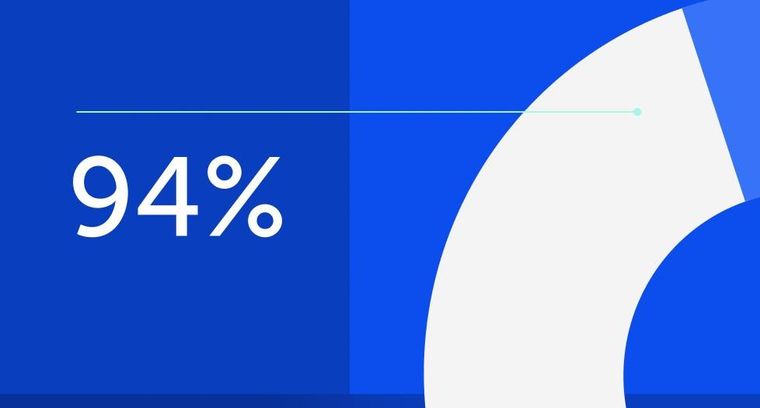
94% of researchers rate our articles as excellent or good
Learn more about the work of our research integrity team to safeguard the quality of each article we publish.
Find out more
REVIEW article
Front. Plant Sci., 05 September 2023
Sec. Crop and Product Physiology
Volume 14 - 2023 | https://doi.org/10.3389/fpls.2023.1206829
This article is part of the Research TopicMaize Stress Ecophysiology and RegulationView all 10 articles
The growth of yield outputs is dwindling after the first green revolution, which cannot meet the demand for the projected population increase by the mid-century, especially with the constant threat from extreme climates. Cereal yield requires carbon (C) assimilation in the source for subsequent allocation and utilization in the sink. However, whether the source or sink limits yield improvement, a crucial question for strategic orientation in future breeding and cultivation, is still under debate. To narrow the knowledge gap and capture the progress, we focus on maize, rice, and wheat by briefly reviewing recent advances in yield improvement by modulation of i) leaf photosynthesis; ii) primary C allocation, phloem loading, and unloading; iii) C utilization and grain storage; and iv) systemic sugar signals (e.g., trehalose 6-phosphate). We highlight strategies for optimizing C allocation and utilization to coordinate the source–sink relationships and promote yields. Finally, based on the understanding of these physiological mechanisms, we envisage a future scenery of “smart crop” consisting of flexible coordination of plant C economy, with the goal of yield improvement and resilience in the field population of cereals crops.
Global primary food production needs to double by 2050 to meet the growing demand for food and nutrition (Grassini et al., 2013; Ray et al., 2013). Simultaneously, there is increasing pressure from sustainable development and global climate change, including bioenergy demand, arable land constraints, and extreme weather (Clark et al., 2020; Ortiz-Bobea et al., 2021). Crops in the future will have to be “resilient” and “smart” to cope with unpredictable stresses and thus increase yields in practice. Carbohydrates are pivotal for a crop to balance its maintenance, growth, and yield formation, providing a carbon (C) skeleton, energy substrates, and indispensable sugar signals. Assimilation of C in leaves by light energy conversion export to growing shoots and root systems in specific spatiotemporal patterns is mediated by transporters. Many relevant reviews, based mainly on new findings on carbohydrate transport, sugar sensing, and systemic improvement in model plants, such as Arabidopsis and tobacco, have been published (for example, Rolland et al., 2006; Ruan, 2014; Fichtner and Lunn, 2021; Burgess et al., 2023). Maize, wheat, and rice are major food crops worldwide, but their carbohydrate flow and correlated regulation measures have not been well summarized. Here, we briefly review the pathways of C fixation, transport, and storage, mainly in the three cereals, and the key sugar signals that have come to light in systemic regulation during the past few years. We discuss published strategies for the regulation of the plant C economy (“C economy” in this paper refers to the production, circulation, and use of carbohydrates) for crop yield and resilience. We proposed that “systemic enhancement” from source to sink together with “specific optimization” in C allocation management depending on spatial–temporal demand may be the way for both crop yield potential and stress resistance and/or resilience.
Plants convert light energy into chemical energy via photosynthesis (Figure 1A). The theoretical maximum efficiencies of photosynthetic energy conversion are approximately 4.6% and 6% for C3 and C4 plants, respectively, as estimated using biomass (Zhu et al., 2010). However, efficiency in the real world, even under favorable conditions, is only half or less than the theoretical value (Yin and Struik, 2015). As a well-studied pathway, the limitations of photosynthesis have been modeled. The improvement of the photosynthetic system is mainly projected into three phases: near-term (including photorespiration bypass, canopy structure improvement, RuBP regeneration, and chlorophyll optimization), mid-term (including photoprotective recovery and RubisCO carboxylation improvement), and long-term (including RubisCO oxygenase decline, mesophyll conductance, and conversion of C3 to C4) (Zhu et al., 2010; von Caemmerer and Furbank, 2016; Leister, 2023).
Figure 1 Carbon fixation and sugar flux from leaves to grains, taking maize plants as an example. (A) C4 photosynthesis in maize leaves in mesophyll and bundle sheath cells. C3 is also shown in gray in mesphyll cells only. (B) Primary carbon partitioning in the diurnal cycle, demonstrating three fates of leaf sugars: glycolysis, temporary storage in chloroplasts or vacuoles, and transport as sucrose; (C) Phloem loading of sucrose by SWEET and SUT; (D) Sucrose retrieval in vascular; (E) Phloem unloading from maternal to filial tissues; (F) Starch synthesis in endosperm cells. ADPG, adenosine 5’-diphosphoglucose; AGPase, ADPG pyrophosphorylase; BE, branching enzymes; CBC, Calvin Benson cycle; CC, companion cells; CWIN, cell wall invertase; DBE, debranching enzymes; DHAP, dihydroxyacetone phosphate; Fru, fructose; GBSS, Granule-bound starch synthase; Glu, glucose; G3P, glucose 3-phosphate; INV, invertase; ISA, Mal, maleic acid; Mat, maltose; MT, monosaccharide transporter; OAA, oxaloacetic acid; PEP, phosphoenolpyruvate; RUBP, ribulose 1,5-bisphosphate; SE, sieve elements; SS, soluble starch synthase; SWEET, sugars will eventually be exported transporters; SUT, sucrose transporter; SuS, sucrose synthase; PGM, phosphoglucomutase; Pyr, pyruvate; SSS, soluble starch synthase; TCA cycle, tricarboxylic acid cycle; TP, triose phosphate. UDPG, Uridine 5’-diphosphoglucose; UTP, uridine triphosphate.
In the past two decades, researchers have proposed yield-improvement strategies based on enhanced photosynthesis using synthetic biology or gene editing in Arabidopsis and tobacco (Long et al., 2018; Hart et al., 2019; Papanatsiou et al., 2019; Chen et al., 2020; Lopez-Calcagno et al., 2020). A few recent studies have shown promise for field crops. One synthetic photorespiratory pathway boosted tobacco biomass by up to 40%, whereas another led to an observable increase in photosynthesis and grain yield under high light in field-grown rice plants (Shen et al., 2019; South et al., 2019). Transgenic rice overexpressing a RuBisCO subunit improved yield performance and nitrogen (N) use efficiency for biomass production when receiving sufficient N fertilization in an experimental paddy field (Yoon et al., 2020). The total spikelet number of transgenic rice did not change, but the ratio of filled spikelets increased, resulting in a 20%–28% higher yield than the wild type (Yoon et al., 2020). In Arabidopsis and soybean, a large increase in grain yield under fluctuating light conditions was achieved by accelerating the recovery from photoprotection. However, when lodging (caused by storms) and/or reduced cloud cover resulted in a lack of sun-flecks in the canopy, the yield gain brought by genetically modified soybeans disappeared (Kromdijk et al., 2016; De Souza et al., 2022). These examples show that increases in photosynthetic efficiency can improve crop yield, under nutrient availability or specific light conditions. However, under adverse conditions such as drought or barren, which occur frequently around the world today, the yield gains shrink or disappear. As Sinclair et al. (2019) refuted, photosynthesis depends on N (and other nutrients). When nitrogen is reduced, plants with high photosynthetic efficiency may not increase crop yield but will cause resource competition and waste, further leading to insufficient transport of sugars into sink tissues. Cross-scale model studies validated that under water-limited conditions, high photosynthetic efficiency could lead to early consumption of soil water and later-growth-period drought and reduce crop yield; however, under water-abundant conditions, improvement of RubisCO and SeBP increased the yield of wheat and sorghum in Australia (Wu et al., 2019a; Wu et al., 2023). In contrast, elevated CO2 and phosphate pools have been modeled to synergistically enhance C3 photosynthesis (Khurshid et al., 2020).
Scholars engaged in photosynthetic efficiency research have notice that proper field nutrition management and coordinated plant C economy are critical to yield as photosynthesis. Transgenic rice plants with modified photorespiration and enhanced photosynthesis undergo massive grain abortion, consistent with a marked reduction in sugar transport from source to sink, as tracked by 13C isotope labeling (Wang et al., 2020). Therefore, sustainable C economic growth of plants is proposed here to depend on further timely and reasonable allocation and utilization after photosynthesis enhancement.
While photosynthesis occurs only in light, growth and respiration occur throughout the day–night cycle (Smith and Stitt, 2007). The immediate photo-assimilate is partitioned into a fraction for glycolysis consumption, a fraction for sucrose transport, and a fraction for temporary storage in leaves and remobilized during the night (Figure 1B). In Arabidopsis, starch is the main transitional reserve in leaves (up to 50% or more) and is synthesized and degraded linearly in a diurnal cycle to maximize C utilization and prevent starvation in changing environments (Stitt and Zeeman, 2012; Ruan, 2014). Furthermore, precisely controlled starch turnover has been shown to be negatively correlated with growth rate or biomass accumulation (Cross et al., 2006; Sulpice et al., 2009; Sulpice et al., 2014), reflecting that the more C reserved in leaves, the less sink obtained for growth. Similarly, the ratio of daily starch accumulation to net C assimilation is negatively correlated with the ground biomass and final yield of three representative maize hybrids (Liang et al., 2019). Under low light or prolonged darkness, maize leaves allocate an even lower proportion of reduced photosynthetic products to sink ends, such as the developing ears, leading to biomass and/or yield losses (Liang et al., 2020; Liang et al., 2021). Thus, it may be possible to increase crop yield potential and resilience by allocating more primary C to sinks under certain circumstances (Oszvald et al., 2018). However, compared to the starchy leaves in Arabidopsis, crops such as wheat and rice prefer soluble sugar leaves, while maize leaves are intermediate (Smith and Stitt, 2007; Liang et al., 2021), the role of C storage in cereal leaves deserves further validation.
Some transporters are directly responsible for the primary C allocation. Sucrose transporter 2 (SUT2), located on the vacuolar membrane, transiently stores sucrose for subsequent growth in cereals (Leach et al., 2017; Prasad et al., 2023). Both loss-of-function mutants of ossut2 and zmsut2 exhibit severe growth restriction and accumulate more sucrose, fructose, glucose, and starch in the leaves (Eom et al., 2011; Leach et al., 2017). Rice cultivars with increased yield under elevated CO2 conditions exhibited elevated expression of OsSUT1 and OsSUT2 and increased photosynthetic capacity of flag leaves, suggesting that enhanced export can prevent inhibition of photosynthesis by sugar accumulation (Zhang et al., 2020a). Other transporters that control sugar transport across vacuole in mesophyll cells, such as the tonoplast monosaccharide transporters (TMTs, TMT1, 2), the class IV sugars will eventually be exported transporters (SWEETs, SWEET16, 17), has also been shown to regulate plant growth and stress resistance in several species (Wingenter et al., 2010; Liu et al., 2022b; Zhu et al., 2022). But as far as we know, related studies on major cereal crops are rare.
In summary, our preliminary findings indicate that daily C turnover in expanded leaves is directly related to crop growth and resistance. Boosting diurnal leaf sucrose export appears to be a strategy to improve cereal yield or stress tolerance in sink tissues, at least in some cases. However, it remains unclear whether this sacrifices the ability of the source leaves to survive extreme stress with fewer sugar reserves. The primary C allocation characteristics and underlying physiological mechanisms in different crops and varieties require further investigation.
Long-distance sugar transport requires phloem loading and unloading to coordinate leaf C supply and sink growth in different environments. Phloem tissue is composed of three cell types: companion cells (CC), sieve elements (SE), and phloem parenchyma cells (PP), which act as highway linking sources and sinks for sugar transport. Proteins responsible for sugar transport, including SUT, SWEETs, and monosaccharide transporters (MTs), have receive much attention (Julius et al., 2017; Braun, 2022; Xue et al., 2022; Singh et al., 2023). Here, we focus on maize, rice, and wheat and discuss the importance of the coordination of C transport for crop production in some recent cases.
Sucrose, the principal sugar for transport, is produced in mesophyll cells (C3) or bundle sheath cells (C4) and moves into adjacent phloem parenchyma cells through plasmodesmata (symplastic movement). In the subsequent apoplastic transport, sucrose is excreted into the intercellular space by clade III SWEETs and collected by SUT1, which is located on the plasma membrane of CC cells against the concentration gradient in the CC–SE complex (Figure 1C; Xue et al., 2022). Mutants of Zmsut1 and Ossut1 are severely debilitated in growth and grain filling (Scofield et al., 2002; Slewinski et al., 2009). However, both mutants could grow to maturity and produce fertile seeds, suggesting that sugars could be transferred by other SUTs (perhaps OsSUT5 in rice, see Wang et al., 2021b) or paths. Through hydrostatic pressure established in the phloem, sucrose is transported to the sink organ by the SE, where sucrose leakage is retrieved by SUT1 during long-distance transport (Figure 1D; Ohshima et al., 1990; Knoblauch et al., 2016). Sucrose unloading occurs symplasmically in growing radicles and shoot apices, while in cereal grains, SWEETs, SUT1, cell wall invertases (CWINs), and MTs are required for maternal-to-filial transport (Figure 1E; Apoplastic path, see details below; Haupt et al., 2001; Dhungana and Braun, 2021; Ruan, 2022; Shen et al., 2022).
SWEETs may be among the most complex sugar transporter families (Chen et al., 2012; Eom et al., 2015; Xue et al., 2022; Singh et al., 2023). The number of SWEETs are 24, 21, and 59, while the SUTs are 5, 5, and 18 in maize, rice, and wheat, respectively (Zhu et al., 2022; Prasad et al., 2023). In maize, SWEET13, including ZmSWEET13a, b, and c, is responsible for sucrose efflux to the SE–CC complex. The triple-knockout mutants exhibited similar but milder growth to Zmsut1, implying greater genetic redundancy among clade III SWEETs (Bezrutczyk et al., 2018). Similarly, OsSWEET11, 13, 14, and 15 are expressed in the rice leaf phloem and are thought to play a role in phloem loading (Yuan et al., 2014; Eom et al., 2019; Mathan et al., 2021a). Single-knockout mutants of Ossweet 11, 13, and 14 showed milder or no yield penalty, whereas double-knockout mutants of Ossweet11 and 14 had severe phenotypes (Eom et al., 2019; Fei et al., 2021). Blocking sugar transmembrane loading by overexpressing CWIN or by knocking down OsDOF11 (DNA binding with one finger 11), which binds and activates gene expression of OsSUT1, OsSWEET11, and OsSWEET14, resulted in restricted vegetable growth and decreased grain yields (Wu et al., 2018; Wang et al., 2021b). In contrast, enhanced apoplastic phloem loading under low nitrogen conditions was attributed to increased gene expression of OsSUT1, OsSWEET11, and OsSWEET14 in leaves and stems (Li et al., 2022a). Furthermore, the lack of symplastic connection between the SE–CC complex and surrounding parenchyma cells in leaves and stems was verified by the phloem-mobile symplastic tracer carboxyfluorescein (Li et al., 2022a).
Both SUTs and SWEETs are believed to have undergone post-domestication selection for higher-caloric harvests (Sosso et al., 2015; Mathan et al., 2021a; Singh et al., 2023). Researchers have attempted to modify the expression of SUT1 and/or clade III SWEETs to coordinate C transport. However, unexpectedly, constitutive overexpression of OsSWEET11, OsSWEET14, OsDOF1, OsSUT1, and OsSWEET11 and 14 in rice resulted in attenuated growth and yield penalty, similar to the AtSWEET11 and 12 OE lines in Arabidopsis (Gao et al., 2018; Kim et al., 2021; Singh et al., 2021; Fatima et al., 2023). Surprisingly, the OsDOF11 and OsSWEET 14 OE lines showed improved resistance to plant pathogens, such as Xanthomonas oryzae pv oryzae and Rhizoctonia solani, which are known to induce the expression of SWEETs for sugar secretion and nutrition hijacking (Eom et al., 2019; Oliva et al., 2019; Wu et al., 2022). Constitutive overexpression of SWEET may induce a series of plant defense reactions, leading to a trade-off between growth and resistance (Xue et al., 2022). Thus, specific regulations are more reasonable. Field rice plants overexpressing AtSUT2 under the control of a phloem-specific promoter, showed a 16% increase in grain yield (Wang et al., 2015). Tissue-specific activation of OsDOF11 increases both yield and resistance to R. solani (Kim et al., 2021). More ingeniously, by creating genomic mutations in the SWEET (OsSWEET11, 13, 14)-specific promoter, where pathogen-secreted transcription activator-like effectors bind to induce gene expression, endowing rice lines with robust, broad-spectrum resistance (Eom et al., 2019; Oliva et al., 2019).
Sugar loading and unloading clues and the significance of these transporters in improving crop performance remain to be explored. First, new sugar transporters are yet to be discovered, although it has been suggested that most sugar transporters have been identified (Chen et al., 2015). Recently, a nitrate transporter 1/peptide transporter family member named ZmSUGCAR1 was shown to carry both sucrose and glucose for grain filling and was proposed to be conserved in wheat and sorghum (Yang et al., 2022). Whether other nitrate/peptide transporters in this family are involved in sugar transport and whether substrate competition affects transporter selectivity is unclear. Second, the substrate selectivity of sugar transporters in crops and their correlation with growth and abiotic stress resistance need to be explored. Clade III SWEETs and clade I SWEET3a glucose transporters can transport gibberellin hormones in addition to sugars (Morii et al., 2020; Wu et al., 2022). OsSWEET13 and 15 were strongly expressed under drought, salt, and ABA treatment, which revealed that the ABA-responsive transcription factor OsbZIP72 directly binds to the promoters of OsSWEET13 and 15 and activates their expression, likely to improve the root-shoot ratio for higher tolerance (Mathan et al., 2021b; Chen et al., 2022a). Third, functional redundancy within the family or clade and interactions among different types of sugar transporters are largely unknown. In general, exploration of the above issues would further deepen our understanding of the critical role of sugar transporters in coordinating the plant C economy and simultaneously improving crop yield and resilience.
Besides the endosperm and embryo, cereal grains also comprise multiple distinctive or even transgenerational tissues, such as the maternal placentachalaza in maize, filial basal endosperm transfer cells (named endosperm transfer cells in wheat), and embryo-surrounding region (ESR) (see details in Liu et al., 2022a; Shen et al., 2022). Within developmentally specific but functionally coordinated tissues, sugar transporters and CWIN set a typical manifestation in which their locations mandate functions in phloem unloading and determine grain development. We recently built a holistic view of sugar transporters that control sucrose unloading in maize grains (Shen et al., 2022). ZmSWEET11 and 13b located in the placento-chalazal zone, expel sucrose into the apoplasmic space and, ZmSUT1, ZmSWEET11/13a (sucrose transporters), and ZmSTP3, ZmSWEET3a/4c (monosaccharide transporters), located in the basal endosperm transfer cells, retrieved sucrose or hexoses after hydrolysis by CWIN (Figure 1E). Sucrose could be further transported by the embryo-surrounding region (ESR) located in ZmSWEET14a/15a, broken down by the ESR-embryo junction located in CWIN, and retrieved by embryo-located ZmSUT4 for embryo development (Shen et al., 2022). Sucrose synthase (SUS) and invertase are responsible for sucrose cleavage in the endosperm and embryo. Similarly, sucrose or monosaccharides derived from GIF1 (also named OsCWIN2) in the vascular bundle are transported by OsSWEET11, 14, and 15 in rice. OsSUT1, 3, and 4, OsSWEET4, 11, and 14, as well as possibly OsMT4 and 6, are responsible for transporting sugar to the aleurone layer for grain growth and C storage (Ma et al., 2017; Yang et al., 2018; Solomon and Drea, 2019; Fei et al., 2021; Li et al., 2022b; Liu et al., 2022a). Although the assimilate acquisition route in wheat differs that from rice and maize (Solomon and Drea, 2019; Liu et al., 2022a), the transporters responsible for apoplast transport may be similar. A recent study showed that TraesCS4B02G287800 and TraesCS4D02G286500 (homologous to OsSUT1), and TraesCS2D02G293200 and TraesCS2B02G311900 (homologous to OsGIF1) are involved in low-light induced sugar transport in wheat grains (Yang et al., 2023).
CWIN is proposed to play a major role in early grain development, probably promoting glucose-activated nuclear division for large endosperm capacity and embryo fertility (Ruan, 2014; Ruan, 2022). Both mutants of Mn1 (also named ZmCWIN2) in maize and the ortholog gene OsGIF1 in rice exhibited reduced grain size, indicating the irreplaceable roles of hexose supply and sugar signaling generated by CWIN (Wang et al., 2008; Li et al., 2013). Ubiquitously expressed Mn1 has the highest expression in developing maize seeds, specifically at the grain set stage (Li et al., 2013). Constitutive overexpression of AtGIF1, OsGIF1, or Mn1 in the maize inbred line Ye478 results in increased grain number, grain weight, starch content, and final yield (Li et al., 2013). In rice, 35S or Waxy-promoted ectopic expression of the OsGIF1 gene showed small grains similar to the gif1 mutant, but the native promotor-driven OsGIF1 increased yield production (Wang et al., 2008). The interactions between CWIN and sugar transporters remain largely unknown. CWIN is co-expressed with hexose transporters located at the plasma membrane of sinks. Sosso et al. (2015) proposed that SWEET4-mediated hexose transport acts downstream of a CWIN in maize and rice. Both ZmSWEET4c and OsSWEET4 mutants are defective in seed-filling (Sosso et al., 2015).
Sugar transporters and invertases in phloem loading and unloading are essential for yield. However, the specific function of each transporter and its responses to C availability (and external stimuli) need to be elucidated. During drought-induced kernel abortion, C shortage suppressed ZmSWEET effluxers (located on the PC and ESR), CWIN, and SUS, but stimulated ZmSTPs and ZmSUTs, which are responsible for sugar uptake in filial tissues (Shen et al., 2020; Shen et al., 2022). When the C supply was boosted, the doomed kernels were reformed, and drought-induced changes in the transporters were mostly prevented (Shen et al., 2022). Sugar signals may regulate transporters and their coordination; however, the specific mechanisms remain unclear. In addition, how these proteins respond to other signaling pathways, such as phytohormones, remains largely unknown.
After maize kernel capacity was established, CWIN expression was repressed and sucrose was directly transported into the endosperm in maize, where SUSs such as shrunken1 (ZmSh1) and sucrose synthase 1, 2, and 4 (ZmSUS1/2/4) were highly expressed during the grain-filling stage (Figure 1F; Larkins, 2017; Shen et al., 2022). Starch is the main storage site in the cereal endosperm, accounting for more than 70% of the endosperm dry weight. Starch synthesis is highly regulated by enzymes including adenosine diphosphate (ADP)-glucose pyrophosphorylases (AGPases), soluble starch synthases (SSs), granule-bound starch synthases (GBSSs), starch branching enzymes (BEs), and starch debranching enzymes (DBEs) (Jeon et al., 2010). Interestingly, the order of starch accumulation in different parts of one grain is highly conserved in maize, rice, and probably wheat, starting from the distal end of the sugar unloading position and gradually moving to the proximal end (Chen, 2022; Liu et al., 2022a). Sucrose, but not hexose, is thought to be resynthesized at the base of the maize endosperm (the site of sugar unloading) and transported to the site of starch synthesis, which is inconsistent with the substantial upregulation of SUSs during grain filling (Shannon et al., 1986; Olsen, 2020; Shen et al., 2022). AGPase is a key enzyme in starch synthesis. Overexpression of AGPase or SS has been reported to increase cereal grain weight, starch content, and yield (Smidansky et al., 2002; Tian et al., 2018; Paul et al., 2020). A recent study engineered heat-stable 6-phosphogluconate dehydrogenase in maize to improve grain yield under heat stress (Ribeiro et al., 2020). By altering these critical metabolic enzymes, yield performance can be improved by increasing sink demand; however, further efforts are needed in field applications.
Many of the details related to sugar sensing, signaling, and crosstalk with phytohormones and environmental nutrients are largely performed in model plants (Wu et al., 2019b; Baena-Gonzalez and Lunn, 2020; Fichtner et al., 2021; Li et al., 2021; Meng et al., 2022), while for cereals, the understanding of sugar signal transduction and regulation is still insufficient. Here, we briefly introduce the core networks of sugar sensing and signaling. Specifically, recent examples of the regulation of trehalose-6-phosphate in cereal crops are discussed, with the aim of revealing the potential of systemic regulation to coordinate source-sink C balance and synchronously enhance crop yield and resilience.
There are two main mechanisms for sensing and transducing sugar signals in plants, called: direct and indirect (Figure 2; Li et al., 2021). The former is triggered by sugar-binding sensors, such as the glucose signaling sensor hexokinase (HXK), and possibly the regulators of G-protein signaling1 (RGS1), trehalose 6-phosphate (T6P) synthase1 (TPS1), and T6P phosphatase (TPP). The latter includes sugar-derived bioenergetic molecules and metabolite-regulated signaling proteins, such as the glucose-activated target of rapamycin (TOR) and sugar-inhibited SNF1-related protein kinase 1 (SnRK1). HXK1 controls multiple biological processes, including photosynthesis, phytohormone production, growth, and senescence, which are uncoupled from sugar metabolism (Moore et al., 2003). T6P, known as plant “insulin” is a key signal indicating sucrose availability and regulating sucrose homeostasis systemically (Fichtner and Lunn, 2021). TOR kinase acts as a GPS of nutrient, energy, and environmental cues to orchestrate growth and development, whereas SnRK1 antagonizes TOR by phosphorylating RAPTOR, a subunit of the TOR complex (Figure 2A). The SnRK1 complex plays a central role in nutrient sensing and stress responses and is activated under nutrient deprivation, such as darkness and starvation, but is inhibited by sugar phosphates, such as glucose 1-phosphate and T6P (Zhang et al., 2009; Li et al., 2021). By downregulating anabolism and upregulating catabolism, SnRK1 restores cellular energy homeostasis and coordinates tissue response to the environment.
Figure 2 Systemic sugar signals. (A) Interactions in core sugar sensing and signaling; (B) Schematic diagram of source-sink carbon balance regulated by a possible T6P/SnRK1 complex in cereals. Recent reports on the regulation of T6P signaling in maize, rice, and wheat have been summarized. bZIP, The basic leucine zipper domain; F6P, fructose 6-phosphate; G6P, glucose 6-phosphate; HXK, hexokinase; INV, invertase; RGS1, regulator of G-protein signaling1; SnRK1, sucrose non-fermenting related kinase 1; T6P, trehalose 6-phosphate; TOR, target of Rapamycin; TPP, T6P phosphatase; TPS, T6P synthase; TRE, trehalase; UDPG, Uridine 5’-diphosphoglucose.
T6P is an intermediate in the trehalose biosynthesis pathway mediated by TPS1 and TPP. As a signal and regulator of sucrose status, T6P functions, at least partly, if not all, through the inhibition of SnRK1, and trehalose has long been implicated in plant biotic and abiotic stress responses (Baena-Gonzalez and Lunn, 2020). Recent studies on the regulation of T6P have shown promising results in major crops, with a significant increase in both yield and stress resistance or resilience (Figure 2B; Paul et al., 2018). In maize, floral promoter OsMads6 driven overexpression of the rice TPP1 gene in developing maize ears improved yield under both drought and non-drought conditions over multiple field sites and seasons (Nuccio et al., 2015). OsMads6 is most active in phloem CC cells in florets and piths, leading to the largest decrease in T6P levels, but significantly increased expression of SWEETs in these tissues. Hence, an increase in both sucrose in ear spikelets and photosynthesis in leaves during the flowering period can be explained by boosted phloem unloading (Nuccio et al., 2015; Oszvald et al., 2018). A common drought-induced phenomenon in maize production is the abortion of apical kernels, where the expression of TPS is elevated, but TPP is lower when compared to set kernels. Abortion can be largely rescued by synchronous pollination and/or incomplete basal pollination, accompanied by downregulation of TPS and upregulation of TPP expression (Shen et al., 2018; Shen et al., 2020). ZmTPS9 was recently identified as a non-starch pathway gene that contributes to starch synthesis. Gene editing to knockout ZmTPS9 results in increased starch accumulation and kernel weight (Hu et al., 2021).
The contributions of T6P, TPS, and TPP genes to source- and sink-related traits were further confirmed by gene-based mapping and T6P-precursors in wheat (Griffiths et al., 2016; Lyra et al., 2021). Using plant-permeable analogs and sunlight-triggered release of T6P, a chemical intervention was proposed to increase wheat grain yield spraying during grain filling and to prevent drought during the vegetative stage (Griffiths et al., 2016). However, unlike maize, the increased yield was associated with increased T6P in wheat grains, suggesting that the role of T6P may differ among species. Another possibility is that T6P has different effects on the source and the sink. The sprayed T6P-precursors to the ears only or to the whole plant cannot enter the grain without affecting other tissues, although the T6P in the grain was elevated (Griffiths et al., 2016). T6P in other parts (including leaves, glumes, and vasculature) is not known, and there may be huge differences in the pericarp and endosperm in one grain owing to unclear transport characteristics. T6P-precursors spraying not only increased gene expression related to the cell wall, starch, and protein synthesis in grains, and increased sugar availability in new-born leaves after post-drought spraying, but also increased photo-assimilation and preserved less C in source tissues, including flag leaves, aging leaves, and potentially pericarps (Liang, 2019). As a systemic signaling pathway, the role of T6P in cereal crops may be similar, but there are existing spatiotemporal differences.
In general, we propose that the increase in T6P could accelerate sucrose transport and/or conversion in source tissues (here, where net C outcomes above zero are defined as “source”) and hence boosts photosynthesis in leaves, while increased sugar accumulation in the sink tissues (where net C incomes above zero are defined as “sink”) could be associated with decreased T6P (Figure 2B). Consistent with this speculation, TaTPP-7A was detected as a QTL that was significantly associated with grain weight, and overexpression of TaTPP-7A greatly enhanced grain weight and wheat yield (Liu et al., 2023). Overexpression of OsTPP7 located in coleoptile tips enhanced rice germination under both anaerobic stress and an aerobic environment by stimulation of endosperm starch remobilization, whereas Ostpp1 mutants germinated slower than the wild type (Kretzschmar et al., 2015; Wang et al., 2021a). Recently, a sugar-inducible rice transcription factor, OsNAC23, was found to directly repress OsTPP1 expression to simultaneously elevate T6P, thereby facilitating C partitioning from the source to sink. Plants overexpressing OsNAC23 showed elevated T6P levels, sugar transport, and photosynthesis in flag leaves and increased sink organ size and rice yields in three elite-variety backgrounds and two locations (Li et al., 2022c). These results further confirmed our speculation and showed promise for future T6P modulation of both yield potential and resistance/resilience (Figure 2B). Future research may need to explore different strategies to regulate T6P in sources and/or sinks and to further clarify the roles of different TPSs and TPPs in the processes of yield production and resilience.
High photoassimilation is the basis for yield and resistance only if the carbohydrates can be effectively stored or transported in downstream processes. First, triose phosphate (TP) from the Calvin cycle must be exported from the chloroplast into the cytoplasm in exchange for inorganic phosphate (Pi). High TP in chloroplasts results in high levels of 3-phosphoglyceraldehyde, low Pi, and photosynthetic inhibition, thereby activating AGPase and starch synthesis. However, a low TP leads to the opposite (Mugford et al., 2014). Starch accumulation in leaves can relieve photosynthetic inhibition but is negatively correlated with maize growth (Liang et al., 2019; Liang et al., 2020). Hence, increasing P availability could be a feasible way to improve the yield of photosynthetically improved crops (Khurshid et al., 2020). Second, excessive soluble sugars in the cytoplasm also feedback-inhibit photosynthesis, which could be alleviated by TMT-mediated temporary storage in the vacuole or by an efficient transport system that is co-controlled by SWEETs and SUT1 in leaves. Third, stems have multiple roles in carbohydrate coordination, acting as a transfer tissue, temporary sink, and donator as needed (Slewinski, 2012). Regulation of stem sugars has been shown to boost yield and resilience as early as the first green revolution. Finally but most importantly, sugars loaded into the phloem must be utilized promptly and appropriately. Sugar unloading strength and sink growth activity ultimately determine the persistence of the source strength.
There is still no verdict about the debate on whether cereal yield is source or sink-limited. Researchers focusing on the source (e.g., photosynthesis system) and sink (e.g., sugar unloading and utilization) both believe that their work could solely improve yield output, while usually reaching an opposite or unexpected result. The so-called “source limitation” or “sink limitation” is more a matter of, at least partly, synergies between organ growth, phase transition, and environmental changes. As mentioned above, the flow of C (and other nutrients) in plants determines that the development of the source-sink relationship always maintains a dynamic balance, rather than mutual independence or even antagonism. The status between the source and sink can be described as Yin and Yang in Tai Ji, the two opposing and unifying principles in nature (Figure 3). Uncoordinated relationships in the crop are generally divided into two cases, i.e., sufficient source supply with insufficient sink demand and the opposite, in which the plant as a whole system tries to turn to but probably never attains a balance. There is no doubt that the strengthening or weakening of one can pull or feedback inhibits the development of the other. For example, evaluated leaf photosynthesis by CO2 concentration increased yield performance, whereas a larger sink capacity facilitated higher power of C fixation and higher ratios of transfer (Arp, 1991; Paul and Foyer, 2001; O'Leary et al., 2015).
Figure 3 The dialectical relationship between source and sink from a systemic perspective with the example of carbohydrates. This relationship is described as the balance of Yin and Yang, the wisdom crystallization of ancient Chinese. The different scenarios of the source-sink relationship during the grain set and filling stages are briefly summarized. It is proposed that the C supply from the source determines the sink capacity during the grain-set stage, whereas in the grain-filling stage, the realization of a potentially high yield requires a high-level balance between the source and sink. C, carbon; Suc, sucrose.
The question is, will the improvement of one be sufficient to stimulate the other to achieve a high level of source-sink balance? A combined approach using both ‘pull’ and ‘push’ has been reported for yield improvement (Rossi et al., 2015). The long time-scale adaptation of the crop to the environment makes it difficult to bring out ideal results by one or two gene modifications, although silencing or knockout of particular genes has resulted in phenotype defects. Metabolic engineering also suggests that an optimal balance of enzyme activities is more important than simply overexpressing a suite of enzymes (Sweetlove et al., 2017). Therefore, rather than improving only the source or sink, systemic improvement and whole-plant C balance may be more important for crop production. This can be achieved by introducing multiple targeted engineered genes from both the source and sink tissues, such as transporters, critical enzymes, and systemic signals, into crops.
Empirically, crop yield and stress resistance often contradicts each other. However, by optimizing the plant C economy, several genetic strategies have emerged that can increase crop tolerance to stress while increasing, or at least not reducing, yield. Editing of the SWEETs promoter mentioned above is a precise strategy to improve disease resistance while maintaining functional SWEETs for crops (Eom et al., 2019; Oliva et al., 2019). ABA is a key response signal for abiotic stresses such as drought. Sustained ABA signaling is considered to significantly increase plant resistance but at the expense of a growth penalty. Overexpression of ABA signaling receptors (TaPYLs), which can respond rapidly to drought-induced ABA signals, improves wheat yield under drought conditions without affecting non-drought growth and yield (Mega et al., 2019). Overexpression of the brassinosteroid receptor BRL3 confers drought resistance without affecting plant growth (Fàbregas et al., 2018). Trehalose accumulates under various conditions and protects plants from damage. Researchers have used fusion gene coding for TPS and TPP driven by an ABA-inducible promoter to generate transgenic rice. Trehalose overproduction contributes to lower yield penalties under drought, saline, and sodic conditions, while the yield potential remains unchanged (Joshi et al., 2020). These examples, together with the above-mentioned T6P modulation are not representative of all, but raise the point of view that carbohydrates can be allocated to the right place at the right time by spatiotemporal expression of certain gene(s) through manipulation of specific promoters (conditionally induced or tissue-specific). This flexible C economy strategy could simultaneously endow crops with both high-yield traits and good resistance/resilience.
In field production, several strategies for simultaneously improving crop resistance and yield by targeting C allocation have gradually emerged. Ovary or grain abortion is an adaptive response in cereal domestication, but is agronomically undesirable as it prevents crop yields from reaching their potential. The window period for ovary pollination and grain establishment is considered highly sensitive to stress (Shen et al., 2018; Gao et al., 2023). Shortening maize ASI in the past has improved grain yield due to, at least partly, increased C allocation into the ear tissue. Furthermore, the pollination time gap (PTG) of ovaries on different parts of a panicle/cob will lead to uneven distribution of C allocation among grain siblings, resulting in abortion of inferior spikelets, which usually occur at the top of maize ears, the upper and lower parts of rice, and wheat spikes, especially under adverse conditions such as drought (Shen et al., 2018; Shen et al., 2023). Shortening PTG by measures, such as synchronizing pollination or adoption of stubby ear hybrids, has been shown to coordinate C allocation within siblings and improve both yield and drought resistance in maize (Shen et al., 2018; Shen et al., 2020; Chen et al., 2022b). Strategies aimed at shortening PTG for rice and wheat might work equally well. Moreover, increased distribution of C in the ovary or grain can be achieved by stem manipulation. In wheat, drought-induced abortion of inferior ovaries or grains is associated with suppressed ABA signal transduction in the stems (Zhang et al., 2020b). In contrast, moderate post-anthesis drought in rice-induced ABA signaling and ABA–IAA interactions promotes the remobilization of stem-stored C reserves and enhances inferior grain filling (Wang and Zhang, 2020; Teng et al., 2022). Similarly, recent research has highlighted ways to increase maize grain number and final yield under both optimal and unsuitable environments by tuning stem elongation and ear development. Two maize genotypes with similar plant heights and yield potentials but different drought tolerances were subjected to water scarcity. The ear grain number and final yield of the tolerant genotype were 38.1% and 35.1% higher, respectively, but the plant height was 17.6% short than that under drought (Gao et al., 2023). 13C labeling, together with transcript analysis revealed that the inhibited stem elongation and promoted assimilate allocation to the ear in the tolerant hybrid were induced by signals including ABA and T6P in the stem (Gao et al., 2023). Exogenous application of plant growth regulators, such as ethephon and cycocel at the V15 stage of maize hybrids was proved to reduce internode length and facilitate assimilate partitioning to the ear, which in turn increases the final yield (Zhao et al., 2022). In general, as evidenced by the first green revolution, it is still an important way to increase yield and resistance by reducing or reactivating stem carbohydrates and increasing ovary or grain allocation during the critical growth period.
In summary, some promising strategies, such as rapid environmental response, optimized ear traits, and specific gene regulation in certain tissues or circumstances, aimed at optimizing the C economy within plant systems and increasing C flow to sink organs, have achieved synergistic improvements in yield and resistance/resilience. Based on these strategies, future research may lead to yield breakthroughs in multiple crops using different means (breeding and/or cultivation).
In conclusion, systemic improvement of crops should include the synergistic promotion of C fixation, transport, and utilization. Although the photosynthetic capacity of the field population of modern varieties is believed to be relatively high, as indicated by the traits of canopy leaf area, stay green, and stress tolerance, further improvements in photosynthetic efficiency and coupling with nutrients (N, P, and others) are still needed. One of the keys to high yields in the field is the efficient and economical use of photosynthetically produced assimilates. Unlike currently common adaptation strategies (for example, less C allocation under drought, seed abortion due to insufficient C supply, etc.), the C economy of future “smart crops” needs to be well designed, and the key lies in the flexible adjustment of C flow according to tissue needs at specific growth periods, aiming to improve yield and/or resilience. Both breeding and cultivation methods as well as crop physiology should be considered. In particular, understanding how a field crop manipulates its C economy is the basis for precise control. A recent study found that rapid phosphorylation of SWEET11 and 12 in Arabidopsis promotes carbohydrate transport to the roots during drought (Chen et al., 2022a). However, root C allocation and adjustment in crops have received limited attention. Hence, further research is needed to determine which tissue(s) should be stimulated during the growth process in the face of changing environments. Currently, some appropriate strategies, such as specifically overexpressing the TPP gene in maize and spraying T6P precursors after flowering or seedling drought in wheat, have been developed and applied (Griffiths et al., 2016; Oszvald et al., 2018). It is believed that in the future, “smart crops” created through means such as targeted gene editing, or “smart cultivation systems” based on precise C regulation will significantly contribute to the actual yield improvement.
X-GL and ZG drafted and edited the manuscript and figure. XXF surveyed and analyzed the literature. X-GL and X-MC revised the manuscript. SS and S-LZ conceived the study, contributed to drafting, and edited both the text and figure. X-MC did a lot of work in the revision of the paper, including language & logic revision, re-creation and addition of figures. All authors contributed to the article and approved the submitted version.
This work was supported by the National Natural Science Foundation of China (32160445), the fellowship of China Postdoctoral Science Foundation (2022M723432), the Natural Science Funds of Jiangxi Province (20202BABL215004, 20202BABL215008), the earmarked fund for Innovation team of Jiangxi Agricultural University (JXAUCXTD002), and the earmarked fund for CARS (CARS-02-16). Support is acknowledged from the State Key Laboratory of North China Crop Improvement and Regulation to X-GL.
The authors declare that the research was conducted in the absence of any commercial or financial relationships that could be construed as a potential conflict of interest.
The reviewer BY declared a shared affiliation with the author ZG to the handling editor at the time of review.
All claims expressed in this article are solely those of the authors and do not necessarily represent those of their affiliated organizations, or those of the publisher, the editors and the reviewers. Any product that may be evaluated in this article, or claim that may be made by its manufacturer, is not guaranteed or endorsed by the publisher.
Arp, W. J. (1991). Effects of source-sink relations on photosynthetic acclimation to elevated CO2. Plant Cell Environ. 14, 869–875. doi: 10.1111/j.1365-3040.1991.tb01450.x
Baena-Gonzalez, E., Lunn, J. E. (2020). SnRK1 and trehalose 6-phosphate - two ancient pathways converge to regulate plant metabolism and growth. Curr. Opin. Plant Biol. 55, 52–59. doi: 10.1016/j.pbi.2020.01.010
Bezrutczyk, M., Hartwig, T., Horschman, M., Char, S. N., Yang, J., Yang, B., et al. (2018). Impaired phloem loading in zmsweet13a,b,c sucrose transporter triple knock-out mutants in Zea mays. New Phytol. 218, 594–603. doi: 10.1111/nph.15021
Braun, D. M. (2022). Phloem loading and unloading of sucrose: what a Long, strange trip from source to sink. Annu. Rev. Plant Biol. 73, 553–584. doi: 10.1146/annurev-arplant-070721-083240
Burgess, A. J., Masclaux-Daubresse, C., Strittmatter, G., Weber, A. P. M., Taylor, S. H., Harbinson, J., et al. (2023). Improving crop yield potential: underlying biological processes and future prospects. Food Energy Secur. 12, e435. doi: 10.1002/fes3.345
Chen, X. M. (2022). Spatio-temporal heterogeneity of carbon metabolism and its regulational mechanism in maize endosperm. PhD thesis China Agric. University Beijing.
Chen, J. H., Chen, S. T., He, N. Y., Wang, Q. L., Zhao, Y., Gao, W., et al. (2020). Nuclear-encoded synthesis of the D1 subunit of photosystem II increases photosynthetic efficiency and crop yield. Nat. Plants 6, 570–580. doi: 10.1038/s41477-020-0629-z
Chen, L. Q., Cheung, L. S., Feng, L., Tanner, W., Frommer, W. B. (2015). Transport of sugars. Ann. Rev. Biochem. 84, 865–894. doi: 10.1146/annurev-biochem-060614-033904
Chen, Q., Hu, T., Li, X., Song, C. P., Zhu, J. K., Chen, L., et al. (2022a). Phosphorylation of SWEET sucrose transporters regulates plant root:shoot ratio under drought. Nat. Plants 8, 68–77. doi: 10.1038/s41477-021-01040-7
Chen, X. M., Li, F. Y., Dong, S., Liu, X. F., Li, B. B., Xiao, Z. D., et al. (2022b). Stubby or slender? ear architecture is related to drought resistance in maize. Front. Plant Sci. 13, 901186. doi: 10.3389/fpls.2022.901186
Chen, L. Q., Qu, X. Q., Hou, B. H., Sosso, D., Osorio, S., Fernie, A. R., et al. (2012). Sucrose efflux mediated by SWEET proteins as a key step for phloem transport. Science 335, 207–211. doi: 10.1126/science.1213351
Clark, M. A., Domingo, N. G. G., Colgan, K., Thakrar, S. K., Tilman, D., Lynch, J., et al. (2020). Global food system emissions could preclude achieving the 1.5° and 2°C climate change targets. Science 370, 705–708. doi: 10.1126/science.aba7357
Cross, J. M., von Korff, M., Altmann, T., Bartzetko, L., Sulpice, R., Gibon, Y., et al. (2006). Variation of enzyme activities and metabolite levels in 24 Arabidopsis accessions growing in carbon-limited conditions. Plant Physiol. 142, 1574–1588. doi: 10.1104/pp.106.086629
De Souza, A. P., Burgess, S. J., Doran, L., Hansen, J., Manukyan, L., Maryn, N., et al. (2022). Soybean photosynthesis and crop yield are improved by accelerating recovery from photoprotection. Science 377, 851–854. doi: 10.1126/science.adc9831
Dhungana, S. R., Braun, D. M. (2021). Sugar transporters in grasses: Function and modulation in source and storage tissues. J. Plant Physiol. 266, 153541. doi: 10.1016/j.jplph.2021.153541
Eom, J. S., Chen, L. Q., Sosso, D., Julius, B. T., Lin, I. W., Qu, X. Q., et al. (2015). SWEETs, transporters for intracellular and intercellular sugar translocation. Curr. Opin. Plant Biol. 25, 53–62. doi: 10.1016/j.pbi.2015.04.005
Eom, J. S., Cho, J. I., Reinders, A., Lee, S. W., Yoo, Y., Tuan, P. Q., et al. (2011). Impaired function of the tonoplast-localized sucrose transporter in rice, OsSUT2, limits the transport of vacuolar reserve sucrose and affects plant growth. Plant Physiol. 157, 109–119. doi: 10.1104/pp.111.176982
Eom, J. S., Luo, D., Atienza-Grande, G., Yang, J., Ji, C., Thi Luu, V., et al. (2019). Diagnostic kit for rice blight resistance. Nat. Biotechnol. 37, 1372–1379. doi: 10.1038/s41587-019-0268-y
Fàbregas, N., Lozano-Elena, F., Blasco-Escamez, D., Tohge, T., Martinez-Andujar, C., Albacete, A., et al. (2018). Overexpression of the vascular brassinosteroid receptor BRL3 confers drought resistance without penalizing plant growth. Nat. Commun. 9, 4680. doi: 10.1038/s41467-018-06861-3
Fatima, U., Balasubramaniam, D., Khan, W. A., Kandpal, M., Vadassery, J., Arockiasamy, A., et al. (2023). AtSWEET11 and AtSWEET12 transporters function in tandem to modulate sugar flux in plants. Plant Direct 7, e481. doi: 10.1002/pld3.481
Fei, H., Yang, Z., Lu, Q., Wen, X., Zhang, Y., Zhang, A., et al. (2021). OsSWEET14 cooperates with OsSWEET11 to contribute to grain filling in rice. Plant Sci. 306, 110851. doi: 10.1016/j.plantsci.2021.110851
Fichtner, F., Dissanayake, I. M., Lacombe, B., Barbier, F. (2021). Sugar and nitrate sensing: A multi-billion-year story. Trends Plant Sci. 26, 352–374. doi: 10.1016/j.tplants.2020.11.006
Fichtner, F., Lunn, J. E. (2021). The Role of Trehalose 6-Phosphate (Tre6P) in plant metabolism and development. Annu. Rev. Plant Biol. 72, 3.1–3.24. doi: 10.1146/annurev-arplant-050718-095929
Gao, Y., Zhang, C., Han, X., Wang, Z. Y., Ma, L., Yuan, P., et al. (2018). Inhibition of OsSWEET11 function in mesophyll cells improves resistance of rice to sheath blight disease. Mol. Plant Pathol. 19, 2149–2161. doi: 10.1111/mpp.12689
Gao, J., Zhang, Y., Xu, C., Wang, X., Wang, P., Huang, S. (2023). Abscisic acid collaborates with lignin and flavonoid to improve pre-silking drought tolerance by tuning stem elongation and ear development in maize (Zea mays L.). Plant J. 114, 437–454. doi: 10.1111/tpj.16147
Grassini, P., Eskridge, K. M., Cassman, K. G. (2013). Distinguishing between yield advances and yield plateaus in historical crop production trends. Nat. Commun. 4, 2918. doi: 10.1038/ncomms3918
Griffiths, C. A., Sagar, R., Geng, Y., Primavesi, L. F., Patel, M. K., Passarelli, M. K., et al. (2016). Chemical intervention in plant sugar signalling increases yield and resilience. Nature 540, 574–578. doi: 10.1038/nature20591
Hart, J. E., Sullivan, S., Hermanowicz, P., Petersen, J., Diaz-Ramos, L. A., Hoey, D. J., et al. (2019). Engineering the phototropin photocycle improves photoreceptor performance and plant biomass production. P. Natl. Acad. Sci. 116, 12550–12557. doi: 10.1073/pnas.1902915116
Haupt, S., Duncan, G. H., Holzberg, S., Oparka, K. J. (2001). Evidence for symplastic phloem unloading in sink leaves of barley. Plant Physiol. 125, 209–218. doi: 10.1104/pp.125.1.209
Hu, S., Wang, M., Zhang, X., Chen, W., Song, X., Fu, X., et al. (2021). Genetic basis of kernel starch content decoded in a maize multi-parent population. Plant Biotechnol. J. 19, 2192–2205. doi: 10.1111/pbi.13645
Jeon, J. S., Ryoo, N., Hahn, T. R., Walia, H., Nakamura, Y. (2010). Starch biosynthesis in cereal endosperm. Plant Physiol. Biochem. 48, 383–392. doi: 10.1016/j.plaphy.2010.03.006
Joshi, R., Sahoo, K. K., Singh, A. K., Anwar, K., Pundir, P., Gautam, R. K., et al. (2020). Enhancing trehalose biosynthesis improves yield potential in marker-free transgenic rice under drought, saline, and sodic conditions. J. Exp. Bot. 71, 653–668. doi: 10.1093/jxb/erz462
Julius, B. T., Leach, K. A., Tran, T. M., Mertz, R. A., Braun, D. M. (2017). Sugar transporters in plants: New insights and discoveries. Plant Cell Physiol. 58, 1442–1460. doi: 10.1093/pcp/pcx090
Khurshid, G., Abbassi, A. Z., Khalid, M. F., Gondal, M. N., Naqvi, T. A., Shah, M. M., et al. (2020). A cyanobacterial photorespiratory bypass model to enhance photosynthesis by rerouting photorespiratory pathway in C3 plants. Sci. Rep. 10, 20879. doi: 10.1038/s41598-020-77894-2
Kim, P., Xue, C. Y., Song, H. D., Gao, Y., Feng, L., Li, Y., et al. (2021). Tissue-specific activation of DOF11 promotes rice resistance to sheath blight disease and increases grain weight via activation of SWEET14. Plant Biotechnol. J. 19, 409–411. doi: 10.1111/pbi.13489
Knoblauch, M., Knoblauch, J., Mullendore, D. L., Savage, J. A., Babst, B. A., Beecher, S. D., et al. (2016). Testing the Munch hypothesis of long distance phloem transport in plants. eLife 5, e15341. doi: 10.7554/eLife.15341.025
Kretzschmar, T., Pelayo, M. A., Trijatmiko, K. R., Gabunada, L. F., Alam, R., Jimenez, R., et al. (2015). A trehalose-6-phosphate phosphatase enhances anaerobic germination tolerance in rice. Nat. Plants 1, 15124. doi: 10.1038/nplants.2015.124
Kromdijk, J., Glowacka, K., Leonelli, L., Gabilly, S. T., Iwai, M., Niyogi, K. K., et al. (2016). Improving photosynthesis and crop productivity by accelerating recovery from photoprotection. Science 354, 857–861. doi: 10.1126/science.aai8878
Larkins, B. A. (Ed.) (2017). Maize kernel development (Boston, MA: CABI), ISBN: ISBN: 978 1 78639 123 3.
Leach, K. A., Tran, T. M., Slewinski, T. L., Meeley, R. B., Braun, D. M. (2017). Sucrose transporter2 contributes to maize growth, development, and crop yield. J. Integr. Plant Biol. 59, 390–408. doi: 10.1111/jipb.12527
Leister, D. (2023). Enhancing the light reactions of photosynthesis: Strategies, controversies, and perspectives. Mol. Plant 16, 4–22. doi: 10.1016/j.molp.2022.08.005
Li, L., Liu, K. H., Sheen, J. (2021). Dynamic nutrient signaling networks in plants. Annu. Rev. Cell Dev. Bi. 37, 341–367. doi: 10.1146/annurev-cellbio-010521-015047
Li, B., Liu, H., Zhang, Y., Kang, T., Zhang, L., Tong, J., et al. (2013). Constitutive expression of cell wall invertase genes increases grain yield and starch content in maize. Plant Biotechnol. J. 11, 1080–1091. doi: 10.1111/pbi.12102
Li, P., Wang, L., Liu, H., Yuan, M. (2022b). Impaired SWEET-mediated sugar transportation impacts starch metabolism in developing rice seeds. Crop J. 10, 98–108. doi: 10.1016/j.cj.2021.04.012
Li, Z., Wei, X., Tong, X., Zhao, J., Liu, X., Wang, H., et al. (2022c). The OsNAC23-Tre6P-SnRK1a feed-forward loop regulates sugar homeostasis and grain yield in rice. Mol. Plant 15, 706–722. doi: 10.1016/j.molp.2022.01.016
Li, G., Zhou, C., Yang, Z., Zhang, C., Dai, Q., Huo, Z., et al. (2022a). Low nitrogen enhances apoplastic phloem loading and improves the translocation of photoassimilates in rice leaves and stems. Plant Cell Physiol. 63, 991–1007. doi: 10.1093/pcp/pcac066
Liang, X. G. (2019). The quantification of photoassimilate in crop Leaves and the adjustment of C-balance by trehalose pathway (Beijing: China Agricultural University).
Liang, X. G., Gao, Z., Shen, S., Paul, M. J., Zhang, L., Zhao, X., et al. (2020). Differential ear growth of two maize varieties to shading in the field environment: effects on whole plant carbon allocation and sugar starvation response. J. Plant Physiol. 251, 153194. doi: 10.1016/j.jplph.2020.153194
Liang, X. G., Gao, Z., Zhang, L., Shen, S., Zhao, X., Liu, Y. P., et al. (2019). Seasonal and diurnal patterns of non-structural carbohydrates in source and sink tissues in field maize. BMC Plant Biol. 19, 1–11. doi: 10.1186/s12870-019-2068-4
Liang, X. G., Shen, S., Gao, Z., Zhang, L., Zhao, X., Zhou, S. L. (2021). Variation of carbon partitioning in newly expanded maize leaves and plant adaptive growth under extended darkness. J. Integr. Agr. 20, 2360–2371. doi: 10.1016/S2095-3119(20)63351-2
Liu, T., Kawochar, M. A., Liu, S., Cheng, Y., Begum, S., Wang, E., et al. (2022b). Suppression of the tonoplast sugar transporter, StTST3.1, affects transitory starch turnover and plant growth in potato. Plant J. 113, 342–356. doi: 10.1111/tpj.16050
Liu, H., Si, X., Wang, Z., Cao, L., Gao, L., Zhou, X., et al. (2023). TaTPP-7A positively feedback regulates grain filling and wheat grain yield through T6P-SnRK1 signalling pathway and sugar-ABA interaction. Plant Biotechnol. J 21, 1159–1175. doi: 10.1111/pbi.14025
Liu, J., Wu, M. W., Liu, C. M. (2022a). Cereal endosperms: Development and storage product accumulation. Annu. Rev. Plant Biol. 73, 255–291. doi: 10.1146/annurev-arplant-070221-024405
Long, B. M., Hee, W. Y., Sharwood, R. E., Rae, B. D., Kaines, S., Lim, Y.-L., et al. (2018). Carboxysome encapsulation of the CO2-fixing enzyme Rubisco in tobacco chloroplasts. Nat. Commun. 9, 3570. doi: 10.1038/s41467-018-06044-0
Lopez-Calcagno, P. E., Brown, K. L., Simkin, A. J., Fisk, S. J., Vialet-Chabrand, S., Lawson, T., et al. (2020). Stimulating photosynthetic processes increases productivity and water-use efficiency in the field. Nat. Plants 6, 1054–1063. doi: 10.1038/s41477-020-0740-1
Lyra, D. H., Griffiths, C. A., Watson, A., Joynson, R., Molero, G., Igna, A. A., et al. (2021). Gene-based mapping of trehalose biosynthetic pathway genes reveals association with source- and sink-related yield traits in a spring wheat panel. Food Energy Secur. 10, e292. doi: 10.1002/fes3.292
Ma, L., Zhang, D., Miao, Q., Yang, J., Xuan, Y., Hu, Y. (2017). Essential role of sugar transporter OsSWEET11 during the early stage of rice grain filling. Plant Cell Physiol. 58, 863–873. doi: 10.1093/pcp/pcx040
Mathan, J., Singh, A., Ranjan, A. (2021a). Sucrose transport and metabolism control carbon partitioning between stem and grain in rice. J. Exp. Bot. 72, 4355–4372. doi: 10.1093/jxb/erab066
Mathan, J., Singh, A., Ranjan, A. (2021b). Sucrose transport in response to drought and salt stress involves ABA-mediated induction of OsSWEET13 and OsSWEET15 in rice. Physiol. Plant 171, 620–637. doi: 10.1111/ppl.13210
Mega, R., Abe, F., Kim, J. S., Tsuboi, Y., Tanaka, K., Kobayashi, H., et al. (2019). Tuning water-use efficiency and drought tolerance in wheat using abscisic acid receptors. Nat. Plants 5, 153–159. doi: 10.1038/s41477-019-0361-8
Meng, Y., Zhang, N., Li, J., Shen, X., Sheen, J., Xiong, Y. (2022). TOR kinase, a GPS in the complex nutrient and hormonal signaling networks to guide plant growth and development. J. Exp. Bot. 73, 7041–7054. doi: 10.1093/jxb/erac282
Moore, B., Zhou, L., Rolland, F., Hall, Q., Cheng, W.-H., Liu, Y.-X., et al. (2003). Role of the Arabidopsis glucose sensor HXK1 in nutrient, light, and hormonal signaling. Science 300, 332–336. doi: 10.1126/science.1080585
Morii, M., Sugihara, A., Takehara, S., Kanno, Y., Kawai, K., Hobo, T., et al. (2020). The dual function of OsSWEET3a as a gibberellin and glucose transporter is important for young shoot development in rice. Plant Cell Physiol. 61, 1935–1945. doi: 10.1093/pcp/pcaa130
Mugford, S. T., Fernandez, O., Brinton, J., Flis, A., Krohn, N., Encke, B., et al. (2014). Regulatory properties of ADP glucose pyrophosphorylase are required for adjustment of leaf starch synthesis in different photoperiods. Plant Physiol. 166, 1733–1747. doi: 10.1104/pp.114.247759
Nuccio, M. L., Wu, J., Mowers, R., Zhou, H. P., Meghji, M., Primavesi, L. F., et al. (2015). Expression of trehalose-6-phosphate phosphatase in maize ears improves yield in well-watered and drought conditions. Nat. Biotechnol. 33, 862–869. doi: 10.1038/nbt.3277
O'Leary, G. J., Christy, B., Nuttall, J., Huth, N., Cammarano, D., Stockle, C., et al. (2015). Response of wheat growth, grain yield and water use to elevated CO2 under a Free-Air CO2 Enrichment (FACE) experiment and modelling in a semi-arid environment. Global Change Biol. 21, 2670–2686. doi: 10.1111/gcb.12830
Ohshima, T., Hayashi, H., Chino, M. (1990). Collection and chemical composition of pure phloem sap from Zea mays L. Plant Cell Physiol. 31, 735–737. doi: 10.1093/oxfordjournals.pcp.a077972
Oliva, R., Ji, C., Atienza-Grande, G., Huguet-Tapia, J. C., Perez-Quintero, A., Li, T., et al. (2019). Broad-spectrum resistance to bacterial blight in rice using genome editing. Nat. Biotechnol. 37, 1344–1350. doi: 10.1038/s41587-019-0267-z
Olsen, O. A. (2020). The modular control of cereal endosperm development. Trends Plant Sci. 25, 279–290. doi: 10.1016/j.tplants.2019.12.003
Ortiz-Bobea, A., Ault, T. R., Carrillo, C. M., Chambers, R. G., Lobell, D. B. (2021). Anthropogenic climate change has slowed global agricultural productivity growth. Nat. Clim. Change 11, 306–312. doi: 10.1038/s41558-021-01000-1
Oszvald, M., Primavesi, L. F., Griffiths, C. A., Cohn, J., Basu, S. S., Nuccio, M. L., et al. (2018). Trehalose 6-Phosphate regulates photosynthesis and assimilate partitioning in reproductive tissue. Plant Physiol. 176, 2623–2638. doi: 10.1104/pp.17.01673
Papanatsiou, M., Petersen, J., Henderson, L., Wang, Y., Christie, J. M., Blatt, M. R. (2019). Optogenetic manipulation of stomatal kinetics improves carbon assimilation, water use, and growth. Science 363, 1456–1459. doi: 10.1126/science.aaw0046
Paul, M. J., Foyer, C. H. (2001). Sink regulation of photosynthesis. J. Exp. Bot. 52, 1383–1400. doi: 10.1093/jexbot/52.360.1383
Paul, M. J., Gonzalez-Uriarte, A., Griffiths, C. A., Hassani-Pak, K. (2018). The role of trehalose 6-phosphate in crop yield and resilience. Plant Physiol. 177 (1), 12–23. doi: 10.1104/pp.17.01634
Paul, M. J., Watson, A., Griffiths, C. A. (2020). Linking fundamental science to crop improvement through understanding source and sink traits and their integration for yield enhancement. J. Exp. Bot. 71, 2270–2280. doi: 10.1093/jxb/erz480
Prasad, D., Jung, W. J., Seo, Y. W. (2023). Identification and molecular characterization of novel sucrose transporters in the hexaploid wheat (Triticum aestivum L.). Gene 860, 147245. doi: 10.1016/j.gene.2023.147245
Ray, D. K., Mueller, N. D., West, P. C., Foley, J. A. (2013). Yield trends are insufficient to double global crop production by 2050. PloS One 8, e66428. doi: 10.1371/journal.pone.0066428
Ribeiro, C., Hennen-Bierwagen, T. A., Myers, A. M., Cline, K., Settles, A. M. (2020). Engineering 6-phosphogluconate dehydrogenase improves grain yield in heat-stressed maize. P. Natl. Acad. Sci. 117, 33177–33185. doi: 10.1073/pnas.2010179117
Rolland, F., Baena-Gonzalez, E., Sheen, J. (2006). Sugar sensing and signaling in plants: conserved and novel mechanisms. Annu. Rev. Plant Biol. 57, 675–709. doi: 10.1146/annurev.arplant.57.032905.105441
Rossi, M., Bermudez, L., Carrari, F. (2015). Crop yield: challenges from a metabolic perspective. Curr. Opin. Plant Biol. 25, 79–89. doi: 10.1016/j.pbi.2015.05.004
Ruan, Y. L. (2014). Sucrose metabolism: gateway to diverse carbon use and sugar signaling. Annu. Rev. Plant Biol. 65, 33–67. doi: 10.1146/annurev-arplant-050213-040251
Ruan, Y. L. (2022). CWIN-sugar transporter nexus is a key component for reproductive success. J. Plant Physiol. 268, 153572. doi: 10.1016/j.jplph.2021.153572
Scofield, G. N., Hirose, T., Gaudron, J. A., Furbank, R. T., Upadhyaya, N. M., Ohsugi, R. (2002). Antisense suppression of the rice transporter gene, OsSUT1, leads to impaired grain filling and germination but does not affect photosynthesis. Funct. Plant Biol. 29, 815–826. doi: 10.1071/PP01204
Shannon, J. C., Porter, G. A., Knlevel, D. P. (1986). “Phloem unloading and transfer of sugars into developing corn endosperm,”. In: Phloem Transport Eds. Cronshaw, J., Lucas, W. J., Giaquinta, R. T. (New York: Phloem Transport, Alan Liss, Inc.), 265–277.
Shen, S., Liang, X. G., Zhang, L., Zhao, X., Liu, Y. P., Lin, S., et al. (2020). Intervening in sibling competition for assimilates by controlled pollination prevents seed abortion under postpollination drought in maize. Plant Cell Eviron. 43, 903–919. doi: 10.1111/pce.13704
Shen, S., Ma, S., Chen, X. M., Yi, F., Li, B. B., Liang, X. G., et al. (2022). A transcriptional landscape underlying sugar import for grain set in maize. Plant J. 110, 228–242. doi: 10.1111/tpj.15668
Shen, S., Ma, S., Wu, L., Zhou, S. L., Ruan, Y. L. (2023). Winners-take-all: Competition of carbon resource for grain set. Trends Plant Sci 28, 893–901. doi: 10.1016/j.tplants.2023.03.015
Shen, B. R., Wang, L. M., Lin, X. L., Yao, Z., Xu, H. W., Zhu, C. H., et al. (2019). Engineering a new chloroplastic photorespiratory bypass to increase photosynthetic efficiency and productivity in rice. Mol. Plant 12, 199–214. doi: 10.1016/j.molp.2018.11.013
Shen, S., Zhang, L., Liang, X. G., Zhao, X., Lin, S., Qu, L. H., et al. (2018). Delayed pollination and low availability of assimilates are major factors causing maize kernel abortion. J. Exp. Bot. 69, 1599–1613. doi: 10.1093/jxb/ery013
Sinclair, T. R., Rufty, T. W., Lewis, R. S. (2019). Increasing photosynthesis: Unlikely solution for world food problem. Trends Plant Sci. 24, 1032–1039. doi: 10.1016/j.tplants.2019.07.008
Singh, J., Das, S., Jagadis Gupta, K., Ranjan, A., Foyer, C. H., Thakur, J. K. (2023). Physiological implications of SWEETs in plants and their potential applications in improving source-sink relationships for enhanced yield. Plant Biotechnol. J 21, 1528–1541. doi: 10.1111/pbi.13982
Singh, J., James, D., Achary, V. M. M., Patel, M. K., Thakur, J. K., Reddy, M. K., et al. (2021). Coordinated overexpression of OsSUT1, OsSWEET11 and OsSWEET14 in rice impairs carbohydrate metabolism that has implications in plant growth, yield and susceptibility to Xanthomonas oryzae pv oryzae (Xoo). bioRxiv, 425507. doi: 10.1101/2021.01.07.425507
Slewinski, T. L. (2012). Non-structural carbohydrate partitioning in grass stems: a target to increase yield stability, stress tolerance, and biofuel production. J. Exp. Bot. 63, 4647–4670. doi: 10.1093/jxb/ers124
Slewinski, T. L., Meeley, R., Braun, D. M. (2009). Sucrose transporter1 functions in phloem loading in maize leaves. J. Exp. Bot. 60, 881–892. doi: 10.1093/jxb/ern335
Smidansky, E. D., Clancy, M., Meyer, F. D., Lanning, S. P., Blake, N. K., Talbert, L. E., et al. (2002). Enhanced ADP-glucose pyrophosphorylase activity in wheat endosperm increases seed yield. P. Natl. Acad. Sci. 99, 1724–1729. doi: 10.1073/pnas.022635299
Smith, A. M., Stitt, M. (2007). Coordination of carbon supply and plant growth. Plant Cell Environ. 30, 1126–1149. doi: 10.1111/j.1365-3040.2007.01708.x
Solomon, C. U., Drea, S. (2019). Delineation of post-phloem assimilate transport pathway into developing caryopsis of Brachypodium distachyon. bioRxiv. doi: 10.1101/718569
Sosso, D., Luo, D., Li, Q. B., Sasse, J., Yang, J., Gendrot, G., et al. (2015). Seed filling in domesticated maize and rice depends on SWEET-mediated hexose transport. Nat. Genet. 47, 1489–1493. doi: 10.1038/ng.3422
South, P. F., Cavanagh, A. P., Liu, H. W., Ort, D. R. (2019). Synthetic glycolate metabolism pathways stimulate crop growth and productivity in the field. Science. 363, eaat9077. doi: 10.1126/science.aat9077
Stitt, M., Zeeman, S. C. (2012). Starch turnover: pathways, regulation and role in growth. Curr. Opin. Plant Biol. 15, 282–292. doi: 10.1016/j.pbi.2012.03.016
Sulpice, R., Flis, A., Ivakov, A. A., Apelt, F., Krohn, N., Encke, B., et al. (2014). Arabidopsis coordinates the diurnal regulation of carbon allocation and growth across a wide range of photoperiods. Mol. Plant 7, 137–155. doi: 10.1093/mp/sst127
Sulpice, R., Pyl, E. T., Ishihara, H., Trenkamp, S., Steinfath, M., Witucka-Wall, H., et al. (2009). Starch as a major integrator in the regulation of plant growth. P. Natl. Acad. Sci. 106, 10348–10353. doi: 10.1073/pnas.0903478106
Sweetlove, L. J., Nielsen, J., Fernie, A. R. (2017). Engineering central metabolism - a grand challenge for plant biologists. Plant J. 90, 749–763. doi: 10.1111/tpj.13464
Teng, Z., Yu, H., Wang, G., Meng, S., Liu, B., Yi, Y., et al. (2022). Synergistic interaction between ABA and IAA due to moderate soil drying promotes grain filling of inferior spikelets in rice. Plant J. 109, 1457–1472. doi: 10.1111/tpj.15642
Tian, B., Talukder, S. K., Fu, J., Fritz, A. K., Trick, H. N. (2018). Expression of a rice soluble starch synthase gene in transgenic wheat improves the grain yield under heat stress conditions. In Vitro Cell.Dev.Biol.-Plant 54, 216–227. doi: 10.1007/s11627-018-9893-2
von Caemmerer, S., Furbank, R. T. (2016). Strategies for improving C4 photosynthesis. Curr. Opin. Plant Biol. 31, 125–134. doi: 10.1016/j.pbi.2016.04.003
Wang, G., Li, X., Ye, N., Huang, M., Feng, L., Li, H., et al. (2021a). OsTPP1 regulates seed germination through the crosstalk with abscisic acid in rice. New Phytol. 230, 1925–1939. doi: 10.1111/nph.17300
Wang, L., Lu, Q., Wen, X., Lu, C. (2015). Enhanced sucrose loading improves rice yield by increasing grain size. Plant Physiol. 169, 2848–2862. doi: 10.1104/pp.15.01170
Wang, L. M., Shen, B. R., Li, B. D., Zhang, C. L., Lin, M., Tong, P. P., et al. (2020). A synthetic photorespiratory shortcut enhances photosynthesis to boost biomass and grain yield in rice. Mol. Plant 13, 1802–1815. doi: 10.1016/j.molp.2020.10.007
Wang, E., Wang, J., Zhu, X., Hao, W., Wang, L., Li, Q., et al. (2008). Control of rice grain-filling and yield by a gene with a potential signature of domestication. Nat. Genet. 40, 1370–1374. doi: 10.1038/ng.220
Wang, G., Wu, Y., Ma, L., Lin, Y., Hu, Y., Li, M., et al. (2021b). Phloem loading in rice leaves depends strongly on the apoplastic pathway. J. Exp. Bot. 72, 3723–3738. doi: 10.1093/jxb/erab085
Wang, G., Zhang, J. (2020). Carbohydrate, hormone and enzyme regulations of rice grain filling under post-anthesis soil drying. Environ. Exp. Bot. 178, 104165. doi: 10.1016/j.envexpbot.2020.104165
Wingenter, K., Schulz, A., Wormit, A., Wic, S., Trentmann, O., Hoermiller, I. I., et al. (2010). Increased activity of the vacuolar monosaccharide transporter TMT1 alters cellular sugar partitioning, sugar signaling, and seed yield in Arabidopsis. Plant Physiol. 154, 665–677. doi: 10.1104/pp.110.162040
Wu, A., Brider, J., Busch, F. A., Chen, M., Chenu, K., Clarke, V. C., et al. (2023). A cross-scale analysis to understand and quantify the effects of photosynthetic enhancement on crop growth and yield across environments. Plant Cell Environ. 46, 23–44. doi: 10.1111/pce.14453
Wu, L. B., Eom, J. S., Isoda, R., Li, C., Char, S. N., Luo, D., et al. (2022). OsSWEET11b, a potential sixth leaf blight susceptibility gene involved in sugar transport-dependent male fertility. New Phytol. 234, 975–989. doi: 10.1111/nph.18054
Wu, A., Hammer, G. L., Doherty, A., von Caemmerer, S., Farquhar, G. D. (2019a). Quantifying impacts of enhancing photosynthesis on crop yield. Nat. Plants 5, 380–388. doi: 10.1038/s41477-019-0398-8
Wu, Y., Lee, S. K., Yoo, Y., Wei, J., Kwon, S. Y., Lee, S. W., et al. (2018). Rice transcription factor OsDOF11 modulates sugar transport by promoting expression of sucrose transporter and SWEET genes. Mol. Plant 11, 833–845. doi: 10.1016/j.molp.2018.04.002
Wu, Y., Shi, L., Li, L., Fu, L., Liu, Y., Xiong, Y., et al. (2019b). Integration of nutrient, energy, light, and hormone signalling via TOR in plants. J. Exp. Bot. 70, 2227–2238. doi: 10.1093/jxb/erz028
Xue, X., Wang, J., Shukla, D., Cheung, L. S., Chen, L. Q. (2022). When SWEETs turn tweens: Updates and perspectives. Annu. Rev. Plant Biol. 73, 379–403. doi: 10.1146/annurev-arplant-070621-093907
Yang, H., Li, Y., Qiao, Y., Sun, H., Liu, W., Qiao, W., et al. (2023). Low light stress promotes new tiller regeneration by changing source-sink relationship and activating expression of expansin genes in wheat. Plant Cell Environ. 46, 1562–1581. doi: 10.1111/pce.14548
Yang, J., Luo, D., Yang, B., Frommer, W. B., Eom, J. S. (2018). SWEET11 and 15 as key players in seed filling in rice. New Phytol. 218, 604–615. doi: 10.1111/nph.15004
Yang, B., Wang, J., Yu, M., Zhang, M., Zhong, Y., Wang, T., et al. (2022). The sugar transporter ZmSUGCAR1 of the nitrate transporter 1/peptide transporter family is critical for maize grain filling. Plant Cell 34, 4232–4254. doi: 10.1093/plcell/koac256
Yin, X., Struik, P. C. (2015). Constraints to the potential efficiency of converting solar radiation into phytoenergy in annual crops: from leaf biochemistry to canopy physiology and crop ecology. J. Exp. Bot. 66, 6535–6549. doi: 10.1093/jxb/erv371
Yoon, D.-K., Ishiyama, K., Suganami, M., Tazoe, Y., Watanabe, M., Imaruoka, S., et al. (2020). Transgenic rice overproducing Rubisco exhibits increased yields with improved nitrogen-use efficiency in an experimental paddy field. Nat. Food 1, 134–139. doi: 10.1038/s43016-020-0033-x
Yuan, M., Zhao, J., Huang, R., Li, X., Xiao, J., Wang, S. (2014). Rice MtN3/saliva/SWEET gene family: Evolution, expression profiling, and sugar transport. J. Integr. Plant Biolo. 56, 559–570. doi: 10.1111/jipb.12173
Zhang, Z., Huang, J., Gao, Y., Liu, Y., Li, J., Zhou, X., et al. (2020b). Suppressed ABA signal transduction in the spike promotes sucrose use in the stem and reduces grain number in wheat under water stress. J. Exp. Bot. 71, 7241–7256. doi: 10.1093/jxb/eraa380
Zhang, J., Li, D., Xu, X., Ziska, L. H., Zhu, J., Liu, G., et al. (2020a). The potential role of sucrose transport gene expression in the photosynthetic and yield response of rice cultivars to future CO2 concentration. Physiol. Plantarum 168, 218–226. doi: 10.1111/ppl.12973
Zhang, Y., Primavesi, L. F., Jhurreea, D., Andralojc, P. J., Mitchell, R. A., Powers, S. J., et al. (2009). Inhibition of SNF1-related protein kinase1 activity and regulation of metabolic pathways by trehalose-6-phosphate. Plant Physiol. 149, 1860–1871. doi: 10.1104/pp.108.133934
Zhao, Y., Zhang, S., Lv, Y., Ning, F., Cao, Y., Liao, S., et al. (2022). Optimizing ear-plant height ratio to improve kernel number and lodging resistance in maize (Zea mays L.). Field Crops Res. 276, 108376. doi: 10.1016/j.fcr.2021.108376
Zhu, X. G., Long, S. P., Ort, D. R. (2010). Improving photosynthetic efficiency for greater yield. Annu. Rev. Plant Biol. 61, 235–261. doi: 10.1146/annurev-arplant-042809-112206
Keywords: photosynthesis, carbon utilization, sugar transport, systemic signaling, trehalose 6-phosphate, carbon allocation, source-sink relationship, smart crop
Citation: Liang X-G, Gao Z, Fu X-X, Chen X-M, Shen S and Zhou S-L (2023) Coordination of carbon assimilation, allocation, and utilization for systemic improvement of cereal yield. Front. Plant Sci. 14:1206829. doi: 10.3389/fpls.2023.1206829
Received: 16 April 2023; Accepted: 14 August 2023;
Published: 05 September 2023.
Edited by:
Baizhao Ren, Shandong Agricultural University, ChinaReviewed by:
Baozhong Yin, Hebei Agricultural University, ChinaCopyright © 2023 Liang, Gao, Fu, Chen, Shen and Zhou. This is an open-access article distributed under the terms of the Creative Commons Attribution License (CC BY). The use, distribution or reproduction in other forums is permitted, provided the original author(s) and the copyright owner(s) are credited and that the original publication in this journal is cited, in accordance with accepted academic practice. No use, distribution or reproduction is permitted which does not comply with these terms.
*Correspondence: Si Shen, c2hlbnNpQGNhdS5lZHUuY24=; Shun-Li Zhou, emhvdXNobEBjYXUuZWR1LmNu
†These authors have contributed equally to this work
Disclaimer: All claims expressed in this article are solely those of the authors and do not necessarily represent those of their affiliated organizations, or those of the publisher, the editors and the reviewers. Any product that may be evaluated in this article or claim that may be made by its manufacturer is not guaranteed or endorsed by the publisher.
Research integrity at Frontiers
Learn more about the work of our research integrity team to safeguard the quality of each article we publish.