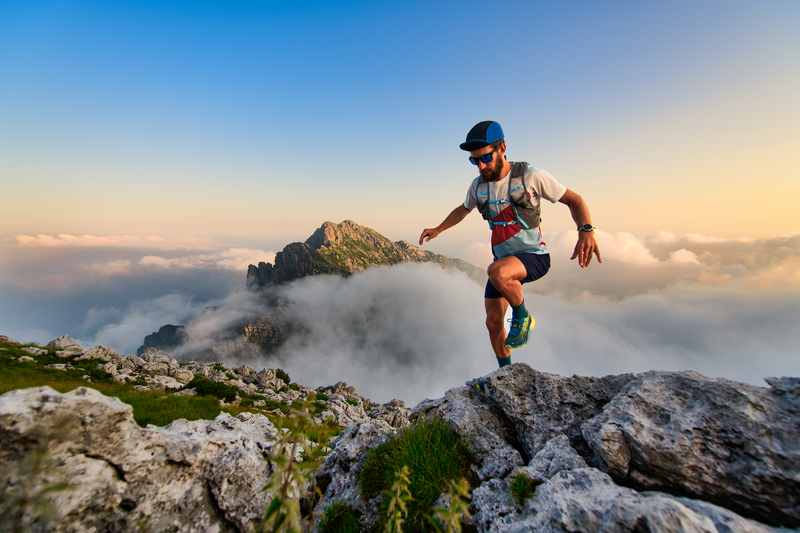
94% of researchers rate our articles as excellent or good
Learn more about the work of our research integrity team to safeguard the quality of each article we publish.
Find out more
REVIEW article
Front. Plant Sci. , 12 July 2023
Sec. Plant Symbiotic Interactions
Volume 14 - 2023 | https://doi.org/10.3389/fpls.2023.1169310
Arbuscular mycorrhizal fungi (AMF) are ubiquitous in soil and form nutritional symbioses with ~80% of vascular plant species, which significantly impact global carbon (C) and nitrogen (N) biogeochemical cycles. Roots of plant individuals are interconnected by AMF hyphae to form common AM networks (CAMNs), which provide pathways for the transfer of C and N from one plant to another, promoting plant coexistence and biodiversity. Despite that stable isotope methodologies (13C, 14C and 15N tracer techniques) have demonstrated CAMNs are an important pathway for the translocation of both C and N, the functioning of CAMNs in ecosystem C and N dynamics remains equivocal. This review systematically synthesizes both laboratory and field evidence in interplant C and N transfer through CAMNs generated through stable isotope methodologies and highlights perspectives on the system functionality of CAMNs with implications for plant coexistence, species diversity and community stability. One-way transfers from donor to recipient plants of 0.02-41% C and 0.04-80% N of recipient C and N have been observed, with the reverse fluxes generally less than 15% of donor C and N. Interplant C and N transfers have practical implications for plant performance, coexistence and biodiversity in both resource-limited and resource-unlimited habitats. Resource competition among coexisting individuals of the same or different species is undoubtedly modified by such C and N transfers. Studying interplant variability in these transfers with 13C and 15N tracer application and natural abundance measurements could address the eco physiological significance of such CAMNs in sustainable agricultural and natural ecosystems.
• Plants interconnected by arbuscular mycorrhizal (AM) fungi form common AM networks
• 13C and 15N labeling can trace the amount of AM-mediated interplant C and N transfers
• 0.02‒41% C transfers are from a donor to a receiver, but< 10% in the reverse route
• 0.04‒80% N transfers are from a donor to a receiver, but< 15% in the reverse route
• Interplant C and N transfers should enhance plant survival under nutrient-limitations
Arbuscular mycorrhizas (AM) are formed between arbuscular mycorrhizal fungi (AMF) and roots of ~70% of ~391,000 higher plant species (Wang and Qiu, 2006; Smith and Read, 2008; Brundrett, 2009; Brundrett, 2017; Brundrett and Tedersoo, 2018). Currently 25 genera and ~338 fungal species belonging to the sub-Phylum Glomeromycota form AMF globally (Schüßler and Walker, 2010). AMF acquire soil nutrients, such as nitrogen (N), phosphorus (P) and other mineral nutrients, and transport them to their host plant in exchange for up to 20% of photosynthetically fixed carbon (C) (Smith and Read, 2008; Roth and Paszkowski, 2017). In an arbuscular mycorrhiza, the intraradical mycelium (IRM) often penetrates root cortical cells to form arbuscules, while the extraradical mycelium (ERM) extends into soil, far beyond the root zone. The ERM forages for N, P, potassium and other soil nutrients, and translocates them to the IRM, where they are exchanged for C from the host (Smith et al., 2009). The ERM is extensive enabling plant access to nutrient resources well beyond the root depletion zone (Li et al., 1991). In addition, several findings revealed that sources of carbon for mutualistic AMF include fatty acids exported from the host plants, as well as lipids and sugars (Pfeffer et al., 1999; Keymer et al., 2017; Jiang et al., 2017).
AMF are ubiquitous components of most soil ecosystems, where they grow through soil, colonize plant roots, and can form links between plants (Newman et al., 1992; Newman et al., 1994; He et al., 2003; He et al., 2009; Molina and Horton, 2015). The plants suppling AMF with labile carbon often grow close together, primarily in multiple species communities. Because AMF exhibit little host specificity (Smith and Read, 2008), and plant roots can thus be linked by a common AM network (CAMN) (Wipf et al., 2019). Such CAMNs, being formed among individual plants of the same species or genus, or from different genera or families (Ronsheim and Anderson, 2001; Southworth et al., 2005), are usually woven into an even larger network of fungi and roots in natural communities (Smith and Read, 2008; Wipf et al., 2019). In this way, plant species within CAMNs may be joined together as a functional guild and become pathways for movement or transfer of nutrients (Figure 1), including C (Francis and Read, 1984; Martins, 1992; Martins, 1993; Watkins et al., 1996; Fitter et al., 1998; Mikkelsen et al., 2008; Voets et al., 2008; Walder et al., 2012), N (Hamel et al., 1991a; Hamel et al., 1991b; He et al., 2003; He et al., 2009; Frey and Schüepp, 1993; Rogers et al., 2001; Moyer-Henry et al., 2006), P (Tuffen et al., 2002; Smith and Smith, 2011; Merrild et al., 2013), arsenic (P analog, Meding and Zasoski, 2008), cadmium (Ding et al., 2022), K (Gao et al., 2021), cesium (Meding and Zasoski, 2008; Gyuricza et al., 2010), rubidium (K analogs) and strontium (Ca analog) (Meding and Zasoski, 2008, and zinc (Cardini et al., 2021). Water (Egerton-Warburton et al., 2007) and genetic material (Giovannetti et al., 2004) can also move within these networks. Movement of these materials can thus promote coexistence and biodiversity among plants (Read, 1991; Smith and Read, 2008).
Figure 1 Milestones in nutrient transfers through common mycorrhizal networks (CMNs) (Note that the year of a reference pointing to the green arrow bar is not to scale).
Despite the considerable evidence of the functional role of CAMNs, they have not been directly visualized in natural ecosystems due to their cryptic, fragile, and microscopic nature (Newman et al., 1994; Ronsheim and Anderson, 2001; Southworth et al., 2005; Wipf et al., 2019). Plants invest photosynthetic products to feed their fungal partners, which, in return, provide mineral nutrients foraged in soil by their intricate hyphal networks (Bever et al., 2010). The Driver (AMF partners drive plant communities) and Passenger (AMF community dynamics follows changes in the host plant community) hypotheses were suggested to explain the mutual relationships of plant and AMF communities (Zobel and Öpik, 2014). Research into this complex system of plant-fungus interactions indicates that plants and fungi can choose their trading partners (Kiers et al., 2011; Walder et al., 2012).
An understanding of the stoichiometry of C, N, or other nutrients mediated by CAMNs could better elucidate the potential roles of CAMNs in C and N functioning in plant-soil systems (Figure 2), although at present CAMNs have not been directly visualized in natural ecosystems due to their fragile and microscopic nature. Application of high-throughput genome sequences or all sorts of omics and BONCAT-FACS (bioorthogonal non-canonical amino acid tagging + fluorescence-activated cell sorting, Couradeau et al., 2019) could have an in situ observation of these underground cryptic microorganisms. Meanwhile, the employment of other emerging technologies, such as cryo-scanning electron microscope (Cryo-SEM), DNA stable isotope probing (DNA-SIP), quantitative multi-isotope imaging mass spectrometry (MIMS), nanoscale secondary ion mass spectrometry (NanoSIMS), single-molecule electronic device and synchrotron radiation facility, could enable the mapping the interplant flow of 13C and 15N through CAMNs. Given this demonstrated autonomy and the key role that CAMNs play in interplant nutrient transfers and biodiversity in ecosystems, it is crucial to understand how nutrient resources (e.g., C, N, P, other elements, see Figure 1) are shared among plants through CAMNs. And whether there may be a mechanism between CAMNs and ecosystems by which a greater biodiversity is associated with a greater productivity.
Figure 2 A conceptual framework of roles played by common mycorrhizal networks (CMNs) in regulating carbon (C) and nitrogen (N) flow or transfer within and between plants.
The abundance level of stable isotopes is theoretically expressed as delta (δ) in parts per thousand or per mil (‰), which is calculated as δ13C or δ15N (‰) = [(RSample/RStandard) –1] × 1,000, where R is the 13C/12C or 15N/14N (atom%) ratio of the sample and standard, and “Vienna”-Pee Dee Belemnite (0.0112372) or atmospheric N2 (0.0036765) is their respective standard material. The 13C and 15N isotopic composition (also expressed as δ13C and δ15N signatures) of plant materials can provide information on (i) inputs of photosynthetic C or uptake of fertilizer N, (ii) plant N derived from N2 fixation by symbiotic microorganisms, (iii) C or N cycling and (iv) the sources of N available for host plant growth (Dawson et al., 2002). For instance, the δ13C or δ15N signatures in vegetation could reflect the relative availability of C sources to fungi and N sources to plants differing in isotopic composition (Querejeta et al., 2003). Here we examine the unique and common characteristics of CMN-mediated interplant C and N transfers that are demonstrated by 13C and 15N labeling (sometimes referred to as 13C and 15N enrichment) or variations in their isotopic composition for exploring the beneficial functionality of CMNs in sustaining managed and natural systems in a changing climate (Dawson et al., 2002; He et al., 2003; Querejeta et al., 2003; Moyer-Henry et al., 2006; He et al., 2009; Jalonen et al., 2009; Kurppa et al., 2010; Walder et al., 2012; Ren et al., 2013; Meng et al., 2015; Wang et al., 2016; Řezáčová et al., 2018; Wipf et al., 2019; Muneer et al., 2020a; Alaux et al., 2021; Avital et al., 2022; Reay et al., 2022).
Estimates of C or N transfer from a donor to a receiver plant are based on the assumption that an equal proportion of applied and unapplied C or N are transferred. The percentage of total C or N transferred to the receiver (% Ntransfer) is then assessed from the ratio of applied C or N in the receiver and total applied C or N in the receiver and donor. Based mostly on the calculations from Giller et al. (1991); Ikram et al. (1994); Johansen and Jensen (1996), the following equations are commonly employed by almost all relevant studies to calculate C or N transfers.
where 13Ccontentplant or 15Ncontentplant = atom%13Cexcessplant or 15Nexcessplant ×
and atom%13Cexcessplant or atom%15Nexcessplant = atom%13Cplant or
The amount of C or N (mg plant−1) transferred from the donor (Ctransfer or Ntransfer) is calculated as:
The % of C or N in the receiver derived from transfer (% CDFT or % NDFT) is calculated as:
In the AM associations, C is the major flux from plant to fungus while P, and possibly N, are the primary fluxes from fungus to plant (Smith and Read, 2008). In general, 5–20% of plant assimilated net C was transferred between linked plants via the CAMNs (Pearson and Jakobsen, 1993). Using 13CO2 to label Hypochaeris radicata growing in Danish coastal grasslands and tracing that labeled C for one growing season, Lekberg et al. (2013) concluded that plants allocated C to AMF even at temperatures close to freezing and that fungal structures persisted in the roots during periods of little C-allocation. Plants could release 13C into rhizosphere soil through AM mycelia. These results suggest that the host plant maintained a supply of C to its AMF symbionts to ensure its own ability to obtain soil mineral nutrition from the AMF’s mycelia (Fitter et al., 1998; Lekberg et al., 2010). On the other hand, C transfer via an AM network does not allow resource sharing among linked plants (Robinson and Fitter, 1999). The mycocentric view is that fungal structures within roots are parts of extended mycelia through which fungi move C according to their own C demands, not those of their autotrophic hosts (Fitter et al., 1998).
Since the growth of AMF completely depends on supply of photosynthetically fixed C from their hosts, the C supply from the plant can be regarded as an infinitely large benefit for AMF fitness (Bago et al., 2000) (Figure 2). From the perspective of the plant, the amount of C provided to the fungal symbiont represents the symbiotic costs (Konvalinková and Jansa, 2016). The dynamics of C exchange between plants and fungi in AM associations is conceptualized as a biological market, in which C sources are reciprocally exchanged in both directions, with preferential allocation to the partner offering the best rate of exchange (Dawson et al., 2002; Werner et al., 2014; Konvalinková and Jansa, 2016). Previous studies have suggested that there is an asymmetry in C-for-nutrient exchange between AMF and host plants. AMF acquire C from their hosts not only as carbohydrates but also as fatty acids (Pfeffer et al., 1999; Trépanier et al., 2005; Jiang et al., 2017; Keymer et al., 2017; Luginbuehl et al., 2017). It is known that the extraradical hyphae and spores of AMF secrete a special glycoprotein, glomalin into the soil, defined as glomalin-related soil protein (GRSP) (Rillig, 2004). GRSP is released to cover the surface of soil organic matter and aggregates, and can store C in protein and carbohydrate subunits, forming a protective layer that avoids the loss of nutrients (e.g., C) in soil aggregates (Schindler et al., 2007; Wang et al., 2023).
AMF manage plant-soil interactions, suppling mineral nutrients to host plants while providing a conduit of C to soil microbial community. Field studies applying 13CO2 pulse labeling have demonstrated that AMF ERM provides a rapid and important pathway of C flux from plants to soil and the atmosphere (Johnson et al., 2002). By tracing in situ flows of photo-assimilated C of 13CO2-exposed wheat (Triticum aestivum) through AMF into root- and hyphae-associated soil microbial communities over an eight-hour period, Kaiser et al. (2015) found that intraradical AMF hyphae were significantly 13C-enriched compared to the root-cortex area, suggesting an efflux of photosynthate C from the plant to the mycorrhizosphere over time. In addition, they showed that 13C photosynthate was delivered to general bacteria and Gram-negative bacteria primarily through the AM pathway rather than directly through roots. These results suggest that AMF play a vital role in the translocation of new fixed plant C to soil microbes (Kaiser et al., 2015).
In mixed-species communities connected through CAMNs, plant species benefit differently depending on the AMF species involved and plant coexistence can be significantly affected by these differences (Wagg et al., 2011; van der Heijden et al., 2015). Differences in the natural abundance of 13C between plants of the C3 and C4 photosynthetic pathways were used in several studies of AMF-linked plants to quantify C transfer. In one study, C transfer of AMF-linked Plantago lanceolata (C3) and Cynodon dactylon (C4) was quantified. This varied from 0 to 41% with median at approximately 5%for individual C. dactylon plants but was not determined for P. lanceolata individuals (Watkins et al., 1996). Walder et al. (2012) found that C3 flax (Linum usitatissimum) contributed 30% of the CAMN carbon but gained up to 90% of N from the CAMNs formed by R. intraradices, which highly facilitated its growth, while the CAMN-interconnected neighboring C4 sorghum (Sorgum bicolor) contributed 70% of CAMN carbon with little N but was barely affected growth. One possible mechanism affecting these results could be the exchange of “luxury goods” between plants and AMF symbionts (Kiers and van der Heijden, 2006). Consequently, resource trading through networks of plant-AMF assemblages could be weakly reciprocal, depending on the sink strength and exchange efficiency at the symbiotic interfaces, which should differ with different plant-fungus combinations (Helgason et al., 2007). Walder et al. (2012) demonstrated that plants transferred underground resources to each other through such a mycelial network, so that nutrients could be quickly transported between different plants.
Recent observations show that mycorrhizal fungi are important regulators of C dynamics because of slow decomposition of fungal residues (van der Heijden et al., 2015) and that C storage is increased in AM-dominated ecosystems (Averill et al., 2014; van der Heijden et al., 2015; Wurzburger et al., 2017). To quantify the involvement of AMF in the intraspecific transport of C between plants, Graves et al. (1997) fumigated a mycorrhizal Festuca ovina turf with 13C-depleted CO2 for one week and found that 41% of the newly fixed C that was exported belowground was subsequently transported to neighbouring F. ovina (Table 1). Although Francis and Read (1984) showed that transfer of C between plants connected by AM mycelia occurred primarily by the direct hyphal pathway, levels of C in whole receiver plants (F. ovina) only reached to 0.058 ± 0.023% of that in donors (Plantago lanceolata). In contrast, by labeling plants with 14CO2 in the field, Lerat et al. (2002) reported that a direct 0.064 ± 0.049% transfer of 14C in the sugar maple (Acer saccharum) were from Erythronium americanum, while 14C was detected in 7 of 22 E. americanum roots from the sugar maple, with labeling in those 7 only 0.018 ± 0.021% that of sugar maple. Both the enrichment and natural abundance of 13C methods show one-way transfer of C between mycorrhizal plants to be 0 to 41%, in controlled or field conditions (He et al., 2003; He et al., 2009; Table 1). For instance, such C transfers have been detected between Allium cepa plants (Hirrel and Gerdemann, 1979), Festuca idahoensis and Centaurea maculosa (Carey et al., 2004), F. ovina and F. ovina (Graves et al., 1997), Lolium perenne and Plantago lanceolata (Martins, 1992), Oryza sativa and Citrullus lanatus (Carey et al., 2004), and Trifolium subterraneum and P. lanceolata (Nakano-Hylander and Olsson, 2007) (Table 1). Most recently, by labelling 13CO2 to one of the four tree species growing in “community boxes” using natural forest soil as fungal inoculums, 6.4 to 29.0% C transfers were facilitated by shared AMF of R. fasciculatus and R. irregularis, with oak (Quercus calliprinos) being a better donor, while pistacia (Pistacia lentiscus) and cypress (Cupressus sempervirens) better recipients (Avital et al., 2022). They concluded that an asymmetric C exchange between co-existing plant species could contribute to forest resilience. However, the mechanism of C transfer and role of mycorrhizal hyphae in the direct transfer of C are not well established (Robinson and Fitter, 1999; Smith and Read, 2008). Therefore, more needs to be done to lay out the arguments for why and how CAMN transfer of C could contribute to the accumulation of C in ecosystems.
Table 1 Transfer of C from one plant to another via CAMNs (see Section 1.4 for transfer calculations).
In contrast to C, AMF were previously thought to play no roles in organic N acquisition for their host plant (Read, 1991). In ecosystems where decomposition and nitrification processes are favored, although both poorly mobile ammonium (NH4+) and highly mobile nitrate (NO3−, most available in non-waterlogging habitats, compared to NH4+) are the principal plant-available N forms, the enhancement of plant N acquisition by AMF may be small (Read, 1991). However, studies have shown that AMF can acquire N from both inorganic and organic N sources and transfer some of this N to their host plant (Johnson et al., 2002; Leigh et al., 2008; Hodge and Fitter, 2010; Hodge and Storer, 2015; Jansa et al., 2019). Since N is a key limiting nutrient in terrestrial ecosystems, if AMF enhance inorganic, organic or unspecified N uptake, this could improve host plant fitness (Hodge and Storer, 2015). In 15N labelling studies, inorganic (Johansen et al., 1993; Tobar et al., 1994a; Tobar et al., 1994b; Hawkins et al., 2000) and organic (Hawkins et al., 2000) N uptake by host plants is positively correlated to AMF colonization rates. In a recent 15N natural abundance study, 30% of total N in maize was AMF-mediated when maize grew within 5 m of N2-fixing Faidherbia albida (Dierks et al., 2021). Generally, inorganic N absorbed by the fungal ERM could be incorporated into amino acids and then transported to the fungal IRM (Johansen et al., 1996).
In most natural and productivity ecosystems most nutrient inputs to soils are as organic materials. These materials vary widely in their physical and chemical complexity, nutrient quality and quantity, and source (Read and Perez-Moreno, 2003). Single 15N-labelled organic materials have been used to trace the flow of N in the soil-plant system, showing that plants, or their mycorrhizal symbionts, acquired N in intact glycine (Näsholm et al., 1998). Subsequently, Leigh et al. (2008) demonstrated that R. intraradices could increase the N concentration of its host plant, apparently by taking up N from a decomposing patch of organic matter. AMF can obtain substantial amounts of N from decomposing organic materials, thereby enhancing plant fitness (Hodge and Fitter, 2010). However, in the absence of other microbes, there is so far no experimental evidence for any quantitative acquisition of N by AMF hyphae from organic sources (Jansa et al., 2019). On the other hand, there is partly equivocal evidence from experiments using quantum dot technology indicating that organic N could be taken up via AMF hyphae and that uptake of N in the form of certain simple amino acids was enhanced in mycorrhizal compared with non-mycorrhizal plant roots (Whiteside et al., 2012). A surprising finding, revealed through feeding 13C-acetate or 15N-Arginine to the ERM, showed that N is transported from fungus to plant as NH4+, not amino acid (Fellbaum et al., 2012). A possible mechanism to explain these observations is that arginine delivered to the fungal IRM was broken down and the NH4+ was then released to the symbiotic interface and transferred from fungus to plant ((Parniske, 2008). The external hyphae of AM fungi can directly take up 15NH4+ or 15NO3–, reduce nitrate to NH4+, and then assimilate NH4+ into the pool of free amino acids. Understanding the link between CAMN transfer of nutrient and accumulation of C and N in ecosystems is crucial to clarify potential C-for-N trades between symbionts (Hodge and Storer, 2015; Thirkell et al., 2016). Indeed, interplant nutrient exchanges could play a vital ecological role in promoting plant coexistence in ecosystems under future climatic and anthropogenic pressures with profound relevance to restoration of plant communities (Figure 2).
Changes in plant δ15N values may reflect N inputs, N outputs and N isotope fractionation processes in ecosystems (Dawson et al., 2002). Use of natural 15N variability to investigate the role of mycorrhizae in N transfer is increasing (Dawson et al., 2002; He et al., 2003; Moyer-Henry et al., 2006; He et al., 2009). Earlier studies showed one-way AM-mediated N transfer from N2-fixing soybean (Glycine max) to non-N2-fixing maize (Zea mays) (van Kessel et al., 1985) and from N2-fixing Trifolium pratense to non-N2-fixing Lolium perenne (Haystead et al., 1988), indicating that such N transfers could be important for non-N2-fixing plants under N-limited conditions. Transfers of both NH4+ and NO3– between N2-fixing and non-N2-fixing plants were mediated by CMNs (Frey and Schüepp, 1993; Johansen et al., 1996; Moyer-Henry et al., 2006). Likewise, He et al. (2006) found that N moved quickly between AM and EM mycorrhizal plant individuals in a California oak woodland, indicating a CMN-mediated nutrient distribution mechanism between plants, including the release and recapture of N from the rhizosphere soil. By foliar 15NO3 labeling to quantify N transfer between non-N2-fixation plants (Eucalyptus marginata, ectomycorrhizal (EM) species; Melaleuca preissiana, AM/EM species; Verticordia nitens AM species), Teste et al. (2015) demonstrate that plants with cluster-roots (Banksia menziesii, non-mycorrhizal species) or ectomycorrhizal plants were more 15N enriched than with AM-only plants. Nitrogen transfer was relatively high (4% of the donor plant N) among these non-N2-fixation plants with contrasting N-acquisition strategies. Montesinos-Navarro et al. (2016) found that CAMNs mediated N transfer between facilitated plants, suggesting that nutrient transfer through CAMNs might be a potential mechanism allowing persistent benefits for their adult facilitated plants. The potential pathways for CAMNs-mediated N transfer could be (1) direct transfer of N via connecting hyphae across the symbiotic interface, (2) increased root surface area and a reduced distance for nutrient diffusion, and (3) increased assimilation or exudation of N in the AM-colonized plants (Haystead et al., 1988; Trannin et al., 2000; He et al., 2003; He et al., 2009).
Both 15N enrichment studies and natural abundance measurements showed a one-way movement of 0 to 80% of receiver N from N2-fixing mycorrhizal to non-N2-fixing mycorrhizal plants, under controlled or field conditions (He et al., 2003; He et al., 2009). For instance, one-way transfer through CAMNs of ~0.09‒80% of plant N was reported in a white clover (Trifolium repens) ‒ ryegrass (Lolium perenne) system with F. mosseae (Haystead et al., 1988), a berseem (Trifolium alexandrinum) ‒ maize (Zea mays) or pea (Pisum sativum) ‒ barley (Hordeum vulgare) system with R. intraradices (Frey and Schüepp, 1993; Johansen and Jensen, 1996), a soybean (Glycine max) ‒ semen cassiae (Senna obtusifolia) and a peanut (Arachis hypogaea) ‒ prickly sida (Sida spinosa) or sicklepod (Senna obtusifolia) (Moyer-Henry et al., 2006), a Cinnamomum camphora ‒ C. camphora system with Claroideoglomus etunicatum (He et al., 2019), a Vachellia seyal ‒ Sporobolus robustus system with R. irregularis (Table 2). In addition, N can also be transferred from non-N2-fixing mycorrhizal plants to N2-fixing mycorrhizal plants via a CAMN, although N transfer is generally less than 10% of plant N budgets (Johansen and Jensen, 1996; Li et al., 2009). For instance, the respective N transfer was 4.2% ‒ 9.9% of plant N budgets from the donor mung bean (Vigna radiata) to its associated receiver rice (Oryza sativa) or from the rice (O. sativa) to mung bean (Li et al., 2009). AM-mediated N transfer can be from N2-fixing to non-N2-fixing plants or from non-N2-fixing to N2-fixing plants, indicating bi-directional transfer. Recently, 15N labeling demonstrated that CMNs increased 15N enrichment of Trifolium pratense, but did not affect its biomass production, when the holoparasite Cuscuta australis was absent (Yuan YG. et al., 2021). In contrast, both 15N enrichment and biomass production in T. pratense plants were increased by CAMNs when the holoparasite was present. These results indicated that CAMNs could preferentially distribute more N to a non-parasitized neighboring T. pratense, while resulting in negative feedback on the growth of the parasite C. australis (Yuan YG. et al., 2021).
Table 2 Transfer of N from one plant to another via CAMNs (see Section 1.4 for transfer calculations).
A range of 0.02 to 41% (C) and 0.04 to 80% (N) of one-way transfer have been observed from donor to recipient plants through the determination of 13C and 15N signatures (Tables 1, 2). Interplant C and N transfers can affect not only the growth and competition between donor and recipient plants but also ecosystem stability. For example, Weremijewicz et al. (2016) observed that Andropogon gerardii plants in intact CMNs under sunlight acquired 9% of their N, but shaded plants (~35% photosynthetically active radiation) acquired only 1% N, from their conspecific neighbors. They suggested that AM fungi in CAMNs preferentially provide N to conspecific hosts of with fixed C or presenting the strongest sinks, thus potentially expanding asymmetric underground competition. Castro-Delgado et al. (2020) showed that the mycelium could transfer diverse compounds and signals among plants that would modify plant behavior in favor of protection of the whole network. In general, stable isotope tracing has provided an effective way to study the exchange of mineral nutrients between plants through CAMNs. Although 13C and 15N labeling techniques have demonstrated that CAMNs are an important pathway for the translocation of both C and N, the functioning of CAMNs in ecosystem C and N dynamics remains equivocal. To make an explicit link between nutrient transfer in CAMNs and nutrient cycling in ecosystems new approaches are needed. For example, a combination of high-throughput genome sequence techniques with model-based assessments could further identify the extent of CAMNs in interplant C and N translocation in natural and managed ecosystems (Orwin et al., 2011; Zhou et al., 2021). The following issues about the physiological and ecological functions of AMF or CAMNs should be addressed.
1. Can 13C and 15N natural abundance, like 13C and 15N external labeling, be employed to detect C and N transfer? Study have shown that plant δ13C signatures could reflect the δ13C of the C sources of associated fungi and δ15N signatures could reflect the δ15N of N sources to plants (Querejeta et al., 2003). However, the reliability of using 15N natural abundance to estimate AMF-mediated N transfer has been recently questioned (Choi et al., 2020; Jach-Smith and Jackson, 2020).
2. In what form are C and N transferred through CAMNs? Amino acids, lipids, or carbohydrates for C, amino acids or ammonium for N? Does a pollen development encompass a mechanism that is shared with CAMNs symbiosis? What may the two phenomena have in common (Nouri and Reinhardt, 2015)? Can fluorescent nanoscale semiconductors or quantum dots (Whiteside et al., 2012) be combined with 13C and 15N labelling to trace the transfer of organic nutrients through CAMNs (Govindarajulu et al., 2005; Parniske, 2008)?
3. Can network theory and computer modeling (Southworth et al., 2005; Wipf et al., 2019) simulate the direction and distribution of interplant C and N transfer facilitated by CMNs and thus predict both positive and negative effects of CMNs in natural and managed systems (Alaux et al., 2021)?
4. 15N labeling showed that AMF could not directly decompose organic matter, but the interaction between AMF and other decomposers enhanced organic matter decomposition and hence the absorption of N by AMF (Hodge and Fitter, 2010). However, how can CAMNs regulate the process of C and N translocation and absorption between AMF mycelia and host plants? In addition, a coupled concurrent C and N movement through CAMNs has not been reported.
5. What determines the net effect of CAMN-mediated interplant nutrient transfer on plant C assimilation and N metabolism? Does the transferred C and N affect the performance or fitness of the donor, receiver, or both? What is the ecological significance of CAMN mediated nutrient transfers in natural and managed ecosystems? Whether AMF-mediated interplant C and N transferred is agronomically important to managed ecosystems, including agroforestry, forestry, croplands, and grasslands, is debated (Rillig et al., 2019; Ryan et al., 2019). How do modern agricultural practices, such as long-term organic farming, no-till, or fertigation affect the establishment and performance of CMNs and subsequent effects on fertilizer use efficiency, crop agronomic characters and productivity?
6. How the abundance and function of soil bacterial and other fungal communities could be manipulated and promoted through a CAMN-mediated interplant C and N transfers (Bonfante and Anca, 2009; Yuan MTM. et al., 2021)? Is plant C investment in AM fungal growth related to soil N acquisition within a CAMN? How is the N for C trade between mycorrhizal symbionts regulated if plants are linked through a CAMN? What determines the magnitude and direction of such C and N transfer within the same or different plant species in mono-species or mixed-species systems, particularly along their complete plant growth and development cycle? How exogenous and endogenous factors can interplay with CAMNs, and how a nutrient can impinge on AM symbiotic signaling and also on a later cellular program in host plants (Nouri et al., 2014).
7. Irrespective of photosynthetic capabilities or N2-fixation characteristics of plant species, what the phylogenetic and functional diversity of plant species can benefit from nutrient transfer through CAMNs? These species would be in a diverse range as C3, C4, C3-C4, CAM and parasitic plants. Are there interactions between AM and EM networks on C and N transfers since some plants do have dual AM/EM associations (Wang and Qiu, 2006; Teste et al., 2015)? How can technical problems be overcome in demonstrating unequivocally that a C or N transfer directly occurs through CMNs rather than indirectly through root exudates or soils (Zhang et al., 2019; Fall et al., 2022; Reay et al., 2022)?
8. How will drivers of global environment change including elevated CO2 concentration, N deposition, drought and temperature affect interplant C and N transfer through CAMNs? Each can have substantial impacts on the direction and magnitude of such C and N transfers and ultimately on resource sharing or competition (Fellbaum et al., 2014; Řezáčová et al., 2018; Mickan et al., 2021). To answer these issues, it is important to keep in mind that mycorrhizal symbiotic benefits are interactively formed between plants and fungi under specific habitats and soil properties.
Conceptualization, XH. Methodology, XH and SL. Data analysis, XL and YL. Original draft, XL and XH. Review and editing, XH, XL and SL. Funding acquisition, XH and XL. All authors contributed to the article and approved the submitted version.
This work received financial support from the Key Laboratory of Eco-environments in Three Gorges Reservoir Region (Ministry of Education), Chongqing Key Laboratory of Plant Resource Conservation and Germplasm Innovation, National Base of International S&T Collaboration on Water Environmental Monitoring and Simulation in the Three Gorges Reservoir Region and Centre of Excellence for Soil Biology at Southwest University to XH, and the Scientific Research Foundation of Wuhan Institute of Technology to XL (22QD65). Researches in the authors’ laboratory are jointly supported by projects from the Key Laboratory of Eco-environments in Three Gorges Reservoir Region (Ministry of Education), Chongqing Key Laboratory of Plant Resource Conservation and Germplasm Innovation, National Base of International S&T Collaboration on Water Environmental Monitoring and Simulation in the Three Gorges Reservoir Region and Centre of Excellence for Soil Biology at Southwest University to XH, and the Scientific Research Foundation of Wuhan Institute of Technology to XL (22QD65).
For valuable comments on the manuscript before submission, we thank Dr. Julie Deslippe at Victoria University of Wellington, New Zealand and Dr. Erik Hobbie at University of New Hampshire University, USA.
The authors declare that the research was conducted in the absence of any commercial or financial relationships that could be construed as a potential conflict of interest.
All claims expressed in this article are solely those of the authors and do not necessarily represent those of their affiliated organizations, or those of the publisher, the editors and the reviewers. Any product that may be evaluated in this article, or claim that may be made by its manufacturer, is not guaranteed or endorsed by the publisher.
Alaux, P. L., Zhang, Y. Q., Gilbert, L., Johnson, D. (2021). Can common mycorrhizal fungal networks be managed to enhance ecosystem functionality? Plants People Planet 3, 433‒444. doi: 10.1002/ppp3.10178
Averill, C., Turner, B. L., Finzi, A. C. (2014). Mycorrhiza-mediated competition between plants and decomposers drives soil carbon storage. Nature 505, 543‒545. doi: 10.1038/nature12901
Avital, S., Rog, I., Livne-Luzon, S., Cahanovitc, R., Klein, T. (2022). Asymmetric belowground carbon transfer in a diverse tree community. Mol. Ecol. 31, 3481‒95. doi: 10.1111/mec.16477
Awaydul, A., Zhu, W. Y., Yuan, Y. G., Xiao, J., Hu, H., Chen, X., et al. (2019). Common mycorrhizal networks influence the distribution of mineral nutrients between an invasive plant, Solidago canadensis, and a native plant, Kummerowa striata. Mycorrhiza 29, 29–38. doi: 10.1007/s00572-018-0873-5
Bago, B., Pfeffer, P. E., Shachar-Hill, Y. (2000). Carbon metabolism and transport in arbuscular mycorrhizas. Plant Physiol. 124, 949–957. doi: 10.1104/pp.124.3.949
Bever, J. D., Dickie, I. A., Facelli, E., Facelli, J. M., Klironomos, J., Moora, M., et al. (2010). Rooting theories of plant community ecology in microbial interactions. Trends Ecol. Evol. 25, 468–478. doi: 10.1016/j.tree.2010.05.004
Bonfante, P., Anca, I. A. (2009). Plants, mycorrhizal fungi, and bacteria: a network of interactions. Annu. Rev. Microbiol. 63, 363–383. doi: 10.1146/annurev.micro.091208.073504
Brundrett, M. C. (2009). Mycorrhizal associations and other means of nutrition of vascular plants: understanding the global diversity of host plants by resolving conflicting information and developing reliable means of diagnosis. Plant Soil 320, 37‒77. doi: 10.1007/s11104-008-9877-9
Brundrett, M. C. (2017). “Global diversity and importance of mycorrhizal and nonmycorrhizal plants,” in Biogeography of mycorrhizal symbiosis. Ed. Tedersoo, L. (Switzerland: Springer, Cham), 533‒556. doi: 10.1007/978-3-319-56363-3_21
Brundrett, M. C., Tedersoo, L. (2018). Evolutionary history of mycorrhizal symbioses and global host plant diversity. New Phytol. 220, 1108–1115. doi: 10.1111/nph.14976
Cardini, A., Pellegrino, E., Declerck, S., Calonne-Salmon, M., Mazzolai, B., Ercoli, L. (2021). Direct transfer of zinc between plants is channelled by common mycorrhizal network of arbuscular mycorrhizal fungi and evidenced by changes in expression of zinc transporter genes in fungus and plant. Environ. Microbiol. 23, 5883–5900. doi: 10.1111/1462-2920.15542
Carey, E. V., Marler, M. J., Callaway, R. M. (2004). Mycorrhizae transfer carbon from a native grass to an invasive weed: evidence from stable isotopes and physiology. Plant Ecol. 172, 133‒141. doi: 10.1023/B:VEGE.0000026031.14086.f1
Castro-Delgado, A. L., Elizondo-Mesen, S., Valladares-Cruz, Y., Rivera-Mendez, W. (2020). Wood wide web: communication through the mycorrhizal network. Tecnol Marcha 33, 114‒125. doi: 10.18845/tm.v33i4.4601
Chen, X., Li, Q., Wang, L. T., Meng, Y. L., Jiao, S. N., Yin, J. L., et al. (2021). Nitrogen uptake, not transfer of carbon and nitrogen by CMN, explains the effect of AMF on the competitive interactions between Flaveria bidentis and native species. Front. Ecol. Evol. 9. doi: 10.3389/fevo.2021.625519
Cheng, X. M., Baumgartner, K. (2004). Arbuscular mycorrhizal fungi-mediated nitrogen transfer from vineyard cover crops to grapevines. Biol. Fertil Soils 40, 406–412. doi: 10.1007/s00374-004-0797-4
Choi, W. J., Chang, S. X., Jin-Hyeob Kwak, J. H. (2020). Comment on inorganic n addition replaces n supplied to switchgrass (Panicum virgatum) by arbuscular mycorrhizal fungi. Ecol. Appl. 31, e2270. doi: 10.1002/eap.2270
Couradeau, E., Sasse, J., Goudeau, D., Nath, N., Hazen, T. C., Bowen, B. P., et al. (2019). Probing the active fraction of soil microbiomes using BONCAT-FACS. Nat. Commun. 10, 2770. doi: 10.1038/s41467-019-10542-0
Dawson, T. E., Mambelli, S., Plamboeck, A. H., Templer, P. H., Tu, K. P. (2002). Stable isotopes in plant ecology. Annu. Rev. Ecol. Syst. 33, 507–559. doi: 10.1146/annurev.ecolsys.33.020602.095451
Dierks, J., Blaser-Hart, W. J., Gamper, H. A., Six, J. (2021). Mycorrhizal fungi-mediated uptake of tree-derived nitrogen by maize in smallholder farms. Nat. Sustain 5, 64–70. doi: 10.1038/s41893-021-00791-7
Ding, C. H., Zhao, Y., Zhang, Q. R., Lin, Y. B., Xue, R. R., Chen, C. Y., et al. (2022). Cadmium transfer between maize and soybean plants via common mycorrhizal networks. Ecotox Environ. Safe 232, 113273. doi: 10.1016/j.ecoenv.2022.113273
Egerton-Warburton, L. M., Querejeta, J. I., Allen, M. F. (2007). Common mycorrhizal networks provide a potential pathway for the transfer of hydraulically lifted water between plants. J. Exp. Bot. 58, 3484–3484. doi: 10.1093/jxb/erm009
Eissenstat, D. M. A. (1990). A comparison of phosphorus and nitrogen transfer between plants of different phosphorus status. Oecologia 82, 342–347. doi: 10.1007/BF00317481
Fall, F., Ndoye, D., Galiana, A., Diouf, D., Ba, A. M. (2022). Arbuscular mycorrhizal fungi-mediated biologically fixed n transfer from Vachellia seyal to Sporobolus robustus. Symbiosis 86, 205–214. doi: 10.1007/s13199-022-00833-4
Fang, L. F., He, X. H., Zhang, X. L., Yang, Y. H., Liu, R., Shi, S. M., et al. (2021). A small amount of nitrogen transfer from legume cover crop to citrus seedling via common arbuscular mycorrhizal networks. Agronomy 11, 32. doi: 10.3390/agronomy11010032
Fellbaum, C. R., Gachomo, E. W., Beesetty, Y., Choudhari, S., Strahan, G. D., Pfeffer, P. E., et al. (2012). Carbon availability triggers fungal nitrogen uptake and transport in arbuscular mycorrhizal symbiosis. P Natl. Acad. Sci. U.S.A. 109, 2666–2671. doi: 10.1073/pnas.1118650109
Fellbaum, C. R., Mensah, J. A., Cloos, A. J., Strahan, G. D., Pfeffer, P. E., Kiers, E. T., et al. (2014). Fungal nutrient allocation in common mycorrhizal networks is regulated by the carbon source strength of individual host plants. New Phytol. 203, 646–656. doi: 10.1111/nph.12827
Fitter, A. H., Graves, J. D., Watkins, N. K., Robinson, D., Scrimgeour, C. (1998). Carbon transfer between plants and its control in networks of arbuscular mycorrhizas. Funct. Ecol. 12, 406–412. doi: 10.1046/j.1365-2435.1998.00206.x
Francis, R., Read, D. J. (1984). Direct transfer of carbon between plants connected by vesicular arbuscular mycorrhizal mycelium. Nature 307, 53–56. doi: 10.1038/307053a0
Frey, B., Schüepp, H. (1993). A role of vesicular-arbuscular (VA) mycorrhizal fungi in facilitating interplant nitrogen transfer. Soil Biol. Biochem. 25, 651–658. doi: 10.1016/0038-0717(93)90104-J
Gao, D. M., Pan, X. J., Rahman, M. K. U., Zhou, X. G., Wu, F. Z. (2021). Common mycorrhizal networks benefit to the asymmetric interspecific facilitation via K exchange in an agricultural intercropping system. Biol. Fertil. Soils 57, 959–971. doi: 10.1007/s00374-021-01561-5
Giller, K. E., Ormesher, J., Awah, F. M. (1991). Nitrogen transfer from Phaseolus bean to intercropped maize measured using 15N-enrichment and 15N-isotope dilution methods. Soil Biol. Biochem. 23, 339–346. doi: 10.1016/0038-0717(91)90189-Q
Giovannetti, M., Sbrana, C., Avio, L., Strani, P. (2004). Patterns of below-ground plant interconnections established by means of arbuscular mycorrhizal networks. New Phytol. 164, 175–181. doi: 10.1111/j.1469-8137.2004.01145.x
Govindarajulu, M., Pfeffer, P., Jin, H., Abubaker, J., Douds, D. D., Allen, J. W., et al. (2005). Nitrogen transfer in the arbuscular mycorrhizal symbiosis. Nature 435, 819–823. doi: 10.1038/nature03610
Graves, J. D., Watkins, N. K., Fitter, A. H., Robinson, D., Scrimgeour, C. (1997). Intraspecific transfer of carbon between plants linked by a common mycorrhizal network. Plant Soil 192, 153‒159. doi: 10.1023/A:1004257812555
Grime, J. P., Mackey, J. M. L., Hillier, S. H., Read, D. J. (1987). Floristic diversity in a model system using experimental microcosms. Nature 328, 420–422. doi: 10.1038/328420a0
Gyuricza, V., Thiry, Y., Wannijn, J., Declerck, S., de Boulois, H. D. (2010). Radiocesium transfer between Medicago truncatula plants via a common mycorrhizal network. Environ. Microbiol. 12, 2180–2189. doi: 10.1111/j.1462-2920.2009.02118.x
Hamel, C., Barrantes-Cartin, U., Furlan, V., Smith, D. L. (1991a). Endomycorrhizal fungi in nitrogen transfer from soybean to maize. Plant Soil 138, 33–40. doi: 10.1007/BF00011805
Hamel, C., Nesser, C., Barrantes-Cartin, U., Smith, D. L. (1991b). Endomycorrhizal fungal species mediate 15N transfer from soybean to maize in non-fumigated soil. Plant Soil 138, 41–47. doi: 10.1007/BF00011806
Hawkins, H. J., Johansen, A., George, E. (2000). Uptake and transport of organic and inorganic nitrogen by arbuscular mycorrhizal fungi. Plant Soil 226, 275–285. doi: 10.1023/A:1026500810385
Haystead, A., Malajczuk, N., Grove, T. S. (1988). Underground transfer of nitrogen between pasture plants infected with vesicular arbuscular mycorrhizal fungi. New Phytol. 108, 417–423. doi: 10.1111/j.1469-8137.1988.tb04182.x
He, X. H. (2002). Nitrogen exchange between plants through common mycorrhizal networks (Brisbane, Australia: The University of Queensland).
He, X. H., Bledsoe, C. S., Zasoski, R. J., Southworth, D., Horwath, W. R. (2006). Rapid nitrogen transfer from ectomycorrhizal pines to adjacent ectomycorrhizal and arbuscular mycorrhizal plants in a California oak woodland. New Phytol. 170, 143–151. doi: 10.1111/j.1469-8137.2006.01648.x
He, Y. J., Cornelissen, J. H. C., Wang, P. P., Dong, M., Ou, J. (2019). Nitrogen transfer from one plant to another depends on plant biomass production between conspecific and heterospecific species via a common arbuscular mycorrhizal network. Environ. Sci. pollut. R 26, 8828–8837. doi: 10.1007/s11356-019-04385-x
He, X. H., Critchley, C., Bledsoe, C. (2003). Nitrogen transfer within and between plants through common mycorrhizal networks (CMNs). Crit. Rev. Plant Sci. 22, 531–567. doi: 10.1080/713608315
He, X. H., Xu, M. G., Qiu, G. Y., Zhou, J. B. (2009). Use of 15N stable isotope to quantify nitrogen transfer between mycorrhizal plants. J. Plant Ecol. 2, 107–118. doi: 10.1093/jpe/rtp015
Helgason, T., Merryweather, J. W., Young, J. P. W., Fitter, A. H. (2007). Specificity and resilience in the arbuscular mycorrhizal fungi of a natural woodland community. J. Ecol. 95, 623‒630. doi: 10.1111/j.1365-2745.2007.01239.x
Hirrel, M. A., Gerdemann, J. W. (1979). Enhanced carbon transfer between onions infected with a vesicular-arbuscular mycorrhizal fungus. New Phytol. 83, 731–738. doi: 10.1111/j.1469-8137.1979.tb02303.x
Hodge, A., Fitter, A. H. (2010). Substantial nitrogen acquisition by arbuscular mycorrhizal fungi from organic material has implications for n cycling. P Natl. Acad. Sci. U.S.A. 107, 13754–13759. doi: 10.1073/pnas.1005874107
Hodge, A., Storer, K. (2015). Arbuscular mycorrhiza and nitrogen: implications for individual plants through to ecosystems. Plant Soil 386, 1–19. doi: 10.1007/s11104-014-2162-1
Hupe, A., Naether, F., Haase, T., Bruns, C., Hess, J., Dyckmans, J., et al. (2021). Evidence of considerable c and n transfer from peas to cereals via direct root contact but not via mycorrhiza. Sci. Rep. 11, 11424. doi: 10.1038/s41598-021-90436-8
Ikram, A., Jensen, E. S., Jakobsen, I. (1994). No significant transfer of n and p from Pueraria phaseoloides to Hevea brasiliensis via hyphal links of arbuscular mycorrhiza. Soil Biol. Biochem. 26, 1541–1547. doi: 10.1016/0038-0717(94)90096-5
Ingraffia, R., Amato, G., Frenda, A. S., Giambalvo, D. (2019). Impacts of arbuscular mycorrhizal fungi on nutrient uptake, N2 fixation, n transfer, and growth in a wheat/faba bean intercropping system. PloS One 14, e0213672. doi: 10.1371/journal.pone.0213672
Ingraffia, R., Giambalvo, D., Frenda, A. S., Roma, E., Ruisi, P., Amato, G. (2021). Mycorrhizae differentially influence the transfer of nitrogen among associated plants and their competitive relationships. Appl. Soil Ecol. 168, 104127. doi: 10.1016/j.apsoil.2021.104127
Jach-Smith, L. C., Jackson, R. D. (2020). Inorganic n addition replaces n supplied to switchgrass (Panicum virgatum) by arbuscular mycorrhizal fungi. Ecol. Appl. 30, e2047. doi: 10.1002/eap.2047
Jalonen, R., Nyqren, P., Sierra, J. (2009). Transfer of nitrogen from a tropical legume tree to an associated fodder grass via root exudation and common mycelial networks. Plant Cell Environ. 32, 1366–1376. doi: 10.1111/j.1365-3040.2009.02004.x
Jansa, J., Forczek, S. T., Rozmos, M., Puschel, D., Bukovska, P., Hrselova, H. (2019). Arbuscular mycorrhiza and soil organic nitrogen: network of players and interactions. Chem. Biol. Technol. Agric. 6, 10. doi: 10.1186/s40538-019-0147-2
Jiang, Y. N., Wang, W. X., Xie, Q. J., Liu, N., Liu, L. X., Wang, D. P., et al. (2017). Plants transfer lipids to sustain colonization by mutualistic mycorrhizal and parasitic fungi. Science 356, 1172‒1175. doi: 10.1126/science.aam9970
Johansen, A., Finlay, R. D., Olsson, P. A. (1996). Nitrogen metabolism of external hyphae of the arbuscular mycorrhizal fungus Glomus intraradices. New Phytol. 133, 705–712. doi: 10.1111/j.1469-8137.1996.tb01939.x
Johansen, A., Jakobsen, I., Jensen, E. S. (1993). External hyphae of vesicular-arbuscular mycorrhizal fungi associated with trifolium subterraneum l. III. hyphal transport of 32P and 15N. New Phytol. 124, 61–68. doi: 10.1111/j.1469-8137.1993.tb03797.x
Johansen, A., Jensen, E. S. (1996). Transfer of n and p from intact or decomposing roots of pea to barley interconnected by an arbuscular mycorrhizal fungus. Soil Biol. Biochem. 28, 73–81. doi: 10.1016/0038-0717(95)00117-4
Johnson, D., Leake, J. R., Ostle, N., Ineson, P., Read, D. J. (2002). In situ (CO2)-C-13 pulse-labelling of upland grassland demonstrates a rapid pathway of carbon flux from arbuscular mycorrhizal mycelia to the soil. New Phytol. 153, 327–334. doi: 10.1046/j.0028-646X.2001.00316.x
Kaiser, C., Kilburn, M. R., Clode, P. L., Fuchslueger, L., Koranda, M., Cliff, J. B., et al. (2015). Exploring the transfer of recent plant photosynthates to soil microbes: mycorrhizal pathway vs direct root exudation. New Phytol. 205, 1537‒1551. doi: 10.1111/nph.13138
Keymer, A., Pimprikar, P., Wewer, V., Huber, C., Brands, M., Bucerius, S., et al. (2017). Lipid transfer from plants to arbuscular mycorrhiza fungi. eLife 6, e29107. doi: 10.7554/eLife.29107
Kiers, E. T., Duhamel, M., Beesetty, Y., Mensah, J. A., Franken, O., Verbruggen, E., et al. (2011). Reciprocal rewards stabilize cooperation in the mycorrhizal symbiosis. Science 333, 880–882. doi: 10.1126/science.1208473
Kiers, E. T., van der Heijden, M. G. A. (2006). Mutualistic stability in the arbuscular mycorrhizal symbiosis: exploring hypotheses of evolutionary cooperation. Ecology 87, 1627‒1636. doi: 10.1890/0012-9658(2006)87[1627:msitam]2.0.co;2
Konvalinková, T., Jansa, J. (2016). Lights off for arbuscular mycorrhiza: on its symbiotic functioning under light deprivation. Front. Plant Sci. 7. doi: 10.3389/fpls.2016.00782
Kurppa, M., Leblanc, H. A., Nygren, P. (2010). Detection of nitrogen transfer from N2-fixing shade trees to cacao saplings in 15N labelled soil: ecological and experimental considerations. Agrofor Syst. 80, 223–239. doi: 10.1007/s10457-010-9327-6
Leigh, J., Hodge, A., Fitter, A. H. (2008). Arbuscular mycorrhizal fungi can transfer substantial amounts of nitrogen to their host plant from organic material. New Phytol. 181, 199–207. doi: 10.1111/j.1469-8137.2008.02630.x
Lekberg, Y., Hammer, E. C., Olsson, P. A. (2010). Plants as resource islands and storage units–adopting the mycocentric view of arbuscular mycorrhizal networks. FEMS Microbiol. Ecol. 74, 336–345. doi: 10.1111/j.1574-6941.2010.00956.x
Lekberg, Y., Rosendahl, S., Michelsen, A., Olsson, P. A. (2013). Seasonal carbon allocation to arbuscular mycorrhizal fungi assessed by microscopic examination, stable isotope probing and fatty acid analysis. Plant Soil 368, 547–555. doi: 10.1007/s11104-012-1534-7
Lerat, S., Gauci, R., Catford, J. G., Vierheilig, H., Piche, Y., Lapointe, L. (2002). 14C transfer between the spring ephemeral Erythronium americanum and sugar maple saplings via arbuscular mycorrhizal fungi in natural stands. Oecologia 132, 181–187. doi: 10.1007/s00442-002-0958-9
Li, X. L., Marschner, H., George, E. (1991). Acquisition of phosphorus and copper by VA mycorrhizal hyphae and root-to-shoot transport in white clover. Plant Soil 136, 49–57. doi: 10.1007/BF02465219
Li, Y. F., Ran, W., Zhang, R. P., Sun, S. B., Xu, G. H. (2009). Facilitated legume nodulation, phosphate uptake and nitrogen transfer by arbuscular inoculation in an upland rice and mung bean intercropping system. Plant Soil 315, 285–296. doi: 10.1007/s11104-008-9751-9
Luginbuehl, L. H., Menard, G. N., Kurup, S., van Erp, H., Radhakrishnan, G. V., Breakspear, A., et al. (2017). Fatty acids in arbuscular mycorrhizal fungi are synthesized by the host plant. Science 356, 1175–1178. doi: 10.1126/science.aan0081
Malcova, R., Albrechtova, J., Vosatka, M. (2001). The role of the extraradical mycelium network of arbuscular mycorrhizal fungi on the establishment and growth of Calamagrostis epigejos in industrial waste substrates. Appl. Soil Ecol. 18, 129‒142. doi: 10.1016/S0929-1393(01)00156-1
Martins, M. A. (1992). The role of the external mycelial network of vesicular-arbuscular mycorrhizal fungi: a study of carbon transfer between plants interconnected by a common mycelium. Mycorrhiza 2, 69–73. doi: 10.1007/BF00203252
Martins, M. A. (1993). The role of the external mycelium of vesicular-arbuscular mycorrhizal fungi in the carbon transfer process between plants. Mycol Res. 97, 807–810. doi: 10.1016/S0953-7562(09)81155-6
Martins, M. A., Cruz, A. F. (1998). The role of the external mycelial network of arbuscular mycorrhizal fungi: III. a study of nitrogen transfer between plants interconnected by a common mycelium. Rev. Microbiol. 29, 289‒294. doi: 10.1590/S0001-37141998000400011
Meding, S. M., Zasoski, R. J. (2008). Hyphal-mediated transfer of nitrate, arsenic, cesium, rubidium, and strontium between arbuscular mycorrhizal forbs and grasses from a California oak woodland. Soil Biol. Biochem. 40, 126‒134. doi: 10.1016/j.soilbio.2007.07.019
Meng, L. B., Zhang, A. Y., Wang, F., Han, X. G., Wang, D. J., Li, S. M. (2015). Arbuscular mycorrhizal fungi and rhizobium facilitate nitrogen uptake and transfer in soybean/maize intercropping system. Front. Plant Sci. 6. doi: 10.3389/fpls.2015.00339
Merrild, M. P., Ambus, P., Rosendahl, S., Jakobsen, I. (2013). Common arbuscular mycorrhizal networks amplify competition for phosphorus between seedlings and established plants. New Phytol. 200, 229–240. doi: 10.1111/nph.12351
Mickan, B. S., Hart, M., Solaiman, Z. M., Renton, M., Siddique, K. H. M., Jenkins, S. N., et al. (2021). Arbuscular mycorrhizal fungus-mediated interspecific nutritional competition of a pasture legume and grass under drought-stress. Rhizosphere 18, 100349. doi: 10.1016/j.rhisph.2021.100349
Mikkelsen, B. L., Rosendahl, S., Jakobsen, I. (2008). Underground resource allocation between individual networks of mycorrhizal fungi. New Phytol. 180, 890–898. doi: 10.1111/j.1469-8137.2008.02623.x
Molina, R., Horton, T. (2015). “Mycorrhiza specificity: its role in the development and function of common mycelial networks,” in Mycorrhizal networks. Ed. Horton, T. (Dordrecht, Netherlands: Springer), 1‒39. doi: 10.1007/978-94-017-7395-9_1
Montesinos-Navarro, A., Verdu, M., Querejetac, J. I., Sortibrán, L., Valiente-Banuet, A. (2016). Soil fungi promote nitrogen transfer among plants involved in long-lasting facilitative interactions. Perspect. Plant Ecol. 18, 45–51. doi: 10.1016/j.ppees.2016.01.004
Moyer-Henry, K. A., Burton, J. W., Israel, D. W., Rufty, T. W. (2006). Nitrogen transfer between plants: a 15N natural abundance study with crop and weed species. Plant Soil 282, 7–20. doi: 10.1007/s11104-005-3081-y
Muneer, M. A., Chen, X. H., Munir, M. Z., Nisa, Z. U., Saddique, M. A. B., Mehmood, S., et al. (2022). Interplant transfer of nitrogen between C3 and C4 plants through common mycorrhizal networks under different nitrogen availability. J. Plant Ecol. 16, rtac058. doi: 10.1093/jpe/rtac058
Muneer, M. A., Wang, P., Zaib-un-Nisa, CC, Li, Ji, B. M. (2020a). Potential role of common mycorrhizal networks in improving plant growth and soil physicochemical properties under varying nitrogen levels in a grassland ecosystem. Glob Ecol. Conserv. 24, e01352. doi: 10.1016/j.gecco.2020.e01352
Muneer, M. A., Wang, P., Zhang, J., Li, Y. M., Munir, M. Z., Ji, B. M. (2020b). Formation of common mycorrhizal networks significantly affects plant biomass and soil properties of the neighboring plants under various nitrogen levels. Microorganisms 8, 230. doi: 10.3390/microorganisms8020230
Nakano-Hylander, A., Olsson, P. A. (2007). Carbon allocation in mycelia of arbuscular mycorrhizal fungi during colonisation of plant seedlings. Soil Biol. Biochem. 39, 1450–1458. doi: 10.1016/j.soilbio.2006.12.031
Näsholm, T., Ekblad, A., Nordin, A., Giesler, R., Högberg, M., Högberg, P. (1998). Boreal Forest plants take up organic nitrogen. Nature 392, 914–916. doi: 10.1038/31921
Newman, E. I., Devoy, A. L. N., Basen, N. J., Fowles, K. J. (1994). Plant species that can be linked by VA mycorrhizal fungi. New Phytol. 126, 691–693. doi: 10.1111/j.1469-8137.1994.tb02963.x
Newman, E. I., Eason, W. R., Eissenstat, D. M., Ramos, M. I. R. F. (1992). Interaction between plants: the role of mycorrhizae. Mycorrhiza 1, 47–53. doi: 10.1007/BF00206135
Nouri, E., Breuillin-Sessoms, F., Feller, U., Reinhardt, D. (2014). Phosphorus and nitrogen regulate arbuscular mycorrhizal symbiosis in Petunia hybrida. PloS One 9, e90841. doi: 10.1371/journal.pone.0090841
Nouri, E., Reinhardt, D. (2015). Flowers and mycorrhizal roots–closer than we think? Trends Plant Sci. 20, 344–350. doi: 10.1016/j.tplants.2015.03.012
Orwin, K. H., Kirschbaum, M. U., St John, M. G., Dickie, I. A. (2011). Organic nutrient uptake by mycorrhizal fungi enhances ecosystem carbon storage: a model-based assessment. Ecol. Lett. 14, 493‒502. doi: 10.1111/j.1461-0248.2011.01611.x
Parniske, M. (2008). Arbuscular mycorrhiza: the mother of plant root endosymbiosis. Nat. Rev. Microbiol. 6, 763‒775. doi: 10.1038/nrmicro1987
Pearson, J. N., Jakobsen, I. (1993). Symbiotic exchange of carbon and phosphorus between cucumber and three arbuscular mycorrhizal fungi. New Phytol. 124, 481–488. doi: 10.1111/j.1469-8137.1993.tb03839.x
Pfeffer, P. E., Douds, D. D., Becard, G., Shachar-Hill, Y. (1999). Carbon uptake and the metabolism and transport of lipids in an arbuscular mycorrhiza. Plant Physiol. 120, 587‒598. doi: 10.1104/pp.120.2.587
Pfeffer, P. E., Douds, D. D., Bücking, H., Schwartz, D. P., Shachar-Hill, Y. (2004). The fungus does not transfer carbon to or between roots in an arbuscular mycorrhizal symbiosis. New Phytol. 163, 617–627. doi: 10.1111/j.1469-8137.2004.01152.x
Querejeta, J. I., Barea, J. M., Allen, M. F., Caravaca, F., Roldan, A. (2003). Differential response of δ13C and water use efficiency to arbuscular mycorrhizal infection in two aridland woody plant species. Oecologia 135, 510–515. doi: 10.1007/s00442-003-1209-4
Rains, K. C., Bledsoe, C. S. (2007). Rapid uptake of 15N-ammonium and glycine 13C, 15N by arbuscular and ericoid mycorrhizal plants native to a Northern California coastal pygmy forest. Soil. Biol. Biochem. 39, 1078–1086. doi: 10.1016/j.soilbio.2006.11.019
Read, D. J., Perez-Moreno, J. (2003). Mycorrhizas and nutrient cycling in ecosystems - a journey towards relevance? New Phytol. 157, 475–492. doi: 10.1046/j.1469-8137.2003.00704.x
Reay, M. K., Pears, K. A., Kuhl, A., Evershed, R. P., Murray, P. J., Cardenas, L. M., et al. (2022). Mechanisms of nitrogen transfer in a model clover-ryegrass pasture: a 15N-tracer approach. Plant Soil 480, 369–389. doi: 10.1007/s11104-022-05585-0
Ren, L. X., Lou, Y. S., Zhang, N., Zhu, X. D., Hao, W. Y., Sun, S. B., et al. (2013). Role of arbuscular mycorrhizal network in carbon and phosphorus transfer between plants. Biol. Fert Soils 49, 3‒11. doi: 10.1007/s00374-012-0689-y
Řezáčová, V., Zemková, L., Beskid, O., Püschel, D., Konvalinková, T., Hujslová, M., et al. (2018). Little cross-feeding of the mycorrhizal networks shared between C3-Panicum bisulcatum and C4-Panicum maximum under different temperature regimes. Front. Plant Sci. 9. doi: 10.3389/fpls.2018.00449
Rillig, M. C. (2004). Arbuscular mycorrhizae glomalin and soil aggregation. Can. J. Soil Sci. 84, 355–363. doi: 10.4141/S04-003
Rillig, M. C., Aguilar-Trigueros, C. A., Camenzind, T., Cavagnaro, T. R., Degrune, F., Hohmann, P., et al. (2019). Why farmers should manage the arbuscular mycorrhizal symbiosis. New Phytol. 222, 1171–1175. doi: 10.1111/nph.15602
Robinson, D., Fitter, A. (1999). The magnitude and control of carbon transfer between plants linked by a common mycorrhizal network. J. Exp. Bot. 50, 9–13. doi: 10.1093/jxb/50.330.9
Rodrigues, L. A., Martins, M. A., Salomao, M. S. M. B. (2003). Use of mycorrhizas and rhizobium in intercropping system of Eucalyptus and Sesbania. I: growth, uptake and transfer of nitrogen between plants. Rev. Bras. Cienc Solo 27, 583–591. doi: 10.1590/S0100-06832003000400002
Rogers, J. B., Laidlaw, A. S., Christie, P. (2001). The role of arbuscular mycorrhizal fungi in the transfer of nutrients between white clover and perennial ryegrass. Chemosphere 42, 153–159. doi: 10.1016/S0045-6535(00)00120-X
Ronsheim, M. L., Anderson, S. E. (2001). Population level specificity in the plant-mycorrhizae association alters intraspecific interactions among neighbouring plants. Oecologia 128, 77–84. doi: 10.1007/s004420000625
Roth, R., Paszkowski, U. (2017). Plant carbon nourishment of arbuscular mycorrhizal fungi. Curr. Opin. Plant Biol. 39, 50–56. doi: 10.1016/j.pbi.2017.05.008
Ryan, M. H., Graham, J. H., Morton, J. B., Kirkegaard, J. A. (2019). Research must use a systems agronomy approach if management of the arbuscular mycorrhizal symbiosis is to contribute to sustainable intensification. New Phytol. 222, 1176–1178. doi: 10.1111/nph.15600
Schindler, F. V., Mercer, E. J., Rice, J. A. (2007). Chemical characteristics of glomalin-related soil protein (GRSP) extracted from soils of varying organic matter content. Soil Biol. Biochem. 39, 320–329. doi: 10.1016/j.soilbio.2006.08.017
Schüßler, A., Walker, C. (2010). “The glomeromycota,” in A species list with new families and new genera. (California: CreateSpace Independent Publishing Platform), 1–58. Available at: http://www.amf-phylogeny.com.
Smith, F. A., Grace, E. J., Smith, S. E. (2009). More than a carbon economy: nutrient trade and ecological sustainability in facultative arbuscular mycorrhizal symbioses. New Phytol. 182, 347–358. doi: 10.1111/j.1469-8137.2008.02753.x
Smith, S. E., Smith, F. A. (2011). Roles of arbuscular mycorrhizas in plant nutrition and growth: new paradigms from cellular to ecosystem scales. Annu. Rev. Plant Biol. 62, 227–250. doi: 10.1146/annurev-arplant-042110-103846
Southworth, D., He, X. H., Swenson, W. S., Bledsoe, C. S., Horwath, W. R. (2005). Application of network theory to potential mycorrhizal networks. Mycorrhiza 15, 589–595. doi: 10.1007/s00572-005-0368-z
Teste, F. P., Veneklaas, E. J., Dixon, K. W., Lambers, H. (2015). Is nitrogen transfer among plants enhanced by contrasting nutrient-acquisition strategies? Plant Cell Environ. 38, 50–60. doi: 10.1111/pce.12367
Thirkell, J. D., Cameron, D. D., Hodge, A. (2016). Resolving the “nitrogen paradox” of arbuscular mycorrhizas: fertilization with organic matter brings considerable benefits for plant nutrition and growth. Plant Cell Environ. 39, 1683–1690. doi: 10.1111/pce.12667
Tobar, R. M., Azcón, R., Barea, J. M. (1994a). The improvement of plant n acquisition from an ammonium-treated, drought stressed soil by the fungal symbiont in arbuscular mycorrhizae. Mycorrhiza 4, 105–108. doi: 10.1007/BF00203769
Tobar, R., Azcón, R., Barea, J. M. (1994b). Improved nitrogen uptake and transport from 15N-labelled nitrate by external hyphae of arbuscular mycorrhiza under water-stressed conditions. New Phytol. 126, 119–122. doi: 10.1111/j.1469-8137.1994.tb07536.x
Trannin, W. S., Urquiaga, S., Guerra, G., Ibijbijen, J., Cadisch, G. (2000). Interspecies competition and n transfer in a tropical grass-legume mixture. Biol. Fertil Soils 32, 441–448. doi: 10.1007/s003740000271
Trépanier, M., Bécard, G., Moutoglis, P., Willemot, C., GagnéS, TJ, A., et al. (2005). Dependence of arbuscular-mycorrhizal fungi on their plant host for palmitic acid synthesis. Appl. Environ. Microb. 71, 5341–5347. doi: 10.1128/AEM.71.9.5341-5347.2005
Tuffen, F., Eason, W. R., Scullion, J. (2002). The effect of earthworms and arbuscular mycorrhizal fungi on growth of and 32P transfer between Allium porrum plants. Soil Biol. Biochem. 34, 1027–1036. doi: 10.1016/S0038-0717(02)00036-6
van der Heijden, M. G. A., Martin, F. M., Selosse, M. A., Sanders, I. R. (2015). Mycorrhizal ecology and evolution: the past, the present and the future. New Phytol. 205, 1406‒1423. doi: 10.1111/nph.13288
van Kessel, C., Singleton, P. W., Hoben, H. J. (1985). Enhanced n transfer from a soybean to maize by vesicular-arbuscular mycorrhizal (VAM). Plant Physiol. 79, 562–563. doi: 10.1104/pp.79.2.562
van Tuinen, D., Tranchand, E., Hirissou, F., Wipf, D., Courty, P. E. (2020). Carbon partitioning in a walnut-maize agroforestry system through arbuscular mycorrhizal fungi. Rhizosphere 15, 100230. doi: 10.1016/j.rhisph.2020.100230
Voets, L., Goubau, I., Olsson, P. A., Merckx, R., Declerck, S. (2008). Absence of carbon transfer between Medicago truncatula plants linked by a mycorrhizal network, demonstrated in an experimental microcosm. FEMS Microbiol. Ecol. 65, 350–360. doi: 10.1111/j.1574-6941.2008.00503.x
Wagg, C., Jansa, J., Stadler, M., Schmid, B., van der Heijden, M. G. A. (2011). Mycorrhizal fungal identity and diversity relaxes plant-plant competition. Ecology 92, 1303–1313. doi: 10.1890/10-1915.1
Wahbi, S., Maghraoui, T., Hafidi, M., Sanguin, H., Oufdou, K., Prin, Y., et al. (2016). Enhanced transfer of biologically fixed n from faba bean to intercropped wheat through mycorrhizal symbiosis. Appl. Soil Ecol. 107, 91‒98. doi: 10.1016/j.apsoil.2016.05.008
Walder, F., Niemann, H., Natarajan, M., Lehmann, M. F., Boller, T., Wiemken, A. (2012). Mycorrhizal networks: common goods of plants shared under unequal terms of trade. Plant Physiol. 159, 789–797. doi: 10.1104/pp.112.195727
Wang, Y. J., He, X. H., Meng, L. L., Zou, Y. N., Wu, Q. S. (2023). Extraradical mycorrhizal hyphae promote soil carbon sequestration through difficultly extractable glomalin−related soil protein in response to soil water stress. Microb. Ecol. doi: 10.1007/s00248-022-02153-y
Wang, B., Qiu, Y. L. (2006). Phylogenetic distribution and evolution of mycorrhizas in land plants. Mycorrhiza 16, 299‒363. doi: 10.1007/s00572-005-0033-6
Wang, G., Sheng, L., Zhao, D., Sheng, J., Wang, X., Liao, H. (2016). Allocation of nitrogen and carbon is regulated by nodulation and mycorrhizal networks in soybean/maize intercropping system. Front. Plant Sci. 7. doi: 10.3389/fpls.2016.01901
Waters, J. R., Borowicz, V. A. (1994). Effect of clipping, benomyl and genet on 14C transfer between mycorrhizal plants. Oikos 71, 246–252. doi: 10.2307/3546272
Watkins, N. K., Fitter, A. H., Graves, J. D., Robinson, D. (1996). Carbon transfer between C3 and C4 plants linked by a common mycorrhizal network, quantified using stable carbon isotopes. Soil Biol. Biochem. 28, 471–477. doi: 10.1016/0038-0717(95)00189-1
Weremijewicz, J., Sternberg, L. D. L. O., Janos, D. P. (2016). Common mycorrhizal networks amplify competition by preferential mineral nutrient allocation to large host plants. New Phytol. 212, 461‒471. doi: 10.1111/nph.14041
Werner, G. D. A., Strassmann, J. E., Ivens, A. B. F., Engelmoer, D. J. P., Verbruggen, E., Queller, D. C., et al. (2014). Evolution of microbial markets. P Natl. Acad. Sci. U.S.A. 111, 1237–1244. doi: 10.1073/pnas.1315980111
Whiteside, M. D., Digman, M. A., Gratton, E., Treseder, K. K. (2012). Organic nitrogen uptake by arbuscular mycorrhizal fungi in a boreal forest. Soil Biol. Biochem. 55, 7–13. doi: 10.1016/j.soilbio.2012.06.001
Wipf, D., Krajinski, F., Courty, P. E. (2019). Trading on the arbuscular mycorrhiza market: from arbuscules to common mycorrhizal networks. New Phytol. 223, 1127–1142. doi: 10.1111/nph.15775
Wurzburger, N., Brookshire, E. N. J., McCormack, M. L., Lankau, R. A. (2017). Mycorrhizal fungi as drivers and modulators of terrestrial ecosystem processes. New Phytol. 213, 996–999. doi: 10.1111/nph.14409
Yuan, M. T. M., Kakouridis, A., Starr, E., Nguyen, N., Shi, S. J., Pett-Ridge, J., et al. (2021). Fungal-bacterial cooccurrence patterns differ between arbuscular mycorrhizal fungi and nonmycorrhizal fungi across soil niches. mBio 12, e03509–e03520. doi: 10.1128/mBio.03509-20
Yuan, Y. G., van Kleunen, M., Li, J. M. (2021). A parasite indirectly affects nutrient distribution by common mycorrhizal networks between host and neighboring plants. Ecology 102, e03339. doi: 10.1002/ecy.3339
Zabinski, C. A., Quinn, L., Callaway, R. M. (2002). Phosphorus uptake, not carbon transfer, explains arbuscular mycorrhizal enhancement of Centaurea maculosa in the presence of native grassland species. Funct. Ecol. 16, 758‒765. doi: 10.1046/j.1365-2435.2002.00676.x
Zhang, H. L., Wang, X. Y., Gao, Y. Z., Sun, B. R. (2019). Short-term n transfer from alfalfa to maize is dependent more on arbuscular mycorrhizal fungi than root exudates in n deficient soil. Plant Soil 446, 23‒41. doi: 10.1007/s11104-019-04333-1
Zhou, X. Q., Li, J. Y., Tang, N. W., Xie, H. Y., Fan, X. N., Chen, H., et al. (2021). Genome-wide analysis of nutrient signaling pathways conserved in arbuscular mycorrhizal fungi. Microorganisms 9, 1557. doi: 10.3390/microorganisms9081557
Keywords: 13C, 15N, carbon and nitrogen cycling, interplant nutrient exchange, plant coexistence, resource competition, resource share
Citation: Luo X, Liu Y, Li S and He X (2023) Interplant carbon and nitrogen transfers mediated by common arbuscular mycorrhizal networks: beneficial pathways for system functionality. Front. Plant Sci. 14:1169310. doi: 10.3389/fpls.2023.1169310
Received: 19 February 2023; Accepted: 27 June 2023;
Published: 12 July 2023.
Edited by:
Shixiao Yu, Sun Yat-sen University, ChinaCopyright © 2023 Luo, Liu, Li and He. This is an open-access article distributed under the terms of the Creative Commons Attribution License (CC BY). The use, distribution or reproduction in other forums is permitted, provided the original author(s) and the copyright owner(s) are credited and that the original publication in this journal is cited, in accordance with accepted academic practice. No use, distribution or reproduction is permitted which does not comply with these terms.
*Correspondence: Siyue Li, c3lsaTIwMDZAMTYzLmNvbQ==; Xinhua He, eGluaHVhLmhlQHV3YS5lZHUuYXU=
Disclaimer: All claims expressed in this article are solely those of the authors and do not necessarily represent those of their affiliated organizations, or those of the publisher, the editors and the reviewers. Any product that may be evaluated in this article or claim that may be made by its manufacturer is not guaranteed or endorsed by the publisher.
Research integrity at Frontiers
Learn more about the work of our research integrity team to safeguard the quality of each article we publish.