- Department of Agricultural Sciences, University of Naples Federico II, Portici, Italy
Background: Long-term space missions will necessarily require producing viable seeds to be used for plant cultivation over time under altered gravity conditions. Pollen is known to play a key role in determining seed and fruit production over seed-to-seed cycles, but very few studies have evaluated pollen functionality under altered gravity.
Methods: We performed ground-based experiments to test how simulated microgravity can affect the directional growth of pollen tubes as a potential bottleneck in seed and fruit sets. The effect of clinorotation was assessed in the pollen of Solanum lycopersicum L. cv. ‘Micro-Tom’ and Brassica rapa L. var. silvestris, both eligible for cultivation in space. Pollen tube length and tortuosity were compared under 1g and simulated microgravity with a uniaxial clinostat.
Results: The main results highlighted that simulated microgravity significantly increased pollen tube length and tortuosity compared to 1g conditions. Further, clinorotation prompted a differential effect on pollen germination between S. lycopersicum and B. rapa. A more in-depth analysis evaluating the effect of gravity on the directional growth of pollen tubes excluded gravitropic responses as responsible for the tube tip position reached after germination.
Discussion: This research provides new insights into how altered gravity can interfere with plant reproduction and, in particular, microgametophyte functionality. Our findings represent a basis for further studies aimed at understanding the effect of real microgravity on plant reproduction and developing countermeasures to ensure seed-to-seed cultivation in long-term space missions and achieve self-sufficiency in food supplies from Earth.
1 Introduction
Future space exploration requires plant-based bioregenerative life support systems (BLSS) to produce edible biomass and oxygen, recycle water, absorb carbon dioxide, and support astronauts’ psychological health (Kordyum and Hasenstein, 2021). Indeed, plants are essential components of BLSS, and for decades, many experiments with plants have been performed to test the feasibility of plant cultivation in space (e.g., Zabel et al., 2016; De Pascale et al., 2021; Romano and Aronne, 2021).
Early efforts to cultivate plants for extended periods in microgravity resulted in reduced growth and complications in the transition to the reproductive stage (Kordyum et al., 1983; Kuang et al., 1995). In 1982, Arabidopsis thaliana became the first plant species to flower in space and produce seeds (Merkies and Laurinavichyus, 1983). However, only half of the seeds were viable and germinated in developing plants without growth defects (Salisbury, 1999). Environmental constraints such as low ventilation and high ethylene concentrations have been considered the main causes of interrupted or reduced reproductive success in many early experiments in space (De Micco et al., 2013). Recently, the advancement of plant growth systems has led to numerous successful seed sets in space and to a seed-to-seed-to-seed cycle of A. thaliana that was achieved entirely on the International Space Station (Link et al., 2014).
Overall, plant biology research in space indicates that real and simulated microgravity do not prevent plant reproduction, but they can affect the quality of embryos and the development of seedlings (Kuang et al., 2000b; Musgrave et al., 2000; Link et al., 2014; Izzo et al., 2022). Various alterations in seeds produced in space include delayed embryonic development, modification of storage reserves, delayed starch use in cotyledons, and decreased cell number in cotyledons (Kuang et al., 2000a; Kuang et al., 2000b; Musgrave et al., 2005). Currently, the identification of mechanisms by which space factors can affect plant reproduction is becoming increasingly critical for plant cultivation in future human settlements on the Moon and Mars (Kordyum et al., 2020). Indeed, the completion of the seed-to-seed cycle in the BLSSs is essential to producing viable seeds to be used for plant cultivation over time independently of Earth’s supply.
Within the plant reproductive cycle, viable and germinable pollen is needed to trigger the double fertilization responsible for seed formation. Indeed, both pollen viability and germination are positively correlated with fruit and seed set (Paupière et al., 2017; Herrera et al., 2018). Therefore, possible effects of space factors on successful seed production can be addressed by defects in pollen viability and germinability. Previous experiments showed that most Arabidopsis and Brassica pollen grains produced under microgravity conditions were viable (Kuang et al., 1996; Kuang et al., 2000b). Nevertheless, this is not enough to fulfill the reproductive cycle. Once formed, viable pollen needs to be transferred to the stigma, posing some challenges in microgravity, especially for self-incompatible species that require cross-pollination to succeed in seed production (Kuang et al., 2000a). Furthermore, once it arrives on the stigma surface, pollen must germinate and guide its pollen tube across the ovary into the ovule to trigger double fertilization and seed formation. In the alternation between sporophyte (diploid) and gametophyte (haploid) generation in sexual plant reproduction, pollen represents the male gametophyte and is therefore an independent organism with a very short lifespan whose survival is essential to achieve the full completion of the plant reproductive cycle (Pacini and Dolferus, 2019).
Pollen is very sensitive to environmental factors that could affect pollen tube development. The earliest stage of pollen development was revealed to be the most sensitive to temperature changes, with a drastic reduction of pollen germination caused by high temperatures during microsporogenesis (Iovane and Aronne, 2021). Moreover, pollen germination is strongly influenced by relative humidity and its combination with temperature conditions (Güçlü and Koyuncu, 2017; Aronne et al., 2021). Indeed, a drastic loss in pollen viability occurs when pollen is exposed to a combination of high humidity and high temperature, whereas this does not happen when pollen is exposed to the same temperatures but lower humidity levels (Iovane et al., 2022a). Additionally, the possibility of plants growing and reproducing in space will depend on their capability to accomplish both sporophyte and gametophyte generations under altered microgravity conditions. In this scenario, investigations into how simulated microgravity can affect pollen germination and tube growth might provide useful insights into space-related issues in plant reproduction.
Among the instruments used to simulate microgravity, clinostats are meant to reduce the effect of constant gravity acceleration on Earth, and they have been largely used to perform ground-based experiments on the effects of simulated microgravity on plant growth and development (Dedolph and Dipert, 1971; Kiss et al., 2019). Even if clinostats do not reproduce weightlessness, ground-based research in simulated microgravity provides useful understandings to predict plant responses in real microgravity (Kiss et al., 2019). Although plants are known to be extremely sensitive to gravity (Muthert et al., 2020; Sathasivam et al., 2021), gravitropism in pollen tubes has not yet been assessed. To date, the microgametophyte (pollen) tube path towards the ovary is known to be guided exclusively by chemotropic stimuli due to specialized pistil tissues (Kandasamy et al., 1994), whereas the possible co-existence with pollen gravitropism has been generally overlooked.
To date, a few studies have shown that simulated microgravity affects several aspects of pollen tube development, such as alterations in sperm cell migration and callose plug formation (De Micco et al., 2005; De Micco et al., 2006), but gravitropism in pollen tubes has not yet been assessed. Previous studies were performed under in vitro conditions where the absence of a Ca2+ gradient allowed isolation of the effect of simulated microgravity. Thus, to test the hypothesis that chemotropism always coexists with gravitropism in germinating pollen, in vitro conditions would be ideal to reveal the effect of altered gravity in orientating pollen tubes. In the framework of future space missions with seed-to-seed crop cycles, it is crucial to study how altered gravity could interfere with pollen germination and tube directional growth to achieve fertilization.
Among candidate space crops, Solanum lycopersicum L. cv. ‘Micro-Tom’ and Brassica rapa L. are two promising crops for cultivation on board spacecrafts, mainly for their compact size and short life cycle (Musgrave et al., 2005; Matsukura et al., 2008; Shikata and Ezura, 2016; Burgner et al., 2020). ‘Micro-Tom’ is a macro-thermal fruity crop that has already achieved a seed-to-seed cycle under simulated microgravity, even though fruit setting was almost halved under clinorotation (Colla et al., 2007). B. rapa is a micro-thermal leafy crop that has already been grown from seed to seed under microgravity conditions on the Mir space station in 1997; however, seeds and siliques formed from the second generation of plants on board resulted in compromise (Musgrave et al., 2005). Brassica spp. are close relatives of A. thaliana, and there is substantial homology between their nuclear genomes (Tatematsu et al., 2004). Particularly, the pollen of B. rapa L. var. sylvestris has already been investigated for aberrant callose plug deposition during tube development under simulated microgravity (De Micco et al., 2006).
Research on possible gravitropic responses of pollen is needed to have a better understanding of how altered gravity can limit seed and fruit production over repeated seed-to-seed cycles in candidate space crops. In this context, our study is the first to perform in vitro pollen germination on a clinostat to isolate the effect of simulated microgravity and unravel the possible gravitropism of the pollen tube. Specifically, our aim was to compare the effect of simulated microgravity and Earth gravity on pollen tube orientation in S. lycopersicum L. cv. ‘Micro-Tom’ and Brassica rapa L var. sylvestris as potential bottlenecks in regular seed and fruit development for cultivation in space.
2 Material and methods
2.1 Plant material
The effect of simulated microgravity on pollen germination and tube orientation was assessed in S. lycopersicum L. cv. ‘Micro-Tom’ and B. rapa L var. sylvestris, both recognized as candidate space crops. ‘Micro-Tom’ and Brassica plants were grown from seeds to flower in two different growth chambers. In ‘Micro-Tom’ plants, a photosynthetic photon flux density (PPFD) of 400 ± 15 μmol m−2 s−1 was provided by white LEDs in a 16 h d−1 photoperiod. Relative humidity was kept at 70 ± 5% and the air temperature was set at 22 ± 0.5°C both reported as the optimum for tomatoes (Sato et al., 2001; Matsuda et al., 2014). As for Brassica plants, the PPFD from white LEDs was 250 ± 10 μmol m−2 s−1 in a 12 h d−1 photoperiod. Relative humidity and air temperature were kept at 70 ± 5% and 18 ± 0.5°C, respectively. PPFD was measured during each treatment using a spectroradiometer (SS-110; Apogee Instruments Inc., Logan, UT).
2.2 Pollen sampling and viability measurement
Pollen was collected from flowers sampled shortly before anthesis to prevent possible pollen damage once exposed to the external environment. More specifically, undehisced anthers were detached from 200 flower buds per species, distributed in two different Petri dishes, and left to dehisce in a closed container filled with dry silica gel. This method sped up pollen release from anthers and allowed for the production of one bulk sample of pollen per species within a few hours of flower sampling (Iovane et al., 2022b).
Before clinorotation, the pollen viability of the bulk samples was assessed through the diaminobenzidine (DAB) reaction (Dafni, 1992) to ensure that the pollen used for the experiment was of high quality. We evaluated pollen viability on five pollen samples per species taken from the respective bulk. Each pollen sample was spread into a 10 μl droplet of water previously placed on a slide. One droplet of 10 μl of DAB reagent was added to each pollen sample, and slides were gently warmed on a heating plate and mounted. Pollen grains were scored as viable when stained dark/brown and as not viable when they remained faint/colorless. Each slide mounting a single pollen sample was photographed under a light microscope, and pollen viability was scored through image analyses on six photos per slide. The viability percentage was measured by counting at least 100 pollen grains per photo on a total of 30 photos per species (two species × five slides × six photos).
2.3 Pollen germination and gravity treatments
To test the effect of simulated microgravity on pollen germination, Petri dishes with germinating pollen were split between a control (1g) and a clinostat treatment (cl). In the control treatment, pollen germinated on agar germination medium inside Petri dishes vertically positioned according to the 1g gravity vector. Simulated microgravity treatment was performed on a uniaxial clinostat consisting of two metallic supports and two rotating dishes joined by a rigid shaft. Petri dishes with germinating pollen were fixed on the rotating shaft at a specific distance from the rotating axis to obtain ineffective centrifuge acceleration on pollen, according to a spin velocity of the rigid shaft set at two revolutions per minute. In vitro pollen germination was tested using two different agar media optimized for ‘Micro-Tom’ and B. rapa (Sato et al., 1998; Karapanos et al., 2010). In more detail, pollen from the bulk samples was placed in 10 Petri dishes per species lined with an agar medium made of 0.9% agar and 15% sucrose (w/v) for ‘Micro-Tom’ pollen and 0.9% agar and 20% sucrose (w/v) for B. rapa.
2.4 Microscopy and image analysis of germinating pollen
The effect of clinorotation on pollen germination and tube development in agar media was assessed through image analyses comparing pollen germinability, tube length, and tortuosity index between control (1g) and clinostat (simulated microgravity) treatments. Image analysis of germinating pollen was performed with ImageJ v1.53 software on photos taken with a digital camera (EOS 60D, Canon) mounted on a light microscope (Olympus® BX-60). Each photo was taken keeping track of the Petri dish orientation with respect to the direction of the gravity vector. Germinating pollen was photographed after 24 and 48 h, and germination scoring was performed through image analyses on 400 photos (two species × two gravity treatments × five Petri dishes × two germination times × 10 photos). Germination percentages were assessed by considering as germinated grains those with a pollen tube longer than the grain diameter. Pollen tube length (L) was assessed on 600 pollen tubes and scored on 200 photos tracing the whole length of the pollen tube from the exine apertures to the pollen tube tip. On the same photos, the pollen tortuosity index was measured to estimate bending/directionality throughout the pollen tube path (Figure 1). The tortuosity index (T) was calculated using the following equation:
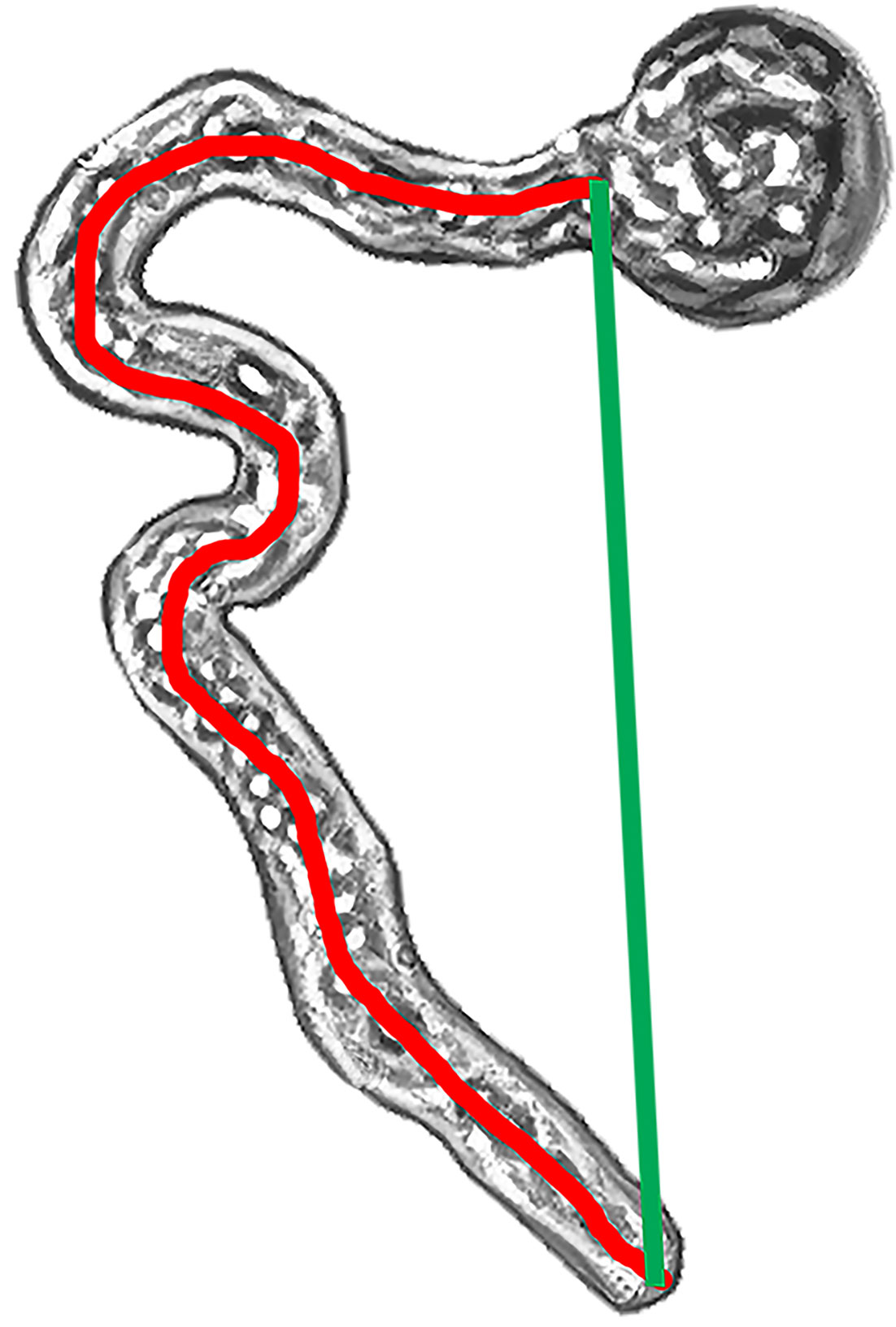
Figure 1 Tortuosity index calculated as L/C—length/chord through image analyses on pollen grains germinated in agar medium. Pollen tube length (L—red line) was traced throughout the whole length of the pollen tube. The pollen tube chord (C—green line) was traced as the straight line between the pollen exine aperture and the tube tip.
where L (length) is the total length of the pollen tube and C (chord) is the distance between the pollen exine aperture and the pollen tube tip. The reported tortuosity index can vary from 1 (L = C) to +∞ (L > C). More specifically, tortuosity values close to 1 indicate that the pollen tube developed straight from the exine to the tube tip, whereas increasing tortuosity values indicate an increase in direction changes during elongation of the pollen tube.
Furthermore, to detect a possible gravitropic response of pollen tubes, we investigated the direction of tube elongation in the control and clinostat treatments. For this purpose, we divided the images of each germinated pollen grain into four quadrants with respect to the direction of the gravity vector, scored the direction of 300 pollen tubes inside the agar medium, and calculated the percentage of pollen tube tips reaching each quadrant (Figure 2).
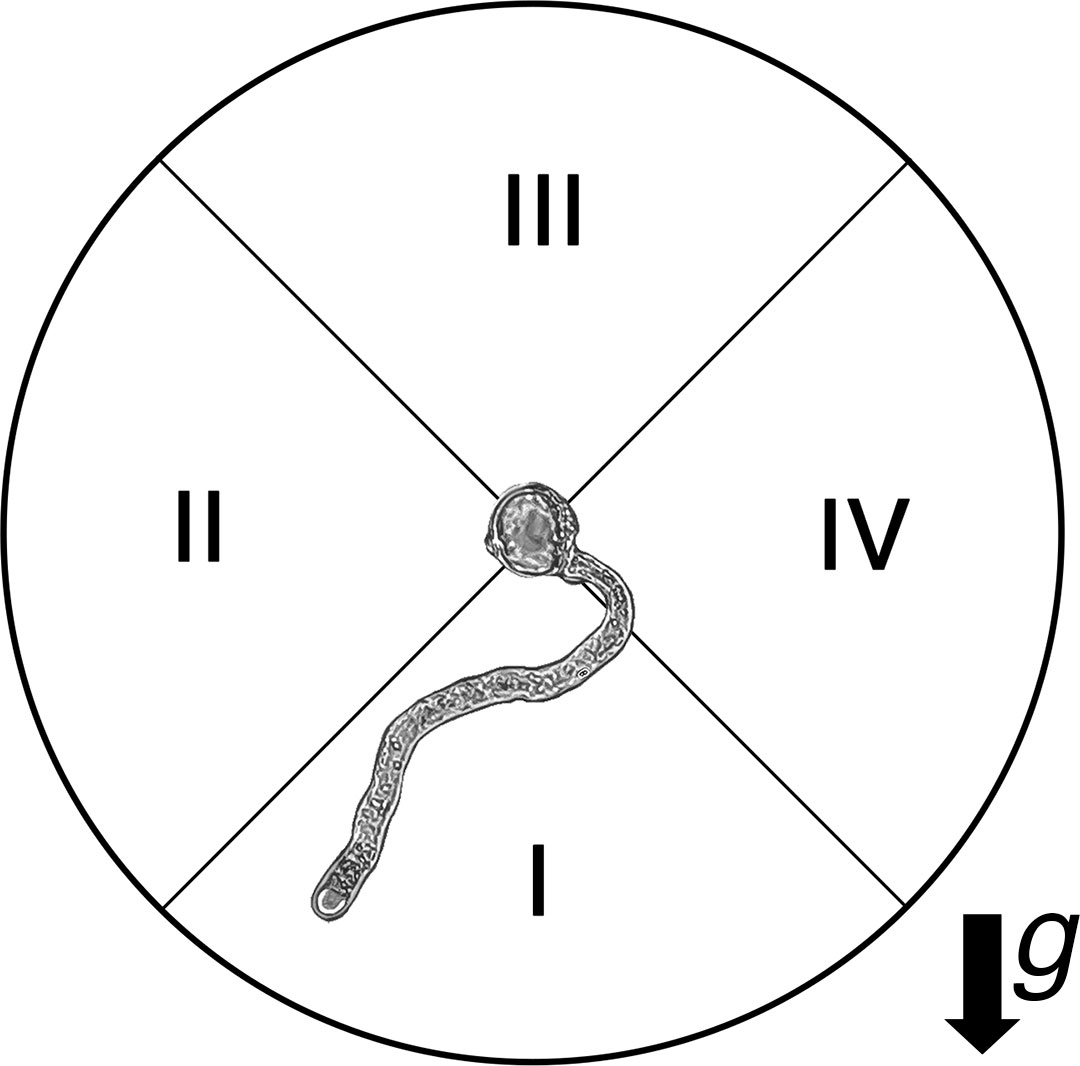
Figure 2 Evaluation of pollen tube tip location with respect to gravity vector (g) under 1g and clinorotation treatments through image analyses.
We hypothesized that the expected frequencies of pollen tube tip location would be equally distributed across the Petri dish containing the germination medium (25% in each quadrant). In the case of P <0.05, the observed frequencies would differ from those expected, therefore showing a preferential growth direction of pollen tube tips. Specifically, in the control treatment, a positive or negative gravitropic response would result if pollen tube tips were preferentially located in quadrants I or III, respectively.
2.5 Data analysis
Data were analyzed with IBM® SPSS Statistics. Shapiro–Wilk’s test and Levene’s test were respectively used to assess the normality and homogeneity of the variances of the datasets. Differences in pollen germination, tube length, and tortuosity between ground and clinostat treatments were tested using ANOVA (P <0.05) and the Brown–Forsythe robust test for equality of means. The observed and expected frequencies of pollen tube tip location in the different quadrants under control and simulated microgravity treatments were compared through a Chi-square test.
3 Results
The DAB test for pollen viability showed that pollen was well formed and functional in both species. Moreover, the viability of all pollen samples taken from the bulk of each species was high and uniform, ranging between 87%–91% in ‘Micro-Tom’ and 86%–94% in B. rapa, and no significant differences were detected among pollen samples intra-species (Figure 3).
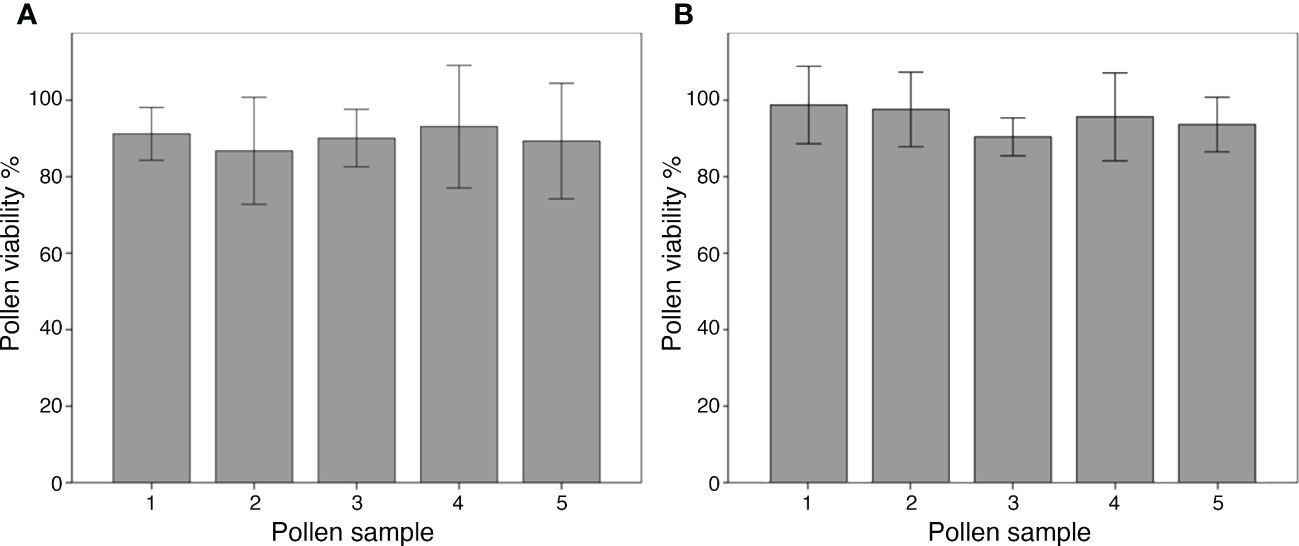
Figure 3 Viability of bulk pollen from flowers of ‘Micro-Tom’ (A) and Brassica rapa (B). Each bar shows the pollen viability of a single pollen sample from the bulk, expressed as the mean of the viability percentage scored on six photos per sample. ANOVA reported no significant differences intra-species among pollen samples (P <0.05). Bars represent ± SE.
Data on pollen germination showed clear differences between control and clinostat treatments (Figure 4). More specifically, the two-way ANOVA showed a significant (P <0.05) effect of germination time, gravity, and their interaction. The two species reacted differently to gravity since simulated microgravity reduced pollen germination in ‘Micro-Tom’ but increased pollen germination in B. rapa compared to the control condition. However, in both species, simulated microgravity significantly increased pollen germination when prolonging pollen incubation from 24 to 48 h, whereas no differences in pollen germination were found between 24 and 48 h of incubation under the 1g condition.
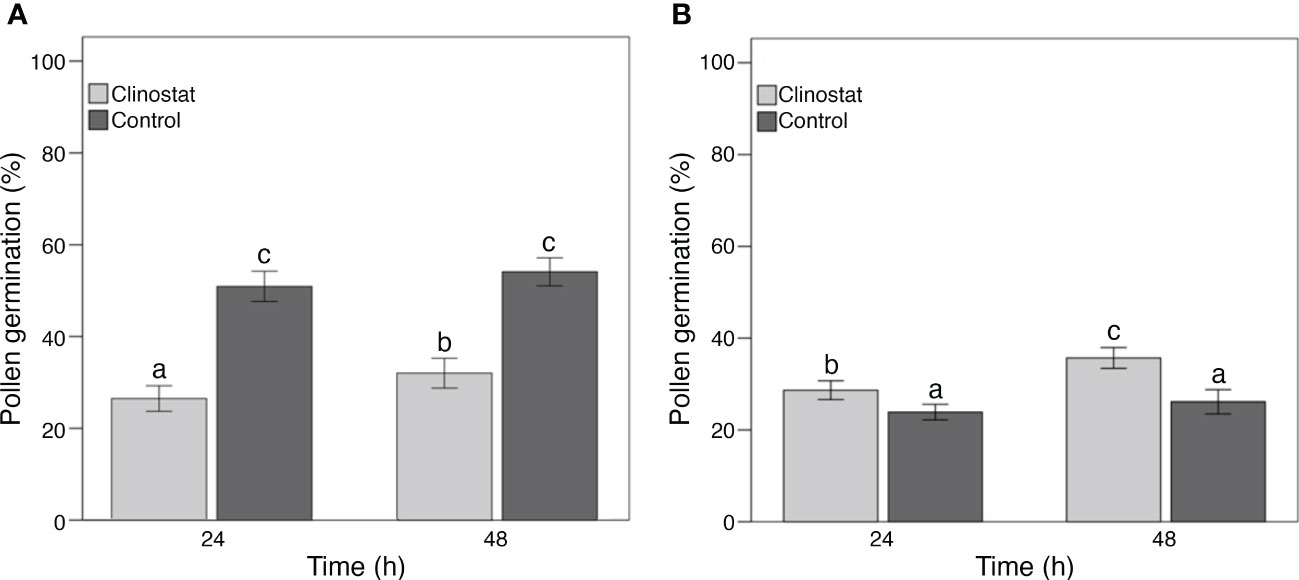
Figure 4 Germination of bulk pollen samples from flowers of ‘Micro-Tom’ (A) and Brassica rapa (B). Pollen germination was scored after 24 and 48 h both in clinostat (simulated microgravity) and control (1g) conditions. Each bar shows the mean pollen germination percentage scored on 50 photos. Significant differences intra-species are reported with different letters (P <0.05). Bars represent ± SE.
Thereafter, the pairwise comparison of pollen tube length between control and clinostat treatments highlighted a similar response in ‘Micro-Tom’ and B. rapa. Despite the great difference in tube length, pollen of both species developed longer tubes in simulated microgravity than in control conditions (Figure 5). Considering pollen tube length and pollen germination data, results highlighted that simulated microgravity promoted pollen tube length in both species despite reducing the germination rate in B. rapa.
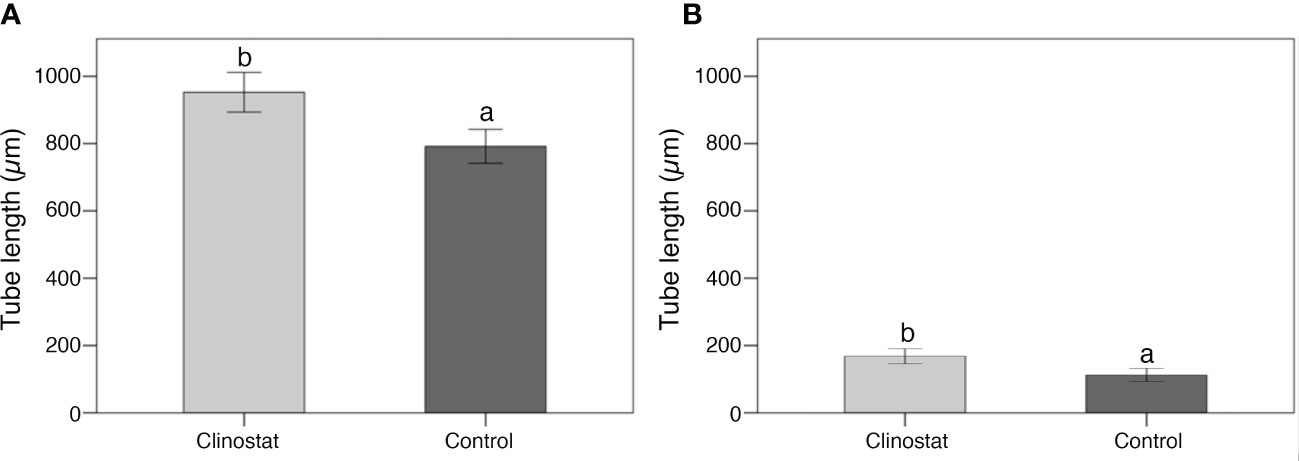
Figure 5 Pollen tube length in clinostat (simulated microgravity) and control (1g) treatments in ‘Micro-Tom’ (A) and Brassica rapa (B) (300 pollen tubes per species). Each bar shows the mean of 150 pollen tube lengths scored on 50 photos. Significant differences intra-species are reported with different letters (P <0.05). Bars represent ± SE.
As far as pollen tube path in germinated grains, a first visual overview of pollen tubes showed a clear difference between control and clinostat treatments, especially in ‘Micro-Tom’ (Figure 6). More in detail, control pollen developed straighter tubes compared to the simulated microgravity condition, in which tube tips changed their direction more frequently throughout the pollen tube path. The straightness/curviness of pollen tube paths was compared between control and clinostat by measuring the tortuosity index in 300 pollen tubes per species. Results showed that simulated microgravity significantly increased pollen tube tortuosity compared to control. Moreover, ANOVA reported that the difference in tortuosity between control and simulated microgravity was more significant in ‘Micro-Tom’ (P <0.001, Figure 7A) than in B. rapa (P <0.05, Figure 7B), which is also highlighted in the box plots showing the distribution of tortuosity values in the two gravity treatments (Figure 7). Indeed, in ‘Micro-Tom,’ most of the values of the tortuosity index are distributed consistently around the median in the control treatment, whereas a much wider distribution with increasing tortuosity values results in the clinostat treatment. Differently, the tortuosity index of B. rapa pollen tubes were similarly distributed around the median both in control and in simulated microgravity, but still had significantly higher values in the clinostat treatment.
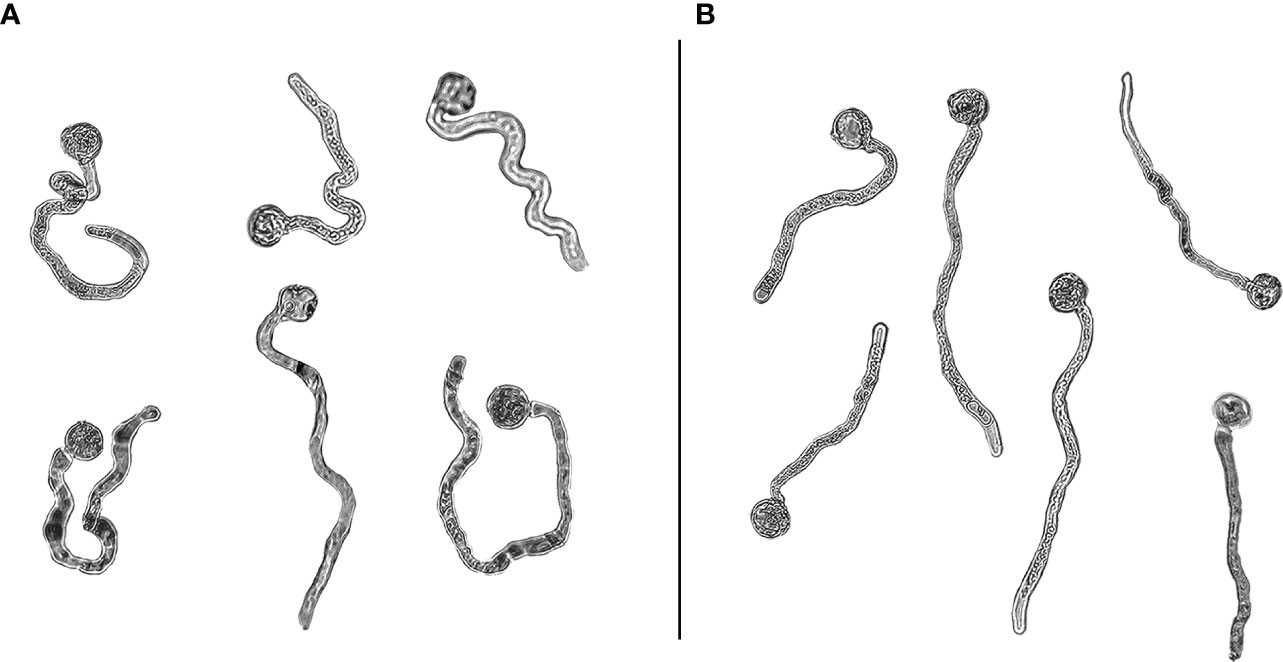
Figure 6 ‘Micro-Tom’ pollen grains germinated in simulated microgravity (A) and in control (B) treatments.
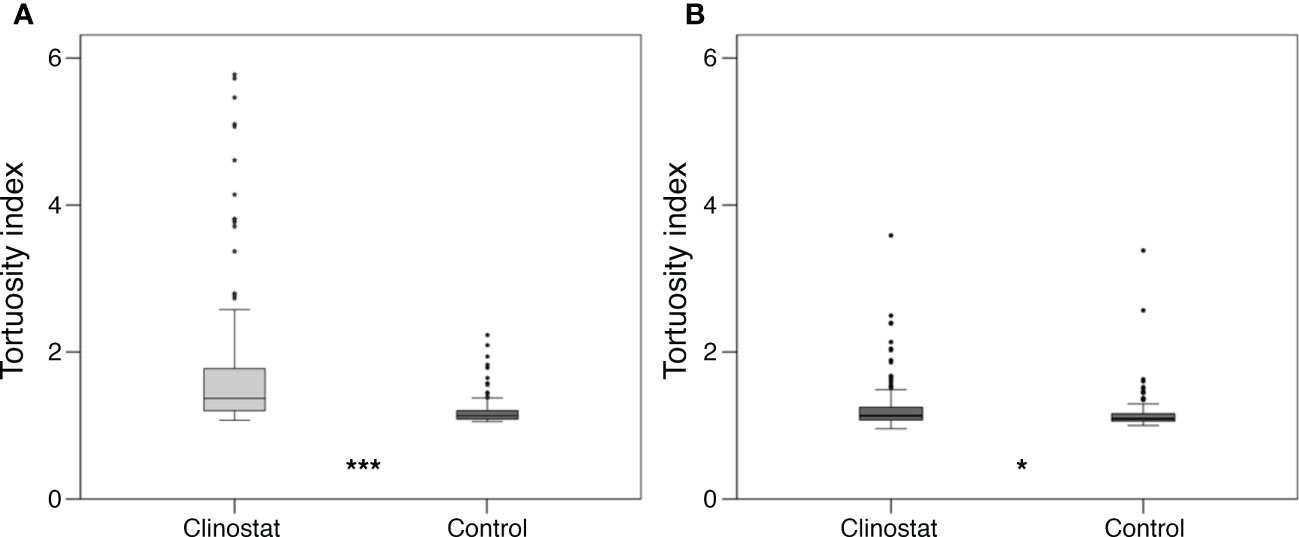
Figure 7 Pollen tube tortuosity of ‘Micro-Tom’ (A) and Brassica rapa (B) (300 pollen tubes per species) in clinostat (simulated microgravity) and control (1g) treatments. Each boxplot shows the values of 150 pollen tubes (***P <0.001; *P <0.05).
Finally, the evaluation of possible gravitropic responses in pollen tubes showed no preferential distribution of pollen tube tips across the Petri dishes, both in control and simulated microgravity treatments (Figure 8). Specifically, we analyzed data assuming no gravitropic response of pollen tubes of ‘Micro-Tom’ and B. rapa, therefore hypothesizing an equal distribution (25% in each quadrant) of tube tips across the Petri dish in both control and simulated microgravity. The Chi-square test revealed no significant differences between observed and expected frequencies of pollen tube tips in the four quadrants, independently of the gravity treatments and the species tested (Table S1, P <0.05). Therefore, we confirmed the null hypothesis that the orientation of pollen tubes of ‘Micro-Tom’ and B. rapa was not subjected to gravitropic responses.
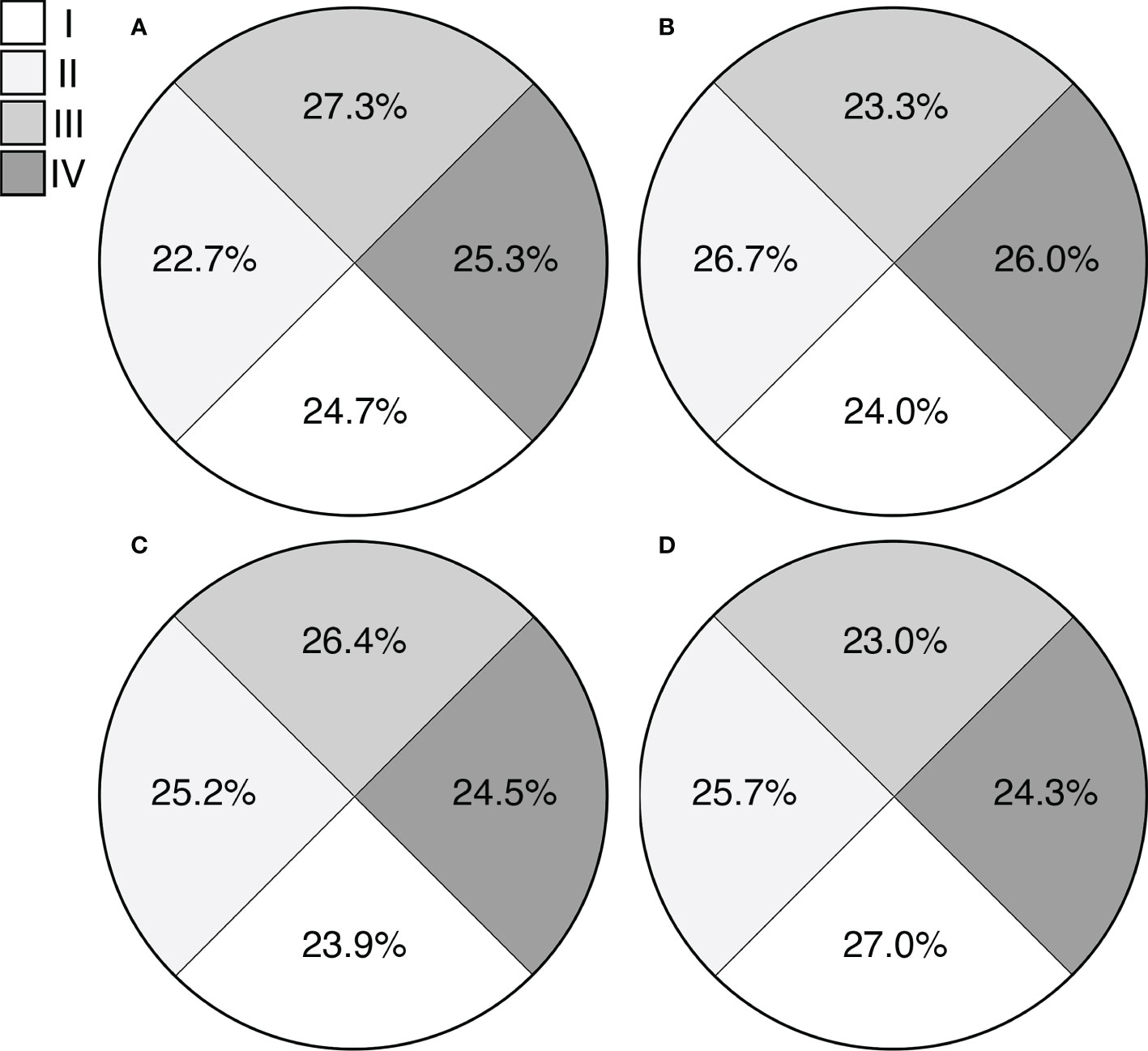
Figure 8 Evaluation of tube tip orientation of ‘Micro-Tom’ in simulated microgravity (A) and control (B) treatments and of Brassica rapa in simulated microgravity (C) and control (D) treatments. Percentage values indicate the observed frequencies of pollen tube tips reaching each quadrant.
4 Discussion
Results on initial pollen viability revealed that growing conditions were optimally set to avoid any interference of abiotic factors with reproductive features. Indeed, pollen samples of both species reported viability over 80%, and no significant differences intraspecies between pollen samples proved high uniformity in pollen grains taken from the same bulk (Figure 3). Therefore, the two bulk pollen samples (one bulk per species) represented a proper starting point to perform further analyses and compare results intra- and inter-species concerning further pollen traits under simulated microgravity conditions.
The viability of pollen grains is not sufficient to predict pollen’s capability to elongate a pollen tube and achieve fertilization (Dafni et al., 2005; Pacini and Dolferus, 2019). Our results also demonstrated that pollen with high viability can germinate under simulated microgravity with a significantly different ratio according to the species tested (Figure 4). Considering this, pollen germination rate may be applied as an inclusion criterion in ranking space crops for seed-to-seed cultivation in space (Aronne et al., 2020). Both pollen viability and germination are strongly correlated with fruit and seed set (Paupière et al., 2017; Herrera et al., 2018) and are therefore essential to ensuring repeated seed-to-seed cycles on board a spacecraft.
Since most plant breeding research has been conducted through the sporophyte, the influence of the gametophytic phase, including pollen tube development, has been generally overlooked. However, the possibility of applying gametophytic selection in plant breeding programs on Earth had already been supported by Hormaza and Herrero (1992), who highlighted the overlap in the genetic expression between the sporophyte and the gametophyte in relation to different abiotic stresses (Mulcahy, 1984; Ottaviano and Mulcahy, 1989; Ottaviano et al., 1990). Therefore, the selection of space candidate species and cultivars with the highest germination rate of pollen developed under altered gravity conditions can be considered a potential application of gametophytic selection in a space environment. Since it can be performed in a small environment and over a short period, this breeding procedure could be conducted by astronauts with the aim of screening and selecting species and cultivars that best adapt to real microgravity (De Micco et al., 2013).
To date, research has shown that simulated microgravity affects several aspects of pollen tube development (De Micco et al., 2005; De Micco et al., 2006), but how the gravity vector could affect pollen tube movements is still unknown. Despite this study proving a clear effect of simulated microgravity on the tortuosity of pollen tube movements (Figure 6), our results excluded gravitropism as a pollen tube response affecting the final position of tube tips in the germination medium (Figure 8).
How clinorotation affect the tortuosity of pollen tubes might be addressed by the key role of the cytoskeleton in coordinating vesicle trafficking responsible for pollen tube elongation (Sack, 1991). More specifically, clinorotation may have altered the production of metabolites related to pollen tube development, such as starch and callose, involved in the same ultrastructural changes occurring in the actin cytoskeleton of pollen tubes during the self-incompatibility reactions (De Micco et al., 2006; Cai and Del Duca, 2019). Indeed, the actin filaments located in the sub-apical portion of elongating pollen tubes are reported to be involved in the vesicle trafficking responsible for the formation and maintenance of the tube apex (Vidali et al., 2009; Qin and Yang, 2011; Hepler and Winship, 2014; Fu, 2015). Actin filaments of the cytoskeleton have a crucial role in tube development and growth direction, but how altered gravity can regulate the directionality of pollen tubes remains to be determined (Cheung et al., 2010).
To date, the microgametophyte (pollen) tube path towards the ovary is known to be mainly driven by chemotropic stimuli related to the Ca2+ gradient throughout the style (Kandasamy et al., 1994; Kim et al., 2019), whereas the possible co-existence with pollen gravitropism has been generally overlooked. Despite in vitro conditions being assumed to mimic the natural state of pollen during pollen tube elongation, functional differences with in vivo or in semi-vivo conditions might occur. For instance, pollen tubes elongate faster, tubes live longer, and sperm cells (in bicellular species) form sooner and more reliably in vivo than in vitro (Kakani et al., 2005; Shi et al., 2018). Pollen tube growth in vivo involves interactions with pistil tissues, especially at critical stages such as tube reorientation and the cessation of growth. Thus, considering the role of chemotropism in the directional growth of pollen tubes, we performed the experiment in vitro to isolate the effect of the gravity vector on pollen tube directionality and exclude interferences related to chemotropic stimuli occurring in vivo.
Overall, our analyses investigating male gametophyte functionality in simulated microgravity suggested that pollen germination and tube directional growth are affected by changes in gravity and highlighted different responses between ‘Micro-Tom’ and B. rapa. To date, research has never considered gravitropism as a driver for pollen tube movement. Our in vitro germination tests represented the best first approach to exclude possible chemotropic interferences and isolate the effect of the altered gravity vector on the directional growth of pollen tubes.
Even though our results excluded gravitropism in pollen tubes, further tests are necessary to confirm our results in real microgravity conditions and in other candidate space crops. In conclusion, a better understanding of how gravity can interfere with plant reproduction is needed to ensure seed and fruit production over repeated seed-to-seed-to-seed cycles, and it is a first step towards self-sufficiency from Earth supplies in long-term space missions.
Data availability statement
The raw data supporting the conclusions of this article will be made available by the authors, without undue reservation.
Author contributions
MI: Conceptualization, methodology, investigation, visualization, and writing—original draft preparation. LI: Investigation, visualization, and writing—review & editing. LR: Writing—review & editing. GA: Conceptualization, methodology, writing—review & editing, and funding acquisition. All authors listed have made a substantial, direct, and intellectual contribution to the work and approved it for publication.
Funding
This research has been financially supported by the project In-situ REsource Bio-Utilization for life Support system (ReBUS), unique project code (CUP) F74I16000000005 financed by the Italian Space Agency.
Conflict of interest
The authors declare that the research was conducted in the absence of any commercial or financial relationships that could be construed as a potential conflict of interest.
Publisher’s note
All claims expressed in this article are solely those of the authors and do not necessarily represent those of their affiliated organizations, or those of the publisher, the editors and the reviewers. Any product that may be evaluated in this article, or claim that may be made by its manufacturer, is not guaranteed or endorsed by the publisher.
Supplementary material
The Supplementary Material for this article can be found online at: https://www.frontiersin.org/articles/10.3389/fpls.2023.1186967/full#supplementary-material
References
Aronne, G., Iovane, M., Strumia, S. (2021). Temperature and humidity affect pollen viability and may trigger distyly disruption in threatened species. Ann. D. Bot. 11, 77–82. doi: 10.13133/2239-3129/17157
Aronne, G., Romano, L. E., Izzo, L. G. (2020). Subsequent inclusion/exclusion criteria to select the best species for an experiment performed on the ISS in a refurbished hardware. Life Sci. Space Res. 27, 19–26. doi: 10.1016/j.lssr.2020.07.002
Burgner, S. E., Nemali, K., Massa, G. D., Wheeler, R. M., Morrow, R. C., Mitchell, C. A. (2020). Growth and photosynthetic responses of Chinese cabbage (Brassica rapa l. cv. Tokyo bekana) to continuously elevated carbon dioxide in a simulated space station “Veggie” crop-production environment. Life Sci. Space Res. 27, 83–88. doi: 10.1016/j.lssr.2020.07.007
Cai, G., Del Duca, S. (2019). Pollen tube and plant reproduction. Int. J. Mol. Sci. 20 (3), 531. doi: 10.3390/ijms20030531
Cheung, A. Y., Niroomand, S., Zou, Y., Wu, H. M. (2010). A transmembrane formin nucleates subapical actin assembly and controls tip-focused growth in pollen tubes. Proc. Natl. Acad. Sci. 107 (37), 16390–16395. doi: 10.1073/pnas.1008527107
Colla, G., Rouphael, Y., Cardarelli, M., Mazzucato, A., Olimpieri, I. (2007). Growth, yield and reproduction of dwarf tomato grown under simulated microgravity conditions. Plant Biosyst. 141, 75–81. doi: 10.1080/11263500601153735
Dafni, A., Kevan, P. G., Husband, B. C. (2005). Practical pollination biology. (Cambridge: Enviroquest Ltd).
Dedolph, R. R., Dipert, M. H. (1971). The physical basis of gravity stimulus nullification by clinostat rotation. Plant Physiol. 47 (6), 756–764. doi: 10.1104/pp.47.6.756
De Micco, V., Aronne, G., De Pascale, S. (2005). Effect of simulated microgravity on seedling development and vascular differentiation of soy. Acta Astronautica 58 (3), 139–148. doi: 10.1016/j.actaastro.2005.06.002
De Micco, V., Pascale, S., Paradiso, R., Aronne, G. (2013). Microgravity effects on different stages of higher plant life cycle and completion of the seed-to-seed cycle. Plant Biol. J. 16, 31–38. doi: 10.1111/plb.12098
De Micco, V., Scala, M., Aronne, G. (2006). Effects of simulated microgravity on male gametophyte of prunus, pyrus, and Brassica species. Protoplasma 228, 121–126. doi: 10.1007/s00709-006-0161-7
De Pascale, S., Arena, C., Aronne, G., De Micco, V., Pannico, A., Paradiso, R., et al. (2021). Biology and crop production in space environments: challenges and opportunities. Life Sci. Sp. Res. 29, 30–37. doi: 10.1016/j.lssr.2021.02.005
Fu, Y. (2015). The cytoskeleton in the pollen tube. Curr. Opin. Plant Biol. 28, 111–119. doi: 10.1016/j.pbi.2015.10.004
Güçlü, F., Koyuncu, F. (2017). Effects of relative humidity on in vitro pollen germination and tube growth in sweet cherries (Prunus avium l.). Sci. Pap. Ser. B Hortic. 61, 15–19.
Hepler, P. K., Winship, L. J. (2014). The pollen tube clear zone: clues to the mechanism of polarized growth. J. Integr. Plant Biol. 57, 79–92. doi: 10.1111/jipb.12315
Herrera, S., Lora, J., Hormaza, J. I., Herrero, M., Rodrigo, J. (2018). Optimizing production in the new generation of apricot cultivars: self-incompatibility, s-RNase allele identification, and incompatibility group assignment. Front. Plant Sci. 9. doi: 10.3389/fpls.2018.00527
Hormaza, J. I., Herrero, M. Pollen selection. Theor. Appl. Genet. (1992) 83 (6-7), 663–672. doi: 10.1007/BF00226682
Iovane, M., Aronne, G. (2021). High temperatures during microsporogenesis fatally shorten pollen lifespan. Plant Reprod. 35, 9–17. doi: 10.1007/s00497-021-00425-0
Iovane, M., Cirillo, A., Izzo, L. G., Di Vaio, C., Aronne, G. (2022a). High temperature and humidity affect pollen viability and longevity in olea europaea l. Agronomy 12, 1–12. doi: 10.3390/agronomy12010001
Iovane, M., Izzo, L. G., Cirillo, A., Romano, L. E., Di Vaio, C., Aronne, G. (2022b). Flowering and pollen resilience to high temperature of apricot cultivars. Scientia Hortic. 304, 111261. doi: 10.1016/j.scienta.2022.111261
Izzo, L. G., Romano, L. E., Muthert, L. W. F., Iovane, M., Capozzi, F., Manzano, A., et al. (2022). Interaction of gravitropism and phototropism in roots of Brassica oleracea. environ. Exp. Bot. 193, 104700. doi: 10.1016/j.envexpbot.2021.104700
Kakani, V. G., Reddy, K. R., Koti, S., Wallace, T. P., Prasad, P. V. V., Reddy, V. R., et al. (2005). Differences in in vitro pollen germination and pollen tube growth of cotton cultivars in response to high temperature. Ann. Bot. 96 (1), 59–67. doi: 10.1093/aob/mci149
Kandasamy, M. K., Nasrallah, J. B., Nashrallah, M. E. (1994). Pollen-pistil interactions and developmental regulation of pollen tube growth in Arabidopsis. Development 120, 3405–3418. doi: 10.1242/dev.120.12.3405
Karapanos, I. C., Akoumianakis, K. A., Olympios, C. M., Passam, H. C. (2010). Tomato pollen respiration in relation to in vitro germination and pollen tube growth under favourable and stress-inducing temperatures. Sex Plant Reprod. 23, 219–224. doi: 10.1007/s00497-009-0132-1
Kim, Y. J., Zhang, D., Jung, K. H. (2019). Molecular basis of pollen germination in cereals. Trends Plant Sci. 24 (12), 1126–1136. doi: 10.1016/j.tplants.2019.08.005
Kiss, J. Z., Wolverton, C., Wyatt, S. E., Hasenstein, K. H., van Loon, J. J. W. A. (2019). Comparison of microgravity analogs to spaceflight in studies of plant growth and development. Front. Plant Sci. 10, 1577. doi: 10.3389/fpls.2019.01577
Kordyum, E., Hasenstein, K. H. (2021). Plant biology for space exploration – building on the past, preparing for the future. Life Sci. Sp. Res. 29, 1–7. doi: 10.1016/j.lssr.2021.01.003
Kordyum, E., Hedukha, O., Artemeko, O., Ivanenko, G. (2020). Seed and vegetative propagation of plants in microgravity 7 (5), 500–507. doi: 10.15982/j.issn.2096-9287.2020.20191113001
Kordyum, E. L., Sytnik, K. M., Chernyaeva, I. I. (1983). Peculiarities of genital organ formation in Arabidopsis thaliana (L) heynh. under spaceflight conditions. Adv. Space Res. 3 (9), 247–250. doi: 10.1016/0273-1177(83)90064-9
Kuang, A., Musgrave, M. E., Matthews, S. W. (1996). Modification of reproductive development in Arabidopsis thaliana under spaceflight conditions. Planta 198, 588–594. doi: 10.1007/BF00262646
Kuang, A., Musgrave, M. E., Matthews, S. W., Cummins, D. B., Tucker, S. C. (1995). Pollen and ovule development in Arabidopsis thaliana under spaceflight conditions. Am. J. Bot. 82 (5), 585–595. doi: 10.1002/j.1537-2197.1995.tb11503.x
Kuang, A., Popova, A., Xiao, Y., Musgrave, M. E. (2000a). Pollination and embryo development in Brassica rapa l. @ in microgravity. Int. J. Plant Sci. 161, 203–211. doi: 10.1086/314254
Kuang, A., Xiao, Y., McClure, G., Musgrave, M. E. (2000b). Influence of microgravity on ultrastructure and storage reserves in seeds of Brassica rapa l. Ann. Bot. 85, 851–859. doi: 10.1006/anbo.2000.1153
Link, B. M., Busse, J. S., Stankovic, B. (2014). Seed-to-seed-to-seed growth and development of Arabidopsis in microgravity. Astrobiology 14, 866–875. doi: 10.1089/ast.2014.1184
Matsuda, R., Ozawa, N., Fujiwara, K. (2014). Leaf photosynthesis, plant growth, and carbohydrate accumulation of tomato under different photoperiods and diurnal temperature differences. Sci. Hortic. 170, 150–158. doi: 10.1016/j.scienta.2014.03.014
Matsukura, C., Aoki, K., Fukuda, N., Mizoguchi, T., Asamizu, E., Saito, T., et al. (2008). Comprehensive resources for tomato functional genomics based on the miniature model tomato micro-tom. Curr. Genomics 9 (7), 436–443. doi: 10.2174/138920208786241225
Merkies, A. I., Laurinavichyus, R. S. (1983). Complete cycle of individual development of Arabidopsis thaliana haynh plants at salyut orbital station. Doklady Akademii Nauk SSSR 271 (2), 509–512.
Mulcahy, D. L. (1984). “Manipulation of gametophytic populations,” in Efficiency in plant breeding: proceedings 10th congress on eucarpia. Eds. Lan-gel, W., Zeven, A. C., Hogenboom, N. G. (Wageningen, the Netherlands: Pudoc, Wageningen), p 167–p 175.
Musgrave, M. E., Kuang, A., Tuominen, L. K., Levine, L. H., Morrow, R. C. (2005). Seed storage reserves and glucosinolates in Brassica rapa l. grown on the international space station. J. Am. Soc Hortic. Sci. 130, 848–856. doi: 10.21273/jashs.130.6.848
Musgrave, M. E., Kuang, A., Xiao, Y., Stout, S. C., Bingham, G. E., Briarty, L. G., et al. (2000). Gravity independence of seed-to-seed cycling in Brassica rapa. Planta 210 (3), 400–406. doi: 10.1007/PL00008148
Muthert, L. W. F., Izzo, L. G., van Zanten, M., Aronne, G. (2020). Root tropisms: investigations on earth and in space to unravel plant growth direction. Front. Plant Sci. 10, 1807. doi: 10.3389/fpls.2019.01807
Ottaviano, E., Mulcahy, D. L. (1989). Genetics of angiosperm pollen. Adv. Genet. 26, 1–64. doi: 10.1016/S0065-2660(08)60222-9
Ottaviano, E., Sari-Gorla, M., Mulcahy, D. L. (1990). “Pollen selection: efficiency and monitoring,” in Isozymes: structure, function, and use in biology and medicine. Eds. Ogita, Z. I., Market, C. L. (New York: Wiley-Liss), p 575–p 588.
Pacini, E., Dolferus, R. (2019). Pollen developmental arrest: maintaining pollen fertility in a world with a changing climate. Front. Plant Sci. 10. doi: 10.3389/fpls.2019.00679
Paupière, M. J., van Haperen, P., Rieu, I., Visser, R. G. F., Tikunov, Y. M., Bovy, A. G. (2017). Screening for pollen tolerance to high temperatures in tomato. Euphytica 213, 230. doi: 10.1007/s10681-017-1927-z
Qin, Y., Yang, Z. (2011). Rapid tip growth: insights from pollen tubes. Seminars Cell & Development. biol. 22 (8), 816–824. doi: 10.1016/j.semcdb.2011.06.004
Romano, L. E., Aronne, G. (2021). The world smallest plants (Wolffia sp.) as potential species for bioregenerative life support systems in space. Plants 10 (9), 1896. doi: 10.3390/plants10091896
Sack, F. D. (1991). Plant gravity sensing. Int. Rev. Cytol 127, 193–252. doi: 10.1016/S0074-7696(08)60695-6
Salisbury, F. B. (1999). “Chapter 5 growing crops for space explorers on the moon, Mars, or in space,” in Advances in space biology and medicine. Ed. Bontinc, S. L. (Stamford, Connecticut: Elsevier), 131–162.
Sathasivam, M., Hosamani, R., K Swamy, B., Kumaran G, S. (2021). Plant responses to real and simulated microgravity. Life Sci. Sp. Res 28, 74–86. doi: 10.1016/j.lssr.2020.10.001
Sato, S., Katoh, N., Iwai, S., Hagimori, M. (1998). Establishment of reliable methods of in vitro pollen germination and pollen preservation of Brassica rapa (syn. B. campestris). Euphytica 103, 29–33. doi: 10.1023/A:1018381417657
Sato, S., Peet, M. M., Gardner, R. G. (2001). Formation of parthenocarpic fruit, undeveloped flowers and aborted flowers in tomato under moderately elevated temperatures. Sci. Hortic. 90, 243–254. doi: 10.1016/S0304-4238(00)00262-4
Shi, W., Li, X., Schmidt, R. C., Struik, P. C., Yin, X., Jagadish, S. K. (2018). Pollen germination and in vivo fertilization in response to high-temperature during flowering in hybrid and inbred rice. Plant Cell Environ. 41 (6), 1287–1297. doi: 10.1111/pce.13146
Shikata, M., Ezura, H. (2016). Micro-tom tomato as an alternative plant model system: mutant collection and efficient transformation. Plant Signal Transduction: Methods Protoc. 1363, 47–55. doi: 10.1007/978-1-4939-3115-6_5
Tatematsu, K., Kumagai, S., Muto, H., Sato, A., Watahiki, M. K., Harper, R. M., et al. (2004). Massugu2 encodes Aux/IAA19, an auxin-regulated protein that functions together with the transcriptional activator NPH4/ARF7 to regulate differential growth responses of hypocotyl and formation of lateral roots in Arabidopsis thaliana. Plant Cell 16, 379–393. doi: 10.1105/tpc.018630
Vidali, L., Rounds, C. M., Hepler, P. K., Bezanilla, M. (2009). Lifeact-mEGFP reveals a dynamic apical f-actin network in tip growing plant cells. PloS One 4 (5), e5744. doi: 10.1371/journal.pone.0005744
Keywords: simulated microgravity, pollen tube, pollen germination, clinostat, space crops, Micro-Tom, Brassica rapa, seed-to-seed
Citation: Iovane M, Izzo LG, Romano LE and Aronne G (2023) Simulated microgravity affects directional growth of pollen tubes in candidate space crops. Front. Plant Sci. 14:1186967. doi: 10.3389/fpls.2023.1186967
Received: 15 March 2023; Accepted: 09 May 2023;
Published: 02 June 2023.
Edited by:
Maurice Bosch, Aberystwyth University, United KingdomReviewed by:
Ioannis-Dimosthenis S. Adamakis, National and Kapodistrian University of Athens, GreeceSarah E. Wyatt, Ohio University, United States
Copyright © 2023 Iovane, Izzo, Romano and Aronne. This is an open-access article distributed under the terms of the Creative Commons Attribution License (CC BY). The use, distribution or reproduction in other forums is permitted, provided the original author(s) and the copyright owner(s) are credited and that the original publication in this journal is cited, in accordance with accepted academic practice. No use, distribution or reproduction is permitted which does not comply with these terms.
*Correspondence: Maurizio Iovane, bWF1cml6aW8uaW92YW5lQHVuaW5hLml0; Luigi Gennaro Izzo, bHVpZ2lnZW5uYXJvLml6em9AdW5pbmEuaXQ=