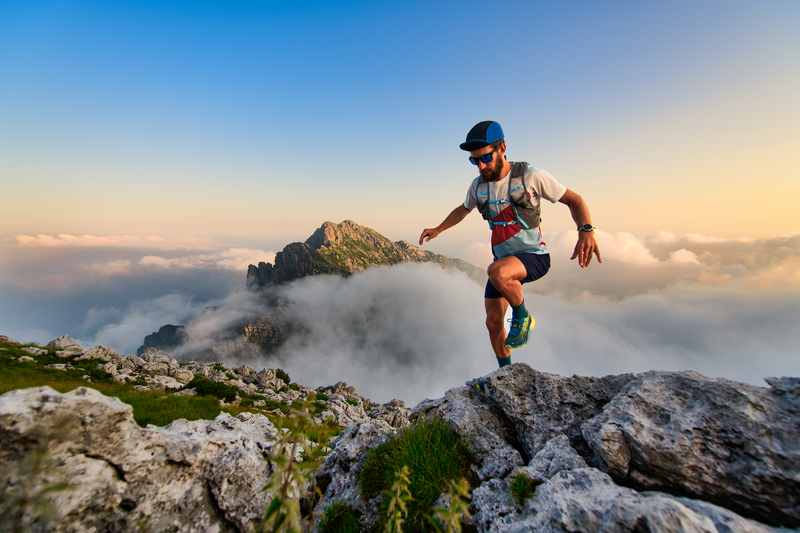
95% of researchers rate our articles as excellent or good
Learn more about the work of our research integrity team to safeguard the quality of each article we publish.
Find out more
EDITORIAL article
Front. Plant Sci. , 21 February 2023
Sec. Plant Abiotic Stress
Volume 14 - 2023 | https://doi.org/10.3389/fpls.2023.1146326
This article is part of the Research Topic Multiple abiotic stresses: molecular, physiological, and genetic responses and adaptations in cereals View all 11 articles
Editorial on the Research Topic
Multiple abiotic stresses: Molecular, physiological, and genetic responses and adaptations in cereals
Cereal crops provide approximately 40% of the energy and protein components of the human diet and are vital to the food security of the world (Dunwell, 2014). With the increasing incidence of global warming (expected 1.2–1.9°C higher than ambient during 2021–2040) and extreme weather events, the intensity of various climatic constraints is expected to accelerate, ultimately affecting global cereal crop production (IPCC, 2021). Most of the climatic constraints are abiotic stresses (drought, heat, cold, waterlogging, salinity, mineral deficiency, heavy metal stress, and ultraviolet-B (UV-B), etc.) causing extensive yield losses (Paul et al., 2019; Chaudhry and Sidhu, 2021; Rivero et al., 2022). Estimates have shown that each degree Celsius rise in the global mean temperature may lead to yield losses of 6.0, 3.2, and 7.4% in major cereals, i.e., wheat, rice, and maize, respectively, without effective adaptation and genetic improvement (Zhao et al., 2017).
The occurrence of abiotic stresses singly has less effect than when occurring in combination at different growth stages (Mittler, 2006; Suzuki et al., 2014; Shaar-Moshe et al., 2017). Under field conditions, frequently occurring combined stresses are drought and heat (Mittler, 2006; Lamaoui et al., 2018; Lawas et al., 2018; Nasser et al., 2020), drought and salinity (Paul et al., 2019; Abobatta, 2020), and salinity and waterlogging (Lamaoui et al., 2018; Lawas et al., 2018). The effect of these stresses in combination on crop plants depends on the nature of the interactions between them (Ramu et al., 2016). These multiple stresses induce unique mechanisms (morphophysiological, biochemical, molecular, and genetic) in crop plants for adaptations and cannot be predicted by simply studying each of the different stresses (Suzuki et al., 2014; Zandalinas et al., 2021). Understanding the mechanisms of plant response to multiple stresses is crucial to unravel the complexities of plant responses to stress combinations for the development of climate-resilient crops for future food security (Rivero et al., 2022). Therefore, keeping the above under consideration, the present Research Topic has been designed to demonstrate the current level of research and progress in the study of molecular, physiological, and genetic responses and adaptation strategies toward multiple abiotic stresses in cereals. The insights of this Research Topic are divided into the following headings:
Characterization and identification of the differential responses of crop plants are one of the major essential steps for the development of climate-resilient crop plants. Amro et al. studied the growth responses and genetic variation among wheat genotypes for salinity tolerance (seawater) and identified a high genetic diversity among the studied genotypes, which could be utilized for breeding programs. Likewise, Radha et al. reviewed the individual and interactive effects of various abiotic stresses (drought, salinity, high temperature, eCO2, submergence, nutrient deficiency) and their combined effect on rice physiology with the possible adaptation strategies for improving grain quality parameters and yield traits. With the advancement of various tools in system biology (high-throughput phenotyping and genome sequencing), different approaches have been developed, such as quantitative trait locus (QTL) mapping, candidate gene association studies, and genome-wide association studies (GWAS), in order to link phenotypes and genotypes in crop plants for the identification of genetic factors associated with the various traits under consideration (Mir et al., 2019). Among them, GWAS is one of the most powerful tools for investigating complex traits associated with single or multiple abiotic stresses. It detects marker–trait association (MTA) using conserved linkage disequilibrium (LD) present in the selected panel of accessions (Myles et al., 2009; Saini et al., 2022). GWAS has a high capacity to identify small-effect genes/MTAs on a genome-wide scale by efficiently using the multiple historical crossover events that occur in the diverse association panel used (Saini et al., 2022; Xiao et al., 2022). In the present Research Topic, Devate et al. used a 35k SNP wheat breeder’s genotyping array to identify 57 unique markers associated with various traits across the locations for drought and heat tolerance in wheat. Out of these associations, 23 MTAs were deemed to be stable. Similarly, in another study, Devate et al. identified six unique marker–trait associations for grain iron (GFeC), zinc (GZnC) contents, and thousand-grain weight (TGW) under drought and heat stress conditions in wheat. These identified MTAs could be utilized in the breeding program after validation through marker-assisted selection (MAS).
The trait-specific markers (linked markers) allow the efficient introgression of targeted genomic loci from the donor genotype into an elite breeding line, facilitate indirect selection for difficult traits (i.e., root traits under drought stress conditions), and cut the number of genes/QTL into a single genotype using the marker-assisted selection approach (Pandurangan et al., 2022; Rai and Pandey-Rai, 2021). This method is very rapid and cost-effective for genetic improvement after the identification of tightly linked markers associated with the trait under consideration. It is effectively implemented for the improvement of multiple abiotic stress tolerance in various crops, i.e., rice (heat tolerance (Lang et al., 2015); submergence and drought tolerance (Kumar et al., 2020); drought, salinity, and submergence (Muthu et al., 2020); drought and heat stress (Withanawasam et al., 2022), wheat (drought tolerance (Ciucă et al., 2009; Merchuk-Ovnat et al., 2016; Rai et al., 2018; Gautam et al., 2021), and maize (drought (Ribaut and Ragot, 2007)). Similarly, in the present Research Topic, Sunilkumar et al. introgressed the rust resistance gene Lr24 and QTLs linked to moisture deficit stress tolerance in the background of HD3086 (a high-yielding, stress-susceptible genotype of wheat) from the HI1500 donor genotype.
Understanding differential levels of plant mechanisms under multiple stress conditions is essential for combating their effect. Adopting different omics approaches (genomics, transcriptomics, proteomics, and metabolomics) and understanding their overall phenotypic effects on crop plants under abiotic stress conditions are crucial to developing strategies for designing crops with superior tolerance mechanisms (Bhardwaj et al., 2021; Jeyasri et al., 2021). Among the different omics approaches, transcription factors (TFs) are crucial for recognizing the appropriate molecular processes and pathways under stress conditions (Muthuramalingam et al., 2018; Muthuramalingam et al., 2020). In the present Research Topic, Annum et al. studied a phospholipase C (PLC) signaling pathway in spring wheat and evaluated its four AtPLC5 overexpressed (OE)/transgenic lines under heat and osmotic stresses through 32Pi radioactive labeling. The results indicate that heat stress and osmotic stress activate several lipid responses in wild-type and transgenic wheat conforming to osmotic stress tolerance. Kumar et al. studied the differential transcript expression of K+ transport genes in different tissues (root, stem, and leaf) under different abiotic stresses, such as salt, drought, heat, and cold, to elucidate their role in ion homeostasis and stress tolerance mechanisms in sorghum. Maheshwari et al. reviewed paclobutrazol (PBZ) as a plant growth regulator and multistress protectant; they discussed current findings and the prospective application of PBZ in regulating crop growth and ameliorating abiotic stresses. In another study, Kumar et al. used the 20S proteasome gene family in rapeseed and identified 20S proteasome genes for α- (PA) and β-subunits (PB) through systematically performed gene structure analysis. Out of 82 BnPA/PB genes, three exhibited high expression under abiotic stresses. Likewise, Ahmed et al. proposed a novel activation function, the Gaussian Error Linear Unit with Sigmoid (SIELU), for the deep learning (DL) model along with other hyperparameters for the classification of unknown abiotic stress protein sequences from cereal crops.
The papers presented in the current Research Topic are associated with the multiple abiotic stresses on various crops and show wider scope to understand the molecular, physiological, and genetic responses of multiple abiotic stresses. At the same time, the findings of the papers presented show a wide range of advanced scientific approaches and research ideas to understand and identify the effects of multiple abiotic stress and the implementation of their adaptation strategies for the development of climate-resilient crop plants.
NB wrote the draft editorial. All authors edited it. All authors contributed to the article and approved the submitted version.
The authors declare that the research was conducted in the absence of any commercial or financial relationships that could be construed as a potential conflict of interest.
All claims expressed in this article are solely those of the authors and do not necessarily represent those of their affiliated organizations, or those of the publisher, the editors and the reviewers. Any product that may be evaluated in this article, or claim that may be made by its manufacturer, is not guaranteed or endorsed by the publisher.
Abobatta, W. F. (2020). “Plant responses and tolerance to combined salt and drought stress,” in Salt and drought stress tolerance in plants (Cham: Springer), 17–52.
Bhardwaj, A., Devi, P., Chaudhary, S., Rani, A., Jha, U. C., Kumar, S., et al. (2021). ‘Omics’ approaches in developing combined drought and heat tolerance in food crops. Plant Cell Rep. 41, 699–739. doi: 10.1007/s00299-021-02742-0
Chaudhry, S., Sidhu, G. P. S. (2021). Climate change regulated abiotic stress mechanisms in plants: a comprehensive review. Plant Cell Rep. 41, 1–31. doi: 10.1007/s00299-021-02759-5
Ciucă, M., Todorvska, E., Kolev, S., Nicolae, R., Guinea, I., Saulescu, N. (2009). Marker-assisted selection (MAS) for drought tolerance in wheat using markers associated with membrane stability. FUNDULEA 12, 7–12.
Dunwell, J. M. (2014). Transgenic cereals: Current status and future prospects. J. Cereal Sci. 59 (3), 419–434. doi: 10.1016/j.jcs.2013.08.008
Gautam, T., Saripalli, G., Kumar, A., Gahlaut, V., Gadekar, D. A., Oak, M., et al. (2021). Introgression of a drought insensitive grain yield QTL for improvement of four Indian bread wheat cultivars using marker assisted breeding without background selection. J. Plant Biochem. Biotechnol. 30 (1), 172–183. doi: 10.1007/s13562-020-00553-0
IPCC (2021). “Summary for policymakers,” in Climate change 2021: The physical science basis. contribution of working group I to the sixth assessment report of the intergovernmental panel on climate change. Eds. Masson-Delmotte, V., Zhai, P., Pirani, A., Connors, S. L., Péan, C., Berger, S., et al (Cambridge University Press).
Jeyasri, R., Muthuramalingam, P., Satish, L., Pandian, S. K., Chen, J. T., Ahmar, S., et al. (2021). An overview of abiotic stress in cereal crops: Negative impacts, regulation, biotechnology and integrated omics. Plants 10 (7), 1472. doi: 10.3390/plants10071472
Kumar, A., Sandhu, N., Venkateshwarlu, C., Priyadarshi, R., Yadav, S., Majumder, R. R., et al. (2020). Development of introgression high-yielding semi-dwarf genetic backgrounds to enable improvement of modern rice varieties for tolerance to multiple abiotic stresses free from undesirable linkage drag. Sci. Rep. 10 (1), 1–13. doi: 10.1038/s41598-020-70132-9
Lamaoui, M., Jemo, M., Datla, R., Bekkaoui, F. (2018). Heat and drought stresses in crops and approaches for their mitigation. Front. Chem. 6. doi: 10.3389/fchem.2018.00026
Lang, N. T., Ha, P. T. T., Tru, P. C., Toan, T. B., Buu, B. C., Cho, Y. C. (2015). Breeding for heat tolerance rice based on marker-backcrossing in Vietnam. Plant Breeding and Biotechnology 3, 274–281. doi: 10.9787/PBB.2015.3.3.274
Lawas, L. M. F., Zuther, E., Jagadish, S. K., Hincha, D. K. (2018). Molecular mechanisms of combined heat and drought stress resilience in cereals. Curr. Opin. Plant Biol. 45, 212–217. doi: 10.1016/j.pbi.2018.04.002
Merchuk-Ovnat, L., Barak, V., Fahima, T., Ordon, F., Lidzbarsky, G. A., Krugman, T., et al. (2016). Ancestral QTL alleles from wild emmer wheat improve drought resistance and productivity in modern wheat cultivars. Front. Plant Sci. 7. doi: 10.3389/fpls.2016.00452
Mir, R. R., Reynolds, M., Pinto, F., Khan, M. A., Bhat, M. A. (2019). High-throughput phenotyping for crop improvement in the genomics era. Plant Sci. 282, 60–72. doi: 10.1016/j.plantsci.2019.01.007
Mittler, R. (2006). Abiotic stress, the field environment, and stress combination. Trends Plant Sci. 11 (1), 15–19. doi: 10.1016/j.tplants.2005.11.002
Muthu, V., Abbai, R., Nallathambi, J., Rahman, H., Ramasamy, S., Kambale, R., et al. (2020). Pyramiding QTLs controlling tolerance against drought, salinity, and submergence in rice marker-assisted breeding. PloS One 15 (1), e0227421. doi: 10.1371/journal.pone.0227421
Muthuramalingam, P., Jeyasri, R., Bharathi, R. K. A. S., Suba, V., Pandian, S. T. K., Ramesh, M. (2020). Global integrated omics expression analyses of abiotic stress signaling HSF transcription factor genes in oryza sativa l.: An in-silico approach. Genomics 112 (1), 908–918. doi: 10.1016/j.ygeno.2019.06.006
Muthuramalingam, P., Krishnan, S. R., Saravanan, K., Mareeswaran, N., Kumar, R., Ramesh, M. (2018). Genome-wide identification of major transcription factor superfamilies in rice identifies key candidates involved in abiotic stress dynamism. J. Plant Biochem. Biotechnol. 27 (3), 300–317. doi: 10.1007/s13562-018-0440-3
Myles, S., Peiffer, J., Brown, P. J., Ersoz, E. S., Zhang, Z., Costich, D. E., et al. (2009). Association mapping: critical considerations shift from genotyping to experimental design. Plant Cell 21 (8), 2194–2202. doi: 10.1105/tpc.109.068437
Nasser, L. M., Badu-Apraku, B., Gracen, V. E., Mafouasson, H. N. (2020). Combining ability of early-maturing yellow maize inbreds under combined drought and heat stress and well-watered environments. Agronomy 10 (10), 1585. doi: 10.3390/agronomy10101585
Pandurangan, S., Workman, C., Nilsen, K., Kumar, S. (2022). Introduction to marker-assisted selection in wheat breeding. in accelerated breeding of cereal crops (New York, NY: Humana), 77–117.
Paul, K., Pauk, J., Kondic-Spika, A., Grausgruber, H., Allahverdiyev, T., Sass, L., et al. (2019). Co-Occurrence of mild salinity and drought synergistically enhances biomass and grain retardation in wheat. Front. Plant Sci. 10. doi: 10.3389/fpls.2019.00501
Rai, N., Bellundagi, A., Kumar, P. K., Kalasapura Thimmappa, R., Rani, S., Sinha, N., et al. (2018). Marker-assisted backcross breeding for improvement of drought tolerance in bread wheat (Triticum aestivum l. em thell). Plant Breed. 137 (4), 514–526. doi: 10.1111/pbr.12605
Rai, S. K., Pandey-Rai, S. (2021). “Marker-assisted breeding for abiotic stress tolerance in horticultural crops,” in Stress tolerance in horticultural crops (Woodhead Publishing), 63–74.
Ramu, V. S., Paramanantham, A., Ramegowda, V., Mohan-Raju, B., Udayakumar, M., Senthil-Kumar, M. (2016). Transcriptome analysis of sunflower genotypes with contrasting oxidative stress tolerance individual and combined biotic-biotic and abiotic stress tolerance mechanisms. PloS One 11 (6), e0157522. doi: 10.1371/journal.pone.0157522
Ribaut, J. M., Ragot, M. (2007). Marker-assisted selection to improve drought adaptation in maize: the backcross approach, perspectives, limitations, and alternatives. J. Exp. Bot. 58 (2), 351–360. doi: 10.1093/jxb/erl214
Rivero, R. M., Mittler, R., Blumwald, E., Zandalinas, S. I. (2022). Developing climate-resilient crops: improving plant tolerance to stress combination. Plant J. 109 (2), 373–389. doi: 10.1111/tpj.15483
Saini, D. K., Chopra, Y., Singh, J., Sandhu, K. S., Kumar, A., Bazzer, S., et al. (2022). Comprehensive evaluation of mapping complex traits in wheat using genome-wide association studies. Mol. Breed. 42 (1), 1–52. doi: 10.1007/s11032-021-01272-7
Shaar-Moshe, L., Blumwald, E., Peleg, Z. (2017). Unique physiological and transcriptional shifts under combinations of salinity, drought, and heat. Physiologyysiology 174 (1), 421–434. doi: 10.1104/pp.17.00030
Suzuki, N., Rivero, R. M., Shulaev, V., Blumwald, E., Mittler, R. (2014). Abiotic and biotic stress combinations. New Phytol. 203 (1), 32–43. doi: 10.1111/nph.12797
Withanawasam, D. M., Kommana, M., Pulindala, S., Eragam, A., Moode, V. N., Kolimigundla, A., et al. (2022). Improvement of grain yield under moisture and heat stress conditions through marker-assisted pedigree breeding in rice (Oryza sativa l.). Crop Pasture Sci. 73 (4), 356–369. doi: 10.1071/CP21410
Xiao, Q., Bai, X., Zhang, C., He, Y. (2022). Advanced high-throughput plant phenotyping techniques for genome-wide association studies: A review. J. Advanced Res. 35, 215–230. doi: 10.1016/j.jare.2021.05.002
Zandalinas, S. I., Fritschi, F. B., Mittler, R. (2021). Global warming, climate change, and environmental pollution: recipe for a multifactorial stress combination disaster. Trends Plant Sci. 26 (6), 588–599. doi: 10.1016/j.tplants.2021.02.011
Keywords: abiotic stress, molecular response, physiological response, genetic response, adaptation, cereals
Citation: Bhusal N, Sharma P, Kumar RR and Sareen S (2023) Editorial: Multiple abiotic stresses: Molecular, physiological, and genetic responses and adaptations in cereals. Front. Plant Sci. 14:1146326. doi: 10.3389/fpls.2023.1146326
Received: 17 January 2023; Accepted: 31 January 2023;
Published: 21 February 2023.
Edited by:
Luisa M. Sandalio, Department of Biochemistry, Cell and Molecular Biology of Plants, (CSIC), SpainReviewed by:
Rosa M. Rivero, Center for Edaphology and Applied Biology of Segura (CSIC), SpainCopyright © 2023 Bhusal, Sharma, Kumar and Sareen. This is an open-access article distributed under the terms of the Creative Commons Attribution License (CC BY). The use, distribution or reproduction in other forums is permitted, provided the original author(s) and the copyright owner(s) are credited and that the original publication in this journal is cited, in accordance with accepted academic practice. No use, distribution or reproduction is permitted which does not comply with these terms.
*Correspondence: Sindhu Sareen, c2FyZWVuOUBob3RtYWlsLmNvbQ==
Disclaimer: All claims expressed in this article are solely those of the authors and do not necessarily represent those of their affiliated organizations, or those of the publisher, the editors and the reviewers. Any product that may be evaluated in this article or claim that may be made by its manufacturer is not guaranteed or endorsed by the publisher.
Research integrity at Frontiers
Learn more about the work of our research integrity team to safeguard the quality of each article we publish.