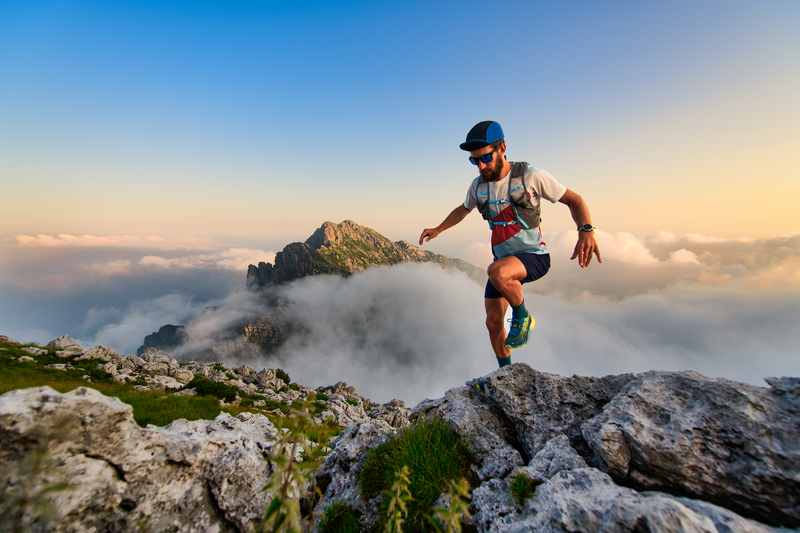
95% of researchers rate our articles as excellent or good
Learn more about the work of our research integrity team to safeguard the quality of each article we publish.
Find out more
ORIGINAL RESEARCH article
Front. Plant Sci. , 20 June 2023
Sec. Functional Plant Ecology
Volume 14 - 2023 | https://doi.org/10.3389/fpls.2023.1070472
This article is part of the Research Topic Carbon Sequestration and Climate Change in Crops, Natural Vegetation, and Wetland Dynamics in the High Andes View all 6 articles
Chenopodium quinoa Willd. is a native species that originated in the High Andes plateau (Altiplano) and its cultivation spread out to the south of Chile. Because of the different edaphoclimatic characteristics of both regions, soils from Altiplano accumulated higher levels of nitrate () than in the south of Chile, where soils favor ammonium (NH4+) accumulation. To elucidate whether C. quinoa ecotypes differ in several physiological and biochemical parameters related to their capacity to assimilate and NH4+, juvenile plants of Socaire (from Altiplano) and Faro (from Lowland/South of Chile) were grown under different sources of N ( or NH4+). Measurements of photosynthesis and foliar oxygen-isotope fractionation were carried out, together with biochemical analyses, as proxies for the analysis of plant performance or sensitivity to NH4+. Overall, while NH4+ reduced the growth of Socaire, it induced higher biomass productivity and increased protein synthesis, oxygen consumption, and cytochrome oxidase activity in Faro. We discussed that ATP yield from respiration in Faro could promote protein production from assimilated NH4+ to benefit its growth. The characterization of this differential sensitivity of both quinoa ecotypes for NH4+ contributes to a better understanding of nutritional aspects driving plant primary productivity.
Carbon (C) assimilation and release in plants are key plant biochemical processes for global primary productivity. It is dependent on multiple processes ranging from photosynthesis, which absorbs energy from sunlight to build carbohydrates, to aerobic cellular respiration, which releases metabolic energy to be captured by the cell in the form of ATP during mitochondrial oxidative phosphorylation (Del-Saz et al., 2018; O’Leary et al., 2019). Both photosynthesis and respiration and, thus, plant growth are dependent on nitrogen (N) plant status (Miller and Cramer, 2005). This essential macronutrient is mostly uptaken from soils by roots in different inorganic forms (Miller and Cramer, 2005; Kant et al., 2011; Xu et al., 2012). Nitrate () can be a dominant form of N in arid and semi-arid regions with basic and aerated soils, where alkali compounds are accumulated. Conversely, NH4+ is a common N source in regions with high precipitations and acidic soils, where soluble basic molecules including are leached (Luzio et al., 2002; Blake, 2005; Miller and Cramer, 2005; Hachiya and Sakakibara, 2017). Several studies suggest that climate variations could change the ratios of the inorganic forms of N ( and NH4+) available for plants (Myers et al., 2014; Coskun et al., 2016; Greaver et al., 2016; Schittek et al., 2016; Ackerman et al., 2019). Climate change may potentially increase NH4+ over by slowing down the rate of nitrification (the aerobic oxidation of NH4+ to ) (Auyeung et al., 2015). Precisely, the decrease in rainfall, together with an increase in temperature and a decrease of pH in soils, could compromise biological nitrification rather than denitrification, altering the availability of inorganic forms of N in soils (Breuer et al., 2002; Barnard et al., 2006; Auyeung et al., 2015; Coskun et al., 2016; Greaver et al., 2016; Daryanto et al., 2019).
Compared to , NH4+ assimilation entails a lower energy cost, and can be beneficial for plant growth in many circumstances, including elevated levels of CO2 (Rubio-Asensio and Bloom, 2017). However, some plants are sensitive to NH4+ due to its toxicity, displaying growth suppression and chlorosis (Rubio-Asensio et al., 2015). Alterations in photosynthesis and PSII performance have been described in plants growing under NH4+ because of increased synthesis of reactive oxygen species (ROS) (Zhu et al., 2000; Guo et al., 2005; Alencar et al., 2019). Regarding respiration, several studies have shown increases in O2 consumption in plants growing under as the sole N source (Rigano et al., 1996; Britto et al., 2001; Escobar et al., 2006). It was suggested that exposure to NH4+ could alter respiratory activity in leaves for the benefit of redox homeostasis through the modulation of the activities of the cytochrome oxidase pathway (COP) and the non-phosphorylating alternative oxidase pathway (AOP; Rigano et al., 1996; Britto et al., 2001; Escobar et al., 2006; Ortiz et al., 2020), which can be measured in vivo by mass spectrometry (Del-Saz et al., 2018). However, no previous studies have characterized the respiratory activities of COP and AOP in plants grown under different N sources.
Chenopodium quinoa Willd. (Amaranthaceae family) is an important crop for food security worldwide (FAO, 2011). It has been reported that quinoa was originated in the Altiplano of the Andes (3500 m.a.s.l., shared by Peru, Bolivia, and Chile) and spread out to Southern Chile by the Inca Empire (Martinez et al., 2009). However, it has recently been suggested that highland and lowland/coastal plants were domesticated independently in these environments (Planella et al., 2015; Jarvis et al., 2017; Maughan et al., 2019). In the Altiplano, quinoa plants deal with extreme drought, high-flux solar radiation, very strong daytime temperature changes, limited volume of annual rainfall (150–300 mm/year), and saline-alkali soils (García et al., 2007; Cárdenas-Castillo et al., 2021; Rascón et al., 2021). In the South of Chile, quinoa plants face acidic soils, uneven N content in the soil, and rainfall ranging from 500 to 1,500 mm/year (Luzio et al., 2010; Bascuñán-Godoy et al., 2018). Previous studies described that availability is an important factor that helps determine differences between these ecotypes at both biochemical and metabolic levels (Pinto-Irish et al., 2020). These authors found that the Andean ecotype displayed a more efficient mechanism of uptake than the southern ecotype, displaying a higher level of proteins in leaves and roots. Additionally, seeds presented higher levels of amino acids, and metabolites from shikimate, ornithine, purine, and nicotinamide metabolism. It remains to be determined whether the N source is a factor that may lead to different plant physiological performance. It has been described that alternative respiration plays different roles under abiotic stress conditions (Del-Saz et al., 2018) depending on plant species or genotypes (Florez-Sarasa et al., 2016; Del-Saz et al., 2018; Del-Saz et al., 2021). Bearing in mind that Chile is one of the most climatically diverse places on the planet, there is a need to characterize respiration metabolism under different scenarios of N forms available for uptake. In this sense, quinoa is an optimal species because the distribution of this species is geographically wide in Chile and diverse ecotypes have been described (Bazile et al., 2014).
In the present research, we categorized two places in Chile with a different predominance of or NH4+ forms (Luzio et al., 2010) according to the existence of a longitudinal gradient of pH from the North (alkaline) to the South (acidic) of this country (SoilGrids, 2021). We performed measurements of photosynthesis, oxygen-isotope fractionation, total soluble sugars, starch, NH4+, protein, chlorophylls, and betacyanin contents in two ecotypes of quinoa plants from Altiplano (Socaire) and South of Chile (Faro) grown under different N sources as proxies for the evaluation of plant performance or sensitivity to NH4+. Thus, our main objective was to characterize plant respiratory parameters in leaves of both C. quinoa ecotypes grown under and NH4+. Secondly, we discuss possible respiratory differences based on other biochemical parameters related to plant performance and N assimilation that help to provide first insights into the regulation of the respiratory pathways by this nutrient.
Seeds of two Chilean ecotypes of C. quinoa (Willd.) from contrasting agro-ecological origins were used in the experiments: Socaire from the Chilean Altiplano (Socaire, 23°35’31.58” S, 67°53’17.69” W, and 3,500 m.a.s.l.) and the Lowland/Southern coastal Faro ecotype (Chillan, 36°35’ 43.2” S, 72°04’ 39.9” W and 140 m.a.s.l.). Seeds of Socaire were collected in a private field (23°34′S 67°54′W, 3,500 m.a.s.l.). The soil in the zone is basic (pH between 7.8 and 8.8) and the total percent and available N were 0.09% and 46 mg/kg, respectively. Soil and climate characterizations of the zone can be found in García et al. (2007). Faro seeds were collected in Chillan at “El Nogal Experimental Station” (36°35’ 43.2” S, 72°04’ 39.9” W and 140 m.a.s.l.). The pH of the soil was approximately 4.5, and the total percent and available N were 0.01% and 44 mg/kg, respectively. Soil and climate characterizations in this station are found in Fischer et al. (2013) and Stolpe (2006). Seeds of both ecotypes (Socaire and Faro) were collected in summer (February) in each location (in Socaire soils and in Chillan soils, respectively). Seeds of both ecotypes are included in the National Seed Bank of Chile managed by the Genetic Resources section of the National Institute of Agriculture Research (http://163.247.128.32/gringlobal/search.aspx, INIA-Intihuasi Vicuña, Chile).
In order to compare the performance of both ecotypes under and NH4+, we established an optimal N concentration for plant growth. We set up biomass curves under the supply of different amounts of N, using as a referential condition because it is generally the preferred N source for plants without toxic effects. Germinated seeds of both ecotypes with similar lengths of emerged radicle were transplanted into 700-ml pots containing sand:perlite (1:1), and six concentrations of (using KNO3 salt) and NH4+ [using (NH4)2SO4 salt] were tested: 0.0, 5.0, 10, 20, 40, and 100 mM. Ten pots (with three plants each) were submitted to each N concentration of or NH4+. The conditions of the growing chamber and the irrigation solution were the same as explained below. Thirty-day-old plants were collected for total biomass determination.
Socaire and Faro plants reached maximum biomass at 20 mM of N (Supplementary Figure 1). Therefore, this amount was used for the physiological experiments comparing N sources. Plants were germinated and grown in pots supplied with 20 mM of or NH4+ using the following growing conditions: light intensity of 575 µmol/m2/s, 21°C/19°C day/night, 16 h light/8 h dark photoperiod, and 75% relative humidity. Plants were supplied once with MS 407 nutrient medium described by Murashige and Skoog (1962), and consisting of the following: 0.30 mM MgSO4.7H2O, 0.22 mM CaCl2, 0.62 mM KH2PO4, 12.7 mM KCl, 0.05 µM KI, 1.00 µM H3BO3, 1.32 µM MnSO4.4H2O, 0.30 µM ZnSO4.7H2O, 0.01 µM Na2MoO4.2H2O, 0.001 µM CuSO4.5H2O, 0.001 µM CoCl2.6H2O, 0.51 µM Na2.EDTA, 0.50 µM FeSO4.7H2O, 2.78 µM inositol, 0.02 µM nicotinic acid, 0.01 µM pyridoxine HCl, 0.001 µM thiamine-HCl, and 0.13 µM Glycine. KNO3 and (NH4)2SO4 varied according to the treatment. The pH was set at 5.8.
Thirty pots of 700 ml (three plants per pot) were used for each N source and ecotype. The experiment was run in a completely randomized design and additional plants were grown to prevent the bordering effect. Juvenile plants were collected 30 days after sowing at midday. Harvested plants for biochemical analysis were fractionated into belowground and aboveground (leaves and biomass). The root samples were rinsed in distilled water and dried with a paper towel. The tissue samples were frozen in liquid N and then freeze-dried. Tissue was ground to a fine powder in liquid N using a mortar and pestle and stored in Falcon tubes at −80°C. Measurements were performed belowground and aboveground, except for pigments that were performed in leaves.
Images of plants and leaves submitted to the different treatments were taken with the Scanner Epson Perfection V850 Pro Photo (Epson Corporation, San Jose, CA). The image acquisition parameter was set to “high” accuracy (600 dpi; image size 18 MB). Leaf area was measured through image analysis using the ImageJ software (NIMH, Bethesda, Maryland, USA). Above and belowground biomasses were determined by drying the tissues at 60°C for 48 h till constant weight.
N and C elements were measured in the whole plant from the different N treatments and ecotypes (n = 3). Briefly, plant tissue was oven-dried at 60°C for 48 h and ground. A subsample of 5 mg was weighed and stored in plastic vials. Samples were measured using the Elemental Combustion System CHNS-O (Costech Analytical Technologies Inc., Valentia, USA). C and N are reported as the percentage of elements per dry matter.
We used above- and belowground tissue from each ecotype and N source (n = 6). Changes in total soluble sugars (TSS), starch, and total nonstructural carbon (NSC) in aerial and roots were assayed following the method of Marquis et al. (1997). Total soluble sugars were separated and extracted with methanol/chloroform/water according to and determined by colorimetry using 2% phenol and sulfuric acid at 490 nm according to Dickinson (1979) and Chow et al (2004). The insoluble fraction that contains starch was hydrolyzed to glucose overnight using a sodium acetate buffer and amyloglucosidase (Sigma-Aldrich 10115, St. Louis, MO, USA) at 45°C and then measured with a phenol–sulfuric acid reaction as in Marquis et al. (1997).
Total soluble protein and ammonium contents were determined in the above- and belowground tissue of the two quinoa ecotypes studied. NH4+ was determined according to Forster (1995). Absorbance was measured at 660 nm in a spectrophotometer (Infinite 200 Pro, Tecan, Männedorf, Switzerland). We used the TCA/acetone procedure for protein extraction (Wang et al., 2008). The Quick Start Bradford Assay kit (Bradford, 1976) was used for protein quantification using BSA as the standard protein (n = 4), according to manufacturer instructions (Bio-Rad, Hercules, CA, USA).
Chlorophylls a and b were extracted from leaves of plants of all treatments (n = 6) in 80% of acetone overnight and centrifuged at 12,000g for 10 min. The content of chlorophylls was determined at 664 and 647 nm following the method described by Lichtenthaler and Buschmann (2001).
Betacyanins were extracted from leaves (n = 6) in water/methanol and spectrophotometrically determined at 536 nm. The betacyanin content of the plant aqueous extracts was estimated according to Abderrahim et al. (2015).
The lipid peroxidation in the above- and belowground tissue of the two quinoa ecotypes studied (n = 5) was determined in vitro by estimating the formation of malondialdehyde (MDA) according to the method described by Ortega-Villasante et al. (2005). Frozen leaf tissue (0.1–0.2 g) was homogenized in 1 ml of TCA–TBA–HCl reagent [15% (w/v)] trichloroacetic acid, 0.37% (w/v) 2-thiobarbituric acid, 0.25 M HCl, and 0.01% butylated hydroxytoluene. After homogenization, samples were incubated at 90°C for 30 min and centrifuged at 12,000 g for 10 min. Absorbance was measured at 535 nm and 600 nm.
Gas exchange measurements of net photosynthesis (AN) were performed in leaves from both ecotypes and N sources in six plants per treatment, using a portable photosynthesis system (Li-6400XT, LI-COR Inc., Lincoln, NE, USA) equipped with a light source (6200-02B LED, Li-Cor).
Light curves were run to determine the light saturation intensity at which plants reached maximum photosynthesis. AN rates were measured at mid-morning (between 11 a.m. and 1 p.m.) with the gas exchange previously stabilized. Conditions in the leaf chamber were as follows: block temperature of 25°C, 1,500 µmol photon m−2 s−1, an air flow of 300 (mol s−1), and a CO2 concentration (Ca) of 400 mol mol−1. Relative humidity ranged between 45% and 50% and VPD ranged between 1.6 and 1.9 kPa. AN data were normalized by the area of leaves (n = 6).
For respiratory measurements, leaves of quinoa plants, which were grown at the University of the Balearic Islands under similar growing conditions, were placed in a 3-ml stainless-steel closed cuvette and maintained at a constant temperature of 25°C. Air samples were sequentially removed from the cuvette and fed into the mass spectrometer (Delta XPlus; Thermo LCC, Bremen, Germany). Changes in the 18O/16O ratios and O2 concentration were obtained to calculate the oxygen-isotope fractionation and the electron partitioning to the AOP (τa), allowing calculations of the in vivo activities of AOP and COP as described in Del-Saz et al. (2017). End point fractionation values of the AOP (Δa) were determined in leaves with a solution of 25 mM potassium cyanide (KCN) for 20 min. A value of 32.8 ± 0.69‰ (n = 3) was obtained in leaves of the Faro ecotype. Owing to a limitation in the number of Socaire plants, we assumed a similar value of Δc of 32.8‰. We also assumed a value of 20.0‰ for the endpoint fractionation values of the COP (Δc) as this has been shown to be constant in most leaves and species examined (Ribas-Carbo et al., 2005). Values presented are the mean of one measurement in four plants per ecotype that were performed from 9 a.m. to 5 p.m. during five consecutive days.
For the determination of sufficient N source supply, we used three-way ANOVA (level of significance p < 0.05) using ecotype, source of N, and concentration as factors. Data from the effects of N source ( or NH4+) on the different quinoa ecotypes (Socaire and Faro) were analyzed by two-way ANOVA. Tukey HSD test was used to identify means with significant differences (level of significance p < 0.05).
The total dry biomass of both ecotypes enhanced with increased N supply, until reaching mean maximum biomass values at 20 mM N (Supplementary Figure 1). Consistently with previous results published by Pinto-Irish et al. (2020), we considered 20 mM N as optimal for growing conditions (because both ecotypes reached the highest biomass either under or NH4+). At higher concentrations (40 and 100 mM), plant biomass decreased under , and mortality increased under NH4+; for this reason, these data were omitted in Supplementary Figure 1. Considering that is generally the preferred N source without deleterious effects, our results focused on the comparison of the ecotypes under NH4+ regarding their performance under .
The two-way ANOVA reveals interactions between ecotypes (E) and nitrogen (N) source (E × N) in aboveground, belowground, and total leaves biomass (Figure 1 and Table 1). Ecotypes presented similar aboveground biomass under (Figures 1A, E); however, under NH4+ supply, Socaire showed a 72% reduction in plant biomass, while Faro maintained similar values to those observed under (p < 0.031) (Figures 1A, E). Regarding belowground biomass, ecotypes exhibited a large contrasting response under NH4+ (E × N), with a 80% reduction in Socaire under NH4+ compared to , while a twofold increase (p < 0.001) was observed in Faro (Figure 1C). Both the total leaf area and total leaf biomass per plant (sum of all leaves) (Figures 1B, D, E) correlated well with aboveground biomass. No differences in leaf area or leaf biomass were observed between N sources in Faro.
Figure 1 Biometrical parameters: aboveground biomass (A), total leaf area (B), belowground biomass (C), total leaves biomass (D), and representative images of whole plants and leaves (E) under different N sources in two ecotypes of C. quinoa. Plants were subjected to 20 mM or NH4+ supply per 30 days. Values are means ± SE (n = 4). Different letters show statistical differences using two-way ANOVA considering ecotypes and source of N as factors. Tukey HSD was used as a post-hoc test (p < 0.05).
Table 1 p-values (p < 0.05) and the size effects (η2; Eta squared) for the effects of E, N, and their interaction determined by two-way ANOVA analysis on biometrics and physiological attributes: above- and belowground biomass, total leaves biomass, total leaves area, %C, %N, C:N ratio, NH4+, protein, TSS, starch, NSC, and MDA at both above- and belowground, Chlorophyll a and b, betacyanins, AN, Vtotal, τa, vcyt, and valt in leaves of two genotypes of Chenopodium quinoa grown at two sources of nitrogen supplementation for 30 days.
The %C, %N, and the C:N ratio were significantly affected by E × N interaction (Table 1). Both ecotypes presented similar %C values under supply; however, their content was significantly reduced under NH4+ supply (p < 0.001) (Figure 2A).
Figure 2 Percent of C (A), N (B), and C:N ratio (C) under different N sources ( and NH4+) in two ecotypes of C. quinoa. Plants were subjected to 20 mM or NH4+ supply per 30 days. Values are means ± SE (n = 3). Different letters show statistical differences using two-way ANOVA considering ecotypes and source of N as factors. Tukey HSD was used as a post-hoc test (p < 0.05).
Under both N sources, Socaire displayed an enhanced level of %N compared to Faro (Figure 2B). A significant increase in %N was observed in Socaire plants under NH4+ compared to conditions. Contrastingly, Faro showed a similar %N under both N sources. Under, the %N in Socaire was twice as high as in Faro.
The C:N ratio was higher in Faro than in Socaire at both conditions. However, both ecotypes showed a significant decrease in the C:N ratio under NH4+, with the lowest values in Socaire (Figure 2C).
The two-way ANOVA revealed that NH4+ content belowground was affected by E × N interaction (p < 0.001). The NH4+ content in roots increased by 30% in Faro ecotype under NH4+ treatment compared to NH4+, while it was maintained in Socaire (Figure 3). Aboveground NH4+ was not affected by N sources and differences were based on E (p < 0.001).
Figure 3 NH4+ and protein content at aboveground (A, B) and belowground (C, D) under different N sources in two ecotypes of C. quinoa. Plants were subjected to 20 mM NH4+ or NH4+ supply per 30 days. Values are means ± SE (n = 4). Different letters show statistical differences using two-way ANOVA considering ecotypes and source of N as factors. Tukey HSD was used as a post-hoc test (p < 0.05).
The two-way ANOVA reveals that protein content at above- and belowground (p < 0.001) tissues was affected by E × N interaction (Table 1 and Figures 3A, B). Under , protein level was three times higher in Socaire than in Faro (in both above- and belowground tissues). Under NH4+, Faro showed a fourfold increase in protein content in both shoot and root, while Socaire showed an increase of 33% in protein content only aboveground.
A non-significant E × N interaction was observed in the storage of carbohydrates aboveground (normalized by dry weight) (p > 0.05); however, significant E × N interaction was observed for TSS, starch, and NSC belowground (Table 1 and Figure 4).
Figure 4 Effect of N source on TSS (A, D), starch (B, E), and NSC (C, F) in aboveground and belowground tissues in juvenile plants of two ecotypes of C. quinoa. Plants were subjected to 20 mM or NH4+ supply per 30 days. Values are means ± SE (n = 5). Different letters show statistical differences using two-way ANOVA considering ecotypes and source of N as factors. Tukey HSD was used as a post-hoc test (p < 0.05).
In aboveground tissues (stem plus leaves), TSS tended to increase under NH4+ in both ecotypes and significant differences were observed in Socaire (Figure 4A). Starch only depended on E aboveground (p < 0.001) (Figure 3B). In belowground tissues, Faro showed higher TSS and starch contents than Socaire under conditions (Figures 4D, E). Under , Faro showed the highest values of NSC in both above- and belowground tissues. However, a significant decrease in belowground NSC was observed under NH4+, while NSC values in the aboveground tissues reached similar values to those observed in plants under (Figures 4C, F). On the other hand, Socaire displays similar NSC values under both sources of N (Figure 4). These results changed drastically when they are interpreted as organs (Supplementary Figure 2), where strong reductions were observed in NSC of Socaire under NH4+ at both aboveground tissues and roots.
A significant E × N interaction was observed for chlorophylls, betacyanins, and MDA (p < 0.001). Chlorophylls a and b increased in Faro under NH4+ compared to , while no significant changes were observed in Socaire (Figures 5A, B). Regarding betacyanins, an increase of 30% was observed in Socaire under NH4+ compared to NO3, displaying significantly higher values than those observed in Faro (Figure 5C).
Figure 5 The effect of N sources in Chlorophyll a (A), Chlorophyll b (B), and Betacyanins (C) in leaves of two Quinoa ecotypes. Plants were subjected to 20 mM or NH4+ supply per 30 days. Values are means ± SE (n = 6). Different letters show statistical differences using two-way ANOVA considering ecotypes and source of N as factors. Tukey HSD was used as a post-hoc test (p < 0.05).
An increase of 50% in belowground MDA content was observed in the Socaire ecotype under NH4+ compared to (Figure 6), while similar values were observed aboveground in comparison to Faro under and NH4+. Faro maintained MDA levels under both N sources (Figure 6D).
Figure 6 Lipid peroxidation (measured as MDA) at aboveground (A) and belowground (B) under different N sources in two ecotypes of C. quinoa. Plants were subjected to 20 mM or NH4+ supply per 30 days. Values are means ± SE (n = 5). Different letters show statistical differences using two-way ANOVA considering ecotypes and source of N as factors. Tukey HSD was used as a post-hoc test (p < 0.05).
Both Faro and Socaire plants displayed similar AN rates in leaves (normalized by area) under . Under NH4+, AN was approximately 50% higher in Socaire than in Faro (p < 0.05) (Figure 7).
Figure 7 Net photosynthesis rates were taken in well-developed leaves on juvenile plants of two ecotypes of quinoa. Plants were subjected to 20 mM or NH4+ supply per 30 days. Bars are means ± SD (n = 6). Two-way ANOVA and Tukey test analysis (p < 0.05) were used for to detect differences.
Oxygen consumption and electron partitioning to the COP and AOP under and NH4+A significant E × N interaction was observed in total O2 uptake (Vt) (p < 0.05) and in the electron partitioning to the AOP (τa) (p < 0.05). There was a significant reduction (by 30%) of τa in Faro under NH4+ compared to , together with a significant increase (by 80%) in Vt via COP (Figure 8). No respiratory changes were observed in Socaire (E × N, p < 0.05) (Figure 8).
Figure 8 The effect of N sources in total respiration (Vtotal) (A), cytochrome pathway activity (vcyt) (B), the electron partitioning to the AOP (τa) (C), and alternative pathway activity (valt) (D) in leaves of two quinoa ecotypes. Plants were subjected to 20 mM or NH4+ supply per 30 days. Values are means ± SE (n = 6). Different letters show statistical differences using two-way ANOVA considering ecotypes and source of N as factors. Tukey HSD was used as a post-hoc test (p < 0.05).
The Chenopodiaceae family has been considered a specialist and sensitive to NH4+ (Smirnoff et al., 1984; Britto and Kronzucker, 2002); however, our data showed evidence that the preference for N source depends on the geographical origin of the genotype. When comparing ecotypes under source, Socaire showed a physiological performance more linked to the N metabolism than Faro, through higher %N, NH4+, protein, and pigment levels, together with higher root biomass and lower content of MDA (Figures 1–3, 5, 6). However, Socaire showed NH4+-sensitive growth, even under low NH4+ concentrations, and under different :NH4+ ratios (Figure 1; Supplementary Figures 3, 4). Conversely, Faro showed similar biomass accumulation under both or NH4+ sources.
Maintaining high C levels is critical to tolerate NH4+ in soils due to an increased requirement for C skeletons that allow the incorporation of NH4+ into organic molecules (Wang et al., 2022). Despite the fact that a significant decrease in the C:N ratio was observed in both ecotypes under NH4+ (Figure 2), except for TSS in Faro roots, non-significant changes in non-structural carbohydrates were observed under both N sources (Figure 4), which correlated well with the maintenance of photosynthesis in both ecotypes (Figure 7). Thus, the biomass restriction in the Socaire ecotype under NH4+ source appeared to be unrelated to either photosynthesis or carbon storage impairment, which has been observed in other species previously (Ariz et al., 2013; Podgórska et al., 2013; Bittsánszky et al., 2015). Therefore, we searched for other mechanisms that could explain these responses, such as the AOP and COP in vivo activities.
Oxygen uptake and the electron partitioning to the AOP were similar in Socaire under both N sources (Figure 8). In contrast to Socaire, Faro showed better yielding of respiration by higher Vt via COP (Figure 8). A possible explanation for this phenomenon can be based on the occurrence of a “futile ammonium cycling” (Hachiya and Noguchi, 2011), in which the increase of NH4+ fluxes across the plasma membrane is accompanied by H+ extrusion (by the plasma membrane proton-ATPase) to maintain the cytosolic charge balance (Britto and Kronzucker, 2005; Szczerba et al., 2008). This would require large amounts of ATP, helping to explain the increase in O2 consumption via COP (Kronzucker et al., 2001). In fact, an active H+ efflux to avoid cytosolic acidification and to release both proton and acid compounds from inside the cell to the rhizosphere was related to an ammonium-dependent increase of O2 uptake in species adapted to acidic soils (Findenegg, 1987; Britto and Kronzucker, 2002; Zhu et al., 2009). Thus, it seems that increases in Vt and COP activity can contribute to adaptation to acidic soils. On top of this, cell replication and the production of proteins are processes that require the highest quantities of ATP in plants (Liang et al., 2015). In this sense, higher rates of COP in Faro could be a key strategy for the benefit of energy and protein production required for plant development.
Interestingly, Socaire displayed a significantly higher valt than Faro under NH4+ source. Previous studies suggested a role for AOP during the dissipation of reducing equivalents in cytosol to compensate for the lack of the reductant sink exerted by nitrate reductase under NH4+ source (Britto et al., 2002; Escobar et al., 2006; Hachiya et al., 2010). Furthermore, the AOP has an important role in dissipating NAD(P)H, under different stress conditions when the COP is impaired, including those prevailing in high mountain habitats, such as cold, low oxygen, and high light intensities (Angert et al., 2012; Jayawardhane et al., 2020). In this sense, the AOP could be more important in Socaire (from Andes mountains) than in Faro (from Lowlands).
In roots, the higher increase of protein content under NH4+ compared to in Faro (Figure 3) could contribute to avoid toxicity by NH4+. This is consistent with lower changes in lipid peroxidation under NH4+ compared to Socaire (Figure 6). It has been proposed that the accumulation of NH4+ in shoots is more deleterious than in roots (Hachiya et al., 2021); however, the NH4+ per se is not the inductor of ammonium toxicity, but rather an excessive proton production by the incorporation of NH4+ in Glu to form Gln by glutamine synthase in the chloroplast (Fangmeier et al., 1994; Tobin and Yamaya, 2001; Hofmockel et al., 2010; Hachiya et al., 2021). Thus, the high production of amino acids and proteins in roots could act as a barrier to prevent the transport of NH4+ to shoots (Hachiya et al., 2021). The increase of proteins in Faro roots under NH4+ compared to was related to TTS and starch reductions, supporting the idea that an enhanced NH4+ assimilation takes place belowground in this ecotype (Figure 4). These changes could be related to the donor of C skeletons for the different processes associated with respiration and protein production (glycolysis, tricarboxylic acid cycle, and amino acid production) (De la Peña et al., 2019). Besides amino acids and proteins, pigments are also sinks of NH4+. Socaire, which showed the highest %N under NH4+, displayed small changes in protein content under NH4+ supply compared to (Figure 3), but presented alternative sinks to cope with the excess of NH4+. Betacyanins (Figure 5C) constitute a class of secondary metabolites in Quinoa derived from the amino acids Tyr and DOPA (Sepúlveda-Jiménez et al., 2005). Betacyanins have been related to scavenging ROS under stress conditions but have been inversely related to growth in quinoa (Bascuñán-Godoy et al., 2018). In contrast to Socaire, Faro increased chlorophylls (derived from Glu) under NH4+ compared to (Figures 5A, B). Chlorophyll has been positively related to both performance of PSII and growth in quinoa (Bascuñán-Godoy et al., 2018). The improvement of betacyanins in Socaire, and the higher level of valt, when compared to Faro, may indicate a role of AOP in dissipating energy excess from chloroplasts, helping to maintain homeostasis of metabolism under NH4+ source. In this sense, a described “trade-off” between the traits of resistance and productivity (Bechtold and Field, 2018) may help to explain the growth reduction observed in Socaire. Conversely, the maintenance of photosynthesis, an enhancement in Vt, and the production of soluble proteins and pigments related to the light collection in Faro suggest the existence of a tight metabolic coordination between chloroplasts and mitochondria. The application of “omics” technologies in future experiments would shed more light on important metabolite pathways for plant performance under NH4+ source.
Considering that agroecosystems have the potential to store a vast amount of C, in this work, we highlight the role of soil N sources in the ability to grow and store biomass in two ecotypes of C. quinoa. Similar physiological performance was observed in Andean and Lowland ecotypes under source, but under NH4+, these showed contrasting C:N relationships that were not related to photosynthesis, but to biomass accumulation and ATP yield of respiration. The enhanced respiration via COP in Faro under NH4+ turned out to be beneficial through the increased energy efficiency of respiration, allowing it to maintain growth, in contrast to Socaire, whose biomass was severely affected. Studies under field conditions and using a wider range of genotypes of each environment are necessary to establish that our findings are a general response. In view of the suggestions about alterations in the N forms available for plants due to climatic variations, increasing our understanding of plant nutrition is relevant, especially in places acutely threatened by climate change, such as the Andean zone.
The original contributions presented in the study are included in the article/Supplementary Material. Further inquiries can be directed to the corresponding author.
LB-G, ND-S, EO-G, SF and PC designed the assays and led the writing of the manuscript. MJ conducted all the experimental analysis. JO, CC, EE and CS performed assays and measurements. LB-G, ND-S, MR-C, TP and PC led the supported projects and edited the manuscript. All authors contributed to the article and approved the submitted version.
This work was supported by ANID Fondecyt Regular N° 1211473. Others funding were provided by FONDECYT No. 1191118 from National Agency for Research and Development (ANID), and Europea-Next Generation EU. However, views and opinions expressed are however those of the author(s) only and do not necessarily reflect those of the European Union.
We thank INIA-Intihuasi Chile National Seed Bank Repository for providing quinoa seeds. We are very grateful to Dr. Biel Martorell at the Serveis Cientifico-Tecnics of the Universitat de les Illes Balears for his help while running IRMS experiments. We also thank Miquel Truyols and collaborators of the UIB Experimental Field and Greenhouses.
The authors declare that the research was conducted in the absence of any commercial or financial relationships that could be construed as a potential conflict of interest.
All claims expressed in this article are solely those of the authors and do not necessarily represent those of their affiliated organizations, or those of the publisher, the editors and the reviewers. Any product that may be evaluated in this article, or claim that may be made by its manufacturer, is not guaranteed or endorsed by the publisher.
The Supplementary Material for this article can be found online at: https://www.frontiersin.org/articles/10.3389/fpls.2023.1070472/full#supplementary-material
Supplementary Figure 1 | Total dry biomass under different N sources and concentrations in two ecotypes of C. quinoa. Plants were subjected to different or supplies from 0 to 100 mM per 30 days. Values are means ± SE (n = 7). Different letters show statistical differences using three-way ANOVA considering ecotypes and source of N and concentration as factors (Tukey test; p < 0.05).
Supplementary Figure 2 | C allocation between aboveground and belowground in response to and . TSS (A, D), Starch (B, E) and NSC (C, F) in aboveground and belowground tissues in whole juvenile plants of two ecotypes of C. quinoa. Bars are means ± SD (n = 5). Two-way ANOVA of and Tukey test analysis (p < 0.05) were used to detect differences.
Supplementary Figure 3 | Total dry biomass of Socaire under different concentrations compared to 20mM . Plants were grown at different N treatments per 30 days at the same growing conditions described in Material and Methods. Values are means ± SE (n = 5). Different letters show statistical differences using three-way ANOVA considering ecotypes and source of N and concentration as factors (Tukey test; p < 0.05).
Supplementary Figure 4 | Percent changes in total dry biomass of Socaire (orange) and Faro (blue) under different : ratios in two ecotypes of C. quinoa. Plants were subjected to 20 mM of N, differing or ratios at the same growing conditions described in Material and Methods per 40 days. The studied proportions were: 100:0; 75:25; 50/50; 25:75 and 0:100 of : ratios. The 100:0 of : ratio was used as Control (100%). Tukey test was used to identify differences regarding 100: 0 : (p < 0.05). Values are means ± SE (n = 5). For each genotype, ns indicates no significant difference, * indicates p < 0.05, ** indicates p < 0.01, and *** indicates p < 0.001.
Abderrahim, F., Huanatico, E., Segura, R., Arribas, S., Gonzalez, M. C., Condezo-Hoyos, L. (2015). Physical features, phenolic compounds, betalains and total antioxidant capacity of coloured quinoa seeds (Chenopodium quinoa willd.) from Peruvian altiplano. Food Chem. 183, 83–90. doi: 10.1016/j.foodchem.2015.03.029
Ackerman, D., Millet, D. B., Chen, X. (2019). Global estimates of inorganic nitrogen deposition across four decades. Global Biogeochem. Cy. 33, 100–107. doi: 10.1029/2018GB005990
Alencar, V. T. C. B., Lobo, A. K. M., Carvalho, F. E. L., Silveira, J. A. G. (2019). High ammonium supply impairs photosynthetic efficiency in rice exposed to excess light. Photosynth. Res. 140, 321–335. doi: 10.1007/s11120-019-00614-z
Angert, A., Rodeghiero, M., Griffin, K. (2012). High alternative oxidase activity in cold soils and its implication to the dole effect. Geophys. Res. Lett. 39, 16710. doi: 10.1029/2012GL052719
Ariz, I., Asensio, A. C., Zamarreño, A. M., García-Mina, J. M., Aparicio-Tejo, P. M., Moran, J. F. (2013). Changes in the C/N balance caused by increasing external ammonium concentrations are driven by carbon and energy availabilities during ammonium nutrition in pea plants: the key roles of asparagine synthetase and anaplerotic enzymes. Physiol. Plant 148 (4), 522–537. doi: 10.1111/j.1399-3054.2012.01712.x
Auyeung, D. S. N., Martiny, J. B. H., Dukes, J. S. (2015). Nitrification kinetics and ammonia-oxidizing community respond to warming and altered precipitation. Ecosphere 6 (5), 83. doi: 10.1890/ES14-00481.1
Barnard, R., Roux, X. L. E., Hungate, B. A., Cleland, E. E., Blankinship, J. C., Barthes, L., et al. (2006). Several components of global change alter nitrifying and denitrifying activities in an annual grassland. Funct. Ecol. 20 (4), 557–564. doi: 10.1111/j.1365-2435.2006.01146.x
Bascuñán-Godoy, L., Sanhueza, C., Pinto, K., Cifuentes, L., Reguera, M., Briones, V., et al. (2018). Nitrogen physiology of contrasting genotypes of Chenopodium Quinoa Willd. (Amaranthaceae). Sci. Rep. 8, 17524. doi: 10.1038/s41598-018-34656-5
Bazile, D., Martínez, E. A., Fuentes, F. (2014). Diversity of quinoa in a biogeographical island: a review of constraints and potential from arid to temperate regions of Chile. Not. Bot. Horti. Agrobo. 42 (2), 289–298. doi: 10.1583/nbha4229733
Bechtold, U., Field, B. (2018). Molecular mechanisms controlling plant growth during abiotic stress. J. Exp. Bot. 69 (11), 2753–2758. doi: 10.1093/jxb/ery157
Bittsánszky, A., Pilinszky, K., Gyulai, G., Komives, T. (2015). Overcoming ammonium toxicity. Plant Sci. 231, 184–190. doi: 10.1016/j.plantsci.2014.12.005
Blake, L. (2005). “Acid rain and soil acidification. reference module in earth systems and environmental sciences,” in Encyclopedia of soils in the environment (Ireland: Elsevier) (2005), 1–11. doi: 10.1016/B0-12-348530-4/00083-7
Bradford, M. M. (1976). A rapid and sensitive method for the quantitation of microgram quantities of protein utilizing the principle of protein-dye binding. Anal Biochem. 72, 248–54.
Breuer, L., Kiese, R., Butterbach-Bahl, K. (2002). Temperature and moisture effects on nitrification rates in tropical rain-forest soils. Soil Sci. Soc Am. J. 66 (3), 834–844. doi: 10.2136/sssaj2002.8340
Britto, D. T., Kronzucker, H. J. (2002). NH4+ toxicity in higher plants: a critical review. J. Plant Physiol. 159, 567–584. doi: 10.1078/0176-1617-0774
Britto, D. T., Siddiqi, M. Y., Glass, A. D. M., Kronzucker, H. J. (2001). Futile transmembrane NH4+ cycling: a cellular hypothesis to explain ammonium toxicity in plants. P. Nat. A. Sci. 98 (7), 4255–4258. doi: 10.1073/pnas.061034698
Britto, D. T., Kronzucker, H. J. (2005). Nitrogen acquisition, PEP carboxylase, and cellular pH homeostasis: new views on old paradigms. Plant Cell Environ 28 (11), 1396–1409. doi: 10.1111/j.1365-3040.2005.01372.x
Britto, D. T., Siddiqi, M. Y., Glass, A. D. M., Kronzucker, H. J. (2002). Subcellular NH4+ flux analysis in leaf segments of wheat (Triticum aestivum). New Phytologist 155 (3), 373–380. doi: 10.1046/j.1469-8137.2002.00471.x
Cárdenas-Castillo, J. E., Delatorre-Herrera, J., Bascuñán-Godoy, L., Rodriguez, J. P. (2021). Quinoa (Chenopodium quinoa Wild.) seed yield and efficiency in soils deficient of nitrogen in the Bolivian altiplano: an analytical review. Plants 10, 2479. doi: 10.3390/plants10112479
Chow, P. S., Landhäusser, S. M. (2004). A method for routine measurements of total sugar and starch content in woody plant tissues. Tree Physiol. 24 (10), 1129–1136. doi: 10.1093/treephys/24.10.1129
Coskun, D., Britto, D. T., Kronzucker, H. J. (2016). Nutrient constraints on terrestrial carbon fixation: the role of nitrogen. J. Plant Physiol. 203, 95–109. doi: 10.1016/j.jplph.2016.05.016
Daryanto, S., Wang, L., Gilhooly, W. P., III, Jacinthe, P. A. (2019). Nitrogen preference across generations under changing ammonium nitrate ratios. J. Plant Ecol. 12 (2), 235–244. doi: 10.1093/jpe/rty014
De la Peña, M., González-Moro, M. B., Marino, D. (2019). Providing carbon skeletons to sustain amide synthesis in roots underlines the suitability of brachypodium distachyon for the study of ammonium stress in cereals. AoB. Plants 11 (3), plz029. doi: 10.1093/aobpla/plz029
Del-Saz, N. F., Douthe, C., Carriquí, M., Ortíz, J., Sanhueza, C., Rivas-Medina, A., et al. (2021). Different metabolic roles for alternative oxidase in leaves of palustrine and terrestrial species. Front. Plant Sci. 12. doi: 10.3389/fpls.2021.752795
Del-Saz, N. F., Ribas-Carbo, M., Martorell, G., Fernie, A. R., Florez-Sarasa, I. (2017). “Measurements of electron partitioning between cytochrome and alternative oxidase pathways in plant tissues,” in Plant respiration and internal oxygen (New York, NY: Humana Press), 203–217.
Del-Saz, N. F., Ribas-Carbo, M., McDonald, A. E., Lambers, H., Fernie, A. R., Florez-Sarasa, I. (2018). An In Vivo perspective of the role(s) of the alternative oxidase pathway. Trends Plant Sci. 23 (3), 206–219. doi: 10.1016/j.tplants.2017.11.006
Dickinson, R. E. (1979). Analytical procedures for the sequential extraction of 14C-labeled constituents from leaves, bark and wood of cottonwood plants. Physiol. Plant 45 (4), 480–488. doi: 10.1111/j.1399-3054.1979.tb02618.x
Escobar, M. A., Geisler, D. A., Rasmusson, A. G. (2006). Reorganization of the alternative pathways of the arabidopsis respiratory chain by nitrogen supply: opposing effects of ammonium and nitrate. Plant J. 45, 775–788. doi: 10.1111/j.1365-313X.2005.02640.x
Fangmeier, A., Hadwiger-Fangmeier, A., van der Eerden, L., Jäger, H. J. (1994). Effects of atmospheric ammonia on vegetation–a review. Environ. pollut. 86 (1), 43–82. doi: 10.1016/0269-7491(94)90008-6
FAO (2011) The state of food insecurity in the world: how does international price volatility affect domestic economies and food security? Available at: http://www.fao.org/docrep/014/i2330e/i2330e00.htm.
Findenegg, G. R. (1987). A comparative study of ammonium toxicity at different constant pH of the nutrient solution. Plant Soil. 103, 239–243. doi: 10.1007/BF02370395
Fischer, S., Wilckens, R., Jara, J., Aranda, M. (2013). Controlled water stress to improve functional and nutritional quality in quinoa seed. Boletín Latinoamericano y del. Caribe Plantas Medicinales y Aromáticas (BLACPMA) 12, 457–468.
Florez-Sarasa, I., Ribas-Carbo, M., Del-Saz, N., Schwahn, K., Nikoloski, Z., Fernie, A. R., et al. (2016). Unravelling the in vivo regulation and metabolic role of the alternative oxidase pathway in C3 species under photoinhibitory conditions. New Phytol. 212, 66–79. doi: 10.1111/nph.14030
Forster, J. C. (1995). “Soil nitrogen,” in Methods in applied soil microbiology and biochemistry. chapter 3: soil sampling, handling, storage andanalysis. Eds. Alef, K., Nanniperi, P. (San Diego, CA: Academic Press Inc.), 79–116.
García, M., Raes, D., Jacobsen, S. E., Michel, T. (2007). Agroclimatic constraints for rainfed agriculture in the Bolivian altiplano. J. Arid. Environ. 71, 109–121. doi: 10.1016/j.jaridenv.2007.02.005
Greaver, T. L., Clark, C. M., Compton, J. E., Vallano, D., Talhelm, A. F., Weaver, C. P., et al. (2016). Key ecological responses to nitrogen are altered by climate change. Nat. Clim. Change 6 (9), 836–843. doi: 10.1038/nclimate3088
Guo, S., Schinner, K., Sattelmacher, B., Hansen, U.-P. (2005). Different apparent CO2 compensation points in nitrate- and ammonium-grown phaseolus vulgaris and the relationship to non-photorespiratory CO2 evolution. Physiol. Plant 123, 288–301. doi: 10.1111/j.1399-3054.2005.00467.x
Hachiya, T., Inaba, J., Wakazaki, M., Sato, M., Toyooka, K., Miyagi, A., et al. (2021). Excessive ammonium assimilation by plastidic glutamine synthetase causes ammonium toxicity in Arabidopsis thaliana. Nat. Commun. 12, 4944. doi: 10.1038/s41467-021-25238-7
Hachiya, T., Noguchi, K. (2011). Integrative response of plant mitochondrial electron transport chain to nitrogen source. Plant Cell Rep. 30, 195–204. doi: 10.1007/s00299-010-0955-0
Hachiya, T., Sakakibara, T. (2017). Interactions between nitrate and ammonium in their uptake, allocation, assimilation, and signaling in plants. J. Exp. Bot. 68 (10), 2501–2512. doi: 10.1093/jxb/erw449
Hachiya, T., Watanabe, C. K., Boom, C., Tholen, D., Takahara, K., Kawai-Yamada, M., et al (2010). Ammonium-dependent respiratory increase is dependent on the cytochrome pathway in Arabidopsis thaliana shoots. Plant Cell Environ 33 (11), 1888–97. doi: 10.1111/j.1365-3040.2010.02189.x
Hofmockel, K. S., Fierer, N., Colman, B. P., Jackson, R. B. (2010). Amino acid abundance and proteolytic potential in north American soils. Oecologia 163 (4), 1069–1078. doi: 10.1007/s00442-010-1601-9
Jarvis, D. E, Ho, Y. S., Lightfoot, D. J., Schmöckel, S. M., Li, B., Borm, T. J. A., et al. (2017). The genome of Chenopodium quinoa. Nature 542, 307–312. doi: 10.1038/nature21370
Jayawardhane, J., Cochrane, D. W., Vyas, P., Bykova, N. V., Vanlerberghe, G. C., Igamberdiev, A. U. (2020). Roles for plant mitochondrial alternative oxidase under normoxia, hypoxia, and reoxygenation conditions. Front. Plant Sci. 11. doi: 10.3389/fpls.2020.00566
Kant, S., Bi, Y. M., Rothstein, S. J. (2011). Understanding plant response to nitrogen limitation for the improvement of crop nitrogen use efficiency. J. Exp. Bot. 62 (4), 1499–1509. doi: 10.1093/jxb/erq297
Kronzucker, H. J., Britto, D. T., Davenport, R. J., Tester, M. (2001). Ammonium toxicity and the real cost of transport. Trends Plant Sci. 6 (8), 335–337. doi: 10.1016/S1360-1385(01)02022-2
Liang, C., Zhang, Y., Cheng, S., Osorio, S., Sun, Y., Fernie, A. R., et al. (2015). Impacts of high ATP supply from chloroplasts and mitochondria on the leaf metabolism of arabidopsis thaliana. Front. Plant Sci. 6. doi: 10.3389/fpls.2015.00922
Lichtenthaler, H. K., Buschmann, C. (2001). Chlorophylls and carotenoids: measurement and characterization by UV-VIS spectroscopy. Curr. Protoc. Food Anal. Chem. 1, F4.3.1–F4.3.8. doi: 10.1002/0471142913.faf0403s01
Luzio, W., Casanova, M., Seguel, O. (2010). “Suelos de la zona desértica,” in Suelos de Chile, vol. 2010. Ed. Luzio, W. (Chile: Departamento de Ingeniería y Suelos. Facultad de Ciencias Agronómicas. Universidad de Chile) 364. Available at: https://bibliotecadigital.ciren.cl/handle/20.500.13082/21767.
Luzio, W., Norambuena, P., Vera, W., Casanova, M. (2002). Génesis y propiedades de algunos suelos del altiplano de Chile. Ciencia del suelo y Nutricion Vegetal 3 (1), 37–52.
Marquis, R. J., Newell, E. A., Villegas, A. C. (1997). Non-structural carbohydrate accumulation and use in an understorey rain-forest shrub and relevance for the impact of leaf herbivory. Funct. Ecol. 11 (5), 636–643. doi: 10.1046/j.1365-2435.1997.00139.x
Martinez, E. A., Veas, E., Jorquera, C., San Martín, R., Jara, P. (2009). Re-introduction of quínoa into arid Chile: cultivation of two lowland races under extremely low irrigation. J. Agron. Crop Sci. 195, 1–10. doi: 10.1111/j.1439-037X.2008.00332.x
Maughan, P. J., Chaney, L., Lightfoot, D. J., Cox, B. J., Mark, T., Jellen, R., et al. (2019). Mitochondrial and chloroplast genomes provide insights into the evolutionary origins of quinoa (Chenopodium quinoa willd.). Sci. Rep. 9, 185. doi: 10.1038/s41598-018-36693-6
Miller, A. J., Cramer, M. D. (2005). Root nitrogen acquisition and assimilation. Plant Soil 274, 1–36. doi: 10.1007/s11104-004-0965-1
Murashige, T., Skoog, F. (1962). A revised medium for rapid growth and bio assays with tobacco tissue cultures. Physiol. Plant 15, 474–497. doi: 10.1111/j.1399-3054.1962.tb08052.x
Myers, S. S., Zanobetti, A., Kloog, I., Huybers, P., Leakey, A. D. B., Bloom, A. J., et al. (2014). Increasing CO2 threatens human nutrition. Nature 510, 139–142. doi: 10.1038/nature13179
O’Leary, B. M., Asao, S., Millar, A. H., Atkin, O. K. (2019). Core principles which explain variation in respiration across biological scales. New Phytol. 222 (2), 670–686. doi: 10.1111/nph.15576
Ortega-Villasante, C., Rellán-Álvarez, R., Del Campo, F. F., Carpena-Ruiz, R. O., Hernández, L. E. (2005). Cellular damage induced by cadmium and mercury in medicago sativa. J. Exp. Bot. 56 (418), 2239–2251. doi: 10.1093/jxb/eri223
Ortiz, J., Sanhueza, C., Romero-Munar, A., Hidalgo-Castellanos, J., Castro, C., Bascuñán-Godoy, L., et al. (2020). In Vivo Metabolic regulation of alternative oxidase under nutrient deficiency–interaction with arbuscular mycorrhizal fungi and rhizobium bacteria. Int. J. Mol. Sci. 21 (12), 4201. doi: 10.3390/ijms21124201
Pinto-Irish, K., Coba de la Peña, T., Ostria-Gallardo, E., Ibáñez, C., Briones, V., Vergara, A., et al. (2020). Seed characterization and early nitrogen metabolism performance of seedlings from altiplano and coastal ecotypes of quinoa. BMC Plant Biol. 20 (343), 1–18. doi: 10.21203/rs.2.24363/v2
Planella, M. T., López, M. L., Bruno, M. C. (2015). “Domestication and prehistoric distribution. chapter 1.3,” in FAO & CIRAD. state of the art report of quinoa in the world in 2013. Eds. Bazile, D., Bertero, D., Nieto, C.(Rome: FAO & CIRAD), 29–55. Available at: https://www.fao.org/documents/card/fr//3638200e-1fbb-4d7e-a359-d8f582b1d082/.
Podgórska, A., Gieczewska, K., Lukawska-Kuźma, K., Rasmusson, A. G., Gardeström, P., Szal, B. (2013). Long-term ammonium nutrition of arabidopsis increases the extrachloroplastic NAD(P)H/NAD(P)+ ratio and mitochondrial reactive oxygen species level in leaves but does not impair photosynthetic capacity. Plant Cell Environ. 36 (11), 2034–2045. doi: 10.1111/pce.12113
Rascón, J., Gosgot-Angeles, W., Quiñones-Huatangari, L., Oliva, M., Barrena Gurbillón, M. Á. (2021). Dry and wet events in Andean populations of northern Peru: a case study of chachapoyas, Peru. Front. Environ. Sci. 9. doi: 10.3389/fenvs.2021.614438
Ribas-Carbo, M., Robinson, S. A., Giles, L. (2005). “The application of the oxygen-isotope technique to assess respiratory pathway partitioning,” in Plant respiration. advances in photosynthesis and respiration, vol. 18 . Eds. Lambers, H., Ribas-Carbo, M. (Dordrecht: Springer). doi: 10.1007/1-4020-3589-6_3
Rigano, C., Di Martino Rigano, V., Vona, V., Carfagna, S., Carillo, P., Esposito, S. (1996). Ammonium assimilation by young plants of hordeum vulgare in light and darkness: effects on respiratory oxygen consumption by roots. New Phytol. 132, 375–382. doi: 10.1111/j.1469-8137.1996.tb01857.x
Rubio-Asensio, J. S., Bloom, A. J. (2017). Inorganic nitrogen form: a major player in wheat and arabidopsis responses to elevated CO2. J. Exp. Bot. 68 (10), 2611–2625. doi: 10.1093/jxb/erw465
Rubio-Asensio, J. S., Rachmilevitch, S., Bloom, A. J. (2015). Responses of arabidopsis and wheat to rising CO2 depend on nitrogen source and nighttime CO2 levels. Plant Physiol. 168 (1), 156–163. doi: 10.1104/pp.15.00110
Schittek, K., Kock, S. T., Lücke, A., Hence, J., Ohlendorf, C., Kulemeyer, J. J., et al. (2016). A high-altitud peatland record of environmental changes in the NW Argentine Andes (24° s) over the last 2100 years. Climate past 12, 1165–1180. doi: 10.5194/cp-12-1165-2016
Sepúlveda-Jiménez, G., Rueda-Benítez, P., Porta, H., Rocha-Sosa, M. (2005). A red beet (Beta vulgaris) UDP-glucosyltransferase gene induced by wounding, bacterial infiltration and oxidative stress. J. Exp. Bot. 56 (412), 605–611. doi: 10.1093/jxb/eri036
Smirnoff, N., Todd, P., Stewart, G. R. (1984). The occurrence of nitrate reductase in the leaves of woody plants. Ann. Bot. 54, 363–3374. doi: 10.1093/oxfordjournals.aob.a086806
SoilGrids (2021) A system for digital soil mapping based on global compilation of soil profile data and environmental layers. Available at: https://soilgrids.org (Accessed 3 January 2023).
Szczerba, M. W., Britto, D. T., Balkos, K. D., Kronzucker, H. J. (2008). Alleviation of rapid, futile ammonium cycling at the plasma membrane by potassium reveals k+-sensitive and -insensitive components of NH4+ transport. J. Exp. Bot. 59 (2), 303–313. doi: 10.1093/jxb/erm309
Tobin, A. K., Yamaya, T. (2001). Cellular compartmentation of ammonium assimilation in rice and barley. J. Exp. Bot. 52 (356), 591–604. doi: 10.1093/jexbot/52.356.591
Wang, W., Tai, F., Chen, S. (2008). Optimizing protein extraction from plant tissues for enhanced proteomics analysis. J. Sep. Sci. 11, 2032–2039. doi: 10.1002/jssc.200800087
Wang, F., Wang, Q., Yu, Q., Ye, J., Gao, J., Liu, H., et al. (2022). Is the NH4 +-induced growth inhibition caused by the NH4 + form of the nitrogen source or by soil acidification? Front. Plant Sci. 13. doi: 10.3389/fpls.2022.968707
Xu, G., Fan, X., Miller, A. J. (2012). Plant nitrogen assimilation and use efficiency. Ann. Rev. Plant Biol. 63, 153–182. doi: 10.1146/annurev-arplant-042811-105532
Zhu, Y., Di, T., Xu, G., Chen, X., Zeng, H., Yan, F., et al. (2009). Adaptation of plasma membrane h+-ATPase of rice roots to low pH as related to ammonium nutrition. Plant Cell Environ. 32, 1428–1440. doi: 10.1111/j.1365-3040.2009.02009.x
Keywords: ammonium, nitrate, ammonium-toxicity, landraces, photosynthetic performance, C metabolism, oxygen-isotope fractionation, alternative- oxidase
Citation: Jerez MP, Ortiz J, Castro C, Escobar E, Sanhueza C, Del-Saz NF, Ribas-Carbo M, Coba de la Peña T, Ostria-Gallardo E, Fischer S, Castro PA and Bascunan-Godoy L (2023) Nitrogen sources differentially affect respiration, growth, and carbon allocation in Andean and Lowland ecotypes of Chenopodium quinoa Willd. Front. Plant Sci. 14:1070472. doi: 10.3389/fpls.2023.1070472
Received: 14 October 2022; Accepted: 21 March 2023;
Published: 20 June 2023.
Edited by:
Barbara Vento, CONICET Mendoza, ArgentinaReviewed by:
Ana K. M. Lobo, Lancaster University, United KingdomCopyright © 2023 Jerez, Ortiz, Castro, Escobar, Sanhueza, Del-Saz, Ribas-Carbo, Coba de la Peña, Ostria-Gallardo, Fischer, Castro and Bascunan-Godoy. This is an open-access article distributed under the terms of the Creative Commons Attribution License (CC BY). The use, distribution or reproduction in other forums is permitted, provided the original author(s) and the copyright owner(s) are credited and that the original publication in this journal is cited, in accordance with accepted academic practice. No use, distribution or reproduction is permitted which does not comply with these terms.
*Correspondence: Luisa Bascunan-Godoy, bHViYXNjdW5AdWRlYy5jbA==; Néstor Fernández Del-Saz, bmVzdG9yLmZlcm5hbmRlekB1aWIuZXU=
Disclaimer: All claims expressed in this article are solely those of the authors and do not necessarily represent those of their affiliated organizations, or those of the publisher, the editors and the reviewers. Any product that may be evaluated in this article or claim that may be made by its manufacturer is not guaranteed or endorsed by the publisher.
Research integrity at Frontiers
Learn more about the work of our research integrity team to safeguard the quality of each article we publish.