- 1College of Horticulture, Nanjing Agricultural University, Nanjing, Jiangsu, China
- 2Fruit Crop Variety Improvement and Seedling Propagation Engineering Research Center of Jiangsu Province, Nanjing, Jiangsu, China
- 3School of Agronomy and Horticulture, Jiangsu Vocational College of Agriculture and Forestry, Jurong, Jiangsu, China
- 4Institute of Pomology, Jiangsu Academy of Agricultural Sciences, Nanjing, Jiangsu, China
- 5College of Life Sciences, Tarim University, Alar, Xinjiang, China
Drought is a common and serious abiotic stress in viticulture, and it is urgent to select effective measures to alleviate it. The new plant growth regulator 5-aminolevulinic acid (ALA) has been utilized to alleviate abiotic stresses in agriculture in recent years, which provided a novel idea to mitigate drought stress in viticulture. The leaves of ‘Shine Muscat’ grapevine (Vitis vinifera L.) seedlings were treated with drought (Dro), drought plus 5-aminolevulinic acid (ALA, 50 mg/L) (Dro_ALA) and normal watering (Control) to clarify the regulatory network used by ALA to alleviate drought stress in grapevine. Physiological indicators showed that ALA could effectively reduce the accumulation of malondialdehyde (MDA) and increase the activities of peroxidase (POD) and superoxide dismutase (SOD) in grapevine leaves under drought stress. At the end of treatment (day 16), the MDA content in Dro_ALA was reduced by 27.63% compared with that in Dro, while the activities of POD and SOD reached 2.97- and 5.09-fold of those in Dro, respectively. Furthermore, ALA reduces abscisic acid by upregulating CYP707A1, thus, relieving the closure of stomata under drought. The chlorophyll metabolic pathway and photosynthetic system are the major pathways affected by ALA to alleviate drought. Changes in the genes of chlorophyll synthesis, including CHLH, CHLD, POR, and DVR; genes related to degradation, such as CLH, SGR, PPH and PAO; the RCA gene that is related to Rubisco; and the genes AGT1 and GDCSP related to photorespiration form the basis of these pathways. In addition, the antioxidant system and osmotic regulation play important roles that enable ALA to maintain cell homeostasis under drought. The reduction of glutathione, ascorbic acid and betaine after the application of ALA confirmed the alleviation of drought. In summary, this study revealed the mechanism of effects of drought stress on grapevine, and the alleviating effect of ALA, which provides a new concept to alleviate drought stress in grapevine and other plants.
1 Introduction
With the intensification of global warming, the occurrence of drought stress will be more frequent, which will exacerbate problems with agricultural cultivation (Chaves et al., 2010). Drought seriously affects plant growth and development and reduces the growth rate of crops. This is primarily because drought affects the leaf size, stem elongation and root proliferation, stomatal movement, and water and nutrient relations of plants (Farooq et al., 2012). Under conditions of water shortage, plant leaves wither, and the edges turn yellow, which inhibits leaf development (Fanizza and Ricciardi, 2015). Furthermore, drought can result in the destruction of chloroplasts and photosynthetic machinery, which leads to a reduction in the content of chlorophyll and a significant decrease in the efficiency of plant photosynthesis (Cornic and Massacci, 1996; Reddy et al., 2004; Farooq et al., 2009; Zargar et al., 2017). The reduction in photosynthesis primarily occurs owing to stomatal or non-stomatal factors (Bota et al., 2004; Zhou et al., 2007). Moreover, drought will cause oxidative stress in plants from the cellular level, the closure of stomata, and the inhibition of photosynthetic electron transport chain, which results in the overproduction of reactive oxygen species (ROS) (Moran et al., 1994; Sade et al., 2011; Hasanuzzaman et al., 2013). Plants have evolved efficient mechanisms to adapt to drought. Stomatal closure is the first reaction to reduce transpiration under drought, which is primarily maintained by the accumulation of phytohormones, such as ABA (Pirasteh-Anosheh et al., 2016). Antioxidant and scavenging defense systems are the important bases of drought tolerance. The activities of enzymatic components, such as superoxide dismutase (SOD) and peroxidase (POD), as well as the contents of non-enzymatic components, such as glutathione (GSH), ascorbate (AsA) and α-tocopherol will change in response to oxidative stress under drought (Terzi and Kadioglu, 2006; Laxa et al., 2019). Moreover, osmotic accumulation (OA) is also a key mechanism of drought tolerance in plants, which involves the accumulation of organic solutes, such as proline, betaine (N, N, N-trimethyl glycine), soluble sugars, and sugar alcohols, and a series of inorganic salt ions, such as Ca2+, K+ and Cl-, to reduce the cell osmotic potential and maintain water relationship (Serraj and Sinclair, 2002; Fang and Xiong, 2015). Together, these mechanisms provide drought tolerance to plants.
Various exogenous applications of substances have been proposed to mitigate drought, which is the most common and harmful abiotic stress. For example, the combined application of 24-epibrassinolide and spermine alleviates drought-induced oxidative stress in maize (Zea mays L.) (Talaat et al., 2015). The application of melatonin can improve the drought tolerance of loquat (Eriobotrya japonica L.) seedlings (Wang et al., 2021a). The exogenous application of betaine and potassium fertilizer can improve water relationship and the yield of wheat (Triticum aestivum L.) under drought conditions (Raza et al., 2014). The application of exogenous glycine betaine and salicylic acid can improve the water relationship of hybrid sunflower (Helianthus) under conditions of water shortage (Hussain et al., 2009). As a non-toxic endogenous plant growth regulator, 5-aminolevulinic acid (ALA) has a substantial potential to alleviate abiotic stress on plants. Studies have shown that ALA can improve photosynthesis, photosystem efficiency, and the antioxidant capacity of plants (Memon et al., 2009). ALA also has the potential to alleviate some common abiotic stresses in plants, including salinity, temperature, and drought stresses (Hodgins and Van Huystee, 1986; Watanabe et al., 2000; Phung et al., 2011; Zhang et al., 2012). However, most of these studies focused on physiological aspects, and the exact mechanism of action of ALA’s mitigating effect still needs to be fully elucidated.
There is a large and growing global market for grapes (V. vinifera) and grape-based products. In addition to its huge economic value, as the first fruit crop whose whole genome was sequenced (Jaillon et al., 2007), many studies related to grapevine have been reported, making grapevine the model perennial fruit crop species (Gambetta et al., 2020). During the process of viticulture, vineyards are often affected by various abiotic stresses, of which drought is the most serious. Most vineyards face prolonged drought during the summer, thus, limiting grapevine growth (Lovisolo et al., 2016). ALA, a plant growth regulator that can enhance plant stress resistance, could provide a new concept to reduce drought damage in vineyards. In this study, leaves of the grapevine cultivar ‘Shine Muscat’ (‘SM’) (Vitis labruscana × Vitis vinifera) were used as research materials. The leaves were classified into control, drought treatment (Dro), and drought plus ALA (Dro_ALA) treatment. The morphological and physiological characteristics of three treated leaves were measured to examine the content of malondialdehyde (MDA) and the activities of antioxidant enzymes, such as SOD and POD. Furthermore, we combined transcriptomic and metabolome analyses to compare differentially expressed genes (DEGs) and differentially abundant metabolites (DAMs) between the ALA-treated group and the non-treated group under drought stress. The purpose of this study was to determine the protective mechanism of exogenous ALA on grapevine leaves under drought and to construct the regulatory network of drought resistance in grapevine leaves, thus, providing theoretical support for subsequent related studies.
2 Materials and methods
2.1 Plant materials, treatments, and sampling
Two-year-old ‘SM’ grapevines were grown in the heliogreenhouse (relative humidity of ~85% and a temperature regime of 25 °C day/15 °C night) of the Baima Teaching and Research Base of Nanjing Agricultural University, Nanjing, China (31°36′36′′ N, 119°10′48′′ E). They were used as the plant material for this study. Equal proportions of perlite, peat and horticultural vermiculite (1:1:1, v/v/v) were used to grow the grape seedlings. First, each pot of seedling soil was flooded with water and then allowed to dry out. Ten days after the watering had stopped was defined as 0 d, and the soil water content was below 9% at this time. The leaves in the Dro_ALA treatment was sprayed with 50 mg·L-1 ALA on both sides until the leaf surface was soaked once every three days from 0 d. The same amount of distilled water was sprayed on the leaves for the Dro treatment. The control plants were watered daily to field capacity. Leaves were collected at 0, 2, 4, 6, 8, 10, 12, 14, and 16 days, and phenotypic observations and physiological indices were measured.
2.2 Measurement of physiological and biochemical responses
The content of MDA and activities of POD and SOD were measured as previously described (Beauchamp and Fridovich, 1971; Zheng and Van Huystee, 1992; Tewari et al., 2002). Cellulose acetate glue was applied to the leaf surface to form a thin layer, and the glue was dried into a thin film, which was imaged in an automatic positive fluorescence microscope (DM6 B; Leica Microsystems, Wetzlar, Germany). The stomatal apertures were also measured using this system. The soil water content (WC) was determined by the gravimetric method (Little et al., 1998). Each sample had three biological replicates.
2.3 Transcriptome and metabolome sequencing and multi-omics analysis
Transcriptome sequencing was performed on samples from the 16th day of the treatments. Total RNA was extracted and sequenced as described by Huang et al. (2020). Reads obtained from the sequencing machines included raw reads that contained adapters or low-quality bases that would affect the following assembly and analysis. Thus, to obtain high quality clean reads, the reads were further filtered by FASTP version 0.18.0 (Chen et al., 2018). The parameters were as follows: (1) removal of the reads that contained adapters; (2) removal of the reads that contained > 10% of unknown nucleotides (N); and (3) removal of low quality reads that contained > 50% of low quality (Q-value ≤ 20) bases. |log2 fold change (FC)| ≥ 1.0 and adjusted P-value (padj) < 0.05 were used as the screening criteria for DEGs. Metabolome sequencing was determined by liquid chromatography-mass spectrometry (LC-MS) based on high-performance liquid chromatography (HPLC) (UltiMate 3000; Thermo Fisher Scientific, Waltham, MA, USA) and mass spectrometry (Q Exactive; Thermo Fisher Scientific). As for DAMs, the variable importance in the projection (VIP) value of a multivariate statistical analysis of orthogonal projections to latent structure discriminant analysis (OPLS-DA) and the t-test P-values of univariate statistical analysis were combined to screen the metabolites with significant differences between the different comparison groups (VIP > 1 and P-value < 0.05) (Saccenti et al., 2014). The transcriptomic and metabolomic data were integrated by a two-way orthogonal partial least squares (O2PLS) analysis (Bylesjö et al., 2007) using the Omics PLS package. Pearson correlation coefficients were calculated to integrate the metabolome and transcriptome data. Gene and metabolite pairs were ranked in the descending order of absolute correlation coefficients. The top 50 genes and metabolites were selected for heatmap analysis using pheatmap packages in the R project. Additionally, the top 250 pairs of genes and metabolites (with an absolute Pearson correlation > 0.5) were subjected to metabolite-transcript network analysis using igraph packages in the R project (Csardi and Nepusz, 2006). Cytoscape software (version 3.6.1) was used to visualize the pairs of genes and metabolites.
2.4 Analysis of the experimental results
The DEGs and DAMs between the groups were measured to analyze the major response of the ‘SM’ grapevine to drought stress and the mitigating effects of ALA. Comparison groups were established as follows: (I) DEGs and DAMs between the control versus Dro (control vs. Dro) comparison group were analyzed to determine the effect of drought stress on grapevine. (II) The control versus Dro_ALA (control vs. Dro_ALA) comparison group was used to analyze the difference between the ALA treatment after drought stress and the control treatment, and (III) The alleviating effect of ALA on drought stress was determined by analyzing the DEGs and DAMs between the Dro and Dro_ALA (Dro vs. Dro_ALA) treatments.
2.5 Statistical analysis
TBtools software (version 1.0692) and MapMan software (version 3.6.0) were used to analyze the functions of DEGs (Thimm et al., 2004). The data were expressed as the mean ± standard deviation (SD). A one-way analysis of variance (ANOVA) was used to analyze the data. Tukey’s multi-range test was conducted using GraphPad Prism software (version 8.0.2) (GraphPad Software, San Diego, CA, USA) to determine significant differences between and within groups at P < 0.05 (Saccenti et al., 2014).
2.6 Validation of RNA-seq using RT-qPCR
Ten DEGs were randomly selected for quantitative reverse transcription PCR (RT-qPCR) analysis to verify the precision and repetitiveness of the transcriptome analytical results. Purified RNA samples were reverse-transcribed using the Revert Aid™ First-Strand cDNA Synthesis Kit (Fermentas, Glen Burnie, MD, USA) following the manufacturer’s instructions. Real-time quantitative reverse transcription PCR (qRT-PCR) was performed using a Quantagene q225 system (Kubo Tech, Beijing, China). Specific primers were designed using Primer Premier 5 software (Table S1; Premier Biosoft, Palo Alto, CA, USA). The V. vinifera actin gene (VvActin, AB073011) was used as the internal control gene. Each sample had three replicates. Gene expression was calculated using the 2-ΔΔCT method (Livak and Schmittgen, 2001).
3 Results
3.1 ALA can alleviate drought stress on grapevine leaves by increasing the activity of antioxidant enzymes
The leaf morphology of ‘SM’ grapevine seedlings was significantly affected by drought treatment. Under drought conditions, the leaves first wilted on the fourth day, and they were significantly withered and became yellowed on the tenth and the 16th days, respectively. The leaves were already producing significant drought symptoms. However, the Dro_ALA leaves showed only mild symptoms of drought stress (Figures 1A-C and S1). The drought-induced MDA accumulation in grapevine leaves was significantly reduced by the ALA treatment, and on the tenth day, the MDA content in Dro_ALA leaves was 28.94% lower than that in the Dro leaves (Figure 1D and Table S2). The POD activity in the Dro treatment kept increasing until the sixth day, and then it gradually decreased, while the POD activity in Dro_ALA was higher than that in the Dro treatment (Figure 1E and Table S2). The activity of SOD increased first and then decreased under drought. In the Dro_ALA treatment, the SOD activity showed the same trend as that in the Dro, which peaked on the fourth day and then decreased. However, the SOD activity in the ALA treatment group decreased more slowly (Figure 1F and Table S2). These results indicate that exogenous ALA may effectively prevent ‘SM’ grapevine seedlings from prolonged drought by increasing the activity of antioxidant enzymes. Moreover, on the 16th day, the symptoms of drought stress were evident, so the leaf samples of the control, Dro and Dro_ALA plants from the 16th day of treatment were collected for transcriptome and metabolome sequencing.
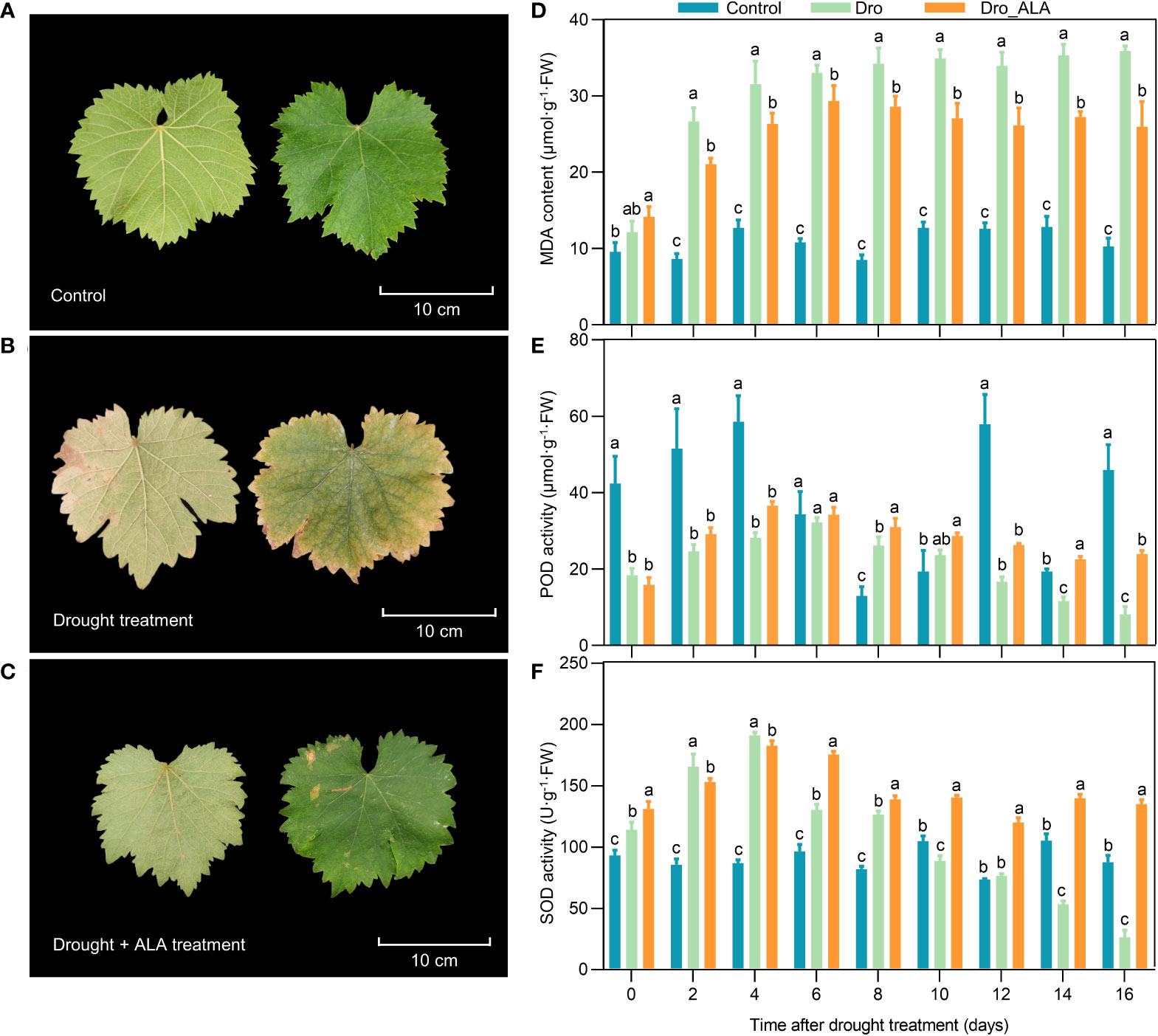
Figure 1 Leaf phenotype observation and physiological index determination (A) Leaf phenotype of control on the 16th day. (B) Leaf phenotype of drought treatment (Dro) on the 16th day. (C) Leaf phenotype of drought plus 5-aminolevulinic acid treatment (Dro_ALA) on the 16th day. (D) Malondialdehyde (MDA) content changes with time under control, Dro and Dro_ALA groups. (E) Peroxidase (POD) activity changes with time under control, Dro and Dro_ALA groups. (F) Superoxide dismutase (SOD) activity changes with time under control, Dro and Dro_ALA groups. In Figure 1C, the left side of each picture shows the bottom surface of the leaf, and the right side shows the top side of the leaf. Different letters in a column indicate significance of difference between treatments (P ≤ 0.05).
3.2 Mechanisms of the response of grapevine leaves to drought and ALA
The control, Dro, and Dro_ALA samples were subjected to RNA-seq and metabolome analyses (Table S3). A total of 6,326, 5,790, and 2,680 DEGs (Figure 2A) and 255, 147, and 327 DAMs (Figure 2B) were detected between the three comparison groups (control vs. Dro; control vs. Dro_ALA; and Dro vs. Dro_ALA). The intersection of different numbers of DEGs in each comparison is shown in Figure 2A. We demonstrated the correlation of each sample in Figures S2 and S3 and Tables S3 and S4, and we found that the DEGs significantly correlated with the DAMs (PCC) > 0.8 (Figure S4). Details of the DEGs and DAMs are shown in Tables S5-S20. A Gene Ontology (GO) analysis indicated that ‘thylakoid’, ‘thylakoid part’, ‘chloroplast’, and ‘chloroplast part’ were enriched in the control vs. Dro and control vs. Dro_ALA comparison group. This result suggests that drought could affect chlorophyll anabolism. In the meantime, the enrichment of ‘photosystem’, ‘photosynthesis membrane’ in the control vs. Dro and control vs. Dro_ALA indicated that photosynthesis was affected under drought stress (Figures 2C and S5; Table S17). Consistent with this, the Kyoto Encyclopedia of Genes and Genomes (KEGG) enrichment analysis of the DEGs revealed that ‘porphyrin and chlorophyll metabolism’, ‘photosynthesis-antenna proteins,’ and ‘photosynthesis’ were significantly enriched in the control vs. Dro comparison group (Figures 2D and S6; Table S18). In the control vs. Dro_ALA comparison group, most of the DEGs in GO terms that were related to chlorophyll metabolism and photosynthesis were upregulated (Figures 2C and S5; Table S17), indicating that ALA could play a positive role in mitigating the inhibition of photosynthesis in grapevine under drought. Furthermore, phytohormones play a role in drought tolerance, and ALA alleviates drought in grapevine. ‘Response to hormone,’ ‘cellular response to hormone stimulus’ in GO and ‘plant hormone signal transduction’ in KEGG were significantly enriched. Moreover, the enrichment of molecular function, such as ‘oxidoreductase activity’ in GO and ‘peroxisome’ and ‘ascorbate and aldarate metabolism’ in the KEGG analysis in each comparison group showed that antioxidant systems also play a vital role in drought resistance in grapevine and the process of alleviating drought stress by ALA (Figures 2D and S6; Table S18). The KEGG analysis of the metabolome also detected enriched terms, such as the ‘biosynthesis of secondary metabolism’ (Figure S7 and Table S19). Moreover, qRT-PCR was used to validate the reliability of RNA-seq (Figure S8; Table S1). The function of DEGs was analyzed using MapMan software as shown in Tables S20 and S21.
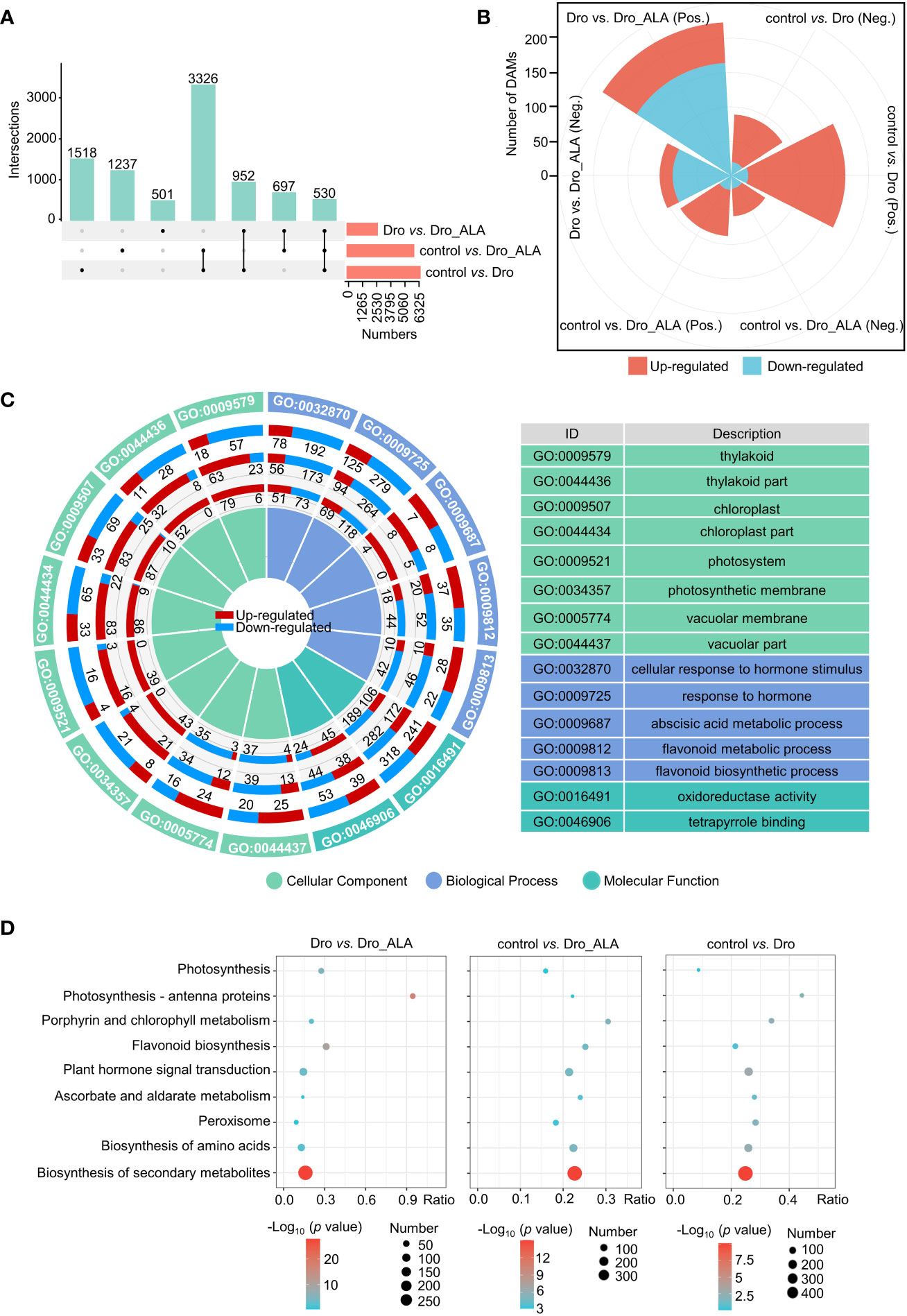
Figure 2 Functional annotations of total DEGs and DAMs. (A) Upset plot of DEGs between control and drought treatment (control vs. Dro); control and drought plus 5-aminolevulinic acid treatment (control vs. Dro_ALA); drought and drought and 5-aminolevulinic acid treatment (Dro vs. Dro_ALA). (B) Statistics of significant difference of DAMs between control vs. Dro, control vs. Dro_ALA, and Dro vs. Dro_ALA. The threshold of significant difference was VIP≥1 and T-test P<0.05.(Pos. means positive and Neg. means negative) (C) Information about key GO terms of control vs. Dro, control vs. Dro_ALA, and Dro vs. Dro_ALA. The first circle represents the GO ID, the second circle represents the DEGs between control vs. Dro, the third circle for control vs. Dro_ALA, and the fourth for Dro vs. Dro_ALA. Red and blue indicate up-regulated and down-regulated DEGs, respectively. (D) Information about key pathways in the KEGG enrichment pathways of control vs. Dro, control vs. Dro_ALA, and Dro vs. Dro_ALA.
3.3 ALA inhibits stomatal closure induced by abscisic acid in grapevine leaves under drought stress
In this study, the grapevine stomatal aperture was found to be significantly reduced compared with the control under drought. Although it was still smaller than the control, the stomatal aperture increased after the ALA treatment (Figures 3A, B; Table S22). In the 16th day, the stomatal aperture of control was 103.7% larger than that of Dro, while Dro_ALA was 58.4% larger than that of Dro. (Figures 3A, B, Table S22). It is a consensus that ABA induces plants to close their stomata to resist stress under drought (Bray, 1997; Buckley, 2019; Ilyas et al., 2020). The DEGs of factors associated with ABA synthase, such as neoxanthin synthase (ABA4, |log2 FC| = 1.26) and 9-cis-epoxycarotenoid dioxygenase6 (NCED6, |log2 FC| = 3.35) were significantly upregulated in the control vs. Dro comparison. Simultaneously, in the Dro treatment, the genes of factors related to the decomposition of ABA, such as abscisic acid 8’-hydroxylase (CYP707A1, CYP707A2, and CYP707A4), were expressed at lower levels than those in the control. This led to the accumulation of endogenous ABA under drought. In the metabolome, (S)-abscisic acid (POS_M265T406; Log10 content from 8.71 to 8.82) increased after drought treatment, which was consistent with the results described above. It is worth noting that the upregulation of ABF2 (|log2 FC| = 1.48), an ABRE-binding bZIP factor, was also detected as upregulated in the control vs. Dro comparison, which confirmed the accumulation of ABA (Figure 3C). The downregulation of some genes, such as ABA4 (|log2 FC| = 0.53), NCED6 (|log2 FC| = 0.41), and ABF2 (|log2 FC| = 0.04), were detected in the Dro vs. Dro_ALA comparison. Moreover, CYP707A1 (|log2 FC| = 1.79) was significantly upregulated after the application of ALA. In the metabolome, the content of (S)-abscisic acid in Dro_ALA was lower than that of Dro (Log10 content from 8.82 to 8.70). These findings suggest that ALA could primarily reduce the accumulation of ABA by accelerating the degradation of ABA, thus, resulting in the re-enlargement of stomatal aperture.
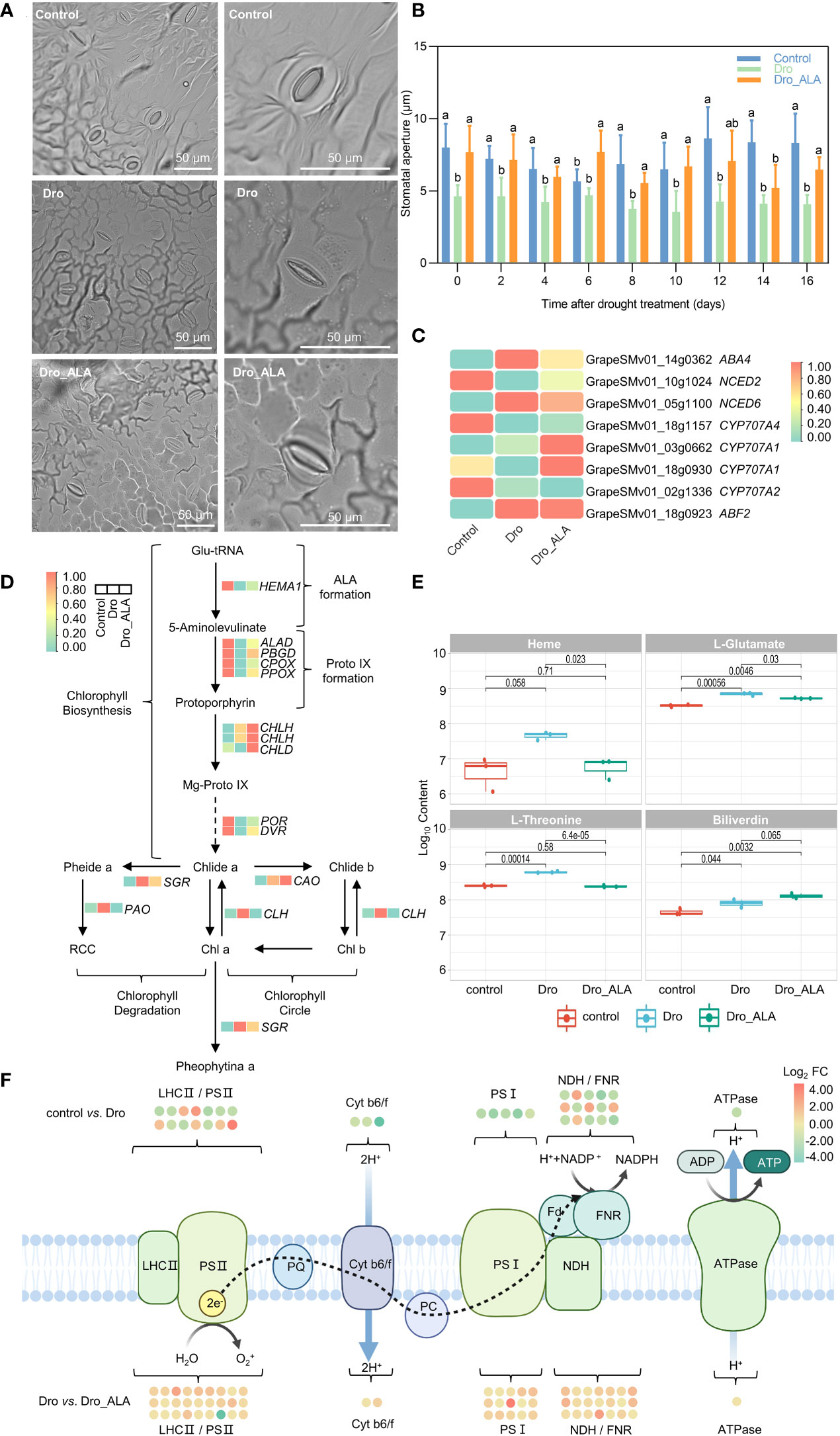
Figure 3 Stomatal movement, chlorophyll metabolism and photosynthesis in grapevine leaves under drought stress and drought plus ALA treatment. (A) Stomatal aperture observation of control, drought treatment (Dro), and drought plus 5-aminolevulinic acid treatment (Dro_ALA) on the 16th day (via upright fluorescent microscope). (B) Variation of stomatal aperture with drought treatment time. (C) Key DEGs related to phytohormones action between control vs. Dro, control vs. Dro_ALA, and Dro vs. Dro_ALA. (D) Pathway of chlorophyll metabolism and key genes in grapevine under drought stress and drought plus 5-aminolevulinic acid treatment. Arrows (↑) and (↓) indicate up-regulation and down-regulation, respective. Red indicates the control vs. Dro comparison group and blue indicates the Dro vs. Dro_ALA comparison group. (E) Key metabolites in chlorophyll metabolism pathway under control, Dro and Dro_ALA treatments. (F) Effects of drought and drought plus 5-aminolevulinic acid on photosynthesis. Each dots represents a DEG, green and red indicate up-regulation and down-regulation, respectively. Different letters indicate significant differences among experimental groups (p < 0.05).
3.4 ALA alleviates the inhibition of photosynthesis by drought on grapevine leaves
Phenotypic observations showed that the leaves suffered from severe chlorosis under drought (Figures 1 and S1), which could be related to the degradation of chlorophyll. In the control vs. Dro comparison, the level of expression of glutamyl-tRNA reductase (HEMA1) was downregulated by 31.2%, which led to the inhibition of endogenous ALA formation (Figure 3D; Table S25). Transcripts involved in the factors of protoporphyrin synthesis, such as porphobilinogen synthase (ALAD), porphobilinogen deaminase (PBGD), coproporphyrinogen III oxidase (CPOX), and protoporphyrinogen oxidase (PPOX), were all maintained at low levels of expression under drought conditions (Figure 3D; Table S25). The genes for protochlorophyllide oxidoreductase (POR) and divinyl chlorophyllide a 8-vinyl-reductase (DVR) were also downregulated in the control vs. Dro comparison (Figure 3D; Table S25). These results indicate that chlorophyll biosynthesis is inhibited under drought stress. In contrast, chlorophyll conjugation and degradation genes, such as the genes that encode chlorophyllide a oxygenase (CAO), chlorophyllase (CLH), magnesium dechelatase (SGR), and pheophorbide a oxygenase (PAO), were all downregulated in the control vs. Dro comparison. Changes in these genes led to a reduction in chlorophyll accumulation in grapevine leaves under drought (Figure 3D; Table S25). In porphyrin metabolism, an increase of L-glutamate (Log10 content from 8.52 to 8.85) and L-threonine (Log10 content from 8.52 to 8.85) were detected (Figures 3E and S9).
In this study, the application of ALA upregulated the levels of expression of genes related to chlorophyll synthesis and inhibited the levels of expression of the genes related to degradation. Compared with Dro, the levels of expression of genes, such as HEMA1, ALAD, PBGD, CPOX, and PPOX, all increased in Dro_ALA. Simultaneously, in the Dro vs. Dro_ALA comparison, CHLH, CHLD, POR, and DVR were all upregulated, indicating that the inhibition of chlorophyll synthesis was relieved. Moreover, the downregulation of CLH, SGR, PPH, and PAO was detected in Dro vs. Dro_ALA, which resulted in the inhibition of chlorophyll degradation (Figure 3D; Table S25). In the metabolome, the contents of L-glutamate (Log10 content from 8.85 to 8.72) and biliverdin (Log10 content from 7.91 to 8.11) increased, while those of heme (Log10 content from 7.67 to 6.80) and L-threonine (Log10 content from 8.79 to 8.39) decreased (Figures 3E and S9). These combined effects resulted in an increase in chlorophyll synthesis and a decrease in chlorophyll degradation after the application of ALA.
The photosynthetic electron transport chains were also inhibited under drought. A comparison of Dro to the Control showed that approximately 66% DEGs that are involved in photosynthesis were downregulated (Figure 3F; Table S24). Eight of the 14 DEGS related to photosystem II were downregulated in the Control vs. Dro comparison. Most of them were associated with the LHC-II complex and PSII assembly and maintenance. The functions of cytochrome b6/f complex, photosystem I, and ATP synthase complex were also inhibited, and the corresponding three, five and one DEGs detected in the control vs. Dro, respectively, were all downregulated. Furthermore, seven of the DEGs related to the NADH dehydrogenases were downregulated in the control vs. Dro. As a vital enzyme in the Calvin cycle, the activity of Rubisco was also inhibited under drought. This could be owing to the downregulation of DEGs related to the CPN20 auxiliary co-chaperone (CPN20, |log2FC| = 7.21) and BSD2 assembly factor (BSD2). The downregulation of genes described above resulted in a significant decrease in the photosynthetic efficiency under drought stress. Moreover, the DEGs related to glycolate oxidase (GLO1), glutamate-glyoxylate transaminase (GGAT2), and serine-glyoxylate transaminase (AGT1) were all maintained at high levels of expression in Dro, which resulted in enhanced photorespiration (Table S20). This could further weaken photosynthesis.
In the Dro vs. Dro_ALA comparison, only two of 82 DEGs related to photosynthesis were downregulated, and most of them were also downregulated in the control vs. Dro treatment (Figure 3F; Table S24). Changes in the DEGs associated with PSII (26 upregulated and one downregulated), cytb6/f complex (four upregulated), PSI (15 upregulated), ferredoxin electron carrier (one upregulated), ferredoxin NADP+ oxidoreductase (FNR) (three upregulated), NDH complex (17 upregulated) and ATP synthase complex (one upregulated) were detected (Table S24). A significant upregulation of the DEGs related to the activity, assembly, and regulation of Rubisco, such as CPN60B4, RBCX1, RBCX2, BSD2, and RCA, was detected after ALA treatment, indicating that the inhibition of Rubisco under drought was relieved. Moreover, treatment with ALA led to upregulation of the genes that encoded serine-glyoxylate transaminase (AGT1), glycine dehydrogenase component P-protein of the glycine cleavage system (GDCSP), aminomethyl transferase component T-protein of the glycine cleavage system (GDCST), and lipoamide-containing component H-protein of the glycine cleavage system (GDCSH), which indicated that ALA reduces photorespiration, and thus, alleviates drought stress (Table S21).
3.5 ALA alleviates oxidative stress in grapevine leaves
In this study, the upregulation of NADPH oxidase (RBOHA, 21.52-29.21 fragments per kilobase of transcripts per million mapped reads [FPKM]) related to ROS generation was detected in the control vs. Dro comparison, indicating that there is a mass production of ROS under drought stress (Table S26). Drought inhibited the expression of some genes related to antioxidant scavenging. For example, the DEGs related to iron superoxide dismutase (FSD3 and FSD2) and copper/zinc superoxide dismutase (SODCP) were significantly downregulated (Figure 4C; Table S26). The genes of some low-molecular weight scavengers, such as phosphomannose isomerase (PMI), related to the biosynthesis of ascorbate also showed a similar trend. The α-, β-, γ-, and δ-forms of tocopherol are active antioxidants that are primarily located in chloroplast membranes where they detoxifying singlet oxygen and lipid peroxy radicals (Munné-Bosch, 2005). In the control vs. Dro comparison group, the genes related to tocopherol biosynthesis, such as VTE1 and VTE3, were both downregulated (Figure 4B and Table S26). As expected, the levels of α-tocopherol and ascorbate were indeed decreased in the metabolome (Figure S10; Table S27). In the ascorbate-glutathione cycle, the level of expression of the gene related to ascorbate peroxidase (APX, 20.97 to 6.46 FPKM) was also suppressed. The chloroplast redox homeostasis was disrupted under drought stress, which could inhibit the photosynthesis of grapevine leaves even further. DEGs related to typical 2-Cys peroxiredoxin (2-CysPrx), atypical 2-Cys peroxiredoxin (PrxQ), M-type thioredoxin (TRM) and atypical thioredoxin (ACHT) maintained low levels of expression. Simultaneously, the levels of monodehydroascorbate reductase (MDHAR, 29.26 to 31.50 FPKM) in the ascorbic acid-glutathione (AsA-GSH) cycle and γ-glutamyl cysteine ligase (GSH) associated with glutathione biosynthesis were upregulated. The DEGs related to catalase (CAT, 305.9 to 747.97 FPKM), glutathione peroxidase (GPX6, 238.037 to 429.523 FPKM), and type-2 peroxiredoxin (PrxII) showed the same trend (Figure 4C; Table S26). In contrast, the DEGs associated with glutathione degradation, such as glutathione reductase (GR, 92.6 to 77.30 FPKM), γ-glutamyl cyclotransferase (GGCT), oxoprolinase (OXP), and dehydroascorbic acid reductase (DHAR, 42.34 to 26.08 FPKM), were downregulated (Figure 4C and Table S26). This could indicate that the glutathione content was increased to remove the ROS. Together, changes in the genes described above led to the accumulation of intracellular ROS, which caused drought oxidative stress. The metabolome data were consistent with the results described above and showed that the contents of glutathione, L-cysteine and oxidized glutathione increased (Figure S10; Table S27).
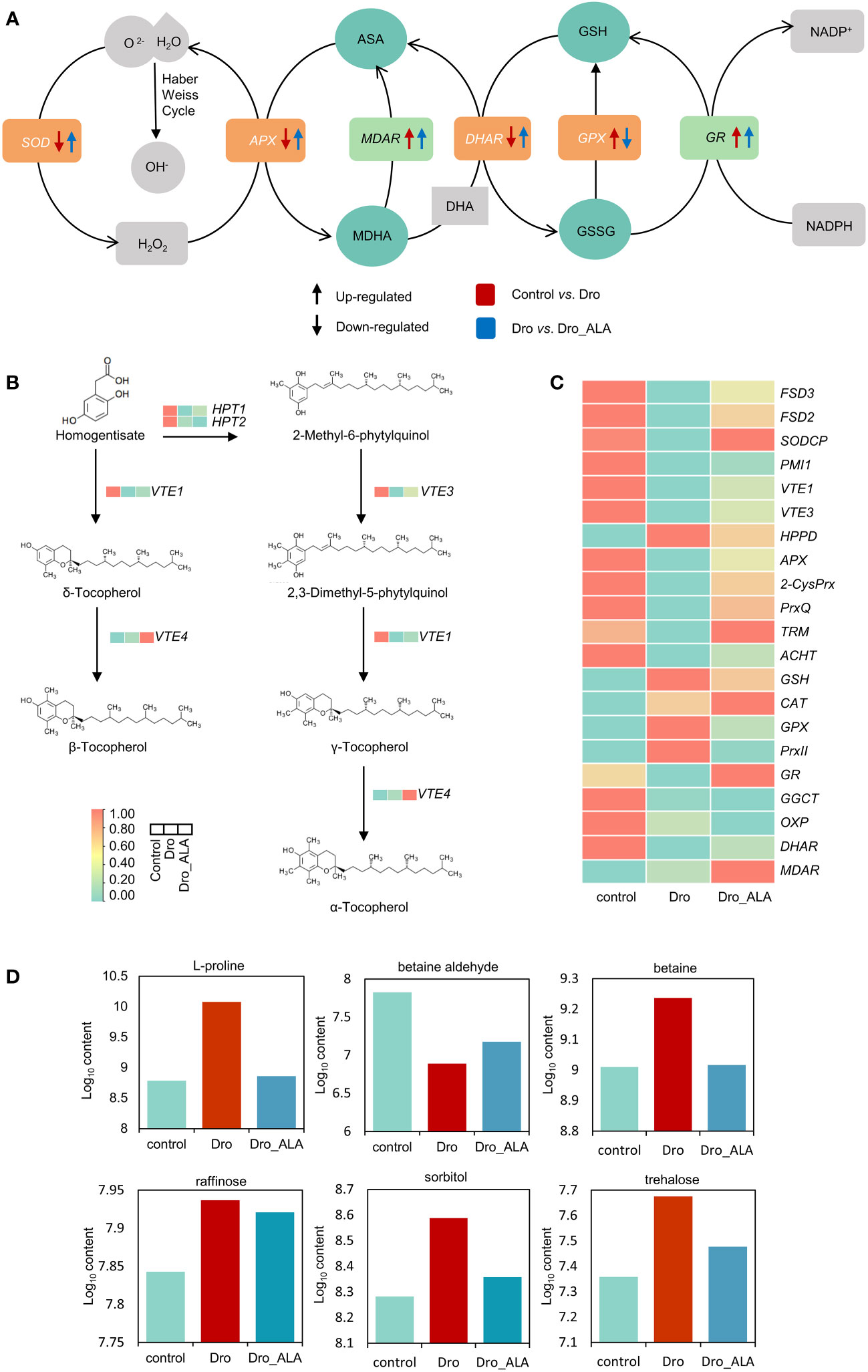
Figure 4 Cellular homeostasis under drought treatment and drought plus ALA treatment. (A) AsA-GSH cycle of grapevine leaves under drought stress and drought plus 5-aminolevulinic acid treatments, Arrows (↑) and (↓) represent up-regulation and down-regulation, respectively. Red and blue represent control vs. Dro and Dro vs. Dro_ALA groups, respectively. (B) Biosynthesis of tocopherol in grapevine leaves under control, under drought stress and drought plus 5-aminolevulinic acid treatments. Red and green represent higher and lower expression, respectively. (C) Information of DEGs related to antioxidant system of grapevine in control, Dro and Dro _ALA. (D) Information of important DAMs related to osmotic regulation and autophagy of grapevine in control, Dro and Dro _ALA. (Dro represents drought treatment; Dro_ALA represents drought plus 5-aminolevulinic acid treatment).
The application of ALA inhibited the generation of ROS, RBOHA, RBOHB, and RBOHC, which were all downregulated in the Dro vs. Dro_ALA comparison group (Table S26). ALA could enhance the function of ROS scavenging system in grapevine under drought stress. A comparison of Dro with Dro_ALA showed that FSD3, FSD2 and SODCP restored upregulation (Figure 4C; Table S26). In addition, the upregulation of VTE1 and VTE3 indicated that tocopherol was resynthesized, and the content of α-tocopherol increased in the metabolome (Figure S10 and Table S27). In the AsA-GHS cycle, ascorbate peroxidase (APX) and glutathione peroxidase (GPX8), and the genes related to type-2 peroxiredoxin (PrxII) all tended to be downregulated after application with ALA (Figure 4C; Table S26). This suggests that the drought stress was easing. The effect of ALA on photosynthesis was shown in the recovery of chloroplast redox homeostasis, and the genes of atypical 2-Cys peroxiredoxin (PrxQ), M-type thioredoxin (TRM1), and atypical thioredoxin (ACHT) were significantly upregulated. These processes reduce the production of ROS and ease the cellular damage caused by drought stress. In the metabolome of the Dro vs. Dro_ALA comparison group, the contents of glutathione and ascorbate decreased (Figure S10; Table S27). Changes in the genes and metabolites described above suggest that ALA does alleviate the oxidative stress caused by drought.
3.6 Osmotic regulation under drought stress
OA has often been considered to be a key mechanism of the resistance of plants to drought stress (Serraj and Sinclair, 2002). Proline has long been thought to accumulate in plants that experience water restriction (Verslues and Sharma, 2010). Pyrroline-5-carboxylate synthetase (PRO2, 52.19 to 202.07 FPKM), ornithine aminotransferase (OAT, 15.88 to 60.90 FPKM) and PHR1 transcription factor (PHR1, 3.61 to 13.42 FPKM) involved in the regulation of proline synthesis were significantly upregulated in the Control vs. Dro comparison group (Table S28). Consistent with this result, increased levels of L-proline were detected in the metabolome (Figure 4D; Table S29). As a quaternary ammonium compound, betaine (N, N, N-trimethyl glycine) is also a vital solute involved in osmotic regulation. Betaine aldehyde dehydrogenase (BADH) catalyzes the conversion of betaine aldehyde to betaine (Weretilnyk and Hanson, 1990). BADH4 associated with BADH tended to be upregulated after drought stress, and this corresponded to a significant decrease in the contents of betaine aldehyde and an increase in the content of betaine in the metabolome (Figure 4D; Table S29). The contents of some soluble sugars and sugar alcohol also changed. For example, trehalose, raffinose, and sorbitol were all elevated under water deficiency (Figure 4D; Table S29). A sharp decrease in proline content (Log10 content from 10.08 to 8.86) was detected in the metabolome of Dro vs. Dro_ALA comparison group, which was caused by the downregulation of PRO2 (202.07 to 101.14 FPKM), OAT (60.90 to 33.71 FPKM), and PHR1 (13.42 to 7.64 FPKM). In this study, BADH4 was downregulated, which resulted in an increase in betaine aldehyde, and a decrease in betaine (Figure 4D; Tables S28 and 29). The decrease in the contents of soluble sugars and sugar alcohols also indicated that drought stress tended to be alleviated after the application of ALA. The contents of raffinose, sorbitol, and trehalose all decreased in the Dro vs. Dro_ALA comparison group (Figure 4D; Table S29). These results indicate that the cell homeostasis tends to moderate.
4 Discussion
4.1 ALA uses multiple synergistic mechanisms to alleviate drought stress
Exogenous plant growth regulators are widely used to alleviate drought stress in grapevine. For example, exogenous melatonin can improve the resistance of wine grape ‘Riesling’ seedlings to water deficiency by alleviating PSII damage and protecting the chloroplasts (Meng et al., 2014). The application of exogenous 24-epibrassinolide (EBR) has also been shown to alleviate the inhibition of drought stress on grape photosynthesis by increasing the content of chlorophyll and alleviating stomatal and non-stomatal limitations on photosynthetic performance (Wang et al., 2015). Consistent with this, ALA alleviates the stomatal closure caused by drought and thus, moderates the decrease in photosynthesis to some extent (Figures 3A, B; Table S22). As a precursor of chlorophyll biosynthesis, ALA can directly increase chlorophyll synthesis and inhibit chlorophyll degradation under drought stress (Figure 3D). The inhibition of photosynthetic electron transport chain was also relieved by ALA under drought (Figure 3F). This also impacted the genes related to Rubisco and alleviated photorespiration (Table S24). Exogenous growth regulators can also alleviate the imbalance of grapevine cell homeostasis caused by drought stress. Strigolactones upregulate the antioxidant enzyme genes CAT1 and APX6 to alleviate drought stress in ‘Cabernet Sauvignon’ seedlings (Wang et al., 2021b). The application of ABA increases the contents of proline and soluble sugars and the activities of SOD and POD in ‘Red Globe’ grape. ALA mitigated drought in a manner similar to that of the study described above. In this study, the application of exogenous ALA reduced the contents of MDA and inhibited the production of ROS in ‘SM’ seedlings under drought, activated antioxidant system by upregulating FSD and SODCP and increased the content of α-tocopherol and other non-enzymatic antioxidant scavengers (Figure 4C, D). Therefore, this study provides a novel idea for ALA to alleviate grapevine drought stress.
4.2 Mitigating effects of ALA on grapevine photosynthesis under drought stress
The formation of ALA is a rate-limiting step in chlorophyll biosynthesis (Beale, 1990), and many studies (Kosar et al., 2015; Wang et al., 2018; Rasheed et al., 2020) have shown that ALA enhances the resistance of plants to drought stress by enhancing photosynthesis. For example, pretreatment with ALA increases stomatal conductance and thus, stabilizes photosynthesis in wheat under drought. Rasheed et al. (2020) demonstrated that ALA alleviates the drought stress of sunflower (H. annuus L.) by protecting chlorophyll from degradation. The exogenous application of ALA alleviates drought stress by enhancing the chlorophyll pigments of spring wheat seedlings. However, these studies are based on physiological indicators, and the changes in genes and metabolites during drought were unclear. In our study, ALA treatment significantly increased the stomatal aperture during drought (Figure 3A, B; Table S22). ALA leads to an increase in the level of expression of CYP707A1 under drought, which reduces the content of ABA and leads to further stomatal opening (Figure 3C). In addition, ROS are important signals that regulate stomatal closure (Song et al., 2014), our study shows that the antioxidant system reduces ROS production after ALA application, which may also lead to stomatal reopening, but the specific mechanism is still unclear. GSH plays a role in stomatal movement, it is generally believed that increased GSH content leads to stomatal opening. For example, studies on the negative regulation of glutathione in Arabidopsis thaliana on stomatal closure induced by methyl jasmonate have been reported (Akter et al., 2013). In this study, although we detected stomatal opening caused by ALA, GSH content decreased after application of ALA, which was inconsistent with the above study. We hypothesized that GSH did not play a major role in ROS reduction and stomatal opening after ALA application. Although the inhibition of water lost by transpiration that is limited by the closure of stomata induced by ABA is an important mechanism to improve drought tolerance in plants (Li et al., 2006), a previous study indicated that the closure of stomata induced by ABA does not increase plant sensitivity to drought stress (An et al., 2016). In this study, we hypothesized that during mild drought, the positive effect of stomatal opening and enhancing photosynthesis by ALA was greater than the negative effect of water loss caused by transpiration. Nevertheless, the molecular mechanisms behind the paradox between the prohibition of stomatal opening and an enhancement of drought tolerance merits further study. As for non-stomatal factors, first, after the application of ALA, genes related to chlorophyll synthesis, such as HEMA1, ALAD, PBGD, CPOX, and PPOX, were significantly upregulated, while the levels of expression of CLH, SGR, PPH, and PAO were downregulated, which resulted in the inhibition of chlorophyll degradation (Figure 3D and Table S25). Consistent with the DEGs, the contents of L-glutamate and biliverdin increased, while those of heme and L-threonine decreased. These changes in the genes and metabolites together serve as the basis for ALA to alleviate the loss of chlorophyll in grape leaves under drought (Figures 3D, E and S9). It is worth mentioning that the relation between porphyrin metabolism and the content of L-glutamate and L-threonine is not well followed. Our data support the results that the above metabolites are related to porphyrin and chlorophyll metabolism, the specific mechanisms are still meriting further study. Furthermore, Cai et al. (2020) found that spraying 10 mg/L ALA on the leaves alleviated the reduction in the activities of PSI and PSII reaction centers induced by PEG 6000, electron transport activity, and photosynthetic performance indices in strawberry (Fragaria × annanasa Duch. cv. ‘Benihoppe’). The transcriptomic data showed that ALA upregulated the genes related to PSII and those related to the cytochrome B6/F complex in plants that had been subjected to drought stress (Figure 3F), which was consistent with our conclusions above. Notably, ALA treatment alleviates drought stress by upregulating the levels of expression of AGT1, GDCSP, GDCST and GDCSH, and thus, reducing photorespiration. To our knowledge, this concept has not been mentioned in other papers. Therefore, we hypothesize that ALA enhances photosynthesis and reduces photorespiration by promoting chlorophyll accumulation and alleviating the inhibition of photosynthetic electron transport chain, thus, alleviating drought stress. However, the specific photosynthetic indices related to this effect still merit further study and determination.
4.3 Effects of ALA on grapevine cell homeostasis under drought stress
There is a consensus that drought causes an imbalance in plant cell homeostasis. It has been reported that the foliar application of 3 µM ALA can not only increase the activities of SOD, CAT, GPX, GSH-Px, APX, DHAR, MDHAR, and GR but also increase the contents of AsA and GSH in cucumber (Cucumis sativus L.) under drought (Li et al., 2011). Similarly, we found that treatment with ALA significantly increased the activities of POD and SOD activities in ‘SM’ leaves over time. Furthermore, treatment with ALA upregulated antioxidant enzymes, such as FSD, SODCP, APX, MDAR, DHAR, and GR, under drought (Figure 4C), which could explain the increase in ALA antioxidant enzyme activity from a genetic perspective. In contrast to the results of the study described above, we found that the application of ALA reduced the levels of AsA and GSH in the metabolome (Figures 4A and S10). This could be owing to different results observed when different concentrations of ALA were sprayed in different types of drought mitigation. OA is a fundamental mechanism of drought adaptation in higher plants (Sanders and Arndt, 2012), Ji-Xuan et al. (2017) suggested that 50 mg L-1 of ALA could increase the contents of soluble proteins and the sugars and proline of Chinese ryegrass (Leymus chinensis [Trin.] Tzvel) to alleviate drought stress (Song et al., 2017). In the metabolome, we found that spraying ALA caused a sharp decrease in the contents of proline in grapevine leaves, which was caused by the downregulation of PRO2, OAT, and PHR1. In contrast to that study, the contents of raffinose, sorbitol, and trehalose all decreased after the application of ALA (Figure 4D) and alternatively, they were involved in the alleviation of drought by ALA. Autophagy plays an important role in the resistance of plants to drought stress (Tang and Bassham, 2022). Nevertheless, no study has reported that ALA alleviates drought stress by affecting autophagy. We found that many autophagy-related genes (ARGs) were highly expressed in the transcriptome of control vs. Dro comparison group, only one out of seven key ARGs that were identified were downregulated (Table S28). Among them, ATG11 and ATG2 were DEGs that play an important role in drought response. ATG8F, ATG8I and ATG8C were also expressed at high levels. After ALA treatment, the ARGs detected in the control vs. Dro comparison group were all downregulated (Table S28). Owing to the lack of direct experimental evidence, we anticipate that there will be subsequent studies on whether ALA affects plant drought tolerance by affecting autophagy. Nonetheless, our study interpreted the role of ALA in the maintenance of grapevine cell homeostasis under drought from the perspectives of genes and metabolites, and it was our goal that this study could provide some ideas for future studies on the mitigation of abiotic stress by ALA.
5 Conclusion
In this study, we confirmed that ALA can mitigate drought stress in grapevine. Stomatal movement, the chlorophyll biosynthetic pathway, metabolic pathway, photosynthetic mechanism, and cellular homeostasis constitute the basis of ALA’s regulatory network for alleviating drought stress. First, the application of ALA upregulated the genes related to ABA degradation, thus, inhibiting the stomatal closure induced by ABA, which alleviates the inhibition of drought on photosynthesis to some extent. The current data indicate that the DEGs associated with chlorophyll biosynthesis, such as HEMA1, ALAD, PBGD, CPOX, PPOX, CHLH, CHLD, POR, and DVR, were upregulated after ALA treatment, while the chlorophyll degradation genes CLH, SGR, PPH, and PAO, were downregulated, thus, mitigating the inhibition of chlorophyll accumulation caused by drought. ALA treatment also alleviated the inhibition of photosynthetic electron transport chain by drought. The genes related to Rubisco activity were upregulated by ALA, and photorespiration was attenuated. ALA down-regulated RBOH, thus, reducing the production of ROS and activating the antioxidant system, which changed the contents of antioxidants, such as tocopherol, ascorbate, and glutathione, to reduce the oxidative damage induced by ROS. FSD and SODCP were also restored to high levels of expression after the application of ALA. In addition, the decrease in the contents of betaine, raffinose, sorbitol and trehalose indicated that the degree of drought stress was reduced after the ALA had been applied. Therefore, this study explains the regulatory network of ALA to mitigate drought stress in grapevine (Figure 5) and provides a new concept to study the regulatory network of grapevine drought stress and apply plant growth regulators to alleviate other abiotic stresses.
Data availability statement
The datasets presented in this study can be found in online repositories. The names of the repository/repositories and accession number(s) can be found below: https://www.ncbi.nlm.nih.gov/, PRJNA769649.
Author contributions
YY wrote the original draft of the manuscript, managed and formalized the data. YY, JX, XF and HJ conducted the experiment. YY and YL carried out statistical analysis. JF and SL provided funds. XW, MG, SL, YP and XF polished the manuscript. All authors contributed to the article and approved the submitted version.
Funding
This project was funded by the National Key Research and Development Project [grant number: 2018YFD1000300], Natural Science Foundation of Jiangsu Province [grant number: BK20201321], Jiangsu Agricultural Science and Technology Innovation Fund [grant number: CX(21)2027], National Natural Science Foundation of China [grant number: 31772283], Postdoctoral Research Fund Project of Jiangsu Province [grant number: 2020Z052], the Fundamental Research Funds for the Central Universities [grant number: KYLH201903], Priority Academic Program Development of Jiangsu Higher Education Institutions (PAPD), and President's Foundation of Tarim University [grant number: NMLH201902].
Acknowledgments
We thank the Bioinformatics Center at Nanjing Agricultural University for computational support.
Conflict of interest
The authors declare that the research was conducted in the absence of any commercial or financial relationships that could be construed as a potential conflict of interest.
Publisher’s note
All claims expressed in this article are solely those of the authors and do not necessarily represent those of their affiliated organizations, or those of the publisher, the editors and the reviewers. Any product that may be evaluated in this article, or claim that may be made by its manufacturer, is not guaranteed or endorsed by the publisher.
Supplementary material
The Supplementary Material for this article can be found online at: https://www.frontiersin.org/articles/10.3389/fpls.2023.1129114/full#supplementary-material
References
Akter, N., Okuma, E., Sobahan, M. A., Uraji, M., Munemasa, S., Nakamura, Y., et al. (2013). Negative regulation of methyl jasmonate-induced stomatal closure by glutathione in arabidopsis. J. Plant Growth Regul. 32 (1), 208–215. doi: 10.1007/s00344-012-9291-7
An, Y., Liu, L., Chen, L., Wang, L. (2016). ALA inhibits ABA-induced stomatal closure via reducing H2O2 and Ca2+ levels in guard cells. Front. Plant Sci. 7. doi: 10.3389/fpls.2016.0048
Beale, S. I. (1990). Biosynthesis of the tetrapyrrole pigment precursor, δ-aminolevulinic acid, from glutamate. Plant Physiol. 93 (4), 1273–1279. doi: 10.1104/pp.93.4.1273
Beauchamp, C., Fridovich, I. (1971). Superoxide dismutase: improved assays and an assay applicable to acrylamide gels. Analytical Biochem. 44 (1), 276–287. doi: 10.1016/0003-2697(71)90370-8
Bota, J., Medrano, H., Flexas, J. (2004). Is photosynthesis limited by decreased rubisco activity and RuBP content under progressive water stress? New Phytol. 162 (3), 671–681. doi: 10.1111/j.1469-8137.2004.01056.x
Bray, E. A. (1997). Plant responses to water deficit. Trends Plant Sci. 2 (2), 48–54. doi: 10.1016/S1360-1385(97)82562-9
Buckley, T. N. (2019). How do stomata respond to water status? New Phytol. 224 (1), 21–36. doi: 10.1111/nph.15899
Bylesjö, M., Eriksson, D., Kusano, M., Moritz, T., Trygg, J. (2007). Data integration in plant biology: the O2PLS method for combined modeling of transcript and metabolite data. Plant J. 52 (6), 1181–1191. doi: 10.1111/j.1365-313X.2007.03293.x
Cai, C., He, S., An, Y., Wang, L. (2020). Exogenous 5-aminolevulinic acid improves strawberry tolerance to osmotic stress and its possible mechanisms. Physiologia Plantarum 168 (4), 948–962. doi: 10.1111/ppl.13038
Chaves, M., Zarrouk, O., Francisco, R., Costa, J., Santos, T., Regalado, A., et al. (2010). Grapevine under deficit irrigation: hints from physiological and molecular data. Ann. Bot. 105 (5), 661–676. doi: 10.1093/aob/mcq030
Chen, S., Zhou, Y., Chen, Y., Gu, J. (2018). Fastp: an ultra-fast all-in-one FASTQ preprocessor. Bioinformatics 34 (17), i884–i890. doi: 10.1093/bioinformatics/bty560
Cornic, G., Massacci, A. (1996). Leaf photosynthesis under drought stress. In: Baker, N. R. (eds) Photosynthesis and the environment. Dordrecht: Springer. 5. doi: 10.1007/0-306-48135-9_14
Csardi, G., Nepusz, T. (2006). The igraph software package for complex network research. InterJournal Complex Syst. 1695 (5), 1–9.
Fang, Y., Xiong, L. (2015). General mechanisms of drought response and their application in drought resistance improvement in plants. Cell. Mol. Life Sci. 72 (4), 673–689. doi: 10.1007/s00018-014-1767-0
Fanizza, G., Ricciardi, L. (2015). Influence of drought stress on shoot, leaf growth, leaf water potential, stomatal resistance in wine grape genotypes (Vitis vinifera l.). VITIS-Journal Grapevine Res. 29, 371. doi: 10.5073/vitis.1990.29.special-issue.371-381
Farooq, M., Hussain, M., Wahid, A., Siddique, K. H. M. (2012). Drought Stress in Plants: An Overview. In: Aroca, R. (eds) Plant Responses to Drought Stress. Springer, Berlin, Heidelberg. doi: 10.1007/978-3-642-32653-0_1
Farooq, M., Wahid, A., Kobayashi, N., Fujita, D., Basra, S. M. A., Lichtfouse, E., et al. (2009). “Plant Drought Stress: Effects, Mechanisms and Management,” in Sustainable Agriculture, eds. Lichtfouse, E., Navarrete, M., Debaeke, P., Véronique, S., Alberola, C.. (Dordrecht: Springer Netherlands), 153–188. doi: 10.1007/978-90-481-2666-8_12
Gambetta, G. A., Herrera, J. C., Dayer, S., Feng, Q. S., Hochberg, U., Castellarin, S. D. (2020). The physiology of drought stress in grapevine: towards an integrative definition of drought tolerance (vol 71, pg 4658, 2020). J. Exp. Bot. 71 (18), 5717–5717. doi: 10.1093/jxb/eraa245
Hasanuzzaman, M., Nahar, K., Gill, S. S., Fujita, M. (2013). Drought stress responses in plants, oxidative stress, and antioxidant defense. In Climate Change and Plant Abiotic Stress Tolerance (eds Tuteja, N., Gill, S. S.). 209–250. doi: 10.1002/9783527675265.ch09
Hodgins, R., Van Huystee, R. (1986). Porphyrin metabolism in chill stressed maize (Zea mays l.). J. Plant Physiol. 125 (3-4), 325–336. doi: 10.1016/S0176-1617(86)80154-7
Huang, Y., Liang, D., Xia, H., Lin, L. J., Wang, J., Lv, X. L. (2020). Lignin and quercetin synthesis underlies berry russeting in ‘Sunshine muscat’ grape. Biomolecules 10 (5), 690. doi: 10.3390/biom10050690
Hussain, M., Malik, M. A., Farooq, M., Khan, M. B., Akram, M., Saleem, M. F. (2009). Exogenous glycinebetaine and salicylic acid application improves water relations, allometry and quality of hybrid sunflower under water deficit conditions. J. Agron. Crop Sci. 195 (2), 98–109. doi: 10.1111/j.1439-037X.2008.00354.x
Ilyas, M., Nisar, M., Khan, N., Hazrat, A., Khan, A. H., Hayat, K., et al. (2020). Drought tolerance strategies in plants: A mechanistic approach. J. Plant Growth Regul. 40, 1–19. doi: 10.1007/s00344-020-10174-5
Jaillon, O., Aury, J. M., Noel, B., Policriti, A., Clepet, C., Casagrande, A., et al. (2007). The grapevine genome sequence suggests ancestral hexaploidization in major angiosperm phyla. Nature 449 (7161), 463–467. doi: 10.1038/nature06148
Ji-Xuan, S., Anjum, S., Zong, X., Yan, R., Wang, L., Yang, A., et al. (2017). "Combined foliar application of nutrients and 5-aminolevulinic acid (ALA) improved drought tolerance in leymus chinensis by modulating its morpho-physiological characteristics. Crop Pasture Sci. 68, 474–482. doi: 10.1071/CP16187
Kosar, F., Akram, N., Ashraf, M. (2015). Exogenously-applied 5-aminolevulinic acid modulates some key physiological characteristics and antioxidative defense system in spring wheat (Triticum aestivum l.) seedlings under water stress. South Afr. J. Bot. 96, 71–77. doi: 10.1016/j.sajb.2014.10.015
Laxa, M., Liebthal, M., Telman, W., Chibani, K., Dietz, K.-J. (2019). The role of the plant antioxidant system in drought tolerance. Antioxidants 8 (4), 94. doi: 10.3390/antiox8040094
Li, S., Assmann, S. M., Albert, R. (2006). Predicting essential components of signal transduction networks: a dynamic model of guard cell abscisic acid signaling. PloS Biol. 4 (10), e312. doi: 10.1371/journal.pbio.0040312
Li, D. M., Zhang, J., Sun, W. J., Li, Q., Dai, A. H., Bai, J. G. (2011). 5-aminolevulinic acid pretreatment mitigates drought stress of cucumber leaves through altering antioxidant enzyme activity. Scientia Hortic. 130 (4), 820–828. doi: 10.1016/j.scienta.2011.09.010
Little, K., Metelerkamp, B., Smith, C. (1998). A comparison of three methods of soil water content determination. South Afr. J. Plant Soil 15 (2), 80–89. doi: 10.1080/02571862.1998.10635121
Livak, K. J., Schmittgen, T. D. (2001). Analysis of relative gene expression data using real-time quantitative PCR and the 2– ΔΔCT method. methods 25 (4), 402–408. doi: 10.1006/meth.2001.1262
Lovisolo, C., Lavoie-Lamoureux, A., Tramontini, S., Ferrandino, A. (2016). Grapevine adaptations to water stress: new perspectives about soil/plant interactions. Theor. Exp. Plant Physiol. 28 (1), 53–66. doi: 10.1007/s40626-016-0057-7
Memon, S. A., Hou, X., Wang, L., Li, Y. (2009). Promotive effect of 5-aminolevulinic acid on chlorophyll, antioxidative enzymes and photosynthesis of pakchoi (Brassica campestris ssp. chinensis var. communis tsen et Lee). Acta Physiologiae Plantarum 31 (1), 51. doi: 10.1007/s11738-008-0198-7
Meng, J. F., Xu, T. F., Wang, Z. Z., Fang, Y. L., Xi, Z. M., Zhang, Z. W. (2014). The ameliorative effects of exogenous melatonin on grape cuttings under water-deficient stress: antioxidant metabolites, leaf anatomy, and chloroplast morphology. J. Pineal Res. 57 (2), 200–212. doi: 10.1111/jpi.12159
Moran, J. F., Becana, M., Iturbe-Ormaetxe, I., Frechilla, S., Klucas, R. V., Aparicio-Tejo, P. (1994). Drought induces oxidative stress in pea plants. Planta 194 (3), 346–352. doi: 10.1007/BF00197534
Munné-Bosch, S. (2005). The role of α-tocopherol in plant stress tolerance. J. Plant Physiol. 162 (7), 743–748. doi: 10.1016/j.jplph.2005.04.022
Phung, T.-H., Jung, H.-i., Park, J.-H., Kim, J.-G., Back, K., Jung, S. (2011). Porphyrin biosynthesis control under water stress: sustained porphyrin status correlates with drought tolerance in transgenic rice. Plant Physiol. 157 (4), 1746–1764. doi: 10.1104/pp.111.188276
Pirasteh-Anosheh, H., Saed-Moucheshi, A., Pakniyat, H., Pessarakli, M. (2016). Stomatal responses to drought stress. In Water Stress and Crop Plants, Ahmad, P. (Ed.). doi: 10.1002/9781119054450.ch3
Rasheed, R., Yasmeen, H., Hussain, I., Iqbal, M., Ashraf, M. A., Parveen, A. (2020). Exogenously applied 5-aminolevulinic acid modulates growth, secondary metabolism and oxidative defense in sunflower under water deficit stress. Physiol. Mol. Biol. Plants 26 (3), 489–499. doi: 10.1007/s12298-019-00756-3
Raza, M., Saleem, M., Shah, G., Khan, I., Raza, A. (2014). Exogenous application of glycinebetaine and potassium for improving water relations and grain yield of wheat under drought. J. Soil Sci. Plant Nutr. 14 (2), 348–364. doi: 10.4067/S0718-95162014005000028
Reddy, A. R., Chaitanya, K. V., Vivekanandan, M. (2004). Drought-induced responses of photosynthesis and antioxidant metabolism in higher plants. J. Plant Physiol. 161 (11), 1189–1202. doi: 10.1016/j.jplph.2004.01.013
Saccenti, E., Hoefsloot, H. C. J., Smilde, A. K., Westerhuis, J. A., Hendriks, M. M. W. B. (2014). Reflections on univariate and multivariate analysis of metabolomics data. Metabolomics 10 (3), 361–374. doi: 10.1007/s11306-013-0598-6
Sade, B., Soylu, S., Soylu, E. (2011). Drought and oxidative stress. Afr. J. Biotechnol. 10 (54), 11102–11109. doi: 10.5897/AJB11.1564
Sanders, G. J., Arndt, S. K. (2012). Osmotic Adjustment Under Drought Conditions. In: Aroca, R. (eds) Plant Responses to Drought Stress. Springer, Berlin, Heidelberg. doi: 10.1007/978-3-642-32653-0_8
Serraj, R., Sinclair, T. (2002). Osmolyte accumulation: can it really help increase crop yield under drought conditions? Plant Cell Environ. 25 (2), 333–341. doi: 10.1046/j.1365-3040.2002.00754.x
Song, J.-X., Anjum, S. A., Zong, X.-F., Yan, R., Wang, L., Yang, A.-J., et al. (2017). Combined foliar application of nutrients and 5-aminolevulinic acid (ALA) improved drought tolerance in leymus chinensis by modulating its morpho-physiological characteristics. Crop Pasture Sci. 68 (5), 474–482. doi: 10.1071/CP16187
Song, Y., Miao, Y., Song, C. P. (2014). Behind the scenes: the roles of reactive oxygen species in guard cells. New Phytol. 201 (4), 1121–1140. doi: 10.1111/nph.12565
Talaat, N. B., Shawky, B. T., Ibrahim, A. S. (2015). Alleviation of drought-induced oxidative stress in maize (Zea mays l.) plants by dual application of 24-epibrassinolide and spermine. Environ. Exp. Bot. 113, 47–58. doi: 10.1016/j.envexpbot.2015.01.006
Tang, J., Bassham, D. C. (2022). Autophagy during drought: function, regulation, and potential application. Plant J. 109 (2), 390–401. doi: 10.1111/tpj.15481
Terzi, R., Kadioglu, A. (2006). Drought stress tolerance and the antioxidant enzyme system. Acta Biologica Cracoviensia Ser. Botanica 48, 89–96. doi: 10.1016/j.jep.2005.09.020
Tewari, R. K., Kumar, P., Sharma, P. N., Bisht, S. S. (2002). Modulation of oxidative stress responsive enzymes by excess cobalt. Plant Sci. 162 (3), 381–388. doi: 10.1016/S0168-9452(01)00578-7
Thimm, O., Bläsing, O., Gibon, Y., Nagel, A., Meyer, S., Krüger, P., et al. (2004). MAPMAN: a user-driven tool to display genomics data sets onto diagrams of metabolic pathways and other biological processes. Plant J. 37 (6), 914–939. doi: 10.1038/s41598-022-14606-y
Verslues, P. E., Sharma, S. (2010). Proline metabolism and its implications for plant-environment interaction. Arabidopsis Book/American Soc. Plant Biologists 8, 3. doi: 10.1199/tab.0140
Wang, D., Chen, Q., Chen, W., Guo, Q., Xia, Y., Wang, S., et al. (2021a). Physiological and transcription analyses reveal the regulatory mechanism of melatonin in inducing drought resistance in loquat (Eriobotrya japonica lindl.) seedlings. Environ. Exp. Bot. 181, 104291. doi: 10.1016/j.envexpbot.2020.104291
Wang, W.N., Min, Z., Wu, J.R., Liu, B.C., Xu, X.l., Fang, Y.l., et al. (2021b). Physiological and transcriptomic analysis of Cabernet sauvginon (Vitis vinifera l.) reveals the alleviating effect of exogenous strigolactones on the response of grapevine to drought stress. Plant Physiol. Biochem. 167, 400–409. doi: 10.1016/j.plaphy.2021.08.010
Wang, Y., Wei, S., Wang, J., Su, X., Suo, B., Qin, F., et al. (2018). Exogenous application of 5-aminolevulinic acid on wheat seedlings under drought stress enhances the transcription of psbA and psbD genes and improves photosynthesis. Braz. J. Bot. 41 (2), 275–285. doi: 10.1007/s40415-018-0455-y
Wang, Z., Zheng, P., Meng, J., Xi, Z. (2015). Effect of exogenous 24-epibrassinolide on chlorophyll fluorescence, leaf surface morphology and cellular ultrastructure of grape seedlings (Vitis vinifera L.) under water stress. Acta Physiologiae Plantarum 37, 1729. doi: 10.1007/s11738-014-1729-z
Watanabe, K., Tanaka, T., Hotta, Y., Kuramochi, H., Takeuchi, Y. (2000). Improving salt tolerance of cotton seedlings with 5-aminolevulinic acid. Plant Growth Regul. 32 (1), 97–101. doi: 10.1023/A:1006369404273
Weretilnyk, E. A., Hanson, A. D. (1990). Molecular cloning of a plant betaine-aldehyde dehydrogenase, an enzyme implicated in adaptation to salinity and drought. Proc. Natl. Acad. Sci. 87 (7), 2745–2749. doi: 10.1199/tab.0140
Zargar, S. M., Gupta, N., Nazir, M., Mahajan, R., Malik, F. A., Sofi, N. R., et al. (2017). Impact of drought on photosynthesis: Molecular perspective. Plant Gene 11, 154–159. doi: 10.1016/j.plgene.2017.04.003
Zhang, J., Li, D.-M., Gao, Y., Yu, B., Xia, C.-X., Bai, J.-G. (2012). Pretreatment with 5-aminolevulinic acid mitigates heat stress of cucumber leaves. Biol. Plantarum 56 (4), 780–784. doi: 10.1007/s10535-012-0136-9
Zheng, X., Van Huystee, R. (1992). Peroxidase-regulated elongation of segments from peanut hypocotyls. Plant Sci. 81 (1), 47–56. doi: 10.1016/0168-9452(92)90023-F
Keywords: grapevine, drought stress, ALA, transcriptome, metabolome, alleviation
Citation: Yang Y, Xia J, Fang X, Jia H, Wang X, Lin Y, Liu S, Ge M, Pu Y, Fang J and Shangguan L (2023) Drought stress in ‘Shine Muscat’ grapevine: Consequences and a novel mitigation strategy–5-aminolevulinic acid. Front. Plant Sci. 14:1129114. doi: 10.3389/fpls.2023.1129114
Received: 21 December 2022; Accepted: 24 February 2023;
Published: 15 March 2023.
Edited by:
Guanlin Li, Jiangsu University, ChinaReviewed by:
Juan B. Arellano, Institute of Natural Resources and Agrobiology of Salamanca, Spanish National Research Council (CSIC), SpainDinakaran Elango, Iowa State University, United States
Copyright © 2023 Yang, Xia, Fang, Jia, Wang, Lin, Liu, Ge, Pu, Fang and Shangguan. This is an open-access article distributed under the terms of the Creative Commons Attribution License (CC BY). The use, distribution or reproduction in other forums is permitted, provided the original author(s) and the copyright owner(s) are credited and that the original publication in this journal is cited, in accordance with accepted academic practice. No use, distribution or reproduction is permitted which does not comply with these terms.
*Correspondence: Jinggui Fang, MjAxMzE1MUBuamF1LmVkdS5jbg==; Lingfei Shangguan, c2hhbmdndWFubGZAbmphdS5lZHUuY24=
†These authors have contributed equally to this work