- 1Agriculure in Marginal Environment Program, African Sustainable Agriculture Research Institute (ASARI), Mohammed VI Polytechnic University (UM6P), Laayoune, Morocco
- 2Agri-Edge, Mohammed VI Polytechnic University (UM6P), Benguerir, Morocco
- 3Department of Food Science and Agricultural Chemistry, McGill University, Sainte-Anne-de-Bellevue, QC, Canada
- 4Department of Plant Protection, Agronomic and Veterinary Institute Hassan II, Agadir, Morocco
- 5AgroBioSciences Program, Mohammed VI Polytechnic University, Ben Guerir, Morocco
- 6Agrobiotechnology & Bioengineering Centre, Cadi Ayyad University, FST, Marrakech, Morocco
- 7Soil, Water, and Agronomy (SWA) Program, International Center for Agricultural Research in the Dry Areas (ICARDA), Rabat, Morocco
In the Sahara Desert, due to drought and salinity and poor soil fertility, very limited crop choice is available for the farmers to grow crops. Quinoa (Chenopodium quinoa Willd.) has shown promising under such conditions in the South of Morocco, a true representative site of Sahara Desert. Soil organic amendments have the potential to minimize negative effects of soil salinity and improve crop production. Thus, this study aimed to elucidate the impact of nine organic amendments on quinoa (var. ICBA-Q5) growth, productivity, and biochemical parameters under saline irrigation water application (4, 12, and 20 dS·m-1). Results of the experiment indicate a significant effect of organic amendments on major agro-morphological and productivity parameters. Biomass and seed yield tends to decrease with the rise of salinity level, and organic amendments have improved productivity compared to the non-treated control. However, salinity stress alleviation was assessed by determining pigments concentration, proline content, phenolic compounds, and antioxidant activity. Therefore, the action of organic amendments varies from one level of salinity to another. Furthermore, a remarkably significant decrease in total saponin content was reached due to the application of amendments even at high saline conditions (20 dS·m-1). The results demonstrate the possibility of enhancing the productivity of quinoa as an alternative food crop under salinity conditions by using organic amendments and improving the quality of grains (saponin reduction) during the pre-industrialization process.
1 Introduction
Crop production is threatened and limited by various challenges including salinity. FAO (2021) estimated that salinity affects more than 424 million hectares of topsoil (0-30 cm) and 833 million hectares of subsoil (30-100 cm). In Africa, 80 million hectares of lands are saline, sodic, or saline-sodic, of which the Sahel, in West Africa, is the most affected (Shahid et al., 2018). Salinization occurs under all climatic conditions and can result from both natural (primary salinization) and human-induced actions (secondary salinization). In agricultural area, secondary salinization is the most dominant form of salinity and is caused by inadequate irrigation, misuse of fertilizers, over-pumping, improper drainage, seawater intrusion, etc. In general, salinization occurs in arid and semi-arid regions where precipitation is very low, and evaporation is high which can result in salt accumulation in the rootzone and lack of leaching (Stavi et al., 2021). Salinity affects plants and reduces their growth and productivity by limiting water and nutrient uptake (osmotic stress), accumulating toxic ions such as sodium, reducing transpiration and photosynthetic activity, damaging cell membranes and causing plant senescence (Machado and Serralheiro, 2017). Salt-tolerant plants have the ability to deal with salinity by deploying several mechanisms including osmotic adjustment, sodium compartmentalization, salt exclusion, etc (Volkov and Beilby, 2017).
Quinoa (Chenopodium quinoa Willd.) is a good example of salt-tolerant crops that recently attracted a lot of attention worldwide thanks to its wide adaptation to various climatic and soil conditions, rusticity and resilience, and its high nutritional value. Quinoa originates from the Andean countries, where it was domesticated over 7000 years ago by the local people. Quinoa was neglected for many centuries following the Spanish conquest and was geographically limited to the Andes, where it was a basic staple food for the indigenous people (Bazile et al., 2016). The potential of quinoa was rediscovered during the second half of the 20th century and since then, quinoa was tested and introduced to over 120 countries around the world (Alandia et al., 2020). Peru and Bolivia are both leading exporters of quinoa grains worldwide, contributing 78% of the total export (exporting 44,353 and 29,416 tons, respectively, out of a total of 93,809 tons/year) (Alandia et al., 2020; Schmidt et al., 2021).
Quinoa was first introduced in Morocco in 2000 and since then its value chain significantly improved. In the beginning of its introduction, research works mainly focused on quinoa agronomic adaptation (Jellen et al., 2006), diversity screening (Mhada et al., 2014), irrigation (Hirich et al., 2014a; Hirich et al., 2014b; Fghire et al., 2015), organic amendment (Hirich et al., 2014b), salinity (Brakez et al., 2013; Hirich et al., 2014c), and sowing date (Hirich et al., 2014d). Recent research is oriented towards production transformation (Mhada et al., 2020; Hirich et al., 2021; Rafik et al., 2021b, 2021c), marketing (Rafik et al., 2021a), value chain analysis (Hirich et al., 2021; Rafik et al., 2021a), etc.
In order to enhance tolerance level to salinity in quinoa plants and improve their yield, it is necessary to increase the nutrient and water availability in the soil and their absorption through the application of organic amendments or fertilizers. Organic amendments were shown to be highly beneficial for crop production, especially under stress conditions (Leogrande and Vitti, 2019). In fact, by adding organic matter to the soil, the nutrient availability for the plant and water holding capacity increases, and the soil’s biological activity gets improved. Another role of organic amendments is they improve soil structure and properties resulting in accelerating salt leaching in the rootzone (Ding et al., 2020; Wichern et al., 2020).
To mitigate the drastic negative effects of abiotic stress such as salinity, halophytes have developed an array of salt-tolerance mechanisms by controlling the uptake and canalization of Na+, K+ and Cl- and the biosynthesis of osmolytes (Flowers and Colmer, 2008). Quinoa is one of the famous halophytes that contains a good balance of starch, protein, lipids and fiber, minerals, and also contains a good amount of non-nutritional compounds such as saponin, polyphenols, flavonoids, carotenoids, that control various biological activities (Miranda et al., 2012; Gómez-Caravaca et al., 2014).
Saponins are bitter, soapy substances that protect quinoa plant from fungal disease and insect attacks. They also contain toxins that can cause irritation and other health issues to some people (Liao et al., 2017). While the level of toxicity is low, some people may be sensitive to this compound. Saponins are also considered secondary metabolites with numerous health promoting compounds (Puente-Garza et al., 2017; Lim et al., 2020; Yi, 2021) commonly used in the agro-food industry as food additives, and emulsifiers in cosmetic products. While in some cases, saponins could be harmful for human consumption due to their weak absorption, and toxic effect (Yi, 2021).
Nevertheless, little information is available so far about the influence of organic amendments on saponins content. Therefore, the aim of this study is to investigate the influence of organic amendments on plant growth, seed yield, and grain quality parameters such as saponins, phenolic contents, and antioxidant activity in quinoa under different salinity levels.
2 Materials and methods
2.1 Experimental site
The study was conducted at the experimental farm of National Institute of Agricultural Research in Foum El Oued area, Laayoune, south of Morocco (X = -13.341°; X = 27.186°; Z = 13 m). The soil in the experimental site was sandy loam, characterized by strong salinity and low content of organic matter and nutrients (Table 1).
2.2 Experimental design and treatments
The experiment was conducted using a split-plot design with four replications. The salinity treatment was applied in the main plot, and the organic amendments were applied in the subplots. The area of each plot was 15 m2, each consisting of ten rows (Figure 1A). The crop was sown manually on April 13th, 2021, using the ICBA-Q5 quinoa variety. Irrigation was applied using a drip irrigation system (row-to-row spacing: 50 cm, distance between drippers: 20 cm, and flow rate: 2L/hr). The crop was irrigated twice a week and received a total irrigation amount of 240 mm during its growing cycle.
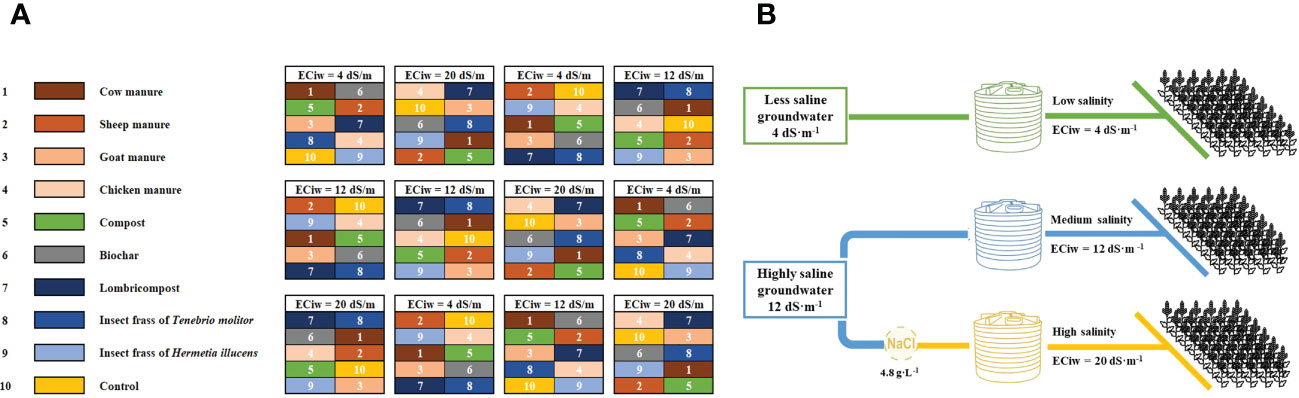
Figure 1 Experimental design showing allocation of main and sub-plot treatments (A), Layout of the irrigation water distribution system in the experimental plots (B).
Irrigation water salinity treatments were applied by filling water tank with two sources of groundwater for low (4 dS·m-1) and medium salinity level (12 dS·m-1), which corresponds to the lowest and the medium groundwater salinity level in the region, respectively. While 20 dS·m-1 was made by adding sodium chloride to the medium salinity treatment (Figure 1B).
Three irrigation water salinity levels, i.e., 4, 12 and 20 dS·m-1 were applied in main-plots. Characteristics of the irrigation water used in this experiment are presented in Table 2.
Nine different organic amendments in addition to a non-treated control were tested in sub-plots. Their characteristics and applied doses are presented in Table 3. The application rate of organic amendments was based on the local farmers’ practices and the cost of the products in the region.
2.3 Climatic condition
Figure 2 shows the variation of different climate parameters measured at the local weather station in the experimental site. The crop received a total amount of rainfall equal to 7 mm. Temperature and relative humidity were almost stable during the growing season, with 2 picks of high temperatures exceeding 30°C. The wind speed increased during the growing period (from April to July) and had an average speed of 5.5 m·s-1.
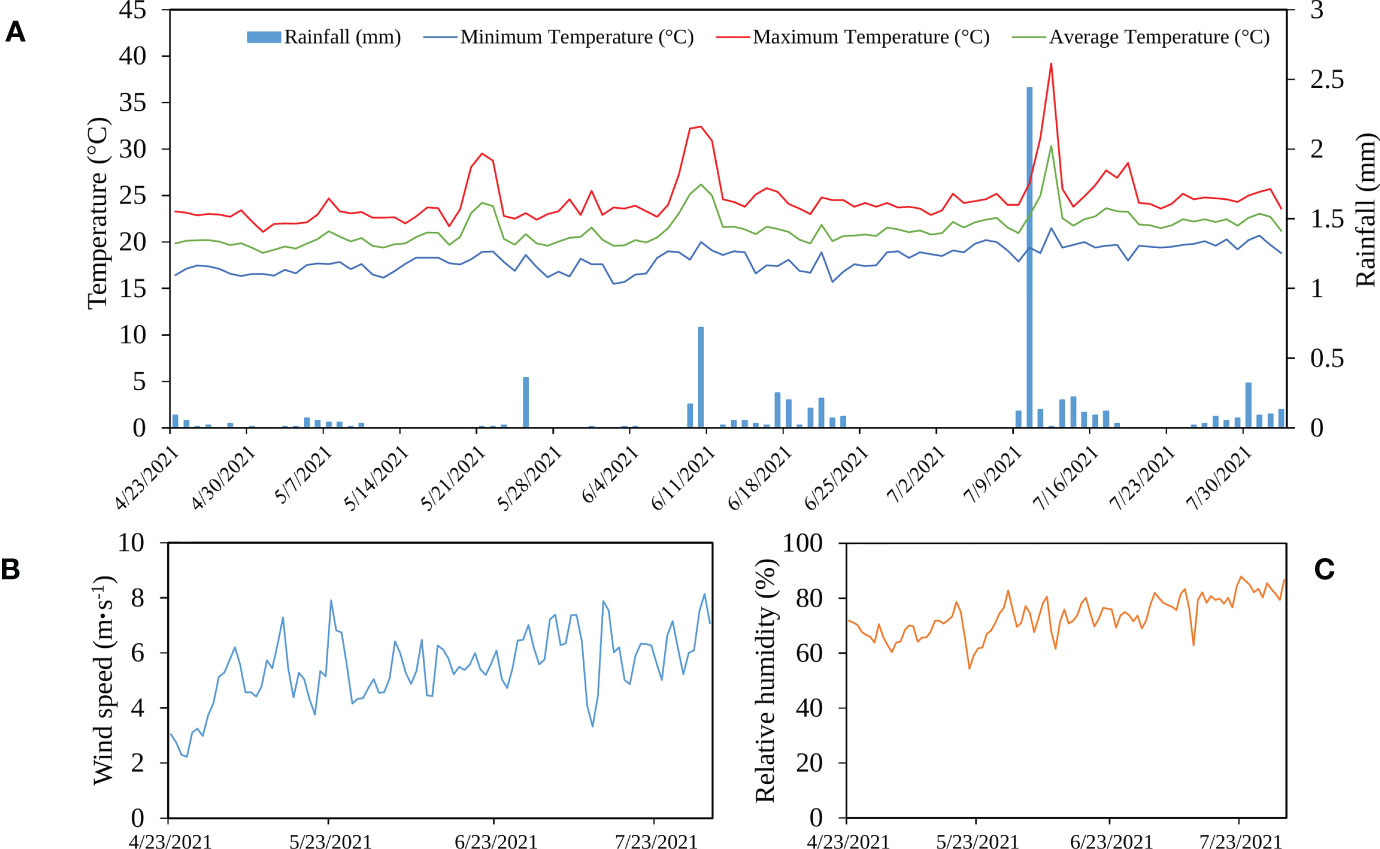
Figure 2 Variation of rainfall and temperature (A), wind speed (B), and relative humidity (C) during the growing period in Laayoune, Morocco.
2.4 Measurement parameter
2.4.1 Morphological traits
Four individual representative plants (sampled from the average height of randomly measured 10 plants) per plot were harvested (with root) at the beginning of the seed-filling stage, when leaves were still in the plants. All plant organs, including roots, stems, leaves, and panicles were separated and weighed (fresh weight). Root volume, length, and width of different organs were recorded, and then all plant materials were oven dried for 48 hours at 60°C to obtain a constant dry weight. Phenological observation such as days to appearance of 1st flowers; and the number of flowering plants were counted every 2 days interval until reached the 50% of the whole subplot were measured.
2.4.2 Crop productivity
The harvest was manually carried out for each row on July 29th, 2021. Fresh biomass was first determined, then the harvest product was oven dried until a constant weight of dry biomass was reached. Afterward, the biomass was threshed, and grains were collected, cleaned, and weighed to determine the seed yield. The harvest index (HI) was calculated as the ratio of seed yield to total dry biomass. Furthermore, crop water productivity (CWP) was calculated by dividing the seed yield by the total irrigation amount applied (also with rainfall).
2.4.3 Soil salinity and humidity
Soil electrical conductivity (ECe) and humidity were measured using Decagon TEROS 10 and 12 at the rootzone depth of 15 cm. Data were recorded every 15 minutes and stored in the Decagon ZL6 data logger. Soil salinity and humidity sensors were placed for sheep manure application’s plot under three irrigation water salinity treatments. While, soil moisture sensors were installed for biochar, compost, and sheep manure plots under low salinity treatment (4 dS·m-1) to compare the soil water retention for this three amendments.
2.4.4 Chemical analysis
Samples of soil, irrigation water, and organic amendments were analyzed in the AITTC-UM6P (Agricultural Innovation and Technology Transfer Center-Mohammed VI Polytechnic University) central laboratory following the Kjeldahl method for nitrogen, ICP-OES (Inductively Coupled Plasma-Optical Emission Spectrometry) for other elements such as phosphorus, calcium, magnesium, potassium, copper, manganese, iron, zinc, and boron. While the bicarbonates (HCO3) concentrations in the irrigation water were measured using the method ISO 9963-1 (ISO, 1994).
2.4.5 Biochemical content
2.4.5.1 Chlorophyll a and chlorophyll b content
Chlorophyll a (Chl a), Chlorophyll b (Chl b), and total chlorophyll were analyzed using the methodology as described by Pérez-Patricio et al. (2018). 0.5 g of fresh quinoa leaves were macerated under low luminosity conditions. The macerate was mixed with 4 mL of 99% acetone and 2 mL of ethanol (2:1 v/v), then stirred for 1 min. After 30 minutes in a dark freezer, the solution was centrifuged at 2000 rpm for 10 minutes, then 5 mL of acetone/ethanol (2:1 v/v) was added to the mixture and stirred for 1 min. The spectrophotometer was used to measure photosynthetic pigments at 663 nm and 645 nm. The solution of acetone/ethanol (2:1 v/v) was used as a blank solution. The following equations are used to calculate the concentration of chlorophyll a and b pigments:
A645 and A663 represent the absorbance values at 645 nm and 663 nm, respectively.
2.4.5.2 Leaf mineral content
The determination of mineral content of quinoa leaves was performed according to the protocol as described by Pequerul et al. (1993). The dried leaf samples were finely ground and sieved through a 2 mm pore sieve. Samples were further prepared by adding 8 ml of nitric acid (HNO3) to the grounded material (0.5 g), it was placed in a digestion tube and left overnight. The mixture was then heated at 90°C for 60 minutes and 3-4 ml of 30% hydrogen peroxide (H2O2) was added. The digestion process was stopped once the solution became colorless, and after cooling, the digest was filtered and diluted with hydrochloric acid (HCl). The elemental determination of sodium and chloride in extracted samples was carried out by using a flame photometer (FP902, PG Instruments).
2.4.5.3 Leaf proline content
The proline content of quinoa leaf was determined as described by Bates et al. (1973) with minor modifications. Briefly, 0.5 g of grounded quinoa leaf was weighed and homogenized with 3% 10 mL of sulfosalicylic acid. After that, 2 mL of the mixture was added to 2 mL of glacial acetic acid, and 2 mL of 2% ninhydrin solution were added. The mixture is incubated in a water bath at 95°C, after cooling in an ice bath, proline was extracted with 4 mL of toluene, the mixture is discarded, and the top layer was extracted, and its absorbance was measured at 520 nm. Proline concentration was calculated using a calibration curve of L-proline (0-40 µg/mL) and expressed as µmole Proline/g of fresh weight.
2.4.5.4 Extraction of bioactive components
Saponins extraction was carried out using the method of (Navarro del Hierro et al., 2018) with slight modifications. Briefly, 5 g of each quinoa seed’s powder was placed in a filter paper cartridge and defatted using the Soxhlet apparatus with Hexane (1:10 w/v) as solvent. Ultrasound-assisted extraction of saponins was performed with methanol (1:10 w/v). Extraction was carried out using an ultrasonic probe at 60% amplitude for 15 min (3 cycles of 5 min). The mixture was filtered through Whatman paper N°1. The mixture was centrifuged, and the supernatant was dried under vacuum and then reconstituted in 5 mL of methanol.
2.4.5.5 Total Saponin Content (TSC)
The total saponins content was determined using the modified method of Lim et al. (2020). 0.25 ml of saponin extract was added to 1 mL of reagent mixture (glacial acetic acid/sulfuric acid 1:1 v/v) and vortexed. After that, the mixture was incubated at 60°C in a water bath for 30 min and then placed in an ice bath to cool. The absorbance of the sample was read at 527 nm using a UV-visible spectrophotometer (VWR International, USA). Oleanolic acid was used to prepare the calibration curve (0 – 1000 μg/mL). Total saponins content was expressed as g of oleanolic acid equivalent/100 g DW.
2.4.5.6 Total Phenolic Content (TPC)
Total phenolic content was determined as reported by Gómez-Caravaca et al. (2011). Aliquots of 200 mL of each sample were mixed with 1 mL of 10% Folin reagent and left to stand for 6 min at room temperature in the dark. Then, 800 mL of Na2CO3 7.5% (w/v) was added to the mixture and placed in the dark to react for 30 min before measuring the optical densities at 750 nm. A control was prepared also and gallic acid was used as a reference standard to prepare the calibration curve. The total phenolic content was expressed as mg gallic acid equivalent (GAE)/100 g of DW.
2.4.5.7 Antioxidant activity (AOX)
Determination of the scavenging activity of quinoa extract was conducted using the 2.2-diphenyl-2-picryl-hydrazyl (DPPH) assay according to the procedure previously described by Fischer et al. (2013). Briefly, 4.9 mL of a 0.1 mM methanolic solution of DPPH (violet color) was mixed with 100 μL of different concentrations of quinoa extract. The tubes were allowed to react in the dark for 30 min at room temperature. The loss of violet color of the mixture was measured at 517 nm. A positive control containing methanol instead of the sample was prepared and Trolox (0-12 µmole) was used to prepare the standard curve. Results are expressed as µmol of Trolox per gram of extract (µmol TE/g E).
The method described by Yang et al. (2022) was used to measure the activity of scavenging ABTS radical cation (ABTS+). Initially, a stock solution of ABTS was created by mixing 7.4 mM of an aqueous solution of ABTS with 2.6 mM of an aqueous solution of potassium persulfate and allowing it to sit in the dark at room temperature for 12 hours. The ABTS+ cation solution was then diluted with methanol until its absorbance value was 0.7 ± 0.02 at 734 nm. Next, 100 μL of phenolic extracts were added to 2 mL of the ABTS+ cation solution and incubated in the dark for 10 minutes at room temperature. Finally, the absorbance of the mixture was measured at 734 nm. Results are expressed as µmol of Trolox equivalents per gram of extract (µmol TE/g E).
The modified method of Lim et al. (2020) was used to determine the reducing power of quinoa extract. In brief, each sample’s extract (0.5 ml) was mixed with 1.25 ml of 0.2 M phosphate buffer (pH 6.6) and 1.25 ml of 30 mM potassium hexacyanoferrate (III) and incubated at 68°C for 20 min. Then, 1.25 ml of 0.6 M trichloroacetic acid was added, and the mixture was centrifuged at 4032xg for 10 min. The supernatant was collected, and 1 ml of distilled water and 0.2 ml of iron (III) chloride were added to 1 ml of the supernatant. This mixture was kept at room temperature for 15 min, and the absorbance of the final test solution was measured at 700 nm using a spectrophotometer. Results are expressed as µmol of Trolox equivalents per gram of Dry weight (µmol TE/g DW).
2.5 Statistical Analysis
Statistical analysis was performed using the statistical programming language R 4.1.3. The additive model of the analysis of variance (ANOVA) was used to assess the effects of salinity and organic amendments on the monitored parameters. Then, Tukey’s multiple comparison tests was conducted to appraise the significant differences between the mean values at a 5% level of significance. Pearson’s correlation matrix was performed to investigate the strength of the linear relationship between variables and visualized using the “corrplot” package. Principal component analysis (PCA) was utilized to examine the correlation among the traits and evaluate the impact of the factors on the identified correlation patterns. To perform the PCA, the “factominer” and “factoextra” packages were used, and the factors were projected as supplementary qualitative variables.
3 Results
3.1 Agro-morphological traits
Variations of different monitored morphological traits of quinoa as affected by salinity level and organic amendments are presented in Table 4. Statistical analysis revealed a significant difference between tested salinity levels and organic amendments. In general, the salinity effect was not consistent from one parameter to another. For instance, an increase in salinity level resulted in a reduction of the most investigated parameters, while salinity did not significantly affect root length, root volume, and the number of panicles per plant. For some parameters such as panicle width, panicle dry weight, leaves dry weight, and plant dry weight, the highest values were recorded under the highest salinity level (20 dS·m-1). However, organic amendments effects vary across salinity levels. On average, the highest values of different investigated parameters are recorded under goat manure (PH, PnDW, LDW, and PDW), chicken manure (No.Pn, StDia, RV, RDW, StDW), or Lombricompst for panicle width. Days to flowering were significantly increased with increasing salinity. Under high salinity levels, compost and chicken manure recorded the highest no. of days to flowering (44 days), while sheep manure recorded the lowest (37) days.
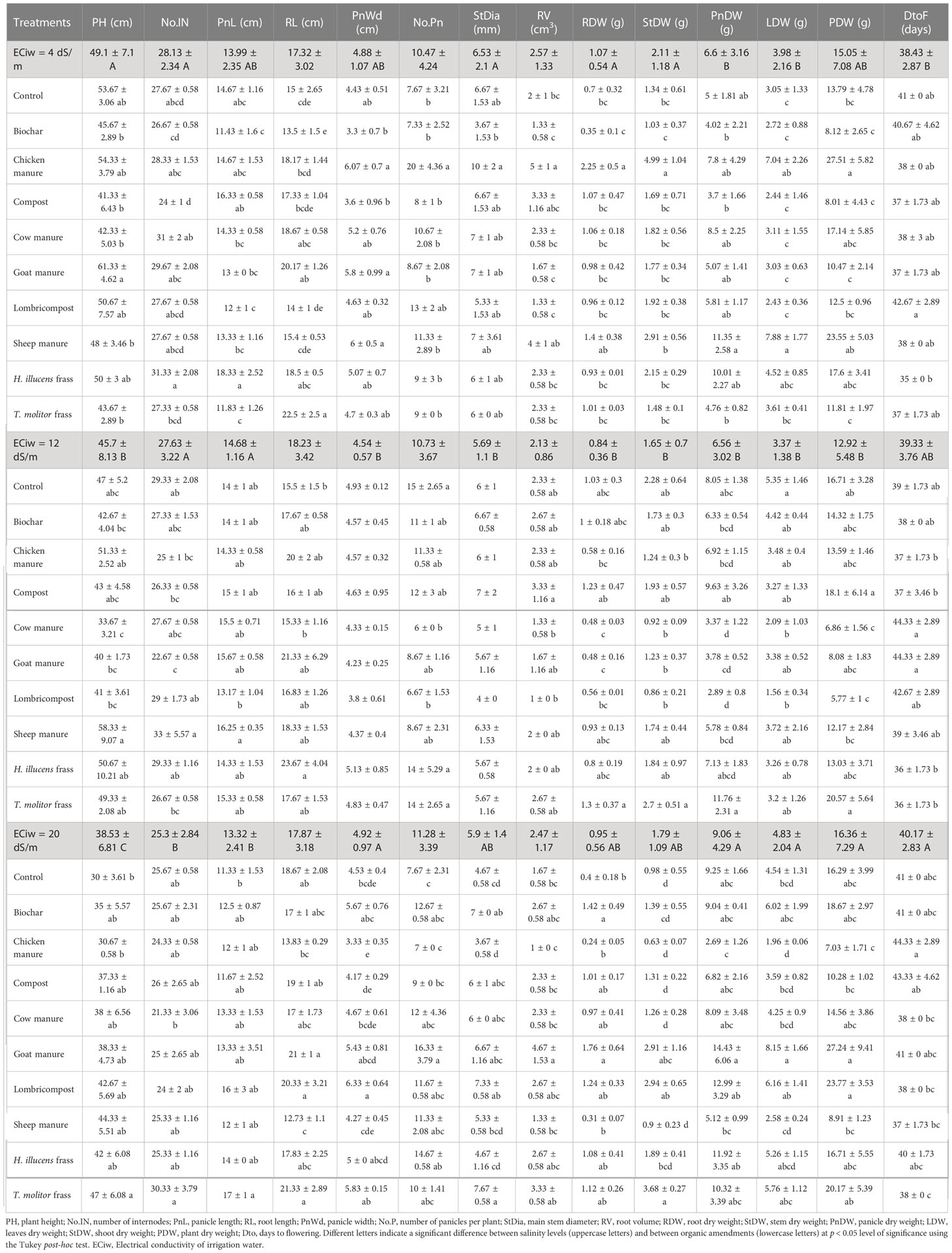
Table 4 Variation of different monitored quinoa morphological traits as affected by salinity level and organic amendments.
3.2 Crop productivity
The analysis of variance revealed that all investigated productivity parameters are affected (p<0.05) by salinity and organic amendments (Table 5). Salinity had a tremendous negative impact on biomass and seed yield, with an average reduction of 21 and 36% for fresh biomass, 25 and 37% for dry biomass, 31 and 52% for seed yield, and 29 and 51% for crop water productivity (CWP) under 12 and 20 dS·m-1 salinity levels, respectively compared to low salinity level (4 dS·m-1). Similarly, harvest index was significantly affected (p<0.05) at the high saline condition, with a decrease of 17% compared to the low salinity (4 dS·m-1). Regarding organic amendments, their response was inconsistent between one salinity level to another. For instance, averaged across all salinity levels, the insect frass of Hermetia illucens recorded the highest value in fresh biomass. In contrast, the Tenebrio molitor frass recorded the highest values of dry biomass and seed yield under high salinity. However, under high salinity conditions (20 dS·m-1), sheep manure recorded the highest values for dry biomass (1.91 t·ha-1), seed yield (1.42 t·ha-1), and crop water productivity (0.59 Kg·m-3).
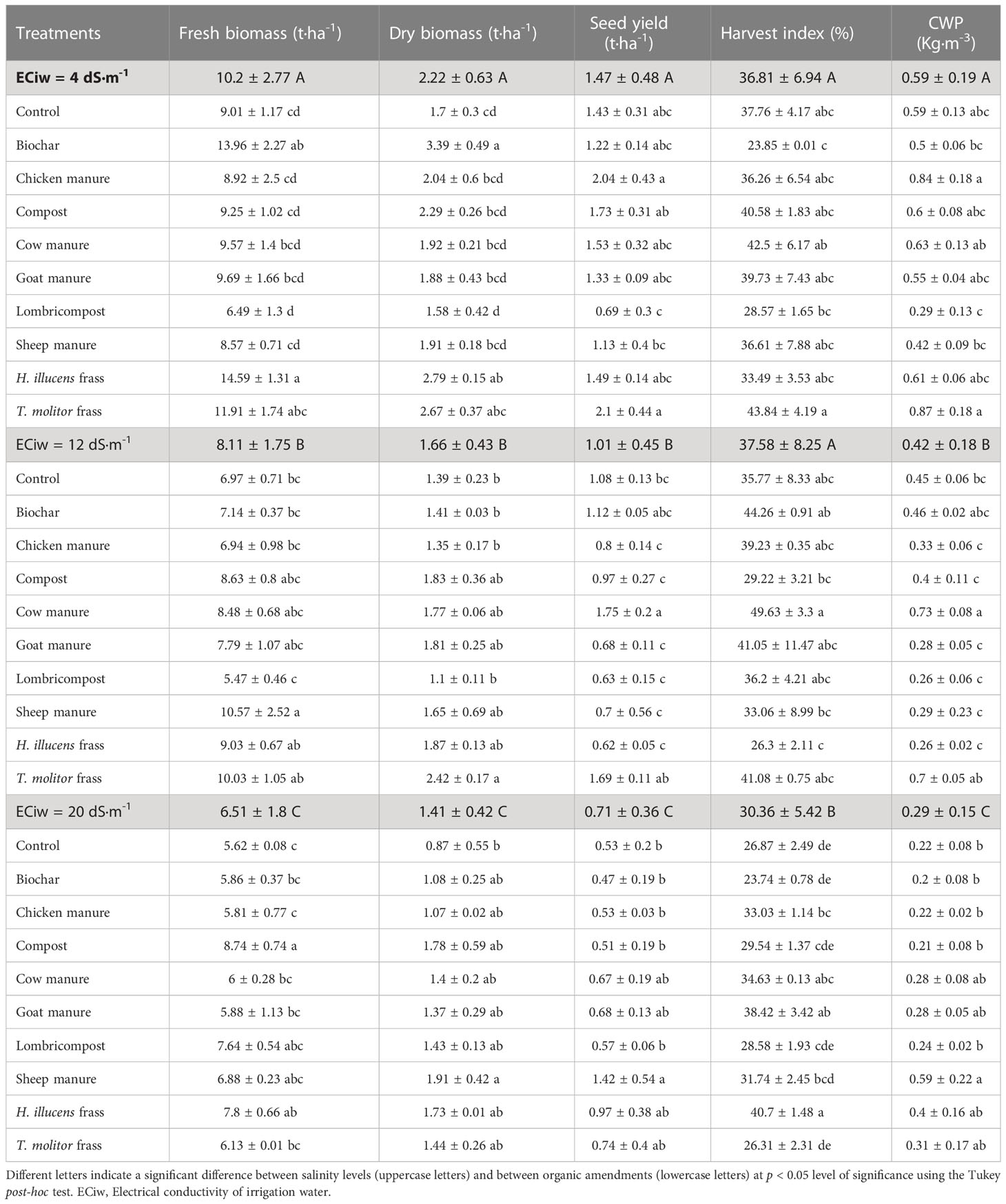
Table 5 Variation of quinoa fresh and dry biomass yield, seed yield, harvest index (HI), and crop water productivity (CWP) as affected by both salinity and organic amendments.
3.3 Soil salinity and humidity
Results of the soil salinity dynamics under sheep manure and different salinity levels are shown as stacked area plot in Figure 3A. The obtained data indicated that the measured soil ECe is well correlated with the applied salt concentration and varied according to soil moisture, as the soil EC sensors are very sensitive to soil humidity. The daily average of ECe varies from 5 to 15 dS·m-1, from 4 to 12 dS·m-1, and from 3 to 8 dS·m-1, under 4, 12, and 20 dS·m-1 of irrigation water salinity, respectively.
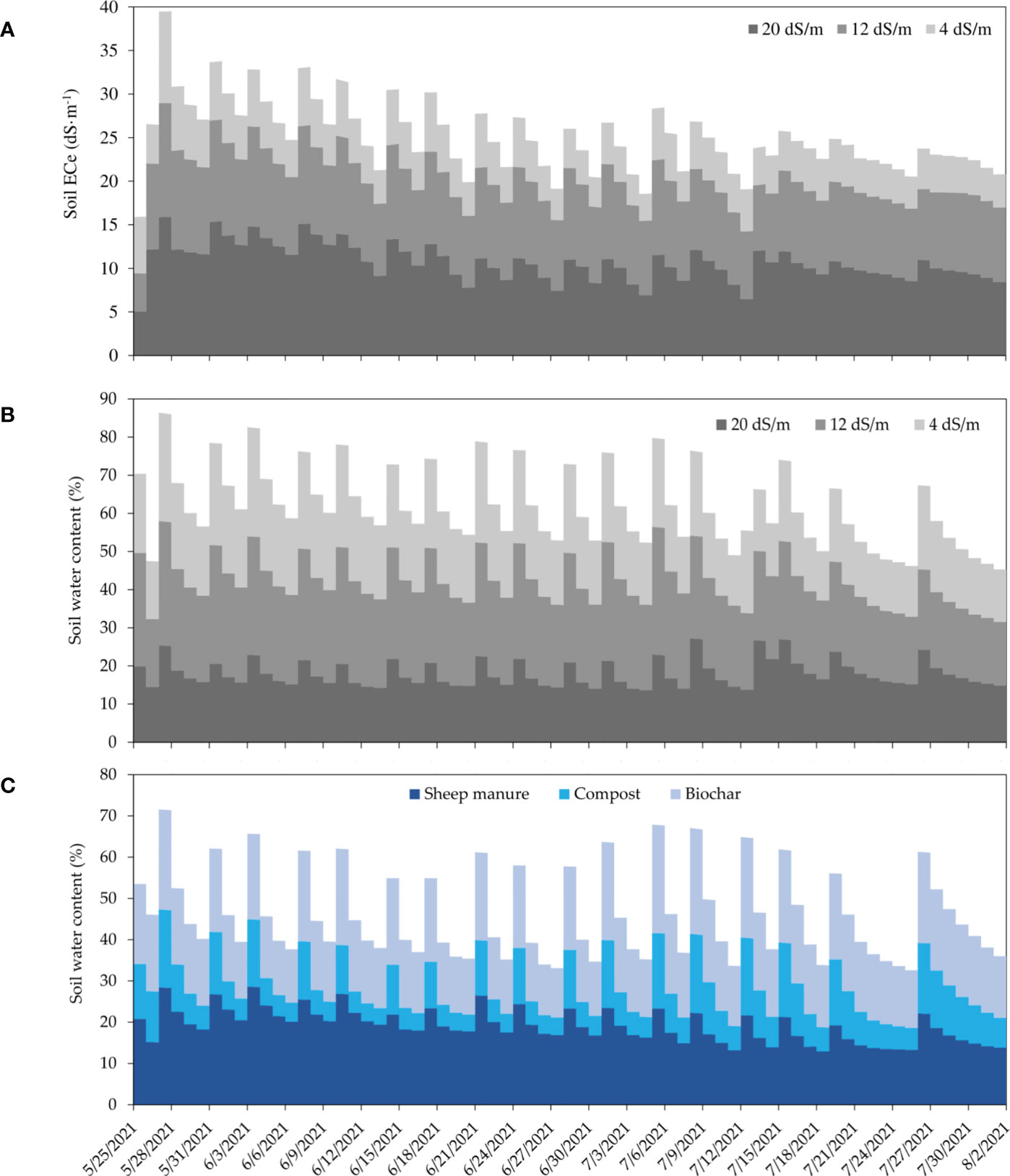
Figure 3 Stacked area plot of daily soil salinity (ECe) dynamics during the crop growing period measured under sheep manure amendment and three irrigation water salinity levels at 0-10 cm soil depth (A). Stacked area plot of daily soil moisture dynamics during the crop growing period measured under sheep manure amendment and three irrigation water salinity levels at 0-10 cm soil depth. (B), and under three organic amendments (sheep manure, compost, and biochar) measured at irrigation water salinity of (4 dS·m-1) (C), at 0-15 cm soil depth.
Soil moisture was less affected by salinity, and the average daily soil moisture were 19, 24, and 18% under low, medium, and high salinity levels, respectively (Figure 3B). Variations of soil moisture under low salinity level (4 dS·m-1) and three different organic amendments are shown in Figure 3C. Measured data clearly indicate that soil moisture varied from one amendment to another. For instance, biochar applied with 5 t·ha-1 holds more water than the compost applied with the same rate. Moreover, it holds more or less the same moisture (20%) as sheep manure applied at a rate of 30 t·ha-1.
3.4 Chlorophyll content
Chlorophyll in leaves is essential for plant growth and can be used to determine a plant’s reaction to stress. The analysis of variance was conducted to assess the effect of irrigation water salinity on chlorophyll pigments concentration under tested organic amendments (Figure 4). Results indicate a significant effect of irrigation water salinity on chlorophyll a (Chl a), chlorophyll b (Chl b), and total chlorophyll (Chl tot) contents. As an average of all tested amendments, the maximum concentration in terms of total chlorophyll was about 9.84 ± 1.43 mg/g of FW under medium salinity (12 dS·m-1). However, organic amendments significantly affected the contents of all measured pigments, except for chlorophyll b content under low salinity levels. While the insect frass of H. illucens and sheep manure significantly increased the total chlorophyll concentration by 76% and 61%, respectively, compared to the control under high salinity conditions (20 dS·m-1). Additionally, no significant difference was recorded for the chlorophyll a to chlorophyll b ratio under salinity conditions and for all tested organic amendments.
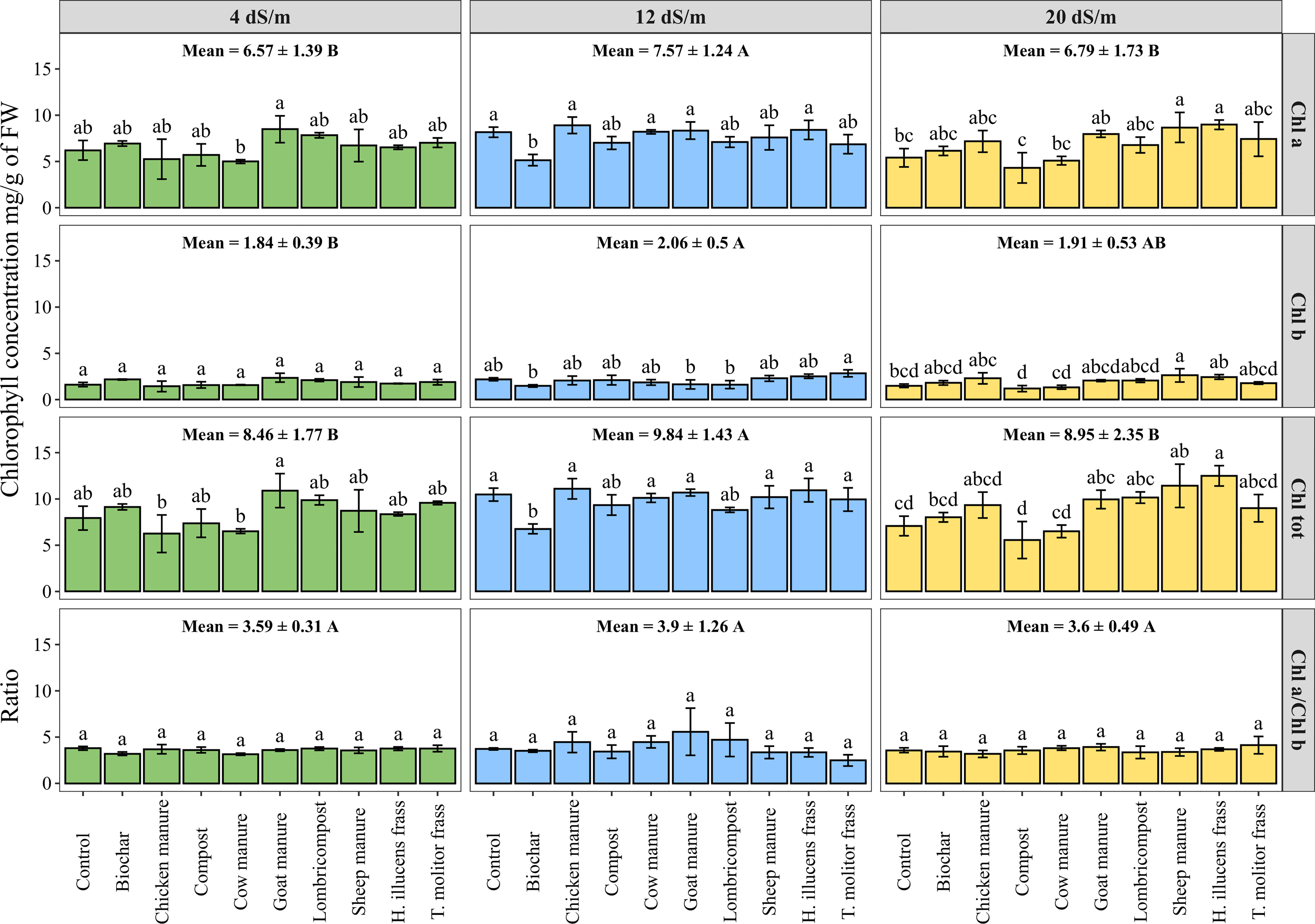
Figure 4 Photosynthetic pigments content measured on fresh leaves of quinoa as affected by different irrigation water salinity and organic amendments. Chlorophyll a (Chl a), chlorophyll b (Chl b), total chlorophyll (Chl tot), and chlorophyll a to chlorophyll b ratio (Chl a/Chl b). Values represent mean ± standard deviation. Different letters indicate a significant difference between salinity levels (uppercase letters) and between organic amendments (lowercase letters) at p < 0.05 level of significance using the Tukey post-hoc test.
3.5 Leaf mineral content
Analysis of variance revealed a significant effect (p < 0.01) of salinity and organic amendments on sodium content of quinoa. Conversely, the potassium content was not affected by the salinity treatments, and the effects of the organic amendments were only noticeable in the medium and high salinity conditions, which is demonstrated in Table 6. Results indicate an increase of 75% in sodium accumulation under high salinity (20 dS/m) compared to low salinity conditions (4 dS/m). In terms of amendment, goat manure was found to decrease sodium accumulation on the leaves of quinoa with 0.85 and 1.45 g/100g DW, under low and medium irrigation water salinity (12 dS/m), respectively. However, under high salinity conditions, sheep manure was more efficient in decreasing the sodium content (1.66 g/100g DW), increasing the potassium accumulation (12.53 g/100g DW), and subsequently resulting in a higher K/Na ratio (7.88).
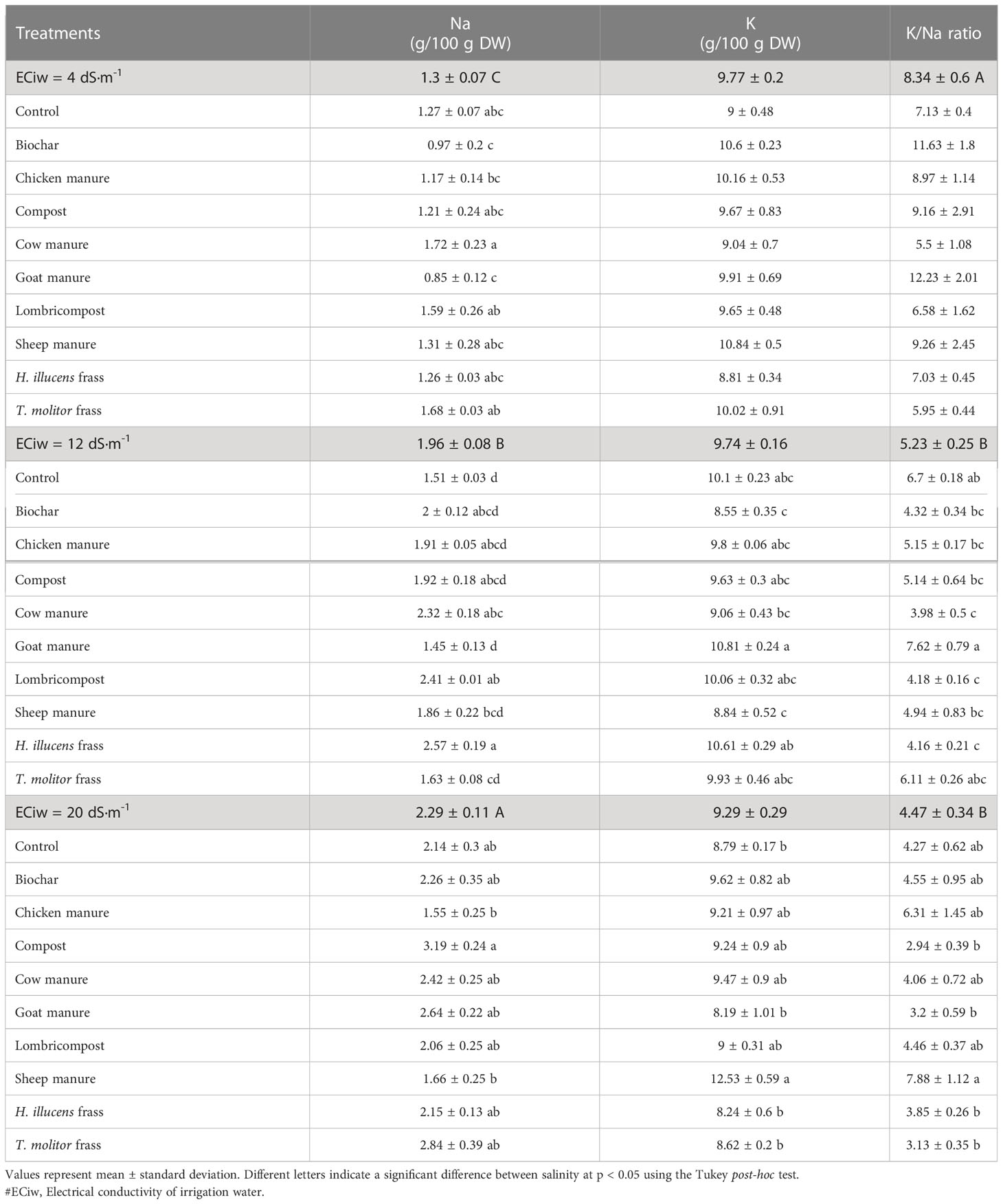
Table 6 Sodium and potassium contents, and K/Na ratio in quinoa leaves as affected by different salinity levels and various organic amendments.
3.6 Leaf proline content
Accumulating endogenous osmolytes in a plant’s leaf is a valuable indicator of the tolerance to osmotic stress. Free proline content measured under different irrigation water salinity levels and various organic amendments in quinoa leaves is shown in Figure 5. Data showed a significant increase of proline content by 38% from low to high salinity levels. However, amendments significantly decreased the proline level compared to the control at high salinity conditions. Meanwhile, Compost exhibited the highest proline content (24.9 µmole of Proline/g of FW), while sheep manure showed the lowest value (3.81 µmole of Proline/g of FW).
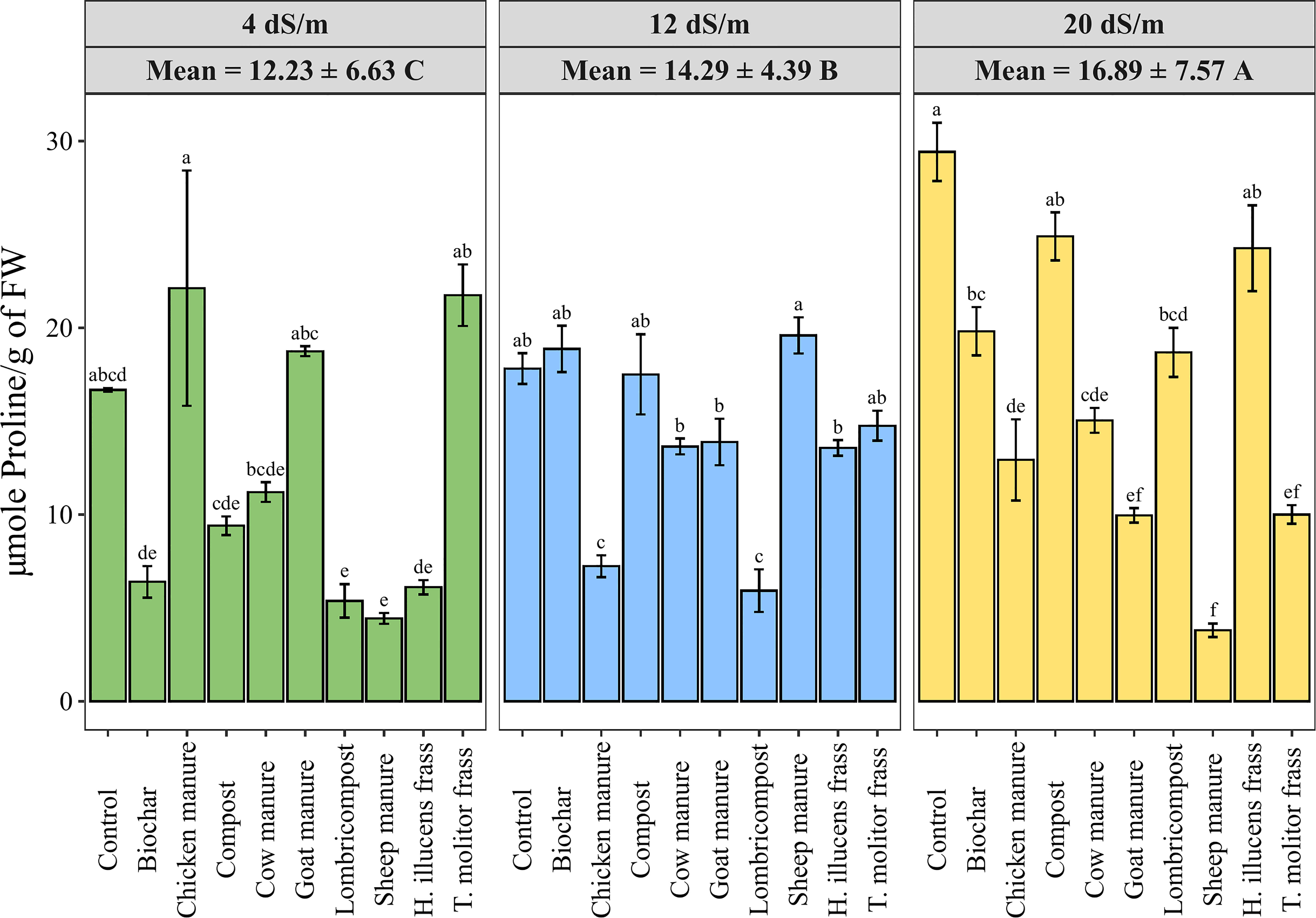
Figure 5 Variation of quinoa’s leaf Proline content under different salinity levels and various organic amendments. Values represent mean ± standard deviation. Different letters indicate a significant difference between salinity levels (uppercase letters) and between organic amendments (lowercase letters) at p < 0.05 level of significance using the Tukey post-hoc test.
3.7 Seed saponin content
Saponins are a class of compounds found in a wide variety of plants and are known for their characteristic bitter taste. The saponins content was measured in quinoa seeds in response to various organic amendments under three different salinity levels. Results presented in Figure 6 showed a significant increase in saponins content when salinity increased in different amendments averaging values of 0.45 ± 0.21, 0.59 ± 0.29, 1 ± 0.48% of DW under salinity levels of 4, 12, and 20 dS·m-1, respectively. Meanwhile, applying organic amendments significantly decreased the seed’s saponin content compared to the control in medium and high salinity levels. Hence, the application of goat manure decreased the saponin content by 76% at high salinity conditions compared to the control treatment.
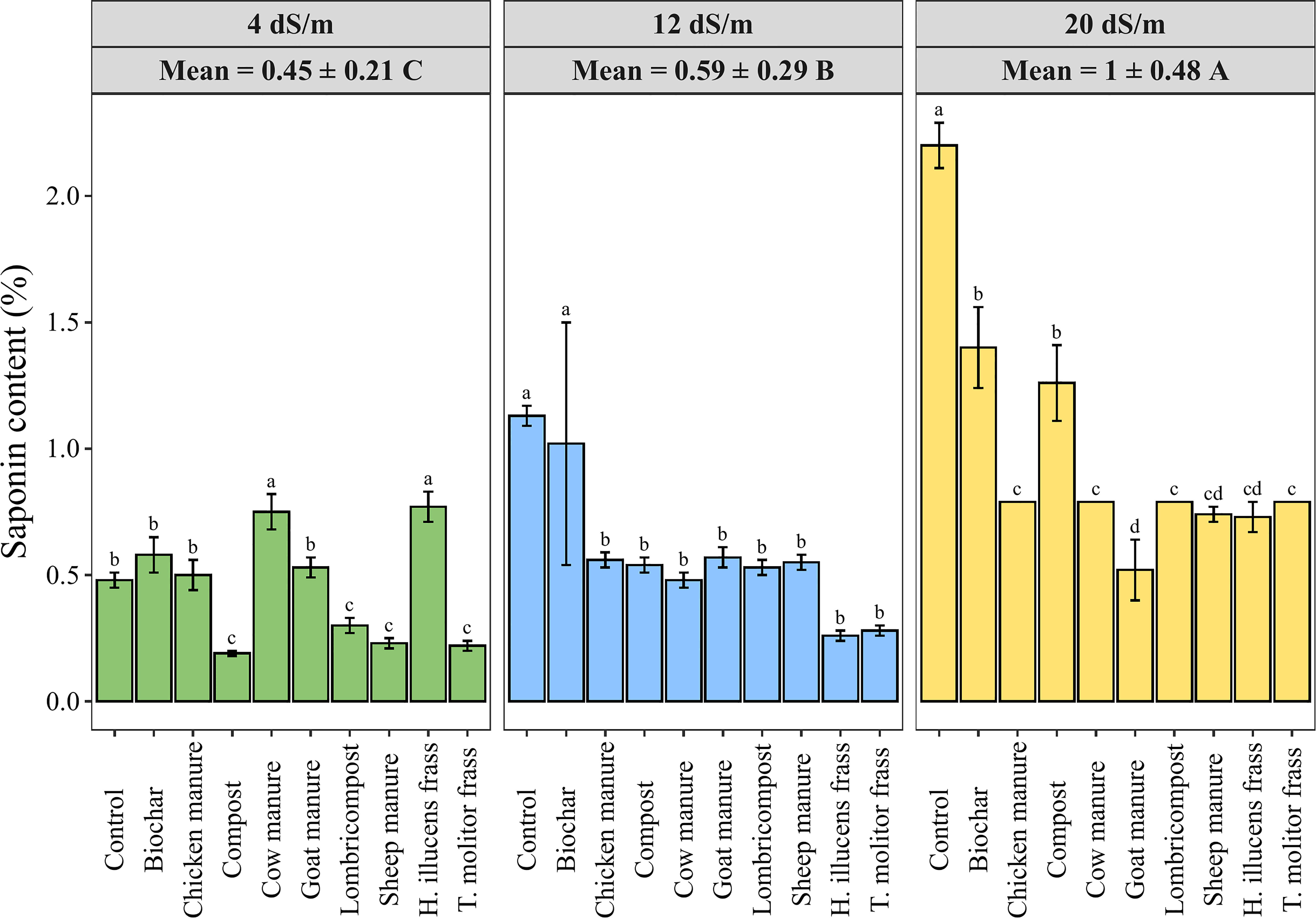
Figure 6 Variation of seed saponin content in quinoa as affected by different irrigation water salinity levels and organic amendments. Values represent mean ± standard deviation. Different letters indicate a significant difference between salinity levels (uppercase letters) and between organic amendments (lowercase letters) at p < 0.05 level of significance using the Tukey post-hoc test.
3.8 Seed polyphenol content
Polyphenols are a large group of naturally occurring compounds that are known for their antioxidant properties and are found in a wide variety of plants. The effects of salinity level and organic amendments on the polyphenols content (TPC) in quinoa seeds are presented in Figure 7. Data showed a significant effect of salinity on the production of polyphenolic compounds. Hence, higher salinity levels led to higher phenolic content in different organic amendments. Furthermore, applying different amendment treatments significantly decreased the total phenolic content in quinoa seeds compared to the untreated group. The use of sheep manure recorded the lowest value of TPC under a highly saline irrigation supply (133.52 mg GAE/g of DW). At the same time, the compost significantly increases the TPC in quinoa seed from 53.55 ± 1.23 to 349.48 ± 7.99 mg GAE/100 g of DW under 4 and 20 dS·m-1, respectively.
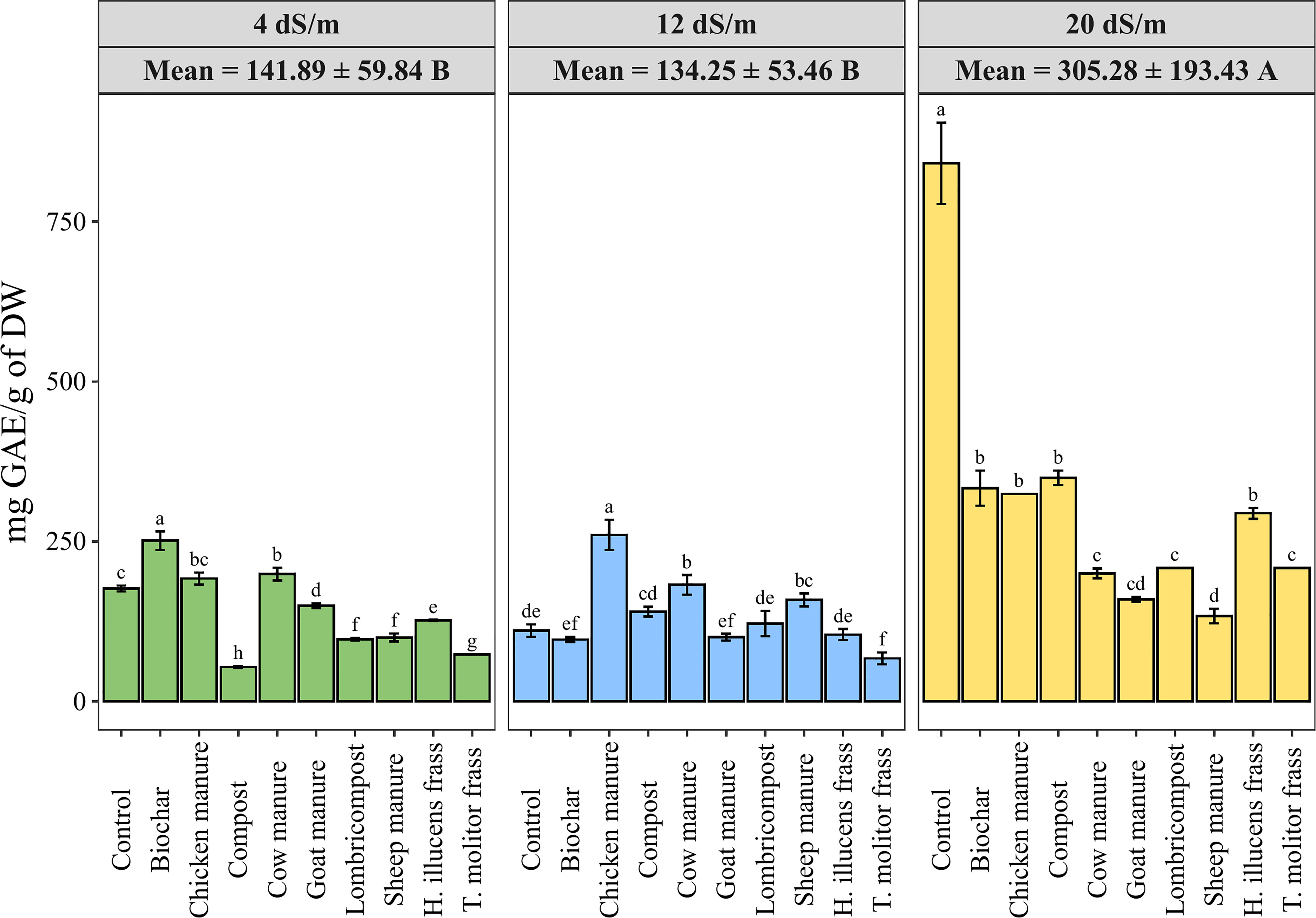
Figure 7 Variation of quinoa phenolic content under different salinity levels and various organic amendments. Values represent mean ± standard deviation. Different letters indicate a significant difference between salinity levels (uppercase letters) and between organic amendments (lowercase letters) at p < 0.05 level of significance using the Tukey post-hoc test.
3.9 Seed antioxidant activity
Plants’ antioxidant mechanism is triggered in response to the abiotic stress caused by excess salt exposure. This mechanism involves the activation of various enzymatic and non-enzymatic antioxidants that work to scavenge reactive oxygen species (ROS) produced under abiotic stress. The antioxidant capacity of quinoa seed is measured by DPPH, ABTS, and ferric-reducing antioxidant capacity. Quinoa seeds exhibited good antioxidant activity (AOX) across different groups of amendments, as depicted in Table 7. Compared to the control group, all amendment groups showed higher AOX when measured using the ABTS and FRAP assays, although there was no significant variation in antioxidant activity when measured using the DPPH assay. At ECiw = 4 dS/m, the highest AOX value was recorded in T. molitor frass using the DPPH assay (147.45 ± 1.15 µmol TE/g E), followed by H. illucens frass (147.22 ± 1.7 µmol TE/g E). Goat manure exhibited the highest FRAP value of 11.13 ± 0.99 µmol TE/g DW at ECiw = 20 dS/m, while compost showed the maximum value of 34.78 ± 2.3 µmol TE/g DW when measured using the ABTS assay at ECiw = 20 dS/m.
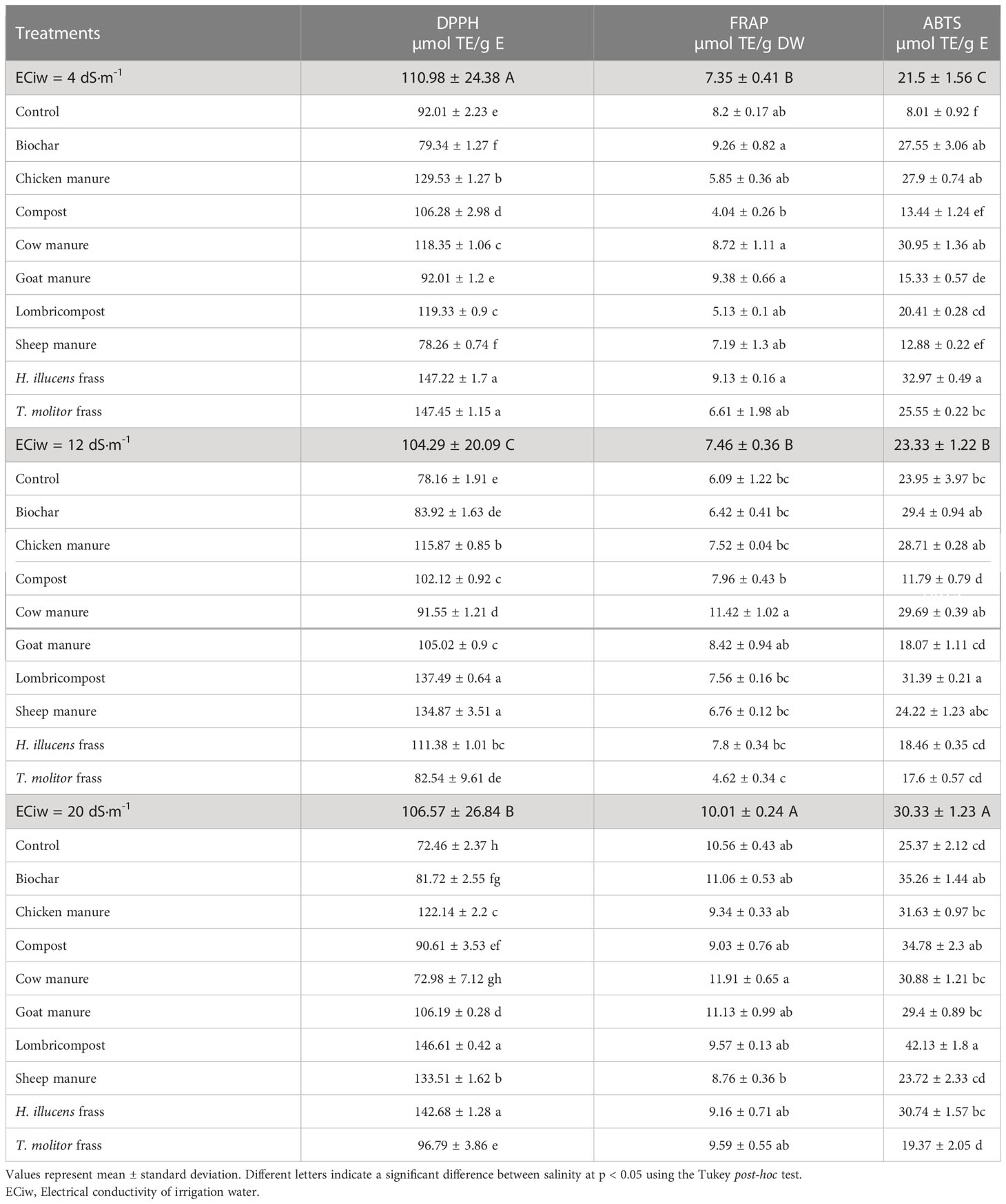
Table 7 Antioxidant content in quinoa as affected by different salinity levels and various organic amendments.
3.10 Correlation matrix and principal component analysis
Figure 8A presents the correlation between the investigated parameters. Results indicate a significant positive correlation among agro-morphological traits. In contrast, days to flowering negatively correlate with almost all agro-morphological and crop productivity parameters. However, total saponin content (TSC), total polyphenol (TPC), and proline content have a significant negative correlation with dry biomass and seed yield.
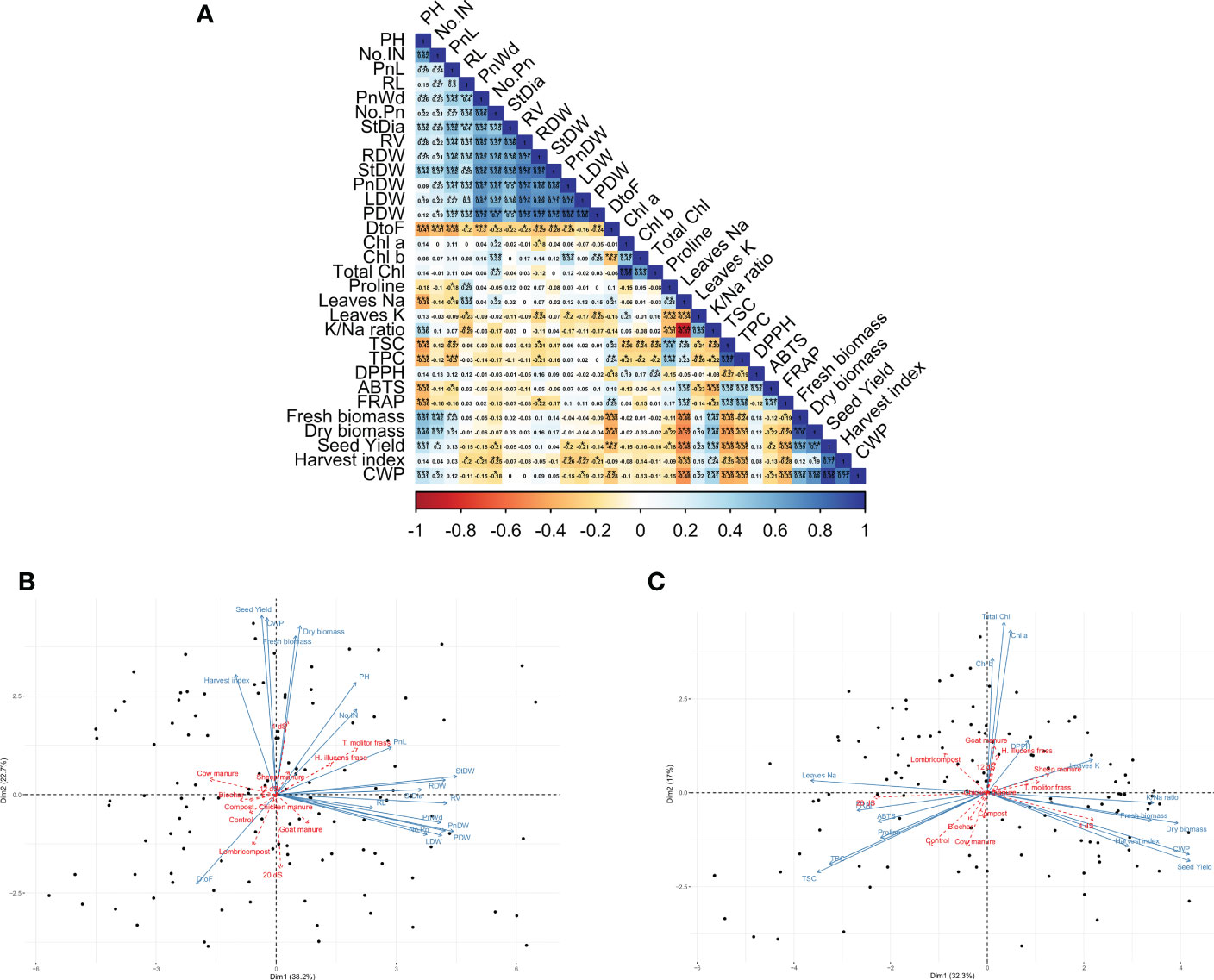
Figure 8 Pearson’s correlation matrix for the investigated parameters. Values in the matrix represent Pearson’s correlation coefficient. *, **, *** indicate the significance of the correlation coefficient at p < 0.05, 0.01, and 0.001, respectively (A). PCA-biplot projection of individuals (black points), variables (blue arrows), and supplementary qualitative variables (red arrows) on the main axis for agro-morphological and productivity traits (B), and biochemical and physiological parameters (C).
The principal plan explains 61% of the total variability in agro-morphological and productivity parameters (Figure 8B), and 49% for the relationship between productivity traits and biochemical and physiological measurements (Figure 8C). Plotting the treatments as a supplementary qualitative variable indicates that the clustering could be revealed, especially for the irrigation water salinity treatment. However, most of the organic amendments are not well presented on the first two dimensions. The results indicate that productivity parameters, such as biomass production and seed yield, are positively correlated with low salinity treatment (4 dS/m). Consequently, the same productivity parameters had a strong negative correlation with high salinity treatment (20 dS/m).
In terms of biochemical composition, the factor analysis revealed a strong positive correlation between TSC, TPC, ABTS, FRAP, Proline, and sodium content with the high salinity treatment (20 dS/m), while low salinity treatment is mainly associated with a high K/Na ratio. Regarding the organic amendments, T. molitor and H. illucens frass are positively correlated with most of the agro-morphological traits, while the non-treated control is positively correlated with TSC and TPC.
4 Discussion
It is well known that quinoa is a facultative halophyte that resists to high salinity levels without a notable effect on growth and development (Adolf et al., 2013). In contrast, in our experiment the effect of salinity on different measured morphological traits was pronounced, and a significant effect of salinity was noticed. Our findings are aligned with several previous studies reporting the impact of salinity on quinoa growth and productivity. For instance, Long (2016) found that plant height, root length, shoot dry weight, root dry weight, and panicle length were slightly reduced under high salinity conditions with a reduction rate of 13, 17, 9, 12 and 10% under high salinity level (150 mM) compared to the control (0 mM). Similarly, Talebnejad and Sepaskhah (2015) found that salinity increase from 10 to 20 dS·m-1 slightly reduced plant height, shoot and root dry weight by about 3, 10 and 15%, respectively. Contrarily to our results where number of panicles per plant increased by 8% under high salinity, Rezzouk et al. (2020) reported that salinity reduced the number of inflorescence or panicle per plant by 12% under saline compared to non-saline conditions. However, their results in terms of plant height and panicle length are in agreement with the finding of the present study. In relation to the quinoa genotype ICBA-Q5 utilized in our experiment, numerous studies have been carried out in arid regions to evaluate the impact of salinity on quinoa’s morphological characteristics. However, a recent study by Shahid and Thushar (2021) indicated a reduction in plant height, number of panicles, and panicle length by 22%, 8%, and 12%, respectively, when subjected to saline irrigation (ECiw = 15 dS/m) in comparison to freshwater irrigation (ECiw = 0.3 dS/m).
Regarding quinoa productivity including seed and biomass yield, several studies reported a similar findings for other tested varieties. For example, Jacobsen et al. (2003) found that seed yield of quinoa was reduced by 10% under 20 dS·m-1 compared to 5 dS·m-1, while under fresh water conditions, the quinoa seed yield was similar to that obtained under 20 dS·m-1. They also found that the highest seed yield was obtained under 15 dS·m-1 and the lowest yield under seawater salinity level (40 dS·m-1) with a reduction rate of only 44% compared to the highest yield. Similarly, (Koyro and Eisa, 2008) showed that quinoa seed yield only decreased by about 15 and 16% under 10 and 20 mM NaCl, respectively compared to the control (0 mM NaCl). While quinoa seed yield was greatly declined under 300 and 500 mM NaCl by 44 and 97% respectively, compared to the control. Similarly to our finding (Iftikhar Hussain et al., 2020) reported that salinity has negatively affected quinoa growth and productivity. In fact, seed yield was reduced by 44 and 60% under 10 and 20 dS·m-1, respectively compared to the control (0 dS·m-1). However, dry biomass yield seemed to be less affected by salinity as it was only reduced by 2 and 3% under 10 and 20 dS·m-1 respectively. Contrarily, in a previous experiment in the same experiment site using the same quinoa variety (ICBA-Q5), Bouras et al. (2022) showed that yield was significantly increased by 27 and 13% under saline irrigation water with EC values up to 12 and 17 dS·m-1 respectively, compared with the control. Similarly, the same experiment showed an increase in biomass yield by 17 and 29% higher at saline irrigation with EC values of 12 and17 dS·m-1, respectively, compared with the control.
All those reported findings showed that quinoa has the ability to resist and produce under high salinity conditions. Quinoa resistance to salinity involves several mechanisms. One of them is its ability to accumulate salt ions in its cells and tissues in order to control and adjust leaf water potential. This enables the plants to maintain cell turgor and therefore, limit transpiration under saline conditions, thus, avoiding physiological damage (Jacobsen et al., 2003). Adolf et al. (2013) summarized the different physiological mechanisms that quinoa deploys to resist to salinity including: (1) stomata regulation and density by maintaining stomatal conductance and reducing stomata density under salinity conditions, (2) exclusion of toxic ions in the salt bladders, (3) osmo-protection by accumulating compatible osmolytes such as proline and mannitol, (4) osmotic adjustment by using inorganic ions such as Na, K and Cl as cheap osmotic in shoot tissue, (5) sodium translocation at xylem parenchyma rather than Na extrusion and (6) potassium retention by increasing K uptake and maintain a high K/Na ratio under salinity conditions. In our experiment, the determination of mineral content indicates a high accumulation of sodium on quinoa leaves under high salinity conditions, resulting in an increase of the K/Na ratio. However, Oumasst et al. (2022) observed a similar trend for sodium accumulation in the ICBA-Q5 variety under arid conditions in the south of Morocco. They found that the sodium content increased tenfold under 10 dS/m of irrigation water salinity compared to 0.9 dS/m salinity level. On the other hand, Bouras et al. (2022) reported no significant effect on the K/Na ratio. However, they found that both sodium and potassium contents increased by 60% and 65%, respectively, under high salinity conditions (17 dS/m) compared to the control (5 dS/m) at the same experimental site using ICBA-Q5 as a quinoa cultivar.
The organic amendment effect on quinoa was reported by several studies to improve its growth and development. Organic amendment mitigates the adverse effects of salinity by several ways including nutrient availability increase, improving soil structure which ease the salt leaching and improve soil aeration, increasing soil water holding capacity, improving soil micro-organisms activity (Diacono and Montemurro, 2015). Thus, plants assimilate more nutrients and water and accumulate more reserves and biomass. The organic amendment can also be beneficial under salinity conditions. Our finding indicates clearly that all tested organic amendments resulted in average more or less similar or higher values of seed and biomass yield compared to the control. Under high salinity conditions (20 dS·m-1), most of tested organic amendments have improved quinoa seed yield. For instance, sheep manure and insect frass of Hermetia illucens increased seed yield by 167 and 83% compared to the control. Very few reports are available on the impact of organic amendment on quinoa productivity under salinity conditions. Abdrabou et al. (2022) reported that applying 20 t·ha-1 farmyard manure to quinoa increased seed yield by 6-fold compared to non-treated control. Alcívar et al. (2018) found that application of biochar, humic substances or both combined significantly improved quinoa root dry weight, root length and seed yield, conversely our study showed that applying biochar at a rate of 5 t·ha-1 under high salinity conditions reduced yield by 11% compared to the control. However, under medium salinity level biochar significantly improved yield by 4% compared with the control. Gill et al. (2020) reported that an application of 20 and 30 t·ha-1 of biochar resulted in a significant increase in quinoa biomass by 54 and 113%, respectively compared to the control. Similarly, seed yield also increased by 116 and 153%, respectively under 20 and 30 t·ha-1 of biochar.
The finding of this study revealed that biochar significantly improved seed yield by 4% under medium salinity (12 dS·m-1) level compared with the control, with no improvement under high salinity conditions (20 dS·m-1). While compost improved seed yield by 21% under low (4 dS·m-1) salinity conditions, while it reduced seed yield by 11% under medium salinity level (12 dS·m-1) compared to the control. The finding also indicates that soil moisture under low salinity conditions and biochar application was maintained much higher than compost applied with the same dose. It is more probable that the high-water holding capacity of biochar compared to compost is among the factors that led to an improvement of the quinoa biomass and seed yield, especially under salinity conditions (Yang et al., 2020). While under high salinity conditions, quinoa responded better to compost rather than biochar due to its high carbon to nitrogen ratio compared with biochar in which the organic matter is more stable (Table 3).
It is very difficult to explain the quinoa yield improvement under different organic amendments and different salinity levels as the behavior of each amendment varies from salinity level to another. Moreover, the yield improvement can be explained by amendment nutrient content, mineralization rate and speed, ability to improve soil water holding capacity, and biological activity. Several soil physical properties are adversely affected by salinity, making it challenging for plants to establish and thrive. The high concentration of sodium ions in the soil causes dispersion and swelling of aggregates, leading to reduced permeability and increased crusting of the soil surface (Arora and Dagar, 2019). To counter these effects, organic amendments such as manures and composts can be added to increase the concentration of humic colloids in the soil, thereby improving the structural stability of soil aggregates and enhancing water-holding capacity (Dorado et al., 2003). Diacono and Montemurro (2011) have reported that animal manure’s high negative charge increases the organic carbon content, resulting in a greater cation exchange capacity, which helps in reducing nutrient loss. This is important in enhancing the organic carbon content, available phosphorus, and extractable potassium, making them readily available for plant growth. On the other hand, Goss et al. (2013) conducted a review of several contaminants associated with animal manure that can potentially pose health risks and environmental hazards. These contaminants include pathogens such as bacteria, viruses, protozoa, and helminthic worms, as well as macronutrients and trace elements. These contaminants can infect both farm animals and humans through contaminated feed, food, and water supplies, and thus pose potential limitations and challenges associated with the use of organic amendments in large-scale agricultural production. Other limitations that may face farmers using large amounts of organic amendments under salinity is the consequent costs associated to their purchase and application.
Proline is a proteinogenic amino acid that accumulates naturally in plants under various biotic and abiotic stress (Hare and Cress, 1997; El Moukhtari et al., 2020; Pingle et al., 2022). Accumulation of proline in cytosol and plastids of plant tissues engenders the adjustment of cellular osmotic pressure and maintains the plant’s cell turgidity (Szepesi and Szollosi, 2018). In this present study, increased salinity level led to an increasing free proline level in different groups of amendments and control. These results agreed with previous studies reporting that salt stress caused a significant increase of proline accumulation by 5-folds in shoots of Indian green gram (Misra and Gupta, 2005), similarly, (Hmidi et al., 2018) showed that proline content and amino acids increase with increasing salinity and duration treatment. Meanwhile, the application of organic amendments in quinoa significantly increased the proline content. In agreement, previous studies reported the accumulation of osmoprotectants in amendments-treated plants under salt-stress conditions (Flowers and Colmer, 2008; Talaat and Shawky, 2014; Kumari et al., 2022). According to (Patel et al., 2018), an increase in proline content was observed under abiotic-induced stress in Triticum durum treated with Kappaphycus alvarezii sap (K-sap). Overall, the accumulation of proline drastically improves the salt-stress tolerance of plants through different mechanisms, a part of being an osmoprotectant, proline can act as a regulator of redox status and attenuates cell acidity (Talaat and Shawky, 2014). Generally, proline level depends on the crop varieties, duration, and severity of salt stress. However, the accumulation of proline is not a specific indicator of salt stress, as it can be accumulated under different types of stress (drought, temperature) (Szepesi and Szollosi, 2018).
Phenolic compounds are a wide range of phytochemicals classified as secondary metabolites, endowed with diverse biological activities, including antioxidant (Sabraoui et al., 2020), antiproliferative (Paludo et al., 2022), hepatoprotective (Jia et al., 2022), and antidiabetic properties (Tatipamula and Kukavica, 2021). Phenolic acids are produced naturally in fruits and vegetable plants under various forms of stress (oxidative stress, salt stress, and drought stress) but also found abandonly in the grain fraction of cereals and crops (Tiozon et al., 2022). In Quinoa, phenolic compounds are present in two forms (soluble free or soluble conjugated to polysaccharides), with the predominance of gallic acid and ferulic acids followed by flavonoids (Pereira et al., 2020). Thus, free phenolic compounds contribute effectively to the sweet flavor of quinoa compared to the conjugated (Suárez-Estrella et al., 2018). In the current study, the TPC in quinoa seed ranged from 53.55 ± 1.23 to 841.01 ± 3.16 mg GAE/100 g of DW. In similar studies, (Han et al., 2019) studied the phenolic content of seven different varieties of coloured quinoa, the TPC content varied from 167.2 to 303.8 mg GAE/100 g of DW. Another work by (Mhada et al., 2020) reported values of TPC of 31.67 ± 7.26 and 105.85 ± 5.21 mg GAE/100 g of DW respectively in raw quinoa of two variety (Puno and Titicaca). In addition, at higher concentrations of salinity, TPC tends to increase significantly which is inconsistent with previous reports in which salinity enhances the biosynthesis of phenolic compounds (Petridis et al., 2012; Bistgani et al., 2019). Moreover, the finding of this study revealed that the application of amendments lowered significantly the TPC compared to the untreated quinoa. In a similar study, (Chehab et al., 2020) indicated that compost negatively influenced the phenolic content in olive fruits grown under saline conditions. Generally, environmental stress and particularly salinity stress regulates the biosynthesis of secondary metabolites. Organic amendments are a rich source of natural antioxidants such as vitamins, and amino acids which could attenuate salt-induced oxidative stress by modulating the antioxidant defense and the chelating activity of this crop (Liu et al., 2021).
Saponins are classified as anti-nutritional secondary metabolites of the glycoside family, naturally produced by plants as a response to a large spectrum of environmental abiotic stress;(Szakiel et al., 2011; El Aziz et al., 2019). Based on their chemical, saponins are divided into two main groups: steroidal and triterpenoid, their content varies from one plant to another depending on the variety, geographic location, and genetics (Cheok et al., 2014; Liao et al., 2017). Quinoa is a pseudo-cereal characterized by an abundant level of saponins, 86% is located mainly in the pericarp of the seed (Ando et al., 2002) their concentration in the upper layer is responsible for the bitterness or sweetness of the seed (Suárez-Estrella et al., 2018). In the current study, saponins’ level content raged from 0.22 ± 0.01 to 2.21 ± 0.07% of the dry weight at different salinity levels. (Mhada et al., 2020), reported similar values: 1.41 ± 0.05 to 2.09 ± 0.29% of dry weight evaluating the saponins content of raw quinoa (Puno and Titicaca). (El Hazzam et al., 2020) indicated a higher value of 6.34%. The bitter flavor of quinoa is a limiting factor and an organoleptic default to its introduction to the human diet. Therefore, different removal techniques have been reported in the literature: wet, dry, and genetics. To the best of our knowledge, this study is the first of its kind to describe the saponin response to organic amendment. When applied, organic amendments effectively reduced the saponin content at different salinity levels, such effect can be attributed to different mechanisms of amendments on attenuating the salinity-induced stress (Kumari et al., 2022). A study (Alam et al., 2020) suggested that organic amendments enhanced antioxidant activity and pea biosynthesis in pea crops grown under arsenic-contaminated soil. (Diacono and Montemurro, 2015) also indicated that organic amendments influence the physicochemical properties of soil due to the flocculation of minerals to organic polymers.
The damaging effects of salinity on plants, such as oxidative stress leading to harm to plant proteins, lipid peroxidation, and DNA damage, have been widely recognized. This occurs when excessive accumulation of reactive oxygen species (ROS) interferes with regular metabolism, (Petridis et al., 2012; Bistgani et al., 2019). The study employed three assays (DPPH, ABTS, and FRAP) to evaluate the antioxidant capacity of plant extracts, which involved the transfer of electrons from antioxidants to radicals to stabilize them. The findings indicated that quinoa seed exhibited notable antioxidant activity across various groups, regardless of the salinity level, while amendments significantly boosted the AOX compared to the untreated group. As previous studies have established, the production of phenolic compounds, which are secondary metabolites generated in response to abiotic stress, played a noteworthy role in the antioxidant capacity of a variety of plant species (Tang et al., 2015; Rusli et al., 2022). However, in our study, we did not observe any correlation between the total phenol levels in quinoa extracts and the antioxidant activity. The phenol content in these extracts showed a higher correlation with the results of the reducing power (FRAP) assay than with the DPPH and ABTS scavenging activity (Table 7). It has been noted by other researchers that the total phenol levels in extracts from pseudocereal seeds may not always be linked to their antioxidant activity. Hence, polyphenols from quinoa may be effectively reducing agents for metal ions but may not scavenge DPPH and ABTS efficiently due to steric hindrance (Yawadio Nsimba et al., 2008; Dini et al., 2010; Starzyńska-Janiszewska et al., 2016). The limited correlation observed between the overall phenolic content and the antioxidative activity implies that the primary antioxidants present in the examined seeds could be of a non-phenolic nature such as ascorbic acid, tocopherols, and carotenoids. This indicates that the understanding of the sources and mechanisms of antioxidant activity in quinoa is complex and cannot rely solely on total phenol levels. Further investigation is needed to identify the specific compounds responsible for the observed antioxidant activity in quinoa extracts. This could involve the isolation, identification, and quantification of individual polyphenols in quinoa extracts as well as more detailed analyses of their mechanisms of action.
5 Conclusions
This field experiment examined the ability of quinoa (Chenopodium quinoa Willd.) ICBA-Q5 variety to grow under different irrigation water salinity in combination with organic amendments. The finding of this study revealed considerable potential for improving quinoa yield under high salinity conditions through the application of organic amendments. Application of amendments alleviated salinity stress at the physiological level and ensured nutrient availability for plants. Further, saponin accumulation significantly decreased in response to the application of amendments, which represents an excellent opportunity to facilitate post-harvest operation and improve the grain quality of quinoa. Considering the several criteria such as yield, biochemical, and physiological, application of organic amendment is therefore suggested to improve quinoa productivity undersalt affected irrigated drylands such as South of Morocco.
Data availability statement
The raw data supporting the conclusions of this article will be made available by the authors, without undue reservation.
Author contributions
Conceptualization and methodology: AH, KD, AM, Talal Sabraoui. Data curation: AM, TS, AH. Investigation: AM, TS, IM, MB, FS, RE, MK, JZ. Chemical analysis: TS, AM, MI, KL, IM. Supervision: AH. Writing original draft: AM, TS, SR, AH. Review-editing: KD, CG AN. Resources mobilization: AH, CG, LK. All authors contributed to the article and approved the submitted version.
Funding
This research was funded by the Moroccan Ministry of National Education, Professional Training, Higher Education and Scientific Research within the ERA-Net Cofund on Food Systems and Climate, SALAD project under the contract number 5.
Acknowledgments
We would like to thank the National Agricultural Research Institute in Foum El Oued, Laayoune for providing field facility to undertake this research.
Conflict of interest
The authors declare that the research was conducted in the absence of any commercial or financial relationships that could be construed as a potential conflict of interest.
Publisher’s note
All claims expressed in this article are solely those of the authors and do not necessarily represent those of their affiliated organizations, or those of the publisher, the editors and the reviewers. Any product that may be evaluated in this article, or claim that may be made by its manufacturer, is not guaranteed or endorsed by the publisher.
References
Abdrabou, M. R., Gomah, H., Darweesh, A., Eissa, M., Selmy, S. (2022). Response of saline irrigated quinoa (Chenopodium quinoa wild) grown on coarse texture soils to organic manure. Egypt. J. Soil Sci. 62 (2), 169–178. doi: 10.21608/ejss.2022.146571.1511
Adolf, V. I., Jacobsen, S. E., Shabala, S. (2013). Salt tolerance mechanisms in quinoa (Chenopodium quinoa willd.). Environ. Exp. Bot. 92, 43–54. doi: 10.1016/j.envexpbot.2012.07.004
Alandia, G., Rodriguez, J. P., Jacobsen, S.-E., Bazile, D., Condori, B. (2020). Global expansion of quinoa and challenges for the Andean region. Glob Food Sec 26, 100429. doi: 10.1016/j.gfs.2020.100429
Alcívar, M., Zurita-Silva, A., Sandoval, M., Muñoz, C., Schoebitz, M. (2018). Reclamation of saline-sodic soils with combined amendments: impact on quinoa performance and biological soil quality. Sustainability 10 (9), 3083. doi: 10.3390/su10093083
Ando, H., Chen, Y., Tang, H., Shimizu, M., Watanabe, K., Mitsunaga, T. (2002). Food components in fractions of quinoa seed. Food Sci. Technol. Res. 8 (1), 80–84. doi: 10.3136/fstr.8.80
Arora, S., Dagar, J. C. (2019). “Salinity tolerance indicators,” in Research developments in saline agriculture. Eds. Dagar, J. C., Yadav, R. K., Sharma, P. C. (Singapore: Springer Singapore), 155–201. doi: 10.1007/978-981-13-5832-6_5
Bates, L. S., Waldren, R. P., Teare, I. D. (1973). Rapid determination of free proline for water-stress studies. Plant Soil 39, 205–207. doi: 10.1007/BF00018060
Bazile, D., Jacobsen, S.-E., Verniau, A. (2016). The global expansion of quinoa: trends and limits. Front. Plant Sci. 7. doi: 10.3389/fpls.2016.00622
Bistgani, Z. E., Hashemi, M., DaCosta, M., Craker, L., Maggi, F., Morshedloo, M. R. (2019). Effect of salinity stress on the physiological characteristics, phenolic compounds and antioxidant activity of thymus vulgaris l. and thymus daenensis celak. Ind. Crops Prod 135, 311–320. doi: 10.1016/j.indcrop.2019.04.055
Bouras, H., Choukr-Allah, R., Amouaouch, Y., Bouaziz, A., Devkota, K. P., El Mouttaqi, A., et al. (2022). How does quinoa (Chenopodium quinoa willd.) respond to phosphorus fertilization and irrigation water salinity? Plants 11 (2), 216. doi: 10.3390/plants11020216
Brakez, M., El Brik, K., Daoud, S., Harrouni, M. C. (2013). “Performance of chenopodium quinoa under salt stress,” in Developments in soil salinity assessment and reclamation: innovative thinking and use of marginal soil and water resources in irrigated agriculture. Eds. Shahid, S., Abdelfattah, M., Taha, F. (Dordrecht: Springer), 463–478. doi: 10.1007/978-94-007-5684-7_32
Chehab, H., Tekaya, M., Hajlaoui, H., Abdelhamid, S., Gouiaa, M., Sfina, H., et al. (2020). Complementary irrigation with saline water and soil organic amendments modified soil salinity, leaf na+, productivity and oil phenols of olive trees (cv. chemlali) grown under semiarid conditions. Agric. Water Manag 237, 106183. doi: 10.1016/j.agwat.2020.106183
Cheok, C. Y., Salman, H. A. K., Sulaiman, R. (2014). Extraction and quantification of saponins: a review. Food Res. Int. 59, 16–40. doi: 10.1016/j.foodres.2014.01.057
Diacono, M., Montemurro, F. (2011). “Long-term effects of organic amendments on soil fertility,” in Sustainable agriculture volume 2. Eds. Lichtfouse, E., Hamelin, M., Navarrete, M., Debaeke, P. (Dordrecht: Springer Netherlands), 761–786. doi: 10.1007/978-94-007-0394-0_34
Diacono, M., Montemurro, F. (2015). Effectiveness of organic wastes as fertilizers and amendments in salt-affected soils. Agriculture 5 (2), 221–230. doi: 10.3390/agriculture5020221
Ding, Z., Kheir, A. M. S., Ali, M. G. M., Ali, O. A. M., Abdelaal, A. I. N., Lin, X., et al. (2020). The integrated effect of salinity, organic amendments, phosphorus fertilizers, and deficit irrigation on soil properties, phosphorus fractionation and wheat productivity. Sci. Rep. 10, 2736. doi: 10.1038/s41598-020-59650-8
Dini, I., Tenore, G. C., Dini, A. (2010). Antioxidant compound contents and antioxidant activity before and after cooking in sweet and bitter chenopodium quinoa seeds. LWT - Food Sci. Technol. 43, 447–451. doi: 10.1016/j.lwt.2009.09.010
Dorado, J., Zancada, M.-C., Almendros, G., López-Fando, C. (2003). Changes in soil properties and humic substances after long-term amendments with manure and crop residues in dryland farming systems. J. Plant Nutr. Soil Sci. 166, 31–38. doi: 10.1002/jpln.200390009
El Aziz, M. M. A., Ashour, A. S., Melad, A. G. (2019). A review on saponins from medicinal plants: chemistry, isolation, and determination. J. Nanomed. Res. 8, 282–288. doi: 10.15406/jnmr.2019.07.00199
El Hazzam, K., Hafsa, J., Sobeh, M., Mhada, M., Taourirte, M., Kacimi, K. E. L., et al. (2020). An insight into saponins from quinoa (Chenopodium quinoa willd): a review. Molecules 25 (5), 1059. doi: 10.3390/molecules25051059
El Moukhtari, A., Cabassa-Hourton, C., Farissi, M., Savouré, A. (2020). How does proline treatment promote salt stress tolerance during crop plant development? Front. Plant Sci. 11. doi: 10.3389/fpls.2020.01127
FAO (2021) Global map of salt affected soils. Available at: https://www.fao.org/soils-portal/data-hub/soil-maps-and-databases/global-map-of-salt-affected-soils/en/ (Accessed November 15, 2022).
Fghire, R., Wahbi, S., Anaya, F., Issa Ali, O., Benlhabib, O., Ragab, R. (2015). Response of quinoa to different water management strategies: field experiments and saltmed model application results. Irrigation Drainage 64 (1), 29–40. doi: 10.1002/ird.1895
Fischer, S., Wilckens, R., Jara, J., Aranda, M. (2013). Variation in antioxidant capacity of quinoa (Chenopodium quinoa will) subjected to drought stress. Ind. Crops Prod 46, 341–349. doi: 10.1016/j.indcrop.2013.01.037
Flowers, T. J., Colmer, T. D. (2008). Salinity tolerance in halophytes. New Phytol. 179 (4), 945–963. doi: 10.1111/j.1469-8137.2008.02531.x
Gill, S., Alshankiti, A., Shahid, S. A., Calle, J. P. R. (2020). “Amending soil health to improve productivity of alternate crops in marginal sandy soils of the UAE,” in Emerging research in alternative crops. Eds. Hirich, A., Choukr-Allah, R., Ragab, R. (Environment & Policy 58. Cham, Switzerland: Springer). doi: 10.1007/978-3-319-90472-6_4
Gómez-Caravaca, A. M., Segura-Carretero, A., Fernández-Gutiérrez, A., Caboni, M. F. (2011). Simultaneous determination of phenolic compounds and saponins in quinoa (Chenopodium quinoa willd) by a liquid chromatography-diode array detection-electrospray ionization-time-of-flight mass spectrometry methodology. J. Agric. Food Chem. 59, 10815–10825. doi: 10.1021/jf202224j
Gómez-Caravaca, A. M., Iafelice, G., Verardo, V., Marconi, E., Caboni, M. F. (2014). Influence of pearling process on phenolic and saponin content in quinoa (Chenopodium quinoa Willd). Food Chem 157, 174–178. doi: 10.1016/j.foodchem.2014.02.023
Goss, M. J., Tubeileh, A., Goorahoo, D. (2013). “Chapter five - a review of the use of organic amendments and the risk to human health,” in Advances in agronomy. Ed. Sparks, D. L. (Cambridge, MA: Academic Press), 275–379. doi: 10.1016/B978-0-12-407686-0.00005-1
Han, Y., Chi, J., Zhang, M., Zhang, R., Fan, S., Huang, F., et al. (2019). Characterization of saponins and phenolic compounds: antioxidant activity and inhibitory effects on α-glucosidase in different varieties of colored quinoa (Chenopodium quinoa willd). Biosci. Biotechnol. Biochem. 83 (11), 2128–2139. doi: 10.1080/09168451.2019.1638756
Hare, P. D., Cress, W. A. (1997). Metabolic implications of stress-induced proline accumulation in plants. Plant Growth Regul. 21, 79–102. doi: 10.1023/A:1005703923347
Hirich, A., Choukr-Allah, R., Jacobsen, S.-E. (2014a). The combined effect of deficit irrigation by treated wastewater and organic amendment on quinoa (Chenopodium quinoa willd.) productivity. Desalination Water Treat 52, 2208–2213. doi: 10.1080/19443994.2013.777944
Hirich, A., Choukr-Allah, R., Jacobsen, S.-E. (2014b). Deficit irrigation and organic compost improve growth and yield of quinoa and pea. J. Agron. Crop Sci. 200 (5), 390–398. doi: 10.1111/jac.12073
Hirich, A., Choukr-Allah, R., Jacobsen, S.-E. (2014d). Quinoa in Morocco - effect of sowing dates on development and yield. J. Agron. Crop Sci. 200, 371–377. doi: 10.1111/jac.12071
Hirich, A., Jelloul, A., Choukr-Allah, R., Jacobsen, S.-E. (2014c). Saline water irrigation of quinoa and chickpea: seedling rate, stomatal conductance and yield responses. J. Agron. Crop Sci. 200, 378–389. doi: 10.1111/jac.12072
Hirich, A., Rafik, S., Rahmani, M., Fetouab, A., Azaykou, F., Filali, K., et al. (2021). Development of quinoa value chain to improve food and nutritional security in rural communities in rehamna, Morocco: lessons learned and perspectives. Plants 10 (2), 301. doi: 10.3390/plants10020301
Hmidi, D., Abdelly, C., Athar, H. U. R., Ashraf, M., Messedi, D. (2018). Effect of salinity on osmotic adjustment, proline accumulation and possible role of ornithine-δ-aminotransferase in proline biosynthesis in cakile maritima. Physiol. Mol. Biol. Plants 24, 1017–1033. doi: 10.1007/s12298-018-0601-9
Hussain, M. I., Muscolo, A., Ahmed, M., Asghar, M. A., Al-Dakheel, A. J. (2020). Agro-morphological, yield and quality traits and interrelationship with yield stability in quinoa (Chenopodium quinoa willd.) genotypes under saline marginal environment. Plants 9 (12), 1763. doi: 10.3390/plants9121763
ISO (1994) ISO 9963-1:1994 water quality — determination of alkalinity — part 1: determination of total and composite alkalinity. Available at: https://www.iso.org/standard/17868.html (Accessed December 3, 2022).
Jacobsen, S.-E., Mujica, A., Jensen, C. R. (2003). The resistance of quinoa (Chenopodium quinoa willd.) to adverse abiotic factors. Food Rev. Int. 19, 99–109. doi: 10.1081/FRI-120018872
Jellen, E. N., Benlhabib, O., Maughan, P. J., Stevens, M. R., Dilg, M., Sederberg, M., et al. (2006). Introduction of the Andean crop quinoa in Morocco (Salt Lake City, UT, USA: The ASA-CSSA-SSSA International Annual Meetings. ASA-CSSA-SSSA).
Jia, X., Dong, L., Wen, Y., Huang, F., Chi, J., Zhang, R. (2022). Discovery of possible hepatoprotective components from lychee pulp phenolic extract by online knockout methods. Food Biosci. 50, 102053. doi: 10.1016/j.fbio.2022.102053
Koyro, H. W., Eisa, S. S. (2008). Effect of salinity on composition, viability and germination of seeds of chenopodium quinoa willd. Plant Soil 302, 79–90. doi: 10.1007/s11104-007-9457-4
Kumari, R., Bhatnagar, S., Mehla, N., Vashistha, A. (2022). Potential of organic amendments (AM fungi, PGPR, vermicompost and seaweeds) in combating salt stress – a review. Plant Stress 6, 100111. doi: 10.1016/j.stress.2022.100111
Leogrande, R., Vitti, C. (2019). Use of organic amendments to reclaim saline and sodic soils: a review. Arid Land Res. Manage. 33, 1–21. doi: 10.1080/15324982.2018.1498038
Liao, P., Liu, D., Xu, T. R., Yang, Y., Cui, X. (2017). Soil water stress attenuate the growth and development but enhance the saponin synthesis of panax notogesing during flowering stage. Ind. Crops Prod 108, 95–105. doi: 10.1016/j.indcrop.2017.05.052
Lim, J. G., Park, H. M., Yoon, K. S. (2020). Analysis of saponin composition and comparison of the antioxidant activity of various parts of the quinoa plant (Chenopodium quinoa willd.). Food Sci. Nutr. 8, 694–702. doi: 10.1002/fsn3.1358
Liu, D., Ding, Z., Ali, E. F., Kheir, A. M. S., Eissa, M. A., Ibrahim, O. H. M. (2021). Biochar and compost enhance soil quality and growth of roselle (Hibiscus sabdariffa l.) under saline conditions. Sci. Rep. 11, 8739. doi: 10.1038/s41598-021-88293-6
Long, N. V. (2016). Effects of salinity stress on growth and yield of quinoa (Chenopodium quinoa willd.) at flower initiation stages. Vietnam J. Agric. Sci. 14, 321–327.
Machado, R. M. A., Serralheiro, R. P. (2017). Soil salinity: effect on vegetable crop growth. management practices to prevent and mitigate soil salinization. Horticulturae 3 (2), 30. doi: 10.3390/horticulturae3020030
Mhada, M., Jellen, E. N., Jacobsen, S. E., Benlhabib, O. (2014). Diversity Analysis of a Quinoa (Chenopodium quinoa Willd.) Germplasm during Two Seasons. World Academy of Science, Engineering and Technology, International Journal of Biological, Biomolecular, Agricultural, Food and Biotechnological Engineering 8, 273–276.
Mhada, M., Metougui, M. L., El Hazzam, K., El Kacimi, K., Yasri, A. (2020). Variations of saponins, minerals and total phenolic compounds due to processing and cooking of quinoa (Chenopodium quinoaWilld.) seeds. Foods 9. doi: 10.3390/foods9050660
Miranda, M., Vega-Gálvez, A., Martinez, E., López, J., Rodríguez, M. J., Henríquez, K., et al (2012). Genetic diversity and comparison of physicochemical and nutritional characteristics of six quinoa (Chenopodium quinoa willd.) genotypes cultivated in Chile. Food Sci Tech 32. doi: 10.1590/S0101-20612012005000114
Misra, N., Gupta, A. K. (2005). Effect of salt stress on proline metabolism in two high yielding genotypes of green gram. Plant Sci. 169, 331–339. doi: 10.1016/j.plantsci.2005.02.013
Navarro del Hierro, J., Herrera, T., García-Risco, M. R., Fornari, T., Reglero, G., Martin, D. (2018). Ultrasound-assisted extraction and bioaccessibility of saponins from edible seeds: quinoa, lentil, fenugreek, soybean and lupin. Food Res. Int. 109, 440–447. doi: 10.1016/j.foodres.2018.04.058
Oumasst, A., Azougay, S., Mimouni, A., Hallam, J. (2022). The effect of different irrigation water salinity levels on nutrients uptake, biochemical content and growth response of blue panicum, quinoa and silage maize. Environ. Sci. Proc. 16. doi: 10.3390/environsciproc2022016060
Paludo, M. C., de Oliveira, S. B. P., de Oliveira, L. F., Colombo, R. C., Gómez-Alonso, S., Hermosín-Gutiérrez, I., et al. (2022). Phenolic composition of peels from different jaboticaba species determined by HPLC-DAD-ESI/MSn and antiproliferative activity in tumor cell lines. Curr. Plant Biol. 29, 100233. doi: 10.1016/j.cpb.2021.100233
Patel, K., Agarwal, P., Agarwal, P. K. (2018). Kappaphycus alvarezii sap mitigates abiotic-induced stress in triticum durum by modulating metabolic coordination and improves growth and yield. J. Appl. Phycology 30, 2659–2673. doi: 10.1007/s10811-018-1423-4
Pequerul, A., Pérez, C., Madero, P., Val, J., Monge, E. (1993). “A rapid wet digestion method for plant analysis,” in Optimization of plant nutrition: refereed papers from the eighth international colloquium for the optimization of plant nutrition, 31 august – 8 September 1992, Lisbon, Portugal. Eds. Fragoso, M. A. C., Beusichem, M.L.V., Houwers, A. (Netherlands: Dordrecht: Springer), 3–6. doi: 10.1007/978-94-017-2496-8_1
Pereira, E., Cadavez, V., Barros, L., Encina-Zelada, C., Stojković, D., Sokovic, M., et al. (2020). Chenopodium quinoa willd. (quinoa) grains: a good source of phenolic compounds. Food Res. Int. 137, 109574. doi: 10.1016/j.foodres.2020.109574
Pérez-Patricio, M., Camas-Anzueto, J. L., Sanchez-Alegría, A., Aguilar-González, A., Gutiérrez-Miceli, F., Escobar-Gómez, E., et al. (2018). Optical method for estimating the chlorophyll contents in plant leaves. Sensors 18 (2), 650. doi: 10.3390/s18020650
Petridis, A., Therios, I., Samouris, G., Tananaki, C. (2012). Salinity-induced changes in phenolic compounds in leaves and roots of four olive cultivars (Olea europaea l.) and their relationship to antioxidant activity. Environ. Exp. Bot. 79, 37–43. doi: 10.1016/j.envexpbot.2012.01.007
Pingle, S. N., Suryawanshi, S. T., Pawar, K. R., Harke, S. N. (2022). The effect of salt stress on proline content in maize (Zea mays). Environ. Sci. Proc. 16 (1), 64. doi: 10.3390/environsciproc2022016064
Puente-Garza, C. A., Meza-Miranda, C., Ochoa-Martínez, D., García-Lara, S. (2017). Effect of in vitro drought stress on phenolic acids, flavonols, saponins, and antioxidant activity in agave salmiana. Plant Physiol. Biochem. 115, 400–407. doi: 10.1016/j.plaphy.2017.04.012
Rafik, S., Chaoui, M., Assabban, Y., Jazi, S., Choukr-Allah, R., El Gharouss, M., et al. (2021a). Quinoa value chain, adoption, and market assessment in Morocco. Environ. Sci. pollut. Res. 28, 46692–46703. doi: 10.1007/s11356-020-11375-x
Rafik, S., Rahmani, M., Choukr-Allah, R., El Gharous, M., Calle, J. P. R., Filali, K., et al. (2021b). Techno-economic assessment of quinoa production and transformation in Morocco. Environ. Sci. pollut. Res. 28, 46781–46796. doi: 10.1007/s11356-021-12665-8
Rafik, S., Rahmani, M., Rodriguez, J. P., Andam, S., Ezzariai, A., El Gharous, M., et al. (2021c). How does mechanical pearling affect quinoa nutrients and saponin contents? Plants 10 (6), 1133. doi: 10.3390/plants10061133
Rezzouk, F. Z., Shahid, M. A., Elouafi, I. A., Zhou, B., Araus, J. L., Serret, M. D. (2020). Agronomic performance of irrigated quinoa in desert areas: comparing different approaches for early assessment of salinity stress. Agric. Water Manag 240, 106205. doi: 10.1016/j.agwat.2020.106205
Rusli, L. S., Abdullah, R., Yaacob, J. S., Osman, N. (2022). Organic amendments effects on nutrient uptake, secondary metabolites, and antioxidant properties of melastoma malabathricum l. Plants 11 (2), 153. doi: 10.3390/plants11020153
Sabraoui, T., Khider, T., Nasser, B., Eddoha, R., Moujahid, A., Benbachir, M., et al. (2020). Determination of punicalagins content, metal chelating, and antioxidant properties of edible pomegranate (Punica granatum l) peels and seeds grown in Morocco. Int. J. Food Sci. 2020, 4–11. doi: 10.1155/2020/8885889
Schmidt, D., Verruma-Bernardi, M. R., Forti, V. A., Borges, M. T. M. R. (2021). Quinoa and amaranth as functional foods: a review. Food Rev. Int. doi: 10.1080/87559129.2021.1950175
Shahid, M., Thushar, S. (2021). “Response of quinoa to high salinity under arid conditions,” in Future of sustainable agriculture in saline environments. Eds. Negacz, K., Vellinga, P., Barrett-Lennard, E., Choukr-Allah, R., Elzenga, T. (Boca Raton, FL: CRC Press), 427–441. doi: 10.1201/9781003112327-29
Shahid, S. A., Zaman, M., Heng, L. (2018). “Soil salinity: historical perspectives and a world overview of the problem,” in Guideline for salinity assessment, mitigation and adaptation using nuclear and related techniques. Eds. Shahid, S. A., Zaman, M., Heng, L. (Springer, Cham), 43–53. doi: 10.1007/978-3-319-96190-3_2
Starzyńska-Janiszewska, A., Duliński, R., Stodolak, B., Mickowska, B., Wikiera, A. (2016). Prolonged tempe-type fermentation in order to improve bioactive potential and nutritional parameters of quinoa seeds. J. Cereal Sci. 71, 116–121. doi: 10.1016/j.jcs.2016.08.001
Stavi, I., Thevs, N., Priori, S. (2021). Soil salinity and sodicity in drylands: a review of causes, effects, monitoring, and restoration measures. Front. Environ. Sci. 9. doi: 10.3389/fenvs.2021.712831
Suárez-Estrella, D., Torri, L., Pagani, M. A., Marti, A. (2018). Quinoa bitterness: causes and solutions for improving product acceptability. J. Sci. Food Agric. 98, 4033–4041. doi: 10.1002/jsfa.8980
Szakiel, A., Pączkowski, C., Henry, M. (2011). Influence of environmental abiotic factors on the content of saponins in plants. Phytochem. Rev. 10, 471–491. doi: 10.1007/s11101-010-9177-x
Szepesi, Á., Szollosi, R. (2018). “Mechanism of proline biosynthesis and role of proline metabolism enzymes under environmental stress in plants,” in Plant metabolites and regulation under environmental stress. Eds. Ahmad, P., Ahanger, M. A., Singh, V. P., Tripathi, D. K., Alam, P., Alyemeni, M. N. (Cambridge, MA: Academic Press), 337–353. doi: 10.1016/B978-0-12-812689-9.00017-0
Talaat, N. B., Shawky, B. T. (2014). Protective effects of arbuscular mycorrhizal fungi on wheat (Triticum aestivum l.) plants exposed to salinity. Environ. Exp. Bot. 98, 20–31. doi: 10.1016/j.envexpbot.2013.10.005
Talebnejad, R., Sepaskhah, A. R. (2015). Effect of different saline groundwater depths and irrigation water salinities on yield and water use of quinoa in lysimeter. Agric. Water Manag 148, 177–188. doi: 10.1016/j.agwat.2014.10.005
Tang, Y., Li, X., Zhang, B., Chen, P. X., Liu, R., Tsao, R. (2015). Characterisation of phenolics, betanins and antioxidant activities in seeds of three chenopodium quinoa willd. genotypes. Food Chem. 166, 380–388. doi: 10.1016/j.foodchem.2014.06.018
Tatipamula, V. B., Kukavica, B. (2021). Phenolic compounds as antidiabetic, anti-inflammatory, and anticancer agents and improvement of their bioavailability by liposomes. Cell Biochem. Funct. 39, 926–944. doi: 10.1002/cbf.3667
Tiozon, R. J. N., Sartagoda, K. J. D., Serrano, L. M. N., Fernie, A. R., Sreenivasulu, N. (2022). Metabolomics based inferences to unravel phenolic compound diversity in cereals and its implications for human gut health. Trends Food Sci. Technol. 127, 14–25. doi: 10.1016/j.tifs.2022.06.011
Volkov, V., Beilby, M. J. (2017). Editorial: salinity tolerance in plants: mechanisms and regulation of ion transport. Front. Plant Sci. 8. doi: 10.3389/fpls.2017.01795
Wichern, F., Islam, M. R., Hemkemeyer, M., Watson, C., Joergensen, R. G. (2020). Organic amendments alleviate salinity effects on soil microorganisms and mineralisation processes in aerobic and anaerobic paddy rice soils. Front. Sustain Food Syst. 4. doi: 10.3389/fsufs.2020.00030
Yang, A., Akhtar, S. S., Li, L., Fu, Q., Li, Q., Naeem, M. A., et al. (2020). Biochar mitigates combined effects of drought and salinity stress in quinoa. Agronomy 10 (6), 912. doi: 10.3390/agronomy10060912
Yang, P., Huang, K., Zhang, Y., Li, S., Cao, H., Song, H., et al. (2022). Biotransformation of quinoa phenolic compounds with monascus anka to enhance the antioxidant capacity and digestive enzyme inhibitory activity. Food Bioscience 46, 101568. doi: 10.1016/j.fbio.2022.101568
Yawadio Nsimba, R., Kikuzaki, H., Konishi, Y. (2008). Antioxidant activity of various extracts and fractions of chenopodium quinoa and amaranthus spp. seeds. Food Chem. 106, 760–766. doi: 10.1016/j.foodchem.2007.06.004
Keywords: quinoa, osmotic stress, organic amendment, growth, seed yield, saponin
Citation: El Mouttaqi A, Sabraoui T, Belcaid M, Ibourki M, Mnaouer I, Lazaar K, Sehbaoui F, Ait Elhaj R, Khaldi M, Rafik S, Zim J, Nilahyane A, Ghoulam C, Devkota KP, Kouisni L and Hirich A (2023) Agro-morphological and biochemical responses of quinoa (Chenopodium quinoa Willd. var: ICBA-Q5) to organic amendments under various salinity conditions. Front. Plant Sci. 14:1143170. doi: 10.3389/fpls.2023.1143170
Received: 12 January 2023; Accepted: 10 April 2023;
Published: 08 May 2023.
Edited by:
Maurizio Ruzzi, University of Tuscia, ItalyReviewed by:
Zina Flagella, University of Foggia, ItalyLahcen Hssaini, Institut National de la Recherche Agronomique, Morocco
Copyright © 2023 El Mouttaqi, Sabraoui, Belcaid, Ibourki, Mnaouer, Lazaar, Sehbaoui, Ait Elhaj, Khaldi, Rafik, Zim, Nilahyane, Ghoulam, Devkota, Kouisni and Hirich. This is an open-access article distributed under the terms of the Creative Commons Attribution License (CC BY). The use, distribution or reproduction in other forums is permitted, provided the original author(s) and the copyright owner(s) are credited and that the original publication in this journal is cited, in accordance with accepted academic practice. No use, distribution or reproduction is permitted which does not comply with these terms.
*Correspondence: Abdelaziz Hirich, YWJkZWxheml6LmhpcmljaEB1bTZwLm1h
†These authors have contributed equally to this work and share first authorship