- 1Department of Biotechnology and Crop Improvement, Kittur Rani Chennamma College of Horticulture, Arabhavi, University of Horticultural Sciences, Bagalkot, Karnataka, India
- 2Center for Ayurveda Biology and Holistic Nutrition, The University of Trans-Disciplinary Health Sciences and Technology (TDU), Bengaluru, Karnataka, India
- 3Amity Institute of Genome Engineering, Amity University Uttar Pradesh, Noida, India
The tropical tree, D. binectariferum, is a prominent source of chromone alkaloid rohitukine, which is used in the semi-syntheses of anticancer molecules such as flavopiridol and P-276-00. The biosynthetic pathway of rohitukine or its derivatives is currently unknown in plants. Here, we explored chromone alkaloid biosynthesis in D. binectariferum through targeted transcriptome sequencing. Illumina sequencing of leaves and roots of a year-old D. binectariferum seedling generated, 42.43 and 38.74 million paired-end short reads, respectively. Quality filtering and de novo assembly of the transcriptome generated 274,970 contigs and 126,788 unigenes with an N50 contig length of 1560 bp. The assembly generated 117,619 translated unigene protein sequences and 51,598 non-redundant sequences. Nearly 80% of these non-redundant sequences were annotated to publicly available protein and nucleotide databases, suggesting the completeness and effectiveness of the transcriptome assembly. Using the assembly, we identified a chalcone synthase (CHS) and three type III polyketide synthases (PKS-III; non-CHS type) that are likely to be involved in the biosynthesis of chromone ring/noreugenin moiety of rohitukine. We also identified key enzymes like lysine decarboxylase in the piperidine pathway that make the piperidine moiety of rohitukine. Besides these, the upstream enzymes in flavonoid biosynthesis like phenylalanine ammonia-lyase (PAL), trans-cinnamate 4-hydroxylase (C4H),4-coumarate-CoA ligase (4CL), and chalcone isomerase (CHI) have also been identified. Also, terpene synthases that are likely to be involved in the biosynthesis of various terpenoid scaffolds have been identified. Together, the D. binectariferum transcriptome resource forms a basis for further exploration of biosynthetic pathways of these valuable compounds through functional validation of the candidate genes and metabolic engineering in heterologous hosts. Additionally, the transcriptome dataset generated will serve as an important resource for research on functional genomics and enzyme discovery in D. binectariferum and comparative analysis with other Meliaceae family members.
1 Introduction
Rohitukine, a prominent chromone alkaloid currently known to occur in five plant species belonging to Meliaceae and Rubiaceae families (Khadem and Marles, 2012; Varun et al., 2023). Rohitukine is a unique chromone alkaloid having a noreugenin chromone scaffold conjugated to a ring containing one or more nitrogen atoms (Houghton, 2002; Mohanakumara et al., 2010; Mohana Kumara, 2012). Flavopiridol (Sanofi) and P-276-00 (Piramal) two semi-synthetic derivatives of rohitukine are in the advanced stages of clinical trials for various cancer treatments (Jain et al., 2012). Flavopiridol (alvocidib; L868275; HMR-1275; NSC 649890 of Sanofi-Aventis + NCI) is an established cyclin-dependent kinases (CDK) inhibitor with broad specificity to CDK1, CDK2, and CDK4 leading to cell cycle arrest at both G1 and G2 phases (Sedlacek et al., 1996; Stadler et al., 2000; Łukasik et al., 2021). Flavopiridol is also a promising agent in inducing p53-independent apoptosis in Chronic Lymphocytic Leukaemia (CLL) and therefore this has been approved as an orphan drug for treating CLL (Christian et al., 2009; Albert et al., 2014; Mandal et al., 2021). Whereas P-276-00 is currently in phase II clinical studies for advanced refractory neoplasms and multiple myeloma (Christian et al., 2009; Borowczak et al., 2022). In addition to cancer, flavopiridol has also been shown to be effective in the treatment of arthritis and atherosclerotic plaque formation (Sekine et al., 2008; Chen et al., 2021).
Rohitukine was first reported in Amoora rohituka and later in Dysoxylum binectariferum, Dysoxylum acutangulum (Meliaceae), Schumanniophyton magnificum and S. problematicum (Rubiaceae) (Harmon et al., 1979; Naik et al., 1988; Ismail et al., 2009; Mohanakumara et al., 2010). Among these species, D. binectariferum accumulates the highest amount of rohitukine in stem bark (3-7% by dry weight). Whereas the closest relative of D. binectariferum, D. malabaricum does not accumulate rohitukine (Houghton, 2002; Mohanakumara et al., 2010). Also, various rohitukine derivatives such as dysoline, schumaniofioside A and chrotacumines have been reported from D. binectariferum (Ismail et al., 2009; Izwan Mohd Lazim et al., 2013; Morita et al., 2014; Mohana Kumara et al., 2016). Besides plants, endophytic fungi associated with A. rohituka and D. binectariferum have also been shown to produce rohitukine in culture (Mohana Kumara, 2012; Mohana Kumara et al., 2012; Kumara et al., 2014). But the biosynthetic pathway of chromone alkaloids in general has not been elucidated so far (Abe et al., 2005; Morita et al., 2007; Izwan Mohd Lazim et al., 2013). Earlier, desorption electrospray ionization mass spectrometry imaging (DESI-MSI) shows that rohitukine in germinating seedlings is largely restricted to the cotyledonary tissue, followed by the embryo and the seed coat (Mohana Kumara et al., 2015; Mohana Kumara et al., 2016; Varun et al., 2023). Within seedlings, rohitukine was predominantly distributed in the roots, collar region of the stem, and young leaves. In the stem and roots, rohitukine was primarily restricted to the cortex region (Mohana Kumara et al., 2016). DESI-MSI and electrospray ionization (ESI) tandem mass spectrometry (MS/MS) analysis revealed the presence of oxidized, acetylated glycosylated, and methylated derivatives of rohitukine (Mohana Kumara et al., 2015; Mohana Kumara et al., 2016). In addition to chromone alkaloids, Dysoxylum is also known to contain as many as 279 triterpenoids belonging to different scaffolds like dammarane, nortriterpenoid, oleanane, lupane, tirucallane, lanostane, cycloartane, glabretal and cyclopropane types (Yan et al., 2021; Naini et al., 2022). With recent advancements in sequencing technologies like genome and transcriptome sequencing of medicinal plants has become an important tool in understanding the biosynthetic pathway of metabolites of therapeutic relevance. For example, the genomes and transcriptomes of medicinal plants such as Asparagus racemosus, Curcuma longa, Polygonum cuspidatum, Ocimum spp., and Azadirachta indica have helped in establishing the different metabolic pathways (Narnoliya et al., 2014; Rajakani et al., 2014; Krishnan et al., 2016; Pandreka et al., 2021; Joudaki et al., 2023). These sequence resources form a base for further elucidation and functional characterization of the constituent metabolic pathways facilitating metabolic engineering in heterologous systems (Ma et al., 2021; Hu et al., 2023; Kwan et al., 2023). In the current study, we report the de novo transcriptome sequencing, assembly of the leaf and root tissues of D. binectariferum and annotation of genes in specialized metabolic pathways including chromones, alkaloids, flavonoids, sesquiterpenes and triterpene pathways. We also report differentially expressed genes in leaf and root tissues and study their tissue-specific gene expression. Finally, we identified potential genes involved in the above biosynthetic pathways and showed relative expression of their transcripts in leaves and roots.
2 Materials and methods
2.1 Plant material
D. binectariferum was identified, collected and the voucher specimen was deposited at The University of Transdisciplinary Health Sciences and Technology herbarium, Bangalore (voucher specimen number; 122951-55). During the fruiting season D. binectariferum seeds were collected from Jog, Central Western Ghats, India (140 13’ 65” N and 740 48’ 35” E). Seeds were sown in polybags and seedlings were kept under shade with continuous watering and maintained in a nursery at the University of Transdisciplinary Health Sciences and Technology, Bengaluru. The leaves and roots of one-year-old seedlings of similar age and size were used in transcriptome sequencing and metabolite analysis (Figure 1A). The sampling was non-invasive with no impact on the natural growth or regeneration of D. binectariferum populations in the wild. And the study was conducted following relevant national and institutional guidelines.
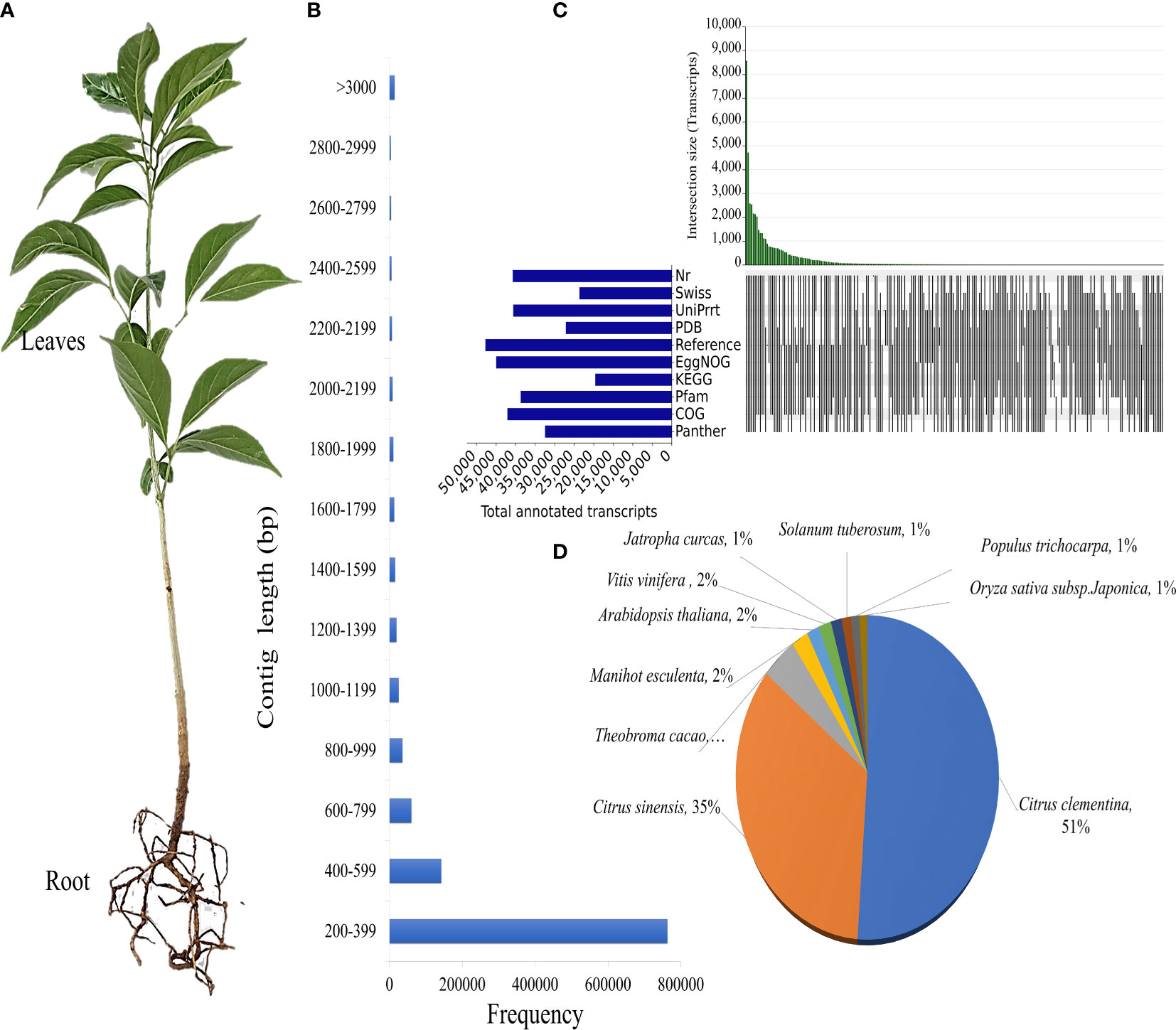
Figure 1 Annotation of D. binectariferum seedling transcriptome. (A) One-year-old D. binectariferum seedling used in transcriptome sequencing and metabolite analysis. (B) The contig’s length distribution of de novo assembled transcripts. (C) Upset plot showing the number of assembled unigenes, annotated to Nr, Swiss, TrEMBL/UniProt, PDB, Reference sequence, EggNOG, KEGG and Pfam databases, (D) Distribution of top hits (species) for the non-redundant unigenes identified in the transcriptome.
2.2 RNA isolation from D. binectariferum and differential expression analysis
Total RNA was isolated from the root and leaf tissues of one-year-old D. binectariferum seedlings using TRIZOL reagent (Sigma Life Science, USA) (Meng and Feldman, 2010). Each sample included three biological replicates. The quantity and quality of total RNA was determined by NanoDdrop (Thermo Scientific) and agarose gel electrophoresis. The purity of total RNA was estimated using the absorbance ratio at 260/280 and 260/230, and the RNA integrity number (RIN). Samples showing acceptable RNA integrity numbers above 7 were used in library preparation. Sequencing was done from both 5’ and 3’ ends on the Illumina platform (NovaSeq 6000) according to the manufacturer’s instructions (Illumina Inc., San Diego, CA, USA).
About 500ng of total RNA was used in first-strand cDNA synthesis using the Takara cDNA synthesis kit according to the manufacturer’s instructions. qRT PCR analysis was carried out in triplicates using SYBR Green Universal Master Mix (Takara) in 98-well optical plates using Applied Biosystems, Quantum studio 3 Real-time qPCR system. Each (10μl) reaction contained a 10ng (2μL) cDNA template, 0.4µl of 5 pM each primer, and 5μl SYBR Green mix. Cycling conditions were as follows: 1 cycle of 50°C for 2 min, 95°C for 3 min, 40 cycles of 95°C for 10 sec, 55°C for 30 sec and 1 cycle of 95°C for 15 sec, 55°C for 1 min and 95°C for 15 sec. The EF2 gene (elongation factor 2) was used as a normalization control, and all samples were analysed in triplicates, and a dissociation curve validated the specificity of each primer pair (Xu et al., 2011; Moraes et al., 2015; Linardić and Braybrook, 2021; Xu et al., 2023). Relative quantification for levels of transcripts between the samples was calculated using 2−ΔΔCT method.
2.3 De novo transcriptome assembly
D. binectariderum roots and leaves (three biological replicates) were sequenced. The raw data obtained was quality checked by trimming and removing adaptor sequences and other low-quality sequences using the FastQC tool (http://www.bioinformatics.babraham.ac.uk/projects/). The raw reads were also processed using Trimmomatic v0.38.2 to remove low-quality reads using default parameters (Bolger et al., 2014). The clean reads were assembled using Trinity Version 2.9.1(Grabherr et al., 2011) with default parameters. The level of completeness of the final transcript assembly was evaluated using BUSCO v 5.4.4 tool (Seppey et al., 2019). Coding regions of the assembled transcripts were predicted using Transdecoder Version 5.5.0 (Haas et al., 2013). We removed redundant sequences, identified non-redundant or representative protein sequences using CD-HIT version 1.2, and retained the longest sequence with a minimum sequence identity threshold of 0.9 contigs in each cluster (Fu et al., 2012). Non-redundant or representative sequences (>200 amino acids cut off) were annotated based on sequence similarity using blastp against the following databases; NCBI non-redundant (Nr), Swiss-Prot 2018, TrEMBL/UniProt, Protein database, Reference sequence database with e value 10− 3 (NCBI BLAST+ blastp Galaxy Version 2.10.1+galaxy2; Christiam Camacho et al., 2009; Peter et al., 2015), HMMER/Protein family (Pfam) v3.3.2 (https://www.ebi.ac.uk/Tools/hmmer/), EggNOG (http://eggnog5.embl.de/#/app/home), Clusters of Orthologous Groups of proteins (COGs), Gene Ontology (GO)(http://www.pantherdb.org/), and Kyoto Encyclopaedia of Genes and Genomes (KEGG) (https://www.kegg.jp/) (Figure S1).
2.4 Differential expression analysis
Global differentially expressed genes (DEGs) analysis between root and leaf samples was performed using DESeq2 (ver 2.11.40.7) tool with the Benjamini-Hochberg procedure (Love et al., 2014). The expression levels were calculated and normalized using TPM methods (Lin et al., 2016; Patro et al., 2017). DEGs were identified with adjusted FDR ≤ 0.05 (false discovery rate), log2 (fold change) of > 2 and FPKM value of >11. An online enrichment tool, ShinyGO v0.75, was used to identify different KEGG pathways enriched in DEGs (http://bioinformatics.sdstate.edu/go75/). The significance of KEGG terms was determined by a p-value or q-value of ≤0.05. Further investigation was performed with selected DEGs. Within this list, we concentrated on genes associated with 17 different metabolic pathways comprising terpenoids, flavonoids, piperidine, and chromone alkaloids etc.
2.5 Extraction of rohitukine and quantification
Metabolites were extracted from the leaf and root tissues of D. binectariferum using methods described earlier (Mohanakumara et al., 2010). Briefly, the freeze-dried samples were ground to a fine powder. Extraction was carried out using methanol (10 mL). The extracts were vortexed, sonicated (30 min) and centrifuged (8,000 rpm for 10 min). Next, extracts were passed through membrane filters (0.2 µm) and kept in airtight vials at -20°C until further use. Samples were analyzed using reverse-phase HPLC (Shimadzu, LC20AT, Japan), RP-18 column (4.6 x 250 mm, 5μm) with UV absorbance at 254 nm. The standard rohitukine was prepared with a series of concentrations (0.2 – 1.0 mg/ml) using liquid chromatography-mass spectrometry (LC-MS) grade methanol and filtered using 0.2µ syringe filters. Acetonitrile and 0.1% TFA were used in gradient mode as the mobile phase. The linear graph obtained (y = mx) was used in quantification of rohitukine in samples (R2 = 0.99) (Mohana Kumara et al., 2016). The significance of rohitukine content in the leaf and root of D. binectariferum was tested using t-tests (unpaired), F-tests, and Kruskal-Wallis tests using Past 4.11 (Hammer et al., 2001).
2.6 GC-MS analysis
Leaf and root tissues of D. binectariferum were also subjected to volatile analysis using GC-MS (Sharma et al., 2021). Leaf and root samples were dried at 40 °C for 8-12 hrs in a hot air oven and 1.0 ± 0.01 g of fine powder was subjected to headspace analysis using GC-MS fitted with RTx-volatiles capillary column (3.0 m × 0.25 mm × 0.25 μm). The analysis was done using a Shimadzu® - Nexis GCMS 2030 coupled to a mass spectrometer with a triple quadrupole TQ8040NX, equipped with an HS-20 auto-sampler (Shimadzu, Tokyo, Japan). The following GC temperature program was used; the column oven temperature was maintained at 80°C for 1 min., followed by two heating ramps of 5 and 10°C/min. until reaching temperatures of 150 °C and 200 °C, respectively. Mass spectra were obtained using electron impact at 70 eV and a start and end mass-to-charge ratio (m/z) of 30 and 500, respectively. The compounds were identified by comparison to the mass spectra from library databases (NIST 98; http://www.nist.gov) and by calculating Kovat’s indices using alkane standards (C8-C24) RT values.
3 Results and discussion
3.1 De novo assembly
The D. binectariferum transcriptome generated 42.43 and 38.74 million paired-end short reads (150 bp) for leaves and roots, respectively. Filtering for quality resulted in 41.52 (97.85%) and 38.09 (98.33%) million clean reads for the leaf and roots, respectively (Table 1). De novo assembly of the short reads generated 274,970 contigs and 126,788 unigenes from the whole transcriptome with an N50 length of 1,560 bp (Table 2). The average GC content of the contigs derived from the transcriptome was 42.6% (Table S1). Of the 2,326 BUSCOs in the Eudicots dataset, 2,142 (92.1%) complete BUSCOs were detected in the assembly (Table S2). The results indicated that the assembly was almost complete with an adequate representation of the gene directory. From the assembly, 67.67% of the contigs (763,242) were 200-400 bp in length, 21.0% (237,930) were 400-1,000 bp, 7.43% (83,771) were 1,000-2,000 bp, 2.55% (28,784) were 2,000-3,000 bp, and only 1.26% (14,233) exceeded 3,000 bp (Figure 1B). Using the CD-HIT tool (>200 amino acids cut off), we identified 51,598 nonredundant protein sequences from a total of 117,619 translated unigenes/protein sequences. After removing the redundancy, De novo assembly generated short reads of 117,619 contigs and 51,340 unigenes from the whole transcriptome with an N50 length of 1,176bp. The average GC content of the contigs derived from the transcriptome was 46.89% (Table S1) (Table 2).
3.2 Functional annotation
To identify putative protein functions, all the assembled unigenes were annotated using the Basic Local Alignment Search Tool (BLAST) against ten publicly available protein databases. Out of 51,598 unigenes, 40,699 (78.88%) were annotated to Nr, 23,618 (45.77%) to Swiss-Prot, 40,552 (78.59%) to TrEMBL/UniProt, 27,101 (52.52%) to Protein database, 47,675 (92.4%) to a Reference sequence database, 44,919 (87.06%) to EggNOG, 19548 (37.89%) to KEGG, 38,297 (74.22%) to Pfam, 42,040 (81.48%) to COG and 36,530 (70.8%) to Panther GO (Figure 1C; Additional File 1). Citrus clementina (7,174), Citrus sinensis (4,837), Theobroma cacao (621), Manihot esculenta (296), and A. thaliana (224) contributed to the most gene annotations (Figure 1D). Two Citrus species were the major hits because both Citrus and D. binectariferum are related phylogenetically as well as encode shared metabolic pathways (Levitsky and Dembitsky, 2014; Bhambhani et al., 2017; Du et al., 2021; Hou et al., 2022).
Further, Nr unigenes were annotated to three major ontologies (GO): molecular function (MF), biological process (BP), and cellular component (CC). BP comprises 41.63% of the total assigned annotations, whereas CC and MF comprised 27.63% and 30.74% respectively. Among biological processes, the unigenes are predominantly annotated to the cellular process (32.6%) and metabolic process (28.1%), followed by biological regulation (7.4%). Similarly, for the CC category, the largest number of unigenes were assigned to cellular anatomical entities (45.2%) and protein-containing complexes (7.4%). While, in the MF category, the catalytic activity (31.1%), and binding (15.6%) were the most annotated (Figure 2A). A total of 42,040 transcripts were assigned to 25 COG classifications with the majority in the category “function unknown” (10,854, 21.04%), followed by “post-translational modification, protein turnover, chaperone functions” (3,351, 6.49%), “transcription” (2,928, 5.67%) and “carbohydrate metabolism and transport” (2,237, 4.34%) (Figure 2B).
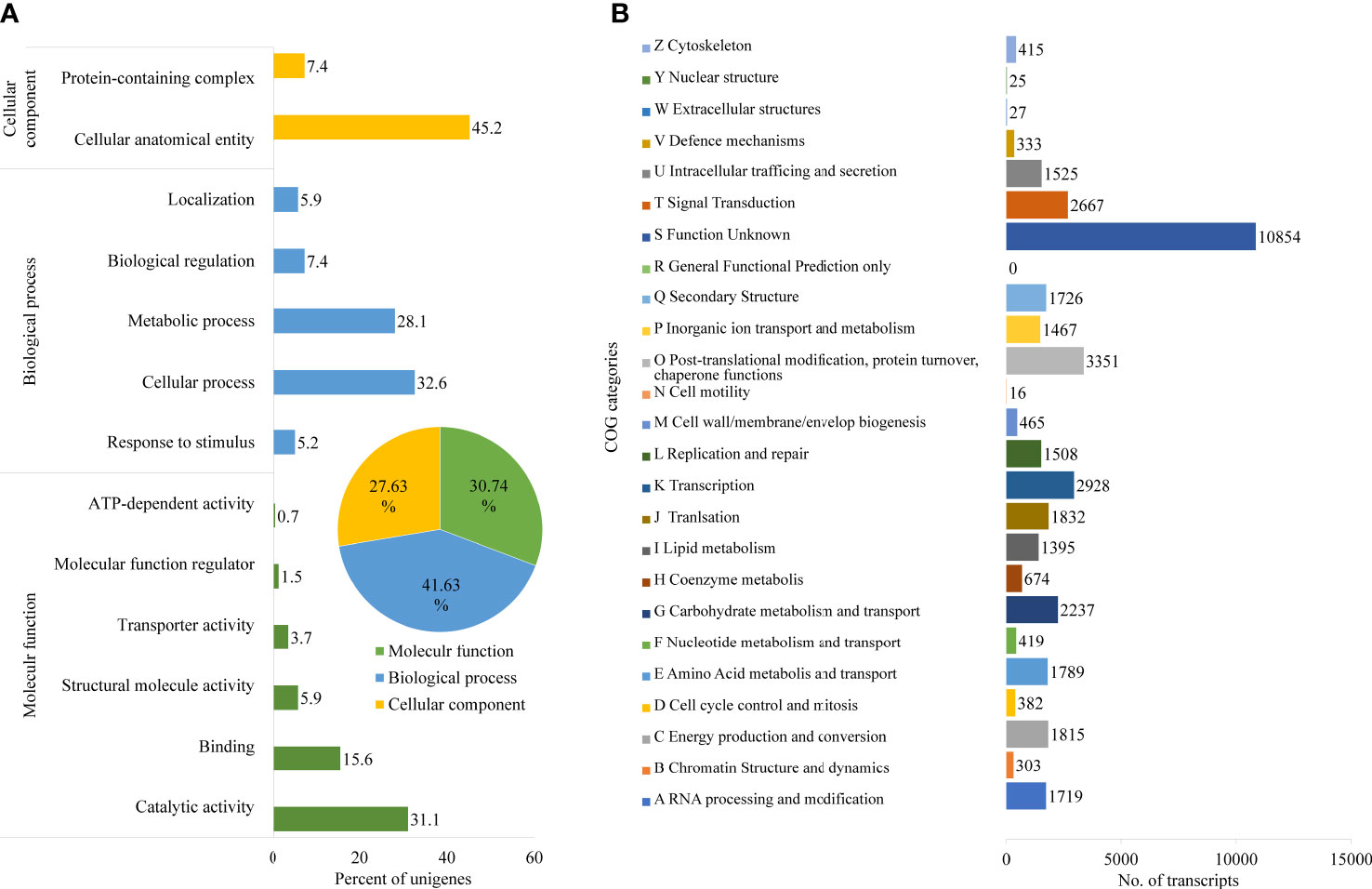
Figure 2 Functional annotation of D. binectariferum transcriptome based on Gene Ontology (GO). (A) GO functional classifications of assembled D. binectariferum unigenes. Insert in pie chart showing percentage of annotation to three different classes of gene ontology. (B) Clusters of Orthologous Groups (COG) functional classifications of assembled D. binectariferum unigenes and associated number of transcripts with COG function categories.
The KEGG classifications for the assembled unigenes were used to evaluate the completeness of the transcriptome library as well as the effectiveness of the annotation process for identifying the specialized metabolic pathways. A total of 19,548 assembled unigenes were assigned into six main functional categories (Metabolism, Genetic Information Processing, Environmental Information Processing, Cellular Processes, Organismal Systems, and Human Diseases) and 46 subcategories (Figure 3A) and 431 KEGG pathways. The two most abundant sub-categories were “metabolism” and “human diseases”, covering 57.47% and 23.14% of the total annotations, respectively. The rest were covered by the remaining categories of Genetic Information Processing (7.28%), Environmental Information Processing (6.22%), Cellular Processes (6.39%) and Organismal Systems (10.13%). Furthermore, the unigenes coding for specialized metabolite biosynthesis were analyzed. The 17 major specialized metabolic pathways were selected and their respective KO and unigene counts are shown in Figure 3B and Additional File 2). Of these, 213 unigenes were assigned to “Phenylpropanoid biosynthesis”, followed by 124 unigenes for “Terpenoid backbone biosynthesis”, 102 for steroid biosynthesis, and others. These annotations form a basis for the functional characterization of genes involved in the specialized metabolism and regulation of D. binectariferum (Kanehisa and Goto, 2000; Liu et al., 2013; Bhambhani et al., 2017).
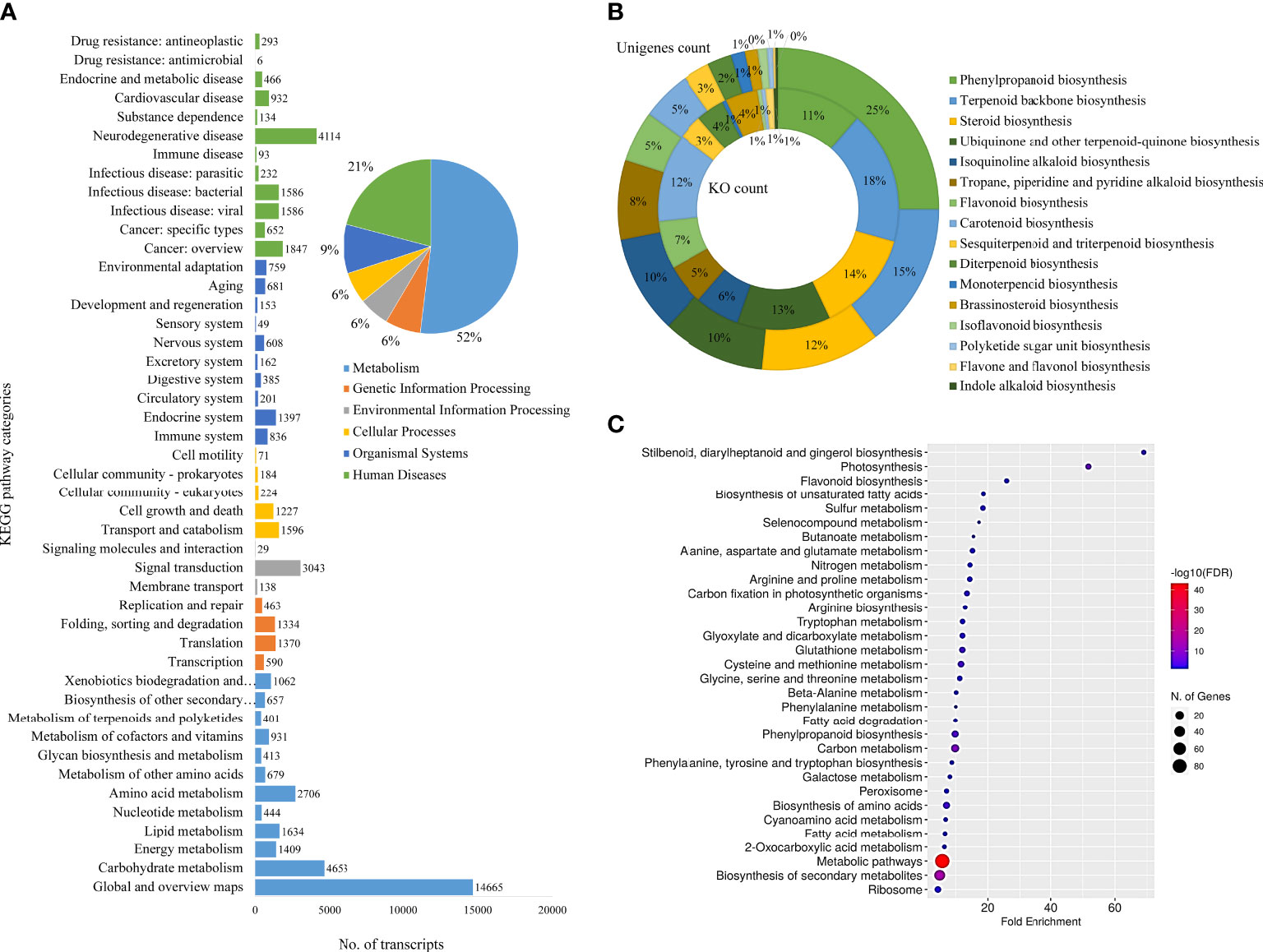
Figure 3 Functional annotation of D. binectariferum transcriptome based on KEGG. (A) Assembled D. binectariferum unigenes annotated to the functional classification of the Kyoto Encyclopedia of Genes and Genomes (KEGG) database and their associated number of transcripts are shown for each of the KEGG functional category. (B) Pie chart showing the selected KEGG pathways related to specialized metabolic pathways and the inner ring represents the KO category while the outer ring represents its respective unigenes count, (C) KEGG pathway enrichment from final annotated transcripts of D. binectariferum. The vertical axis represents the pathway’s name, and the horizontal axis represents the fold enrichment. The size and color of bubbles indicate the number and degree of enrichment of different metabolites, respectively.
3.3 Metabolic pathway analysis
We identified 6,495 DEGs in total, including 3,532 genes that were upregulated in roots and 2,963 genes that were downregulated in leaves. Further, unigenes related to 17 different specialized metabolic pathways were analyzed for their expression levels (log2fold) in roots and leaves. Of the 736 DEGs that were found to be involved in specialized metabolism, 284 of them were upregulated and 452 of them were downregulated in the root compared to the leaf (Additional file 3 and 4). Based on KEGG pathway enrichment of the bubble diagram, carbon metabolism, stilbenoid, flavonoid, unsaturated fatty acids, and phenylpropanoid biosynthesis were the most dominant pathways, and the majority of KEGG-identified genes were associated with metabolic pathways and secondary metabolite biosynthesis (Figure 3C).
3.3.1 Identification of unigenes involved in the terpenoid pathway
Terpenoids comprise the largest group of structurally diverse natural compounds and are known to be biosynthesized via two biosynthetic routes; the 2-C-methyl-D-erythritol 4-phosphate (MEP) pathway and the mevalonic acid (MVA) pathway (Sandeep and Ghosh, 2020). The isoprene unit (C5) is synthesized from the MEP pathway and is engaged in the formation of mono-(C10), Di-(C20) and other terpenoids. Whereas the isoprene unit from the MVA pathway is used in the synthesis of triterpene (C30) and sesquiterpenes (C15) (Schilmiller et al., 2009; Zhao et al., 2013; Ghissing et al., 2023). In the D. binectariferum transcriptome, around 269 unigenes (70 key enzymes) were found to be associated with the terpenoid pathways (Figure 4; Figure S2). Of these, we identified 48 unigenes encoding 6 key enzymes in the mevalonate pathway (MVA) and 29 unigenes encoding 8 key enzymes in the MEP pathway leading to the formation of isopentenyl diphosphate (IPP) and dimethylallyl diphosphate (DMAPP) (Kuzuyama, 2002). IPP and DMAPP go through a series of condensation reactions by prenyl diphosphate synthases to form prenyl diphosphates like geranyl diphosphate (GPP; C10), farnesyl diphosphate (FPP; C15), geranylgeranyl diphosphate (GGPP; C20) and other diphosphates. These prenyl diphosphate precursors form a basis for the biosynthesis of a diverse classes of terpenoids currently observed in plants (Figure 4; Figure S2) (Lorigooini et al., 2020). The formation of FPP from IPP by the enzyme short-chain Z-isoprenyl diphosphate synthase (K12503, 1 unigene) leads to triterpenoid synthesis via squalene. The squalene is oxidized to form 2, 3 oxidosqualene by squalene monooxygenase (SQLE, 9 unigenes) and 2, 3 oxidosqualene is the central precursor in biosynthesis of diverse triterpenoids including dammarane, cabraleadiol, 3-epiocotillol, methyl shoreate, and others (Yan et al., 2014; Fan et al., 2021). The 2, 3 oxidosqualene also leads to the formation of sterols (phyto- and ergosterols) involved in sitosterol and phytosterols biosynthesis in plants. Farnesyl diphosphate synthase (FDPS, 4 unigenes) accepts both DMAPP and IPP from MEP and MVA pathways in the mono and sesquiterpenoids (Szkopińska and Płochocka, 2005). We analyzed the volatile components of the leaf and root tissues of D. binectariferum using GC-MS to identify the possible precursors of the terpenoids. The leaf contained 14% monoterpenes, 6.5% sesquiterpenes, 1.5% ketones, and 1.27% cycloalkanes, while the root contained 49.75% sesquiterpenes, 8.86% sesquiterpenoids, 0.2% aldehydes, and 0.11% ketones (Table S3). The farnesyl diphosphate synthase (FDPS, 4 unigene) is involved in the synthesis of the sesquiterpenoids. The enzyme (-)-germacrene D synthase (GERD, 6 unigenes) makes germacren-type sesquiterpenes. In GC-MS we detected several sesquiterpenenes including α- and β-cadinenes, α- and β-copaene α-ylangene, caryophyllene, alloaromadendrene, α-guaiene, and germacrene-D in leaves and α-muurolene in the root. Additionally, monoterpene (+)-4-carene was also detected in D. binectariferum leaves (Table S3; Figure S3). All these sesquiterpenes detected in GC-MS analysis were mapped to different terpenoid biosynthetic routes (Figure 4; Figure S3). The transcriptome showed 12 unigenes involved in monoterpenoid biosynthesis and 21 unigenes encoding 7 enzymes in gibberellin biosynthesis (2 beta-dioxygenase GA2ox, 7 unigenes, ent-kaurenoic acid monooxygenase KAO, 4 unigenes) (Figures 3B, 4). In total, the MEP pathway predominates in the roots and MVA pathway in the leaf which contributes to the generation of many volatile molecules (Inaba and Ito-Inaba, 2010; Tomar et al., 2013; Pérez et al., 2022) and they play key roles in the biosynthesis of various defensive compounds (Yan et al., 2021; Naini et al., 2022; Ghissing et al., 2023).
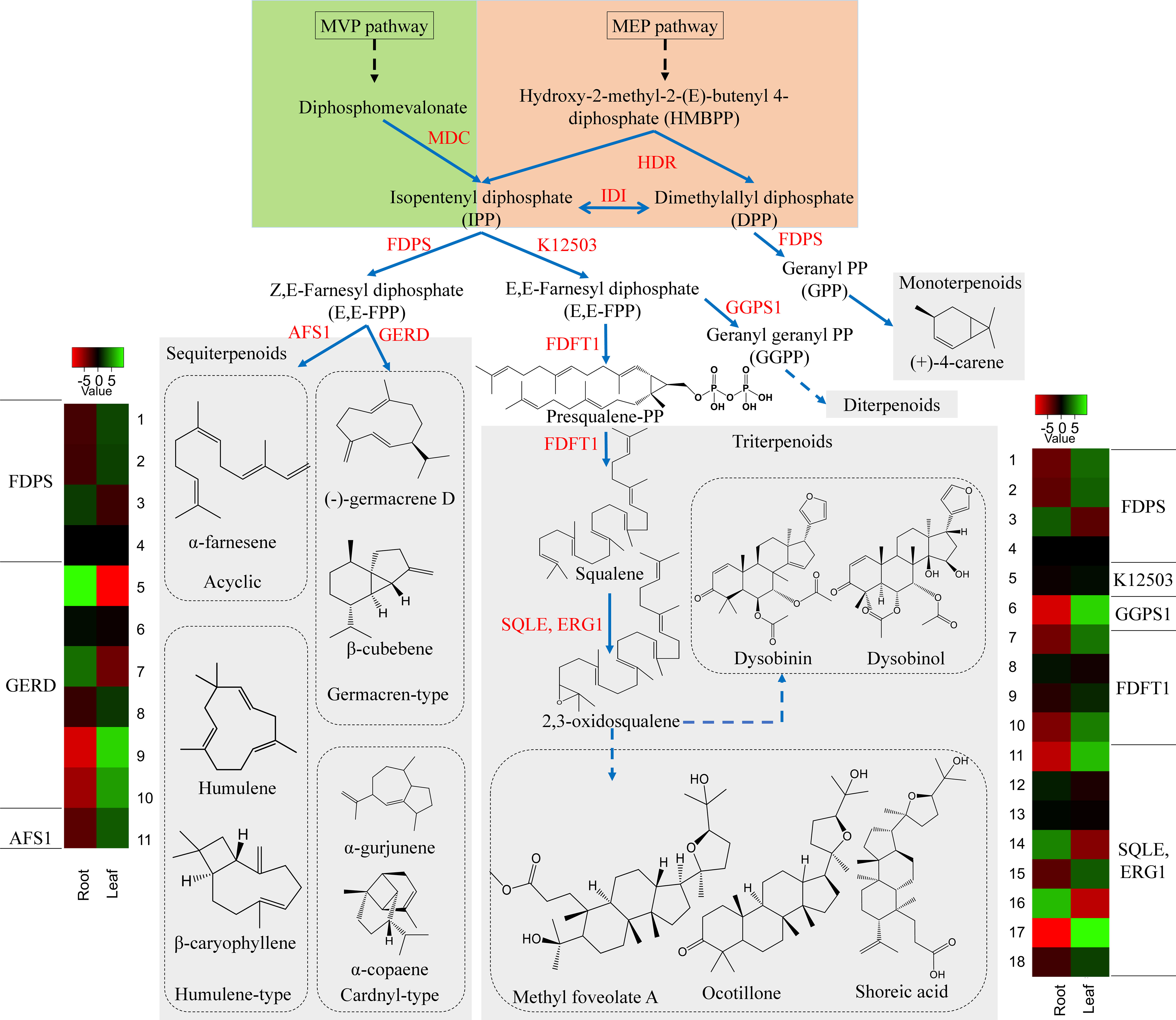
Figure 4 D. binectariferum candidates involved in the biosynthetic pathways of terpenoids. Key biosynthetic enzymes (red)) identified from the D. binectariferum transcriptome are shown at each step. Heat-map displaying differential expression of unigene transcripts (enzymes) of D. binectariferum transcriptome are shown for roots and leaves. Details of numbers labelled in the Heat-map are provided in the additional file 3. MDC-Mevalonate pyrophosphate decarboxylase; HDR- 4-Hydroxy-3-methylbut-2-enyl diphosphate reductase (HDR)/HMBPP reductase; IDI- Isopentenyl diphosphate isomerase; FDPS- Farnesyl diphosphate synthase; FDPS-Farnesyl diphosphate synthase; K12503: short-chain Z-isoprenyl diphosphate synthase; AFS 1-α-farnesene synthase; GERD- (-)-Germacrene D synthase; GGPS1-Geranylgeranyl pyrophosphate synthase1; SQLE/ERG1- Squalene epoxidase/Squalene monooxygenase.
3.3.2 Identification of unigenes involved in the flavonoid pathway
In flavonoid biosynthesis, twelve key enzymes are involved in the conversion of p-coumaroyl CoA to naringenin, and D. binectariferum revealed 44 unigenes to be associated with all the 12 key enzymes (Figure 3B). Chalcone synthase (CHS, 6 unigenes) is the first key enzyme in flavonoid biosynthesis that converts 4-coumaroyl CoA to naringenin chalcone. Then the isomerization of naringenin chalcone to noreugenin is catalyzed by the enzyme chalcone isomerase (CHI, 2 unigenes). Noreugenin forms a central precursor from which all other flavonoids are derived (Liu et al., 2013). The naringenin is converted to dihydrokaempferol by an enzyme naringenin 3-dioxygenase (F3H, 1 unigene) and dihydrokaempfero is further converted to leucopelargonidin by a bifunctional enzyme dihydroflavonol 4-reductase/flavanone 4-reductase (DFR, 3 unigenes). Leucopelargonidin is a key molecule, where (+)-Afzelechin and pelargonidin are formed by the enzymes leucoanthocyanidin reductase (LAR, 4 unigenes) and anthocyanidin synthase (ANS, 1 unigene) respectively, and also converted back to the dihydrokaempferol by ANS (Zhao et al., 2017; Wang et al., 2021). Further, pelargonidin is reduced to form (-)-Epiafzelechin by an enzyme anthocyanidin reductase (ANR, 1 unigene) (Figure 5). The genes that encode these enzymes, PAL (3 unigenes), C4H (5 unigene), CHS (6 unigenes), CHI (2 unigene) and 4CL (22 unigenes) were found to be significantly upregulated in roots compared to leaves (Figure S4).
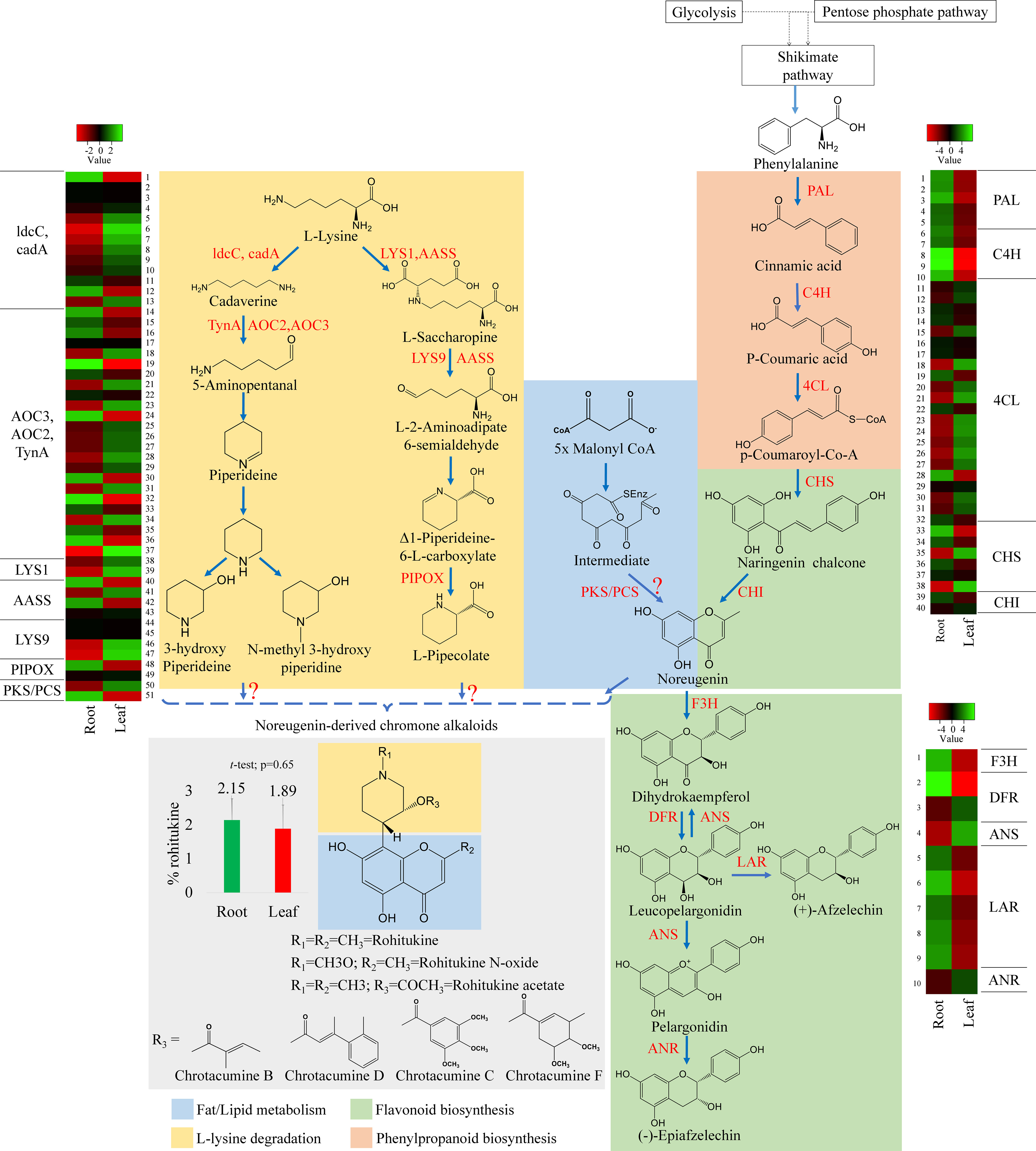
Figure 5 Putative biosynthetic pathway of rohitukine. Convergence of piperidine (also called lysine degradation) and shikimate routes (highlighted with different colours) in biosynthesis of chromone alkaloids in D. binectariferum. (rohitukine). Key biosynthetic enzymes (red) identified from the D. binectariferum transcriptome are shown at each. Steps highlighted with question marks denote unknown enzymatic conversions or candidate enzymes. Dotted arrows represent multiple steps or enzymes. Heat-map displaying differential expression of unigene transcripts (enzymes) D. binectariferum transcriptome are shown for roots and leaves. Details of numbers labelled in the Heat-map are provided in the additional file 3. The graph (insert) showing rohitukine content in the root and leaves of a one-year-old D. binectariferum seedling that was used in transcriptome sequencing. Mean (percent rohitukine, % of dry weight) and the error bars derived from three independent biological replicates (n =3). Piperidine (Lysine degradation) pathway; ldcC-Lysine decarboxylase; cadA- Lysine decarboxylase; LYS1- Lysine-forming saccharopine dehydrogenase; AASS- Alpha-aminoadipic semialdehyde synthase; AOC2/AOC3/TynA- primary-amine oxidase; LYS9- L-glutamate-forming saccharopine dehydrogenase 9; PIPOX- Sarcosine oxidase/L-pipecolate oxidase. Shikimate/Flavonoid/chromone alkaloid pathways: PAL- Phenylalanine ammonia-lyase; C4H- Trans-cinnamate 4-hydroxylase;4CL-4-coumarate-CoA ligase; PKS/PCS, Type III Polyketide synthase/Pentaketide chromone synthase; CHI, Chalcone isomerase; F3H, flavanone 3-hydroxylase; DFR, Dihydroflavonol 4-reductase/flavanone 4-reductase; LAR, Leucoanthocyanidin reductase; ANS, Anthocyanidin synthase; ANR, Anthocyanidin reductase.
In parallel, using HPLC, we also measured rohitukine content in D. binectariferum leaf and the root tissues and the data showed that rohitukine content was comparatively more in root (2.15 ± 0.62%) than in the leaf (1.89 ± 0.69%) (Figure 5; p > 0.05; not significant). To test if the expression pattern of flavonoid and other associated pathway genes is correlated with rohitukine content we measured their expression patterns using quantitative Realtime PCR (qRT) in leaves and roots. The upstream genes PAL, C4H, 4CL, and CHI are involved in the biosynthesis of the key precursor naringenin (Table S4; Figure S4) and all these four genes were highly expressed in the roots compared to the leaves and this was comparable to DEseq-RNA-seq expression data (Figure S4). Further, using BLASTP with Arabidopsis CHS as a query sequence, we identified CHS like genes from D. binectariferum transcriptome. In total four full-length CHS-like genes were identified. Of these, one of them corresponds to the CHS (DN149243) and it is likely to be involved in the biosynthesis of noreugenin. The other three unigenes DN1192 (PKS1), DN4064 (PKS2), and DN567668 belong to type-III polyketide synthases (PKS-III) (Figure 6A; Table S5). We subjected these candidates for phylogenetic analysis along with other functionally characterized CHSs and PKS-IIIs from plants together with bacterial PKSs as out groups. These genes were grouped into three clusters; chalcone synthases (CHS), plant non CHS/PKS-III, and bacterial PKS (Figure 6; Table S5). D. binectariferum PKS-III candidates DN1192 (PKS1), DN4064 (PKS2), and DN567668 were clustered with known plant PKS-III’s and it is likely that one of these could be involved in the biosynthesis of chromone alkaloids (Figure 6). The candidates PKS1 and PKS2 are highly expressed in the root with low to negligible expression in the leaves of D. binectariferum (Figure 6B) corresponding roughly with the rohitukine content in roots. For example, a pentaketide chromone synthase (PCS) that makes noreugenin (5,7-dihydroxy-2-methylchromone) by successive condensation of five malonyl-CoA precursor units is known from the plant Aloe arborescens (Izwan Mohd Lazim et al., 2013). Therefore, functional characterization of the PKS-III like candidates from D. binectariferum likely reveal PCS like enzyme in the biosynthesis of rohitukine or chromone alkaloids.
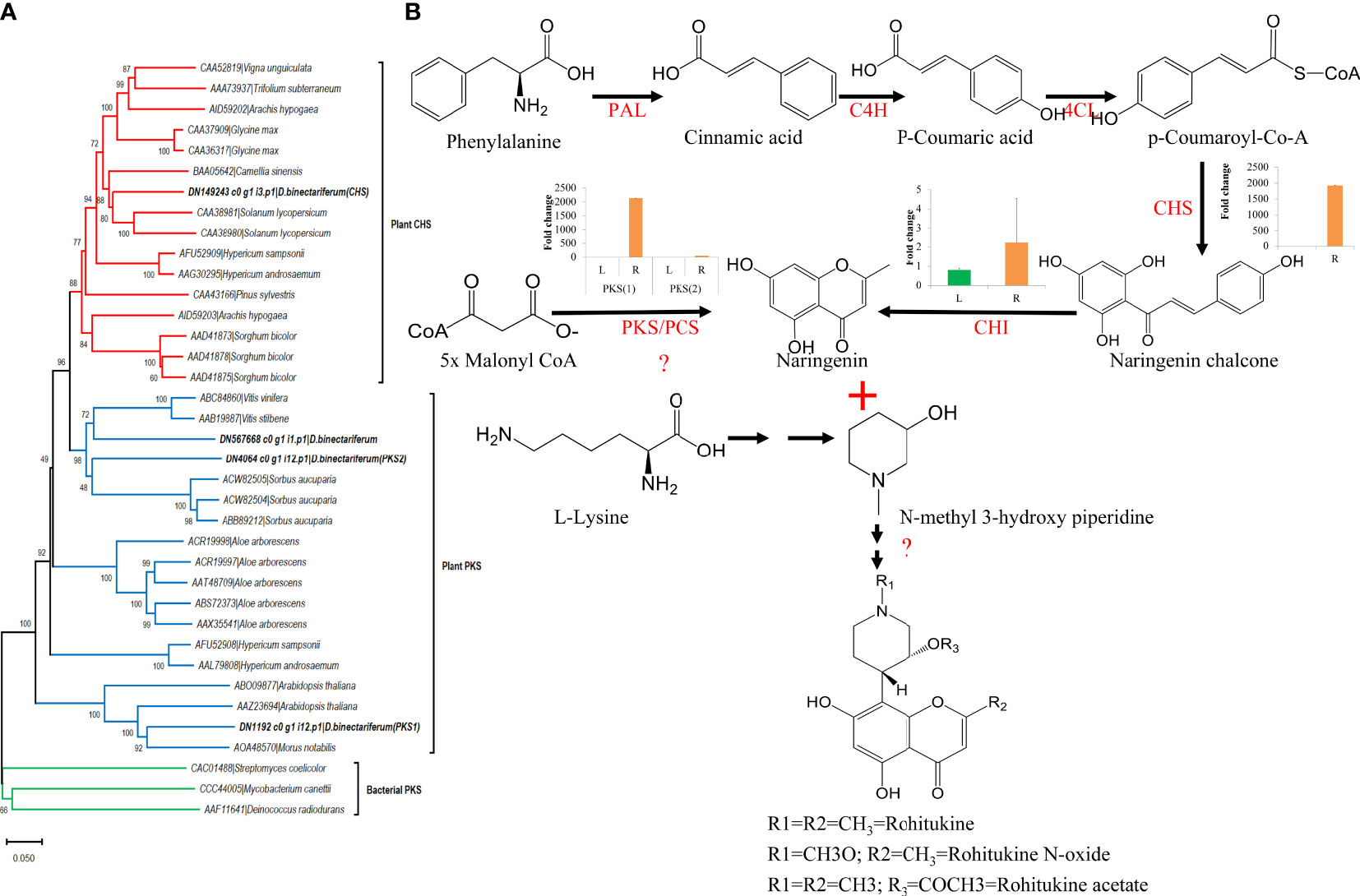
Figure 6 Candidate polyketide synthases identified from D. binectariferum transcriptome and their likely role in rohitukine/chromone alkaloids biosynthesis. (A) Phylogenetic tree of functionally characterized chalcone synthase (CHS) and polyketide synthase IIIs (PKSIII) from diverse plants along with CHS and PKS-III candidates identified from D. binectariferum transcriptome. Tree was created using MEGA7 with 1000 bootstrap values. Candidates highlighted in bold represent the candidates from D. binectariferum. (B) Predicted biosynthetic pathway of rohitukine/chromone alkaloids in D. binectariferum. Graphs in the inserts represent quantitative expression of key genes in the chromone alkaloid biosynthetic pathways (qRT mean ± SD, n=3, L; Leaves, R; Roots). PAL, Phenylalanine ammonia-lyase; C4H, Trans-cinnamate 4-hydroxylase; 4CL, 4-coumarate-CoA ligase; PKS/PCS, Type III Polyketide synthase/Pentaketide chromone synthase; CHI, Chalcone isomerase.
3.3.3 Identification of unigenes involved in the piperidine pathway
The L-lysine degradation pathway seem to provide N-containing phenol ring in chromone alkaloids. The amino acid L-lysine is degraded by a known enzyme lysine decarboxylase (ldcC, cadA, 13 unigenes) leading to the formation of cadaverine (Tomar et al., 2013). Next, primary-amine oxidase (AOC3/AOC2/tynA, 24 unigenes) converts cadaverine to 5-aminopentanal. Cadaverine and 5-aminopentanal are the central precursors in the biosynthesis of L-pipecolate and piperidine (Reimer et al., 2017) (Figure 5). The following enzymes are known to be involved in pipecolate and peridine biosynthesis from cadaverine; L-lysine-forming saccharopine dehydrogenase (LYS1, 2 unigenes), alpha-aminoadipic semialdehyde synthase (AASS, 5 unigenes), alpha-aminoadipic semialdehyde synthase, saccharopine dehydrogenase (LYS9, 3 unigenes) and sarcosine oxidase/L-pipecolate oxidase (PIPOX, 2 unigenes) (Figure 5) (Hartmann and Zeier, 2018). Presence of these candidates/unigenes and their expression in leaves suggest that the L-lysine degradation pathway is likely operative in D. binectariferum (Figures 5, 6B). In plants with L-lysine degradation pathway the associated pathway genes are normally expressed in leaves and the pathway end products like pyridine and piperidine alkaloids are also present in leaves (defence related) (Inaba and Ito-Inaba, 2010; Khadem and Marles, 2012; Hartmann and Zeier, 2018).
3.3.4 Putative chromone alkaloid biosynthetic pathway
Rohitukine is a chromone alkaloid consisting of noreugenin or flavone scaffold attached to a nitrogen containing piperidine ring (Harmon et al., 1979; Mohanakumara et al., 2010). Noreugenin chromone scaffold is a central precursor in biosynthesis of diverse chromone alkaloids including rohitukine and its derivatives. Noreugenin is made either through; a) a flavonoid pathway or b) through successive condensation of multiple malonyl co-A units by type-III polyketide synthase-like enzymes. The presence of the unigenes coding for PKS-III candidate enzymes as well as their high expression in roots where rohitukine is highly accumulated suggests that the route ‘b’ is more plausible (Figure 6B). And the piperidine ring is likely derived from the L-lysine degradation pathway and condensation of piperidine moiety and noreugenin yields rohitukine or chromone alkaloids (Figures 5, 6). The results also highlight the convergence of multiple biosynthetic pathways including the shikimic acid/phenylpropanoid pathway, flavonoids, acetate to pentaketide pathway, and L-lysine degradation pathway in the biosynthesis of complex chromone alkaloids like rohitukine. These results form a base for the further comprehensive investigation of the chromone alkaloid biosynthesis that is required for engineering heterologous hosts to make these valuable molecules and their derivatives.
4 Conclusions
D. binectariferum, an endemic medicinal plant of the Western Ghats, India, is well known to produce a chromone alkaloid called rohitukine and as well as a variety of triterpenoids and flavonoids. Rohitukine is a natural precursor for the semi-synthetic of anticancer drugs flavopiridol and P-276-00. To understand the biosynthetic pathway of rohitukine, we generated a comprehensive transcriptome assembly of leaf and root tissues and identified 51,598 non-redundant protein sequences of more than 200 amino acids. About 78.95% of these unigenes were annotated to the Nr database highlighting the completeness of the assembly. Next, with a combination of metabolite profiling and transcriptome assembly, we presented a biosynthetic route to these diverse compounds including terpenoids, flavonoids, and chromone alkaloids. More specifically, we discover candidate genes in rohitukine biosynthesis, and these enzymes strongly suggest the possibility of involvement of noreugenin pathway in the production of rohitukine and these biosynthetic routes have not been described previously. Therefore, these results pave the way for further functional characterization of these genes and clarify the biosynthesis pathway of chromone alkaloids, specifically rohitukine in D. binectariferum.
Data availability statement
The data presented in the study are deposited found in the NCBI repository, BioProject-PRJNA943416, and SRA accession numbers, SRR23901936 and SRR23901937.
Author contributions
PK and RT designed the research. PK and EV carried out experiments and analyzed the data. EV, JS and AM participated in sample collection and carried out the qRT-PCR assays. PK, RT and EV wrote the manuscript. The authors read and approved the final manuscript.
Funding
The current study was financially supported by SERB Early Career Grant ECR/2017/002686.
Acknowledgments
MP thanks SERB Early Career Grant ECR/2017/002686 for the financial support. RT acknowledges DBT-Ramalingaswami fellowship. We acknowledge usegalaxy.org for the server support. We acknowledge Prof. Uma Shaanker R, UAS Bangalore for his constructive suggestions while developing the manuscript.
Conflict of interest
The authors declare that the research was conducted in the absence of any commercial or financial relationships that could be construed as a potential conflict of interest.
Publisher’s note
All claims expressed in this article are solely those of the authors and do not necessarily represent those of their affiliated organizations, or those of the publisher, the editors and the reviewers. Any product that may be evaluated in this article, or claim that may be made by its manufacturer, is not guaranteed or endorsed by the publisher.
Supplementary material
The Supplementary Material for this article can be found online at: https://www.frontiersin.org/articles/10.3389/fpls.2023.1098987/full#supplementary-material
Additional File 1 | Functional annotation of final transcriptome of D. binectariferum with different databases.
Additional File 2 | Annotated (17 selected) KEGG metabolite pathways of D. binectariferum.
Additional File 3 | Differential expression of unigenes in leaves and roots of D. binectariferum.
Additional File 4 | Differential expression of unigenes (DEGs) in root and leaf tissues of D. binectariferum of selected 17 specialized metabolic pathways.
Glossary
References
Abe, I., Utsumi, Y., Oguro, S., Morita, H., Sano, Y., Noguchi, H. (2005). A plant type III polyketide synthase that produces pentaketide chromone. J. Am. Chem. Soc. 127, 1362–1363. doi: 10.1021/ja0431206
Albert, T. K., Rigault, C., Eickhoff, J., Baumgart, K., Antrecht, C., Klebl, B., et al. (2014). Characterization of molecular and cellular functions of the cyclin-dependent kinase CDK9 using a novel specific inhibitor. Br. J. Pharmacol. 171, 55–68. doi: 10.1111/bph.12408
Bhambhani, S., Lakhwani, D., Gupta, P., Pandey, A., Dhar, Y. V., Kumar Bag, S., et al. (2017). Transcriptome and metabolite analyses in Azadirachta indica: identification of genes involved in biosynthesis of bioactive triterpenoids. Sci. Rep. 7, 5043. doi: 10.1038/s41598-017-05291-3
Bolger, A. M., Lohse, M., Usadel, B. (2014). Trimmomatic: a flexible trimmer for Illumina sequence data. Bioinformatics 30, 2114–2120. doi: 10.1093/bioinformatics/btu170
Borowczak, J., Szczerbowski, K., Ahmadi, N., Szylberg, Ł. (2022). CDK9 inhibitors in multiple myeloma: a review of progress and perspectives. Med. Oncol. 39, 39. doi: 10.1007/s12032-021-01636-1
Camacho, C., Coulouris, G., Avagyan, V., Ma, N., Papadopoulos, J., Bealer, K., et al. (2009). BLAST+: architecture and applications. BMC Bioinformatics 15 (10), 421. doi: 10.1186/1471-2105-10-421
Chen, K. T. J., Militao, G. G. C., Anantha, M., Witzigmann, D., Leung, A. W. Y., Bally, M. B. (2021). Development and characterization of a novel flavopiridol formulation for treatment of acute myeloid leukemia. J. Control Release 333, 246–257. doi: 10.1016/j.jconrel.2021.03.042
Christian, B. A., Grever, M. R., Byrd, J. C., Lin, T. S. (2009). Flavopiridol in chronic lymphocytic leukemia: a concise review. Clin. Lymphoma Myeloma 9 (Suppl 3), S179–S185. doi: 10.3816/CLM.2009.s.009
Du, Y., Song, W., Yin, Z., Wu, S., Liu, J., Wang, N., et al. (2021). Genomic analysis based on chromosome-level genome assembly reveals an expansion of terpene biosynthesis of Azadirachta indica. bioRxiv 13, 853861. doi: 10.1101/2021.11.11.468207
Fan, W., Fan, L., Wang, Z., Yang, L. (2021). Limonoids from the genus melia (Meliaceae): phytochemistry, synthesis, bioactivities, pharmacokinetics, and toxicology. Front. Pharmacol. 12. doi: 10.3389/fphar.2021.795565
Fu, L., Niu, B., Zhu, Z., Wu, S., Li, W. (2012). CD-HIT: accelerated for clustering the next-generation sequencing data. Bioinformatics 28, 3150–3152. doi: 10.1093/bioinformatics/bts565
Ghissing, U., Kutty, N. N., Bimolata, W., Samanta, T., Mitra, A. (2023). Comparative transcriptome analysis reveals an insight into the candidate genes involved in anthocyanin and scent volatiles biosynthesis in colour changing flowers of Combretum indicum. Plant Biol. 25 (1), 85–95. doi: 10.1111/plb.13481
Grabherr, M. G., Haas, B. J., Yassour, M., Levin, J. Z., Thompson, D. A., Amit, I., et al. (2011). Full-length transcriptome assembly from RNA-Seq data without a reference genome. Nat. Biotechnol. 29, 644–652. doi: 10.1038/nbt.1883
Haas, B. J., Papanicolaou, A., Yassour, M., Grabherr, M., Blood, P. D., Bowden, J., et al. (2013). De novo transcript sequence reconstruction from RNA-seq using the Trinity platform for reference generation and analysis. Nat. Protoc. 8, 1494–1512. doi: 10.1038/nprot.2013.084
Hammer, Ø., Harper, D. A. T., Ryan, P. D. (2001). PAST: paleontological statistics software package for education and data analysis. Palaeontologia Electronica 4, 9.
Harmon, A. D., Weiss, U., Silverton, J. v (1979). The structure of rohitukine, the main alkaloid of Amoora rohituka (Syn. Aphanamixis polystachya) (meliaceae). Tetrahedron Lett. 20, 721–724. doi: 10.1016/S0040-4039(01)93556-7
Hartmann, M., Zeier, J. (2018). l-lysine metabolism to N-hydroxypipecolic acid: an integral immune-activating pathway in plants. Plant J. 96, 5–21. doi: 10.1111/tpj.14037
Hou, Q., Li, S., Shang, C., Wen, Z., Cai, X., Hong, Y., et al. (2022). Genome-wide characterization of chalcone synthase genes in sweet cherry and functional characterization of CpCHS1 under drought stress. Front. Plant Sci. 13. doi: 10.3389/fpls.2022.989959
Houghton, P. J. (2002). Chromatography of the chromone and flavonoid alkaloids. J. Chromatogr A 967, 75–84. doi: 10.1016/S0021-9673(01)01555-2
Hu, Y., Zhang, Y., Šmarda, P., Bureš, P., Guo, Q. (2023). Transcriptome and proteome associated analysis of flavonoid metabolism in haploid Ginkgo biloba. Int. J. Biol. Macromol. 224, 306–318. doi: 10.1016/j.ijbiomac.2022.10.125
Inaba, T., Ito-Inaba, Y. (2010). Versatile roles of plastids in plant growth and development. Plant Cell Physiol. 51, 1847–1853. doi: 10.1093/pcp/pcq147
Ismail, I. S., Nagakura, Y., Hirasawa, Y., Hosoya, T., Lazim, M. I. M., Lajis, N. H., et al. (2009). Chrotacumines A–D, chromone alkaloids from Dysoxylum acutangulum. J. Nat. Prod 72, 1879–1883. doi: 10.1021/np9003849
Izwan Mohd Lazim, M., Safinar Ismail, I., Shaari, K., Abd Latip, J., Ali Al-Mekhlafi, N., Morita, H. (2013). Chrotacumines E and F, two new chromone-alkaloid analogs from Dysoxylum acutangulum (Meliaceae) leaves. Chem. Biodivers 10, 1589–1596. doi: 10.1002/cbdv.201200391
Jain, S. K., Bharate, S. B., Vishwakarma, R. A. (2012). Cyclin-dependent kinase inhibition by flavoalkaloids. Mini Rev. Med. Chem. 12, 632–649. doi: 10.2174/138955712800626683
Joudaki, F., Ismaili, A., Sohrabi, S. S., Hosseini, S. Z., Kahrizi, D., Ahmadi, H. (2023). Transcriptome analysis of gall oak (Quercus infectoria): De novo assembly, functional annotation and metabolic pathways analysis. Genomics 115 (2), 110588. doi: 10.1016/j.ygeno.2023.110588
Kanehisa, M., Goto, S. (2000). KEGG: kyoto encyclopedia of genes and genomes. Nucleic Acids Res. 28, 27–30. doi: 10.1093/nar/28.1.27
Khadem, S., Marles, R. J. (2012). Chromone and flavonoid alkaloids: occurrence and bioactivity. Molecules 17, 191–206. doi: 10.3390/molecules17010191
Krishnan, N. M., Jain, P., Gupta, S., Hariharan, A. K., Panda, B. (2016). An improved genome assembly of Azadirachta indica A. Juss. G3: Genes Genomes Genet. 6, 1835–1840. doi: 10.1534/g3.116.030056
Kumara, P. M., Soujanya, K. N., Ravikanth, G., Vasudeva, R., Ganeshaiah, K. N., Shaanker, R. U. (2014). Rohitukine, a chromone alkaloid and a precursor of flavopiridol, is produced by endophytic fungi isolated from Dysoxylum binectariferum Hook.f and Amoora rohituka (Roxb).Wight & Arn. Phytomedicine 21, 541–546. doi: 10.1016/j.phymed.2013.09.019
Kuzuyama, T. (2002). Mevalonate and nonmevalonate pathways for the biosynthesis of isoprene units. Biosci. Biotechnol. Biochem. 66, 1619–1627. doi: 10.1271/bbb.66.1619
Kwan, B. D., Seligmann, B., Nguyen, T.-D., Franke, J., Dang, T.-T. T. (2023). Leveraging synthetic biology and metabolic engineering to overcome obstacles in plant pathway elucidation. Curr. Opin. Plant Biol. 71, 102330. doi: 10.1016/j.pbi.2022.102330
Levitsky, D. O., Dembitsky, V. M. (2014). Anti-breast cancer agents derived from plants. Nat. Prod. Bioprospect 5, 1–16. doi: 10.1007/s13659-014-0048-9
Lin, Y., Golovnina, K., Chen, Z.-X., Lee, H. N., Negron, Y. L. S., Sultana, H., et al. (2016). Comparison of normalization and differential expression analyses using RNA-Seq data from 726 individual Drosophila melanogaster. BMC Genomics 17, 28. doi: 10.1186/s12864-015-2353-z
Linardić, M., Braybrook, S. A. (2021). Identification and selection of optimal reference genes for qPCR-based gene expression analysis in Fucus distichus under various abiotic stresses. PloS One 16 (4), e0233249. doi: 10.1371/journal.pone.0233249
Liu, X., Lu, Y., Yuan, Y., Liu, S., Guan, C., Chen, S., et al. (2013). De novo transcriptome of Brassica juncea seed coat and identification of genes for the biosynthesis of flavonoids. PloS One 8, e71110. doi: 10.1371/journal.pone.0071110
Lorigooini, Z., Jamshidi-kia, F., Dodman, S. (2020). “Analysis of sesquiterpenes and sesquiterpenoids,” in Recent Advances in Natural Products Analysis (Elsevier), 289–312.
Love, M. I., Huber, W., Anders, S. (2014). Moderated estimation of fold change and dispersion for RNA-seq data with DESeq2. Genome Biol. 15, 550. doi: 10.1186/s13059-014-0550-8
Łukasik, P., Baranowska-Bosiacka, I., Kulczycka, K., Gutowska, I. (2021). Inhibitors of cyclin-dependent kinases: types and their mechanism of action. Int. J. Mol. Sci. 22 (6), 2806. doi: 10.3390/ijms22062806
Ma, C., Zhang, K., Zhang, X., Liu, G., Zhu, T., Che, Q., et al. (2021). Heterologous expression and metabolic engineering tools for improving terpenoids production. Curr. Opin. Biotechnol. 69, 281–289. doi: 10.1016/j.copbio.2021.02.008
Mandal, R., Becker, S., Strebhardt, K. (2021). Targeting CDK9 for anti-cancer therapeutics. Cancers (Basel) 13 (9), 2181. doi: 10.3390/cancers13092181
Meng, L., Feldman, L. (2010). A rapid TRIzol-based two-step method for DNA-free RNA extraction from Arabidopsis siliques and dry seeds. Biotechnol. J. 5, 183–186. doi: 10.1002/biot.200900211
Mohana Kumara, P. (2012). Assessing the genetic diversity of Dysoxylum binectariferum and prospecting for anticancer metabolite rohitukine from its phylogenetically related species in the Western Ghats, India (Doctoral dissertation, University of Agricultural Science, GKVK, Bangalore, India).
Mohanakumara, P., Sreejayan, N., Priti, V., Ramesha, B. T., Ravikanth, G., Ganeshaiah, K. N., et al. (2010). Dysoxylum binectariferum Hook.f (Meliaceae), a rich source of rohitukine. Fitoterapia 81, 145–148. doi: 10.1016/j.fitote.2009.08.010
Mohana Kumara, P., Srimany, A., Arunan, S., Ravikanth, G., Uma Shaanker, R., Pradeep, T. (2016). Desorption electrospray ionization (DESI) mass spectrometric imaging of the distribution of rohitukine in the seedling of Dysoxylum binectariferum hook. F. PloS One 11, e0158099. doi: 10.1371/journal.pone.0158099
Mohana Kumara, P., Srimany, A., Ravikanth, G., Uma Shaanker, R., Pradeep, T. (2015). Ambient ionization mass spectrometry imaging of rohitukine, a chromone anti-cancer alkaloid, during seed development in Dysoxylum binectariferum Hook.f (Meliaceae). Phytochemistry 116, 104–110. doi: 10.1016/j.phytochem.2015.02.031
Mohana Kumara, P., Zuehlke, S., Priti, V., Ramesha, B. T., Shweta, S., Ravikanth, G., et al. (2012). Fusarium proliferatum, an endophytic fungus from Dysoxylum binectariferum Hook.f, produces rohitukine, a chromane alkaloid possessing anti-cancer activity. Antonie van Leeuwenhoek Int. J. Gen. Mol. Microbiol. 101, 323–329. doi: 10.1007/s10482-011-9638-2
Moraes, G. P., Benitez, L. C., do Amaral, M. N., Vighi, I. L., Auler, P. A., da Maia, L. C., et al. (2015). Evaluation of reference genes for RT-qPCR studies in the leaves of rice seedlings under salt stress. Genet. Mol. Res. 14, 2384–2398. doi: 10.4238/2015.March.27.24
Morita, H., Kondo, S., Oguro, S., Noguchi, H., Sugio, S., Abe, I., et al. (2007). Structural Insight into Chain-Length Control and Product Specificity of Pentaketide Chromone Synthase from Aloe arborescens. Chem. Biol. 14, 359–369. doi: 10.1016/j.chembiol.2007.02.003
Morita, H., Nugroho, A. E., Nagakura, Y., Hirasawa, Y., Yoshida, H., Kaneda, T., et al. (2014). Chrotacumines G-J, chromone alkaloids from Dysoxylum acutangulum with osteoclast differentiation inhibitory activity. Bioorg Med. Chem. Lett. 24, 2437–2439. doi: 10.1016/j.bmcl.2014.04.020
Naik, R. G., Kattige, S. L., Bhat, S., Alreja, B., de Souza, N. J., Rupp, R. H. (1988). An antiinflammatory cum immunomodulatory piperidinylbenzopyranone from Dysoxylum binectariferum: isolation, structure and total synthesis. Tetrahedron 44, 2081–2086. doi: 10.1016/S0040-4020(01)90352-7
Naini, A. A., Mayanti, T., Supratman, U. (2022). Triterpenoids from Dysoxylum genus and their biological activities. Arch. Pharm. Res. 45, 63–89. doi: 10.1007/s12272-022-01371-9
Narnoliya, L. K., Rajakani, R., Sangwan, N. S., Gupta, V., Sangwan, R. S. (2014). Comparative transcripts profiling of fruit mesocarp and endocarp relevant to secondary metabolism by suppression subtractive hybridization in Azadirachta indica (neem). Mol. Biol. Rep. 41, 3147–3162. doi: 10.1007/s11033-014-3174-x
Pandreka, A., Chaya, P. S., Kumar, A., Aarthy, T., Mulani, F. A., Jennifer, C., et al. (2021). Limonoid biosynthesis 3: Functional characterization of crucial genes involved in neem limonoid biosynthesis. Phytochemistry 184, 112669. doi: 10.1016/j.phytochem.2021.112669
Patro, R., Duggal, G., Love, M. I., Irizarry, R. A., Kingsford, C. (2017). Salmon provides fast and bias-aware quantification of transcript expression. Nat. Methods 14, 417–419. doi: 10.1038/nmeth.4197
Peter, J. A., Cock, J. M., Grüning, C. B, Johnson, J. E., Soranzo, N. (2015). NCBI BLAST+ integrated into Galaxy. GigaScience 4, 39. doi: 10.1186/s13742-015-0080-7
Pérez, L., Alves, R., Perez-Fons, L., Albacete, A., Farré, G., Soto, E., et al. (2022). Multilevel interactions between native and ectopic isoprenoid pathways affect global metabolism in rice. Transgenic Res. 31, 249–268. doi: 10.1007/s11248-022-00299-6
Rajakani, R., Narnoliya, L., Sangwan, N. S., Sangwan, R. S., Gupta, V. (2014). Subtractive transcriptomes of fruit and leaf reveal differential representation of transcripts in Azadirachta indica. Tree Genet. Genomes 10, 1331–1351. doi: 10.1007/s11295-014-0764-7
Reimer, L. C., Will, S. E., Schomburg, D. (2017). The fate of lysine: Non-targeted stable isotope analysis reveals parallel ways for lysine catabolization in Phaeobacter inhibens. PloS One 12, e0186395. doi: 10.1371/journal.pone.0186395
Sandeep, Ghosh, S. (2020). “Chapter 12 - Triterpenoids: Structural diversity, biosynthetic pathway, and bioactivity,” in Bioactive Natural Products Studies in Natural Products Chemistry. Ed. Atta-ur-Rahman (Elsevier), 411–461. doi: 10.1016/B978-0-12-819483-6.00012-6
Schilmiller, A. L., Schauvinhold, I., Larson, M., Xu, R., Charbonneau, A. L., Schmidt, A., et al. (2009). Monoterpenes in the glandular trichomes of tomato are synthesized from a neryl diphosphate precursor rather than geranyl diphosphate. Proc. Natl. Acad. Sci. USA 106, 10865–10870. doi: 10.1073/pnas.0904113106
Sedlacek, H., Czech, J., Naik, R., Kaur, G., Worland, P., Losiewicz, M., et al. (1996). Flavopiridol (L86 8275; NSC 649890), a new kinase inhibitor for tumor therapy. Int. J. Oncol. 9, 1143–1168. doi: 10.3892/ijo.9.6.1143
Sekine, C., Sugihara, T., Miyake, S., Hirai, H., Yoshida, M., Miyasaka, N., et al. (2008). Successful treatment of animal models of rheumatoid arthritis with small-molecule cyclin-dependent kinase inhibitors. J. Immunol. 180, 1954–1961. doi: 10.4049/jimmunol.180.3.1954
Seppey, M., Manni, M., Zdobnov, E. M. (2019). BUSCO: Assessing Genome Assembly and Annotation Completeness. 227–245. doi: 10.1007/978-1-4939-9173-0_14
Sharma, A., Bhardwaj, G., Cannoo, D. S. (2021). Antioxidant potential, GC/MS and headspace GC/MS analysis of essential oils isolated from the roots, stems and aerial parts of Nepeta leucophylla. Biocatal Agric. Biotechnol. 32, 101950. doi: 10.1016/j.bcab.2021.101950
Stadler, W. M., Vogelzang, N. J., Amato, R., Sosman, J., Taber, D., Liebowitz, D., et al. (2000). Flavopiridol, A novel cyclin-dependent kinase inhibitor, in metastatic renal cancer: A University of Chicago phase II consortium study. J. Clin. Oncol. 18, 371. doi: 10.1200/JCO.2000.18.2.371
Szkopińska, A., Płochocka, D. (2005). Farnesyl diphosphate synthase; regulation of product specificity. Acta Biochim. Pol. 52, 45–55. doi: 10.18388/abp.2005_3485
Tomar, P. C., Lakra, N., Mishra, S. N. (2013). Cadaverine: a lysine catabolite involved in plant growth and development. Plant Signal Behav. 8, e25850. doi: 10.4161/psb.25850
Varun, E., Bhakti, K., Aishwarya, K., Suraj, R. H., Jagadish, M. R., Mohana Kumara, P. (2023). Rohitukine content across the geographical distribution of Dysoxylum binectariferum Hook F. and its natural derivatives are potential sources of CDK inhibitors. Heliyon 9 (2), e13469. doi: 10.1016/j.heliyon.2023.e13469
Wang, X., Hu, H., Wu, Z., Fan, H., Wang, G., Chai, T., et al. (2021). Tissue-specific transcriptome analyses reveal candidate genes for stilbene, flavonoid and anthraquinone biosynthesis in the medicinal plant Polygonum cuspidatum. BMC Genomics 22, 353. doi: 10.1186/s12864-021-07658-3
Xu, B., Liu, L., Song, G. (2023). Functions and regulation of translation elongation factors. Front. Mol. Biosci. 19 (8). doi: 10.3389/fmolb.2021.816398
Xu, M., Zhang, B., Su, X., Zhang, S., Huang, M. (2011). Reference gene selection for quantitative real-time polymerase chain reaction in Populus. Anal. Biochem. 408 (2), 337–339. doi: 10.1016/j.ab.2010.08.044
Yan, H., Si, H., Zhao, H., Chen, L., Yu, J., Zhao, H., et al. (2021). Four new cycloartane triterpenoids from the leaves of Dysoxylum binectariferum. Phytochem. Lett. 41, 101–105. doi: 10.1016/j.phytol.2020.11.013
Yan, H.-J., Wang, J.-S., Kong, L.-Y. (2014). Cytotoxic steroids from the leaves of Dysoxylum binectariferum. Steroids 86, 26–31. doi: 10.1016/j.steroids.2014.04.014
Zhao, L., Chang, W., Xiao, Y., Liu, H., Liu, P. (2013). Methylerythritol phosphate pathway of isoprenoid biosynthesis. Annu. Rev. Biochem. 82, 497–530. doi: 10.1146/annurev-biochem-052010-100934
Keywords: Dysoxylum binectariferum, de novo transcriptome, rohitukine, chromone alkaloids biosynthesis, chalcone synthases, polyketide synthases
Citation: Kumara PM, Varun E, Sanjay JR, Madhushree AH and Thimmappa R (2023) De novo transcriptome analysis of Dysoxylum binectariferum to unravel the biosynthesis of pharmaceutically relevant specialized metabolites. Front. Plant Sci. 14:1098987. doi: 10.3389/fpls.2023.1098987
Received: 15 November 2022; Accepted: 05 July 2023;
Published: 09 August 2023.
Edited by:
Luan Luong Chu, Phenikaa University, VietnamReviewed by:
Charu Chandra Giri, Osmania University, IndiaClément Cuello, Université de Tours, France
Pankaj Kumar Sharma, Birla Institute of Technology and Science, India
Qi Tang, Hunan Agricultural University, China
Copyright © 2023 Kumara, Varun, Sanjay, Madhushree and Thimmappa. This is an open-access article distributed under the terms of the Creative Commons Attribution License (CC BY). The use, distribution or reproduction in other forums is permitted, provided the original author(s) and the copyright owner(s) are credited and that the original publication in this journal is cited, in accordance with accepted academic practice. No use, distribution or reproduction is permitted which does not comply with these terms.
*Correspondence: Patel Mohana Kumara, bW9uYXBhdGVscGdhdHRpQGdtYWlsLmNvbQ==; bW9oYW5ha3VtYXJhLnBAdWhzYmFnYWxrb3QuZWR1Lmlu