- 1Department of Botany and Plant Sciences, University of California, Riverside, Riverside, CA, United States
- 2Institute of Integrative Genome Biology, University of California, Riverside, Riverside, CA, United States
Tomato (Solanum lycopersicum) is a model species for studying fruit development, wounding, herbivory, and pathogen attack. Despite tomato’s world-wide economic importance and the role of chloroplasts as metabolic hubs and integrators of environmental cues, little is known about the stromal proteome of tomato. Using a high-yielding protocol for chloroplast and stromal protein isolation, MudPIT nano-LC-MS/MS analyses, a robust in-house protein database (the Atlas) for predicting the plastid localization of tomato proteins, and rigorous selection criteria for inclusion/exclusion in the stromal proteome, we identified 1,278 proteins of the tomato stromal proteome. We provide one of the most robust stromal proteomes available to date with empirical evidence for 545 and 92 proteins not previously described for tomato plastids and the Arabidopsis stroma, respectively. The relative abundance of tomato stromal proteins was determined using the exponentially modified protein abundance index (emPAI). Comparison of the abundance of tomato and Arabidopsis stromal proteomes provided evidence for the species-specific nature of stromal protein homeostasis. The manual curation of the tomato stromal proteome classified proteins into ten functional categories resulting in an accessible compendium of tomato chloroplast proteins. After curation, only 91 proteins remained as unknown, uncharacterized or as enzymes with unknown functions. The curation of the tomato stromal proteins also indicated that tomato has a number of paralogous proteins, not present in Arabidopsis, which accumulated to different levels in chloroplasts. As some of these proteins function in key metabolic pathways or in perceiving or transmitting signals critical for plant adaptation to biotic and abiotic stress, these data suggest that tomato may modulate the bidirectional communication between chloroplasts and nuclei in a novel manner. The stromal proteome provides a fertile ground for future mechanistic studies in the field of tomato chloroplast-nuclear signaling and are foundational for our goal of elucidating the dynamics of the stromal proteome controlled by the solanaceous-specific, stromal, and wound-inducible leucine aminopeptidase A of tomato.
Introduction
Plastids are essential organelles of green algae, land plants and some protists. Differentiating from proplastids, plastids develop into numerous forms, are tissue-specific and formed in response to endogenous signals (Jarvis and López-Juez, 2013). Well known for their role in photosynthesis, chloroplasts are metabolic hubs engaged in the biosynthesis of amino acids, starch, fatty acids, lipids, terpenoids, purine and pyrimidine bases, various pigments, vitamins, co-factors, as well as major biochemical pathways, such as nitrogen and sulfur metabolism (Rolland et al., 2012; Buchanan et al., 2015).
Approximately 2,500 proteins reside within chloroplasts (Abdallah et al., 2000). The vast majority are nuclear genome encoded, synthesized in the cytosol, imported into the chloroplast, and sorted into one of six sub-compartments (Cline and Dabney-Smith, 2008; Nakai, 2018; Thomson et al., 2020). N-terminal transit peptides facilitate the import of these proteins, while other proteins use non-canonical pathways for entering the chloroplast, including transit through the endoplasmic reticulum (Armbruster et al., 2009; Jarvis and López-Juez, 2013; Thomson et al., 2020).
Due to the emergence of its well-annotated genome in 2000 (Initiative, 2000), proteomes of Arabidopsis thaliana organelles including chloroplasts, mitochondria, peroxisomes, and vacuoles have been intensively studied (Carter et al., 2004; Kleffmann et al., 2004; Millar et al., 2006; Reumann et al., 2007; Zybailov et al., 2008). This includes the protein cohorts in Arabidopsis chloroplast sub-compartments: the envelope, stroma, thylakoid membrane, and lumen (Peltier et al., 2002; Schubert et al., 2002; Ferro et al., 2003; Friso et al., 2004; Peltier et al., 2006; Olinares et al., 2010). Several studies have combined gel or column fractionation in conjunction with mass spectroscopy (MS/MS) to elucidate the oligomeric complexes of the chloroplast (Peltier et al., 2006; Olinares et al., 2010; Lundquist et al., 2017). Finally, the proteomes of different plastid forms have also been established for developing plastids and chloroplasts from maize and eucalyptus (Majeran et al., 2012; Baldassi and Balbuena, 2022), potato leaf chloroplasts (Liu et al., 2022), wheat amyloplasts (Andon et al., 2002), rice and barley etioplasts (Von Zychlinski et al., 2005; Ploscher et al., 2011), tobacco proplastids (Baginsky et al., 2004), and chromoplasts from seven species (Siddique et al., 2006; Barsan et al., 2010; Barsan et al., 2012; Wang et al., 2013).
Of particular interest is the chloroplast’s role in sensing and transmitting signals to report organellar and cellular homeostasis (de Souza et al., 2017; Krupinska et al., 2020; Unal et al., 2020; Wang Y. et al., 2020). Chloroplasts have intimate and dynamic relationships with other organelles such as the nucleus, peroxisomes, mitochondria, and endomembrane system to enable signaling of cellular stress (Mehrshahi et al., 2013; Oikawa et al., 2019; Mullineaux et al., 2020). The diversity of signal pathways has primarily been elucidated genetically and biochemically in Arabidopsis allowing the discovery of a diverse set of metabolites (e.g., reactive oxygen species, isoprenoid intermediates, phosphonucleotides, chlorophyll precursors, carotenoid metabolites) and transcription factors to orchestrate these crucial communications (de Souza et al., 2017; Wang Y. et al., 2020). In addition, recent studies in Arabidopsis and other plants have shown that the chloroplast serves as a critical signaling hub in plant-pathogen interactions (Fernandez and Burch-Smith, 2019; Yang et al., 2021).
Defining the constituents of chloroplast proteomes and their dynamics in response to biotic and abiotic stress in crop plants is an emerging research area. In tomato, the stromal protein leucine aminopeptidase (LAP-A) controls expression of nuclear genes after herbivory, wounding and treatments with methyl jasmonate (Fowler et al., 2009; Scranton et al., 2013). The bifunctional LAP-A has both aminopeptidase and chaperone activities (Gu et al., 1999; Scranton et al., 2012) and LAP-A-dependent signal(s) may be generated post-translationally to orchestrate chloroplast-to-nucleus communication. With our long-term objective of understanding the LAP-A-dependent stromal proteome dynamics, we have determined a foundational component – tomato’s chloroplast stromal proteome.
Recent advances in sensitivity and accuracy in mass spectrometry joined with the availability of the annotated tomato nuclear and chloroplast genomes and a high-yielding tomato chloroplast and stromal protein isolation protocol, has allowed for an unprecedented in-depth understanding of tomato’s chloroplast stroma (Sato et al., 1999; Kahlau et al., 2006; Bhattacharya et al., 2020). Using nanoLC-MS/MS and two strategies to detect stromal proteins, we provide strong empirical evidence for 1,278 proteins in the tomato stromal proteome. With minimal contamination from other subcellular fractions of the chloroplast, this represents the largest stromal proteome to date and provides an important insight into the complexity of the eudicot stromal proteome. Our proteome adds 545 new proteins to previous studies that characterized the tomato chromoplast (Barsan et al., 2010; Barsan et al., 2012) and 130 proteins not previously identified in a wide range of Arabidopsis thaliana proteomics studies (Sun et al., 2009; Hooper et al., 2017). Tomato’s stromal proteins were manually curated and classified into ten protein functional categories allowing accessibility of our dataset.
Materials and methods
Chloroplast and stromal protein isolation
Tomato plants (Solanum lycopersicum UC82b) were grown to the three-to-four true-leaf stage (five-weeks-old) as described in Bhattacharya et al. (2020). Briefly, surface-sterilized tomato seeds were grown in UC Soil Mix 3 in flats with 18-section inserts in a growth chamber at 28 °C for 16 hr with 400 µmol m-2 s-1 light and 22 °C for 8 hr (dark). Plants were watered daily and fertilized weekly with a 0.35% (w/v) Miracle-Gro Tomato Plant Food solution. Twenty-seven hr prior to the chloroplast isolation, tomato plants were transferred to the dark to reduce starch. Five independent chloroplast preparations were made using leaves from 18 dark-adapted plants per preparation. Chloroplasts were isolated using a high-yielding chloroplast and stromal protein isolation method optimized for tomato leaves (Bhattacharya et al., 2020).
For each biological replicate, chloroplast soluble proteins (110 µg) were precipitated with four volumes of acetone for 16 hr at -20 °C and pelleted at 15,000 g for 30 min at 4 °C. The supernatant was discarded. The pellet was manually dislodged and washed with 1 mL of methanol to remove residual water. The sample was centrifuged at 15,000 g for 15 min at 4 °C. Supernatant was removed. The protein pellet was air-dried and stored at -20 °C until use.
To enhance identification of chloroplast stromal proteins, which may be obscured by abundant proteins in the 55- to 75-kDa range, stromal proteins (100 µg/lane) were fractionated by 12% SDS-PAGE and gels were stained with Coomassie Blue R-250 (Gu et al., 1996b; Rosenberg et al., 1997). The gel section with the 50- to 75-kDa proteins was excised and discarded. The proteins in remaining gel fragments were separated into three fractions based on mass (Figure S1). Proteins that were > 75-kDa (high mass) and < 20-kDa (low mass) proteins were pooled for analysis. The high plus low mass and the intermediate mass protein (50- to 20-kDa) samples had similar protein levels. Gel pieces were minced and destained in 50 mM ammonium bicarbonate in 50% acetonitrile with vigorous shaking at room temperature for 30 min. Destaining was repeated until gel pieces were devoid of Coomassie Blue R-250. After the final wash, gel pieces were dehydrated in 100% acetonitrile for 50 min at room temperature with vigorous shaking. Gel pieces were dried using a SpeedVac for 15 min at 30 °C and stored at -20 °C until use.
Acetone protein pellets were resuspended in 100 µL trypsin solution (10 µg/mL trypsin, 50 mM ammonium bicarbonate (pH 8), 10% acetonitrile) and incubated at 37 °C overnight. The gel protein samples were soaked with sufficient volume of trypsin solution (10 µg/ml trypsin, 50 mM ammonium bicarbonate) and incubated overnight at 37 °C. After trypsin digestion, five acetone-precipitated and three gel-extracted stromal protein samples were analyzed by nanoLC-MS/MS.
NanoLC-MS/MS
A MudPIT approach was employed to analyze the trypsin-treated samples at the UC Riverside Institute of Integrative Biology Core by Dr. Songqin Pan. A nanoAcquity UPLC (Waters, Milford, MA) and an Orbitrap Fusion MS (Thermo Scientific, San Jose, CA) were configured to perform online 2D-nanoLC/MS/MS analysis. 2D-nanoLC was performed online using the nanoAcquity UPLC in an At-Column Dilution configuration. The first-dimension LC mobile phases were 20 mM ammonium formate (pH 10) (mobile phase A) and acetonitrile (mobile phase B) and was achieved with five-min elutions off a NanoEase trap column (Waters) using five stepwise increases in acetonitrile (13%, 18%, 21.5%, 27%, and 50% acetonitrile). A final flushing step with 80% acetonitrile was used to clean the column. Each fraction was then analyzed online using a second dimension LC gradient. The second dimension nano-UPLC method was described previously (Drakakaki et al., 2012).
Orbitrap Fusion MS method was based on a data-dependent acquisition (DDA) survey. The MS-acquired data from 1 to 69 min over a 70-min gradient. The nanoESI source was used with spray voltage at 2000 V, sweep gas at 0, and ion transfer tube temperature at 275 °C. Orbitrap mass analyzer was used for MS1 scan with resolution set at 60,000. MS mass range was 300-1800 m/z. AGC target for each scan was set at 500,000 with maximal ion injection time set at 100 ms.
Precursor ions with intensity 10,000 or higher were selected for MS2 scans, which were performed with the Ion-Trap mass analyzer in the rapid scan mode. The sequence of individual MS2 scans was from the most- to least-intense precursor ions using the top-speed mode and a cycle time of 4 sec. Precursor ions apex peak detection was enabled, using an expected peak width of 10 sec and Desired Apex Window set to 30%. The minimum peak intensity threshold was set to 1e4. Higher-energy collisional dissociation (HCD) with 25-35% normalized activation energy was used for fragmentation. The quadrupole was used for precursor isolation with 2 m/z isolation window. MS2 mass range was set to auto/normal with the first mass set at 120 m/z. Maximal injection time was 100 msec with the AGC target set at 10,000. Ions were injected for all available parallelizable time. A 120-sec exclusion window was applied to all abundant ions to avoid repetitive MS2 scanning on the same precursor ions using 10 ppm error tolerance. Charge states from 2 to 8 were selected for MS2 scan and undetermined charge states were excluded. All MS2 spectra were recorded in the centroid mode.
The raw MS files were processed and analyzed using Proteome Discoverer version 2.1 (Thermo Scientific, San Jose, CA). Sequest HT search engine was used to match all MS data to a tomato protein database (ITAG 2.4 annotation release) or the tomato Atlas (see below) and concatenated target/decoy databases were used for determining false discovery rates (Elias et al., 2005). The search parameters were the following: trypsin with two missed cleavages, minimal peptide length of six amino acids, MS1 mass tolerance 20 ppm, MS2 mass tolerance 0.6 Da, and Gln→pyro-Glu (N-term Q), oxidation (M), and N-terminal acetylation as variable modifications. Only proteins with 1% FDR cut-off were considered in the final result. Primary data is summarized in Table S1. The mass spectrometry proteomics data have been deposited to the ProteomeXchange Consortium via the PRIDE partner repository with the dataset identifier PXD035944.
Annotation of the stromal proteome
All identified proteins (1% FDR) were manually annotated. Peptide spectral matches (PSMs) and frequency of detection in tomato eight stromal samples were the first criteria for inclusion/exclusion of the tomato chloroplast soluble proteome. Proteins that were detected once with 1 PSM, identified with a single peptide or sporadically identified (in less than 40% of the samples analyzed) were removed from consideration (Bhattacharya et al., 2020). The exceptions were proteins that had empirical evidence for residence within the chloroplast based on the tomato literature or Arabidopsis orthologs identified in the Plant Proteome Database (PPDB; http://ppdb.tc.cornell.edu/) (Sun et al., 2009), the Plastid Protein Database (plprot; http://www.plprot.ethz.ch/) (Kleffmann et al., 2006), and Subcellular Localization Database for Arabidopsis (SUBA4; http://suba.live/) (Hooper et al., 2017). The PPDB database was filtered for chloroplast-localized proteins with empirical evidence for localization within the chloroplast. The plprot database describes proteins localized in all plastid forms and was filtered for Arabidopsis homologs. SUBA4 was filtered for proteins with experimentally validated localizations within Arabidopsis plastids.
Proteins that were predicted to be chloroplast localized by more than two or more localization algorithms were also retained (see below). Gene names were based on the tomato literature, Sol Genomics database, updated with recent NCBI annotations, and, when appropriate, Arabidopsis thaliana orthologs, which were identified by the program Eggnog (http://eggnog5.embl.de/#/app/home) (Huerta-Cepas et al., 2019)(Table S2). Data from the primary literature and/or The Arabidopsis Information Resource site (TAIR; https://www.arabidopsis.org/) and Mercator and MapMan BIN ontologies (http://www.plabipd.de/portal/mercator-sequence-annotation/) were used for protein curation (Thimm et al., 2004; Lohse et al., 2014; Berardini et al., 2015). The full set of manually annotated proteins of the tomato stromal proteome is found in Table S2A. During manual annotation, we found that 63 genes/proteins were misannotated in the tomato genome (Table S2B).
The tomato chloroplast protein Atlas
The 34,727 proteins of the deduced proteome of tomato (ITAG 2.4 annotation release) were downloaded from the Sol Genomics Network (http://www.solgenomics.net/) and imported into an R file, which included the amino acid sequences and gene annotations. Subcellular predictions for all deduced proteins were performed using four stand-alone software programs on the UCR Linux Biocluster, which included: TargetP version 1.1b (http://www.cbs.dtu.dk/services/TargetP/), ChloroP version 1.1 (http://www.cbs.dtu.dk/services/ChloroP/), WoLF PSORT version 2.0 (http://www.wolfpsort.org/), and YLoc (http://abi.inf.uni-tuebingen.de/Services/YLoc/webloc.cgi/) (Emanuelsson et al., 1999; Emanuelsson et al., 2000; Horton et al., 2007; Briesemeister et al., 2010). Subcellular predictions using the online version Predotar (http://urgi.versailles.inra.fr/predotar/predotar.html) were also made (Small et al., 2004). Proteins predicted to have a plastid location by one or more organellar prediction algorithms were included in the tomato chloroplast protein Atlas. Of the 87 conserved open-reading frames in the tomato chloroplast genome, six are in the inverted repeat and encode identical proteins; therefore, 81 chloroplast-genome encoded proteins were added to the Atlas (Daniell et al., 2006). The Atlas was maintained in an MS Excel file, with Sol Genomics Network (SGN) loci identifiers. In the absence of functional or experimental evidence from Arabidopsis databases or the literature, the reproducible detection and strong Atlas predictions were the criteria for retention of a protein in the tomato stromal proteome. As transmembrane domain algorithms often provide different predictions, TMpred (Hofmann and Stoffel, 1993), DeepTMHMM (Hallgren et al., 2022), and CCTOP (Dobson et al., 2015) were used to confirm the presence of transmembrane domains of tomato proteins. Lumenal transit peptides were predicted using PredSL (http://aias.biol.uoa.gr/PredSL/) and TargetP-2.0 (https://services.healthtech.dtu.dk/services/TargetP-2.0/) (Petsalaki et al., 2006; Almagro Armenteros et al., 2019). Venn diagrams were drawn using the VennDiagram package in RStudio Version 1.4.1717 open-source software (Chen and Boutros, 2011).
Relative protein abundance
Relative protein abundance was calculated based on emPAI (exponentially modified protein abundance index) (Ishihama et al., 2005) using the acetone-precipitated protein data. PAI is the ratio of the number of detected proteins to the number of observable peptides per protein (Rappsilber et al., 2002) and was obtained for each protein from Thermo Scientific Proteome Discoverer (PD) 2.1 output. emPAI is calculated by PD as 10PAI -1. The relative protein abundance (mol fraction) was calculated by dividing the emPAI of a protein by the sum of emPAIs of all the proteins in the entire dataset. The molar fraction was multiplied by 100 to obtain the mol % of each protein.
Results
Isolation and nanoLC-MS/MS analysis of the tomato chloroplast stromal proteome
A high-yielding chloroplast and stromal protein isolation protocol was used to identify the protein complement of the tomato chloroplast stromal proteome (Bhattacharya et al., 2020). Given the enhanced accuracy and sensitivity of the Orbitrap Fusion MS, we directly analyzed soluble chloroplast extracts that had chloroplast membranes removed by ultracentrifugation. A robust set of 2,325 proteins with a 1% FDR were obtained from the five biological replicates precipitated in 80% acetone and/or the three samples analyzed after 12% PAGE. The different methods of protein isolation were complementary. The acetone-precipitated and PAGE gel samples yielded 287 and 27 unique proteins, respectively (Table S1). Proteins were curated using a tomato chloroplast protein Atlas, databases with empirical evidence for a protein’s plastidial localization (plprot, SUBA4 and PPDB), relatedness to Arabidopsis orthologs, and evidence present in the literature (Table S2).
Rigorous criteria were used to define the tomato stromal proteome. Of the 2,325 proteins detected, 790 were removed from further analysis based on the fact that they were identified once by 1 peptide spectral match (PSM), with a single unique peptide, or sporadically (in less than 40% of the samples analyzed) (Figure 1). However, we retained any protein with a known chloroplast location to gain insights into low-abundance proteins in our stromal preparations. The remaining 1,535 proteins were unambiguously identified with 7,916 unique peptides and 60,830 peptide spectral matches (PSMs) from which 1,278 proteins were designated as the stromal proteome and 257 were classified as co-isolating proteins (CIPs), which were excluded from the stromal proteome (Tables S1, S2). CIPs were reproducibly isolated but their Arabidopsis homologs had empirical evidence for and/or protein localization algorithms strongly predicted residence in other subcellular compartments (Bhattacharya et al., 2020). CIPs may have dual localization within tomato cells; however, if chloroplast localized, CIPs do not use canonical transit peptides (Armbruster et al., 2009; Jarvis and López-Juez, 2013; Nakai, 2018; Thomson et al., 2020). It is also possible that the CIPs reflect the close proximity of and connections between other organelles such as the nucleus, peroxisome, mitochondria, and endomembrane system (Andersson et al., 2007; Islam and Takagi, 2010; Mehrshahi et al., 2013; Higa et al., 2014; Gao et al., 2016; Exposito-Rodriguez et al., 2017; Hooper et al., 2017; Barton et al., 2018; Oikawa et al., 2019; Mullineaux et al., 2020).
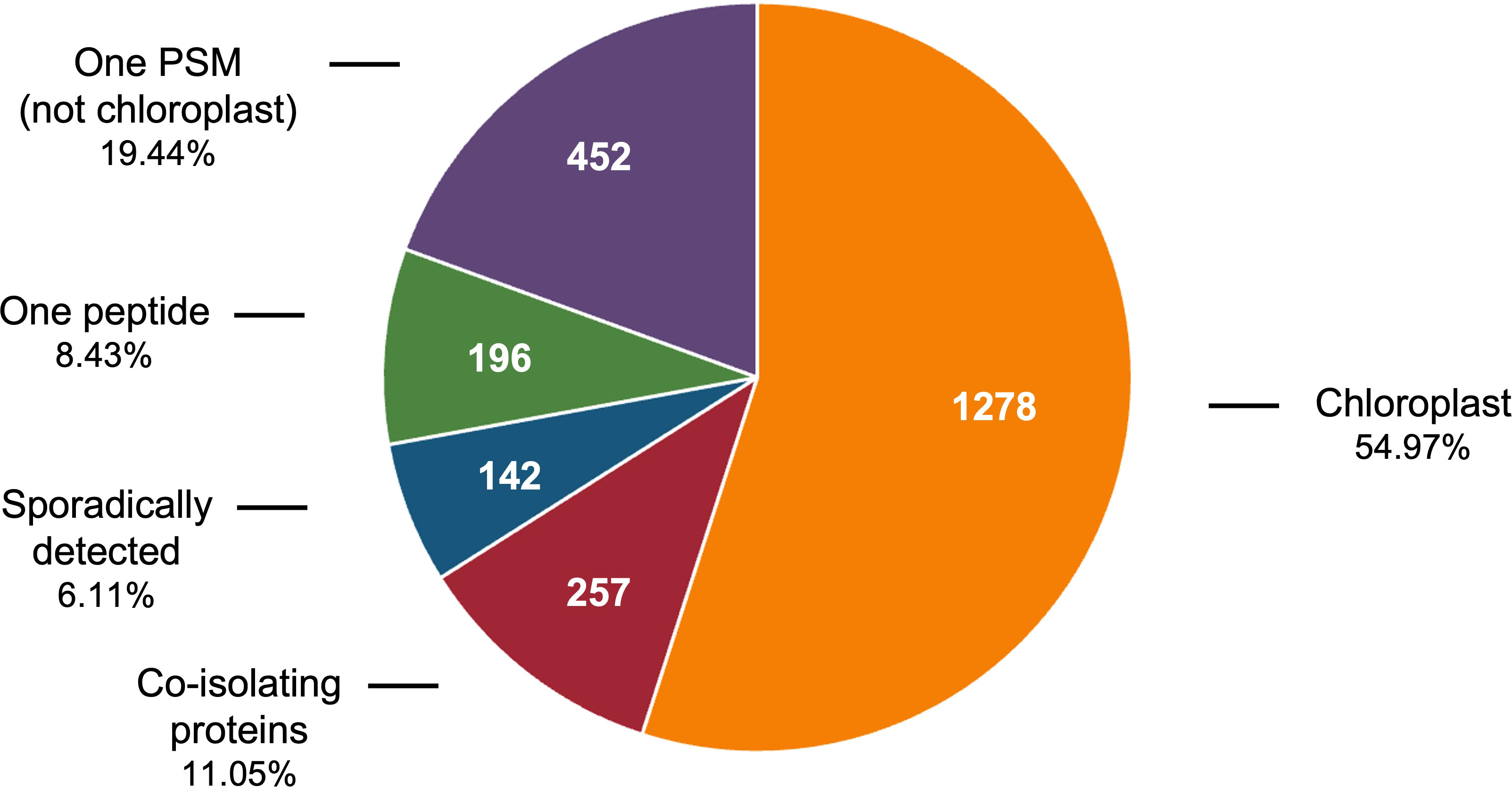
Figure 1 Classification of 1% FDR proteins identified in tomato chloroplast soluble extracts. The 2,325 proteins identified in the soluble extracts of tomato chloroplasts includes 1,278 chloroplast proteins, 257 co-isolating proteins (CIPs) that were reproducibly detected and 790 proteins that were removed from consideration because they were detected with one PSM, with one unique peptide, or sporadically (in less than 40% of the acetone or PAGE samples).
Curation of the tomato stromal proteome: leveraging the tomato chloroplast protein Atlas and Arabidopsis protein localization databases
The use of multiple machine-learning algorithms is best practice for predicting the residence of plant proteins in subcellular compartments such as the chloroplast (Richly and Leister, 2004; Hooper et al., 2017). Here, five subcellular-localization programs (TargetP, ChloroP, Predotar, WoLF PSORT, and YLoc) were used to construct a theoretical tomato chloroplast proteome (the Atlas) (Emanuelsson et al., 1999; Emanuelsson et al., 2007; Horton et al., 2007; Briesemeister et al., 2010; Hooper et al., 2017) (Table S3A). The Atlas included 81 chloroplast genome-encoded proteins (Daniell et al., 2006; Kahlau et al., 2006) and 7,473 nuclear genome-encoded proteins predicted to be localized in the plastid by one or more programs (Figure 2A, Table S3A). The Atlas constitutes ~ 22% of the tomato genome making it a liberal predictor of chloroplast localization. This approach was reasonable since each algorithm brought different computational approaches to predict protein locations and was trained on different sets of proteins.
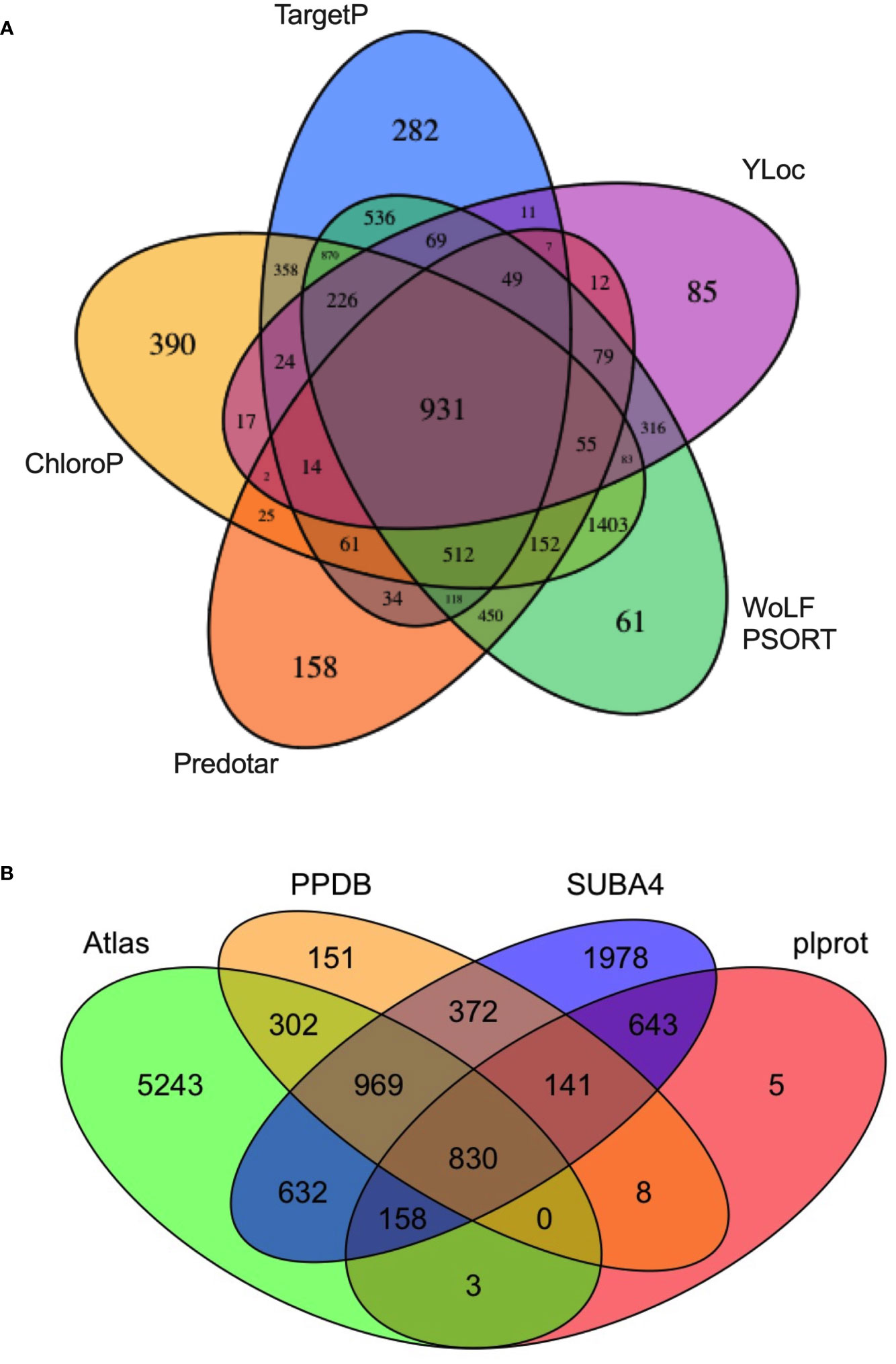
Figure 2 The tomato protein Atlas. (A) Source of proteins assigned to the tomato Atlas. A total of 7,473 nuclear-genome encoded proteins were predicted to be chloroplast localized by one or more of five subcellular localization programs: WoLF PSORT, Predotar, ChloroP, TargetP, and YLoc. The 81 plastid-genome encoded proteins, which are part of the Atlas, are not displayed. (B) A four-way Venn diagram compares the overlap of the tomato protein Atlas with Arabidopsis thaliana orthologs present in plprot, SUBA4, and PPDB. Proteins in the tomato chloroplast Atlas are found in Table S3A. Arabidopsis orthologs of predicted tomato chloroplast proteins were identified by Eggnog v5.0 and are found in Table S3B.
At the core of the Atlas are 931 proteins that were predicted to be chloroplast localized by all five programs (Figure 2A; Table S3A). No single algorithm identified all 1,278 proteins of the tomato stromal proteome and each algorithm identified a set of unique proteins ranging from 61 (WoLF PSORT) to 390 (ChloroP), stressing the contributions of each program to the Atlas (Table S2). Finally, based on the PPDB, plprot, and SUBA4 databases, only 2,903 of the proteins in the tomato Atlas (38.8%) had an Arabidopsis ortholog with empirical evidence for residence in the chloroplast (Figure 2B, Table S3B).
Of the 1,278 proteins in the tomato stromal proteome, 89% were predicted by the Atlas and 84%, 88% and 43% of these proteins had one or more Arabidopsis homologs in PPDB, SUBA4 and plprot databases, respectively (Tables S2, S3C). A core of 469 proteins (36.7%) was detected in all three databases (Table S2; Figure 3A). These proteins were enriched for proteins involved in protein folding and targeting, tetrapyrrole synthesis, redox, and TCA metabolism; while proteins associated with DNA synthesis, amino acid metabolism, photosynthesis, and glycolysis were under represented.
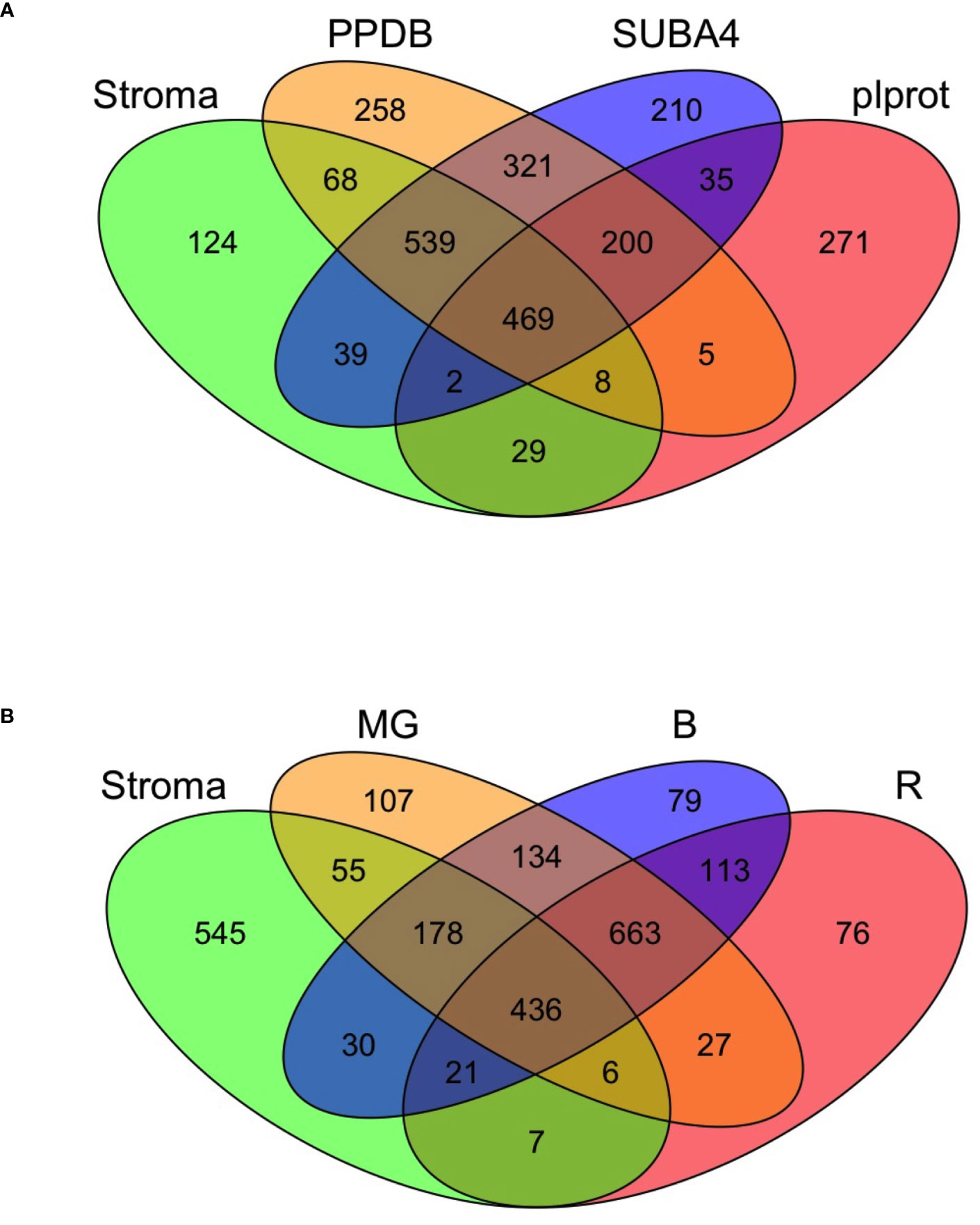
Figure 3 The tomato stromal proteome comparisons to Arabidopsis chloroplast proteins and tomato fruit proteins. (A) Comparison of the tomato stromal proteome with Arabidopsis thaliana chloroplast proteins present in the plprot, SUBA4 and PPDB databases. A core of 469 proteins with one or more Arabidopsis orthologs was detected in all three databases. Sixty proteins in the tomato stromal proteome had multiple Arabidopsis orthologs in all three databases, which brings the number of unique tomato proteins shared with the databases to 518 (Tables S2, S3B). (B) A four-way Venn diagram illustrates the overlap of the leaf stromal proteome with three tomato plastid proteomes from fruit in the mature green (MG), breaker and 10-d post breaker (B), and red (R) stages of fruit development.
Sub-organellar localization of proteins and molar abundance
Immunoblots indicated that the tomato stromal proteome may harbor thylakoid lumenal proteins and should be depleted of thylakoid integral membrane proteins (Bhattacharya et al., 2020). TMpred, DeepTMHMM and CCTOP predicted 159 proteins with one or more transmembrane domains (Hofmann and Stoffel, 1993)(Table S4A). While 17 of these proteins had an unknown location within the chloroplast, proteins associated with the thylakoid membrane (95), the envelope (30), both chloroplast membrane systems (3), and plastoglobules (3) were detected. Based on these numbers, membrane proteins constituted 12.4% of the stromal proteome. However, it should be noted that 46 of the membrane proteins were sporadically detected (<40% of acetone or gel samples), making estimated percentage of membrane proteins 8.8% (Table S4A).
Fifty-nine proteins that reside within the tomato chloroplast lumen were identified and represented 4.6% of the stromal proteome (Table 1). The lumenal proteins had a diverse array of functions including 12 immunophilins (cyclophilins and FKPBs), three C-terminal processing proteases, three DEG protease subunits, 11 lumenal proteins associated with the PSI, PSII, CytB6/f, and NAD(P)H complexes, as well as 22 proteins involved photosystem maintenance or assembly (Table 1). We detected nine of the ten tomato FKBP proteins predicted to be within the chloroplast (Waseem et al., 2018); only FKBP12, which was predicted to be localized to both the cytosol and chloroplast, was not detected. We also detected eight lumenal proteins with orthologs in Arabidopsis that were not detected in earlier studies (Table S4B), as well as tomato’s PPO-F and PPO-A (Newman et al., 1993).
The total number of chloroplast membrane and lumenal proteins overestimated their contribution to the stromal proteome (17.1%). A better assessment was provided by the exponentially modified protein abundance index (emPAI) (Table S2). We used the emPAI to normalize the abundance of stromal proteins in acetone-precipitated samples. emPAI is based on the number of detected peptides versus the number of observable peptides per protein to provide an estimate of a protein’s molar abundance (Ishihama et al., 2005). The mol % of tomato’s stromal proteins varied over a 5.7 x 104-fold range, with the majority of proteins in the 10-3 to 10-2 mol % categories (Figure 4; Table S2). Membrane proteins represented a 1.9 mol % of the stromal proteome (Table S4A), while the 59 lumenal proteins accounted for 5.8 mol % (Table 1). The most abundant lumenal protein was TL19, constituting 33% of the lumen protein mass. Collectively, tomato chloroplast membrane and lumenal proteins constituted 7.7% of the mass of proteins in the stromal proteome, representing a minor proportion of the tomato stromal proteome. These data strongly support previous immunoblot data indicating low levels of proteins from other compartments of the chloroplast (Bhattacharya et al., 2020).
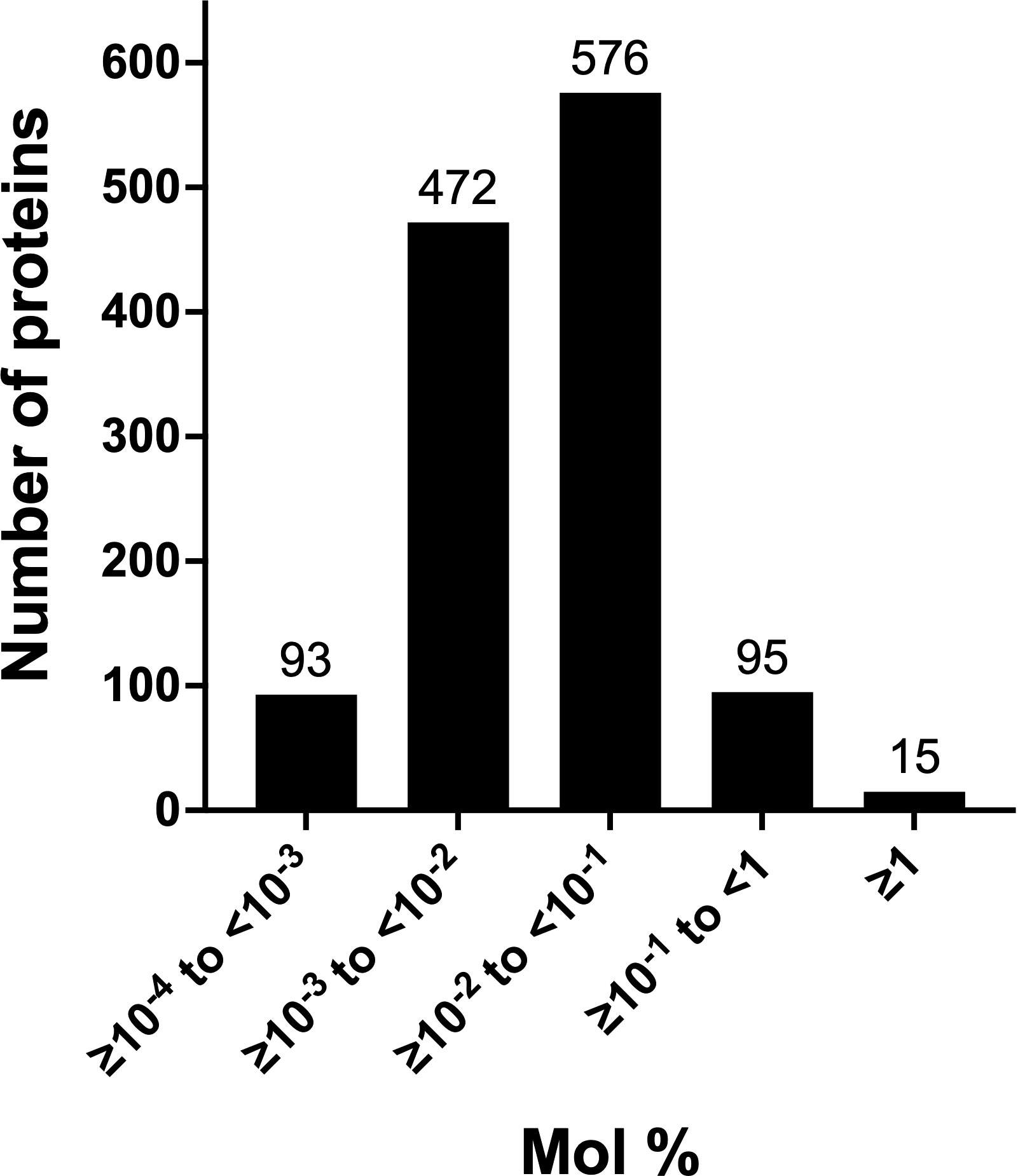
Figure 4 Abundance classes of leaf stromal proteins. The abundance of the 1,251 acetone-precipitated proteins of the leaf stromal proteome was determined by calculating the emPAI and mol % of the proteome. Five protein classes were defined by their relative abundance. The numbers of proteins in each emPAI class are provided above the bar.
Relative abundance of proteins and novel proteins in the tomato chloroplast stromal proteome
A small number of studies have provided insights into eudicot stromal proteomes. To elucidate chloroplast complexes and soluble proteomes in Arabidopsis, these studies used SDS-PAGE (Ferro et al., 2003; Peltier et al., 2006), size exclusion chromatography (Peltier et al., 2006), affinity chromatography (Bayer et al., 2011), or blue native-PAGE (Lundquist et al., 2017) to prefractionate proteins prior to MS analyses. To benchmark the tomato stromal proteome relative to the Arabidopsis stromal proteome, we compared the relative abundance of the tomato stromal proteins to the relative normalized abundance of the 241 Arabidopsis stromal proteins identified by Peltier et al. (2006).
The top two classes of tomato stromal proteins had mol % values ranging from > 0.1 to 13.9 (Figure 4; Table 2). The rankings of these 110 proteins were compared to their Arabidopsis orthologs (Peltier et al., 2006). Although of varying abundance and rankings, 19 of the 23 most abundant proteins in Arabidopsis were detected in tomato’s top two abundance classes (Table S4C); the other abundant Arabidopsis proteins were detected but at lower levels (Table S4C). For the most abundant tomato stromal proteins, there were 26 proteins with two or more orthologs in tomato relative to a single protein in Arabidopsis (Table 2). For eleven, both orthologous proteins of tomato were in the top two abundance classes including: RuBisCo activase2 (RCA2A, RCA2B), chaperone DnaK (HSC70-2a, HSC70-2b), Clp protease subunit C (CLPC1, CLPC2), elongation factor Tu (EFTuA, EFTuB), fructose-bisphosphate aldolase (FBA1, FBA2), glycolate oxidase1 (GLO1A, GLO1B), glyceraldehyde-3-phosphate hydrogenase (GAPB1, GAPB2), ketol-acid reductoisomerase (At3g58610-like1 and 2), 29-kDa ribonucleoprotein (CP29A, CP29B), superoxidase dismutase (Fe-SOD2A, Fe-SOD2B), and triosephosphate isomerase (pdTPI1, pdTPI2). For the remaining sixteen, the two orthologous proteins accumulated to different levels suggesting different mechanisms of regulation. Forty-seven proteins in the top-two protein cohorts were not detected by Peltier et al. (2006) (Table 2).
Reciprocally, of the 23 most abundant Arabidopsis stromal proteins, all but one (a ROC4-like protein with no tomato ortholog) were detected in the tomato stromal proteome but their relative rankings (by mol %) were significantly different (Table S4C) (Peltier et al., 2006). While the RuBisCo large subunit (RBCL) was one of the most abundant proteins in both studies, there was a striking difference in the abundance of the RuBisCo small subunits. Peltier et al. (2006) reported the abundance of an RBCS protein pool, which ranked 2 in abundance. In contrast, the analogous tomato RBCS pool had a combined mol % of 0.228, which ranked the pool as 51 in the tomato stromal proteome (Table S4C). Furthermore, some tomato proteins, such as 2-CYS-Prx1, 2-CYS-Prx2, CPN20, and LOX2, were not even in the top 110 most-abundant proteins of the tomato stromal proteome. Collectively, these data indicate the mechanisms that dictate stromal protein abundance are significantly different in these plant species.
Comparisons of tomato stromal proteome with Arabidopsis chloroplast proteins catalogued in PPDB, SUBA4 or plprot showed that 130 stromal proteins were not previously detected in plastids (Table 3). A majority (72%) of the novel proteins were reproducibly detected (in >40% of acetone and/or gel samples) and 82.4% of the novel proteins were predicted to reside within the chloroplasts by two or more algorithms (Table 3, Table S2A). The abundance of the novel stromal proteins ranged from 1.95 mol % to < 3.8 x 10-4 mol % and totaled 4.96 mol % of the stromal proteome. Strikingly, six defense-associated proteins (LAP-A1, LAP-A2, PPOE, PPOF, AIG2-like, and KIROLA) were abundant and, collectively, accounted for 81% of the mass of the novel proteins based on mol %. Most novel stromal proteins were not abundant and were likely identified due to the enhanced sensitivity, accuracy and resolution of the Orbitrap Fusion MS.
Forty-two of the novel proteins had roles in RNA biogenesis, protein biogenesis, redox, or stress responses, ten were transcription factors or DNA-binding proteins, and 32 proteins had roles in cellular metabolism spanning amino acid to secondary metabolism (Table 3). Unknown proteins and proteins with uncharacterized functions dominated, representing 28% of the novel proteins. Finally, 14 proteins did not have orthologs in Arabidopsis including: two leucine aminopeptidases (LAP-A1, LAP-A2) (Gu et al., 1996a), three tomato polyphenol oxidases (PPO-F, PPO-E, and PPO-A) (Newman et al., 1993; Tran et al., 2012), YCF23, and a methyltransferase.
Functional comparisons of the tomato leaf stromal and fruit plastid proteomes
While the proteomes of tomato fruit are well-characterized (Sant’Ana and Lefsrud, 2018), few studies have focused on the plastids of tomato fruit or leaves (Barsan et al., 2010; Barsan et al., 2012; Tamburino et al., 2017). Barsan et al. (2010; 2012) identified 1,932 proteins in plastids undergoing the chloroplast to chromoplast transition associated with fruit ripening (Table S3D). A core of 436 proteins were shared with our leaf stromal proteome and the proteomes of mature-green, breaker and red fruit plastids with reflecting shared housekeeping and biochemical functions. In addition, 545 proteins unique to the leaf stromal proteome were identified (Figure 3B; Table S3). Of the 81 chloroplast-genome encoded proteins, 44 were detected in the leaf stromal proteome (Table S5A). Collectively the leaf stromal and fruit plastid proteomes provided empirical evidence for 55 of the chloroplast-genome encoded proteins (Tables S3D).
To infer function, stromal proteins were assigned MapMan function bins using Mercator (Lohse et al., 2014). Four of the five largest bins (>59 proteins) were associated with well-known chloroplast functions - photosystems, protein synthesis, amino acid metabolism, and RNA (Figure 5 (top panel); Table S5). There was a surprising lack of correlation of numbers of proteins and the relative protein mass (based on mol %) for the top five bins (Figure 5) (bottom panel). For example, approximately 37.6% of the stromal protein mass was associated with the 94 proteins in the photosystems bin. In contrast, the 77 proteins in the RNA and the 94 proteins in the amino acid metabolism bins were 8.3% and 2.75% of the proteome, respectively. Manual curation of the proteins in the not-assigned bin (311 proteins) allowed specific or general functions to be assigned most proteins, leaving only 39 proteins as uncharacterized/unknown and 52 enzymes with unknown functions (Tables S4D–F). This curation grouped the stromal proteins into ten functional categories (Table 4; Tables S5–S10). Below we highlight several of these functional groups.
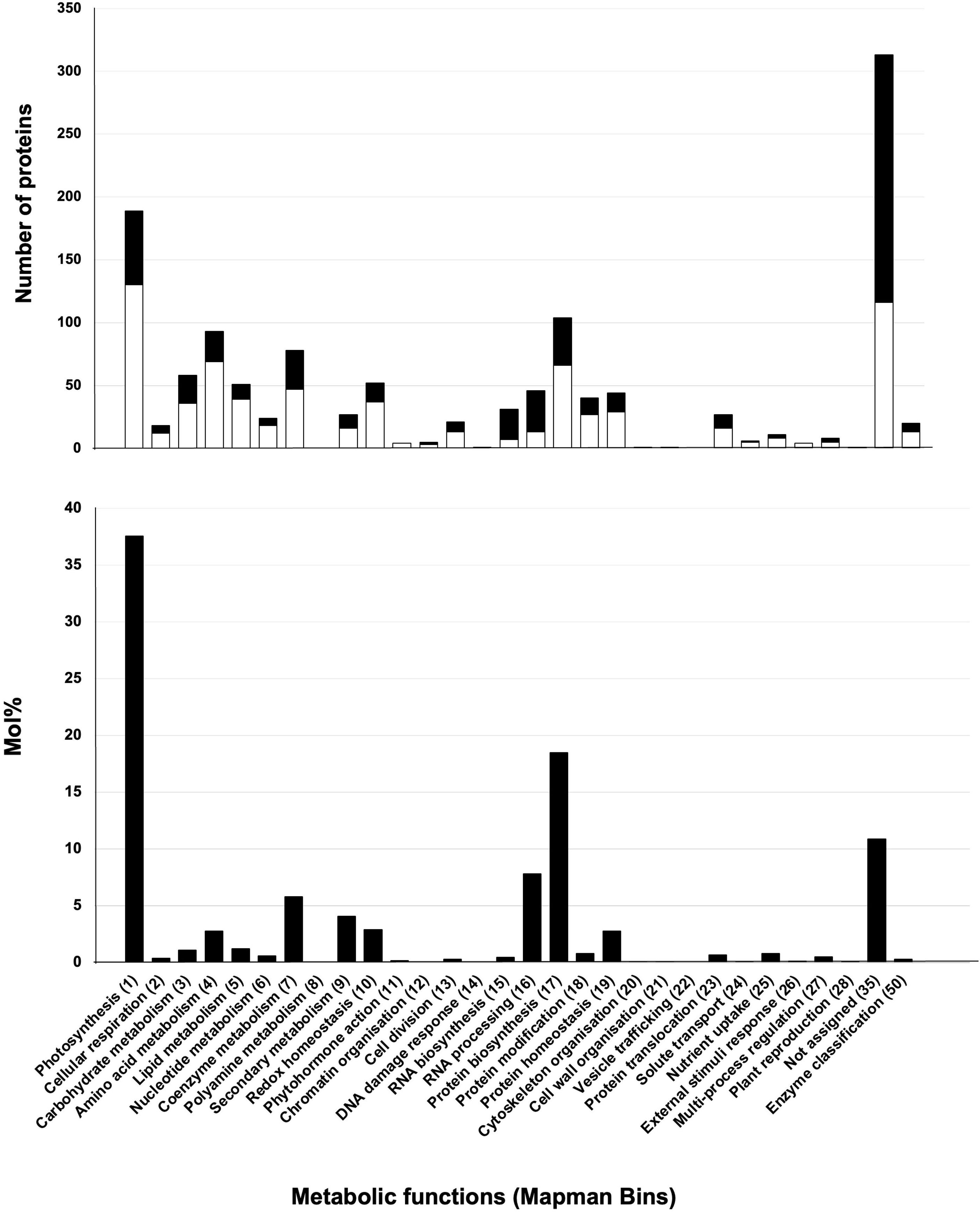
Figure 5 Functions and abundance of proteins detected in the tomato stromal proteome. The 1,278 proteins of the tomato stromal proteome were categorized into MapMan functional categories. MapMan bin numbers are within parentheses. The 733 proteins shared with the fruit plastid proteomes (white) and 545 proteins detected only in the leaf stromal proteome (black) are displayed. Proteins were detected in all but two MapMan bins. There were no proteins assigned to polyamine metabolism (Bin 8) or the vesicular trafficking (Bin 22) bins. The abundance of the proteins in each MapMan bin is displayed as mol % of the stromal proteome.
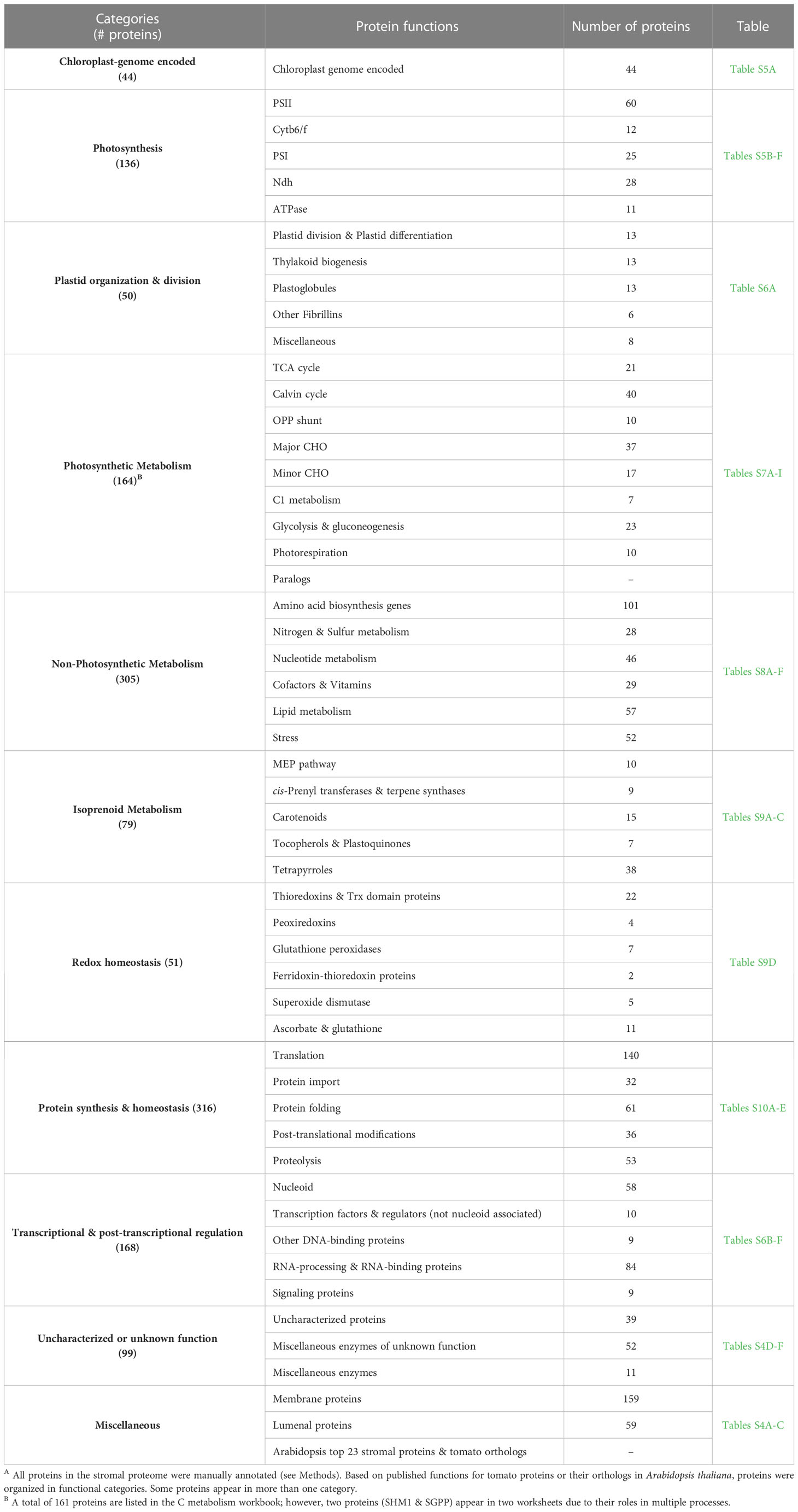
Table 4 Functional categories of proteins after manual annotation of the tomato leaf stromal proteomeA.
Photosynthetic complexes
Over 19.8% of the stromal proteome mass was associated with the major multimeric photosynthetic complexes - photosystem I-Light Harvesting Complex I (PSI-LHCI), PSII-LHCII, cytochrome b6f, ATP synthase, and NADH dehydrogenase (NDH) complexes – and the proteins involved in complex stability and assembly (Table S5). Photosynthesis initiates with the absorbance of light energy by light-harvesting complex proteins (LHCII) and photosystem II (PSII) (Buchanan et al., 2015). The vast majority of PSII-associated proteins are integral-membrane proteins and were not detected (Table S5B). The chloroplast genome-encoded PSBA-E were detected infrequently, at low levels and with non-molar ratios. The nuclear-genome encoded PSII subunits (PSBR and PSBS) and five LHCII subunits (LHCB13, 1A, 1B, 3C, and CP29.1) were detected at low levels (≤0.007 and ≤0.006 mol%, respectively) and sporadically identified. Whereas PSB33 and LHCB9 were 2.6- and 3.2-fold more abundant, respectively, and detected in all samples analyzed, suggesting a looser association with the thylakoid membranes. The most abundant proteins were the lumenal oxygen-evolving proteins (PSBO-1, PSBO-2, PSBP, and PSBQ). Dozens of proteins important for PSII protein and pigment assembly, stability or repair are known in Arabidopsis (Lu, 2016; Liu and Last, 2017; Sato et al., 2017; de Luna-Valdez et al., 2019; Li et al., 2019). We detected 29 of these orthologous proteins, as well as thio/disulfide-modulating proteins critical for PSII assembly/maintenance and protein processing/turnover (Table S5B; Table 4).
Linking PSI and PSII, the cytochrome b6/f complex has eight subunits (Malone et al., 2019) and two integral membrane proteins (PETA and PETB) and the lumenal PETC were detected. All proteins associated with photosynthetic electron transport (PETE, PETJ, two PETFs, and two PETHs) were detected (Table S5C). PETH1 is the most abundant of these proteins (0.27 mol%) (Table 2) and is 6-fold more abundant than its paralog. Two complex assembly/stability factors were detected - HCF164 and LIR1 (Yang et al., 2016; Lennartz et al., 2001) (Table S5C).
PSI and its light-harvesting complex is an asymmetric assemblage of 15 PSI proteins, LHCA proteins, and PSI assembly proteins (Table S5D) (Amunts et al., 2010). We detected 11 PSI subunits including six integral membrane proteins (PSA-A, B, F, G, K, and L) and extrinsic proteins exposed on the stromal (PSA-C, D, E) and lumenal (PSA-N) side. Of the PSI-associated LHC proteins, proteins similar to AtLHCA1 and AtLHCA2 were not detected, but two AtLHCA3-like (LHCA8A, LHCA8B) and one AtLHCA4-like (LHCA11) proteins were detected. Finally, we detected five PSI assembly proteins including: YCF3, YCF3-interacting factor, PPD1, PSA2, and PSA3 (Naver et al., 2001; Liu et al., 2012; Fristedt et al., 2014; Shen et al., 2017; Nellaepalli et al., 2018). Notably, the chloroplast genome-encoded YCF4 was not detected, although it was detected in the tomato fruit proteome (Barsan et al., 2012) (Table S3).
The NAD(P)H-dehydrogenase-like complex (NDH) associates with two PSI complexes and is active in photorespiration (Shikanai, 2016), as well cyclic electron flow to preferentially contribute to ATP synthesis (Munekage et al., 2002; Yamamoto and Shikanai, 2019). Proteins associated with electron flow (PGR5, PGRL1A and PIFI) were detected, as were many subunits of the NDH complex and several NDH assembly proteins (Table S5E) (Wang and Portis, 2007; Shikanai, 2016). NDH is the largest complex with 29 proteins organized into subcomplexes (Shikanai, 2016). Six NDH subunits of the stroma-facing of subcomplex A (NDH-H, I, J, M, N, O) and five proteins critical for assembly (CRR1, 6, 7, 9, and 41) were detected (Table S5E). In addition, subcomplex E (NDH-S, U, and V) and all subunits of stroma-exposed subcomplex B (PNSB1-PNSB5) and lumenal subcomplex L (PNSL1-PNSL5) were detected; whereas, none of the proteins in the thylakoid membrane-associated subcomplexes SubL nor SubM were detected. The subunits for the NDH subcomplexes A, B and L were not detected in equimolar ratios. Finally, the minor LHCA proteins (similar to AtLHCA5 and AtLHCA6) that mediate the PSI-NDH super-complex formation were not detected (Peng et al., 2009).
The ATP synthase complex is composed of eight different subunits (Hahn et al., 2018). All subunits of the extrinsic CF1 complex, which are peripheral thylakoid membrane proteins, were detected (Table S5F). While ATPA, ATPB, ATPD, ATPE, and ATPC are present in a 3:3:1:1:1 ratio in CF1, their abundance in the tomato stroma did not reflect this stoichiometry. ATPB was 27-, 32-, 22-, and 55-fold more abundant than ATPA, ATPD, ATPE, and ATPC, respectively. While the integral membrane subunits ATPH and ATPI were not detected, the ATPF and ATPG subunits, which are tethered to ATPH, were detected at substantially lower levels than the CF1 complex proteins (Table S5F). Finally, four ATP synthase biogenesis proteins were detected (ALB4, BFA1, BFA2, and PAB) (Mao et al., 2015; Trösch et al., 2015; Zhang et al., 2016; Zhang et al., 2018). In contrast, the assembly proteins P11 (Solyc02g093690) and P12 (Solyc02g031770) were not detected (Duan et al., 2020).
Of critical importance to the function of the photosynthetic complexes is the biogenesis and maintenance of the thylakoid membranes. In addition, proteins associated with plastid fission, chloroplast differentiation, and plastoglobules are important for chloroplast structure and function (Table S6). Of the 53 proteins in this group, 19 were fibrillins (Laizet et al., 2004). Ten different types of fibrillins were detected in 87-100% of the samples and ranging from 0.06 mol % (FBN-like) to 0.07 mol % (FBN4).
Photosynthetic metabolism in chloroplasts
The chloroplast is a metabolic hub synthesizing a broad spectrum of molecules essential for plant growth, development and adaptation to stress (Rolland et al., 2012; Buchanan et al., 2015). A significant proportion of the tomato stromal proteome was associated with the central (or primary) metabolic pathways of photosynthetic metabolism (Wise and Hooper, 2006). These pathways include the Calvin cycle, TCA cycle, OPP pathway, major and minor carbohydrate metabolism, C1 metabolism, glycolysis, gluconeogenesis, and photorespiration (Table 4, Tables S7A–H). A total of 165 proteins associated with carbon metabolism were detected and, collectively, they constituted 19.3 mol % of the stromal proteome. Notably, 27 of these proteins were encoded by single-copy genes in Arabidopsis and by two paralogs in tomato (Table S7I). The majority of the paralogous proteins accumulated to different levels in the tomato stroma ranging from 1.1- to 161-fold different. For example, the RuBisCo large subunit methyl transferase LMST2 was 48-fold more abundant than LMST1 (Table S7I). These data suggest that the duplicated genes have allowed for changes in paralog abundance and, potentially, in function.
Non-photosynthetic metabolism in plastids: amino acids, nitrogen, sulfur, nucleotides, co-factors, and vitamins
Numerous non-photosynthetic central metabolic pathways are active within chloroplasts including N and S metabolism and biosynthesis of nucleotides, co-factors and vitamins, amino acids, lipids, and defense-associated oxylipins (Table S8A). We also detected 11 enzymes with roles in other metabolic pathways and identified 52 enzymes that could not be reliably assigned to a pathway (Tables S4E, F).
The largest group of proteins associated with non-photosynthetic central metabolism were the 101 enzymes that catalyze amino acid biosynthesis (Lancien et al., 2007) (Table S8A). Four enzymes associated with aromatic amino acid (TSA, SK, ASB2) or histidine (HIS-N5B) biosynthesis were identified in the stroma for the first time (Table 3). In addition, three ACT-domain proteins with unknown function were identified; ACT domains bind amino acids and are often used in amino acid feedback-regulated enzymes.
The chloroplast contributes to N and S metabolism (Table S8B) (Masclaux-Daubresse et al., 2010; Chan et al., 2013). Seven enzymes in N metabolism were detected with glutamine synthase 2 (GS2) being most abundant (0.4 mol %). We detected 21 proteins associated with S metabolism, which centers on Cys biosynthesis and catabolism. Cys is essential for protein biosynthesis and is a critical residue in enzyme active sites, protein tertiary structure, protein-protein interactions, redox sensitive enzyme activity, [Fe-S] groups, vitamins, and cofactors (Table S8B). Proteins involved in sulfate catabolism (APS1, APR3, SiR), Cys biosynthesis (OASC, CS26), as well as Cys-derived methionine (GS, CBL), cystathione (CBX1A-C), and glutathione (GSH1, GSH2) biosynthesis were detected. Finally, SAL1, a critical redox-responsive regulator of the retrograde stress signal PAP, was detected (Chan et al., 2013); while the integral-membrane antiporters of PAPS/PAP (PAPST1 and PAPST2) that help to control the levels of cytosolic PAP were not detected (Ashykhmina et al., 2019).
Forty-six enzymes associated with nucleotide metabolism were detected (Table S8C). Of these, six were detected for the first time, including an Appr-1-p processing domain protein (Kumaran, 2005), a nucleoside diphosphate kinase (NDK3) and a ribose-phosphate pyrophosphokinase 3 (PRS3) (Table 3). Surprisingly, we reproducibly detected two enzymes of pyrimidine biosynthesis, dihydroorotate dehydrogenase (DHODH) and orotidine 5’-phosphate decarboxylase (ODCase), which catalyze tandem steps in pyrimidine biosynthesis in the stroma. The tomato DHODH had no predicted targeting signals and was previously detected in plant mitochondria (Bellin et al., 2021). In contrast, the tomato ODCase had strong predictors for plastid localization (Table S8C); although previous studies suggest it resides in the cytosol. The stromal localization of both proteins may provide new insights into pyrimidine metabolism in tomato.
Non-photosynthetic metabolism: lipids and oxylipins
The central metabolic pathways for lipids and phytohormone biosynthesis are highly conserved (Li-Beisson et al., 2013; Wasternack and Song, 2017). Fifty-seven enzymes associated with lipid metabolism (1.24 mol %) were identified (Table S8E). Enzymes for the synthesis of acetyl-CoA (ACS and the pyruvate dehydrogenase complex), all soluble enzymes for lipid elongation, many lipases, and lipid-binding proteins were detected. The inner membrane-associated enzymes and enzymes associated with lipid desaturation were not detected. An acyl carrier protein (ACP5) and the oleoyl-acyl carrier protein thioesterase 2 (FATA) were not previously reported in the Arabidopsis proteomics databases (Table 3; Table S8E). The enzymes essential for the synthesis of jasmonic acid (JA), which is critical for plant defense and development, and numerous oxylipins with roles in defense signaling including the HPL branch that produces C6 volatiles were detected (Table S8E) including two lipoxygenases (LOXC and LOXF), allene oxide synthase (AOS), allene oxide cyclase (AOC), and hydroperoxide lyase (HPL).
Isoprenoid metabolism, retrograde signals, and other metabolic pathways
Isoprenoids are the largest and most diverse group of natural products in plants, with over 35,000 different compounds (Kirby and Keasling, 2009). The plastid-derived isoprenoid metabolites (heme, chlorophylls, carotenoids, ABA, gibberellins, strigolactones, plastoquinones, phylloquinones, tocopherols, and terpenoid volatiles) are derived from the five-carbon isopentenyl diphosphate (IPP) and DMAPP, which are primarily synthesized by the MEP pathway (Zhou and Pichersky, 2020). Seventy-nine proteins associated with isoprenoid production were detected in the stromal proteome (Tables S9A-C). All enzymes of the plastidial MEP pathway, as well as two IPP isomerases, were detected. DXS, which creates the substrates for the MEP pathway and thiamine biosynthesis, is encoded by two tomato paralogs. DXS1 was 17-fold more abundant than DXS2 in leaf chloroplasts (Table S9A), which consistent with DXS1 and DXS2 RNA levels in leaves and fruit (Paetzold et al., 2010). Additional enzymes detected included three cis-prenyl transferases, two geranylgeranyl pyrophosphate synthases (GGPPS), a GGPPS small subunit (SSU-II), and three terpene synthases (Table S9A). While Barja et al. (2021) and Zhou and Pichersky (2020) reported three plastidial GGPP synthases (SIG1-3) with similar kinetic parameters, only SlG2 and SlG3 were detected in our leaf stromal proteome. The absence of SIG1 protein (Solyc11g011240) was consistent with low levels of SIG1 mRNAs, relative to SIG2 and SIG3 (Barja et al., 2021). It is also noteworthy that SSU-I (Solyc07g064660), which is known to modify SIG1-3 activity was not detected (Zhou and Pichersky, 2020).
GGPP is used for the synthesis of carotenoids, which are important for stabilization of the photosynthetic apparatus, light capture, and photoprotection (Stanley and Yuan, 2019). The carotenoid-derived apocarotenoids are important for synthesis of abscisic acid and strigolactone, as well as producing a suite of volatiles important in development and stress signaling (e.g., β-cyclocitral). Fifteen enzymes associated with carotenoid metabolism were detected; although the rating-limiting leaf phytoene synthase 1 (PSY1), orange chaperones, and carotenoid-cleavage enzymes were not detected (Table S9B).
GGPP is also used to synthesize tocopherols, chlorophylls, plastoquinones, and phylloquinones (Table S9B). Tocopherols scavenge singlet oxygen (1O2) derived from photosynthesis. The biosynthetic enzymes (VTE1, VTE3, and VTE4) and regulatory kinases (ABCK1 and ABCK3) were detected (Tables S9B). In addition, the plastoquinione biosynthesis enzyme, solanesyl diphosphate synthase, was detected. The tetrapyrrole pathway yields hemes, the chlorophylls for the PSI and PSII light-harvesting antennae, and protochlorophyllide (PChlide), which is a critical photosensor role in chloroplast-nuclear communication. We detected 38 enzymes associated with tetrapyrrole biosynthesis and catabolism (Table S9C). The complete tetrapyrrole pathway was represented with the exception of the membrane-bound chlorophyllide A oxygenase and uroporphyrinogen III methylase. Tomato also has expanded its tetrapyrrole protein complement with two UROD and three POR paralogs (Table S9C) (Gabruk and Mysliwa-Kurdziel, 2020).
PChlide is a photosensitizer that is critical in retrograde signaling (de Souza et al., 2017). By transferring its excitation energy to oxygen, PChlide creates the highly reactive 1O2. To limit 1O2 production and photosensitivity, AtFLU controls PChlide levels (op den Camp et al., 2003). We detected two FLU proteins (FLU1 and FLU2) that are 64% identical and FLU1 is 4-fold more abundant than FLU2. Neither have been studied to date and it is unclear if they are functionally redundant (Table S9C). In Arabidopsis, the EXECUTER proteins (AtEX1 and AtEX2) have critical but distinct roles in perception of 1O2 and triggering the reprogramming of nuclear gene expression for stress adaptation (Lee et al., 2007; Dogra et al., 2017; Duan et al., 2019). In tomato, EX2 is 8-fold more abundant than EX1 (Table S9C), which may reflect differences in the roles of the tomato EX proteins, the tightness of association or location within the grana margins of the thylakoid. Finally, SAFEGUARD1, which suppress 1O2 production at the thylakoid grana margins (Wang L. et al., 2020) is 1.8-fold more abundant than EX2 (Table S9C).
Redox regulation: damage control to cellular homeostasis
Chloroplasts use redox-regulatory systems to limit cellular damage from ROS and adapt plant metabolism to fluctuating light/dark cycles and environmental insults, such as abiotic stress or pathogen/pest attack (Exposito-Rodriguez et al., 2017; Cejudo et al., 2019; Yoshida et al., 2019; Fichman and Mittler, 2020). Redox regulation is dependent on the electron transport chain of the thylakoid’s photosynthetic complexes to produce reducing power, which is transferred from ferredoxin (Fd) to a thioredoxin (Trx) via Fd-Trx reductase (FTR). The diversity of proteins with Trx and Trx-like motifs and down-stream redox proteins provides flexibility and specificity in responses. We identified 51 redox-regulation proteins including: FTRs, thioredoxin domain-proteins, peroxiredoxins, glutathione peroxidases, superoxide dismutases, ascorbate/glutathione cycle proteins, and proteins with a cystathionine β-synthase (CBX) domain (Table S9D). The abundance of the redox proteins varied within a 1020-fold range with Fe-SOD2A (1.01 mol %) as the most abundant protein. The tomato redox systems are distinguished from Arabidopsis by the facts that: (1) the tomato Trx-m4 family is expanded (three paralogs), (2) there are two NTRC proteins (with one detected), (3) there are two Fe-SOD2 paralogs, (4) the 2-CYS-Prxs collectively are the most abundant peroxiredoxin in the tomato stroma, but their abundance is significantly lower than in Arabidopsis (Table S4C), and (5) the CBX1 protein family (with probable roles regulation of redox signaling) is expanded (three paralogs) (Table S9D, Table 3) (Cheng et al., 2014).
Protein homeostasis
Approximately 3,000 plastid-localized proteins are encoded by nuclear genes, translated on cytosolic ribosomes and imported into plastids (Thomson et al., 2020), while the remaining 81 proteins are synthesized on chloroplast ribosomes (Daniell et al., 2006; Kahlau et al., 2006). Within the chloroplast, proteins must be folded, post-translationally modified, transported to their sub-compartment within the chloroplast, associated with their cofactors, assembled into their multimeric complexes, and ultimately be targeted for proteolytic turnover. Protein homeostasis is carefully regulated to ensure metabolic responses are coordinated with light/dark cycles and can adapt to the stresses imposed by PS-generated ROS and the environment. Not surprisingly, we detected over 322 proteins that orchestrate the life and death of proteins (Table S10).
The plastid’s 50S and 30S ribosome complexes are essential for synthesizing chloroplast genome-encoded proteins. Perturbations in translation are perceived and communicated to the nucleus (via GUN1) to coordinate plastid biogenesis and mediate adaptation to stress (Marino et al., 2019; Wu et al., 2019). We detected 33 RPL subunits, 23 RPS subunits, 5 plastid-specific ribosomal proteins (PRSPs), as well as 29 proteins were associated with rRNA, tRNA, or ribosomal protein modifications (Table S10A). The ribosomal protein subunits were not present at equimolar levels. Six subunits were particularly abundant including the chloroplast genome-encoded RPS19, RPS15 and RPL23 and nuclear-genome encoded RPL12A, RPL12B and RPS1A. In addition, 27 amino-acyl tRNA synthases and 20 proteins associated with translational initiation, elongation, termination or regulation were identified. Seven of the tRNA synthases lacked an identifiable transit peptide, while 17 had predicted chloroplast or mitochondrial transit peptides (Table S10A). If similar to Arabidopsis, many of these proteins may have dual localization in the chloroplast and mitochondrion or cytosol (Duchene et al., 2005).
Import of proteins into plastids is a regulated process and disruption of import provides a retrograde signal to mediate stress adaptation (Wu et al., 2019). There are several routes for entry into the chloroplast including the canonical import via the outer and inner membranes (TOC and TIC complexes) and inter-organellar channels (Cline and Dabney-Smith, 2008; Armbruster et al., 2009; Nakai, 2018; Thomson et al., 2020). We identified 32 proteins involved in subcellular targeting (Table S10B). Few of the membrane-associated TOC/TIC translocation machinery proteins were detected, while the associated chaperones were readily detected. The proteases (PREP1, SPP, TOP1) that remove the N-terminal transit peptide from imported proteins (Table S10E) and ten other proteins critical for translocating proteins into the thylakoid membrane or lumen were also identified (Table S10B).
To establish and maintain their secondary, tertiary, and quaternary structures to preserve protein function, the chloroplast has an impressive array proteins to facilitate protein folding with 61 different proteins identified in the tomato stroma (Table S10C). This included: 30 chaperones or chaperonins; three ATP-dependent chaperones of the Clp protease (ClpC1, ClpC2, and ClpD), the ClpB3 disaggregase, 19 peptidyl-prolyl cis-trans isomerases, and seven protein disulfide isomerases. Three of these proteins (DJC65, DJC73 and FKBP17-1) were not previously detected (Table 3).
Proteins are also post-translationally modified by addition/removal of chemical moieties or by proteolytic processing to influence protein function or stability. We detected 36 modification enzymes in six functional categories: kinases, phosphatases, methylases, acetylases, deformylases (PDFs), and peptide methionine sulfoxide reductases (Table S10D) and 13 N-terminal peptidases (Tables S10D, E) (Walling, 2006; Gibbs et al., 2016). Unique to tomato are the wound-induced LAPs (LAP-A1 and LAP-A2) that control the expression of nuclear genes associated with the wound- and stress-responses via their aminopeptidase and/or chaperone activities (Table 3) (Gu and Walling, 2000; Fowler et al., 2009; Scranton et al., 2012; Scranton et al., 2013).
The chloroplast also has a robust complement of oligopeptidases and endoproteases to mediate protein turnover (Kmiec et al., 2014; Nishimura et al., 2017). These proteinases and proteolytic complexes are located within envelope, stroma, lumen, or thylakoid membranes. We detected a total of 53 proteins associated with proteolysis (2.6 mol %) (Table S10E). While these proteins primarily remove damaged or unfolded proteins from the chloroplast, it is also clear that peptidase activity is critical for chloroplast signaling, as evidenced by the requirement of FtsH2 protease-mediated turnover of EX1 for signaling 1O2 damage (Wang et al., 2016), role of LapA in tomato defense gene expression (Fowler et al., 2009), and role of chloroplast peptides in defense signaling (Kmiec et al., 2018).
The stroma-localized Clp complex is well characterized structurally and known to have a critical role in protein homeostasis and proteome remodeling (Nishimura et al., 2017; Rowland et al., 2022). We detected all subunits of the stromal Clp complex (Table S10E), three Clp chaperones, as well as the ClpS, ClpF, ClpT1, and ClpT2 proteins that help deliver or provide substrate specificity to the Clp protease (Nishimura et al., 2017). The tomato has two ClpC paralogs with ClpC1 being 2.7-fold more abundant than ClpC2.
Little is known of the function of tomato’s chloroplast DEG proteases (Table S10E) (Nishimura et al., 2017). We detected two stromal DEG2 paralogs in tomato, and three lumenal DEGs (DEG1, DEG5 and DEG8), but the stromal DEG7 (Solyc02g091410) was not detected. The filamentation temperature-sensitive H (FtsH) proteases are associated with membranes, turnover of proteins damaged by ROS, and thermotolerance. In tomato, the thylakoid FtsH6 has a role in thermotolerance (Sun et al., 2006). Of the nine FtsH proteins, the thylakoid-localized (FtsH2 and FtsH5) and inner envelope-localized (FtsH7 and FtsH11) were detected (Table S10E). If similar to the AtFtsH2, the tomato FtsH2 may be critical for retrograde signaling by controlling the turnover of D1 (a reaction center protein of PSII) and the 1O2 sensor EX1 at the margins of the grana (Wang et al., 2016). Finally, three C-terminal processing peptidases (CTPA1-3) and two subunits of the EGY (ethylene-dependent gravitropism-deficient and yellow-green) protease were detected.
The replication and transcriptional hub of the chloroplast
The proteomes of nucleoids and transcriptionally active chromosomes (pTAC) from plastids are influenced by the differentiation state of plastids and/or environmental factors and have been characterized in Arabidopsis and maize (Huang M. S. et al., 2013; Melonek et al., 2016). We detected 58 nucleoid- and TAC-associated proteins (Table S6B). This included all plastid-encoded RNA polymerase (PEP) subunits, 20 PEP-associated proteins, nine DNA replication and repair proteins, four redox proteins, ten RNA biogenesis enzymes, two kinases, and six other proteins with diverse functions. Surprisingly, we did not detect the seven sigma factors (SigA-F) that interact with PEP. Collectively, the nucleoid/pTAC proteins detected in the tomato stroma constituted 2.32 mol % of the proteome ranging from 1.02 mol % (Fe-SOD2A) to 0.0002 mol % (DNA topoisomerase) (Table S6B).
For the conserved nucleoid core, we detected the MURE-like protein and all but three (pTAC9, pTAC11 and pTAC13) of the 18 pTACs (Melonek et al., 2016)(Table S6B). While tomato genome has pTAC9 (OSB2, Solyc09g007430) and pTAC13 (Solyc09g011830) genes, it does not encode a pTAC11-like protein (WHIRLY3) (Akbudak and Filiz, 2019). We detected pTAC7, pTAC10, pTAC12, and pTAC14, as well as the FNL1 and FNL2 kinases, that are known to interact with one another to regulate the activity of PEP (Gao et al., 2012; Huang C. et al., 2013; Chang et al., 2017). While the function of pTAC17 is unknown, we detected two tomato pTAC17s; the tomato pTAC17A was the most abundant pTAC protein identified (0.07 mol %) and was 106-fold more abundant than pTAC17B.
In addition to the proteins associated with transcriptionally active nucleoids, we detected proteins involved with DNA replication, chromatin assembly, recombination, transcription factors, RNA processing and binding, and signaling (Tables S6C-F). There were 82 proteins important for post-transcriptional control (Table S6E). While there is substantial evidence for transcription factors being dual-localized in Arabidopsis, only ten transcription factors and regulators were detected (Tables S6B, C) (Krause et al., 2012; Krupinska et al., 2020). Three histone proteins (two H3-2 proteins and one H2B.1) were detected; their roles within the chloroplast are unknown (Table S6D).
Discussion
The tomato stromal proteome is an important contribution to the field of plastid proteomics, providing novel insights into the protein complement of a eudicot’s stroma, as few stromal proteomes are currently available (Peltier et al., 2006; Olinares et al., 2010). The unprecedented depth of the tomato stromal proteome with 1,278 rigorously identified proteins was achieved due to the purity of our stromal preparations (Bhattacharya et al., 2020) and accuracy and sensitivity of the Orbitrap Fusion MS. Our data complements the plastid proteomes of tomato fruit and leaves (Barsan et al., 2010; Barsan et al., 2012; Tamburino et al., 2017), as well as Arabidopsis stromal proteomes (Peltier et al., 2006; Olinares et al., 2010). Our endeavors provided empirical evidence for 545 tomato plastid proteins and 92 Arabidopsis stromal proteins that were not previously reported (Sun et al., 2009; Barsan et al., 2012). Furthermore, using emPAI as a measure of protein abundance, we showed that when the most abundant proteins in the tomato vs Arabidopsis stroma were compared, there were significant differences in the abundance of orthologous proteins suggesting that the mechanisms that regulate protein homeostasis may have diverged in these model plants. This diversity has the potential to impact the ability of a plastid to sense and transmit signals to inform organellar networks of deviations from plastidial and cellular homeostasis (de Souza et al., 2017; Fernandez and Burch-Smith, 2019; Unal et al., 2020; Wang Y. et al., 2020).
One of these diverged protein homeostasis mechanisms is likely to involve the Solanaceae-specific, wound-induced and stromal LAP-A (Chao et al., 1999; Narváez-Vásquez et al., 2008; Fowler et al., 2009; Scranton et al., 2012) (Table 3). LAP-A upregulates nuclear-genome encoded genes associated with the late branch of wound signaling; LAP-A acts downstream of JA perception and accumulation (Fowler et al., 2009) and, also, downregulates a set of stress-response genes (Scranton et al., 2013). Given LAP-A’s residence in the stroma and ability to modulate nuclear gene expression, LAP-A appears to generate a signal to enable chloroplast-nucleus communication and, thereby, deploy adaptations to cope with ROS, mechanical damage, herbivory, and pathogen attack. To understand its global impact on tomato defense and chloroplast-to-nucleus signaling, the tomato stromal proteome sets the foundations for the multi-omics approaches that are being pursued to characterize of the MeJA- and LAP-A-dependent proteome, N-terminome, metabolome, and transcriptome.
Well studied in Arabidopsis, less is known about retrograde signaling in crops (de Souza et al., 2017; Marino et al., 2019). The tomato stromal proteome provided empirical evidence for accumulation of proteins associated with the synthesis of plastidial metabolites known as retrograde signals including proteins associated with sulfur (PAP, 3’-phosphoadenosine 5’-phosphate), carotenoid (β-cyclocitral), isoprenoid (MEcPP, 2-C-methyl-D-erythritol 2, 4-cyclodiphosphate), and fatty acid metabolism (Table S9). In addition, a robust complement of proteins associated the generation and dissipation of reactive oxygen species (ROS) or serving as photosensitizers (tetrapyrroles, FLU, EX), as well as protein homeostasis were identified (Tables S8, S9). The manual curation of the proteins of tomato stroma identified additional diversity that may be important for the ability of tomato chloroplasts to act as stress sensors and modulate these operational retrograde signals allowing rapid adaptation to biotic and abiotic stress. Significantly, tomato had expansions of some of these gene families and there were substantial differences in protein abundance between paralogs. Examples, included 27 proteins associated with: photosynthetic metabolism, redox and ROS scavenging (NTRC1/NTRC2 and Trx-domain proteins), tetrapyrrole accumulation (UROD1/2, POR1/2/3, FLU1/FLU2) and perception of 1O2 (EX1/EX2), protein homeostasis (ClpC1/C2, LAP-A1/A2, DEG2A/2B, CPN20) and regulation of transcriptionally active chromosomes (pTAC17A/B) (Tables S7, S9, S10). These discoveries present new avenues for understanding the biochemical and signaling complexities of tomato’s stromal compartment.
Data availability statement
The datasets presented in this study can be found in online repositories. The names of the repository/repositories and accession number(s) can be found below: www.proteomexchange.org, PXD035944.
Author contributions
OB isolated tomato chloroplasts and purified stromal proteins. NH enabled critical analysis of datasets. IO created the tomato chloroplast protein Atlas and assembled the data from Arabidopsis databases, NCBI and Mercator. IO, OB and LW performed data analysis and manual curation. OB, IO and LW wrote the manuscript collaboratively. All authors read and approved the final version of the manuscript.
Funding
The work was supported by National Science Foundation grants IOS0725093 and IOS1450331 to LW. A Guru Gobind Singh Fellowship provided partial support for OB. Several fellowships supported IO: NSF/California Louis Stokes Alliance for Minority Participation Bridge to the Doctorate (CAMP-BD) Fellowship, Ford Foundation Predoctoral Fellowship, American Association of University Women (AAUW) Dissertation Fellowship, and US Department of Education Graduate Assistance in Areas of National Need (GAANN) Award. The Orbitrap Fusion mass spectrometer was purchased with a shared instrumentation grant (S10 OD010669).
Acknowledgments
We thank members of the Walling lab for helpful discussions. We thank Frances Holzer for assistance with chloroplast isolations, Dr. A.L.N Rao (UC Riverside) for use of his rotors and ultracentrifuge, and Dr. T. Girke (UC Riverside) for training IO in RStudio. MudPit nano-LC-MS/MS was contracted to the IIGB Proteomics Core and performed by Songqin Pan.
Conflict of interest
The authors declare that the research was conducted in the absence of any commercial or financial relationships that could be construed as a potential conflict of interest.
Publisher’s note
All claims expressed in this article are solely those of the authors and do not necessarily represent those of their affiliated organizations, or those of the publisher, the editors and the reviewers. Any product that may be evaluated in this article, or claim that may be made by its manufacturer, is not guaranteed or endorsed by the publisher.
Supplementary material
The Supplementary Material for this article can be found online at: https://www.frontiersin.org/articles/10.3389/fpls.2023.1020275/full#supplementary-material
Supplementary Figure 1 | SDS-PAGE gels, gel fragment excision and LC-MS/MS analysis. Stromal proteins were isolated as described by Bhattacharya et al. (2020) and fractionated by 12% SDS PAGE. Gels were stained with Coomassie blue. The MW markers and stromal proteins were run on the same gel and two intervening lanes were excised for this figure. The gel section with 50- to 75-kDa proteins was excised (see the arrows). The <50-kDa sections was separated into two fractions containing proteins that were between < 20-kDa (low mass) and a fraction that had proteins from <50-kD and > 20-kDa (intermediate mass). The low mass proteins were pooled with the > 70-kDa (high mass) proteins. The low/high and intermediate mass pools had nearly equivalent amounts of protein and were processed for LC-MS/MS as described in Methods.
References
Abdallah, F., Salamini, F., Leister, D. (2000). A prediction of the size and evolutionary origin of the proteome of chloroplasts of Arabidopsis. Trends Plant Sci. 5, 141–142. doi: 10.1016/S1360-1385(00)01574-0
Akbudak, M. A., Filiz, E. (2019). Whirly (Why) transcription factors in tomato (Solanum lycopersicum L.): genome-wide identification and transcriptional profiling under drought and salt stresses. Mol. Biol. Rep. 46, 4139–4150. doi: 10.1007/s11033-019-04863-y
Almagro Armenteros, J. J., Salvatore, M., Emanuelsson, O., Winther, O., Von Heijne, G., Elofsson, A., et al. (2019). Detecting sequence signals in targeting peptides using deep learning. Life Sci. Alli. 2, e201900429. doi: 10.26508/lsa.201900429
Amunts, A., Toporik, H., Borovikova, A., Nelson, N. (2010). Structure determination and improved model of plant photosystem I. J. Biol. Chem. 285, 3478–3486. doi: 10.1074/jbc.m109.072645
Andersson, M. X., Goksor, M., Sandelius, A. S. (2007). Optical manipulation reveals strong attracting forces at membrane contact sites between endoplasmic reticulum and chloroplasts. J. Biol. Chem. 282, 1170–1174. doi: 10.1074/jbc.M608124200
Andon, N. L., Hollingworth, S., Koller, A., Greenland, A. J., Yates, J. R., 3rd, Haynes, P. A. (2002). Proteomic characterization of wheat amyloplasts using identification of proteins by tandem mass spectrometry. Proteomics 2, 1156–1168. doi: 10.1002/1615-9861(200209)2:9<1156::aid-prot1156>3.0.co;2-4
Armbruster, U., Hertle, A., Makarenko, E., Zühlke, J., Pribil, M., Dietzmann, A., et al. (2009). Chloroplast proteins without cleavable transit peptides: rare exceptions or a major constituent of the chloroplast proteome? Mol. Plant 2, 1325–1335. doi: 10.1093/mp/ssp082
Ashykhmina, N., Lorenz, M., Frerigmann, H., Koprivova, A., Hofsetz, E., Stührwohldt, N., et al. (2019). PAPST2 plays critical roles in removing the stress signaling molecule 3′-phosphoadenosine 5′-phosphate from the cytosol and its subsequent degradation in plastids and mitochondria. Plant Cell 31, 231–249. doi: 10.1105/tpc.18.00512
Baginsky, S., Siddique, A., Gruissem, W. (2004). Proteome analysis of tobacco bright yellow-2 (BY-2) cell culture plastids as a model for undifferentiated heterotrophic plastids. J. Prot. Res. 3, 1128–1137. doi: 10.1021/pr0499186
Baldassi, A. C., Balbuena, T. S. (2022). The Eucalyptus grandis chloroplast proteome: seasonal variations in leaf development. PloS One 17, e0265134. doi: 10.1371/journal.pone.0265134
Barja, M. V., Ezquerro, M., Beretta, S., Diretto, G., Florez-Sarasa, I., Feixes, E., et al. (2021). Several geranylgeranyl diphosphate synthase isoforms supply metabolic substrates for carotenoid biosynthesis in tomato. New Phytol. 231, 255–272. doi: 10.1111/nph.17283
Barsan, C., Sanchez-Bel, P., Rombaldi, C., Egea, I., Rossignol, M., Kuntz, M., et al. (2010). Characteristics of the tomato chromoplast revealed by proteomic analysis. J. Exp. Bot. 61, 2413–2431. doi: 10.1093/jxb/erq070
Barsan, C., Zouine, M., Maza, E., Bian, W., Egea, I., Rossignol, M., et al. (2012). Proteomic analysis of chloroplast-to-chromoplast transition in tomato reveals metabolic shifts coupled with disrupted thylakoid biogenesis machinery and elevated energy-production components. Plant Physiol. 160, 708–725. doi: 10.1104/pp.112.203679
Barton, K. A., Wozny, M. R., Mathur, N., Jaipargas, E. A., Mathur, J. (2018). Chloroplast behaviour and interactions with other organelles in Arabidopsis thaliana pavement cells. J. Cell Sci. 131, jcs202275. doi: 10.1242/jcs.202275
Bayer, R. G., Stael, S., Csaszar, E., Teige, M. (2011). Mining the soluble chloroplast proteome by affinity chromatography. Proteomics 11, 1287–1299. doi: 10.1002/pmic.201000495
Bellin, L., Melzer, M., Hilo, A., Garza Amaya, D. L., Keller, I., Meurer, J., et al. (2021). Pyrimidine nucleotide availability is essential for efficient photosynthesis, ROS scavenging, and organelle development. BioRxv. doi: 10.1101/2021.01.22.427776
Berardini, T. Z., Reiser, L., Li, D., Mezheritsky, Y., Muller, R., Strait, E., et al. (2015). The Arabidopsis information resource: making and mining the “gold standard” annotated reference plant genome. Genesis 53, 474–485. doi: 10.1002/dvg.22877
Bhattacharya, O., Ortiz, I., Walling, L. L. (2020). Methodology: an optimized, high-yield tomato leaf chloroplast isolation and stroma extraction protocol for proteomics analyses and identification of chloroplast co-localizing proteins. Plant Meth 16, 131. doi: 10.1186/s13007-020-00667-5
Briesemeister, S., Rahnenführer, J., Kohlbacher, O. (2010). YLoc–an interpretable web server for predicting subcellular localization. Nuc. Acids Res. 38, W497–W502. doi: 10.1093/nar/gkq477
Buchanan, B. B., Gruissem, W., Jones, R. L. (2015). Biochemistry & molecular biology of plants (Chichester, England: Wiley Blackwell), 1280.
Cai, B., Li, Q., Xu, Y., Yang, L., Bi, H., Ai, X. (2016). Genome-wide analysis of the fructose 1,6-bisphosphate aldolase (FBA) gene family and functional characterization of FBA7 in tomato. Plant Physiol. Bioch. 108, 251–265. doi: 10.1016/j.plaphy.2016.07.019
Carter, C., Pan, S., Zouhar, J., Avila, E. L., Girke, T., Raikhel, N. V. (2004). The vegetative vacuole proteome of Arabidopsis thaliana reveals predicted and unexpected proteins. Plant Cell 16, 3285–3303. doi: 10.1105/tpc.104.027078
Cejudo, F. J., Ojeda, V., Delgado-Requerey, V., González, M., Pérez-Ruiz, J. M. (2019). Chloroplast redox regulatory mechanisms in plant adaptation to light and darkness. Front. Plant Sci. 10. doi: 10.3389/fpls.2019.00380
Chan, K. X., Wirtz, M., Phua, S. Y., Estavillo, G. M., Pogson, B. J. (2013). Balancing metabolites in drought: the sulfur assimilation conundrum. Trends Plant Sci. 18, 18–29. doi: 10.1016/j.tplants.2012.07.005
Chang, S. H., Lee, S., Um, T. Y., Kim, J.-K., Do Choi, Y., Jang, G. (2017). pTAC10, a key subunit of plastid-encoded RNA polymerase, promotes chloroplast development. Plant Physiol. 174, 435–449. doi: 10.1104/pp.17.00248
Chao, W. S., Gu, Y. Q., Pautot, V., Bray, E. A., Walling, L. L. (1999). Leucine aminopeptidase RNAs, proteins, and activities increase in response to water deficit, salinity, and the wound signals systemin, methyl jasmonate, and abscisic acid. Plant Physiol. 120, 979–992. doi: 10.1104/pp.120.4.979
Chen, H., Boutros, P. C. (2011). VennDiagram: a package for the generation of highly-customizable Venn and Euler diagrams in r. BMC Bioinform. 12, 35. doi: 10.1186/1471-2105-12-35
Cheng, F., Zhou, Y.-H., Xia, X.-J., Shi, K., Zhou, J., Yu, J.-Q. (2014). Chloroplastic thioredoxin-f and thioredoxin-m1/4 play important roles in brassinosteroids-induced changes in CO(2) assimilation and cellular redox homeostasis in tomato. J. Expt. Bot. 65, 4335–4347. doi: 10.1093/jxb/eru207
Cline, K., Dabney-Smith, C. (2008). Plastid protein import and sorting: different paths to the same compartments. Curr. Opin. Plant Biol. 11, 585–592. doi: 10.1016/j.pbi.2008.10.008
Daniell, H., Lee, S.-B., Grevich, J., Saski, C., Quesada-Vargas, T., Guda, C., et al. (2006). Complete chloroplast genome sequences of Solanum bulbocastanum, Solanum lycopersicum and comparative analyses with other Solanaceae genomes. Theoret. Appl. Genet. 112, 1503–1518. doi: 10.1007/s00122-006-0254-x
D’Andrea, L., Simon-Moya, M., Llorente, B., Llamas, E., Marro, M., Loza-Alvarez, P., et al. (2018). Interference with clp protease impairs carotenoid accumulation during tomato fruit ripening. J. Expt. Bot. 69, 1557–1568. doi: 10.1093/jxb/erx491
de Luna-Valdez, L. A., Villaseñor-Salmerón, C. I., Cordoba, E., Vera-Estrella, R., León-Mejía, P., Guevara-García, A. A. (2019). Functional analysis of the chloroplast GrpE (CGE) proteins from Arabidopsis thaliana. Plant Physiol. Bioch. 139, 293–306. doi: 10.1016/j.plaphy.2019.03.027
de Souza, A., Wang, J. Z., Dehesh, K. (2017). Retrograde signals: integrators of interorganellar communication and orchestrators of plant development. Annu. Rev. Plant Biol. 68, 85–108. doi: 10.1146/annurev-arplant-042916-041007
Dobson, L., Reményi, I., Tusnády, G. E. (2015). CCTOP: a consensus constrained TOPology prediction web server. Nucleic Acids Res. 43, W408–W412. doi: 10.1093/nar/gkv451
Dogra, V., Duan, J. L., Lee, K. P., Lv, S. S., Liu, R. Y., Kim, C. H. (2017). FtsH2-dependent proteolysis of EXECUTER1 is essential in mediating singlet oxygen-triggered retrograde signaling in Arabidopsis thaliana. Front. Plant Sci. 8. doi: 10.3389/fpls.2017.01145
Drakakaki, G., van de Ven, W., Pan, S., Miao, Y., Wang, J., Keinath, N. F., et al. (2012). Isolation and proteomic analysis of the SYP61 compartment reveal its role in exocytic trafficking in Arabidopsis. Cell Res. 22, 413–424. doi: 10.1038/cr.2011.129
Duan, J. L., Lee, K. P., Dogra, V., Zhang, S. Y., Liu, K. W., Caceres-Moreno, C., et al. (2019). Impaired PSII proteostasis promotes retrograde signaling via salicylic acid. Plant Physiol. 180, 2182–2197. doi: 10.1104/pp.19.00483
Duan, Z., Li, K., Zhang, L., Che, L., Lu, L., Rochaix, J.-D., et al. (2020). F-type ATP synthase assembly factors Atp11 and Atp12 in Arabidopsis. Front. Plant Sci. 11. doi: 10.3389/fpls.2020.522753
Duchene, A. M., Giritch, A., Hoffmann, B., Cognat, V., Lancelin, D., Peeters, N. M., et al. (2005). Dual targeting is the rule for organellar aminoacyl-tRNA synthetases in Arabidopsis thaliana. proc. Natl. Acad. Sci. U.S.A. 102, 16484–16489. doi: 10.1073/pnas.0504682102
Elias, J. E., Haas, W., Faherty, B. K., Gygi, S. P. (2005). Comparative evaluation of mass spectrometry platforms used in large-scale proteomics investigations. Nat. Methods 2, 667–675. doi: 10.1038/nmeth785
Emanuelsson, O., Brunak, S., von Heijne, G., Nielsen, H. (2007). Locating proteins in the cell using TargetP, SignalP and related tools. Nat. Proto. 2, 953–971. doi: 10.1038/nprot.2007.131
Emanuelsson, O., Nielsen, H., Brunak, S., von Heijne, G. (2000). Predicting subcellular localization of proteins based on their N-terminal amino acid sequence. J. Mol. Biol. 300, 1005–1016. doi: 10.1006/jmbi.2000.3903
Emanuelsson, O., Nielsen, H., Von Heijne, G. (1999). ChloroP, a neural network-based method for predicting chloroplast transit peptides and their cleavage sites. Prot. Sci. 8, 978–984. doi: 10.1110/ps.8.5.978
Exposito-Rodriguez, M., Laissue, P. P., Yvon-Durocher, G., Smirnoff, N., Mullineaux, P. M. (2017). Photosynthesis-dependent H2O2 transfer from chloroplasts to nuclei provides a high-light signalling mechanism. Nat. Commun. 8, 4. doi: 10.1038/s41467-017-00074-w
Fernandez, J. C., Burch-Smith, T. M. (2019). Chloroplasts as mediators of plant biotic interactions over short and long distances. Curr. Opin. Plant Biol. 50, 148–155. doi: 10.1016/j.pbi.2019.06.002
Ferro, M., Salvi, D., Brugière, S., Miras, S., Kowalski, S., Louwagie, M., et al. (2003). Proteomics of the chloroplast envelope membranes from Arabidopsis thaliana. mol. Cell Prot. 2, 325–345. doi: 10.1074/mcp.M300030-MCP200
Fichman, Y., Mittler, R. (2020). Rapid systemic signaling during abiotic and biotic stresses: is the ROS wave master of all trades? Plant J. 102, 887–896. doi: 10.1111/tpj.14685
Fowler, J. H., Aromdee, D. N., Pautot, V., Holzer, F. M., Walling, L. L. (2009). Leucine aminopeptidase regulates defense and wound signaling downstream of jasmonic acid. Plant Cell 21, 1239–1251. doi: 10.1105/tpc.108.065029
Friso, G., Giacomelli, L., Ytterberg, A. J., Peltier, J. B., Rudella, A., Sun, Q., et al. (2004). In-depth analysis of the thylakoid membrane proteome of Arabidopsis thaliana chloroplasts: new proteins, new functions, and a plastid proteome database. Plant Cell 16, 478–499. doi: 10.1105/tpc.017814
Fristedt, R., Williams-Carrier, R., Merchant, S. S., Barkan, A. (2014). A thylakoid membrane protein harboring a DnaJ-type zinc finger domain is required for photosystem I accumulation in plants. J. Biol. Chem. 289, 30657–30667. doi: 10.1074/jbc.m114.587758
Gabruk, M., Mysliwa-Kurdziel, B. (2020). The origin, evolution and diversification of multiple isoforms of light-dependent protochlorophyllide oxidoreductase (LPOR): focus on angiosperms. Biochem. J. 477, 2221–2236. doi: 10.1042/bcj20200323
Gao, Z.-P., Chen, G.-X., Yang, Z.-N. (2012). Regulatory role of Arabidopsis pTAC14 in chloroplast development and plastid gene expression. Plant Sig. Behav. 7, 1354–1356. doi: 10.4161/psb.21618
Gao, H. B., Metz, J., Teanby, N. A., Ward, A. D., Botchway, S. W., Coles, B., et al. (2016). In vivo quantification of peroxisome tethering to chloroplasts in tobacco epidermal cells using optical tweezers. Plant Physiol. 170, 263–272. doi: 10.1104/pp.15.01529
Gibbs, D. J., Bailey, M., Tedds, H. M., Holdsworth, M. J. (2016). From start to finish: amino-terminal protein modifications as degradation signals in plants. New Phytol. 211, 1188–1194. doi: 10.1111/nph.14105
Gu, Y.-Q., Chao, W. S., Walling, L. L. (1996a). Localization and post-translational processing of the wound-induced leucine aminopeptidase proteins of tomato. J. Biol. Chem. 271, 25880–25887. doi: 10.1074/jbc.271.42.25880
Gu, Y. Q., Holzer, F. M., Walling, L. L. (1999). Overexpression, purification and biochemical characterization of the wound-induced leucine aminopeptidase of tomato. Eur. J. Biochem. 263, 726–735. doi: 10.1046/j.1432-1327.1999.00548.x
Gu, Y. Q., Pautot, V., Holzer, F. M., Walling, L. L. (1996b). A complex array of proteins related to the multimeric leucine aminopeptidase of tomato. Plant Physiol. 110, 1257–1266. doi: 10.1104/pp.110.4.1257
Gu, Y.-Q., Walling, L. L. (2000). Specificity of the wound-induced leucine aminopeptidase (LAP-a) of tomato: activity on dipeptide and tripeptide substrates. Eur. J. Biochem. 267, 1178–1187. doi: 10.1046/j.1432-1327.2000.01116.x
Hahn, A., Vonck, J., Mills, D. J., Meier, T., Kühlbrandt, W. (2018). Structure, mechanism, and regulation of the chloroplast ATP synthase. Science 360, eaat4318. doi: 10.1126/science.aat4318
Hallgren, J., Tsirigos, K. D., Pedersen, M. D., Armenteros, J. J. A., Marcatili, P., Nielsen, H., et al. (2022). DeepTMHMM predicts alpha and beta transmembrane proteins using deep neural networks. bioRxiv 2004, 487609. doi: 10.1101/2022.04.08.487609
Higa, T., Suetsugu, N., Kong, S. G., Wada, M. (2014). Actin-dependent plastid movement is required for motive force generation in directional nuclear movement in plants. Proc. Natl. Acad. Sci. U.S.A. 111, 4327–4331. doi: 10.1073/pnas.1317902111
Hofmann, K., Stoffel, W. (1993). TMbase - a database of membrane spanning protein segments biol. Chem. Hoppe-Seyler 374, 166. doi: 10.1515/bchm3.1993.374.1-6.143
Hooper, C. M., Castleden, I. R., Tanz, S. K., Aryamanesh, N., Millar, A. H. (2017). SUBA4: the interactive data analysis centre for Arabidopsis subcellular protein locations. Nuc. Acids Res. 45, D1064–D1074. doi: 10.1093/nar/gkw1041
Horton, P., Park, K. J., Obayashi, T., Fujita, N., Harada, H., Adams-Collier, C. J., et al. (2007). WoLF PSORT: protein localization predictor. Nucleic Acids Res. 35, W585–W587. doi: 10.1093/nar/gkm259
Huang, M. S., Friso, G., Nishimura, K., Qu, X., Olinares, P. D. B., Majeran, W., et al. (2013). Construction of plastid reference proteomes for maize and Arabidopsis and evaluation of their orthologous relationships: the concept of orthoproteomics. J. Prot. Res. 12, 491–504. doi: 10.1021/pr300952g
Huang, C., Yu, Q.-B., Lv, R.-H., Yin, Q.-Q., Chen, G.-Y., Xu, L., et al. (2013). The reduced plastid-encoded polymerase-dependent plastid gene expression leads to the delayed greening of the Arabidopsis fln2 mutant. PloS One 8, e73092. doi: 10.1371/journal.pone.0073092
Huerta-Cepas, J., Szklarczyk, D., Heller, D., Hernández-Plaza, A., Forslund, S. K., Cook, H., et al. (2019). eggNOG 5.0: a hierarchical, functionally and phylogenetically annotated orthology resource based on 5090 organisms and 2502 viruses. Nuc. Acids Res. 47, D309–D314. doi: 10.1093/nar/gky1085
Initiative, T. A. G. (2000). Analysis of the genome sequence of the flowering plant Arabidopsis thaliana. Nature 408, 796–815. doi: 10.1038/35048692
Ishihama, Y., Oda, Y., Tabata, T., Sato, T., Nagasu, T., Rappsilber, J., et al. (2005). Exponentially modified protein abundance index (emPAI) for estimation of absolute protein amount in proteomics by the number of sequenced peptides per protein. Mol. Cell Prot. 4, 1265–1272. doi: 10.1074/mcp.M500061-MCP200
Islam, M. S., Takagi, S. (2010). Co-Localization of mitochondria with chloroplasts is a light-dependent reversible response. Plant Sig. Behav. 5 (146), 147–146. doi: 10.4161/psb.5.2.10410
Jarvis, P., López-Juez, E. (2013). Biogenesis and homeostasis of chloroplasts and other plastids. Nat. Rev. Mol. Cell Biol. 14, 787–802. doi: 10.1038/nrm3702
Kahlau, S., Aspinall, S., Gray, J. C., Bock, R. (2006). Sequence of the tomato chloroplast DNA and evolutionary comparison of Solanaceous plastid genomes. J. Mol. Evol. 63, 194–207. doi: 10.1007/s00239-005-0254-5
Kirby, J., Keasling, J. D. (2009). Biosynthesis of plant isoprenoids: perspectives for microbial engineering. Annu. Rev. Plant Biol. 60, 335–355. doi: 10.1146/annurev.arplant.043008.091955
Kleffmann, T., Hirsch-Hoffmann, M., Gruissem, W., Baginsky, S. (2006). Plprot: a comprehensive proteome database for different plastid types. Plant Cell Physiol. 47, 432–436. doi: 10.1093/pcp/pcj005
Kleffmann, T., Russenberger, D., von Zychlinski, A., Christopher, W., Sjölander, K., Gruissem, W., et al. (2004). The Arabidopsis thaliana chloroplast proteome reveals pathway abundance and novel protein functions. Curr. Biol. 14, 354–362. doi: 10.1016/j.cub.2004.02.039
Kmiec, B., Branca, R. M. M., Berkowitz, O., Li, L., Wang, Y., Murcha, M. W., et al. (2018). Accumulation of endogenous peptides triggers a pathogen stress response in Arabidopsis thaliana. Plant J. 96, 705–715. doi: 10.1111/tpj.14100
Kmiec, B., Teixeira, P. F., Glaser, E. (2014). Shredding the signal: targeting peptide degradation in mitochondria and chloroplasts. Trends Plant Sci. 19, 771–778. doi: 10.1016/j.tplants.2014.09.004
Krause, K., Oetke, S., Krupinska, K. (2012). Dual targeting and retrograde translocation: regulators of plant nuclear gene expression can be sequestered by plastids. Int. J. Mol. Sci. 13, 11085–11101. doi: 10.3390/ijms130911085
Krupinska, K., Blanco, N. E., Oetke, S., Zottini, M. (2020). Genome communication in plants mediated by organelle–nucleus-located proteins. Phil. Trans. R. Soc B: Biol. Sci. 375, 20190397. doi: 10.1098/rstb.2019.0397
Kumaran, D. (2005). Structure and mechanism of ADP-ribose-1''-monophosphatase (Appr-1''-pase), a ubiquitous cellular processing enzyme. Prot. Sci. 14, 719–726. doi: 10.1110/ps.041132005
Laizet, Y. H., Pontier, D., Mache, R., Kuntz, M. (2004). Subfamily organization and phylogenetic origin of genes encoding plastid lipid-associated proteins of the fibrillin type. J. Genome Sci. Tech. 3, 19–28.
Lancien, M., Lea, P. J., Azevedo, R. A. (2007). “Amino acid synthesis in plastids,” in The structure and function of plastids (Dordrecht, Netherlands: Springer Netherlands), 355–385. doi: 10.1007/978-1-4020-4061-0_18
Lee, K. P., Kim, C., Landgraf, F., Apel, K. (2007). EXECUTER1- and EXECUTER2-dependent transfer of stress-related signals from the plastid to the nucleus of Arabidopsis thaliana. Proc. Natl. Acad. Sci. U.S.A. 104, 10270–10275. doi: 10.1073/pnas.0702061104
Lennartz, K., Plücken, H., Seidler, A., Westhoff, P., Bechtold, N., Meierhoff, K. (2001). HCF164 encodes a thioredoxin-like protein involved in the biogenesis of the cytochrome b6f complex in Arabidopsis. Plant Cell 13, 2539–2551. doi: 10.1105/tpc.010245
Li, Y., Liu, B., Zhang, J., Kong, F., Zhang, L., Meng, H., et al. (2019). OHP1, OHP2, and HCF244 form a transient functional complex with the photosystem II reaction center. Plant Physiol. 179, 195–208. doi: 10.1104/pp.18.01231
Li-Beisson, Y., Shorrosh, B., Beisson, F., Andersson, M. X., Arondel, V., Bates, P. D., et al. (2013). Acyl-lipid metabolism. Arabidopsis Book 11, e0161. doi: 10.1199/tab.0161
Liu, J., Last, R. L. (2017). A chloroplast thylakoid lumen protein is required for proper photosynthetic acclimation of plants under fluctuating light environments. Proc. Natl. Acad. Sci. U.S.A. 114, E8110–E8117. doi: 10.1073/pnas.1712206114
Liu, S., Liu, T., Wang, E., Cheng, Y., Liu, T., Chen, G., et al. (2022). Dissecting the chloroplast proteome of the potato (Solanum tuberosum L.) and its comparison with the tuber amyloplast proteome. Plants (Basel) 11, 1915. doi: 10.3390/plants11151915
Liu, J., Yang, H., Lu, Q., Wen, X., Chen, F., Peng, L., et al. (2012). PSBP-DOMAIN PROTEIN1, a nuclear-encoded thylakoid lumenal protein, is essential for photosystem I assembly in Arabidopsis. Plant Cell 24, 4992–5006. doi: 10.1105/tpc.112.106542
Lohse, M., Nagel, A., Herter, T., May, P., Schroda, M., Zrenner, R., et al. (2014). Mercator: A fast and simple web server for genome scale functional annotation of plant sequence data. Plant Cell Environ. 37, 1250–1258. doi: 10.1111/pce.12231
Lu, Y. (2016). Identification and roles of photosystem II assembly, stability, and repair factors in Arabidopsis. Front. Plant Sci. 7. doi: 10.3389/fpls.2016.00168
Lundquist, P. K., Mantegazza, O., Stefanski, A., Stuhler, K., Weber, A. P. M. (2017). Surveying the oligomeric state of Arabidopsis thaliana chloroplasts. Mol. Plant 10, 197–211. doi: 10.1016/j.molp.2016.10.011
Majeran, W., Friso, G., Asakura, Y., Qu, X., Huang, M. S., Ponnala, L., et al. (2012). Nucleoid-enriched proteomes in developing plastids and chloroplasts from maize leaves: a new conceptual framework for nucleoid functions. Plant Physiol. 158, 156–189. doi: 10.1104/pp.111.188474
Malone, L. A., Qian, P., Mayneord, G. E., Hitchcock, A., Farmer, D. A., Thompson, R. F., et al. (2019). Cryo-EM structure of the spinach cytochrome b6 f complex at 3.6 Å resolution. Nature 575, 535–539. doi: 10.1038/s41586-019-1746-6
Mao, J., Chi, W., Ouyang, M., He, B., Chen, F., Zhang, L. (2015). PAB is an assembly chaperone that functions downstream of chaperonin 60 in the assembly of chloroplast ATP synthase coupling factor 1. Proc. Natl. Acad. Sci. U.S.A. 112, 4152–4157. doi: 10.1073/pnas.1413392111
Marino, G., Naranjo, B., Wang, J., Penzler, J. F., Kleine, T., Leister, D. (2019). Relationship of GUN1 to FUG1 in chloroplast protein homeostasis. Plant J. 99, 521–535. doi: 10.1111/tpj.14342
Masclaux-Daubresse, C., Daniel-Vedele, F., Dechorgnat, J., Chardon, F., Gaufichon, L., Suzuki, A. (2010). Nitrogen uptake, assimilation and remobilization in plants: challenges for sustainable and productive agriculture. Ann. Bot. 105, 1141–1157. doi: 10.1093/aob/mcq028
Mehrshahi, P., Stefano, G., Andaloro, J. M., Brandizzi, F., Froehlich, J. E., DellaPenna, D. (2013). Transorganellar complementation redefines the biochemical continuity of endoplasmic reticulum and chloroplasts. Proc. Natl. Acad. Sci. U.S.A. 110, 12126–12131. doi: 10.1073/pnas.1306331110
Melonek, J., Oetke, S., Krupinska, K. (2016). Multifunctionality of plastid nucleoids as revealed by proteome analyses. Biochim. Biophys. Acta Prot. Proteom. 1864, 1016–1038. doi: 10.1016/j.bbapap.2016.03.009
Millar, A. H., Whelan, J., Small, I. (2006). Recent surprises in protein targeting to mitochondria and plastids. Curr. Opin. Plant Biol. 9, 610–615. doi: 10.1016/j.pbi.2006.09.002
Mullineaux, P. M., Exposito-Rodriguez, M., Laissue, P. P., Smirnoff, N., Park, E. (2020). Spatial chloroplast-to-nucleus signalling involving plastid–nuclear complexes and stromules. Phil. Trans. R. Soc B: Biol. Sci. 375, 20190405. doi: 10.1098/rstb.2019.0405
Munekage, Y., Hojo, M., Meurer, J., Endo, T., Tasaka, M., Shikanai, T. (2002). PGR5 is involved in cyclic electron flow around photosystem I and is essential for photoprotection in Arabidopsis. Cell 110, 361–371. doi: 10.1016/s0092-8674(02)00867-x
Nakai, M. (2018). New perspectives on chloroplast protein import. Plant Cell Physiol. 59, 1111–1119. doi: 10.1093/pcp/pcy083
Narváez-Vásquez, J., Tu, C. J., Park, S. Y., Walling, L. L. (2008). Targeting and localization of wound-inducible leucine aminopeptidase a in tomato leaves. Planta 227, 341–351. doi: 10.1007/s00425-007-0621-0
Naver, H., Boudreau, E., Rochaix, J. D. (2001). Functional studies of Ycf3: its role in assembly of photosystem I and interactions with some of its subunits. Plant Cell 13, 2731–2745. doi: 10.1105/tpc.010253
Nellaepalli, S., Ozawa, S.-I., Kuroda, H., Takahashi, Y. (2018). The photosystem I assembly apparatus consisting of Ycf3–Y3IP1 and Ycf4 modules. Nat. Comm. 9, 2439. doi: 10.1038/s41467-018-04823-3
Newman, S. M., Eannetta, N. T., Yu, H., Prince, J. P., Carmen De Vicente, M., Tanksley, S. D., et al. (1993). Organisation of the tomato polyphenol oxidase gene family. Plant Mol. Biol. 21, 1035–1051. doi: 10.1007/bf00023601
Nishimura, K., Kato, Y., Sakamoto, W. (2017). Essentials of proteolytic machineries in chloroplasts. Mol. Plant 10, 4–19. doi: 10.1016/j.molp.2016.08.005
Oikawa, K., Hayashi, M., Hayashi, Y., Nishimura, M. (2019). Re-evaluation of physical interaction between plant peroxisomes and other organelles using live-cell imaging techniques. J. Integ. Plant Biol. 61, 836–852. doi: 10.1111/jipb.12805
Olinares, P. D. B., Ponnala, L., van Wijk, K. J. (2010). Megadalton complexes in the chloroplast stroma of Arabidopsis thaliana characterized by size exclusion chromatography, mass spectrometry, and hierarchical clustering. Mol. Cell Prot. 9, 1594–1615. doi: 10.1074/mcp.M000038-MCP201
op den Camp, R. G. L., Przybyla, D., Ochsenbein, C., Laloi, C., Kim, C., Danon, A., et al. (2003). Rapid induction of distinct stress responses after the release of singlet oxygen in Arabidopsis. Plant Cell 15, 2320–2332. doi: 10.1105/tpc.014662
Paetzold, H., Garms, S., Bartram, S., Wieczorek, J., Urós-Gracia, E.-M., Rodríguez-Concepción, M., et al. (2010). The isogene 1-Deoxy-D-Xylulose 5-phosphate synthase 2 controls isoprenoid profiles, precursor pathway allocation, and density of tomato trichomes. Mol. Plant 3, 904–916. doi: 10.1093/mp/ssq032
Peltier, J. B., Cai, Y., Sun, Q., Zabrouskov, V., Giacomelli, L., Rudella, A., et al. (2006). The oligomeric stromal proteome of Arabidopsis thaliana chloroplasts. Mol. Cell Prot. 5, 114–133. doi: 10.1074/mcp.M500180-MCP200
Peltier, J. B., Emanuelsson, O., Kalume, D. E., Ytterberg, J., Friso, G., Rudella, A., et al. (2002). Central functions of the lumenal and peripheral thylakoid proteome of Arabidopsis determined by experimentation and genome-wide prediction. Plant Cell 14, 211–236. doi: 10.1105/tpc.010304
Peng, L., Fukao, Y., Fujiwara, M., Takami, T., Shikanai, T. (2009). Efficient operation of NAD(P)H dehydrogenase requires supercomplex formation with photosystem I via minor LHCI in Arabidopsis. Plant Cell 21, 3623–3640. doi: 10.1105/tpc.109.068791
Petsalaki, E. I., Bagos, P. G., Litou, Z. I., Hamodrakas, S. J. (2006). PredSL: a tool for the N-terminal sequence-based prediction of protein subcellular localization. Genom. Proteo. Bioinfo. 4, 48–55. doi: 10.1016/S1672-0229(06)60016-8
Ploscher, M., Reisinger, V., Eichacker, L. A. (2011). Proteomic comparison of etioplast and chloroplast protein complexes. J. Prot. 74, 1256–1265. doi: 10.1016/j.jprot.2011.03.020
Rappsilber, J., Ryder, U., Lamond, A. I., Mann, M. (2002). Large-Scale proteomic analysis of the human spliceosome. Genome Res. 12, 1231–1245. doi: 10.1101/gr.473902
Reumann, S., Babujee, L., Ma, C., Wienkoop, S., Siemsen, T., Antonicelli, G. E., et al. (2007). Proteome analysis of Arabidopsis leaf peroxisomes reveals novel targeting peptides, metabolic pathways, and defense mechanisms. Plant Cell 19, 3170–3193. doi: 10.1105/tpc.107.050989
Richly, E., Leister, D. (2004). An improved prediction of chloroplast proteins reveals diversities and commonalities in the chloroplast proteomes of Arabidopsis and rice. Gene 329, 11–16. doi: 10.1016/j.gene.2004.01.008
Rolland, N., Curien, G., Finazzi, G., Kuntz, M., Marechal, E., Matringe, M., et al. (2012). The biosynthetic capacities of the plastids and integration between cytoplasmic and chloroplast processes. Annu. Rev. Genet. 46, 233–264. doi: 10.1146/annurev-genet-110410-132544
Rosenberg, L. A., Padgett, P. E., Assmann, S. M., Walling, L. L., Leonard, R. T. (1997). Identification of mRNAs and proteins in higher plants using probes from the band 3 anion transporter of mammals. J. Expt. Bot. 48, 857–868. doi: 10.1093/jxb/48.4.857
Rowland, E., Kim, J., Friso, G., Poliakov, A., Ponnala, L., Sun, Q., et al. (2022). The CLP and PREP protease systems coordinate maturation and degradation of the chloroplast proteome in Arabidopsis thaliana. New Phytol. 236, 1339–1357. doi: 10.1111/nph.18426
Sant’Ana, D. V. P., Lefsrud, M. (2018). Tomato proteomics: tomato as a model for crop proteomics. Sci. Hortic. 239, 224–233. doi: 10.1016/j.scienta.2018.05.041
Sato, R., Kono, M., Harada, K., Ohta, H., Takaichi, S., Masuda, S. (2017). FLUCTUATING-LIGHT-ACCLIMATION PROTEIN1, conserved in oxygenic phototrophs, regulates H+ homeostasis and non-photochemical quenching in chloroplasts. Plant Cell Physiol. 58, 1622–1630. doi: 10.1093/pcp/pcx110
Sato, S., Nakamura, Y., Kaneko, T., Asamizu, E., Tabata, S. (1999). Complete structure of the chloroplast genome of Arabidopsis thaliana. DNA Res. 6, 283–290. doi: 10.1093/dnares/6.5.283
Schubert, M., Petersson, U. A., Haas, B. J., Funk, C., Schroder, W. P., Kieselbach, T. (2002). Proteome map of the chloroplast lumen of Arabidopsis thaliana. J. Biol. Chem. 277, 8354–8365. doi: 10.1074/jbc.M108575200
Scranton, M., Fowler, J. H., Girke, T., Walling, L. L. (2013). Microarray analysis of tomato’s early and late wound response reveals new regulatory targets for leucine aminopeptidase A. PloS One 8, e77889. doi: 10.1371/journal.pone.0077889
Scranton, M., Yee, A., Park, S. Y., Walling, L. L. (2012). Plant leucine aminopeptidases moonlight as molecular chaperones. J. Biol. Chem. 287, 18408–18417. doi: 10.1074/jbc.M111.309500
Shen, J., Williams-Carrier, R., Barkan, A. (2017). PSA3, a protein on the stromal face of the thylakoid membrane, promotes photosystem I accumulation in cooperation with the assembly factor PYG7. Plant Physiol. 174, 1850–1862. doi: 10.1104/pp.17.00524
Shikanai, T. (2016). Chloroplast NDH: a different enzyme with a structure similar to that of respiratory NADH dehydrogenase. Biochim. Biophys. Acta Bioenerg. 1857, 1015–1022. doi: 10.1016/j.bbabio.2015.10.013
Siddique, M. A., Grossmann, J., Gruissem, W., Baginsky, S. (2006). Proteome analysis of bell pepper (Capsicum annuum L.) chromoplasts. Plant Cell Physiol. 47, 1663–1673. doi: 10.1093/pcp/pcl033
Small, I., Peeters, N., Legeai, F., Lurin, C. (2004). Predotar: a tool for rapidly screening proteomes for N-terminal targeting sequences. Proteomics 4, 1581–1590. doi: 10.1002/pmic.200300776
Stanley, L., Yuan, Y.-W. (2019). Transcriptional regulation of carotenoid biosynthesis in plants: so many regulators, so little consensus. Front. Plant Sci. 10. doi: 10.3389/fpls.2019.01017
Sun, A.-Q., Yi, S.-Y., Yang, J.-Y., Zhao, C.-M., Liu, J. (2006). Identification and characterization of a heat-inducible FtsH gene from tomato (Lycopersicon esculentum Mill.). Plant Sci. 170, 551–562. doi: 10.1016/j.plantsci.2005.10.010
Sun, Q., Zybailov, B., Majeran, W., Friso, G., Olinares, P. D., van Wijk, K. J. (2009). PPDB, the plant proteomics database at Cornell. Nucleic Acids Res. 37, D969–D974. doi: 10.1093/nar/gkn654
Tamburino, R., Vitale, M., Ruggiero, A., Sassi, M., Sannino, L., Arena, S., et al. (2017). Chloroplast proteome response to drought stress and recovery in tomato (Solanum lycopersicum L.). BMC Plant Biol. 17, 40. doi: 10.1186/s12870-017-0971-0
Thimm, O., Bläsing, O., Gibon, Y., Nagel, A., Meyer, S., Krüger, P., et al. (2004). MAPMAN: a user-driven tool to display genomics data sets onto diagrams of metabolic pathways and other biological processes. Plant J. 37, 914–939. doi: 10.1111/j.1365-313x.2004.02016.x
Thomson, S. M., Pulido, P., Jarvis, R. P. (2020). Protein import into chloroplasts and its regulation by the ubiquitin-proteasome system. Biochem. Soc Trans. 48, 71–82. doi: 10.1042/bst20190274
Tran, L. T., Taylor, J. S., Constabel, C. (2012). The polyphenol oxidase gene family in land plants: Lineage-specific duplication and expansion. BMC Genomics 13, 395. doi: 10.1186/1471-2164-13-395
Trösch, R., Töpel, M., Flores-Pérez, Ú., Jarvis, P. (2015). Genetic and physical interaction studies reveal functional similarities between ALBINO3 and ALBINO4 in Arabidopsis. Plant Physiol. 169, 1292–1306. doi: 10.1104/pp.15.00376
Unal, D., García-Caparrós, P., Kumar, V., Dietz, K.-J. (2020). Chloroplast-associated molecular patterns as concept for fine-tuned operational retrograde signalling. Phil. Trans. R. Soc B: Biol. Sci. 375, 20190443. doi: 10.1098/rstb.2019.0443
Von Zychlinski, A., Kleffmann, T., Krishnamurthy, N., Sjölander, K., Baginsky, S., Gruissem, W. (2005). Proteome analysis of the rice etioplast. Mol. Cell Prot. 4, 1072–1084. doi: 10.1074/mcp.m500018-mcp200
Vu, N. T., Kamiya, K., Fukushima, A., Hao, S., Ning, W., Ariizumi, T., et al. (2019). Comparative co-expression network analysis extracts the SlHSP70 gene affecting to shoot elongation of tomato. Plant Biotech. 36, 143–153. doi: 10.5511/plantbiotechnology.19.0603a
Walling, L. L. (2006). Recycling or regulation? The role of amino-terminal modifying enzymes. Curr. Opin. Plant Biol. 9, 227–233. doi: 10.1016/j.pbi.2006.03.009
Wang, L., Kim, C., Xu, X., Piskurewicz, U., Dogra, V., Singh, S., et al. (2016). Singlet oxygen- and EXECUTER1-mediated signaling is initiated in grana margins and depends on the protease FtsH2. Proc. Natl. Acad. Sci. U.S.A. 113, E3792–E3800. doi: 10.1073/pnas.1603562113
Wang, L., Leister, D., Guan, L., Zheng, Y., Schneider, K., Lehmann, M., et al. (2020). The Arabidopsis SAFEGUARD1 suppresses singlet oxygen-induced stress responses by protecting grana margins. Proc. Natl. Acad. Sci. U.S.A. 117, 6918–6927. doi: 10.1073/pnas.1918640117
Wang, D., Portis, A. R. (2007). A novel nucleus-encoded chloroplast protein, PIFI, is involved in NAD(P)H dehydrogenase complex-mediated chlororespiratory electron transport in Arabidopsis. Plant Physiol. 144, 1742–1752. doi: 10.1104/pp.107.103218
Wang, Y., Selinski, J., Mao, C., Zhu, Y., Berkowitz, O., Whelan, J. (2020). Linking mitochondrial and chloroplast retrograde signalling in plants. Phil. Trans. R. Soc B: Biol. Sci. 375, 20190410. doi: 10.1098/rstb.2019.0410
Wang, Y. Q., Yang, Y., Fei, Z., Yuan, H., Fish, T., Thannhauser, T. W., et al. (2013). Proteomic analysis of chromoplasts from six crop species reveals insights into chromoplast function and development. J. Exp. Bot. 64, 949–961. doi: 10.1093/jxb/ers375
Waseem, M., Ahmad, F., Habib, S., Gao, Y., Li, Z. (2018). Genome-wide identification of FK506-binding domain protein gene family, its characterization, and expression analysis in tomato (Solanum lycopersicum L.). Gene 678, 143–154. doi: 10.1016/j.gene.2018.08.021
Wasternack, C., Song, S. S. (2017). Jasmonates: biosynthesis, metabolism, and signaling by proteins activating and repressing transcription. J. Expt. Bot. 68, 1303–1321. doi: 10.1093/jxb/erw443
Wise, R. R., Hooper, J. K. (2006). “The structure and function of plastids,” in Advances in photosynthesis and respiration (Dordrecht, Netherlands: Springer).
Wu, G. Z., Meyer, E. H., Richter, A. S., Schuster, M., Ling, Q. H., Schottler, M. A., et al. (2019). Control of retrograde signalling by protein import and cytosolic folding stress. Nat. Plants 5, 525–538. doi: 10.1038/s41477-019-0415-y
Yamamoto, H., Shikanai, T. (2019). PGR5-dependent cyclic electron flow protects photosystem I under fluctuating light at donor and acceptor sides. Plant Physiol. 179, 588–600. doi: 10.1104/pp.18.01343
Yang, C., Hu, H., Ren, H., Kong, Y., Lin, H., Guo, J., et al. (2016). LIGHT-INDUCED RICE1 regulates light-dependent attachment of LEAF-TYPE FERREDOXIN-NADP+ OXIDOREDUCTASE to the thylakoid membrane in rice and Arabidopsis. Plant Cell 28, 712–728. doi: 10.1105/tpc.15.01027
Yang, F., Xiao, K., Pan, H., Liu, J. (2021). Chloroplast: the emerging battlefield in plant–microbe interactions. Front. Plant Sci. 12. doi: 10.3389/fpls.2021.637853
Yoshida, K., Yokochi, Y., Hisabori, T. (2019). New light on chloroplast redox regulation: molecular mechanism of protein thiol oxidation. Front. Plant Sci. 10. doi: 10.3389/fpls.2019.01534
Zhang, L., Duan, Z., Zhang, J., Peng, L. (2016). BIOGENESIS FACTOR REQUIRED FOR ATP SYNTHASE 3 facilitates assembly of the chloroplast ATP synthase complex. Plant Physiol. 171, 1291–1306. doi: 10.1104/pp.16.00248
Zhang, L., Pu, H., Duan, Z., Li, Y., Liu, B., Zhang, Q., et al. (2018). Nucleus-encoded protein BFA1 promotes efficient assembly of the chloroplast ATP synthase coupling factor 1. Plant Cell 30, 1770–1788. doi: 10.1105/tpc.18.00075
Zhou, F., Pichersky, E. (2020). The complete functional characterisation of the terpene synthase family in tomato. New Phytol. 226, 1341–1360. doi: 10.1111/nph.16431
Keywords: chloroplast, leucine aminopeptidase, lumenal proteins, stroma, redox, protein homeostasis, proteomics, Solanum lycopersicum
Citation: Bhattacharya O, Ortiz I, Hendricks N and Walling LL (2023) The tomato chloroplast stromal proteome compendium elucidated by leveraging a plastid protein-localization prediction Atlas. Front. Plant Sci. 14:1020275. doi: 10.3389/fpls.2023.1020275
Received: 16 August 2022; Accepted: 22 June 2023;
Published: 28 August 2023.
Edited by:
Renu Deswal, University of Delhi, IndiaReviewed by:
Yusuke Kato, Setsunan University, JapanJean-David Rochaix, University of Geneva, Switzerland
Copyright © 2023 Bhattacharya, Ortiz, Hendricks and Walling. This is an open-access article distributed under the terms of the Creative Commons Attribution License (CC BY). The use, distribution or reproduction in other forums is permitted, provided the original author(s) and the copyright owner(s) are credited and that the original publication in this journal is cited, in accordance with accepted academic practice. No use, distribution or reproduction is permitted which does not comply with these terms.
*Correspondence: Linda L. Walling, bHdhbGxpbmdAdWNyLmVkdQ==
†Present address: Oindrila Bhattacharya, The Douglas Research Center, McGill University, Montreal, QC, Canada
Irma Ortiz, Beltsville Agricultural Research Center, Food Quality Laboratory, U.S. Department of Agriculture, Agricultural Research Service, Beltsville, MD, United States
Nathan Hendricks, Cedars Sinai Precision Biomarker Laboratories, Beverly Hills, CA, United States
‡ These authors contributed equally to this work and share first authorship