- Department of Biosciences and Technology, Dr. Vishwanath Karad MIT-World Peace University, Pune, Maharashtra, India
Plants are continuously threatened by a plethora of biotic stresses caused by microbes, pathogens, and pests, which often act as the major constraint in crop productivity. To overcome such attacks, plants have evolved with an array of constitutive and induced defense mechanisms— morphological, biochemical, and molecular. Volatile organic compounds (VOCs) are a class of specialized metabolites that are naturally emitted by plants and play an important role in plant communication and signaling. During herbivory and mechanical damage, plants also emit an exclusive blend of volatiles often referred to as herbivore-induced plant volatiles (HIPVs). The composition of this unique aroma bouquet is dependent upon the plant species, developmental stage, environment, and herbivore species. HIPVs emitted from infested and non-infested plant parts can prime plant defense responses by various mechanisms such as redox, systemic and jasmonate signaling, activation of mitogen-activated protein (MAP) kinases, and transcription factors; mediate histone modifications; and can also modulate the interactions with natural enemies via direct and indirect mechanisms. These specific volatile cues mediate allelopathic interactions leading to altered transcription of defense-related genes, viz., proteinase inhibitors, amylase inhibitors in neighboring plants, and enhanced levels of defense-related secondary metabolites like terpenoids and phenolic compounds. These factors act as deterrents to feeding insects, attract parasitoids, and provoke behavioral changes in plants and their neighboring species. This review presents an overview of the plasticity identified in HIPVs and their role as regulators of plant defense in Solanaceous plants. The selective emission of green leaf volatiles (GLVs) including hexanal and its derivatives, terpenes, methyl salicylate, and methyl jasmonate (MeJa) inducing direct and indirect defense responses during an attack from phloem-sucking and leaf-chewing pests is discussed. Furthermore, we also focus on the recent developments in the field of metabolic engineering focused on modulation of the volatile bouquet to improve plant defenses.
1 Introduction
Plants are sessile organisms that are very often exposed to several biotic and abiotic stresses during their lifetime. To cope with adverse conditions, plants produce an array of organic compounds called secondary or specialized metabolites (Tisser et al., 2014). Specialized metabolites can be defined as those metabolites that are directly or indirectly involved in plant reproduction, development, defense, and help in mediating ecological interactions with the environment (Tisser et al., 2014). These are diverse compounds that may or may not be produced in all parts of the plant. Accumulation of specialized metabolites also vary from plant species to species, thus contributing to the diversity of these compounds (Knudsen et al., 2006; Dudareva et al., 2013). Based on the chemical structures, they can be broadly classified as polyphenols (lignin, flavonoids, and phenolic acids), nitrogen-/sulfur-containing compounds (alkaloids, glucosinolates, and thiophenes), and terpenoids (including carotenoids) (Tisser et al., 2014). Specialized metabolites also include volatile organic compounds (VOCs), which are low molecular weight molecules with low water solubility and high vapor pressure (Muhlemann et al., 2014). VOCs mediate several functions and act as chemical messengers during different biotic/abiotic stress situations through their elevated levels of secretion and emission (Muhlemann et al., 2014). Biotic stress affecting plants includes attacks from herbivores and infections from bacteria, fungi, and viruses (Suzuki et al., 2014). Herbivores can be further classified based on their attacking mode into chewing herbivores, which cause more cellular damage; mesophyll-feeding stylet feeders; and phloem-feeding stylet feeders, which cause less structural damage to plant tissue but can cause the source to sink shifts by emptying the cellular contents (Kant et al., 2009). This review focuses on how volatile compounds are produced dynamically within the family Solanaceae under the attack of herbivores. The diversity of VOCs produced by Solanaceous crops constitutively and upon attack by different classes of herbivores is also discussed in detail. Secretion and emission of VOCs from these crops during pest attacks can also be affected by abiotic factors (Vázquez-González et al., 2022). However, the influence of these factors affecting plant defense is beyond the scope of this review and have limited to biotic stresses. Furthermore, an attempt is made towards understanding the mechanism of volatile-induced selective defense strategies in these crops. Solanaceous crop plants are considered as most important crops for not only fulfilling the nutritional requirements of vegetables but also as a source of drugs, ornamentals, and medicines (Yadav et al., 2016). Solanaceous plants are attacked by major plant pathogens and pests like bacteria, fungi, nematodes, oomycetes, parasites, and lepidopteran insect pests (Strange and Scott, 2005). Solanaceous plants are known for their distinct evolutionary history driven by natural selection resulting into unique adaptations, signaling molecules, and biochemical pathways involving several genes and products (Poczai et al., 2022). Consequently, they have served as important model plants for studying plant defense mechanisms. We further highlight the ecological importance of these defense mechanisms in the Solanaceae family concerning different types of pests and conclude by listing the possible modifications for improved pest resistance through metabolic engineering in Solanaceous crops.
2 Herbivore- induced plant VOCs
Approximately 1,700 VOCs have been identified from different plant species (Dudareva et al., 2006). Based on their core chemical structure and biosynthesis pathways, VOCs are classified as terpenoids, benzenoids/phenylpropanoids, fatty acid derivatives, and amino acid derivatives (Dudareva et al., 2006). These compounds were initially considered metabolic waste products but were eventually discovered to have important physiological roles (Harrewijn and Piron, 1995). Plants produce VOCs from almost all parts, including leaves, roots, flowers, and fruits into the atmosphere (Dudareva et al., 2006). Among these plant parts, floral volatiles constitute almost 90% of the total mixture of compounds. Floral VOCs play a major role in the attraction of pollinators and thereby help in plant reproduction (Dudareva et al., 2006). Plants also emit VOCs from vegetative tissues, which are normally elevated under biotic/abiotic stress conditions (Dicke and Baldwin, 2010). These compounds also mediate plant defense and carry out tri-trophic and allelopathic interactions. Root volatiles play a major role in defense against nematodes and are actively involved in plant–microbe interactions at the underground level (Huang et al., 2019). The emitted aerial VOCs constitute the headspace of the plants. Along with the emitted bouquet of VOCs, these compounds can be secreted and stored as glycosyl-bound volatiles in plant tissues (Song et al., 2018). The emission of VOCs is influenced by different physical factors, including the circadian clock, light, temperature, and humidity (Dudareva et al., 2006). The emission rate of VOCs is also influenced by the developmental stage of the plant, environmental factors, and presence/absence of abiotic/biotic stress elements (Dudareva et al., 2006).
Herbivore-induced plant volatiles (HIPVs) refer to the class of VOCs that are specifically released by plants during herbivory (Dicke et al., 2009). Several HIPVs have been identified from almost 200 families of plants (War et al., 2011). These compounds may be released by the infected tissues or uninfected tissues, thereby leading to an altered scent profile of the plant. Furthermore, HIPVs act as information molecules conveying pest attacks in host plants to parasitoids/predators and other neighboring plants (Unsicker et al., 2009). Along with acting as info chemicals, these VOCs also increase the defense responses in plants (Mumm and Dicke, 2010). In recent times, several articles have been published regarding the diversity of HIPVs. These include the major classes, including GLVs, terpenoids, phenylpropanoids/benzenoids, and even small molecules like methanol and ethylene (Mumm and Dicke, 2010). The biosynthesis of green leaf volatiles (GLVs) or fatty acid derivative compounds is initiated by the deacetylation of galactolipids to free linolenic acid and linoleic acid in the plastids (Feussner and Wasternack, 2002). This is followed by the enzymatic activities of lipoxygenases, alcohol dehydrogenases, and alcohol acyl transferases, leading to the production of C6 alcohols, aldehydes, and esters (Kim and Grosch, 1981; Matsui et al., 1996; Grechkin, 1998; Howe et al., 2000). Linolenic acid also acts as a precursor compound for the synthesis of jasmonic acid (JA), which is further converted to methyl jasmonate (MeJA) and cis-jasmone (Seo et al., 2001; Song et al., 2005). Another important class of HIPVs includes terpenoid classes of isoprene (C5), monoterpenes (C10), sesquiterpenes (C15), and irregular terpenes. The diversity of terpenoid compounds is due to terpene synthases, which produce these compounds from two inter convertible C5 units, isopentenyl diphosphate (IPP) and its allelic isomer dimethylallyl diphosphate (DMAPP) (McGarvey and Croteau, 1995; Bohlmann et al., 1998; Dudareva et al., 2003; Sharkey et al., 2005). The biosynthesis pathways of terpenoids are also compartmentalized in plastids and cytosol (Poulter et al., 1981). The two terpenoid pathways, the mevalonic acid (MVA) pathway (sesquiterpenes synthesis) and the methylerythritol phosphate (MEP) pathway (monoterpenes synthesis), and their regulatory factors have been studied in model systems like Petunia hybrida, Arabidopsis thaliana, and many other species (Dudareva et al., 2004). Phenylpropanoid/benzenoid volatiles are derived from the aromatic amino acid, phenylalanine (Humphreys and Chapple, 2002). Like terpenoid compounds, extensive studies have been done to understand their biosynthesis pathways and regulatory factors. Benzenoid volatiles are synthesized from phenylalanine originating via the shikimate–chorismate pathway and followed by the deamination of phenylalanine to cinnamic acid by phenylalanine ammonia-lyase (PAL) (Vogt, 2010). A few of the volatiles under this class include methyl benzoate, methyl salicylate, benzyl alcohol, benzyl benzoate, benzyl salicylate, eugenol, and isoeugenol (Vogt, 2010; Muhlemann et al., 2014). Nitrogen-containing compounds such as 1H-indole and methyl-2-amino benzoic acid are also produced from the shikimate pathway (Muhlemann et al., 2014). A brief overview of select classes of HIPVs along with their biosynthetic routes is illustrated in Figure 1.
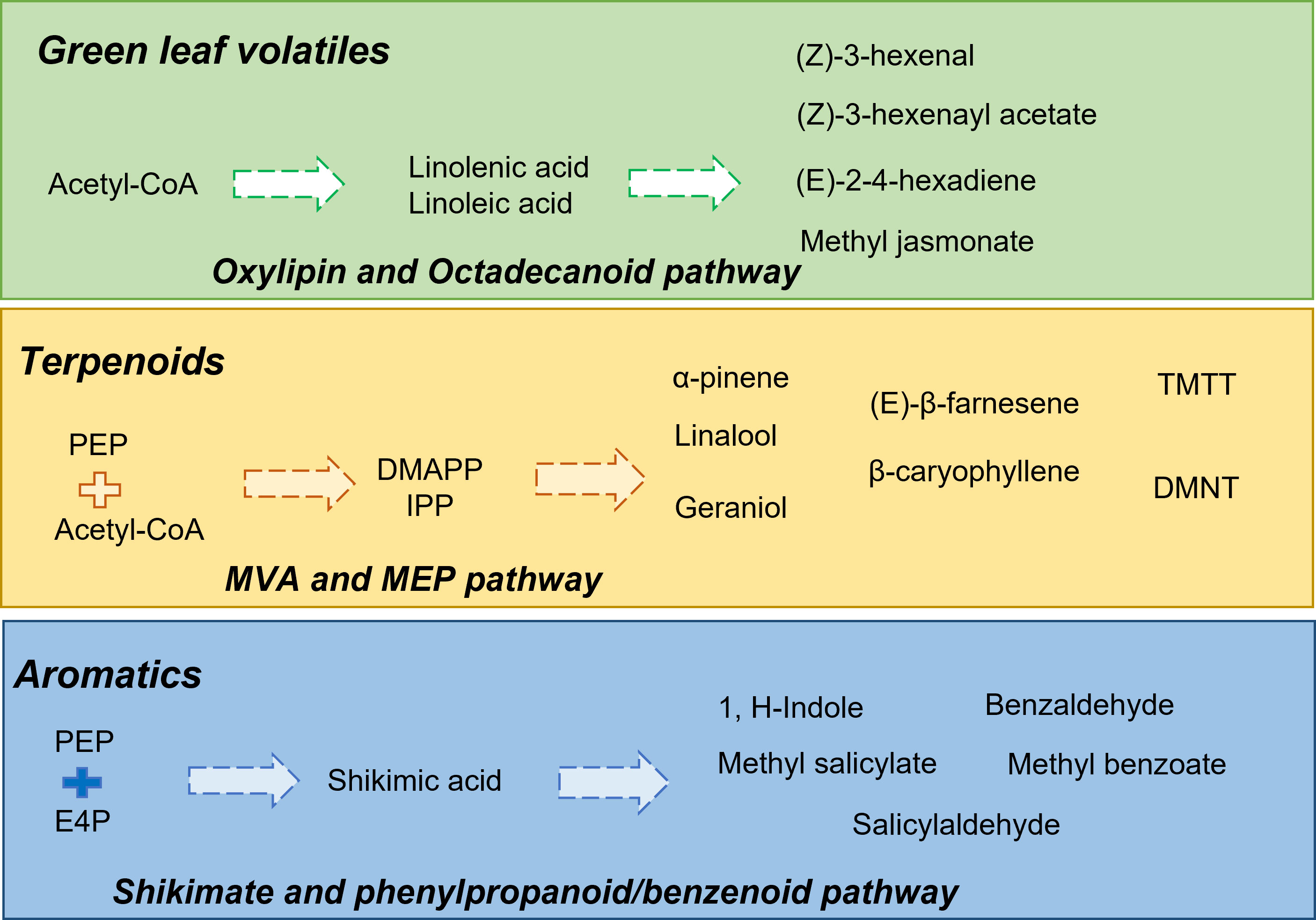
Figure 1 Overview of different classes of herbivore induced plant volatiles (HIPVs), including green leaf volatiles, terpenoids, and aromatic compounds along with their biosynthesis pathways. In the figure, block arrows represent multiple biosynthetic steps involved. Abbreviations: acetyl-CoA, acetyl-coenyzme A; DMAPP, dimethylallyl pyrophosphate; DMNT, 4,8-dimethylnona-1,3,7-triene; E4P, erythrose 4-phosphate; IPP, isopentenyl pyrophosphate; PEP, phosphoenolpyruvate; TMTT, 4,8,12-trimethyltrideca-1,3,7,11-tetraene.
The diversity of these VOCs enables the plants to produce specific cues under biotic stress such as herbivory. The production of these volatile compounds during herbivory can be either triggered by tissue damage or damage-associated molecular patterns (DAMPs) or specific elicitors released by the pests referred to as herbivore-associated molecular patterns (HAMPs) (Meents and Mithöfer, 2020). The former is referred to as damage-induced volatiles (DIVs), while the latter is often referred to as herbivore-induced plant volatiles (HIPVs). Over the years, most of the studies have been taken up on HIPVs as compared to DIVs (Meents and Mithöfer, 2020). Recent studies have reported that mechanical damage inflicted on the plant can trigger systemic responses and herbivore resistance (Quintana-Rodriguez et al., 2018; Heil, 2009; Duran-Flores and Heil, 2016). Here, we try to identify the dynamic plasticity of the emitted volatiles from aerial tissues of Solanaceae crops under herbivory and the consecutive molecular responses related to defense and tri-trophic interactions.
3 Aroma compounds of Solanaceae plants during herbivory
The Solanaceae family includes flowering plants, many of them being economically important crops related to horticulture (petunia), pharmacology (tobacco, mandrake, and jimsonweed), and food (eggplant, potato, tomato, cherry, and gooseberry) (Samuels, 2015). According to the Food and Agricultural Organization (FAO) report, potatoes ranked sixth in the production of primary crops almost accounting for 359,071 thousand tons in 2022 (https://www.fao.org/3/CC2211EN/online/CC2211EN.html#). This also indicates the economic importance of these crops worldwide. One of the major problems faced by these crops is the attack from pests and pathogens. According to FAO, pests account for 20%–40% of the yield losses worldwide (https://www.fao.org/3/CC2211EN/online/CC2211EN.html#). These crops are affected by a variety of herbivores, including chewing herbivores, mesophyll feeding stylet feeders, and phloem-feeding stylet feeders (Kant et al., 2009). In the following sections, we describe the diversity of VOCs produced under the attack of major pests affecting agriculturally important Solanaceae crops (Table 1).
3.1 HIPVs and DIVs during attack of chewing herbivores
Chewing herbivores such as caterpillars, miners, and borers cause more harm to the plant tissues than the sucking pests. These pests directly damage the cell membranes and cell walls. Along with the tissue damage, it has been observed that chewing herbivores trigger defense responses, including the emission of volatiles via effectors. Effectors are molecules that can uplift/trigger defense responses in host plants. They include oral secretions or regurgitant, saliva, ventral eversible gland secretions, waste products, ovipositional fluids, and herbivore-associated endosymbionts (Kant et al., 2009). Chemically, they can be fatty acid conjugates (e.g., volicitin), β-glucosidases, and small peptide molecules (Mattiacci et al., 1995; Alborn et al., 1997; Mori et al., 2001). The detection of the presence of volicitin and other volatile-inducing fatty acid conjugates in oral secretions of tobacco budworm and tobacco hornworm is one of the earliest reports in Solanaceae crops (Halitschke et al., 2001; Mori et al., 2001). Ventral eversible gland secretions of Spodoptera exigua caterpillars have also been shown to significantly increase the production and emission of GLVs ((E)-2-hexenal, (Z)-3-hexenal, (Z)-3-hexenyl acetate, and (Z)-2-hexenol), monoterpenes (β-linalool and γ-terpinene), sesquiterpenes ((E)-β-caryophyllene, α-humulene, and β-elemene), and methyl salicylate in tomato plants (Zebelo et al., 2014). These effector compounds not only trigger the defense responses of the host plant but also induce responses that can attract predators of the pest. A study in potato plants, showing that the treatment with volicitin, regurgitant from the insect larvae, and MeJA treatment increased the attraction of Colorado potato beetles, Leptinotarsa decemlineata (Say), in comparison to the mechanically damaged potato plants even after 24 h (Landolt et al., 1999). This indicates that the HIPVs produced in response to the effector molecules secreted by the insect pests may also increase the attractiveness of the host plant to the same/other pests.
Tuta absoluta (Lepidoptera: Gelechiidae), a major pest of Solanaceae crops, has shown differential behavioral responses to VOCs emitted by the tomato and potato plants in their natural conditions (Caparros Megido et al., 2014). According to the report, enhanced emission of monoterpenes (α-pinene, sabinene, myrcene, δ-2-carene, α-phellandrene, δ-3-carene, and β-phellandrene) was found to be attractive for the pests in tomato plants and enhanced emission of sesquiterpenes (β-caryophyllene, (E)-β-farnesene, germacrene-D, and germacrene-D-4-ol) was found to be attractive in potato plants. However, T. absoluta females did not show any preference for oviposition according to these volatile cues. Floral volatiles from tobacco, including (E)-β-ocimene, octanal, (Z)-3-hexenyl acetate, (Z)-3-hexen-1-ol, nonanal, (Z)-3-hexenyl-2-methyl butyrate, decanal, linalool, and (E)-β-caryophyllene, have been found to be attractive for another chewing herbivore, Helicoverpa assulta (Guene´e) (Lepidoptera: Noctuidae) (Sun et al., 2012). The attraction of the pest Phthorimaea operculella to tobacco plants mediated by GLVs has also been reported by Xiang et al. (2020). Interestingly, geraniol, a monoterpene volatile has been found to deter the oviposition of shoot and fruit borer on eggplant (Ghosh et al., 2022).
Qualitative and quantitative differences in the emitted volatiles have been observed in the tomato plants infested with T. absoluta (Silva et al., 2017). Headspace analysis of these plants has shown a consistent association with the emission of fatty acid derivative compounds, including 3-methyl butan-1-ol, (Z)-2-penten-1-ol, (Z)-3-hexen-1-yl-formate, (Z)-2-penten-1-yl butyrate, and few other related compounds. An almost 10-fold increase in the emission of volatiles, including terpenes, aromatic compounds, and fatty acid esters, has been observed post-infestation. A recent metabolome and volatilome analysis of eggplant and tomato post-infestation revealed that differential accumulation of both metabolites and VOCs was responsible for the pest resistance in eggplants (Chen et al., 2021). Interestingly, the study also reported that the borer showed differential behavioral responses during pre-and post-infestation in both eggplants and tomatoes. Terpenes (nerolidol, beta-Cyclocitral, 1,3-cyclohexadiene-1-carboxaldehyde, 2,6,6-trimethyl, and beta-iso-methyl ionone) and a few other ketones, heterocyclic esters, aldehydes, and alcohols emitted from tomato plants could have attracted and/or stimulated the attack of pests, while emission of nerolidol (terpene), 1,3-cyclopentadiene, 5,5-dimethyl-1,2-dipropyl (olefin), and 2-butenoic acid, 3-hexenyl ester (ester) due to mechanical damage or borer infestation from eggplants could have repelled the pest or decreased its survival (Chen et al., 2021). Among the other metabolites, two deterrent compounds, viz., flavonoid compounds (6-hydroxy kaempferol-3-O-rutin-6-O-glucoside) and quercetin-3-O-apiosyl (1 → 2) galactoside), were found to be produced in higher quantities in eggplants. These flavonoids have been shown to modulate the oviposition and feeding of herbivores in different crops (Simmonds, 2001). The induction or enhanced emission of VOCs during the attack of chewing herbivores has also been reported in maize, rice, cotton, and legume crops (Leitner et al., 2005; Sobhy et al., 2017; Qi et al., 2018; Arce et al., 2021).
Recently, GLVs have been shown to emit immediately because of the mechanical damage caused during herbivory in plants, including tomatoes, potatoes, lima beans, Arabidopsis, and even trees (Meents and Mithöfer, 2020). Farag and Paré (2002) explained how C6 GLV, (E)-2-hexenal triggers the defense responses, including the emission of VOCs in tomatoes. Similar reports of induction of defense through mechanical damage in cotton leaves and Arabidopsis have also been reported (Rodriguez-Saona et al., 2003, Yamauchi et al., 2018; Arce et al., 2021). The effects of damage-induced volatiles seem to be contradictory in a few plants even among Solanaceae family. Studies by Landolt et al. (1999) reported that mechanical- damage-induced VOCs did not attract Colorado potato beetles significantly in comparison to the VOCs released in response to effector molecules released by larvae feeding. Treatment with cis-3-hexenyl acetate (z3HAC), another GLV often grouped under DIVs, did trigger defense responses in Capsicum annuum (Freundlich et al., 2021). These reports are in line with a well-known fact that damage helps in the recognition of attack by plants but then is not completely sufficient to trigger the full plant defense (Heil, 2009; Fürstenberg-Hägg et al., 2013; Acevedo et al., 2015).
3.2 HIPVs during attack of mesophyll-feeding stylet feeders
Mesophyll-feeding stylet feeders include mites and thrips. These insects can empty the cell contents without causing more damage to cell walls and plasma membranes. They primarily feed on the leaf tissues via their stylet penetrating the stomatal openings or the intercellular spaces of cells located on tissue surfaces (Bensoussan et al., 2016). Most of the studies have focused on the emission of VOCs from plants under attack by chewing herbivores, while limited reports exist on the emission of HIPVs from plants under attack by other types of feeding herbivores, including mesophyll-feeding stylet feeders (Delphia et al., 2007). Two-spotted spider mite (Tetranychus urticae) is a generalist pest that infects almost 150 crop species, including those from the Solanaceae family. Upon infestation with these mites, a susceptible line of chili showed increased emission of VOCs, specifically, (E)-2-4-Hexadiene and methyl salicylate, while the resistant line showed enhanced emission of sesquiterpene compounds. The emission of benzenoid volatiles was found repressed in both the lines (Zhang et al., 2020). In tomatoes, the infestation of T. urticae induced the emission of terpenoids and methyl salicylate mediated by JA (Ament et al., 2004). Contradictorily, in a different tomato cultivar under the attack of T. urticae, enhanced emission of terpenoids, methyl salicylate, and methyl benzoate induced via salicylic acid (SA) signaling was observed (Weinblum et al., 2021). Time course profiling of VOCs emitted from tomato leaves upon spider mite infestation showed the enhanced emission of methyl salicylate, 4,8,12-trimethyltrideca-1,3,7,11-tetraene (TMTT), trans-β-ocimene, trans-nerolidol, and linalool, which relatively kept increasing up to 5 days (Kant et al., 2004). Secondary metabolites, including anthocyanins, were induced in tomato plants almost 5 days after the infestation, thus suggesting that the emission of volatiles is part of the secondary response when mites infect the plants. Delayed emission of terpenoid compounds upon spider mite infection almost after 5 days has also been reported in lima beans and cucumber (Bouwmeester et al., 1999; Mercke et al., 2004). In the case of chewing herbivores, which cause more damage to the foliar tissues, emission of GLVs almost happens immediately in comparison to the VOCs emitted by the plants infected by mites. Another specialist herbivore, i.e., the tomato red spider mite Tetranychus evansi, showed similar attractiveness to the foliar volatiles emitted by cultivated African nightshade plants (Solanum sarrachoides Sendtner, S. villosum Miller, and S. scabrum Miller) and VOCs (unsaturated fatty acids) from glandular trichomes of one of the plants studied, which deterred the oviposition of the pest (Murungi et al., 2016). JA-mediated induction of linalool synthase in trichomes of the leaf tissues during spider mite infestation has been reported in tomato (van Schie et al., 2007). Furthermore, Falara et al. (2014) identified a class of geranyl linalool synthases in the Solanaceae family and other angiosperms that are responsible for the production of defensive compounds. They also reported that the corresponding genes can be expressed by treatment with MeJA.
Western Flower Thrips, Onion thrips, and melon thrips have also been shown to prey on the Solanaceae crops. Responses of western flower thrips (Frankliniella occidentalis) to plant volatiles when analyzed led to the identification of attractant and repellant VOC molecules for the pest. p-Anisaldehyde, nerol, ethyl nicotinate, and (E)-β-farnesene were found to be attractive at several concentrations, while salicylaldehyde, a benzenoid compound, was found to be repellent for the thrips (Koschier et al., 2000). One of the early reports describing the emission of induced VOCs by white flower thrips feeding was by Delphia et al. (2007) in tobacco plants. VOCs, when analyzed after 4 days of a high level of infection, showed the presence of terpenoid compounds, including (E)-β-ocimene, linalool, β-caryophyllene, unidentified sesquiterpene, farnesene, and nicotine, consistently. Pest attack by western flower thrips in tomatoes has shown a delayed increase in the production of terpenoid compounds (α-pinene, δ-carene, α-phellandrene, α-terpinene, limonene, and β-phellandrene) via JA regulatory pathway by increasing the trichome density in leaf tissues of the plant (Escobar-Bravo et al., 2017; Chen et al., 2018).
3.3 HIPVs during attack of phloem-feeding stylet feeders
Phloem-feeding stylet feeders include aphids and whiteflies often grouped as sucking pests. They cause minimal damage to the mesophyll cells and rather cause a shift in the plant’s source to sink flow (Kant et al., 2009). One of the earliest report of the production of HIPVs upon aphid (Myzus persicae (Sulzer)) attack was in Solatium berthaultii Hawkes, which released (E)-β-farnesene, a sesquiterpenoid compound from glandular hairs of the shoot system (Gibson and Pickett, 1983). Interestingly, this compound is also believed to be an aphid alarm pheromone (Bowers et al., 1972). Harmel et al. (2007) also reported the changes in the emission levels of terpene, (E)-β-farnesene, upon infection of potato plants with Myzus persicae. Consistent emission of few fatty acid derivatives (hexanal, E-2-hexenal, Z-2-hexenal, and their alcohols, decanal and phthalic acid, and cis-hexen-1-ol), terpenoids (α-pinene, β-ocimene), and a benzenoid compound (methyl salicylate) during aphid attack has been reported in eggplant, chili, and tomato (Digilio et al., 2012; Cascone et al., 2015; Ali et al., 2022). Consistent emission of limonene and (E)-β-farnesene from Rhopalosiphum padi (Hemiptera: Aphididae)- infected rice has been reported to increase the resistance of crops against the pest (Sun et al., 2017). Attack of Lipaphis erysimi in Arabidopsis plants showed enhanced emission of HIPVs when the intensity of attack increased within 24 h. A few volatiles detected include limonene, α-terpineol, benzaldehyde, phenylacetaldehyde, and decan-3-ol (Lin et al., 2016). The emission of methyl salicylate has also been reported from different crops under the attack of aphids (Zhu and Park, 2005).
Whiteflies are another class of sucking pests that attack major crops worldwide. They not only suck up the nutrients from the plant tissue but also promote the growth of pathogenic fungi and act as vectors for several viruses (Perring et al., 2018). Bemisia tabaci (Gennadius) and Trialeurodes vaporariorum Westwood are two prominent pests affecting several crops worldwide. The production of a sesquiterpene zingiberene (Antonious and Kochhar, 2003) and a fatty acid derivative (Fridman et al., 2005) by glandular trichomes of wild tomatoes have shown deterrence to the infection of the pest. The study by Bleeker et al. (2009) also showed that monoterpene p-cymene was putatively repellent and revealed two additional candidates, i. e., α-terpinene and α-phellandrene, against the pest in tomato lines. Emission of Z-3-hexen-1-ol, α-pinene, α-humulene, (E)-β-caryophyllene, methoxyphenyl oxime, azulene, and 1,1-dimethyl-3-methylene-2-vinylcyclohexane was shown to increase in egg plants infested by greenhouse whitefly, Trialeurodes vaporariorum Westwood (Darshanee et al., 2017).
To summarize, feeding by chewing herbivores induces the emission of GLVs, terpenes (monoterpenes, sesquiterpenes, and homoterpenes), and, at times, methyl salicylate (limited reports) due to the mechanical damage caused to the tissues, released effector molecules, and the eggs laid by the pests on the Solanaceae crops (Table 1). Mesophyll-feeding stylet feeders have been shown to induce the emission of terpenoids, and fatty acid esters present in the trichomes of the leaf tissue via JA signaling. Very few reports are available on the effector molecules released by mites/thrips inducing defense responses in the host plants (Steenbergen et al., 2018). Phloem-feeding stylet feeders causing minimal damage to the plant tissues release HIPVs like phenylpropanoids/benzenoids (methyl salicylate, benzaldehyde), terpenes (E-β-farnesene), and fatty acid esters (Figure 2). Leaf-chewing herbivores are known to activate the JA signaling pathway. While phloem-sucking pests activate the SA signaling pathway at times, even by switching off the JA signaling pathway (Clavijo McCormick et al., 2012). A recent meta-analysis of 236 experiments dealing with HIPVs released by chewing herbivores vs. sucking pests reported higher total amounts of volatiles, including GLVs and terpenoids being released by the chewing herbivores as compared to the sucking pests that selectively induce fewer compounds belonging to benzenoid/phenylpropanoid and terpenoid classes (Rowen and Kaplan, 2016). Differential emission of volatiles when two different types of pests are attacking the same crop plant has also been studied in the Solanaceae family (Gosset et al., 2009; Silva et al., 2017). Davidson-Lowe and Ali (2021) also studied the VOCs emission pattern in potato plants under the co-occurrence of chewing and sucking pests. The study reported that sucking pests preferred uninfected plants over the plants that were already infected with the Colorado potato beetle. Analysis of the emission of HIPVs by plants under different biotic stress conditions can help in identifying the volatile signatures, which may be used for early detection of pest attacks, thereby reducing the crop losses incurred. This review being limited to a few important agricultural crops of the Solanaceae family may have overlooked any other classes of VOCs emitted upon herbivory. In the following sections, we further discuss the dynamic role of plant volatiles affecting direct and indirect defense mechanisms in plants.
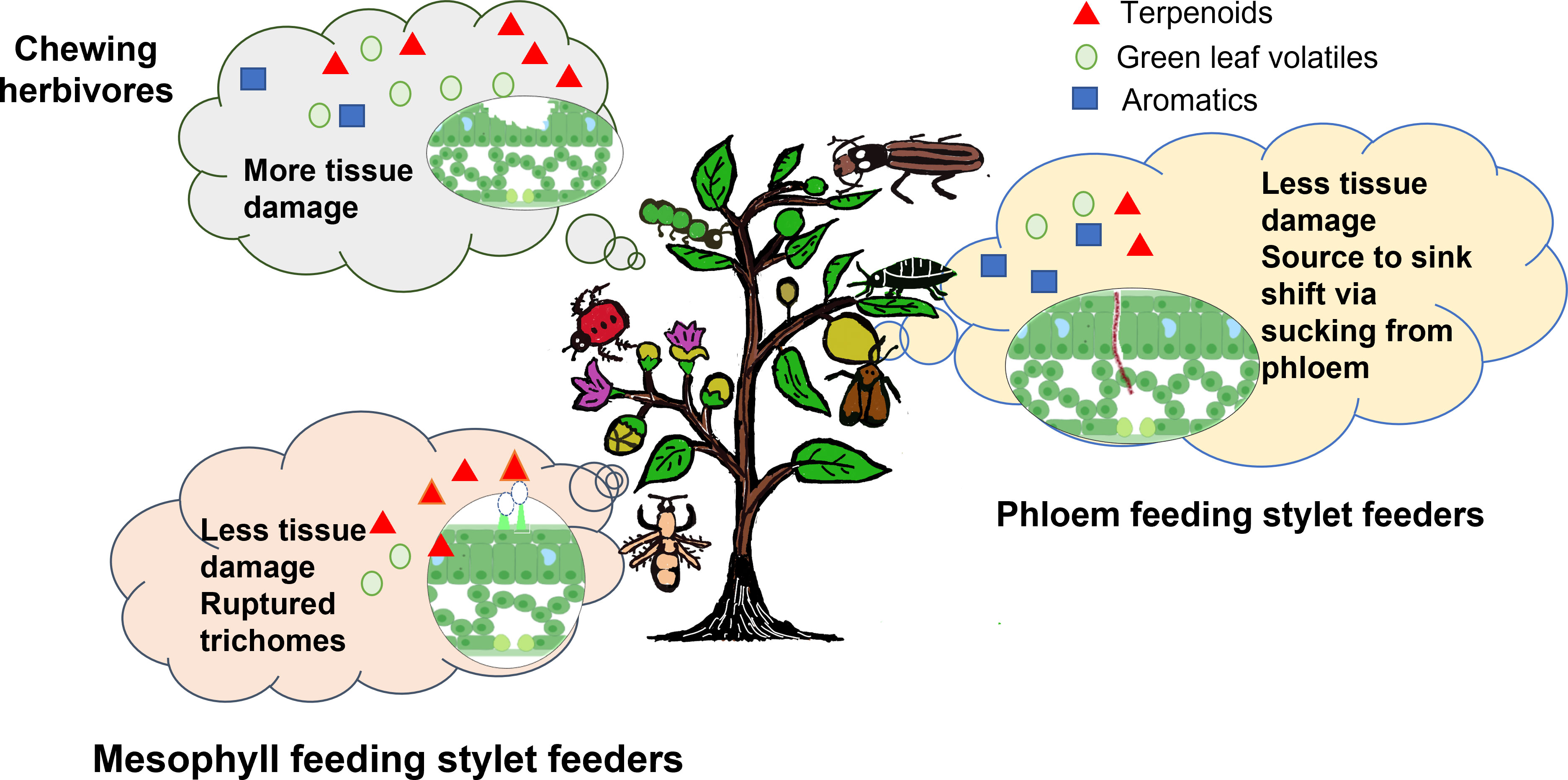
Figure 2 Diversity of HIPVs emitted in Solanaceae crops under herbivory. Emission of higher amounts of VOCs including terpenoids, GLVs, and few aromatics under the attack of chewing herbivores and mesophyll-feeding stylet feeders along with selective emission of aromatics, terpenoids, and few GLVs under the attack of phloem feeding stylet feeders is shown. In set in the figures shows a representative cross-section of leaf tissue damage under respective herbivory.
4 Multifunctionality of HIPVs in plant defense
Plants emit specific blends of info chemicals upon mechanical tissue damage and herbivore attack. HIPVs act as physiological regulators that can prime plant defenses (Hu et al., 2021). Faster and stronger activation of plant defenses in HIPV-exposed plants leading to enhanced resistance in subsequent herbivore attacks is known as “defense priming” (Engelberth et al., 2004; Kim and Felton, 2013). HIPVs prime JA accumulation by modulating the early defense signaling components and subsequently regulating the transcription of jasmonate-responsive genes (Engelberth et al., 2013; Erb et al., 2015). Jasmonates are known to be the key regulators of plant defense and herbivore resistance. Therefore, it is assumed that HIPVs increase plant resistance by regulating the JA signaling pathway. The intricate molecular mechanisms underlying the volatile-mediated defense priming have been explored in several plants and discussed below.
4.1 Regulation of GLVs in response to herbivory
GLVs, namely, six-carbon aldehydes ((E)-2-hexenal), alcohols ((Z)-3-hexenol), and their esters ((Z)-3-hexenyl acetate) are dramatically emitted by plants upon tissue damage or herbivore attack (Engelberth et al., 2013; Ameye et al., 2017). GLVs being produced from existing precursors are produced very rapidly upon tissue damage. They are produced via the lipoxygenase (LOX) pathway, the first product (Z)-3-hexenal being formed by the oxygenation of linolenic acid catalyzed by LOX. The isomerization of (Z)-3-hexenal yields (E)-2-hexenal, which is directly toxic for the infesting herbivores. C6 aldehyde forms are converted into corresponding C6 alcohols by alcohol dehydrogenases followed by the action of acetyltransferases to form esters (D’Auria et al., 2007). The intermediate product in this pathway, linolenic acid 13-hydroperoxide (13HPOT), also serves as a precursor for JA, which regulates the production of herbivore-induced volatile terpenoids in damaged and undamaged tissues. Hence, GLV and JA bio syntheses both support the effective regulation of HIPV production by plants upon herbivory (Arimura et al., 2009) (Figure 3). GLVs are released within seconds of tissue damage from leaves and stem. Real-time volatile analysis studies in Arabidopsis have shown peaking of (Z)-3-hexenal at 30–45 s, and alcohol and ester form at 5 min following the damage (D’Auria et al., 2007). It is assumed that (Z)-3-hexenal is the predominant product at the tissue damage site, while alcohol and acetate are formed in the vicinity of the wounded site owing to the ample supply of (Z)-3- hexenal from directly disrupted tissues. Presumably, NAD(P)H and acetyl-CoA from healthy leaves support the production of alcohols and acetates, respectively. Many studies have suggested the spatial differentiation of GLVs between local and distal sites of herbivore damage or mechanical tissue disruption. For instance, in maize and cotton leaves, acetate forms were significantly emitted from the distal sites of herbivory or when treated with MeJA. However, emission of all the GLVs with local and distal differentiation was observed when leaves were artificially damaged or subjected to herbivory (Farag et al., 2005; Arimura et al., 2009). The detailed mechanism leading to the rapid release of hexenal after herbivory or wounding is not yet clarified, but high GLV emissions also upon photooxidative stress have suggested that oxidative damage of membranes could be the primary cause of induction of GLV emissions. Oxidative stress is also considered one of the consequences of herbivore damage (Mithöfer et al., 2004; Mithöfer et al., 2005). GLVs are released immediately at the site of tissue damage and result in moderate plant response specifically, priming the neighboring plants against impending herbivory by inducing the chemical defenses. The induction of several plant- defense-related genes that are induced upon MeJA treatment has been observed upon treatment of Arabidopsis plants with C6 volatiles— phenylpropanoid- related genes such as phenylalanine ammonia-lyase, chalcone synthase, dihydro flavonol reductase, and LOX pathway genes, including LOX and allene oxide synthase (AOS) (Bate and Rothstein, 1998).
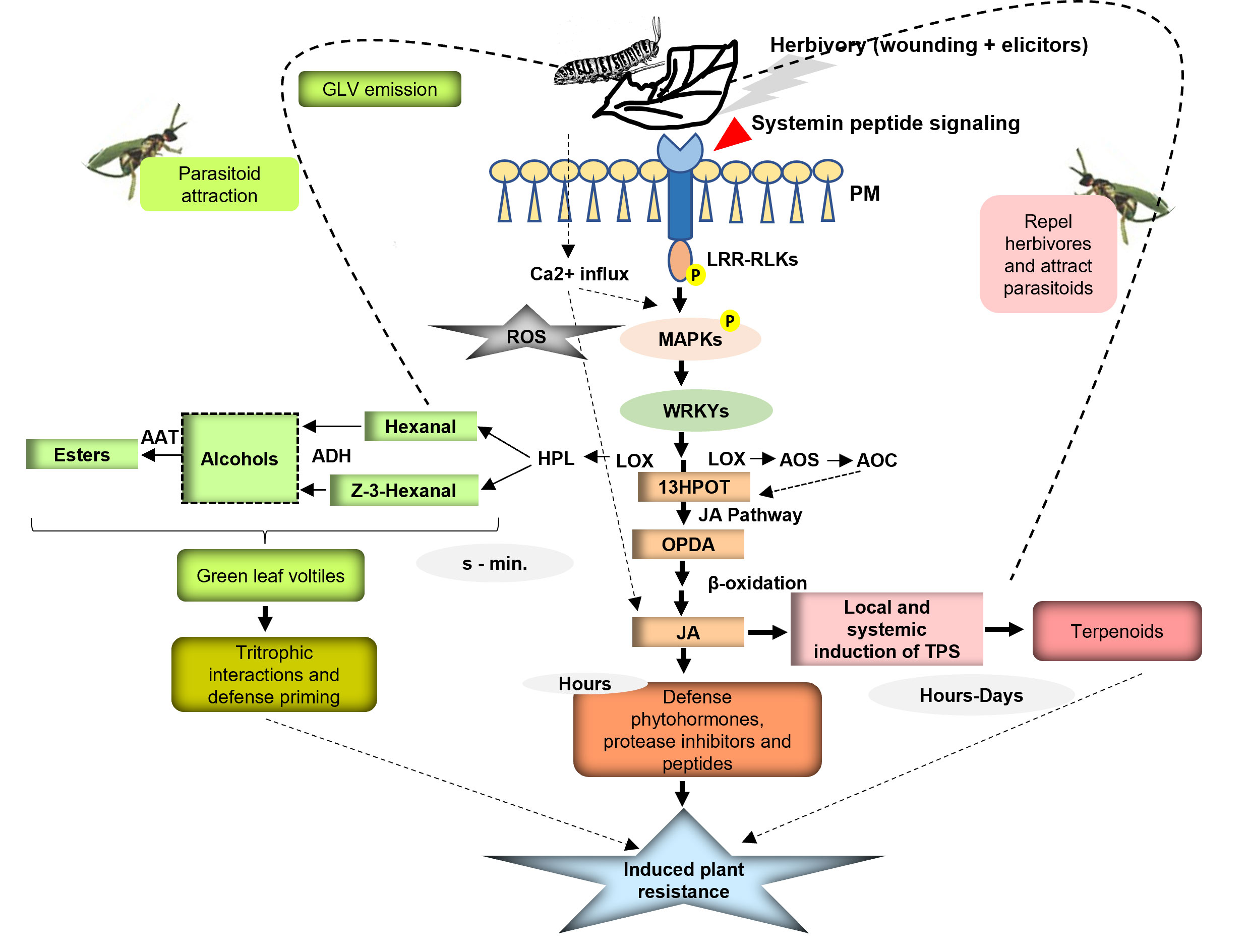
Figure 3 Schematic model of herbivore-induced defense signaling in plants. Role of specific early defense regulators and components comprising of Ca2+, LRR-RLKs, MAPKs, WRKY transcription factors, and jasmonate biosynthesis genes leading to downstream defense gene expression to activate plant defenses is illustrated. The role of GLVs and terpenoids in mediating the direct and indirect responses via tritrophic interactions is also exemplified.
4.2 Regulation of volatile terpenoids in response to herbivory
Terpenoids, the structurally diverse natural compounds, are produced in plants via two biosynthetic pathways: i) the mevalonate (MVA) pathway in the cytoplasm and ii) 2- C -methyl- D -erythritol 4-phosphate (MEP) pathway in the plastids (Arimura et al., 2009; Nagegowda and Gupta, 2020). Both pathways are independent of each other but produce the same intermediate, the five-carbon compound isopentenyl diphosphate (IDP). Substantial contribution of IDP from the MEP pathway has been reported towards total sesquiterpene biosynthesis upon herbivory (Bartram et al., 2006). The structural diversity of terpenoids is brought about by the terpene synthases (TPSs), which can utilize different prenyl diphosphates as substrates to synthesize hemeterpenes (C5), monoterpenes (C10), sesquiterpenes (C15), homoterpenes (C11 and C16), and diterpenes (C20). Hence, TPSs significantly contribute to the plasticity of terpenoid blends produced in response to herbivory (Arimura et al., 2008a). JA and its precursors act as master switches for upregulating a specific set of defense genes for terpenoid production. As a direct response to mechanical tissue damage mimicking herbivory, JA accumulation is observed locally leading to immediate upregulation of the ocimene synthase gene in lima bean leaves (Arimura et al., 2008b). Gene expression studies have shown the upregulation of terpene synthase genes TPS7 (encoding for monoterpene, β-ocimene) and TPS12 (encoding for (E)-β-caryophyllene) in tomato plants damaged by caterpillars (Zebelo et al., 2014). However, the release of de novo synthesized terpenes from plants may take several hours to days (Turlings et al., 1998; Danner et al., 2015).
The synergistic and antagonistic crosstalk among the influx of calcium ions, JA, and ethylene signaling regulates terpenoid biosynthesis. Plants show varied responses to sucking arthropods and chewing insect pests. In plants damaged by sucking arthropods, the specific terpenoid blend is regulated by the antagonistic crosstalk of SA with JA, while chewing herbivores stimulate the calcium influx, which acts as a secondary messenger for the activation of the JA signaling pathway (Arimura et al., 2008a). Generally, negative interactions between JA and SA signaling have been reported, but this crosstalk can show variations depending upon the degree of damage, timing, and the specific herbivore leading to huge differences in the blend of terpenoids being emitted. Ethylene plays a role in modulating the early signaling events like cytoplasmic Ca2+ influx and downstream JA-dependent biosynthesis, which in turn can regulate terpenoid production (Figure 3). Moreover, chemical elicitors like fatty acid-amino acid conjugates (FACs) present in oral secretions of lepidopteran larvae synergistically induce plants to release HIPVs (Halitschke et al., 2001; Yoshinaga et al., 2010). Oral secretions (OS) from fall armyworm, Spodoptera frugiperda, have shown the presence of proteolytic fragments Inceptin [+ ICDINGVCVDA −] and the related peptides [+ (GE) ICDINGVCVDA −] eliciting rapid production of JA, SA, and ethylene (Schmelz et al., 2007). β-Glucosidase, volicitin, and caeliferins are other reported insect oral elicitors known for inducing the production of HIPVs. Contrary to this, very little is known about oral elicitors from sucking arthropods except for oligogalacturonides, which are assumed to induce Ca2+ influx due to cell wall digestion (Will and van Bel, 2008).
4.3 Regulation of early defense signaling and downstream gene expression
The molecular mechanism of integration of HIPVs into early defense signaling leading to JA accumulation and subsequent defense gene expression remains elusive demanding more exploration. However, the role of specific early defense regulators and components has been contemplated based on several research studies. The first event after the damage of tissues by chewing caterpillars is the influx of cytosolic Ca2+ owing to plasma trans-membrane potential (Vm) depolarization (Asai et al., 2009; Zebelo et al., 2012). Exposure to volatiles such as GLVs has shown increased cytosolic Ca2+ flux in tomato and Arabidopsis plants. The rise in cytosolic calcium ions is also accompanied by a burst of reactive oxygen species (ROS), including hydrogen peroxide (H2O2) and nitric oxide (NO) (Maffei et al., 2006). These events occurring within seconds to hour(s) of insect damage are considered as early plant defense responses. Ca2+ binding proteins like calmodulins and Ca 2+ -dependent protein kinases further integrate the signal to mitogen-activated kinases. Ye et al., 2019 reported direct induction of leucine-rich repeat receptor-like kinase genes (OsLRR-RLK1) in rice upon exposure to indole, a commonly emitted HIPV. This study also reported subsequent priming of mitogen-activated protein kinase (OsMPK3) and the WRKY transcription factor gene (OsWRKY70) for stronger expression leading to jasmonate accumulation and herbivore resistance (Ye et al., 2019). It was concluded that LRR kinases have an upstream function in perceiving the HIPVs. The activation of receptor-like kinases and apoplastic O2− and H2O2 production are supposed to be linked, but the exact connection between these links is still not clear. H2O2 accumulation is known to upregulate the genes that encode for antioxidative enzymes like superoxide dismutase, catalase, ascorbate peroxidase, glutathione reductase, and monodehydro ascorbate reductase under biotic and abiotic stress (González-Bosch, 2018). It is also anticipated that it may have specific transcription factor targets within the WRKY gene family. In maize, pre-exposure to GLVs has shown direct increased expression of ZmMAPK6 and ZmWRKY12 genes (Engelberth et al., 2013). These findings suggest that GLVs can directly induce defense genes and strengthen the jasmonate signaling pathway. Certainly, OsMPK3, OsWRKY70, and JA form a signaling cascade and positively regulate the resistance to chewing herbivores in plants (Figure 3). In addition, a clear role of reactive oxygen species in the early perception and signaling to activate plant defenses is established. Experiments with mutant plants deficient in JA biosynthesis/signaling have also validated that a functional jasmonate signaling pathway is required for HIPV-mediated defense priming in plants (Ye et al., 2019). Therefore, changes in the transcript level of defense-related genes and production of subsequent metabolites, including terpenoids, occur hour(s) to day(s) after the insect damage and comprise the late plant defense responses.
Alternate mechanisms for the molecular basis of VOC recognition have been suggested by a few studies advocating a prominent involvement of transcriptional co-repressors bound to VOCs in regulating the gene expression in plant cells (Nagashima et al., 2019). Direct binding of caryophyllene analogs (sesquiterpenes) to TPL-like proteins (encoded by NtTPLs) and dose-dependent responses upon overexpression were observed in vitro and in vivo in tobacco BY-2 cells and tobacco plants, respectively (Nagashima et al., 2019). Hence, a dual role of TPLs as co-repressors for JA-mediated signaling and as VOC-binding proteins have been proposed, which acts upstream of other transcription factors. However, to generalize this finding, more studies with other VOCs need to be performed. Nevertheless, such reports lead to suggest that plants use VOC-sensing mechanisms via nuclear proteins and not membrane-bound receptors. Therefore, the exact mechanism of sense that leads to transcriptional regulation of defense genes is an appealing topic to be explored.
Defense-related enzymes like peroxidase (POD), polyphenol oxidase (PPO), and lipoxygenase (LOX) show significantly higher expression in plants damaged by caterpillars or aphids or mechanically injured plants treated with insect oral secretions (Zebelo et al., 2014; Pingault et al., 2021). All three enzymes are components of the octadecanoid signal pathway, which regulates JA production (Felton et al., 1989). LOXs catalyze the oxidation of linolenic acid in the JA signaling pathway. PODs and LOXs are anti-oxidative enzymes that limit the nutritional quality of the plants to the insect herbivores, thereby increasing the resistance (Felton et al., 1992). PPO is also an inducible enzyme known for its defensive role against insect herbivores and pathogens (Zebelo et al., 2014). In succession to high POD activity, upregulation of several genes, including proteinase inhibitors, has been reported in tomatoes and capsicum upon herbivory by caterpillars or treatment with insect oral secretions (Damle et al., 2005; Mishra et al., 2012). In Solanaceous plants, accumulation of proteinase inhibitors (PIs) in the damaged and the undamaged leaves has been reported as one of the major consequences of mechanical wounding or insect herbivory. Generally, the responses to insect feeding have been observed to be more complex as compared to mechanical wounding owing to the elicitors present in insect OS or regurgitant (Hermsmeier et al., 2001; Mishra et al., 2012; Zebelo et al., 2014). Systemin, an 18-amino acid peptide derived from a 200-amino acid precursor prosystemin is known as proteinase inhibitor-inducing factor (Pearce et al., 1991; Orozco-Cardenas et al., 1993) in Solanaceous plants. Systemin has been identified as the systemic signal owing to its phloem mobility, and its treatment shows induced PI accumulation in plants (Narvaez-Vasquez et al., 1994). In addition, coordinated synthesis of immunoregulatory signals like ethylene, hydrogen peroxide, cytosolic calcium ion influx, and plasma membrane depolarization leading to transcriptional reprogramming by systemin has been reported (Ryan, 2000; Kandoth et al., 2007). However, peptide mediator-like systemin has not been identified in other families of plants in the context of ant–herbivore defense. A series of elicitor peptides (ZmPeps) have been reported in maize, which trigger the biosynthesis of herbivory-associated VOCs and also regulate phytohormone biosynthesis and the accumulation of transcripts related to anti-herbivore defense, including proteinase inhibitors (Huffaker et al., 2013; Huffaker, 2015).
4.4 Indirect effects of HIPVs during herbivory
HIPVs attract arthropod predators and parasitoids of herbivores acting as an indirect means to repel insect pests (Dicke et al., 1998; Reddy, 2002; Ayelo et al., 2021) (Figure 3). Parasitoids use these volatiles as cues to search for their preys. Most of the vegetative volatiles that have been identified in repelling herbivores and attracting herbivore enemies are either terpenes ((E)-β-Ocimene, (E)-β-Caryophyllene, (E)-β-Farnesene), or GLVs (Isoprene) (Unsicker et al., 2009). For example, monoterpene volatiles have been reported to repel the ovipositing females of diamondback moth, and isoprene was shown to deter the tobacco hornworm caterpillars from feeding on the isoprene-releasing transgenic tobacco lines (Laothawornkitkul et al., 2008; Wang et al., 2008). These interactions are specific to each insect–plant interaction. For instance, Cotesia marginiventris, a parasitoid of Spodoptera litura, gets attracted to Arabidopsis thaliana plants emitting (E)-β-farnesene, (E)-α-bergamotene, and other sesquiterpenes (Schnee et al., 2006). The indirect effect is extended to even vertebrate predators of herbivores in a few instances. More attacks from birds were observed on caterpillars attached to infested trees emitting terpenes, specifically, (E)-β-ocimene, linalool, and 4,8-dimethylnona-1,3,7-triene (DMNT) (Mäntylä et al., 2008). While most of the reports on the volatile attraction of herbivore enemies are on the aerial parts of the plants, emissions from roots attracting the nematodes that prey on attacking insect larvae are also known (Rasmann et al., 2005). For example, a root-feeding pest, Diuraphis noxia, induces the emission of a monoterpene volatile, 1,8-cineole, which is toxic and acts as a repellant to Coleopteran insect pests (Tripathi et al., 2001). Roots of Thuja occidentalis upon attack by Black vine weevils release volatiles that attract the entomopathogenic nematodes (Van Tol et al., 2001). However, the tri-trophic interactions resulting from volatile emissions are a result of the combined effects of above- and below-ground herbivory. Methyl salicylate, a constituent of insect-induced plant volatiles, has been reported to be very effective for the indirect defense of plants by attracting many insect predators and mites and inhibiting the oviposition of the moths (Zhu and Park, 2005; Ulland et al., 2008).
HIPVs mediate the attraction of predators and induce defense responses in neighboring plants (Baldwin and Schultz, 1983; Rhoades, 1983; Turlings and Erb et al., 2018). Maize plants exposed to GLVs from neighboring plants emit increased quantities of sesquiterpenes, thereby activating the direct defenses and attracting an important parasitoid of S. littura larvae, i.e., C. marginiventris (Ton et al., 2007). Similarly, approximately 30 volatiles including methyl salicylate and methyl benzoate have been reported from rice plants infested with S. frugiperda, which collectively result in the attraction of natural enemies of S. frugiperda such as C. marginiventris (Yuan et al., 2008). The study by Arimura et al. (2001) reported that emitted VOCs from lima- bean-infested plants by T. urticae activated the transcription of pathogenesis-related and phenylalanine ammonia-lyase genes in undamaged neighboring plants. Studies also showed where the defense of receiver plants has increased upon exposure to HIPVs in Solanaceae crops (Cascone et al., 2015; Angeles Lopez et al., 2012; Dahlin et al., 2015). A recent study by Abdala-Roberts (2022) has reported a lack of upregulation of insect resistance in receiving potato plants due to exposure to HIPVs from Colorado potato beetle-infested plants (emitter plants). Engelberth et al. (2004) has shown priming of defense responses in maize plants upon exposure to airborne VOCs. Holopainen et al. (2013) discussed the fate of HIPVs once emitted from the host plant. VOCs such as terpenoids, fatty acid esters, and methyl salicylate show different atmospheric lifetimes under different environmental conditions in the presence or absence of contaminants. Furthermore, these VOCs, when up taken or absorbed by diffusion into plant tissues, undergo reduction/oxidation reactions (metabolism), glycosylation, and glutathionylation (Matsui, 2016). Thus, responses induced by them can also vary from plant to plant and environment to environment.
5 Metabolomics as a tool to understand HIPVs and plant defense
The importance of plant VOCs are very crucial as signaling molecules in plant defense and plant–plant/plant–insect communication. Plant VOCs are the info chemicals that mediate intra- and interspecific interactions (Dudareva et al., 2013). However, several challenges concerning abiotic conditions, the lifetime of VOCs in different environments, and limitations of performing experiments at the field level to understand the actual effect of HIPVs in inducing defense in crop systems must be addressed. In recent times, metabolomics and transcriptomics analysis have been carried out to understand metabolite networking during the attack of pests especially for identifying metabolites responsible for inducing pest resistance in plants. For example, Macel et al. (2019) have identified diterpene glycosides as defensive compounds in pepper plants against thrips. Similarly, Liu et al. (2022) identified quinic acid as a metabolite that offers resistance against western flower thrips in eggplants. Progress in -omics technologies in recent times have intensified our knowledge of VOCs diversity, genes encoding enzymes that are responsible for their biosynthesis, the regulatory mechanisms involved in their formation, and downstream defense gene expression. Metabolomic analysis, including volatilome studies, must be designed and taken up in the field level studies to gain complete insight into the dynamic plasticity of HIPVs produced, defense responses induced in plants, behavioral changes in pests, allelopathic interactions within the kingdom, and cross-kingdom species. However, several technical challenges do exist in this domain including developing a single sampling method to profile both volatile and non-volatile metabolites (Maag et al., 2015).
6 Conclusion and future prospective
In this review, we try to summarize the diversity of HIPVs induced by the attack of different types of herbivores in Solanaceae crops. Furthermore, we highlight the multifunctional role of HIPVs in regulating direct and indirect defense responses in Solanaceae crops and provide a brief overview of upcoming “ omics” driven methodologies in understanding plant–herbivore interactions. Truly, these compounds act as infochemicals during herbivory in plants and mediate several interactions at different trophic levels. Metabolic engineering of floral and defense-related VOCs is a promising approach to enhance plant chemo-diversity and mediate plant–insect interactions to enhance insect resistance in crop plants. The introduction of new gene(s) or upregulation or downregulation of existing biochemical components have been some of the strategies implemented in recent studies. Constitutive overexpression of (E)-β-caryophyllene synthases in rice has shown improved above-ground plant defense by attracting parasitoids (Degenhardt et al., 2009; Xiao et al., 2012), while in maize, significant improvement in below-ground plant defense from root pests was observed on restored emission of (E)-β-caryophyllene (Degenhardt et al., 2009). The production of volatile patchoulol with sesquiterpenes in transgenic tobacco shows deterrence to tobacco hornworms, which otherwise show 20%–50% more damage to the wild-type plants (Wu et al., 2006). Overexpression of strawberry linalool/nerolidol synthase gene (FaNES1) in Arabidopsis results in high emission of linalool, thereby repelling the aphids, Myzus persicae (Aharoni et al., 2003). Transgenic tobacco plants overexpressing yeast acyl-CoA D9 desaturase or the insect acyl-CoA D11 desaturase showed elevated levels of GLV (Z)-3-hexenal, thereby leading to an increased 13-lipoxygenase activity, which regulates the defense pathway (Hong et al., 2004). Heterologous expression of a sesquiterpene synthase gene in Solanum lycopersicum (cultivated tomato) showed the production of a novel insecticidal compound that increased resistance to whiteflies and spider mites (Bleeker et al., 2012). Such reports suggest that it is possible to improve the natural plant defense mechanisms by metabolic engineering of VOCs, providing an alternate pest management strategy (Khan et al., 2000; Dudareva and Pichersky, 2008; Zhou and Jander, 2021). However, the impact of altered VOC emissions on insect behavior, effects on other tri-trophic interactions, and overall ecological implications need to be explored to a larger extent to implement these approaches in an agriculture setting. Other significant methodological challenges that VOC engineering encounters are negative effects on plant growth and development owing to the limited carbon availability, the toxicity of the volatile compounds to the non-target organisms, the formation of unpredicted compounds, and no yield of desired volatile due to lack of biosynthetic precursors (Dudareva et al., 2013). A more holistic view of plant metabolic networks is the need of the hour to engineer plant- defense-specialized metabolites to enhance insect resistance. The evolutionary context of HIPVs must also be considered when designing such modified crops. As Dicke and Baldwin (2010) suggested, these HIPVs also function beyond distress signals and rather act as infochemicals in an infochemical web in an ecosystem. Therefore, metabolic engineering to improve plant fitness is a very fruitful area for future research work.
Author contributions
The authors MM and NK have contributed equally towards collecting, interpreting, analyzing, and writing the manuscript. All authors contributed to the article and approved the submitted version.
Acknowledgments
Authors would like to thank Dr. Vishwanath Karad MIT-World Peace University for the support provided. Authors also thank Ms. Rajeshree Patil for the help received while finalizing the draft, and NK also thanks Srividya Nadimpalli for helping to make illustration related to the manuscript.
Conflict of interest
The authors declare that the research was conducted in the absence of any commercial or financial relationships that could be construed as a potential conflict of interest.
Publisher’s note
All claims expressed in this article are solely those of the authors and do not necessarily represent those of their affiliated organizations, or those of the publisher, the editors and the reviewers. Any product that may be evaluated in this article, or claim that may be made by its manufacturer, is not guaranteed or endorsed by the publisher.
References
Acevedo, F. E., Rivera-Vega, L. J., Chung, S. H., Ray, S., Felton, G. W. (2015). Cues from chewing insects–the intersection of DAMPs, HAMPs, MAMPs and effectors. Curr. Opin. Plant Biol. 26, 80–86. doi: 10.1016/j.pbi.2015.05.029
Abdala-Roberts, L., Vázquez-González, C., Rasmann, S., Moreira, X. (2022). Test of communication between potato plants in response to herbivory by the Colorado potato beetle. Agri. Forest Ent 24, 212–218. doi: 10.1111/afe.12484
Aharoni, A., Giri, A. P., Deuerlein, S., Griepink, F., de Kogel, W. J., Verstappen, F. W., et al. (2003). Terpenoid metabolism in wild-type and transgenic arabidopsis plants. Plant Cell. 15, 2866–2884. doi: 10.1105/tpc.016253
Alborn, H. T., Turlings, T. C. J., Jones, T., Stenhagen, G., Loughrin, J. H., Tumlinson, J. H. (1997). An elicitor of plant volatiles from beet armyworm oral secretion. Science 276, 945–949. doi: 10.1126/science.276.5314.945
Ali, M. Y., Naseem, T., Zhang, J., Pan, M., Zhang, F., Liu, T. X. (2022). Plant volatiles and herbivore induced plant volatiles from chili pepper act as attractant of the aphid parasitoid Aphelinus varipes (Hymenoptera: aphelinidae). Plants. 11, 1350. doi: 10.3390/plants11101350
Ament, K., Kant, M. R., Sabelis, M. W., Haring, M. A., Schuurink, R. C. (2004). Jasmonic acid is a key regulator of spider mite-induced volatile terpenoid and methyl salicylate emission in tomato. Plant Physiol. 135, 2025–2037. doi: 10.1104/pp.104.048694
Ameye, M., Allmann, S., Verwaeren, J., Smagghe, G., Haesaert, G., Schuurink, R. C., et al. (2017). Green leaf volatile production by plants: a meta-analysis. New Phytologist. 220, 666–683. doi: 10.1111/nph.14671
Ángeles López, Y. I., Martínez-Gallardo, N. A., Ramírez-Romero, R., López, M. G., Sánchez-Hernández, C., Délano-Frier, J. P. (2012). Cross-kingdom effects of plant-plant signaling via volatile organic compounds emitted by tomato (Solanum lycopersicum) plants infested by the greenhouse whitefly (Trialeurodes vaporariorum). J. Chem. Ecol. 38 (11), pp.1376–1386. doi: 10.1007/s10886-012-0201-z
Antonious, G. F., Kochhar, T. S. (2003). Zingiberene and curcumene in wild tomato. J. Environ. Sci. Health B. 38, 489–500. doi: 10.1081/PFC-120021668
Arce, C. M., Besomi, G., Glauser, G., Turlings, T. C. (2021). Caterpillar-induced volatile emissions in cotton: the relative importance of damage and insect-derived factors. Front. Plant Sci. 12, 1503. doi: 10.3389/fpls.2021.709858
Arimura, G. I., Garms, S., Maffei, M., Bossi, S., Schulze, B., Leitner, M., et al. (2008a). Herbivore-induced terpenoid emission in Medicago truncatula: concerted action of jasmonate, ethylene and calcium signaling. Planta. 227, 453–464. doi: 10.1007/s00425-007-0631-y
Arimura, G. I., Kopke, S., Kunert, M., Volpe, V., David, A., Brand, P., et al. (2008b). Effects of feeding spodoptera littoralis on lima bean leaves: IV. diurnal and nocturnal damage differentially initiate plant volatile emission. Plant Physiol. 146, 965–973. doi: 10.1104/pp.107.111088
Arimura, G. I., Matsui, K., Takabayashi, J. (2009). Chemical and molecular ecology of herbivore-induced plant volatiles: proximate factors and their ultimate functions. Plant Cell Physiol. 50, 911–923. doi: 10.1093/pcp/pcp030
Arimura, G. I., Ozawa, R., Horiuchi, J. I., Nishioka, T., Takabayashi, J. (2001). Plant–plant interactions mediated by volatiles emitted from plants infested by spider mites. Biochem. System. Ecol. 29, 1049–1061. doi: 10.1016/S0305-1978(01)00049-7
Asai, N., Nishioka, T., Takabayashi, J., Furuichi, T. (2009). Plant volatiles regulate the activities of Ca2+-permeable channels and promote cytoplasmic calcium transients in arabidopsis leaf cells. Plant Signal. Behav. 4, 294–300. doi: 10.4161/psb.4.4.8275
Ayelo, P. M., Yusuf, A. A., Pirk, C. W., Chailleux, A., Mohamed, S. A., Deletre, E. (2021). Terpenes from herbivore-induced tomato plant volatiles attract Nesidiocoris tenuis (Hemiptera: miridae), a predator of major tomato pests. Pest Manage. Sci. 77, 5255–5267. doi: 10.1002/ps.6568
Baldwin, I. T., Schultz, J. C. (1983). Rapid changes in tree leaf chemistry induced by damage: evidence for communication between plants. Science. 221, 277–279. doi: 10.1126/science.221.4607.277
Bartram, S., Jux, A., Gleixner, G., Boland, W. (2006). Dynamic pathway allocation in early terpenoid biosynthesis of stress-induced lima bean leaves. Phytochem. 67, 1661–1672. doi: 10.1016/j.phytochem.2006.02.004
Bate, N. J., Rothstein, S. J. (1998). C6-volatiles derived from the lipoxygenase pathway induce a subset of defense-related genes. Plant J. 16, 561–569. doi: 10.1046/j.1365-313x.1998.00324.x
Bensoussan, N., Santamaria, M. E., Zhurov, V., Diaz, I., Grbić, M., Grbić, V. (2016). Plant-herbivore interaction: dissection of the cellular pattern of Tetranychus urticae feeding on the host plant. Front. Plant Sci. 7, 1105. doi: 10.3389/fpls.2016.01105
Bleeker, P. M., Diergaarde, P. J., Ament, K., Guerra, J., Weidner, M., Schutz, S., et al. (2009). The role of specific tomato volatiles in tomato-whitefly interaction. Plant Physiol. 151, 925–935. doi: 10.1104/pp.109.142661
Bleeker, P. M., Diergaarde, P. J., Ament, K., Schütz, S., Johne, B., Dijkink, J., et al. (2011). Tomato-produced 7-epizingiberene and r-curcumene act as repellents to whiteflies. Phytochem 72, 68–73. doi: 10.1016/j.phytochem.2010.10.014
Bleeker, P. M., Mirabella, R., Diergaarde, P. J., VanDoorn, A., Tissier, A., Kant, M. R., et al. (2012). Improved herbivore resistance in cultivated tomato with the sesquiterpene biosynthetic pathway from a wild relative. Proc. Nat. Acad. Sci. 109, 20124–20129. doi: 10.1073/pnas.1208756109
Bohlmann, J., Meyer-Gauen, G., Croteau, R. (1998). Plant terpenoid synthases: molecular biology and phylogenetic analysis. Proc. Nat. Acad. Sci. 95, 4126–4133. doi: 10.1073/pnas.95.8.4126
Bouwmeester, H. J., Verstappen, F. W., Posthumus, M. A., Dicke, M. (1999). Spider mite-induced (3 s)-(E)-nerolidol synthase activity in cucumber and lima bean. the first dedicated step in acyclic C11-homoterpene biosynthesis. Plant Physiol. 121, 173–180. doi: 10.1104/pp.121.1.173
Bowers, W. S., Nault, L. R., Webb, R. E., Dutky, S. R. (1972). Aphid alarm pheromone: isolation, identification, synthesis. Science. 177, 1121–1122. doi: 10.1126/science.177.4054.1121
Caparros Megido, R., De Backer, L., Ettaïb, R., Brostaux, Y., Fauconnier, M. L., Delaplace, P., et al. (2014). Role of larval host plant experience and solanaceous plant volatile emissions in Tuta absoluta (Lepidoptera: gelechiidae) host finding behavior. Arthro-Plant Interact. 8, 293–304. doi: 10.1007/s11829-014-9315-2
Cascone, P., Iodice, L., Maffei, M. E., Bossi, S., Arimura, G. I., Guerrieri, E. (2015). Tobacco overexpressing β-ocimene induces direct and indirect responses against aphids in receiver tomato plants. J. Plant Physiol. 173, 28–32. doi: 10.1016/j.jplph.2014.08.011
Chen, G., Klinkhamer, P. G., Escobar-Bravo, R., Leiss, K. A. (2018). Type VI glandular trichome density and their derived volatiles are differently induced by jasmonic acid in developing and fully developed tomato leaves: implications for thrips resistance. Plant Sci. 276, 87–98. doi: 10.1016/j.plantsci.2018.08.007
Chen, L., Li, X., Zhang, J., He, T., Huang, J., Zhang, Z., et al. (2021). Comprehensive metabolome and volatilome analyses in eggplant and tomato reveal their differential responses to Tuta absoluta infestation. Front. Plant Sci. 12, 757230. doi: 10.3389/fpls.2021.757230
Clavijo McCormick, A., Unsicker, S. B., Gershenzon, J. (2012). The specificity of herbivore-induced plant volatiles in attracting herbivore enemies. Trends Plant Sci. 17, 303–310. doi: 10.1016/j.tplants.2012.03.012
Dahlin, I., Vucetic, A., Ninkovic, V. (2015). Changed host plant volatile emissions induced by chemical interaction between unattacked plants reduce aphid plant acceptance with intermorph variation. J. Pest Science. 88, 249–257. doi: 10.1007/s10340-014-0625-z
Damle, M. S., Giri, A. P., Sainani, M. N., Gupta, V. S. (2005). Higher accumulation of proteinase inhibitors in flowers than leaves and fruits as a possible basis for differential feeding preference of Helicoverpa armigera on tomato (Lycopersicon esculentum Mill, Cv. Dhanashree). Phytochem. 66, 2659–2667. doi: 10.1016/j.phytochem.2005.09.006
Danner, H., Brown, P., Cator, E. A., Harren, F. J., van Dam, N. M., Cristescu, S. M. (2015). Aboveground and belowground herbivores synergistically induce volatile organic sulfur compound emissions from shoots but not from roots. J. Chem. Ecol. 41, 631–640. doi: 10.1007/s10886-015-0601-y
Darshanee, H. L., Ren, H., Ahmed, N., Zhang, Z. F., Liu, Y. H., Liu, T. X. (2017). Volatile-mediated attraction of greenhouse whitefly Trialeurodes vaporariorum to tomato and eggplant. Front. Plant Sci. 8, 1285. doi: 10.3389/fpls.2017.01285
D’Auria, J. C., Pichersky, E., Schaub, A., Hansel, A., Gershenzon, J. (2007). Characterization of a BAHD acyltransferase responsible for producing the green leaf volatile (Z) -3-hexen-1-yl acetate in Arabidopsis thaliana. Plant J. 49, 194–207. doi: 10.1111/j.1365-313X.2006.02946.x
Davidson-Lowe, E., Ali, J. G. (2021). Herbivore-induced plant volatiles mediate behavioral interactions between a leaf-chewing and a phloem-feeding herbivore. Basic Appl. Ecol. 53, 39–48. doi: 10.1016/j.baae.2021.03.005
Degenhardt, J., Hiltpold, I., Köllner, T. G., Frey, M., Gierl, A., Gershenzon, J., et al. (2009). Restoring a maize root signal that attracts insect-killing nematodes to control a major pest. Proc. Nat. Acad. Sci. 106, 13213–13218. doi: 10.1073/pnas.0906365106
Delphia, C. M., Mescher, M. C., De Moraes, C. M. (2007). Induction of plant volatiles by herbivores with different feeding habits and the effects of induced defenses on host-plant selection by thrips. J. Chem. Ecol. 33, 997–1012. doi: 10.1007/s10886-007-9273-6
Dicke, M., Baldwin, I. T. (2010). The evolutionary context for herbivore-induced plant volatiles: beyond the ‘cry for help’. Trends. Plant Sci. 15, 167–175. doi: 10.1016/j.tplants.2009.12.002
Dicke, M., Takabayashi, J., Posthumus, M. A., Schütte, C., Krips, O. E. (1998). Plant–phytoseiid interactions mediated by herbivore-induced plant volatiles: variation in production of cues and responses of predatory mites. Exper. Applied. Acarology 22, 311–333. doi: 10.1023/A:1024528507803
Dicke, M., Van Loon, J. J., Soler, R. (2009). Chemical complexity of volatiles from plants induced by multiple attack. Nat. Chem. Biol. 5, 317–324. doi: 10.1038/nchembio.169
Digilio, M. C., Cascone, P., Iodice, L., Guerrieri, E. (2012). Interactions between tomato volatile organic compounds and aphid behaviour. J. Plant Interact. 7, 322–325. doi: 10.1080/17429145.2012.727104
Disi, J. O., Zebelo, S., Ngumbi, E., Fadamiro, H. Y. (2017). cis-Jasmone primes defense pathways in tomato via emission of volatile organic compounds and regulation of genes with consequences for Spodoptera exigua oviposition. Arthropod-Plant Interact. 11, 591–602.
Dudareva, N., Klempien, A., Muhlemann, J. K., Kaplan, I. (2013). Biosynthesis, function and metabolic engineering of plant volatile organic compounds. New Phytol. 198, 16–32. doi: 10.1111/nph.12145
Dudareva, N., Martin, D., Kish, C. M., Kolosova, N., Gorenstein, N., Faüldt, J., et al. (2003). (E)-β-Ocimene and myrcene synthase genes of floral scent biosynthesis in snapdragon: function and expression of three terpene synthase genes of a new terpene synthase subfamily. Plant Cell 15, 1227–1241. doi: 10.1105/tpc.011015
Dudareva, N., Negre, F., Nagegowda, D. A., Orlova, I. (2006). Plant volatiles: recent advances and future perspectives. Crit. Rev. Plant Sci. 25, 417–440. doi: 10.1080/07352680600899973
Dudareva, N., Pichersky, E. (2008). Metabolic engineering of plant volatiles. Curr. Opin. Biotech. 19, 181–189. doi: 10.1016/j.copbio.2008.02.011
Dudareva, N., Pichersky, E., Gershenzon, J. (2004). Biochemistry of plant volatiles. Plant Physiol. 135, 1893–1902. doi: 10.1104/pp.104.049981
Duran-Flores, D., Heil, M. (2016). Sources of specificity in plant damaged-self recognition. Curr. Opin. Plant Biol. 32, 77–87. doi: 10.1016/j.pbi.2016.06.019
Engelberth, J., Alborn, H. T., Schmelz, E. A., Tumlinson, J. H. (2004). Airborne signals prime plants against insect herbivore attack. Proc. Nat. Acad. Sci. 101, 1781–1785. doi: 10.1073/pnas.0308037100
Engelberth, J., Contreras, C. F., Dalvi, C., Li, T., Engelberth, M. (2013). Early transcriptome analyses of z-3-hexenol-treated Zea mays revealed distinct transcriptional networks and anti-herbivore defense potential of green leaf volatiles. PloS One 8 (10), e77465. doi: 10.1371/journal.pone.0077465
Erb, M., Veyrat, N., Robert, C. A., Xu, H., Frey, M., Ton, J., et al. (2015). Indole is an essential herbivore-induced volatile priming signal in maize. Nature. Commun. 6, 1–10. doi: 10.1038/ncomms7273
Escobar-Bravo, R., Klinkhamer, P. G., Leiss, K. A. (2017). Induction of jasmonic acid-associated defenses by thrips alters host suitability for conspecifics and correlates with increased trichome densities in tomato. Plant Cell Physiol. 58, 622–634. doi: 10.1093/pcp/pcx014
Falara, V., Alba, J. M., Kant, M. R., Schuurink, R. C., Pichersky, E. (2014). Geranyllinalool synthases in solanaceae and other angiosperms constitute an ancient branch of diterpene synthases involved in the synthesis of defensive compounds. Plant Physiol. 166, 428–441. doi: 10.1104/pp.114.243246
Farag, M. A., Fokar, M., Abd, H., Zhang, H., Allen, R. D., Pare, P. W. (2005). (Z)-3-Hexenol induces defense genes and downstream metabolites in maize. Planta 220, 900–909. doi: 10.1007/s00425-004-1404-5
Farag, M., Paré, P. W. (2002). C6-green leaf volatiles trigger local and systemic VOC emissions in tomato. Phytochem. 61, 545–554. doi: 10.1016/S0031-9422(02)00240-6
Felton, G. W., Donato, K. K., Broadway, R. M., Duffey, S. S. (1992). Impact of oxidized plant phenolics on the nutritional quality of dietary protein to a noctuid herbivore, Spodoptera exigua. J. Insect Physiol. 38 (4), 277–285. doi: 10.1016/0022-1910(92)90128-Z
Felton, G. W., Donato, K., Del Vecchio, R. J., Duffey, S. S. (1989). Activation of plant foliar oxidases by insect feeding reduces nutritive quality of foliage for noctuid herbivores. J. Chem. Ecol. 15, 2667–2694. doi: 10.1007/BF01014725
Feussner, I., Wasternack, C. (2002). The lipoxygenase pathway. Annu. Rev. Plant Biol. 53, 275. doi: 10.1146/annurev.arplant.53.100301.135248
Freundlich, G. E., Shields, M., Frost, C. J. (2021). Dispensing a synthetic green leaf volatile to two plant species in a common garden differentially alters physiological responses and herbivory. Agronomy 11, 958. doi: 10.3390/agronomy11050958
Fridman, E., Wang, J., Iijima, Y., Froehlich, J. E., Gang, D. R., Ohlrogge, J., et al. (2005). Metabolic, genomic, and biochemical analyses of glandular trichomes from the wild tomato species Lycopersicon hirsutum identify a key enzyme in the biosynthesis of methylketones. Plant Cell 17, 1252–1267. doi: 10.1105/tpc.104.029736
Fürstenberg-Hägg, J., Zagrobelny, M., Bak, S. (2013). Plant defense against insect herbivores. Int. J. Mol. Sci. 14, 10242–10297. doi: 10.3390/ijms140510242
Ghosh, R., Metze, D., Shaikh, M., Deshpande, A., Firake, D. M., Pandit, S. (2022). Chemical ecology of Himalayan eggplant variety’s antixenosis: identification of geraniol as an oviposition deterrent against the eggplant shoot and fruit borer. bioRxiv. doi: 10.1101/2022.04.28.489959
Gibson, R. W., Pickett, J. A. (1983). Wild potato repels aphids by release of aphid alarm pheromone. Nature 302, 608–609. doi: 10.1038/302608a0
González-Bosch, C. (2018). Priming plant resistance by activation of redox-sensitive genes. Free Rad. Biol. Med. 122, 171–180. doi: 10.1016/j.freeradbiomed.2017.12.028
Gosset, V., Harmel, N., Göbel, C., Francis, F., Haubruge, E., Wathelet, J. P., et al. (2009). Attacks by a piercing-sucking insect (Myzus persicae sultzer) or a chewing insect (Leptinotarsa decemlineata say) on potato plants (Solanum tuberosum l.) induce differential changes in volatile compound release and oxylipin synthesis. J. Exp. Bot. 60 (4), 1231–1240. doi: 10.1093/jxb/erp015
Grechkin, A. (1998). Recent developments in biochemistry of the plant lipoxygenase pathway. Prog. Lipid Res. 37, 317–352. doi: 10.1016/S0163-7827(98)00014-9
Halitschke, R., Schittko, U., Pohnert, G., Boland, W., Baldwin, I. T. (2001). Molecular interactions between the specialist herbivore Manduca sexta (Lepidoptera, sphingidae) and its natural host Nicotiana attenuata. III. fatty acid-amino acid conjugates in herbivore oral secretions are necessary and sufficient for herbivore-specific plant responses. Plant Physiol. 125, 711–717. doi: 10.1104/pp.125.2.711
Halitschke, R., Stenberg, J. A., Kessler, D., Kessler, A., Baldwin, I. T. (2008). Shared signals–’alarm calls’ from plants increase apparency to herbivores and their enemies in nature. Ecol. Lett. 11, 24–34. doi: 10.1111/j.1461-0248.2007.01123.x
Harmel, N., Almohamad, R., Fauconnier, M. L., Du Jardin, P., Verheggen, F., Marlier, M., et al. (2007). Role of terpenes from aphid-infested potato on searching and oviposition behavior of Episyrphus balteatus. Insect Sci. 14, 57–63. doi: 10.1111/j.1744-7917.2007.00126.x
Harrewijn, P., Piron, P. G. (1995). “Resistance of plants (including wild plants) to aphids and thrips,” in Meeting of the Swiss Society of Phytomedicine. Zürich, Switzerland, SGP Info 3/95, 6–11.
Heil, M. (2009). Damaged-self recognition in plant herbivore defence. Trends. Plant Sci. 14, 356–363. doi: 10.1016/j.tplants.2009.04.002
Hermsmeier, D., Schittko, U., Baldwin, I. T. (2001). Molecular interactions between the specialist herbivore manduca sexta (Lepidoptera, sphingidae) and its natural host nicotiana attenuata. i. Large-scale changes in the accumulation of growth-and defense-related plant mRNAs. Plant Physiol. 125, 683–700. doi: 10.1104/pp.125.2.683
Holopainen, J. K., Nerg, A. M., Blande, J. D. (2013). “Multitrophic signalling in polluted atmospheres,” in Biology, controls and models of tree volatile organic compound emissions (Dordrecht: Springer), 285–314.
Hong, M., Zilinskas, B. A., Knipple, D. C., Chin, C. K. (2004). Cis-3-Hexenal production in tobacco is stimulated by 16-carbon monounsaturated fatty acids. Phytochem. 65, 159–168. doi: 10.1016/j.phytochem.2003.10.013
Howe, G. A., Lee, G. I., Itoh, A., Li, L., DeRocher, A. E. (2000). Cytochrome P450-dependent metabolism of oxylipins in tomato. cloning and expression of allene oxide synthase and fatty acid hydroperoxide lyase. Plant Physiol. 123, 711–724. doi: 10.1104/pp.123.2.711
Hu, L., Zhang, K., Wu, Z., Xu, J., Erb, M. (2021). Plant volatiles as regulators of plant defense and herbivore immunity: molecular mechanisms and unanswered questions. Cur. Opin. Insect Sci. 44, 82–88. doi: 10.1016/j.cois.2021.03.010
Huang, W., Gfeller, V., Erb, M. (2019). Root volatiles in plant–plant interactions II: Root volatiles alter root chemistry and plant–herbivore interactions of neighbouring plants. Plant, Cell & Environ 42, 1964–1973. doi: 10.1111/pce.13534
Huffaker, A. (2015). Plant elicitor peptides in induced defense against insects. Cur. Opin. Insect Sci. 9, 44–50. doi: 10.1016/j.cois.2015.06.003
Huffaker, A., Pearce, G., Veyrat, N., Erb, M., Turlings, T. C., Sartor, R., et al. (2013). Plant elicitor peptides are conserved signals regulating direct and indirect antiherbivore defense. Proc. Nat. Acad. Sci. 110, 5707–5712. doi: 10.1073/pnas.1214668110
Humphreys, J. M., Chapple, C. (2002). Rewriting the lignin roadmap. Curr. Opin. Plant Biol. 5, 224–229. doi: 10.1016/S1369-5266(02)00257-1
Kandoth, P. K., Ranf, S., Pancholi, S. S., Jayanty, S., Walla, M. D., Miller, W., et al. (2007). Tomato MAPKs LeMPK1, LeMPK2, and LeMPK3 function in the systemin-mediated defense response against herbivorous insects. Proc. Nat. Acad. Sci. 104, 12205–12210. doi: 10.1073/pnas.0700344104
Kant, M. R., Ament, K., Sabelis, M. W., Haring, M. A., Schuurink, R. C. (2004). Differential timing of spider mite-induced direct and indirect defenses in tomato plants. Plant Physiol. 135, 483–495. doi: 10.1104/pp.103.038315
Kant, M. R., Bleeker, P. M., Van Wijk, M., Schuurink, R. C., Haring, M. A. (2009). Plant volatiles in defence. Adv. botanical Res. 51, 613–666. doi: 10.1016/S0065-2296(09)51014-2
Khan, Z. R., Pickett, J. A., Berg, J. V. D., Wadhams, L. J., Woodcock, C. M. (2000). Exploiting chemical ecology and species diversity: stem borer and striga control for maize and sorghum in Africa. Pest Manage. Science: Formerly Pesticide Sci. 56, 957–962. doi: 10.1002/1526-4998(200011)56:11<957::AID-PS236>3.0.CO;2-T
Kim, J., Felton, G. W. (2013). Priming of antiherbivore defensive responses in plants. Insect Sci. 20, 273–285. doi: 10.1111/j.1744-7917.2012.01584.x
Kim, I. S., Grosch, W. (1981). Partial purification and properties of a hydroperoxide lyase from fruits of pear. J. Agric. Food Chem. 29, 1220–1225. doi: 10.1021/jf00108a030
Knudsen, J. T., Eriksson, R., Gershenzon, J., Ståhl, B. (2006). Diversity and distribution of floral scent. Bot. Rev. 72 (1), 1–120. doi: 10.1663/0006-8101(2006)72[1:DADOFS]2.0.CO;2
Koschier, E. H., De Kogel, W. J., Visser, J. H. (2000). Assessing the attractiveness of volatile plant compounds to western flower thrips Frankliniella occidentalis. J. Chem. Ecol. 26, 2643–2655. doi: 10.1023/A:1026470122171
Landolt, P. J., Tumlinson, J. H., Alborn, D. H. (1999). Attraction of Colorado potato beetle (Coleoptera: chrysomelidae) to damaged and chemically induced potato plants. Environ. Entomol. 28, 973–978. doi: 10.1093/ee/28.6.973
Laothawornkitkul, J., Paul, N. D., Vickers, C. E., Possell, M., Taylor, J. E., Mullineaux, P. M., et al. (2008). Isoprene emissions influence herbivore feeding decisions. Plant Cell Environ. 31, 1410–1415. doi: 10.1111/j.1365-3040.2008.01849.x
Leitner, M., Boland, W., Mithöfer, A. (2005). Direct and indirect defences induced by piercing-sucking and chewing herbivores in Medicago truncatula. New Phytol. 167, 597–606. doi: 10.1111/j.1469-8137.2005.01426.x
Lin, Y., Hussain, M., Avery, P. B., Qasim, M., Fang, D., Wang, L. (2016). Volatiles from plants induced by multiple aphid attacks promote conidial performance of Lecanicillium lecanii. PloS One 11, e0151844. doi: 10.1371/journal.pone.0151844
Liu, Y., Wang, X., Luo, S., Ma, L., Zhang, W., Xuan, S., et al. (2022). Metabolomic and transcriptomic analyses identify quinic acid protecting eggplant from damage caused by western flower thrips. Pest Manage. Sci. 78, 5113–5123. doi: 10.1002/ps.7129
Maag, D., Erb, M., Glauser, G. (2015). Metabolomics in plant–herbivore interactions: challenges and applications. Entomol. Exper. Applicat. 157, 18–29. doi: 10.1111/eea.12336
Macel, M., Visschers, I. G., Peters, J. L., Kappers, I. F., de Vos, R. C., van Dam, N. M. (2019). Metabolomics of thrips resistance in pepper (Capsicum spp.) reveals monomer and dimer acyclic diterpene glycosides as potential chemical defenses. J. Chem. Ecol. 45, 490–501. doi: 10.1007/s10886-019-01074-4
Maffei, M. E., Mithoüfer, A., Arimura, G. I., Uchtenhagen, H., Bossi, S., Bertea, C. M., et al. (2006). Effects of feeding spodoptera littoralis on lima bean leaves. III. membrane depolarization and involvement of hydrogen peroxide. Plant Physiol. 140, 1022–1035. doi: 10.1104/pp.105.071993
Mäntylä, E., Alessio, G. A., Blande, J. D., Heijari, J., Holopainen, J. K., Laaksonen, T., et al. (2008). From plants to birds: higher avian predation rates in trees responding to insect herbivory. PloS One 3, e2832. doi: 10.1371/journal.pone.0002832
Matsui, K. (2016). A portion of plant airborne communication is endorsed by uptake and metabolism of volatile organic compounds. Cur. Opin. Plant Biol. 32, 24–30. doi: 10.1016/j.pbi.2016.05.005
Matsui, K., Kaji, Y., Kajiwara, T., Hatanaka, A. (1996). Developmental changes of lipoxygenase and fatty acid hydroperoxide lyase activities in cultured cells of marchantia polymorpha. Phytochem. 41, 177–182. doi: 10.1016/0031-9422(95)00560-9
Mattiacci, L., Dicke, M., Posthumus, M. A. (1995). Beta-glucosidase: an elicitor of herbivore-induced plant odor that attracts host-searching parasitic wasps. Proc. Nat. Acad. Sci. 92, 2036–2040. doi: 10.1073/pnas.92.6.2036
McGarvey, D. J., Croteau, R. (1995). Terpenoid metabolism. Plant Cell 7, 1015–26. doi: 10.1105/tpc.7.7.1015
Meents, A. K., Mithöfer, A. (2020). Plant–plant communication: is there a role for volatile damage-associated molecular patterns? Front. Plant Sci. 11, 583275. doi: 10.3389/fpls.2020.583275
Mercke, P., Kappers, I. F., Verstappen, F. W., Vorst, O., Dicke, M., Bouwmeester, H. J. (2004). Combined transcript and metabolite analysis reveals genes involved in spider mite induced volatile formation in cucumber plants. Plant Physiol. 135, 2012–2024. doi: 10.1104/pp.104.048116
Mishra, M., Mahajan, N., Tamhane, V. A., Kulkarni, M. J., Baldwin, I. T., Gupta, V. S., et al. (2012). Stress inducible proteinase inhibitor diversity in Capsicum annuum. BMC Plant Biol. 12, 1–14. doi: 10.1186/1471-2229-12-217
Mithöfer, A., Wanner, G., Boland, W. (2005). Effects of feeding spodoptera littoralis on lima bean leaves. II. continuous mechanical wounding resembling insect feeding is sufficient to elicit herbivory-related volatile emission. Plant Physiol. 137, 1160–1168. doi: 10.1104/pp.104.054460
Mithöfer, A., Schulze, B., Boland, W. (2004). Biotic and heavy metal stress response in plants: evidence for common signals. FEBS Lett. 566, 1–5. doi: 10.1016/j.febslet.2004.04.011
Mori, N., Alborn, H. T., Teal, P. E., Tumlinson, J. H. (2001). Enzymatic decomposition of elicitors of plant volatiles in heliothis virescens and helicoverpa zea. J. Insect Physiol. 47, 749–757. doi: 10.1016/S0022-1910(00)00171-2
Muhlemann, J. K., Klempien, A., Dudareva, N. (2014). Floral volatiles: from biosynthesis to function. Plant Cell Environ. 37, 1936–1949. doi: 10.1111/pce.12314
Mumm, R., Dicke, M. (2010). Variation in natural plant products and the attraction of bodyguards involved in indirect plant defense. Canad. J. Zoo. 88, 628–667. doi: 10.1139/Z10-032
Murungi, L. K., Kirwa, H., Salifu, D., Torto, B. (2016). Opposing roles of foliar and glandular trichome volatile components in cultivated nightshade interaction with a specialist herbivore. PloS One 11, e0160383. doi: 10.1371/journal.pone.0160383
Nagashima, A., Higaki, T., Koeduka, T., Ishigami, K., Hosokawa, S., Watanabe, H., et al. (2019). Transcriptional regulators involved in responses to volatile organic compounds in plants. J. Biol. Che. 294, 2256–2266. doi: 10.1074/jbc.RA118.005843
Nagegowda, D. A., Gupta, P. (2020). Advances in biosynthesis, regulation, and metabolic engineering of plant specialized terpenoids. Plant Sci. 294, 110457. doi: 10.1016/j.plantsci.2020.110457
Narvaez-Vasquez, J., Orozco-Cardenas, M. L., Ryan, C. A. (1994). A sulfhydryl reagent modulates systemic signaling for wound-induced and systemin-induced proteinase inhibitor synthesis. Plant Physiol. 105, 725–730. doi: 10.1104/pp.105.2.725
Nusra, M. S. F., Udukala, D. N., Amarasinghe, L. D., Paranagama, P. A. (2021). Volatiles from host plant brinjal attract the brinjal fruit and shoot borer-leucinodes orbonalis guenee. J. Asia-Pacific Entomol. 24, 695–703. doi: 10.1016/j.aspen.2021.06.002
Orozco-Cardenas, M., McGurl, B., Ryan, C. A. (1993). Expression of an antisense prosystemin gene in tomato plants reduces resistance toward manduca sexta larvae. Proc. Nat. Acad. Sci. 90, 8273–8276. doi: 10.1073/pnas.90.17.8273
Pearce, G., Strydom, D., Johnson, S., Ryan, C. A. (1991). A polypeptide from tomato leaves induces wound-inducible proteinase inhibitor proteins. Science 253, 895–897. doi: 10.1126/science.253.5022.895
Perring, T. M., Stansly, P. A., Liu, T. X., Smith, H. A., Andreason, S. A. (2018). “Whiteflies: biology, ecology, and management,” in Sustainable management of arthropod pests of tomato (Academic Press), 73–110.
Pingault, L., Varsani, S., Palmer, N., Ray, S., Williams, W. P., Luthe, D. S., et al. (2021). Transcriptomic and volatile signatures associated with maize defense against corn leaf aphid. BMC Plant Biol. 21, 1–15. doi: 10.1186/s12870-021-02910-0
Poczai, P., D’Agostino, N., Deanna, R., Portis, E. (2022). Editorial: solanaceae VII: biology, genetics, and evolution. Front. Genet. 13, 932421. doi: 10.3389/fgene.2022.932421
Poulter, C. D., Wiggins, P. L., Le, A. T. (1981). Farnesylpyrophosphate synthetase. a stepwise mechanism for the 1’-4 condensation reaction. J. Am. Chem. Soc. 103, 3926–3927. doi: 10.1021/ja00403a054
Qi, J., ul Malook, S., Shen, G., Gao, L., Zhang, C., Li, J., et al. (2018). Current understanding of maize and rice defense against insect herbivores. Plant Diversity 40, 189–195. doi: 10.1016/j.pld.2018.06.006
Quintana-Rodriguez, E., Duran-Flores, D., Heil, M., Camacho-Coronel, X. (2018). Damage-associated molecular patterns (DAMPs) as future plant vaccines that protect crops from pests. Sci. hort. 237, 207–220. doi: 10.1016/j.scienta.2018.03.026
Rasmann, S., Köllner, T. G., Degenhardt, J., Hiltpold, I., Toepfer, S., Kuhlmann, U., et al. (2005). Recruitment of entomopathogenic nematodes by insect-damaged maize roots. Nature 434, 732–737. doi: 10.1038/nature03451
Reddy, G. V. P. (2002). Plant volatiles mediate orientation and plant preference by the predator chrysoperla carnea stephens (Neuroptera: chrysopidae). Biol. Control 25 (1), 49–55. doi: 10.1016/S1049-9644(02)00038-5
Rhoades, H. L. (1983). Effects of cover crops and fallowing on populations of Belonolaimus longicaudatus and Meloidogyne incognita and subsequent crop yields. Nematropica 13, 9–16.
Rodriguez-Saona, C., Crafts-Brandner, S. J., Cañas, L. A. (2003). Volatile emissions triggered by multiple herbivore damage: beet armyworm and whitefly feeding on cotton plants. J. Chem. Ecol. 29, 2539–2550. doi: 10.1023/A:1026314102866
Rowen, E., Kaplan, I. (2016). Eco-evolutionary factors drive induced plant volatiles: a meta-analysis. New Phytol. 210, 284–294. doi: 10.1111/nph.13804
Ryan, C. A. (2000). The systemin signaling pathway: differential activation of plant defensive genes. Biochem. Biophys. Acta (BBA)-Prot. Struct. Mol. Enzy. 1477, 112–121. doi: 10.1016/S0167-4838(99)00269-1
Samuels, J. (2015). Biodiversity of food species of the solanaceae family: a preliminary taxonomic inventory of subfamily solanoideae. Resources 4, 277–322. doi: 10.3390/resources4020277
Schettino, M., Grasso, D. A., Weldegergis, B. T., Castracani, C., Mori, A., Dicke, M., et al. (2017). Response of a predatory ant to volatiles emitted by aphid-and caterpillar-infested cucumber and potato plants. J. Chem. Ecol. 43, 1007–1022. doi: 10.1007/s10886-017-0887-z
Schmelz, E. A., LeClere, S., Carroll, M. J., Alborn, H. T., Teal, P. E. (2007). Cowpea chloroplastic ATP synthase is the source of multiple plant defense elicitors during insect herbivory. Plant Physiol. 144, 793–805. doi: 10.1104/pp.107.097154
Schnee, C., Köllner, T. G., Held, M., Turlings, T. C. J., Gershenzon, J., Degenhardt, J. (2006). The products of a single maize sesquiterpene synthase form a volatile defense signal that attracts natural enemies of maize herbivores. Proc. Natl. Acad. Sci. U.S.A. 103, 1129–1134. doi: 10.1073/pnas.0508027103
Seo, H. S., Song, J. T., Cheong, J. J., Lee, Y. H., Lee, Y. W., Hwang, I., et al. (2001). Jasmonic acid carboxyl methyltransferase: a key enzyme for jasmonate-regulated plant responses. Proc. Nat. Acad. Sci. 98, 4788–4793. doi: 10.1073/pnas.081557298
Sharkey, T. D., Yeh, S., Wiberley, A. E., Falbel, T. G., Gong, D., Fernandez, D. E. (2005). Evolution of the isoprene biosynthetic pathway in kudzu. Plant Physiol. 137, 700–712. doi: 10.1104/pp.104.054445
Silva, D. B., Weldegergis, B. T., Van Loon, J. J., Bueno, V. H. (2017). Qualitative and quantitative differences in herbivore-induced plant volatile blends from tomato plants infested by either Tuta absoluta or bemisia tabaci. J. Chem. Ecol. 43, 53–65. doi: 10.1007/s10886-016-0807-7
Simmonds, M. S. (2001). Importance of flavonoids in insect–plant interactions: feeding and oviposition. Phytochem. 56, 245–252. doi: 10.1016/S0031-9422(00)00453-2
Sobhy, I. S., Miyake, A., Shinya, T., Galis, I. (2017). Oral secretions affect HIPVs induced by generalist (Mythimna loreyi) and specialist (Parnara guttata) herbivores in rice. J. Chem. Ecol. 43, 929–943. doi: 10.1007/s10886-017-0882-4
Song, C., Härtl, K., McGraphery, K., Hoffmann, T., Schwab, W. (2018). Attractive but toxic: emerging roles of glycosidically bound volatiles and glycosyltransferases involved in their formation. Mol. Plant 11, 1225–1236. doi: 10.1016/j.molp.2018.09.001
Song, M. S., Kim, D. G., Lee, S. H. (2005). Isolation and characterization of a jasmonic acid carboxyl methyltransferase gene from hot pepper (Capsicum annuum l.) J. Plant Biol. 48, 292–297. doi: 10.1007/BF03030525
Steenbergen, M., Abd-el-Haliem, A., Bleeker, P., Dicke, M., Escobar-Bravo, R., Cheng, G., et al. (2018). Thrips advisor: exploiting thrips-induced defences to combat pests on crops. J. Exper. Bot. 69, 1837–1848. doi: 10.1093/jxb/ery060
Strange, R. N., Scott, P. R. (2005). Plant disease: a threat to global food security. Annu. Rev. Phytopathol. 43, 83–116. doi: 10.1146/annurev.phyto.43.113004.133839
Sun, Y., Huang, X., Ning, Y., Jing, W., Bruce, T. J., Qi, F., et al. (2017). TPS46, a rice terpene synthase conferring natural resistance to bird cherry-oat aphid, Rhopalosiphum padi (Linnaeus). F. Plant Sci. 8, 110. doi: 10.3389/fpls.2017.00110
Sun, J. G., Huang, L. Q., Wang, C. Z. (2012). Electrophysiological and behavioral responses of Helicoverpa assulta (Lepidoptera: noctuidae) to tobacco volatiles. Arthropod. -Plant Interact. 6, 375–384. doi: 10.1007/s11829-012-9190-7
Suzuki, N., Rivero, R. M., Shulaev, V., Blumwald, E., Mittler, R. (2014). Abiotic and biotic stress combinations. New Phytol. 203, 32–43. doi: 10.1111/nph.12797
Tissier, A., Ziegler, J., Vogt, T. (2014). Specialized plant metabolites: diversity and biosynthesis. Ecol. biochemistry: Environ. interspecies Interact. (Wiley-VCH Verlag GmbH & Co. KGaA), 14–37. doi: 10.1002/9783527686063.ch2
Ton, J., D’Alessandro, M., Jourdie, V., Jakab, G., Karlen, D., Held, M., et al. (2007). Priming by airborne signals boosts direct and indirect resistance in maize. Plant J. 49, 16–26. doi: 10.1111/j.1365-313X.2006.02935.x
Tripathi, A. K., Prajapati, V., Aggarwal, K. K., Kumar, S. (2001). Toxicity, feeding deterrence, and effect of activity of 1,8-cineole from artemisia annua on progeny production of tribolium castanaeum (Coleoptera: tenebrionidae). J. Econ Entomol. 94, 979–983. doi: 10.1603/0022-0493-94.4.979
Turlings, T. C., Erb, M. (2018). Tritrophic interactions mediated by herbivore-induced plant volatiles: mechanisms, ecological relevance, and application potential. Annu. Rev. entomology 63, 433–452. doi: 10.1146/annurev-ento-020117-043507
Turlings, T. C., Lengwiler, U. B., Bernasconi, M. L., Wechsler, D. (1998). Timing of induced volatile emissions in maize seedlings. Planta 207, 146–152. doi: 10.1007/s004250050466
Ulland, S., Ian, E., Mozuraitis, R., Borg-Karlson, A. K., Meadow, R., Mustaparta, H. (2008). Methyl salicylate, identified as primary odorant of a specific receptor neuron type, inhibits oviposition by the moth mamestra brassicae l. (Lepidoptera, noctuidae). Chem. Senses 33, 35–46. doi: 10.1093/chemse/bjm061
Unsicker, S. B., Kunert, G., Gershenzon, J. (2009). Protective perfumes: the role of vegetative volatiles in plant defense against herbivores. Curr. Opin. Plant Biol. 12, 479–485. doi: 10.1016/j.pbi.2009.04.001
Van Den Boom, C. E., Van Beek, T. A., Posthumus, M. A., De Groot, A., Dicke, M. (2004). Qualitative and quantitative variation among volatile profiles induced by Tetranychus urticae feeding on plants from various families. J. Chem. Ecol. 30, 69–89. doi: 10.1023/B:JOEC.0000013183.72915.99
van Schie, C. C., Haring, M. A., Schuurink, R. C. (2007). Tomato linalool synthase is induced in trichomes by jasmonic acid. Plant Mol. Biol. 64, 251–263. doi: 10.1007/s11103-007-9149-8
Van Tol, R. W. H. M., van der Sommen, A. T. C., Boff, M. I. C., van Bezooijen, J., Sabelis, M. W., Smits, P. H. (2001). Plants protect their roots by alerting the enemies of grubs. Ecol. Lett. 4, 292–294. doi: 10.1046/j.1461-0248.2001.00227.x
Vázquez-González, C., Pombo-Salinas, L., Martín-Cacheda, L., Rasmann, S., Röder, G., Abdala-Roberts, L., et al. (2022). Effect of water availability on volatile-mediated communication between potato plants in response to insect herbivory. Fun. Ecol. 36, 2763–2773. doi: 10.1111/1365-2435.14159
Wang, H., Guo, W. F., Zhang, P. J., Wu, Z. Y., Liu, S. S. (2008). Experience-induced habituation and preference towards non-host plant odors in ovipositing females of a moth. J. Chem. Ecol. 34, 330–338. doi: 10.1007/s10886-008-9433-3
War, A. R., Sharma, H. C., Paulraj, M. G., War, M. Y., Ignacimuthu, S. (2011). Herbivore induced plant volatiles: their role in plant defense for pest management. Plant Signal. Behav. 6, 1973–1978. doi: 10.4161/psb.6.12.18053
Weinblum, N., Cna’Ani, A., Yaakov, B., Sadeh, A., Avraham, L., Opatovsky, I., et al. (2021). Tomato cultivars resistant or susceptible to spider mites differ in their biosynthesis and metabolic profile of the monoterpenoid pathway. Front. Plant Sci. 12, 630155. doi: 10.3389/fpls.2021.630155
Will, T., van Bel, A. J. (2008). Induction as well as suppression: how aphid saliva may exert opposite effects on plant defense. Plant Signal. Behav. 3, 427–430. doi: 10.4161/psb.3.6.5473
Wu, S., Schalk, M., Clark, A., Miles, R. B., Coates, R., Chappell, J. (2006). Redirection of cytosolic or plastidic isoprenoid precursors elevates terpene production in plants. Nat. Biotech. 24, 1441–1447. doi: 10.1038/nbt1251
Xiang, L. I., Zhang, X. G., Chun, X. I. A. O., Gao, Y. L., Dong, W. X. (2020). Behavioral responses of potato tuber moth (Phthorimaea operculella) to tobacco plant volatiles. J. Integrat. Agric. 19, 325–332. doi: 10.1016/S2095-3119(19)62663-8
Xiao, Y., Wang, Q., Erb, M., Turlings, T. C., Ge, L., Hu, L., et al. (2012). Specific herbivore-induced volatiles defend plants and determine insect community composition in the field. Ecol. Lett. 15, 1130–1139. doi: 10.1111/j.1461-0248.2012.01835.x
Yadav, R., Rathi, M., Pednekar, A., Rewachandani, Y. (2016). A detailed review on solanaceae family. Eur. J. Pharm. Med. Res. 3, 369–378.
Yamauchi, Y., Matsuda, A., Matsuura, N., Mizutani, M., Sugimoto, Y. (2018). Transcriptome analysis of arabidopsis thaliana treated with green leaf volatiles: possible role of green leaf volatiles as self-made damage-associated molecular patterns. J. Pest. Sci. 43, 207–213. doi: 10.1584/jpestics.D18-020
Ye, M., Glauser, G., Lou, Y., Erb, M., Hu, L. (2019). Molecular dissection of early defense signaling underlying volatile-mediated defense regulation and herbivore resistance in rice. Plant Cell 31, 687–698. doi: 10.1105/tpc.18.00569
Yoshinaga, N., Alborn, H. T., Nakanishi, T., Suckling, D. M., Nishida, R., Tumlinson, J. H., et al. (2010). Fatty acid-amino acid conjugates diversification in lepidopteran caterpillars. J. Chem. Ecol. 36, 319–325. doi: 10.1007/s10886-010-9764-8
Yuan, J. S., Kollner, T. G., Wiggins, G., Grant, J., Degenhardt, J., Chen, F. (2008). Molecular and genomic basis of volatile mediated indirect defense against insects in rice. Plant J. 55, 491–503. doi: 10.1111/j.1365-313X.2008.03524.x
Zebelo, S. A., Matsui, K., Ozawa, R., Maffei, M. E. (2012). Plasma membrane potential depolarization and cytosolic calcium flux are early events involved in tomato (Solanum lycopersicon) plant-to-plant communication. Plant Sci. 196, 93–100. doi: 10.1016/j.plantsci.2012.08.006
Zebelo, S., Piorkowski, J., Disi, J., Fadamiro, H. (2014). Secretions from the ventral eversible gland of spodoptera exigua caterpillars activate defense-related genes and induce emission of volatile organic compounds in tomato, Solanum lycopersicum. BMC Plant Biol. 14, 1–12. doi: 10.1186/1471-2229-14-140
Zhang, Y., Bouwmeester, H. J., Kappers, I. F. (2020). Combined transcriptome and metabolome analysis identifies defence responses in spider mite-infested pepper (Capsicum annuum). J. Expt. Botany. 71, 330–343. doi: 10.1093/jxb/erz422
Zhou, S., Jander, G. (2021). Engineering insect resistance using plant specialized metabolites. Curr. Opin. Biotech. 70, 115–121. doi: 10.1016/j.copbio.2021.03.005
Keywords: herbivory, defense responses, VOCs (volatile organic compounds), HIPVs, terpenoids, biotic stress, metabolomics
Citation: Kutty NN and Mishra M (2023) Dynamic distress calls: volatile info chemicals induce and regulate defense responses during herbivory. Front. Plant Sci. 14:1135000. doi: 10.3389/fpls.2023.1135000
Received: 31 December 2022; Accepted: 10 May 2023;
Published: 19 June 2023.
Edited by:
Deepak Kasote, North Carolina State University, United StatesReviewed by:
Ken Keefover-Ring, University of Wisconsin-Madison, United StatesGuoxing Wu, Yunnan Agricultural University, China
Copyright © 2023 Kutty and Mishra. This is an open-access article distributed under the terms of the Creative Commons Attribution License (CC BY). The use, distribution or reproduction in other forums is permitted, provided the original author(s) and the copyright owner(s) are credited and that the original publication in this journal is cited, in accordance with accepted academic practice. No use, distribution or reproduction is permitted which does not comply with these terms.
*Correspondence: Nithya N. Kutty, bml0aHlhLmt1dHR5QG1pdHdwdS5lZHUuaW4=; Manasi Mishra, bWFuYXNpLm1pc2hyYUBtaXR3cHUuZWR1Lmlu