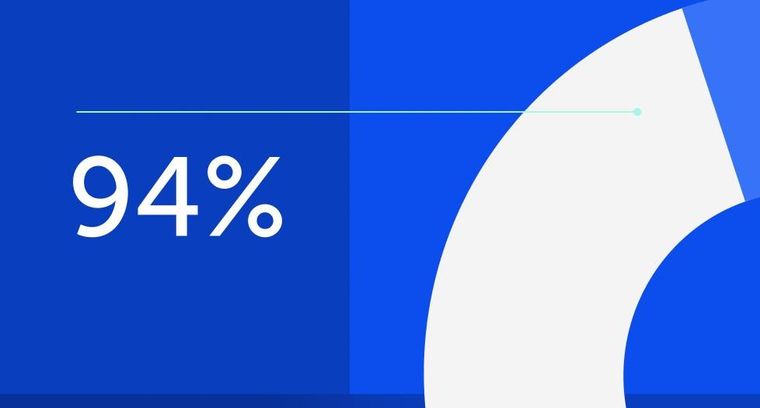
94% of researchers rate our articles as excellent or good
Learn more about the work of our research integrity team to safeguard the quality of each article we publish.
Find out more
REVIEW article
Front. Plant Sci., 19 April 2023
Sec. Plant Biotechnology
Volume 14 - 2023 | https://doi.org/10.3389/fpls.2023.1159588
Plant micropropagation has been adapted in the fields of agriculture, horticulture, forestry, and other related fields for large-scale production of elite plants. The use of liquid media and adoption of bioreactors have escalated the production of healthy plants. Several liquid-phase, gas-phase, temporary immersion, and other modified bioreactors have been used for plant propagation. The design, principle, operational mode, merits, and demerits of various bioreactors used for the regeneration of propagules, such as bulblets, cormlets, rhizomes, microtubers, shoots (subsequent rooting), and somatic embryos, are discussed here. In addition, various parameters that affect plant regeneration are discussed with suitable examples.
Micropropagation of plants is a useful technique for large-scale propagation of crops, medicinal and ornamental plants, tree species, and other economically important plants (Rout et al., 2000; Rout et al., 2006). Micropropagation is a valuable method for rapid production of disease-free plants, rapid multiplication of rare species, genetic transformation of plants, and production of plant-derived bioactive compounds (Rout et al., 2000; Espinosa-Leal et al., 2018). Micropropagation technique involves aseptic culturing of explants, such as apical or axillary meristems, or other parts of the plant body on chemically defined nutrient medium, and maintaining the cultures in controlled environmental conditions for temperature, light, and humidity. The cultured cells from explants are involved in expressing totipotency and morphogenetic responses and follow a definite developmental pathway of dedifferentiation, redifferentiation, and organization of meristematic centers under the influence of phytohormones/growth regulators. Upon expression of totipotency, actively dividing cells induce organogenesis or somatic embryogenesis (Xu and Huang, 2014). Developmental events for the formation of organs or somatic embryos may occur via direct and indirect morphogenesis (Rout et al., 2006). Development of shoots from preexisting meristem i.e., regeneration of shoots from apical meristem or axillary meristem is designated as direct organogenesis. Whereas, the regeneration of somatic embryos from single cells or group of cells without the mediation of callus regeneration is referred to as direct embryogenesis. However, in some plants, regeneration of shoots or embryos may develop from the callus regenerated from the cultured explants. Micropropagation involves four concrete stages during organogenesis: initiation of culture, shoot multiplication, induction of roots from regenerated shoots, and acclimatization (Rout et al., 2006). The first stage involves culture initiation when specific and actively growing organs or explants and juveniles are selected and cultured on a well-defined medium. In the second stage, explant cells respond to the stimulus of growth regulators (auxin and cytokinins) and are involved in the division and development of organs (organogenesis). The third stage involves elongation of shoots derived from the multiplication stage, and is subsequently rooted either ex vitro or in vitro. The fourth stage involves the acclimatization of plants grown in vitro, which is a crucial stage in the micropropagation procedure (Rout et al., 2006). Somatic embryogenesis involves five stages: initiation of embryogenesis or induction, during which embryogenic cultures are initiated by culturing the primary explant on a medium supplemented with plant growth regulators, mainly auxin and cytokinin. The second stage involves proliferation of embryogenic cultures on solidified or liquid media supplemented with plant growth regulators, similar to that during initiation. The third stage is pre-maturation of somatic embryos or developmental stage in a medium lacking a plant growth regulator or cytokinin, which stimulates somatic embryo formation and early development. The fourth stage involves the maturation of somatic embryos by culturing on a medium supplemented with abscisic acid and/or reduced osmotic potential (Lema-Ruminska et al., 2013). The fifth stage involves conversion of embryos into plantlets, normally on a medium lacking a plant growth regulator (Arnold et al., 2002). In orchids, in vitro cultured explants develop protocorm-like bodies (PLBs) that are regenerated from shoot tips, flower stalk buds, root tips, and leaf segments directly or via callus mediation, and are similar to somatic embryos based on their nature and mode of development (Lee et al., 2013). In geophytes, vegetative propagules/organogenic propagules/storage organs, such as bulbs/bulblets (onion, garlic), tubers/microtubers (potato), corms/cormlets (gladiolus, crocus), and rhizomes (ginger, turmeric, lily), are regenerated when different explants are cultured on nutrient medium (Paek and Murthy, 2002; Lian et al., 2003a; Lian et al., 2003b; Jo et al., 2008).
Conventional micropropagation is considered a labor-intensive and costly technology because it involves the maintenance of a large number of culture vessels, semi-solid media, and periodic transfer of plant material to fresh media after subculturing for 4–6 weeks owing to the exhaustion of nutrients in the medium (Maene and Debergh, 1985). Gelling agents substantially raise the expense of in vitro production and restrict the extent to which commercial propagation may be automated (Garcia-Ramirez, 2023). Scaled-up and automated methods are consequently preferable to reduce handling throughout the processes necessary for micropropagation, boost multiplication rates, and overcome or minimize production costs (Watt, 2012; Garcia-Ramirez, 2023). Therefore, the use of liquid medium and culture of propagules in bioreactors have evolved as attractive alternatives to conventional propagation methods. The advantages of using liquid medium and bioreactor cultures for micropropagation include: i) a large number of plantlets are easily produced and scaled up; ii) handling of cultures, such as inoculation or harvest, is easy, saving labor and time; iii) cultures are always in contact with the medium, which helps in easy uptake of nutrients and results in stimulation of growth rate; and iv) forced air supply in bioreactor cultures facilitates growth and metabolism of cultured cells and organs (Takayama and Akita, 1994; Paek et al., 2001; Etienne and Berthouly, 2002; Paek et al., 2005). However, liquid cultures have disadvantages, including asphyxia, hyperhydricity, and physiological disorders exhibited by the continuously immersed cultures (Gao et al., 2018; Schuchovski et al., 2020). Several bioreactors designed for microbial cultures cannot be used for plant micropropagation because plant cells and organs may experience shear stress, mechanical damage in stirred tank bioreactors, and foam formation in bubble-aerated bioreactors (Teisson and Alvard, 1999). Therefore, several modifications have been carried out with available bioreactors, and new designs have been developed and adopted exclusively for culturing plant cells and organs, specifically for micropropagation. Temporary immersion, wave, and balloon-type bubble bioreactors have been developed. The design and modification of existing bioreactors for plant micropropagation have been extensively reviewed (Paek et al., 2001; Etienne and Berthouly, 2002; Paek et al., 2005; Watt, 2012; Steingroewer et al., 2013; Mamun et al., 2015; Vidal and Sanchez, 2019). However, bioreactors for regenerating shoots (axillary and adventitious shoots), somatic embryos, and bulbs/corms/micro-tubers/rhizomes require special designs. Different plant species and propagation materials have various requirements that demand specific settings for internal bioreactor environments and determine the most appropriate bioreactor design. The growth and development of plant cells in vitro mostly depends on liquid medium circulation, mixing, and aeration for the distribution of oxygen and nutrients (Curtis, 2005; Mamun et al., 2015). The present review focuses on various configurations of bioreactors that have been specifically designed or modified for plant propagation, their advantages, and limitations. Because of the differences in their requirements, the parameters that should be managed in bioreactors for regenerating organogenic propagules, such as bulbs, corms, micro-tubers, rhizomes, shoots, and somatic embryos, have been covered in separate sections.
Bioreactors are self-contained and sterile environments, which use liquid nutrient media or liquid/air inflow and outflow systems. They are designed for intensive culture and provide the highest opportunity for monitoring and controlling microenvironmental conditions such as agitation, aeration, temperature, and hydrogen ion concentration (pH) (Leathers et al., 1995). The internal environment of a bioreactor is typically controlled at different levels, depending on its model and the plant material. The parameters, including circulation of medium, mixing, aeration, temperature, pH, and dissolved oxygen, are efficiently controlled in bioreactors. Different plant species and propagation materials have different requirements that dictate specific settings for the internal environment of bioreactors and determine the most appropriate bioreactor model to use (Mamun et al., 2015). Based on the environment in the cultivation chamber, bioreactors are broadly classified into four types: liquid-phase, gas-phase, temporary immersion, and modified bioreactors. Liquid-phase bioreactors are of two types: mechanically agitated bioreactors and pneumatically agitated bioreactors based on the mode of circulation of medium inside the container.
Liquid-phase bioreactors are designed such that cultured organs, propagules, or plants are submerged in the liquid medium within the culture vessel. Mixing of the medium and aeration is performed by mechanical stirring using impellers along with an air supply, or mixing is achieved pneumatically by supplying sterile air. Several liquid-phase bioreactors, such as stirred tanks, bubble columns, and airlift bioreactors, adopted for plant propagation are described below.
Conventional stirred-tank bioreactors consist of an impeller or agitator to stir the medium inside the container (Figure 1A). Frequently used stirrers are marine impellers and pinched blade turbines, which create axial fluid flow patterns at low speeds. Various ports for aeration, addition or removal of liquid medium, and distribution of oxygen and nutrients are provided (Mamun et al., 2015). These bioreactors were originally called fermenters and were designed to cultivate microorganisms. They have various advantages in terms of efficient fluid mixing, high oxygen mass transfer, and better control of pH, temperature, dissolved oxygen, and nutrient concentrations, which are ideal for cultivating microorganisms. However, high energy input, shear stress on cultured cells, issues with inoculation and harvesting of biomass, and contamination are the major disadvantages of these bioreactors (Table 1). Features such as high specific power input, high energy dissipation rate, turbulence around the agitator, and shear may damage the cultured plant tissues (Huang and McDonald, 2009). Nevertheless, mechanically agitated bioreactors have been used to culture bulblets of Lilium auratum and shoot primordia of Stevia rebaudiana (Takayama and Akita, 1994; Lim et al., 1998). Severe damage to shoots/propagules have been experienced owing to the high shear stress caused by mechanical agitation. Daucus carota somatic embryos have been cultured in stirred-tank reactors (3-L capacity with working volume of 1.7 L) by Jay et al. (1992) to study the effect of dissolved oxygen and pH on embryo growth; however, poor plantlet formation has been observed. Synchronization of growth of developing embryos and a decrease in the viability of harvested embryos have been reported by Ducos et al. (1993). To reduce shear forces, slow-speed stirring bioreactors have been developed by introducing thin silicone tubing hanging inside the periphery of bioreactors (Hvoslef-Eide et al., 2005) and somatic embryos of carrot, Norway spruce, birch, and cyclamen have been cultured. However, none of these modifications have resulted in healthy plantlets.
Figure 1 Configurations of different bioreactors used for plant propagation. (A) Stirred-tank bioreactor. (B) Bubble column bioreactor. (C) Airlift bioreactor. (D) Nutrient mist bioreactor. (E) Trickle bed bioreactor.
Table 1 Micropropagation of plants via organogenesis and somatic embryogenesis: Merits and demerits of bioreactor configurations.
Another conventional bioreactor is the pneumatically agitated bioreactor (Figure 1B). It consists of a cylindrical vessel with an air sparger at the bottom of the cylinder, and mixing and agitation are performed by rising air bubbles without any mechanical energy input. These bioreactors are efficient in terms of high heat and mass transfer and involve low operational and maintenance costs (Mamun et al., 2015). The absence of moving parts reduces the risk of contamination (Table 1). However, the demerits of these bioreactors are high foam formation under enhanced airflow rates, poor mixing of highly viscous fluids, and gas–liquid separation in the headspace region (Paek et al., 2001).
Another pneumatically agitated bioreactor is the airlift bioreactor that is operated by sparging air into a liquid medium placed at the base of the vessel. It contains an additional draft tube that is used to create an internal or external loop (Figure 1C). This creates a high circulation rate and oxygen supply for cultured cells (Mamun et al., 2015). The advantages of these bioreactors are easy maintenance and reduced risk of contamination owing to the absence of mobile parts. This reduces the effect of shear stress and maintains well-defined flow pattern of the medium, and these features are beneficial to produce secondary metabolites from cell suspension cultures (Mamun et al., 2015). The disadvantages of airlift reactors include excessive foam formation under high gas flow rates and relatively poor oxygen transfer capabilities (Paek et al., 2001; Valdiani et al., 2019).
These are modified bubble bioreactors (Figure 2D) containing balloon-shaped vessels with a concentric tube, with which a circular sparger is installed, and air passing through the sparger and tube helps to lift the cultured biomass at the raiser of the vessel bottom. They have a wide-open area/mouth at the top of a balloon-like cylinder, which helps in easy harvesting of biomass. At the bottom of the vessel, a ‘Y’- or ‘T’- shaped tube helps in collecting sample-suspended cells/biomass and medium. Various probes are used to monitor dissolved oxygen and hydrogen ion concentration (pH). Provision is made with inlet gas to mix oxygen, carbon dioxide, ethylene, or nitrogen with sterile air supplied to the bioreactors. These bioreactors have undergone several modifications for culturing cells, roots, somatic embryos, and organogenic propagules (Paek et al., 2001; Paek et al., 2005). Balloon-type bubble bioreactors are efficient in culturing organogenic propagules/storage organs, such as bulbs/bulblets, pseudobulbs, corms, and rhizomes (Table 2). Furthermore, with balloon-type bubble bioreactors, various modes of cultivation have been followed to produce organogenic propagules, such as the complete immersion method and raft method (in the raft method, propagules are grown in the bioreactor with a plastic net or raft; Figure 2E), in which the explants are in contact with the liquid medium at the base. In the ebb and flood method, medium is pumped from the storage tank into culture vessels (Figure 2F). A series of channels helps in evenly supplying nutrient solution to the plant materials, resulting in uniform growth. The medium remains in the vessel for a few minutes, after which it is drained back to the storage tank for reuse. The drainage process is controlled by a solenoid valve at intervals of 3–6 h depending on the programs set by the timer. In this process, explants/plant materials receive nutrients at regular time intervals at the same time they are in aerated conditions inside culture vessels. Kim et al. (2004) used balloon-type bubble bioreactors (5-L capacity and 4 L liquid medium), followed by immersion, raft, and ebb and flood methods to induce bulblets from shoot tip cultures (to produce virus-free bulblets) of Allium sativum (garlic). They could produce 4,049, 5,129, and 3,099 bulblets of varied sizes (0.02–2 gm) after 12 weeks of culture using immersion, raft, and ebb and flood methods, respectively. Balloon-type bubble bioreactors are efficient in culturing somatic embryos and protocorm-like bodies (PLBs; also, somatic embryos of orchids). Shohael et al. (2005) induced somatic embryogenesis in Eleutherococcus sessiliflorus and cultured 10 gm embryogenic cells aggregated in 3-L balloon-type bubble bioreactors containing 2-L liquid medium and obtained 128.8 gm mature somatic embryos within four weeks. Subsequently, they cultured 10 gm matured embryos in a liquid medium supplemented with gibberellic acid in balloon-type bubble bioreactors and obtained plantlets after four weeks. Similarly, Yang et al. (2010) induced PLBs in Oncidium ‘Sugar Sweet” orchid from shoot tip explants using balloon-type bubble bioreactors. They followed the immersion and ebb and flood methods to cultivate PLBs, and the maximum PLB biomass was obtained in the immersion culture method after 50 days. Furthermore, PLBs regenerated in the bioreactor were successfully developed into the plantlets.
Figure 2 Configurations of (A) Wave bioreactor, (B) Box-in-bag bioreactor, (C) Vertical-column bioreactor, (D) Balloon-type bubble bioreactor, (E) Balloon-type bubble bioreactor operating on raft method, (F) Balloon-type bubble bioreactor operating on ebb and flood method.
Table 2 Selected examples of propagation of bulblets, corms, microtubers, and rhizomes in bioreactor cultures.
In gas-phase bioreactors, cultured explants are maintained in ambient sterile air conditions and are intermittently supplied with liquid medium in the form of bubbles, spray, or mist. Gas-phase bioreactors were originally developed for hairy root cultures to overcome the limitations of oxygen supply, shear stress, and physical and physiological problems experienced by cultured roots, and such reactors were also used for plant propagation. The merits and demerits of gas-phase bioreactors for cultivation of organogenic propagules and somatic embryos are explained below.
Nutrient mist bioreactors are gas-phase bioreactors that provide in vitro plants with small droplets of culture medium fully infused with sterile gas generated as an aerosol into the growth chamber (Figure 1D). Mist bioreactors with various configurations promote growth with increased shoot and somatic embryogenesis. Correll and Weathers (2001a; 2001b) propagated Dianthus plants that were cultured in mist chambers with well-rooted plantlets and obtained quality plants with good physiological characteristics. Fei and Weathers (2014) cultured embryogenic cells of Daucus carrota (carrot) in a mist bioreactor and regenerated plants in a single culture system from early-stage embryos to germinated plantlets. Mist bioreactors offer excellent growth of embryos and plantlets when the liquid medium is delivered as an aerosol with droplets on the immobilized mesh. This offers the advantages of improved gas exchange, oxygen supply, and nutrient availability inside the growth chamber. However, such bioreactors have the disadvantages of scale up (Table 1).
In this type of bioreactors, medium is usually supplied from the top of the culture vessel through nozzles integrated into the headspace (Kim et al., 2002; Kuzma et al., 2009). Medium is supplied in the form of droplets that trickle over growing explants (shoots/somatic embryo biomass) that are maintained on the mesh or support. Spent medium is drained from the bottom of the bioreactor to a reservoir, and medium is recirculated at specific time intervals and rates (Figure 1E). Szopa et al. (2019) tested various systems, such as a cone bioreactor (continuous immersion system), cylindrical tube bioreactor (continuous immersion system), nutrient sprinkle bioreactor, and temporary immersion bioreactor (RITA and Plantform), to produce microshoots of Schisandara chinensis. Among them, culture in nutrient sprinkle bioreactor yielded the highest accumulated biomass. These results indicate that nutrient sprinkler bioreactors can also be used for shoot propagation. A disadvantage of these bioreactors is the difficulty in scaling up cultures (Table 1).
Temporary immersion bioreactors or temporary immersion systems (TIS) are designed for periodic immersion of cultured plant tissues or propagules in liquid medium, followed by draining and exposing plant tissues to a sterile gaseous environment. Majority of TIS contains two or more compartments in the container or separate vessels; medium is pushed from a reservoir compartment/vessel to a compartment/vessel in which explants or plants are cultured. Usually, immersion period can be programmed from a few minutes to a few hours. An air pump and solenoid valve are used to circulate the medium. An automated TIS was first developed by Tisserat and Vandercook (1985), and was designated as an automated plant culture system, in which shoot tips of orchids, aster, cow tree, callus of date palm, and carrot were cultured. Better growth of plants and calli was noticed in the automated system than in the manual system. Subsequently, several systems were developed by Aitken-Christie and Davies (1988); Simonton et al. (1991); Alvard et al. (1993, RITA system), and Escalona et al. (1999, twin flask or BIT system). TISs are free from contamination and help propagules with an adequate supply of nutrients. They can overcome the issues of hyperhydricity in cultured plant tissues by creating conditions for optimal humidity (Alabarran et al., 2005). The gaseous environment in TISs overcomes the issue of oxygen limitation. Furthermore, excellent growth and involvement of propagules can be achieved by enriching the percentage of CO2 and light intensity in culture vessels (Aragon et al., 2005; Aragon et al., 2010; Curtis and Tuerk, 2008). Trauger et al. (2022) also showed how CO2 enrichment affected the growth of cocoa somatic embryos and yam nodal cultures. According to their findings, increased CO2 during plant propagation considerably enhanced the development of cocoa and yam propagules and eliminated the requirement for added sugars in the tissue culture growth medium. The designs and operations of various TISs are briefly described here, and detailed configurations of TIS, principles, and technological advances have been presented in previous reviews (Etienne and Berthouly, 2002; Afreen, 2006; Watt, 2012; Georgiev et al., 2014; Garcia-Ramirez, 2023).
The twin-flask system consists of two vessels connected by silicone tubing (Figure 3A). One vessel works as a culture chamber, and the other is a medium reservoir. The vessels are connected to airlines and controlled by timers and solenoid valves. This system maintains constant sterility and allows easy cultivation of plant propagules for a long period. Demerits of this system include a lack of operation for renewal of growth medium, forced ventilation, and automation processes (Georgiev et al., 2014). Shoots, nodule clusters, and embryos have been cultivated using this system. For example, Niemenak et al. (2008) cultured somatic embryos of Theobroma cacao in a twin-flask system, and subsequently, matured embryos were converted into plants after sowing. Recently, Orozco-Ortiz et al. (2023) tested three temporary immersion systems namely RITA, SETIS, and BIT systems in addition to a semisolid medium for the multiplication of sugarcane (Saccharum spp. variety LAICA 04-809) shoots. Of all the temporary immersion cultures BIT bioreactor generated the highest quality of shoots without hyperhydricity.
Figure 3 Configurations of temporary immersion bioreactors. (A) Twin flask bioreactor. (B) Ebb and flow bioreactor. (C) RITA bioreactor. (D) Rocker system. (E) Bio-MINT bioreactor. (F) RALM bioreactor. (G) SETIS bioreactor. (H) PLANTIMA system. (I) PLANTFORM bioreactor.
This system consists of two vessels interconnected by silicone tubing; the larger vessel acts as a culture vessel, and the smaller vessel is used for storing liquid medium or as a medium reservoir (Figure 3B). Medium is supplied from the reservoir to the main culture vessel using an air pump, solenoid valve, and timer. With the main vessel, a plastic or polypropylene net is sometimes used as a raft to hold materials away from the container. The main vessel is aerated using sterile air through a sparger during immersion period. This system is simple and reliable; however, supplementation of gases, such as CO2 and ethylene, is a limitation of these bioreactors (Georgiev et al., 2014). This system has been used to propagate shoots, bulblets, cormlets, PLBs, and regeneration plants (Chakrabarty et al., 2003; Lian et al., 2003a; b; Jo et al., 2008, Park et al., 2000). Lian et al. (2003a) compared semisolid cultures with liquid cultures using an ebb and flow system for propagation of Lilium bulblets and obtained 81 and 1,025 bulblets respectively. These results demonstrate the superiority of the ebb and flow system over semi-solid cultures.
The Recipient for Automated Temporary Immersion system (RITA) was developed by Teisson and Alvard (1995) (supplied by VITROPIC, France), which is a single autoclavable polypropylene vessel with two compartments (Figure 3C). The upper compartment is the culture vessel, and the lower one is the medium reservoir. The two compartments are interconnected, and flow of medium is controlled by a solenoid valve and timer. These systems are convenient for handling and culturing; however, no provisions for medium renewal and forced ventilation are some issues (Georgiev et al., 2014). RITA has been used for the propagation of plants such as lingonberry, lowbush blueberry, and plum (Debnath, 2008; Debnath, 2009; Arigundam et al., 2020; Gogo et al., 2022). However, some plants regenerated in this system develop hyperhydricity; therefore, this system requires further improvement of air circulation.
In this system, culture vessels or boxes are kept on a platform that tilts by mechanical energy. Explants are normally placed directly into the culture vessel or placed using different support materials, such as nets, rock wool cubes, and polyurethane foam. Medium reaches the explants through mechanical moment of the entire vessel (Figure 3D). The cultivation vessels or boxes are made of polycarbonate and are usually rectangular in shape with lateral mouth openings. These systems might be useful in some types of plant cultures; however, they are electromagnetically driven and involve energy and investment costs (Georgiev et al., 2014). A liquid Lab rocker system is used to produce microtubers in potatoes (Kamarainen-Karppinen et al., 2010).
The newly developed BioMINT systems consist of two cylindrical polycarbonate vessels that are fixed opposite to each other (Figure 3E). Of the two vessels, one is used for culturing explants/plants, and the other is the medium reservoir. Medium flows through adaptors from one to another vessel and facilitates imbibing of cultures alternately. CO2 enrichment is accomplished through a port. These systems have been used for in vitro proliferation and elongation of pepper shoots (Bello-Bello et al., 2010) and micropropagation of Cedar plants (Pena-Ramirez et al., 2010).
RALM (Ralm Industries, Brazil) (Figure 3F) is a twin-flask system. SETIS (Vervit, Belgium) (Figure 3G) operates on the principle of an ebb and flow system. PLANTIMA (A-Tech Bioscientific Co., Ltd., Taiwan) (Figure 3H) and PLANTFORM (Plant Form AB, Sweden & TC propagation Ltd., Ireland) (Figure 3I) work on the principle followed by RITA. These systems have been used to propagate plants such as Dioscorea alata (Yan et al., 2011), Siraitia grosvenorii (Yan et al., 2010), Chrysanthemum morifolium, Fragaria x ananassa, and Cnidium officinale (Hwang et al., 2022).
The idea behind wave bioreactors is wave-induced agitation of cultures. This device loads culture bags onto a rocking platform that generates waves inside the bags to enable the contents (medium and cultured propagules) to mix and shake and facilitate a liquid or gaseous environment (Eibl et al., 2009; Eibl et al., 2010; Figure 2A). Changes can be made to the operating conditions such as the rocking rate, angle, aeration rate, and dimension of culture bags. The term “disposable bioreactors” refers to culture bags that are typically composed of polyethylene, polystyrene, polytetrafluoroethylene, polypropylene, or ethylene vinyl acetate and are delivered in a presterilized state (Eibl et al., 2010). Without foaming and shear stress, aeration and oxygen transfer may be effectively accomplished in these systems. Online monitoring options are available for temperature, pH, and dissolved oxygen. However, these bioreactors are expensive.
The modified version called “Box-in-bag” temporary immersion system (10-L capacity) has been developed by Ducos et al. (2009) specifically to propagate Coffea canephora by culturing somatic embryos. The bags are made of a transparent film composed of polyethylene and nylon and are supplied closed on three sides. They are 750 × 420 mm in size and have two polyphenylene ports molded into the film (one above and another below). A rigid transparent box of 50 × 30 × 10 cm made of polycarbonate is kept inside the bag. Port A is connected to the inoculum chamber, and port B is connected to the medium reservoir below (Figure 2B). The entire system is kept in a culture room and is loaded with 5 L culture medium along with the inoculum. Ducos et al. (2009) have used these bioreactors to convert torpedo-stage embryos into plantlets after germination.
These are vertical column-shaped bioreactors with a capacity of 10 L, in which a circular sparger is placed at the bottom of the bioreactors, and sterile air is pumped from the basal region of the bioreactors (Figure 2C). These are modified bubble column bioreactors, in which a net is provided above the sparger to place propagules/explants. Ports are used for inlet and outlet of medium at the base of culture vessels. Additional ports are available for supplying gases, such as CO2, and they can be easily modified to work on the ebb and flow principles. These bioreactors are quite useful in cultivating plants that can grow vertically, and nodal cuttings can be prepared for ex vitro rooting of shoots from these in vitro grown plants. Such bioreactors are useful in producing chrysanthemum shoots (Hahn and Paek, 2005) and microtubers in potatoes (Piao et al., 2003).
Micropropagation of plants in vitro is affected by several factors, including selection and source of explants, selection of medium, optimization of chemical factors of medium, such as macroelements and microelements, concentration and type of sugars, type and concentration of phytohormones, and physical factors such as light, photoperiod, temperature, and humidity; these factors should be optimized for particular species considered for regeneration by using semisolid cultures. Bioreactors offer some advantages for culturing propagules, particularly, large volumes for cultivation, providing nutrients optimally to the developing propagules, and facilitating physical conditions that are involved in morphogenetic events. Micropropagation is achieved in bioreactors mostly in batch mode; however, the mode of differentiation and development, i.e., the process involved in multiplication of propagules, shoots, and somatic embryos, varies in different plant species. For example, cultured explants may be involved in the differentiation of bulblets, cormlets, or rhizomes, as in the case of onions, garlic, lilies, and orchids. Similarly, explants may be involved in regenerating axillary or adventitious shoot and the subsequent rooting of shoots, as in the case of several crops and ornamentals and medicinal plants. Some plants propagate via somatic embryogenesis that involves the induction, differentiation, maturation, and germination of embryos. Therefore, depending on the mode of regeneration, operating parameters, such as replenishment of medium (control of medium factors), mode of operation (continuous immersion, periodic immersion, or deep flow method), substrate or inoculum density, control of the gaseous environment (oxygen, carbon dioxide, and ethylene), shear stress, and light are essential, even in bioreactor cultures.
Various factors affect the regeneration of propagules in bioreactor cultures; therefore, selection of bioreactor and mode of operation are very important. Lian et al. (2014) investigated the regeneration of bulblets from the bulb-scale segments of various Lilium cultivars. They selected 5-L balloon-type bubble bioreactors with 1 L Murashige and Skoog medium supplemented with 0.3 mg·L-1 naphthalene acetic acid, 1 mg·L-1 benzyl adenine, and 30 gm·L-1 sucrose and adopted continuous immersion and ebb and flow for regeneration of bulblets of Lilium ‘Casa Blanca’. No bulblet regeneration was observed with continuous immersion, whereas in the ebb and flow method, explants developed bulblet regeneration. Furthermore, they tested the effects of the number (four, six, and eight times per day for 30 min immersion in liquid medium) and duration (four times per day for 15, 30, 60, and 120 min) of medium supply on the regeneration of bulblets with ebb and flow type bioreactors. They reported the highest percentage of bulblet formation (75.8%) when medium was supplied four times a day for 15 min.
Lian et al. (2014) conducted several experiments on Lilium bulblet enlargement/growth after induction in vitro, because bulblets that had reached a minimum size of 1 gm could successfully acclimatize after transplantation of potting medium. They cultured 0.1-gm-sized bulblets in Balloon-type bubble bioreactors (BTBBs) using continuous immersion and temporary immersion (ebb and flow) and reported that a continuous immersion system was superior to temporary immersion during the bulblet enlargement/growth stage (Figure 4C). Lian et al. (2014) reported that inoculation density, aeration, temperature, and light intensity had a profound influence on growth (enlargement of bulblets) in bioreactor cultures. Enlargement of Lilium bulblets in vitro in liquid medium/culture is a lengthy process and takes 16 weeks. They also studied the kinetics of nutrient uptake by bulblets during bioreactor cultures and observed depletion of several minerals and sugars in the medium every four weeks. Therefore, they adopted the fed-batch culture method, that is, supplementation of fresh medium with cultures, and could mass-propagate several Lilium cultivars.
Figure 4 Application of bioreactors for micropropagation. (A, B) Somatic embryos of Siberian ginseng cultured in balloon-type bubble bioreactor. (C) Lilium bulblets cultured in balloon-type bubble bioreactor. (D) Phalaenopsis PLBs cultured in balloon-type bubble bioreactor with raft system. (E) Garlic bulblets harvested from balloon-type bubble bioreactor. (F) Potato microtubers developed in vertical column bioreactors. (G) Chrysanthemum plants in the greenhouse. (H, I) Chrysanthemum plantlets after ex vitro rooting by hydroponic method and direct planting to the potting medium by dipping in indole acetic acid powder.
Piao et al. (2003) tested three different bioreactor systems, including temporary immersion using ebb and flow, continuous immersion with net/raft, or without a net, to produce microtubers of potato ‘Atlantic’. Again, factors, such as inoculum density and medium exchange, affected tuber size during shoot multiplication and tuber induction stages. Table 2 presents several examples of regeneration of bulblets, corms/cormlets, minitubers, and rhizomes in bioreactor cultures.
Regeneration of axillary and adventitious shoots has been achieved in various plant species using bioreactor systems (Table 3), and such regenerated shoots have been used for in vitro or ex vitro rooting. However, in certain other plant species, shoot induction and root regeneration has been simultaneously achieved (Jeon et al., 2009; Thul and Kukreja, 2010). Among various bioreactors, temporary immersion systems have been found to be suitable for shoot regeneration, and continuous immersion systems have not been found to be suitable for axillary or adventitious shoot regeneration because these systems induce asphyxia and hyperhydricity of regenerated shoots (Etienne and Berthouly, 2002; Chakrabarty et al., 2003). Varied factors such as inoculum density, aeration volume, and light intensity that affect shoot regeneration should be thoroughly analyzed in certain bioreactor systems. For example, Jin et al. (2013) applied balloon-type bubble bioreactors to propagate grape rootstocks and analyzed the effects of inoculum density, air volume/aeration, and light intensity on plant growth. Inoculum density is an important physical parameter that influences growth during micropropagation. An optimum inoculation density is necessary for dynamic growth and proliferation of cultures in vitro. Hahn and Paek (2005) found 80 nodes as the best inoculum density for shoot multiplication of chrysanthemum in bioreactors. Piao et al. (2003) reported the maximal growth of potatoes when 50 nodal explants were cultured in a bioreactor. Similarly, Jin et al. (2013) reported that an initial inoculum density of 65 nodes was suitable for regenerating axillary shoots. Air volume is responsible for medium circulation and oxygen transfer in explants (Wu et al., 2011). A lower aeration volume may be responsible for oxygen deficiency, and higher aeration has been reported to be owing to the stripping off of essential gases such as carbon dioxide and ethylene (Gao and Lee, 1992). An air volume of 150 mL·min-1 was found to be appropriate for shoot regeneration in nodal cultures of grape with varied air volumes (50, 100, 150, and 200 mL·min-1). Light intensity is another important factor affecting the growth and photosynthesis of cultured propagules (Mukherjee et al., 2010). Of various light intensities (30, 50, and 70 µmol·m-2·s-1) tested by Jin et al. (2013), 50 µmol·m-2·s-1 was found to be suitable for regeneration of grape plants using balloon-type bubble bioreactors. RITA and SETIS temporary immersion cultures have been used to regenerate horticultural and medicinal plants (Monja-Mio et al., 2021; Hwang et al., 2022).
Gaseous environment, shear stress, and light are major factors that affect the formation and culture of somatic embryos in small-scale cultures and bioreactors (Fei and Weathers, 2016). Oxygen, carbon dioxide, and ethylene are the major gases that affect the induction, differentiation, and maturation of somatic embryos (Shigeta et al., 1996; El Meskaoui and Tremblay, 2001; Fisichella and Morini, 2003). A comparatively low oxygen concentration stimulates somatic embryogenesis in wheat (Carman, 1988). In contrast, elevated carbon dioxide (0.3–5%) improves somatic embryo formation (Buddendorf-Joosten and Woltering, 1994; Huang et al., 2006). Ethylene is detrimental during somatic embryogenesis (Jimenez, 2005). Therefore, controlling the gaseous environment of bioreactors during somatic embryo culture is essential. Mixing embryogenic cultures without shear stress is critical for bioreactor cultures. Conventional bioreactors, such as stirred tank bioreactors, create excess shear stress, whereas airlift bioreactors develop foaming within growth chambers; consequently, balloon-type bioreactors are found to be suitable for cultivating somatic embryogenesis (Park et al., 2000; Park et al., 2005; Shohael et al., 2014) in which gaseous environments can be controlled very easily. These bioreactors are attached to cylinders of oxygen, carbon dioxide, and ethylene, and the concentration of these gases is properly controlled and monitored (Jeong et al., 2006; Shohael et al., 2014). Light inhibits the induction and development of somatic embryogenesis. For example, in carrot and Siberian ginseng suspension cultures, darkness induces the production of somatic embryos (Michler and Lineberger, 1987; Shohael et al., 2014). In contrast, red light induces a higher frequency of somatic embryogenesis (D’Onofrio et al., 1998). For coffee, fluorescence is essential, particularly during embryo germination (Ducos et al., 2009). Bioreactor cultures for plant regeneration via somatic embryogenesis have been extensively studied (Table 4). Temporary immersion RITA, box-in-bag, and temporary root zone immersion bioreactors have been used for regenerating Coffea arabica, C. canephora, and C. arabusta plants, respectively (Etienne-Barry et al., 1999; Afreen et al., 2002; Ducos et al., 2009). Balloon-type bubble bioreactors have been used for culturing somatic embryos and for subsequent plant regeneration (Park et al., 2000; Park et al., 2005; Shohael et al., 2014). Somatic embryos of orchids, called PLBs, have also been cultured in balloon-type bubble bioreactors for regenerating plants (Park et al., 2000; Yang et al., 2010).
Table 4 Selected examples of multiplication of somatic embryos and plant regeneration in bioreactors.
Several decades of studies have demonstrated that liquid cultures are superior to semi-solid cultures for micropropagation of plants because they facilitate fast growth rates, rapid uptake of nutrients by propagules, and involvement of their growth and differentiation. In contrast, the application of bioreactor cultures for micropropagation is useful for large-scale propagation of plants and to reduce production costs (Paek et al., 2005; Etienne and Berthouly, 2002; Georgiev et al., 2014; Mamun et al., 2015). Propagules, such as bulblets, cormlets, pseudobulbs, rhizomes, and microtubers, regenerated in bioreactors do not face the issues of hyperhydricity and can be directly used for in vivo transplantation. However, shoots, embryos, and plantlets regenerated in bioreactor cultures (immersion and temporary immersion cultures) are sometimes hyperhydric and show morphological, anatomical, and biochemical variations. This affects up to 30–70% loss of micropropagated plants upon transplantation to in vivo conditions (Debnath, 2008; Snyman et al., 2011). Increased ventilation and/or gas supply may control hyperhydricity (Chakrabarty et al., 2003). In addition, the enrichment of carbon dioxide in a bioreactor vessel facilitates the growth of shoots and results in healthy plantlets of sweet potato, potato, and chrysanthemum and Chinese fox gloves (Paek et al., 2001; Paek et al., 2005). Aragon et al. (2010) reported that enhanced light intensity in the presence of carbon dioxide enrichment improved leaf and root growth in Musa culture using temporary immersion cultures. Therefore, forced ventilation increases carbon dioxide concentration, and enrichment of light intensity in bioreactors may facilitate the growth of regenerated propagules. Moreover, the usage of CO2-enriched air could assist developing propagules in engaging in autotrophy and enhance the physiological and anatomical circumstances of developing plantlets (Xiao et al., 2011; Nguyen et al., 2020; Gago et al., 2021). Additionally, photosynthesis can be improved in bioreactors with forced ventilation, eliminating the need for medium sugars and reducing microbial contamination, producing plants that are physiologically healthier and better suited for acclimatization (Xiao et al., 2011; Nguyen et al., 2020; Rico et al., 2022). Figure 4 shows examples of the propagation of several plants in bioreactors on a large scale.
Micropropagation using a bioreactor system using liquid cultures is a reliable system for large-scale propagation of plants with genetic uniformity, however, sometimes regenerated plants do exhibit genetic variability and this might be due to various factors. The method of plant regeneration, genotype, age of the culture, explant selection, and culture conditions are all crucial elements for the genetic stability of regenerated plants (Rani and Raina, 2000). Direct regeneration shoots, embryos, and propagules such as bulbs, corms, and protocorms invariably maintain genetic stability compared to callus-mediated regeneration (Dale et al., 2008). Evaluation of the genetic purity of regenerated plants is crucial because both genetic (heritable) and epigenetic (non-heritable) variations are documented with in vitro regenerated plants. In vitro regenerated plants’ genetic homogeneity has been evaluated at the morphological, physiological, biochemical, and genetic levels. Plants produced from tissue culture have been characterized using DNA fingerprinting and several DNA-based markers. For the genetic analysis of tissue-cultured plants, a variety of newly developed special markers have been used, including amplified fragment length polymorphism (AFLP), sequence-tagged sites (STSs), arbitrarily primed polymerase chain reaction (AP-PCR), DNA amplification fingerprinting (DAE), and inter-simple sequence repeat (ISSR) (Rani and Raina, 2000). For instance, Snyman et al. (2011) evaluated sugarcane plants grown in temporary immersion bioreactors in the field and found many variances during the first six months of growth, such as variations in stem diameter and length, but these differences vanished as culture time was extended. They used AFLP analysis to characterize sugarcane plants that had been micropropagated and reported modest levels of polymorphism (0-0.9%). However, bioreactor-cultivated American ginseng and date palm plants have displayed typical phenotypic traits (Fki et al., 2011; Uchendu et al., 2011).
In bioreactors, a liquid medium is utilized to culture plant explants for regenerating elite clones. Bioreactors provide ideal conditions for mixing cultured tissues with a culture medium, and maintain optimal culture conditions, such as aeration (oxygen, carbon dioxide, and other essential gases), temperature, pH, and illumination regimes, so that cultured tissues grow and develop for regeneration of plants via organogenesis or embryogenesis. During plant regeneration, a particular bioreactor does not meet the needs of all short cultures, and immersion-type bioreactors are useful for the regeneration of bulblets, cormlets, rhizomes, and microtubers. Temporary immersion cultures are ideal for regenerating axillary and adventitious shoots and their subsequent rooting. A single bioreactor does not meet all needs of embryogenic cultures because concrete steps, such as induction, differentiation, development, maturation, and germination of somatic embryos, exist. Consequently, different bioreactors are used for different stages to successfully regenerate plants via somatic embryogenesis. Future studies should be focused on the adoption of large-scale cultures based on the plant type and mode of regeneration. For the regeneration of bulblets, cormlets, rhizomes, and microtubers adoption of fed-batch cultures are suitable since many nutrients are consumed by growing propagules on a selective/requirement basis. Additionally, changing the composition of the medium—particularly the level of carbohydrates—can aid in getting the best yield and lowering the cost of growing plantlets. The problem of morphological, anatomical, and physiological abnormalities is a bottleneck for plants regenerated in liquid cultures. Although temporary immersion systems eliminate these issues, modifications, including improved aeration, adequate supplementation of essential gases, and balanced light penetration through bioreactors, are necessary. Containers made of glass or other materials that are efficient in light penetration, proper aeration/supplementation designs, and mixing of cultures without endangering aseptic nature are essential. Containers should also be simplistic for the inoculation of explants, transfer of cultures, maintenance, and harvesting of regenerated propagules. Scope for scale-up is necessary, which may efficiently reduce the cost of production
HM, KJ, KP, and SP designed the study. HM has written the manuscript. KJ has developed figures. KP and SP supervised research work. All authors contributed to the article and approved the submitted version.
This work was supported by a National Research Foundation of Korea (NRF) grant funded by the Korean government (MSIT) (No. NRF-2020R1A2C2102401) and Technology Innovation Program funded by the Ministry of Trade, Industry & Energy (MOTIE, Korea) (Grant No. P0018148).
Hosakatte Niranjana Murthy is thankful for the “Brain Pool” (BP) program, Grant No. 415 2022H1D3A2A02056665.
The authors declare that the research was conducted in the absence of any commercial or financial relationships that could be construed as a potential conflict of interest.
All claims expressed in this article are solely those of the authors and do not necessarily represent those of their affiliated organizations, or those of the publisher, the editors and the reviewers. Any product that may be evaluated in this article, or claim that may be made by its manufacturer, is not guaranteed or endorsed by the publisher.
Afreen, F. (2006). “Temporary immersion bioreactor,” in Plant tissue culture engineering. Eds. Gupta, S. D., Ibaraki, Y. (Dordrecht, The Netherlands: Springer), 187–201. doi: 10.1007/978-1-4020-3694-1_11
Afreen, F., Zohayad, S. M. A., Kozai, T. (2002). Photoautotrophic culture of Coffea arabusta somatic embryos: development of a bioreactor for large-scale plantlet conversion from cotyledonary embryos. Ann. Bot. 90, 21–29. doi: 10.1093/aob/mcf151
Aitken-Christie, J., Davies, H. E. (1988). Development of a semi-automated micropropagation system. Acta Hortic. 230, 81–88. doi: 10.17660/ActaHortic.1988.230.7
Akita, M., Takayama, S. (1994). Induction and development of potato tubers in a jar fermenter. Plant Cell Tiss. Organ Cult. 36, 177–182. doi: 10.1007/BF00037717
Alabarran, J., Bertand, B., Lartaud, M., Etienne, H. (2005). Cycle characteristics in a temporary immersion bioreactor affect regeneration, morphology, water, and mineral status of coffee (Coffea arabica) somatic embryos. Plant Cell Tiss. Organ Cult. 81, 27–36. doi: 10.1007/s11240-004-2618-8
Alvard, D., Cote, T., Teisson, C. (1993). Comparison of methods of liquid medium culture for banana micropropagation: effects of temporary immersion of explants. Plant Cell Tiss. Organ Cult. 32, 55–60. doi: 10.1007/BF00040116
Aragon, C. E., Escalona, M., Capote, I., Pina, D., Cejas, I., Rodriguez, R., et al. (2005). Photosynthesis and carbon metabolism in plantain (Musa AAB) growing in temporary immersion bioreactor (TIB) and ex-vitro acclimatization. In Vitro Cell. Dev. Biol. Plant 41, 550–554. doi: 10.1079/IVP2005640
Aragon, C. E., Escalona, M., Rodriguez, R., Canal, M. J., Capote, I., Pina, D., et al. (2010). Effect of sucrose, light, and carbon dioxide on plantain micropropagation in temporary immersion bioreactors. In Vitro Cell. Dev. Biol. Plant 46, 89–94. doi: 10.1007/s11627-009-9246-2
Arigundam, U., Variyath, A. M., Siow, Y. L., Marshall, D., Debnath, S. C. (2020). Liquid culture for efficient in vitro propagation of adventitious shoots in wild Vaccinium vitis-idaea ssp. minus (lingonberry) using temporary immersion and stationary bioreactors. Sci. Hortic. 264, 109199. doi: 10.1016/j.scienta.2020.109199
Arnold, S. V., Sabla, I., Bozhkov, P., Dyachok, J., Filonova, L. (2002). Developmental pathways of somatic embryogenesis. Plant Cell Tiss. Organ Cult. 69, 233–249. doi: 10.1023/A:1015673200621
Bello-Bello, J. J., Canto-Flick, A., Balam-Uc, E., Gomez-UC, E., Robert, M. L. (2010). Improvement of in vitro proliferation and elongation of habanero pepper shoot (Capsicum chinense jacq.) by temporary immersion. HortScience 45, 1093–1098. doi: 10.21273/HORTSCI.45.7.1093
Buddendorf-Joosten, J. M. C., Woltering, E. J. (1994). Components of the gaseous environment and their effects on plant growth and development in vitro. Plant Growth Regul. 15, 1–16. doi: 10.1007/BF00024671
Carman, J. G. (1988). Improved somatic embryogenesis in wheat by partial simulation of the in-ovulo oxygen, growth-regulator and desiccation environments. Planta 175, 417–424. doi: 10.1007/BF00396349
Chakrabarty, D., Hahn, E. J., Yoon, Y. J., Paek, K. Y. (2003). Micropropagation of apple root stock M.9 EMLA using bioreactor. J. Hortic. Sci. Biotechnol. 78, 605–609. doi: 10.1080/14620316.2003.11511671
Correll, M. J., Weathers, P. J. (2001a). One-step acclimatization of plantlets using a mist reactor. Biotechnol. Bioeng. 73, 253–258. doi: 10.1002/bit.1058
Correll, M. J., Weathers, P. J. (2001b). Effects of light, CO2, and humidity on carnation growth, hyperhydration and cuticular wax development in a mist reactor. In vitro cell. dev. Biol. Plant 37, 405–413. doi: 10.1007/s11627-001-0071-5
Cuenca, B., Sanchez, C., Aldrey, A., Bogo, B., Blanco, B., Correa, B., et al. (2017). Micropropagation of axillary shoots of hybrid chestnut (Castanea sativa x C. crenta) in liquid medium in a continuous immersion system. Plant Cell Tissue Organ Cult. 131, 307–320. doi: 10.1007/s11240-017-1285-5
Curtis, W. R. (2005). Application of bioreactor design principles to plant micropropagation. Plant Cell Tiss. Organ Cult. 81, 255–264. doi: 10.1007/s11240-004-6646-1
Curtis, W. R., Tuerk, A. L. (2008). "Oxygen transport In plant tissue culture systems: Oxygen transport limitations", in Plant tissue culture engineering, Eds. Dutta Gupta, S., Ibaraki, Y. (Dordrecht: Springer), 173–186. doi: 10.1007/978-1-4020-3694-1_10
Dale, A., Hughes, B. R., Donnelly, D. (2008). The role of micropropagation in producing specific pathogen-tested plants. HortScience 43, 74–77. doi: 10.21273/HORTSCI.43.1.74
Debnath, S. C. (2008). Developing a scale-up system for the in vitro multiplication of thidiazuron-induced strawberry shoots using a bioreactor. Can. J. Plant Sci. 88, 737–746. doi: 10.4141/CJPS07147
Debnath, S. C. (2009). A scale-up system for lowbush blueberry micropropagation using a bioreactor. HortScience 44, 1962–1966. doi: 10.21273/HORTSCI.44.7.1962
Dewir, Y. H., Alsadon, A., Al-Aizari, A. A., Al-Mohidib, M. (2022). In vitro floral emergence and improved formation of saffron daughter corms. Horticulturae 8, 973. doi: 10.3390/horticulturae8100973
D’Onofrio, C., Morini, S., Bellocchi, G. (1998). Effect of light quality on somatic embryogenesis of quince leaves. Plant Cell Tiss. Organ Cult. 53, 91–98. doi: 10.1023/A:1006059615088
Ducos, J. P., Bollon, H., Pettard, V. (1993). Production of carrot somatic embryos in a bioreactor. Appl. Microbiol. Biotechnol. 39, 465–470. doi: 10.1007/BF00205034
Ducos, J. P., Terrier, B., Courtois, D. (2009). Disposable bioreactors for plant micropropagation and mass plant cell culture. Adv. Biochem. Eng. Biotechnol. 115, 89–115. doi: 10.1007/10_2008_28
Eibl, R., Kaiser, S., Lombriser, R., Eibl, D. (2010). Disposable bioreactors: the current state-of-art and recommended applications in biotechnology. Appl. Microbiol. Biotechnol. 86, 41–49. doi: 10.1007/s00253-009-2422-9
Eibl, R., Werner, S., Eibl, D. (2009). Disposable bioreactors for plant liquid cultures at litre-scale. Eng. Life Sci. 9, 156–164. doi: 10.1002/elsc.200800102
Ekmekcigil, M., Bayraktar, M., Akkus, O., Gurel, A. (2019). High-frequency protocorm like bodies and shoot regeneration through a combination of thin cell layer and RITA® temporary immersion bioreactor in Cattleya forbesii lindl. Plant Cell Tissue Organ Cult. 136, 451–464. doi: 10.1007/s11240-018-1526-2
El Meskaoui, A., Tremblay, F. M. (2001). Involvement of ethylene in the maturation of black spruce embryogenic cell lines with different maturation capacities. J. Exp. Bot. 52, 761–769. doi: 10.1093/jexbot/52.357.761
Escalona, M., Lorenzo, J. C., Gonzales, B. L., Daquinta, M., Borroto k, C. G., Gonzales, J. I., et al. (1999). Pineapple (Ananas cosmos l. merr) micropropagation in temporary immersion systems. Plant Cell Rep. 18, 743–748. doi: 10.1007/s002990050653
Espinosa-Leal, C., Puente-Garza, C. A., Gracia-Lara, S. (2018). In vitro plant tissue culture: means for production of biological active compounds. Planta 248, 1–18. doi: 10.1007/s00425-018-2910-1
Etienne, H., Berthouly, M. (2002). Temporary immersion systems in plant micropropagation. Plant Cell Tiss. Organ Cult. 69, 215–231. doi: 10.1023/A:1015668610465
Etienne-Barry, D., Bertrand, B., Vasquez, N., Etienne, H. (1999). Direct sowing of Coffea arabica somatic embryos mass-produced in a bioreactor and regeneration of plants. Plant Cell Rep. 19, 111–117. doi: 10.1007/s002990050720
Fei, L., Weathers, P. J. (2014). From cells to embryos to rooted plantlets in a mist bioreactor. Plant Cell Tiss. Organ Cult. 116, 37–46. doi: 10.1007/s11240-013-0380-5
Fei, L., Weathers, P. (2016). “Bioreactors for plant embryogenesis and beyond,” in In vitro embryogenesis in higher plants, method in molecular biology. Eds. Germana, M. A., Lambardi, M. (New York: Springer), 245–258. doi: 10.1007/978-1-4939-3061-6_10
Fisichella, M., Morini, S. (2003). Somatic embryo and root regeneration from quince leaves cultured in ventilated vessels or under different oxygen and carbon dioxide levels. In Vitro Cell. Dev. Biol. Plant 39, 402–408. doi: 10.1079/ivp20033429
Fki, L., Bouaziz, N., Kriaa, W., Benjamaa-Masmoudi, R., Gargouri-Bouzid, R., Rival, A., et al. (2011). Multiple bud cultures of ‘Barhee’ date palm (Phoenix dactylifera) and physiological status of regenerated plants. J. Plant Physiol. 168, 1694–1700. doi: 10.1016/j.jplph.2011.03.013
Gago, D., Vilavert, S., Bernal, M. A., Sanchez, C., Aldrey, A., Vidal, N. (2021). The effect of sucrose supplementation on the micropropagation of Salix viminalis l. shoots in semisolid medium and temporary immersion bioreactors. Forests 12, 1408. doi: 10.3390/f12101408
Gao, J., Lee, J. M. (1992). Effect of oxygen supply on the suspension cultures of genetically modified tobacco cells. Biotechnol. Prog. 8, 285–290. doi: 10.1021/bp00016a004
Gao, H., Li, J., Ji, H., An, L., Xia, X. (2018). Hyperhydricity -induced ultrastructural and physiological changes in blueberry (Vaccinium spp.). Plant Cell Tissue Organ Cult. 133, 65–76. doi: 10.1007/s11240-017-1361-x
Gao, R., Wu, S. Q., Piao, X. C., Park, S. Y., Lian, M. L. (2014). Micropropagation of Cymbidium sinense using continuous and temporary airlift bioreactor systems. Acta Physiol. Plant 36, 117–124. doi: 10.1007/s11738-013-1392-9
Georgiev, M. I. (2014). “Design of bioreactors for plant cell organ cultures,” in Production of biomass and bioactive compounds using bioreactor technology. Eds. Paek, K. Y., Murthy, H. N., Zhong, J. J. (Dordrecht: Springer), 3–14. doi: 10.1007/978-94-017-9223-3_1
Georgiev, V., Schumann, A., Pavlov, A., Bley, T. (2014). Temporary immersion systems in plant biotechnology. Eng. Life Sci. 14, 607–621. doi: 10.1002/elsc.201300166
Gogo, D., Sanchez, C., Aldrey, A., Christie, C. B., Bernal, M. A., Vidal, N. (2022). Micropropagation of plum (Prunus domestica l.) in bioreactors using photomixotrophic and photoautotrophic conditions. Horticultrurae 8, 286. doi: 10.3390/horticulturae8040286
Garcia-Ramirez, Y. (2023). Temporary immersion system for in vitro propagation via organogenesis of forest plant species. Trees. doi: 10.1007/s00468-022-02379-w
Garcia-Ramirez, Y., Barrera, G. P., Freire-Seijo, M., Barbon, R., Cancepcion-Hernandez, M., Mendoza-Rodriguez, M. F., et al. (2019). Effect of sucrose on physiological and biochemical changes of proliferated shoots of Bambusa vulgaris schrad. ex wendl in temporary immersion. Plant Cell Tissue Organ Cult. 137, 239–247. doi: 10.1007/s11240-019-01564-z
Hahn, E. J., Paek, K. Y. (2005). Multiplication of chrysanthemum shoots in bioreactors as affected by culture method and inoculation density of single node stems. Plant Cell Tiss. Organ Cult. 81, 301–306. doi: 10.1007/s11240-004-6655-0
Hao, Z., Ouyang, F., Geng, Y., Geng, Y., Deng, X., Hu, Z., et al. (1998). Propagation of potato tuber in nutrient mist bioreactor. Biotehcnol. Tech. 12, 641–644. doi: 10.1023/A:1008892332242
Huang, S. Y., Chan, H. S., Wang, T. T. (2006). Induction of somatic embryos of celery by control of gaseous compositions and other physical conditions. Plant Growth Regul. 49, 219–227. doi: 10.1007/s10725-006-9113-7
Huang, T. K., McDonald, K. A. (2009). Bioreactor engineering for recombinant protein production in plant cell suspension cultures. Biochem. Eng. J. 45, 168–184. doi: 10.1016/j.bej.2009.02.008
Hvoslef-Eide, A. K., Olsen, O. A. S., Lyngved, R., Munster, C., Heyerdahl, P. H. (2005). Bioreactor design for propagation of somatic embryos. Plant Cell Tiss. Organ Cult. 81, 265–276. doi: 10.1007/s11240-004-6647-0
Hwang, H. D., Kwon, S. H., Murthy, H. N., Yun, S. W., Pyo, S. S., Park, S. Y. (2022). Temporary immersion bioreactor system as an efficient method for mass production of in vitro plants in horticulture and medicinal plants. Agronomy 12, 346. doi: 10.3390/agronomy12020346
Jang, H. R., Lee, H. J., Shohael, A. M., Park, B. J., Paek, K. Y., Park, S. Y. (2016). Production of biomass and bioactive compounds from shoot cultures of Rosa rugosa using a bioreactor culture system. Hortic. Environ. Biotechnol. 57, 79–87. doi: 10.1007/s13580-016-0111-z
Jay, V., Genestier, S., Courduroux, J. C. (1992). Bioreactor studies on the effect of dissolved oxygen concentrations on growth and differentiation of carrot (Daucus carota l.) cell cultures. Plant Cell Rep. 11, 605–608. doi: 10.1007/BF00236382
Jeon, S. B., Kan, S. W., Kim, W. S., Lee, G. P., Kim, S. H., Seo, S. G. (2009). In vitro plant regeneration from axillary buds of Hibiscus syriacus l. J. Plant Biotechnol. 2, 174–178. doi: 10.5010/JPB.2009.36.2.174
Jeong, C. S., Chakarabarty, D., Hahn, E. J. (2006). Effects of oxygen, carbon dioxide and ethylene on growth and bioactive compound production in bioreactor culture of ginseng adventitious roots. Biochem. Eng. J. 27, 252–263. doi: 10.1016/j.bej.2005.08.025
Jimenez, V. M. (2005). Involvement of plant hormones and plant growth regulators on in vitro somatic embryogenesis. Plant Growth Regul. 47, 91–110. doi: 10.1007/s10725-005-3478-x
Jimenez, E., Perez, N., de Feria, M., Barbon, R., Capote, A., Chavez, M., et al. (1999). Improved production of potato microtubers using a temporary immersion system. Plant Cell Tiss. Organ Cult. 59, 19–23. doi: 10.1023/A:1006312029055
Jin, M. Y., Piao, X. C., Xiu, J. R., Park, S. Y., Lian, M. L. (2013). Micropropagation using a bioreactor system and subsequent acclimatization of grape rootstock ‘5BB’. Sci. Hortic. 164, 35–40. doi: 10.1016/j.scienta.2013.09.004
Jo, E. A., Murthy, H. N., Hahn, E. J., Paek, K. Y. (2008). Micropropagation of alocasia amazonica using semisolid and liquid cultures. In Vitro Cell. Dev. Biol. Plant 44, 26–32. doi: 10.1007/s11627-007-9081-2
Kamarainen-Karppinen, T., Virtanen, E., Rokka, V. M., Pirttila, A. M. (2010). Novel bioreactor technology for mass propagation of potato microtubers. Plant Cell Tiss. Organ Cult. 101, 245–249. doi: 10.1007/s11240-010-9679-7
Kim, N. Y., Hwang, H. D., Kim, J. H., Kwon, B. M., Kim, D., Park, S. Y. (2020). Efficient production of virus-free apple plantlets using the temporary immersion bioreactor system. Hortic. Environ. Biotechnol. 61, 779–785. doi: 10.1007/s13580-020-00257-3
Kim, E. K., Hahn, E. J., Murthy, H. N., Paek, K. Y. (2004). Enhanced shoot and bulblet proliferation of garlic (Allium sativum l.) in bioreactor system. J. Hortic. Sci. Biotechnol. 79, 818–822. doi: 10.1080/14620316.2004.11511848
Kim, Y. J., Wyslouzil, B. E., Weathers, P. J. (2002). Secondary metabolism of hairy root cultures in bioreactors. In Vitro Cell. Dev. Biol. Plant 38, 1–10. doi: 10.1079/IVP2001243
Kuzma, L., Bruchajzer, E., Wysokinska, H. (2009). Methyl jasmonate effect on diterpenoid accumulation in Salvia sclarea hairy root culture in shake flasks and sprinkle bioreactor. Enzyme Microb. Technol. 44, 406–410. doi: 10.1016/j.enzmictec.2009.01.005
Leathers, R. R., Smith, M. A. L., Aitken-Christie, J. (1995). “Automation of the bioreactor process for mass propagation and secondary metabolism,” in Automation and environmental control in plant tissue culture. Eds. Aitken-Christie, J., Kozai, T., Smith, M. A. L. (Dordrecht: Springer), 187–214. doi: 10.1007/978-94-015-8461-6_9
Lee, Y. I., Hsu, S. T., Yeung, E. C. (2013). Orchid protocorm-like bodies are somatic embryos. Am. J. Bot. 100, 2121–2131. doi: 10.3732/ajb.1300193
Lema-Ruminska, J., Goncerzewicz, K., Garbriel, M. (2013). Influence of abscisic acid and sucrose on somatic embryogenesis of cactus Copiapoa tenuissima ritt. forma mostruosa. Sci. World J. 2013, 513985. doi: 10.1155/2013/513985
Lian, M. L., Chakarabarty, D., Paek, K. Y. (2003a). Bulblet formation from bulbscale segments of Lilium using bioreactor system. Biol. Plant 46, 199–203. doi: 10.1023/A:1022890208500
Lian, M. L., Chakrabarty, D., Paek, K. Y. (2002). Growth and uptake of sucrose and mineral ionsby bulblets of Lilium oriental hybrid ‘Casablanca’ during bioreactor culture. J. Hortic. Sci. Biotechnol. 77, 253–257. doi: 10.1080/14620316.2002.11511488
Lian, M. L., Murthy, H. N., Paek, K. Y. (2003b). Photoautotrophic culture conditions and photosynthetic photon flux influence growth of Lilium bulblets in vitro. In Vitro Cell. Dev. Biol. Plant 39, 532–535. doi: 10.1079/IVP2003440
Lian, M. L., Piao, X. C., Park, S. Y. (2014). “Mass production of lilium bulblets in bioreactors,” in Production of biomass and bioactive compounds using bioreactor technology. Eds. Paek, K. Y., Murthy, H. N. (Dordrecht: Springer), 389–415. doi: 10.1007/978-94-017-9223-3_16
Lim, S., Seon, J. H., Paek, K. Y., Son, S. H., Han, B. H. (1998). Development of pilot scale process for mass production of Lilium bulblets in vitro. Acta Hortic. 461, 237–242. doi: 10.17660/ActaHortic.1998.461.25
Maene, P., Debergh, P. (1985). Liquid medium additions to established tissue cultures to improve elongation and rooting in vivo. Plant Cell Tiss. Organ Cult. 67, 25–35. doi: 10.1007/BF00033566
Mallon, R., Covelo, P., Vieitez, A. M. (2012). Improving secondary embryogenesis in Quercus robur: application of temporary immersion for mass propagation. Trees 26, 731–741. doi: 10.1007/s00468-011-0639-6
Mamun, N. H., Egertsdotter, U., Aidun, C. K. (2015). Bioreactor technology for clonal propagation of plants and metabolite production. Front. Biol. 10, 177–193. doi: 10.1007/s11515-015-1355-1
Michler, C., Lineberger, R. D. (1987). Effects of light on somatic embryo development and abscisic levels in carrot suspension cultures. Plant Cell Tiss. Organ Cult. 11, 189–207. doi: 10.1007/BF00040425
Monja-Mio, K. M., Olvera-Casanova, D., Herrera-Alamillo, M. A., Sanchez-Teyer, F. L., Robert, M. L. (2021). Comparison of conventional and temporary immersion systems on micropropagation (multiplication phase) of Agave angustifolia haw. ‘Bacanora’. 3 Biotech. 11, 77. doi: 10.1007/s13205-020-02604-8
Mukherjee, P., Husain, N., Misra, S. C., Rao, V. S. (2010). In vitro propagation of a grape rootstock, deGrasset (Vitis champinni planch.): effects of medium compositions and plant growth regulators. Sci. Hortic. 126, 13–19. doi: 10.1016/j.scienta.2010.06.002
Nguyen, Q. T., Xiao, Y., Kozai, T. (2020). “Photoautotrophic micropropagation,” in Plant factory-an indoor vertical forming system for efficient quality food production, 2nd Edn. Eds. Kozai, T., Niu, G., Takagaki, M. (New York, NY: Elsevier, Academic Press), 333–346. doi: 10.1016/B978-0-12-816691-8.00023-6
Niemenak, N., Saare-Surminski, K., Rohsius, C., Ndoumou, D. O., Lieberei, R. (2008). Regeneration of somatic embryos in Theobroma cacao L. in temporary immersion bioreactor and analyses of free amino acids in different tissue. Plant Cell Rep. 27, 667–676. doi: 10.1007/s00299-007-0497-2
Orozco-Ortiz, C., Sanchez, L., Araya-Mattey, J., Vargas-Solorzano, I., Araya-Valverde, E. (2023). BIT® bioreactor increases in vitro multiplication of quality shoots in sugarcane (Saccharum spp. variety LAICA 04-809). Plant Cell Tissue Organ Cult. 152, 115–128. doi: 10.1007/s11240-022-02392-4
Paek, K. Y., Chakrabarty, D., Hahn, E. J. (2005). Application of bioreactor systems for large scale production of horticultural and medicinal plants. Plant Cell Tiss. Organ Cult. 81, 287–300. doi: 10.1007/s11240-004-6648-z
Paek, K. Y., Hahn, E. J., Son, S. H. (2001). Application of bioreactors for large-scale micropropagation systems of plants. In Vitro Cell. Dev. Biol. Plant 37, 149–157. doi: 10.1007/s11627-001-0027-9
Paek, K. Y., Murthy, H. N. (2002). High frequency of bulblet regeneration from bulb scale sections of Fritillaria thunbergii. Plant Cell Tiss. Organ Cult. 68, 247–252. doi: 10.1023/A:1013952803887
Park, S. Y., Ahn, J. K., Lee, W. Y., Murthy, H. N., Paek, K. Y. (2005). Mass production of Eleutherococcus koreanum plantlets via somatic embryogenesis from root cultures and accumulation of eleutherosides in regenerants. Plant Sci. 168, 1221–1225. doi: 10.1016/j.plantsci.2004.12.023
Park, J. A., Park, B. J., Kim, A. H., Park, S. Y., Paek, K. Y. (2015). Airlift bioreactor system and nitrogen sources for biomass and antioxidant compound production from in vitro culture of Vitis flexuosa plantlets. Hort. Environ. Biotechnol., 56, 358–365. doi: 10.1007/s13580-015-0006-4
Park, S. Y., Murthy, H. N., Paek, K. Y. (2000). Mass multiplication of protocorm-like bodies using bioreactor system and subsequent plant regeneration in Phalaenopsis. Plant Cell Tiss. Organ Cult. 63, 67–72. doi: 10.1023/A:1006420116883
Pena-Ramirez, Y. J., Juarez-Gomez, J., Gomez-Lopez, L., Jeronimo-Perez, J. L., Gracia-Shesena, I., Gonzalez-Rodriguez, J. A., et al. (2010). Multiple adventitious shoot formation in Spanish red cedar (Cedrela odorata l.) cultured in vitro using juvenile and mature tissues: an improved micropropagation protocol for a highly valuable tropical tree species. In Vitro Cell. Dev. Biol. Plant 46, 149–160. doi: 10.1007/s11627-010-9280-0
Piao, X. C., Chakrabarty, D., Hahn, E. J., Paek, K. Y. (2003). A simple method for mass production of potato microtubers using a bioreactor system. Curr. Sci. 84, 1129–1132.
Rani, V., Raina, S. N. (2000). Genetic fidelity of organized meristem-derived micropropagated plants: A critical reappraisal. In Vitro Cell. Dev. Biol. Plant 36, 319–330. doi: 10.1007/s11627-000-0059-6
Regueira, M., Rial, E., Blanco, B., Bogo, B., Aldrey, A., Correa, B., et al. (2018). Micropropagation of axillary shoots of Salix viminalis using a temporary immersion system. Trees 32, 61–71. doi: 10.1007/s00468-017-1611-x
Rico, S., Garrido, J., Sanchez, C., Ferreiro-Vera, C., Codesido, V., Vidal, N. (2022). A temporary immersion system to improve Cannabis sativa micropropagation. Frot. Plant Sci. 13. doi: 10.3389/fpls.2022.895971
Rout, G. R., Mohapatra, A., Jain, S. M. (2006). Tissue culture of ornamental pot plant: A critical review on present scenario and future prospects. Biotechnol. Adv. 24, 531–560. doi: 10.1016/j.biotechadv.2006.05.001
Rout, G. R., Samantharay, S., Das, P. (2000). In vitro manipulation and propagation of medicinal plants. Biotechnol. Adv. 18, 91–120. doi: 10.1016/s0734-9750(99)00026-9
San Jose, M. C., Blazquez, N., Cernada, M. J., Janeiro, L. V., Cuenca, B., Sanchez, C., et al. (2020). Temporary immersion systems to improve alder micropropagation. Plant Cell Tissue Organ Cult. 143, 265–275. doi: 10.1007/s11240-020-01937-9
Schuchovski, C., Sant’Anna-Santos, B., Marra, R., Biasi, L. (2020). Morphological and anatomical insights into de novo shoot organogenesis of in vitro ‘Delite rabbiteye’ blueberries. Heliyon 6, e05468. doi: 10.1016/j.heliyon.2020.e54468
Shigeta, J., Sato, K. J., Mii, M. (1996). Effects of initial cell density, pH and dissolved oxygen on bioreactor production of carrot somatic embryos. Plant Sci. 115, 109–114. doi: 10.1016/s0168-9452(96)04327-o
Shohael, A. M., Chakrabarty, K. W., Yu, K. W., Hahn, E. J., Paek, K. Y. (2005). Application of bioreactor system for large-scale production of Eleutherococcus sessiliflorus somatic embryos in an air-lift bioreactor and production of eleutherosides. J. Biotechnol. 120, 228–236. doi: 10.1016/j.jbiotec.2005.06.010
Shohael, A. M., Murthy, H. N., Paek, K. Y. (2014). Pilot-scale culture of somatic embryos of Eleutherococcus senticosus in airlift bioreactors for the production of eleutherosides. Biotechnol. Lett. 36, 1727–1733. doi: 10.1007/s10529-014-1534-1
Simonton, W., Robacker, C., Krueger, S. (1991). A programmable micropropagation apparatus using cycled medium. Plant Cell Tiss. Organ Cult. 27, 211–218. doi: 10.1007/BF00041292
Snyman, S. J., Nkwanyana, P. D., Watt, M. P. (2011). Alleviation of hyperhydricity of sugarcane plantlets produced in RITA® vessels and genotypic and phenotypic characterization of acclimated plants. South Afr. J. Bot. 77, 685–692. doi: 10.1016/j.sajb.2011.03.004
Steingroewer, J., Bley, T., Georgiev, V., Ivanov, I., Lenk, F., Marchev, A., et al. (2013). Bioprocessing of differentiated plant in vitro systems. Eng. Life Sci. 13, 26–38. doi: 10.1002/elsc.201100226
Szopa, A., Kokotkiewicz, A., Bednarz, M., Jafernik, K., Luczkiewicz, M., Ekiert, H. (2019). Bioreactor types affects the accumulation of phenolic acids and flavonoids in microshoot cultures of Schisandra chinensis (Turcz.) baill. Plant Cell Tiss. Organ Cult. 139, 199–206. doi: 10.1007/s11240-019-01676-6
Takayama, S., Akita, M. (1994). The types of bioreactors used for shoots and embryos. Plant Cell Tiss. Organ Cult. 39, 147–156. doi: 10.1007/BF00033922
Teisson, C., Alvard, D. (1995). “A new concept of plant in vitro cultivation liquid medium: Temporary immersion,” in Current issues in plant molecular and cellular biology. current plant science and biotechnology in agriculture. Eds. Terzi, M., Cella, R., Falavigna, A. (Dordrecht: Spriger), 105–110. doi: 10.1007/978-94-011-0307-7_12
Teisson, C., Alvard, D. (1999). In vitro production of potato microtubers in liquid medium using temporary immersion. Potato Res. 42, 499–504. doi: 10.1007/BF02358166
Thul, S. T., Kukreja, K. (2010). An efficient protocol for high-frequency direct multiple shoot regeneration from internodes of peppermint (Mentha x pierita). Nat. Prod. Res. 5, 1945–1946. doi: 10.1177/1934578X1000501223
Tisserat, B., Vandercook, C. E. (1985). Development of an automated plant culture system. Plant Cell Tiss. Organ Cult. 5, 107–117. doi: 10.1007/BF00040307
Trauger, M., Hile, A., Sreenivas, K., Shouse, E. V., Bhatt, J., Lai, T., et al. (2022). CO2 supplementation eliminates sugar-rich media requirement for plant propagation using a simple inexpensive temporary immersion photobioreactor. Plant Cell Tissue Organ Cult. 150, 57–71. doi: 10.1007/s11240-021-02210-3
Uchendu, E. E., Poliyath, G., Brwon, D. C. W., Saxena, P. K. (2011). In vitro propagation of north American ginseng (Panax quinquefolius l.). In Vitro Cell. Dev. Biol. Plant 47, 710–718. doi: 10.1007/s11627-011-9379-y
Uma, S., Karthic, R., Kalpana, S., Backyarani, S., Saraswathi, M. S. (2021). A novel temporary immersion bioreactor system for large scale multiplication of banana (Rasthali AAB-silk). Sci. Rep. 11, 20371. doi: 10.1038/s41598-021-99923-4
Valdiani, A., Hansen, O. K., Nielsen, U. B., Johannsen, V. K., Shariat, M., Georgiev, M. I., Odmidvar, V., Ebrahimi, M., Tavakoli Dinanai, E., Abiri, R. (2019). Bioreactor-based advances in plant tissue and cell culture: challenges and prospects. Crit. Rev. Biotechnol. 39, 20–34. doi: 10.1080/07388551.2018.1489778
Vidal, N., Blanco, B., Cuenca, B. (2015). A temporary immersion system for micropropagation of axillary shoots of hybrid chestnut. Plant Cell Tissue Organ Cult. 123, 229–243. doi: 10.1007/s11240-015-0827-y
Vidal, N., Sanchez, C. (2019). Use of bioreactor systems in the propagation of forest trees. Eng. Life Sci. 19, 896–915. doi: 10.1002/elsc.201900041
Watt, M. P. (2012). The status of temporary immersion system (TIS) technology for plant micropropagation. Afr. J. Biotechnol. 11, 14025–14035. doi: 10.5897/AJB12.1693
Wu, S. Q., Lian, M. L., Gao, R., Park, S. Y., Piao, X. C. (2011). Bioreactor application on adventitious root culture of Astragalus membranaceus. In Vitro Cell. Dev. Biol. Plant 47, 719–724. doi: 10.1007/s11627-011-9376-1
Xiao, Y., Niu, G., Kozai, T. (2011). Development and application of photoautotrophic micropropagation plant system. Plant Cell Tissue Organ Cult. 105, 149–158. doi: 10.1007/s11240-010-9863-9
Xu, L., Huang, H. (2014). Genetic and epigenetic controls of plant regeneration. Curr. Top. Dev. Biol. 108, 1–33. doi: 10.1016/B978-0-12-391498-9.00009-7
Yan, H., Liang, C., Li, Y. (2010). Improved growth and quality of Siraitia grosvenorii plantlets using a temporary immersion system. Plant Cell Tiss. Organ Cult. 103, 131–135. doi: 10.1007/s11240-010-9752-2
Yan, H., Yang, L., Li, Y. (2011). Improved growth and quality of Dioscorea fordii prain et Burk and Dioscorea alata plantlets using a temporary immersion system. Afr. J. Biotechnol. 10, 19444–19448. doi: 10.5897/AJB11.2684
Yang, J. F., Piao, X. C., Sun, D., Lian, M. L. (2010). Production of protocorm-like bodies with bioreactor and regeneration of in vitro of Oncidium ‘Sugar sweet’. Sci. Hortic. 125, 712–717. doi: 10.1016/j.scienta.2010.05.003
Yu, W. C., Joyce, P. J., Cameron, D. C., McCown, B. H. (2000). Sucrose utilization during potato microtuber growth in bioreactors.. Plant Cell Rep. 19, 407–413. doi: 10.1007/s002990050748
Keywords: Bioreactor, embryogenesis, immersion culture, in vitro propagation, liquid culture, organogenesis, somatic embryo, temporary immersion culture
Citation: Murthy HN, Joseph KS, Paek KY and Park SY (2023) Bioreactor systems for micropropagation of plants: present scenario and future prospects. Front. Plant Sci. 14:1159588. doi: 10.3389/fpls.2023.1159588
Received: 06 February 2023; Accepted: 27 March 2023;
Published: 19 April 2023.
Edited by:
Chunzhao Liu, Qingdao University, ChinaReviewed by:
Atаhас Паbлоb, University of Food Technologies, BulgariaCopyright © 2023 Murthy, Joseph, Paek and Park. This is an open-access article distributed under the terms of the Creative Commons Attribution License (CC BY). The use, distribution or reproduction in other forums is permitted, provided the original author(s) and the copyright owner(s) are credited and that the original publication in this journal is cited, in accordance with accepted academic practice. No use, distribution or reproduction is permitted which does not comply with these terms.
*Correspondence: Hosakatte Niranjana Murthy, aG5tdXJ0aHk2MEBnbWFpbC5jb20=; So Young Park, c295cGFyazdAY2JudS5hYy5rcg==
Disclaimer: All claims expressed in this article are solely those of the authors and do not necessarily represent those of their affiliated organizations, or those of the publisher, the editors and the reviewers. Any product that may be evaluated in this article or claim that may be made by its manufacturer is not guaranteed or endorsed by the publisher.
Research integrity at Frontiers
Learn more about the work of our research integrity team to safeguard the quality of each article we publish.