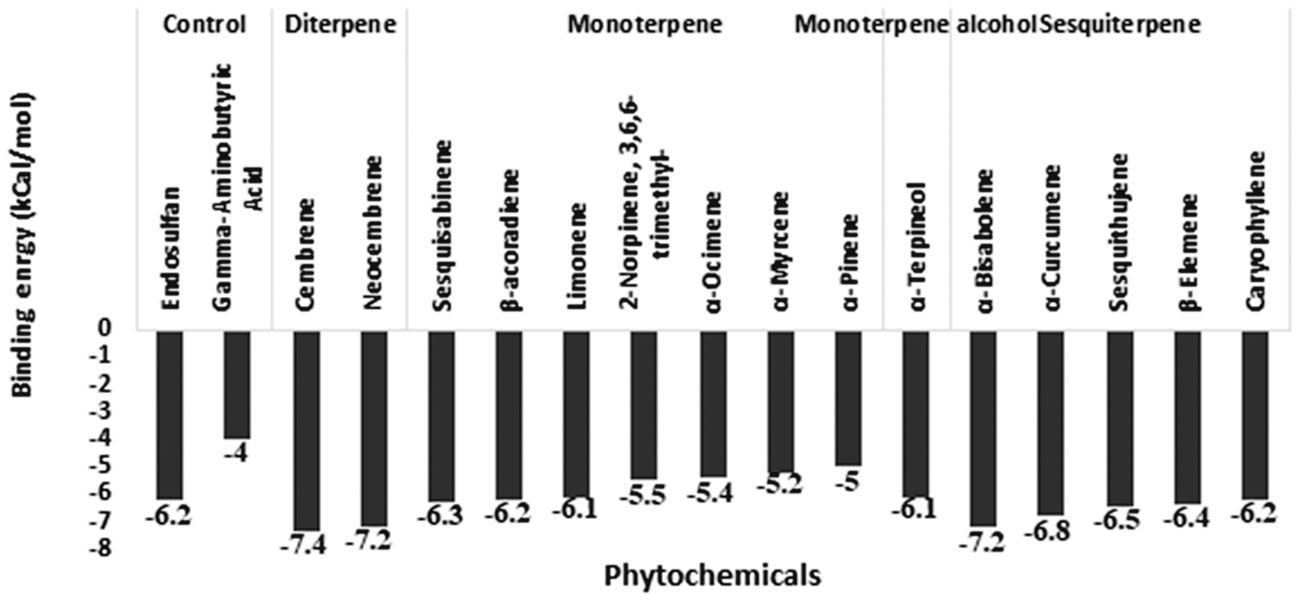
Figure 4 Molecular docking binding energy of Commiphora wightii phytochemicals against the GABA receptor.
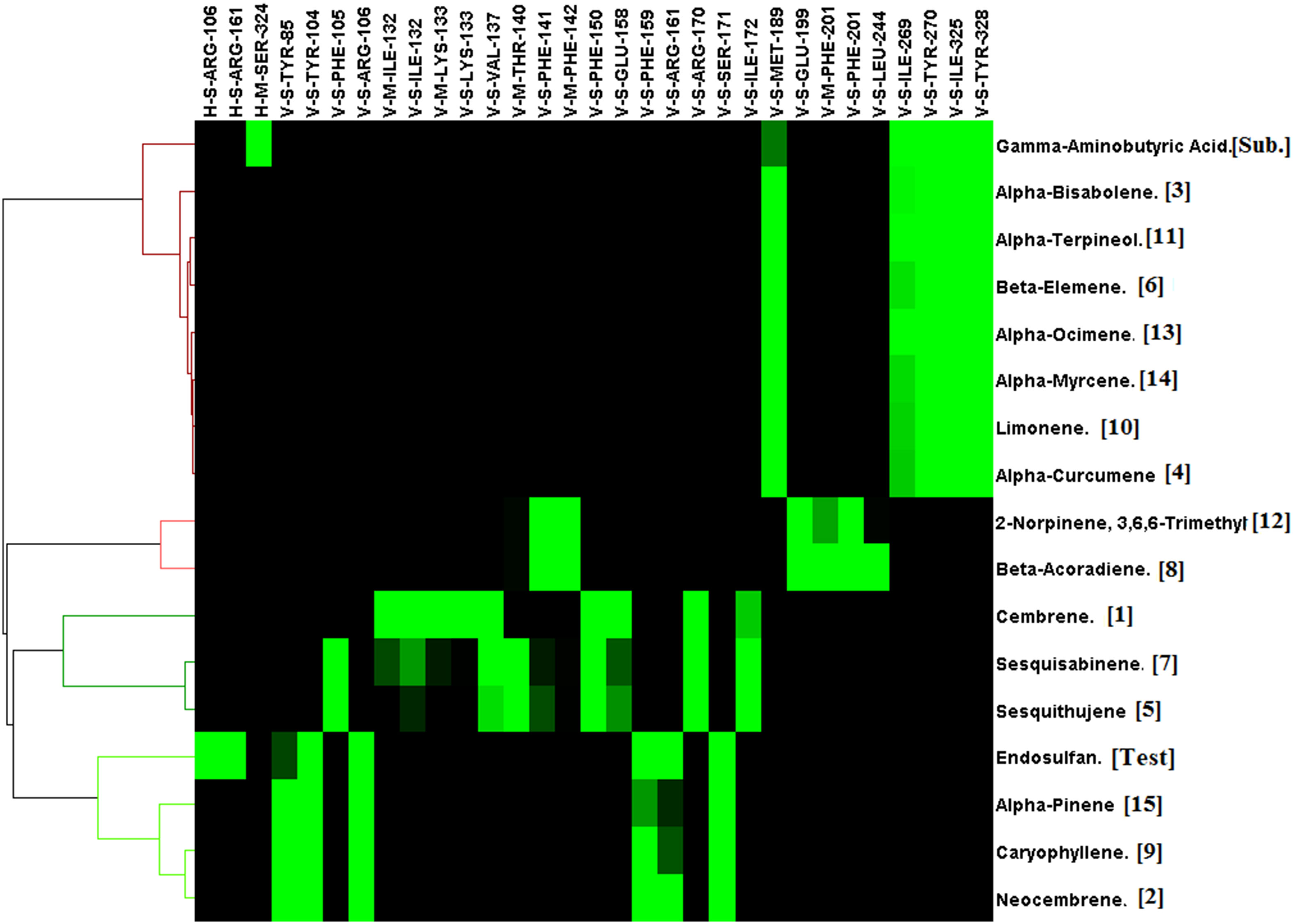
Figure 5 Interaction profile of Commiphora wightii phytochemicals best pose against the GABA receptor. Numbers in the bracket indicate the docking rank, black color indicates zero value, and green color indicates negative energy.
Octopamine receptor docking
For the octopamine receptor protein docking experiment, the binding energy ranged from –4.5 to -6.8 (Figure 6). The overall binding interaction for octopamine was relatively lower compared to that of acetylcholinesterase and GABA receptor, which may be due to the lower quality of the model. The minimum docking binding energy was obtained for 2-Norpinene, 3,6,6-trimethyl (-6.8), α-Bisabolene (-6.4), and Cembrene (-6.4), followed by other compounds (Figure 6). The interaction analysis for Octopamine receptor molecular docking against phytochemicals revealed four clusters, generated with a single member of with first-ranking 2-Norpinene, 3,6,6-trimethyl, α-Curcumene, β-Elemene, and inhibitor Formamidine hydrochloride. Second- and third-ranking compounds α-Bisabolene and Cembrene are also assorted in a separate group (Figure 7). In contrast, substrate octopamine was grouped together with the other three phytochemicals with a lower rank. The substrate octopamine had a lower binding energy of -5.4 when compared to the -2.5 of acaricide insecticide Formamidine hydrochloride.
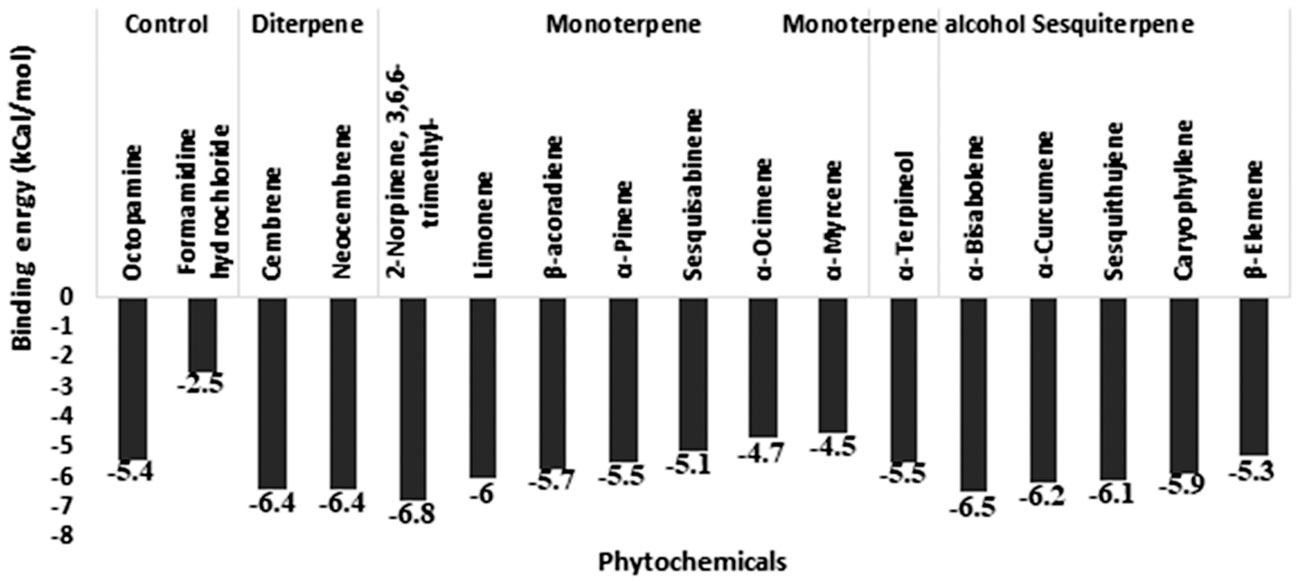
Figure 6 Molecular docking binding energy of Commiphora wightii phytochemicals against the octopamine receptor.
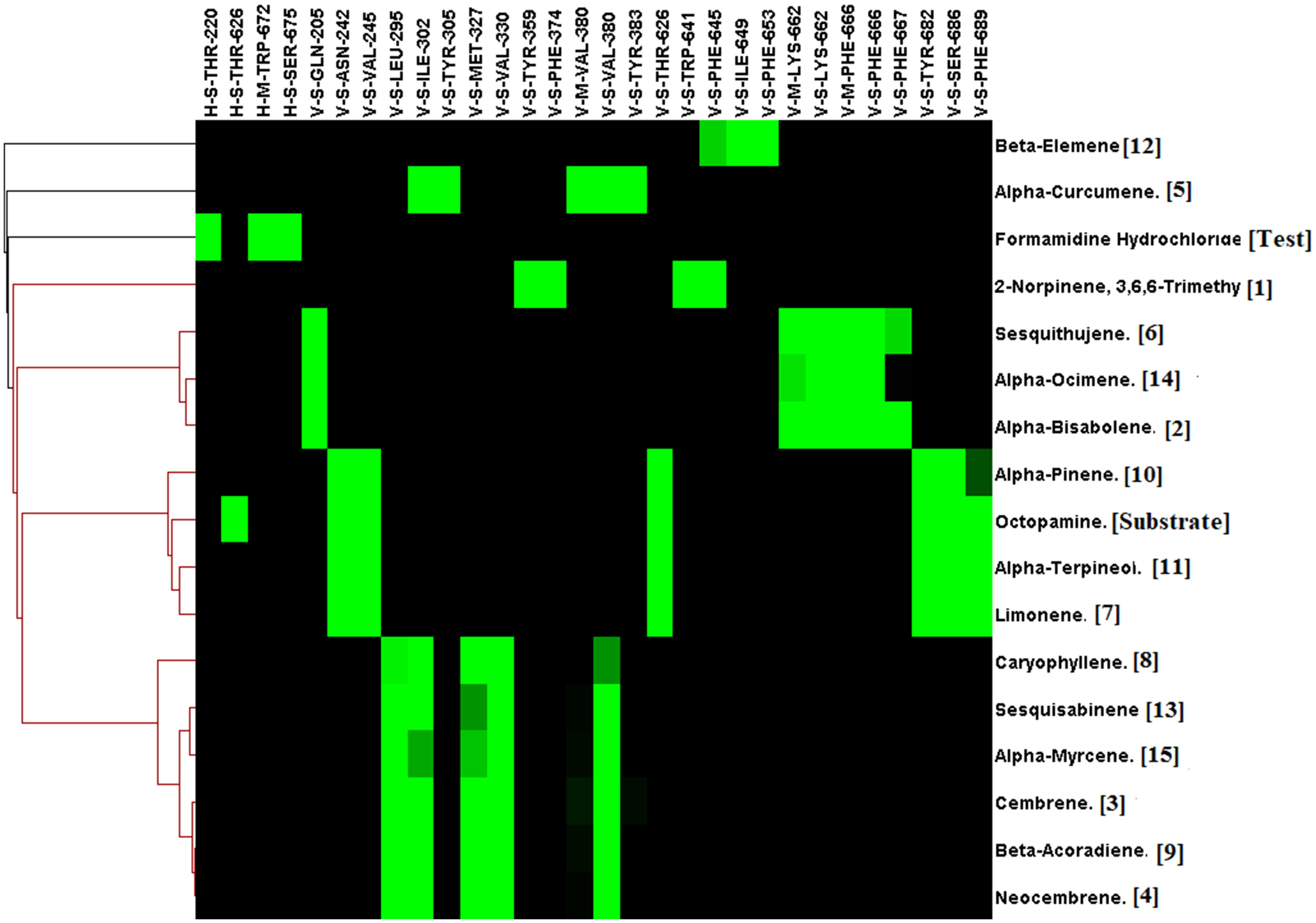
Figure 7 Interaction profile of Commiphora wightii phytochemicals best pose against the octopamine receptor. Numbers in the bracket indicate the docking rank, black color indicates zero value, and green color indicates negative energy.
Grouping of the three top-ranking compounds against generated leads the group of a total of six compounds, α-Bisabolene, Caryophyllene, Neocembrene, β-Acoradiene, 2-Norpinene, and 3,6,6-trimethyl that had better binding energy in comparison to their natural substrate and commercial inhibitor.
Discussion
Since ancient times, extracts prepared from different parts of plants like flowers, bark, roots, leaves, and seeds have been used as insecticides (Sir et al., 2020). Various studies have reported the efficacy of plant extracts against mosquitoes. Studies have proven that these natural extracts have certain compounds that help in controlling the pest population (Jamiołkowska and Kopacki, 2020). These extracts have certain volatile compounds that can act on insects as insect repellents, insecticides, insect antifeedants, insect growth inhibitors, acetylcholinesterase enzyme inhibitors, oviposition inhibitors, ovicides, or insect growth-reducing agents (Hikal et al., 2017).
Numerous studies have reported the larvicidal activity of Commiphora species. The larvicidal activity of the following Commiphora species against different species of mosquitoes has been reported that includes oil-resins of Commiphora molmol (1,500 ppm acetone and aqueous extracts) against mosquito larvae of Culex pipiens (Baz et al., 2021); arabinofuranosidetridecanol from C. merkeri against A. aegypti (LC50 = 40.66 µg mL−1), Anopheles gambiae (LC50 = 22.86 µg mL−1), and C. quinquefasciatus (LC50 = 15.88 µg mL−1) (Samwel et al., 2021); essential oil of C. erythraea against C. restuans, C. pipiens, and A. aegypti (Muturi et al., 2020). As per the researchers, LC50<50 µg mL−1 is very active; LC50 50 µg mL−1–100 µg mL−1 is active, and LC50 >100 µg mL−1 is a weak or inactive larvicidal compound (Milugo et al., 2021).
It has been suggested that monoterpenoids including Terpineol are very effective in inhibiting insect reproduction (Regnault-Roger et al., 2004), and the occurrence of monoterpenes and sesquiterpenes leads to fumigant toxicity (Hamraoui and Regnault-Roger, 1997; Regnault-Roger et al., 2004). Gum extracts are known as potential sources of larvicides due to their ability to alter the activity of the insect’s acetylcholinesterase enzyme (Ashokkumar et al., 2021), which is required for neuro-neuronal and neuromuscular junctions in insects. Among these, Limonene and α-Pinene are the most effective compounds that alter the activity of the acetylcholinesterase enzyme (Marei et al., 2012; Vieira et al., 2018).
Monoterpenes have natural pesticidal properties that are effective, safe, and biodegradable, which make them the best alternative to synthetic pesticides. Various pesticidal activities of monoterpenes, including bactericidal (Badawy et al., 2019), fungicidal, herbicidal (Liu et al., 2022), and insecticidal properties (Gad et al., 2022), are known. The larvicidal activity of monoterpenes against certain mosquito species (Gad et al., 2022), including toxic effects on A. aegypti (Giang An et al., 2020) and anti-oviposition effects on A. aegypti (Waliwitiya et al., 2009), has also been reported. It has been observed that monoterpenes and terpenoids are efficient acetylcholinesterase enzyme inhibitors in different insects and larvae (Waliwitiya et al., 2009), whereas diterpenes like Cembrene have antifeed activity against herbivores (Sun et al., 2019).
Previous research conducted on 24 species of the genus Commiphora, including C. wightii, identified 230 phytoconstituents, including phytoconstituents α-Bisabolene, Caryophyllene, Cembrene, Limonene, α-Pinene, β-Elemene, α-Myrcene, and α-Ocimene (Selvamani et al., 2008), which have also been obtained in the present study. However, Sesquisabinene and 2-Norpinene, 3,6,6-Trimethyl have not been reported from any Commiphora species so far.
The research conducted on five Piper species (Piper nigrum, Piper mutabile, Piper longum, Piper montium, and Piper caninum) showed excellent larvicidal activities with LC50 and LC90 values<10 μg mL-1 and were correlated with β-Caryophyllene, β-Bisabolene, β-Pinene, and α-Pinene concentrations (Huong et al., 2019), which support our molecular docking findings for β-Caryophyllene and β-Bisabolene.
α-Bisabolene was best docked against the acetylcholinesterase with binding energy −8.2. It has been observed that niloticin (−8.4 kcal/mol) had higher binding affinities and energy values than temephos (−4.75 kcal/mol), which is a commercial larvicide (Reegan et al., 2016). Similarly, the inhibitory effect of natural alkaloids on the acetylcholinesterase present in A. aegypti had the best fit into the AChE1 binding pocket with a minimum binding energy of −8.13 (Balachandran et al., 2021).
The gamma-aminobutyric acid receptor, also known as the GABA receptor, has four distinct but overlapping and coupled targets of pesticide action. These targets are associated with very little or no cross-resistance (Setlur et al., 2023). In the present research work, besides acetylcholinesterase, α-Bisabolene also ranked second and third for the octopamine receptor and GABA receptor, indicating its possibility to act as a multitarget ligand inhibiting the nervous system. Thus, α-Bisabolene emerged as one of the potent multitarget inhibitors for application as a bio-insecticide to control the resistant A. aegypti.
Bisabolene, a plant-based Sesquiterpene, is used as a precursor for the synthesis of several industrially relevant chemicals (Zhao et al., 2021). Bisabolene’s low plant abundance makes its isolation uneconomical. Therefore, industrial Bisabolene production using synthetic biology and metabolic engineering to create microbial cell factories is more competitive and environmentally sustainable. In this proof-of-principle engineering, the oleaginous yeast Yarrowia lipolytica was explored to produce α- and β-Bisabolene by heterologously expressing the synthase genes from Abies grandis, Zingiber officinale, and Helianthus annuus.
The current research findings suggest that C. wightii could be beneficial as a biological agent in the fight against A. aegypti. It was found that Bisabolene could be one of the key larvicidal compounds that employ a multitarget strategy and act on proteins associated with the nervous system of A. aegypti larvae. Even in cases where A. aegypti larva has developed resistance to commercially used pesticides, being a natural compound, Bisabolene can be effective against them.
Conclusion
A. aegypti mosquito, also known as the yellow fever mosquito, is a potent transmitter of several vector-borne diseases such as dengue fever, chikungunya, Zika fever, the Mayaro virus, and yellow fever, in addition to other pathogens. Guggulu, also known as C. wightii, has recently been recognized as a reliable source of traditional medicines for the treatment of a variety of conditions, including inflammation, arthritis, obesity, microbial infection, wounds, pain, fractures, tumors, and gastrointestinal diseases. The current investigation posed an additional application of C. wightii gum resin for the environment-friendly biological control of A. aegypti larva (fourth instar) due to its multitarget action. A total of 15 volatile compounds were identified belonging to diterpene, monoterpene, monoterpene alcohol, and sesquiterpene groups. A total of 230 phytoconstituents have been identified from Commiphora species, out of which five unique compounds were identified from C. wightii, which were Limonene, α-Terpineol, α-Pinene, α-Myrcene, and Cembrene. The molecular docking study of acetylcholinesterase, GABA receptor, and octopamine receptor revealed certain compounds of the C. wightii gum resin having very low binding energy, revealing their role in larvicidal properties. These compounds are known to act upon the nervous system leading to the ultimate death of the larvae.
Data availability statement
The datasets presented in this study can be found in online repositories. The names of the repository/repositories and accession number(s) can be found in the article/Supplementary Material.
Ethics statement
The article presents research on animals that do not require ethical approval for their study.
Author contributions
Conceptualization and supervision: AP, JGT, VY, and DS. Investigation and methodology: KP, DA, RVP, VM, and SW. Original draft preparation: AP, VY, KP, and JGT. Review and final editing: RP, AP, JGT, and JT. All authors contributed to the article and approved the submitted version.
Acknowledgments
The authors are grateful to the Department of Life Sciences, Hemchandracharya North Gujarat University, Patan, Gujarat, India, for providing the laboratory facilities to carry out the research work.
Conflict of interest
The authors declare that the research was conducted in the absence of any commercial or financial relationships that could be construed as a potential conflict of interest.
Publisher’s note
All claims expressed in this article are solely those of the authors and do not necessarily represent those of their affiliated organizations, or those of the publisher, the editors and the reviewers. Any product that may be evaluated in this article, or claim that may be made by its manufacturer, is not guaranteed or endorsed by the publisher.
Supplementary material
The Supplementary Material for this article can be found online at: https://www.frontiersin.org/articles/10.3389/fpls.2023.1220339/full#supplementary-material
References
Acock, M. C., Wang, Z., Acock, B., Jones, R., Abba, E., Shehu, Z., et al. (2022). Plant natural products for the control of Aedes aEgypti: The main vector of important arboviruses. PloS One 14, 1–11. doi: 10.1016/j.foodres.2011.11.017
Ashokkumar, K., Murugan, M., Dhanya, M. K., Pandian, A., Warkentin, T. D. (2021). Phytochemistry and therapeutic potential of black pepper [Piper nigrum (L.)] essential oil and piperine: a review. Clin. Phytosci. 7, 52. doi: 10.1186/s40816-021-00292-2
Badawy, M. E. I., Marei, G. I., Rabea, E. I., Taktak, N. E. M. (2019). Antimicrobial and antioxidant activities of hydrocarbon and oxygenated monoterpenes against some foodborne pathogens through in vitro and in silico studies. Pestic Biochem. Physiol. 158, 185–200. doi: 10.1016/j.pestbp.2019.05.008
Balachandran, C., Anbalagan, S., Kandeepan, C., Arun Nagendran, N., Jayakumar, M., Fathi Abd Allah, E., et al. (2021). Molecular docking studies of natural alkaloids as acetylcholinesterase (AChE1) inhibitors in Aedes aEgypti. J. Asia Pac Entomol 24, 645–652. doi: 10.1016/j.aspen.2021.05.011
Baz, M. M., Hegazy, M. M., Khater, H. F., El-Sayed, Y. A. (2021). Comparative evaluation of five oil-resin plant extracts against the mosquito larvae, Culex pipiens say (Diptera: Culicidae). Pak Vet. J. 41, 191–196. doi: 10.29261/pakvetj/2021.010
Benelli, G., Mehlhorn, H. (2016). Declining malaria, rising of dengue and Zika virus: insights for mosquito vector control. Parasitol. Res. 115, 1747–1754. doi: 10.1007/S00436-016-4971-Z/FIGURES/6
Bharati, M., Saha, D. (2018). Multiple insecticide resistance mechanisms in primary dengue vector, Aedes aEgypti (Linn.) from dengue endemic districts of sub-Himalayan West Bengal, India. PloS One 13, e0203207. doi: 10.1371/JOURNAL.PONE.0203207
Braga, I. A., Lima, J. B. P., Da Silva Soares, S., Valle, D. (2004). Aedes aEgypti resistance to temephos during 2001 in several municipalities in the states of Rio de Janeiro, Sergipe, and Alagoas, Brazil. Mem Inst Oswaldo Cruz 99, 199–203. doi: 10.1590/S0074-02762004000200015
Chandran, H., Meena, M., Barupal, T., Sharma, K. (2020). Plant tissue culture as a perpetual source for production of industrially important bioactive compounds. Biotechnol. Rep. 26, e00450. doi: 10.1016/j.btre.2020.e00450
Dusfour, I., Thalmensy, V., Gaborit, P., Issaly, J., Carinci, R., Girod, R. (2011). Multiple insecticide resistance in aedes aEgypti (Diptera: Culicidae) populations compromises the effectiveness of dengue vector control in French Guiana. Mem Inst Oswaldo Cruz 106, 346–352. doi: 10.1590/S0074-02762011000300015
Eberhardt, J., Santos-Martins, D., Tillack, A. F., Forli, S. (2021). AutoDock Vina 1.2.0: new docking methods, expanded force field, and python bindings. J. Chem. Inf Model. 61, 3891–3898. doi: 10.1021/acs.jcim.1c00203
Gad, H. A., Ramadan, G. R. M., El-Bakry, A. M., El-Sabrout, A. M., Abdelgaleil, S. A. M. (2022). Monoterpenes: Promising natural products for public health insect control- A review. Int. J. Trop. Insect Sci. 42, 1059–1075. doi: 10.1007/s42690-021-00692-4
Giang An, N. T., Huong, L. T., Satyal, P., Tai, T. A., Dai, D. N., Hung, N. H., et al. (2020). Mosquito larvicidal activity, antimicrobial activity, and chemical compositions of essential oils from four species of myrtaceae from central Vietnam. Plants 9. doi: 10.3390/plants9040544
Guzman, M. G., Harris, E. (2015). Dengue. Lancet 385 (9966), 453–465. doi: 10.1016/S0140-6736(14)60572-9
Hamraoui, A., Regnault-Roger, C. (1997). Comparaison des activités insecticides des monoterpènes sur deux espèces d’insectes ravageurs des cultures: Ceratitis capitata et Rhopalosiphum padi. Acta Botanica Gallica 144, 413–417. doi: 10.1080/12538078.1997.10515780
Harbach, R. E., Besansky, N. J. (2014). Mosquitoes. Curr. Biol. 24 (1). 14–15. doi: 10.1016/J.CUB.2013.09.047
Hemingway, J., Hawkes, N. J., McCarroll, L., Ranson, H. (2004). The molecular basis of insecticide resistance in mosquitoes. Insect Biochem. Mol. Biol. 34 (7), 653–665. doi: 10.1016/j.ibmb.2004.03.018
Hikal, W. M., Baeshen, R. S., Said-Al Ahl, H. A. H. (2017). Botanical insecticide as simple extractives for pest control. Cogent Biol. 3, 1404274. doi: 10.1080/23312025.2017.1404274
Hsu, K. C., Chen, Y. F., Lin, S. R., Yang, J. M. (2011). Igemdock: A graphical environment of enhancing gemdock using pharmacological interactions and post-screening analysis. BMC Bioinf. 12, 1–11. doi: 10.1186/1471-2105-12-S1-S33
Huong, L. T., Hung, N. H., Dai, D. N., Tai, T. A., Hien, V. T., Satyal, P., et al. (2019). Chemical compositions and mosquito larvicidal activities of essential oils from piper species growing wild in central Vietnam. Molecules 24, 3871. doi: 10.3390/MOLECULES24213871
Jamiołkowska, A., Kopacki, M. (2020). “Chapter 5 - natural compounds against plant pests and pathogens,” in Natural Remedies for Pest, Disease and Weed Control. Eds. Egbuna, C., Sawicka, B. (Cambridge, Massachusetts, United States: Academic Press), 55–63.
Kaavya, K., Tharakan, J., Joshi, C. O., Aneesh, E. M. (2022). Role of vertically transmitted viral and bacterial endosymbionts of Aedes mosquitoes. Does Paratransgenesis influence vector-borne disease control? Symbiosis 86, 139–153. doi: 10.1007/s13199-022-00836-1
Kantheti, P., Padma, A. (2017). Ethnobotanical tribal practices for mosquito repellency followed by people of north India. J. Pharmacogn Phytochem. 6, 942–944.
Kumar, S., Warikoo, R., Wahab, N. (2010). Larvicidal potential of ethanolic extracts of dried fruits of three species of peppercorns against different instars of an Indian strain of dengue fever mosquito, Aedes aEgypti L. (Diptera: Culicidae). Parasitol. Res. 107, 901–907. doi: 10.1007/S00436-010-1948-1
Liu, Z., Li, Q. X., Song, B. (2022). Pesticidal activity and mode of action of monoterpenes. J. Agric. Food Chem. 70, 4556–4571. doi: 10.1021/acs.jafc.2c00635
Marei, G. I., Abdel Rasoul, M. A., Abdelgaleil, S. A. M. (2012). Comparative antifungal activities and biochemical effects of monoterpenes on plant pathogenic fungi. Pestic Biochem. Physiol. 103, 56–61. doi: 10.1016/j.pestbp.2012.03.004
Medronho, R. D. A. (2008). Dengue no Brasil: Desafios para o seu controle. Cad Saude Publica 24, 948–949. doi: 10.1590/S0102-311X2008000500001
Milugo, T. K., Tchouassi, D. P., Kavishe, R. A., Dinglasan, R. R., Torto, B. (2021). Naturally occurring compounds with larvicidal activity against malaria mosquitoes. Front. Trop. Dis. 2. doi: 10.3389/fitd.2021.718804
Muturi, E. J., Hay, W. T., Doll, K. M., Ramirez, J. L., Selling, G. (2020). Insecticidal activity of commiphora erythraea essential oil and its emulsions against larvae of three mosquito species. J. Med. Entomol 57, 1835–1842. doi: 10.1093/JME/TJAA097
Reegan, A. D., Stalin, A., Paulraj, M. G., Balakrishna, K., Ignacimuthu, S., Al-Dhabi, N. A. (2016). In silico molecular docking of niloticin with acetylcholinesterase 1 (AChE1) of Aedes aEgypti L. (Diptera: Culicidae): a promising molecular target. Med. Chem. Res. 7, 1411–1419. doi: 10.1007/S00044-016-1579-X
Regnault-Roger, C., Ribodeau, M., Hamraoui, A., Bareau, I., Blanchard, P., Gil-Munoz, M.-I., et al. (2004). Polyphenolic compounds of Mediterranean Lamiaceae and investigation of orientational effects on Acanthoscelides obtectus (Say). J. Stored Prod. Res. 40, 395–408. doi: 10.1016/S0022-474X(03)00031-6
Samwel, B., Innocent, E., Machumi, F., Kisinza, W. N., Heydenreich, M. (2021). Two mosquito larvicidal arabinofuranosidetridecanol from Commiphora merkeri exudate. Nat. Prod. Res. 36, 2821–2829. doi: 10.1080/14786419.2021.1931866
Sarup, P., Bala, S., Kamboj, S. (2015). Pharmacology and phytochemistry of oleo-gum resin of commiphora wightii (Guggulu). Scientifica (Cairo) 2015, 1–14. doi: 10.1155/2015/138039
Selvamani, P., Gupta, J. K., Sen, D. J. (2008). Pharmacognostical standardization of Commiphora berryi (Arn) Engl and phytochemical studies on its crude extracts. Article Afr. J. Pharm. Pharmacol. 3, 37–046. doi: 10.5897/AJPP.9000219
Setlur, S., Karunakaran, C., Pandey, S., Sarkar, M., NIranjan, V. (2023). Molecular interaction studies of thymol via molecular dynamic simulations and free energy calculations using multi-target approach against Aedes aEgypti proteome to decipher its role as mosquito repellent. Mol. Simul. 49, 325–340. doi: 10.1080/08927022.2022.2159054
Sir, K., Ali, E., Altaib, T., Salih, A., Daffalla, H. M. (2020). In vitro Phytochemical, Larvicidal and Antimicrobial Activities of Gum Arabic Extract. Walailak Journal of Science and Technology (WJST). 17(3), 192–199. doi: 10.48048/wjst.2020.5540
Sun, X., Geng, Y., Wang, X., Qin, D., Yu, J. (2019). Cembrane-type diterpenoids from the gum resin of Boswellia carterii and their biological activities. RSC Adv. 10, 746–755. doi: 10.1039/c9ra09776g
Udayanga, L., Ranathunge, T., Iqbal, M. C. M., Abeyewickreme, W., Hapugoda, M. (2019). Predatory efficacy of five locally available copepods on Aedes larvae under laboratory settings: An approach towards bio-control of dengue in Sri Lanka. PloS One 14, e0216140. doi: 10.1371/JOURNAL.PONE.0216140
Van Den Dool, H. A. N. D., Kratz, P. D. (1963). A generalization of the retention index system including linear temperature programmed gas-liquid partition chromatography. J. Chromatogr. 11, 463–471. doi: 10.1016/S0021-9673(01)80947-X
Vasishth, A., Guleria, V. (2017). Standardized gum tapping techniques to maximize yield from high-value Indian tree, Sterculia urens. J. For Res. (Harbin) 28, 615–619. doi: 10.1007/s11676-016-0315-1
Vieira, A. J., Beserra, F. P., Souza, M. C., Totti, B. M., Rozza, A. L. (2018). Limonene: Aroma of innovation in health and disease. Chem. Biol. Interact. 283, 97–106. doi: 10.1016/j.cbi.2018.02.007
Waliwitiya, R., Kennedy, C. J., Lowenberger, C. A. (2009). Larvicidal and oviposition-altering activity of monoterpenoids, trans-anithole and rosemary oil to the yellow fever mosquito Aedes aEgypti (Diptera: Culicidae). Pest Manag Sci. 65, 241–248. doi: 10.1002/ps.1675
Waterhouse, A., Bertoni, M., Bienert, S., Studer, G., Tauriello, G., Gumienny, R., et al. (2018). SWISS-MODEL: Homology modelling of protein structures and complexes. Nucleic Acids Res. 46, W296–W303. doi: 10.1093/nar/gky427
Yang, T., Lu, L., Fu, G., Zhong, S., Ding, G., Xu, R., et al. (2009). Epidemiology and vector efficiency during a dengue fever outbreak in Cixi, Zhejiang Province, China. J. Vector Ecol. 34, 148–154. doi: 10.1111/J.1948-7134.2009.00018.X
Keywords: Aedes aegypti, crude extracts, larvicidal activity, volatile compounds, plant gum extract, viral vector
Citation: Patel K, Akbari D, Pandya RV, Trivedi J, Mevada V, Wanale SG, Patel R, Yadav VK, Tank JG, Sahoo DK and Patel A (2023) Larvicidal proficiency of volatile compounds present in Commiphora wightii gum extract against Aedes aegypti (Linnaeus, 1762). Front. Plant Sci. 14:1220339. doi: 10.3389/fpls.2023.1220339
Received: 10 May 2023; Accepted: 07 August 2023;
Published: 30 August 2023.
Edited by:
Petr Maděra, Mendel University in Brno, CzechiaReviewed by:
Lucie Vanickova, Mendel University in Brno, CzechiaSeema Ramniwas, Chandigarh University, India
Copyright © 2023 Patel, Akbari, Pandya, Trivedi, Mevada, Wanale, Patel, Yadav, Tank, Sahoo and Patel. This is an open-access article distributed under the terms of the Creative Commons Attribution License (CC BY). The use, distribution or reproduction in other forums is permitted, provided the original author(s) and the copyright owner(s) are credited and that the original publication in this journal is cited, in accordance with accepted academic practice. No use, distribution or reproduction is permitted which does not comply with these terms.
*Correspondence: Ashish Patel, dW5pLmFzaGlzaEBnbWFpbC5jb20=; Dipak Kumar Sahoo, ZHNhaG9vQGlhc3RhdGUuZWR1; Jigna G. Tank, amlnbmFndGFua0BnbWFpbC5jb20=; Virendra Kumar Yadav, eWFkYXZhOTRAZ21haWwuY29t