- 1Microbiologie Adaptation et Pathogénie, UMR 5240 CNRS, University Lyon, INSA Lyon, Villeurbanne, France
- 2Institute of Ecology and Environmental Sciences, Sorbonne University, CNRS, INRAE, Paris, France
The genus Dickeya includes plant pathogenic bacteria attacking a wide range of crops and ornamentals as well as a few environmental isolates from water. Defined on the basis of six species in 2005, this genus now includes 12 recognized species. Despite the description of several new species in recent years, the diversity of the genus Dickeya is not yet fully explored. Many strains have been analyzed for species causing diseases on economically important crops, such as for the potato pathogens D. dianthicola and D. solani. In contrast, only a few strains have been characterized for species of environmental origin or isolated from plants in understudied countries. To gain insights in the Dickeya diversity, recent extensive analyzes were performed on environmental isolates and poorly characterized strains from old collections. Phylogenetic and phenotypic analyzes led to the reclassification of D. paradisiaca (containing strains from tropical or subtropical regions) in the new genus, Musicola, the identification of three water species D. aquatica, D. lacustris and D. undicola, the description of a new species D. poaceaphila including Australian strains isolated from grasses, and the characterization of the new species D. oryzae and D. parazeae, resulting from the subdivision of the species D. zeae. Traits distinguishing each new species were identified from genomic and phenotypic comparisons. The high heterogeneity observed in some species, notably for D. zeae, indicates that additional species still need to be defined. The objective of this study was to clarify the present taxonomy of the genus Dickeya and to reassign the correct species to several Dickeya strains isolated before the current classification.
Introduction
Members of the genus Dickeya belong to the family Pectobacteriaceae in the order Enterobacterales (Adeolu et al., 2016). Most of them are phytopathogenic bacteria and several species have a broad host range; they infect numerous vegetable crops and ornamental plants, including both monocot and dicot plants (Charkowski et al., 2012; Toth et al., 2021; van der Wolf et al., 2021). These pathogens cause either soft rots or vascular wilts in their plant hosts in temperate, tropical and subtropical climates (Charkowski et al., 2012). The soft rot symptoms are due to the action of bacterial pectinases associated with other plant cell wall-degrading enzymes (PCWDEs), which degrade the main structural components of the middle lamella and primary plant cell wall (Van Gijsegem et al., 2021). These devastating plant pathogens have a significant impact on agriculture, causing crop losses both in fields and during storage. Dickeya was classified among the top ten most important bacterial plant pathogens based on its economic and scientific impact (Mansfield et al., 2012). While most Dickeya strains have been isolated from diseased plants, some strains have also been found in surface water (Parkinson et al., 2014; Potrykus et al., 2016; Hugouvieux-Cotte-Pattat et al., 2019; Oulghazi et al., 2019; Pedron and Van Gijsegem, 2019; Ben Moussa et al., 2022).
The history of Dickeya classification began in 2005 with the proposal to place strains formerly designated Pectobacterium chrysanthemi (formerly Erwinia chrysanthemi) or Brenneria paradisiaca into the new genus Dickeya (Samson et al., 2005). At this time, the genus Dickeya comprised six recognized species, namely Dickeya chrysanthemi, Dickeya dadantii, Dickeya dianthicola, Dickeya dieffenbachiae, Dickeya paradisiaca, and Dickeya zeae (Samson et al., 2005). Thereafter, new changes were proposed in the genus Dickeya. Members of the species D. dieffenbachiae were reclassified as a subspecies of D. dadantii (i.e. D. dadantii subsp. dieffenbachiae) (Brady et al., 2012). Then, classification largely evolved with the identification of new Dickeya species and the contribution of genomics. Dickeya solani was identified as the causal agent of severe disease outbreaks of potatoes in Europe (van der Wolf et al., 2014). The novel species Dickeya fangzhongdai was isolated from pear trees in China (Tian et al., 2016) and from orchids in different countries (Alic et al., 2018). Recently, some members of the heterogeneous species D. zeae were reclassified into Dickeya oryzae including rice strains (Wang et al., 2020) and Dickeya parazeae (Hugouvieux-Cotte-Pattat and Van Gijsegem, 2021). Three new Dickeya species were also isolated from water sources. Dickeya aquatica was isolated from freshwaters in Scotland and Finland (Parkinson et al., 2014). Dickeya lacustris was found in lake water and in the rhizosphere of waterside plants in France (Hugouvieux-Cotte-Pattat et al., 2019). Dickeya undicola was found in water samples collected in Malaysia and France (Oulghazi et al., 2019). In addition, old strains from collection isolated in Australia from sugarcane or other Poaceae were classified as D. poaceiphila (Hugouvieux-Cotte-Pattat et al., 2020). More recently, the species D. paradisiaca has been reassigned to the new genus Musicola, including two species Musicola paradisiaca and Musicola keenii (Hugouvieux-Cotte-Pattat et al., 2021). Thus, the genus Dickeya currently comprises twelve validly accepted species: D. aquatica, D. chrysanthemi, D. dadantii, D. dianthicola, D. fangzhongdai, D. lacustris, D. oryzae, D. paradisiaca, D. poaceiphila, D. solani, D. undicola, and D. zeae.
Assigning the correct bacterial species to new and old Dickeya strains is necessary. The large host-range and non-specific symptoms of the soft-rot diseases increase the importance of taxonomy for accurate identification of the causal pathogens (Toth et al., 2021). Phenotypic analyses, mainly based on biochemical and nutritional traits, were previously used to differentiate species. This method remains interesting for a first classification of a large number of strains. However, the taxonomy has rapidly evolved with the use of genetic and genomic tools. Sequencing of the housekeeping genes recA or gapA is routinely used to refine interspecific phylogenetic positions of strains from the genus Dickeya (Parkinson et al., 2009; Suharjo et al., 2014; Cigna et al., 2017). Whole-genome sequence data are now widely used to understand the evolutionary and taxonomic relationships in bacteria. Thus, both phenotypic analysis and comparative genomics are key tools to improve the taxonomy of cultivable strains.
The virulence equipment of the phytopathogenic Dickeya species is based on the production and secretion of a battery of PCWDEs (Van Gijsegem et al., 2021). These bacteria have a high capacity of production and secretion of enzymes possessing pectinase, cellulase or protease activity. The maceration symptom is mainly due to the activity of pectate lyases of the PL1 family (Hugouvieux-Cotte-Pattat et al., 2014), acting together with several accessory pectinases, the cellulase CelZ and a few metalloproteases (Van Gijsegem et al., 2021). The multiplication of PCWDEs in D. dadantii 3937 is partly due to gene duplications leading to clusters of homologous genes, namely pelADE, pelBC, pehXVW, and prtABC (Hugouvieux-Cotte-Pattat et al, 1996; Hugouvieux-Cotte-Pattat et al, 2014). However, virulence is multifactorial and additional virulence determinants have been identified (Van Gijsegem et al., 2021). Different protein secretion systems (T1SS to T6SS) are present in Dickeya. The T2SS Out drives the secretion of extracellular pectinases and of the cellulase CelZ; it is essential for virulence and present in all Dickeya members. Most Dickeya members possess the T1SS Prt devoted to the secretion of the proteases and the T3SS Hrp secreting a few effectors. In contrast, the equipment in T4SS, T5SS or T6SS largely varies among Dickeya species (Van Gijsegem et al., 2021). Dickeya members produce an array of secondary metabolites involved in plant-bacteria interactions, competition to other microorganisms, or adaptation to diverse environments. In particular, they carry large gene clusters that encode complex non-ribosomal peptide synthetases (NRPS) and polyketide synthases (PKS) (Van Gijsegem et al., 2021).
Many Dickeya strains have been stored in international bacterial collections but this material deserves to be better valued (Broders et al., 2022). For example, the analysis of old Dickeya strains from the French Collection of Phytopathogenic Bacteria, CFBP (https://cirm-cfbp.fr/) contributed to clarify the taxonomy and epidemiology of the Pectobacterium species (Portier et al., 2020) and allowed the description of the two species D. poaceiphila and M. keenii (Hugouvieux-Cotte-Pattat et al., 2020; Hugouvieux-Cotte-Pattat et al., 2021). Most Dickeya strains isolated and analyzed before 2010s came from diseased crop plants, and there was a need to expand exploration to other environments. Dickeya strains are more difficult to isolate from environmental samples which contain many other bacteria, whereas rotten tissues contain almost only the causal agent. This approach was however possible thanks to a powerful semi-selective medium (Hélias et al., 2012) which allowed the isolation of the new aquatic species D. aquatica, D. lacustris, and D. undicola (Parkinson et al., 2014; Hugouvieux-Cotte-Pattat et al., 2019; Oulghazi et al., 2019).
The objective of this study was to present an updated view of the taxonomy of the genus Dickeya and to reassign the correct species to Dickeya strains isolated before the current classification. We resumed knowledge on the genus Dickeya and added new data from phenotypic, genomic and phylogenetic analyses. We tried to favour inter-species comparison by applying the analyses uniformly to all the Dickeya species. As the taxonomy within the Dickeya genus has been subject to several changes over the past 10 years, this update on the genomic and phenotypic biodiversity within this taxon is supported by information on the strains belonging to each Dickeya species.
Materials and methods
Strains
Strains of each species are listed in Tables 1–7; S1, with the plant hosts, country of origin and isolation date when these data were available. Musicola strains are listed in Table 1, D. aquatica, D. lacustris, D. poaceiphila and D. undicola strains in Table 2, D. dadantii strains in Tables 3; S1, D. dianthicola and D. solani strains in Tables 4; S1, D. fangzhongdai strains in Tables 5; S1, D. chrysanthemi in Table 6, andD. oryzae, D. parazeae and D. zeae strains in Tables 7; S1.
Growth conditions and phenotypic analysis
To test their growth with different carbon sources, strains were inoculated onto M63 minimal medium plates supplemented with a sole carbon source (2 g l-1). The enzyme secretion was assessed on plates containing an enzyme substrate (Hugouvieux-Cotte-Pattat et al., 2019). For the detection of pectinase activity, bacteria were grown on M63 agar plates supplemented with 2 g l-1 glycerol and 4 g l-1 polygalacturonic acid. After incubation for 24 h at 30°C, plates were flooded with a saturated solution of copper acetate. Clear haloes appear around colonies secreting pectate-lyases. Cellulase activity was detected on M63 agar plates supplemented with 2 g l-1 glycerol and 10 g l-1 carboxymethylcellulose. After incubation for 24 h at 30°C, the plates were overflowed with 10 mg ml-1 Congo red solution for 10 min and washed for 5 min with 1 M NaCl. Clear haloes surround colonies secreting cellulases. Protease production was tested on LB plates supplemented with skim milk (12.5 g l-1). After incubation at 30°C for 24 to 48 h, clear haloes are visible around colonies secreting proteases.
PCR, amplicon sequencing and recA phylogenetic analysis
The strains were subjected to PCR with primers specific for the gene gapA (gapAF and gapAR, 0.8 kb amplicon) (Cigna et al., 2017). The PCR products were sequenced in both directions with the same set of primers, using a commercial service (Microsynth France, Vaulx-en-Velin France). These gapA sequences were used for strain identification using BlastN comparison and construction of phylogenetic trees including the type strains. In addition, several recA and 16S rRNA sequences were retrieved from NCBI databases and used to define or verify the species affectation of the strain. Several strains firstly identified as E. chrysanthemi or Dickeya sp. were assigned to species that were not recognized at the time of their isolation.
The phylogenetic tree based on the recA gene was constructed using the pipeline Phylogeny.fr (http://www.phylogeny.fr/phylogeny.cgi); the nucleotide sequences are aligned with MUSCLE (Edgar, 2004), the phylogenetic tree is reconstructed using the maximum likelihood method implemented in the PhyML program (Guindon and Gascuel, 2003) and graphical representation is performed with TreeDyn (Dereeper et al., 2008). The recA sequences were retrieved from the NCBI database; accession numbers are given in the tree.
Comparative genomic analysis
The dDDH values were calculated using the webserver Genome-to-Genome Distance Calculator (GGDC) version 2.1 with the formula 2 (Meier-Kolthoff et al., 2013). The Average Nucleotide Identity (ANI) based on the Nucleotide MUMmer algorithm (Delcher et al., 2002) was calculated using the JSpecies Web Server with default parameters (Richter and Rosselló-Móra, 2009). The dDDH value of 70% and the cut-off ANI values of 95–96% between two strains were considered for species delineation (Goris et al., 2007).
To determine the phylogenetic position of Dickeya species, the phylogenomic tree was constructed from concatenated protein sequences of 963 unique homologous proteins (293566 sites). It was computed using the BioNJ distance method (Criscuolo and Gascuel, 2008). Two hundred bootstrap replicates were performed to assess the statistical support of each node.
Results and discussion
The new genus Musicola, analysis of old strains from collections
After analysis of a large panel of strains, Samson et al. (2005) proposed the species D. paradisiaca which comprised the former Brenneria paradisiaca type strain CFBP 4178T and six E. chrysanthemi strains (CFBP 1445, CFBP 1446, CFBP 1451, CFBP 3477, CFBP 3696, CFBP 3699). This classification was mainly based on phylogenetic analyses of 16S rRNA gene sequences which suggested that these strains were phylogenetically distant to all Brenneria species. Recently, genomic analyses have suggested that the differences between D. paradisiaca and other Dickeya species justify the creation of a separate genus (Pritchard et al, 2016; Pedron and Van Gijsegem, 2019). The genus Musicola was recognized in 2021, on the basis of phenotypic, phylogenetic and genomic arguments (Hugouvieux-Cotte-Pattat et al., 2021). Most D. paradisiaca strains were isolated from banana trees (Musa paradisiaca) in tropical or subtropical countries (Dickey and Victoria, 1980) (Table 1).
The species previously named D. paradisiaca was considered as the earliest branching lineage in the Dickeya genus in previous evolution studies (Duprey et al., 2019). However, data on the genetic diversity of this species was scarce, and it was poorly characterized at the genomic level. Only two genome sequences were available, including those of the type strain CFBP 4178T (Pritchard et al., 2013). Calculation of aligned fraction values between Dickeya and Musicola genomes showed that less than 33% of the genomes of these two genera could be aligned together (Hugouvieux-Cotte-Pattat et al., 2021).
A new Musicola strain was identified among poorly characterized strains of the CFBP collection. This strain, A3967 (CFBP 722), is related to M. paradisiaca CFBP 4178T but showed atypical phenotypes for sugar assimilation. Genomic comparison (ANI and dDDH values of 96.21% and 68.3%, respectively) confirmed the divergence and justified the description of a novel species Musicola keenii (Hugouvieux-Cotte-Pattat et al., 2021). Since the main phenotypic differences between M. keenii and M. paradisiaca members concern sugar assimilation, a simple distinction between the two species can be obtained by testing the bacterial growth in the presence of myo-inositol, melibiose or raffinose as the sole carbon source (Table 8). Sequencing of the genomes of two other Musicola strains, CFBP 3477 and CFBP 3699, confirms their assignation to the species M. paradisiaca, as suggested by phenotypic analysis (Table 1).
Musicola strains are less equipped than Dickeya members in PCWDEs and other virulence factors. They lack genes encoding PelA, PelI, PnlH, PehN, Rhi, PemB, GanA and Prt (Table 9). The reduced number of PCWDE genes could explain the low pectinase activity and the absence of protease secretion observed for Musicola members (Table 8). While the number of T1SS, T3SS, T4SS and T6SS varies among Dickeya species, Musicola members have none of them. In addition, they are deprived of factors important to deal with the plant stress responses occuring during infection, such as the nitric oxide dioxygenase HmpX that detoxify nitric oxide produced by plants, the suf cluster involved in the repair of damaged Fe/S clusters, and the ind cluster encoding the ROS scavenging pigment indigoïdine. These dissimilarities suggest notable differences in the virulence strategies of Dickeya and Musicola members. Indeed, virulence tests showed that the Musicola strains have a weak maceration activity on potato tubers and chicory leaves, two dicot models classically used to evaluate the virulence of Dickeya strains (Hugouvieux-Cotte-Pattat et al., 2021). With a low pectate lyase activity, Musicola strains are less equipped than Dickeya strains to degrade the high pectin content of dicot cell wall. Musicola strains may be better adapted to monocots such as Poaceae whose primary cell wall has a low pectin content (Jarvis et al., 1988).
Only 16 Musicola strains have been characterized up to now (Table 1). These strains were mainly isolated from banana and in a few cases from another monocot, corn, and from dicots of the Solanaceae family, potato and tomato. They were isolated between 1968 and 1987 (Table 1). Unfortunately, no Musicola strain has been isolated more recently.
The water species D. aquatica and D. lacustris, true Dickeya or members of another genus?
Some water isolates belong to phylogenetic groups differing from plant-related Dickeya species. The two species D. aquatica and D. lacustris form a clade distinct from the other Dickeya species in phylogenomic studies (Duprey et al., 2019; Hugouvieux-Cotte-Pattat et al., 2019) (Figure 1). Some D. lacustris strains were found associated to the rhizosphere of bittersweet nightshade (Solanum dulcamara), a weed whose roots are in contact with water (Hugouvieux-Cotte-Pattat et al., 2019). Since water strains deserve less interest than pathogenic isolates, they were less studied. Only a few genomes have been sequenced, three for D. aquatica and one for D. lacustris (Table 2). Very recently, a new D. lacustris genome sequence (GCA_027571425.1) became available, it corresponds to strain isb1 isolated in Pakistan from Human stool. The surprising origin of this strain needs further investigation.
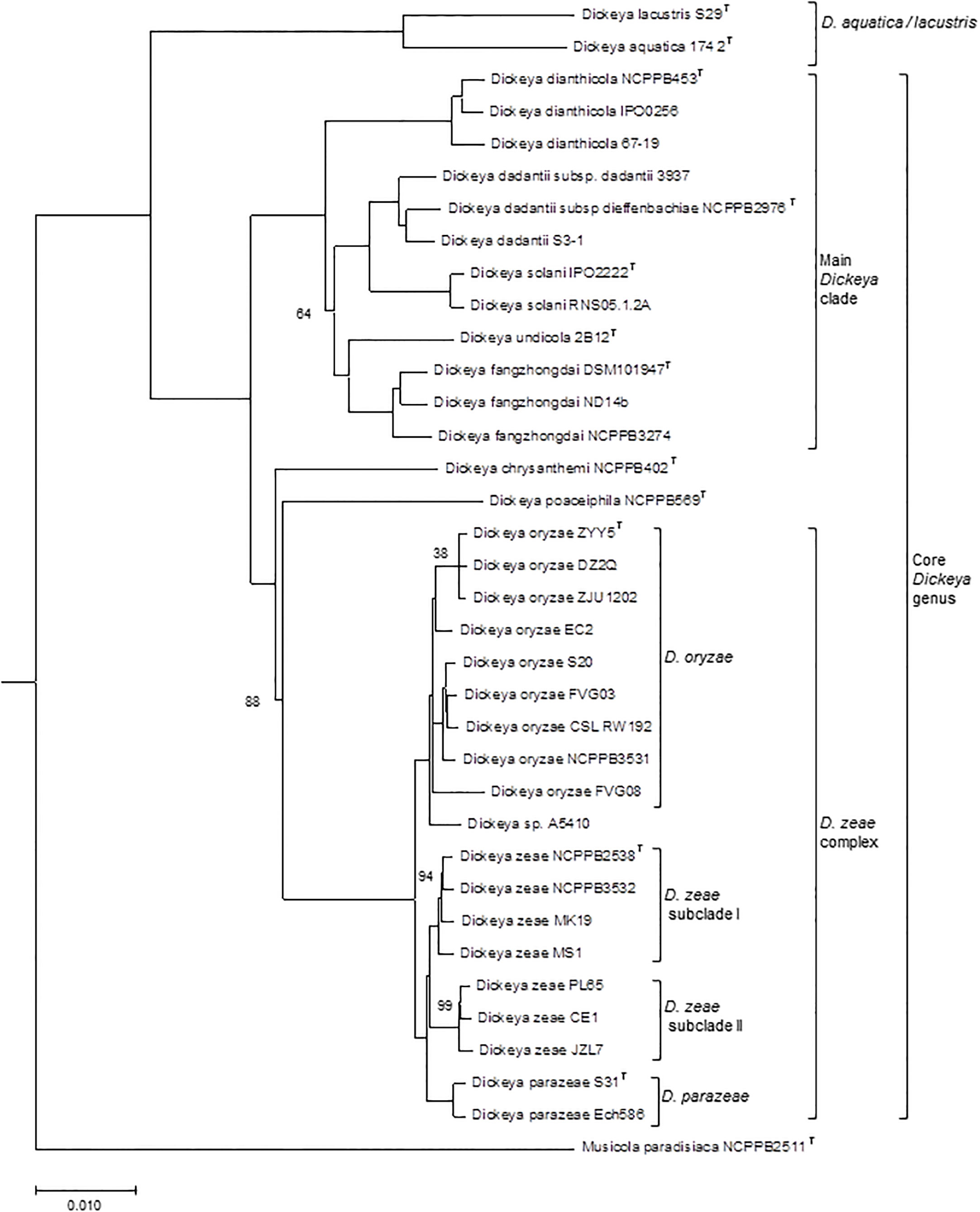
Figure 1 Phylogenomic tree of the Dickeya species. The phylogenetic tree, constructed from concatenated amino acid sequences of 963 unique homologous proteins (293566 sites), was computed using the BioNJ distance method. Two hundred bootstrap replicates were performed to assess the statistical support of each node. The tree includes 35 Dickeya genomes, representative of the different species and subclades discussed in the text. A Musicola genome was used as outgroup. Only boostrap values below 100 are indicated. T, type strain. The scale bar represents the average number of changes per nucleotide position.
Although there is no formal definition of genus delineation on the basis of genome similarity, recent approaches used the genome aligned fraction to discriminate at genus level, showing that genus boundary corresponds to aligned fraction values around 60% (Barco et al., 2020). Indeed, for strains of the ten species D. chrysanthemi, D. dadantii, D. dianthicola, D. fangzhongdai, D. oryzae, D. parazeae, D. poaceiphila, D. solani, D. undicola and D. zeae, paired aligned fractions correspond to at least 58% of the genome lengths (Hugouvieux-Cotte-Pattat et al., 2021). These ten species constitute a coherent group corresponding to the “core Dickeya genus” (Figure 1). With aligned fractions of 73% between D. lacustris and D. aquatica genomes, these two species correspond to a homogeneous group. In contrast, the aligned fraction of D. lacustris and D. aquatica genomes is less than 41% with other Dickeya species (Hugouvieux-Cotte-Pattat et al., 2021). At the phenotypic level, D. lacustris and D. aquatica showed some metabolic particularities in comparison to the ten species of the “core Dickeya genus”, such as the non-assimilation of xylose or mannitol (Table 8). However, their PCWDE equipment is quite similar to that of other Dickeya species. They lack a few accessory pectinases PnlG, PehN and PemB (Table 9), but they have the capacity to macerate different plants in laboratory conditions (Duprey et al., 2019). A notable difference is their ecology as D. lacustris and D. aquatica seem to inhabit only water. An exception could be an isolate from carrot in Northern Ireland which was putatively identified as D. aquatica on the basis of a partial recA sequence (Zaczek-Moczydłowska et al., 2019). However, phylogenetic analysis of the corresponding sequences (MH688057, MH688058) suggests that strain Ca3 is quite distant from D. aquatica (Figure 2). Further analysis will be required to clarify the classification of this strain. The isolation of new strains and sequencing of new genomes is essential in order to obtain more information on the phenotypic and genetic diversity of these water species.
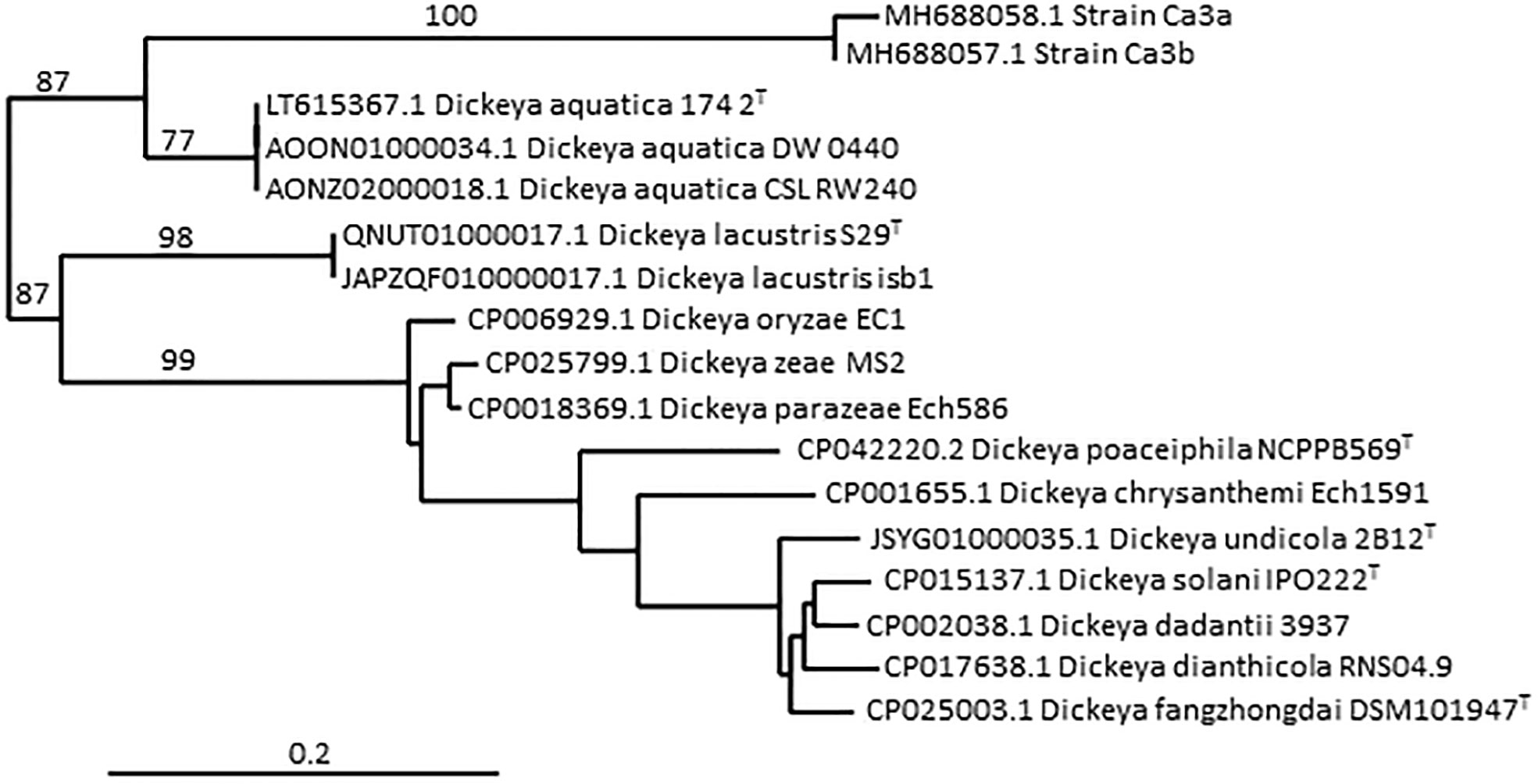
Figure 2 Phylogenetic position of strain Ca3a among the Dickeya species, based on partial recA gene sequences. The tree was constructed using the pipeline Phylogeny.fr (http://www.phylogeny.fr/phylogeny.cgi). The nucleotide sequences are aligned with MUSCLE, the phylogenetic tree is reconstructed using the maximum likelihood method implemented in the PhyML program and graphical representation is performed with TreeDyn. Numbers correspond to bootstrap values (500 replicates). The scale bar represents the average number of substitutions per site. The two strains Ca3a and Ca3b were isolated from carrots in Northern Ireland (Zaczek-Moczydłowska et al., 2019) and their partial recA gene sequences (MH688058 and MH688057, respectively) consisted of 503 nt.
Analysis of old strains from collections, the species Dickeya poaceiphila
The phenotypic and genetic analysis of poorly characterized strains from collections allowed the characterization of the species Dickeya poaceiphila, including a group of strains isolated in Australia from sugarcane and other Poaceae (commonly known as grasses) (Hugouvieux-Cotte-Pattat et al., 2020). Between 1955 and 1957, a plant disease affecting sugarcane in Australia was designated by bacterial mottle (Steindl, 1964). The symptoms consisted of chlorotic striping of the leaves which were heavily invaded by bacteria as were also the stems, causing severe stunting and wilting (Dowson and Hayward, 1960). Symptoms of chlorosis and wilting were also observed on various grasses occurring near sugarcane fields, such as Pennisetum purpureum (elephant grass), Megathyrsus maximus (Guinea grass), and Brachiaria mutica (para grass). The strain NCPPB 569T was sampled during this outbreak in Australia; its genome sequence was reported in 2013 (Pritchard et al., 2013). Genomic comparisons indicated that this strain was a candidate for assignment to a novel Dickeya species (Pritchard et al., 2016; Duprey et al., 2019) and the species D. poaceiphila was recognized in 2020 (Hugouvieux-Cotte-Pattat et al., 2020). In addition to the type strain, it includes strain CFBP 2040 whose genome was also sequenced (dDDH values of 90.2%) and strain CFBP 1537 also isolated in Australia (Table 2).
The three D. poaceiphila strains showed substantial phenotypic differences compared to other Dickeya species. They produce a low level of pectinases and proteases and no cellulase activity (Table 8). This low secretion of PCWDEs is associated with a low capacity to macerate potato tubers and chicory leaves (Hugouvieux-Cotte-Pattat et al., 2020). The absence of extracellular cellulase activity in D. poaceiphila results from the absence of the cellulase gene celZ present in all other Dickeya species (Table 9). The weak protease production could be due to the presence of a single prt gene in D. poaceiphila, while several prt genes are present in other Dickeya species (Table 9). Similarly, the low level of pectinase activity could be due to a low number of pectinase genes. D. poaceiphila has only two genes encoding secreted pectate lyases of the polysaccharide lyase family 1 (PL1), one of the pelADE cluster and one of the pelBC cluster. PL1 are responsible for the major pectate lyase activity of Dickeya (Hugouvieux-Cotte-Pattat et al., 2014) and the other Dickeya species contain 5 to 6 genes encoding PL1 pectate lyases (Table 9). D. poaceiphila is the only Dickeya species lacking the pectate lyase genes pelL and pelZ. It also lacks the accessory pectinase genes pnlG, pnlH, pehK, pehN, pemB and the galactanase gene ganA. It has only one polygalacturonase gene of the cluster pehXVW (Table 9). Several gene duplications leading to clusters of pel, peh or prt genes in other Dickeya species are not found in D. poaceiphila. This restricted number of PCWDE activities and genes could explain why D. poaceiphila strains are poorly effective in causing soft rot symptoms (Tables 8, 9) and instead produce other types of symptoms, such as stunting or wilting (Dowson and Hayward, 1960).
Additional phenotypic differences between D. poaceiphila and other Dickeya species were observed for sugar assimilation, including an absence of growth with D-gluconic acid, D-fructose-6-phosphate, D-glucose-1-phosphate, D-glucose-6-phosphate, myo-inositol, or polygacturonate (PGA), the pectin backbone (Table 8). The absence of growth of D. poaceiphila on pectin or polygalacturonate may result from the inactivation of kduI, a gene involved in the intracellular pectin catabolic pathway (Hugouvieux-Cotte-Pattat et al., 1996). This gene is annotated as a pseudogene in D. poaceiphila because it has an internal deletion of 555 bp. A defect in pectin assimilation is clearly a factor that could affect the bacterial growth in macerated plant tissues. It is less troublesome for a species that does not cause maceration of its host plants but other types of symptoms.
While D. poaceiphila strains show clear differences in phenotypic and genomic features from strains of the other characterized Dickeya species, phylogenomic studies confirmed that these strains clearly belong to the genus Dickeya (Hugouvieux-Cotte-Pattat et al., 2020). However, the D. poaceiphila genomes appear to be the smallest ones (4.22-4.32 Mb) among the Dickeya species and they have the lowest GC content (52.60-52.70%) (Table 10). A reduced genome size is consistent with the numerous gene losses observed in this species and suggests an evolution of these genomes towards an adaptation to a more restricted habitat. This could correspond to an adaptation to a specialized host range and type of symptom as D. poaceiphila has only been shown to cause wilt disease on monocot herbaceous plants of the Poaceae family.
Only three D. poaceiphila strains have been characterized and only two genome sequences have been reported (Table 2). Since the three strains were isolated from the same country, isolation and characterization of new strains from different origins will be necessary to access to the diversity of the species D. poaceiphila and to confirm its genomic divergence and potential specialization within the genus Dickeya.
The main Dickeya clade includes D. dadantii, D. dianthicola, D. fangzhongdai, D. solani and D. undicola
Using phylogenetic studies, two clades are observed in the core Dickeya genus (Figure 1). One includes D. chrysanthemi, D. poaceiphila, D. oryzae, D. parazeae and D. zeae. The other, called the “main Dickeya clade”, regroups the five species D. dadantii, D. dianthicola, D. fangzhongdai, D. solani and D. undicola (Figure 1). These five species form a clade sharing high ANI values (89 to 94%) and share more than 50% of their protein families (around 2600) including most virulence genes (Pedron and Van Gijsegem, 2019). They have a large equipment in PCWDEs (Table 9). Their genomes encode at least ten pectate lyases (PelB, PelC, PelD, PelE, PelI, PelL, PelN, PelW, PelX and PelZ), the rhamnogalacturonate lyase RhiE, the predicted pectin lyase PnlG, the pectin acetyl esterases PaeX and PaeY, the feruloyl esterases FaeD and FaeT, and the two pectin methyl esterases PemA and PemB (Table 9). The arsenal of polygalacturonases is more variable, ranging from three to five, including at least PehN and PehX. All species of the main Dickeya clade produce the cellulase CelZ and the galactanase GanA. Their genome harbor at least two protease genes (Table 9). While the siderophore clusters cbs and acs belongs to the Dickeya core genome, most clusters encoding secondary metabolite biosynthesis are members of the Dickeya accessory genomes, as they variably distributed among species and sometimes among strains of the same species (Table 11).
Despite their high genetic proximity and sharing of virulence related genes, the closeness and host ranges of these five Dickeya species differ, ranging from narrow to wide host ranges and genomic diversities. The two species D. dadantii and D. fangzongdai are even able to cause tree diseases in orchards, such as the bleeding canker necrosis of pear trees (Tian et al., 2016) and the bacterial quick decline of apple trees (Fujikawa et al., 2019).
D. dadantii, a wide host range, the model strain 3937
Noticeably, the D. dadantii species includes the strain 3937 that has been used for a long time as a model of the genus Dickeya for genetic studies leading to the characterization of virulence factors, including the different enzymes constituting the PCWDE equipment, and the regulatory network controlling expression of the virulence genes (Hugouvieux-Cotte-Pattat et al., 1996; Hugouvieux-Cotte-Pattat et al., 2014; Reverchon et al., 2016). The 3937 genome was the first Dickeya genome to be sequenced (Glasner et al., 2011).
Among all Dickeya species, D. dadantii has the widest host range and geographical distribution. It was isolated in all continents, from several dicots and monocot plants. More than 100 strains have been isolated from at least 25 countries and 26 different plants, including apple, peach and pear trees, vegetables (potato, tomato, eggplant, carrot, sweet potato), and numerous ornamentals (Aglaonema, Dieffenbachia, Drimiopsis, Euphorbia, Kalenchoe, Pelargonium, Phylodendron, Saintpaulia, Syngonium, etc). It was occasionally found in corn (Zea mays), cactus (Gymnocalicium), alpine plants (Erygium alpinum) and water (Table 3; Table S1).
First described as a separate species, D. dieffenbachiae was included as a subspecies of D. dadantii (D. dadantii subsp. dieffenbachiae) since both species type strains shared 96.6 ANI and 71.4% dDDH values. The main D. dadantii clade was renamed D. dadantii subsp. dadantii (Brady et al., 2012). The main phenotypic difference between the two subspecies is the assimilation of melibiose (Table 8). D. dadantii subsp. dieffenbachiae strains were nearly all isolated from ornamentals of the genus Dieffenbachia (Araceae family). With the isolation of new Asiatic strains of D. dadantii (Fujikawa et al., 2019; Wei et al., 2021; Tan et al., 2022), the heterogeneity within this species may increase. The strain S3-1 isolated from an Araceae (Zantedeschia aethiopica) in Taiwan (Wei et al., 2021) is distinct from members of both D. dadantii subspecies, with 96.3-96.7 ANI values and 68-72% dDDH values. This raises the possibility of an additional D. dadantii subspecies including strains S3-1, FZ06 and A622-S1-A17 (Table 3, Figure 1). A classification at the subspecies level can be justified when each subspecies has a specific feature or is linked to a particular host. However, newly isolated strains are rarely classified at this level, except when there is a clear phenotypic difference between the subspecies. So far, no specific traits have been described for the three D. dadantii strains not assigned to a recognized subspecies. Nevertheless, with 17 genome sequences available (Table 3), the genomic diversity of the D. dadantii species is exemplified by the large range of genome sizes, from 4.66 to 5.35 Mb (Table 10).
Another intriguing type of diversity among D. dadantii members concerns the vfm cluster which is present in all Dickeya strains (Table 11). The VFM molecule plays a major role in intercellular communication by acting as a quorum sensing molecule (Nasser et al., 2013). However, differences in the enzymatic specificity of the proteins VfmO and VfmP generate the production of different analogs of the VFM molecule, which vary according to the strain (Hugouvieux-Cotte-Pattat et al., 2022). As these analogs are differentially recognized, signaling through the Vfm quorum sensing system is limited to strains belonging to compatible groups. For instance, the D. dadantii strains are divided in two groups and the production of two different VFM analogs affects the intra-species communication (Table 11).
D. dianthicola, a potato pathogen known since 1950s
The two species D. dianthicola and D. solani deserved special attention because they caused severe damages in the important crop potato. Most analyzed genomes are from strains isolated from diseased potatoes introducing a severe bias for diversity analyses. Despite its reputation of potato pathogen, D. dianthicola has a broad host range (van der Wolf et al., 2021). It was first described in an outbreak on carnation in Europe in the 1950s. Nearly 300 strains have now been characterized, including about 80% potato isolates (Table 4; Table S1). Other isolates were reported from vegetables, such as chicory or artichoke, and ornamentals, frequently carnation, kalanchoe, begonia and dahlia (Table 4). Potato infections in Europe have been detected since the 1970s but losses caused by D. dianthicola remained generally low and sporadic (Toth et al., 2011) except in Switzeland and the Netherlands where D. dianthicola dominated till the advent of D. solani in the 2000s (Pedron et al., 2021; Pedron et al., 2022). Since 2015, D. dianthicola caused a severe blackleg outbreak in the US that originated from Maine and further spread in at least eighteen US states (Charkowski, 2018). D. dianthicola also caused a blackleg outbreak in Western Australia in 2017 (Wright et al., 2018).
All D. dianthicola genomes contain the cluster ooc involved in the biosynthesis of an oocydin A-like molecule that may contribute to the maintenance of these species in the potato environment, by favouring the competition with other microorganisms (Brual et al., 2023). At the genomic level, D. dianthicola has a truncated pectate lyase gene pelA and lacks two protease genes (Table 9). At the phenotypic level, it is the sole member of the main Dickeya clade unable to grow with D-arabinose as the sole carbon source and it is the sole Dickeya species unable to grow at 39°C (Table 8). Such phenotypic differences could reflect a divergent adaptation of D. dianthicola to environmental conditions.
Due to the large interest for this potato pathogen, more than 70 D. dianthicola genome sequences are now available (Tables 4, 10). Most show strong relatedness illustrated by ANI values greater than 99% and clonal origin of the strains isolated during the US potato outbreak (Ge et al., 2021). Analysis of additional genomes highlights the diversity in D. dianthicola, with two strains isolated from potato in the Netherlands in 1975 and from impatiens in the US in 2019 (Liu et al, 2021) (Table 4), sharing 97 to 98% ANI values with other D. dianthicola genomes (Pedron et al., 2022). A recent report of D. dianthicola presence in Asteraceae weeds (fleabane and butterbur) close to potato fields points to another possible route of transmission from weeds to potato through surface water flow (Aono et al., 2022).
D. solani, a potato pathogen emerging in the 2000s
In contrast to D. dianthicola, D. solani has a narrow host range, it was recognized as the agent of a potato outbreak in Europe in the 2000s (Slawiak et al., 2009; van der Wolf et al., 2014), then it spread in Asia (Israel, Syria, Turkey, Georgia, oriental Russia), North Africa (Morocco) and Latin America (Brazil) (van der Wolf et al., 2021). On 65 D. solani characterized strains so far (Table 4; Table S1), only two other hosts have been identified apart to potato, the ornamentals muscari (one strain) and hyacinth (2 strains), and two strains were isolated from water (Table 4). A large majority of D. solani isolates collected in Europe and the Mediterranean Basin are clonal (Khayi et al., 2015; Golanowska et al., 2018; Blin et al., 2021). Even strains isolated more than 20 years apart are highly related, indicating a high genomic stability (Pedron et al., 2021). Genomic variations in D. solani genomes consist mainly in the presence of genes related to phages and even complete prophages (Golanowska et al., 2018; Khayi et al., 2022). The first exception was observed with strain RNS05.1.2A isolated from potato in France, which showed an ANI value of only 98% with other D. solani genomes (Khayi et al., 2015). Recently, two other French strains, RNS10-105-1A and A623S-20A-17, isolated from potato and surface water respectively, were shown to belong to the same rare subclade (Khayi et al., 2022). The other genetic variations observed in D. solani results from horizontal gene transfers leading to gene replacement. A few potato strains collected in France (RNS07.7.3B, RNS13-30-1A, RNS13-31-1A and RNS13-48-1A) acquired genomic regions related to the RNS05.1.2A subclade (Khayi et al., 2022). Two D. solani strains isolated from ornamentals (PPO9019 and PPO9134) acquired gene clusters from D. dianthicola. The strain PPO9019 also possesses a 45 kb plasmid identical to a plasmid of Burholderia ambifaria (Khayi et al., 2015). Interestingly, D. solani and B. ambifaria beside sharing an identical plasmid, exhibit similarities in the O-polysaccharide compositions that both contain 6-deoxyaltropyranose (Ossowska et al., 2017). Even if divergences remain rather limited, the D. solani strains from ornamentals or water seem to show more diversity than the potato isolates. Strains showing genomic variations were collected in The Netherlands and France and it would be interesting to further analyze the potential D. solani diversification in other countries.
A genomic comparison revealed the presence of three genomic regions encoding NRPS, PKS and associated proteins which are present in all D. solani genomes but absent in D. dadantii 3937 (Garlant et al., 2013; Pedron et al., 2014). First described for some D. oryzae strains (Zhou et al., 2011), the cluster zms is found in all D. solani and D. fangzhongdai genomes (Table 11). It encodes proteins involved in the biosynthesis of toxins of the zeamine family. The zeamine produced by D. solani is involved in antibacterial activity (Effantin et al., 2021; Brual et al., 2023). The D. solani cluster ooc comprises genes involved in the biosynthesis of an oocydin A-like molecule, a compound inhibiting Ascomycetes growth (Effantin et al., 2021; Brual et al., 2023). This cluster is also found in all the D. dianthicola genomes and some strains of D. fangzongdai, D. oryzae, D. chryanthemi and D. aquatica (Table 11). The cluster sol (or ssm) produces an antifungal compound recently named solanimycin (Matilla et al., 2022). Solanimycin is active against a broad range of plant-pathogenic fungi and the human pathogen Candida albicans (Effantin et al., 2021; Matilla et al., 2022; Brual et al., 2023). The sol cluster is conserved in most Dickeya species, including all the genomes of D. fangzongdai, D. poaceiphila, D. parazeae and D. zeae and in some strains of D. dadantii, D. undicola and D. oryzae (Table 11). The presence of the sol cluster appears to be correlated with signs of horizontal genetic transfer (Matilla et al., 2022).
D. fangzhongdai, a tree and orchid pathogen
D. fangzhongdai was the first Dickeya species found to infect trees, where it causes bleeding canker necrosis (Tian et al., 2016). Most D. fangzhongdai isolates originated from Asia (Table 5, Table S1), but the species was also identified in Europe, mainly in orchids (Alic et al., 2017). Analysis of old bacterial collections showed that D. fangzhongdai was introduced to Europe as early as 1985, through the propagation of ornamental plants (Parkinson et al., 2009; Van Vaerenbergh et al., 2012; Suharjo et al., 2014; Alic et al., 2018). Beside pear trees, D. fangzhongdai is able to cause soft rot on orchids (Phalenopsis, Oncidium, Vanda, Cattleya), and it is also found in water (Alic et al., 2018) (Table 5). Unlike other members of the main Dickeya clade, D. fangzhongdai strains are able to assimilate the rare sugar D-psicose (Table 8). The D-psicose catabolic pathway was not yet characterized but this capacity was also observed for D. oryzae and D. poaceiphila (Table 8). Genome mining in the three Dickeya species able to utilize D-psicose demonstrates the correlation between the presence of the gene cluster alsRBACDEKS and the bacterial utilization of D-psicose (Figure 3A). The importance of this pathways during pathogenesis is unknown but D-psicose was shown to induce some plant defense genes, conferring plant resistance to diseases (Kano et al., 2011).
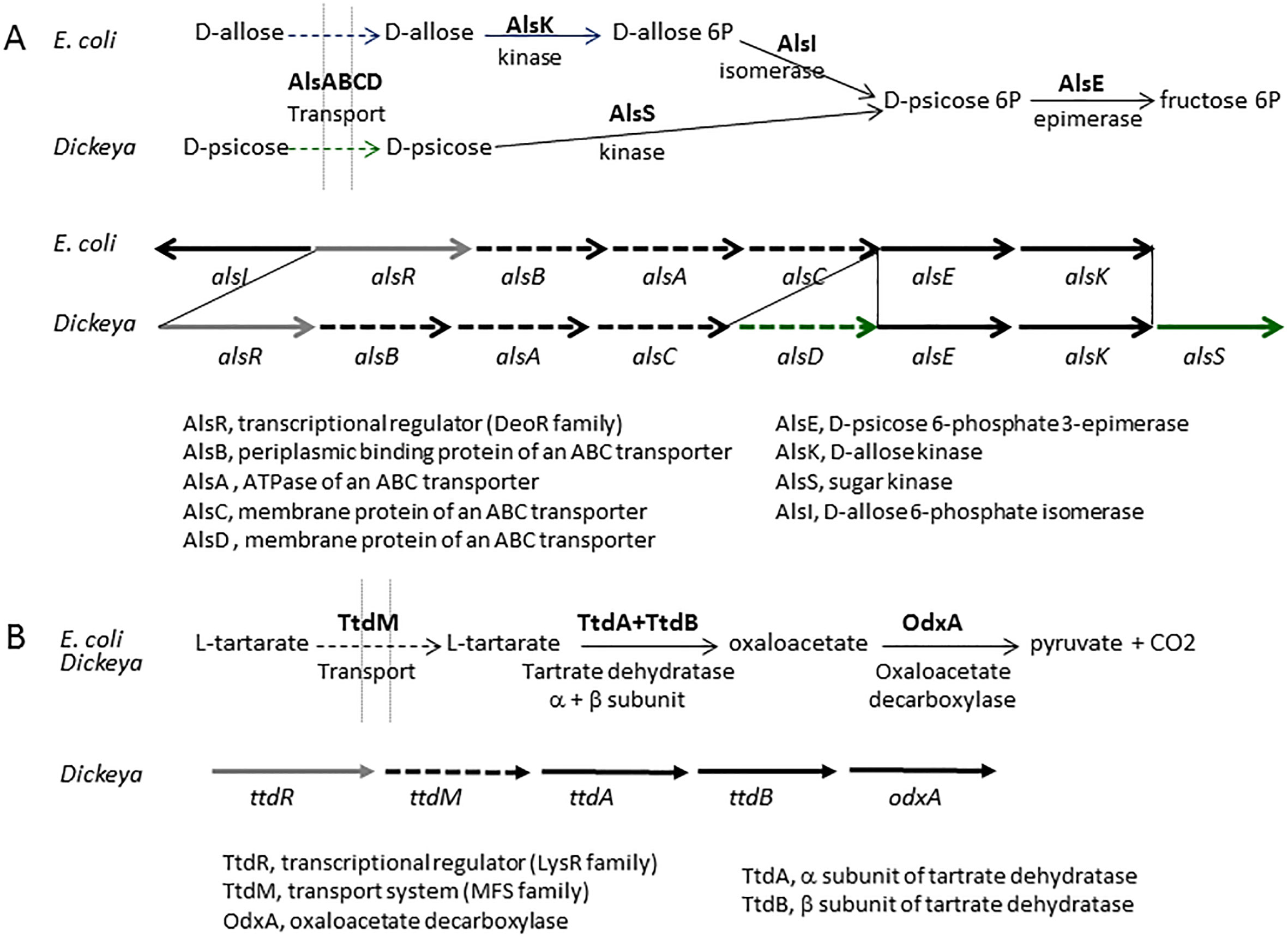
Figure 3 The Dickeya clusters involved in D-pscicose and L-tartarate catabolism. (A) Comparison of the pathways and gene clusters involved in D-allose and D-pscicose utilization in E. coli and Dickeya, respectively. The E. coli cluster involved in D-allose utilization contains the additional gene alsI, encoding the isomerase necessary for D-allose assimilation. The Dickeya cluster involved in D-pscicose utilization includes two additional genes,encoding a second permease (AlsD) and a second sugar kinase (AlsS). The cluster alsRBACDEKS is found in the three Dickeya species able to utilize D-pscicose, D. angzhongdai, D. oryzae, and D. poaceiphila. (B) The L-tartarate catabolic pathway and the Dickeya cluster involved in L-tartarate utilization. The three-step pathway of L-tartarate assimilation is biochemically similar in E. coli and Dickeya. The genes ttdA and ttdB encode the two subunits of tartrate dehydratase. The Dickeya genes ttdR, ttdM and odxA encode a regulator, a transporter and a decarboxylase which belong to different protein families but are predicted to have similar functions. The cluster ttdRMABodxA is found in the different Dickeya species able to utilize L-tartarate, namely D. aquatica, D. fangzhongdai, D. parazeae, D. undicola and D. zeae.
When analyzed for their maceration ability in laboratory conditions, D. fangzhongdai members appear to be more efficient than any other Dickeya species (Alic et al., 2018). In addition to classical PCWDE equipment, the D. fangzhongdai genome contains a gene encoding a pectate lyase of the rare family PL10 (Table 9). PL10 enzymes exhibit catalytic properties similar to those of pectate lyases of the PL1 family such as an alkaline optimal pH, a Ca2+ requirement and a preference for low methylated pectin as substrate (Hugouvieux-Cotte-Pattat et al., 2014). They adopt an (α/α)3 barrel topology in place of the parallel β-helix topology found for PL1 pectate lyases, but the catalytic machinery used by PL10 and PL1 displays a striking resemblance, presumably due to convergent evolution (Charnock et al., 2002).
D. fangzhongdai clearly shows intra-species diversity with ANI values ranging from 96 to 99% between its members. The D. fangzhongdai strains are distributed in three clades in phylogenetic trees (Figure 1). One strain, NCPPB3274, is at the limit of being part of the species, with ANI values of ~96% and dDDH values of 68.6-70.5% with other D. fangzhongdai isolates. The D. fangzhongdai strain S1 carries a 23 kb plasmid similar to an Acidovorax plasmid. Besides genes involved in plasmid replication, stabilization and conjugative transfer, this plasmid contains two streptomycin kinase genes responsible for the resistance to the antibiotic streptomycin (Alic et al., 2019).
D. fangzhongdai is particularly well armed in secondary metabolites. In addition to the clusters zms and sol involved in the biosynthesis of the antibiotic zeamine and the antifungal compound solanimycin, respectively (Table 11), all the D. fangzhongdai genomes possess additional NRPS/PKS clusters (Alic et al., 2019; Van Gijsegem et al., 2021). Two of them are predicted to be involved in the biosynthesis of cyanobactin-related and thiopeptide-related metabolites, respectively. The atypical strain NCPPB3274 harbours the cluster ooc involved in the biosynthesis of an oocydin A-like molecule.
D. undicola, only a water species?
The species D. undicola was only recently described after isolation of three strains from surface waters, one from a lake in Malaysia and two from an irrigation canal in the South of France (Oulghazi et al., 2019) (Table 2). Phylogenetic analyses identified D. fangzhongdai as the closest Dickeya species (Figure 1). Carbon sources utilization analysis identified galactonic acid as a potential discriminative character for distinguishing between these two species (Table 8). The PCWDE repertoire of D. undicola is identical to that of D. dadantii, except that it produces only one polygalacturonase of the pelXVW cluster (Table 9). The genes involved in resistance to stresses encountered during plant infection are shared by all species of the main Dickeya clade, except that D. undicola harbors a truncated flavohemoglobin gene hmpX (Van Gijsegem et al., 2021). D. undicola genomes contain the car locus involved in the synthesis of the β-lactam antibiotic, 1-carbapen-2-em-3-carboxylic acid (carbapenem) as well as genes encoding the carbapenem immunity proteins. This cluster is also found in the water species D. aquatica and D. lacustris, some strains of D. chrysanthemi, D. oryzae and D. zeae (Table 11) and some Pectobacterium species (Van Gijsegem et al., 2021).
Despite they were isolated from far regions, the three D. undicola strains are closely related as they shared ANI and dDDH values higher than 99 and 92%, respectively, but each genome carries hundreds of specific genes (Pedron and Van Gijsegem, 2019). The low number of genomes sequenced, only three, remains insufficient to relate the closeness of the accessory genomes to the geographical origin. Recently, a new D. undicola strain was isolated from carrot in Taiwan (Wei et al., 2021) and we identified as D. undicola a strain from the CFBP collection isolated from onion in 2005 in Spain (Table 2). These two isolates suggest that D. undicola is not only a water species but can also infect plants, perhaps after irrigation with contaminated water. To better understand the D. undicola diversity and habitat, it will clearly be interesting to analyze new strains and to sequence the genomes of isolates from diverse origins.
D. chrysanthemi, the type species of the genus Dickeya
The species D. chrysanthemi is the type species of the genus Dickeya (Samson et al., 2005). The type strain was isolated in 1956 from Chrysanthemum morifolium displaying signs of soft rot and wilt. Other members of the species have been isolated from various plants, mostly Chrysanthemum sp., Parthenium argentatum, carnation, sunflower, tobacco, and from several vegetables including potato, tomato, carrot, eggplant, chicory, and artichoke. Several members were also isolated from surface water (Table 6).
At the phenotypic level, D. chrysanthemi is unable to grow with D-arabinose as the sole carbon source, a feature shared by a few other Dickeya species such as D. dianthicola, D. aquatica, and D. lacustris (Table 8). The D. chrysanthemi PCWDE equipment is quite typical of the genus Dickeya; the genome just lacks the secondary pectinase genes pnlG and pehK, one pel gene of the cluster pelADE and the protease gene prtG (Table 9).
Phylogenomic data suggest that the species D. chrysanthemi contains at least two subclades. Since dDDH values of 69%−70% were observed between most distant members, at the border of the species delimitation; this species clearly includes a high genetic diversity (Table 10). However, although this species has been known for a long time, only eight D. chrysanthemi genome sequences are currently available (Table 6); this is insufficient to propose a new classification in this heterogeneous species.
The Dickeya zeae complex: D. oryzae, D. parazeae, D. zeae and maybe others
Since classification of the strains previously classified as D. zeae has recently evolved, the term “D. zeae complex” was used to describe all these isolates (Hugouvieux-Cotte-Pattat and Van Gijsegem, 2021). In addition to the species D. zeae, the D. zeae complex comprises the species D. oryzae, initially described for a rice isolate (Wang et al., 2020), and D. parazeae whose type strain is a water isolate (Hugouvieux-Cotte-Pattat and Van Gijsegem, 2021). Several genome sequences of strains belonging to the D. zeae complex are avaible in public databases but most of the corresponding strains have not been deposited in collections and are not publicly available for phenotypic analyses, restraining their microbiological characterization.
A high diversity was noticed within genomes of the D. zeae complex which form distinct clades in phylogenetic analyses (Pedron and Van Gijsegem, 2019). The two main clades includes a group of genomes clustering with the D. zeae type strain, and another group clustering with the D. oryzae type strain (Figure 1). It should be noted that the majority of strains previously classified as D. zeae are reclassified as D. oryzae (Table 7; Table S1). In addition, a separate branch is formed by strains of the species D. parazeae. The strain CE1 clusters with the strains PL65 and JZL7 isolated from taro and clivia, respectively (Hu et al., 2018; Boluk et al., 2021) (subclade II, Figure 1). These three strains share dDDH values of about 90%, and values of ~68%, ~65% and ~56% with D. zeae subclade I (Figure 1), D. parazeae and D. oryzae, respectively. Thus, they may correspond to a novel species of the D. zeae complex and, indeed, strain PL65 was recently proposed as the type strain of a new species, Dickeya colocasiae (Boluk et al., 2022), but this classification is not officially recognized. The D. zeae strain A5410, which was isolated from pineapple (Boluk et al., 2021), also forms a separate branch (Figure 1); it shares dDDH similarities of 66−70% to D. oryzae, and 57-58% to D. zeae and D. parazeae. Strain FVG08, isolated from water is another example of strains not clearly positionned in the phylogenetic tree (Figure 1); it is at the limit of belonging to the species D. oryzae with dDDH values of 67.1-69.2% with other D. oryzae strains. These examples highlight the residual heterogeneity of the D. zeae complex. Interesting, the different D. oryzae subclades show some host preference, suggesting an evolution towards host adaptation. The rice strains form a homogeneous subclade also including a millet strain. A second D. oryzae branch brings together mostly maize strains. A third branch includes isolates from water and potato; these later may result from contaminations of potato crops by irrigation water (Hugouvieux-Cotte-Pattat and Van Gijsegem, 2021).
The D. oryzae, D. parazeae and D. zeae strains exhibit high secreted pectinase, protease and cellulase activities (Table 8). Their repertoire of PCWDEs is similar to those of the main Dickeya clade (Table 9). Differences were observed only for accessory pectinases; the genes encoding PnlH, PemB and RhiF are absent in all genomes of the D. zeae complex. The genes encoding PehN and RhiE are absent in D. parazeae and D. oryzae. Some differences in their virulence equipment disclose particularities of rice strains among D. oryzae members. The D. oryzae rice strains possess the zeamine cluster zms, the gene pnlG, and the genes cyt encoding entomotoxins but not the glycopeptidase gene avrL. In contrast, the other D. oryzae strains possess avrL but not zms, pnlG, or cyt. The toxin zeamine was shown to be involved in the rice foot rot disease caused by D. oryzae (Zhou et al., 2011). Despite its name, zeamine is not produced by D. zeae but by D. solani, D. fangzhondai and some D. oryzae strains isolated from rice (Table 11).
A phenotypic difference was observed between D. oryzae and D. zeae for the assimilation of L-tartarate, a compound abundant in many fruits (Table 8) (Hugouvieux-Cotte-Pattat and Van Gijsegem, 2021). Genomic comparison allowed the identification of the gene cluster ttdRMAB-odxA encoding the L-tartarate catabolic pathway in Dickeya (Figure 3B). This pathway was previously described in Salmonella typhimurium and Escherichia coli (Hurlbert and Jakoby, 1965), but the Dickeya cluster encodes proteins of different families sharing the same function, indicating a convergent evolution among Enterobacterales. The six species D. zeae, D. parazeae D. fangzhongdai, D. aquatica, D. lacustris and D. undicola are able to assimilate L-tartarate (Table 8). Their genome contains the cluster ttdRMAB-odxA, confirming the correlation between phenotypic and genomic data.
To reassign strains previously classified as D. zeae, we took advantage of the many available recA sequences and we sequenced the gapA gene from available strains (Table 7). This study also gives information into the host range or habitat of each species. Most D. oryzae isolates come from corn and they are frequently found in potato, rice and water. Most of the D. zeae isolates originate from corn, banana, potato, and water. Similarly, the D. parazeae strains were mostly isolated from corn and water (Table 7). Thus, members of the D. zeae complex are noticeably most common on monocots (corn, rice, banana, pineapple), but also frequent in surface water and in a few dicots, such as potato.
Conclusion
Since the definition of the genus Dickeya and the six first historic species D. chrysanthemi, D. dadantii, D. dianthicola, D. dieffenbachiae, D. paradisiaca, and D. zeae (Samson et al., 2005), mainly based on phenotypes and 16S rRNA comparison, we recently faced numerous assignation modifications and new species occurrence due to sharp genomic comparisons of known Dickeya members, new disease outbreaks, data mining in old collections and enlargement of habitat surveys. Genomic analyses resulted in the re-assignation of D. paradisiaca to the new genus Musicola. They also led to the split of D. zeae into three species and putatively more, given the high diversity among the D. zeae members. The severe outbreak in potato crops in Europe in the 2000s led to the identification of the new species D. solani. Similarly, D. fangzhongdai was described in 2016 after outbreaks in apple trees and in orchids. The atypical species D. poaceiphila was described after analysis of the diversity of old strains present in collections. Surveys of surface waters allowed the characterization of three new species, D. aquatica, D. lacustris and D. undicola, absent or rarely present on plants. New investigations in other environments such as soils but also alternative hosts such as insects or nematodes could also expand the range of Dickeya diversity. It should also be noted that, apart from the Musicola strains isolated almost exclusively in Latin America, international bacterial collections contain very few isolates from Central and South America or Central Africa. Given their climatic particularities, investigations in these underscored geographical locations should also broaden our vision of the Dickeya diversity.
Even if they are usually described as pathogens presenting a large host range, some Dickeya species seem to have a preference for herbaceous monocots. For instance, D. poaceiphila was only isolated from Poaceae and members of D. oryzae, D. parazeae and D. zeae were more often isolated from monocots than from dicots (Tables 2, 7). Similarly, Musicola members were mostly identified on monocots (banana trees) (Table 1). Pectate lyases are critical virulence factors for causing the soft rot symptoms; as these enzymes have no strict substrate specificity (Hugouvieux-Cotte-Pattat et al, 2014) they allow Dickeya to attack a large range of plants. Adaptation to a preferential host may depend on the variety and abundance of PCWDEs. For instance, D. poaceiphila has the poorest PCWDE repertoire among Dickeya members and shows a low production of pectate lyases that would disfavor the breakdown of the cell walls rich in pectin. D. poaceiphila is less well equipped to degrade the high pectin content of dicot cell wall and may be better adapted to monocots whose primary cell wall has low pectin content, such as Poaceae (Jarvis et al., 1988). The inactivation of pectin catabolism in D. poaceiphila is also a strong argument indicating that pectin degradation has become a secondary virulence factor for this species that produces mostly symptoms of chlorosis and wilting.
Genomic analyses are powerful but not suitable to the first screening of a large number of isolates as they need sequencing facilities and remains costly in time and resources. Thus, the phenotypic analysis of simple growth traits should not be forgotten for a preliminary strain classification (Table 8). In addition, the sequencing of a quality marker gene, such as recA or gapA is recommended in order to obtain a reliable taxonomic assignation of newly isolated strains. In any case, a correct classification of phytopathogenic bacteria in genera and species requires many strains of various origins (in terms of host plants, habitats and countries), a phenotypic analysis of these strains and a sufficient number of sequenced genomes to propose a solid phylogeny.
Data availability statement
The original contributions presented in the study are included in the article/Supplementary Material. Further inquiries can be directed to the corresponding author.
Author contributions
NH-C-P – conceptualization of the study, phenotypic analysis, writing, reviewing, and editing of the manuscript. JP – comparative genomics, phylogenetic analysis. FV – genome analysis, writing, reviewing, and editing of the manuscript. All authors contributed to the article and approved the submitted version.
Funding
The work was financially supported by funding of CNRS, University Lyon 1, and INSA Lyon to UMR 5240.
Acknowledgments
We thank Veronique Utzinger for medium preparation and contribution to phenotypic analysis, and the members of the team MTSB (MAP, UMR 5240) for sharing of unpublished information. We also thank Sebastien Santini (CNRS UMR7256) and the PACA Bioinfo platform for the availability and management of the phylogeny.fr website used to construct the recA tree.
Conflict of interest
The authors declare that the research was conducted in the absence of any commercial or financial relationships that could be construed as a potential conflict of interest.
Publisher’s note
All claims expressed in this article are solely those of the authors and do not necessarily represent those of their affiliated organizations, or those of the publisher, the editors and the reviewers. Any product that may be evaluated in this article, or claim that may be made by its manufacturer, is not guaranteed or endorsed by the publisher.
Supplementary material
The Supplementary Material for this article can be found online at: https://www.frontiersin.org/articles/10.3389/fpls.2023.1168480/full#supplementary-material
Supplementary Table 1 | Complete list of D. dadantii, D. dianthicola, D. solani, D. fangzhongdai D. oryzae, and D. zeae strains.
Supplementary Table 2 | List of the genome sequences used in this study with their accession numbers and references.
Abbreviations
NCPPB, National Collection of Plant Pathogenic Bacteria, UK; CFBP, Collection Française de Bacteíries Phytopathogènes, France; IPO, Institute for Plant Protection, Netherlands; PD, Plant Protection Service, Netherlands; IFB, Intercollegiate Faculty of Biotechnology, Poland; SUPP, Shizuoka University of Plant Pathology, Japan; MAFF, Ministry of Agriculture, Forestry and Fisheries, Japan; LMG, Laboratory of Microbiology of Ghent University, Belgium; DSM, DSMZ-German Collection of Microorganisms and Cell Cultures, Germany; ICMP, International Collection of Microorganisms from Plants, New Zealand; PCWDE, plant cell wall degrading enzyme; NRPS, non-ribosomal peptide synthetase; PKS, polyketide synthase.
References
Adeolu, M., Alnajar, S., Naushad, S., Gupta, R. S. (2016). Genome based phylogeny and taxonomy of the ‘Enterobacteriales’: proposal for enterobacterales ord. nov. divided into the families Enterobacteriaceae, erwiniaceae fam. nov., Pectobacteriaceae fam. nov., Yersiniaceae fam. nov., Hafniaceae fam. nov., Morganellaceae fam. nov., and Budviciaceae fam. nov. Int. J. Syst. Evol. Microbiol. 66, 5575–5599. doi: 10.1099/ijsem.0.001485
Alic, S., Pedron, J., Dreo, T., Van Gijsegem, F. (2019). Genomic characterisation of the new dickeya fangzhongdai species regrouping plant pathogens and environmental isolates. BMC Genomics 20, 34. doi: 10.1186/s12864-018-5332-3
Alic, S., Tusek-Znidaric, M., Peterka, M., Ravnikar, M., Dreo, T. (2017). Putative new species of the genus dickeya as major soft rot pathogens in Phalaenopsis orchid production. Plant Pathol. 66, 1357–1368. doi: 10.1111/ppa.12677
Alic, S., Van Gijsegem, F., Pedron, J., Ravnikar, M., Dreo, T. (2018). Diversity within the novel dickeya fangzhongdai sp., isolated from infected orchids, water and pears. Plant Pathol. 67, 1612–1620. doi: 10.1111/ppa.12866
Aono, Y., Nakayama, T., Ogawa, S., Fujimoto, T., Ohki, T., Oka, N., et al. (2022). Asteraceae weeds may be an alternative host of dickeya dianthicola, a causal agent of potato blackleg in Japan. Eur. J. Plant Pathol. 63, 257–268. doi: 10.1007/s10658-022-02474-1
Barco, R. A., Garrity, G. M., Scott, J. J., Amend, J. P., Nealson, K. H., Emerson, D. (2020). A genus definition for bacteria and archaea based on a standard genome relatedness index. mBio 11 (1), e02475–e02419. doi: 10.1128/mBio.02475-19
Ben Moussa, H., Bertrand, C., Rochelle-Newall, E., Fiorini, S., Pedron, J., Barny, M. A. (2022). The diversity of soft rot Pectobacteriaceae along the durance river stream in the south-east of France revealed by multiple seasonal surveys. Phytopathology 112 (8), 1676–1685. doi: 10.1094/phyto-12-21-0515-r
Blin, P., Robic, K., Khayi, S., Cigna, J., Munier, E., Dewaegeneire, P., et al. (2021). Pattern and causes of the establishment of the invasive bacterial potato pathogen Dickeya solani and of the maintenance of the resident pathogen d. dianthicola. Mol. Ecol. 30, 608–624. doi: 10.1111/mec.15751
Boluk, G., Arizala, D., Dobhal, S., Zhang, J., Hu, J., Alvarez, A. M., et al. (2021). Genomic and phenotypic biology of novel strains of dickeya zeae isolated from pineapple and taro in Hawaii: insights into genome plasticity, pathogenicity, and virulence determinants. Front. Plant Sci. 12, 663851. doi: 10.3389/fpls.2021.663851
Boluk, G., Dobhal, S., Arizala, D., Alvarez, A. M., Arif, M. (2022). Dickeya colocasiae sp. nov. isolated from wetland taro, Colocasia esculentum. bioRxiv. doi: 10.1101/2022.01.14.476417
Brady, C. L., Cleenwerck, I., Denman, S., Venter, S. N., Rodríguez-Palenzuela, P., Coutinho, T. A., et al. (2012). Proposal to reclassify Brenneria quercina (Hildebrand & schroth 1967) hauben et al. 1999 into a novel genus, Lonsdalea gen. nov., as Lonsdalea quercina comb. nov., descriptions of Lonsdalea quercina subsp. quercina comb. nov., Lonsdalea quercina subsp. iberica subsp. nov., and Lonsdalea quercina subsp. britannica subsp. nov., emendation of the description of the genus Brenneria, reclassification of Dickeya dieffenbachiae as Dickeya dadantii subsp. dieffenbachiae comb. nov., and emendation of the description of Dickeya dadantii. Int. J. Syst. Evol. Microbiol. 62, 1592–1602. doi: 10.1099/ijs.0.035055-0
Broders, K., Aspin, A., Bailey, J., Chapman, T., Portier, P., Weir, B. S. (2022). Building more resilient culture collections: a call for increased deposits of plant-associated bacteria. Microorganisms 10, 741. doi: 10.3390/microorganisms10040741
Brual, T., Effantin, G., Baltenneck, J., Attaiech, L., Grosbois, C., Royer, M., et al. (2023). A natural single nucleotide mutation in the small regulatory RNA ArcZ of dickeya solani switches off the antimicrobial activities against yeast and bacteria. PloS Genet. 19 (4), e1010725. doi: 10.1371/journal.pgen.1010725
Charkowski, A. O. (2018). The changing face of bacterial soft-rot diseases. Annu. Rev. Phytopathol. 56, 269–288. doi: 10.1146/annurev-phyto-080417-045906
Charkowski, A., Blanco, C., Condemine, G., Expert, D., Franza, T., Hayes, C., et al. (2012). The role of secretion systems and small molecules in soft-rot Enterobacteriaceae pathogenicity. Annu. Rev. Phytopathol. 50, 425–449. doi: 10.1146/annurev-phyto-081211-173013
Charnock, S. J., Brown, I. E., Turkenburg, J. P., Black, G. W., Davies, G. J. (2002). Convergent evolution sheds light on the anti-beta -elimination mechanism common to family 1 and 10 polysaccharide lyases. Proc. Natl. Acad. Sci. U. S. A. 99, 12067–12072. doi: 10.1073/pnas.182431199
Cigna, J., Dewaegeneire, P., Beury, A., Gobert, V., Faure, D. (2017). A gapA PCR-sequencing assay for identifying the dickeya and Pectobacterium potato pathogens. Plant Dis. 101, 1278–1282. doi: 10.1094/PDIS-12-16-1810-RE
Criscuolo, A., Gascuel, O. (2008). Fast NJ-like algorithms to deal with incomplete distance matrices. BMC Bioinf. 9, 166. doi: 10.1186/1471-2105-9-166
Delcher, A. L., Phillippy, A., Carlton, J., Salzberg, S. L. (2002). Fast algorithms for large-scale genome alignment and comparison. Nucleic Acids Res. 30, 2478–2483. doi: 10.1093/nar/30.11.2478
Dereeper, A., Guignon, V., Blanc, G., Audic, S., Buffet, S., Chevenet, F., et al. (2008). Phylogeny.fr: robust phylogenetic analysis for the non-specialist. Nucleic Acids Res. 36, W465–W469. doi: 10.1093/nar/gkn180
Dickey, R. S., Victoria, J. I. (1980). Taxonomy and emended description of strains of erwinia isolated from Musa paradisiaca lineaus. Int. J. Syst. Bacteriol. 30, 129–134. doi: 10.1099/00207713-30-1-129
Dowson, W. J., Hayward, A. C. (1960). The bacterial mottle pathogen of Queensland sugar cane. Intern. Sugar J. 62, 275.
Drula, E., Garron, M. L., Dogan, S., Lombard, V., Henrissat, B., Terrapon, N. (2022). The carbohydrate-active enzyme database: functions and literature. Nucleic Acids Res. 50 (D1), D571–D577. doi: 10.1093/nar/gkab1045
Duprey, A., Taib, N., Leonard, S., Garin, T., Flandrois, J. P., Nasser, W., et al. (2019). The phytopathogenic nature of Dickeya aquatica 174/2 and the dynamic early evolution of dickeya pathogenicity. Environ. Microbiol. 21, 2809–2835. doi: 10.1111/1462-2920.14627
Edgar, R. C. (2004). MUSCLE: a multiple sequence alignment method with reduced time and space complexity. BMC Bioinf. 32, 1792–1797. doi: 10.1186/1471-2105-5-113
Effantin, G., Brual, T., Rahbé, Y., Hugouvieux-Cotte-Pattat, N., Gueguen, E. (2021). Dickeya solani d s0432-1 produces an arsenal of secondary metabolites with anti-prokaryotic and anti-eukaryotic activities against bacteria, yeasts, fungi, and aphids. bioRxiv. doi: 10.1101/2021.07.19.452942
Fujikawa, T., Ota, N., Sasaki, M., Nakamura, T., Iwanami, T. (2019). Emergence of apple bacterial quick decline caused by Dickeya dadantii in Japan. J. Gen. Plant Pathol. 85, 314–319. doi: 10.1007/s10327-019-00852-y
Garlant, L., Koskinen, P., Rouhiainen, L., Laine, P., Paulin, L., Auvinen, P., et al. (2013). Genome sequence of dickeya solani, a new soft rot pathogen of potato, suggests its emergence may be related to a novel combination of non-ribosomal peptide/polyketide synthetase clusters. Diversity 5, 824–842. doi: 10.3390/d5040824
Ge, T., Jiang, H., Tan, E. H., Johnson, S. B., Larkin, R. P., Charkowski, A. O., et al. (2021). Pangenomic analysis of dickeya dianthicola strains related to the outbreak of blackleg and soft rot of potato in USA. Plant Dis. 105, 3946–3955. doi: 10.1094/PDIS-03-21-0587-RE
Glasner, J. D., Yang, C. H., Reverchon, S., Hugouvieux-Cotte-Pattat, N., Condemine, G., Bohin, J. P., et al. (2011). Genome sequence of the plant-pathogenic bacterium dickeya dadantii 3937. J. Bacteriol. 193, 2076–2077. doi: 10.1128/JB.01513-10
Golanowska, M., Potrykus, M., Motyka-Pomagruk, A., Kabza, M., Bacci, G., Galardini, M., et al. (2018). Comparison of highly and weakly virulent Dickeya solani strains, with a view on the pangenome and panregulon of this species. Front. Microbiol. 9, 1940. doi: 10.3389/fmicb.2018.01940
Goris, J., Konstantinidis, K. T., Klappenbach, J. A., Coenye, T., Vandamme, P., Tiedje, J. M. (2007). DNA–DNA Hybridization values and their relationship to whole genome sequence similarities. Int. J. Syst. Evol. Microbiol. 57, 81–91. doi: 10.1099/ijs.0.64483-0
Guindon, S., Gascuel, O. (2003). A simple, fast and accurate algorithm to estimate larges phylogenies by maximum likelihood. Syst. Biol. 52, 696–704. doi: 10.1080/10635150390235520
Hélias, V., Hamon, P., Huchet, E., van der Wolf, J., Andrivon, D. (2012). Two new effective semiselective crystal violet pectate media for isolation of pectobacterium and Dickeya: isolating pectolytic bacteria on CVP. Plant Pathol. 61, 339–345. doi: 10.1111/j.1365-3059.2011.02508.x
Hu, M., Li, J., Chen, R., Li, W., Feng, L., Shi, L., et al. (2018). Dickeya zeae strains isolated from rice, banana and clivia rot plants show great virulence differentials. BMC Microbiol. 18, 136. doi: 10.1186/s12866-018-1300-y
Hugouvieux-Cotte-Pattat, N., Brochier-Armanet, C., Flandrois, J. P., Reverchon, S. (2020). Dickeya poaceaphila sp. nov., a plant-pathogenic bacterium isolated from sugar cane (Saccharum officinarum). Int. J. Syst. Evol. Microbiol. 70, 4508–4514. doi: 10.1099/ijsem.0.004306
Hugouvieux-Cotte-Pattat, N., Condemine, G., Nasser, W., Reverchon, S. (1996). Regulation of pectinolysis in erwinia chrysanthemi. Annu. Rev. Microbiol. 50, 213–257. doi: 10.1146/annurev.micro.50.1.213
Hugouvieux-Cotte-Pattat, N., Condemine, G., Shevchik, V. E. (2014). Bacterial pectate lyases, structural and functional diversity. Environ. Microbiol. Rep. 6, 427–440. doi: 10.1111/1758-2229.12166
Hugouvieux-Cotte-Pattat, N., des-Combes, C. J., Briolay, J., Pritchard, L. (2021). Proposal for the creation of a new genus musicola gen. nov., reclassification of Dickeya paradisiaca (Samson et al. 2005) as Musicola paradisiaca comb. nov. and description of a new species Musicola keenii sp. nov. Int. J. Syst. Evol. Microbiol. 71, 005037. doi: 10.1099/ijsem.0.005037
Hugouvieux-Cotte-Pattat, N., Jacot-des-Combes, C., Briolay, J. (2019). Dickeya lacustris sp. nov., a water-living pectinolytic bacterium isolated from lakes in France. Int. J. Syst. Evol. Microbiol. 69, 721–726. doi: 10.1099/ijsem.0.003208
Hugouvieux-Cotte-Pattat, N., Royer, M., Gueguen, E., Le Guen, P., Süssmuth, R. D., Reverchon, S., et al. (2022). Allelic variations within the vfm locus define quorum sensing specificity groups in plant pathogenic bacteria of the genus Dickeya. Environ. Microbiol. 24, 1467–1483. doi: 10.1111/1462-2920.15889
Hugouvieux-Cotte-Pattat, N., Van Gijsegem, F. (2021). Diversity within the Dickeya zeae complex, identification of dickeya zeae and Dickeya oryzae members, proposal of the novel species Dickeya parazeae sp. nov. Int. J. Syst. Evol. Microbiol. 71, 005059. doi: 10.1099/ijsem.0.005059
Hurlbert, R. E., Jakkoby, W. B. (2022). Tartaric acid metabolism. I. Subunits of L(+)-tartaric acid dehydrase. J. Biol. Chem. 240, 2772–2777. doi: 10.1016/0926-6569(64)90298-6
Jarvis, M. C., Forsyth, W., Duncan, H. J. (1988). A survey of the pectic content of nonlignified monocot cell walls. Plant Physiol. 88, 309–314. doi: 10.1104/pp.88.2.309
Kano, A., Hosotani, K., Gomi, K., Yamasaki-Koduko, Y., Shirakawa, C., Fukumoto, T., et al. (2011). D-psicose induces upregulation of defense-related genes and resistance in rice against bacterial blight. J. Plant Physiol. 168, 1852–1857. doi: 10.1016/j.jplph.2011.04.003
Khayi, S., Blin, P., Pedron, J., Chong, T. M., Chan, K. G., Moumni, M., et al. (2015). Population genomics reveals additive and replacing horizontal gene transfers in the emerging pathogen Dickeya solani. BMC Genom. 16, 788. doi: 10.1186/s12864-015-1997-z
Khayi, S., Chan, K. G., Faure, D. (2022). Patterns of genomic variations in the plant pathogen dickeya solani. Microorganisms 10, 2254. doi: 10.3390/microorganisms10112254
Liu, Y., Tyler, C., Helmann, T. C., Stodghill, P., Filiatrault, M. J. (2021). Complete genome sequence resource for the necrotrophic plant pathogenic bacterium Dickeya dianthicola 67-19 isolated from new Guinea impatiens. Plant Dis. 105, 1174–1176. doi: 10.1094/PDIS-09-20-1968-A
Mansfield, J., Genin, S., Magori, S., Citovky, V., Sriariyanum, M., Ronald, P., et al. (2012). Top 10 plant pathogenic bacteria in molecular plant pathology. Mol. Plant Pathol. 13, 614–629. doi: 10.1111/j.1364-3703.2012.00804.x
Matilla, M. A., Monson, R. E., Murphy, A., Schicketanz, M., Rawlinson, A., Duncan, C., et al. (2022). Solanimycin: biosynthesis and distribution of a new antifungal antibiotic regulated by two quorum-sensing systems. mBio 13 (6), e0247222. doi: 10.1128/mbio.02472-22
Meier-Kolthoff, J. P. M., Auch, A. F., Klenk, H. P., Göker, M. (2013). Genome sequence-based species delimitation with confidence intervals and improved distance functions. BMC Bioinf. 14, 60. doi: 10.1186/1471-2105-14-60
Nasser, W., Dorel, C., Wawrzyniak, J., Van Gijsegem, F., Groleau, M. C., Déziel, E., et al. (2013). Vfm a new quorum sensing system controls the virulence of Dickeya dadantii. environ. Microbiol 15, 865–880. doi: 10.1111/1462-2920.12049
Ossowska, K., Czerwicka, M., Sledz, W., Zoledowska, S., Motyka, A., Golanowska, M., et al. (2017). The uniform structure of O-polysaccharides isolated from dickeya solani strains of different origin. Carbohydr. Res. 445, 40–43. doi: 10.1016/j.carres.2017.04.001
Oulghazi, S., Pedron, J., Cigna, J., Lau, Y. Y., Moumni, M., Van Gijsegem, F., et al. (2019). Dickeya undicola sp. nov., a novel species for pectinolytic isolates from surface waters in Europe and Asia. Int. J. Syst. Evol. Microbiol. 69, 2440–2444. doi: 10.1099/ijsem.0.003497
Parkinson, N., DeVos, P., Pirhonen, M., Elphinstone, J. (2014). Dickeya aquatica sp. nov., isolated from waterways. Int. J. Syst. Evol. Microbiol. 64, 2264–2266. doi: 10.1099/ijs.0.058693-0
Parkinson, N., Stead, D., Bew, J., Heene, J., Tsror Lahkim, L., Elphinstone, J. (2009). Dickeya species relatedness and clade structure determined by comparison of recA sequences. Int. J. Syst. Evol. Microbiol. 59, 2388–2393. doi: 10.1099/ijs.0.009258-0
Pedron, J., Mondy, S., Raoul des Essarts, Y., Van Gijsegem, F., Faure, D. (2014). Genomic and metabolic comparison with Dickeya dadantii 3937 reveals the emerging dickeya solani potato pathogen to display distinctive metabolic activities and T5SS/T6SS-related toxin repertoire. BMC Genom. 15, 283. doi: 10.1186/1471-2164-15-283
Pedron, J., Schaerer, S., Kellenberger, I., Van Gijsegem, F. (2021). Early emergence of dickeya solani revealed by analysis of Dickeya diversity of potato blackleg and soft rot causing pathogens in Switzerland. Microorganisms 9, 1187. doi: 10.3390/microorganisms9061187
Pedron, J., van der Wolf, J. M., Portier, P., Caullireau, E., Van Gijsegem, F. (2022). The broad host range plant pathogen dickeya dianthicola shows a high genetic diversity. Microorganisms 10, 1024. doi: 10.3390/microorganisms10051024
Pedron, J., Van Gijsegem, F. (2019). Diversity in the bacterial genus dickeya grouping plant pathogens and waterways isolates. OBM Genet. 3 (4), 098. doi: 10.21926/obm.genet.1904098
Portier, P., Pedron, J., Taghouti, G., Dutrieux, C., Barny, M.-A. (2020). Updated taxonomy of pectobacterium genus in the CIRM-CFBP bacterial collection: when newly described species reveal “old” endemic population. Microorganisms 8, 1441. doi: 10.3390/microorganisms8091441
Potrykus, M., Golanowska, M., Sledz, W., Zoledowska, S., Motyka, A., Kolodziejska, A., et al. (2016). Biodiversity of dickeya spp. isolated from potato plants and water sources in temperate climate. Plant Dis. 100, 408–417. doi: 10.1094/PDIS-04-15-0439-RE
Pritchard, L., Glover, R. H., Humphris, S., Elphinstone, J. G., Toth, I. K. (2016). Genomics and taxonomy in diagnostics for food security: soft-rotting enterobacterial plant pathogens. Anal. Methods 8, 12–24. doi: 10.1039/C5AY02550H
Pritchard, L., Humphris, S., Saddler, G. S., Elphinstone, J. G., Pirhonen, M., Toth, I. K. (2013). Draft genome sequences of 17 isolates of the plant pathogenic bacterium Dickeya. Genome Announc 1 (6), e00978–e00913. doi: 10.1128/genomeA.00978-13
Reverchon, S., Muskhelisvili, G., Nasser, W. (2016). Virulence program of a bacterial plant pathogen: the dickeya model. Prog. Mol. Biol. Transl. Sci. 142, 51–92. doi: 10.1016/bs.pmbts.2016.05.005
Richter, M., Rosselló-Móra, R. (2009). Shifting the genomic gold standard for the prokaryotic species definition. Proc. Natl. Acad. Sci. U.S.A. 106, 19126–19131. doi: 10.1073/pnas.0906412106
Samson, R., Legendre, J. B., Christen, R., Fischer-Le Saux, M., Achouak, W., Gardan, L. (2005). Transfer of Pectobacterium chrysanthemi (Burkholder et al 1953) Brenner et al. 1973 and Brenneria paradisiaca to the genus Dickeya gen. nov. as Dickeya chrysanthemi comb. nov. and Dickeya paradisiaca comb. nov. and delineation of four novel species, Dickeya dadantii sp. nov., Dickeya dianthicola sp. nov., Dickeya dieffenbachiae sp. nov. and Dickeya zeae sp. nov. Int. J. Syst. Evol. Microbiol. 55, 1415–1427. doi: 10.1099/ijs.0.02791-0
Slawiak, M., van Beckhoven, J. R. C. M., Speksnijde, A. G. C. L., Czajkowski, R. L., Grabe, G., van der Wolf, J. M. (2009). Biochemical and genetical analysis reveal a new clade of biovar 3 dickeya spp. strains isolated from potato in Europe. Eur. J. Plant Pathol. 125, 245–261. doi: 10.1007/s10658-009-9479-2
Steindl, D. R. L. (1964). “Bacterial mottle,” in Sugar cane diseases of the world. Eds. Hughes, C. G., Abbott, E. V., Wismer, C. A. (New York: Elsevier Publishing Company) 2, 2–11.
Suharjo, R., Sawada, H., Takikawa, Y. (2014). Phylogenetic study of Japanese dickeya spp. and development of new rapid identification methods using PCR–RFLP. J. Gen. Plant Pathol. 80, 237–254. doi: 10.1007/s10327-014-0511-9
Tan, C., Li, C., Hu, M., Hu, A., Xue, Y., Zhou, X., et al. (2022). Comparative pathogenomic analysis of two banana pathogenic Dickeya strains isolated from China and the Philippines. Int. J. Mol. Sci. 23, 12758. doi: 10.3390/ijms232112758
Tian, Y., Zhao, Y., Yuan, X., Yi, J., Fan, J., Xu, Z., et al. (2016). Dickeya fangzhongdai sp. nov., a plant-pathogenic bacterium isolated from pear trees (Pyrus pyrifolia). Int. J. Syst. Evol. Microbiol. 66, 2831–2835. doi: 10.1099/ijsem.0.001060
Toth, I. K., Barny, M., Brurberg, M. B., Condemine, G., Czajkowski, R., Elphinstone, J. G., et al. (2021). “Pectobacterium and dickeya: environment to disease development,” in Plant diseases caused by dickeya and pectobacterium species. Eds. Van Gijsegem, F., van der Wolf, J. M., Toth, I. K. (Cham, Switzerland: Springer International Publishing), 39–84.
Toth, I. K., van der Wolf, J. M., Saddler, G., Lojkowska, E., Hélias, V., Pirhonen, M., et al. (2011). Dickeya species: an emerging problem for potato production in Europe. Plant Pathol. 60, 385–399. doi: 10.1111/j.1365-3059.2011.02427.x
van der Wolf, J., Acuña, I., De Boer, S. H., Brurberg, M. B., Cahill, G., Charkowski, A. O., et al. (2021). “Diseases caused by pectobacterium and dickeya species around the world,” in Plant diseases caused by dickeya and pectobacterium species. Eds. Van Gijsegem, F., van der Wolf, J. M., Toth, I. K. (Cham, Switzerland: Springer International Publishing), 215–270.
van der Wolf, J. M., Nijhuis, E. H., Kowalewska, M. J., Saddler, G. S., Parkinson, N., Elphinstone, J. G., et al. (2014). Dickeya solani sp. nov., a pectinolytic plant-pathogenic bacterium from potato (Solanum tuberosum). Int. J. Syst. Evol. Microbiol. 64, 768–774. doi: 10.1099/ijs.0.052944-0
Van Gijsegem, F., Hugouvieux-Cotte-Pattat, N., Kraepiel, Y., Lojkowska, E., Moleleki, L. N., Gorshkov, V., et al. (2021). “Molecular interactions of pectobacterium and dickeya with plants,” in Plant diseases caused by dickeya and pectobacterium species. Eds. Van Gijsegem, F., van der Wolf, J. M., Toth, I. K. (Cham, Switzerland: Springer International Publishing), 85–147.
Van Vaerenbergh, J., Baeyen, S., De Vos, P., Maes, M. (2012). Sequence diversity in the dickeya fliC gene: phylogeny of the Dickeya genus and TaqMan PCR for ‘D. solani’, new biovar 3 variant on potato in Europe. PloS One 7, e35738. doi: 10.1371/journal.pone.0035738
Wang, X., He, S. W., Guo, H. B., Han, J. G., Thin, K. K., Gao, J. S., et al. (2020). Dickeya oryzae sp. nov., isolated from the roots of rice. Int. J. Syst. Evol. Microbiol. 70, 4171–4178. doi: 10.1099/ijsem.0.004265
Wei, X.-Y., Deng, W.-L., Chu, C.-C. (2021). Phylogenetic and phenotypic analyses on dickeya spp. isolated from different host plants in Taiwan. J. Phytopathol. 169, 678–691. doi: 10.1111/jph.13038
Wright, D., Bwye, A., Banovic, M., Baulch, J., Wang, C., Hair, S., et al. (2018). First report of dickeya dianthicola in potatoes in Australia. Plant Dis. 102, 2029. doi: 10.1094/PDIS-01-18-0094-PDN
Zaczek-Moczydłowska, M. A., Fleming, C. C., Young, G. K., Campbell, K., Hanlon, R. O. (2019). Pectobacterium and Dickeya species detected in vegetables in northern Ireland. Eur. J. Plant Pathol. 154, 635–647. doi: 10.1007/s10658-019-01687-1
Keywords: Pectobacteriaceae, soft-rot, Musicola, Dickeya, phytopathogens, pectinases
Citation: Hugouvieux-Cotte-Pattat N, Pédron J and Van Gijsegem F (2023) Insight into biodiversity of the recently rearranged genus Dickeya. Front. Plant Sci. 14:1168480. doi: 10.3389/fpls.2023.1168480
Received: 17 February 2023; Accepted: 22 May 2023;
Published: 20 June 2023.
Edited by:
Vittoria Catara, University of Catania, ItalyReviewed by:
David J. Studholme, University of Exeter, United KingdomAgata Motyka-Pomagruk, Intercollegiate Faculty of Biotechnology of University of Gdańsk and Medical University of Gdańsk, Poland
Copyright © 2023 Hugouvieux-Cotte-Pattat, Pédron and Van Gijsegem. This is an open-access article distributed under the terms of the Creative Commons Attribution License (CC BY). The use, distribution or reproduction in other forums is permitted, provided the original author(s) and the copyright owner(s) are credited and that the original publication in this journal is cited, in accordance with accepted academic practice. No use, distribution or reproduction is permitted which does not comply with these terms.
*Correspondence: Nicole Hugouvieux-Cotte-Pattat, bmljb2xlLmNvdHRlLXBhdHRhdEBpbnNhLWx5b24uZnI=