- 1Indian Council of Agricultural Research (ICAR) -National Rice Research Institute, Odisha, India
- 2Odisha University of Agriculture and Technology, Odisha, India
- 3Cornell University, Ithaca, NY, United States
- 4Norwegian Institute of Bioeconomy Research, Oslo, Norway
Introduction: Conventional rice production techniques are less economical and more vulnerable to sustainable utilization of farm resources as well as significantly contributed GHGs to atmosphere.
Methods: In order to assess the best rice production system for coastal areas, six rice production techniques were evaluated, including SRI-AWD (system of rice intensification with alternate wetting and drying (AWD)), DSR-CF (direct seeded rice with continuous flooding (CF)), DSR-AWD (direct seeded rice with AWD), TPR-CF (transplanted rice with CF), TPR-AWD (transplanted rice with AWD), and FPR-CF (farmer practice with CF). The performance of these technologies was assessed using indicators such as rice productivity, energy balance, GWP (global warming potential), soil health indicators, and profitability. Finally, using these indicators, a climate smartness index (CSI) was calculated.
Results and discussion: Rice grown with SRI-AWD method had 54.8 % higher CSI over FPR-CF, and also give 24.5 to 28.3% higher CSI for DSR and TPR as well. There evaluations based on the climate smartness index can provide cleaner and more sustainable rice production and can be used as guiding principle for policy makers.
1 Introduction
Sustainable rice cultivation in Asia is important for global food security, as rice fulfills the calorie requirement of more than 50% of the world population. As per the future projection, the rice production should be increased by 30% to meet out the future food demand (Pathak et al., 2020). Currently, rice is grown on 43.79 million hectares (Mha) of cultivated land in India, with a production of 116.4 million tons (Mt) (GOI, 2020). Farmers often wet till their paddies to transplant young seedlings of rice. Tillage at inappropriate moisture destructs the soil structure and declines the soil health over the long run to support a healthy crop (Altieri, 2018). In addition, rice is blamed for its high-water requirement and greenhouse gas emission due to its cultivation in completely saturated and flooded condition. The water footprint of rice production in India is 2,020 M3 a year compared with 970 M3 a year in China and a global average of 1,325 M3 a year (Bouraima et al., 2015). Rice requires 800–5,000 L of water with an average of 2,500 L/kg of grain yield (Bouman and Tuong, 2000; Najmuddin et al., 2018), and the water productivity of rice ranges between 0.20 and 1.20 kg m−3, which is one of the least among the cereal crops with equal caloric requirements (Najmuddin et al., 2018; Kumar et al., 2019). Globally, rice fields account approximately 30% and 11% of agricultural emissions of CH4 and N2O, respectively (Hussain et al., 2015). In India, rice fields emit 4.09 ± 1.19 Tg CH4 year−1 of methane, which is approximately 5% of the total methane emission from agriculture (Gupta et al., 2021). Thus, in the era of climate change and energy crisis, focusing and popularizing the sustainable crop production practices are the needs of the hour. The crop production should be associated with minimum use of water, energy, fertilizer, and other farm inputs to reduce the environmental foot print associated with rice production
In past decades, alternative rice establishment techniques have been advocated for sustainable intensification of rice production systems (Jat et al., 2019), namely, system of rice intensification (SRI), direct seeded rice (DSR), line transplanting, alternate wetting and drying (AWD), etc. These techniques are popularly called as resource conservation techniques (RCTs), as they improve the use efficiency of applied resources. SRI has been adopted by more than 10 million farmers in 70 or more countries (Thakur et al., 2016). Rice grown under SRI methods has merit of improving resource use efficiency and crop productivity while mitigating greenhouse gas (GHG) emissions (Gathorne-Hardy, 2013). Adaptation of SRI methods reduced 28%–30% global warming potential (GWP) and 36% water use over the farmer practice (Jain et al., 2014). DSR rice production is another popular crop establishment technique with coverage of 29 Mha in Asia (Pathak et al., 2011). The main advantage of DSR rice production techniques is 10%–30% saving of irrigation water (Kumar et al., 2022; Rana et al., 2022), reduction in GWP by 16%–33% (Pathak and Aggarwal, 2012; Chakraborty et al., 2017; Susilawati et al., 2019), and reduction in the production cost by 6%–32% (Kumar and Ladha, 2011). The alternate wet and dry method of irrigation is usually followed along with SRI and DSR establishment techniques (Rao et al., 2017). The mechanized line transplanting of the seedlings is often advised for better stand establishment as compared to random transplanting in the farmer’s field. The benefits of mechanized transplanting are higher yield and nutrient and water use efficiency.
Although there are many studies evaluating the benefits of RCTs for crop productivity and profitability, studies evaluating multi-criteria assessment of different RCTs for sustainability indicators are very few (Kakraliya et al., 2018). Again, most of the studies have been conducted on the research stations, which does not account for the socioeconomic conditions of farmers. The on-farm assessment of crop production techniques for multiple indicators of rice production is more important (Nayak et al., 2022). Nowadays, along with productivity and profitability, the environmental footprints are more focused due to the environmental pollution/footprints associated with crop production (Sapkota et al., 2019). Common rice production/crop establishment techniques are evaluated for GHG emission, energy, fertilizer, and water use, and soil health (Parihar et al., 2019). Thus, in order to select a cleaner, efficient, and profitable rice production practices, which are climate resilient, a comprehensive approach is required. For this, the development of a common index combining the effect of multiple indicators can be more appropriate. Although many researchers have proposed the use of numerical index for evaluating climate smart agriculture practices (Arenas-Calle et al. (2019); Arenas-Calle et al. (2021)); Challinor et al., 2022), those were less comprehensive (not based on three principles of climate smart agriculture). There is no single available study assessing the performance of rice production techniques against crop and water productivity, soil health, nutrient use efficiency, energy productivity, GHG emission, and profitability, in totality based on three principles of climate smart agriculture (production, adaptation, and mitigation). In this connection, there lies an excellent opportunity to develop a unique index to compare multiple criteria at one go while screening the most efficient rice production techniques. Therefore, a 2-year experiment was conducted with the following objectives: i) to measure or compute multiple indicators of sustainability for diversified rice establishment techniques in the eastern coastal rice production system of India, ii) to identify the important soil properties affecting productivity and greenhouse gas emission from the soil, and iii) to develop an index to represent multiple indicators of sustainability (climate smartness index) to screen for a cleaner, profitable, and resource-efficient rice establishment technique. We hypothesized that the SRI techniques of rice production are more sustainable, with minimum resource use and ecological impact.
2 Materials and methods
2.1 Experimental site characteristics
A field experiment was conducted in a farmer’s field in Tangi-Choudwar block of Cuttack districts of Odisha state, India during the winter season of 2020 and 2021. This area is located at mid-central table land zone (20°66′ N latitudes and 85° 95 E′ longitudes) of Odisha and receives a mean annual rainfall of 1,400 mm. Soil type was sandy clay loam (Bouyoucos, 1962) with 1.44 Mg m−3 bulk density, 0.29 dS m−1 electrical conductivity (EC), 5.7–5.9 pH (using 1:2.5, soil:water suspension) (Jackson, 1973), 5.8 g kg−1 organic C (Walkley and Black, 1934), 176.5 kg ha−1 available N (Subbiah and Asija, 1956), 15.8 kg ha−1 available P (Olsen, 1954), and 87.8 kg ha−1 NH4OAc-K (Jackson, 1973) content, respectively.
2.2 Details of the treatment and crop management
The experiment was carried out in a randomized completely block design (RCBD) replicated four times. The rice cultivar Pooja of 130–135days duration with 5.5–6 t ha−1 of yield potential was cultivated during both years. Five climate-resilient rice production techniques along with farmer regular practice as control were evaluated. The treatment detail of each rice production technique has been described in Table 1. The treatments included I) system of rice intensification with alternate wetting and drying (SRI-AWD), II) direct seeded rice with continuous flooding (DSR-CF), III) direct seeded rice with alternate wetting and drying (DSR-AWD), IV) transplanted rice with continuous flooding (TPR-CF), V) transplanted rice with alternate wetting and drying (TPR-AWD), and VI) farmer’s practice (FPR-CF). The land was prepared once with mold board plow up to 20 cm, followed by harrowing and leveling. Each plots (100 m2) were isolated by bunds (30×50 cm2 with polythene sheets up to a depth of 45 cm to prevent interplot seepage. The treatments details related to seed rate, method of transplanting, seedling age, irrigation water management, and amount of fertilizer applied were presented in Table 1. Urea, di-ammonium phosphate (DAP) and muriate of potash (MOP) was applied to supply the NPK to the rice. Half dose of N and full dose of P and K were applied as basal, and the remaining half-dose of N was applied at maximum tillering stage and panicle initiation stage in two equal splits. In both DSR and transplanted condition after 1 week of showing/transplanting, 5 cm irrigation was applied when soil moisture tension dropped to −20 kPa in alternate wetting and drying (AWD) treatments throughout the rice growing season, excluding the flowering period. In conventional flooding (CF) conditions, irrigation was regularly applied to maintain a flooding condition from the day of transplanting to physiological maturity. The grain and biological yield were recorded after harvesting at harvest maturity of the crops. The grain yield was recorded at 14% moisture content. The harvest index (HI) was calculated as:
2.3 Irrigation water productivity and nitrogen partial factor productivity
The amount of applied irrigation water was measured by measuring the discharge of Parshall flume installed at the field multiplied by the water application duration (Parshall, 1928). Furthermore, the irrigation water productivity was measured across the treatments using Equation 2.
Nitrogen partial factor productivity (NPFP) was calculated by using Equation 3 as described in Dobermann (2007).
2.4 Energy budgeting
The consumption of input energy from the use of all the farm input and agricultural activities and energy output from rice grain, and straw yield were included for energy budgeting (Scholz, 1997; Sørensen et al., 2014). At first, the inventory of input use in all the agricultural operations, such as tillage, sowing, weeding, fertilizer, and agrochemical applications, harvesting, and threshing, was built. Furthermore, the amount of fertilizers, seeds, agrochemicals, human labor, diesel, and irrigation water were multiplied with their respective energy conversion coefficients (Table 2) for the calculation of input energy for different treatments. To determine the system’s energy efficiency, the following parameters were taken and calculated (Chaudhary et al., 2017) using the following equation:
Net energy (NE) = Output energy − input energy
2.5 Soil sampling and processing for determination of soil properties
For the laboratory analysis of soil properties, four replicated composite soil samples were collected randomly from each treatment using an augur (depth of 0–15 cm) at panicle initiation (PI) stage and after harvesting of rice, using the quartering method. For enzymatic assays, one portion of fresh soil samples was stored in the refrigerator at 4°C. The remaining portion was air dried, ground, and passed through a 2-mm sieve to prepare a final processed soil sample for analysis. Plant residues, root debris, and gravels were thoroughly removed before processing the soil sample.
2.6 Soil chemical and biological soil parameters
Soil chemical properties like soil pH and Eh (mV) were monitored at 3–7-day intervals throughout the seasons using pH and ORP (Star A3210 series) portable meter with a platinum-calomel electrode submerged in the reduced zone. For fractional analysis of labile carbon pool, air dried, ground, and passed through a 2-mm sieve, soil samples were taken. For the extraction of readily mineralizable carbon (RMC), 0.5 M K2SO4 (Inubushi et al., 1991) was used followed by wet digestion with dichromate (Vance et al., 1987). Chloroform fumigation extraction method described by Witt et al. (2000) was used for the analysis of microbial biomass carbon (MBC). For the analysis of other three fractions of carbon, viz., permanganate oxidizable carbon (POXC), water soluble carbohydrate carbon (WSC), and soil oxidizable organic carbon, the methods described by Blair et al. (1995); Haynes and Swift (1990), and Walkley and Black (1934), respectively, were followed. The analysis of microbial population and enzymatic activities was done with field moist soil sample. Before analysis, moisture content of soil was determined on oven-dry equivalent weight basis. The soil dehydrogenase activity was determined colorimetrically using the triphenyl tetrazolium chloride (TTC) reduction technique (Casida et al., 1964). The determination of soil urease activity was done colorimetrically with diacetyl monoxim assay method described by Tabatabai (1972). On the other hand, β-glucosidase enzyme activity was determined with the help of the following method described by Dick (1994) in which colorimetric estimation of p-nitrophenol was done, which was produced by incubating soil at 37°C for 1 h with p-nitrophenol-d-glucopyranoside, modified universal buffer pH 6.0 and toluene followed by extraction with calcium chloride and tris (hydroxymethyl) amino methane. The total microbial count was estimated using serial dilution plate count method (Sikora et al., 1983), bacterial colony counts by Waksman (1927), fungal colony count by Ryckeboer et al. (2003), and Actinomycetes colony count by Williams and Davies (1965), and expressed as colony forming units (CFU) g−1 dry soil. Finally, soil health index was calculated taking labile carbon pool, microbial population, and soil enzyme activity.
2.8 Greenhouse gas flux measurement
Throughout the growth season, the flux of methane (CH4), carbon dioxide (CO2), and nitrous oxide (N2O) from each treatment was measured using a manual closed chamber technique. Aluminum base plate (53 cm × 37 cm × 10 cm length × width × height) with 2-cm-wide channel on the rim were inserted into the soil covering four rice plants. During the time of gas sampling, a manual closed chamber (53 cm × 37 cm × 71 cm length × width × height) was placed on the channel of the base, and channel was filled with water in order to keep the base and chamber air tight. Gas samples were collected shortly after showing/transplanting and at 3–7-day intervals during the crop growing season (depending on phenological phases of the crop) (Mohanty et al., 2018). CH4 (ppm), CO2 (ppm), and N2O (ppb) concentrations in the gas samples were analyzed using a gas chromatograph (M/s Thermo Scientific) using the equation as follows:
XCH4 = the difference in CH4 concentrations (ppm) at 0 min and at 30 min.
XN2O = the difference in N2O concentrations (ppb) at 0 and 30 min.
EBVSTP = effective chamber volume at standard temperature and pressure (liter).
T = time interval (minutes) between the initial (0 min) and final (30 min) sample after chamber implantation.
A = base plate area (m2).
Fluxes of CH4, N2O, and CO2 were computed for days without a sample event using sequential linear interpolation of the sample days’ mean emissions (Kumar et al., 2016). The season’s cumulative CH4, N2O, and CO2 emissions (kg ha−1) were calculated by adding daily fluxes in sampling and non-sampling days.
2.9 Global warming potential and greenhouse gas intensity
The global warming potential (GWP), greenhouse gas intensity (GHGI), and carbon equivalent emission (CEE) of each rice production techniques were calculated as per IPCC (2007) and protocols proposed in Bhatia et al. (2005).
where GWP is global warming potential in kg CO2 eq. ha−1, and RY is rice grain yield in kg.
2.10 Estimation of profitability of different rice establishment techniques
The economics of rice cultivation in all the rice production techniques was estimated using the following equation (Gulati et al., 2022).
where Qi is the quantity of agricultural input used, Pi is the market price of agricultural input, OCi is the agricultural operational cost, YR is the yield of rice grain and straw yield (Mg ha-1), and Pr is the minimum support price (MSP) (CACP, 2021) for rice grain and market price for straw. After calculating the cost of cultivation, gross return, and net profit, these figures are converted from Indian rupees to US dollars by dividing by 73.5.
2.11 Drivers of yield and greenhouse gas emissions
A forward stepwise regression was used to establish a relationship between crop yield and greenhouse gas emissions with different soil properties and to screen the important soil properties governing the yield and GHG emission variability. For this, at first, two intercept models were fitted, i.e., rice yield ~ intercept and GHG emission ~ intercept. Furthermore, a full model was developed, i.e., yield and emission dependent on all the soil properties. Finally, the step function of R was used to create a stepwise model in R to screen the important variables.
2.12 Estimation of climate smartness index
A modified climate smartness index (CSI) for different rice production techniques was calculated based on the procedure proposed in Arenas-Calle et al. (2019). For the calculation of CSI, a set of indicators were selected to represent different indicators of sustainability (productivity, Irrigation water productivity, energy productivity, nitrogen partial factor productivity (PFPN), global warming potential, and economy). The indicators were normalized using the minimum (min) and maximum (max) normalization (N) approach (OECD, 2008; Mazziotta and Pareto, 2013). This normalization rescaled the CSI indicator values from 0 to 1. Furthermore, either the “more is better” or “less is better” technique was used depending on whether the indicator has a beneficial or cost criteria, to ease the comprehension of each indicator’s contribution in the CSI (Pollesch and Dale, 2016). For this type of normalization, min and max thresholds of productivity, nitrogen partial factor productivity (PFPN), water productivity (WP), energy productivity (EP), global warming potential (GWP), and benefit–cost ratio (B:C ratio) data for rice production system were used. The maximum and minimum threshold values of different rice production techniques were derived from different published articles of the same location. Finally, the normalized indicators were weighted. In this regard, three approaches were used for weighting these indicators: (1) expert opinion (Brooks et al., 2005); (2) by giving equally weight to each indicator (Gan et al., 2017); and (3) Statistical approach by principal components analysis (PCA) (Jiang et al., 2020). Here, we used PCA to assign significant weight to each CSA indicators so that different indicators affect CSI unequally. The following equations were used for normalization and weightage computation.
more is better
less is better
where CSI= climate smartness index, W= weightage of each indicator, ev = Eigen vector from PCA analysis, N= normalized value, P=productivity (min= 1.97 t ha−1, max= 6.55 t ha−1), WP (min= 0.1 kg grain m−3, max = 70.1 kg kg−1), (PFPN (min = 25 kg kg−1, max 70.1 kg kg−1) EP (min = 0.05 max= 0.3), GWP (min = 1,970 kg CO2-eq/ha, max= 5,109 kg CO2-eq/ha), and B:C ratio (min=1.15, max = 2.2) are the minimum and maximum value these indicators collected from systematic review.
2.12 Statistical analysis
The data for different indicators were analyzed using the SPSS version 21.0 software. The data for various indicators were analyzed using the one-way analysis of variance (ANOVA). If the ANOVA was found significant at the 0.05 level of probability, Duncan’s multiple range test (DMRT) was performed to differentiate the effect of treatment means (Gomez and Gomez, 1984).
3 Result
3.1 Crop yield and water and nitrogen use efficiency
The rice production techniques had significant effect on rice grain yield, biomass yield, and harvest index (Table 3). Rice grown under SRI-AWD method produced 5.04%–39.4% and 4.73%–40.1% higher grain yield than other production techniques in the winter season of 2020 and 2021, respectively. The DSR-based AWD and CF rice production technique had 15.6% and 22.5% higher grain yield than farmer practices (FPR-CF); however, the rice yield under AWD and CF under DSR was similar. In addition, there was no significant difference observed in yield of DSR-CF and TPR-AWD during both the season. TPR methods had 21.9% higher yield in AWD and 33.3% in CF compared to farmer practices (Table 3). The straw yield was largest in the SRI-AWD treatment followed by TPR-CF> DSR-CF>TPR-AWD>DSR-AWD > FPR-CF, while the harvest index was highest in the SRI-AWD and lowest in FPR-CF among different rice production techniques (Table 3).
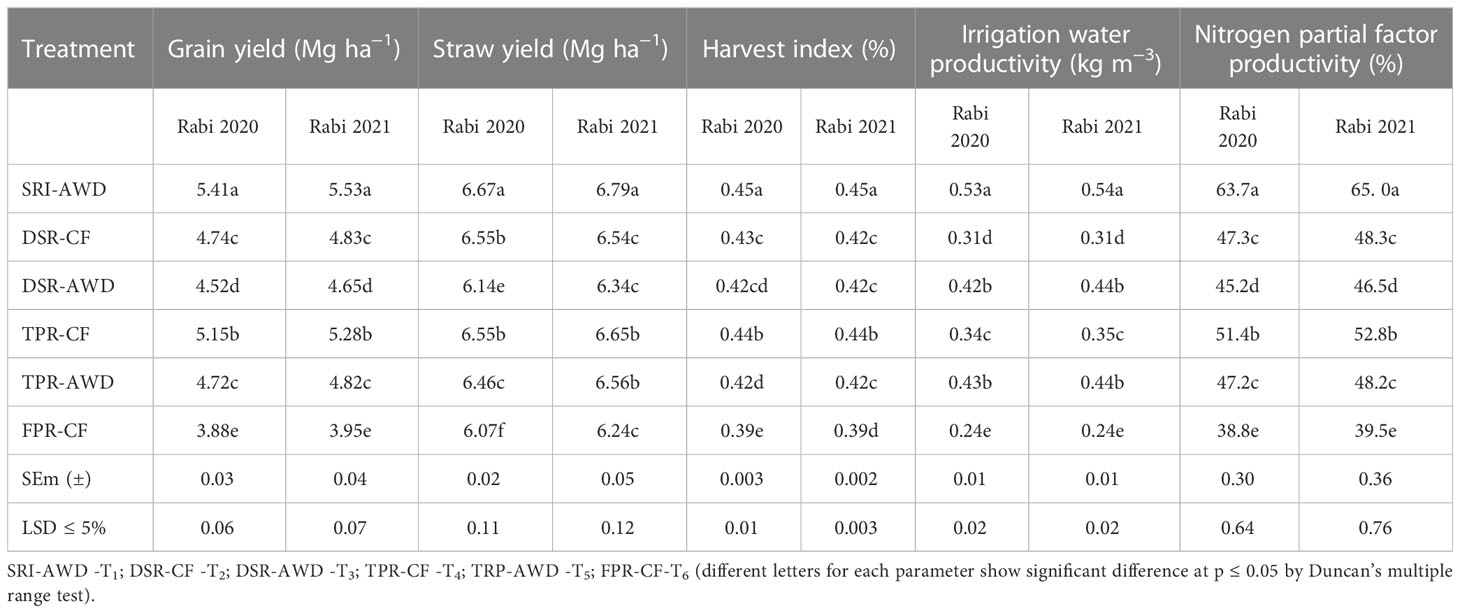
Table 3 Yield, irrigation water productivity, and nitrogen partial factor productivity of rice crop as influenced by different rice production techniques.
Total water use was higher in conventional method of rice cultivation (FPR-CF), followed by TPR-CF, DSR-CF, TPR-AWD, SRI-AWD, and DSR-AWD, respectively (Table 3). The water saving was largest under SRI-AWD (37.5%), followed by DSR-AWD (34.7%) and TPR-AWD (33.5%) as compared to FPR-CF. Significantly largest grain yield per unit quantity of water used (water productivity) was found under SRI-AWD (0.53, 0.54 kg m−3) and least in FPR-CF (0.24 and 0.24 kg m−3) during both the seasons. Similarly, the water productivity for DSR-AWD was observed 0.42 and 0.44 kg m−3, whereas the water productivity under DSR-CF was 0.31 and 0.31 kg m−3 during both the crop growing season. Among all the rice production techniques, on an average, the AWD technique increased the water productivity by 78.3% compared to CF water management (Table 3). Nitrogen partial factor productivity (PFPN) was significantly varied among the rice production techniques (Table 3). It varied from 38.8 to 65.0 kg grain per kg N applied, whereas the highest was observed in SRI-AWD and least in FPR-CF. The DSR- CF had on an average 4.25% higher PFPN as compared to DSR-AWD, whereas the TPR-CF had 8.4% higher NUE as compared to TPR-AWD. The PFPNs under FPR-CF were 38.8 and 39.5 kg grain per kg N.
3.2 Energy budgeting
The input energy requirement for rice production varied under different rice production techniques (Table 4). The total energy requirement was largest in TPR-CF, which was nearly similar to the energy requirement under farmer’s practices (1.74% higher over farmer practices). The least total energy requirement was recoded in DSR-AWD, which was on an average 26.1% lesser than FPR in both seasons (Table 4). DSR production technique required 23.8% less energy as compared to conventional rice cultivation, with the majority of the energy savings obtained from diesel, machinery, and labor. The energy output was found largest in SRI-AWD, which was 19.8% more than the FPR-CF (Table 4). All the indices of energy use efficiency, i.e., EUE, net energy, energy productivity, and profitability, differed significantly among the rice production practices (Table 4). The largest net energy was observed in SRI-AWD technique, which was on an average 28% higher than FPR-CF during both the year. The largest energy use efficiency was obtained under DRS-AWD method of rice production, which was 45.7% larger than FPR-CF technique. The different energy-efficient rice production practices improved the EUE by 27.3%–45.4% and 27.3–46.1% during 2020 and 2021, respectively.
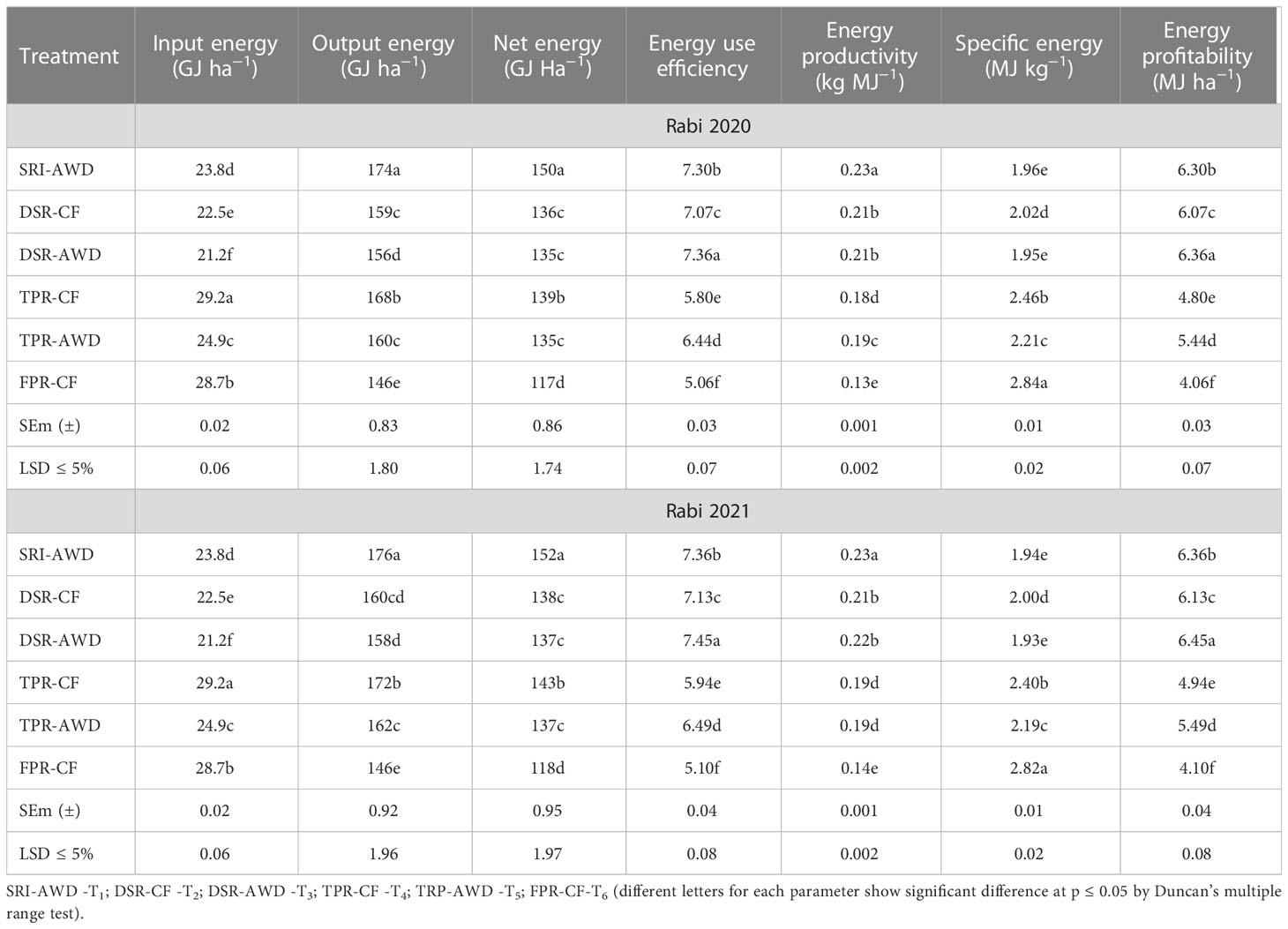
Table 4 Energy input–output relationship of rice crop as influenced by different rice production techniques.
3.3 Soil redox potential, greenhouse gas emission and global warming potential
Soil pH and Eh value during rice growing period varied in between 5.5–7.1 and 20 to −261 mV among different rice production techniques during both years. After transplanting/sowing under continues flooding conditions, soil Eh declined until panicle initiation stage, then gradually increased in all CF treatments (Figure 1). The lowest Eh value was recorded under DSR-AWD, TPR-AWD, and SRI-AWD treatment, which was significantly lower than other CF treatments. The average seasonal redox potential under AWD based techniques was 26.2% more than the CF treatments.
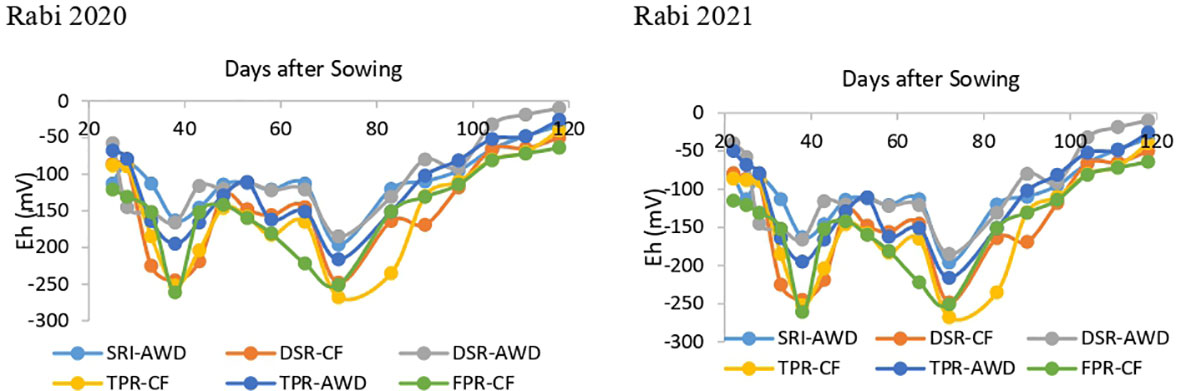
Figure 1 Effect of different rice production techniques on soil Eh (redox potential) in rice crop. SRI-AWD-T1; DSR-CF-T2; DSR-AWD-T3 TPR-CF-T4; TPR-AWD-T5; FPR-CF-T6.
Methane flux under different rice production techniques varied significantly (p< 0.05) in between 1.06–9.01 mg m−2 h−1 and 0.72–7.83 mg m−2 h−1, during the rice production season of 2020 and 2021, respectively (Figure 2). The highest methane flux (9.201 and 7.83 mg m−2 h−1) was recorded during panicle initiation stage (at 72th Julian day of 2020 and 2021) under different rice production technique during both the season (Figure 2). The cumulative CH4 emission under FPR-CF was significantly (p< 0.05) higher than that under SRI-AWD and DSR-AWD during both years (Table 5). Alike methane emission, the N2O emissions varied significantly under different rice establishment techniques. The largest N2O emission (127.0 and 147 μg m2 h−1) was observed under the TPR-AWD treatments, which was at per with DSR-AWD (126.8 and 147 μg m−2 h−1), whereas TPR-CF had the least nitrous oxide emission (126.8 and 147g m2 h−1) in both years (Figure 2). The cumulative N2O emission was significantly (p< 0.05) higher in AWD treatments (DSR-AWD, TPR-AWD, and SRI-AWD), which was 27.9% to 42.3% higher compared to CF treatments (DSR-CF, TPR-CF, and FPR-CF) during both years (Table 5). Significant effects of different rice production techniques on CO2 emission from rice field were observed (Figure 2 and Table 5). The CO2 fluxes varied from 12.3 to 115.2 mg CO2 m−2 h−1 in the rice production system during both years. Regardless of the treatments, the CO2 flux increased till flowering and decreased thereafter. The average cumulative CO2 emissions under different rice production techniques ranged between 1,146–1,647 kg ha−1 and 1,147 to 1,625 kg ha−1 in 2020 and 2021, respectively (Figure 2 and Table 5).
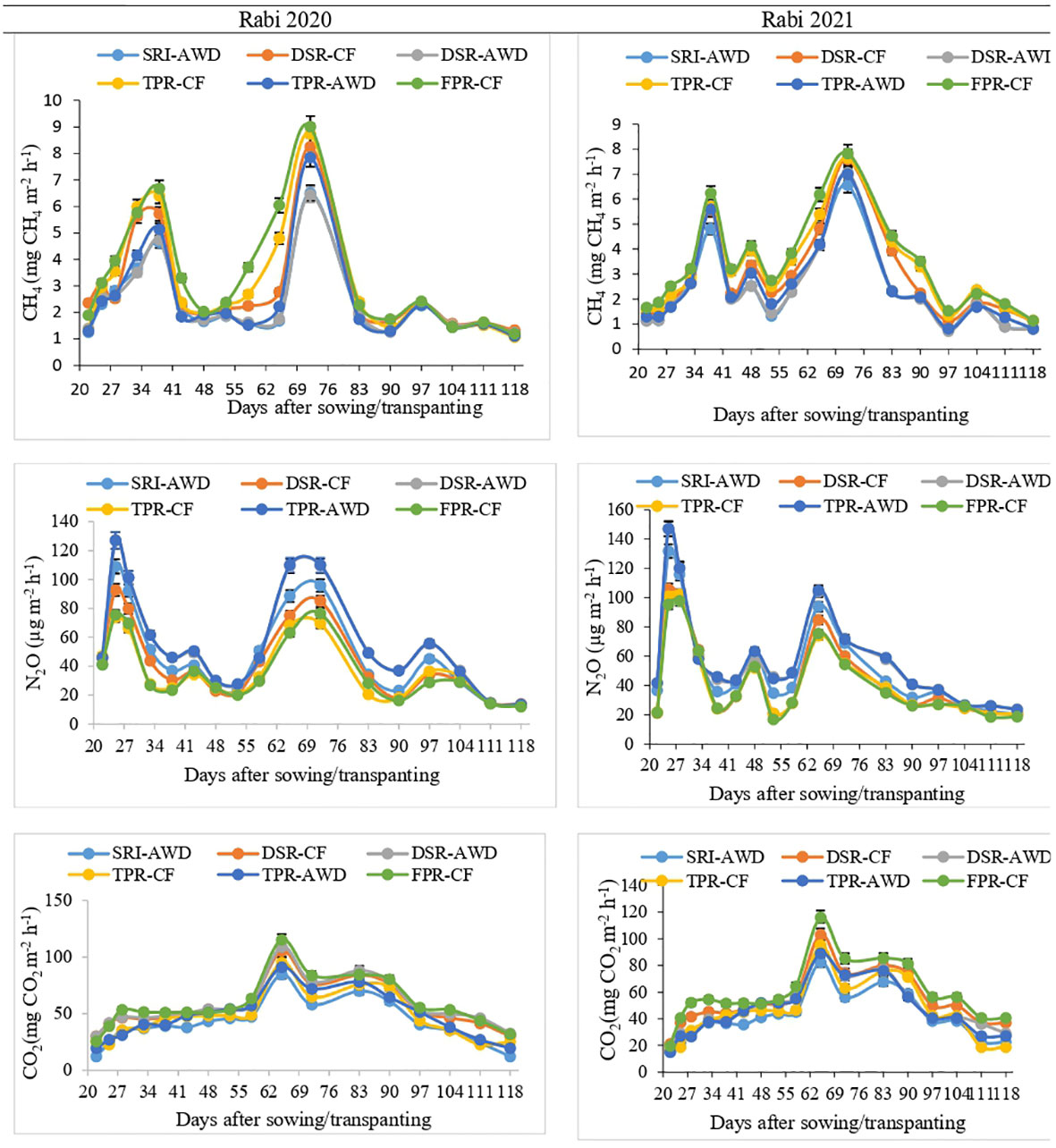
Figure 2 Methane (CH4), nitrous oxide (N2O), and carbon dioxide (CO2) flux from soils during different rice production technique of rice crop.
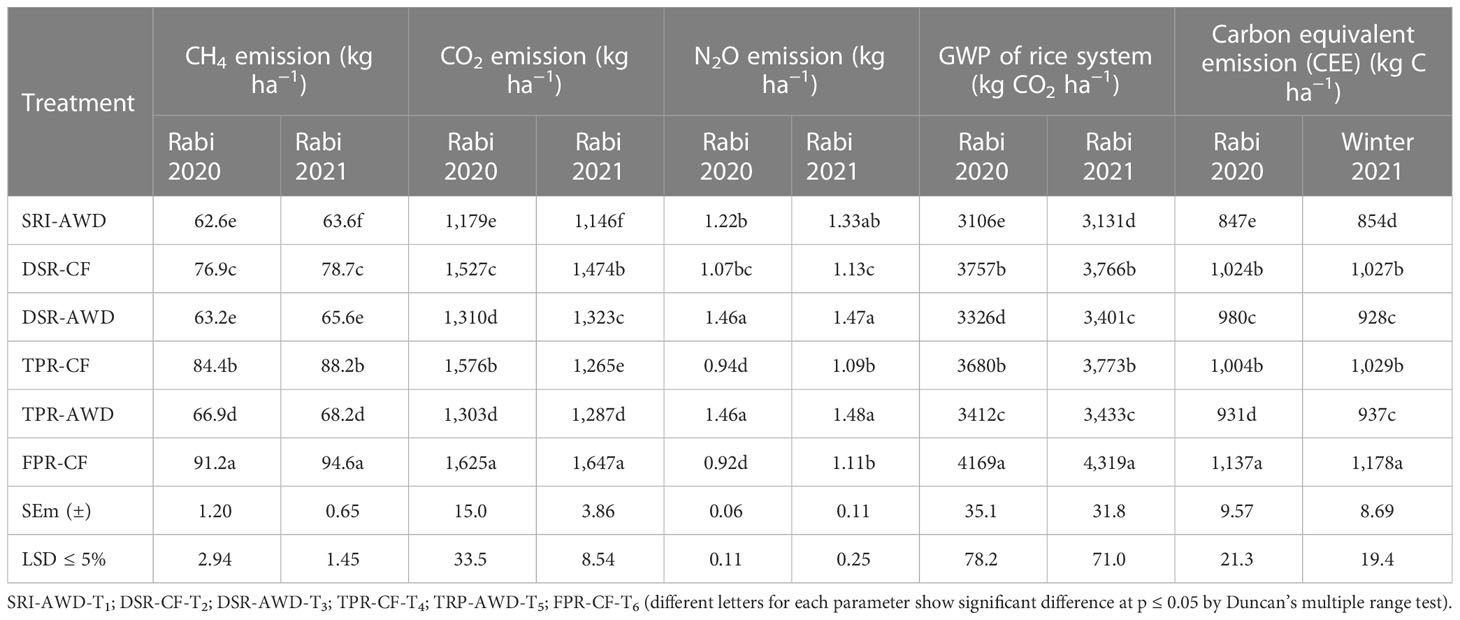
Table 5 Greenhouse gas emissions, GWP, and carbon equivalent emission in different rice production techniques.
The methane, nitrous oxide, and carbon dioxide emission were converted to GWP based on CO2 equivalent values of respective GHGs. The GWP under different rice production techniques varied significantly in both years. The largest GWP was observed in FPR-CF, which was 9.9%–25.5% and 12.8%–27.5% higher than other rice production techniques during 2020 and 2021, respectively. The AWD irrigation with SRI establishment techniques had least (3,106–3,131kg CO2 ha-1) GWP, which was 25.5% and 27.5% lesser than the GWP under FPR-CF during both seasons (Table 5). The DSR-AWD and TPR-AWD had 20.2%–21.3% and 18.2%–20.5% lesser GWP than FPR-CF during both the seasons.
3.4 Soil health indicators
The soil health indicators, i.e., the labile carbon fractions, soil enzymes, and microbial parameters at panicle initiation state of rice soil, varied significantly across different rice production techniques (Figure 3, 4 and Table 6). In addition, the rice production practices significantly affected different C-fractions (RMC, WSC, and POXC) (Figures 3, 4 and Table 6). The mean RMC, POXC, and WSC contents were significantly higher in SRI-AWD, which were 1.54, 1.58, and 2.05 times higher than the FPR-CF treatment. The RMC concentration was significantly increased in SRI-AWD practices by 156 and 163 μg kg−1 during 2020 and 2021, respectively, than FPR-CF. Soil POXC concentration was greater in DSR-AWD and TPR-AWD, as compared to FPR-CF. MBC of soil remained unaffected by different rice production techniques, and the largest MBC was observed under SRI-AWD (211–251 μg kg−1 soil), while the least MBC was observed under FPR-CF (168–198 μg kg−1 soil). The microbial populations of bacteria, fungi, actinomycetes, heterotrophs, denitrifiers, and methanogen recorded at PI stages varied significantly across different rice production techniques (Table 6). The largest microbial population was observed in the SRI-AWD method of rice cultivation, which was 20%–45% higher as compared to FPR-CF. The trend in the build-up of bacteria, fungi, and Actinomycetes populations was largest under SRI-AWD, followed by TPR-AWD, and least in FPR-CF. Similarly, the heterotrophs and methanogens population were higher in FPR-CF, followed by TPR-CF, and least in SRI-AWD, whereas the denitrifiers population was higher in DSR-AWD and TPR-AWD in both the season. The soil enzymes activities, like DHA, urease, β-glucosidase, and FDA, were significantly improved with different rice production practices (Figure 4). Among the different rice production techniques, the SRI-AWD practices had largest enzymatic activities like urease, β-glucosidase, and FDA, whereas the FPR-CF had least enzymatic activities, which were at par with DSR-CF and TPR-CF. The urease, β-glucosidase, and FDA activities increased by 19.4%, 16.7%, and 61.2%, respectively, in SRI-AWD, as compared to FPR-CF during 2021 and 16.7%, 12.1%, and 57.3% during 2020, respectively. The maximum DHA activity was found in FPR-CF (362 and 357 g kg−1) and least in TPR-AWD (296.5 and 282 g kg−1).
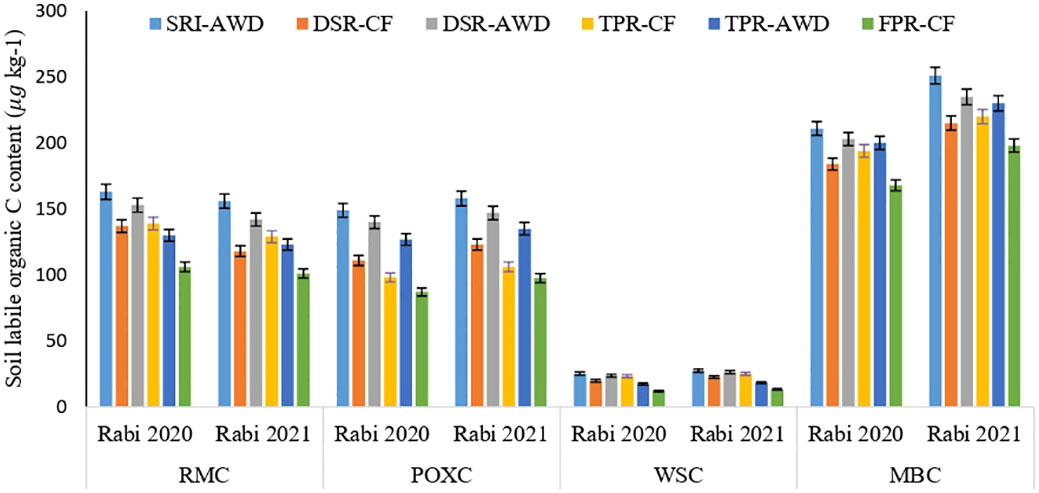
Figure 3 Soil labile C content such as readily mineralizable carbon (RMC), permanganate oxidizable carbon (POXC), water soluble carbohydrate carbon (WSC), and microbial biomass carbon (MBC) (g kg−1) in Rabi 2020 and Rabi 2021 under different rice production techniques in rice–rice cropping system.
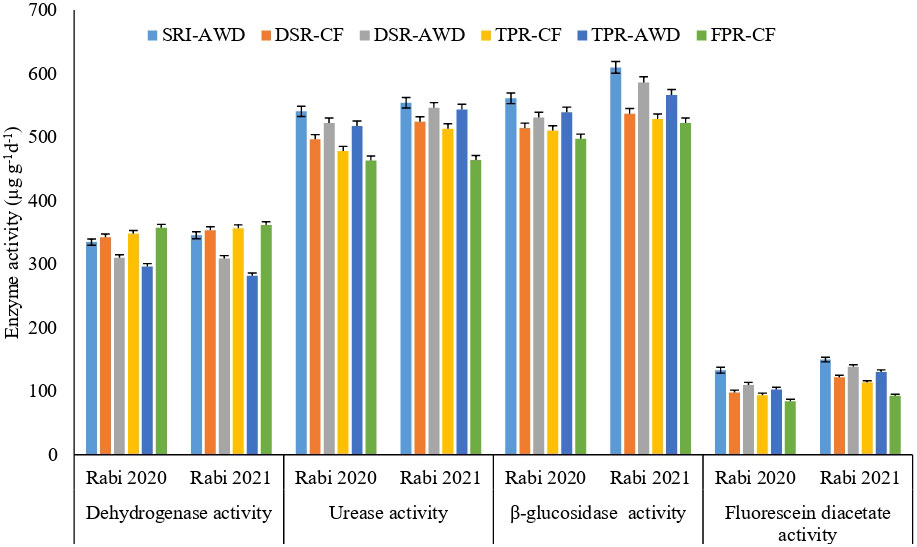
Figure 4 Soil dehydrogenase activity, urease activity, and fluorescein diacetate activity (μg TPF g−1 day−1) during Rabi 2020 and Rabi 2021 under different rice production techniques in rice crop.
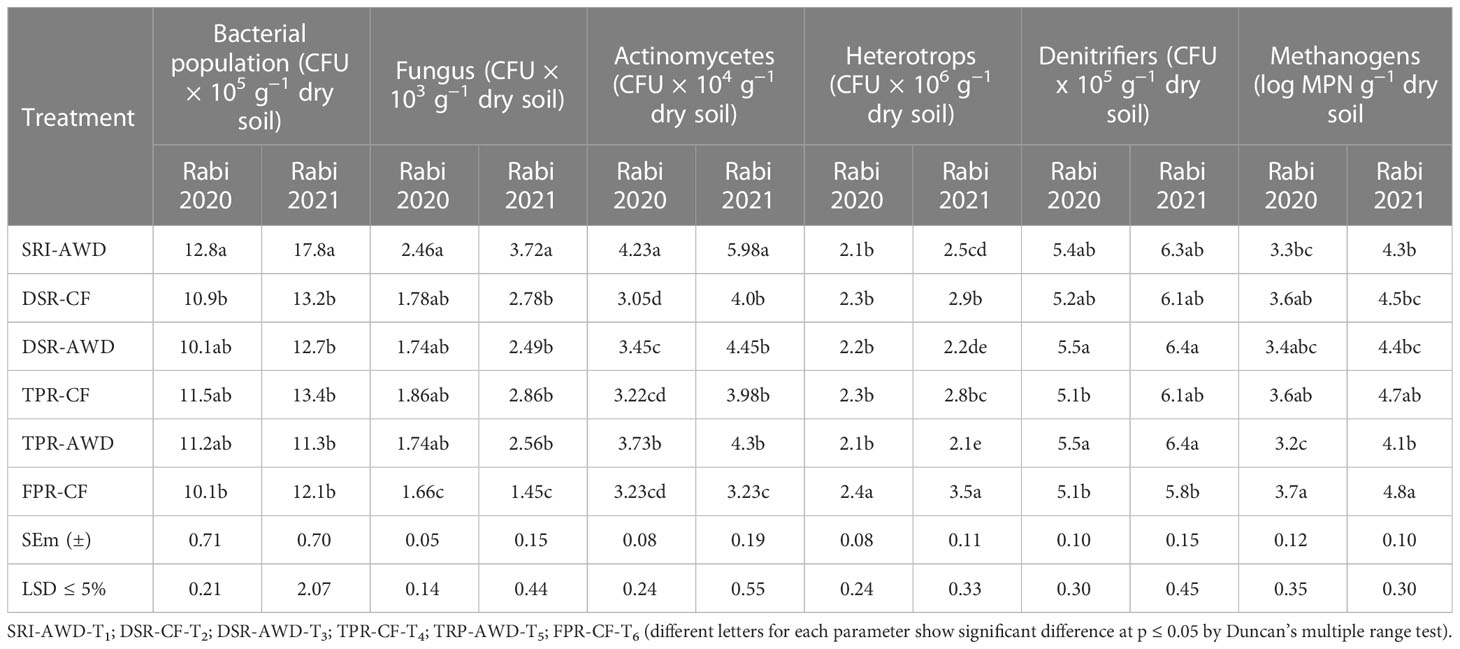
Table 6 Soil microbial population dynamics of rice crop influenced by different rice production techniques.
3.5 Drivers of crop yield and greenhouse gas emission
The stepwise regression was used to screen the important soil properties governing the yield and emission variability. WSC and bacterial population (BP) positively affected the rice yield, whereas the methanogenesis activity and POXC negatively affected the rice yield (although very small negative effect of permanganate oxidizable carbon). A unit change in WSC was associated with 0.06 t increase in rice yield, whereas the unit increase in methanogenesis was associated with 0.33 t ha decrease in rice yield when all other factors are present at their mean level (Table 7). As expected, the denitrifiers and methanogenesis population positively affected the GHG emission from the rice field, i.e., a unit increase in methanogens and denitrifiers population was associated with 208 and 189 CO2-eq increase in GWP when all other factors are present at their mean level. The bacterial population, urease activity, and readily mineralizable carbon negatively affected the GWP (Table 7).
3.6 Profitability of different rice production techniques
Production cost for various rice production techniques varied significantly across different crop production techniques (Table 7). The cost of production was largest in TPR-CF, followed by TPR-AWD, which was at par with FPR-CF and least in DSR-AWD. The cost of production under FPR-CF was 1.12%–4.62% higher than other rice production techniques, i.e., SRI AWD, DSR-AWD, and DSR-CF during both years. Gross and net return and benefit–cost ratio varied significantly across different rice production techniques (Table 7). SRI-AWD method fetched significantly largest gross and net return over other rice production techniques. The gross and net return under SRI-AWD were 34.9% and 122%, respectively, higher than FPR-CF in both years. The largest B:C ratio was observed in SRI-AWD and least in FPR-CF in both years. Among different rice production techniques, the production efficiency (PE) varied from 25.8% to 36.2% and 31.0% to 36.9% in 2020 and 2021, respectively, whereas the monetary efficiency (ME) varied from 9.65% to 15.2% and 9. 32% to 14.7%. The maximum PE was observed in SRI-AWD, whereas the least PE was observed under FPR-CF. The SRI-AWD techniques were most profitable than all other rice production practices.
3.7 Climate smartness index
The multiple indicators were evaluated using a composite index, i.e., climate smart index, to screen the production practices that are sustainable over long run. The CSI varied between −1 to +1. The composite CSI varied significantly across different rice production methods (Figure 5). The SRI-AWD had the largest CSI, i.e., 0.80 and 0.82, which indicates that CSI had higher grain yield, higher water productivity, higher energy efficiency, higher profitability, and least GHG emission, whereas the least CSI score was observed under FPR-CF, i.e., 0.53 during both years, which indicates the least yield, energy efficiency, and largest emission under FPR-CF. The CSI under CSI-AWD was 1.50 and 1.55 times greater than the farmer’s practices. The performance of the DSR-AWD and TPR-AWD was in between that of SRI-AWD and FPR-CF. The CSI score under DSR-AWD and TPR-AWD techniques of rice production was 4.6% and 1.54% higher than DSR-CF and TPR-CF, respectively.
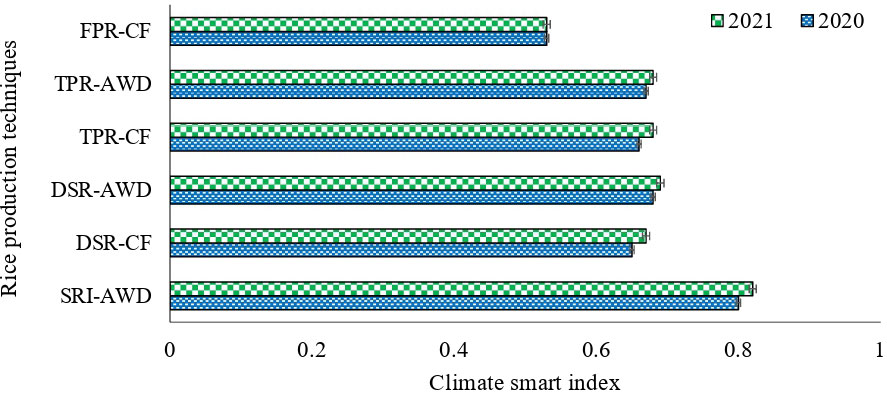
Figure 5 Climate smartness index (CSI) during both the season under different rice production techniques in rice crop.
4 Discussion
4.1 Effect of different rice production techniques on productivity, profitability, and energy use efficiency.
The congenial growth conditions prevailed because AWD under SRI method allowed the rice plant to have a greater number of phyllochrons, increased number of tillers, and highly developed roots, before the flowering phase (Deb, 2020) and produced highest grain yield over all the rice production techniques evaluated. Our findings were in accordance with the findings of Chapagain and Yamaji (2010); Mishra and Salokhe (2011), and Nazir et al. (2022). Talla and Jena (2014) and Kumar et al. (2016) have also reported approximately 7%–30% higher grain yield in SRI-AWD over the conventional method. Lal et al. (2016) reported that younger seedlings with higher tillering ability, optimum plant population, efficient nutrient, and moisture utilization enabled SRI to produce higher grain yield. Higher grain yield in DSR was due to early seeding vigor, avoidance of transplanting shock, increased panicle number, higher test weight, and reduced poor grain sterility percentage (Gangwar et al., 2008). Compared to continuous flooding, AWD considerably reduced water use while maintaining or even increasing yields (Mishra and Salokhe, 2011; Thakur et al., 2014; Malyan et al., 2016). Although the mechanisms underlying these adaptations are unknown, AWD was found to be strongly associated with root development and access to water and nutrients at a deeper level in the soil profile (Thakur et al., 2014). AWD-induced rhizosphere drying also improved plant hormone signaling and increased grain filling rate, especially in inferior spikelet (Davies et al., 2011; Zhang et al., 2012). Zhang et al. (2009) reported an increase in rice yield by 11% due to AWD compared to continuous flooding. Moreover, yield decreased in DSR, which was also reported by recent studies (Farooq et al., 2007; Farooq et al., 2009). The yield and yield attributes are often higher in TPR because of proper utilization of available resources and efficient partitioning of photosynthates in comparison to the conventional method (Kumar et al., 2016). However, yield attributes were lower in TPR compared to that in SRI and higher over DSR in sandy clay loam soil, since the older seedlings took relatively longer time to mature. In line transplanted rice, the flowering was delayed by 12–16 days compared to DSR, which might be ascribed to transplanting shock (Joshi et al., 2013). Soil texture plays an important role in water management in rice production. Similarly, rice yield was reduced under AWD management in clay texture soil compared to loam soil because of dryness in clay soil at −20kPa compared to loam (Carrijo et al., 2017). Under the conventional system, cultural practices of transplanting of older seedlings at three to four seedlings/hill, continuous flooding of the field throughout crop would have resulted in poor root growth with quicker root degeneration (Chakraborty et al., 2017). Nirmala et al. (2021) reported that conventional method of rice production yield was reduced up to 55% compared to SRI methods. Thus, adaptation of rice production technique like SRI, DSR, and TPR (mechanical) with AWD in rice ecology may enhance overall productivity along with resilience to climate change (Lal et al., 2020; Kumar et al., 2022).
SRI-AWD rice production technique required less water and have higher grain yield, whereas DSR-AWD and TPR-AWD methods of rice production required less water use but had less yield compared to SRI-AWD. This might be attributed to less irrigation water and chemical fertilizer lowering the cost of cultivation without affecting grain yield. Increased water productivity in AWD systems could be attributed to reduced seepage and percolation loss compared to flood irrigation. However, such losses are highly reliant on a soil’s hydrological characteristics (Sharma et al., 2002; Pérez-Sánchez et al., 2012). Saving of water in AWD, SRI-AWD (20%–50%), and DSR (13%–60%) as compared to flooding was also reported by several workers (Singh et al., 2013; Dass et al., 2016; Carrijo et al., 2017; Mboyerwa et al., 2021). In SRI-AWD rice production technique, 1 kg of N fertilizer produced 65 kg of grain on average; however, when 1 kg of N fertilizer was applied to rice using the FPR-CF technique, only 38.8 kg of grain was produced. Higher N use efficiency in SRI-AWD treatment might be due to higher root growth, photosynthetic rate, and N uptake. In AWD practices, there were different losses of N, viz., leaching and denitrification by draining less water and leaving less stagnant water in the field. Contrarily, hypoxic conditions in continuous flooding with faulty N management in farmer’s practice inhibit root growth and N uptake. Thakur et al. (2013) reported the same, as SRI-AWD had higher PFPN than FPR-CF.
Energy was consumed at every stage of the rice production, from tillage operation to threshing. Total energy requirement for optimum production of a crop is the function of input used (seed rate, manure and fertilizer rate, herbicide and pesticide rate, irrigation, etc.), crop production method, duration of cultivar, and cropping system (Lal et al., 2020). The total energy requirement was highest in TPR-CF. It might be due to mechanical transplanter, which consumed higher energy than manual transplantation. However, FPR-CF had demanded more human labor, fertilizer, seed, and area to get more seedling to transplanting per unit area than other rice production techniques. Fertilizers used the majority of total input energy consumption, accounting for 40%–70% of total input energy compared to other input requirement, which was supported by recent findings (Singh et al., 2019; Lal et al., 2020). The second important factor of energy consumption was land preparation, which includes tillage operation, irrigation for seedbed preparation, and machinery. It was similar to the finding of Eskandari and Attar (2015). SRI-AWD had higher output energy, which could be attributed to higher yield than the other tested techniques (Lal et al., 2020). Highest EUE in DSR technique might be due to high resource use efficiency (radiation use efficiency, water productivity, and nutrient use efficiency) (Lal et al., 2020; Htwe et al., 2021). DSR technique needed less energy consumption than transplanting rice production technique, resulting in a greater EUE (Eskandari and Attar, 2015). Here, higher specific energy in FPR-CF technique indicated that more energy input is needed to produce one-unit rice grain. The B:C ratio of SRI-AWD was in accordance with findings of Singh et al. (2013) and Soni and Soe (2016). DSR-AWD was a cost-effective alternative to DSR-CF, TPR-CF, and TPR-AWD with a similar B:C ratio. Gautam et al. (2021) reported less gross return in DSR-AWD compared to transplanted rice. The least benefit–cost ratio for FPR-CF was due to higher seed rate, higher disease and weed infestation, lower grain yield, and knowledge gap in rice production (Devkota et al., 2022).
4.2 Effect of different rice production techniques on greenhouse gas emission and GWP
Methane gas emissions included production, oxidation, and transportation systems (Brye et al., 2016). Alternate wetting and drying (AWD) increased irrigation water efficacy in mitigating 45%–90% CH4 emissions compared to continuous flooding (Nalley et al., 2015; Xu et al., 2015; Liang et al., 2016). During dry spells in AWD, the reduction in CH4 emission is the consequence of a subtle decrease in methanogenic and methanotrophic activity along with an increased supply of oxygen (Tirol-Padre et al., 2018; Islam et al., 2020). Continuous flooding maintained anoxic soil environment (redox potential<−150 mV), which facilitated anaerobic organic matter decomposition and increased CH4 emissions (Minamikawa et al., 2006; Tirol-Padre et al., 2018; Islam et al., 2020). DSR reduced GHG emission by 82%–87% than TPR (Gupta et al., 2016; Mishra et al., 2021). DSR provided non-puddled soil condition, higher water percolation rate, and increased soil macro-porosity and soil pore continuity (Singh et al., 2020), thus improved gas diffusivity and increased CH4 oxidation. However, in TPR-CF, puddled condition restricted percolation of water and anaerobic condition was formed, which was favorable for CH4 emission (Harada et al., 2007; Kumar et al., 2019). Anoxic conditions were produced in FPR-CF, allowing methanogens to produce CH4 by anaerobic decomposition of organic materials (Bhattacharyya et al., 2016; Swain et al., 2016, Swain et al.,2018). In the AWD water regime treatment, N2O emissions were greater than continuous flooding plots, which varied from 0.88 and 1.5 kg N2O ha−1 worldwide (Romasanta et al., 2017; Islam et al., 2020). Previous studies have also reported the similar findings of higher N2O emissions in AWD (Miniotti et al., 2016; Tariq et al., 2017; Islam et al., 2020). The anaerobic conditions found in continuous flooding techniques are estimated to significantly impede NO3 − flux from nitrification while also promoting complete denitrification of any NO3 − to N2, resulting in minimal N2O emissions. Conversely, the AWD method involved a shift to more aerobic conditions and might have accelerated nitrification and NO3 − production increasing N2O production (Verhoeven et al., 2019; Islam et al., 2020). The integrative impact of soil moisture content in different rice production techniques and rice development stage could be ascribed to temporal CO2 flux fluctuation. The increased CO2 fluxes at panicle initiation stage were owing to increased carbon substrate and microbial activities (Swain et al., 2018). In comparison to continuously flooded systems, AWD technique mitigated global warming potential (GWP: CO2+CH4+N2O) by 45%–90% (Linquist et al., 2012).
4.3 Effect of different rice production techniques on soil health indicators and climate smartness index
The post-harvest soils of SRI-AWD technique had higher plant biomass that might result in secretion of root exudates and metabolites that improved water-soluble carbon (Thakur et al., 2011; Mandal et al., 2020; Wen et al., 2022). Generally, soil moisture regime of 60%–80% field capacity is best suitable for microbial activity (Linn and Doran, 1984; Zhang et al., 2020). Hence, MBC content was higher in SRI-AWD, DSR-AWD, and TPR-AWD as compared to FPR-CF. Tian et al. (2013) reported abundance of bacteria and AM fungi, and actinomycetes population decreased under continuous submerged situation. Anoxic flooding condition favored methanogenic archaea activities over alternate wetting and drying condition, whereas a reverse trend occurred in denitrifier’s activity (Lagomarsino et al., 2016). In SRI technique, organic manure was added to soil during land preparation, which could have improved microbial and enzyme activities (Lakshmi et al., 2019). The urease activity was higher under AWD condition as compared to CF, which could be referred to a decrease in oxygen diffusion rate (ODR) value (Naher et al., 2021). Similarly, β-glucosidase activities are primarily responsible for the conversion of low-molecular-weight carbon molecules to sugar in response to soil moisture regimes (Turner et al., 2002; Nayak et al., 2007; Watts et al., 2010). Continuous submerged soils had higher dehydrogenase activity than soils with alternately wet and dry. Low ODR was shown to be favorable for dehydrogenase activities in soil in previous studies (Wolińska and Bennicelli, 2010; Lakshmi et al., 2019).
The CSI presented here suggests universal improvements in the selection of climate-smart rice production techniques when compared with farmer practices. The approach to developing a CSI presented here offers a means for quantitatively measuring and comparing the combined mitigation, adaptation, and productivity properties of agricultural practices. The specific CSI presented is a suitable metric for contexts of climate-driven constraint relating to water stress like drought, changing rainfall patterns, increasing temperatures, and the socioeconomic condition of farmers. The selection of the indicators for the development of CSI was done by combining mitigation, adaptation, and productivity properties such as GHG, PFPN, IWP, EP, BC ratio, and yield of rice farming. SRI-AWD, DSR-AWD, and TPR-AWD had higher CSIs, which might be due to their higher yield potential, water productivity, nutrient use efficiency, global warming mitigation potential, and economics compared to continuous flooding rice production techniques (Yang et al., 2012; Fangueiro et al., 2017; Arenas-Calle et al., 2019). Linquist et al. (2015) and Tarlera et al. (2016) reported that in AWD trials, water savings and emissions reductions outweighed yield costs when compared with continuous flooding practices. Consequently, we cannot explain the climate smartness associated with rice establishment method, irrigation, and nutrient management without considering suitability, and the situations in which individual CSA pillars improve considerably with respect to others, or even at the expense of others, should be carefully considered, as CSA priorities may not be the same in all cases (Campbell et al., 2014; Lipper et al., 2014; Totin et al., 2018). This is important because rice is also threatened by sub-emergence, soil salinity, and high temperatures (Mohanty et al., 2013), which means that the meaning of “climate smart” may change. The CSI could offer an easy interpretation and a transparent measure of climate smartness.
5 Conclusion
The present research calculates a climate smartness index and then applies it to screening different rice production techniques based on their score. The CSI was constructed by taking relevant indicators such as grain yield, irrigation water productivity, nutrient use efficiency, energy productivity, global warming potential, and the economy, then normalizing and weighing those indicators, and finally calculating the CSI by aggregating indicator scores and weightage using additive methods. Among the climate-resilient rice methods, SRI-AWD is the best method in terms of CSI score. The approach provides a pragmatic solution to meet the several needs and advantages of adaptation progress monitoring, effectiveness, and reduced supply chain risks resulting from climate change. Even so, as this is still a preliminary research, more investigation and assessment in multiple years, multiple locations, and multiple climatic situations are needed to reduce the uncertainty in CSI assessments, particularly in terms of spatial and temporal variability in yield, GHG mitigation potential, soil health, water productivity, and profitability in large-scale agricultural systems.
Data availability statement
The original contributions presented in the study are included in the article/supplementary material. Further inquiries can be directed to the corresponding author.
Author contributions
AN laid and managed experiments. KM and CS collected data, analyzed, and wrote the first draft of manuscript. AN reviewed and edited the manuscript. The rest of the authors read the manuscripts and corrected where ever necessary. All authors have read and agreed to the published version of the manuscript.
Funding
The Norwegian Ministry of Foreign Affairs, the Norwegian Embassy in New Delhi, and the Director of the ICAR-National Rice Research Institute in Cuttack provided funding for this study.
Acknowledgments
Sincere gratitude is extended by the first author to ICAR-NRRI and Odisha University of Agriculture and Technology for their assistance in crop-related data, GHGs, and soil sampling, laboratory analysis, and other technical advice. The authors acknowledge the field staff of ICAR-NRRI, Cuttack for helping in conducting the experiments.
Conflict of interest
The authors declare that the research was conducted in the absence of any commercial or financial relationships that could be construed as a potential conflict of interest.
Publisher’s note
All claims expressed in this article are solely those of the authors and do not necessarily represent those of their affiliated organizations, or those of the publisher, the editors and the reviewers. Any product that may be evaluated in this article, or claim that may be made by its manufacturer, is not guaranteed or endorsed by the publisher.
References
Arenas-Calle, L. N., Ramirez-Villegas, J., Whitfield, S., Challinor, A. J. (2021). Design of a soil-based climate-smartness index (SCSI) using the trend and variability of yields and soil organic carbon. Agric. Syst. 190, 103086. doi: 10.1016/j.agsy.2021.103086
Arenas-Calle, L. N., Whitfield, S., Challinor, A. J. (2019). A climate smartness index (CSI) based on greenhouse gas intensity and water productivity: application to irrigated rice. Front. Sustain. Food Syst. 3. doi: 10.3389/fsufs.2019.00105
Bhatia, A., Pathak, H., Jain, N., Singh, P. K., Singh, A. K. (2005). Global warming potential of manure amended soils under rice–wheat system in the indo-gangetic plains. Atmospheric Environ. 39 (37), 6976–6984. doi: 10.1016/j.atmosenv.2005.07.052
Bhattacharyya, P., Roy, K. S., Das, M., Ray, S., Balachandar, D., Karthikeyan, S., et al. (2016). Elucidation of rice rhizosphere metagenome in relation to methane and nitrogen metabolism under elevated carbon dioxide and temperature using whole genome metagenomic approach. Sci. Total Environ. 542, 886–898. doi: 10.1016/j.scitotenv.2015.10.154
Blair, G. J., Lefroy, R. D., Lisle, L. (1995). Soil carbon fractions based on their degree of oxidation, and the development of a carbon management index for agricultural systems. Aust. J. Agric. Res. 46 (7), 1459–1466. doi: 10.1071/AR9951459
Bouman, B. A. M., Tuong, T. P. (2000). Field water management to save water and increase its productivity in irrigated lowland rice. Agric. Water Manag 1615 (1), 20.
Bouraima, A. K., Zhang, W., Wei, C. (2015). Irrigation water requirements of rice using cropwat model in northern Benin. Int. J. Agric. Biol. Eng. 8 (2), 58–64.
Bouyoucos, G. J. (1962). Hydrometer method improved for making particle size analyses of soils 1. Agron. J. 54 (5), 64–465. doi: 10.2134/agronj1962.00021962005400050028x
Brooks, N., Adger, W. N., Kelly, P. M. (2005). The determinants of vulnerability and adaptive capacity at the national level and the implications for adaptation. Glob. Environ. Change. 15 (2), 151–163. doi: 10.1016/j.gloenvcha.2004.12.006
Brye, K. R., Nalley, L. L., Tack, J. B., Dixon, B. L., Barkley, A. P., Rogers, C. W., et al. (2016). Factors affecting methane emissions from rice production in the lower Mississippi river valley, USA. Geoderma Reg. 7 (2), 223–229. doi: 10.1016/j.geodrs.2016.04.005
Campbell, B. M., Thornton, P., Zougmoré, R., Van Asten, P., Lipper, L. (2014). Sustainable intensification: What is its role in climate smart agriculture? Curr. Opin. Environ. Sustainability 8, 39–43. doi: 10.1016/j.cosust.2014.07.002
Carrijo, D. R., Lundy, M. E., Linquist, B. A. (2017). Rice yields and water use under alternate wetting and drying irrigation: A meta-analysis. Field Crops Res. 203, 173–180. doi: 10.1016/j.fcr.2016.12.002
Casida, L. E., Jr., Klein, D. A., Santoro, T. (1964). Soil dehydrogenase activity. Soil Sci. 98 (6), 371–376. doi: 10.1097/00010694-196412000-00004
Chakraborty, D., Ladha, J. K., Rana, D. S., Jat, M. L., Gathala, M. K., Yadav, S., et al. (2017). A global analysis of alternative tillage and crop establishment practices for economically and environmentally efficient rice production. Sci. Rep. 7 (1), 1–11. doi: 10.1038/s41598-017-09742-9
Challinor, A. J., Arenas-Calles, L. N., Whitfield, S. (2022). Measuring the effectiveness of climate-smart practices in the context of food systems: Progress and challenges. Front. Sustain. Food Syst. 6. doi: 10.3389/fsufs.2022.853630
Chapagain, T., Yamaji, E. (2010). The effects of irrigation method, age of seedling and spacing on crop performance, productivity and water-wise rice production in Japan. Paddy Water Environ. 8 (1), 81–90. doi: 10.1007/s10333-009-0187-5
Chaudhary, V. P., Singh, K. K., Pratibha, G., Bhattacharyya, R., Shamim, M., Srinivas, I., et al. (2017). Energy conservation and greenhouse gas mitigation under different production systems in rice cultivation. Energy 130, 307–317. doi: 10.1016/j.energy.2017.04.131
Commission for Agricultural Costs and Prices (CACP) (2021). Department of agriculture, cooperation and farmers welfare, ministry of agriculture and farmers welfare government of India.
Dass, A., Chandra, S., Choudhary, A. K., Singh, G., Sudhishri, S. (2016). Influence of field re-ponding pattern and plant spacing on rice root–shoot characteristics, yield, and water productivity of two modern cultivars under SRI management in Indian mollisols. Paddy Water Environ. 14, 45–59. doi: 10.1007/s10333-015-0477-z
Davies, W. J., Zhang, J., Yang, J., Dodd, I. C. (2011). Novel crop science to improve yield and resource use efficiency in water-limited agriculture. J. Agric. Sci. 149, 123–131. doi: 10.1017/S0021859610001115
Deb, D. (2020). Is the system of rice intensification (SRI) consonant with agroecology? Agroecology Sustain. Food Syst. 44 (10), 1338–1369. doi: 10.1080/21683565.2020.1779165
Devkota, K. P., Beebout, S. E., Bunquin, M. A. (2022). Setting sustainability targets for irrigated rice production and application of the sustainable rice platform performance indicators. Environ. Impact Assess. Rev. 92, 106697. doi: 10.1016/j.eiar.2021.106697
Dick, R. P. (1994). Soil enzyme activities as indicators of soil quality. Defining Soil Qual. Sustain. Environ. 35, 107–124. doi: 10.2136/sssaspecpub35.c7
Eskandari, H., Attar, S. (2015). Energy comparison of two rice cultivation systems. Renewable Sustain. Energy Rev. 42, 666–671. doi: 10.1016/j.rser.2014.10.050
Fangueiro, D., Becerra, D., Albarrán, Á., Peña, D., Sanchez-Llerena, J., Rato-Nunes, J. M., et al. (2017). Effect of tillage and water management on GHG emissions from Mediterranean rice growing ecosystems. Atmospheric Environ. 150, 303–312. doi: 10.1016/j.atmosenv.2016.11.020
Farooq, M. S. M. A., Basra, S. M. A., Ahmad, N. (2007). Improving the performance of transplanted rice by seed priming. Plant Growth Regul. 51, 129–137. doi: 10.1007/s10725-006-9155-x
Farooq, M., Basra, S. M., Ahmad, N., Murtaza, G. (2009). Enhancing the performance of transplanted coarse rice by seed priming. Paddy Water Environ. 7, 55–63. doi: 10.1007/s10333-008-0143-9
Gan, X., Fernandez, I. C., Guo, J., Wilson, M., Zhao, Y., Zhou, B., et al. (2017). When to use what: Methods for weighting and aggregating sustainability indicators. Ecol. Indic. 81, 491–502. doi: 10.1016/j.ecolind.2017.05.068
Gangwar, K. S., Tomar, O. K., Pandey, D. K. (2008). Productivity and economics of transplanted and direct-seeded rice (Oryza sativa)-based cropping systems in indo-gangetic plains.
Gathorne-Hardy, A. (2013). A life cycle assessment (LCA) of greenhouse gas emissions from SRI and flooded rice production in SE India. doi: 10.35648/20.500.12413/11781/ii250
Gautam, P., Lal, B., Panda, B. B., Bihari, P., Chatterjee, D., Singh, T., et al. (2021). Alteration in agronomic practices to utilize rice fallows for higher system productivity and sustainability. Field Crops Res. 260, 108005. doi: 10.1016/j.fcr.2020.108005
GOI (2020). Directorate of economics and statistics, ministry of agriculture and farmers’ welfare, government of India.
Gomez, K. A., Gomez, A. A. (1984). Statistical procedures for agricultural research (John wiley & sons).
Gulati, A., Ganguly, K., Wardhan, H. (2022). Agricultural value chains in India: Ensuring competitiveness, inclusiveness, sustainability, scalability, and improved finance Vol. 298 (Springer Nature). doi: 10.1007/978-981-33-4268-2
Gupta, D. K., Bhatia, A., Kumar, A., Das, T. K., Jain, N., Tomer, R., et al. (2016). Mitigation of greenhouse gas emission from rice–wheat system of the indo-gangetic plains: Through tillage, irrigation and fertilizer management. Agriculture Ecosyst. Environ. 230, 1–9. doi: 10.1016/j.agee.2016.05.023
Gupta, K., Kumar, R., Baruah, K. K., Hazarika, S., Karmakar, S., Bordoloi, N. (2021). Greenhouse gas emission from rice fields: a review from Indian context. Environ. Sci. pollut. Res. 28 (24), 30551–30572. doi: 10.1007/s11356-021-13935-1
Harada, H., Kobayashi, H., Shindo, H. (2007). Reduction in greenhouse gas emissions by no-tilling rice cultivation in hachirogata polder, northern Japan: Life-cycle inventory analysis. Soil Sci. Plant Nutr. 53 (5), 668–677. doi: 10.1111/j.1747-0765.2007.00174.x
Haynes, R. J., Swift, R. S. (1990). Stability of soil aggregates in relation to organic constituents and soil water content. J. Soil Sci. 41 (1), 73–83. doi: 10.1111/j.1365-2389.1990.tb00046.x
Htwe, T., Sinutok, S., Chotikarn, P., Amin, N., Akhtaruzzaman, M., Techato, K., et al. (2021). Energy use efficiency and cost-benefits analysis of rice cultivation: A study on conventional and alternative methods in Myanmar. Energy 214, 119104. doi: 10.1016/j.energy.2020.119104
Hussain, S., Peng, S., Fahad, S., Khaliq, A., Huang, J., Cui, K., et al. (2015). Rice management interventions to mitigate greenhouse gas emissions: a review. Environ. Sci. pollut. Res. 22, 3342–3360. doi: 10.1007/s11356-014-3760-4
Inubushi, K., Brookes, P. C., Jenkinson, D. S. (1991). Soil microbial biomass c, n and ninhydrin-n in aerobic and anaerobic soils measured by the fumigation-extraction method. Soil Biol. Biochem. 23 (8), 737–741. doi: 10.1016/0038-0717(91)90143-8
IPCC, C.C (2007). “The physical science basis,” in Contribution of working group I to the fourth assessment report of the intergovernmental panel on climate change, vol. 996. (NY, USA: Cambridge University Press, Cambridge, United Kingdom and New York), 113–119.
Islam, S. F. U., Sander, B. O., Quilty, J. R., De Neergaard, A., Van Groenigen, J. W., Jensen, L. S. (2020). Mitigation of greenhouse gas emissions and reduced irrigation water use in rice production through water-saving irrigation scheduling, reduced tillage and fertiliser application strategies. Sci. Total Environ. 739, 140215. doi: 10.1016/j.scitotenv.2020.140215
Jackson, M. L. (1973). Soil chemical analysis, pentice hall of India pvt. Ltd. New Delhi India 498, 151–154.
Jain, N., Dubey, R., Dubey, D. S., Singh, J., Khanna, M., Pathak, H., et al. (2014). Mitigation of greenhouse gas emission with system of rice intensification in the indo-gangetic plains. Paddy Water Environ. 12, 355–363. doi: 10.1007/s10333-013-0390-2
Jat, S. L., Parihar, C. M., Dey, A., Nayak, H. S., Ghosh, A., Parihar, N., et al. (2019). Dynamics and temperature sensitivity of soil organic carbon mineralization under medium-term conservation agriculture as affected by residue and nitrogen management options. Soil Tillage Res. 190, 175–185. doi: 10.1016/j.still.2019.02.005
Jiang, M., Xu, L., Chen, X., Zhu, H., Fan, H. (2020). Soil quality assessment based on a minimum data set: a case study of a county in the typical river delta wetlands. Sustainability 12 (21), 9033. doi: 10.3390/su12219033
Joshi, E., Kumar, D., Lal, B., Nepalia, V., Gautam, P., Vyas, A. K. (2013). Management of direct seeded rice for enhanced resource-use efficiency. Plant Knowledge J. 2 (3), 119–134.
Kakraliya, S. K., Jat, H. S., Singh, I., Sapkota, T. B., Singh, L. K., Sutaliya, J. M., et al. (2018). Performance of portfolios of climate smart agriculture practices in a rice-wheat system of western indo-gangetic plains. Agric. Water Manage. 202, 122–133. doi: 10.1016/j.agwat.2018.02.020
Kitani, O. (1999). CIGR handbook of agricultural engineering, volume V energy and biomass engineering, chapter 1 natural energy and biomass, part 1.3 biomass resources.
Kumar, V., Ladha, J. K. (2011). Direct seeding of rice: recent developments and future research needs. Adv. Agron. 111, 297–413. doi: 10.1016/B978-0-12-387689-8.00001-1
Kumar, R., Mishra, J. S., Mali, S. S., Mondal, S., Meena, R. S., Lal, R., et al. (2022). Comprehensive environmental impact assessment for designing carbon-cum-energy efficient, cleaner and eco-friendly production system for rice-fallow agro-ecosystems of south Asia. J. Cleaner Production 331, 129973. doi: 10.1016/j.jclepro.2021.129973
Kumar, A., Nayak, A. K., Das, B. S., Panigrahi, N., Dasgupta, P., Mohanty, S., et al. (2019). Effects of water deficit stress on agronomic and physiological responses of rice and greenhouse gas emission from rice soil under elevated atmospheric CO2. Sci. Total Environ. 650, 2032–2050. doi: 10.1016/j.scitotenv.2018.09.332
Kumar, A., Nayak, A. K., Mohanty, S., Das, B. S. (2016). Greenhouse gas emission from direct seeded paddy fields under different soil water potentials in Eastern India. Agriculture Ecosyst. Environ. 228, 111–123. doi: 10.1016/j.agee.2016.05.007
Kumar, S., Verma, S. K., Yadav, A., Taria, S., Alam, B., Banjara, T. R. (2022). Tillage based crop establishment methods and zinc application enhances productivity, grain quality, profitability and energetics of direct-seeded rice in potentially zinc-deficient soil in the subtropical conditions of India. Commun. Soil Sci. Plant Anal. 53 (9), 1085–1099. doi: 10.1080/00103624.2022.2043340
Lagomarsino, A., Agnelli, A. E., Linquist, B., Adviento-Borbe, M. A., Agnelli, A., Gavina, G., et al. (2016). Alternate wetting and drying of rice reduced CH4 emissions but triggered N2O peaks in a clayey soil of central Italy. Pedosphere 26 (4), 533–548. doi: 10.1016/S1002-0160(15)60063-7
Lakshmi, C. S. R., Kumari, M. B. G. S., Sreelatha, T., Sireesha, A. (2019). Influence of different rice establishment methods and nutrient levels on soil enzyme activity, nutrient status and grain yield of rice in north coastal zone of andhra pradesh. ORYZA-An Int. J. Rice 56 (4), 380–387. doi: 10.35709/ory.2019.56.4.6
Lal, B., Gautam, P., Panda, B. B., Tripathi, R., Shahid, M., Bihari, P., et al. (2020). Identification of energy and carbon efficient cropping system for ecological sustainability of rice fallow. Ecol. Indic. 115, 106431. doi: 10.1016/j.ecolind.2020.106431
Liang, K., Zhong, X., Huang, N., Lampayan, R. M., Pan, J., Tian, K., et al. (2016). Grain yield, water productivity and CH4 emission of irrigated rice in response to water management in south China. Agric. Water Manage. 163, 319–331. doi: 10.1016/j.agwat.2015.10.015
Linn, D. M., Doran, J. W. (1984). Aerobic and anaerobic microbial populations in no-till and plowed soils. Soil Sci. Soc. America J. 48 (4), 794–799. doi: 10.2136/sssaj1984.03615995004800040019x
Linquist, B., Van Groenigen, K. J., Adviento-Borbe, M. A., Pittelkow, C., Van Kessel, C. (2012). An agronomic assessment of greenhouse gas emissions from major cereal crops. Global Change Biol. 18 (1), 194–209. doi: 10.1111/j.1365-2486.2011.02502.x
Linquist, B. A., Anders, M. M., Adviento-Borbe, M. A. A., Chaney, R. L., Nalley, L. L., Da Rosa, E. F., et al. (2015). Reducing greenhouse gas emissions, water use, and grain arsenic levels in rice systems. Global Change Biol. 21 (1), 407–417. doi: 10.1111/gcb.12701
Lipper, L., Thornton, P., Campbell, B. M., Baedeker, T., Braimoh, A., Bwalya, M., et al. (2014). Climate-smart agriculture for food security. Nat. Climate Change 4 (12), 1068–1072. doi: 10.1038/nclimate2437
Malyan, S. K., Kumar, A., Kumar, J., Smita Kumar, S. (2016a). “Water management tool in rice to combat two major environmental issues: global warming and water scarcity,” in Environmental concerns of 21st century: Indian and global context (New Delhi: Book Age publication), 46–58.
Mandal, M., Kamp, P., Singh, M. (2020). Effect of long term manuring on carbon sequestration potential and dynamics of soil organic carbon labile pool under tropical rice-rice agro-ecosystem. Commun. Soil Sci. Plant Anal. 51 (4), 468–480. doi: 10.1080/00103624.2020.1718690
Mandal, S., Roy, S., Das, A., Ramkrushna, G. I., Lal, R., Verma, B. C., et al. (2015). Energy efficiency and economics of rice cultivation systems under subtropical Eastern himalaya. Energy Sustain. Dev. 28, 115–121. doi: 10.1016/j.esd.2015.08.002
Mazziotta, M., Pareto, A. (2013). Methods for constructing composite indices: One for all or all for one. Rivista Italiana di Economia Demografia e Statistica 67 (2), 67–80.
Mboyerwa, P. A., Kibret, K., Mtakwa, P. W., Aschalew, A. (2021). Evaluation of growth, yield, and water productivity of paddy rice with water-saving irrigation and optimization of nitrogen fertilization. Agronomy 11 (8), 1629. doi: 10.3390/agronomy11081629
OECD (2008). Handbook on Constructing Composite Indicators: Methodology and User’s Guide (Paris: OECD Publications).
Minamikawa, K., Sakai, N., Yagi, K. (2006). Methane emission from paddy fields and its mitigation options on a field scale. Microbes environments 21 (3), 135–147. doi: 10.1264/jsme2.21.135
Miniotti, E. F., Romani, M., Said-Pullicino, D., Facchi, A., Bertora, C., Peyron, M., et al. (2016). Agro-environmental sustainability of different water management practices in temperate rice agro-ecosystems. Agriculture Ecosyst. Environ. 222, 235–248. doi: 10.1016/j.agee.2016.02.010
Mishra, A., Salokhe, V. M. (2011). Rice root growth and physiological responses to SRI water management and implications for crop productivity. Paddy Water Environ. 9, 41–52. doi: 10.1007/s10333-010-0240-4
Mishra, J. S., Poonia, S. P., Kumar, R., Dubey, R., Kumar, V., Mondal, S., et al. (2021). An impact of agronomic practices of sustainable rice-wheat crop intensification on food security, economic adaptability, and environmental mitigation across eastern indo-gangetic plains. Field Crops Res. 267, 108164. doi: 10.1016/j.fcr.2021.108164
Mittal, J. P., Dhawan, K. C. (1988). “Research manual on energy requirements in agricultural sector, coordinating cell,” in AICRP on energy requirement in rural sector (Ludhiana, India: PAU).
Mohanty, S., Swain, C. K., Tripathi, R., Sethi, S. K., Bhattacharyya, P., Kumar, A., et al. (2018). Nitrate leaching, nitrous oxide emission and n use efficiency of aerobic rice under different n application strategy. Arch. Agron. Soil Sci. 64 (4), 465–479. doi: 10.1080/03650340.2017.1359414
Mohanty, S., Wassmann, R., Nelson, A., Moya, P., Jagadish, S. V. K. (2013). “Rice and climate change: significance for food security and vulnerability,” in IRRI discussion paper series no. 49 (Los Baños: International Rice Research Institute).
Naher, U. A., Haque, M. M., Khan, F. H., Sarkar, M. I. U., Ansari, T. H., Hossain, M. B., et al. (2021). Effect of long-term nutrient management practices on soil health and paddy yield of rice-rice-fallow cropping system in tropic humid climate. Eur. J. Soil Biol. 107, 103362. doi: 10.1016/j.ejsobi.2021.103362
Najmuddin, O., Rasul, G., Hussain, A., Molden, D., Wahid, S., Debnath, B. (2018). Low water productivity for rice in bihar, India–a critical analysis. Water 10 (8), 1082. doi: 10.3390/w10081082
Nalley, L., Linquist, B., Kovacs, K., Anders, M. (2015). The economic viability of alternative wetting and drying irrigation in Arkansas rice production. Agron. J. 107 (2), 579–587. doi: 10.2134/agronj14.0468
Nayak, D. R., Babu, Y. J., Datta, A., Adhya, T. K. (2007). Methane oxidation in an intensively cropped tropical rice field soil under long-term application of organic and mineral fertilizers. J. Environ. Qual. 36 (6), 1577–1584. doi: 10.2134/jeq2006.0501
Nayak, H. S., Silva, J. V., Parihar, C. M., Kakraliya, S. K., Krupnik, T. J., Bijarniya, D., et al. (2022). Rice yield gaps and nitrogen-use efficiency in the northwestern indo-gangetic plains of India: Evidence based insights from heterogeneous farmers’ practices. Field Crops Res. 275, 108328. doi: 10.1016/j.fcr.2021.108328
Nazir, A., Bhat, M. A., Bhat, T. A., Fayaz, S., Mir, M. S., Basu, U., et al. (2022). Comparative analysis of rice and weeds and their nutrient partitioning under various establishment methods and weed management practices in temperate environment. Agronomy 12 (4), 816. doi: 10.3390/agronomy12040816
Nirmala, B., Tuti, M. D., Mahender Kumar, R., Waris, A., Muthuraman, P., Parmar, B., et al. (2021). Integrated assessment of system of rice intensification vs. conventional method of transplanting for economic benefit, energy efficiency and lower global warming potential in India. Agroecology Sustain. Food Syst. 45 (5), 745–766. doi: 10.1080/21683565.2020.1868648
Olsen, S. R. (1954). Estimation of available phosphorus in soils by extraction with sodium bicarbonate (No. 939) (US Department of Agriculture).
Parihar, C. M., Nayak, H. S., Rai, V. K., Jat, S. L., Parihar, N., Aggarwal, P., et al. (2019). Soil water dynamics, water productivity and radiation use efficiency of maize under multi-year conservation agriculture during contrasting rainfall events. Field Crops Res. 241, 107570. doi: 10.1016/j.fcr.2019.107570
Pathak, H., Aggarwal, P. K. (2012). Low carbon technologies for agriculture: a study on rice and wheat systems in the indo-gangetic plains. Indian Agric. Res. Institute 78, xvii.
Pathak, H., Jain, N., Bhatia, A. (2015). Enhancing resilience of Indian agriculture to climate change. Indian J. Fertilisers 11 (4), 102–115.
Pathak, H., Tewari, A. N., Sankhyan, S., Dubey, D. S., Mina, U., Singh, V. K., et al. (2011). Direct-seeded rice: potential, performance and problems-areview. Curr. Adv. Agric. Sci. (An Int. Journal) 3 (2), 77–88.
Pathak, H., Tripathi, R., Jambhulkar, N. N., Bisen, J. P., Panda, B. B. (2020). Eco-regional-based rice farming for enhancing productivity, profitability and sustainability.
Pérez-Sánchez, D., Thorne, M. C., Limer, L. M. C. (2012). A mathematical model for the behaviour of Se-79 in soils and plants that takes account of seasonal variations in soil hydrology. J. Radiological Prot. 32 (1), 11. doi: 10.1088/0952-4746/32/1/11
Pollesch, N. L., Dale, V. H. (2016). Normalization in sustainability assessment: Methods and implications. Ecol. Economics 130, 195–208. doi: 10.1016/j.ecolecon.2016.06.018
Rana, B., Parihar, C. M., Nayak, H. S., Patra, K., Singh, V. K., Singh, D. K., et al. (2022). Water budgeting in conservation agriculture-based sub-surface drip irrigation using HYDRUS-2D in rice under annual rotation with wheat in Western indo-gangetic plains. Field Crops Res. 282, 108519. doi: 10.1016/j.fcr.2022.108519
Rao, A. N., Wani, S. P., Ramesha, M. S., Ladha, J. K. (2017). “Rice production systems,” in Rice production worldwide, 185–205. doi: 10.1007/978-3-319-47516-5_8
Romasanta, R. R., Sander, B. O., Gaihre, Y. K., Alberto, M. C., Gummert, M., Quilty, J., et al. (2017). How does burning of rice straw affect CH4 and N2O emissions? a comparative experiment of different on-field straw management practices. Agriculture Ecosyst. Environ. 239, 143–153. doi: 10.1016/j.agee.2016.12.042
Ryckeboer, J., Mergaert, J., Coosemans, J., Deprins, K., Swings, J. (2003). Microbiological aspects of biowaste during composting in a monitored compost bin. J. Appl. Microbiol. 94 (1), 127–137. doi: 10.1046/j.1365-2672.2003.01800.x
Sørensen, C. G., Halberg, N., Oudshoorn, F. W., Petersen, B. M., Dalgaard, R. (2014). Energy inputs and GHG emissions of tillage systems. Biosyst. Eng. 120, 2–14. doi: 10.1016/j.biosystemseng.2014.01.004
Sapkota, T. B., Vetter, S. H., Jat, M. L., Sirohi, S., Shirsath, P. B., Singh, R., et al. (2019). Cost-effective opportunities for climate change mitigation in Indian agriculture. Sci. Total Environ. 655, 1342–1354. doi: 10.1016/j.scitotenv.2018.11.225
Scholz, V. (1997). Methodik zur ermittlung des energieaufwandes pflanzenbaulicher produkte am beispiel von biofestbrennstoffen. Agrartechnische Forschung 3 (1), 11–18.
Sharma, P. K., Bhushan, L., Ladha, J. K., Naresh, R. K., Gupta, R. K., Balasubramanian, B. V., et al. (2002). “Crop-water relations in rice-wheat cropping under different tillage systems and water-management practices in a marginally sodic, medium-textured soil,” in Water-wise rice production, vol. 8. (Los Baños, Philippines: International Rice Research Institute), 223–235.
Sikora, L. J., Ramirez, M. A., Troeschel, T. A. (1983). Laboratory composter for simulation studies (Vol. 12, no. 2, pp. 219-224). Am. Soc. Agronomy Crop Sci. Soc. America Soil Sci. Soc. America. doi: 10.2134/jeq1983.00472425001200020015x
Singh, M., Kumar, P., Kumar, V., Solanki, I. S., McDonald, A. J., Kumar, A., et al. (2020). Intercomparison of crop establishment methods for improving yield and profitability in the rice-wheat system of Eastern India. Field Crops Res. 250, 107776. doi: 10.1016/j.fcr.2020.107776
Singh, Y. V., Singh, K. K., Sharma, S. K. (2013). Influence of crop nutrition on grain yield, seed quality and water productivity under two rice cultivation systems. Rice Sci. 20 (2), 129–138. doi: 10.1016/S1672-6308(13)60113-4
Singh, P., Singh, G., Sodhi, G. P. S. (2019). Energy auditing and optimization approach for improving energy efficiency of rice cultivation in south-western punjab, India. Energy 174, 269–279. doi: 10.1016/j.energy.2019.02.169
Soni, P., Soe, M. N. (2016). Energy balance and energy economic analyses of rice production systems in ayeyarwaddy region of Myanmar. Energy Efficiency 9, 223–237. doi: 10.1007/s12053-015-9359-x
SRI-Rice (2020) Countries. Available at: http://sri.cals.cornell.edu/countries (Accessed on 5 January 2020).
Subbiah, B., Asija, G. L. (1956). Alkaline permanganate method of available nitrogen determination. Curr Sci 25, 259.
Susilawati, H. L., Setyanto, P., Kartikawati, R., Sutriadi, M. T. (2019). “The opportunity of direct seeding to mitigate greenhouse gas emission from paddy rice field,” in IOP conference series: Earth and environmental science, vol. 393. (IOP Publishing), 012042.
Swain, C. K., Bhattacharyya, P., Singh, N. R., Neogi, S., Sahoo, R. K., Nayak, A. K., et al. (2016). Net ecosystem methane and carbon dioxide exchange in relation to heat and carbon balance in lowland tropical rice. Ecol. Eng. 95, 364–374. doi: 10.1016/j.ecoleng.2016.06.053
Swain, C. K., Nayak, A. K., Bhattacharyya, P., Chatterjee, D., Chatterjee, S., Tripathi, R., et al. (2018). Greenhouse gas emissions and energy exchange in wet and dry season rice: eddy covariance-based approach. Environ. Monit. Assess. 190, 1–17. doi: 10.1007/s10661-018-6805-1
Tabatabai, M. A. (1972). Use of p-nitro-phenyl phosphate for assay of soil phosphatase activity. Soil Biol. Biochem. 4, 479–487. doi: 10.1016/0038-0717(72)90064-8
Talla, A., Jena, S. N. (2014). Evaluation of crop establishment and weed management practices on growth and yield parameters of rice under rainfed conditions. Curr. Biot 8, 257–269.
Tariq, A., Vu, Q. D., Jensen, L. S., de Tourdonnet, S., Sander, B. O., Wassmann, R., et al. (2017). Mitigating CH4 and N2O emissions from intensive rice production systems in northern Vietnam: Efficiency of drainage patterns in combination with rice residue incorporation. Agriculture Ecosyst. Environ. 249, 101–111. doi: 10.1016/j.agee.2017.08.011
Tarlera, S., Capurro, M. C., Irisarri, P., Scavino, A. F., Cantou, G., Roel, A. (2016). Yield-scaled global warming potential of two irrigation management systems in a highly productive rice system. Scientia Agricola 73, 43–50. doi: 10.1590/0103-9016-2015-0050
Thakur, A. K., Mohanty, R. K., Patil, D. U., Kumar, A. (2014). Impact of water management on yield and water productivity with system of rice intensification (SRI) and conventional transplanting system in rice. Paddy Water Environ. 12 (4), 413–424. doi: 10.1007/s10333-013-0397-8
Thakur, A. K., Rath, S., Patil, D. U., Kumar, A. (2011). Effects on rice plant morphology and physiology of water and associated management practices of the system of rice intensification and their implications for crop performance. Paddy Water Environ. 9 (1), 13–24. doi: 10.1007/s10333-010-0236-0
Thakur, A. K., Uphoff, N. T., Stoop, W. A. (2016). Scientific underpinnings of the system of rice intensification (SRI): What is known so far? Adv. Agron. 135, 147–179. doi: 10.1016/bs.agron.2015.09.004
Tian, J., Dippold, M., Pausch, J., Blagodatskaya, E., Fan, M., Li, X., et al. (2013). Microbial response to rhizodeposition depending on water regimes in paddy soils. Soil Biol. Biochem. 65, 195–203. doi: 10.1016/j.soilbio.2013.05.021
Tirol-Padre, A., Minamikawa, K., Tokida, T., Wassmann, R., Yagi, K. (2018). Site-specific feasibility of alternate wetting and drying as a greenhouse gas mitigation option in irrigated rice fields in southeast Asia: a synthesis. Soil Sci. Plant Nutr. 64 (1), 2–13. doi: 10.1080/00380768.2017.1409602
Totin, E., Segnon, A. C., Schut, M., Affognon, H., Zougmoré, R. B., Rosenstock, T., et al. (2018). Institutional perspectives of climate-smart agriculture: A systematic literature review. Sustainability 10 (6), 1990. doi: 10.3390/su10061990
Turner, B. L., Hopkins, D. W., Haygarth, P. M., Ostle, N. (2002). β-glucosidase activity in pasture soils. Appl. Soil Ecol. 20 (2), 157–162. doi: 10.1016/S0929-1393(02)00020-3
Vance, E. D., Brookes, P. C., Jenkinson, D. S. (1987). Microbial biomass measurements in forest soils: determination of kc values and tests of hypotheses to explain the failure of the chloroform fumigation-incubation method in acid soils. Soil Biol. Biochem. 19 (6), 689–696. doi: 10.1016/0038-0717(87)90050-2
Verhoeven, E., Barthel, M., Yu, L., Celi, L., Said-Pullicino, D., Sleutel, S., et al. (2019). Early season n 2 O emissions under variable water management in rice systems: source-partitioning emissions using isotope ratios along a depth profile. Biogeosciences 16 (2), 383–408. doi: 10.5194/bg-16-383-2019-supplement
Walkley, A., Black, I. A. (1934). An examination of the degtjareff method for determining soil organic matter, and a proposed modification of the chromic acid titration method. Soil Sci. 37 (1), 29–38. doi: 10.1097/00010694-193401000-00003
Watts, D. B., Torbert, H. A., Feng, Y., Prior, S. A. (2010). Soil microbial community dynamics as influenced by composted dairy manure, soil properties, and landscape position. Soil Sci. 175 (10), 474–486. doi: 10.1097/SS.0b013e3181f7964f
Wen, T., Yu, G. H., Hong, W. D., Yuan, J., Niu, G. Q., Xie, P. H., et al. (2022). Root exudate chemistry affects soil carbon mobilization via microbial community reassembly. Fundam. Res. 2 (5), 697–707. doi: 10.1016/j.fmre.2021.12.016
Williams, S. T., Davies, F. L. (1965). Use of antibiotics for selective isolation and enumeration of actinomycetes in soil. Microbiology 38 (2), 251–261. doi: 10.1099/00221287-38-2-251
Witt, C., Gaunt, J. L., Galicia, C. C., Ottow, J. C., Neue, H. U. (2000). A rapid chloroform-fumigation extraction method for measuring soil microbial biomass carbon and nitrogen in flooded rice soils. Biol. Fertility Soils 30, 510–519. doi: 10.1007/s003740050030
Wolińska, A., Bennicelli, R. P. (2010). Dehydrogenase activity response to soil reoxidation process described as varied conditions of water potential, air porosity and oxygen availability. Polish J. Environ. Stud. 19 (3).
World Bank. World Bank Institute (2010). Available at: http://info.worldbank.org/etools/docs/library/245848/ (Accessed on 5 January 2020).
Xu, Y., Ge, J., Tian, S., Li, S., Nguy-Robertson, A. L., Zhan, M., et al. (2015). Effects of water-saving irrigation practices and drought resistant rice variety on greenhouse gas emissions from a no-till paddy in the central lowlands of China. Sci. Total Environ. 505, 1043–1052. doi: 10.1016/j.scitotenv.2014.10.073
Yang, S., Peng, S., Xu, J., Luo, Y., Li, D. (2012). Methane and nitrous oxide emissions from paddy field as affected by water-saving irrigation. Phys. Chem. Earth Parts A/B/C 53, 30–37. doi: 10.1016/j.pce.2011.08.020
Zhang, Y., Hou, W., Chi, M., Sun, Y., An, J., Yu, N., et al. (2020). Simulating the effects of soil temperature and soil moisture on CO2 and CH4 emissions in rice straw-enriched paddy soil. Catena 194, 104677. doi: 10.1016/j.catena.2020.104677
Zhang, Y., Tang, Q., Peng, S., Xing, D., Qin, J., Laza, R. C., et al. (2012). Water use efficiency and physiological response of rice cultivars under alternate wetting and drying conditions. Sci. World J 2012. doi: 10.1100/2012/287907
Keywords: rice production techniques, energy budget, global warming potential, climate smart index, nutrient use efficiency
Citation: Mohapatra KK, Nayak AK, Patra RK, Tripathi R, Swain CK, Moharana KC, Kumar A, Shahid M, Mohanty S, Garnaik S, Nayak HS, Mohapatra S, Nagothu US and Tesfai M (2023) Multi-criteria assessment to screen climate smart rice establishment techniques in coastal rice production system of India. Front. Plant Sci. 14:1130545. doi: 10.3389/fpls.2023.1130545
Received: 23 December 2022; Accepted: 23 March 2023;
Published: 18 April 2023.
Edited by:
Sowbiya Muneer, VIT University, IndiaReviewed by:
Ajay Kumar Bhardwaj, Central Soil Salinity Research Institute (ICAR), IndiaAung Zaw Oo, Japan International Research Center for Agricultural Sciences (JIRCAS), Japan
Copyright © 2023 Mohapatra, Nayak, Patra, Tripathi, Swain, Moharana, Kumar, Shahid, Mohanty, Garnaik, Nayak, Mohapatra, Nagothu and Tesfai. This is an open-access article distributed under the terms of the Creative Commons Attribution License (CC BY). The use, distribution or reproduction in other forums is permitted, provided the original author(s) and the copyright owner(s) are credited and that the original publication in this journal is cited, in accordance with accepted academic practice. No use, distribution or reproduction is permitted which does not comply with these terms.
*Correspondence: A. K. Nayak, YWtuYXlhazIwQGdtYWlsLmNvbQ==