- 1Department of Phytology, Faculty of Forestry, Technical University in Zvolen, Zvolen, Slovakia
- 2Centre for Applied Entomology and Parasitology, School of Life Sciences, Keele University, Newcastle-under-Lyme, Staffordshire, United Kingdom
- 3Department of Plant Biology, Faculty of Biological Sciences, Tarbiat Modares University, Tehran, Iran
- 4Department of Integrated Forest and Landscape Protection, Faculty of Forestry, Technical University in Zvolen, Zvolen, Slovakia
- 5Department of Chemistry and Chemical Technologies, Faculty of Wood Sciences and Technology, Technical University in Zvolen, Zvolen, Slovakia
Several polysaccharides augment plant growth and productivity and galvanise defence against pathogens. Such elicitors have ecological superiority over traditional growth regulators, considering their amplified biocompatibility, biodegradability, bioactivity, non-toxicity, ubiquity, and inexpensiveness. Chitosan is a chitin-derived polysaccharide that has recently been spotlighted among plant scientists. Chitosan supports plant growth and development and protects against microbial entities such as fungi, bacteria, viruses, nematodes, and insects. In this review, we discuss the current knowledge of chitosan’s antimicrobial and insecticidal potential with recent updates. These effects are further explored with the possibilities of chitosan’s active correspondence with phytohormones such as jasmonic acid (JA), salicylic acid (SA), indole acetic acid (IAA), abscisic acid (ABA), and gibberellic acid (GA). The stress-induced redox shift in cellular organelles could be substantiated by the intricate participation of chitosan with reactive oxygen species (ROS) and antioxidant metabolism, including hydrogen peroxide (H2O2), superoxide dismutase (SOD), catalase (CAT), and peroxidase (POD). Furthermore, we propose how chitosan could be intertwined with cellular signalling through Ca2+, ROS, nitric oxide (NO), transcription factors (TFs), and defensive gene activation.
1 Introduction
Chitosan is a polysaccharide derived from the second most abundant natural biopolymer on Earth, i.e., chitin. It is a linear cationic polysaccharide that is made up of β-(1,4)-joined N-acetyl-d-glucosamine (GlcNAc) and d-glucosamine (GLcN) units. This biopolymer is obtained commercially by the N-deacetylation of chitin from the crustacean exoskeleton. Some mushrooms, green algae, and yeasts can also biosynthesise it. The chemical structure of chitosan can be determined through three characteristics: the degree of polymerisation (the length of the polymer), the degree of acetylation (the percentage of acetylated units), and the pattern of acetylation (the sequence of GLcN and GlcNAc units) (Shokri et al., 2021). The chemical properties of chitosan depend on the degree of deacetylation (DDA), i.e., the extent to which amine groups have substituted N-acetyl groups in chitin (Mukarram et al., 2022). The more deacetylated the product, the more positive the charges and the higher the solubility under acidic conditions. The less deacetylated the chitosan, the higher the solubility under neutral and alkaline conditions (Aranaz et al., 2021; Linhorst et al., 2021). Figure 1 depicts the chemical structure of chitosan and its common derivatives. Chitosan has several excellent chemical and physical properties that make it useful for many applications, e.g., biocompatibility, biodegradability, and antibacterial activity. These traits have led to using chitosan in various areas, including agriculture, food science, medicine, paper science and technologies, and environmental sciences (Morin-Crini et al., 2019). It also is used as a chelating agent because of its ability to bind with cholesterol, fats, proteins, and metal ions. In agriculture, chitosan has been used as a natural pesticide and plant growth agent due to its ability to improve plant growth and tolerance to environmental stresses. It effectively increases the yield and quality of crops such as vegetables, fruit, and ornamental plants. Chitosan has been used as a wound-healing promoter in medicine due to its ability to stimulate tissue regeneration and antibacterial properties (Croisier and Jérôme, 2013; Wang et al., 2020). In the food industry, chitosan has been used as a food stabiliser due to its ability to prevent food spoilage and improve the texture and stability of food products. In general, chitosan’s unique chemical structure and properties make it a versatile and valuable material with many applications (Ibrahim and El-Zairy, 2015; Rahman and Goswami, 2021; Shahrajabian et al., 2021; Gal et al., 2023).
Chitosan has been shown to act as a growth elicitor in plants, meaning that it can stimulate plant growth and improve crop yields. It could be due to the ability of chitosan to mimic the effects of plant growth regulators such as auxins and stimulate biosynthesis of other regulators such as ethylene (Pichyangkura and Chadchawan, 2015). In addition, chitosan has been shown to improve plant tolerance to environmental stresses such as drought, extreme temperatures, salinity, and heavy metal toxicity by enhancing the plant’s antioxidant defence system (Hidangmayum et al., 2019). The effectiveness of chitosan as a plant growth elicitor is significantly correlated with its chemical structure. The degree of polymerisation, degree of acetylation, the pattern of acetylation, and monomer unit sequences are all essential structural factors (Hosseinnejad and Jafari, 2016; Fan et al., 2023). Applying chitosan to plant roots, leaves, or seeds can increase plant height, root length, biomass production, and the improvement in crop yields. Chitosan has been shown to improve crop quality, including increased fruit size, vitamin and mineral content, and shelf life (Chamnanmanoontham et al., 2015; Faqir et al., 2021). In addition, it has also been used in the bioremediation of contaminated soils, as it can help to decrease the levels of heavy metals and other toxic ingredients in the soil. In general, the ability of chitosan to act as a growth elicitor in plants makes it a valuable compound for improving crop yields and quality and promoting sustainable agricultural methods (Faqir et al., 2021; Ingle et al., 2022; Kugarajah et al., 2023).
2 Chitosan against plant pathogens and pests
Chitosan has been used as an artificial plant defence elicitor for over two decades. It has been tested in various economically essential crops against various plant pathogens (Rabea et al., 2003). Many laboratory studies have investigated the correspondence of chitosan with pathogens and showed that it has a critical role in the triggering plant defence against microbes (fungi, oomycetes, bacteria, and viruses), nematodes, and insect herbivores (El Hadrami et al., 2010; Badawy and Rabea, 2011; Hadwiger, 2013). Chitosan treatment induces several protective reactions inhibiting pathogens spread and providing systemic acquired resistance to plants (Rabea et al., 2003; Chakraborty et al., 2020), including biosynthesis of phytoalexins (soybean) and lignification (antibiotic and antifeeding compounds in plants) (Horbowicz et al., 2009). As discussed, the antimicrobial activity of chitosan depends upon several factors such as degree of polymerisation, type of chitosan (native or modified), the host, pH, molecular weight (MW), degree of acetylation, and climatic conditions (Tsai and Su, 1999). Several studies suggest that chitosan’s pentamers and heptamers show higher antifungal activity than larger ones (Rabea et al., 2003). In other forms, antipathogenic activity increases with MW (Kulikov et al., 2006).
Chitosan-induced plant protection can be broadly categorised into direct and indirect defence. Direct defence affects the performance of attacking pathogens, including reduced growth rate (El Hadrami et al., 2010), lower fecundity (larviposition) (Haas et al., 2018), and higher mortality of pests in plants treated with chitosan and its derivatives (Ibrahim et al., 2022), while indirect defence shows enhanced recruitment of natural enemies (Naree et al., 2021; El-Seedi et al., 2022). The chitosan-induced direct defence could be due to the production of antibiotic and defensive compounds, such as reactive oxygen species (ROS), phytoalexins, and phenolic acid (Yin et al., 2012; Waewthongrak et al., 2015; Singh et al., 2017), which affect the physiological status of plant pathogens and emit plant volatiles to repel herbivores (Walling, 2000; Zhang and Chen, 2009).
Moreover, chitosan-treated plants have been found to release a qualitatively and quantitatively different blend of volatile organic compounds (VOCs) compared to untreated plants (Zhang and Chen, 2009; Badiali et al., 2018). The change in quality and quantity of VOCs depends on the concentration and duration of the induced phase of chitosan application (Yin et al., 2012). For instance, chitosan treatment of crop plants altered VOC emission, enhancing indirect plant defence by repelling and/or recruiting the natural enemies of insect herbivores (Obara et al., 2002). In particular, rice leaves treated with chitosan release a high amount of linalool, methyl salicylate (MeSA), (Z)-3-hexen-1-ol, and β-caryophyllene (Cartwright et al., 1977; Bailey, 1982; Kodama et al., 1992; Obara et al., 2002). Exogenous application of chitosan elicits expression of defence-related genes (1-deoxy-d-xylulose 5-phosphate synthase (DXS), PaDXS2B, and PaDXS2B) in seedlings of Picea glauca (Lapointe et al., 2001). Similarly, tomato plants treated with chitosan show higher levels of jasmonic acid (JA) than untreated plants (Doares et al., 1995). Encapsulation of geraniol in chitosan controls whitefly (De Oliveira et al., 2018). Chitosan induces resistance in Solanum tuberosum against late blight by accumulating salicylic acid (SA) caused by activation of benzoate-2-hydroxylase and hydrolysis of SA conjugates in S. tuberosum (Ozeretskovskaya et al., 2006) (Table 1).
2.1 Against fungi and oomycetes
In an increasing population, the control of fungal plant disease has become vital to meet food supply needs. Phytopathogenic fungi are the most common plant pathogens and cause many severe diseases. Over time, the widespread use of synthetic fungicides to combat fungal crop diseases has increased resistance to fungal pathogens (Bagheri et al., 2019). Most vegetable diseases are due to fungal pathogens (Koike et al., 2007). The role of chitosan as a biocontrol agent against fungal pathogens has been studied extensively (Abbey et al., 2019; dos Santos Gomes et al., 2021; Riseh et al., 2023). Chitosan inhibits mycelial growth, sporulation, spore viability, and germination of the fungal pathogens, probably due to chitosan’s ability to bind DNA to inhibit RNA synthesis in the target organism (Roller and Covill, 1999). Chitosan imposes fatalities against other fungi and oomycetes, such as Phytophthora cinnamomi, Phytophthora palmivora, Gremmeniella abietina, Cryphonectria parasitica, and Heterobasidion annosum (Kuyyogsuy et al., 2018; Silva-Castro et al., 2018; Matei et al., 2020). Chitosan protected the S. tuberosum plant against late blight disease by eliciting the induced systemic resistance, pattern recognition receptors, and several other defence-related genes, hormones, and enzymes (Zheng et al., 2021b). Furthermore, it can restrict mycelial growth and spore germination by regulating several genes of metabolism, cell membrane structure and function, and ribosome biogenesis (Huang et al., 2021). Chitosan and its derivatives attained a growth inhibition of up to 100% against Phytophthora cambivora and significantly reduced the mycelial growth in Phytophthora plurivora and Phytophthora × alni (Silva-Castro et al., 2018). It could result from enhanced activities of catalase (CAT), peroxidase (POD), polyphenol oxidase, and phenylalanine ammonia lyase (PAL) and the expression of HbPR1, HbGLU, HbASI, and HbCAT genes with chitosan application (Kuyyogsuy et al., 2018). The same study found a positive correlation between chitosan and callose and lignin depositions in Hevea brasiliensis leaves, which could provide additional fungal resistance. Furthermore, Guo et al. (2013) reported that 0.5 mg/mL of chitosan reduced growth in Fusarium oxysporum (15%) and Alternaria solani (57%). Similar effects were observed by Younes et al. (2014), where chitosan effectively suppressed the growth of Aspergillus niger, F. oxysporum, and A. solani.
2.2 Against bacteria
The antibacterial activity of chitosan encompasses both discouraging bacterial growth (bacteriostatic) and destroying them (bactericidal). Although the mode of chitosan activity against bacteria is still under discussion, the most accepted model is the electrostatic interaction model governing chitosan binding to the bacterial membrane. The functional NH2 groups of GlcNAc subunits give chitosan a polycationic nature. The positive moiety binds electrostatically with the negatively charged components of bacterial cell membrane including extracellular polymeric substances and proteins (Khan et al., 2020). A few studies noted that chitosan can also bind to DNA and cause extensive nucleic acid degradation (Dananjaya et al., 2016; Wang et al., 2022). Chitosan binding induces permeabilisation of the bacterial cell surface and facilitates intracellular leakage. It degrades bacterial biofilm and causes cell death. However, it all depends on how well chitosan can perforate the plasma membrane, which directly depends on its MW and the bacterial type (Verlee et al., 2017). Several other factors can regulate chitosan’s antibacterial potential, such as the ratio of its monomeric units (GlcNAc and GLcN), the DDA, the acetylation pattern, solubility, and environmental effects (Li and Zhuang, 2020). Nonetheless, no substantial relation was found between the source of chitosan to its antibacterial activity. It is observed in several gram (+) and gram (−) bacteria that the minimum inhibitory concentration (MIC) of chitosan (similar MW) decreases with increasing DDA (Verlee et al., 2017). Thus, a higher DDA would mean more effective chitosan against bacteria. Antibacterial activity of chitosan has been noted against several important gram (+) bacteria (such as Staphylococcus aureus, Bacillus cereus, Enterococcus faecalis, Micrococcus luteus, and Bacillus subtilis) and gram (−) bacteria (including Escherichia coli, Vibrio cholera, Shigella dysenteriae, Bacteroides fragilis, and Pseudomonas aeruginosa) (Benhabiles et al., 2012; Younes et al., 2014; Goy et al., 2016; Yadav et al., 2020).
2.3 Against viruses
Plant viruses negatively impact plants and cause a wide range of symptoms, including discolouration, distortion of plant parts, and loss of vigour, affecting yield. Chitosan application has been found significantly effective against several viruses, including potato virus X, tobacco mosaic and necrosis viruses, alfalfa mosaic virus, peanut stunt virus, cucumber mosaic virus, potato virus X (PVX), and beans mild mosaic virus (Phaseolus vulgaris) (Pospieszny et al., 1991; Pospieszny, 1997; Chirkov et al., 2001; Faoro et al., 2001). Since chitosan’s antiviral activity depends on its MW, low MW chitosan shows higher antiviral activities than high MW. Chitosan application inhibits systemic propagation of viroid (Pospieszny et al., 1991) and induces plant resistance by callose formation and enhanced ribonuclease content. Treated leaves show less accumulation of viruses than the control (Chirkov et al., 2001).
2.4 Against nematodes
The underground plant parasitic nematodes attack various crops’ root systems, causing root galls, stunted growth, and increased susceptibility to pathogen attack and abiotic stress (Yan and Xie, 2015). Accumulating body of evidence has shown that exogenous application of chitosan triggers plant defence-related pathways in various crops, such as potato, tomato (Vasyukova et al., 2001; Fan et al., 2020; Boamah et al., 2023), barley, banana (Maciá-Vicente et al., 2009; Suarez-Fernandez et al., 2021), and soybean (Mwaheb et al., 2017). Consistently, chitosan also promotes host resistance to Meloidogyne spp. root-knot nematodes (RKNs). Similarly, water-soluble chitosan induces resistance against root-knot nematodes (Escudero et al., 2017; Chakraborty et al., 2020).
2.5 Against insects
Insect herbivores are among the most notorious crop pests that cause significant direct and indirect crop loss. The exogenous application of chitosan has been found effective against multiple pests, including chewing and sucking insects. The insecticidal effect of chitosan has been tested against various insect herbivores: lepidopterans (Helicoverpa armigera, Plutella xylostella, Spodoptera littoralis, and Tuta absoluta), hemipterans (Aphis gossypii, Rhopalosiphum padi, Sitobian aveane, Metapophium dirhodum, Myzus persicae, and Hyalopetrus prun), coleopterans (Callosobruchus maculatus), and hymenopterans (Solenopsis invicta) (Rabea et al., 2005; Sahab et al., 2015; Sabbour and Solieman, 2016; Zhang et al., 2003; Zhu et al., 2021). Chitosan-mediated changes have been shown to reduce egg deposition (Sahab et al., 2015), insect performance (Zhang et al., 2003), population densities (Sabbour and Solieman, 2016), and feeding behaviour. It also causes morphological changes in the midgut of insect herbivores and reduces digestive enzymes’ activity, increasing pest mortality (Rabea et al., 2005).
3 How does chitosan work?
There are several theories regarding the antimicrobial mechanism of chitosan. Goy et al. (2009) proposed three faces of the antibacterial mechanism of chitosan: degradation of the cell wall by ionic surface interaction, inhibition of protein and mRNA synthesis through permeation of chitosan into nuclei of microorganisms, and limitation of nutrient availability for microorganism by the formation of external covering over the plant surface. Another researcher stated that the mechanism of chitosan action is based on the destruction of the cell membrane due to a burst of extracellular components, which has been observed in disrupting the growth of fungi (Xing et al., 2015). Recent studies suggested that chitosan is responsible for the hydrolysis of cell wall components (peptidoglycans), disrupting electrolytic balance and increasing pathogen mortality. The exogenous spray of chitosan induces resistance against insect herbivores. Chitosan has been extensively exploited to improve inducible plant defences against insect herbivores (Gatehouse, 2002). Chitosan treatment enhances plant response locally (around the infection sites) and systemically to alert healthy plant parts. These responses include signal transduction, synthesis of resistance-related compounds such as phytoalexins, pathogenesis-related protein (PR-protein) callose formation, lignification, and synthesis of proteinase inhibitors (Katiyar et al., 2015; Chun and Chandrasekaran, 2019). Evidence shows that chitosan also increases the endogenous 2-oxo-phytodeinoic and JA levels in many crops, including Oryza sativa (Rakwal et al., 2002). Furthermore, chitosan has been found responsible for activating the octadecanoic acid pathway that enhances the activity of chitinase, glucanase, and lipoxygenase and accumulates phytoalexins (El Hadrami et al., 1997; El Hadrami et al., 2010). Figure 2 proposes a modus operandi for chitosan-induced changes in the soil and plant and how they influence the cellular physiology of stressed plants. Chitosan seems to have intricate crosstalk with several other signalling pathways to confer biotic tolerance. These pathways can include phytohormones (SA, JA, and ET), ROS and antioxidant metabolisms, and other cell signals.
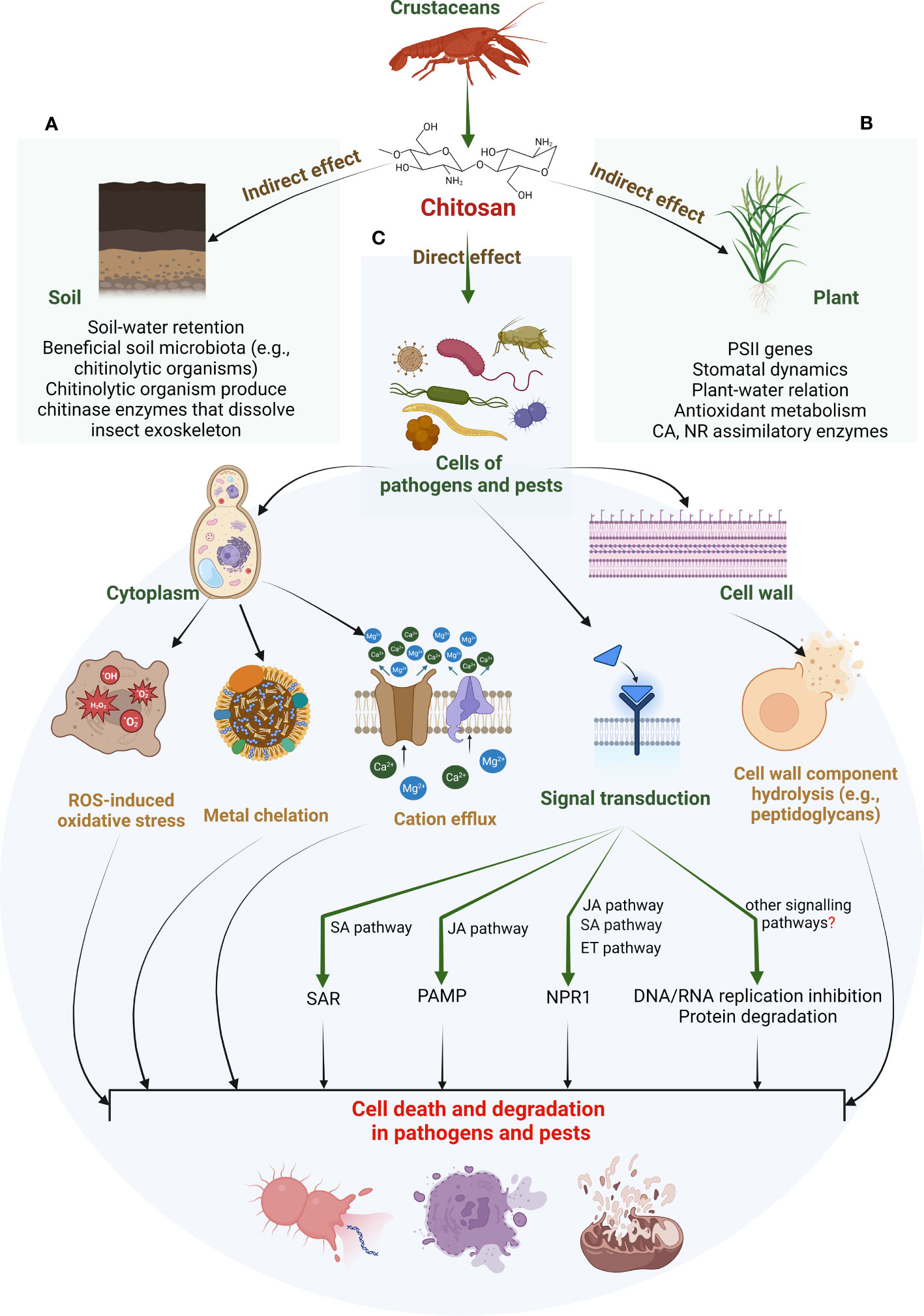
Figure 2 Proposed modus operandi for chitosan-induced biotic stress tolerance in higher plants. These effects can be identified as indirect (A, B) and direct (C). Indirect effects include chitosan action on soil properties that improve water retention. Improved soil–water content promotes soil microbiota. Such microbiota include several chitinolytic organisms that can dissolve insect exoskeletons by chitinase enzymes (see panel A). Other beneficial soil organisms can promote source-sink potential. A higher nutrient status combined with increased soil–water retention promotes plant innate defence, e.g., antioxidant capacity (see panel B). In contrast, the direct effects of chitosan include cytotoxic action on pathogen/pest cells. Chitosan can cause hydrolysis of the cell wall components. This, along with metal chelation and cation efflux from the cytoplasm, disrupts ROS-antioxidant metabolism in pathogen/pest cells. This could result in cell degradation or death of plant invaders. It is suggested that these direct actions can rely on chitosan’s intricate crosstalk with certain phytohormones such as JA, SA, ET, ABA, and GA. A few studies suggested a genomic approach for such adjustments such as activation of defensive genes and TFs (see panel C). Apart from this, other signalling pathways could be facilitating chitosan-induced biotic tolerance in plants. Nevertheless, their understanding is still in preliminary phase and demands more investigation. PSII, photosystem II; CA, carbonic anhydrase; NR, nitrate reductase; ROS, reactive oxygen species; SAR, systemic induced resistance; PAMP, pathogen-associated molecular pattern; NPR1, natriuretic peptide receptor A/guanylate cyclase A; SA, salicylic acid; JA, jasmonic acid; ET, ethylene; GA, gibberellic acid; ABA, abscisic acid; TFs, transcription factors.
4 Chitosan’s crosstalk with phytohormones, antioxidants, and other signalling molecules
4.1 Crosstalk with phytohormones
It is suggested that chitosan induces plant defence through an intricate correspondence with several phytohormones such as JA, abscisic acid (ABA), indole acetic acid (IAA), SA, and gibberellic acid (GA) (Jogaiah et al., 2020; Ji et al., 2022). These phytohormones are critical to inducing defence against several plant pathogens and pests.
Chitosan promotes JA concentration in plant tissues under attack by activating the octadecanoic pathway (Rakwal et al., 2002; Iriti et al., 2009). The pathway is responsible for JA biosynthesis by oxidising linolenic acid and increasing phytoalexin content. Chitosan-induced JA signalling promoted the accumulation of several other secondary metabolites such as glucosinolates and anthocyanins glucosides (Iula et al., 2022). These compounds regulate stress-induced oxidative damage given their ROS scavenging nature. Furthermore, JA can trigger signal transduction and activates defence-related genes against pathogen invasion. Peian et al. (2021) reported that chitosan activated multiple JA-biosynthesis-related genes such as VvLOX, VvAOC, VvAOS, and VvCOI1 to induce production of methyl jasmonate, a vital defence hormone, in Vitis vinifera L. under fungal stress.
ABA-mediated signal transduction is critical for plants to respond to biotic and abiotic stresses (Mauch-Mani and Mauch, 2005; Lee and Luan, 2012). Chitosan is reported to stimulate ABA biosynthesis to protect the plant against P. palmivora in H. brasiliensis (Kuyyogsuy et al., 2018). In Fragaria × ananassa ‘Fugilia’, TOPLESS-related 3 (TPR3) and HISTONE DEACETYLASE 19 (HDA19) are overexpressed during pathogen attack. These proteins interact with the cell wall and reduce its stability. Nonetheless, both TPR3 and HDA19 were restricted by chitosan integration into such plants while restoring cell wall stability and fruit quality (Peian et al., 2021). Chitosan and ABA treatments induced the expression of HbPR1, HbGLU, HbASI, and HbCAST and the deposition of lignin and callose (Kuyyogsuy et al., 2018). ABA also regulates callose deposition intensity and speed (Flors et al., 2005). Furthermore, the same study (Kuyyogsuy et al., 2018) links chitosan with upregulated NCED activity, a crucial enzyme for ABA biosynthesis. Thus, chitosan and ABA seem to work together to defend plants against stressful scenarios. However, their precise crosstalk is still unclear. Chitosan can activate the pathogen-associated molecular pattern (PAMP) and promote H+ and Ca2+ entry in the cytosol; this activates mitogen-activated protein kinases (MAPKs) and the production of JA, ABA, and phytoalexins (El Hadrami et al., 2010; Iula et al., 2022).
In Helianthus annuus L., chitosan magnified IAA and phenol content during stress (Li et al., 2019; Bakhoum et al., 2020). Similarly, chitosan treatment upregulated IAA and SA content in Arabidopsis thaliana by altering their gene expression patterns in the roots. It resulted in restricted expression of WOX5 in the apical root meristem and arrested root development (Lopez-Moya et al., 2017). Chitosan-induced IAA amassing could be triggered by upregulated genes of the tryptophan-dependent biosynthesis pathway (ami1, aao1, and yuc2) and reduced expression of IAA translocation gene (pin1) (Lopez-Moya et al., 2019).
Similar implications of chitosan treatment were observed in GA biosynthesis. Seed priming with chitosan improved germination rate, lipase activity, and seedling growth through increased GA levels in peanut plants (Zhou et al., 2002). Chitosan was also linked with the enhanced impact of GA on plant physiology in another study with P. vulgaris L. (Pereira et al., 2017). Nonetheless, the understanding of chitosan–GA crosstalk is still preliminary and needs more attention.
4.2 Chitosan crosstalk with ROS and antioxidant metabolism
Chitosan triggers signal transduction for phytoalexins production, secondary metabolites, and enzymatic and non-enzymatic antioxidants in defence responses to (a)biotic stresses (Pongprayoon et al., 2022). Chitosan triggered signalling pathways in strawberry fruits during oxidative stresses. It induces chloroplast-related genes. Peroxiredoxin-ROS scavenger genes have related to the cellular levels of ROS in the signalling networks of the chloroplast (Awad et al., 2015). Chitosan application in arbuscular mycorrhizal (AM) tomato (Solanum lycopersicum L.) promoted the growth of plants. Among the possibilities to evaluate the influence of chitosan on tomato growth and flowering may be its anti-transpirant properties to activate ROS scavenging to increase stomatal conductance and xylem vessel growth. In addition, applying chitosan to leaves increased plants’ photosynthesis rate and consequently improved plant growth and development (El Amerany et al., 2020). Plants treated with chitosan developed an increased capacity to produce enzymatic antioxidants such as CAT, POD, superoxide dismutase (SOD), and glutathione reductase (GR) to mitigate the effects of oxidative stress in salinity stress (Alkahtani et al., 2020). Chitosan’s involvement with vide supra specialised molecules has been reported in several plant species under various stresses. Chitosan stimulates the activity of several defence-related enzymes such as POD in Prunus persica L. fruits or Phoenix dactylifera L. roots (Abdellatef et al., 2022). Chitosan treatment can amplify PAL activity as well in many crops like Triticum aestivum, V. vinifera, and O. sativa, resulting in increased levels of phenolic and flavonoid compounds through phenylpropanoid pathway (Li et al., 2013). In V. vinifera, chitosan elevated PAL activity and enhanced the antioxidant defence mechanisms against Botrytis cinerea by upregulated CAT, POD, and SOD activities (Peian et al., 2021).
The foliar application of chitosan encouraged O2− scavenging and restricted H2O2 generation and lipid peroxidation to manage stress in sweet peppers (Alkahtani et al., 2020). In white clover, chitosan in dehydration-responsive element-binding protein (DREB) responsive pathway upregulated DREB2, DREB4, and DREB5 genes (Ling et al., 2022). It activated Y2K and Y2SK genes, which encode dehydrins (DHNs) that produce water-stress tolerance. Notably, these genes are critical to stress tolerance and antioxidant defence.
4.3 Chitosan crosstalk with signalling molecules
Chitosan activates signalling pathways in cells by binding to specific cellular receptors, activating important secondary messengers such as Ca2+, nitric oxide (NO), ROS, and transcription factors (TFs) (vide supra section 4.2 for ROS). These molecules play a critical role in triggering several biochemical responses. It is worth noting that chitosan, along with Ca2+ treatment, increases Ca2+ influx into the cytosol. This elevated cytosolic Ca2+ level is associated with enhanced callose formation. Notably, chitosan without the Ca2+ application did not form callose, suggesting that chitosan-induced callose synthesis depends on the presence of Ca2+ (Koühle et al., 1985).
Similarly, NO plays a critical role in diverse vital physiological phenomena and provides defence against stress scenarios (Corpas et al., 2001; Kolbert et al., 2019). Chitosan promotes the generation of NO and phosphatidic acid. Nonetheless, it inhibits the phospholipase-mediated signalling pathway in the presence of an NO scavenger. Thus, it seems plausible that chitosan elicits defence responses in a NO-dependent pathway (Tocci et al., 2011). Moreover, chitosan can regulate photosynthesis and stomatal movement (Mukarram et al., 2023) in NO-dependent signalling, considering NO has critical roles in stomatal movement in stressed plants (Garcıía-Mata and Lamattina, 2001; Neill et al., 2008).
Many chitosan-modulated genes, including defence-related genes and TFs related to signalling pathways, are involved in biotic stress responses. Povero et al. (2011) demonstrated that several WRKY genes of A. thaliana respond to chitosan treatment. WRKY gene family is attributed to defending against pathogens or pathogen-mimicking stimuli. Among these chitosan-elicited WRKY TFs, At5g13080 (WRKY75), At3g01970 (WRKY45), At2g46400 (WRKY46), and At4g31800 (WRKY18) are specifically involved in pathogen responses. Chitosan also overexpressed other MYB TFs such as MYB31 (At1g74650) and MYB15 (At3g23250). The modulation of these TFs by chitosan highlights its impact on regulating biotic stress responses and emphasises its potential as a valuable tool in enhancing plant defence mechanisms.
5 Technological advances: chitosan oligomers (COS), chitosan microparticles (CS-MPs), and chitosan nanoparticles (CS-NPs)
At high pH (≥6.5), chitosan experiences reduced solubility, high viscosity, and affinity to coagulate proteins. This limits chitosan’s bioactivities. The radiolytic degradation or (acidic, alkaline, or enzymatic) digestion of the β-1,4-glycosidic bonds between monomeric sugar residues in chitosan polymers can form chitosan oligosaccharides (COSs). Such oligosaccharides have higher solubility and surface area and lower viscosity than their polymeric counterparts (Muley et al., 2019). Thus, COSs are equipped with upgraded bioactivities, e.g., antimicrobial (against fungi, bacteria, and viruses), antitumor, antioxidant, anti-inflammatory, hypocholesterolemic, and immunopotentiation bioactivities (Liaqat and Eltem, 2018). Similarly, COS application in agriculture produced superior outcomes in plant growth, development, productivity, and defence against (a)biotic stresses (Wang et al., 2015; Lan et al., 2016; Li et al., 2020; Mukarram et al., 2022). Kim and Rajapakse (2005) reported that COSs discourage pathogenic invasion on the plant by upregulating various genes expression responsible for endogenous plant immunity. It was suggested that poly-d-glucosamine units of chitosan bind with the contagious receptors mimicking a pathogenic invasion (Maurya et al., 2019). It initiates the feedback mechanisms in the plant including upregulated phytoalexins biosynthesis. Phytoalexins are the defence chaperones for endogenous immunity and confer resistance against biotic stress. Others reported a boost in phytoalexin content with COS application in different plants (Pichyangkura and Chadchawan, 2015). Benchamas et al. (2021) discussed antibiotic effects of COSs against several gram (+) bacteria (e.g., M. luteus, Staphylococcus faecalis, S. aureus, B. subtilis, B. cereus, and Lactiplantibacillus plantarum) and gram (−) bacteria (such as E. coli, Aggregatibacter actinomycetemcomitans, Vibrio vulnificus, and P. aeruginosa). Similar cytotoxic effects of COSs were found in many important fungi, e.g., Candida albicans, Candida krusei, B. cinerea, Saccharomyces cerevisiae, Rhodotorula glutinis, Rhodotorula mucilaginosa, and Schizosaccharomyces pombe (Ganan et al., 2019).
Chitosan microparticles (CS-MPs) or nanoparticles (CS-NPs) are even smaller chitosan derivatives than COSs. CS-MPs are produced from the aqueous solution of chitosan mixed with dilute acid. The resulting suspension loses its aqueous phase at low pressure and forms microparticles. CS-MPs formed from low chitosan concentration can stabilise Pickering emulsion (oil-in-water) (Mwangi et al., 2016). This opens new perspectives for optimising stimulus-responsive emulsion and their stable storage. Several studies with CS-MPs or CS-NPs concluded their enhanced beneficial role in agriculture, food storage, and biomedical sectors over chitosan polymers (Mwangi et al., 2016; Iglesias et al., 2019; Malerba and Cerana, 2020). Further, CS-MPs and CS-NPs have special relevance in vaccine delivery, inflammatory diseases, and cancer treatment (see Prabaharan and Mano, 2004; Naskar et al., 2019). These bioactivities could also be true against microbial and pest communities. CS-NPs exhibit cytotoxic effects against many gram (+) and gram (−) bacteria, e.g., E. coli, Staphylococcus choleraesuis, Staphylococcus typhimurium, and S. aureus (Qi et al., 2004). Ahmed and Aljaeid (2016) suggested the crucial roles of CS-MPs and CS-NPs with the influenza vaccine, cholera toxin, hepatitis B surface protein, and antigen protein against several other fungi and viruses. Integrating CS-NPs with certain metals, phenolics, and essential oils produces enhanced antioxidants and scavenging activities against microbes (Hasheminejad et al., 2019; Fahimirad et al., 2021; Rashki et al., 2021). This makes chitosan particles an ideal candidate for encapsulating agents in the food packaging and preservation industry as well as a delivery vehicle for bioactive compounds such as nutrients, essential oils, vitamins, and antioxidants (Hasheminejad et al., 2019; Maleki et al., 2022).
6 Conclusion and perspectives
The past few decades have witnessed exponential growth in chitosan studies in several aspects of crop defence to biomedical applications. It is established now that chitosan boosts plant development and yield during optimal and stressful environments. Nonetheless, there is a vast gap in the molecular understanding of chitosan and its derivatives. In particular, a comprehensive cognition of chitosan’s crosstalk with phytohormones, antioxidants, and signalling molecules is lacking. It is pertinent for future studies to explore the signalling potential of chitosan itself. Although our knowledge of chitosan’s antimicrobial potential has widened over the past two decades, multiple inconsistencies and a well-defined mechanism must be solved. Therefore, another aspect worth exploring is the modus operandi of chitosan against microbial entities such as fungi, bacteria, and viruses. Recent advances in structural modification in chitosan conferred superior results over chitosan. It could be interesting to know whether these modified chitosan oligomers or nanoparticles adopt different pathways or signalling molecules for enhanced bioactivities.
Author contributions
All authors listed have made a substantial, direct, and intellectual contribution to the work and approved it for publication.
Funding
This work was supported by funding from the Slovak scientific grant agency VEGA (1/0117/22 and 1/0108/23).
Acknowledgments
Figures 1 and 2 were created with BioRender.com.
Conflict of interest
The authors declare that the research was conducted in the absence of any commercial or financial relationships that could be construed as a potential conflict of interest.
Publisher’s note
All claims expressed in this article are solely those of the authors and do not necessarily represent those of their affiliated organizations, or those of the publisher, the editors and the reviewers. Any product that may be evaluated in this article, or claim that may be made by its manufacturer, is not guaranteed or endorsed by the publisher.
References
Abbey, J. A., Percival, D., Abbey, L., Asiedu, S. K., Prithiviraj, B., Schilder, A. (2019). Biofungicides as alternative to synthetic fungicide control of grey mould (Botrytis cinerea)–prospects and challenges. Biocontrol Sci. Technol. 29 (3), 207–228. doi: 10.1080/09583157.2018.1548574
Abdellatef, A. E. M., Elagamey, E., Kamel, S. M. (2022). Chitosan is the ideal resource for plant disease management under sustainable agriculture. IntechOpen. doi: 10.5772/intechopen.107958
Ahmed, T. A., Aljaeid, B. M. (2016). Preparation, characterization, and potential application of chitosan, chitosan derivatives, and chitosan metal nanoparticles in pharmaceutical drug delivery. Drug Design Dev. Ther. 10, 483–507. doi: 10.2147/DDDT.S99651
Alkahtani, M., Attia, K., Hafez, Y. M., Khan, N., Eid, A. M., Ali, M. A., et al. (2020). Chlorophyll fluorescence parameters and antioxidant defense system can display salt tolerance of salt acclimated sweet pepper plants treated with chitosan and plant growth promoting rhizobacteria. Agronomy 10, 1180. doi: 10.3390/agronomy10081180
Aranaz, I., Alcántara, A. R., Civera, M. C., Arias, C., Elorza, B., Heras Caballero, A., et al. (2021). Chitosan: An overview of its properties and applications. Polymers 13, 3256. doi: 10.3390/polym13193256
Awad, J., Stotz, H. U., Fekete, A., Krischke, M., Engert, C., Havaux, M., et al. (2015). 2-cysteine peroxiredoxins and thylakoid ascorbate peroxidase create a water-water cycle that is essential to protect the photosynthetic apparatus under high light stress conditions. Plant Physiol. 167, 1592–1603. doi: 10.1104/pp.114.255356
Badawy, M. E. I., Rabea, E. I. (2011). A biopolymer chitosan and its derivatives as promising antimicrobial agents against plant pathogens and their applications in crop protection. Int. J. Carbohydr. Chem. 2011, 1–29. doi: 10.1155/2011/460381
Badiali, C., De Angelis, G., Simonetti, G., Brasili, E., Tobaruela, E., de, C., et al. (2018). Chitosan oligosaccharides affect xanthone and VOC biosynthesis in Hypericum perforatum root cultures and enhance the antifungal activity of root extracts. Plant Cell Rep. 37 (11), 1471–1484. doi: 10.1007/s00299-018-2317-2
Bagheri, A., Bondori, A., Damalas, C. A. (2019). Modeling cereal farmers’ intended and actual adoption of integrated crop management (ICM) practices. J. Rural Stud. 70, 58–65. doi: 10.1016/j.jrurstud.2019.05.009
Bailey, J. A. (1982) Mechanisms of phytoalexin accumulation. Eds. Bailey, J. A., Mansfield, J. W. Glasgow, London: Halsted/Wiley.
Bakhoum, G. S., Sadak, M. S., Badr, E. A. E. M. (2020). Mitigation of adverse effects of salinity stress on sunflower plant (Helianthus annuus L.) by exogenous application of chitosan. Bull. Natl. Res. Centre 44, 1–11. doi: 10.1186/s42269-020-00343-7
Benchamas, G., Huang, G., Huang, S., Huang, H. (2021). Preparation and biological activities of chitosan oligosaccharides. Trends Food Sci. Technol. 107, 38–44. doi: 10.1016/j.tifs.2020.11.027
Benhabiles, M. S., Salah, R., Lounici, H., Drouiche, N., Goosen, M. F. A., Mameri, N. (2012). Antibacterial activity of chitin, chitosan and its oligomers prepared from shrimp shell waste. Food Hydrocolloids 29 (1), 48–56. doi: 10.1016/j.foodhyd.2012.02.013
Boamah, P. O., Onumah, J., Aduguba, W. O., Santo, K. G. (2023). Application of depolymerized chitosan in crop production: A review. Int. J. Biol. Macromolecules 235, 123858. doi: 10.1016/j.ijbiomac.2023.123858
Cartwright, D., Langcake, P., Pryce, R. J., Leworthy, D. P., Ride, J. P. (1977). Chemical activation of host defence mechanisms as a basis for crop protection. Nature 267 (5611), 511–513. doi: 10.1038/267511a0
Chakraborty, M., Hasanuzzaman, M., Rahman, M., Khan, M. A. R., Bhowmik, P., Mahmud, N. U., et al. (2020). Mechanism of plant growth promotion and disease suppression by chitosan biopolymer. Agriculture 10 (12), 624. doi: 10.3390/agriculture10120624
Chamnanmanoontham, N., Pongprayoon, W., Pichayangkura, R., Roytrakul, S., Chadchawan, S. (2015). Chitosan enhances rice seedling growth via gene expression network between nucleus and chloroplast. Plant Growth Regul. 75, 101–114. doi: 10.1007/s10725-014-9935-7
Chatterjee, S., Chatterjee, B. P., Guha, A. K. (2014). A study on antifungal activity of water-soluble chitosan against Macrophomina phaseolina. Int. J. Biol. Macromolecules 67, 452–457. doi: 10.1016/j.ijbiomac.2014.04.008
Chirkov, S. N., Il’ina, A. V., Surgucheva, N. A., Letunova, E. V., Varitsev, Y. A., Tatarinova, N. Y., et al. (2001). Effect of chitosan on systemic viral infection and some defense responses in potato plants. Russian J. Plant Physiol. 48 (6), 774–779. doi: 10.1023/A:1012508625017
Chun, S.-C., Chandrasekaran, M. (2019). Chitosan and chitosan nanoparticles induced expression of pathogenesis-related proteins genes enhances biotic stress tolerance in tomato. Int. J. Biol. Macromolecules 125, 948–954. doi: 10.1016/j.ijbiomac.2018.12.167
Corpas, F. J., Barroso, J. B., del Rıío, L. A. (2001). Peroxisomes as a source of reactive oxygen species and nitric oxide signal molecules in plant cells. Trends Plant Sci. 6 (4), 145–150. doi: 10.1016/S1360-1385(01)01898-2
Croisier, F., Jérôme, C. (2013). Chitosan-based biomaterials for tissue engineering. Eur. Polymer J. 49 (4), 780–792. doi: 10.1016/j.eurpolymj.2012.12.009
Dananjaya, S. H. S., Godahewa, G. I., Jayasooriya, R. G. P. T., Lee, J., De Zoysa, M. (2016). Antimicrobial effects of chitosan silver nano composites (CAgNCs) on fish pathogenic Aliivibrio (Vibrio) salmonicida. Aquaculture 450, 422–430. doi: 10.1016/j.aquaculture.2015.08.023
De Oliveira, J. L., Campos, E. V. R., Pereira, A. E. S., Nunes, L. E. S., Da Silva, C. C. L., Pasquoto, T., et al. (2018). Geraniol encapsulated in chitosan/gum arabic nanoparticles: A promising system for pest management in sustainable agriculture. J. Agric. Food Chem. 66 (21), 5325–5334. doi: 10.1021/acs.jafc.8b00331
Doares, S. H., Syrovets, T., Weiler, E. W., Ryan, C. A. (1995). Oligogalacturonides and chitosan activate plant defensive genes through the octadecanoid pathway. Proc. Natl. Acad. Sci. 92 (10), 4095–4098. doi: 10.1073/pnas.92.10.4095
dos Santos Gomes, A. C., da Silva, R. R., Moreira, S. I., Vicentini, S. N. C., Ceresini, P. C. (2021). Biofungicides: An eco-friendly approach for plant disease management. In: Encyclopedia of Mycology. Eds. Zaragoza, Ó, Casadevall, A. Oxford: Elsevier. p 641–649.
El Amerany, F., Meddich, A., Wahbi, S., Porzel, A., Taourirte, M., Rhazi, M., et al. (2020). Foliar application of chitosan increases tomato growth and influences mycorrhization and expression of endochitinase-encoding genes. Int. J. Mol. Sci. 21, 535. doi: 10.3390/ijms21020535
El Ghaouth, A., Arul, J., Grenier, J., Asselin, A. (1992). Antifungal activity of chitosan on two postharvest pathogens of strawberry fruits. Phytopathology 82 (4), 398–402. doi: 10.1094/Phyto-82-398
El Hadrami, A., Adam, L. R., El Hadrami, I., Daayf, F. (2010). Chitosan in plant protection. Mar. Drugs 8 (4), 968–987. doi: 10.3390/md8040968
El Hadrami, I., Ramos, T., El Bellaj, M., El Idrissi-Tourane, A., Macheix, J. J. (1997). A sinapic derivative as an induced defence compound of date palm against “Fusarium oxysporum” f. sp. “albedinis”, the agent causing bayoud disease. J. Phytopathol. 145 (8-9), 329–333. doi: 10.1111/j.1439-0434.1997.tb00409.x
El-Seedi, H. R., El-Wahed, A. A. A., Naggar, Y., Saeed, A., Xiao, J., Ullah, H., et al. (2022). Insights into the role of natural products in the control of the honeybee gut parasite (Nosema spp.). Animals 12 (21), 3062. doi: 10.3390/ani12213062
Escudero, N., Lopez-Moya, F., Ghahremani, Z., Zavala-Gonzalez, E. A., Alaguero-Cordovilla, A., Ros-Ibañez, C., et al. (2017). Chitosan increases tomato root colonization by Pochonia chlamydosporia and their combination reduces root-knot nematode damage. Front. Plant Sci. 8, 1415. doi: 10.3389/fpls.2017.01415
Fahimirad, S., Abtahi, H., Satei, P., Ghaznavi-Rad, E., Moslehi, M., Ganji, A. (2021). Wound healing performance of PCL/chitosan based electrospun nanofiber electrosprayed with curcumin loaded chitosan nanoparticles. Carbohydr. Polymers 259, 117640. doi: 10.1016/j.carbpol.2021.117640
Fan, Z., Qin, Y., Liu, S., Xing, R., Yu, H., Li, P. (2020). Chitosan oligosaccharide fluorinated derivative control root-knot nematode (Meloidogyne incognita) disease based on the multi-efficacy strategy. Mar. Drugs 18 (5), 273. doi: 10.3390/md18050273
Fan, Z., Wang, L., Qin, Y., Li, P. (2023). Activity of chitin/chitosan/chitosan oligosaccharide against plant pathogenic nematodes and potential modes of application in agriculture: A review. Carbohydr. Polymers 306, 120592. doi: 10.1016/j.carbpol.2023.120592
Faoro, F., Sant, S., Iriti, M., Maffi, D., Appiano, A. (2001). Chitosan-elicited resistance to plant viruses: a histochemical and cytochemical study. Chitin Enzymol 22, 57–62.
Faqir, Y. H., Ma, J. H., Chai, Y. L. (2021). Chitosan in modern agriculture production. Plant Soil Environ. 67, 679–699. doi: 10.17221/332/2021-PSE
Flors, V., Ton, J., Jakab, G., Mauch-Mani, B. (2005). Abscisic acid and callose: team players in defence against pathogens? J. Phytopathol. 153, 377–383. doi: 10.1111/j.1439-0434.2005.00987.x
Gal, M. R., Rahmaninia, M., Hubbe, M. A. (2023). Comprehensive review of chitosan applications in paper science and technologies. Carbohydr. Polymers 309, 120665. doi: 10.1016/j.carbpol.2023.120665
Ganan, M., Lorentzen, S. B., Agger, J. W., Heyward, C. A., Bakke, O., Knutsen, S. H., et al. (2019). Antifungal activity of well-defined chito-oligosaccharide preparations against medically relevant yeasts. PloS One 14 (1), e0210208. doi: 10.1371/journal.pone.0210208
Garcıía-Mata, C., Lamattina, L. (2001). Nitric oxide induces stomatal closure and enhances the adaptive plant responses against drought stress. Plant Physiol. 126, 1196–1204. doi: 10.1104/pp.126.3.1196
Gatehouse, J. A. (2002). Plant resistance towards insect herbivores: a dynamic interaction. New Phytol. 156 (2), 145–169. doi: 10.1046/j.1469-8137.2002.00519.x
Goy, R. C., de Britto, D., Assis, O. B. G. (2009). A review of the antimicrobial activity of chitosan. Polímeros 19 (3), 241–247. doi: 10.1590/S0104-14282009000300013
Goy, R. C., Morais, S. T., Assis, O. B. (2016). Evaluation of the antimicrobial activity of chitosan and its quaternized derivative on E. coli and S. aureus growth. Rev. Bras. Farmacognosia 26, 122–127. doi: 10.1016/j.bjp.2015.09.010
Guo, Z., Ren, J., Dong, F., Wang, G., Li, P. (2013). Comparative study of the influence of active groups of chitosan derivatives on antifungal activity. J. Appl. Polymer Sci. 127 (4), 2553–2556. doi: 10.1002/app.37747
Haas, J., Lozano, E. R., Haida, K. S., Mazaro, S. M., de Souza Vismara, E., Poppy, G. M. (2018). Getting ready for battle: do cabbage seeds treated with jasmonic acid and chitosan affect chewing and sap-feeding insects? Entomologia Experimentalis Applicata 166 (5), 412–419. doi: 10.1111/eea.12678
Hadwiger, L. A. (2013). Multiple effects of chitosan on plant systems: Solid science or hype. Plant Sci. 208, 42–49. doi: 10.1016/j.plantsci.2013.03.007
Hadwiger, L. A., Beckman, J. M. (1980). Chitosan as a component of pea-Fusarium solani interactions. Plant Physiol. 66 (2), 205–211. doi: 10.1104/pp.66.2.205
Hasheminejad, N., Khodaiyan, F., Safari, M. (2019). Improving the antifungal activity of clove essential oil encapsulated by chitosan nanoparticles. Food Chem. 275, 113–122. doi: 10.1016/j.foodchem.2018.09.085
Helander, I. M., Nurmiaho-Lassila, E.-L., Ahvenainen, R., Rhoades, J., Roller, S. (2001). Chitosan disrupts the barrier properties of the outer membrane of Gram-negative bacteria. Int. J. Food Microbiol. 71 (2–3), 235–244. doi: 10.1016/S0168-1605(01)00609-2
Hidangmayum, A., Dwivedi, P., Katiyar, D., Hemantaranjan, A. (2019). Application of chitosan on plant responses with special reference to abiotic stress. Physiol. Mol. Biol. Plants 25, 313–326. doi: 10.1007/s12298-018-0633-1
Horbowicz, M., Mioduszewska, H., Koczkodaj, D., Saniewski, M. (2009). The effect of cis-jasmone, jasmonic acid and methyl jasmonate on accumulation of anthocyanins and proanthocyanidins in seedlings of common buckwheat [Fagipyrum esculentum Moench]. Acta Societatis Botanicorum Poloniae 78 (4), 271–277. doi: 10.5586/asbp.2009.035
Hosseinnejad, M., Jafari, S. M. (2016). Evaluation of different factors affecting antimicrobial properties of chitosan. Int. J. Biol. Macromolecules 85, 467–475. doi: 10.1016/j.ijbiomac.2016.01.022
Huang, X., You, Z., Luo, Y., Yang, C., Ren, J., Liu, Y., et al. (2021). Antifungal activity of chitosan against Phytophthora infestans, the pathogen of potato late blight. Int. J. Biol. Macromolecules 166, 1365–1376. doi: 10.1016/j.ijbiomac.2020.11.016
Ibrahim, S. S., Abou-Elseoud, W. S., Elbehery, H. H., Hassan, M. L. (2022). Chitosan-cellulose nanoencapsulation systems for enhancing the insecticidal activity of citronella essential oil against the cotton leafworm Spodoptera littoralis. Ind. Crops Products 184, 115089. doi: 10.1016/j.indcrop.2022.115089
Ibrahim, H. M., El-Zairy, E. M. R. (2015). “Chitosan as a biomaterial — structure, properties, and electrospun nanofibers,” in Concepts, Compounds and the Alternatives of Antibacterials. Ed. Bobbarala, V. (London: IntechOpen), 81–101. doi: 10.5772/61300
Ibrahim, A. Y., Kurniawati, F. (2020). The efficacy of chitosan to control nematode Aphelenchoides besseyi Christie through seed treatment. IOP Conf. Series: Earth Environ. Sci. 468 (1), 12025. doi: 10.1088/1755-1315/468/1/012025
Iglesias, M. J., Colman, S. L., Terrile, M. C., Paris, R., Martín-Saldaña, S., Chevalier, A. A., et al. (2019). Enhanced properties of chitosan microparticles over bulk chitosan on the modulation of the auxin signaling pathway with beneficial impacts on root architecture in plants. J. Agric. Food Chem. 67, 6911–6920. doi: 10.1021/acs.jafc.9b00907
Ingle, P. U., Shende, S. S., Shingote, P. R., Mishra, S. S., Sarda, V., Wasule, D. H., et al. (2022). Chitosan nanoparticles (ChNPs): A versatile growth promoter in modern agricultural production. Heliyon 8, e11893. doi: 10.1016/j.heliyon.2022.e11893
Iriti, M., Picchi, V., Rossoni, M., Gomarasca, S., Ludwig, N., Gargano, M., et al. (2009). Chitosan antitranspirant activity is due to abscisic acid-dependent stomatal closure. Environ. Exp. Bot. 66, 493–500. doi: 10.1016/j.envexpbot.2009.01.004
Iula, G., Miras-Moreno, B., Rouphael, Y., Lucini, L., Trevisan, M. (2022). The complex metabolomics crosstalk triggered by four molecular elicitors in tomato. Plants 11, 678. doi: 10.3390/plants11050678
Ji, H., Wang, J., Chen, F., Fan, N., Wang, X., Xiao, Z., et al. (2022). Meta-analysis of chitosan-mediated effects on plant defense against oxidative stress. Sci. Total Environ. 851, 158212. doi: 10.1016/j.scitotenv.2022.158212
Jia, Z., Xu, W. (2001). Synthesis and antibacterial activities of quaternary ammonium salt of chitosan. Carbohydr. Res. 333 (1), 1–6. doi: 10.1016/S0008-6215(01)00112-4
Jogaiah, S., Satapute, P., De Britto, S., Konappa, N., Udayashankar, A. C. (2020). Exogenous priming of chitosan induces upregulation of phytohormones and resistance against cucumber powdery mildew disease is correlated with localized biosynthesis of defense enzymes. Int. J. Biol. Macromolecules 162, 1825–1838. doi: 10.1016/j.ijbiomac.2020.08.124
Katiyar, D., Hemantaranjan, A., Singh, B. (2015). Chitosan as a promising natural compound to enhance potential physiological responses in plant: a review. Indian J. Plant Physiol. 20 (1), 1–9. doi: 10.1007/s40502-015-0139-6
Khalil, M. S., Badawy, M. E. I. (2012). Nematicidal activity of a biopolymer chitosan at different molecular weights against root-knot nematode, Meloidogyne incognita. Plant Prot. Sci. 48 (4), 170–178. doi: 10.17221/46/2011-PPS
Khan, F., Pham, D. T. N., Oloketuyi, S. F., Manivasagan, P., Oh, J., Kim, Y. M. (2020). Chitosan and their derivatives: Antibiofilm drugs against pathogenic bacteria. Colloids Surfaces B: Biointerfaces 185, 110627. doi: 10.1016/j.colsurfb.2019.110627
Kim, C. H., Choi, J. W., Chun, H. J., Choi, K. S. (1997). Synthesis of chitosan derivatives with quaternary ammonium salt and their antibacterial activity. Polym Bull 38, 387–393. doi: 10.1007/s002890050064
Kim, S. K., Rajapakse, N. (2005). Enzymatic production and biological activities of chitosan oligosaccharides (COS): A review. Carbohydr. Polymers 62 (4), 357–368. doi: 10.1016/j.carbpol.2005.08.012
Koühle, H., Jeblick, W., Poten, F., Blaschek, W., Kauss, H. (1985). Chitosan-elicited callose synthesis in soybean cells as a Ca2+-dependent process. Plant Physiol. 77, 544–551. doi: 10.1104/pp.77.3.544
Kodama, O., Miyakawa, J., Akatsuka, T., Kiyosawa, S. (1992). Sakuranetin, a flavanone phytoalexin from ultraviolet-irradiated rice leaves. Phytochemistry 31 (11), 3807–3809. doi: 10.1016/S0031-9422(00)97532-0
Koike, S. T., Gladders, P., Paulus, A. O. (2007). Vegetable diseases: a color handbook (San Diego, USA: Gulf Professional Publishing).
Kolbert, Z. S., Barroso, J. B., Brouquisse, R., Corpas, F. J., Gupta, K. J., Lindermayr, C., et al. (2019). A forty year journey: The generation and roles of NO in plants. Nitric. Oxide 93, 53–70. doi: 10.1016/j.niox.2019.09.006
Kulikov, S. N., Chirkov, S. N., Il’Ina, A. V., Lopatin, S. A., Varlamov, V. P. (2006). Effect of the molecular weight of chitosan on its antiviral activity in plants. Appl. Biochem. Microbiol. 42 (2), 200–203. doi: 10.1134/S0003683806020165
Kuyyogsuy, A., Deenamo, N., Khompatara, K., Ekchaweng, K., Churngchow, N. (2018). Chitosan enhances resistance in rubber tree (Hevea brasiliensis), through the induction of abscisic acid (ABA). Physiol. Mol. Plant Pathol. 102, 67–78. doi: 10.1016/j.pmpp.2017.12.001
Laflamme, P., Benhamou, N., Bussières, G., Dessureault, M. (2000). Differential effect of chitosan on root rot fungal pathogens in forest nurseries. Can. J. Bot. 77 (10), 1460–1468. doi: 10.1139/b99-111
Lan, W., Wang, W., Yu, Z., Qin, Y., Luan, J., Li, X. (2016). Enhanced germination of barley (Hordeum vulgare L.) using chitooligosaccharide as an elicitor in seed priming to improve malt quality. Biotechnol. Lett. 38, 1935–1940. doi: 10.1007/s10529-016-2181-5
Lapointe, G., Luckevich, M. D., Seguin, A. (2001). Investigation on the induction of 14-3-3 in white spruce. Plant Cell Rep. 20 (1), 79–84. doi: 10.1007/s002990000275
Lee, S. C., Luan, S. (2012). ABA signal transduction at the crossroad of biotic and abiotic stress responses. Plant Cell Environ. 35, 53–60. doi: 10.1111/j.1365-3040.2011.02426.x
Li, R., He, J., Xie, H., Wang, W., Bose, S. K., Sun, Y., et al. (2019). Effects of chitosan nanoparticles on seed germination and seedling growth of wheat (Triticum aestivum L.). Int. J. Biol. Macromolecules 126, 91–100. doi: 10.1016/j.ijbiomac.2018.12.118
Li, B., Liu, B., Shan, C., Ibrahim, M., Lou, Y., Wang, Y., et al. (2013). Antibacterial activity of two chitosan solutions and their effect on rice bacterial leaf blight and leaf streak. Pest Manage. Sci. 69, 312–320. doi: 10.1002/ps.3399
Li, K., Xing, R., Liu, S., Li, P. (2020). Chitin and chitosan fragments responsible for plant elicitor and growth stimulator. J. Agric. Food Chem. 68, 12203–12211. doi: 10.1021/acs.jafc.0c05316
Li, J., Zhuang, S. (2020). Antibacterial activity of chitosan and its derivatives and their interaction mechanism with bacteria: Current state and perspectives. Eur. Polymer J. 138, 109984. doi: 10.1016/j.eurpolymj.2020.109984
Liaqat, F., Eltem, R. (2018). Chitooligosaccharides and their biological activities: A comprehensive review. Carbohydr. Polymers 184, 243–259. doi: 10.1016/j.carbpol.2017.12.067
Ling, Y., Zhao, Y., Cheng, B., Tan, M., Zhang, Y., Li, Z. (2022). Seed Priming with chitosan improves germination characteristics associated with alterations in antioxidant defense and dehydration-responsive pathway in white clover under water stress. Plants 11, 2015. doi: 10.3390/plants11152015
Linhorst, M., Wattjes, J., Moerschbacher, B. M. (2021). Chitin deacetylase as a biocatalyst for the selective N-acylation of chitosan oligo-and polymers. ACS Catalysis 11 (23), 14456–14466. doi: 10.1021/acscatal.1c04472
Liu, H., Du, Y., Wang, X., Sun, L. (2004). Chitosan kills bacteria through cell membrane damage. Int. J. Food Microbiol. 95 (2), 147–155. doi: 10.1016/j.ijfoodmicro.2004.01.022
Liu, H., Tian, W., Li, B., Wu, G., Ibrahim, M., Tao, Z., et al. (2012). Antifungal effect and mechanism of chitosan against the rice sheath blight pathogen, Rhizoctonia solani. Biotechnol. Lett. 34, 2291–2298. doi: 10.1007/s10529-012-1035-z
López-Mora, L. I., Gutiérrez-Martínez, P., Bautista-Baños, S., Jiménez-García, L. F., Zavaleta-Mancera, H. A. (2013). Evaluación de la actividad antifúngica del quitosano en Alternaria alternata y en la calidad del mango’Tommy Atkins’ durante el almacenamiento. Rev. Chapingo. Serie Horticultura 19 (3), 315–331. doi: 10.5154/r.rchsh.2012.07.038
Lopez-Moya, F., Escudero, N., Zavala-Gonzalez, E. A., Esteve-Bruna, D., Blázquez, M. A., Alabadí, D., et al. (2017). Induction of auxin biosynthesis and WOX5 repression mediate changes in root development in Arabidopsis exposed to chitosan. Sci. Rep. 7, 1–14. doi: 10.1038/s41598-017-16874-5
Lopez-Moya, F., Suarez-Fernandez, M., Lopez-Llorca, L. V. (2019). Molecular mechanisms of chitosan interactions with fungi and plants. Int. J. Mol. Sci. 20, 332. doi: 10.3390/ijms20020332
Maciá-Vicente, J. G., Rosso, L. C., Ciancio, A., Jansson, H., Lopez-Llorca, L. V. (2009). Colonisation of barley roots by endophytic Fusarium equiseti and Pochonia chlamydosporia: effects on plant growth and disease. Ann. Appl. Biol. 155 (3), 391–401. doi: 10.1111/j.1744-7348.2009.00352.x
Maleki, G., Woltering, E. J., Mozafari, M. R. (2022). Applications of chitosan-based carrier as an encapsulating agent in food industry. Trends Food Sci. Technol. 120, 88–99. doi: 10.1016/j.tifs.2022.01.001
Malerba, M., Cerana, R. (2020). Chitin-and chitosan-based derivatives in plant protection against biotic and abiotic stresses and in recovery of contaminated soil and water. Polysaccharides 1 (1), 21–30. doi: 10.3390/polysaccharides1010003
Matei, P. M., Buzón-Durán, L., Pérez-Lebeña, E., Martín-Gil, J., Iacomi, B. M., Ramos-Sánchez, M. C., et al. (2020). In vitro antifungal activity of chitosan-polyphenol conjugates against Phytophthora cinnamomi. AgriEngineering 2 (1), 72–77. doi: 10.3390/agriengineering2010005
Mauch-Mani, B., Mauch, F. (2005). The role of abscisic acid in plant–pathogen interactions. Curr. Opin. Plant Biol. 8, 409–414. doi: 10.1016/j.pbi.2005.05.015
Maurya, S., Kumar, S., Jat, K. K., Kumari, P. (2019). Chitosan: A novel bioactive compound for management of plant diseases: A review. J. Pharmacognosy Phytochem. 8 (5), 2281–2286.
Meng, X., Yang, L., Kennedy, J. F., Tian, S. (2010). Effects of chitosan and oligochitosan on growth of two fungal pathogens and physiological properties in pear fruit. Carbohydr. Polymers 81 (1), 70–75. doi: 10.1016/j.carbpol.2010.01.057
Morin-Crini, N., Lichtfouse, E., Torri, G., Crini, G. (2019). Applications of chitosan in food, pharmaceuticals, medicine, cosmetics, agriculture, textiles, pulp and paper, biotechnology, and environmental chemistry. Environ. Chem. Lett. 17 (4), 1667–1692. doi: 10.1007/s10311-019-00904-x
Mukarram, M., Khan, M. M. A., Kurjak, D., Corpas, F. J. (2023). Chitosan oligomers (COS) trigger a coordinated biochemical response of lemongrass (Cymbopogon flexuosus) plants to palliate salinity-induced oxidative stress. Sci. Rep. 13, 8636. doi: 10.1038/s41598-023-35931-w
Mukarram, M., Naeem, M., Aftab, T., Khan, M. M. A. (2022). Chitin, chitosan, and chitooligosaccharides: recent advances and future perspectives. In: Radiation-processed polysaccharides: Emerging Roles in Agriculture Eds. Naemm, M., Aftab, T., Khan, M. M. A. Academic Press, Elsevier Inc. p. 339–353. doi: 10.1016/B978-0-323-85672-0.00012-X
Muley, A. B., Ladole, M. R., Suprasanna, P., Dalvi, S. G. (2019). Intensification in biological properties of chitosan after γ-irradiation. Int. J. Biol. Macromolecules 131, 435–444. doi: 10.1016/j.ijbiomac.2019.03.072
Mwaheb, M. A. M. A., Hussain, M., Tian, J., Zhang, X., Hamid, M. I., El-Kassim, N. A., et al. (2017). Synergetic suppression of soybean cyst nematodes by chitosan and Hirsutella minnesotensis via the assembly of the soybean rhizosphere microbial communities. Biol. Control 115, 85–94. doi: 10.1016/j.biocontrol.2017.09.011
Mwangi, W. W., Ho, K. W., Tey, B. T., Chan, E. S. (2016). Effects of environmental factors on the physical stability of pickering-emulsions stabilized by chitosan particles. Food Hydrocolloids 60, 543–550. doi: 10.1016/j.foodhyd.2016.04.023
Naree, S., Ponkit, R., Chotiaroonrat, E., Mayack, C. L., Suwannapong, G. (2021). Propolis extract and chitosan improve health of Nosema ceranae infected giant honeybees, Apis dorsata Fabricius 1793. Pathogens 10 (7), 785. doi: 10.3390/pathogens10070785
Naskar, S., Sharma, S., Kuotsu, K. (2019). Chitosan-based nanoparticles: An overview of biomedical applications and its preparation. J Drug Deliv Sci Technol 49, 66–81. doi: 10.1016/j.jddst.2018.10.022
Neill, S., Barros, R., Bright, J., Desikan, R., Hancock, J., Harrison, J., et al. (2008). Nitric oxide, stomatal closure, and abiotic stress. J. Exp. Bot. 59, 165–176. doi: 10.1093/jxb/erm293
Nunes da Silva, M., Cardoso, A. R., Ferreira, D., Brito, M., Pintado, M. E., Vasconcelos, M. W. (2014). Chitosan as a biocontrol agent against the pinewood nematode (Bursaphelenchus xylophilus). For. Pathol. 44 (5), 420–423. doi: 10.1111/efp.12136
Nunes da Silva, M., Santos, C. S., Cruz, A., López-Villamor, A., Vasconcelos, M. W. (2021). Chitosan increases Pinus pinaster tolerance to the pinewood nematode (Bursaphelenchus xylophilus) by promoting plant antioxidative metabolism. Sci. Rep. 11, 3781. doi: 10.1038/s41598-021-83445-0
Obara, N., Hasegawa, M., Kodama, O. (2002). Induced volatiles in elicitor-treated and rice blast fungus-inoculated rice leaves. Bioscience Biotechnol Biochem. 66 (12), 2549–2559. doi: 10.1271/bbb.66.2549
Ozeretskovskaya, O. L., Vasyukova, N. I., Panina, Y. S., Chalenko, G. I. (2006). Effect of immunomodulators on potato resistance and susceptibility to Phytophthora infestans. Russian J. Plant Physiol. 53 (4), 488–494. doi: 10.1134/S1021443706040091
Peian, Z., Haifeng, J., Peijie, G., Sadeghnezhad, E., Qianqian, P., Tianyu, D., et al. (2021). Chitosan induces jasmonic acid production leading to resistance of ripened fruit against Botrytis cinerea infection. Food Chem. 337, 127772. doi: 10.1016/j.foodchem.2020.127772
Pereira, A., Sandoval-Herrera, I., Zavala-Betancourt, S., Oliveira, H., Ledezma-Pérez, A., Romero, J., et al. (2017). γ-Polyglutamic acid/chitosan nanoparticles for the plant growth regulator gibberellic acid: Characterization and evaluation of biological activity. Carbohydr. Polymers 157, 1862–1873. doi: 10.1016/j.carbpol.2016.11.073
Pichyangkura, R., Chadchawan, S. (2015). Biostimulant activity of chitosan in horticulture. Scientia Hortic. 196, 49–65. doi: 10.1016/j.scienta.2015.09.031
Pongprayoon, W., Siringam, T., Panya, A., Roytrakul, S. (2022). Application of chitosan in plant defense responses to biotic and abiotic stresses. Appl. Sci. Eng. Prog. 15, 107–158. doi: 10.14416/j.asep.2020.12.007
Pospieszny, H. (1997). Antiviroid activity of chitosan. Crop Prot. 16 (2), 105–106. doi: 10.1016/S0261-2194(96)00077-4
Pospieszny, H., Chirkov, S., Atabekov, J. (1991). Induction of antiviral resistance in plants by chitosan. Plant Sci. 79 (1), 63–68. doi: 10.1016/0168-9452(91)90070-O
Povero, G., Loreti, E., Pucciariello, C., Santaniello, A., Di Tommaso, D., Di Tommaso, G., et al. (2011). Transcript profiling of chitosan-treated Arabidopsis seedlings. J. Plant Res. 124, 619–629. doi: 10.1007/s10265-010-0399-1
Prabaharan, M., Mano, J. F. (2004). Chitosan-based particles as controlled drug delivery systems. Drug Delivery 12 (1), 41–57. doi: 10.1080/10717540590889781
Qi, L., Xu, Z., Jiang, X., Hu, C., Zou, X. (2004). Preparation and antibacterial activity of chitosan nanoparticles. Carbohydr. Res. 339 (16), 2693–2700. doi: 10.1016/j.carres.2004.09.007
Raafat, D., Von Bargen, K., Haas, A., Sahl, H.-G. (2008). Insights into the mode of action of chitosan as an antibacterial compound. Appl. Environ. Microbiol. 74 (12), 3764–3773. doi: 10.1128/AEM.00453-08
Rabea, E. I., Badawy, M. E. I., Rogge, T. M., Stevens, C. V., Höfte, M., Steurbaut, W., et al. (2005). Insecticidal and fungicidal activity of new synthesized chitosan derivatives. Pest Manage. Sci. 61 (10), 951–960. doi: 10.1002/ps.1085
Rabea, E. I., Badawy, M. E. T., Stevens, C. V., Smagghe, G., Steurbaut, W. (2003). Chitosan as antimicrobial agent: Applications and mode of action. Biomacromolecules 4 (6), 1457–1465. doi: 10.1021/bm034130m
Rahman, L., Goswami, J. (2021). Recent development on physical and biological properties of chitosan-based composite films with natural extracts: A review. J. Bioactive Compatible Polymers 36, 225–236. doi: 10.1177/08839115211014218
Rakwal, R., Tamogami, S., Agrawal, G. K., Iwahashi, H. (2002). Octadecanoid signaling component “burst” in rice (Oryza sativa L.) seedling leaves upon wounding by cut and treatment with fungal elicitor chitosan. Biochem. Biophys. Res. Commun. 295 (5), 1041–1045. doi: 10.1016/S0006-291X(02)00779-9
Rashki, S., Asgarpour, K., Tarrahimofrad, H., Hashemipour, M., Ebrahimi, M. S., Fathizadeh, H., et al. (2021). Chitosan-based nanoparticles against bacterial infections. Carbohydr. Polymers 251, 117108. doi: 10.1016/j.carbpol.2020.117108
Reddy, M. V. B., Angers, P., Castaigne, F., Arul, J. (2000). Chitosan effects on blackmold rot and pathogenic factors produced by Alternaria alternata in postharvest tomatoes. J. Am. Soc. Hortic. Sci. 125 (6), 742–747. doi: 10.21273/JASHS.125.6.742
Riseh, R. S., Vatankhah, M., Hassanisaadi, M., Kennedy, J. F. (2023). Chitosan/silica: A hybrid formulation to mitigate phytopathogens. Int. J. Biol. Macromolecules 239, 124192. doi: 10.1016/j.ijbiomac.2023.124192
Roller, S., Covill, N. (1999). The antifungal properties of chitosan in laboratory media and apple juice. Int. J. Food Microbiol. 47 (1–2), 67–77. doi: 10.1016/S0168-1605(99)00006-9
Román-Doval, R., Torres-Arellanes, S. P., Tenorio-Barajas, A.Y., Gómez-Sánchez, A. A., Valencia-Lazcano, A. (2023). Chitosan: Properties and its application in agriculture in context of molecular weight. Polymers 15 (13), 2867. doi: 10.3390/polym15132867
Sabbour, M. M., Solieman, N. Y. (2016). The efficacy effect of using chitosan and nano-chitosan against Tuta absoluta (Lepidoptera: Gelechiidae). J. Chem. Pharm. Res. 8, 548–554.
Sahab, A. F., Waly, A. I., Sabbour, M. M., Nawar, L. S. (2015). Synthesis, antifungal and insecticidal potential of chitosan (CS)-g-poly (acrylic acid) (PAA) nanoparticles against some seed borne fungi and insects of soybean. Int. J. ChemTech Res. 8 (2), 589–598.
Shahrajabian, M. H., Chaski, C., Polyzos, N., Tzortzakis, N., Petropoulos, S. A. (2021). Sustainable agriculture systems in vegetable production using chitin and chitosan as plant biostimulants. Biomolecules 11, 819. doi: 10.3390/biom11060819
Shokri, Z., Seidi, F., Karami, S., Li, C., Saeb, M. R., Xiao, H. (2021). Laccase immobilization onto natural polysaccharides for biosensing and biodegradation. Carbohydr. Polymers 262, 117963. doi: 10.1016/j.carbpol.2021.117963
Silva-Castro, I., Martín-García, J., Diez, J. J., Flores-Pacheco, J. A., Martín-Gil, J., Martín-Ramos, P. (2018). Potential control of forest diseases by solutions of chitosan oligomers, propolis and nanosilver. Eur. J. Plant Pathol. 150, 401–411. doi: 10.1007/s10658-017-1288-4
Singh, R., Shitiz, K., Singh, A. (2017). Chitin and chitosan: biopolymers for wound management. Int. Wound J. 14 (6), 1276–1289. doi: 10.1111/iwj.12797
Struszczyk, M. H. (2002). Chitin and chitosan. Part II. Applications of chitosan. Polimery 47 (6), 396–403. http://polimery.ichp.vot.pl/index.php/p/article/view/1982.
Suarez-Fernandez, M., Sambles, C., Lopez-Moya, F., Nueda, M. J., Studholme, D. J., Lopez-Llorca, L. V. (2021). Chitosan modulates Pochonia chlamydosporia gene expression during nematode egg parasitism. Environ. Microbiol. 23 (9), 4980–4997. doi: 10.1111/1462-2920.15408
Sudarshan, N. R., Hoover, D. G., Knorr, D. (1992). Antibacterial action of chitosan. Food Biotechnol. 6 (3), 257–272. doi: 10.1080/08905439209549838
Tocci, N., Simonetti, G., D’Auria, F. D., Panella, S., Palamara, A. T., Valletta, A., et al. (2011). Root cultures of Hypericum perforatum subsp. angustifolium elicited with chitosan and production of xanthone-rich extracts with antifungal activity. Appl. Microbiol. Biotechnol. 91, 977–987. doi: 10.1007/s00253-011-3303-6
Tsai, G.-J., Su, W.-H. (1999). Antibacterial activity of shrimp chitosan against Escherichia coli. J. Food Prot. 62 (3), 239–243. doi: 10.4315/0362-028X-62.3.239
Vasyukova, N. I., Zinov’Eva, S. V., Il’Inskaya, L. I., Perekhod, E. A., Chalenko, G. I., Gerasimova, N. G., et al. (2001). Modulation of plant resistance to diseases by water-soluble chitosan. Appl. Biochem. Microbiol. 37 (1), 103–109. doi: 10.1023/A:1002865029994
Verlee, A., Mincke, S., Stevens, C. V. (2017). Recent developments in antibacterial and antifungal chitosan and its derivatives. Carbohydr. Polymers 164, 268–283. doi: 10.1016/j.carbpol.2017.02.001
Waewthongrak, W., Pisuchpen, S., Leelasuphakul, W. (2015). Effect of Bacillus subtilis and chitosan applications on green mold (Penicilium digitatum Sacc.) decay in citrus fruit. Postharvest Biol. Technol. 99, 44–49. doi: 10.1016/j.postharvbio.2014.07.016
Walling, L. L. (2000). The myriad plant responses to herbivores. J. Plant Growth Regul. 19 (2), 195–216. doi: 10.1007/s003440000026
Wang, M., Chen, Y., Zhang, R., Wang, W., Zhao, X., Du, Y., et al. (2015). Effects of chitosan oligosaccharides on the yield components and production quality of different wheat cultivars (Triticum aestivum L.) in Northwest China. Field Crops Res. 172, 11–20. doi: 10.1016/j.fcr.2014.12.007
Wang, Z., Huo, X., Zhang, Y., Gao, Y., Su, J. (2022). Carboxymethyl chitosan nanoparticles loaded with bioactive protein CiCXCL20a effectively prevent bacterial disease in grass carp (Ctenopharyngodon idella). Aquaculture 549, 737745. doi: 10.1016/j.aquaculture.2021.737745
Wang, W., Meng, Q., Li, Q., Liu, J., Zhou, M., Jin, Z., et al. (2020). Chitosan derivatives and their application in biomedicine. Int. J. Mol. Sci. 21 (2), 487. doi: 10.3390/ijms21020487
Xing, K., Zhu, X., Peng, X., Qin, S. (2015). Chitosan antimicrobial and eliciting properties for pest control in agriculture: a review. Agron. Sustain. Dev. 35 (2), 569–588. doi: 10.1007/s13593-014-0252-3
Yadav, S., Mehrotra, G. K., Bhartiya, P., Singh, A., Dutta, P. K. (2020). Preparation, physicochemical and biological evaluation of quercetin based chitosan-gelatin film for food packaging. Carbohydr. Polym. 227, 115348. doi: 10.1016/j.carbpol.2019.115348
Yan, C., Xie, D. (2015). Jasmonate in plant defence: sentinel or double agent? Plant Biotechnol. J. 13 (9), 1233–1240. doi: 10.1111/pbi.12417
Yin, H., Fretté, X. C., Christensen, L. P., Grevsen, K. (2012). Chitosan oligosaccharides promote the content of polyphenols in Greek oregano (Origanum vulgare ssp. hirtum). J. Agric. Food Chem. 60 (1), 136–143. doi: 10.1021/jf204376j
Younes, I., Sellimi, S., Rinaudo, M., Jellouli, K., Nasri, M. (2014). Influence of acetylation degree and molecular weight of homogeneous chitosans on antibacterial and antifungal activities. Int. J. Food Microbiol. 185, 57–63. doi: 10.1016/j.ijfoodmicro.2014.04.029
Zhang, P., Chen, K. (2009). Age-dependent variations of volatile emissions and inhibitory activity toward Botrytis cinerea and Fusarium oxysporum in tomato leaves treated with chitosan oligosaccharide. J. Plant Biol. 52 (4), 332–339. doi: 10.1007/s12374-009-9043-9
Zhang, M., Tan, T., Yuan, H., Rui, C. (2003). Compatible polymers insecticidal and fungicidal. J. Bioactive Compatible Polymers 18, 391–400. doi: 10.1177/088391103039019
Zheng, K., Lu, J., Li, J., Yu, Y., Zhang, J., He, Z., et al. (2021b). Efficiency of chitosan application against Phytophthora infestans and the activation of defence mechanisms in potato. Int. J. Biol. Macromolecules 182, 1670–1680. doi: 10.1016/j.ijbiomac.2021.05.097
Zheng, Q., Wang, R., Qin, D., Yang, L., Lin, S., Cheng, D., et al. (2021a). Insecticidal efficacy and mechanism of nanoparticles synthesized from chitosan and carboxymethyl chitosan against Solenopsis invicta (Hymenoptera: Formicidae). Carbohydr. Polymers 260, 117839. doi: 10.1016/j.carbpol.2021.117839
Zhou, Y. G., Yang, Y. D., Qi, Y. G., Zhang, Z. M., Wang, X. J., Hu, X. J. (2002). Effects of chitosan on some physiological activity in germinating seed of peanut. J. Peanut Sci. 31 (1), 22–25.
Keywords: chitosan, biopolymer, antimicrobial, insecticidal, oxidative stress, phytohormones, antioxidants, chitooligosaccharides
Citation: Mukarram M, Ali J, Dadkhah-Aghdash H, Kurjak D, Kačík F and Ďurkovič J (2023) Chitosan-induced biotic stress tolerance and crosstalk with phytohormones, antioxidants, and other signalling molecules. Front. Plant Sci. 14:1217822. doi: 10.3389/fpls.2023.1217822
Received: 05 May 2023; Accepted: 30 June 2023;
Published: 19 July 2023.
Edited by:
Cristiano Fortuna Soares, University of Porto, PortugalReviewed by:
Hyong Woo Choi, Andong National University, Republic of KoreaDalia Gamil Aseel, Arid Lands Cultivation Research Institute (ALCRI), Egypt
Copyright © 2023 Mukarram, Ali, Dadkhah-Aghdash, Kurjak, Kačík and Ďurkovič. This is an open-access article distributed under the terms of the Creative Commons Attribution License (CC BY). The use, distribution or reproduction in other forums is permitted, provided the original author(s) and the copyright owner(s) are credited and that the original publication in this journal is cited, in accordance with accepted academic practice. No use, distribution or reproduction is permitted which does not comply with these terms.
*Correspondence: Mohammad Mukarram, bWRtdWthcnJhbTAwN0BnbWFpbC5jb20=
†ORCID: Mohammad Mukarram, orcid.org/0000-0002-9034-9366
Jamin Ali, orcid.org/0000-0001-7698-5000
Hamed Dadkhah-Aghdash, orcid.org/0000-0002-6281-0186
Daniel Kurjak, orcid.org/0000-0002-2489-8463
František Kačík, orcid.org/0000-0002-8989-0833
Jaroslav Ďurkovič, orcid.org/0000-0003-2351-7638