- 1Department of Plant and Microbial Biology, North Carolina State University, Raleigh, NC, United States
- 2Department of Mechanical and Aerospace Engineering and Organic and Carbon Electronics Laboratories, North Carolina State University, Raleigh, NC, United States
- 3Department of Physics and Organic and Carbon Electronics Laboratories, North Carolina State University, Raleigh, NC, United States
- 4Department of Chemistry, University of North Carolina, Chapel Hill, NC, United States
The integration of semi-transparent organic solar cells (ST-OSCs) in greenhouses offers new agrivoltaic opportunities to meet the growing demands for sustainable food production. The tailored absorption/transmission spectra of ST-OSCs impacts the power generated as well as crop growth, development and responses to the biotic and abiotic environments. To characterize crop responses to ST-OSCs, we grew lettuce and tomato, traditional greenhouse crops, under three ST-OSC filters that create different light spectra. Lettuce yield and early tomato development are not negatively affected by the modified light environment. Our genomic analysis reveals that lettuce production exhibits beneficial traits involving nutrient content and nitrogen utilization while select ST-OSCs impact regulation of flowering initiation in tomato. These results suggest that ST-OSCs integrated into greenhouses are not only a promising technology for energy-neutral, sustainable and climate-change protected crop production, but can deliver benefits beyond energy considerations.
1 Introduction
Greenhouses enable the production of food crops and ornamental plants year-round outside of their natural growth zones and can therefore produce more crops on less land than conventional field cultivation, giving them an important role in feeding the world as space becomes increasingly limited. Current predictions for global food demand estimate a 70% increase by 2050, while the world population is predicted to grow by roughly 40% over the same period (Bruinsma, 2009). Overall productivity in greenhouses is several times higher than in fields. A comparative analysis of tomato production showed that greenhouse productivity in New York was 12-fold higher than that of Florida fields (De Villiers et al., 2009), while lettuce production was more than ten times higher under greenhouse conditions on a per area basis (Barbosa et al., 2015).
In addition to increased productivity, greenhouses also use less water than conventional farming (Page et al., 2012; Barbosa et al., 2015; Ntinas et al., 2017). This will become increasingly advantageous, because large regions of the world are expected to experience field crop losses as climate change continues to limit the availability of water for irrigation (Elliott et al., 2013). Greenhouse cultivation also allows for the reduction of the ecological impacts of pesticides and fertilizers as well as a reduction in herbicide use. The enclosed nature of greenhouses presents an opportunity to more easily control and monitor chemical contaminants, such as pesticides and fertilizers, in water and soil exiting the system (Barbosa et al., 2015). Furthermore, greenhouses can be designed to recycle nutrients in their irrigation systems to avoid excess fertilizer use (Halbert-Howard et al., 2020). This becomes especially important as the production of N-fertilizer is not only energy intensive, but its field applications lead to eutrophication of aquatic systems. Along with greater control within the enclosed environment, greenhouses shelter crops from extreme weather conditions like drought, heat or flooding, which are worsened by climate change. In 2021 alone, damage to field crops due to extreme weather events in the United States exceeded 8 billion USD (Munch, 2022).
However, this seemingly ideal system for plant productivity requires large amounts of energy for temperature control and supplemental lighting, which reduces its economic and environmental sustainability. Life cycle assessment (LCA) studies have shown that the carbon footprint of greenhouse-grown crops exceeds that of conventional crops when fossil fuels are used for heating and cooling (Page et al., 2012; Ntinas et al., 2017). The studies mentioned above that reported large improvements in greenhouse tomato and lettuce production also found energy demands nearly 19 times higher than field cultivation for tomato (De Villiers et al., 2009) and more than 80 times higher for lettuce (Barbosa et al., 2015). This prevents conventional greenhouses from being a truly sustainable method of food production. Therefore, new technologies are needed to solve the problem of high energy demand in these climate-controlled systems.
One alternative is to grow crops in insulated, fully enclosed environments that avoid the greenhouse effect caused by natural sunlight. These sole-source container farms are an alternative to greenhouse cultivation with a lower cost for climate control, but they suffer from high energy costs for artificial lighting that prevent their economic viability for crops other than microgreens or lettuce (Pattison et al., 2018). These container systems can also produce crops at higher yields than conventional field agriculture (Qian et al., 2022). While these systems require less energy for space conditioning than a greenhouse (Ramin Shamshiri et al., 2018), the elimination of natural lighting requires artificial light sources. This artificial lighting, typically provided by LEDs, is the major energy requirement and limitation of container systems (Liebman-Pelaez et al., 2021). Indeed, one study found that such systems are only economically viable for the production of low-light crops, such as microgreens and, to a lesser extent, lettuce (Pattison et al., 2018), although the high energy cost can possibly be reduced through an intermittent lighting strategy (Song et al., 2019). Container systems trade one high energy requirement for another by excluding sunlight and relying on LEDs. The sustainable production of the majority of crops will require both the integration of a renewable energy source and better utilization of the natural sunlight available for crop production in most of the world.
While other solar-powered greenhouses do improve the sustainability of food production, they require either additional land in the form of solar farms or a reduction in yield in the case of opaque rooftop solar cells (Hassanien et al., 2016). Wavelength-selective semi-transparent organic solar cells (OSCs) are an alternative to both container and conventional solar power systems. The cost of OSC-greenhouses can be kept close to the cost of conventional and photovoltaic-adjacent greenhouse systems without additional land in favorable climates (Hollingsworth et al., 2020). Additionally, these OSC systems allow more light to reach the crops below, avoiding the yield losses seen in opaque systems. However, the light reaching the plants is altered in both light intensity (i.e., quantity) and light spectrum (i.e., quality). Through the selection of distinct organic semiconductors as the OSC active layer, the spectral transmission of the devices can be controlled (Xiao et al., 2017). Modeling has shown that these spectrally flexible net zero energy greenhouses can be economically feasible for crop production in most regions of the world (Ravishankar et al., 2020).
Plants depend on light for their energy supplied by photosynthesis. Light harvesting antenna complexes contain large numbers of chlorophylls that absorb primarily in the blue (400-500 nm) and red (600-700 nm) regions of the light spectrum to produce energy (Kobayashi et al., 2013; Taniguchi and Lindsey, 2018; reviewed in Bilodeau et al., 2019). As a result of these peak absorbance wavelengths, red and blue light are used more efficiently than green light during photosynthesis (Figure 1). The wavelengths from (400-700 nm), termed photosynthetically active radiation (PAR), are the most relevant for plant growth and are measured in PPFD, photosynthetic photon flux density. TPFD, or total photon flux density, is also used to account for the full range of wavelengths sensed by plants (Kim et al., 2019; Zhen and Bugbee, 2020; Kohler and Lopez, 2021).
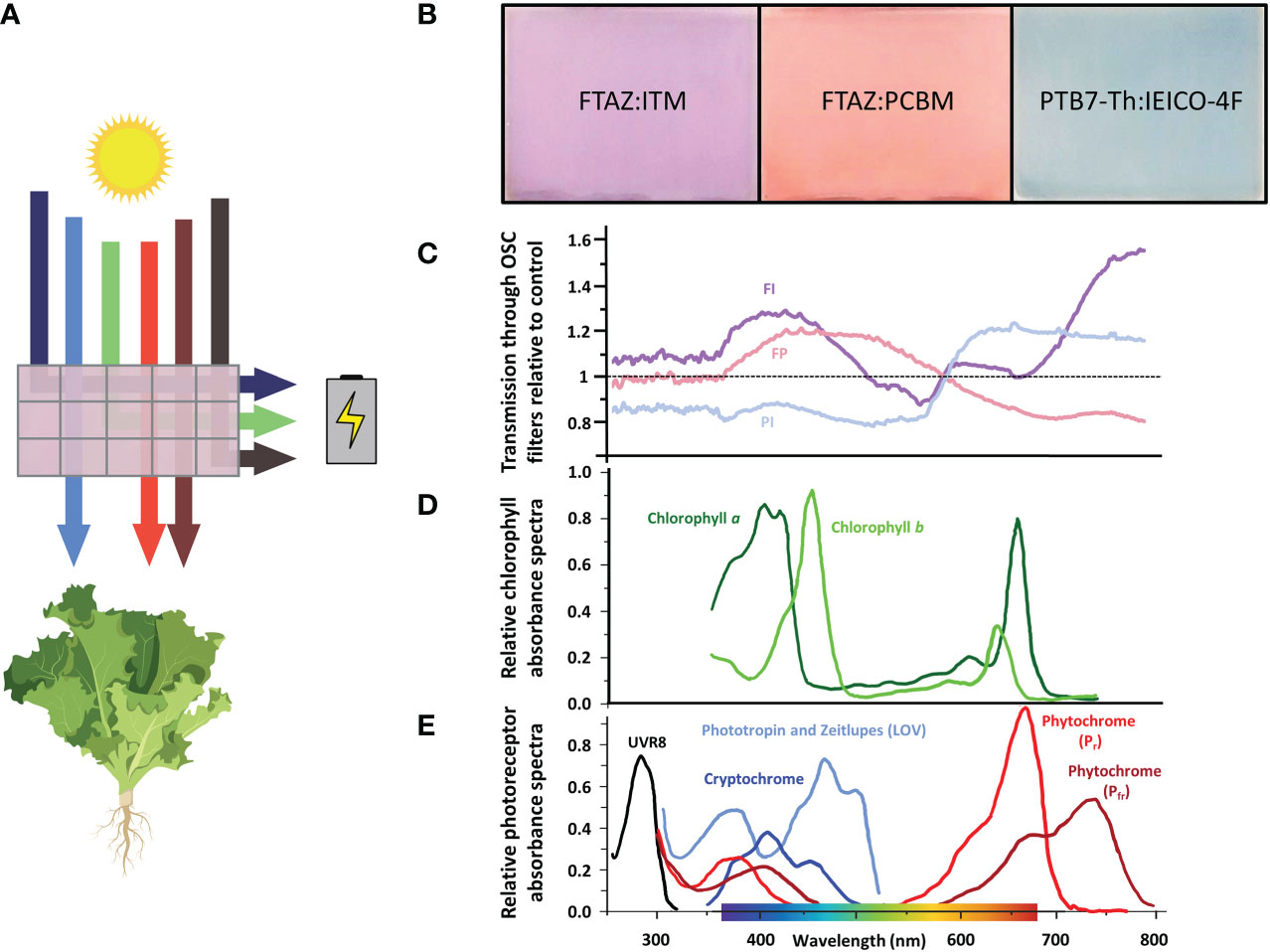
Figure 1 Wavelength selectivity of OSC devices. (A) Schematic of a wavelength-selective OSC device. Specific wavelengths of light are harvested to generate electrical energy to power the greenhouse. The wavelengths most important for photosynthesis (blue, red and far-red) are selectively transmitted through the device to grow greenhouse crops. Created with BioRender.com. (B) The OSC filters are named for the two organic molecules that determine their color and transmission spectrum. Different donor and acceptor molecules can be chosen to tune the wavelengths that reach the plants below. (C) Ratio of light transmission through the OSC filters relative to the light transmitted through the control. Each filter varies in relation to the control and to each other over PAR and in the far-red region (700-750 nm). (D) Absorption spectra of plant chlorophylls. Chlorophyll a and b harvest light energy from sunlight to power photosynthesis over PAR (400-700 nm), especially in the blue and red regions (Kobayashi et al., 2013; Taniguchi and Lindsey, 2018; reviewed in Bilodeau et al., 2019). (E) Absorption spectra of photoreceptors. UVR8 absorbs UV light. Cryptochromes, phototropins and zeitlupes absorb primarily blue light. Phytochromes absorb red and far-red light (Taiz and Zeiger, 2022; Sager et al., 1988; Galvao and Fankhauser, 2015; reviewed in Bilodeau et al., 2019).
Because light is the only energy source for plants, their entire growth and development is dependent upon and regulated by changes in their light environment. To sense these light changes, plants have evolved a complex network of additional chromophores called photoreceptors that sense different wavelengths, intensity, and direction in the 400-700 nm range of PAR and beyond (Sager et al., 1988; Taiz and Zeiger, 2002; Galvao and Fankhauser, 2015; Bilodeau et al., 2019). This sensory input informs photomorphogenesis, the adjustment of growth and development to light conditions (Galvao and Fankhauser, 2015). Photomorphogenesis occurs through large integrated gene networks with other environmental factors such as nutrient availability and temperature (Abbas et al., 2014; Chen et al., 2016; Hahm et al., 2020). Many crop production characteristics are influenced by these gene networks, including height, nutrient content and the timing of flowering and fruit production (Guo et al., 1998; Schmitt et al., 1999; Park et al., 2007).
Due to the tunable nature of OSCs, net zero greenhouses can create a wide variety of light environments for crops. Various models have estimated that sufficient crop and energy harvests can be produced using different organic semiconductors in many climates (Emmott et al., 2015; Ravishankar et al., 2022). Peppers (Zisis et al., 2019) and tomatoes (Waller et al., 2021) have been grown in greenhouses partially covered in OSC panels with minimal impacts on growth. Flowering species, such as tomato, are important greenhouse crops that are particularly sensitive to changes in light spectrum. To address the question of how the altered spectra produced by OSC filters might impact light-sensitive growth and development of common greenhouse crops, we grew a low light-requiring crop, lettuce (Lactuca sativa cv. ‘Red oak leaf’) and a high light-requiring crop, tomato (Solanum lycopersicum cv. ‘Moneymaker’) under simulated OSC greenhouse conditions. Previously studied OSC filters were selected based on their spectral complementarity with chlorophyll and photoreceptor absorbance and ease of fabrication: FTAZ : IT-M (FI), FTAZ : PCBM (FP) and PTB7-Th : IEICO-4F (PI) (Xiao et al., 2017; Ravishankar et al., 2021). We found no detrimental impacts on biomass accumulation in either species. Furthermore, we demonstrate modifications in gene expression detected through a transcriptome analysis that point to agronomically important emerging traits in crop physiology in response to the altered light spectra, including flowering time, nitrogen use, and nutritional content. This analysis demonstrates that OSC greenhouses may present synergistic opportunities to achieve environmentally sustainable agriculture and drive favorable crop traits.
2 Results
Our experiments were designed to identify the molecular and physiological responses of lettuce and tomato plants to altered light spectra provided by different ST-OSCs. Here, we present the results of plants grown under different OSC filters with the same input of total photosynthetic active radiation. A second set of supplemental experiments was conducted where light intensity was allowed to vary as well as light spectra, based on the inherent variable transmittance of the OSC filters. Because many traits are not apparent unless directly tested (e.g., nitrogen use efficiency or drought responses), we used transcriptome analyses to identify differentially regulated genome networks as indicators for underlying changes in plant physiology and development. The results of the variable light intensity are included in the supplemental information.
2.1 Light conditions under OSCs
Growth containers with integrated OSC filters were designed to house plants within a climate-controlled growth chamber equipped with lights that approximated the spectrum of natural sunlight (Figure S1). The distance from the light source to the top of each growth box was kept constant to simulate the irradiation on the roof of actual greenhouses in a previous experiment (Figure S2) (Ravishankar et al., 2021). The distance from the light source and height of the growth boxes was adjusted to minimize differences between light intensity (PPFD) between each filter treatment and control (Table 1). Filter degradation due to exposure to light and oxygen resulted in increased variations in light intensity by the end of both experiments (Figure S3).
2.2 Biomass was largely unaffected by OSC filters
Variation in lettuce and tomato growth was largely insignificant when only spectra varied. This was unsurprising given that there were few significant differences between filter treatments and controls when both light quality and intensity were altered by OSC filters (Ravishankar et al., 2021). There were no significant differences in lettuce fresh weight, dry weight or leaf area at harvest stage (Figures 2A–D) and the transplant stage (Figure S4). The spectral differences between the three OSC filters alone were evidently not large enough to have a significant effect on biomass in lettuce.
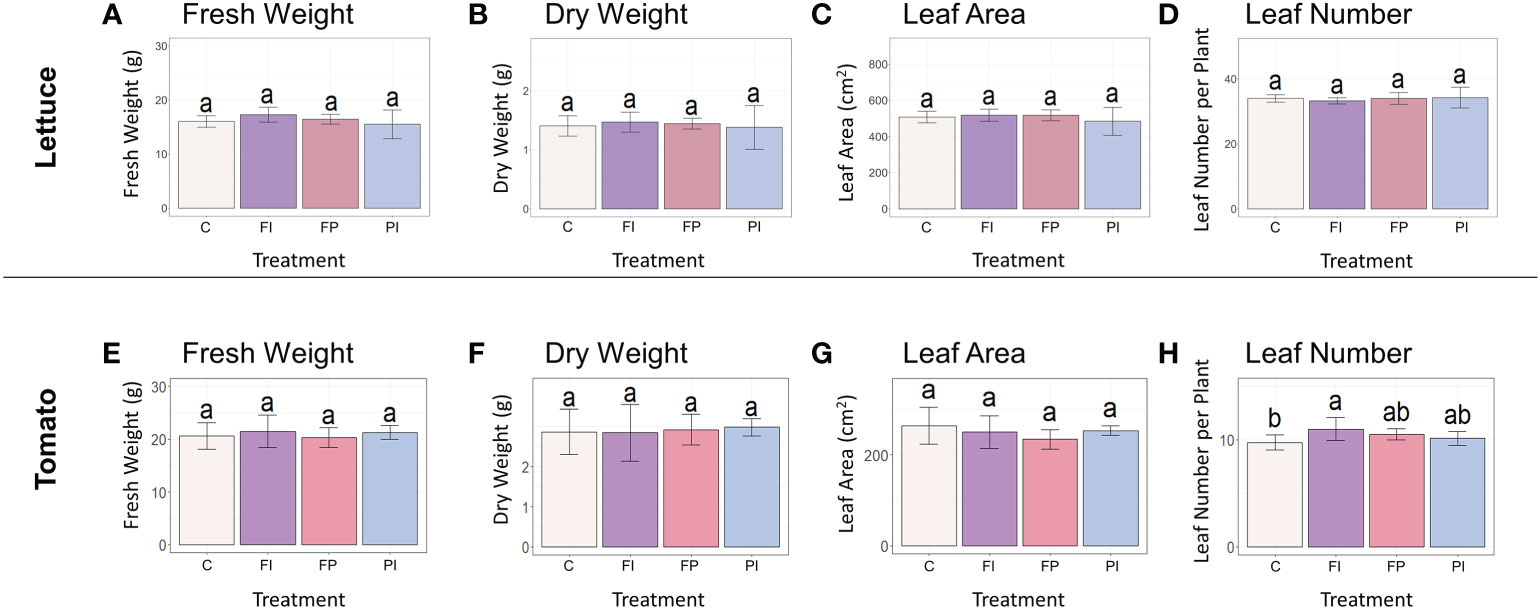
Figure 2 Biomass accumulation of lettuce and tomato under OSC filters. (A) Lettuce fresh weight of lettuce grown under OSC filters and clear glass control (C). (B) Lettuce dry weight grown under OSC filters. (C) Lettuce leaf area under OSC filters. (D) Number of leaves per lettuce plant under OSC filters. (E–H) Tomato fresh weight, dry weight, leaf area and leaf number. Statistical significance was assessed by ANOVA and Tukey test (p<0.05). For each plot, the differences between the means of treatments marked with the same letter are not statistically significant.
Tomato biomass accumulation was measured by the same parameters recorded previously for lettuce in addition to those measurements more relevant to growth and development of a fruit crop species. As seen in lettuce, there were no significant differences in shoot fresh weight, shoot dry weight or leaf area between the filter treatments and control (Figures 2E–G). The FI treatment produced significantly more leaves than the control in tomato (Figure 2H). This differs from the response of lettuce to the OSC filters, where there were no significant differences between the filter treatments and the control in leaf number or other measured biomass parameters.
2.3 Crop-specific responses in photosynthetic CO2-fixation and transpiration
Although overall biomass and growth phenotype did not vary significantly in lettuce plants grown under the different spectra, the rate of photosynthesis was significantly improved in the FI treatment, relative to control (Figure 3). In contrast, the transpiration rate was unaffected. Because differences in light intensity were minimized, the differences in photosynthesis are likely the result of the spectrum of the FI filter. While filter degradation did result in some variation in light intensity by the end of the lettuce experiment (Tables 1, S3; Figure S3), the trends reported here remained after normalization to PPFD recorded at the time of measurement, although they were not always statistically significant (Figure S5).
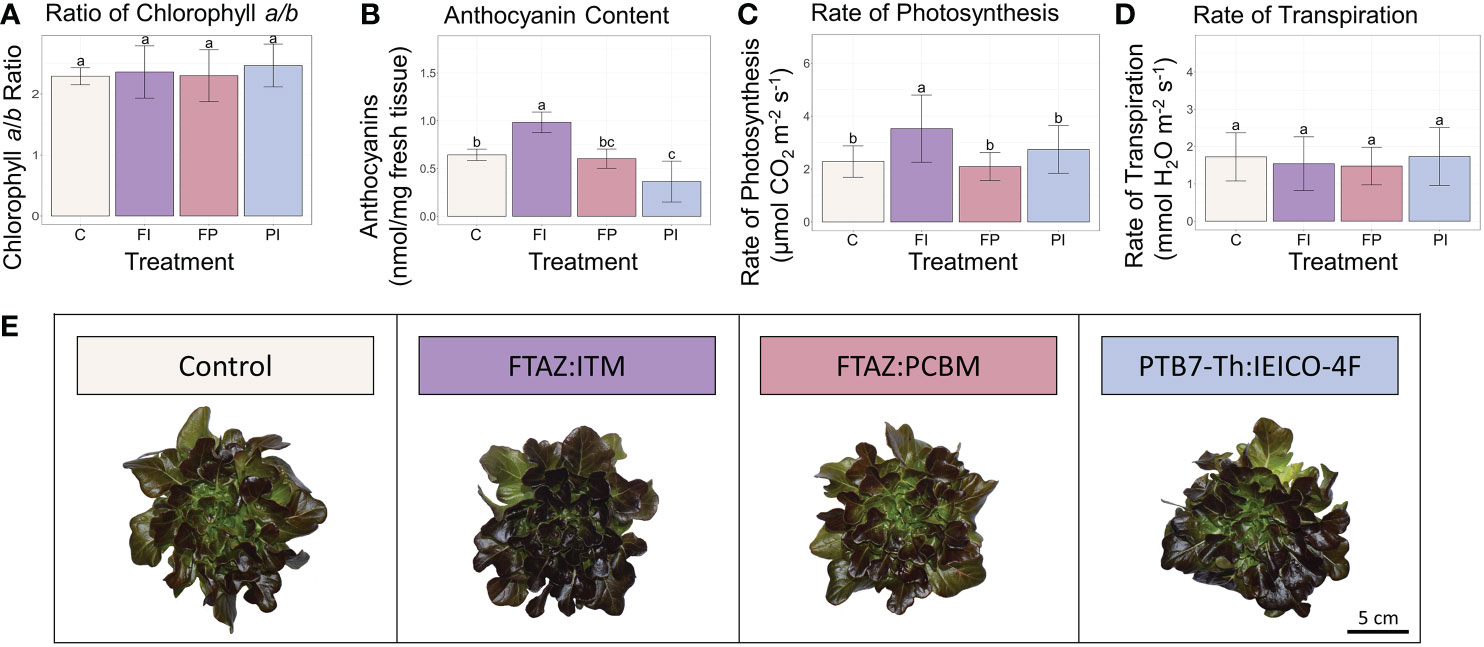
Figure 3 Lettuce secondary metabolites and carbon assimilation under OSC filters. (A) Ratio of chlorophyll a to chlorophyll b in lettuce leaf tissue. (B) Anthocyanin concentration in lettuce leaf tissue. (C) Rate of photosynthesis (μmol CO2 m-2 s-1) measured under each light condition. (D) Transpiration rate (mmol H2O m-2 s-1) measured under each light condition. (E) Representative photos of lettuce plants from each treatment. Error bars represent the standard deviation. Statistical significance was assessed by ANOVA and Tukey test (p<0.05). For each plot, the differences between the means of treatments marked with the same letter are not statistically significant.
In contrast to lettuce, the tomato plants grown under the FI filter showed no significant difference in photosynthesis between control and FI filter treatment, albeit with large variation (Figures 4, S6). A significant decrease was found in the transpiration rate of these tomato plants. The increased photosynthetic rate under the FI filter in lettuce may be due to the proportion of red to blue light in relation to the amount of less efficient green light (Wang et al., 2016). The opposite response seen in tomato could be due to the 3% increase in green light seen in the FI tomato treatment relative to the FI treatment in the lettuce experiment. However, this increase only raises the percent of green light in the FI treatment to be equal to the percent of green in the C treatment (30%). This suggests that this response is more likely to be a species difference between lettuce and tomato, possibly affected by the sensitivity of stomatal aperture.
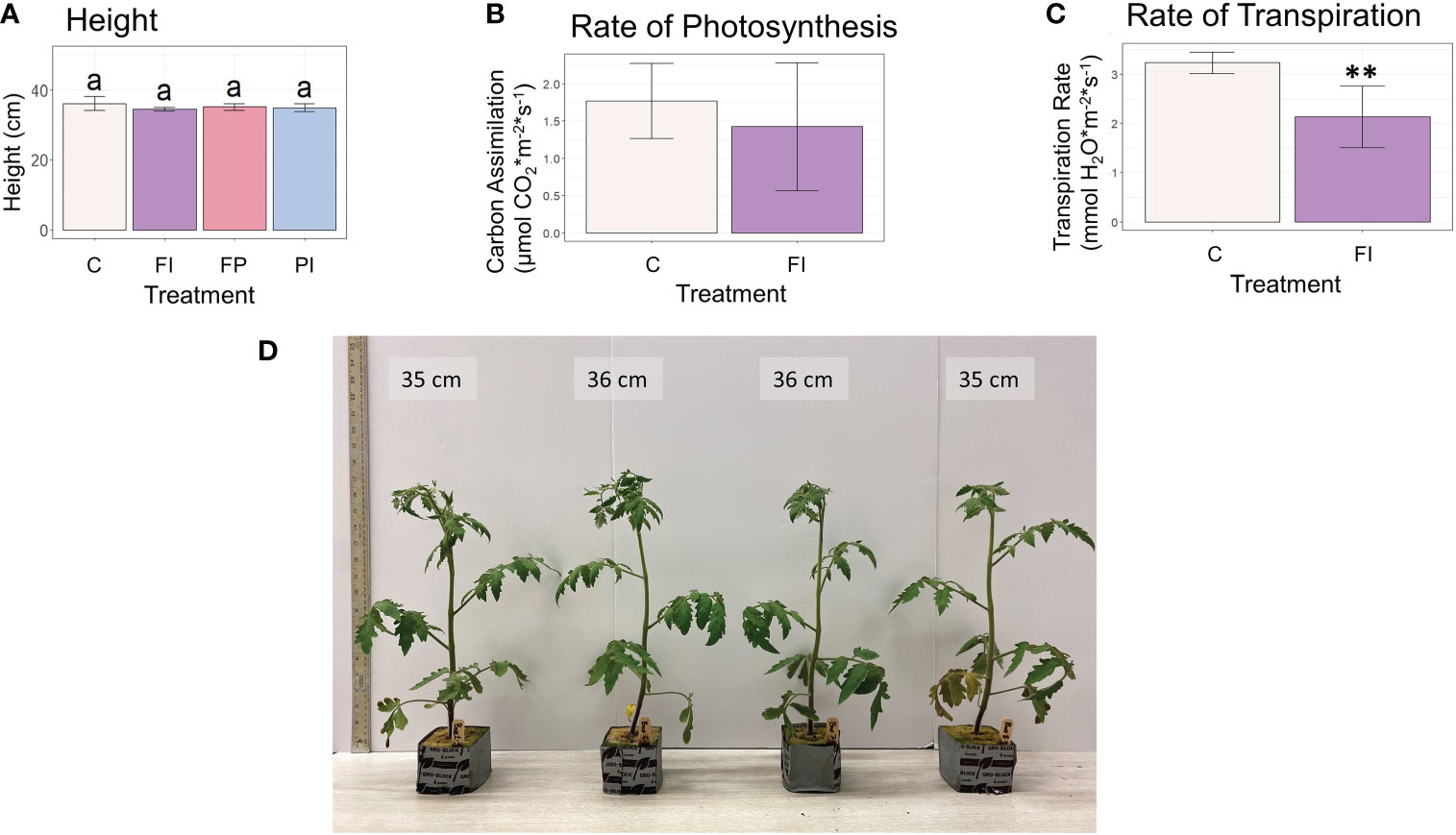
Figure 4 Height and carbon assimilation in tomato plants. (A) Height of tomato plants at 30 days. (B) Rate of photosynthesis of tomato plants under the FI filter relative to the unfiltered control. Although the average rate was lower under FI filtered light, this difference was not statistically significant due to the large variation within the treatment. (C) Rate of transpiration under the FI filter relative to the unfiltered control. The FI treatment was significantly lower than control (** indicates significance at p<0.01). (D) Photo of representative tomato plants at 30 days.
2.4 Anthocyanin content was significantly higher under the FI filter in lettuce
Certain secondary metabolites with photosynthetic relevance were extracted from lettuce leaf tissue to look for acclimation to the altered light environment under the OSC filters. Chlorophyll, for example, has been shown to increase in overall concentration in response to a lower R/B ratio (38). There were no significant differences in the ratio of chlorophyll a to b (Figure 3). Surprisingly, this was also the case when light intensity as well as spectra was varied (Figure S7) (Ravishankar et al., 2021), despite a well-documented correlation between lower light intensity and an increase in chlorophyll b (Evans, 1988; Pattanayak et al., 2004). Similarly, the concentration of carotenoids was not significantly different when light intensity was consistent (Figure S7). Although carotenoid concentrations did vary significantly between treatments under variable light intensity, the treatment with the highest light intensity, C, had a carotenoid concentration that was not significantly different from that of the lowest light intensity, FI (Figure S2). However, anthocyanins that protect the plant by absorbing excess light varied between treatments. The FI treatment had a significantly higher anthocyanin content than all other treatments (Figure 3). Despite receiving approximately the same amount of light, the lettuce plants in this treatment accumulated more anthocyanins than those under the other filters or the white light control. While anthocyanin content in lettuce has been shown to increase with exposure to UV light (Park et al., 2007), increased blue light (Stutte, 2009) and increased light intensity (Zhang et al., 2018), the differences in blue light and total light intensity between the FI treatment and the other treatments were small (~15 μmol m-2 s-1 blue and ~70 μmol m-2 s-1 TPFD), and UV light was negligible in all treatments. This suggests that aspects of the FI transmission spectrum enhance anthocyanin content beyond what could be expected from the amount of blue light it transmits.
2.5 Gene expression networks identified genomic responses
A transcriptome analysis was conducted for each species to detect gene expression differences caused by the variation in filter spectra and light intensity in lettuce and tomato leaf tissue. Such analyses are often used to identify emergent traits caused by the combination of changes in the expression of the tens of thousands of genes in the genome that are not immediately apparent in the phenotype. To examine global patterns of gene expression in response to the filters, we used a network-based approach to identify distinct groups of co-expressed genes that shared a similar relationship with the filter-specific light spectra and were consistent when light intensity was varied. Gene networks were individually constructed for each species using an unsupervised machine learning approach. Datasets collected under varied TPFD were included to differentiate between genomic responses to altered spectrum from those caused by light intensity changes. The resulting networks were used to build a common consensus network for each species containing clusters of genes (i.e. modules) that are shared between the intensity-independent and intensity-dependent networks. The consensus networks identified 35 modules ranging in size from 39 to 3,656 genes in the cluster dendrogram for lettuce (Figure S8) and 43 modules ranging from 46 to 2,326 in tomato (Figure S9).
Genes within a given module are considered to have highly similar expression profiles. Each module is described by a single value called the module eigengene that represents the expression profile of the entire module. These module eigengenes were used to assess relationships between gene expression profiles and quantitative measures of light quality, such as TPFD, red to blue (R/B) ratio, red to far-red (R/FR) ratio. We identified clusters of genes that responded similarly to these parameters by comparing the sign of the correlation between the module eigengene and each quantitative measurement. These clusters or modules are unique to each species.
In lettuce, Module L2 (2,397 genes) showed a strong positive correlation with TPFD (r=0.9, p=1e-07, Figure 5), indicating that genes in this module are strongly influenced by small increases in light intensity. Module L12 (3,656 genes) also showed a strong correlation with TPFD but in the opposite direction (r=-0.8, p=3e-05). This suggests an inverse relationship between genes in Module L2 and Module L12, where higher values of TPFD are associated with higher expression of genes in Module L2 but lower expression in Module L12. The same two modules also had opposite correlations with the R/FR ratio. Module L2 was negatively correlated with the R/FR ratio, meaning that these genes tended to have higher expression when lettuce was grown under a lower R/FR ratio (r=-0.9, p=3e-06). However, when light intensity varied in addition to the spectrum, the correlation between Module L2 eigengene and the R/FR ratio was not significant (p=0.05). A similar pattern was seen in Module L12 where there was a positive correlation with the R/FR ratio (r=0.8, p=1e-05).
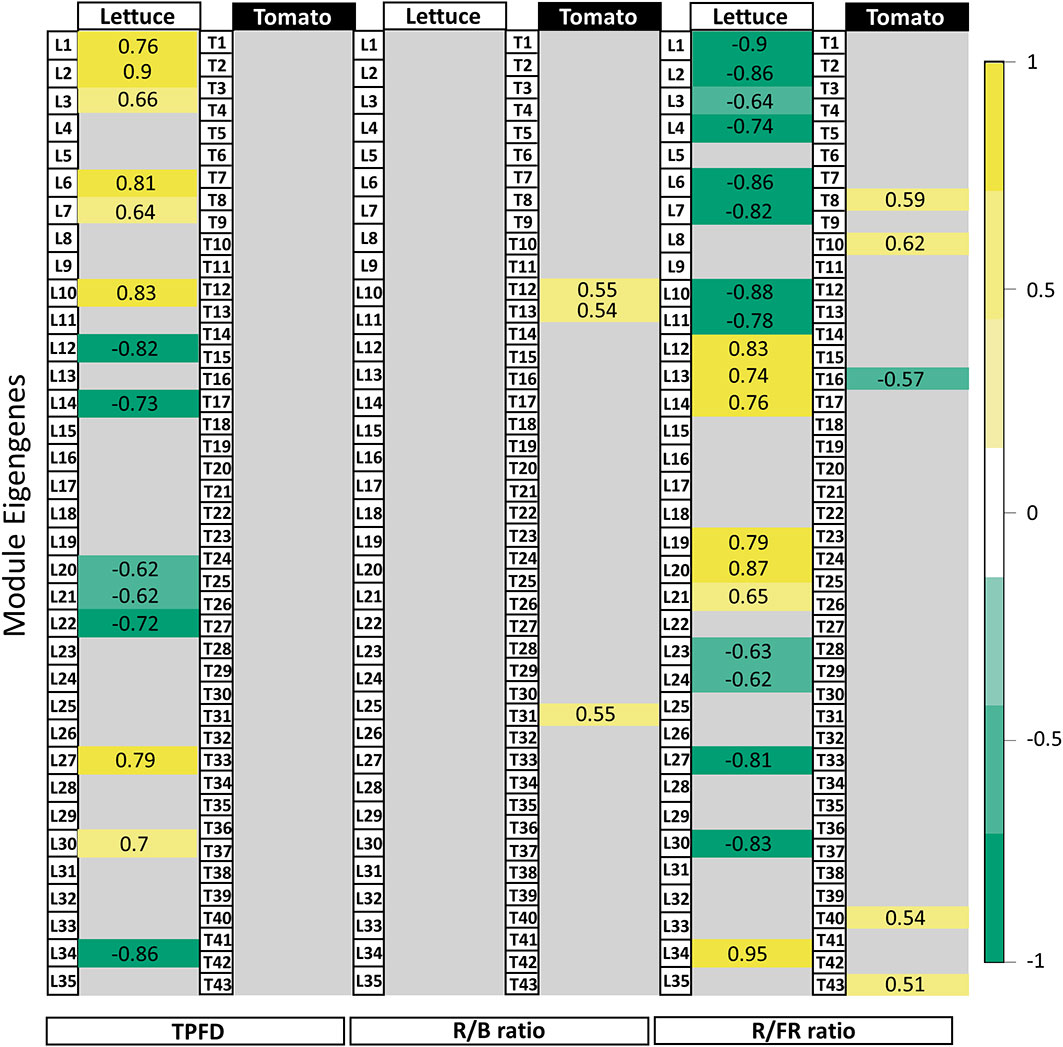
Figure 5 Module-trait relationships with selected light traits. Table of correlations between measured light parameters and the 35 lettuce modules and 43 tomato modules of genes identified in the network analysis. Strong positive correlations are dark yellow while strong negative correlations are dark green. Values given are the correlation coefficient (r) for each relationship. Although gene expression in lettuce varied in response to changes in TPFD, the same responses were not seen in tomato. The responses to light quality traits (i.e., R/B and R/FR ratios) were similarly variable between lettuce and tomato. Tomato gene modules correlated more strongly with the R/B ratio while the R/FR ratio had more influence on gene expression in lettuce. Modules showing weak or no significant correlation with a trait were filtered by applying a p-value threshold (p<1e-05). See Figures S8, 11–13 for the complete module-trait relationships for lettuce and tomato.
In tomato, 43 modules of genes with similar expression were identified (Figure 5). Surprisingly, the correlations between several modules and TPFD observed in lettuce were not observed in tomato. Rather, there were no statistically significant correlations between any modules of genes and TPFD when tomato plants were grown under OSC filter with the same light intensity. This suggests that the small differences in TPFD were not sufficient to modify gene expression in tomato. The lack of correlation with TPFD indicates that the experimental design of the tomato experiment was optimized by reducing the already small light intensity variation in the lettuce experiment. The tomato treatments are closer to each other in TPFD, which allowed for a clearer interpretation of the filters’ spectral effects on gene expression. Although gene expression of very few modules correlates significantly with the spectral traits, the correlations that do exist offer insight into the plant’s response to the modified OSC spectra. In particular, the representative eigengene of Module T12 (219 genes) had a positive correlation with the ratio of R/B light (r=0.55, p=0.02). This indicates that an increase in R/B ratio, such as that produced by the FP filter, increases expression of the genes assigned to this module.
2.6 Hub genes in lettuce and tomato
We focused on specific genes with expression patterns that were highly correlated with a particular module and its representative eigengene. In lettuce, Modules L2 and L12 were selected to investigate the R/FR dependence. One such hub gene in Module L2 is elongated hypocotyl 5 (HY5), which encodes a light-responsive transcription factor important for photomorphogenesis (Abbas et al., 2014). As expected of a hub gene, HY5-1 expression closely matched the pattern of both Module L2 eigengene expression and the overall expression of all Module L2 genes (Figure 6). Accordingly, HY5 expression was also negatively correlated with R/FR, suggesting that HY5 expression increases in response to lower R/FR ratios. Indeed, the treatment with the lowest R/FR ratio (Data S1), also had the highest expression of HY5-1. Conversely, a high affinity nitrate transporter 2.1 gene (NRT2.1-1, LOC111883156), a hub gene of Module L12 (kME=0.9, p=2e-06), had an opposite expression pattern with the lowest expression in FI, compared to the other treatments. Surprisingly, HY5 has been identified as an enhancer of NRT2.1 in roots and is expected to increase, not decrease, expression of its target, a gene important for nitrate uptake and nutrient acquisition (Chen et al., 2016).
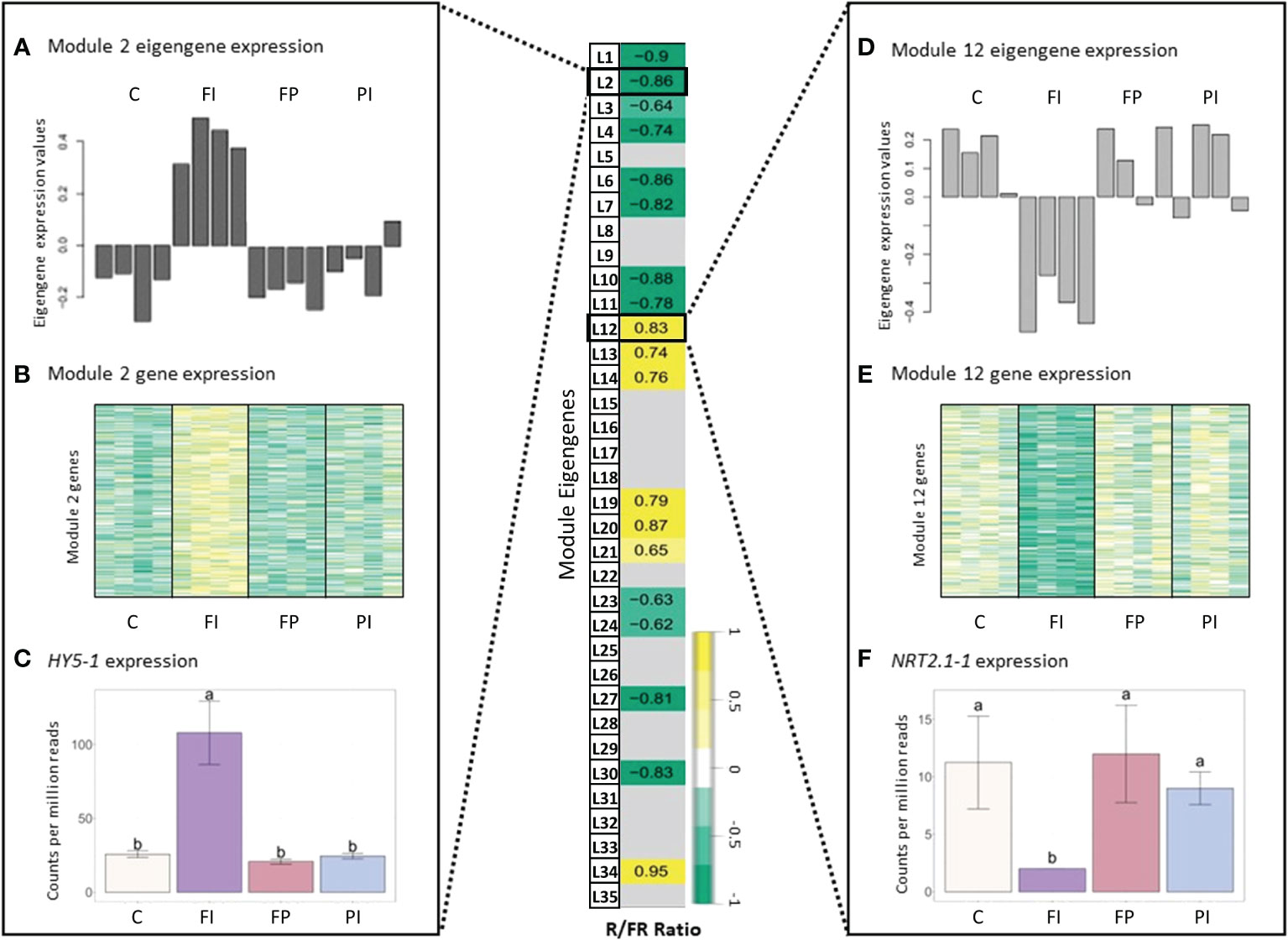
Figure 6 Gene expression in lettuce correlated with R/FR ratio. Module-trait relationships and differential gene expression between lettuce filter treatments and control. (A) Normalized expression of the Lettuce Module 2 eigengene expression for each of the treatments by sample. Table values given are the correlation coefficient (r) for each relationship. Insignificant correlations were removed (p<1e-05). Error bars indicate the standard deviation. Letters indicate significance from ANOVA and Tukey test (p<0.05). See Figure S13 for full table. (B) Heatmap of gene expression for all genes in Lettuce Module 2, where dark yellow indicates a strong increase in expression and dark green indicates a strong decrease in expression. (C) Normalized gene expression of a Lettuce Module 2 hub gene, elongated hypocotyl 5 (HY5-1). (D–F) Eigengene expression, gene expression and hub gene expression (high-affinity nitrate transporter 2.1, NRT2.1-1) for Lettuce Module 12. The treatment with the lowest R/FR ratio, FI, had higher gene expression in Lettuce Module 2, resulting in a negative correlation with the R/FR ratio. In contrast, Lettuce Module 12 had a positive correlation with R/FR ratio due to the downregulation of expression in FI, relative to the other treatments.
A gene that negatively regulates flowering in tomato, self pruning 5G (SP5G) (Zhen and Bugbee, 2020), had a strong correlation with the Module T12 eigengene (kME=0.9, p=3e-07) and was, therefore, a good candidate hub gene (Figure 7). This module had a positive correlation with the R/B ratio. Expression of SP5G increased under the FP filter, which had a relatively high R/B ratio (4.5), and decreased under the FI filter with its relatively low R/B ratio (2.8), although this decrease was not statistically significant. The same correlation between Module T12 and the R/B ratio was not seen when light intensity varied (Figure S10). While FP had the highest expression levels as expected, SP5G expression in the FI treatment was not significantly lower than the high light control.
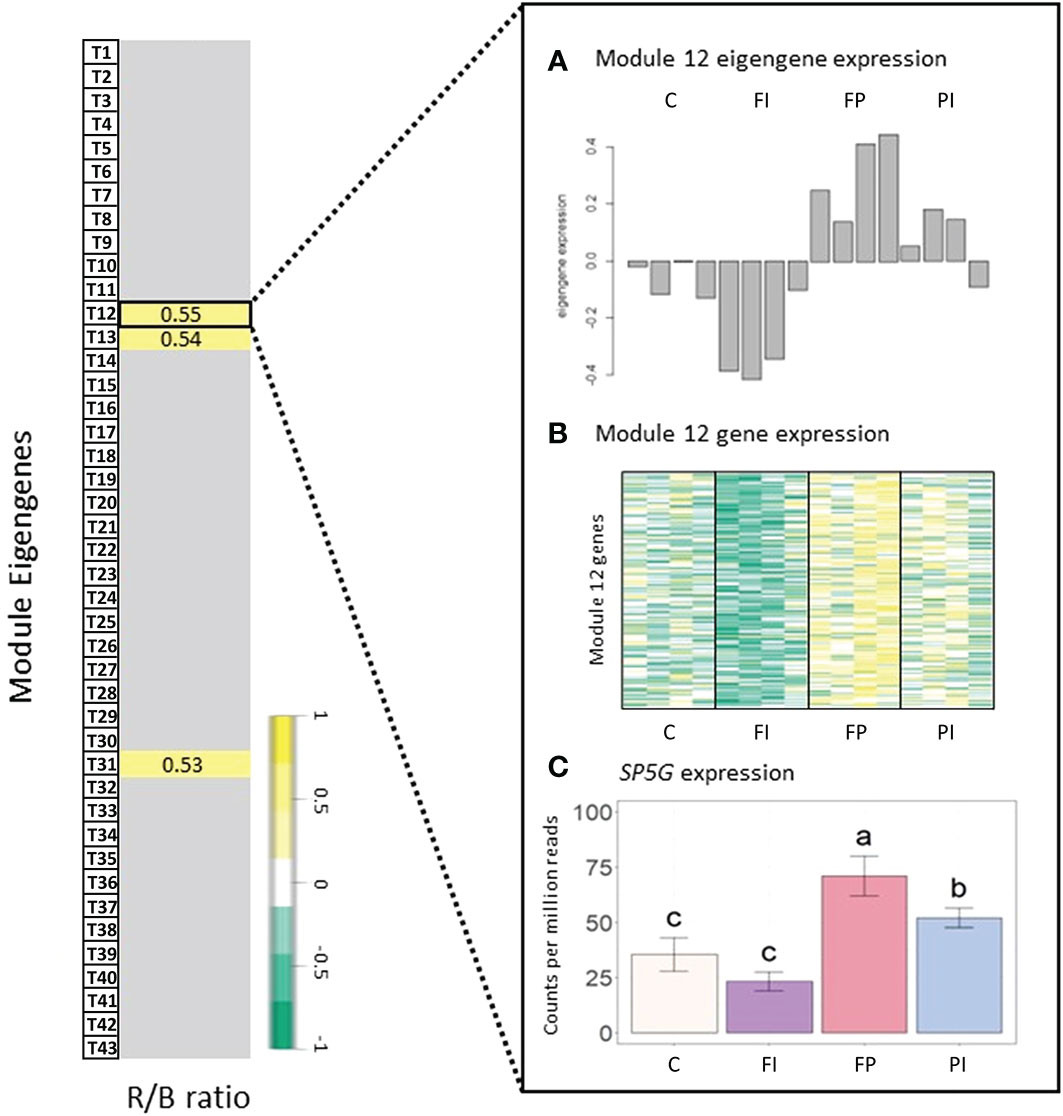
Figure 7 Gene expression in tomato correlated with R/B ratio. Module-trait relationships and differential gene expression between tomato filter treatments and control. (A) Normalized expression of the Tomato Module 12 eigengene expression for each treatment by sample. The correlation coefficient (r) is given for each relationship. Insignificant correlations were removed (p<0.05). See Figures S14, S15 for full module-trait relationships tables. (B) Heatmap of gene expression for all genes in Tomato Module 12, where dark green indicates a strong increase in expression and dark yellow indicates a strong decrease in expression. (C) Normalized gene expression of a Tomato Module 12 hub gene, self pruning 5G (SP5G). The treatment with the highest R/B ratio, FP (FTAZ : PCBM filter), had higher gene expression in Tomato Module 12, resulting in a positive correlation with the R/B ratio. This correlation is exemplified in a hub gene of Tomato Module 12: SPG5. Error bars indicate the standard deviation. Letters indicate significance from ANOVA and Tukey test (p<0.05).
The pattern of expression of SP5G corresponds with the timing of flowering observed. The FI treatment had lower expression of this floral repressor (Data S3). Although flowering data collection was limited by the size restrictions within the growth boxes, our observations indicate that the FI treatment flowered one day earlier than the control treatment (Data S2). In contrast, the FP treatment had high expression of SP5G, and limited data indicated flowering was delayed by one day, relative to the control (Data S2, S3). SP5G is thought to be downstream of both the red light-sensitive photoreceptor, phyB1 (Cao et al., 2018), and the blue-light sensitive photoreceptors, cry1a and cry2 (Fantini et al., 2019). This provides a potential mechanism for the sensitivity of this gene to the differing R/B ratios in the filter treatments. The characterized relationship between phyB1 and SP5G expression also suggests a correlation between gene expression and the R/FR ratio that directly impacts the activation state of phytochromes. A lower R/FR has been shown to increase expression of SP5G and delay tomato flowering (Cao et al., 2018). While the network analysis did identify a correlation between SP5G expression and R/FR ratio, it was a weaker correlation with the opposite sign expected from the literature (GS=0.58, p=0.02).
2.7 Gene expression patterns vary between lettuce and tomato
A differential gene expression analysis was performed to contrast each filter treatment against its respective control to quantify changes in the expression of individual genes. In lettuce, few differences were observed for all filter treatments relative to the control except FI. There were 1,143 differentially expressed genes (DEGs) identified in the FI/C comparison but only 15 DEGs in FP/C and 12 DEGs in PI/C (Figure 8A). While filter degradation did result in changes in TPFD by the end of the experiment (Figure S3), this small variation in light intensity alone can hardly account for the large differences between the FI treatment and the other treatments. As reported earlier, gene expression of several large modules of genes correlated with the R/FR ratio as well as TPFD in this experiment. This suggests that the R/FR ratio and possibly other spectral characteristics unique to the FI filter resulted in differential gene expression. In particular, HY5-1 gene expression was increased by a log2-fold change of 2.2 in FI, which is consistent with a previous study that found that HY5 expression increased with lower R/FR ratios (van Gelderen et al., 2021). Furthermore, HY5-1 expression was much higher in plants grown under the FI filter than in plants grown under higher intensity, unfiltered light, demonstrating that changes in the expression of this key gene cannot be explained by variations in light intensity (Figure S11). Because HY5 is a transcription factor that controls expression of many downstream genes, it is not surprising that many of these genes were also differentially expressed in the FI treatment. Among these were several genes involved in side chain modification of anthocyanins that were upregulated relative to the control, including anthocyanin 3-O-glucoside-6”-O-malonyltransferase (Table S1). While many of the genes in the anthocyanin biosynthesis pathway were not differentially regulated on a statistically significant scale, these genes involved in the modification of anthocyanins into more stable forms were upregulated (Saigo et al., 2020; Suzuki et al., 2002) (Table S1; Data S3). The increase in expression of these genes correlates with the increase in anthocyanin concentration seen in Figure 3, where the FI treatment had a significantly higher concentration than all other treatments. Other genes known to be directly or indirectly regulated by HY5 were also upregulated, including several related to nitrate uptake, defense against predation and the circadian clock.
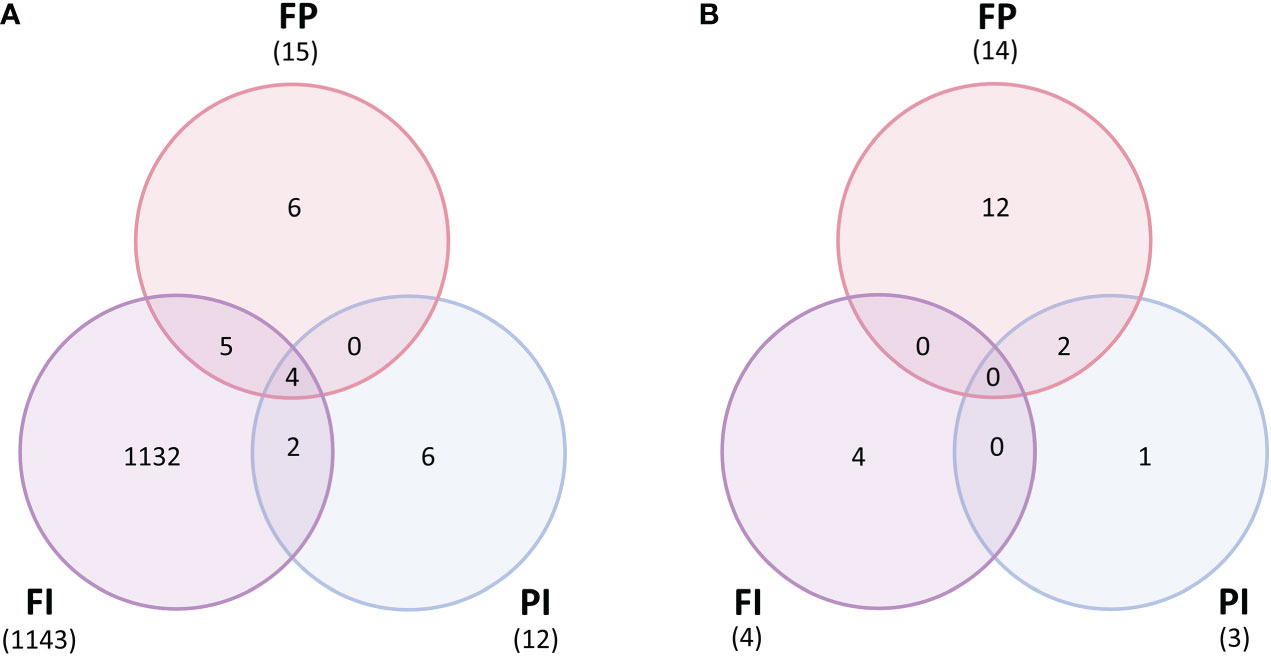
Figure 8 Differentially expressed genes between filter treatments and control. (A) Lettuce normalized gene expression (counts per million) contrasted between each treatment for each filter (FI, FP and PI) and the control (C) (p<1e-05). Lettuce grown under the FI filter had thousands of differentially expressed genes relative to the control, while the FP and PI treatments both had fewer than twenty DEGs each. (B) Tomato normalized gene expression (counts per million) contrasted between each treatment for each filter (FI, FP and PI) and the control (C) (p<1e-05). Very few genes were differentially expressed between each light treatment in tomato, with the majority found in the FP/C contrast.
However, there were very few differences between the tomato filter treatments and the control. There were only 4 DEGs in the FI/C contrast, 3 in the PI/C contrast and 14 in the FP contrast (Figure 8B). Unlike in lettuce, the FI filter did not cause a dramatic expression profile difference relative to the control. The FP filter had a greater impact on the tomato genome, although this was only a few genes more than either FI or PI.
Many of the differentially expressed genes observed in the lettuce experiments were not seen in tomato. The expression of the transcription factor, HY5-1, gene was upregulated in lettuce plants grown under the FI filter. In particular, the FI treatment had higher HY5-1 expression than all other treatments. HY5 mediates light-responsive signals by regulating a diverse range of downstream genes, including NRT2.1-1 (Gangappa and Botto, 2016). The interaction between light, HY5 and downstream targets such as NRT2.1-1 points to modification in nutrient uptake and accumulation driven by the spectrum FI OSC filter in lettuce. However, this interaction was not observed in tomato. Expression of HY5-1 was not significantly higher in the tomato FI treatment compared to the control (Figure S12). Furthermore, there were zero counts of NRT2.1 expressed in sampled tomato leaf tissue. It seems likely that the expression of this nitrate transporter is restricted to root tissue in tomato, which was not analyzed here.
Among the genes that were differentially expressed between OSC filter treatments and the white light control in tomato, the flowering regulators SP5G and GIGANTEA (GI) were upregulated in FP and both FP and PI, respectively (Figure S12; Table S2). GI, in particular, plays many roles in regulating the circadian clock and various light-responsive processes and is downstream of phyB (Nohales et al., 2019). The expression of GI increased with both the high relative amounts of blue light in the PI filter and the high relative amounts of red light in the FP filter. Additionally, genes involved in diverse pathways such as response to low sulfur 3-like, inositol-1,4,5-triphosphate-5-phosphatase (5PT1) and terpene synthase group gene, TPS12, were differentially expressed in response to spectral changes alone (Figure S12).
The expression of the photoreceptors and downstream genes in tomato had patterns different from those seen in lettuce as well (Figure 9). While the activity of photoreceptors as enzymes post-translation is most commonly discussed in studies on plant light responses, photoreceptor gene expression is also responsive to light conditions (Sharrock and Quail, 1989; Jain et al., 2007; Luo et al., 2013; Tian et al., 2019). The gene expression of phytochrome A (phyA), which encodes one of the red light photoreceptors, had the most variation in lettuce and tomato. Phytochrome expression is known to increase in response to high levels of red light, improving the plant’s ability to perceive these wavelengths of light (Tian et al., 2019). Accordingly, this gene was more highly expressed in the FP treatment, although only in tomato. There was less variation in the gene expression of other photoreceptors, which also have a wavelength-sensitive increase in expression (Jain et al., 2007; Luo et al., 2013).
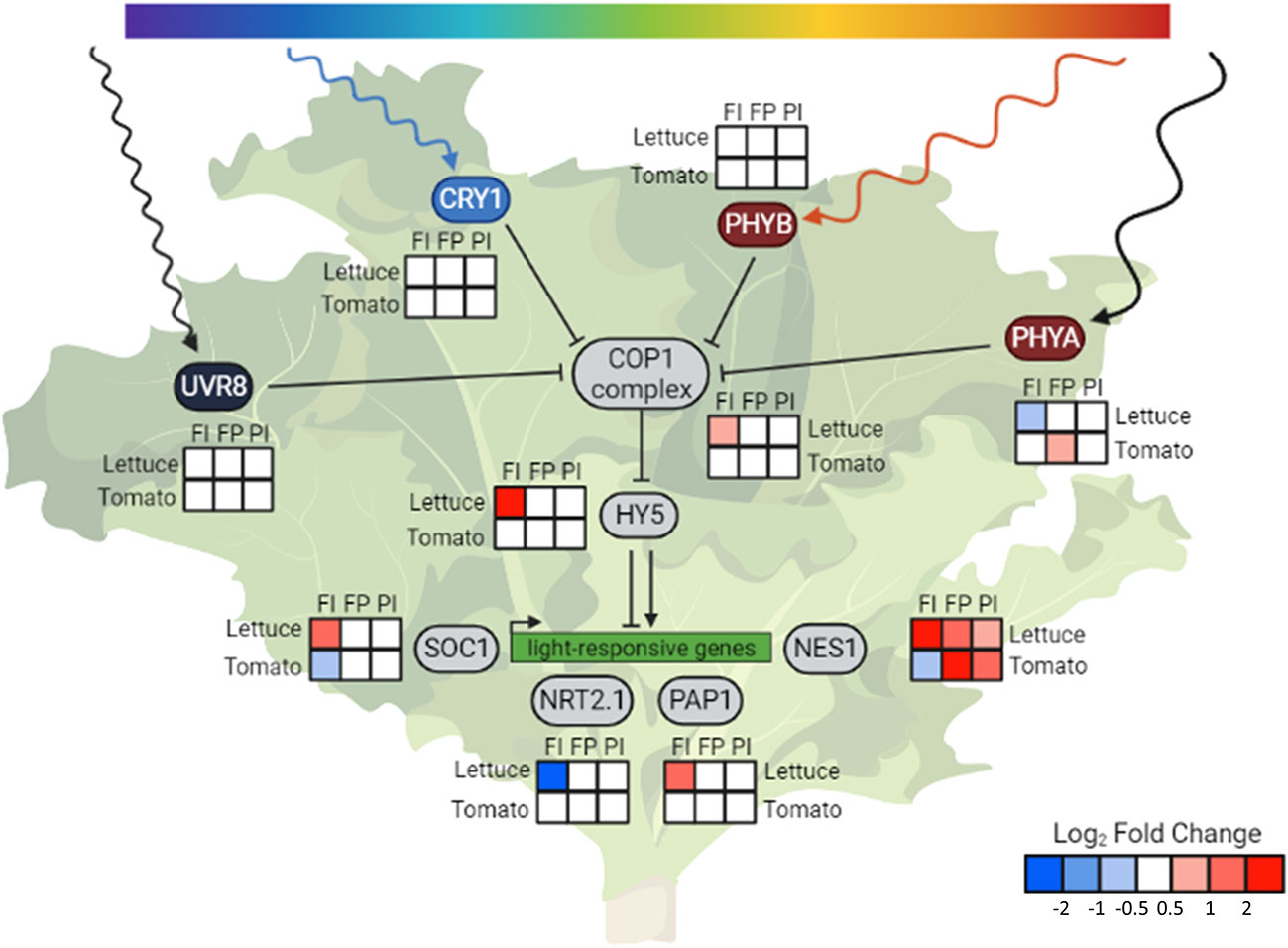
Figure 9 HY5 integrates light signals and regulates downstream genes. Condensed photoreceptor signaling pathway with expression of downstream genes of interest in lettuce and tomato. The photoreceptors indirectly upregulate expression of HY5 through inhibition of the constitutive photomorphogenic 1 (COP1) complex formation. Elongated hypocotyl 5 (HY5-1) is a transcription factor that is a known or hypothesized regulator of many light-responsive genes, including suppressor of overexpression of CO 1 (SOC1), high affinity nitrate transporter 2.1 (NRT2.1-1), production of anthocyanin pigment1/MYB75 (PAP1) and (3S,6E)-nerolidol synthase 1 (NES1-1). COP1 itself was differentially expressed in response to light quality changes in lettuce but not tomato. The changes in COP1 expression are in the opposite direction of many of the trends observed in phyA1 expression, indicating gene-level regulation of this gene. Despite a lack of differential expression of COP1 and HY5, expression of downstream genes, such as NES1, were altered in tomato, indicating another method of light regulation. Relative transcript abundance is represented by log2 fold changes between specified treatments. Created with BioRender.com.
The phytochromes, along with the cryptochromes, inhibit the formation of the complex that COP1 ubiquitin ligase forms with suppressor of phyA 105-1 (SPA1), which prevents the degradation of transcription factors such as HY5 and leads to photomorphogenesis (Abbas et al., 2014). Our analysis revealed that, in addition to the photoreceptors, COP1 itself was differentially expressed in lettuce in response to both light quality changes and intensity changes. The changes in COP1 expression are in the opposite direction of many of the trends observed in phyA expression. The expression of several genes downstream of COP1 was also altered in response to changes in spectrum and the overall amount of light in lettuce. One of these genes is suppressor of overexpression of CO 1 (SOC1), which encodes a transcription factor that regulates flowering and has been identified as a putative target of HY5 (Lee et al., 2007; Lee and Lee, 2010). Production of anthocyanin pigment 1/MYB75 (PAP1) is a transcription factor that regulates anthocyanin accumulation, and whose gene expression is also directly regulated by HY5 (Gangappa and Botto, 2016).
While many of these downstream changes were seen only in lettuce, altered expression of a volatile-producing gene was observed in both species (Figure 7). (3S,6E)-nerolidol synthase 1 (NES1-1) gene expression increased in response to both light intensity and altered spectrum. While it has not been demonstrated that NES1 is directly regulated by HY5, it is a known regulator in the terpenoid synthase pathway of which NES1 is a member (Michael et al., 2020). NES1 encodes an enzyme that produces nerolidol, a volatile compound released by the plant in response to wounding by herbivorous insects. In lettuce, NES1 was upregulated in response to all three OSC filters. In tomato, only the FP and PI filters resulted in an increase in NES1 expression, while the FI treatment showed lower expression.
3 Discussion
Our experiments were designed to account for both the quantitative and qualitative aspects of OSC-filtered light on lettuce and tomato as representative crops. Despite the morphological similarities between treatments, the physiology of the plants was altered by the differences in light quality under the ST-OSC filters on a molecular and transcriptomic level. These molecular tools enable us to predict physiological responses that can now be tested to further improve the productivity and sustainability of crops to be grown in ST-OSC greenhouses and ultimately breeding or engineering of crops to specifically optimize their performance.
3.1 Transcriptome analysis identified physiological and metabolic changes
The transcriptome analysis was a screening tool for the many aspects of plant growth and development that could not be measured directly. Beginning with the photoreceptors that sense light, expression of many genes was altered by changes in either light intensity or light quality, or both (Figure 9). The expression patterns seen in the photoreceptors reflect the light environment of the plants. There was minimal variation in UVR8-1 expression and negligible UV light, while there was greater variability in phyA that corresponded to the variability in red light between treatments (Table 1). That COP1 expression increased while phyA expression decreased in the FI treatment in lettuce may indicate that the expression of COP1 in lettuce, in addition to activity of its protein, is regulated by light. A common theme of the transcriptome analysis in lettuce was the differential expression of HY5, a light-regulated gene that encodes a transcription factor that controls the expression of many genes that drive photomorphogenesis and is regulated by the COP1 complex. HY5 was differentially expressed in response to altered spectrum and altered light quantity only in lettuce, leading to many downstream changes. Among these, PAP1 expression mirrored the trends seen with HY5, providing a genomic basis for the increase in anthocyanin content seen in the lettuce FI treatment.
In contrast, tomato lacked this differential expression of HY5, and expression of many downstream targets showed distinct trends (Figure 9). NES1 expression was upregulated in the lettuce FI treatment, relative to control, while it was downregulated in tomato under the same filter. In tea plants, nerolidol has been shown to attract predator insects, protecting the plants from further damage by reducing the number of pests (Naskar et al., 2021). This varied response may indicate that the pest response of different crop species can be modulated with the selection of OSC device selected for production.
3.2 Impact of OSC-altered spectra on flowering
The upregulation of SOC1 in lettuce grown under the FI filter relative to the control with similar light intensity suggests that this spectrum may trigger an earlier flowering time. The regulation of flowering is an important aspect of crop development that can impact harvest. In particular, the prevention of early flowering in lettuce can improve harvests by extending the growing period before the lettuce begins to bolt, altering its metabolite and flavor profile (Hao et al., 2018). Although this would be undesirable in several crop species, this effect was not seen in the other OSC filters tested, which yielded similar biomass. This is an example of the importance of considering the transmission in the 400-750 nm range when selecting OSCs for greenhouses.
In tomato, probable transcription factor SP5G is a known repressor of the start of flowering (Lee and Lee, 2010). Despite timing of the RNA tissue sampling for the genomic analysis after the initiation of flowering, elevated expression levels of SP5G were detected in combination with the delayed flowering in the FP treatment. The light spectrum of the FP filter is similar to the spectrum of the commercial OSCs used in a greenhouse tomato experiment (Waller et al., 2021). The tomato plants in this experiment also experienced a reduced amount of blue light relative to red, in addition to a decrease in the R/FR ratio. Although the authors did not report a delay in flowering, the first three fruit harvests of the indeterminate tomato plants were lower in yield compared to the control. Taken together, this suggests that the FP filter and other OSC filters with similar spectral profiles may have a negative effect on flowering crop production, similar to the potential negative impact on bolting in lettuce associated with the FI filter. On the other hand, FI, the treatment with a more balanced B/R ratio that flowered one day before the control had the lowest levels of SP5G. Although SP5G was not significantly differentially expressed between this treatment and the control, this may indicate that the FI filter still holds some advantage over others in promoting beneficial agronomic traits in tomato and similar high-light species.
3.3 Impacts from OSC spectra on lettuce nutritional content
Crops rich in anthocyanins are more nutritious and have a positive effect on human health when included in the diet (Cerletti et al., 2017). Anthocyanin content in red cultivars of lettuce has been shown to increase in response to increased light intensity (Zhang et al., 2018) and altered spectrum (Stutte, 2009; Sng et al., 2021). The increase in PAP1 expression provided a genomic and molecular basis for the increase in anthocyanin content seen in the lettuce FI. While many of the genes in the anthocyanin biosynthesis pathway were not differentially regulated on a statistically significant scale, several genes involved in the modification of anthocyanins into more stable forms were upregulated (Suzuki et al., 2002) (Table S1; Data S3). This suggests that the primary mode of anthocyanin accumulation in lettuce may have been through the stabilization of anthocyanins rather than novel biosynthesis. Although the lettuce FI treatment did have a somewhat higher amount of light than other PC treatments, there is reason to believe that the increase in anthocyanins could be the result of the FI spectrum alone. The FI treatment had a wavelength profile with higher amounts of blue and red light and lower amounts of green relative to the other treatments (Table 1), and anthocyanin content has been shown to increase under red and blue light (Stutte, 2009).
While nitrate is required for growth and must be supplied in fertilizer, high nitrate content in lettuce leaves is an important consumer concern with human health effects (Li et al., 2021). Several nitrogen transporter genes that allow the plant to take up nitrogen from the soil and move it within the plant were differentially expressed in the FI treatment relative to control, including NRT2.1-1 (Figure 9; Table S1). Surprisingly, expression of NRT2.1 was downregulated when HY5 was upregulated, although HY5 is typically considered to be a positive regulator of NRT2.1 (Chen et al., 2016). A recent study has demonstrated that HY5 can act as a negative regulator of NRT2.1 under certain conditions (van Gelderen et al., 2021). It should also be noted that much of the research on NRT2.1 is focused on nitrate uptake from the soil and is primarily analyzing expression in root, not leaf, tissue. The downregulation of nitrate transporters in leaf tissue may indicate that nitrate is selectively reduced in the roots instead of the leaves and accumulates less in the leaves. Additionally, the decrease in nitrate reductase (NR) expression could indicate lessened demand for the conversion of nitrate () to nitrite () in the leaves (Figure S11). These changes may correlate with desirable low nitrate levels in leaf tissue and improved nutrition. Nitrate levels in lettuce have been shown to vary in response to different light intensities (Fu et al., 2017). Nitrogen use efficiency and fertilizer requirements may also be affected. Because fertilizer uptake and nitrogen content were not quantified in this study, further experimentation is needed to confirm these changes.
A limitation of our experimental design was the variation in light intensity between treatments, especially in the lettuce experiment. Although this is not unlike real world variation that would result from differences in filter transmission or varied shading from bench to bench in the greenhouse, the changes in TPFD made it more difficult to distinguish between changes that were solely due to the filter spectra. A combinatorial study, where treatments are compared to others that vary in only filter or TPFD and not both at once, would more clearly distinguish between light intensity and light quality effects. Such a study could better predict the changes in crop growth and yield caused by general OSC shading as well as the effects that would vary based on the transmission of the active layer. The many potential metabolic changes that were suggested by differential gene expression but not quantified, especially changes in nitrogen acquisition, fruiting and herbivory defense in other important greenhouse crops should be investigated. Additionally, recent modeling of plant growth and energy harvesting in OSC-greenhouses have identified a number of promising organic semiconductor active layers, which includes the FTAZ : IT-M (FI) and PTB7-Th : IEICO-4F (PI) systems studied here (Ravishankar et al., 2022). Further study of OSC active layers, particularly under natural sunlight, would validate the model’s ability to identify materials that can produce economically viable crops. More broadly, a combinatorial transcriptomic approach to the study of plant light responses in general could yield valuable insight into the signal integration between different aspects of light quality and light intensity.
3.4 Future directions
Our experiments presented here offer molecular insight into plant growth and development under OSC filters. We found no negative impacts on the accumulation of biomass or on the quantified secondary metabolites when light intensity was controlled. The differential gene expression, especially the upregulation of key regulator genes under the FI filter in lettuce and the FP filter in tomato, is worthy of further study to discover how these changes translate to important aspects of crop production. In particular, the gene expression changes related to the initiation of flowering pointed to economic impacts for crops like lettuce, where early flowering can damage harvests by introducing a bitter taste, and tomato, where improved fruit development can increase yields. The advantage of a transcriptome analysis in the study of OSC-grown plants is that these key modifications can be identified without the need to directly measure each aspect of plant growth and development.
A major limitation to commercialization of ST-OSCs in greenhouses is filter fading. This issue is currently being addressed, and we expect to develop commercially meaningful lifetimes for OSC filters in the near future. This will enable scale-up production and commercialization. To have a meaningful impact on sustainable food production, these ST-OSC greenhouses also need to be able to produce a larger variety of crops in different climate zones. While we have modeled the potential for economic value with some crops (Guo et al., 1998), lower material costs and higher efficiency will provide a path to not only grow locally desirable vegetables from strawberries and beans to eggplants, but also row crops such as corn, wheat and root vegetables. Most of the improvements are expected to come from OSC efficiency increases, better materials and different systems like flexible OSC shades that can be used in greenhouses in a similar, more temporary way as current shading techniques and paint are applied.
In addition to the material science and battery storage improvements that can be anticipated, breeding or genetic engineering of crops that are specifically adjusted to these modified growth conditions can be considered. Many of the traits that evolved through natural selection or were bred for field crops are no longer required in controlled environment agriculture. Stress response mechanisms in plants for survival in varying biotic and abiotic environments often reduce yield. When the environment can be controlled, those yield-reducing stress responses are no longer required and can be removed through conventional breeding or engineering (Folta, 2018).
The magnitude of intensification of agricultural crop production in these net zero energy greenhouses would not only contribute to an increased food demand even on marginal land, but it can also spare land, so it can be converted to other ecosystems, which could provide income from carbon credits through reforestation or other ecosystem services. Future research focuses on improving ST-OSC stability and testing of other crop systems for productivity and sustainability traits in ST-OSC GreenERhouses.
4 Materials and methods
4.1 Experimental design
Red oak leaf lettuce (Lactuca sativa) was grown with adjustments made to the height of the growth boxes so that each treatment received similar light intensity in addition to the consistent height, variable intensity setup previously described (Ravishankar et al., 2021). Eight lettuce plants from each treatment were harvested at 21 days post germination (transplant stage) and the remaining eight plants from each treatment at 35 days post germination (harvest stage). Four plants from each harvest per box were used for biomass measurements, while the remaining four were used for tissue sampling. Tomato plants (Solanum lycopersicum cv. Moneymaker) were grown under these conditions with modifications. Seeds were sown on rockwool and germinated in the same growth chamber with metal halide and incandescent lighting to approximate natural sunlight. Eight seedlings of uniform size and age were selected and transplanted into individual blocks of larger rockwool and moved inside the treatment boxes with nearly 100% OSC roof coverage. Tomato plants were harvested after flowering, 30 days past the two-leaf stage when plants were moved under the filters.
The growth boxes were covered with either an OSC filter, a clear glass or a shaded control on top to simulate a greenhouse roof. The positions of the rockwool blocks were rotated to avoid positional light effects as in the lettuce experiment. The consistent light intensity between treatments allowed for comparison of the influence of the light spectra on plant physiology. The results of these experiments were compared to the previously reported experimental design where all filters were positioned at a consistent height to model the roof of a greenhouse and therefore produce different TPFD due to the differences in filter transmission. Five replications of the lettuce experiment with consistent light intensity were conducted. One replication was conducted of both tomato experiments, using consistent and variable light intensity. Lettuce light conditions were measured as previously reported. Reported percent colors for tomato were measured using a spectrophotometer (Black Comet-SR, Stellar Net, Inc., USA) except the high light control treatment, which was assumed to have the percent colors of the low light control. PPFD was measured using a quantum sensor (LI-190R, LI-COR, Inc., USA) and TPFD was calculated from PPFD and percent colors.
4.2 Filter fabrication
OSC filters were made as previously described (Ravishankar et al., 2021). Solutions of the organic semiconductor active layers were wire bar-coated onto glass substrates. A second sheet of glass was adhered under heat to each of the glass substrates with ethylene vinyl acetate films for encapsulation. Optical epoxy (Norland 63) was cured around the edge of the filter stack as an additional seal. Twelve of these filters, each 20x10cm, were arranged in a single layer above a layer of PEDOT : PSS (PH1000, Hareus) coated onto a PET substrate to simulate the transmission of full OSC devices for each filter treatment.
4.3 Biomass measurements
Measurements of fresh weight, dry weight, leaf area and leaf number were collected as previously described at Day 21 and Day 35 after germination for lettuce and at Day 30 for tomato (Ravishankar et al., 2021). The 21-day early harvest corresponds to the age when young lettuce is typically transplanted. Both fresh and dry weights are above ground measurements that do not include root tissue. Dry weight was measured after leaves were dried at 65°C for three days. Leaf area was measured by leaf meter (LI-3000, LI-COR, Inc., USA) and summed per plant. Leaf number was also summed by plant and excluded any emerging leaves less than 1 cm in length. Additional measurements were taken of the tomato plants. Height was measured from the top of the rockwool block to the highest point of the plant. Visible flower buds and open flower buds were recorded per plant after initiation of flowering and at harvest. The number of leaflets was counted per plant. Height and leaf number were collected for all eight plants in each treatment. All other biomass measurements were collected for the four tomato plants per treatment not used for tissue sampling.
4.4 Extraction and quantification of secondary metabolites
Secondary metabolites were extracted from ground frozen lettuce leaf tissue as previously described (Sims and Gamon, 2002; Ravishankar et al., 2021). Leaf tissue was collected from four harvest stage plants and immediately frozen in liquid nitrogen and stored until ground. Ground frozen leaf tissue was weighed and suspended in extraction buffer. A BioTek Synergy HT microplate reader (BioTek Instruments, USA) was used to measure absorbance.
4.5 Photosynthetic data collection
A LI-6400XT (LI-COR, Inc., USA) was used to collect photosynthetic data from lettuce as previously described (Ravishankar et al., 2021). Two sample measurements were collected per leaf, two leaves per plant and four plants per treatment beginning five days before the final harvest. Photosynthesis was measured in situ inside the growth boxes to observe the impact of the light intensity and spectrum created by the OSC filters. The chamber door was kept closed during data collection to minimize changes to the environment and a black cloth was used to block ambient white light from entering around the equipment. A CO2 scrubber was used to prevent elevated CO2 levels from researcher exhalation. PPFD was monitored as measured by the instrument to ensure lighting conditions remained consistent throughout the data collection for each treatment. Photosynthetic data collection was limited in tomato due to experimental constraints.
4.6 RNA extraction
Mature leaf tissue was collected from four plants per treatment in one replicate and ground in liquid nitrogen. The PureLink RNA Mini on-column kit with TRIzol (ThermoFisher Scientific, Inc., USA) was used to extract total RNA. An on-column DNAse treatment with additional off-column DNAse I treatments were used to remove DNA contamination. The mRNA library preparation and sequencing were performed by BGI Genomics Co., Ltd. (Shenzhen, China) with polyA selection by an oligo dT library. All 32 samples were multiplexed, pooled and loaded together. Sequencing was conducted on a DNBSEQ™ Technology Platform.
4.7 Transcriptome analysis
Raw reads were checked for quality standards using FastQC (v. 0.11.9) (http://www.bioinformatics.babraham.ac.uk/projects/fastqc/) and only high-quality read pairs (base score above Q30) were subject to downstream processing. Read pairs were aligned to the L. sativa cv. Salinas RefSeq genome assembly version 7 (genome ID: 5962908) or S. lycopersicum cv. Heinz 1706 RefSeq genome assembly (RefSeq GCF_000188115.4) using HISAT2 (v. 2.2.1) with default parameter settings (Kim et al., 2015). Genes with multiple copies undifferentiated in the genome annotation were assigned numbers in the order they are referred to in the text (e.g., LOC111908039 as HY5-1). Mapped reads were assigned to genomic features based on Lsat_Salinas_v7 or S. lycopersicum RefSeq assembly annotations using featureCounts (v. 2.0.1) (Liao et al., 2014). Read counts were summarized at the gene level and zero-count genes were removed prior to further analysis. Raw data and counts have been deposited in NCBI’s Gene Expression Omnibus (Edgar et al., 2002) and are accessible through GEO Series accession numbers GSE180179 and GSE200978 (https://www.ncbi.nlm.nih.gov/geo/query/acc.cgi?acc=GSE180179; https://www.ncbi.nlm.nih.gov/geo/query/acc.cgi?acc=GSE200978).
Differential expression analysis was performed independently for each species in R using the edgeR package (v. 3.34.0) (Robinson et al., 2010; McCarthy et al., 2012). The estimateGLMCommonDisp function was used to estimate a common gene-wise dispersion parameter suitable for all genes and evaluated on an individual basis and likelihood ratio tests were performed to test for differential expression of genes within pairwise treatment groups. For each test, a single treatment (OSC filter) group was compared to the control (clear or shaded glass) treatment and significance was evaluated based on the Benjamini Hochberg adjusted p-value (threshold of FDR<0.05). A second round of analysis was performed by comparing each treatment with the corresponding treatment with variable light intensity.
4.8 Network analysis
Normalized read counts were extracted using the edgeR cpm function and log-transformed counts per million were used as input for weighted correlation network analysis (WGCNA) (v. 1.69) (Langfelder and Horvath, 2008; Robinson et al., 2010; Langfelder and Horvath, 2012). To reduce spurious correlations, genes with consistently low expression (less than two read counts) for four or more samples were removed prior to analysis. With the remaining genes, expression similarity was calculated using the Pearson correlation metric, and a signed adjacency matrix was constructed using a soft-threshold power of 7 for the lettuce dataset and 14 for the tomato dataset to satisfy the scale-free network topology criterion. The network adjacency matrix was then used to calculate the topological overlap for each of the datasets separately. Average linkage hierarchical clustering was performed on the topological overlap dissimilarity matrices, and modules were detected using the dynamic tree cutting algorithm. The resulting network for each species was compared to networks constructed with transcriptome data where both light intensity and quality varied for each species. Topological overlap measures were used to scale these light intensity-dependent networks to the networks with consistent light intensity prior to consensus module detection.
4.9 Statistical analysis
Physiological data were analyzed by ANOVA and Tukey post-hoc test where p<0.05. Treatments that share a letter were not significantly different. Physiological data reported in the body of this report were collected from the same replication of the experiments used in the transcriptome analysis. Photosynthetic data were further analyzed by dividing by the PPFD recorded at time of measurement to identify potential effects of small changes in light intensity introduced by filter degradation. To minimize variation between lettuce replications, the biomass and secondary data were normalized relative to the control treatment within each round. Lettuce biomass and secondary metabolite data were normalized by replicate relative to their respective controls. Two replicates were performed simultaneously in the same growth chamber and were normalized together. These normalized data are presented in the supplementary information.
Data availability statement
The datasets presented in this study can be found in online repositories. The names of the repository/repositories and accession number(s) can be found below: https://www.ncbi.nlm.nih.gov/, GSE180179, https://www.ncbi.nlm.nih.gov/, GSE200978.
Author contributions
Conceptualization: CS, WY, HA, BO’C, HS. Investigation: MC, ER, JC, RH, JR. Software: MC, BE. Visualization: MC, BE, HS. Supervision: CS, WY, HA, BO’C, HS. Writing—original draft: MC, HS, BE. Writing—review & editing: MC, BE, HA, BO’C, HS. All authors contributed to the article and approved the submitted version.
Funding
NSF INFEWS grant 1639429 to BO'C, HA, WY and HS; NIH Molecular Biotechnology Training Grant # 1T32GM133366-01 Fellowship to MC.
Acknowledgments
We would like to thank Jennifer Swift for technical assistance as well as the NCSU Phytotron and the Hernandez group for equipment and advice.
Conflict of interest
The authors declare that the research was conducted in the absence of any commercial or financial relationships that could be construed as a potential conflict of interest.
Publisher’s note
All claims expressed in this article are solely those of the authors and do not necessarily represent those of their affiliated organizations, or those of the publisher, the editors and the reviewers. Any product that may be evaluated in this article, or claim that may be made by its manufacturer, is not guaranteed or endorsed by the publisher.
Supplementary material
The Supplementary Material for this article can be found online at: https://www.frontiersin.org/articles/10.3389/fpls.2023.1087707/full#supplementary-material
References
Abbas, N., Maurya, J. P., Senapati, D., Gangappa, S. N., Chattopadhyay, S. (2014). Arabidopsis CAM7 and HY5 physically interact and directly bind to the HY5 promoter to regulate its expression and thereby promote photomorphogenesis. Plant Cell 26, 1036–1052. doi: 10.1105/tpc.113.122515
Barbosa, G. L., Gadelha, F. D. A., Kublik, N., Proctor, A., Reichelm, L., Weissinger, E., et al. (2015). Comparison of land, water, and energy requirements of lettuce grown using hydroponic vs. conventional agricultural methods. Int. J. Environ. Res. Public Health 12 (6), 6879–6891. doi: 10.3390/ijerph120606879
Bilodeau, S. B., Wu, B., Rufyikiri, A., MacPherson, S., Lefsrud, M. (2019). An update on plant photobiology and implications for cannabis production. Front. Plant Sci. 10. doi: 10.3389/fpls.2019.00296
Bruinsma, J. (2009). “The resource outlook to 2050: By how much do land, water and crop yields need to increase by 2050?” in Presented at Expert Meeting on How to Feed the World in 2050. Rome, Italy: United Nations FAO, 24–26.
Cao, K., Yan, F., Xu, D., Ai, K., Yu, J., Bao, E., et al. (2018). Phytochrome B1-dependent control of SP5G transcription is the basis of the night break and red to far-red light ratio effects in tomato flowering. BMC Plant Biol. 18 (1), 158. doi: 10.1186/s12870-018-1380-8
Cerletti, C., De Curtis, A., Bracone, F., Digesù, C., Morganti, A. G., Iacoviello, L., et al. (2017). Dietary anthocyanins and health: Data from FLORA and ATHENA EU projects. Br. J. Clin. Pharmacol. 83, 103–106. doi: 10.1111/bcp.12943
Chen, X., Yao, Q., Gao, X., Jiang, C., Harberd, N., Fu, X. (2016). Shoot-to-Root mobile transcription factor HY5 coordinates plant carbon and nitrogen acquisition. Curr. Biol. 26, 640–646. doi: 10.1016/j.cub.2015.12.066
De Villiers, D. S., Wien, H. C., Reid, J. E., Albright, L. D. (2009). “Energy use and yields in tomato production: Field, high tunnel and greenhouse compared for the northern tier of the USA (Upstate new York),” in International Symposium on High Technology for Greenhouse Systems: GreenSys2009 893. Quebec City, Canada 373–380.
Edgar, R., Domrachev, M., Lash, A. E. (2002). Gene expression omnibus: NCBI gene expression and hybridization array data repository. Nucleic Acids Res. 30 (1), 207–210. doi: 10.1093/nar/30.1.207
Elliott, J., Deryng, D., Müller, C., Frieler, K., Konzmann, M., Gerten, D., et al. (2013). Constraints and potentials of future irrigation water availability on agricultural production under climate change. Proc. Natl. Acad. Sci. 111, 3239–3244. doi: 10.1073/pnas.1222474110
Emmott, C. J. M., Röhr, J. A., Campoy-Quiles, M., Kirchartz, T., Urbina, A., Ekins-Daukes, N. J., et al. (2015). Organic photovoltaic greenhouses: A unique application for semi-transparent PV? Energy Environ. Sci. 8 (4), 1317–1328. doi: 10.1039/C4EE03132F
Evans, J. R. (1988). Acclimation by the thylakoid membranes to growth irradiance and the partitioning of nitrogen between soluble and thylakoid proteins. Aust. J. Plant Physiol. 15, 93–106. doi: 10.1071/PP9880093
Fantini, E., Sulli, M., Zhang, L., Aprea, G., Jiménez-Gómez, J. M., Bendahmane, A., et al. (2019). Pivotal roles of cryptochromes 1a and 2 in tomato development and physiology. Plant Physiol. (Bethesda) 179 (2), 732–748. doi: 10.1104/pp.18.00793
Folta, K. M. (2018). Breeding new varieties for controlled environments. Plant Biol. 21, 6–12. doi: 10.1111/plb.12914
Fu, Y., Li, H., Yu, J., Liu, H., Cao, Z., Manukovsky, N. S., et al. (2017). Interaction effects of light intensity and nitrogen concentration on growth, photosynthetic characteristics and quality of lettuce (Lactuca sativa l. var. youmaicai). Sci. Hortic. 214, 51–57. doi: 10.1016/j.scienta.2016.11.020
Galvao, V. C., Fankhauser, C. (2015). Sensing the light environment in plants: Photoreceptors and early signaling steps. Curr. Opin. Neurobiol. 34, 46–53. doi: 10.1016/j.conb.2015.01.013
Gangappa, S., Botto, J. (2016). The multifaceted roles of HY5 in plant growth and development. Mol. Plant 9 (10), 1353–1365. doi: 10.1016/j.molp.2016.07.002
Guo, H., Yang, H., Mockler, T. C., Lin, C. (1998). Regulation of flowering time by arabidopsis photoreceptors. Sci. (American Assoc. Adv. Sci.) 279, 1360–1363. doi: 10.1126/science.279.5355.1360
Hahm, J., Kim, K., Qiu, Y., Chen, M. (2020). Increasing ambient temperature progressively disassemble arabidopsis phytochrome b from individual photobodies with distinct thermostabilities. Nat. Commun. 11 (1), 1660. doi: 10.1038/s41467-020-15526-z
Halbert-Howard, A., Häfner, F., Karlowsky, S., Schwarz, D., Krause, A. (2020). Evaluating recycling fertilizers for tomato cultivation in hydroponics, and their impact on greenhouse gas emissions. Environ. Sci. pollut. Res. 28, 59284–59303. doi: 10.1007/s11356-020-10461-4
Hao, J., Zhang, L., Li, P., Sun, Y., Li, J., Qin, X., et al. (2018). Quantitative proteomics analysis of lettuce (Lactuca sativa l.) reveals molecular basis-associated auxin and photosynthesis with bolting induced by high temperature. Int. J. Mol. Sci. 19, 2967. doi: 10.3390/ijms19102967
Hassanien, R. H. E., Li, M., Lin, W. D. (2016). Advanced applications of solar energy in agricultural greenhouses. Renewable Sustain. Energy Rev. 54, 989–1001. doi: 10.1016/j.rser.2015.10.095
Hollingsworth, J. A., Ravishankar, E., O'Connor, B., Johnson, J. X., DeCarolis, J. F. (2020). Environmental and economic impacts of solar-powered integrated greenhouses. J. Ind. Ecol. 24 (1), 234–247. doi: 10.1111/jiec.12934
Jain, M., Sharma, P., Tyagi, S. B., Tyagi, A. K., Khurana, J. P. (2007). Light regulation and differential tissue-specific expression of phototropin homologues from rice (Oryza sativa ssp. indica). Plant Sci. (Limerick) 172 (1), 164–171. doi: 10.1016/j.plantsci.2006.08.003
Kim, D., Langmead, B., Salzberg, S. L. (2015). HISAT: a fast spliced aligner with low memory requirements. Nat. Methods 12, 357–360. doi: 10.1038/nmeth.3317
Kim, H., Lin, M., Mitchell, C. A. (2019). Light spectral and thermal properties govern biomass allocation in tomato through morphological and physiological changes. Environ. Exp. Bot. 157, 228–240. doi: 10.1016/j.envexpbot.2018.10.019
Kobayashi, M., Akutsu, S., Fujinuma, D., Furukawa, H., Komatsu, H., Hotota, Y., et al. (2013). “Physiochemical properties of chlorophylls in oxygenic photosynthesis – succession of co-factors from anoxygenic to oxygenic photosynthesis,” in Photosynthesis. Ed. Dubinsky, Z. (London, UK: InTech), 47–90.
Kohler, A. E., Lopez, R. G. (2021). Duration of light-emitting diode (LED) supplemental lighting providing far-red radiation during seedling production influences subsequent time to flower of long-day annuals. Sci. Hortic. 281, 109956. doi: 10.1016/j.scienta.2021.109956
Langfelder, P., Horvath, S. (2008). WGCNA: An r package for weighted correlation network analysis. BMC Bioinf. 9 (1), 559. doi: 10.1186/1471-2105-9-559
Langfelder, P., Horvath, S. (2012). Fast r functions for robust correlations and hierarchical clustering. J. Stat. Softw. 46 (11), 1–17. doi: 10.18637/jss.v046.i11
Lee, J., He, K., Stolc, V., Lee, H., Figueroa, P., Gao, Y., et al. (2007). Analysis of transcription factor HY5 genomic binding sites revealed its hierarchical role in light regulation of development. Plant Cell 19, 731–749. doi: 10.1105/tpc.106.047688
Lee, J., Lee, I. (2010). Regulation and function of SOC1, a flowering pathway integrator. J. Exp. Bot. 61, 2247–2254. doi: 10.1093/jxb/erq098
Li, J., Wu, T., Huang, K., Liu, Y., Liu, M., Wang, J. (2021). Effect of LED spectrum on the quality and nitrogen metabolism of lettuce under recycled hydroponics. Front. Plant Sci. 12. doi: 10.3389/fpls.2021.678197
Liao, Y., Smyth, G. K., Shi, W. (2014). Featurecounts: an efficient general purpose program for assigning sequence reads to genomic features. Bioinf. (Oxford England) 30, 923–930. doi: 10.1093/bioinformatics/btt656
Liebman-Pelaez, M., Kongoletos, J., Norford, L. K., Reinhart, C. (2021). Validation of a building energy model of a hydroponic container farm and its application in urban design. Energy Buildings 250, 111192. doi: 10.1016/j.enbuild.2021.111192
Luo, Q., Li, Y., Gu, H., Zhao, L., Gu, X., Li, W. (2013). The promoter of soybean photoreceptor GmPLP1 gene enhances gene expression under plant growth regulator and light stresses. Plant Cell. Tissue Organ Cult. 114 (1), 109–119. doi: 10.1007/s11240-013-0310-6
McCarthy, D. J., Chen, Y., Smyth, G. K. (2012). Differential expression analysis of multifactor RNA-seq experiments with respect to biological variation. Nucleic Acids Res. 40, 4288–4297. doi: 10.1093/nar/gks042
Michael, R., Ranjan, A., Kumar, R., Pathak, P., Trivedi, P. (2020). Light-regulated expression of terpene synthase gene, AtTPS03, is controlled by the bZIP transcription factor, HY5, in Arabidopsis thaliana. Biochem. Biophys. Res. Commun. 529, 437–443. doi: 10.1016/j.bbrc.2020.05.222
Munch, D. (2022). “2021 disaster estimations reveal at least $12.5 billion in crop and forage losses,” in Market Intel (Washington, D.C., USA: American Farm Bureau Federation). Available at: https://www.fb.org/market-intel/2021-disaster-estimations-reveal-at-least-12.5-billion-in-crop-and-forage-l.
Naskar, S., Roy, C., Ghosh, S., Mukhopadhyay, A., Hazarika, L. K., Chaudhuri, R. K., et al. (2021). Elicitation of biomolecules as host defense arsenals during insect attacks on tea plants. Appl. Microbiol. Biotechnol. 105, 7187. doi: 10.1007/s00253-021-11560-z
Nohales, M. A., Liu, W., Duffy, T., Nozue, K., Sawa, M., Pruneda-Paz, J. L., et al. (2019). Multi-level modulation of light signaling by GIGANTEA regulates both the output and pace of the circadian clock. Dev. Cell 49 (6), 840–851.e8. doi: 10.1016/j.devcel.2019.04.030
Ntinas, G. K., Neumair, M., Tsadilas, C. D., Meyer, J. (2017). Carbon footprint and cumulative energy demand of greenhouse and open-field tomato cultivation systems under southern and central European climatic conditions. J. Cleaner Prod. 142, 3617–3626. doi: 10.1016/j.jclepro.2016.10.106
Page, G., Ridoutt, B., Bellotti, B. (2012). Carbon and water footprint tradeoffs in fresh tomato production. J. Cleaner Prod. 32, 219–226. doi: 10.1016/j.jclepro.2012.03.036
Park, J., Choung, M., Kim, J., Hahn, B., Kim, J., Bae, S., et al. (2007). Genes up-regulated during red coloration in UV-b irradiated lettuce leaves. Plant Cell Rep. 26, 507–516. doi: 10.1007/s00299-006-0255-x
Pattanayak, G. K., Biswal, A. K., Reddy, V. S., Tripathy, B. C. (2004). Light-dependent regulation of chlorophyll b biosynthesis in chlorophyllide a oxygenase overexpressing tobacco plants. Biochem. Biophys. Res. Commun. 326, 466–471. doi: 10.1016/j.bbrc.2004.11.049
Pattison, P. M., Tsao, J. Y., Brainard, G. C., Bugbee, B. (2018). LEDs For photons, physiology and food. Nat. (London) 563 (7732), 493–500. doi: 10.1038/s41586-018-0706-x
Qian, Y., Hibbert, L. E., Milner, S., Katz, E., Kliebenstein, D. J., Taylor, G. (2022). Improved yield and health benefits of watercress grown in an indoor vertical farm. Sci. Horticult. 300, 111068. doi: 10.1016/j.scienta.2022.111068
Ramin Shamshiri, R., Kalantari F, C., Ting, K., Thorp K, R., Hameed I, A., Weltzien, C., et al. (2018). Advances in greenhouse automation and controlled environment agriculture: A transition to plant factories and urban agriculture. Int. J. Agric. Biol. Engineering 11, 1–22. doi: 10.25165/j.ijabe.20181101.3210
Ravishankar, E., Booth, R. E., Hollingsworth, J. A., Ade, H., Sederoff, H., DeCarolis, J. F., et al. (2022). Organic solar powered greenhouse performance optimization and global economic opportunity. Energy Environ. Sci. 15, 1659–1671. doi: 10.1039/D1EE03474J
Ravishankar, E., Booth, R. E., Saravitz, C., Sederoff, H., Ade, H. W., O’Connor, B. T. (2020). Achieving net zero energy greenhouses by integrating semitransparent organic solar cells. Joule 4 (2), 490–506. doi: 10.1016/j.joule.2019.12.018
Ravishankar, E., Charles, M., Xiong, Y., Henry, R., Swift, J., Rech, J., et al. (2021). Balancing crop production and energy harvesting in organic solar-powered greenhouses. Cell Rep. Phys. Sci. 2 (3), 100381. doi: 10.1016/j.xcrp.2021.100381
Robinson, M. D., McCarthy, D. J., Smyth, G. K. (2010). edgeR: A bioconductor package for differential expression analysis of digital gene expression data. Bioinformatics 26 (1), 139–140. doi: 10.1093/bioinformatics/btp616
Sager, J. C., Smith, W. O., Edwards, J. L., Cyr, K. L. (1988). Photosynthetic efficiency and phytochrome photoequilibria determination using spectral data. Trans. ASAE 31 (6), 1882–1889. doi: 10.13031/2013.30952
Saigo, T., Wang, T., Watanabe, M., Tohge, T. (2020). Diversity of anthocyanin and proanthocyanin biosynthesis in land plants. Curr. Opin. Plant Biol. 55, 93–99. doi: 10.1016/j.pbi.2020.04.001
Schmitt, J., Dudley, S. A., Pigliucci, M. (1999). Manipulative approaches to testing adaptive plasticity: Phytochrome-mediated shade-avoidance responses in plants. Am. Nat. 154 (S1), S43–S54. doi: 10.1086/303282
Sharrock, R. A., Quail, P. H. (1989). Novel phytochrome sequences in arabidopsis thaliana: Structure, evolution, and differential expression of a plant regulatory photoreceptor family. Genes Dev. 3 (11), 1745–1757. doi: 10.1101/gad.3.11.1745
Sims, D. A., Gamon, J. A. (2002). Relationships between leaf pigment content and spectral reflectance across a wide range of species, leaf structures and developmental stages. Remote Sens. Environ. 81, 337–354. doi: 10.1016/S0034-4257(02)00010-X
Sng, B. J. R., Mun, B., Mohanty, B., Kim, M., Phua, Z. W., Yang, H., et al. (2021). Combination of red and blue light induces anthocyanin and other secondary metabolite biosynthesis pathways in an age-dependent manner in Batavia lettuce. Plant Sci. 310, 110977. doi: 10.1016/j.plantsci.2021.110977
Song, S., Kusuma, P., Carvalho, S. D., Li, Y., Folta, K. M. (2019). Manipulation of seedling traits with pulsed light in close controlled environments. Environ. Exper. Bot. 166, 103803. doi: 10.1016/j.envexpbot.2019.103803
Stutte, G. W. (2009). Light-emitting diodes for manipulating the phytochrome apparatus. HortScience 44, 231–234. doi: 10.21273/HORTSCI.44.2.231
Suzuki, H., Nakayama, T., Yonekura-Sakakibara, K., Fukui, Y., Nakamura, N., Yamaguchi, M., et al. (2002). cDNA cloning, heterologous expressions, and functional characterization of malonyl-coenzyme A: Anthocyanidin 3-O-Glucoside-6"-O-Malonyltransferase from dahlia flowers. Plant Physiol. 130, 2142–2151. doi: 10.1104/pp.010447
Taniguchi, M., Lindsey, J. S. (2018). Database of absorption and fluorescence spectra of >300 common compounds for use in PhotochemCAD. Photochem. Photobiol. 94, 290–327. doi: 10.1111/php.12860
Tian, Y., Wang, H., Sun, P., Fan, Y., Qiao, M., Zhang, L., et al. (2019). Response of leaf color and the expression of photoreceptor genes of Camellia sinensis cv. huangjinya to different light quality conditions. Sci. Hortic. 251, 225–232. doi: 10.1016/j.scienta.2019.03.032
van Gelderen, K., Kang, C., Li, P., Pierik, R. (2021). Regulation of lateral root development by shoot-sensed far-red light via HY5 is nitrate-dependent and involves the NRT2.1 nitrate transporter. Front. Plant Sci. 12, 660870. doi: 10.3389/fpls.2021.660870
Waller, R., Kacira, M., Magadley, E., Teitel, M., Yehia, I. (2021). Semi-transparent organic photovoltaics applied as greenhouse shade for spring and summer tomato production in arid climate. Agron. (Basel) 11 (6), 1152. doi: 10.3390/agronomy11061152
Wang, J., Lu, W., Tong, Y., Yang, Q. (2016). Leaf morphology, photosynthetic performance, chlorophyll fluorescence, stomatal development of lettuce (Lactuca sativa l.) exposed to different ratio of red light to blue light. Front. Plant Sci. 7. doi: 10.3389/fpls.2016.00250
Xiao, S., Zhang, Q., You, W. (2017). Molecular engineering of conjugated polymers for solar cells: An updated report. Adv. Mater. (Weinheim) 29 (20), 1601391–n/a. doi: 10.1002/adma.201601391
Zhang, Y., Xu, S., Cheng, Y., Peng, Z., Han, J. (2018). Transcriptome profiling of anthocyanin-related genes reveals effects of light intensity on anthocyanin biosynthesis in red leaf lettuce. PeerJ. (San Francisco CA) 6, e4607. doi: 10.7717/peerj.4607
Zhen, S., Bugbee, B. (2020). Far-red photons have equivalent efficiency to traditional photosynthetic photons: Implications for redefining photosynthetically active radiation. Plant Cell Environ. 43, 1259–1272. doi: 10.1111/pce.13730
Keywords: agrivoltaic, greenhouse, solar power, sustainability, gene networks, photosynthesis, photomorphogenesis
Citation: Charles M, Edwards B, Ravishankar E, Calero J, Henry R, Rech J, Saravitz C, You W, Ade H, O’Connor B and Sederoff H (2023) Emergent molecular traits of lettuce and tomato grown under wavelength-selective solar cells. Front. Plant Sci. 14:1087707. doi: 10.3389/fpls.2023.1087707
Received: 02 November 2022; Accepted: 30 January 2023;
Published: 13 February 2023.
Edited by:
Peng Wang, Institute of Botany (CAS), ChinaReviewed by:
Xueyun Hu, Yangzhou University, ChinaTanai Cardona, Imperial College London, United Kingdom
Copyright © 2023 Charles, Edwards, Ravishankar, Calero, Henry, Rech, Saravitz, You, Ade, O’Connor and Sederoff. This is an open-access article distributed under the terms of the Creative Commons Attribution License (CC BY). The use, distribution or reproduction in other forums is permitted, provided the original author(s) and the copyright owner(s) are credited and that the original publication in this journal is cited, in accordance with accepted academic practice. No use, distribution or reproduction is permitted which does not comply with these terms.
*Correspondence: Heike Sederoff, aHdzZWRlcm9AbmNzdS5lZA==
†Present addresses: Eshwar Ravishankar, Department of Horticulture, North Carolina State University, Raleigh, North Carolina, United States
John Calero, Pairwise Inc., Durham, North Carolina, United States
Jeromy Rech, Department of Chemical Engineering, Stanford University, Stanford, CA, United States