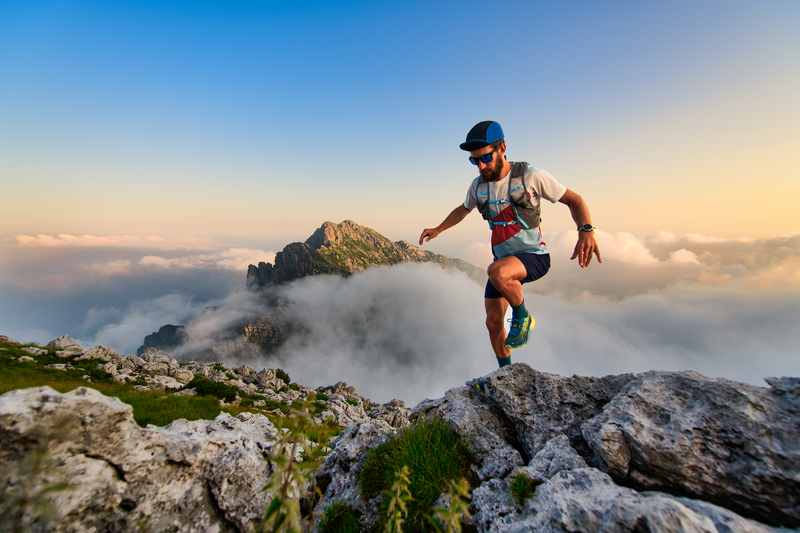
95% of researchers rate our articles as excellent or good
Learn more about the work of our research integrity team to safeguard the quality of each article we publish.
Find out more
REVIEW article
Front. Plant Sci. , 28 March 2023
Sec. Plant Bioinformatics
Volume 14 - 2023 | https://doi.org/10.3389/fpls.2023.1134754
This article is part of the Research Topic Dissecting Antinutrient Traits Using Omics Approaches View all 6 articles
Plants synthesize various compounds for their growth, metabolism, and stress mitigation, and one such group of compounds is the raffinose family of oligosaccharides (RFOs). RFOs are non-reducing oligosaccharides having galactose residues attached to a sucrose moiety. They act as carbohydrate reserves in plants, assisting in seed germination, desiccation tolerance, and biotic/abiotic stress tolerance. Although legumes are among the richest sources of dietary proteins, the direct consumption of legumes is hindered by an excess of RFOs in the edible parts of the plant, which causes flatulence in humans and monogastric animals. These opposing characteristics make RFOs manipulation a complicated tradeoff. An in-depth knowledge of the chemical composition, distribution pattern, tissue mobilization, and metabolism is required to optimize the levels of RFOs. The most recent developments in our understanding of RFOs distribution, physiological function, genetic regulation of their biosynthesis, transport, and degradation in food crops have been covered in this review. Additionally, we have suggested a few strategies that can sustainably reduce RFOs in order to solve the flatulence issue in animals. The comprehensive information in this review can be a tool for researchers to precisely control the level of RFOs in crops and create low antinutrient, nutritious food with wider consumer acceptability.
Plants are sessile and hence must face the real challenges of nature in terms of biotic and abiotic stresses. These inevitable environmental factors often profoundly affect plant metabolism and photosynthesis, leading to a significant decline in crop yields and productivity. Plants have adapted different strategies to cope with the ever-changing climate during evolution. Plants use carbohydrates or their derivatives as stress-sensing and signalling molecules (Cummings, 2019) for coordinating metabolism with developmental features, plant growth and responses to external stimuli (Rolland et al., 2006). Furthermore, low-molecular weight soluble sugars, amino acids, and amines accumulate in the cytosol or vacuoles and help in the cell’s osmotic adjustment and also protect the cell membrane and other cell components from reactive oxygen species (ROS). Raffinose family oligosaccharides (RFOs) and Fructooligosaccharides (FOS) are one such class of soluble sugars which play an important role in abiotic stress response.
RFOs, such as raffinose, stachyose, and verbascose, are the non-reducing carbohydrates formed by α-1,6-galactosyl extensions onto the glucose moiety of sucrose. These compounds are nearly ubiquitous in crops (Minorsky, 2003), with contents varying from species to species and even from plant to plant, depending on the growing conditions. RFOs act as reserve carbohydrates (Gangl and Tenhaken, 2016) and are reported as compatible solutes that function like antioxidants, are a component of carbon partitioning strategies and may act as stress signals (Elsayed et al., 2014). RFOs benefit plants, but humans and other monogastric animals find them difficult to digest due to the absence of the enzyme required for their hydrolysis. Food containing higher RFOs takes a shorter time to pass through the digestive tract, causing reduced absorption of beneficial nutrients. The lack of hydrolysis in the small intestine and subsequent fermentation by the gut flora in the colon results in flatulence (Valentine et al., 2017). This limits the consumption of crops with higher RFOs (Kannan et al., 2018, Kasprowicz-Potocka et al., 2022). The balance between crop quality improvement and plant metabolism/immunity makes RFOs manipulation challenging for researchers.
This review highlights the structural chemistry of RFOs leading to their biosynthesis and subsequent degradation. The latest information on the genetic control of RFOs, their distribution, transport and physiological significance are also discussed. Recent studies targeting the manipulation of RFOs biosynthesis and transport to minimise their content in the human diet have also been highlighted, paving the way for producing low antinutrient, consumer-preferred food crops.
Raffinose family oligosaccharides (RFOs) are formed when galactose units are attached to the glucose moiety of sucrose via α-1,6-galactosidic linkages (Figure 1A). Galactinol serves as the galactose donor to sucrose, producing raffinose (trisaccharide), the first member of the RFOs family (Elango et al., 2022). Further addition of galactosyl residues forms stachyose (tetrasaccharide) and verbascose (pentasaccharide), which accumulate primarily in dicotyledonous seeds (Sengupta et al., 2015). Oligosaccharides with a higher degree of polymerization (DP) include ajugose (hexasaccharide), which is limited to some species of the Lamiaceae family, particularly Ajuga reptans (Haab and Keller, 2002). Higher plants seldom produce RFOs isomers with galactosidic linkages at other carbons of glucose (such as umbelliferose), fructose (such as Planteose and Sesamose), or both glucose and fructose moieties concurrently (such as Lychnose and Isolychnose) (Vanhaecke et al., 2010). However, these classes do not fall under the RFOs (Figure 1B). Unlike RFOs, sugars like lychnose/isolychnose are exclusively produced by the Caryophyllaceae family, acting as chemotaxic markers of this family (Madore, 2001).
Figure 1 The general structure of raffinose family oligosaccharides (RFOs) and their related families. (A) RFOs are α(1-6) galactosyl substituted derivatives of the sucrose molecule, forming a non-reducing oligosaccharide. Raffinose, Stachyose and Verbascose are the major RFOs members in plants. (B) RFOs isomers with galactosidic linkages at other carbons of glucose (Umbelliferose), fructose (Planteose and Sesamose), or raffinose (Lychnose and Isolychnose). These classes do not fall under the RFOs. Structures were drawn with ChemDraw (version 12.0.2) software.
RFOs biosynthesis begins with galactinol synthase (GolS), catalysing the galactosyl transfer to myo-inositol from UDP-D-galactose, synthesizing galactinol (dos Santos and Vieira, 2020). A higher concentration of galactinol than UDP-D-galactose in the developing seed suggests the function of galactinol as a transient galactosyl store, separated from primary carbohydrate metabolism (Peterbauer and Richter, 2001). Raffinose synthase (RS) catalyzes raffinose synthesis by a galactose-transfer (from galactinol) to glucose moiety of sucrose (Li et al., 2020). Myoinositol released in this process returns to the myoinositol pool. Raffinose synthase is said to be the most unstable enzyme in this pathway (Tian et al., 2019). Raffinose can cause a product inhibition effect on RS. So, it is rapidly converted to stachyose by stachyose synthase (SS) (Tian et al., 2019). Further, galactose transfer from galactinol to stachyose is catalyzed by verbascose synthase (VS), yielding verbascose (Figure 2). Unlike RS, SS exhibits a broad substrate specificity, using a range of galactosyl cyclitols (galactosyl ononitol, galactopinitol A, galactinol) and methylated inositols (ononitol and pinitol) or myoinositol as galactosyl donors and acceptors, respectively. (Hoch et al., 1999; Peterbauer and Richter, 2001). Galactosylation of pinitol yields galactopinitol A, which acts as a galactosyl donor (to raffinose) and acceptor (yielding ciceritol). A strong negative correlation between digalactosyl cyclitol (ciceritol) and verbascose was found in two lentil cultivars (Frias et al., 1999), as the two pathways are linked via STS, inhibiting each other.
Figure 2 The RFOs biosynthetic pathway. Myo-inositol and UDP-Galactose serve as the precursors for galactinol. The reaction is catalysed by galactinol synthase (GolS) and Mn2+ as a cofactor. Galactinol is the galactose donor to sucrose, forming raffinose with the help of raffinose synthase (RS). The addition of galactose moiety to raffinose is catalysed by stachyose synthase (SS), producing stachyose. SS has a broad substrate specificity, catalyzing the production of ciceritol in some plants. Verbascose synthase (VS) catalyses the formation of verbascose in the galactinol-dependent pathway. A galactinol-independent pathway also exists in some crops or even in different tissues of the same crop, where galactan: galactan galactosyltransferase (GGT) catalyses the formation of RFOs, utilizing the galactose moiety from lower order RFOs. All the reactions are reversible in galactinol-dependent and independent pathways. HK, Hexokinase; MIPS, Myoinositol-1-Phosphate Synthase; IMPase, Inositol mono phosphatase; MIK, Myoinositol Kinase; PIK, Phosphoinositol Kinase.
Two members of the Lamiaceae family (Ajuga reptans and Coleus blumei) exhibit a galactinol-independent pathway, where the galactan: galactan galactosyltransferase (GGT) enzyme catalyzes the galactosyl transfer from one RFO to another (Haab and Keller, 2002) (Figure 3). Stachyose synthesized in the cytoplasm can be transported via the stachyose/H+ antiporter to the vacuole to participate in the galactinol-independent pathway (Greutert et al., 1998). GolS, RS, STS being extravacuolar and stachyose, verbascose and ajugose being exclusively vacuolar, suggests the synthesis of higher-order RFOs via a galactinol-independent pathway in the vacuole (Peterbauer and Richter, 2001). However, in seeds, the alkaline pH (pH 6.7) of vacuoles creates an unfavorable condition for acidic GGT enzyme, facilitating RFOs biosynthesis in the conventional galactinol-dependent pathway (Herman and Larkins, 1999; Peterbauer and Richter, 2001; Haab and Keller, 2002).
Figure 3 RFOs catabolism. RFOs are hydrolyzed by α-galactosidase (AGAL) and β-fructofuranosidase (BFLUCT) in most plants, producing simple sugars (left). RFOs utilization by gut microbiota in humans and animals via extracellular (levansucrases) or intracellular (raffinose transporter, RT) hydrolysis. AGLUC, α-glucosidases; ScrP, Sucrose Phosphorylase.
The RFOs and phytic acid biosynthetic pathways share a common intermediate (myoinositol). Low phytic acid mutants have increased myoinositol levels, possibly contributing to RFOs accumulation (Zhawar et al., 2011; Redekar et al., 2020). Higher sucrose concentrations also increase the accumulation of raffinose (Borisjuk et al., 2002). From this, it is difficult to say which substrate (myoinositol or sucrose) is more important. Since monocots predominantly accumulate raffinose, sucrose may play an important role, but for dicots, which mainly synthesize stachyose or verbascose, galactinol (from myoinositol) play a critical role rather than sucrose. This implies that all the metabolites are tightly regulated and that any changes can markedly affect RFOs deposition in seeds (Karner et al., 2004). Further study can be conducted to establish the differences between the dicot and monocot raffinose synthase genes, causing differential accumulation of RFOs in such plants.
Genes encoding enzymes involved in RFOs biosynthesis primarily have either seed-specific or phloem tissue-specific expression. Seed-specific GolS expression was reported to confer seed desiccation tolerance in tomatoes (Downie et al., 2003). No GolS expression in flowers, fruits, roots and endosperm, but a very high expression in leaves was observed when coffee plants were exposed to stress (drought/salt/heat) (dos Santos et al., 2011). The spatio-temporal expression patterns of hybrid poplar GolS homologues (Unda et al., 2012) and leaf-specific or anther-specific expression of cloned GolS genes from cotton (Zhou et al., 2012) suggest GolS expression is highly tissue-specific. GolS1 was also reported to facilitate raffinose synthesis in the storage pool of the common bugle (Ajuga reptans). At the same time, GolS2 plays a central role in synthesizing galactinol to transport the RFOs pool (Sprenger and Keller, 2000). Transcriptomic analysis of water stress-treated peanuts identified AdGolS3 as a candidate gene for drought tolerance (Vinson et al., 2020). Similar studies on kiwifruit (Actinidia chinensis) identified AcRS4 as critical during salt stress (Yang et al., 2022). The leaf and latex-specific HbGolS1 and latex-specific expression of HbRS1 were also reported in rubber (Hevea brasiliensis) (Lu et al., 2022). Among six GolS genes and the three RS genes in soybean, GmGolS1_A, GmRS2_A, and GmRS2_B form attractive gene targets because of their seed-specific expression patterns (de Koning et al., 2021). GmGolS1_A expression was highest during seed maturity, whereas soybean vegetative tissues primarily showed GmGolS1_B expression (Le et al., 2020). In Arabidopsis thaliana, among seven GolS isoforms, three isoforms, AtGolS1/AtGolS2 and AtGolS3 were induced during drought/salt/heat stress and cold stress, respectively (Taji et al., 2002). The inability of galactinol and raffinose accumulation in AtGolS1 mutants also suggests AtGolS1 as the major GolS isoform facilitating RFOs accumulation under heat stress (Panikulangara et al., 2004). Among six putative RS genes in Arabidopsis, overexpression of two RS genes showed oxidative stress tolerance in tobacco (Nishizawa et al., 2008). High seed-specific expression of PvGolS1 and PvRS2 in common beans (Phaseolus vulgaris) (de Koning et al., 2021) and AhRS14 and AhSS7 in peanuts (Sanyal et al., 2023) made them good candidates to knock out for low RFOs cultivar development just like the low raffinose soybean cultivars with mutated RS2 (Dierking and Bilyeu, 2008; Bilyeu and Wiebold, 2016).
Three transcription factors (TFs) can regulate GolS gene expression: heat shock factors (HSFs), DREB1A/CBF3, and WRKY transcription factors (Elsayed et al., 2014). HSFs and DREB1A/CBF3 are reported in Arabidopsis (Panikulangara et al., 2004; Maruyama et al., 2009), while WRKY regulates both GolS and RS via W-box cis-elements present in their promoters, as explained in Boea hygrometrica (Wang et al., 2009). GolS1 and GolS2 expression is regulated by HSFs like HsfA1a, HsfA1b, and HsfA2 in Arabidopsis (Nishizawa et al., 2008), HsfA4a in mustard (Lang et al., 2017) and HsfA2 in maize (Gu et al., 2019). HsfA2 and heat shock binding protein (HSBP) physically interact with each other and antagonistically modulate GolS expression. Overexpression of maize ZmDREB1A in the leaf also showed upregulation of ZmRS by binding to the DRE motif in the ZmRS promoter, enhancing raffinose synthesis and chilling stress tolerance (Han et al., 2020). A MYB-like transcription factor (AQUILO) isolated from Amur grapes (Vitis amurensis) improved cold tolerance through the upregulation of GolS and RS and osmoprotectant accumulation (Sun et al., 2018). Ethylene-responsive factors (ERFs) (PtrERF108) from trifoliate orange (Poncirus trifoliata) also target raffinose synthase (PtrRafS) directly, modulating raffinose levels in response to cold stress (Khan et al., 2021). Most TFs regulating RFOs in response to cold stress have been reported, while limited information is available for TF-mediated RFOs modulation in other stress situations.
The interplay of RFOs and phytohormones is also tightly controlled. Studies suggest ABA-induced RFOs accumulation in alfalfa somatic embryos (Blöchl et al., 2005) and regulation of maize GolS2 expression by VIVIPAROUS1- ABA INSENSITIVE5 (ZmVP1- ZmABI5) interaction (Zhang et al., 2019). The studies observed an increase in GolS activity and raffinose accumulation, revealing an ABA-RFOs crosstalk, the mechanism of which is yet to be identified. Promoter-GUS study by Salvi et al., 2020, demonstrated the positive influence of ABA and dehydration stress on chickpea GolS (CaGolS1 and CaGolS2) gene. Improved chlorophyll retention, relative water content and lower H2O2, malondialdehyde (MDA) content, and ion-leakage in transgenic lines suggested the potential role of GolS in modulating ROS and alleviating dehydration stress. OsPP65 (a type 2C protein phosphatase) knockout rice plants showed significant expression of ABA and jasmonic acid biosynthetic genes as well as their high endogenous levels during osmotic (salt) stress (Liu Q. et al., 2022). Metabolomics analysis showed higher endogenous galactose and galactinol content but a lower raffinose content in the transgenic rice suggesting negative regulation of OsPP65 through ABA and JA-mediated modulation of RFOs during salt stress tolerance. The role of Brassinosteroid (24-epibrassinolide/EBR) in the positive regulation of tea GolS gene (CsGolS2) and enhancement in ABA signal transduction (Zhang et al., 2022) also suggests the possible regulation of the RFOs gene by phytohormones.
α-Galactosidases (AGAL) are activated during germination and hydrolyze RFOs into simpler molecules, i.e., sucrose and galactose (Figure 3). The galactokinases act upon the galactose removed during this process, forming D-galactose-1-phosphate. This compound is further metabolized by UDP-D-glucose-hexose-1-phosphate uridyltransferase (via the Leloir pathway) or UDP-D-galactose pyrophosphorylase (via the pyrophosphorylase pathway) (Peterbauer and Richter, 2001). RFOs and AGALs co-occur in protein storage vacuoles, but simultaneous synthesis and degradation are prevented due to the vacuole’s high pH during the reserve deposition and storage phase (Keller and Pharr, 1996). Evidence also negates the function of the protein storage vacuole as a lytic compartment (Jauh et al., 1999; Peterbauer and Richter, 2001). AGAL synthesised de novo (Group II AGAL) plays a role in galactomannan degradation, while pre-existing AGAL (Group I AGAL) appears to be responsible for RFOs hydrolysis (Katrolia et al., 2014). AGALs with acidic pH optima are also present in extracytoplasmic or vacuolar regions, but they are not effective at hydrolyzing larger RFOs, such as stachyose, and generally show a preference for small oligosaccharides (Peterbauer and Richter, 2001).
In animals, due to the lack of α-galactosidase enzyme, RFOs cannot be utilized. It passes to the lower gut and gets fermented by the gut microbiota (Arunraj et al., 2020). Out of thousands of bacteria in the human gut, about 10-15% have the potential to utilize raffinose as their substrate (Mao et al., 2018). Bacteria that prefer galactose to glucose or fructose as an energy source metabolize stachyose better than raffinose, while most bacteria commonly metabolize raffinose (Zartl et al., 2018). All bacteria that utilize raffinose do not necessarily have AGAL activity but still manage to degrade raffinose by using enzymes like β-fructofuranosidases (BFLUCT, hydrolase class) or levansucrases (transferase class). BFLUCT removes the fructosyl moiety of raffinose (yielding melibiose) and stachyose (yielding manninotriose). Bacteria producing both AGAL and BFLUCT can hydrolyze raffinose into galactose, glucose, and fructose. Two types of hydrolysis generally occur: intracellular and extracellular. In the case of intracellular hydrolysis, raffinose hydrolysis occurs inside the cell after transporting it via raffinose transporters. In contrast, for extracellular hydrolysis, raffinose can be hydrolyzed into fructose and melibiose by levansucrases (Figure 3). The hydrolysis products are transported inside the cell and metabolized for energy supply. Hence, glycosidases and transporters play a vital role, enabling gut bacteria to utilize galactosides differently (Teixeira et al., 2012; Mao et al., 2018). However, most studies used raffinose as the substrate for gut bacteria, while the effect of stachyose and verbascose as a substrate needs further validation.
The content and composition of RFOs vary across the genotypes and environmental conditions (Table 1) (Kumar et al., 2010; Redekar et al., 2020). Seeds are the primary storage site for RFOs. Plants may store RFOs in tubers or mesophyll cells of photosynthesizing leaves, sometimes reaching even 25-80% of dry weight (Keller and Matile, 1985). All seed parts, viz., embryo, endosperm or seed coat, can retain α-galactosides at varying levels (Martínez-Villaluenga et al., 2008; Sengupta et al., 2015). Reports suggest that lupin seeds have the highest RFOs, followed by soybean (Ruiz-López et al., 2000; Martínez-Villaluenga et al., 2008). Stachyose seems to be the predominant RFO in dicot crops. However, monocot seeds such as barley and wheat primarily accumulate raffinose (Yan et al., 2022). Among the commonly cultivated crops, ajugose was found exclusively in lupin seeds. In crop plants, the reports suggest that ciceritol, a pinitol digalactoside, is found only in chickpeas and lentils, with chickpeas accumulating the maximum amount (1.2-3.1%). Among legumes, groundnut and faba bean have been reported to have lower amounts of RFOs (0.12-.076% and 1.0-4.5%, respectively) (Bishi et al., 2015; Kannan et al., 2018; Sanyal et al., 2023). Analysis of RFOs in Brassica, barley (Andersen et al., 2005), and wheat (Huynh et al., 2008) suggested that non-legumes contain comparatively lower amounts of these oligosaccharides. Although various studies discussed the variations in RFOs content/composition, the evolutionary reason behind the accumulation of high DP compounds (verbascose, ajugose, ciceritol), having higher energy costs, is still unexplored. Moreover, there is significant research on RFO concentrations in legume crops, while it is still scanty for non-legumes.
Plants belonging to Gamalei’s “Type 1 category” (such as cucurbits) have a high plasmodesmata abundance between companion cells and mesophyll cells and assimilate is loaded via a polymer trap mechanism in a symplastic route (Gamalei, 1989). RFOs are predominantly transported in such plants. On the other hand, “Type 2 plants” with lower plasmodesmata frequency (such as potato and Arabidopsis) primarily transport sucrose via proton symport in an apoplastic route (Turgeon, 1996; Haritatos et al., 2017). The polymer trap model states that the specialized companion cells (intermediary cells) in the minor veins are where RFOs biosynthetic enzymes transform the sucrose generated by photosynthesis in mesophyll cells (source) into RFOs (mainly raffinose and stachyose) (Cao et al., 2013). The plasmodesmata in RFOs-utilizing plants are characteristically branched on the side of companion cells, significantly reducing the plasmodesmatal pore size. The RFOs cannot diffuse back to the mesophyll (source) because they are larger and are trapped in the intermediary cells. Conversion of sucrose into RFOs favors passive entry of sucrose, while RFOs accumulation increases osmotic pressure. This makes it easier for the RFOs to migrate toward the sieve elements, followed by transportation to other areas of the plant (sinks), where AGAL may break them down (Turgeon and Medville, 2004). Plants can maintain a high phloem sugar concentration by producing RFOs in the intermediate cells. Although species-specific, this paradigm is primarily observed in the Cucurbitaceae family. Meagre amounts of RFOs are transported by Type 1 plant species lacking intermediate cells, and they mostly load assimilates via the apoplastic pathway (Hannah et al., 2006).
In leaves that transport, as well as store RFOs, such as Xerosicyos danguyi (Cucurbitaceae) and Ajuga reptans (Lamiaceae), RFOs biosynthetic components are present in both phloem and mesophyll tissues in different isoforms, involving complex cellular partitioning (Madore, 2001). Plants having variegated leaves (such as Coleus blumei Benth) do not use AGAL for RFOs degradation in non-photosynthesizing patches. Instead, they possibly use the reverse (or backward) reaction of RS and SS to degrade RFOs into disaccharides (galactinol and sucrose). The products obtained thereof can support respiration in the absence of photosynthesis (Madore, 2001). 14C-labelling study in Cucumis blumei indicates limited phloem transport of galactinol and efficient retention and transportation of sucrose, raffinose and stachyose. Studies estimating raffinose and galactinol levels observed 30 times lower raffinose in Cucumis leaves but only two-fold lower raffinose in phloem exudates (Ayre et al., 2003; Hannah et al., 2006), suggesting higher transport efficiency of raffinose as compared to galactinol.
Additionally, raffinose can supplement sucrose as phloem-mobile forms of carbon, delivering 1.5 times more carbon than sucrose at the same osmotic cost. This is often seen to support non-photosynthetic tissues and organs (Madore, 2001). When plants with an apoplastic phloem loading strategy (Type 2 plants) were engineered to follow a symplastic route (polymer trap mechanism) via metabolic engineering of RFOs biosynthetic genes, the synthesis of RFOs and their transportation was deficient despite the high sucrose concentration (Hannah et al., 2006; Cao et al., 2013). Theoretically, apoplasmic loaders should synthesize RFOs efficiently due to ample carbon (reduced form) in the companion cell cytoplasm where RFOs synthesis occurs. Moreover, there are no limitations in the plasmodesmatal pore size. The inability of high RFOs accumulation in companion cells can be a biochemical limitation or a cell biology problem (Yadav et al., 2015). There can be limitations in the flux of early RFOs precursors such as UDP-D-galactose and myoinositol or variations in the internal membrane and vacuoles. “Type 1” plants with numerous, highly branched plasmodesmata generally have small vacuoles, extensive endomembrane systems and companion cells larger than “Type 2” plants. Thus, the internal structure can also contribute to the biosynthesis of RFOs in companion cells (Turgeon et al., 2001). The stability, localization and interaction of enzymes with other cellular components can probably explain the inefficient synthesis and transport of RFOs in “Type 2” plants.
Under water deficit conditions, the hydroxyl groups of RFOs provide the hydrophilic interaction needed for cellular membrane and protein stabilization. A higher RFOs concentration prevents sucrose crystallization during desiccation and facilitates stable vitreous/glassy state formation (Leopold et al., 1994; Elango et al., 2022). The concentrated, highly viscous solid crystals formed within cells (intracellular glass) protect the desiccating seeds by providing stability during dormancy (Koster et al., 1991). Moreover, raffinose and its higher homologues stabilize the membrane bilayer by inserting themselves within the lipid headgroups during stress (Hincha et al., 2003). Delayed acquisition of desiccation tolerance was observed in RFOs biosynthesis mutants (gos1, gos2 and rs5) of Arabidopsis. In contrast, the corresponding overexpression lines exhibited higher RFOs and enhanced desiccation tolerance (Jing et al., 2018). Relatively high level of reducing sugars in green chickpea pods and their subsequent reduction from yellow pod stage through post-germination stage, indicated a continual supply of reducing sugars for the seed’s energy requirements as it dries out, preparing it for desiccation and germination (Arunraj et al., 2020). Experiments also demonstrated the raffinose-mediated increase in antioxidant gene expression and their stabilization (Nishizawa et al., 2008, Salvi et al., 2016, Keller et al., 2021), facilitating ROS detoxification. These experiments suggest probable mechanisms protecting the seed and helping them remain viable in the dry state.
Various molecular and biochemical changes occur during the acclimatization of plants to cold and drought (Figure 4). A prominent pathway that transcriptionally induces target gene expression involves cold-responsive element-binding factor/dehydration-responsive element-binding factor CBF/DREB (Jaglo et al., 2001; Lata and Prasad, 2011). During cold acclimation, the transcription factor CBF3 is overexpressed, which induces the accumulation of osmoprotective substances, including RFOs, in Arabidopsis (Gilmour et al., 2000). GolS activity also increased under cold exposure in kidney bean seeds (Liu et al., 1998) and tomato leaves (Downie et al., 2003; Nishizawa et al., 2008). Among the seven GolS members of Arabidopsis, GolS1 and GolS2 mRNAs were expressed in leaf during drought stress and salt stress, while GolS3 was induced during cold stress (Taji et al., 2002; Elsayed et al., 2014). PhGolS1-1 was recognized as a direct target of PhZFP1, a C2H2-type zinc finger protein, modulating galactinol synthesis and contributing to cold tolerance in Petunia hybrida (Zhang et al., 2022). Increased cold tolerance as a result of raffinose accumulation has also been reported in maize (Han et al., 2020), trifoliate orange (Poncirus trifoliata L.) (Khan et al., 2021), and barrel clover (Medicago truncatula) (Sun et al., 2021), where RS and GolS have been proposed to be the primary target of the associated transcription factors (HSFs, DREB1A/CBF3 and WRKY). Additionally, GolS1 was identified as a heat shock factor (HSF)-dependent gene of Arabidopsis involved in vegetative tissue-specific osmolyte synthesis during stress (Panikulangara et al., 2004; Schramm et al., 2006; Nishizawa et al., 2008). Increased heat stress tolerance was observed in Arabidopsis plants overexpressing maize GolS gene (ZmGolS2) with increased raffinose and galactinol levels (Gu et al., 2016), while overexpression of the maize heat shock binding protein (ZmHSBP2) in Arabidopsis decreased stress tolerance due to reduced expression of RFOs genes (AtGolS1, AtGolS2, and AtRS5) (Gu et al., 2019). Increased RFOs content due to overexpression of GolS and RS has also been reported to improve drought tolerance in cucumber (Ma et al., 2021) and Arabidopsis (Li et al., 2020). Recent studies also reported higher galactinol content and increased raffinose catabolism in a type 2C phosphatase protein (OsPP65) knockout line of rice, conferring osmotic and salt stress tolerance (Liu et al., 2022b). Studies on the role of RFOs in alleviating abiotic stresses are ever-increasing, and the differential accumulation of galactinol and raffinose can further be studied. An opposite trend observed in raffinose levels in other instances (Le et al., 2020; Liu et al., 2022b) hints towards an additional mechanism for regulating raffinose biosynthesis.
Figure 4 Functions of Raffinose Family Oligosaccharides (RFOs) in plants and animals. RFOs in plants provide abiotic stress tolerance, serve as a storage compound upon seed germination, maintain osmotic balance, help seed storability, and stabilize membranes. In human and monogastric animals, these same RFOs are responsible for flatulence production, reduced net dietary energy and decreased absorption of other nutrients. However, when RFOs reach the colon, they serve as prebiotics and help in the growth of probiotic microorganisms.
During seed germination, the expression of AGAL increases, utilizing the stored RFOs as a carbon source (Martínez-Villaluenga et al., 2008). A recent report demonstrated the increase in the activity of AGAL during early germination and seed maturation in chickpeas (Cicer arietinum) (Arunraj et al., 2020). An inhibitor of α-galactosidase (1-deoxygalactonojirimycin, DGJ) in germinating pea seeds blocked RFOs breakdown and reduced germination rates to approximately 25% of control, two days after imbibition (Blöchl et al., 2007). Soybean seeds similarly experienced a delay in germination, but the germination rate was not significantly reduced, indicating a secondary role of RFOs rather than a primary one (Dierking and Bilyeu, 2008; Li et al., 2020). The positive correlation of raffinose (Yan et al., 2018) and the negative correlation of galactose (Chen et al., 2022) with seed germination in hybrid rice under natural ageing conditions also suggested the role of RFOs in seed vigour and longevity. Decreased seed longevity was also observed in maize plants with low raffinose (reduced ZmRS expression) (Han et al., 2020) and Arabidopsis plants overexpressing AGAL (ZmAGA1) (Zhang et al., 2021). The seed germination, however, was higher in low raffinose Arabidopsis plants (Zhang et al., 2021). Like phytic acid (phosphate reservoir), RFOs act as galactose reservoirs, interacting with PIF transcription factors to control temperature- and light-dependent germination (Gangl and Tenhaken, 2016). Early studies already reported the influence of sucrose to total oligosaccharide ratio on seed storability. A ratio less than 1 confers seed storability with a half viability period (t1/2) >10 years, while a ratio greater than 1 negatively impacts seed storability with a t1/2 <10 years (Horbowicz and Obendorf, 1994). Accumulation of soluble sugars or alcohols possibly plays a protective role by minimizing harmful effects of ROS (Salvi et al., 2022). These metabolites in high concentration can stabilize the enzymes (Ascorbate, Glutathione) involved in ROS detoxification and they also exhibit higher second-order rate constants for detoxification as compared to common antioxidants (Nishizawa et al., 2008). RFOs can react with ROS using mechanisms similar to fructans (Keller et al., 2021). resulting in the formation of sugar–phenol compounds, higher DP-neutral carbohydrates, or phenolics (Peshev et al., 2013). This mechanism can protect ROS-mediated lipid peroxidation in the tonoplast. In a recent study, Keller et al., 2021, showed ROS production in sugar beet pith tissue due to frost exposure to induce raffinose synthase gene (BvRS5) expression and raffinose levels in the tissue. Previous studies on transgenic Arabidopsis also showed overexpression of galactinol synthase (GolS1, GolS2, GolS4) and raffinose synthase (RS2) along with increased ROS-scavenging/oxidative stress tolerance (Nishizawa et al., 2008). Decreased seed vigour in maize RS mutants and enhanced seed vigour in Arabidopsis lines overexpressing ZmRS, ZmGolS or AtSS have also been reported (Li et al., 2017). These pieces of evidence suggest a potential role of RFOs in supporting seed vigour and longevity via ROS modulation.
Reports on RFOs influencing plant growth/development are also increasing (Unda et al., 2017; Hua et al., 2021; Liu et al., 2022a). Galactinol synthase (AtGolS3) overexpressing poplar plants exhibited higher lignin and cellulose deposition with increased vessels (Unda et al., 2017). AGAL overexpressing cucumber plants also had increased fruit vasculature and size, while its RNAi lines exhibited delayed fruit development and altered sugar metabolism (Hua et al., 2021). Reduced photosynthesis and fewer plasmodesmata decreased phloem loading in AGAL-silenced cucumber plants (CsAGA2), and the opposite trend in CsAGA2-overexpression lines further validated the role of AGAL in increasing fruit size (Liu et al., 2022a).
Plants have evolved various defence strategies with the evolution of pathogens and pests. Galactinol, raffinose and melibiose (a raffinose degradation product) induce systemic resistance to phytopathogens. Experiments on rhizobacterium Pseudomonas chlororaphis O6 colonization in cucumber showed an increase in endogenous galactinol levels within the leaves and conferred resistance to bacterial pathogens (P. syringae and Erwinia carotovora) and the leaf spot fungus Corynespora cassiicola (Ryu et al., 2007; Kim et al., 2008; Cho et al., 2010). Such events in plants are referred to as “sugar-based resistance” or “sweet immunity” (Gómez-Ariza et al., 2007; Bolouri Moghaddam and Van Den Ende, 2013; Morkunas and Ratajczak, 2014). Recent reports also suggest RFOs play a protective role at the initial stages of root nematode infection, but nematodes hijack them as carbon nutrients at later stages of infection (Wang et al., 2022). The study reported increased galactinol, raffinose and stachyose content with overexpression of RS gene at the early infection stage, followed by reduced transcript levels of GolS, RS and STS, reduced RFOs levels and increased AGAL activity during the late infection stage (Wang et al., 2022). GolS and RS overexpressing poplar plants exhibited resistance to leaf rust due to higher galactinol and raffinose levels, but GolS silenced lines exhibited higher disease incidence (La Mantia et al., 2018). An altered translocation stream was also observed in Arabidopsis when RFOs biosynthetic enzymes were expressed in ordinary companion cells, which resulted in reduced fecundity of aphid feeding (Cao et al., 2013). Aphids preferred sucrose-translocating plants over RFOs-translocating plants (Hewer et al., 2010), which presents a clue about the role of RFOs from an ecological viewpoint. Such studies can further be developed to understand the role of RFOs as a phloem mobile metabolite, supporting plant immunity.
Humans and other monogastric animals cannot digest RFOs because their intestinal mucosa lacks the hydrolyzing enzyme AGAL (Rackis, 1975). RFOs pass down to the lower intestinal tract, where the colon microflora metabolizes them via anaerobic fermentation, producing excess carbon dioxide, hydrogen, traces of short-chain fatty acids (SCFAs) and methane (Minorsky, 2003; Sanyal and Bishi, 2021). Flatus accumulation in the gastrointestinal tract causes abdominal rumblings, diarrhea, cramps, pain and discomfort, deterring people from consuming high RFOs food crops (Sasi et al., 2022).
RFOs cause the quick passage of animal feed through the digestive system, negatively affecting the absorption of other nutrients (Figure 4) (Valentine et al., 2017). Improved amino acid digestion by RFO-extracted lupin feed has been observed in swine (van Barneveld, 1999). RFOs create an imbalance in the small intestine’s osmotic pressure, which reduces its absorption capacity for glucose, water, and methionine (Peterbauer et al., 2001; Martínez-Villaluenga et al., 2008). Studies have also supported the assumption that RFOs from lupin reduce nutritional value by reducing protein digestibility (Glencross et al., 2003; Martínez-Villaluenga et al., 2008). Animals fed on an RFO-rich diet see a drop in true metabolic energy (TME) due to extensive fermentation in the large intestine (Coon et al., 1990; Zhu et al., 2020; Jiang et al., 2022). TME is the net energy available for metabolism after excluding the energy lost (in urine, faeces and combustible gases) from the gross energy (Lattimer and Haub, 2010). Improvements were observed when feeding was supplemented with exogenous α-galactosidase (Jang et al., 2019; Llamas-Moya et al., 2021) or silencing the raffinose synthase gene in the food crop (Valentine et al., 2017).
Despite the negative influence of RFOs on human health, recent studies have identified some prebiotic potential of RFOs (mainly raffinose). Prebiotics stimulate calcium, magnesium and iron absorption, regulate lipid metabolism, and help modulation of immune response (Anggraeni, 2022; Kumar et al., 2022). RFOs function as prebiotics, stimulating the growth or activity of good gut bacteria (Zartl et al., 2018; Amorim et al., 2020). RFOs increased the number of Lactobacillus (beneficial bacteria) present in the vaginal microbiota (Collins et al., 2018) and decreased the pathogenic Proteobacteria, which causes GI tract diseases, during fermentation in the human gut (Amorim et al., 2020). Studies on 21-day-old broilers showed increased growth, cecal microbiota and gut health with enhanced immune responses after in-ovo inoculation of B. subtilis, raffinose, and symbiotic (Shehata et al., 2022). The beneficial influence of raffinose on gut microbiota is reviewed elsewhere (Anggraeni, 2022; Bamigbade et al., 2022). However, as mentioned in section 5, most of these studies used raffinose as a substrate, while dicots (especially legumes) generally possess higher RFOs (stachyose, verbascose), which needs consideration. The role of stachyose or verbascose as a substrate can shed light towards the actual potential of the gut microbiota in degrading RFOs.
From the above discussion, it becomes clear that RFOs benefit plant growth and development. However, their adverse effects on humans and monogastric animals require their reduction to an acceptable limit. Such an approach can preserve normal plant growth while reducing flatus production to promote human consumption and animal feed for monogastric animals like pigs and sheep.
α-Galactosidase hydrolyses the α(1→6) linkage to break RFOs. Overexpression of AGAL from coffee reduced the total RFOs in peas (Polowick et al., 2009). Other RFOs degradative enzymes such as levansucrases and β-fructofuranosidases (BFLUCT) can also be targeted. Activation of AGAL after harvesting can be an interesting strategy to reduce RFOs without impacting plant development. AGAL from a thermophilic bacterium (e.g., Thermotoga neapolitana) can be transferred into grain legumes, only to be induced during canning (Wang et al., 2003; Kannan et al., 2018). An alternative strategy to reduce the RFOs concentration is increasing galactosyl cyclitol (e.g., ciceritol) synthesis (Frias et al., 1999; Peterbauer et al., 2001; Kannan et al., 2018). Ciceritol can maintain the α–galactoside activity necessary for the plants, but decrease their flatus potential, as it is hydrolyzed slower than RFOs by α–galactosidase. The stachyose synthase gene, representing a connection between RFOs and galactosyl cyclitol pathways, could be targeted in such situations.
Reducing the expression by knockdown or knockout of critical biosynthetic enzymes (GolS, RS, SS) can be an excellent strategy to minimise RFOs accumulation. Regulating myoinositol synthesis by suppressing myo-inositol phosphate synthase (MIPS) expression can also be a potential strategy (Greutert et al., 1998; Hitz et al., 2002; Ma et al., 2005). However, myoinositol is also required for various other functions, such as membrane biogenesis, light responses, receptor cycling, phosphate accumulation and mineral nutrient storage, auxin physiology, fertilization, senescence signalling, and abiotic stress response (Sengupta et al., 2015). Various approaches, such as antisense RNA technology (Greutert et al., 1998; Bock et al., 2009), RNAi approaches (Valentine et al., 2017) and CRISPR/Cas9 technology (Le et al., 2020), have recently been used to downregulate RFOs biosynthetic enzymes (Table 2). According to most studies, out of the major targets (sucrose concentration, myoinositol concentration, GolS, RS, SS), GolS is the most preferred candidate, as it commits galactose towards RFOs biosynthesis (Gangola, 2014; Kannan et al., 2018).
Table 2 Reports on various strategies used for reducing raffinose family oligosaccharides (RFOs) in plants.
Redirection of carbons involved in RFOs biosynthesis to oil or protein can be a good strategy. It has been hypothesized that carbon derived from lipid and protein turnover contributes to RFOs synthesis during the late seed maturation stage. A 10-15% reduction in lipids coincides with RFOs accumulation during seed maturity (Moretti et al., 2020). A protracted buildup of lipids without a reduction in protein content was also seen in recent research employing fast neutron-mutagenized soybean populations with deletions in genes involved in the central carbon metabolism (Kambhampati et al., 2019).
Transgenics require high energy, more time, money and different regulations depending on the country, which makes the varietal release a cumbersome process, especially for feed and food purposes. Plant breeding presents a good alternative in such cases (Kannan et al., 2018). Soybean lines with high sucrose and low RFOs have been developed via germplasm screening and chemical mutagenesis (Hitz et al., 2002; Kannan et al., 2018), which enhanced the possibility of introgressing low RFOs phenotypes into elite genetic backgrounds (Kannan et al., 2018; Hagely et al., 2020). Studies found increased sucrose levels in low RFOs lines (Hitz et al., 2002), the genetic basis of which was associated with a deletion mutation (deletion of 331st tryptophan residue) in the highly conserved coding sequence of the raffinose synthase (RS2) (Dierking and Bilyeu, 2008; Jo et al., 2019). Using the reverse genetics approach, a missense mutation (T107I) in the RS2 gene was identified in soybean (Dierking and Bilyeu, 2009), and an additional mutation in RS3 was also reported to be associated with ultralow RFOs lines (Hagely et al., 2020) (Table 2). Recurrent selection and traditional plant breeding methods resulted in the development of ultralow RFOs (UL RFO) phenotype (seed raffinose and stachyose content < 0.15% and < 0.54%, respectively) (Hagely et al., 2020). Association studies on indel markers with low stachyose content (Qiu et al., 2015) and genotype/environment-modulated carbohydrate profile (Bilyeu and Wiebold, 2016; Jo et al., 2019) are also available. With accelerated genomic sequencing of legumes (Das and Parida, 2013), molecular breeding is emerging as an attractive strategy (Kannan et al., 2018). It is crucial to remember that RFOs have various vital roles in plants; reducing them completely will take a toll on plant survival and yield. Gene targets with minimum hindrance to plant development and growth should be selected, and targeting seed-specific RFOs genes can be promising (de Koning et al., 2021).
In recent decades, considerable progress has been made in understanding the RFOs structural diversity, biosynthesis, translocation, and catabolism. The varied roles of RFOs in plants and animals ask for the optimization of RFOs level to reduce flatulence production without interfering with the normal metabolism of the crop. Such ideal levels need to be ascertained. In the era of global warming, RFOs have the potential to enhance sugar export to phloem and improve crop performance under elevated carbon dioxide. Superimposition of the polymer trap mechanism on apoplastic phloem loaders or vice versa can be an attractive strategy to increase the economic yield of a crop. Sink-specific expression or catabolism of RFOs can modulate the hydrostatic pressure, allowing for a targeted partitioning of photoassimilates.
In future, the connection of RFOs with phytic acid, fructooligosaccharide phenols and methyl ether derivatives of cyclitols can be studied in detail so that the metabolic shift of high phytate crops into RFOs can be engineered. This will facilitate the reduction of antinutrients in crops and a limited increase in RFOs incapable of causing flatulence. Low RFOs lines with increased protein or oil content can also be prepared by altering central carbon metabolism so that they can be used in the vegetable oil industry. Moreover, using several α-galactosidase crude preparations can enhance the nutritional quality of high RFOs crops and fulfil the protein requirement of the community.
RS and SB compiled and wrote the manuscript. SK, AP, and AK provided revisions of scientific content. All authors contributed to the article and approved the submitted version.
The authors declare that the research was conducted in the absence of any commercial or financial relationships that could be construed as a potential conflict of interest.
All claims expressed in this article are solely those of the authors and do not necessarily represent those of their affiliated organizations, or those of the publisher, the editors and the reviewers. Any product that may be evaluated in this article, or claim that may be made by its manufacturer, is not guaranteed or endorsed by the publisher.
Alajaji, S. A., El-Adawy, T. A. (2006). Nutritional composition of chickpea (Cicer arietinum l.) as affected by microwave cooking and other traditional cooking methods. J. Food Compos. Anal. 19, 806–812. doi: 10.1016/J.JFCA.2006.03.015
Amorim, C., Silvério, S. C., Cardoso, B. B., Alves, J. I., Pereira, M. A., Rodrigues, L. R. (2020). In vitro fermentation of raffinose to unravel its potential as prebiotic ingredient. LWT 126, 109322. doi: 10.1016/J.LWT.2020.109322
Andersen, K., Bjergegaard, C., Møller, P., Sørensen, J., Sørensen, H. (2005). Compositional variations for α-galactosides in different species of leguminosae, brassicaceae, and barley: A chemotaxonomic study based on chemometrics and high-performance capillary electrophoresis. J. Agric. Food Chem. 53, 5809–5817. doi: 10.1021/JF040471V
Anggraeni, A. A. (2022). Mini-review: The potential of raffinose as a prebiotic. IOP Conf. Ser.: Earth Environ. Sci. 980, 12033. doi: 10.1088/1755-1315/980/1/012033
Anisha, G. S., Prema, P. (2008). Reduction of non-digestible oligosaccharides in horse gram and green gram flours using crude α-galactosidase from streptomyces griseoloalbus. Food Chem. 106, 1175–1179. doi: 10.1016/J.FOODCHEM.2007.07.058
Arunraj, R., Skori, L., Kumar, A., Hickerson, N. M. N., Shoma, N., Vairamani, M., et al. (2020). Spatial regulation of alpha-galactosidase activity and its influence on raffinose family oligosaccharides during seed maturation and germination in cicer arietinum. Plant Signal. Behav. 15, 1709707. doi: 10.1080/15592324.2019.1709707
Ayre, B. G., Keller, F., Turgeon, R. (2003). Symplastic continuity between companion cells and the translocation stream: Long-distance transport is controlled by retention and retrieval mechanisms in the phloem. Plant Physiol. 131, 1518–1528. doi: 10.1104/PP.012054
Azeke, M. A., Fretzdorff, B., Buening-Pfaue, H., Betsche, T. (2007). Comparative effect of boiling and solid substrate fermentation using the tempeh fungus (Rhizopus oligosporus) on the flatulence potential of African yambean (Sphenostylis stenocarpa l.) seeds. Food Chem. 103, 1420–1425. doi: 10.1016/J.FOODCHEM.2006.10.058
Bamigbade, G. B., Subhash, A. J., Kamal-Eldin, A., Nyström, L., Ayyash, M. (2022). An updated review on prebiotics: insights on potentials of food seeds waste as source of potential prebiotics. Molecules 27. doi: 10.3390/molecules27185947
Bepary, R. H., Wadikar, D. D. (2019). HPLC profiling of flatulence and non-flatulence saccharides in eleven ricebean (Vigna umbellata) varieties from north-East India. J. Food Sci. Technol. 56 (3), 1655–1662. doi: 10.1007/S13197-019-03675-Z
Bilyeu, K. D., Wiebold, W. J. (2016). Environmental stability of seed carbohydrate profiles in soybeans containing different alleles of the raffinose synthase 2 (RS2) gene. J. Agric. Food Chem. 64, 1071–1078. doi: 10.1021/ACS.JAFC.5B04779
Bishi, S. K., Lokesh, K., Mahatma, M. K., Khatediya, N., Chauhan, S. M., Misra, J. B. (2015). Quality traits of Indian peanut cultivars and their utility as nutritional and functional food. Food. Chem. 167, 107–114. doi: 10.1016/j.foodchem.2014.06.076
Blöchl, A., March, G. G., Sourdioux, M., Peterbauer, T., Richter, A. (2005). Induction of raffinose oligosaccharide biosynthesis by abscisic acid in somatic embryos of alfalfa (Medicago sativa l.). Plant Sci. 168, 1075–1082. doi: 10.1016/j.plantsci.2004.12.004
Blöchl, A., Peterbauer, T., Richter, A. (2007). Inhibition of raffinose oligosaccharide breakdown delays germination of pea seeds. J. Plant Physiol. 164, 1093–1096. doi: 10.1016/J.JPLPH.2006.10.010
Bock, C., Ray, H., Georges, F. (2009). Down-regulation of galactinol synthesis in oilseed brassica napus leads to significant reduction of antinutritional oligosaccharides. Botany 87, 597–603. doi: 10.1139/B09-037
Bolouri Moghaddam, M. R., Van Den Ende, W. (2013). Sweet immunity in the plant circadian regulatory network. J. Exp. Bot. 64, 1439–1449. doi: 10.1093/JXB/ERT046
Borisjuk, L., Walenta, S., Rolletschek, H., Mueller-Klieser, W., Wobus, U., Weber, H. (2002). Spatial analysis of plant metabolism: sucrose imaging within vicia faba cotyledons reveals specific developmental patterns. Plant J. 29, 521–530. doi: 10.1046/J.1365-313X.2002.01222.X
Bryant, R. J., Rao, D. R., Ogutu, S. (2003). α and β-galactosidase activities and oligosaccharide content in peanuts. Plant Foods. Hum. Nutr. 58, 213–223. doi: 10.1023/B:QUAL.0000040351.01307.ED
Bueno, R. D., Borges, L. L., Good God, P. I. V., Piovesan, N. D., Teixeira, A. I., Cruz, C. D., et al. (2018). Quantification of anti-nutritional factors and their correlations with protein and oil in soybeans. An. Acad. Bras. Cienc. 90, 205–217. doi: 10.1590/0001-3765201820140465
Cao, T., Lahiri, I., Singh, V., Louis, J., Shah, J., Ayre, B. G. (2013). Metabolic engineering of raffinose-family oligosaccharides in the phloem reveals alterations in carbon partitioning and enhances resistance to green peach aphid. Front. Plant Sci. 4. doi: 10.3389/fpls.2013.00263
Chen, B. X., Fu, H., Gao, J. D., Zhang, Y. X., Huang, W. J., Chen, Z. J., et al. (2022). Identification of metabolomic biomarkers of seed vigor and aging in hybrid rice. Rice 15, 1–12. doi: 10.1186/s12284-022-00552-w
Cho, S. M., Kim, S. H., Kim, Y. C., Yang, Y., Kim, K. S., Choi, Y. S., et al. (2010). Galactinol is involved in induced systemic resistance against bacterial infection and environmental stresses. Korean. J. Plant Resour. 23, 248–255. Available at: https://koreascience.kr/article/JAKO201025665646813.page.
Collins, S. L., McMillan, A., Seney, S., van der Veer, C., Kort, R., Sumarah, M. W., et al. (2018). Promising prebiotic candidate established by evaluation of lactitol, lactulose, raffinose, and oligofructose for maintenance of a lactobacillus-dominated vaginal microbiota. Appl. Environ. Microbiol. 84. doi: 10.1128/AEM.02200-17
Coon, C., Leske, K., Akavanichan, O., Cheng, T. (1990). Effect of oligosaccharide-free soybean meal on true metabolizable energy and fiber digestion in adult roosters. Poult. Sci. 69, 787–793. doi: 10.3382/PS.0690787
Cummings, R. D. (2019). Stuck on sugars – how carbohydrates regulate cell adhesion, recognition, and signaling. Glycoconjugate. J. 364 (36), 241–257. doi: 10.1007/S10719-019-09876-0
Das, A., Parida, S. K. (2013). Advances in biotechnological applications in three important food legumes. Plant Biotechnol. Rep. 8, 83–99. doi: 10.1007/S11816-013-0299-7
de Koning, R., Kiekens, R., Toili, M. E. M., Angenon, G. (2021). Identification and expression analysis of the genes involved in the raffinose family oligosaccharides pathway of phaseolus vulgaris and glycine max. Plants 10, 1465. doi: 10.3390/plants10071465
Dierking, E. C., Bilyeu, K. D. (2008). Association of a soybean raffinose synthase gene with low raffinose and stachyose seed phenotype. Plant Genome 1, 135–145. doi: 10.3835/plantgenome2008.06.0321
Dierking, E., Bilyeu, K. (2009). Raffinose and stachyose metabolism are not required for efficient soybean seed germination. J. Plant Physiol. 166, 1329–1335. doi: 10.1016/J.JPLPH.2009.01.008
dos Santos, T. B., Budzinski, I. G. F., Marur, C. J., Petkowicz, C. L. O., Pereira, L. F. P., Vieira, L. G. E. (2011). Expression of three galactinol synthase isoforms in coffea arabica l. and accumulation of raffinose and stachyose in response to abiotic stresses. Plant Physiol. Biochem. 49, 441–448. doi: 10.1016/J.PLAPHY.2011.01.023
dos Santos, T. B., Vieira, L. G. E. (2020). Involvement of the galactinol synthase gene in abiotic and biotic stress responses: A review on current knowledge. Plant Gene 24, 100258. doi: 10.1016/J.PLGENE.2020.100258
Downie, B., Gurusinghe, S., Dahal, P., Thacker, R. R., Snyder, J. C., Nonogaki, H., et al. (2003). Expression of a galactinol synthase gene in tomato seeds is upregulated before maturation desiccation and again after imbibition whenever radicle protrusion is prevented. Plant Physiol. 131, 1347–1359. doi: 10.1104/PP.016386
Elango, D., Rajendran, K., van der Laan, L., Sebastiar, S., Raigne, J., Thaiparambil, N. A., et al. (2022). Raffinose family oligosaccharides: Friend or foe for human and plant health? Front. Plant Sci. 13. doi: 10.3389/fpls.2022.829118
Elkowicz, K., Sosulski, F. W. (1982). Antinutritive factors in eleven legumes and their air-classified protein and starch fractions. J. Food Sci. 47, 1301–1304. doi: 10.1111/J.1365-2621.1982.TB07673.X
Elsayed, A. I., Rafudeen, M. S., Golldack, D. (2014). Physiological aspects of raffinose family oligosaccharides in plants: Protection against abiotic stress. Plant Biol. 16, 1–8. doi: 10.1111/plb.12053
Frias, J., Bakhsh, A., Jones, D. A., Arthur, A. E., Vidal-Valverde, C., Rhodes, M. J. C., et al. (1999). Genetic analysis of the raffinose oligosaccharide pathway in lentil seeds. J. Exp. Bot. 50, 469–476. doi: 10.1093/jxb/50.333.469
Gamalei, Y. (1989). Structure and function of leaf minor veins in trees and herbs. Trees 3, 96–110. doi: 10.1007/BF01021073
Gangl, R., Tenhaken, R. (2016). Raffinose family oligosaccharides act as galactose stores in seeds and are required for rapid germination of arabidopsis in the dark. Front. Plant Sci. 7. doi: 10.3389/fpls.2016.01115
Gangola, M. P. (2014). Raffinose family oligosaccharides (RFO) biosynthesis in chickpea (cicer arietinum l.) seeds. [Doctoral dissertation]. Department of Plant Sciences, University of Saskatchewan. Available at: https://scholar.google.com/scholar?hl=en&as_sdt=0%2C5&q=gangola+2014+raffinose&btnG=
Gawłowska, M., Lahuta, L., Boros, L., Sawikowska, A., Kumar, P., Knopkiewicz, M., et al. (2022). Validation of molecular markers significant for flowering time, plant lodging, stem geometry properties, and raffinose family oligosaccharides in pea (Pisum sativum l.). Agriculture 12, 1125. doi: 10.3390/AGRICULTURE12081125
Gilmour, S. J., Sebolt, A. M., Salazar, M. P., Everard, J. D., Thomashow, M. F. (2000). Overexpression of the arabidopsis CBF3 transcriptional activator mimics multiple biochemical changes associated with cold acclimation. Plant Physiol. 124, 1854–1865. doi: 10.1104/PP.124.4.1854
Glencross, B. D., Boujard, T., Kaushik, S. J. (2003). Influence of oligosaccharides on the digestibility of lupin meals when fed to rainbow trout, oncorhynchus mykiss. Aquaculture 219, 703–713. doi: 10.1016/S0044-8486(02)00664-6
Gómez-Ariza, J., Campo, S., Rufat, M., Estopà, M., Messeguer, J., Segundo, B. S., et al. (2007). Sucrose-mediated priming of plant defense responses and broad-spectrum disease resistance by overexpression of the maize pathogenesis-related PRms protein in rice plants. Mol. Plant Microbe Interact. 20, 832–842. doi: 10.1094/MPMI-20-7-0832
Greutert, H., Martinoia, E., Keller, F. (1998). Mannitol transport by vacuoles of storage parenchyma of celery petioles operates by facilitated diffusion. J. Plant Physiol. 153, 91–96. doi: 10.1016/S0176-1617(98)80050-3
Gu, L., Jiang, T., Zhang, C., Li, X., Wang, C., Zhang, Y., et al. (2019). Maize HSFA2 and HSBP2 antagonistically modulate raffinose biosynthesis and heat tolerance in arabidopsis. Plant J. 100, 128–142. doi: 10.1111/TPJ.14434
Gu, L., Zhang, Y., Zhang, M., Li, T., Dirk, L. M. A., Downie, B., et al. (2016). ZmGOLS2, a target of transcription factor ZmDREB2A, offers similar protection against abiotic stress as ZmDREB2A. Plant Mol. Biol. 90, 157–170. doi: 10.1007/s11103-015-0403-1
Haab, C. I., Keller, F. (2002). Purification and characterization of the raffinose oligosaccharide chain elongation enzyme, galactan:galactan galactosyltransferase (GGT), from ajuga reptans leaves. Physiol. Plant 114, 361–371. doi: 10.1034/j.1399-3054.2002.1140305.x
Hagely, K. B., Jo, H., Kim, J.-H., Hudson, K. A., Bilyeu, K. (2020). Molecular-assisted breeding for improved carbohydrate profiles in soybean seed. Theor. Appl. Genet. 133 (4), 1189–1200. doi: 10.1007/S00122-020-03541-Z
Han, Q., Qi, J., Hao, G., Zhang, C., Wang, C., Dirk, L. M. A., et al. (2020). ZmDREB1A regulates RAFFINOSE SYNTHASE controlling raffinose accumulation and plant chilling stress tolerance in maize. Plant Cell Physiol. 61, 331–341. doi: 10.1093/PCP/PCZ200
Hannah, M. A., Zuther, E., Buchel, K., Heyer, A. G. (2006). Transport and metabolism of raffinose family oligosaccharides in transgenic potato. J. Exp. Bot. 57, 3801–3811. doi: 10.1093/jxb/erl152
Haritatos, E., Keller, F., Turgeon, R. (2017). Raffinose oligosaccharide concentrations measured in individual cell and tissue types in cucumis melo l. leaves: implications for phloem loading. Planta. 1996. 198 (4), 614–622. doi: 10.1007/BF00262649
Herman, E., Larkins, B. (1999). Protein storage bodies and vacuoles. Plant Cell 11, 601–613. doi: 10.1105/TPC.11.4.601
Hewer, A., Will, T., van Bel, ,. A. J. E. (2010). Plant cues for aphid navigation in vascular tissues. J. Exp. Biol. 213, 4030–4042. doi: 10.1242/jeb.046326
Hincha, D., Zuther, E., Heyer, A. (2003). The preservation of liposomes by raffinose family oligosaccharides during drying is mediated by effects on fusion and lipid phase transitions. Biochim. Et. Biophys. Acta (BBA). - Biomembranes. 1612 (2), 172–177. doi: 10.1016/s0005-2736(03)00116-0
Hitz, W. D., Carlson, T. J., Kerr, P. S., Sebastian, S. A. (2002). Biochemical and molecular characterization of a mutation that confers a decreased raffinosaccharide and phytic acid phenotype on soybean seeds. Plant Physiol. 128, 650–660. doi: 10.1104/PP.010585
Hoch, G., Peterbauer, T., Richter, A. (1999). Purification and characterization of stachyose synthase from lentil (Lens culinaris) seeds: Galactopinitol and stachyose synthesis. Arch. Biochem. Biophys. 366, 75–81. doi: 10.1006/abbi.1999.1212
Hollung, K., Øverland., M., Hrustič, M., Sekulič, P., Miladinovič, J., Martens, H., et al. (2005). Evaluation of nonstarch polysaccharides and oligosaccharide content of different soybean varieties (Glycine max) by near-infrared spectroscopy and proteomics. J. Agric. Food Chem. 53, 9112–9121. doi: 10.1021/JF051438R
Horbowicz, M., Obendorf, R. L. (1994). Seed desiccation tolerance and storability: Dependence on flatulence-producing oligosaccharides and cyclitols–review and survey. Seed. Sci. Res. 4, 385–405. doi: 10.1017/S0960258500002440
Hua, B., Zhang, M., Zhang, J., Dai, H., Zhang, Z., Miao, M. (2021). CsAGA1 and CsAGA2 mediate RFO hydrolysis in partially distinct manner in cucumber fruits. Int. J. Mol. Sci. 22, 13285. doi: 10.3390/IJMS222413285
Huynh, B. L., Palmer, L., Mather, D. E., Wallwork, H., Graham, R. D., Welch, R. M., et al. (2008). Genotypic variation in wheat grain fructan content revealed by a simplified HPLC method. J. Cereal Sci. 48, 369–378. doi: 10.1016/J.JCS.2007.10.004
Jaglo, K. R., Kleff, S., Amundsen, K. L., Zhang, X., Haake, V., Zhang, J. Z., et al. (2001). Components of the arabidopsis c-repeat/dehydration-responsive element binding factor cold-response pathway are conserved in brassica napus and other plant species. Plant Physiol. 127, 910–917. doi: 10.1104/PP.010548
Jang, J. M., Yang, Y., Wang, R., Bao, H., Yuan, H., Yang, J. (2019). Characterization of a high performance α-galactosidase from irpex lacteus and its usage in removal of raffinose family oligosaccharides from soymilk. Int. J. Biol. Macromol. 131, 1138–1146. doi: 10.1016/j.ijbiomac.2019.04.060
Jauh, G., Phillips, T., Rogers, J. (1999). Tonoplast intrinsic protein isoforms as markers for vacuolar functions. Plant Cell 11, 1867. doi: 10.1105/TPC.11.10.1867
Jiang, Q., Wu, W., Wan, Y., Wei, Y., Kawamura, Y., Li, J., et al. (2022). Energy values evaluation and improvement of soybean meal in broiler chickens through supplemental mutienzyme. Poult. Sci. 101, 101978. doi: 10.1016/j.psj.2022.101978
Jing, Y., Lang, S., Wang, D., Xue, H., Wang, X. F. (2018). Functional characterization of galactinol synthase and raffinose synthase in desiccation tolerance acquisition in developing arabidopsis seeds. J. Plant Physiol. 230, 109–121. doi: 10.1016/J.JPLPH.2018.10.011
Jo, H., Lorenz, A. J., Rainey, K. M., Shannon, J. G., Chen, P., Bilyeu, K. D. (2019). Environmental stability study of soybeans with modified carbohydrate profiles in maturity groups 0 to V. Crop Sci. 59, 1531–1543. doi: 10.2135/CROPSCI2018.09.0600
Kambhampati, S., Aznar-Moreno, J. A., Hostetler, C., Caso, T., Bailey, S. R., Hubbard, A. H., et al. (2019). On the inverse correlation of protein and oil: Examining the effects of altered central carbon metabolism on seed composition using soybean fast neutron mutants. Metabolites 10, 18. doi: 10.3390/METABO10010018
Kannan, U., Sharma, R., Gangola, M. P., Sari, N., Chibbar, R. N. (2018). Improving grain quality in pulses: S trategies to reduce raffinose family oligosaccharides in. Ekin. J. Crop Breed. Genet. 4, 70–88. Available at: https://dergipark.org.tr/en/pub/ekinjournal/issue/36232/408599.
Karner, U., Peterbauer, T., Raboy, V., Jones, D. A., Hedley, C. L., Richter, A. (2004). Myo-inositol and sucrose concentrations affect the accumulation of raffinose family oligosaccharides in seeds. J. Exp. Bot. 55, 1981–1987. doi: 10.1093/jxb/erh216
Kasprowicz-Potocka, M., Gulewicz, P., Zaworska-Zakrzewska, A. (2022). The content of raffinose oligosaccharides in legumes and their importance for animals. J. Anim. Feed Sci. 31, 265–275. doi: 10.22358/jafs/149656/2022
Katrolia, P., Rajashekhara, E., Yan, Q., Jiang, Z. (2014). Biotechnological potential of microbial α-galactosidases. Crit. Rev. Biotechnol. 34, 307–317. doi: 10.3109/07388551.2013.794124
Keller, F., Matile, P. (1985). The role of the vacuole in storage and mobilization of stachyose in tubers of stachys sieboldii. J. Plant Physiol. 119, 369–380. doi: 10.1016/S0176-1617(85)80104-8
Keller, R., Brearley, C. A., Trethewey, R. N., Müller-Röber, B. (1998). Reduced inositol content and altered morphology in transgenic potato plants inhibited for 1D- myo -inositol 3-phosphate synthase. Plant J. 16, 403–410. doi: 10.1046/J.1365-313X.1998.00309.X
Keller, I., Müdsam, C., Rodrigues, C. M., Kischka, D., Zierer, W., Sonnewald, U., et al. (2021). Cold-triggered induction of ROS- and raffinose metabolism in freezing-sensitive taproot tissue of sugar beet. Front. Plant Sci. 12. doi: 10.3389/fpls.2021.715767
Keller, F., Pharr, ,. D. M. (1996). “Metabolism of carbohydrates in sinks and sources: galactosyl-sucrose oligosaccharides,” in Photoassimilate distribution in plants and crops. Eds. Zamski, E., Schaffer, A. A. (Milton Park: Taylor & Francis), 115–184.
Khan, M., Hu, J., Dahro, B., Ming, R., Zhang, Y., Wang, Y., et al. (2021). ERF108 from poncirus trifoliata (L.) raf. functions in cold tolerance by modulating raffinose synthesis through transcriptional regulation of PtrRafS. Plant J. 108, 705–724. doi: 10.1111/TPJ.15465
Kim, M. S., Cho, S. M., Kang, E. Y., Im, Y. J., Hwangbo, H., Kim, Y. C., et al. (2008). Galactinol is a signaling component of the induced systemic resistance caused by pseudomonas chlororaphis O6 root colonization. Mol. Plant Microbe Interact. 21, 1643–1653. doi: 10.1094/MPMI
Koster, K. L. (1991). Glass formation and desiccation tolerance in seeds. Plant Physiol. 96, 302–304. doi: 10.1104/pp.96.1.302
Kumar, S., Sasi, M., Bishi, S., Sanyal, R. (2022). Role of probiotic α-galactosidases in the reduction of flatulence causing raffinose oligosaccharides (RFOs). Biot. Res. Today 4, 640–642. https://www.biospub.com/index.php/biorestoday/article/view/1650.
Kumar, V., Rani, A., Goyal, L., Dixit, A. K., Manjaya, J. G., Dev, J., et al. (2010). Sucrose and raffinose family oligosaccharides (RFOs) in soybean seeds as influenced by genotype and growing location. J. Agric. Food Chem. 58, 5081–5085. doi: 10.1021/jf903141s
La Mantia, J., Unda, F., Douglas, C. J., Mansfield, S. D., Hamelin, R. (2018). Overexpression of AtGolS3 and CsRFS in poplar enhances ROS tolerance and represses defense response to leaf rust disease. Tree Physiol. 38, 457–470. doi: 10.1093/TREEPHYS/TPX100
Lang, S., Liu, X., Xue, H., Li, X., Wang, X. (2017). Functional characterization of BnHSFA4a as a heat shock transcription factor in controlling the re-establishment of desiccation tolerance in seeds. J. Exp. Bot. 68, 2361–2375. doi: 10.1093/jxb/erx097.4
Lata, C., Prasad, M. (2011). Role of DREBs in regulation of abiotic stress responses in plants. J. Exp. Bot. 62, 4731–4748. doi: 10.1093/JXB/ERR210
Lattimer, J. M., Haub, ,. M. D. (2010). Effects of dietary fiber and its components on metabolic health. Nutrients 2, 1266–1289. doi: 10.3390/nu2121266
Le, H., Nguyen, N. H., Ta, D. T., Le, T. N. T., Bui, T. P., Le, N. T., et al. (2020). CRISPR/Cas9-mediated knockout of galactinol synthase-encoding genes reduces raffinose family oligosaccharide levels in soybean seeds. Front. Plant Sci. 11. doi: 10.3389/fpls.2020.612942
Leopold, A. C., Sun, W. Q., Bernal-Lugo, I. (1994). The glassy state in seeds: analysis and function. Seed. Sci. Res. 4, 267–274. doi: 10.1017/S0960258500002294
Li, T., Zhang, Y., Liu, Y., Li, X., Hao, G., Han, Q., et al. (2020). Raffinose synthase enhances drought tolerance through raffinose synthesis or galactinol hydrolysis in maize and arabidopsis plants. J. Biol. Chem. 295, 8064–8077. doi: 10.1074/JBC.RA120.013948
Li, T., Zhang, Y., Wang, D., Liu, Y., Dirk, L. M. A., Goodman, J., et al. (2017). Regulation of seed vigor by manipulation of raffinose family oligosaccharides in maize and arabidopsis thaliana. Mol. Plant 10, 1540–1555. doi: 10.1016/j.molp.2017.10.014
Liu, J. J. J., Krenz, D. C., Galvez, A. F., De Lumen, B. O. (1998). Galactinol synthase (GS): increased enzyme activity and levels of mRNA due to cold and desiccation. Plant Sci. 134, 11–20. doi: 10.1016/S0168-9452(98)00042-9
Liu, H., Liu, X., Zhao, Y., Nie, J., Yao, X., Lv, L., et al. (2022a). Alkaline α-galactosidase 2 (CsAGA2) plays a pivotal role in mediating source–sink communication in cucumber. Plant Physiol. 189, 1501–1518. doi: 10.1093/plphys/kiac152
Liu, Q., Ding, J., Huang, W., Yu, H., Wu, S., Li, W., et al. (2022b). OsPP65 negatively regulates osmotic and salt stress responses through regulating phytohormone and raffinose family oligosaccharide metabolic pathways in rice. Rice 15, 34. doi: 10.1186/s12284-022-00581-5
Llamas-Moya, S., Higgins, N. F., Adhikari, R., Lawlor, P. G., Lacey, S. (2021). Effect of multicarbohydrase enzymes containing α-galactosidase on the growth and apparent metabolizable energy digestibility of broiler chickens: a meta-analysis. Anim. Feed. Sci. Technol. 277, 114949. doi: 10.1016/j.anifeedsci.2021.114949
Lu, J., Wang, Z., Long, X., Fang, Y., Zhou, M., Yang, J., et al. (2022). Characterization and expression profiles of galaetinol synthase and raffinose synthase in rubber tree. Comput. Mol. Biol. 12, 1–7. doi: 10.5376/CMB.2022.12.0002
Ma, J. M., Horbowicz, M., Obendorf, R. L. (2005). Cyclitol galactosides in embryos of buckwheat stem–leaf–seed explants fed d-chiro-inositol, myo-inositol or d-pinitol. Seed. Sci. Res. 15, 329–338. doi: 10.1079/SSR2005221
Ma, S., Lv, J., Li, X., Ji, T., Zhang, Z., Gao, L. (2021). Galactinol synthase gene 4 (CsGolS4) increases cold and drought tolerance in cucumis sativus l. by inducing RFO accumulation and ROS scavenging. Environ. Exp. Bot. 185, 104406. doi: 10.1016/J.ENVEXPBOT.2021.104406
Madore, M. A. (2001). “Biosynthesis and degradation of galactosyloligosaccharides,” in Glycoscience: Chemistry and chemical biology I-III. Eds. Fraser-Reid, B. O., Tatsuta, K., Thiem, J. (Berlin, Heidelberg: Springer-Verlag Berlin Heidelberg), 1662–1690. doi: 10.1007/978-3-642-56874-9
Mao, B., Tang, H., Gu, J., Li, D., Cui, S., Zhao, J., et al. (2018). In vitro fermentation of raffinose by the human gut bacteria. Food Funct. 9, 5824–5831. doi: 10.1039/c8fo01687a
Martín-Cabrejas, M. A., Díaz, M. F., Aguilera, Y., Benítez, V., Mollá, E., Esteban, R. M. (2008). Influence of germination on the soluble carbohydrates and dietary fibre fractions in non-conventional legumes. Food Chem. 107, 1045–1052. doi: 10.1016/J.FOODCHEM.2007.09.020
Martínez-Villaluenga, C., Frias, J., Vidal-Valverde, C. (2008). Alpha-galactosides: Antinutritional factors or functional ingredients? Crit. Rev. Food Sci. Nutr. 48, 301–316. doi: 10.1080/10408390701326243
Maruyama, K., Takeda, M., Kidokoro, S., Yamada, K., Sakuma, Y., Urano, K., et al. (2009). Metabolic pathways involved in cold acclimation identified by integrated analysis of metabolites and transcripts regulated by DREB1A and DREB2A. Plant Physiol. 150, 1972–1980. doi: 10.1104/PP.109.135327
Minorsky, P. V. (2003). The hot and the classic. Plant Physiol. 131, 1159–1160. doi: 10.1104/PP.900066
Morkunas, I., Ratajczak, L. (2014). The role of sugar signaling in plant defense responses against fungal pathogens. Acta Physiol. Plant 36, 1607–1619. doi: 10.1007/S11738-014-1559-Z
Moretti, A., Arias, C. L., Mozzoni, L. A., Chen, P., McNeece, B. T., Mian, M. A. R., et al. (2020). Workflow for the quantification of soluble and insoluble carbohydrates in soybean seed. Molecules 25. doi: 10.3390/molecules25173806
Mulimani, V. H., Devendra, S. (1998). Effect of soaking, cooking and crude α-galactosidase treatment on the oligosaccharide content of red gram flour. Food Chem. 61, 475–479. doi: 10.1016/S0308-8146(97)00142-8
Naczk, M., Amarowicz, R., Shahidi, F. (1997). α-galactosides of sucrose in foods: Composition, flatulence-causing effects, and removal. ACS Symp. Ser. 662, 127–151. doi: 10.1021/BK-1997-0662.CH008
Nishizawa, A., Yabuta, Y., Shigeoka, S. (2008). Galactinol and raffinose constitute a novel function to protect plants from oxidative damage. Plant Physiol. 147, 1251. doi: 10.1104/PP.108.122465
Oboh, H. A., Muzquiz, M., Burbano, C., Cuadrado, C., Pedrosa, M. M., Ayet, G., et al. (2000). Effect of soaking, cooking and germination on the oligosaccharide content of selected Nigerian legume seeds. Plant Foods. Hum. Nutr. 55 (2), 97–110. doi: 10.1023/A:1008133531726
Onigbinde, A. O., Akinyele, I. O. (1983). Oligosaccharide content of 20 varieties of cowpeas in Nigeria. J. Food Sci. 48, 1250–1251. doi: 10.1111/J.1365-2621.1983.TB09203.X
Panikulangara, T., Eggers-Schumacher, G., Wunderlich, M., Stransky, H., Schöffl, F. (2004). Galactinol synthase1. a novel heat shock factor target gene responsible for heat-induced synthesis of raffinose family oligosaccharides in arabidopsis. Plant Physiol. 136, 3148–3158. doi: 10.1104/PP.104.042606
Pattee, H., Isleib, T., Giesbrecht, F., McFeeters, R. (2000). Relationships of sweet, bitter, and roasted peanut sensory attributes with carbohydrate components in peanuts. J. Agric. Food Chem. 48, 757–763. doi: 10.1021/JF9910741
Peshev, D., Vergauwen, R., Moglia, A., Hideg, E., Van den Ende, W. (2013). Towards understanding vacuolar antioxidant mechanisms: a role for fructans? J. Exp. Bot. 64, 1025–1038. doi: 10.1093/jxb/ers377
Peterbauer, T., Karner, U., Mucha, J., Mach, L., Jones, D. A., Hedley, C. L., et al. (2003). Enzymatic control of the accumulation of verbascose in pea seeds. Plant Cell Environ. 26, 1385–1391. doi: 10.1046/J.0016-8025.2003.01063.X
Peterbauer, T., Lahuta, L. B., Blöchl, A., Mucha, J., Jones, D. A., Hedley, C. L., et al. (2001). Analysis of the raffinose family oligosaccharide pathway in pea seeds with contrasting carbohydrate composition. Plant Physiol. 127, 1764–1772. doi: 10.1104/PP.010534
Peterbauer, T., Richter, A. (2001). Biochemistry and physiology of raffinose family oligosaccharides and galactosyl cyclitols in seeds. Seed. Sci. Res. 11, 185–197. doi: 10.1079/SSR200175
Polowick, P. L., Baliski, D. S., Bock, C., Ray, H., Georges, F. (2009). Over-expression of α-galactosidase in pea seeds to reduce raffinose oligosaccharide content. Botany 87, 526–532. doi: 10.1139/B09-020
Pugalenthi, M., Vadivel, V. (2006). Agrobiodiversity of eleven accessions of mucuna pruriens (L.) DC. var. utilis (Wall. ex Wight) baker ex burck (velvet bean) collected from four districts of south India. Genet. Resour. Crop Evol. 54, 1117–1124. doi: 10.1007/S10722-006-9003-X
Qiu, D., Vuong, T., Valliyodan, B., Shi, H., Guo, B., Shannon, J. G., et al. (2015). Identification and characterization of a stachyose synthase gene controlling reduced stachyose content in soybean. Theor. Appl. Genet. 128, 2167–2176. doi: 10.1007/s00122-015-2575-0
Rackis, J. J. (1975). “Oligosaccharides of food legumes: Alpha-galactosidase activity and the flatus problem,” in Physiological effects of food carbohydrates. Jeanes, A., Hodge, J. eds. (Washington, D. C.: American Chemical Society), 207–222. doi: 10.1021/bk-1975-0015.ch013
Reddy, N. R., Pierson, M. D., Sathe, S. K., Salunkhe, D. K. (1984). Chemical, nutritional and physiological aspects of dry bean carbohydrates–a review. Food Chem. 13, 25–68. doi: 10.1016/0308-8146(84)90026-8
Redekar, N. R., Glover, N. M., Biyashev, R. M., Ha, B. K., Raboy, V., Maroof, M. A. S. (2020). Genetic interactions regulating seed phytate and oligosaccharides in soybean (Glycine max l.). PloS One 15, 1–18. doi: 10.1371/journal.pone.0235120
Rolland, F., Baena-Gonzalez, E., Sheen, J. (2006). Sugar sensing and signaling in plants: conserved and novel mechanisms. Annu. Rev. Plant Biol. 57, 675–709. doi: 10.1146/ANNUREV.ARPLANT.57.032905.105441
Ruiz-López, M. A., García-López, P. M., Castañeda-Vazquez, H., Zamora, N. J. F., Garzón-De La Mora, P., Bañuelos Pineda, J., et al. (2000). Chemical composition and antinutrient content of three lupinus species from jalisco, Mexico. J. Food Compos. Anal. 13, 193–199. doi: 10.1006/JFCA.1999.0887
Ryu, C. M., Kang, B. R., Han, S. H., Cho, S. M., Kloepper, J. W., Anderson, A. J., et al. (2007). Tobacco cultivars vary in induction of systemic resistance against cucumber mosaic virus and growth promotion by pseudomonas chlororaphis O6 and its gacS mutant. Eur. J. Plant Pathol. 119, 383–390. doi: 10.1007/S10658-007-9168-Y
Salvi, P., Kamble, N. U., Majee, M. (2020). Ectopic over-expression of ABA-responsive chickpea galactinol synthase (CaGolS) gene results in improved tolerance to dehydration stress by modulating ROS scavenging. Environ. Exp. Bot. 171, 103957. doi: 10.1016/j.envexpbot.2019.103957
Salvi, P., Saxena, S. C., Petla, B. P., Kamble, N. U., Kaur, H., Verma, P., et al. (2016). Differentially expressed galactinol synthase(s) in chickpea are implicated in seed vigor and longevity by limiting the age induced ROS accumulation. Sci. Rep. 6, 35088. doi: 10.1038/srep35088
Sanyal, R., Bishi, S. K. (2021). Reduction of flatus sugars: An approach towards nutritional enhancement. Biot. Res. Today 3, 897–900. Available at: https://www.biospub.com/index.php/biorestoday/article/view/1158.
Sanyal, R., Pradhan, B., Jawed, D. M., Tribhuvan, K. U., Dahuja, A., Kumar, M., et al. (2023). Spatio-temporal expression pattern of raffinose synthase genes determine the levels of raffinose family oligosaccharides in peanut (Arachis hypogaea l.) seed. Sci. Rep. 13, 795. doi: 10.1038/s41598-023-27890-z
Sasi, M., Kumar, S., Hasan, M., Arpitha, S. R., Garcia-Gutierrez, E., Kumari, S., et al. (2022). Current trends in the development of soy-based foods containing probiotics and paving the path for soy-synbiotics. Crit. Rev. Food Sci. Nutr., 1–19. doi: 10.1080/10408398.2022.2078272
Schramm, F., Ganguli, A., Kiehlmann, E., Englich, G., Walch, D., von Koskull-Döring, P. (2006). The heat stress transcription factor HsfA2 serves as a regulatory amplifier of a subset of genes in the heat stress response in arabidopsis. Plant Mol. Biol. 60, 759–772. doi: 10.1007/S11103-005-5750-X
Sengupta, S., Mukherjee, S., Basak, P., Majumder, A. L. (2015). Significance of galactinol and raffinose family oligosaccharide synthesis in plants. Front. Plant Sci. 6. doi: 10.3389/fpls.2015.00656
Sharma, P., Goudar, G., Kumar Chandragiri, A., Ananthan, R., Subhash, K., Chauhan, A., et al. (2023). Assessment of diversity in antinutrient profile, resistant starch, minerals and carbohydrate components in different ricebean (Vigna umbellata) accessions. Food Chem. 405, 134835. doi: 10.1016/j.foodchem.2022.134835
Shehata, A. M., Paswan, V. K., Attia, Y. A., Abougabal, M. S., Khamis, T., Alqosaibi, A. I., et al. (2022). In ovo inoculation of bacillus subtilis and raffinose affects growth performance, cecal microbiota, volatile fatty acid, ileal morphology and gene expression, and sustainability of broiler chickens (Gallus gallus). Front. Nutr. 9. doi: 10.3389/fnut.2022.903847
Sprenger, N., Keller, F. (2000). Allocation of raffinose family oligosaccharides to transport and storage pools in ajuga reptans: the roles of two distinct galactinol synthases. Plant J. 21, 249–258. doi: 10.1046/J.1365-313X.2000.00671.X
Sun, Q., Huang, R., Zhu, H., Sun, Y., Guo, Z. (2021). A novel medicago truncatula calmodulin-like protein (MtCML42) regulates cold tolerance and flowering time. Plant J. 108, 1069–1082. doi: 10.1111/TPJ.15494
Sun, X., Matus, J. T., Wong, D. C. J., Wang, Z., Chai, F., Zhang, L., et al. (2018). The GARP/MYB-related grape transcription factor AQUILO improves cold tolerance and promotes the accumulation of raffinose family oligosaccharides. J. Exp. Bot. 69, 1749–1764. doi: 10.1093/jxb/ery020
Taji, T., Ohsumi, C., Iuchi, S., Seki, M., Kasuga, M., Kobayashi, M., et al. (2002). Important roles of drought- and cold-inducible genes for galactinol synthase in stress tolerance in arabidopsis thaliana. Plant J. 29, 417–426. doi: 10.1046/J.0960-7412.2001.01227.X
Teixeira, J., McNeill, V., Gänzle, M. (2012). Levansucrase and sucrose phoshorylase contribute to raffinose, stachyose, and verbascose metabolism by lactobacilli. Food Microbiol. 31, 278–284. doi: 10.1016/J.FM.2012.03.003
Tian, C., Yang, J., Zeng, Y., Zhang, T., Zhou, Y., Men, Y., et al. (2019). Biosynthesis of raffinose and stachyose from sucrose via an In vitro multienzyme system. Appl. Environ. Microbiol. 85, e02306–18. doi: 10.1128/AEM.02306-18
Trugo, L. C., Almeida, D. C. F., Gross, R. (1988). Oligosaccharide contents in the seeds of cultivated lupins. J. Sci. Food Agric. 45, 21–24. doi: 10.1002/JSFA.2740450104
Turgeon, R. (1996). Phloem loading and plasmodesmata. Trends Plant Sci. 1, 418–423. doi: 10.1016/S1360-1385(96)10045-5
Turgeon, R., Medville, R. (2004). Phloem loading. a reevaluation of the relationship between plasmodesmatal frequencies and loading strategies. Plant Physiol. 136, 3795–3803. doi: 10.1104/PP.104.042036
Turgeon, R., Medville, R., Nixon, K. C. (2001). The evolution of minor vein phloem and phloem loading. Am. J. Bot. 88, 1331–1339. doi: 10.2307/3558441
Unda, F., Canam, T., Preston, L., Mansfield, S. D. (2012). Isolation and characterization of galactinol synthases from hybrid poplar. J. Exp. Bot. 63, 2059–2069. doi: 10.1093/JXB/ERR411
Unda, F., Kim, H., Hefer, C., Ralph, J., Mansfield, S. D. (2017). Altering carbon allocation in hybrid poplar (Populus alba × grandidentata) impacts cell wall growth and development. Plant Biotechnol. J. 15, 865–878. doi: 10.1111/PBI.12682
Valentine, M. F., De Tar, J. R., Mookkan, M., Firman, J. D., Zhang, Z. J. (2017). Silencing of soybean raffinose synthase gene reduced raffinose family oligosaccharides and increased true metabolizable energy of poultry feed. Front. Plant Sci. 8. doi: 10.3389/fpls.2017.00692
van Barneveld, R. (1999). Understanding the nutritional chemistry of lupin (Lupinus spp.) seed to improve livestock production efficiency. Nutr. Res. Rev. 12, 203–230. doi: 10.1079/095442299108728938
Vanhaecke, M., Dyubankova, N., Lescrinier, E., Van Den Ende, W. (2010). Metabolism of galactosyl-oligosaccharides in stellaria media–discovery of stellariose synthase, a novel type of galactosyltransferase. Phytochemistry 71, 1095–1103. doi: 10.1016/J.PHYTOCHEM.2010.04.012
Vidal-Valverde, C., Frias, J., Hernández, A., Martín-Alvarez, P. J., Sierra, I., Rodríguez, C., et al. (2003). Assessment of nutritional compounds and antinutritional factors in pea (Pisum sativum) seeds. J. Sci. Food Agric. 83, 298–306. doi: 10.1002/JSFA.1309
Vidal-Valverde, C., Frías, J., Valverde, S. (1993). Changes in the carbohydrate composition of legumes after soaking and cooking. J. Am. Diet. Assoc. 93, 547–550. doi: 10.1016/0002-8223(93)91814-7
Vinson, C. C., Mota, A. P. Z., Porto, B. N., Oliveira, T. N., Sampaio, I., Lacerda, A. L., et al. (2020). Characterization of raffinose metabolism genes uncovers a wild arachis galactinol synthase conferring tolerance to abiotic stresses. Sci. Rep. 10, 1–19. doi: 10.1038/s41598-020-72191-4
Wang, T. L., Domoney, C., Hedley, C. L., Casey, R., Grusak, M. A. (2003). Can we improve the nutritional quality of legume seeds? Plant Physiol. 131, 886–891. doi: 10.1104/PP.102.017665
Wang, X., Li, S., Zhang, X., Gao, L., Ruan, Y. L., Tian, Y., et al. (2022). From raffinose family oligosaccharides to sucrose and hexoses: Gene expression profiles underlying host-to-Nematode carbon delivery in cucumis sativus roots. Front. Plant Sci. 13. doi: 10.3389/FPLS.2022.823382
Wang, Z., Zhu, Y., Wang, L., Liu, X., Liu, Y., Phillips, J., et al. (2009). A WRKY transcription factor participates in dehydration tolerance in boea hygrometrica by binding to the W-box elements of the galactinol synthase (BhGolS1) promoter. Planta 230 (6), 1155–1166. doi: 10.1007/S00425-009-1014-3
Yadav, U. P., Ayre, B. G., Bush, D. R. (2015). Transgenic approaches to altering carbon and nitrogen partitioning in whole plants: assessing the potential to improve crop yields and nutritional quality. Front. Plant Sci. 0. doi: 10.3389/FPLS.2015.00275
Yan, S., Huang, W., Gao, J., Fu, H., Liu, J. (2018). Comparative metabolomic analysis of seed metabolites associated with seed storability in rice (Oryza sativa l.) during natural aging. Plant Physiol. Biochem. 127, 590–598. doi: 10.1016/J.PLAPHY.2018.04.020
Yan, S., Liu, Q., Li, W., Yan, J., Fernie, A. R. (2022). Raffinose family oligosaccharides: Crucial regulators of plant development and stress responses. CRC. Crit. Rev. Plant Sci. 41, 286–303. doi: 10.1080/07352689.2022.2111756
Yang, J., Ling, C., Liu, Y., Zhang, H., Hussain, Q., Lyu, S., et al. (2022). Genome-wide expression profiling analysis of kiwifruit GolS and RFS genes and identification of AcRFS4 function in raffinose accumulation. Int. J. Mol. Sci. 23, 8836. doi: 10.3390/ijms23168836
Zartl, B., Silberbauer, K., Loeppert, R., Viernstein, H., Praznik, W., Mueller, M. (2018). Fermentation of non-digestible raffinose family oligosaccharides and galactomannans by probiotics. Food Funct. 9, 1638–1646. doi: 10.1039/c7fo01887h
Zhang, H., Sun, Z., Feng, S., Zhang, J., Zhang, F., Wang, W., et al. (2022). The C2H2-type zinc finger protein PhZFP1 regulates cold stress tolerance by modulating galactinol synthesis in petunia hybrida. J. Exp. Bot. 73, 6434–6448. doi: 10.1093/jxb/erac274
Zhang, J., Song, G., Mei, Y., Li, R., Zhang, H., Liu, Y. (2019). Present status on removal of raffinose family oligosaccharides - a review. Czech. J. Food Sci. 37, 141–154. doi: 10.17221/472/2016-CJFS
Zhang, Y., Li, D., Dirk, L. M. A., Downie, A. B., Zhao, T. (2021). ZmAGA1 hydrolyzes RFOs late during the lag phase of seed germination, shifting sugar metabolism toward seed germination over seed aging tolerance. J. Agric. Food Chem. 69, 11606–11615. doi: 10.1021/ACS.JAFC.1C03677
Zhawar, V. K., Kaur, N., Gupta, A. K. (2011). Phytic acid and raffinose series oligosaccharides metabolism in developing chickpea seeds. Physiol. Mol. Biol. Plants 17, 355. doi: 10.1007/S12298-011-0080-8
Zhou, M.-L., Zhang, Q., Zhou, M., Sun, Z.-M., Zhu, X.-M., Shao, J.-R., et al. (2012). Genome-wide identification of genes involved in raffinose metabolism in maize. Glycobiology 22, 1775–1785. doi: 10.1093/GLYCOB/CWS121
Keywords: abiotic stress, antinutritional factors, flatulence, raffinose, stachyose
Citation: Sanyal R, Kumar S, Pattanayak A, Kar A and Bishi SK (2023) Optimizing raffinose family oligosaccharides content in plants: A tightrope walk. Front. Plant Sci. 14:1134754. doi: 10.3389/fpls.2023.1134754
Received: 30 December 2022; Accepted: 08 March 2023;
Published: 28 March 2023.
Edited by:
Mehanathan Muthamilarasan, University of Hyderabad, IndiaReviewed by:
Brendan Michael O’Leary, Agriculture and Agri-Food Canada (AAFC), CanadaCopyright © 2023 Sanyal, Kumar, Pattanayak, Kar and Bishi. This is an open-access article distributed under the terms of the Creative Commons Attribution License (CC BY). The use, distribution or reproduction in other forums is permitted, provided the original author(s) and the copyright owner(s) are credited and that the original publication in this journal is cited, in accordance with accepted academic practice. No use, distribution or reproduction is permitted which does not comply with these terms.
*Correspondence: Sujit K. Bishi, c3VqaXRiaXNoaUBnbWFpbC5jb20=
Disclaimer: All claims expressed in this article are solely those of the authors and do not necessarily represent those of their affiliated organizations, or those of the publisher, the editors and the reviewers. Any product that may be evaluated in this article or claim that may be made by its manufacturer is not guaranteed or endorsed by the publisher.
Research integrity at Frontiers
Learn more about the work of our research integrity team to safeguard the quality of each article we publish.