- 1State Key Laboratory of Plant Physiology and Biochemistry, College of Life Sciences, Zhejiang University, Hangzhou, China
- 2Hainan Institute, Zhejiang University, Yazhou Bay Science and Technology City, Sanya, Hainan, China
Nitrogen (N) and phosphorus (P) are two primary components of fertilizers for crop production. Coordinated acquisition and utilization of N and P are crucial for plants to achieve nutrient balance and optimal growth in a changing rhizospheric nutrient environment. However, little is known about how N and P signaling pathways are integrated. We performed transcriptomic analyses and physiological experiments to explore gene expression profiles and physiological homeostasis in the response of rice (Oryza sativa) to N and P deficiency. We revealed that N and P shortage inhibit rice growth and uptake of other nutrients. Gene Ontology (GO) analysis of differentially expressed genes (DEGs) suggested that N and Pi deficiency stimulate specific different physiological reactions and also some same physiological processes in rice. We established the transcriptional regulatory network between N and P signaling pathways based on all DEGs. We determined that the transcript levels of 763 core genes changed under both N or P starvation conditions. Among these core genes, we focused on the transcription factor gene NITRATE-INDUCIBLE, GARP-TYPE TRANSCRIPTIONAL REPRESSOR 1 (NIGT1) and show that its encoded protein is a positive regulator of P homeostasis and a negative regulator of N acquisition in rice. NIGT1 promoted Pi uptake but inhibited N absorption, induced the expression of Pi responsive genes PT2 and SPX1 and repressed the N responsive genes NLP1 and NRT2.1. These results provide new clues about the mechanisms underlying the interaction between plant N and P starvation responses.
1 Introduction
Phosphorus (P) and nitrogen (N) are two of the most important essential mineral elements for plant growth and are also the most widely used components of fertilizers that cause environmental pollution. The uptake and utilization of N and P by plants are often mutually affected (Gusewell, 2004), with changes in the N:P supply ratio significantly affecting their absorption (Luo et al., 2016). Inorganic phosphate (Pi) and nitrate () and ammonium () are the main forms of P and N taken up by most plant species. Plants have evolved similar regulatory networks to adapt to soil environments with different P and N nutrient contents, known as Pi and N starvation responses, respectively. The underlying pathways involve inducing the expression of genes encoding transporters, recycling internal P and N pools, changing root system architecture, and interacting with soil microbes (Bouain et al., 2019, Li et al., 2021b). Previous studies have mainly focused on P and N signal transduction pathways separately (Wang et al., 2018, Li et al., 2021b, Lu et al., 2022; Paz-Ares et al., 2022), and very few have examined the mutual regulation of the uptake and utilization of these two nutrient elements.
Recent studies have revealed several genes that regulate P–N interactions in plants. Arabidopsis (Arabidopsis thaliana) NITROGEN LIMITATION ADAPTATION (NLA) encodes an E3 ligase that regulates the protein stability of the nitrate transporter NRT1/PTR FAMILY 2.13 (NPF2.13, also named NITRATE TRANSPORTER 1.7 [NRT1.7]) and PHOSPHATE TRANSPORTER 1 (PHT1), resulting in N-dependent P accumulation in shoots (Kant et al., 2011; Lin et al., 2013; Park et al., 2014; Liu et al., 2017). Rice (Oryza sativa) NLA1 mediates the degradation of the phosphate transporters PT2 and PT8 to regulate P acquisition (Yang et al., 2017; Yue et al., 2017). Rice NITRATE-INDUCIBLE, GARP-TYPE TRANSCRIPTIONAL REPRESSOR 1 (NIGT1) is a nitrate-induced GARP-type transcription factor (TF) that binds 5′-GAATC-3′ and 5′-GATATTC-3′ sequences to regulate the expression of downstream genes (Sawaki et al., 2013); however, its biological function has not been studied. Arabidopsis contains four NIGT1 homologs that affect root growth and plant P and N uptake by regulating the expression of SPX1 (SYG1/Pho81/XPR1), PHT, and NRT genes (Medici et al., 2015; Kiba et al., 2018; Maeda et al., 2018, Ueda et al., 2020a; Wang et al., 2020b). In addition, studies have shown that rice NRT1.1B can interact with SPX4 to promote its degradation under high nitrate conditions, thereby relieving the inhibition by SPX4 of nuclear translocation for the Pi signaling regulator PHOSPHATE STARVATION RESPONSE 2 (PHR2) and the N signaling regulator NIN-LIKE PROTEIN 3 (NLP3) (Hu et al., 2019). Studies in Arabidopsis have shown that Pi deficiency promotes ammonium assimilation mediated by AMT1 transporters, accompanied by H+ expulsion, activates STOP1 (SENSITIVE TO PROTON RHIZOTOXICITY 1) and increases organic acid secretion, thereby releasing Pi from Fe (and Al) containing complexes (Tian et al., 2021). At the same time, STOP1 can directly bind to the promoter of NRT1.1 to activate its transcription at low pH, therefore promote uptake (Ye et al., 2021).
In response to low external P and N, plants undergo global physiological changes and transcriptome reprogramming (Ueda et al., 2020c). Transcriptome analysis is a rapid and effective method for studying regulatory networks, allowing us to understand plant stress responses comprehensively and identify potential functional genes, including TF genes and their targets (Ueda et al., 2020c, Nie et al., 2021; Yan et al., 2021; Li et al., 2022). For example, time-series transcriptome analyses revealed CYTOKININ RESPONSE FACTOR 4 (CRF4) as an early player in mediating the N response in Arabidopsis (Varala et al., 2018). Comparative omics analysis also provides a new approach for completely understanding the mechanisms underlying specific physiological responses (Liang et al., 2022; Li et al., 2022; Zhou et al., 2022). Although several genes associated with N and P acquisition have been identified in rice, the precise molecular mechanism behind N–P interaction remains elusive.
In this study, we conducted combined transcriptomic analyses of rice roots responding to N or Pi deficiency to identify genes associated with both N and P homeostasis in rice. Our results highlighted many genes shared by the N and P starvation responses. We identified a list of candidate core genes that may coordinate N and P responses and metabolisms. Furthermore, we functionally identified rice NIGT1 as one such integrator of N and P homeostasis.
2 Materials and methods
2.1 Plant materials and growth conditions
Two wild-type japonica rice (Oryza sativa L.) cultivars, ‘Nipponbare’ and ‘Hei Jing 2’ (HJ2), were used in this study. Nipponbare plants were used for transcriptome analysis. All transgenic rice lines were produced in the HJ2 background. Rice plants were grown hydroponically in a growth chamber at 30/22°C (day/night), 60 to 70% humidity, with a light intensity of ~300 μmol m–2 s–1 provided by a bulb-type light and a photoperiod of 14 h light/10 h dark, as previously described (Wang et al., 2020a). The nutrient solution was adjusted to pH 5.5 using 1 M HCl or NaOH. Concentrations of KH2PO4 and NH4NO3 were adjusted to 200/1,400, 10/1,400, 0/1,400, 200/70, and 200 μM/0 μM for control (CK), low-P (LP), P deficiency (–P), low-N (LN), and N deficiency (–N) experimental conditions, respectively. The other macronutrients are K2SO4 (191.5 μM), CaCl2·2H2O (366 μM) and MgSO4·7H2O (547 μM). The nutrient solution also contained the following micronutrients: MnCl2·4H2O (0.0005 μM), H3BO3 (0.003 μM), (NH4)6Mo7O24·4H2O (0.0001 μM), ZnSO4·7H2O (0.0004 μM), CuSO4·5H2O (0.0002μM) and NaFe(3)-EDTA (40 μM).
2.2 Nutrient content measurements
All plants (the wild type and T2 transgenic lines) were harvested after treatment, separated into roots and shoots, and then dried at 70°C for 3 d. Tissues were weighed and digested with 98% H2SO4 (v/v) and 30% H2O2 (v/v) at 300°C. Total N content was determined using the Kjeldahl N assay (Xia et al., 2015). Both N and P determination were conducted using a continuous flow analyzer (SKALAR, SKALAR San plus system) as previously described (Wang et al., 2020a). Potassium (K), calcium (Ca), and magnesium (Mg) concentrations were determined by inductively coupled plasma–optical emission spectrometry (Yang et al., 2016).
2.3 RNA-seq and gene expression analysis
Fourteen-day-old Nipponbare seedlings cultured in full-strength nutrient solution were transferred to –N and –P solutions and cultured for a further 7 d. Roots from three to four independent plants were harvested, pooled together as one biological replicate, and used for RNA-seq. Three biological repeats were performed per treatment. RNA-seq experiments were performed by Novogene Bioinformatics Technology Co. Ltd (China). Total RNA was extracted using TRIzol reagent (Invitrogen). mRNA libraries were constructed according to the Illumina instructions and sequenced using a HiSeq 2500 instrument; all paired-end reads were mapped to the rice cv Nipponbare genome (http://ftp.ensemblgenomes.org/pub/release-24/plants/fasta/oryza_sativa/dna/Oryza_sativa.IRGSP-1.0.24.dna.toplevel.fa.gz) using TopHat (v2.0.12). All raw sequence read data were uploaded to the NCBI Sequence Read Archive under accession number PRJNA925220.
The DESeq R package (v1.10.1) was used for analyzing differentially expressed genes (DEGs). Thresholds were set as a false discovery rate (FDR) adjusted P < 0.05 (P values were adjusted using the Benjamini and Hochberg method) and an absolute value of Log2(fold-change) > 1 to determine significant differences in gene expression. Clustered genes were assigned to biological process categories based on Gene Ontology (GO) analysis using the web tool THE GENE ONTOLOGY RESOURCE (http://geneontology.org/). GO terms with FDR < 0.05 were considered significantly enriched for DEGs. Heatmaps of DEGs were visualized using TBtools (Chen et al., 2020). TF IDs were downloaded from the database PlantTFDB (http://planttfdb.gao-lab.org/). Predicted regulatory networks between enriched TFs and their potential target genes were identified using the PlantRegMap database (http://plantregmap.gao-lab.org/tf_enrichment.php).
2.4 RT‐qPCR analysis
Total RNA was isolated from rice samples using TRIzol reagent (Invitrogen). First-strand cDNA was synthesized from 1 µg of total RNA using a reverse transcription system (A3500; Promega). qPCR was performed using SYBR Green I Master (Roche) on a LightCycler 480 Real-Time PCR system (Roche) according to the manufacturer’s instructions. Relative expression levels were normalized to those of the housekeeping gene ACTIN1 (Os03g0718100), as previously described (Wang et al., 2020a). Primers used for qPCR are listed in Table S1.
2.5 Vector construction and generation of transgenic plants
To generate nigt1 knockout mutants, two specific targets at different locations in the NIGT1 genomic sequence were selected for creating single guide RNAs (sgRNAs). The target sequences were ligated into the pYLCRISPR/gRNA vector, followed by ligation into the pYLCRISPR/Cas9-MH vector as described (Shao et al., 2019). To generate 35S::NIGT1-GFP overexpression lines, the full-length NIGT1 (1236 bp) coding sequence was amplified by PCR from cDNA using primer pair NIGT-GFP-F and NIGT-GFP-R and introduced into a modified pCAMBIA1300-35S-eGFP plasmid using BamHI and XbaI restriction sites downstream of the 35S promoter. All constructs were confirmed by sequencing. All transgenic plants were produced via Agrobacterium (Agrobacterium tumefaciens) (strain EHA105)-mediated transformation. Primers used for vector construction are listed in Table S1.
2.6 Subcellular localization
35S::NIGT1-GFP was transiently expressed into Nicotiana benthamiana leaves by Agrobacterium-mediated infiltration. Fluorescence in roots of 7-d-old transgenic rice seedlings harboring the 35S::NIGT1-GFP transgene was observed using a confocal laser-scanning microscope (Axiovert LSM 710; Zeiss). A×25 water immersion objective was used for confocal imaging. Fluorescent proteins were excited at 488 nm using an argon ion laser. Fluorescence was detected from 493 to 542 nm.
2.7 Statistical analyses
Statistical analysis was conducted using the program SPSS Statistics v22.0 (IBM).
3 Results
3.1 Nitrogen or phosphate deficiency affects rice growth and N/P homeostasis
To confirm the effects of N and Pi deficiency on rice development, we cultured wild-type Nipponbare seedlings in normal nutrient solution (CK) for 14 d and then transferred them to CK, –P (no Pi), or –N (no N) solutions for another 14 d. Both –P and –N conditions inhibited rice shoot growth but promoted root elongation (Figures 1A, B) and resulted in a lower overall biomass. Furthermore, N deficiency led to a relatively more severe growth inhibition than P deficiency (Figure 1C). The SPAD value (for evaluating chlorophyll content) of rice leaves treated with –N was lower than that for the CK treatment, consistent with the yellowish leaves of N-deficient rice seedlings (Figure 1A and Figure S1A). Moreover, the N content of rice was lowest under N deficiency, and the P content was lowest under Pi deficiency, validating the deficiency conditions (Figures 1D, E). Furthermore, the P and N concentrations in shoots or roots of rice plants grown under either –N or –P conditions were lower than those of rice plants grown under normal conditions (Figures 1D, E). Plants grown under N deficiency showed lower K concentrations than those under CK conditions; plants grown under Pi deficiency displayed lower concentrations of K, Ca, and Mg (Figures S1B-D). These results indicate the mutual influence of N uptake and P acquisition, which is critical for optimizing plant growth under diverse nutrient conditions.
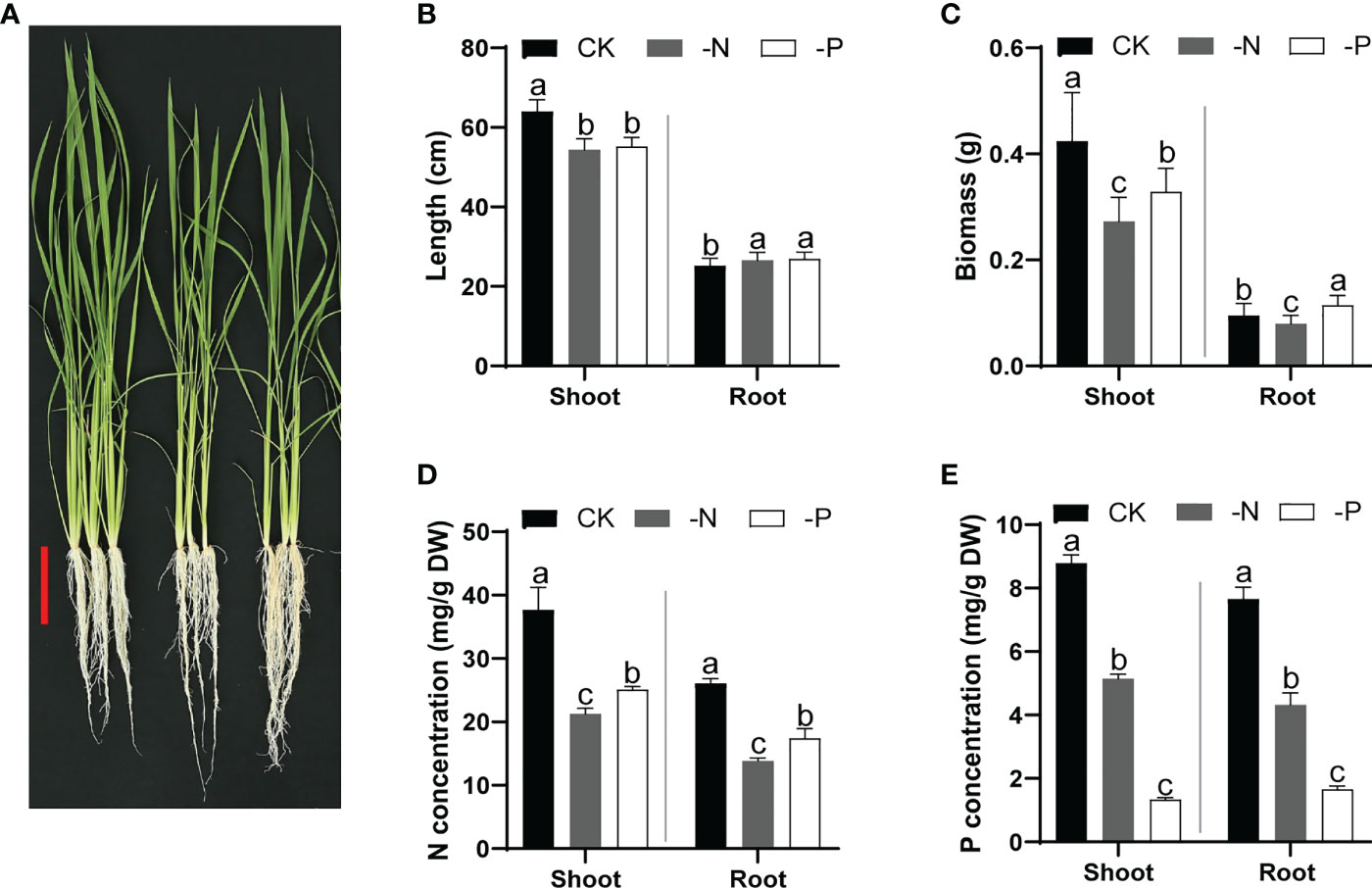
Figure 1 Growth performance and N/P content of rice plants cultured under different nutrient conditions. (A) Growth phenotypes of wild-type Nipponbare in different nutrient solutions. Fourteen-day-old seedlings cultured in replete nutrient solution were transferred to –N or –P solutions and cultured for another 14 d. Scale bar, 10 cm. (B–E) Shoot and root length (B), biomass (C), N concentration (D), and P concentration (E) of rice seedlings shown in (A) Data are means + SD (n = 30 in (B, C), n = 4 in (D, E)). Different lowercase letters indicate significant differences between groups (ANOVA followed by Tukey’s test, P < 0.05).
3.2 Profiling the transcriptional response to nitrogen and phosphate deficiency
To obtain molecular insight into the transcriptional changes in response to N and P availability, we performed transcriptome deep sequencing (RNA-seq) on rice root tissues harvested after 7 d of treatment with N or Pi deficiency. The number of differentially expressed genes (DEGs) between the –N treatment and the control (CK) was much higher than that between the –P treatment and the CK treatment (2,205 vs. 1,306), with an adjusted P value (p-adj) < 0.05 and |Log2(fold-change)| ≥ 1 as cutoffs (Figure 2A). More DEGs were downregulated than upregulated in –N conditions relative to CK (1,147 vs. 1,058; Figure 2A; Dataset S1), while we detected more upregulated DEGs than downregulated DEGs in –P conditions compared to CK (744 vs. 562; Figure 2A; Dataset S2). DEGs triggered by –N or –P included known P or N starvation responsive genes, such as SPX1 (Os06g0603600) (Wang et al., 2009), PT2 (Os03g0150800) (Ai et al., 2009), PT8 (Os10g0444700) (Jia et al., 2011), NRT2.1 (Os02g0112100) (Takayanagi et al., 2011), DEGRADATION OF UREA 3 (DUR3) (Os10g0580400) (Wang et al., 2012), and NLP1 (Os03g0131100) (Alfatih et al., 2020), suggesting that the P and N deficiency treatments were successful. A Venn diagram between the DEGs obtained from individual deficiency conditions revealed 763 common DEGs between the –N treatment and the –P treatment, indicating that these genes are responsive to both N and P deficiency (hereafter referred to as NP-responsive DEGs; Figure 2B; Dataset S3). There were 1,442 DEGs only responding to N deficiency and 543 DEGs only responding to P deficiency (hereafter referred to as N-responsive DEGs and P-responsive DEGs, respectively; Figure 2B; Dataset S3).
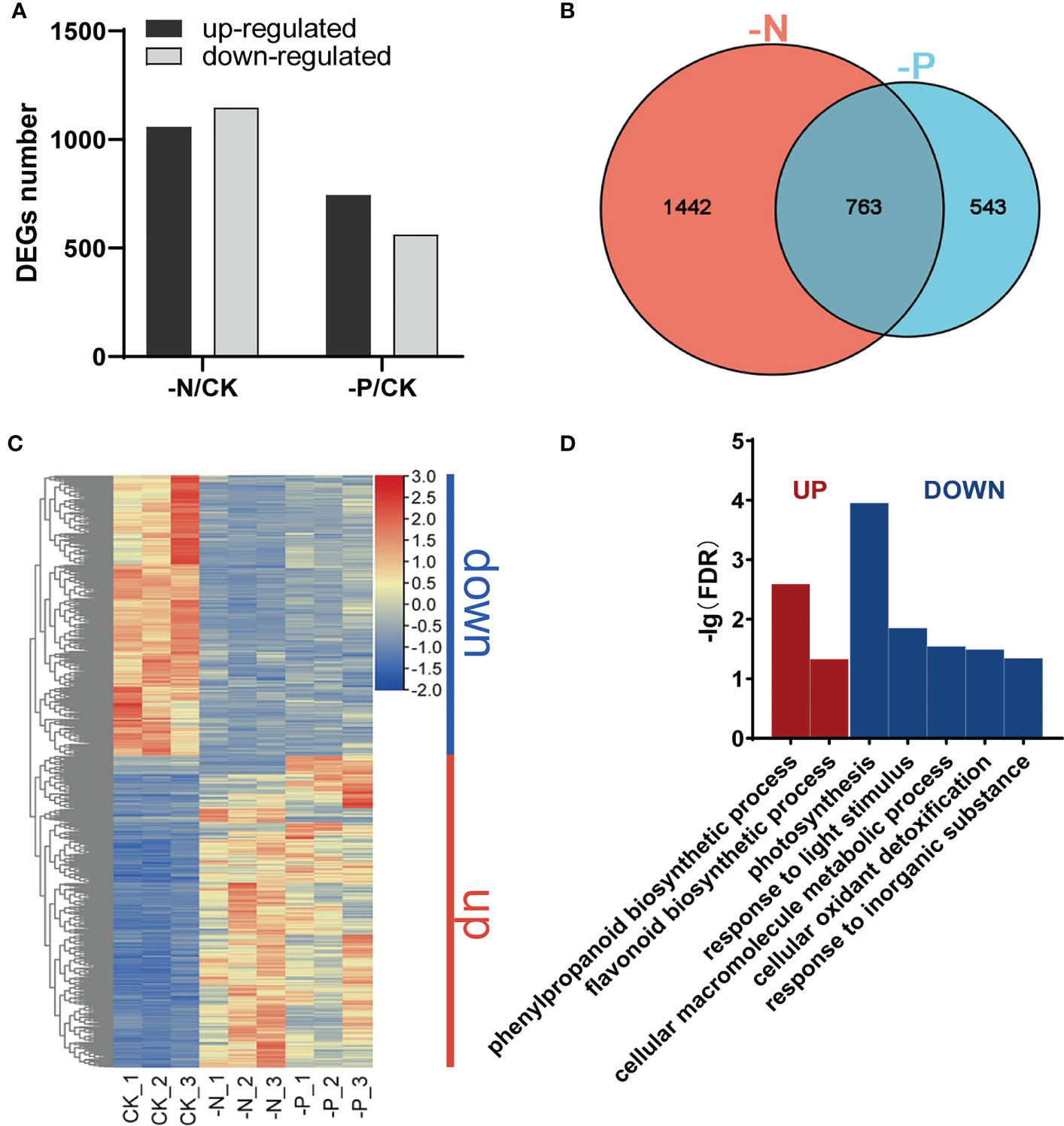
Figure 2 Changes in the rice transcriptome during N/P deficiency. (A) Numbers of upregulated and downregulated genes in Nipponbare exposed to –N or –P compared to normal conditions (CK). (B) Venn diagram of genes responsive to different N/P conditions. (C) Heatmap of DEGs responsive to both N and P deficiency; “down”, genes downregulated under –N or –P; “up”, genes upregulated under –N or –P. (D) Gene Ontology (GO) enrichment analysis of DEGs in (C). Significant categories (FDR < 0.05) are displayed.
We conducted a Gene Ontology (GO) enrichment analysis on these DEGs to determine their biological function (FDR-corrected P value < 0.05). We classified upregulated and downregulated DEGs under N deficiency into more than 20 functional categories in the biological process category (Figure S2A). DEGs upregulated under –N were mainly associated with nitrogen transport and metabolism, biosynthesis of secondary metabolites, and cell wall-related processes, such as ammonium transport, nitrate assimilation, plant-type secondary cell wall biogenesis, cutin biosynthetic process, and isoprenoid biosynthetic process (Figure S2A). DEGs downregulated under –N were enriched in photosynthesis-related terms, including photosynthesis, photosystem II stabilization, response to high-light intensity, and photosystem II repair, as well as terms related to glycometabolism and detoxification, including disaccharide biosynthetic process, fructose 1,6-bisphosphate metabolic process, and cellular oxidant detoxification (Figure S2A). Multiple photosynthesis-related terms showed significant enrichment, indicating that genes involved in photosynthesis are downregulated under N starvation, consistent with the yellowish leaf phenotype under N deficiency. DEGs upregulated under –P were enriched in cellular responses to phosphate starvation, phosphate ion transport, glycerol metabolic process, glycerophospholipid catabolic process, sulfolipid and glycolipid biosynthetic process, and cellular response to cold (Figure S2B). These results suggest that N and P deficiency induce different physiological reactions in plants.
A heatmap of the 763 NP-responsive DEGs showed that most of these genes respond similarly to N and P starvation. Genes upregulated under N deficiency were also upregulated under P deficiency, while genes downregulated under N deficiency were also downregulated under P deficiency (Figure 2C). These genes could be separated into two groups based on their expression patterns, “Up” and “Down.” Genes in the “Up” group were enriched in the phenylpropanoid and flavonoid biosynthetic pathways. Genes in the “Down” group were mainly enriched in photosynthesis, response to light stimulus, cellular macromolecule metabolic process, cellular oxidant detoxification, and response to inorganic substance categories (Figure 2D). These results demonstrate that some of the same physiological and biochemical reactions are stimulated in plants experiencing N or P deficiency.
3.3 Transcriptional regulatory network of rice nitrogen and phosphate deficiency responses
TFs are systematic regulators of global gene expression upon N and P deficiency. From our RNA-seq data, we identified 81 TF genes specifically responding to N, 19 TF genes specifically responding to P, and 34 TF genes responding to NP (Figure S3A and Dataset S4). These TF genes belonged to 28 families, with the N-responsive TF genes mainly in the basic helix-loop-helix (bHLH), MYB, NAC, and WRKY families, P-responsive TF genes mainly belonging to the ETHYLENE-RESPONSE FACTOR (ERF) family, and NP-responsive TF genes mainly comprising MYB and NAC family members (Figure S3A). To explore the potential transcriptional regulatory hierarchy among these DEGs, we constructed a transcriptional regulatory network of these TFs and DEGs considered as targets using PlantRegMap (Tian et al., 2020). This analysis produced a sub-network predominantly centered around 27 TFs and consisting of 2,005 regulatory relationships with 1,210 unique genes (Figure S3B and Dataset S5). These TFs and target genes were widely distributed among N-, NP-, and P-responsive DEGs. We noticed that several TFs target genes, including those encoding other TFs, from all three groups of DEGs. The TF targeting the most DEGs was ERF19, which is involved in responses to abscisic acid and salt stress (Huang et al., 2021). ERF19 is also a P-responsive gene, suggesting a reciprocal influence of P and abscisic acid on plants. N-responsive TF MYB63, regulating the second most target genes (Figure S3B), is reported to regulate cellulose biosynthesis during secondary cell wall formation (Noda et al., 2015). This result is consistent with enrichment of the GO term cellulose biosynthesis under N deprivation (Figure S2A). Os02g0695200, encoding a MYB TF that has not yet been characterized, represented the NP-responsive TF targeting the most genes (Figure S3B).
The 34 NP-responsive TF genes belonged to 16 TF families, indicating that many types of TFs are involved in responses to both N and P deficiency (Figure S3A). MYB and MYB-related TFs formed the largest group. The central phosphate signaling regulator genes AtPHR1 in Arabidopsis and OsPHR2 in rice encode MYB family TFs that participate in nitrate-dependent phosphate signaling networks (Rubio et al., 2001; Zhou et al., 2008; Hu et al., 2019; Medici et al., 2019). Although the transcript levels of AtPHR1 and OsPHR2 are barely responsive to P deprivation, their homologs AtPHL2, AtPHL3, OsPHR3, and OsPHR4 are upregulated upon P starvation (Sun et al., 2016; Ruan et al., 2017). The expression of most NP-responsive TFs (32 of 34) responded the same way to both N deficiency and P starvation (Figures 2C and 3). Two genes showing opposite expression patterns under N and P deficiency were Os02g0325600 and Os02g0136000, encoding NIGT1 and NLP6, respectively. Whether these genes are involved in the response to N and P starvation awaits further study.
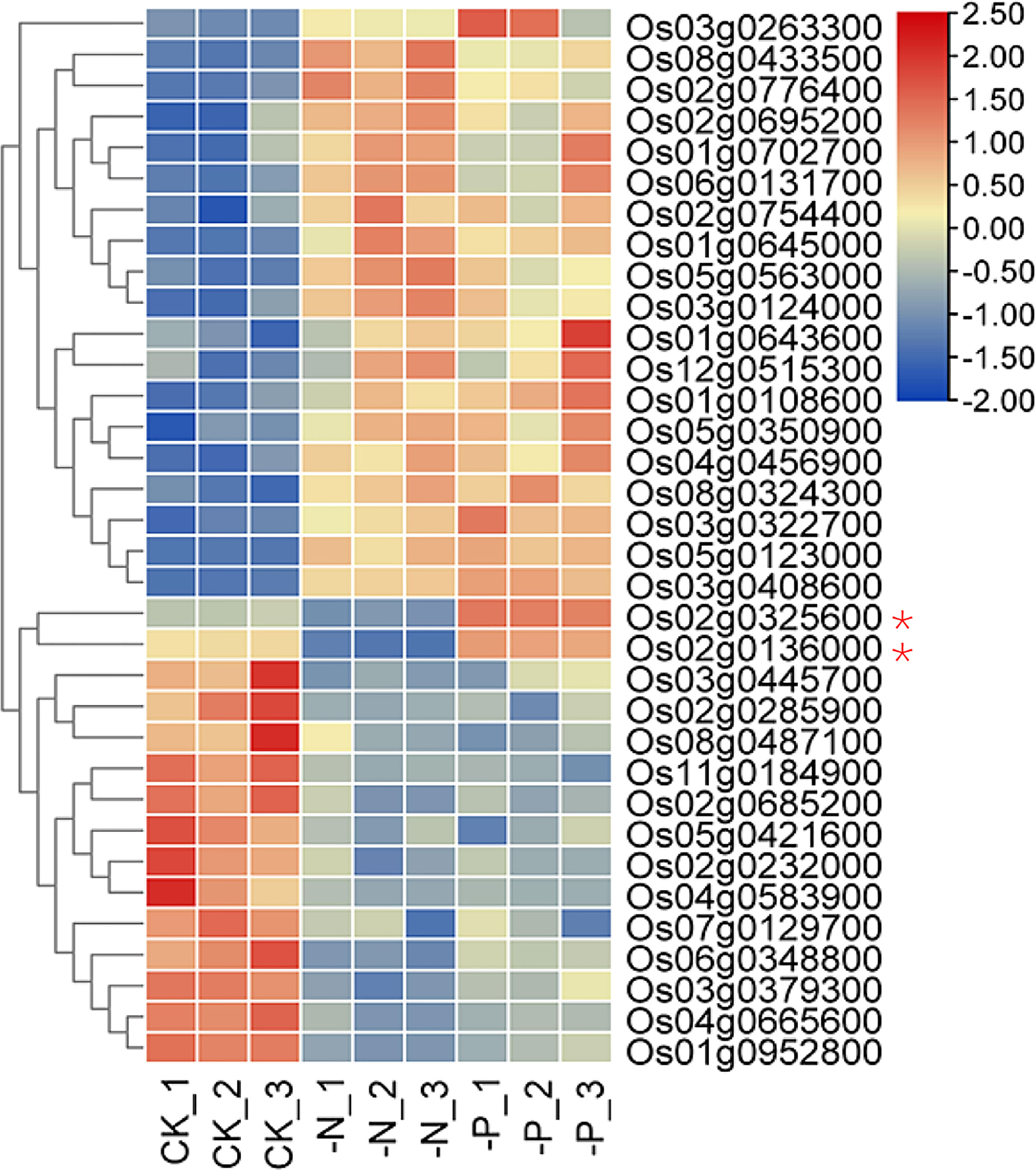
Figure 3 Heatmap of TF genes that respond to both N and P deficiency. Asterisks indicate genes with opposite responses to N and P deficiency.
3.4 NIGT1 regulates nitrogen and phosphate homeostasis in rice
To verify whether NIGT1 responds to N and P deficiency, we performed RT-qPCR analysis using total RNA isolated from the roots of rice plants exposed to N or P deficiency. Consistent with the results of RNA-seq analysis, NIGT1 expression was induced by P deficiency and repressed by N deprivation (Figure 4A). To investigate the potential function of NIGT1, we generated multiple nigt1 mutants using clustered regularly interspaced short palindromic repeat (CRISPR)/CRISPR-associated nuclease 9 (Cas9)-mediated genome editing to knock out NIGT1 in the japonica rice cv. Hei Jing 2 (HJ2) and verified these by sequencing. We selected two independent lines carrying a 30- or 3-bp deletion and a 1-bp insertion in the first exon of the NIGT1 gene, named nigt1-1 and nigt1-2, respectively, for further study (Figure 4B). The introduced mutations in both nigt1-1 and nigt1-2 resulted in premature stop codons and a predicted truncated protein. We also produced transgenic plants overexpressing NIGT1 by ectopically expressing a NIGT1-GFP (green fluorescent protein) construct under the control of the cauliflower mosaic virus (CaMV) 35S promoter in HJ2. We chose two independent lines with the highest expression of NIGT1-GFP for subsequent experiments (Figure 4C). We observed a predominantly nuclear localization when NIGT1-GFP was transiently expressed in N. benthamiana leaves. Furthermore, we detected green fluorescence in NIGT1-GFP transgenic lines in the nuclei of root cells (Figure 4D), suggesting that NIGT1 is a nucleus-localized protein, consistent with NIGT1 functioning as a TF.
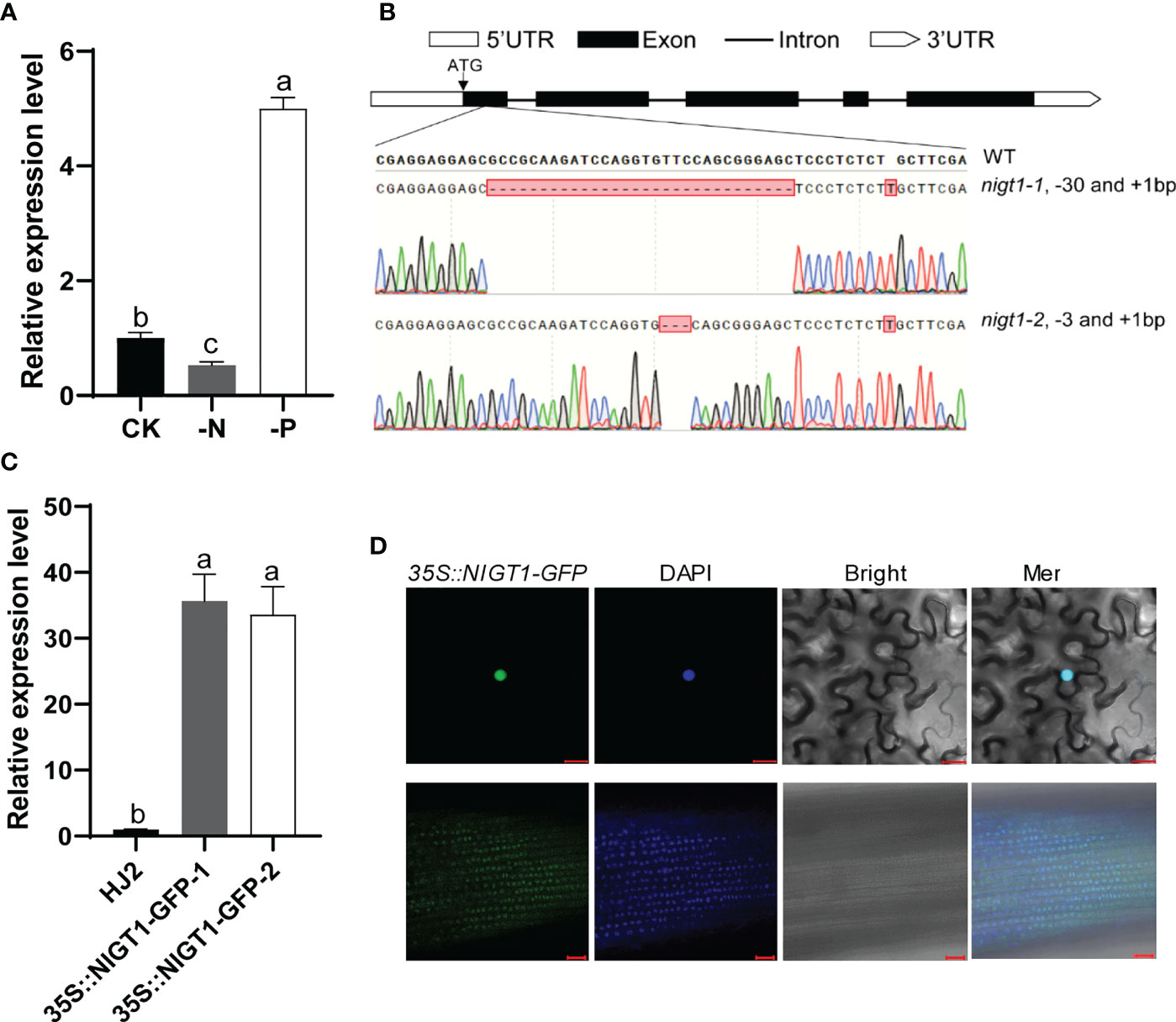
Figure 4 Molecular characterization of NIGT1 mutants and transgenic overexpression lines. (A) Expression of NIGT1 in HJ2 under –N or –P treatment for 7 d. Seedlings were grown under nutrient-replete hydroponic conditions for 7 d before –N or –P treatment. (B) Target sites and mutated sequences in nigt1-1 and nigt1-2 mutants generated using CRISPR/Cas9. (C) Expression of NIGT1 in two NIGT1-overexpression transgenic lines. Seedlings were grown under nutrient-replete conditions for 2 weeks. ACTIN1 was used as a control. NIGT1 expression of the wild type under nutrient-replete conditions was set to 1. (D) GFP fluorescence in N. benthamiana leaf cells (upper panel) and root epidermal cells of 7-d-old transgenic rice seedlings (lower panel) harboring 35S::NIGT1-GFP. Scale bars, 20 µm. Data are means + SD in (A, C) (n = 3); different lowercase letters indicate significant differences between groups (ANOVA followed by Tukey’s test, P < 0.05).
To assess the involvement of NIGT1 in rice N and P homeostasis, we investigated N and P concentration of HJ2, the nigt1 mutants, and NIGT1 overexpression lines cultured in different nutrient solutions. Generally, accumulation of P in rice plants decreased under low-N supply; analogously, reducing the P supply also limited the levels of N in plant tissues (Figures 1A and 5). Both nigt1 mutants had lower P contents and higher N contents than wild-type plants, whereas NIGT1 overexpression plants displayed higher P contents and lower N contents than wild-type plants (Figure 5), suggesting that NIGT1 promotes P uptake and inhibits N absorption. In addition, the shoots of NIGT1 overexpression lines were shorter than those of wild-type plants, irrespective of growth conditions (Figure S4), indicating that NIGT1 also affects rice shoot development. We asked whether NIGT1 mediated N and P signaling by measuring the expression levels of N- or P-responsive genes in the nigt1 mutant and NIGT1 overexpression lines. Transcript abundance of the N transporter gene NRT2.1 was higher and that of the P transporter gene PT2 was lower in nigt1 mutant lines than in wild-type plants; by contrast, we detected lower NRT2.1 and higher PT2 transcript levels in NIGT1 overexpression plants compared to wild-type plants (Figures 6A, C). In addition, SPX1 accumulation induced by P deficiency was repressed and NLP1 expression induced by N deficiency was enhanced in nigt1 mutants compared to wild-type plants; we observed the opposite pattern in NIGT1-overexpressing lines (Figures 6B, D). These data demonstrate that N/P-responsive NIGT1 plays a role in balancing the acquisition of nitrogen and phosphate in rice.
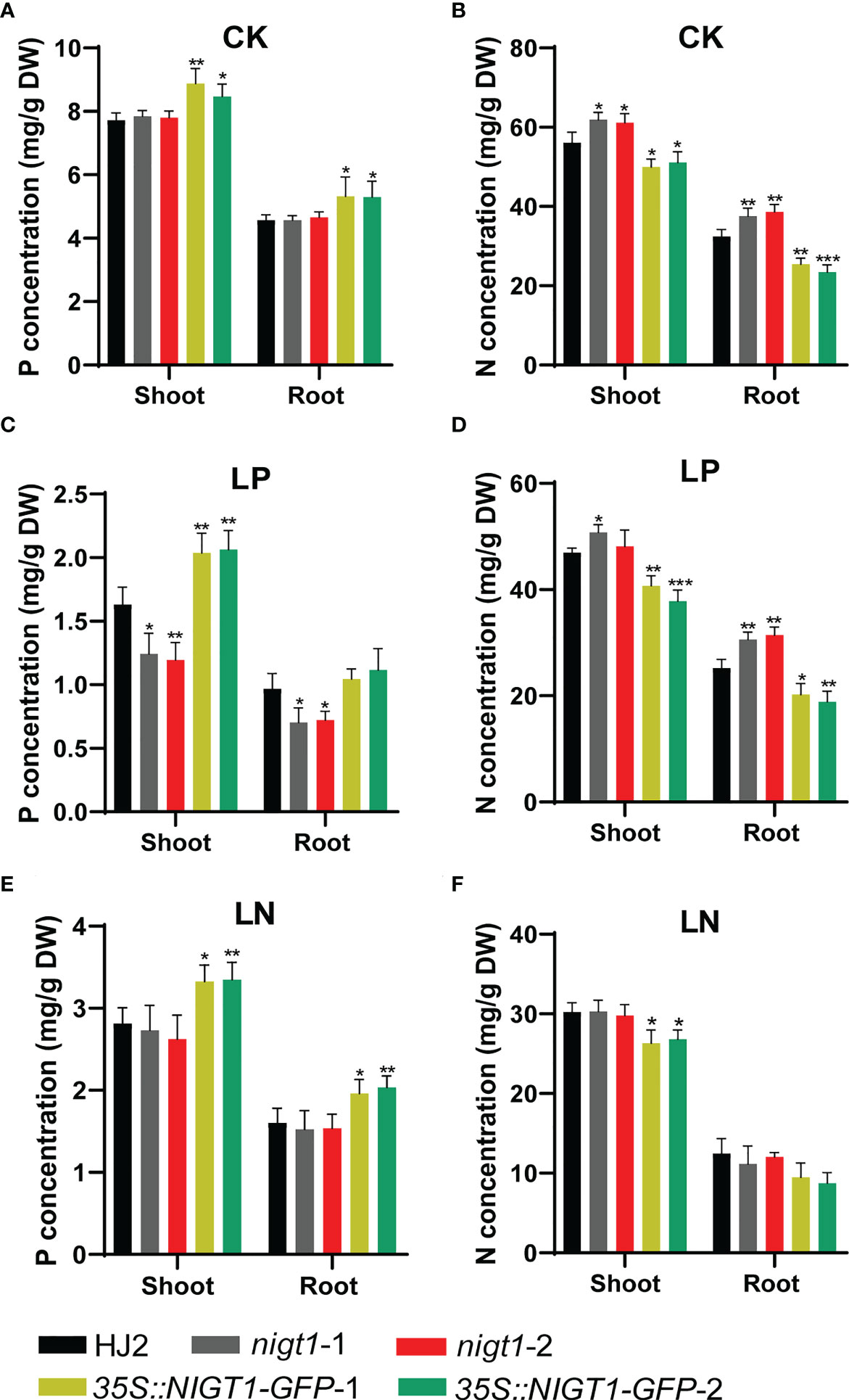
Figure 5 P and N concentrations in shoots and roots of rice nigt1 mutants and NIGT1 overexpression plants grown under different N and P conditions. (A, C, E) P concentration of plants. (B, D, F) N concentration of plants. Plants cultured in replete nutrient solution for 7 d were transferred to CK (normal N and P), LP (10 µM KH2PO4), or LN (70 µM NH4NO3) solutions and grown for another 21 d. Data are means + SD (n = 4). Student’s t-test *, P < 0.05; **, P < 0.01; ***, P < 0.001.
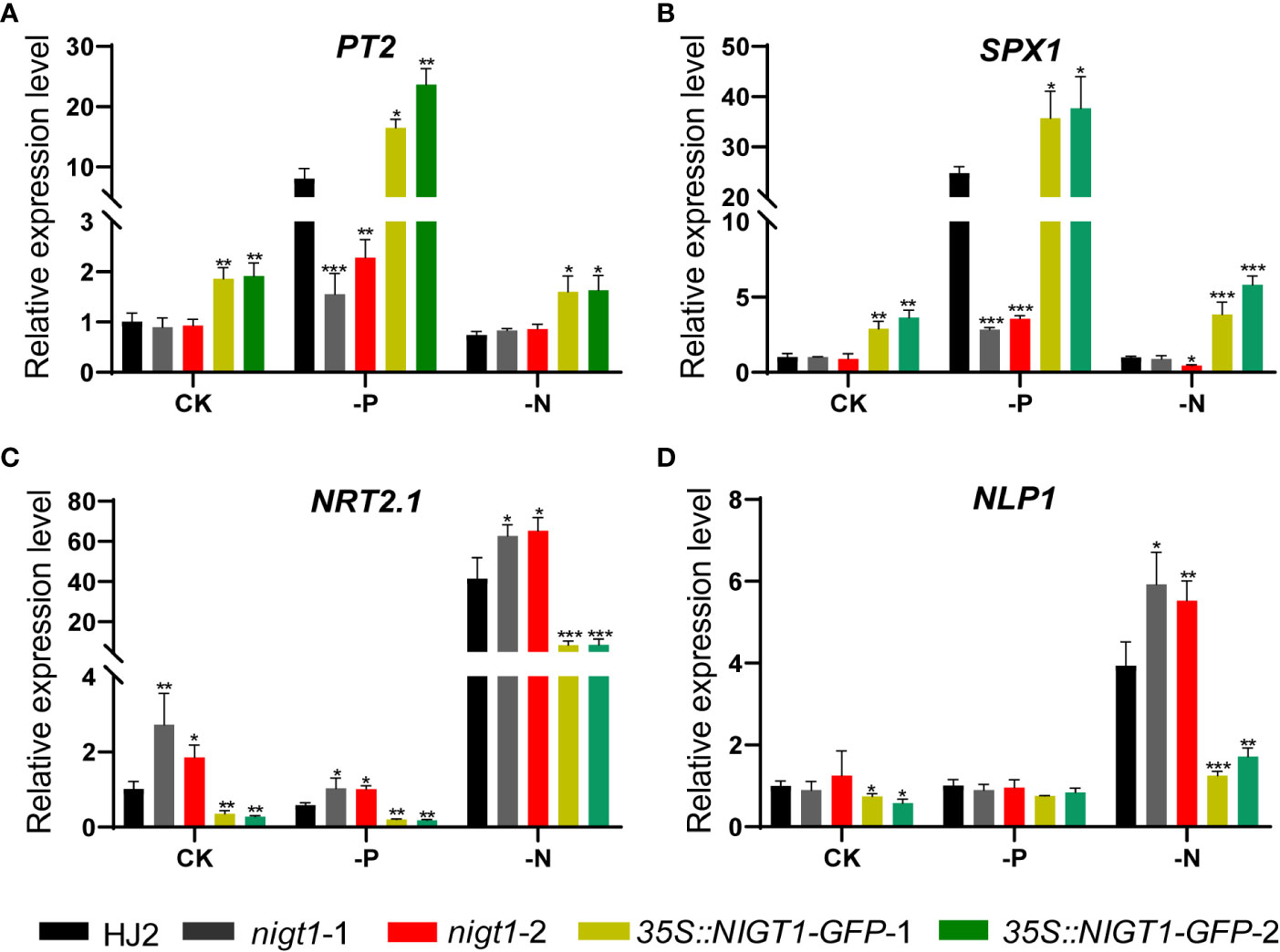
Figure 6 Expression levels of PT2(A), SPX1(B), NRT2.1(C), and NLP1(D) in HJ2, nigt1 mutant, and NIGT1 overexpression plants cultured under CK, LP, or LN conditions. ACTIN1 was used as a control. Expression of the wild type HJ2 was set to 1. Data are means + SD (n = 3). Student’s t-test *, P < 0.05; **, P < 0.01; ***, P < 0.001.
4 Discussion
Studying the mutual regulation of P and N signals is key for understanding the adaptive response of plants to a fluctuating soil environment; however, the mechanisms linking P and N acquisition in plant remain largely unknown. In this study, we investigated the similarities and differences of N and P deficiency on rice growth and transcript profiles. Both N and P deprivation inhibit shoot growth and mutually inhibit the uptake of P and N and even other nutrients in rice (Figure 1 and Figure S1). All the mineral nutrient elements are absorbed via plants root. N or P deficiency changes the root system architecture (Figures 1A, B), which may affect the uptake of other elements. In consistency, the expression of transporter genes of K, Ca or Mg are changed upon N or P deficiency (Datasets S1 and S2). However, deficiency in one of the microelements iron, zinc, or manganese significantly promotes the uptake of the other two elements (Dong et al., 2018), suggesting that the mutual regulatory mechanisms of different nutrient elements vary.
Rice is a typical species that prefers ammonium and lots of ammonium and nitrate coexist in the irrigated soil (Chen et al., 2018). It is of great importance to detect the combined effect of ammonium and nitrate in rice, so we used NH4NO3 as nitrogen source in this study. Nitrate deficiency can regulate the P starvation response in plants (Medici et al., 2019); however, whether P limitation affects the N starvation response remains elusive. Our comparative transcriptomic analysis revealed that one-third (763 out of 2,205) of the N-responsive DEGs accounted for more than one-half of all P-responsive DEGs (763 out of 1,306) (Figure 2B). These results indicate that low-N treatment can inhibit P accumulation and stimulate a P starvation response in plants. Similarly, P deficiency caused an N starvation response to some extent. The common N- and P-responsive genes were enriched in functions associated with phenylpropanoid and flavonoid biosynthesis, cellular oxidant detoxification, and response to inorganic substance, suggesting that plants will initiate basic defense mechanisms in response to N or P deficiency. Many genes were specifically responsive to either N deficiency or P deficiency. These results suggest that there are similarities and differences in plant responses to N and Pi deficiency.
Notably, many genes downregulated in roots were involved in photosynthesis, as evidenced by enriched GO categories such as response to light stimulus, photosystem II repair, photosynthesis, and light harvesting in photosystem I (Figure 2D and Figure S2). This observation suggests that genes involved in photosynthesis are also expressed in roots and that mobile signals contribute to the communication between roots and shoots. Photosynthesis involves many DNA, RNA and proteins that are rich in N or P (Hernandez and Munne-Bosch, 2015; Evans and Clarke, 2019; Mu and Chen, 2021), which may explain the downregulation of photosynthesis-related genes under N or P deficiency. Fluctuation in leaf N is strongly correlated with chlorophyll content (Hou et al., 2020); we observed lower chlorophyll content in rice plants exposed to N deficiency than in control plants, consistent with those genes associated with photosynthesis being affected by N deficiency. In addition, peptides, Ca2+, mRNAs, microRNAs, phytohormones, and nutrient ions themselves can act as mobile signals for long-distance communication between shoots and roots (Michigami et al., 2018; van Gelderen et al., 2018; Wang et al., 2018, Li et al., 2021a, Wheeldon and Bennett, 2021). Our RNA-seq data showed that the expression of the strigolactone (SL) biosynthesis genes DWARF10 (D10) and D27 was enhanced under –P and –N treatment (Datasets S1 and S2). These results are consistent with previous results suggesting that low-phosphate and low-nitrate induce SL biosynthesis, mediating root growth (Mayzlish-Gati et al., 2012; Sun et al., 2014). Whether mobile signal molecules coordinate N and P homeostasis is worth further study.
Many TFs, especially PHR and NLP signaling regulators, regulate gene expression under N and P deficiency conditions (Bustos et al., 2010; Marchive et al., 2013; Guo et al., 2015; Gaudinier et al., 2018). We identified 27 TF-centered transcriptional regulatory networks from our RNA-seq data (Figure S3). Functional characterization of the TF gene NIGT1 using gene-edited mutants and overexpression lines showed that OsNIGT1, which is induced by P starvation and repressed by N starvation (Figure 4A), negatively regulates N homeostasis and positively regulates P homeostasis (Figure 5). These results suggest that NIGT1 might play a role in the mutual inhibition of P uptake by N deficiency and of N uptake by P deprivation in rice. Expression levels of PT2 and SPX1 were lower in nigt1 mutants and higher in NIGT1 overexpression lines, while expression levels of NRT2.1 and NLP1 were higher in nigt1 mutants and lower in NIGT1 overexpression lines than those in wild-type plants (Figure 6). Furthermore, the promoters of these four genes contain a NIGT1-binding site (Table S2), indicating that they may be direct targets of NIGT1, which is worth confirming. In Arabidopsis, NIGT1 (also named HYPERSENSITIVITY TO LOW PI-ELICITED PRIMARY ROOT SHORTENING 1 [HRS1]) and HRS1 HOMOLOGs (HHOs) form a NIGT1-SPX–PHR signaling pathway and play an important role in coordinating N and P uptake and utilization during growth and development (Medici et al., 2015; Nagarajan et al., 2016; Kiba et al., 2018; Maeda et al., 2018, Ueda et al., 2020a, Ueda et al., 2020b). Recently, Wang et al. demonstrated that ZmNIGT1.1 and ZmNIGT1.2, two homologs of AtNIGT1.2, also participate in repression of uptake under P deficiency in maize (Zea mays) (Wang et al., 2020b). These data indicate that the coordinated P–N regulatory pathway mediated by NIGT1 is conserved in monocot and dicot plants.
In summary, this work revealed the interaction between gene networks associated with N and P signaling in rice and the importance of NIGT1 in this interaction (Figure 7). These findings will help identify the N–P signaling interaction network and benefit breeding efforts to generate crops with higher N- and Pi-use efficiency.
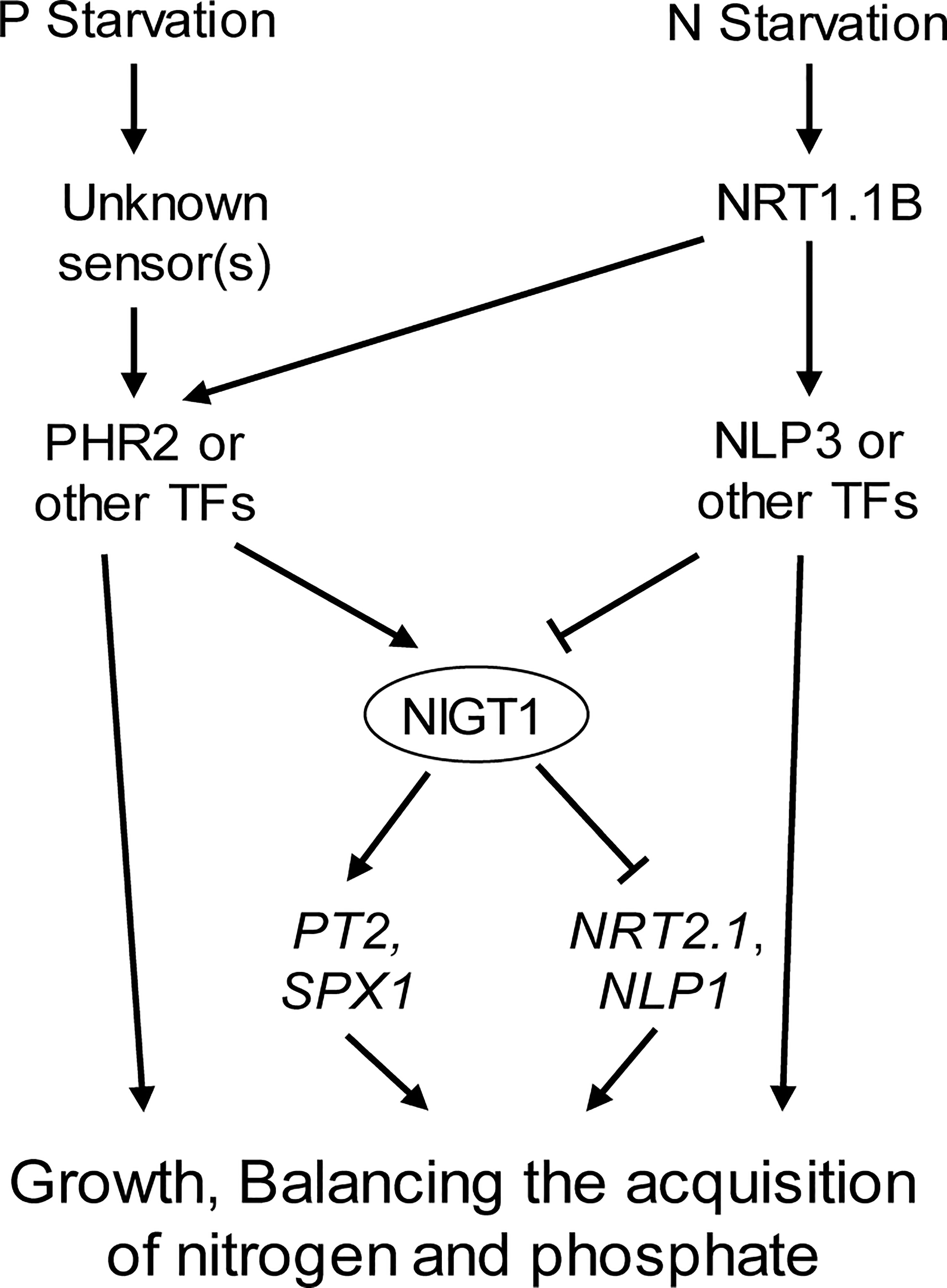
Figure 7 Proposed model for the regulation of plant responses to P and N deficiency. P and N starvation are perceived by respective sensors. The downstream signals are integrated by P deficiency induced and N starvation repressed TF NIGT1, which regulates PT2, SPX1, NRT2.1, and NLP1 expression.
Data availability statement
The datasets presented in this study can be found in online repositories. The names of the repository/repositories and accession number(s) can be found below: https://www.ncbi.nlm.nih.gov/, PRJNA925220.
Author contributions
CM and FW conceived and designed the experiments. FW performed the experiments. YW, LY, HL, YJL, YL, JX, and YRW helped with the experiments. XM and ZW revised the manuscript. CM and FW wrote the manuscript. All authors contributed to the article and approved the submitted version.
Funding
This work was supported by the National Key Research and Development Program of China (2021YFF1000402), the National Natural Science Foundation of China (31972486), the Fundamental Research Funds for the Central Universities (2021FZZX001-28), the Ministry of Education and Bureau of Foreign Experts of China (B14027), and Natural Science Foundation of Zhejiang Province (LQ21C020004).
Conflict of interest
The authors declare that the research was conducted in the absence of any commercial or financial relationships that could be construed as a potential conflict of interest.
Publisher’s note
All claims expressed in this article are solely those of the authors and do not necessarily represent those of their affiliated organizations, or those of the publisher, the editors and the reviewers. Any product that may be evaluated in this article, or claim that may be made by its manufacturer, is not guaranteed or endorsed by the publisher.
Supplementary material
The Supplementary Material for this article can be found online at: https://www.frontiersin.org/articles/10.3389/fpls.2023.1164441/full#supplementary-material
References
Ai, P., Sun, S., Zhao, J., Fan, X., Xin, W., Guo, Q., et al. (2009). Two rice phosphate transporters, OsPht1;2 and OsPht1;6, have different functions and kinetic properties in uptake and translocation. Plant J. 57 (5), 798–809. doi: 10.1111/j.1365-313X.2008.03726.x
Alfatih, A., Wu, J., Zhang, Z. S., Xia, J. Q., Jan, S. U., Yu, L. H., et al. (2020). Rice NIN-LIKE PROTEIN 1 rapidly responds to nitrogen deficiency and improves yield and nitrogen use efficiency. J. Exp. Bot. 71 (19), 6032–6042. doi: 10.1093/jxb/eraa292
Bouain, N., Krouk, G., Lacombe, B., Rouached, H. (2019). Getting to the root of plant mineral nutrition: Combinatorial nutrient stresses reveal emergent properties. Trends Plant Sci. 24 (6), 542–552. doi: 10.1016/j.tplants.2019.03.008
Bustos, R., Castrillo, G., Linhares, F., Puga, M. I., Rubio, V., Perez-Perez, J., et al. (2010). A central regulatory system largely controls transcriptional activation and repression responses to phosphate starvation in arabidopsis. PloS Genet. 6 (9), e1001102. doi: 10.1371/journal.pgen.1001102
Chen, C., Chen, H., Zhang, Y., Thomas, H. R., Frank, M. H., He, Y., et al. (2020). TBtools: An integrative toolkit developed for interactive analyses of big biological data. Mol. Plant 13 (8), 1194–1202. doi: 10.1016/j.molp.2020.06.009
Chen, H., Zhang, Q., Cai, H., Zhou, W., Xu, F. (2018). H2 O2 mediates nitrate-induced iron chlorosis by regulating iron homeostasis in rice. Plant Cell Environ. 41 (4), 767–781. doi: 10.1111/pce.13145
Dong, C., He, F., Berkowitz, O., Liu, J., Cao, P., Tang, M., et al. (2018). Alternative splicing plays a critical role in maintaining mineral nutrient homeostasis in rice (Oryza sativa). Plant Cell 30 (10), 2267–2285. doi: 10.1105/tpc.18.00051
Evans, J. R., Clarke, V. C. (2019). The nitrogen cost of photosynthesis. J. Exp. Bot. 70 (1), 7–15. doi: 10.1093/jxb/ery366
Gaudinier, A., Rodriguez-Medina, J., Zhang, L., Olson, A., Liseron-Monfils, C., Bagman, A. M., et al. (2018). Transcriptional regulation of nitrogen-associated metabolism and growth. Nature 563 (7730), 259–264. doi: 10.1038/s41586-018-0656-3
Guo, M., Ruan, W., Li, C., Huang, F., Zeng, M., Liu, Y., et al. (2015). Integrative comparison of the role of the PHOSPHATE RESPONSE1 subfamily in phosphate signaling and homeostasis in rice. Plant Physiol. 168 (4), 1762–1776. doi: 10.1104/pp.15.00736
Gusewell, S. (2004). N: P ratios in terrestrial plants: variation and functional significance. New Phytol. 164 (2), 243–266. doi: 10.1111/j.1469-8137.2004.01192.x
Hernandez, I., Munne-Bosch, S. (2015). Linking phosphorus availability with photo-oxidative stress in plants. J. Exp. Bot. 66 (10), 2889–2900. doi: 10.1093/jxb/erv056
Hou, W., Trankner, M., Lu, J., Yan, J., Huang, S., Ren, T., et al. (2020). Diagnosis of nitrogen nutrition in rice leaves influenced by potassium levels. Front. Plant Sci. 11. doi: 10.3389/fpls.2020.00165
Hu, B., Jiang, Z., Wang, W., Qiu, Y., Zhang, Z., Liu, Y., et al. (2019). Nitrate-NRT1.1B-SPX4 cascade integrates nitrogen and phosphorus signalling networks in plants. Nat. Plants 5 (4), 401–413. doi: 10.1038/s41477-019-0384-1
Huang, S., Ma, Z., Hu, L., Huang, K., Zhang, M., Zhang, S., et al. (2021). Involvement of rice transcription factor OsERF19 in response to ABA and salt stress responses. Plant Physiol. Biochem. 167, 22–30. doi: 10.1016/j.plaphy.2021.07.027
Jia, H., Ren, H., Gu, M., Zhao, J., Sun, S., Zhang, X., et al. (2011). The phosphate transporter gene OsPht1;8 is involved in phosphate homeostasis in rice. Plant Physiol. 156 (3), 1164–1175. doi: 10.1104/pp.111.175240
Kant, S., Peng, M., Rothstein, S. J. (2011). Genetic regulation by NLA and microRNA827 for maintaining nitrate-dependent phosphate homeostasis in arabidopsis. PloS Genet. 7 (3), e1002021. doi: 10.1371/journal.pgen.1002021
Kiba, T., Inaba, J., Kudo, T., Ueda, N., Konishi, M., Mitsuda, N., et al. (2018). Repression of nitrogen starvation responses by members of the arabidopsis GARP-type transcription factor NIGT1/HRS1 subfamily. Plant Cell 30 (4), 925–945. doi: 10.1105/tpc.17.00810
Li, L., Liu, K. H., Sheen, J. (2021b). Dynamic nutrient signaling networks in plants. Annu. Rev. Cell Dev. Biol. 37, 341–367. doi: 10.1146/annurev-cellbio-010521-015047
Li, H., Testerink, C., Zhang, Y. (2021a). How roots and shoots communicate through stressful times. Trends Plant Sci. 26 (9), 940–952. doi: 10.1016/j.tplants.2021.03.005
Li, Z., Tong, Z., He, F., Li, X., Sun, J. (2022). Integrated mRNA and microRNA expression analysis of root response to phosphate deficiency in medicago sativa. Front. Plant Sci. 13. doi: 10.3389/fpls.2022.989048
Liang, R., Su, Y., Qin, X., Gao, Z., Fu, Z., Qiu, H., et al. (2022). Comparative transcriptomic analysis of two cucumis melo var. saccharinus germplasms differing in fruit physical and chemical characteristics. BMC Plant Biol. 22 (1), 193. doi: 10.1186/s12870-022-03550-8
Lin, W. Y., Huang, T. K., Chiou, T. J. (2013). Nitrogen limitation adaptation, a target of microRNA827, mediates degradation of plasma membrane-localized phosphate transporters to maintain phosphate homeostasis in arabidopsis. Plant Cell 25 (10), 4061–4074. doi: 10.1105/tpc.113.116012
Liu, W., Sun, Q., Wang, K., Du, Q., Li, W. X. (2017). Nitrogen limitation adaptation (NLA) is involved in source-to-sink remobilization of nitrate by mediating the degradation of NRT1.7 in arabidopsis. New Phytol. 214 (2), 734–744. doi: 10.1111/nph.14396
Lu, H., Wang, F., Wang, Y., Lin, R., Wang, Z., Mao, C. (2023). Molecular mechanisms and genetic improvement of low-phosphorus tolerance in rice. Plant Cell Environ. 46 (4), 1104–1119. doi: 10.1111/pce.14457
Luo, X., Mazer, S. J., Guo, H., Zhang, N., Weiner, J., Hu, S. (2016). Nitrogen:phosphorous supply ratio and allometry in five alpine plant species. Ecol. Evol. 6 (24), 8881–8892. doi: 10.1002/ece3.2587
Maeda, Y., Konishi, M., Kiba, T., Sakuraba, Y., Sawaki, N., Kurai, T., et al. (2018). A NIGT1-centred transcriptional cascade regulates nitrate signalling and incorporates phosphorus starvation signals in arabidopsis. Nat. Commun. 9 (1), 1376. doi: 10.1038/s41467-018-03832-6
Marchive, C., Roudier, F., Castaings, L., Brehaut, V., Blondet, E., Colot, V., et al. (2013). Nuclear retention of the transcription factor NLP7 orchestrates the early response to nitrate in plants. Nat. Commun. 4, 1713. doi: 10.1038/ncomms2650
Mayzlish-Gati, E., De-Cuyper, C., Goormachtig, S., Beeckman, T., Vuylsteke, M., Brewer, P. B., et al. (2012). Strigolactones are involved in root response to low phosphate conditions in arabidopsis. Plant Physiol. 160 (3), 1329–1341. doi: 10.1104/pp.112.202358
Medici, A., Marshall-Colon, A., Ronzier, E., Szponarski, W., Wang, R., Gojon, A., et al. (2015). AtNIGT1/HRS1 integrates nitrate and phosphate signals at the arabidopsis root tip. Nat. Commun. 6, 6274. doi: 10.1038/ncomms7274
Medici, A., Szponarski, W., Dangeville, P., Safi, A., Dissanayake, I. M., Saenchai, C., et al. (2019). Identification of molecular integrators shows that nitrogen actively controls the phosphate starvation response in plants. Plant Cell 31 (5), 1171–1184. doi: 10.1105/tpc.18.00656
Michigami, T., Kawai, M., Yamazaki, M., Ozono, K. (2018). Phosphate as a signaling molecule and its sensing mechanism. Physiol. Rev. 98 (4), 2317–2348. doi: 10.1152/physrev.00022.2017
Mu, X., Chen, Y. (2021). The physiological response of photosynthesis to nitrogen deficiency. Plant Physiol. Biochem. 158, 76–82. doi: 10.1016/j.plaphy.2020.11.019
Nagarajan, V. K., Satheesh, V., Poling, M. D., Raghothama, K. G., Jain, A. (2016). Arabidopsis MYB-related HHO2 exerts a regulatory influence on a subset of root traits and genes governing phosphate homeostasis. Plant Cell Physiol. 57 (6), 1142–1152. doi: 10.1093/pcp/pcw063
Nie, Z., Luo, B., Zhang, X., Wu, L., Liu, D., Guo, J., et al. (2021). Combined transcriptome and proteome analysis of maize (Zea mays l.) reveals a complementary profile in response to phosphate deficiency. Curr. Issues Mol. Biol. 43 (2), 1142–1155. doi: 10.3390/cimb43020081
Noda, S., Koshiba, T., Hattori, T., Yamaguchi, M., Suzuki, S., Umezawa, T. (2015). The expression of a rice secondary wall-specific cellulose synthase gene, OsCesA7, is directly regulated by a rice transcription factor, OsMYB58/63. Planta 242 (3), 589–600. doi: 10.1007/s00425-015-2343-z
Park, B. S., Seo, J. S., Chua, N. H. (2014). NITROGEN LIMITATION ADAPTATION recruits PHOSPHATE2 to target the phosphate transporter PT2 for degradation during the regulation of arabidopsis phosphate homeostasis. Plant Cell 26 (1), 454–464. doi: 10.1105/tpc.113.120311
Paz-Ares, J., Puga, M. I., Rojas-Triana, M., Martinez-Hevia, I., Diaz, S., Poza-Carrion, C., et al. (2022). Plant adaptation to low phosphorus availability: Core signaling, crosstalks, and applied implications. Mol. Plant 15 (1), 104–124. doi: 10.1016/j.molp.2021.12.005
Ruan, W., Guo, M., Wu, P., Yi, K. (2017). Phosphate starvation induced OsPHR4 mediates pi-signaling and homeostasis in rice. Plant Mol. Biol. 93 (3), 327–340. doi: 10.1007/s11103-016-0564-6
Rubio, V., Linhares, F., Solano, R., Martin, A. C., Iglesias, J., Leyva, A., et al. (2001). A conserved MYB transcription factor involved in phosphate starvation signaling both in vascular plants and in unicellular algae. Genes Dev. 15 (16), 2122–2133. doi: 10.1101/gad.204401
Sawaki, N., Tsujimoto, R., Shigyo, M., Konishi, M., Toki, S., Fujiwara, T., et al. (2013). A nitrate-inducible GARP family gene encodes an auto-repressible transcriptional repressor in rice. Plant Cell Physiol. 54 (4), 506–517. doi: 10.1093/pcp/pct007
Shao, Y., Zhou, H. Z., Wu, Y., Zhang, H., Lin, J., Jiang, X., et al. (2019). OsSPL3, an SBP-domain protein, regulates crown root development in rice. Plant Cell 31 (6), 1257–1275. doi: 10.1105/tpc.19.00038
Sun, L., Song, L., Zhang, Y., Zheng, Z., Liu, D. (2016). Arabidopsis PHL2 and PHR1 act redundantly as the key components of the central regulatory system controlling transcriptional responses to phosphate starvation. Plant Physiol. 170 (1), 499–514. doi: 10.1104/pp.15.01336
Sun, H., Tao, J., Liu, S., Huang, S., Chen, S., Xie, X., et al. (2014). Strigolactones are involved in phosphate- and nitrate-deficiency-induced root development and auxin transport in rice. J. Exp. Bot. 65 (22), 6735–6746. doi: 10.1093/jxb/eru029
Takayanagi, S., Takagi, Y., Araki, R., Hasegawa, H. (2011). High-affinity nitrate uptake by rice (Oryza sativa) coleoptiles. J. Plant Res. 124 (2), 305–309. doi: 10.1007/s10265-010-0375-9
Tian, F., Yang, D. C., Meng, Y. Q., Jin, J., Gao, G. (2020). PlantRegMap: charting functional regulatory maps in plants. Nucleic Acids Res. 48 (D1), D1104–D1113. doi: 10.1093/nar/gkz1020
Tian, W. H., Ye, J. Y., Cui, M. Q., Chang, J. B., Liu, Y., Li, G. X., et al. (2021). A transcription factor STOP1-centered pathway coordinates ammonium and phosphate acquisition in arabidopsis. Mol. Plant 14 (9), 1554–1568. doi: 10.1016/j.molp.2021.06.024
Ueda, Y., Kiba, T., Yanagisawa, S. (2020a). Nitrate-inducible NIGT1 proteins modulate phosphate uptake and starvation signalling via transcriptional regulation of SPX genes. Plant J. 102 (3), 448–466. doi: 10.1111/tpj.14637
Ueda, Y., Nosaki, S., Sakuraba, Y., Miyakawa, T., Kiba, T., Tanokura, M., et al. (2020b). NIGT1 family proteins exhibit dual mode DNA recognition to regulate nutrient response-associated genes in arabidopsis. PloS Genet. 16 (11), e1009197. doi: 10.1371/journal.pgen.1009197
Ueda, Y., Ohtsuki, N., Kadota, K., Tezuka, A., Nagano, A. J., Kadowaki, T., et al. (2020c). Gene regulatory network and its constituent transcription factors that control nitrogen-deficiency responses in rice. New Phytol. 227 (5), 1434–1452. doi: 10.1111/nph.16627
van Gelderen, K., Kang, C. K., Pierik, R. (2018). Light signaling, root development, and plasticity. Plant Physiol. 176 (2), 1049–1060. doi: 10.1104/pp.17.01079
Varala, K., Marshall-Colon, A., Cirrone, J., Brooks, M. D., Pasquino, A. V., Leran, S., et al. (2018). Temporal transcriptional logic of dynamic regulatory networks underlying nitrogen signaling and use in plants. Proc. Natl. Acad. Sci. U.S.A. 115 (25), 6494–6499. doi: 10.1073/pnas.1721487115
Wang, Y. Y., Cheng, Y. H., Chen, K. E., Tsay, Y. F. (2018). Nitrate transport, signaling, and use efficiency. Annu. Rev. Plant Biol. 69, 85–122. doi: 10.1146/annurev-arplant-042817-040056
Wang, F., Deng, M., Chen, J., He, Q., Jia, X., Guo, H., et al. (2020a). CASEIN KINASE2-dependent phosphorylation of PHOSPHATE2 fine-tunes phosphate homeostasis in rice. Plant Physiol. 183 (1), 250–262. doi: 10.1104/pp.20.00078
Wang, W. H., Kohler, B., Cao, F. Q., Liu, G. W., Gong, Y. Y., Sheng, S., et al. (2012). Rice DUR3 mediates high-affinity urea transport and plays an effective role in improvement of urea acquisition and utilization when expressed in arabidopsis. New Phytol. 193 (2), 432–444. doi: 10.1111/j.1469-8137.2011.03929.x
Wang, X., Wang, H. F., Chen, Y., Sun, M. M., Wang, Y., Chen, Y. F. (2020b). The transcription factor NIGT1.2 modulates both phosphate uptake and nitrate influx during phosphate starvation in arabidopsis and maize. Plant Cell 32 (11), 3519–3534. doi: 10.1105/tpc.20.00361
Wang, C., Ying, S., Huang, H., Li, K., Wu, P., Shou, H. (2009). Involvement of OsSPX1 in phosphate homeostasis in rice. Plant J. 57 (5), 895–904. doi: 10.1111/j.1365-313X.2008.03734.x
Wheeldon, C. D., Bennett, T. (2021). There and back again: An evolutionary perspective on long-distance coordination of plant growth and development. Semin. Cell Dev. Biol. 109, 55–67. doi: 10.1016/j.semcdb.2020.06.011
Xia, X., Fan, X., Wei, J., Feng, H., Qu, H., Xie, D., et al. (2015). Rice nitrate transporter OsNPF2.4 functions in low-affinity acquisition and long-distance transport. J. Exp. Bot. 66 (1), 317–331. doi: 10.1093/jxb/eru425
Yan, H., Shi, H., Hu, C., Luo, M., Xu, C., Wang, S., et al. (2021). Transcriptome differences in response mechanisms to low-nitrogen stress in two wheat varieties. Int. J. Mol. Sci. 22 (22), 12278. doi: 10.3390/ijms222212278
Yang, J., Gao, M. X., Hu, H., Ding, X. M., Lin, H. W., Wang, L., et al. (2016). OsCLT1, a CRT-like transporter 1, is required for glutathione homeostasis and arsenic tolerance in rice. New Phytol. 211 (2), 658–670. doi: 10.1111/nph.13908
Yang, J., Wang, L., Mao, C., Lin, H. (2017). Characterization of the rice NLA family reveals a key role for OsNLA1 in phosphate homeostasis. Rice (N Y) 10 (1), 52. doi: 10.1186/s12284-017-0193-y
Ye, J. Y., Tian, W. H., Zhou, M., Zhu, Q. Y., Du, W. X., Zhu, Y. X., et al. (2021). STOP1 activates NRT1.1-mediated nitrate uptake to create a favorable rhizospheric pH for plant adaptation to acidity. Plant Cell 33 (12), 3658–3674. doi: 10.1093/plcell/koab226
Yue, W., Ying, Y., Wang, C., Zhao, Y., Dong, C., Whelan, J., et al. (2017). OsNLA1, a RING-type ubiquitin ligase, maintains phosphate homeostasis in oryza sativa via degradation of phosphate transporters. Plant J. 90 (6), 1040–1051. doi: 10.1111/tpj.13516
Zhou, J., Jiao, F., Wu, Z., Li, Y., Wang, X., He, X., et al. (2008). OsPHR2 is involved in phosphate-starvation signaling and excessive phosphate accumulation in shoots of plants. Plant Physiol. 146 (4), 1673–1686. doi: 10.1104/pp.107.111443
Zhou, J., Liu, C., Chen, Q., Liu, L., Niu, S., Chen, R., et al. (2022). Integration of rhythmic metabolome and transcriptome provides insights into the transmission of rhythmic fluctuations and temporal diversity of metabolism in rice. Sci. China Life Sci. 65 (9), 1794–1810. doi: 10.1007/s11427-021-2064-7
Keywords: rice, nitrogen, phosphate, interaction, uptake, transcriptome, NIGT1
Citation: Wang F, Wang Y, Ying L, Lu H, Liu Y, Liu Y, Xu J, Wu Y, Mo X, Wu Z and Mao C (2023) Integrated transcriptomic analysis identifies coordinated responses to nitrogen and phosphate deficiency in rice. Front. Plant Sci. 14:1164441. doi: 10.3389/fpls.2023.1164441
Received: 12 February 2023; Accepted: 15 March 2023;
Published: 08 May 2023.
Edited by:
Juan Camacho-Cristóbal, Universidad Pablo de Olavide, SpainReviewed by:
Ki-Hong Jung, Kyung Hee University, Republic of KoreaYuanyue Shen, Beijing University of Agriculture, China
Rafael A. Cañas, University of Malaga, Spain
Copyright © 2023 Wang, Wang, Ying, Lu, Liu, Liu, Xu, Wu, Mo, Wu and Mao. This is an open-access article distributed under the terms of the Creative Commons Attribution License (CC BY). The use, distribution or reproduction in other forums is permitted, provided the original author(s) and the copyright owner(s) are credited and that the original publication in this journal is cited, in accordance with accepted academic practice. No use, distribution or reproduction is permitted which does not comply with these terms.
*Correspondence: Chuanzao Mao, bWN6QHpqdS5lZHUuY24=