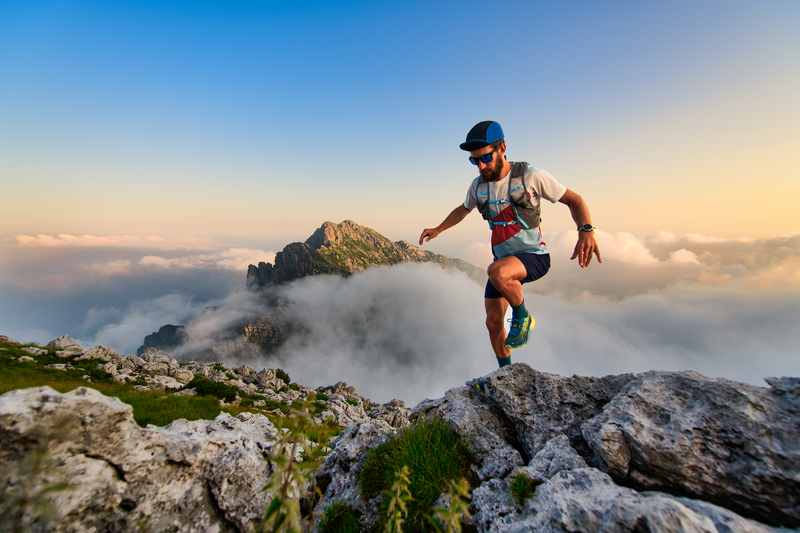
95% of researchers rate our articles as excellent or good
Learn more about the work of our research integrity team to safeguard the quality of each article we publish.
Find out more
REVIEW article
Front. Plant Sci. , 17 March 2023
Sec. Plant Abiotic Stress
Volume 14 - 2023 | https://doi.org/10.3389/fpls.2023.1137923
This article is part of the Research Topic A Large-Scale Biology View of Crop-Environment Interaction: The Influence of Water and Temperature Stresses on the Development of Cereal and Horticultural Crops View all 9 articles
High-temperature stress affects crop yields worldwide. Identifying thermotolerant crop varieties and understanding the basis for this thermotolerance would have important implications for agriculture, especially in the face of climate change. Rice (Oryza sativa) varieties have evolved protective strategies to acclimate to high temperature, with different thermotolerance levels. In this review, we examine the morphological and molecular effects of heat on rice in different growth stages and plant organs, including roots, stems, leaves and flowers. We also explore the molecular and morphological differences among thermotolerant rice lines. In addition, some strategies are proposed to screen new rice varieties for thermotolerance, which will contribute to the improvement of rice for agricultural production in the future.
High-temperature stress is an important environmental factor affecting crop growth and yield (Yang et al., 2017). In 2017, four independent studies estimated that for every 1°C increase in the average global temperature, rice (Oryza sativa) yields would decrease by 3.2%, maize (Zea mays) yields by 7.4%, wheat (Triticum aestivum) yields by 6%, and soybean (Glycine max) yields by 3.1% (Zhao et al., 2017). Therefore, mitigating the decreases in yield caused by high temperatures by developing and cultivating new thermotolerant crop varieties has emerged as an important strategy for sustainable agriculture. Rice represents the main food source for nearly half of the world’s population, accounting for 21% of total global caloric intake. The cultivation area of rice accounts for 11% of the total cultivated land area worldwide. It is estimated that global rice consumption will increase from 480 million tons in 2014 to nearly 550 million tons in 2030 (Yuan et al., 2021). Rice yield is estimated to decrease by up to 10% with every 1°C increase in land surface temperature (Peng et al., 2004). Given the frequency of extreme heat events, it is important to identify existing thermotolerant rice varieties and develop new ones.
In this review, we explore the effects of high temperature on rice at different stages of growth. We then explore the effects of high-temperature stress on cellular processes and phytohormones in rice. Finally, we examine the mechanisms by which thermotolerant rice varieties acclimate to high temperature. Understanding these effects and mechanisms will provide a foundation for producing new thermotolerant rice varieties, which will be essential for the future of agriculture.
The life cycle of rice is divided into vegetative (germination, seedling growth, and tillering) and reproductive (booting, heading, flowering, and maturation/grain filling) stages; high temperatures have different effects on plants at different stages of development (Zhang C et al., 2018). Rice plants are particularly sensitive to temperature stress during reproductive growth and maturation, when exposure to high-temperature stress can significantly affect development and yield (Lawas et al., 2019; Chen et al., 2020). The two subspecies, Japonica and Indica, have different domestication origins. Japonica and Indica originated in temperate regions and tropical regions, respectively. Indica is more thermotolerant than Japonica and has different morphological and physiological characteristics (Lee et al., 2002; Lee et al., 2017). So, these geographic origins should be taken into account, when different kinds of species were used for high temperature treatment. Therefore, during the domestication of modern rice varieties, the two subspecies were used as backgrounds of their respective varieties. Figure 1 shows the impact of high temperature on different developmental stages of rice.
Figure 1 High temperature has different effects on rice at different stages of the plant life cycle: reduced germination rate, loss of water, loss of membrane integrity, inhibited photosynthesis, ROS accumulation, phytohormone imbalance, altered carbohydrate, altered lipid metabolism, dead seedlings, and reduced tiller number at the vegetative stage; deformed floral organs, reduced spikelet fertility, reduced spikelet number, changed flower opening time, reduced spikelet fertility, reduced spikelet number, and altered flower opening time at the reproductive stage; reduce starch biosynthesis, lower starch accumulation, boost senescence of functional leaves, reduced grain filling and increased chalkiness rate at the maturity stage.
Vegetative growth includes seed germination, early seedling growth, and tillering. Long-term exposure to high temperatures affects the germination rate of rice seeds (Liu et al., 2015). In seedlings, high temperature can destroy cell membranes, inhibit photosynthesis, and increase oxidative damage, leading to increased water loss, wilting, damage to root growth, and even plant death (Bahuguna et al., 2015; Liu et al., 2018). To mitigate these effects, rooting agents such as iron chelators, calcium, silicone, and gibberellins can be added during sowing to improve the seed germination rate, promote root growth, and reduce the effects of high temperatures on the resulting plants (Tiwari et al., 2022).
Tillering determines the structure and yield of rice plants and is thus an important agronomic trait of rice. Tillers develop from the leaf axillary bud on the mother stem and grow at an angle centered on the main stem (Wang et al., 2018; Luo et al., 2021). Tiller number and angle affect yield: tillers growing at a large angle reduce harvest efficiency, planting density, and yield, whereas tillers growing at a small angle increase disease risk (Dias de Oliveira et al., 2015; Hu et al., 2020). High temperature can decrease tillering and thus panicle number in rice. For example, Soda reported that panicle number and yield per plant decreased by 35% and 28%, respectively, in rice plants subjected to high-temperature stress (Soda et al., 2018).
Studies aimed at mitigating these effects have focused on the regulation of tillering. For example, heterologous overexpression of TaGAMYB1 (encoding a GAMYB-like family transcription factor) or overexpression of FIBRILLIN 1 (OsFBN1) or OsSTVB-I (a rice stripe virus resistance gene) improved thermotolerance and increased tiller number in rice (Li et al., 2019; Hayano-Saito and Hayashi, 2020; Wang et al., 2012). In addition, the tiller angle between the main stem of rice and its side tillers also directly affects the photosynthetic rate, and thus affects the rice yield per unit area (Li et al., 2020a). Recent studies have shown that the expression of HEAT SHOCK FACTOR A2d (HSFA2d) increases rapidly only 15 min after HS, and the formation of rice tiller angle can be regulated by HSFA2D-LA1 (LAZY1) pathway in rice (Zhang N et al., 2018; Hu et al., 2020; Prerostova et al., 2021). Therefore, the phenotypic characteristics of tillers can also be used as one of the screening criteria for thermoresistant varieties.
The reproductive stage includes booting, heading, and flowering. We will discuss maturation, which includes grain filling, in Section 2.3. The reproductive period, especially heading and flowering, is very sensitive to high-temperature stress (Matsui et al., 2015). Rice belongs to the Poaceae family. The spikelet, representing the basic structure of the Poaceae inflorescence, is composed of stamens, pistils, glumes, and lemmas (Ren et al., 2020). High temperature during the reproductive period primarily affects the fertility and number of spikelets, resulting in a serious decline in rice yield. As early as 1978, Satake and Yoshida reported that exposing rice to temperatures above 35°C for about five days during the reproductive period resulted in spikelet sterility and failure to produce seeds (Satake and Yoshida, 1978). The effect of high temperature on the reproductive period was greater before than after flowering. Exposing pre-flowering spikelets to 33.7°C for one hour was sufficient to cause sterility, but exposing post-flowering spikelets to 38°C or 41°C for one hour did not cause sterility (Jagadish et al., 2007; Ishimaru et al., 2010; Shi et al., 2018).
Stamens are particularly sensitive to high temperature. Indeed, Miura et al. reported that pistils could be successfully fertilized by hand pollination even after five-day exposure to 41°C (Miura et al., 2011). High temperature has several negative effects on rice stamens. Firstly, high temperature interferes with meiosis during pollen development, resulting in the formation of sterile pollen (Endo et al., 2009). Secondly, high temperature inhibits pollen dehiscence and decreases the swelling ability of pollen grains, thus reducing the amount of pollen falling on a stigma and affecting the fertilization rate (Arshad et al., 2017; Hu et al., 2021). Thirdly, the moisture content of pollen grains is crucial to both the formation and diffusion of pollen grains. When pollen falls onto a stigma, the moisture content changes according to environmental conditions; high temperature plays a role in disrupting this process (Das et al., 2014; Shrestha et al., 2022). Finally, high temperature strongly reduces the amount of protein present in pollen, thereby reducing the germination ability of pollen and the elongation rate of the pollen tube, leading to spikelet sterility (Arshad et al., 2017; Jagadish, 2020; Shrestha et al., 2022).
Some thermotolerant rice varieties rapidly release pollen early during flowering, but many thermosensitive varieties fail to release pollen in time to effect fertilization. For example, in the thermotolerant rice variety Nagina 22 (N22), pollen dehiscence occurs rapidly upon opening of the glumes, and the released pollen settles on the stigma (Kobayashi et al., 2015). The anther cavity of thermotolerant Japonica rice varieties (Akitakomachi, Nipponbare) are well developed, and the septum breaks rapidly when the pollen expands, resulting in high spikelet fertility (Matsui and Omasa, 2002). High temperature also causes decreases in pollen protein content and viability. Indeed, a typical characteristic of a thermotolerant variety is its strong pollen viability (Zhao et al., 2018). Pollen tubes are also extremely sensitive to high temperature, which affects grain yield. Therefore, studying the morphology and physiology of floral organs from different thermosensitive and thermotolerant varieties may help breeders screen varieties for thermotolerance (Matsui and Omasa, 2002).
Approaches to mitigating the effect of high temperature on reproductive development have focused on identifying the underlying genetic factors. For example, Ye et al. identified a QTL (qHTSF4.1) on chromosome 4 in 24 rice varieties. Compared with rice varieties without qHTSF4.1, most varieties with this QTL showed increased spikelet fertility under high-temperature stress during the reproductive period, thus improving yield (Ye et al., 2015). Therefore, in addition to detecting pollen viability, changes in the expression levels of related target genes can also be detected after high-temperature stress to evaluate the changes in pollen metabolism and phytohormone levels, representing another effective method for identifying thermotolerant rice varieties.
In addition, rice varieties have different flowering duration and significantly different flower opening time (FOT), ranging from early morning to midnight. High temperatures shorten the flowering time, limiting the time for pollination (Jagadish et al., 2007; Djanaguiraman et al., 2020). Therefore, changing the FOT can help protect rice from high-temperature stress, thereby reducing damage during flowering. This early-morning flowering (EMF) strategy has been effectively applied in rice. This strategy is to breed cultivars that escape high temperature at flowering because of their EMF trait has been effectively applied in rice.
Under simulated continuous warming, Nanjing 11 began flowering after 7 am, with one flowering peak between 9 and 10 am and one between 11 and 12 am. However, qEMF3 advanced the flowering peak of Nanjing 11 to 7-8 am, thereby improving thermotolerance (Hirabayashi et al., 2015; Jagadish, 2020). In addition, when post-flowering stage is exposed to high night temperatures, respiration is accelerated, resulting in higher carbon loss, and affecting starch accumulation, thus reducing rice yield (Bahuguna et al., 2017; Impa et al., 2021). In conclusion, early flowering can protect spike heads and preserve fertility when plants experience high temperatures. We can systematically evaluate the start and peak of the FOT, select suitable varieties, or modify the FOT to protect crops from high temperatures during propagation and thus improve yields.
Grain filling occurs during the maturity stage and involves the conversion of sucrose produced by photosynthesis into starch, which is the major carbohydrate in rice and an important determinant of grain quality and yield (Tang et al., 2018). The starch in rice grains is mainly derived from sugars produced in the grains after anthesis and from sugars that are redistributed from other vegetative tissues, such as stems and leaf sheaths. For reactivating assimilates stored in vegetative tissues and transferring them to grains, whole-plant senescence should be initiated (Yang and Zhang, 2006). Delaying the senescence results in insufficient grain filling, with large amounts of carbohydrates remaining unused in the straw (Plaut et al., 2004).
High temperature during grain filling can reduce rice yields by 50% (Sreenivasulu et al., 2015). It can also cause the failure of grain filling in rice and wheat by affecting the accumulation of starch granules, ultimately resulting in yield losses (Chen et al., 2017; Impa et al., 2021). High-temperature stress during grain filling also induces DNA methylation in the promoters of abscisic acid (ABA) catabolic genes and α-amylase genes, which can delay the germination of the resulting seeds (Suriyasak et al., 2020).
High temperature affects starch accumulation in rice grains via the following mechanisms: Firstly, high temperature can shorten the grain filling period, resulting in insufficient grain filling and inadequate starch accumulation, thus reducing grain yield (Chen et al., 2021b). Secondly, high temperature can reduce the gene expression and bioactivity of key enzymes involved in the conversion of sucrose to starch in endosperm, reducing the rate of starch synthesis and thus affecting total starch content and starch accumulation patterns, especially the amylose content of endosperm starch (Yamakawa and Hakata, 2010; Zhang et al., 2021; Shirdelmoghanloo et al., 2022). Thirdly, high temperature can inhibit photosynthesis in other vegetative organs such as stems and leaf sheaths, resulting in an insufficient supply of fixed carbon from vegetative organs to spikelets, a slower grain filling rate, and a lower grain weight (Yang and Zhang, 2010).
Spermidine (Spd) is an important bioactive polyamine in plants. Exogenous Spd treatment enhances thermotolerance in seeds by regulating starch and polyamine metabolism and reducing high-temperature-induced damage during grain filling. Spd treatment increased the accumulation of OsSAP5 (stress-associated protein 5, an A20/AN1 zinc finger domain protein). When OsSAP5 was heterologously overexpressed in Arabidopsis thaliana, the 1,000-grain weight and thermotolerance of seeds were significantly enhanced. OsSAP5 may be involved in Spd-mediated thermotolerance during seed development (Chen et al., 2021b). Fibrillin 1 (OsFBN1) and the heterodimers formed by ONAC127/129 (two seed-specific NAM/ATAF/CUC domain transcription factors) are also involved in plant growth and grain filling under high-temperature stress (Li et al., 2019; Ren et al., 2021). Rice mutants with deletions of CPHSP70-2 are sensitive to high temperatures and show substantial chalkiness in grains, which seriously affects their yield and quality (Jiang et al., 2021; Yang et al., 2021).
Some studies have shown that grain filling is related to the overall thermotolerance of plants, and most thermosensitive lines show impaired grain filling under high-temperature stress (He et al., 2018; He et al., 2021). The loss-of-function mutants of ERECTA are thermosensitive, and their seeds are smaller than those of the wild type (WT) (Shen et al., 2015; Wu et al., 2022b). High temperature not only reduced the size and yield of rice seeds, but also affected the quality of endosperm (Nevame et al., 2018). The grains of rice grown under normal conditions are round, full, and transparent after filling. However, the rice chalkiness rate (chalkiness is the opaque part of the endosperm in the rice kernel and contain a lower density of starch granules as compared to vitreous ones) increased significantly and the grain weight decreased after high-temperature stress (Wada et al., 2019; Wang et al., 2022a). This has seriously affected the quality of rice and the cooking taste of consumers. Therefore, thermotolerant varieties can be screened by measuring seed size, weight, and the frequency of chalky grains.
How do plants sense high temperatures? Numerous studies on the model plant Arabidopsis have revealed multiple “thermosensors,” including the Ca2+ channel proteins CNGCs (cyclic nucleotide-gated calcium channels) and ANNs (annexins) (Zheng et al., 2012; Li et al., 2018a), RNA thermometers, alternative splicing, changes in DNA/chromatin structure (H2A.Z), photoreceptor phytochrome B, and EARLY FLOWERING 3 (ELF3), which exhibits liquid–liquid phase separation. Recent studies have shown that TT3.1 located on the plasma membrane can transfer heat signals to chloroplasts and improve the thermotolerance of rice (Zhang et al., 2022a). Together, these thermosensors sense high temperatures in the environment and efficiently, repeatedly, and stably transmit heat signals to downstream response factors (Vu et al., 2019; Guihur et al., 2022). On the other hand, research into rice has mainly focused on the response to high-temperature stress in recent years, but it is well established that high-temperature stress can cause protein misfolding and accumulation of high levels of reactive oxygen species (ROS) in rice, which reduce plant thermotolerance (Li et al., 2022).
When plants are under heat stress, they initiate a heat shock response, which is induced by the accumulation of denatured proteins and the transient influx of calcium through specific calcium-permeable channels in the plasma membrane (Zheng et al., 2012; Li et al., 2018a). When rice is subjected to heat stress, a series of heat shock genes are induced, among which heat shock proteins (HSPs) and heat shock factors (HSFs) play crucial role in plant thermotolerance. HSPs can be divided into HSP100, HSP90, HSP70, HSP60, HSP40, and small HSPs; these proteins defend cells against damage (Muller and Rieu, 2016; Wang et al., 2020). HSPs function as molecular chaperones to enhance protein folding and prevent the accumulation of unfolded proteins in cells under high-temperature stress (Ding et al., 2020). OsHSP101 positively regulates tolerance to high temperature and acquired heat shock memory in rice. CHLORPLAST-LOCALIZED HEAT SHOCK PROTEIN 70 (OsHSP70CP1) is essential for chloroplast development under high-temperature conditions (Kim and An, 2013). In addition to HSPs, other related components have been discovered and placed in a complex regulatory network. HSFs are key components of the plant heat-stress response that play important roles in heat-stress memory. To date, 25 OsHSF genes have been identified in rice (Mittal et al., 2009). Exogenous expression of OsHSFA2e enhanced thermotolerance in transgenic Arabidopsis (Yokotani et al., 2008). Under high-temperature conditions, OsHSFA2d is alternatively spliced into OsHSFA2dI, the transcriptionally active form of OsHSFA2d, which participates in the heat-stress response (Cheng et al., 2015).
High-temperature stress inhibits anthocyanin accumulation in rice by suppressing the expression of various genes in the anthocyanin biosynthetic pathway. On the other hand, anthocyanins are widely recognized as antioxidant factors in plant cells with high ROS scavenging ability. And it is known that high-temperature also causes the accumulation of ROS in plant cells, which eventually leads to plant death. The presence of antioxidants is necessary to maintain the dynamic balance of ROS in plants and protect them from the adverse effects of high-temperature stress (Zaidi et al., 2019). High-temperature stress leads to the production of reactive oxygen species, which in turn disrupts the membrane system of cysts, chloroplasts, and plasma membranes. Inactivation of the photosystem, reduction of photosynthesis and inactivation of rubisco affect the production of photoassimilates and their distribution. This ultimately affects flowering, seed filling, size, number, and maturity of rice seeds, thus hindering crop productivity (Miyazaki et al., 2013; Lal et al., 2022). In addition, high temperature is also one of the environmental factors that cause premature leaf senescence in plants. Respiration is very sensitive to high temperatures. The abundance of CYTOCHROME C OXIDASE (COX), the key protein of respiration, decreased significantly when subjected to prolonged high temperature stress (Rashid et al., 2020). The decrease in respiration rate was predicted to be greater for thermotolerant rice (Ferguson et al., 2020). In order to reduce the damage to the plant, when suffer from high temperatures, the leaves open their stomata by increasing transpiration, which in turn has the effect of reducing the surface temperature of the leaves. On the other hand, sustained high temperatures can lead to plant death by causing massive water loss, reduced membrane mobility and permeability, and stomatal closure (Cochard et al., 2007; Liu et al., 2016).
Plant hormones also regulate thermotolerance in rice via independent or interconnected pathways. Moreover, the application of exogenous plant hormones affects the thermotolerance of rice (Fahad et al., 2016). Salicylic acid (SA) enhances stress resistance in plants and plays an important role in regulating rice thermotolerance. Under high-temperature conditions, SA reduces the accumulation of ROS in anthers to prevent premature degradation caused by tapetal programmed cell death, thereby alleviating pollen abortion (Feng et al., 2018; Nadarajah et al., 2021). Brassinosteroids (BRs) function in plant morphogenesis and abiotic stress tolerance. Application of BRs can significantly improve the thermotolerance of plants (Ren et al., 2022). And BRs induce thermotolerance in plants under high-temperature stress by increasing the synthesis of HSPs and the expression of genes encoding protective enzymes (Hwang and Back, 2019). Ethylene-mediated signaling pathways help reduce oxidative damage, maintain chlorophyll content, and enhance thermotolerance in rice seedlings under high-temperature stress. Ethylene also affects rice thermotolerance by regulating carbohydrate metabolism, antioxidant systems, and thus photosynthesis (Gautam et al., 2022).
In general, Abscisic acid (ABA) normally plays a positive regulatory role in rice in response to high-temperature stress. For example, ABA can prevent pollen abortion under high-temperature stress by regulating sugar metabolism in rice spikelets (Rezaul et al., 2019). Rice endogenous ABA content can be regulated by 9-CIS-EPOXYCAROTENOID DIOXYGENASE (OsNCED1), which positively regulates thermotolerance in rice seedlings by increasing endogenous ABA content, thereby improving antioxidant capacity, and activating the expression of heat and ABA-related genes expression (Zhang et al., 2022b). However, ABA also plays a negative regulatory role in regulating thermotolerance in rice. High temperature causes the accumulation of ABA in rice, which inhibits seed germination (Liu et al., 2019). ABA is a negative regulator of thermotolerance in high temperature susceptibility (hts) plants with semi-rolled leaves by modulating energy homeostasis. More interesting, exogenous ABA significantly decreased thermotolerance of hts plants, but clearly enhanced thermoresistance of WT (Li et al., 2020b). The function of ABA on heat stress is important and comprehensive. More research work may be needed to clarify the comprehensive function of ABA in response to heat in detail. Jasmonic acid (JA) and its methyl ester play important roles in plant responses to biotic and abiotic stresses. HEAT-TOLERANCE GENE ON CHROMOSOME 3 (HTG3) regulates thermotolerance in rice by upregulating two heat-responsive JASMONATE ZIM-DOMAIN (JAZ) genes (Wu et al., 2022a). Auxin plays an important role in maintaining spikelet fertility, and decreased levels of the active auxin indole-3-acetic acid (IAA) can cause pollen abortion. High temperature stress induced rice spikelet sterility by inhibiting pollen tube elongation and interfering with auxin homeostasis in the pistil (Zhang C et al., 2018; Zhang N et al., 2018).
Increases in global temperature seriously affect rice yields, making it crucial to understand the roles of different phytohormones and their crosstalk in regulating the thermotolerance of rice. To date, few studies have focused on the roles of endogenous plant hormone signaling in thermotolerance in rice. This line of research will be crucial for maintaining the growth and yield of rice and enhancing its ability to withstand adverse environmental conditions. Designing strategies to reduce yield losses in rice may involve using the components of phytohormone signaling networks to breed varieties with altered expression levels of important stress-response regulators.
A variety of stresses co-occur in rice fields, the most common of which are heat and drought stress. The response of rice to combined stress factors is unique, with rapid adjustments at the physiological and molecular levels (Yadav et al., 2022). High temperatures are often associated with water deficit. Plants normally close their stomata in response to rapid water loss from plant tissues or the soil, but this can lead to increased tissue temperatures due to impaired transpiration. In addition, high temperatures can lead to drought stress through evapotranspiration (Jin et al., 2015). Exposure to combined heat and drought stress can lead to the production of ROS, triggering protective responses such as increasing the expression and activity of ROS-scavenging enzymes and molecules. Rice senses stress signals through signaling sensors including Ca2+ and ROS to activate transcription factors such as bZIP, MYB/MYC, WRKY, AP2/EREBP, and NAC (Yang et al., 2022). In addition, increasing the expression of CATALASE (CAT), ASCORBATE PEROXIDASE (APX), and GATA28a genes helped protect thermotolerant rice variety N22 from lethal damage when heat and drought co-occurred (Yadav et al., 2022). In some parts of the world, where salt stress and high-temperature stress coexist, the accumulation of flavonoids helps regulate endogenous phytohormone levels in rice and balances Na+/K+ levels, allowing plants to resist combined salt and heat stress (Jan et al., 2021).
Some genes might help rice survive under combined high-temperature and salt stress. For example, overexpression of SATIVA INTERMEDIATE FILAMENT (OsIF) helped stabilize photosynthetic mechanisms under both stresses, resulting in significantly higher yields than those of the WT (Soda et al., 2018). DEHYDRATION-RESPONSIVE ELEMENT-BINDING PROTEIN 1C (OsDREB1C) positively regulated heat and salt tolerance (Wang et al., 2022c). Moreover, DEHYDRATION-RESPONSIVE ELEMENT-BINDING PROTEIN 1C (OsDREB1G) and SAP AND MIZ 1 (OsSIZ1) positively regulated drought, heat, and salt tolerance (Mishra et al., 2018; Wang et al., 2022c).
Most studies of the effects of abiotic stress on rice reported to date have focused on the effects of a single stress on plant growth and development. However, rice often faces stressful conditions in addition to high-temperature stress, making it crucial to study the effects of combinations of stresses. Studying the coping mechanisms of rice under combined stress can help maintain plant growth and improve yields in the face of complex environments.
The development of thermotolerant varieties is an important goal of rice improvement. Finding quick and convenient methods for identifying them will help achieve this goal. Diurnal respiration (Rd) is the most plastic physiological characteristic of plants under high-temperature stress. Studies have predicted that plants with higher thermotolerance would show greater decreases in respiration rates under high-temperature stress (Ferguson et al., 2020). However, more research is needed to confirm this notion. Nevertheless, we can preliminarily predict that a certain variety has strong thermotolerance by examining the following thermotolerance-related phenotypes (Table 1) in rice and perhaps other plant species.
Roots play a vital role in absorbing, transporting, and storing water and nutrients, as well as anchoring plants. Thermotolerant rice varieties usually have larger (including more lateral roots and branches) and stronger root systems compared with thermosensitive varieties (Tiwari et al., 2022). For example, plants overexpressing Big Grain 1 (OsRBG1) showed enhanced thermotolerance, and three-week-old plants had longer roots than the WT (Lo et al., 2020). Plants overexpressing HIGHER YIELD RICE (OsHYR) also had more adventitious roots, with greater length and thickness, than the WT (Ambavaram et al., 2014). ZINC-FINGER PROTEIN (OsZFP350)-overexpressing plants showed enhanced thermotolerance, and root volume and length at the seedling stage were significantly larger than those of the WT under normal conditions (Kang et al., 2019), as was the case for OsRCc3 (encoding a rice root special gene)-overexpressing plants (Li et al., 2018b). Loss-of-function mutants of PURPLE LEAF(OsPL) showed enhanced thermotolerance and had longer roots than the WT (Akhter et al., 2019). By contrast, loss-of-function mutants of OsNSUN2 (encoding an RNA 5-methycytosine methyltransferase) were thermosensitive and had significantly shorter roots than the WT (Tang et al., 2020; Zhu and Zhang, 2020). Therefore, examining root length, number of lateral roots and branches, and root biomass can provide important information for studying plant thermotolerance.
Plant height reflects the growth state of a plant and may therefore be related to thermotolerance. Loss-of-function mutants of OsHST1 (encoding a β-ketoacyl carrier protein reductase) showed severe hypersensitivity to high temperature at the seedling stage and a dwarf phenotype under normal conditions (Rana et al., 2019; Chen et al., 2021a). Similarly, loss-of-function mutants of PHOTOPERIOD-THERMOSENSITIVE DWARFISM 1 (OsPTD1), SPTTED LEAF 7 (OsSPL7), HEAT-SENSITIVE ALBINO 1 (OsHSA1), and OsFKBP20-1b (belonging to the immunophilin family) showed decreased thermotolerance and a stunted phenotype under normal conditions (Qiu et al., 2018; Hoang et al., 2019; Deng et al., 2020; Park et al., 2020). By contrast, OsZFP350-overexpressing plants were more thermotolerant and taller than the WT under normal conditions (Kang et al., 2019). Therefore, rice thermotolerance may be related to plant height, that is, thermosensitive plants are short, and thermotolerant ones are tall. However, not all plants conform to this rule. For example, loss-of-function mutants of GLYCOGEN SYNTHASE KINASE 3-LIKE GENE 1 (OsGSK1) showed decreased thermotolerance, but plants overexpressing OsGSK1 showed a dwarf phenotype (Koh et al., 2007). Further experiments are required to examine the potential connection between plant height and thermotolerance.
As the main sites of photosynthesis and transpiration and the largest organs exposed to the environment, leaves play an important role in plant growth and development. Leaf size, color, shape, and other morphological characteristics directly affect photosynthesis in rice, thus affecting grain yield (Kumar et al., 2021; Vitoriano and Calixto, 2021). Leaves of loss-of-function mutants of OsHSP40 were significantly smaller than those of the WT (Barghetti et al., 2017; Wang et al., 2022b). Leaf color is an important morphological characteristic in rice breeding (Liu et al., 2012). Chlorophyll content of the thermosensitive mutant of OsHTS1 (encoding a thylakoid membrane-localized β-ketoacyl carrier protein reductase) was lower than that of the WT, and leaf color was lighter (Chen et al., 2021a). Chloroplasts of the thermosensitive mutant of OsHSA1 developed slowly and showed albinism, which was alleviated during growth, but leaf color was still lighter in the mutant than in the WT (Qiu et al., 2018). By contrast, thermotolerant OsHYR-overexpressing plants had darker green leaves than the WT (Ambavaram et al., 2014).
We propose that leaf color is related to thermotolerance in rice; thermotolerant rice generally has dark leaves, and thermosensitive rice has lighter leaves. Bread wheat genotypes of the stay-green type showed high chlorophyll content and low canopy temperature under both control and high-temperature stress conditions. Maintaining the green phenotype can mitigate the harmful effects of high-temperature stress by maintaining grain yield and biological production (Latif et al., 2020). Increasing chlorophyll content also improved thermotolerance in ryegrass. Treating ryegrass with sodium copper chlorophyllin increased chlorophyll accumulation, downregulated chlorophyll catabolic and senescence genes, and enhanced H2O2 scavenging by the peroxidase pathway, thus inhibiting heat-induced leaf senescence (Yu et al., 2022). Therefore, measuring the chlorophyll content and photosynthetic rate of plants may help in screening plants for thermotolerance. In addition, the senescence rate of leaves, stomatal opening, and leaf temperature regulation might be used as an indicator to identify the thermotolerance of rice. PREMATURE SENESCENCE LEAF 50 (PSL50) mutant are highly sensitive to high temperatures (He et al., 2021). Inhibition of MONODEHYDROASCORBATE REDUCTASE (OsMDHAR4) expression enhances thermotolerance by inducing stomatal closure in rice plants (Liu et al., 2018). Increasing leaf temperature negatively regulates the thermotolerance of hts mutant (Li et al., 2020b). Leaf area and thickness could also be used as an index to identify thermotolerant plants. Thicker leaves have higher chlorophyll content and photosynthetic efficiency. Moreover, the larger the leaf area, the higher the relative number of stomata and the transpiration rate and thus the better the heat dissipation and the thermotolerance.
Abnormalities in both anther and ovary development affect spikelet fertility. The well-known highly thermotolerant rice variety N22 showed higher spikelet fertility than the WT after high-temperature stress (Poli et al., 2013; Shi et al., 2022). Altering the time of flowering, such as flowering early in the morning, could help protect crops from high-temperature stress in the environment, thus reducing the damage that crops suffer during flowering. The near-isogenic line IR64 + qEMF3 advanced flowering time by about 2h, overcoming heat-induced spikelet sterility (Bheemanahalli et al., 2017). Moreover, qEMF3 advanced the flowering time of Nanjing 11 by about 1.5 h, thereby improving the thermoresistance of this variety (Hirabayashi et al., 2015; Jagadish, 2020). Therefore, statistical analysis of flowering time and spikelet fertility might represent another effective means to identify thermoresistant rice varieties.
With the gradual increase in the global population, the worldwide demand for crops is increasing. By 2050, the global agricultural output may need to increase by 60%–110% to meet the demands of the growing population (Ray et al., 2013). However, over the past century, global temperatures have been steadily increasing, which poses great challenges to the global economy and food security. The rise in ambient temperature is a complex variable with many effects depending on duration and degree. Different plant species have relatively stable optimum growth temperatures throughout their life cycles, and the same plant also has different optimum growth temperatures at different stages of growth and development. Hence, plants have evolved complex, rigorous mechanisms to regulate growth and development to adapt to changing environmental temperatures. When the ambient temperature exceeds the optimum temperature for a plant, the plant is in a state of high-temperature stress.
In view of the complexity of plant responses to high temperature and the importance of rice for global agriculture, this review provides an important theoretical basis for identifying new thermotolerant rice varieties according to the physiological morphology and molecular regulatory mechanisms involved in plant growth and development under high-temperature conditions. Higher than optimum temperatures during each period of rice growth and development affect the overall plant growth. High temperatures can lead to delayed germination, reduced pollen viability, and abnormal ovary development and affect the accumulation of starch grains, among other phenotypes, thus affecting rice production (Chen et al., 2017; Zhao et al., 2018; Afzal et al., 2019; Liu et al., 2019).
In recent years, many studies have focused on how to mitigate the effects of high-temperature stress in rice, but we still have a long way to go before fully understanding the thermotolerance mechanism of rice, optimize its quality, and improve its ability to cope with high-temperature stress. The roles of HSPs and HSFs in high-temperature stress are still an important focus of research on rice thermotolerance (Afzal et al., 2019; Wang et al., 2022b). The high-temperature stress response mechanisms in the model organism Arabidopsis have been well investigated by previous studies, the findings of which can be leveraged to expand our understanding of thermotolerance in rice.
This paper also summarizes other physiological phenotypes in roots, stems, leaves, and flowers thereby providing a theoretical basis for further research on genetic variation of rice and screening of thermotolerant varieties. We suggest that root length, leaf color, plant height, flowering time, spikelet fertility and grain filling should be studied as preliminary and rapid statistical indexes for thermoresistant varieties. In addition, leaf senescence rate, stomatal opening and leaf temperature are also very important for rice to cope with high temperature stress. This research direction can help to rapidly screen thermotolerant rice varieties and improve the resistance of rice to unfavorable environmental conditions, but little research has been conducted on this aspect. Of course, the thermoresistant varieties selected need to be moved from the natural living environment into the laboratory to conduct in-depth exploration of their endogenous regulation and metabolic ability, which will be a future research direction. And effects of high temperature on grain quality are also meaningful research filed in the future. The above provides a theoretical basis for ensuring sustainable agricultural development in the face of global warming, as well as achieving high, stable, and safe crop yields to meet the demand for food production from a growing global population.
All authors listed have made a substantial, direct, and intellectual contribution to the work and approved it for publication.
This work was supported by the Natural Science Foundation of Hebei Province (C2020205028, C2021205009, C202105013), High-Level Talent Team Construction Project (225A2902D), the National Natural Science Foundation of China (31870243), Science Foundation of Hebei Normal University (L042018B01, L2019B20), and Postdoctoral Research Foundation of China (2019M651060).
The authors declare that the research was conducted in the absence of any commercial or financial relationships that could be construed as a potential conflict of interest.
All claims expressed in this article are solely those of the authors and do not necessarily represent those of their affiliated organizations, or those of the publisher, the editors and the reviewers. Any product that may be evaluated in this article, or claim that may be made by its manufacturer, is not guaranteed or endorsed by the publisher.
Afzal, S., Sirohi, P., Yadav, A. K., Singh, M. P., Kumar, A., Singh, N. K. (2019). A comparative screening of abiotic stress tolerance in early flowering rice mutants. J. Biotechnol. 302, 112–122. doi: 10.1016/j.jbiotec.2019.07.003
Akhter, D., Qin, R., Nath, U. K., Eshag, J., Jin, X., Shi, C. (2019). A rice gene, OsPL, encoding a MYB family transcription factor confers anthocyanin synthesis, heat stress response and hormonal signaling. Gene 699, 62–72. doi: 10.1016/j.gene.2019.03.013
Ambavaram, M. M., Basu, S., Krishnan, A., Ramegowda, V., Batlang, U., Rahman, L., et al. (2014). Coordinated regulation of photosynthesis in rice increases yield and tolerance to environmental stress. Nat. Commun. 5, 5302. doi: 10.1038/ncomms6302
Arshad, M. S., Farooq, M., Asch, F., Krishna, J. S. V., Prasad, P. V. V., Siddique, K. H. M. (2017). Thermal stress impacts reproductive development and grain yield in rice. Plant Physiol. Biochem. 115, 57–72. doi: 10.1016/j.plaphy.2017.03.011
Bahuguna, R. N., Jha, J., Pal, M., Shah, D., Lawas, L. M., Khetarpal, S., et al. (2015). Physiological and biochemical characterization of NERICA-L-44: A novel source of heat tolerance at the vegetative and reproductive stages in rice. Physiol. Plant 154 (4), 543–559. doi: 10.1111/ppl.12299
Bahuguna, R. N., Solis, C. A., Shi, W., Jagadish, K. S. (2017). Post-flowering night respiration and altered sink activity account for high night temperature-induced grain yield and quality loss in rice (Oryza sativa l.). Physiol. Plant 159 (1), 59–73. doi: 10.1111/ppl.12485
Barghetti, A., Sjögren, L., Floris, M., Paredes, E. B., Wenkel, S., Brodersen, P. (2017). Heat-shock protein 40 is the key farnesylation target in meristem size control, abscisic acid signaling, and drought resistance. Genes Dev 31 22), 2282–2295. doi: 10.1101/gad.301242.117
Bheemanahalli, R., Sathishraj, R., Manoharan, M., Sumanth, H. N., Muthurajan, R., Ishimaru, T., et al. (2017). Is early morning flowering an effective trait to minimize heat stress damage during flowering in rice? Field Crops Res. 203, 238–242. doi: 10.1016/j.fcr.2016.11.011
Chen, F., Dong, G., Wang, F., Shi, Y., Zhu, J., Zhang, Y., et al. (2021a). A beta-ketoacyl carrier protein reductase confers heat tolerance via the regulation of fatty acid biosynthesis and stress signaling in rice. New Phytol. 232 (2), 655–672. doi: 10.1111/nph.17619
Chen, M., Fu, Y., Mou, Q., An, J., Zhu, X., Ahmed, T., et al. (2021b). Spermidine induces expression of stress associated proteins (SAPs) genes and protects rice seed from heat stress-induced damage during grain-filling. Antiox. (Basel) 10 (10), 1–14. doi: 10.3390/antiox10101544
Chen, J., Tang, L., Shi, P., Yang, B., Sun, T., Cao, W., et al. (2017). Effects of short-term high temperature on grain quality and starch granules of rice (Oryza sativa l.) at post-anthesis stage. Protoplasma 254 (2), 935–943. doi: 10.1007/s00709-016-1002-y
Chen, J., Xu, Y., Fei, K., Wang, R., He, J., Fu, L., et al. (2020). Physiological mechanism underlying the effect of high temperature during anthesis on spikelet-opening of photo-thermo-sensitive genic male sterile rice lines. Sci. Rep. 10 (1), 2210. doi: 10.1038/s41598-020-59183-0
Cheng, Q., Zhou, Y., Liu, Z., Zhang, L., Song, G., Guo, Z., et al. (2015). An alternatively spliced heat shock transcription factor, OsHSFA2dI, functions in the heat stress-induced unfolded protein response in rice. Plant Biol. (Stuttg) 17 (2), 419–429. doi: 10.1111/plb.12267
Cochard, H., Venisse, J. S., Barigah, T. S., Brunel, N., Herbette, S., Guilliot, A., et al. (2007). Putative role of aquaporins in variable hydraulic conductance of leaves in response to light. Plant Physiol. 143 (1), 122–133. doi: 10.1104/pp.106.090092
Das, S., Krishnan, P., Nayak, B., Ramakrishnan, B. (2014). High temperature stress effects on pollens of rice (Oryza sativa l.) genotypes. Environ. Exp. Bot. 101, 36–46. doi: 10.1016/j.envexpbot.2014.01.004
Deng, W., Li, R., Xu, Y., Mao, R., Chen, S., Chen, L., et al. (2020). A lipid transfer protein variant with a mutant eight-cysteine motif causes photoperiod- and thermo-sensitive dwarfism in rice. J. Exp. Bot. 71 (4), 1294–1305. doi: 10.1093/jxb/erz500
Dias de Oliveira, E. A., Siddique, K. H., Bramley, H., Stefanova, K., Palta, J. A. (2015). Response of wheat restricted-tillering and vigorous growth traits to variables of climate change. Glob. Chang. Biol. 21 (2), 857–873. doi: 10.1111/gcb.12769
Ding, Y., Shi, Y., Yang, S. (2020). Molecular regulation of plant responses to environmental temperatures. Mol. Plant 13 (4), 544–564. doi: 10.1016/j.molp.2020.02.004
Djanaguiraman, M., Narayanan, S., Erdayani, E., Prasad, P. V. V. (2020). Effects of high temperature stress during anthesis and grain filling periods on photosynthesis, lipids and grain yield in wheat. BMC Plant Biol. 20 (1), 268. doi: 10.1186/s12870-020-02479-0
Endo, M., Tsuchiya, T., Hamada, K., Kawamura, S., Yano, K., Ohshima, M., et al. (2009). High temperatures cause male sterility in rice plants with transcriptional alterations during pollen development. Plant Cell Physiol. 50 (11), 1911–1922. doi: 10.1093/pcp/pcp135
Fahad, S., Hussain, S., Saud, S., Hassan, S., Ihsan, Z., Shah, A. N., et al. (2016). Exogenously applied plant growth regulators enhance the morpho-physiological growth and yield of rice under high temperature. Front. Plant Sci. 7. doi: 10.3389/fpls.2016.01250
Feng, B., Zhang, C., Chen, T., Zhang, X., Tao, L., Fu, G. (2018). Salicylic acid reverses pollen abortion of rice caused by heat stress. BMC Plant Biol. 18 (1), 245. doi: 10.1186/s12870-018-1472-5
Ferguson, J. N., McAusland, L., Smith, K. E., Price, A. H., Wilson, Z. A., Murchie, E. H. (2020). Rapid temperature responses of photosystem II efficiency forecast genotypic variation in rice vegetative heat tolerance. Plant J. 104 (3), 839–855. doi: 10.1111/tpj.14956
Gautam, H., Fatma, M., Sehar, Z., Iqbal, N., Albaqami, M., Khan, N. A. (2022). Exogenously-sourced ethylene positively modulates photosynthesis, carbohydrate metabolism, and antioxidant defense to enhance heat tolerance in rice. Int. J. Mol. Sci. 23 (3), 1–25. doi: 10.3390/ijms23031031
Guihur, A., Rebeaud, M. E., Bourgine, B., Goloubinoff, P. (2022). How do humans and plants feel the heat? Trends Plant Sci. 27 (7), 630–632. doi: 10.1016/j.tplants.2022.03.006
Hayano-Saito, Y., Hayashi, K. (2020). Stvb-i, a rice gene conferring durable resistance to rice stripe virus, protects plant growth from heat stress. Front. Plant Sci. 11. doi: 10.3389/fpls.2020.00519
He, Y., Li, L., Zhang, Z., Wu, J. L. (2018). Identification and comparative analysis of premature senescence leaf mutants in rice (Oryza sativa l.). Int. J. Mol. Sci. 19 (1), 1–17. doi: 10.3390/ijms19010140
He, Y., Zhang, X., Shi, Y., Xu, X., Li, L., Wu, J. L. (2021). PREMATURE SENESCENCE LEAF 50 promotes heat stress tolerance in rice (Oryza sativa l.). Rice (N Y) 14 (1), 53. doi: 10.1186/s12284-021-00493-w
Hirabayashi, H., Sasaki, K., Kambe, T., Gannaban, R. B., Miras, M. A., Mendioro, M. S., et al. (2015). qEMF3, a novel QTL for the early-morning flowering trait from wild rice, oryza officinalis, to mitigate heat stress damage at flowering in rice, o. sativa. J. Exp. Bot. 66 (5), 1227–1236. doi: 10.1093/jxb/eru474
Hoang, T. V., Vo, K. T. X., Rahman, M. M., Choi, S. H., Jeon, J. S. (2019). Heat stress transcription factor OsSPL7 plays a critical role in reactive oxygen species balance and stress responses in rice. Plant Sci. 289, 110273. doi: 10.1016/j.plantsci.2019.110273
Hu, Y., Li, S., Fan, X., Song, S., Zhou, X., Weng, X., et al. (2020). OsHOX1 and OsHOX28 redundantly shape rice tiller angle by reducing HSFA2D expression and auxin content. Plant Physiol. 184 (3), 1424–1437. doi: 10.1104/pp.20.00536
Hu, Q., Wang, W., Lu, Q., Huang, J., Peng, S., Cui, K. (2021). Abnormal anther development leads to lower spikelet fertility in rice (Oryza sativa l.) under high temperature during the panicle initiation stage. BMC Plant Biol. 21 (1), 428. doi: 10.1186/s12870-021-03209-w
Hwang, O. J., Back, K. (2019). Melatonin deficiency confers tolerance to multiple abiotic stresses in rice via decreased brassinosteroid levels. Int. J. Mol. Sci. 20 (20), 1–17. doi: 10.3390/ijms20205173
Impa, S. M., Raju, B., Hein, N. T., Sandhu, J., Prasad, P. V. V., Walia, H., et al. (2021). High night temperature effects on wheat and rice: Current status and way forward. Plant Cell Environ. 44 (7), 2049–2065. doi: 10.1111/pce.14028
Ishimaru, T., Hirabayashi, H., Ida, M., Takai, T., San-Oh, Y. A., Yoshinaga, S., et al. (2010). A genetic resource for early-morning flowering trait of wild rice oryza officinalis to mitigate high temperature-induced spikelet sterility at anthesis. Ann. Bot. 106 (3), 515–520. doi: 10.1093/aob/mcq124
Jagadish, S. V. K. (2020). Heat stress during flowering in cereals - effects and adaptation strategies. New Phytol. 226 (6), 1567–1572. doi: 10.1111/nph.16429
Jagadish, S. V., Craufurd, P. Q., Wheeler, T. R. (2007). High temperature stress and spikelet fertility in rice (Oryza sativa l.). J. Exp. Bot. 58 (7), 1627–1635. doi: 10.1093/jxb/erm003
Jan, R., Kim, N., Lee, S. H., Khan, M. A., Asaf, S., Lubna, et al. (2021). Enhanced flavonoid accumulation reduces combined salt and heat stress through regulation of transcriptional and hormonal mechanisms. Front. Plant Sci. 12. doi: 10.3389/fpls.2021.796956
Jiang, L., Zhong, H., Jiang, X., Zhang, J., Huang, R., Liao, F., et al. (2021). Identification and pleiotropic effect analysis of GSE5 on rice chalkiness and grain shape. Front. Plant Sci. 12. doi: 10.3389/fpls.2021.814928
Jin, R., Wang, Y., Liu, R., Gou, J., Chan, Z. (2015). Physiological and metabolic changes of purslane (Portulaca oleracea l.) in response to drought, heat, and combined stresses. Front. Plant Sci. 6. doi: 10.3389/fpls.2015.01123
Kang, Z., Qin, T., Zhao, Z. (2019). Overexpression of the zinc finger protein gene OsZFP350 improves root development by increasing resistance to abiotic stress in rice. Acta Biochim. Pol. 66 (2), 183–190. doi: 10.18388/abp.2018_2765
Kim, S. R., An, G. (2013). Rice chloroplast-localized heat shock protein 70, OsHsp70CP1, is essential for chloroplast development under high-temperature conditions. J. Plant Physiol. 170 (9), 854–863. doi: 10.1016/j.jplph.2013.01.006
Kobayashi, K., Matsui, T., Murata, Y., Yamamoto, M. (2015). Percentage of dehisced thecae and length of dehiscence control pollination stability of rice cultivarsat high temperatures. Plant Product. Sci. 14 (2), 89–95. doi: 10.1626/pps.14.89
Koh, S., Lee, S. C., Kim, M. K., Koh, J. H., Lee, S., An, G., et al. (2007). T-DNA Tagged knockout mutation of rice OsGSK1, an orthologue of arabidopsis BIN2, with enhanced tolerance to various abiotic stresses. Plant Mol. Biol. 65 (4), 453–466. doi: 10.1007/s11103-007-9213-4
Kumar, S., Tripathi, S., Singh, S. P., Prasad, A., Akter, F., Syed, M. A., et al. (2021). Rice breeding for yield under drought has selected for longer flag leaves and lower stomatal density. J. Exp. Bot. 72 (13), 4981–4992. doi: 10.1093/jxb/erab160
Lal, M. K., Tiwari, R. K., Gahlaut, V., Mangal, V., Kumar, A., Singh, M. P., et al. (2022). Physiological and molecular insights on wheat responses to heat stress. Plant Cell Rep. 41 (3), 501–518. doi: 10.1007/s00299-021-02784-4
Latif, S., Wang, L., Khan, J., Ali, Z., Sehgal, S. K., Babar, M. A., et al. (2020). Deciphering the role of stay-green trait to mitigate terminal heat stress in bread wheat. Agronomy 10 (7), 1–23. doi: 10.3390/agronomy10071001
Lawas, L. M. F., Li, X., Erban, A., Kopka, J., Jagadish, S. V. K., Zuther, E., et al. (2019). Metabolic responses of rice cultivars with different tolerance to combined drought and heat stress under field conditions. Gigascience 8 (5), 1–21. doi: 10.1093/gigascience/giz050
Lee, S. Y., Kim, Y. H., Lee, G. S. (2017). Mapping QTLs associated to germination stability following dry-heat treatment in rice seed. Biotech 7, 220. doi: 10.1007/s13205-017-0807-5
Lee, S. Y., Lee, J. H., Kwon, T. O. (2002). Varietal differences in seed germination and seedling vigor of Korean rice varieties following dry heat treatment. Seed Sci. Technol. 30, 311–321 Available at: https://www.researchgate.net/publication/282180938_Varietal_differences_in_seed_germination_and_seedling_vigor_of_Korean_rice_varieties_following_dry_heat_treatment.
Li, X., Chen, R., Chu, Y., Huang, J., Jin, L., Wang, G., et al. (2018b). Overexpression of RCc3 improves root system architecture and enhances salt tolerance in rice. Plant Physiol. Biochem. 130, 566–576. doi: 10.1016/j.plaphy.2018.08.008
Li, B., Gao, K., Ren, H., Tang, W. (2018a). Molecular mechanisms governing plant responses to high temperatures. J. Integr. Plant Biol. 60 (9), 757–779. doi: 10.1111/jipb.12701
Li, Y., Li, J., Chen, Z., Wei, Y., Qi, Y., Wu, C. (2020a). OsmiR167a-targeted auxin response factors modulate tiller angle via fine-tuning auxin distribution in rice. Plant Biotechnol. J. 18 (10), 2015–2026. doi: 10.1111/pbi.13360
Li, J. Y., Yang, C., Xu, J. M., Lu, H. P., Liu, J. X. (2022). The hot science in rice research: How rice plants cope with heat stress. Plant Cell Environ. 7, 1–17. doi: 10.1111/pce.14509
Li, J., Yang, J., Zhu, B., Xie, G. (2019). Overexpressing OsFBN1 enhances plastoglobule formation, reduces grain-filling percent and jasmonate levels under heat stress in rice. Plant Sci. 285, 230–238. doi: 10.1016/j.plantsci.2019.05.007
Li, G., Zhang, C., Zhang, G., Fu, W., Feng, B., Chen, T., et al. (2020b). Abscisic acid negatively modulates heat tolerance in rolled leaf rice by increasing leaf temperature and regulating energy homeostasis. Rice (N Y) 13 (1), 18. doi: 10.1186/s12284-020-00379-3
Liu, J., Hasanuzzaman, M., Wen, H., Zhang, J., Peng, T., Sun, H., et al. (2019). High temperature and drought stress cause abscisic acid and reactive oxygen species accumulation and suppress seed germination growth in rice. Protoplasma 256 (5), 1217–1227. doi: 10.1007/s00709-019-01354-6
Liu, X., Sun, X., Wang, W., Ding, H., Liu, W., Li, G., et al. (2012). Fine mapping of pa-6 gene for purple apiculus in rice. J. Plant Biol. 55 (3), 218–225. doi: 10.1007/s12374-011-0276-z
Liu, J., Sun, X., Xu, F., Zhang, Y., Zhang, Q., Miao, R., et al. (2018). Suppression of OsMDHAR4 enhances heat tolerance by mediating H2O2-induced stomatal closure in rice plants. Rice (N Y) 11 (1), 38. doi: 10.1186/s12284-018-0230-5
Liu, S. J., Xu, H. H., Wang, W. Q., Li, N., Wang, W. P., Møller, I. M., et al. (2015). A proteomic analysis of rice seed germination as affected by high temperature and ABA treatment. Physiol. Plant 154 (1), 142–161. doi: 10.1111/ppl.12292
Liu, J., Zhang, C., Wei, C., Liu, X., Wang, M., Yu, F., et al. (2016). The RING finger ubiquitin E3 ligase OsHTAS enhances heat tolerance by promoting H2O2-induced stomatal closure in rice. Plant Physiol. 170 (1), 429–443. doi: 10.1104/pp.15.00879
Lo, S. F., Cheng, M. L., Hsing, Y. C., Chen, Y. S., Lee, K. W., Hong, Y. F., et al. (2020). Rice big grain 1 promotes cell division to enhance organ development, stress tolerance and grain yield. Plant Biotechnol. J. 18 (9), 1969–1983. doi: 10.1111/pbi.13357
Luo, Z., Janssen, B. J., Snowden, K. C. (2021). The molecular and genetic regulation of shoot branching. Plant Physiol. 187 (3), 1033–1044. doi: 10.1093/plphys/kiab071
Matsui, T., Omasa, K. (2002). Rice (Oryza sativa l.) cultivars tolerant to high temperature at flowering: anther characteristics. Ann. Bot. 89 (6), 683–687. doi: 10.1093/aob/mcf112
Matsui, T., Omasa, K., Horie, T. (2015). The difference in sterility due to high temperatures during the flowering period among japonica-rice varieties. Plant Product. Sci. 4 (2), 90–93. doi: 10.1626/pps.4.90
Mishra, N., Srivastava, A. P., Esmaeili, N., Hu, W., Shen, G. (2018). Overexpression of the rice gene OsSIZ1 in arabidopsis improves drought-, heat-, and salt-tolerance simultaneously. PloS One 13 (8), e0201716. doi: 10.1371/journal.pone.0201716
Mittal, D., Chakrabarti, S., Sarkar, A., Singh, A., Grover, A. (2009). Heat shock factor gene family in rice: Genomic organization and transcript expression profiling in response to high temperature, low temperature and oxidative stresses. Plant Physiol. Biochem. 47 (9), 785–795. doi: 10.1016/j.plaphy.2009.05.003
Miura, K., Ashikari, M., Matsuoka, M. (2011). The role of QTLs in the breeding of high-yielding rice. Trends Plant Sci. 16 (6), 319–326. doi: 10.1016/j.tplants.2011.02.009
Miyazaki, M., Araki, M., Okamura, K., Ishibashi, Y., Yuasa, T., Iwaya-Inoue, M. (2013). Assimilate translocation and expression of sucrose transporter, OsSUT1, contribute to high-performance ripening under heat stress in the heat-tolerant rice cultivar genkitsukushi. J. Plant Physiol. 170 (18), 1579–1584. doi: 10.1016/j.jplph.2013.06.011
Muller, F., Rieu, I. (2016). Acclimation to high temperature during pollen development. Plant Reprod. 29 (1-2), 107–118. doi: 10.1007/s00497-016-0282-x
Nadarajah, K., Abdul Hamid, N. W., Abdul Rahman, N. S. N. (2021). SA-Mediated regulation and control of abiotic stress tolerance in rice. Int. J. Mol. Sci. 22 (11), 1–16. doi: 10.3390/ijms22115591
Nevame, A. Y. M., Emon, R. M., Malek, M. A., Hasan, M. M., Alam, M. A., Muharam, F. M., et al. (2018). Relationship between high temperature and formation of chalkiness and their effects on quality of rice. BioMed. Res. Int. 2018, 1653721. doi: 10.1155/2018/1653721
Park, H. J., You, Y. N., Lee, A., Jung, H., Jo, S. H., Oh, N., et al. (2020). OsFKBP20-1b interacts with the splicing factor OsSR45 and participates in the environmental stress response at the post-transcriptional level in rice. Plant J. 102 (5), 992–1007. doi: 10.1111/tpj.14682
Peng, S., Huang, J., Sheehy, J. E., Laza, R. C., Visperas, R. M., Zhong, X., et al. (2004). Rice yields decline with higher night temperature from global warming. PNAS 101 (27), 9971–9975. doi: 10.1073/pnas.0403720101
Plaut, Z., Butow, B. J., Blumenthal, C. S., Wrigley, C. W. (2004). Transport of dry matter into developing wheat kernels and its contribution to grain yield under post-anthesis water deficit and elevated temperature. Field Crops Res. 86 (2-3), 185–198. doi: 10.1016/j.fcr.2003.08.005
Poli, Y., Basava, R. K., Panigrahy, M., Vinukonda, V. P., Dokula, N. R., Voleti, S. R., et al. (2013). Characterization of a Nagina22 rice mutant for heat tolerance and mapping of yield traits. Rice (N Y) 6 (1), 36. doi: 10.1186/1939-8433-6-36
Prerostova, S., Jarosova, J., Dobrev, P. I., Hluskova, L., Motyka, V., Filepova, R., et al. (2021). Heat stress targeting individual organs reveals the central role of roots and crowns in rice stress responses. Front. Plant Sci. 12. doi: 10.3389/fpls.2021.799249
Qiu, Z., Kang, S., He, L., Zhao, J., Zhang, S., Hu, J., et al. (2018). The newly identified heat-stress sensitive albino 1 gene affects chloroplast development in rice. Plant Sci. 267, 168–179. doi: 10.1016/j.plantsci.2017.11.015
Rana, M. M., Takamatsu, T., Baslam, M., Kaneko, K., Itoh, K., Harada, N., et al. (2019). Salt tolerance improvement in rice through efficient SNP marker-assisted selection coupled with speed-breeding. Int. J. Mol. Sci. 20 (10), 1–22. doi: 10.3390/ijms20102585
Rashid, F. A. A., Crisp, P. A., Zhang, Y., Berkowitz, O., Pogson, B. J., Day, D. A., et al. (2020). Molecular and physiological responses during thermal acclimation of leaf photosynthesis and respiration in rice. Plant Cell Environ. 43 (3), 594–610. doi: 10.1111/pce.13706
Ray, D. K., Mueller, N. D., West, P. C., Foley, J. A. (2013). Yield trends are insufficient to double global crop production by 2050. PloS One 8 (6), e66428. doi: 10.1371/journal.pone.0066428
Ren, Y., Huang, Z., Jiang, H., Wang, Z., Wu, F., Xiong, Y., et al. (2021). A heat stress responsive NAC transcription factor heterodimer plays key roles in rice grain filling. J. Exp. Bot. 72 (8), 2947–2964. doi: 10.1093/jxb/erab027
Ren, D., Li, Y., He, G., Qian, Q. (2020). Multifloret spikelet improves rice yield. New Phytol. 225 (6), 2301–2306. doi: 10.1111/nph.16303
Ren, H. M., Wu, X. D., Zhao, W. S., Wang, Y. T., Gao, K., Tang, W. Q. (2022). Heat shock-induced accumulation of the glycogen synthase kinase 3-like kinase BRASSINOSTEROID INSENSITIVE 2 promotes early flowering but reduces thermotolerance in Arabidopsis. Front. Plant Sci. 13. doi: 10.3389/fpls.2022.838062
Rezaul, I. M., Baohua, F., Tingting, C., Weimeng, F., Caixia, Z., Longxing, T., et al. (2019). Abscisic acid prevents pollen abortion under high-temperature stress by mediating sugar metabolism in rice spikelets. Physiol. Plant 165 (3), 644–663. doi: 10.1111/ppl.12759
Satake, T., Yoshida, S. (1978). High temperature induced sterility in indica rices at flowering. Japan. J. Crop Sci. 47, 6–17. doi: 10.1626/jcs.47.6
Shen, H., Zhong, X., Zhao, F., Wang, Y., Yan, B., Li, Q., et al. (2015). Overexpression of receptor-like kinase ERECTA improves thermotolerance in rice and tomato. Nat. Biotechnol. 33 (9), 996–1003. doi: 10.1038/nbt.3321
Shi, W., Li, X., Schmidt, R. C., Struik, P. C., Yin, X., Jagadish, S. V. K. (2018). Pollen germination and in vivo fertilization in response to high-temperature during flowering in hybrid and inbred rice. Plant Cell Environ. 41 (6), 1287–1297. doi: 10.1111/pce.13146
Shi, W., Yang, J., Kumar, R., Zhang, X., Impa, S. M., Xiao, G., et al. (2022). Heat stress during gametogenesis irreversibly damages female reproductive organ in rice. Rice (N Y) 15 (1), 32. doi: 10.1186/s12284-022-00578-0
Shirdelmoghanloo, H., Chen, K., Paynter, B. H., Angessa, T. T., Westcott, S., Khan, H. A., et al. (2022). Grain-filling rate improves physical grain quality in barley under heat stress conditions during the grain-filling period. Front. Plant Sci. 13. doi: 10.3389/fpls.2022.858652
Shrestha, S., Mahat, J., Shrestha, J., Madhav, K. ,. C., Paudel, K. (2022). Influence of high-temperature stress on rice growth and development. a review. Heliyon 8 (12), e12651. doi: 10.1016/j.heliyon.2022.e12651
Soda, N., Gupta, B. K., Anwar, K., Sharan, A., Govindjee, Singla-Pareek, S. L., et al. (2018). Rice intermediate filament, OsIF, stabilizes photosynthetic machinery and yield under salinity and heat stress. Sci. Rep. 8 (1), 4072. doi: 10.1038/s41598-018-22131-0
Sreenivasulu, N., Butardo, V. M., Jr., Misra, G., Cuevas, R. P., Anacleto, R., Kavi Kishor, P. B. (2015). Designing climate-resilient rice with ideal grain quality suited for high-temperature stress. J. Exp. Bot. 66 (7), 1737–1748. doi: 10.1093/jxb/eru544
Suriyasak, C., Oyama, Y., Ishida, T., Mashiguchi, K., Yamaguchi, S., Hamaoka, N., et al. (2020). Mechanism of delayed seed germination caused by high temperature during grain filling in rice (Oryza sativa l.). Sci. Rep. 10 (1), 17378. doi: 10.1038/s41598-020-74281-9
Tang, Y., Gao, C. C., Gao, Y., Yang, Y., Shi, B., Yu, J. L., et al. (2020). OsNSUN2-mediated 5-methylcytosine mRNA modification enhances rice adaptation to high temperature. Dev. Cell 53 (3), 272–286 e277. doi: 10.1016/j.devcel.2020.03.009
Tang, S., Zhang, H., Li, L., Liu, X., Chen, L., Chen, W., et al. (2018). Exogenous spermidine enhances the photosynthetic and antioxidant capacity of rice under heat stress during early grain-filling period. Funct. Plant Biol. 45 (9), 911–921. doi: 10.1071/FP17149
Tiwari, M., Kumar, R., Min, D., Jagadish, S. V. K. (2022). Genetic and molecular mechanisms underlying root architecture and function under heat stress-a hidden story. Plant Cell Environ. 45 (3), 771–788. doi: 10.1111/pce.14266
Vitoriano, C. B., Calixto, C. P. G. (2021). Reading between the lines: RNA-seq data mining reveals the alternative message of the rice leaf transcriptome in response to heat stress. Plants (Basel) 10 (8), 1–21. doi: 10.3390/plants10081647
Vu, L. D., Gevaert, K., De Smet, I. (2019). Feeling the heat: Searching for plant thermosensors. Trends Plant Sci. 24 (3), 210–219. doi: 10.1016/j.tplants.2018.11.004
Wada, H., Hatakeyama, Y., Onda, Y., Nonami, H., Nakashima, T., Erra-Balsells, R., et al. (2019). Multiple strategies for heat adaptation to prevent chalkiness in the rice endosperm. J. Exp. Bot. 70 (4), 1299–1311. doi: 10.1093/jxb/ery427
Wang, Y., Sun, F. L., Cao, H., Peng, H. R., Ni, Z. F., Sun, Q. X., et al. (2012). TamiR159 directed wheat TaGAMYB cleavage and its involvement in another development and heat response. Plos One 7 (11), 1–10. doi: 10.1371/journal.pone.0048445
Wang, C., Caragea, D., Kodadinne Narayana, N., Hein, N. T., Bheemanahalli, R., Somayanda, I. M., et al. (2022a). Deep learning based high-throughput phenotyping of chalkiness in rice exposed to high night temperature. Plant Methods 18 (1), 9. doi: 10.1186/s13007-022-00839-5
Wang, H., Lu, S., Guan, X., Jiang, Y., Wang, B., Hua, J., et al. (2022c). Dehydration-responsive element binding protein 1C, 1E, and 1G promote stress tolerance to chilling, heat, drought, and salt in rice. Front. Plant Sci. 13. doi: 10.3389/fpls.2022.851731
Wang, L., Ma, K. B., Lu, Z. G., Ren, S. X., Jiang, H. R., Cui, J. W., et al. (2020). Differential physiological, transcriptomic and metabolomic responses of arabidopsis leaves under prolonged warming and heat shock. BMC Plant Biol. 20 (1), 86. doi: 10.1186/s12870-020-2292-y
Wang, B., Smith, S. M., Li, J. (2018). Genetic regulation of shoot architecture. Annu. Rev. Plant Biol. 69, 437–468. doi: 10.1146/annurev-arplant-042817-040422
Wang, F., Tang, Z., Wang, Y., Fu, J., Yang, W., Wang, S., et al. (2022b). Leaf mutant 7 encoding heat shock protein OsHSP40 regulates leaf size in rice. Int. J. Mol. Sci. 23 (8), 1–13. doi: 10.3390/ijms23084446
Wu, X., Cai, X., Zhang, B., Wu, S., Wang, R., Li, N., et al. (2022b). ERECTA regulates seed size independently of its intracellular domain via MAPK-DA1-UBP15 signaling. Plant Cell 34 (10), 3773–3789. doi: 10.1093/plcell/koac194
Wu, N., Yao, Y., Xiang, D., Du, H., Geng, Z., Yang, W., et al. (2022a). A MITE variation-associated heat-inducible isoform of a heat-shock factor confers heat tolerance through regulation of JASMONATE ZIM-DOMAIN genes in rice. New Phytol. 234 (4), 1315–1331. doi: 10.1111/nph.18068
Yadav, C., Bahuguna, R. N., Dhankher, O. P., Singla-Pareek, S. L., Pareek, A. (2022). Physiological and molecular signatures reveal differential response of rice genotypes to drought and drought combination with heat and salinity stress. Physiol. Mol. Biol. Plants 28 (4), 899–910. doi: 10.1007/s12298-022-01162-y
Yamakawa, H., Hakata, M. (2010). Atlas of rice grain filling-related metabolism under high temperature: Joint analysis of metabolome and transcriptome demonstrated inhibition of starch accumulation and induction of amino acid accumulation. Plant Cell Physiol. 51 (5), 795–809. doi: 10.1093/pcp/pcq034
Yang, W., Liang, J., Hao, Q., Luan, X., Tan, Q., Lin, S., et al. (2021). Fine mapping of two grain chalkiness QTLs sensitive to high temperature in rice. Rice (N Y) 14 (1), 33. doi: 10.1186/s12284-021-00476-x
Yang, Y., Yu, J., Qian, Q., Shang, L. (2022). Enhancement of heat and drought stress tolerance in rice by genetic manipulation: A systematic review. Rice (N Y) 15 (1), 67. doi: 10.1186/s12284-022-00614-z
Yang, J., Zhang, J. (2006). Grain filling of cereals under soil drying. New Phytol. 169 (2), 223–236. doi: 10.1111/j.1469-8137.2005.01597.x
Yang, J., Zhang, J. (2010). Grain-filling problem in 'super' rice. J. Exp. Bot. 61 (1), 1–5. doi: 10.1093/jxb/erp348
Yang, Z., Zhang, Z., Zhang, T., Fahad, S., Cui, K., Nie, L., et al. (2017). The effect of season-long temperature increases on rice cultivars grown in the central and southern regions of China. Front. Plant Sci. 8. doi: 10.3389/fpls.2017.01908
Ye, C., Tenorio, F. A., Redona, E. D., Morales-Cortezano, P. S., Cabrega, G. A., Jagadish, K. S. (2015). Fine-mapping and validating qHTSF4.1 to increase spikelet fertility under heat stress at flowering in rice. Theor. Appl. Genet. 128 (8), 1507–1517. doi: 10.1007/s00122-015-2526-9
Yokotani, N., Ichikawa, T., Kondou, Y., Matsui, M., Hirochika, H., Iwabuchi, M., et al. (2008). Expression of rice heat stress transcription factor OsHsfA2e enhances tolerance to environmental stresses in transgenic arabidopsis. Planta 227 (5), 957–967. doi: 10.1007/s00425-007-0670-4
Yu, G., Xie, Z., Chen, W., Xu, B., Huang, B. (2022). Knock down of NON-YELLOW COLOURING 1-like gene or chlorophyllin application enhanced chlorophyll accumulation with antioxidant roles in suppressing heat-induced leaf senescence in perennial ryegrass. J. Exp. Bot. 73 (1), 429–444. doi: 10.1093/jxb/erab426
Yuan, S., Linquist, B. A., Wilson, L. T., Cassman, K. G., Stuart, A. M., Pede, V., et al. (2021). Sustainable intensification for a larger global rice bowl. Nat. Commun. 12 (1), 7163. doi: 10.1038/s41467-021-27424-z
Zaidi, S. H. R., Zakari, S. A., Zhao, Q., Khan, A. R., Shah, J. M., Cheng, F. (2019). Anthocyanin accumulation in black kernel mutant rice and its contribution to ROS detoxification in response to high temperature at the filling stage. Antiox. (Basel) 8 (11), 1–14. doi: 10.3390/antiox8110510
Zhang, C., Li, G., Chen, T., Feng, B., Fu, W., Yan, J., et al. (2018). Heat stress induces spikelet sterility in rice at anthesis through inhibition of pollen tube elongation interfering with auxin homeostasis in pollinated pistils. Rice (N Y) 11 (1), 14. doi: 10.1186/s12284-018-0206-5
Zhang, Y., Liu, X., Su, R., Xiao, Y., Deng, H., Lu, X., et al. (2022b). 9-cis-epoxycarotenoid dioxygenase 1 confers heat stress tolerance in rice seedling plants. Front. Plant Sci. 13. doi: 10.3389/fpls.2022.1092630
Zhang, H., Xu, H., Jiang, Y., Zhang, H., Wang, S., Wang, F., et al. (2021). Genetic control and high temperature effects on starch biosynthesis and grain quality in rice. Front. Plant Sci. 12. doi: 10.3389/fpls.2021.757997
Zhang, N., Yu, H., Yu, H., Cai, Y., Huang, L., Xu, C., et al. (2018). A core regulatory pathway controlling rice tiller angle mediated by the LAZY1-dependent asymmetric distribution of auxin. Plant Cell 30 (7), 1461–1475. doi: 10.1105/tpc.18.00063
Zhang, H., Zhou, J. F., Kan, Y., Shan, J. X., Ye, W. W., Dong, N. Q., et al. (2022a). A genetic module at one locus in rice protects chloroplasts to enhance thermotolerance. Science 376 (6599), 1293–1300. doi: 10.1126/science.abo5721
Zhao, C., Liu, B., Piao, S., Wang, X., Lobell, D. B., Huang, Y., et al. (2017). Temperature increase reduces global yields of major crops in four independent estimates. PNAS 114 (35), 9326–9331. doi: 10.1073/pnas.1701762114
Zhao, Q., Zhou, L., Liu, J., Du, X., Asad, M. A., Huang, F., et al. (2018). Relationship of ROS accumulation and superoxide dismutase isozymes in developing anther with floret fertility of rice under heat stress. Plant Physiol. Biochem. 122, 90–101. doi: 10.1016/j.plaphy.2017.11.009
Zheng, S. Z., Liu, Y. L., Li, B., Shang, Z. L., Zhou, R. G., Sun, D. Y. (2012). Phosphoinositide-specific phospholipase C9 is involved in the thermotolerance of arabidopsis. Plant J. 69 (4), 689–700. doi: 10.1111/j.1365-313X.2011.04823.x
Keywords: rice, high temperature, heat stress, survival strategies, sustainable agriculture, adaptive traits phenotyping
Citation: Ren H, Bao J, Gao Z, Sun D, Zheng S and Bai J (2023) How rice adapts to high temperatures. Front. Plant Sci. 14:1137923. doi: 10.3389/fpls.2023.1137923
Received: 05 January 2023; Accepted: 01 March 2023;
Published: 17 March 2023.
Edited by:
Keting Chen, Iowa State University, United StatesReviewed by:
Daniel Kean Yuen Tan, The University of Sydney, AustraliaCopyright © 2023 Ren, Bao, Gao, Sun, Zheng and Bai. This is an open-access article distributed under the terms of the Creative Commons Attribution License (CC BY). The use, distribution or reproduction in other forums is permitted, provided the original author(s) and the copyright owner(s) are credited and that the original publication in this journal is cited, in accordance with accepted academic practice. No use, distribution or reproduction is permitted which does not comply with these terms.
*Correspondence: Jiaoteng Bai, anRiYWlAaGVidHUuZWR1LmNu; Shuzhi Zheng, c3p6aGVuZ0BoZWJ0dS5lZHUuY24=
†These authors have contributed equally to this work
Disclaimer: All claims expressed in this article are solely those of the authors and do not necessarily represent those of their affiliated organizations, or those of the publisher, the editors and the reviewers. Any product that may be evaluated in this article or claim that may be made by its manufacturer is not guaranteed or endorsed by the publisher.
Research integrity at Frontiers
Learn more about the work of our research integrity team to safeguard the quality of each article we publish.