- 1Shapotou Desert Research and Experiment Station, Northwest Institute of Eco-Environment and Resources, Chinese Academy of Sciences, Lanzhou, China
- 2College of Biological Sciences and Engineering, North Minzu University, Yinchuan, China
- 3College of Pastoral Agriculture Science and Technology, Lanzhou University, Yinchuan, China
- 4Breeding Base for Key Laboratory Land Degradation and Ecological Restoration in Northwest China, Ningxia University, Yinchuan, China
When plants are subjected to various biotic and abiotic stresses, the root system responds actively by secreting different types and amounts of bioactive compounds, while affects the structure of rhizosphere soil bacterial community. Therefore, understanding plant–soil-microbial interactions, especially the strength of microbial interactions, mediated by root exudates is essential. A short-term experiment was conducted under drought and salt stress to investigate the interaction between root exudates and Nitraria tangutorum rhizosphere bacterial communities. We found that drought and salt stress increased rhizosphere soil pH (9.32 and 20.6%) and electrical conductivity (1.38 and 11 times), respectively, while decreased organic matter (27.48 and 31.38%), total carbon (34.55 and 29.95%), and total phosphorus (20 and 28.57%) content of N. tangutorum rhizosphere soil. Organic acids, growth hormones, and sugars were the main differential metabolites of N. tangutorum under drought and salt stress. Salt stress further changed the N. tangutorum rhizosphere soil bacterial community structure, markedly decreasing the relative abundance of Bacteroidota as r-strategist while increasing that of Alphaproteobacteria as k-strategists. The co-occurrence network analysis showed that drought and salt stress reduced the connectivity and complexity of the rhizosphere bacterial network. Soil physicochemical properties and root exudates in combination with salt stress affect bacterial strategies and interactions. Our study revealed the mechanism of plant–soil-microbial interactions under the influence of root exudates and provided new insights into the responses of bacterial communities to stressful environments.
Introduction
The plant root system is the interface for material exchange between the plant and soil ecosystem. As the life carrier between plants and the soil environment, root exudates play a prominent role in nutrient absorption and cycling (Mommer et al., 2016; Sokol et al., 2019). Root exudates, mainly including sugars, amino acids, organic acids, phenolic acids, fatty acids, sterols, proteins, and growth factors, can be divided into high and low molecular weight (Baetz and Martinoia, 2014; Wu et al., 2014; Canarini et al., 2019). Plants are often subjected to various biotic and abiotic stresses throughout their life cycle and respond by secreting different bioactive compounds through their roots (Badri and Vivanco, 2009). The composition and quantity of plant root exudates increase considerably under abiotic stress, and their organic acid content markedly increases under water stress (Vives-Peris et al., 2018). Furthermore, salt and other abiotic stressors can induce the synthesis and accumulation of plant secondary metabolites such as polyphenols (Zhou et al., 2018). Previous studies have shown that root exudates serve as the medium for co-evolution between plants and microorganisms and can promote the interaction between them by regulating the plant rhizosphere micro-environment to cope with various biotic and abiotic stresses (Pétriacq et al., 2017; Gargallo-Garriga et al., 2018; Guyonnet et al., 2018; Karlowsky et al., 2018; de Vries et al., 2019).
Soil microbial communities are closely associated with plant roots and participate in biogeochemical cycles, thus shaping the structure and function of terrestrial ecosystems (Bardgett and van der Putten, 2014; Fierer, 2017). The interaction between plant root exudates and rhizosphere microorganisms is an important process. For example, plant roots secrete various secondary metabolites affecting rhizosphere microorganism species, quantity, and distribution (Jenkins et al., 2017). Amino acids, vitamins, and carbohydrates in plant root exudates provide a basis for microbial growth and substrate decomposition (Zhalnina et al., 2018). Furthermore, some allelochemicals in root exudates can inhibit the assembly process of rhizosphere microbial communities (Xia et al., 2016). Soil moisture, vegetation type, and climate shape plant rhizosphere microbial communities through changes in plant rhizosphere metabolites (Kong et al., 2018). Plant root exudates and environmental selection drive the change in rhizosphere microbial community structure (Schleuning et al., 2015). Given the ubiquity, diversity, and plasticity of microorganisms, it is important to explore plant–soil-microorganism interactions mediated by root exudates and to have an in-depth understanding of microbial interactions and functions.
In the past decade, the diversity and complexity of microbial communities have made it urgent to understand the construction and characteristics of microbial communities (Duran et al., 2018; Rottjers and Faust, 2018). With the rapid development of high-throughput sequencing technology, microbial community data have become more accurate. The network of microbial interactions provides a multidimensional and more complete view of ecosystems. These networks are closely related to ecosystem functions and play a key role in maintaining biodiversity (Wardle, 2006). Therefore, the application of microbial network analysis to identify alternative community states and ecological niches has become a common tool for studying the structure of microbial communities (Xiao et al., 2017; Kumar et al., 2019). In microbial co-association networks, nodes represent species and edges represent potential interactions between species (Bahram et al., 2014; Ma et al., 2016). There is growing evidence that the properties of ecological networks, such as soil pH (Banerjee et al., 2019), soil water availability (Ma et al., 2020; Hernandez et al., 2021), and soil nutrients (Shi et al., 2020), may represent interactions between coexisting organisms. A microbial community is a well-constructed complex ecological network in which microorganisms collaborate and interact to maximize their ecological functions (Toju et al., 2018; Banerjee et al., 2019).
Given the large number of soil bacteria, the concept of r-and k-strategists can be applied to the study of soil bacterial ecology to understand bacterial interactions (Fierer et al., 2007; Pascault et al., 2013). R-strategists preferentially consume unstable soil nutrients and maximize their intrinsic growth rate when resources are abundant (Kielak et al., 2009). Contrastingly, k-strategists show a slower growth rate and compete for survival under nutrient deficiency (Meyer, 1994). Studies have shown that in the ecological classification of soil bacteria, Betaproteobacteria, Gammaproteobacteria, Deltaproteobacteria, and Bacteroidota are r-strategists, and Alphaproteobacteria and Acidobacteriota are k-strategists (Klappenbach et al., 2000; Lee et al., 2008; Senechkin et al., 2010; Gu et al., 2018). Microbial communities often respond to environmental stress considering their growth, reproduction, competition, and adaptation strategies. However, there is still a limited understanding of the effects of root exudates on the adaptation strategies of plant rhizosphere bacterial communities under environmental stress.
Soil microorganisms are essential for maintaining the structure and function of terrestrial ecosystems. Many environmental factors (soil pH, moisture, and nutrient availability) and plant root exudates directly or indirectly influence soil microbial communities (Bardgett and van der Putten, 2014; Delgado-Baquerizo et al., 2016; Sayer et al., 2017; Su et al., 2020). However, the mechanisms by which plant root exudates influence rhizosphere microbial communities of the dominant plant species in the desert steppe ecosystems in China are not fully understood. Nitraria tangutorum is a typical desert shrub with strong resistance to salinity and drought, and widely distributed in arid areas of Northern China (Luo et al., 2008; Ni et al., 2015; Yan et al., 2018). It is of great significance for sand fixation, soil improvement and has very important ecological functions (Liu et al., 2016). Its fruit is also an important nutritional health food resource with high potential economic value (Kang et al., 2015). In this study, we analyzed the differences in root exudates and microbial communities of N. tangutorum under short-term drought and salt stress through controlled experiments. We proposed that there are differences in the root exudates of N. tangutorum under short-term drought and salt stress, and that the differences in root exudates affect bacterial survival strategies and interactions.
Materials and methods
Plant materials and treatments
In this study, N. tangutorum was obtained from the Lianhuachi Lake in Dingbian County (37°36′20′N, 107°20′25′ E), Shaanxi Province, China, in May 2021. Lianhuachi Lake is 1,310 m above sea level and covers 0.61 km2. The average annual precipitation is less than 200 mm. Nitraria tangutorum with the same growth were transplanted into pots (Φ = 33 cm, height = 20 cm) with sand and vermiculite at a ratio of 4: 1 and placed in a greenhouse. The growth conditions in greenhouse were controlled to maintain a temperature of 26/22°C (day/night), a photoperiod of 16/8 h (light/dark). The plants were watered with 1/2 Hoagland solution and subjected to drought and salt stress after 2 months. In “CK” group, the SWC was maintained at 50% of FWC by irrigated with 1/2 Hoagland solution. In “Drought” group, the SWC was maintained at 30% of FWC by irrigated with 1/2 Hoagland solution. All these values were subsequently maintained constantly by irrigated with corresponding solutions every 5 days for 30 days. In “Salt” group, the SWC was maintained at 50% of FWC by 1/2 Hoagland solution containing 200 mM NaCl, and the treatment solutions were renewed every 5 days to keep constant NaCl concentration (Kang et al., 2016; Pan et al., 2016; Guo et al., 2020).
Sample collections
After 30 days of treatment, N. tangutorum were removed from the pots, and the rhizosphere soil was collected; 27 soil samples were collected, including nine CK, nine drought, and nine salt samples. For each treatment, the soil probe was washed with 75% ethanol before collection and gloves were changed before collection. The soil cores were then placed in a sterile centrifuge tube and taken to the laboratory in an ice box (Pan et al., 2021). After sieving and removing surface vegetation and litter, the soil samples were divided into three parts: one for high-throughput sequencing, one for analysis of physicochemical properties, and the other was frozen for metabolite extraction. Soil samples were air-dried for the determination of soil physicochemical properties, and fresh soil samples were used for the determination of soil microorganisms and root exudates. We took 100 g of fresh soil for extraction and collection of root exudate. Firstly, 500 ml deionized water was added, and then centrifuged for 5 min (20°C,8,000 r/min) after shock extraction for 3 h, and then the supernatant was extracted for filtration. After that, the water was spin-dried at 35°C with the vacuum rotary evaporator, poured on the tin foil until the methanol completely volatilized, and washed repeatedly 2–3 times; and finally stored at −80°C to be tested.
Characterization of physicochemical properties of rhizosphere soil samples
Soil water content (SWC) was measured using the weighing method (Li et al., 2020), soil pH was determined using a pH meter (Mettler S220; Mettler Toledo Solutions, Greifensee, Switzerland), and soil electrical conductivity (EC) was measured using a specific conductivity meter (Leici DDS-307A, Shanghai Leici Instruments, Shanghai, China) with the soil-water ratio of 1:5 (Wang et al., 2019). After the collected soil samples were air-dried, the soil total carbon (TC), total nitrogen (TN), and total phosphorus (TP) were determined using atomic absorption spectrometry with an atomic absorption spectrophotometer (iCE 3,500, Thermo Fisher Scientific, Waltham, MA, United States; Bettinelli et al., 2000; Postma et al., 2016). Soil total organic carbon (TOC) was determined by dichromate oxidation and ammonium ferrous sulfate titration (Gul et al., 2015).
Determination of root metabolites
Nucleic acids were extracted from each sample at the end of the treatment. Plant roots were carefully rinsed with sterile deionized water and soaked in 1,000 ml of sterile deionized water for 8 h, according to Luo et al. (2014). The extract was stored in liquid nitrogen and sent to Novogene Bioinformatics Technology Co. Ltd., Tianjin, China, for the determination of plant root exudates using liquid chromatography-mass spectrometry (LC–MS; Ultimate 3000 LC, Q Exactive; Thermo Fisher Scientific) on a Hyper Gold C18 column [100 mm × 2.1 mm, at 1.9 μm, (Thermo Fisher Scientific)]. Metabolites were detected in positive and negative ion modes. Before sample detection, equal amounts of samples were extracted from 27 root exudates and mixed into a quality control (QC) sample. The total ion flow chromatograms of the QC samples were overlapped to verify the reproducibility of the retention time of the same substance. Compound Discoverer Software (Thermo Fisher Scientific) was used to extract and preprocess the LC–MS detection data, including retention time, molecular weight, sample name, and peak intensity. Subsequently, the ionic characteristics were combined with three predicted components databases (Predicted Compositions, mzCloud Search, and ChemSpider Search) to determine the compound information. Finally, the peak value was converted to the peak value per unit mass using the dry weight of the secretions.
Soil DNA extraction and PCR amplification
The rhizosphere soil bacterial community was examined using Illumina MiSeq sequencing kits (Illumina, San Diego, CA, United States). Total genomic DNA of N. tangutorum rhizosphere soil was extracted using the cetyltrimethylammonium bromide/sodium dodecyl sulfate method. The DNA concentration and purity were examined on a 1% agarose gel. Considering the concentration, DNA was diluted to 1 ng/μl using sterile water. All PCR reactions were carried out using 15 μl of Phusion High-Fidelity PCR Master Mix (New England Biolabs, Ipswich, MA, United States), 0.2 μM of forward and reverse primers, and approximately 10 ng template DNA. Illumina MiSeq sequencing libraries (Illumina) for bacteria were prepared via PCR amplification of the V3–V4 hypervariable regions of the bacterial 16S rRNA gene using the primers 338F (5′-ACTCCTACGGGAGGCAGCAG-3′) and 806R (5′-GGACTACHVGGGTWTCTAAT-3′) using a GeneAmp 9700 PCR thermocycler (Applied Biosystems, Waltham, MA, United States; Caporaso et al., 2012; Langille et al., 2013). The thermal cycling conditions were as follows: an initial denaturation at 98°C for 1 min, followed by 30 cycles of denaturation at 98°C for 10 s, annealing at 50°C for 30 s, and elongation at 72°C for 30 s, and finally extension at 72°C for 5 min (Haas et al., 2011). The purified amplicons were pooled in equimolar concentrations and paired-end sequenced on an Illumina MiSeq platform (Illumina) according to the standard protocols of Novogene Bioinformatics Technology Co., Ltd. The 16S rRNA gene sequences obtained in this study have been submitted to the NCBI Sequence Read Archive (SRA) database with the serial number PRJNA855333.
Sequence processing and statistical analysis
PE libraries were constructed using a NEXTflex Rapid DNA-SEQ Kit (Bioscience, South San Francisco, CA, United States), and an Illumina MiSeq PE300 platform (Illumina) was used for sequencing. Trimmomatic software (Illumina) was used for quality control of Illumina MiSeq sequencing original sequences. FLASH 1.2.11;1 software (Illumina) was used for stitching (Magoč and Salzberg, 2011). UPARSE 7.1 software was used for amplicon sequence variants (ASVs) clustering analysis of the sequences (similarity 97%), and UCHIME software was used to remove chimeras (Caporaso et al., 2012). Each sequence was annotated for species classification by the ribosomal database project classifier and compared with the Silva database (SSU128) at a confidence threshold of 0.7 (Quast et al., 2013). All calculations were performed by sub-sampling each sample to an equivalent sequence of 49,556 (Ye et al., 2017).
The QIIME program (2.0) was used to calculate the alpha diversity indices (Shannon and Chao1; Kuczynski et al., 2012). The linkET2 package in R software (Version 4.1.0) was used to calculate and visualize the correlation between soil physicochemical properties and soil bacterial α-diversity. Canonical correlation analysis (CCA) was applied to distinguish the soil physicochemical properties, differential metabolites community, and bacterial phyla under drought and salt stress (Ramette, 2010). A non-metric multidimensional scale (NMDS) based on the Bray–Curtis distance matrix and ANOSIM test with 9,999 permutations were used to illustrate beta diversity. Differences in community composition between sample groups were analyzed using the vegan package (Pan et al., 2021). The ggalluvial package in R software was used to describe changes in bacteria (top 10 phyla and top 20 genera with the highest abundance). A co-occurrence network was constructed for each treated sample, and coefficients of all possible Spearman correlations among ASVs in all samples were calculated using the psych package (Berry and Widder, 2014; R Core Team, 2020). Cytoscape (3.7.1) was used for network visualization, and the number of ASVs at the phylum level was statistically analyzed. The bacterial phyla or classes for r-and k-strategists were visualized using the ggplot2 package. The psych package was used to calculate the correlation between differential metabolites and soil properties, which was then visualized using the ggplot2 package. We used the fold change value and value of p to screen for significantly upregulated or downregulated differential metabolites and visualized them using the ggplot2 package.
SPSS version 25.0 (SPSS Inc., Chicago, IL, United States) was used for one-way ANOVA of soil physicochemical data, and Duncan’s multiple range test was used to identify the significant differences between means at a 5% significance level. All data are presented as the mean ± SE (n = 9; Pan et al., 2021).
Results
Relationship between rhizosphere soil bacterial community diversity and physicochemical properties under drought and salt stress
The physicochemical properties of N. tangutorum rhizosphere soil were different under drought and salt stress. Salt and drought stress markedly increased rhizosphere soil pH and EC of N. tangutorum. Under salt stress, pH and EC were 20.55% and 5.2-fold higher than those of CK, respectively. Conversely, salt and drought stress decreased rhizosphere SOM, TC, and TP contents. Rhizosphere SOM, TC, and TP contents decreased by 38.03, 52.85, and 24.39%, respectively, under drought stress, and by 45.62, 42.73, and 39.48%, respectively, under salt stress, compared with that in CK (p < 0.05; Supplementary Table 1).
Based on a 97% sequence similarity threshold, 10,535 ASVs were obtained for each sample after normalization. The coverage index of the bacterial ASVs was greater than 0.999, indicating that the sequencing depth was reasonable. Compared with CK, drought decreased the Shannon index in the rhizosphere bacterial community of N. tangutorum, while salt stress increased the number of ASVs and the Chao1 index but not significantly (Supplementary Table 2). The rhizosphere soil bacterial community diversity of N. tangutorum was significantly correlated with soil SWC, TN, and SOM, and SWC and TN were positively correlated. The correlation between pH and EC was stronger under drought stress, whereas that between SWC and EC was stronger under salt stress. The ASVs and Shannon and Chao1 indices of the rhizosphere soil bacterial community of N. tangutorum were correlated with soil pH and EC under salt stress (p < 0.05; Figure 1). CCA analysis showed that Firmicutes and Bacteroidetes were correlated with soil SOM under drought stress; while Acidobacteriota, Chloroflexi, Proteobacteria, Actinobacteriota, and Gemmatimonadota were correlated with soil SWC, TS, and Na+. The analysis explained 27.95 and 14.11% of the variables, respectively (Supplementary Figure 1).
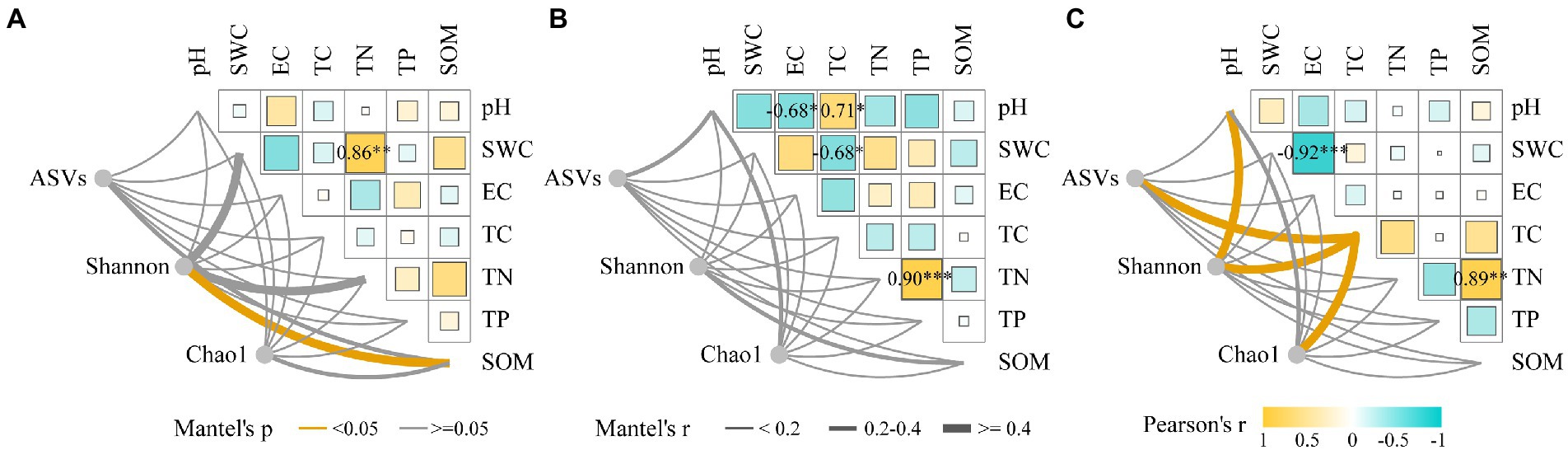
Figure 1. Correlation between environmental factors and alpha diversity indices of Nitraria tangutorum rhizosphere soil under different stress (A: CK; B: Drought; and C: Salt). Pairwise comparisons of environmental factors are shown with a color gradient denoting Pearson’s correlation coefficient. The number of ASVs, Shannon and Chao1 indices were correlated with each environmental factor by Mantel test.
Rhizosphere soil bacterial community composition and r-and k-strategists under drought and salt stress
Non-metric multidimensional scale analysis revealed that the rhizosphere soil bacterial community was clustered under drought stress but dispersed under salt stress. The ASVs of N. tangutorum rhizosphere soil bacteria were classified into 50 phyla and 999 genera. The top 10 phyla in relative abundance under drought stress were Firmicutes, Bacteroidota, Proteobacteria, Actinobacteriota, Cyanobacteria, Acidobacteriota, Gemmatimonadota, Fusobacteriota, Chloroflexi, and Myxococcota. Compared with that of CK, the abundance of Proteobacteria (25.44–19.55%) and Actinobacteriota (17.02–11.19%) decreased, whereas that of Firmicutes (24.45–35.44%) and Bacteroidota (16.56–28.78%) increased under drought stress. When considering the relative abundance under salt stress, the top 10 phyla were Proteobacteria, Actinobacteriota, Firmicutes, Bacteroidota, Cyanobacteria, Acidobacteriota, Gemmatimonadota, Chloroflexi, Myxococcota, and Fusobacterium. Under salt stress, the abundance of Proteobacteria (25.44–31.70%) and Actinobacteriota (17.02–32.36%) increased, while Firmicutes (24.45–7.40%) and Bacteroidota decreased (16.56–8.01%) compared with that in CK. The relative abundance of Cyanobacteria decreased under drought and salt stress conditions. Thus, the relative abundance of Bacteroides, Faecalibacterium, and Escherichia-Shigella decreased under drought and salt stress (Figure 2).
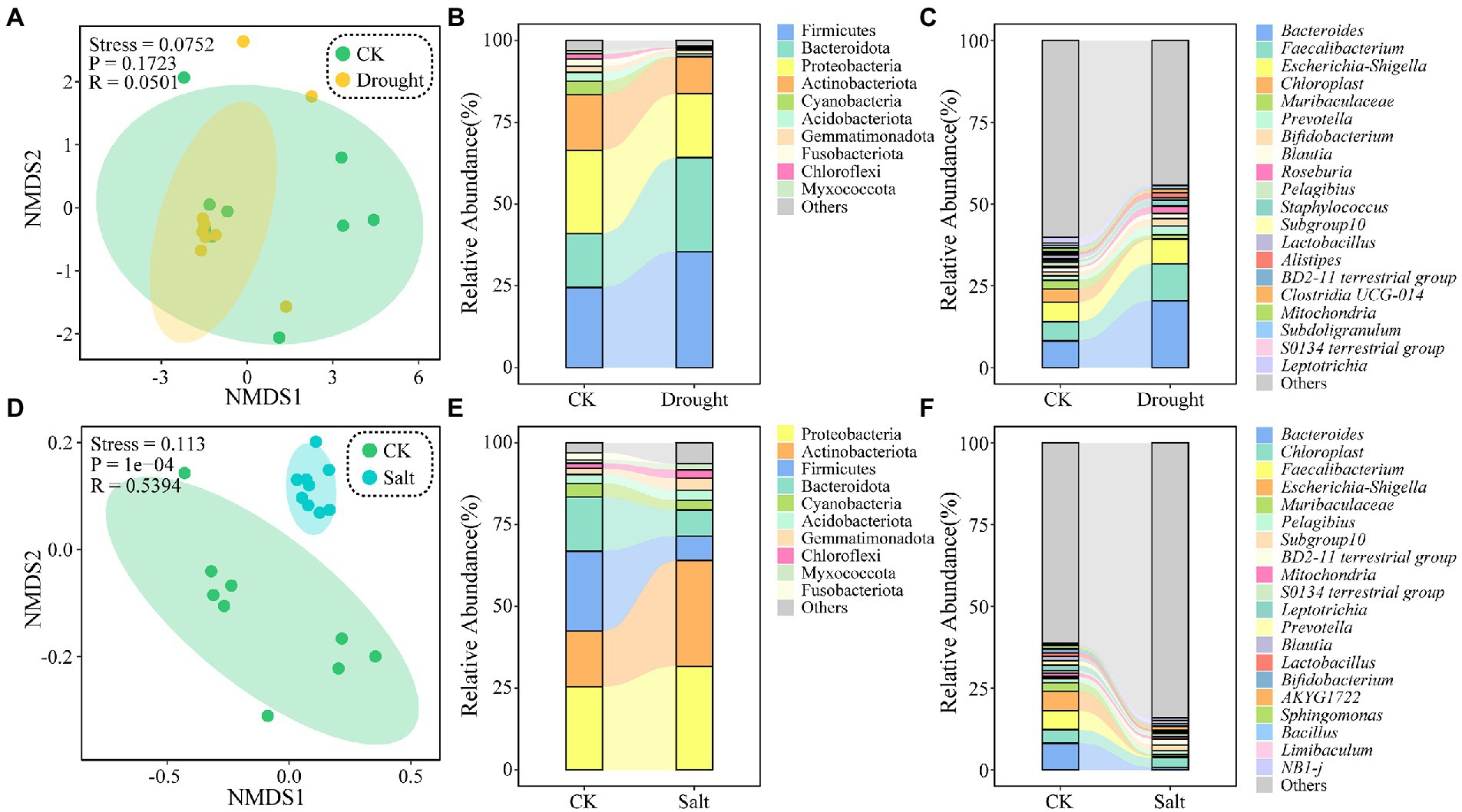
Figure 2. Non-metric multidimensional scaling (NMDS) ordination based on Bray Curtis similarities of bacterial communities under drought (A) and salt (D) stress. Each point represents a replicate, different colors indicate different sample plots. Relative abundance of major taxa (top 10) in the bacterial communities under drought (B) and salt (E) stress at the phylum level. And relative abundance of major taxa (top 20) in the bacterial communities under drought (C) and salt (F) stress at the genera level.
There were two r-strategist bacteria (Gammaproteobacteria and Bacteroidota) and two k-strategists (Alphaproteobacteria and Acidobacteriota) in our study. The relative abundance of Gammaproteobacteria (15.65–16.03%) and Bacteroidota (16.56–28.78%) as r-strategists increased, whereas that of Alphaproteobacteria (9.78–3.52%) and Acidobacteriota (2.70–0.76%) as k-strategists decreased under drought stress compared with that of CK. The relative abundance of Gammaproteobacteria (15.65–10.84%) and Bacteroidota (16.56–8.01%) as r-strategists decreased, whereas Alphaproteobacteria (9.78–20.86%) and Acidobacteriota (2.70–3.06%) as k-strategists increased under salt stress (Figures 3A,B). Gammaproteobacteria were significantly negatively correlated with TC under drought stress; furthermore, Gammaproteobacteria, Acidobacteriota, and Bacteroidota were significantly negatively correlated with TC under salt stress. Gammaproteobacteria and Acidobacteriota were significantly negatively correlated with TN, and Acidobacteriota was significantly negatively correlated with SOM under salt stress (p < 0.05; Figure 3).
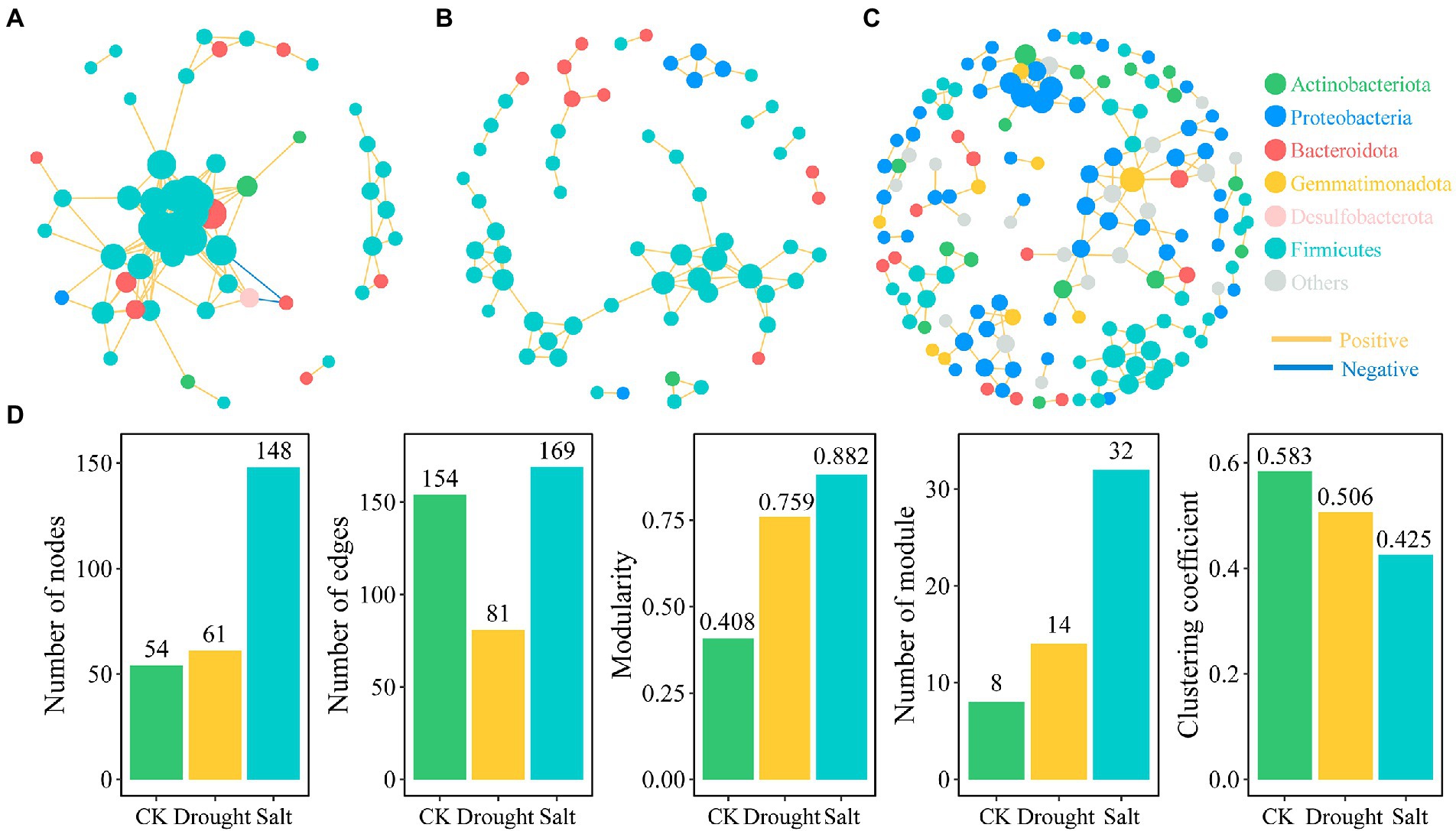
Figure 3. The relative abundance of bacteria for r-and k-strategists (A,B) and the correlation with rhizosphere soil properties of N. tangutorum under different stress (C,D). Drought: A and C; Salt: B and D. “*” represents p < 0.05, “**” represents p < 0.01, and “***” represents p < 0.001.
Rhizosphere soil bacterial network under drought and salt stress
Based on correlation analysis (p < 0.01), we constructed bacterial co-occurrence networks of N. tangutorum under CK, drought, and salt stress. The co-occurrence network showed that drought and salt stress decreased ASV aggregation in the rhizosphere soil bacterial community of N. tangutorum. Under drought stress, the connectivity and complexity of the rhizosphere soil bacterial community network of N. tangutorum and the number of edges (47.4%) decreased compared with those of CK. In contrast, salt stress increased the connectivity and complexity of the bacterial community network, and the number of nodes and edges increased by 174.07 and 9.74%, respectively, compared with those of CK (Figure 4). There were 54 nodes and 154 edges, of which 152 were positive and 2 were negative, for CK. Under drought and salt stress, the number of nodes were 61 and 148, number of edges were 81 and 169, respectively, and all were positive. The number of modules was highest (32) under salt stress, followed by those under drought stress (14) and CK (8). Topological features such as graph density, clustering coefficient, betweenness centralization, and degree centralization were the highest in CK, followed by drought stress, and the lowest in salt stress (Supplementary Table 3).
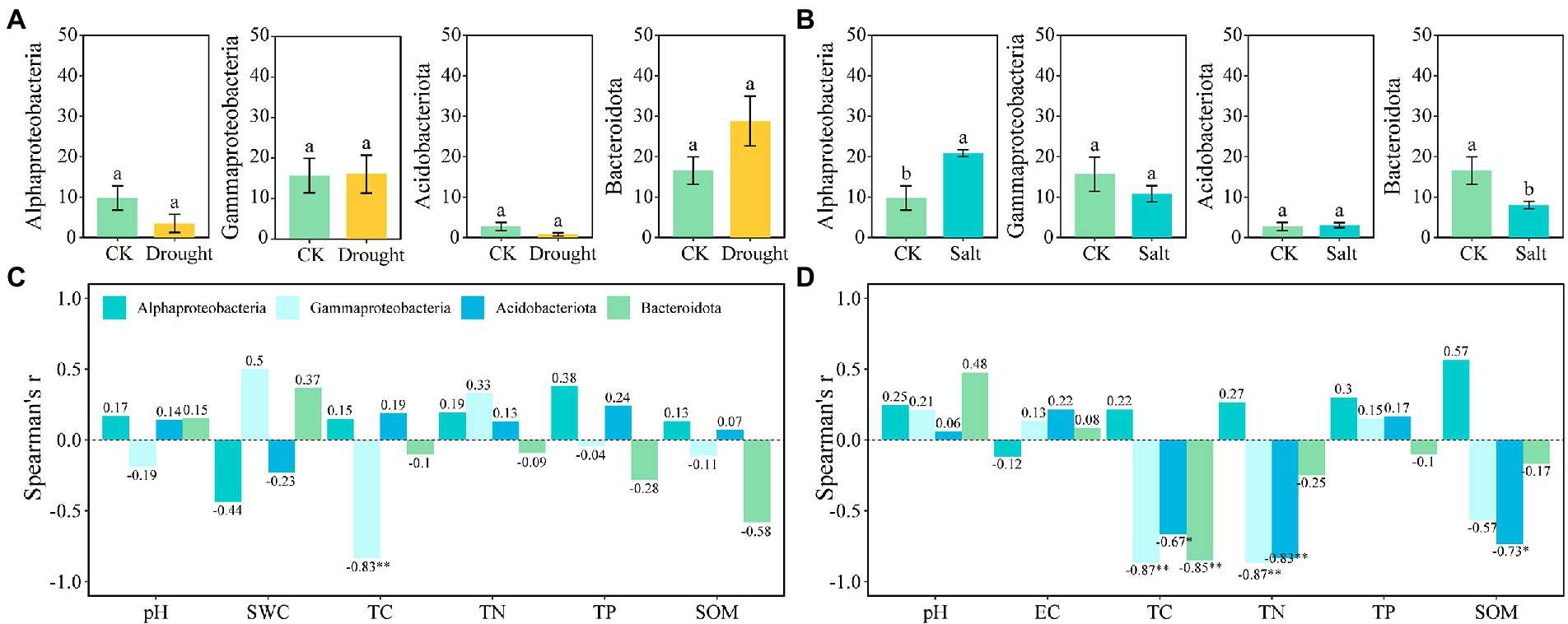
Figure 4. Bacterial networks based on correlation analysis of Nitraria tangutorum rhizosphere soil under different stress (A: CK; B: Drought; and C: Salt) and the correlation between network properties and soil properties (D). A connection stands for a strong (Spearman’s|r|> 0.9) and significant (p < 0.01 after FDR correction). Colours of nodes indicate different major phyla while node size is proportional to node degree calculated from each ASV abundance correlation. The yellow lines indicate positive interactions whereas blue lines indicate negative interactions. (D) The network topological features of N.tangutorum under drought and salt stress.
Correlation among root exudates, r-and k-strategists bacteria, and network properties under drought and salt stress
The characteristic peaks of the chromatograms of N. tangutorum root exudates under different stress conditions were markedly different. By preprocessing the raw data and comparing the database, 152 and 60 compounds in positive and negative ion modes, respectively, were identified. Root exudates contained various organic acids, amides, esters, sugars, olefins, phenols, growth factors, aromatics, ketones, amino acids and their derivatives, and heterocyclic compounds. Most of the exudates were secondary metabolites. Significant differences in metabolites of N. tangutorum root systems under drought and salt stress, with most metabolites correlated with environmental changes (Supplementary Figures 2, 3). Under drought stress, there were 40 differential metabolites in the positive ion mode, of which 14 were upregulated and 26 were downregulated. In addition, there were 13 differential metabolites in the negative ion mode, of which three were upregulated and 10 were downregulated. Under salt stress, there were 56 differential metabolites in the positive ion mode, of which 11 were upregulated and 45 were downregulated. However, in the negative ion mode, there were 13 differential metabolites, of which three were upregulated and 10 were downregulated (Figure 5).
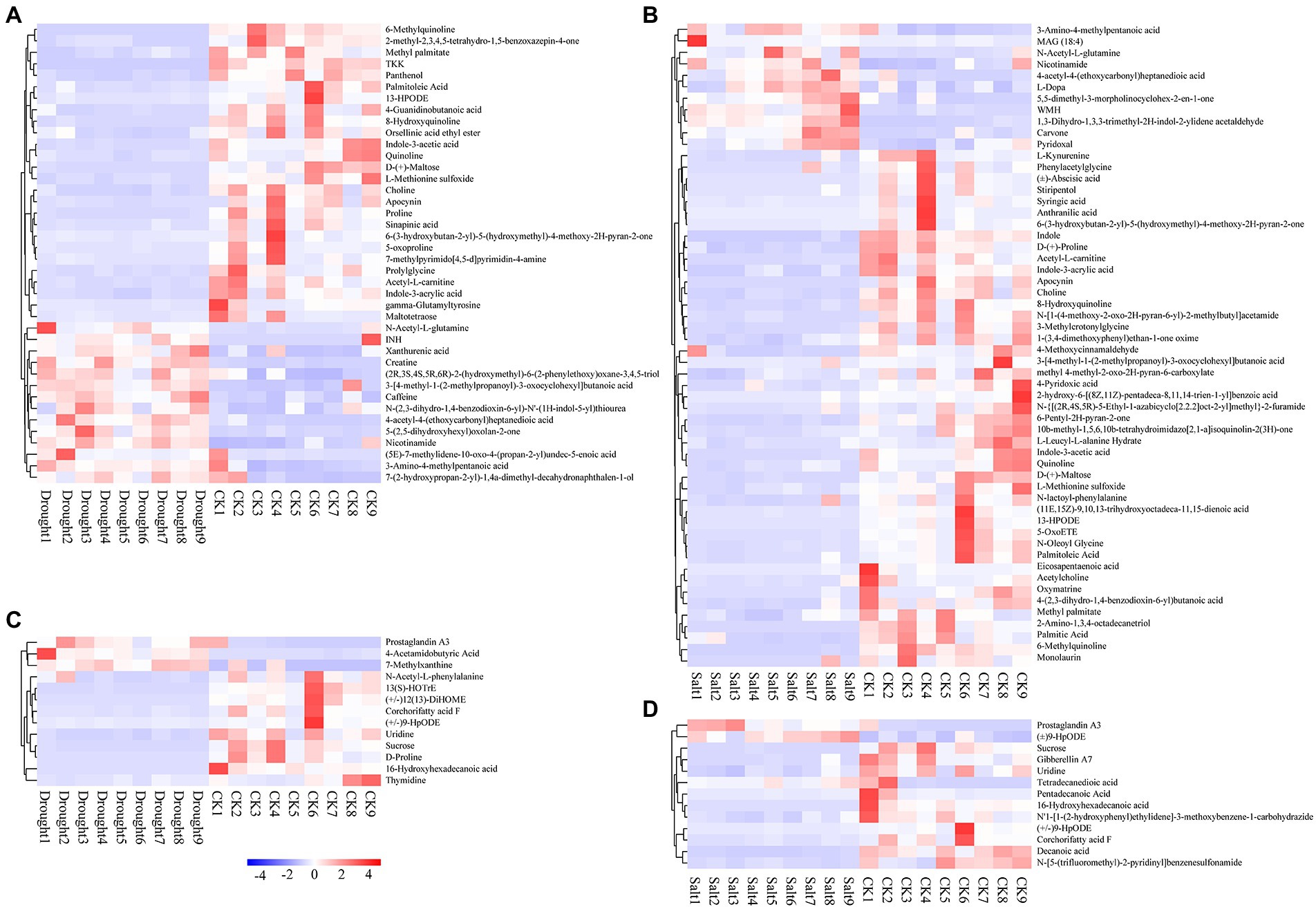
Figure 5. Heat map of clustering of differential metabolites of Nitraria tangutorum under drought (A: pos and C: neg) and salt (B: pos and D: neg) stress.
Under drought stress, indole-3-acrylic acid and panthenol were negatively correlated with pH; 2-methyl-2,3,4,5-tetrahydro-1,5-benzoxazepin-4-one and thymidine were negatively correlated with SWC; caffeine, 6-(3-hydroxybutan-2-yl)-5-(hydroxymethyl)-4-methoxy-2H-pyran-2-one, and 7-methylpyrimido [4,5-d]pyrimidin-4-amine were positively correlated with TN; 3-[4-methyl-1-(2-methylpropanoyl)-3-oxocyclohexyl] butanoic acid, TKK, INH, sucrose, and 4-acetamidobutyric acid were positively correlated with TP; caffeine, xanthurenic acid, and maltotetraose were negatively correlated with SOM; and 3-amino-4-methylpentanoic acid and 16-hydroxyhexadecanoic acid were positively correlated with SOM. Under salt stress, (+/−)9-HpODE was negatively correlated with pH; (+/−)9-HpODE and corchorifatty acid F were negatively correlated with EC; palmitoleic acid, 5-OxoETE, and quinoline were positively correlated with TC; 2-amino-1,3,4-octadecanetriol and prostaglandin A3 were negatively correlated with TC; methyl 4-methyl-2-oxo-2H-pyran-6-carboxylate, D-(+)-maltose, quinoline, and L-dopa were positively correlated with TN; prostaglandin A3 was negatively correlated with TN; pyridoxal, carvone, methyl 4-methyl-2-oxo-2H-pyran-6-carboxylate, acetyl-L-carnitine, anthranilic acid, L-dopa, 5,5-dimethyl-3-morpholinocyclohex-2-en-1-one, 3-[4-methyl-1-(2-methylpropanoyl)-3-oxocyclohexyl] butanoic acid, 4-acetyl-4-(ethoxycarbonyl) heptanedioic acid, and phenylacetylglycine were positively correlated with SOM (Figure 6).
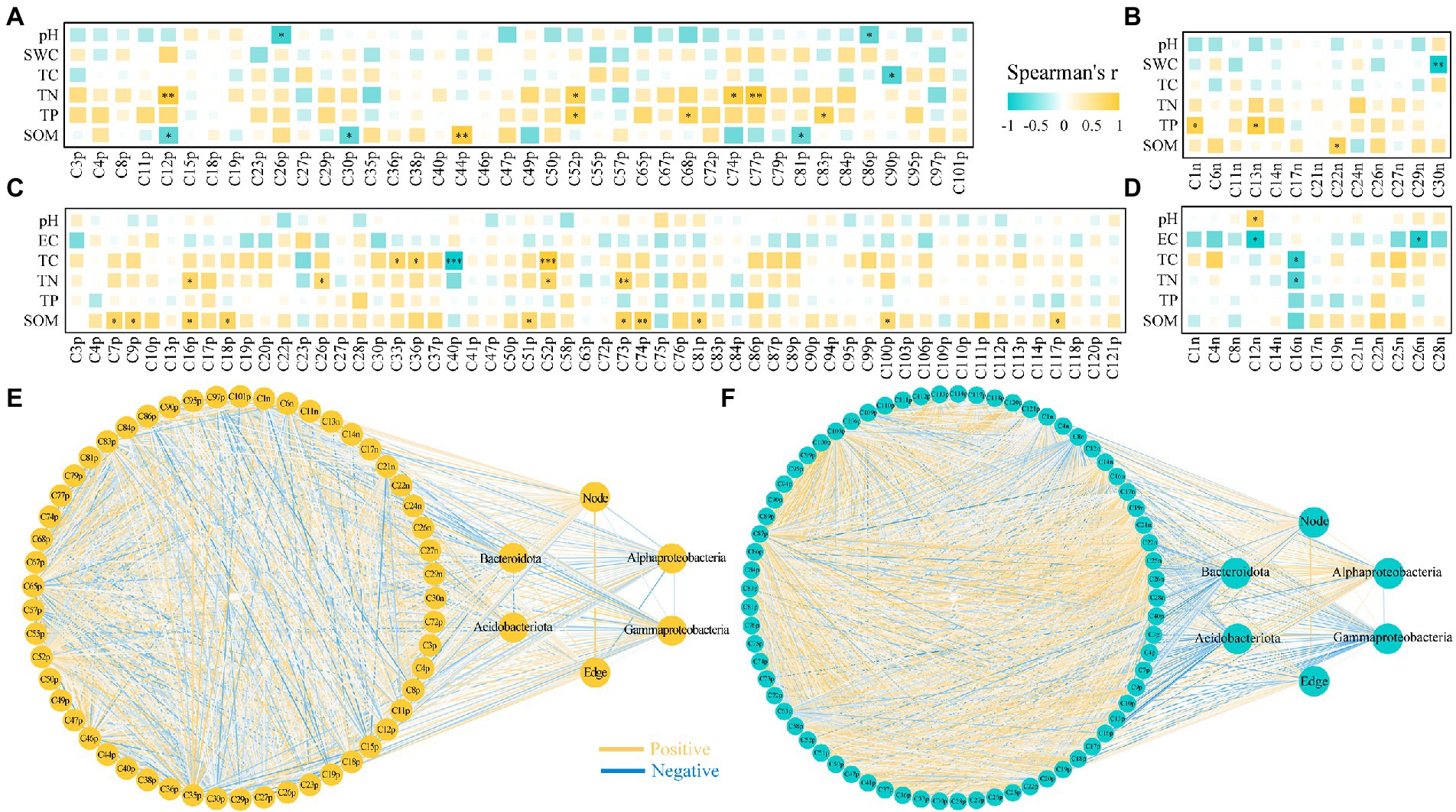
Figure 6. Correlation between root exudates and rhizosphere soil properties, bacteria for r-and k-strategists and network properties of Nitraria tangutorum under drought and salt stress. (A) Spearman correlation coefficients between differential metabolites of positive ion mode and rhizosphere soil physicochemical properties under drought stress. (B) Spearman correlation coefficients between differential metabolites of negative ion mode and rhizosphere soil physicochemical properties under drought stress. (C) Spearman correlation coefficients between differential metabolites of positive ion mode and rhizosphere soil physicochemical properties under salt stress. (D) Spearman correlation coefficients between differential metabolites of negative ion mode and rhizosphere soil physicochemical properties under salt stress. Correlation between differential metabolites of positive and negative ion mode, bacteria for r-and k-strategists and network properties of N. tangutorum under drought (E) and salt (F) stress. The significance of factors and the correlations between factors is marked at the figure, where “*” represents p < 0.05, “**” represents p < 0.01, and “***” represents p < 0.001; yellow lines indicate positive correlations whereas blue lines indicate negative correlations. Name of root exudates under drought stress: C50p: 6-Methylquinoline; C68p: TKK; C3p: Proline; C38p: Creatine; C67p: 8-Hydroxyquinoline; C86p: Panthenol; C19p: Indole-3-acetic acid; C95p: D-(+)-Maltose; C29p: 5-(2,5-dihydroxyhexyl)oxolan-2-one; C27p: Prolylglycine; C4p: Choline; C90p: 2-methyl-2,3,4,5-tetrahydro-1,5-benzoxazepin-4-one; C18p: Acetyl-L-carnitine; C15p: L-Methionine sulfoxide; C26p: Indole-3-acrylic acid; C35p: 4-Guanidinobutanoic acid; C79p: 4-acetyl-4-(ethoxycarbonyl) heptanedioic acid; C55p: Methyl palmitate; C40p: Palmitoleic Acid; C36p: 13-HPODE; C101p: Sinapinic acid; C97p: 5-oxoproline; C77p: 7-methylpyrimido [4,5-d]pyrimidin-4-amine; C47p: Quinoline; C11p: Nicotinamide; C52p: 3-[4-methyl-1-(2-methylpropanoyl)-3-oxocyclohexyl] butanoic acid; C12p: Caffeine; C57p: N-Acetyl-L-glutamine; C65p: gamma-Glutamyltyrosine; C8p: Apocynin; C84p: Orsellinic acid ethyl ester; C83p: INH; C30p: Xanthurenic acid; C72p: (2R,3S,4S,5R,6R)-2-(hydroxymethyl)-6-(2-phenylethoxy)oxane-3,4,5-triol; C46p: (5E)-7-methylidene-10-oxo-4-(propan-2-yl)undec-5-enoic acid; C44p: 3-Amino-4-methylpentanoic acid; C81p: Maltotetraose; C23p: 7-(2-hydroxypropan-2-yl)-1,4a-dimethyl-decahydronaphthalen-1-ol; C49p: N-(2,3-dihydro-1,4-benzodioxin-6-yl)-N′-(1H-indol-5-yl)thiourea; C74p: 6-(3-hydroxybutan-2-yl)-5-(hydroxymethyl)-4-methoxy-2H-pyran-2-one; C1n: Sucrose; C27n: 13(S)-HOTrE; C22n: 16-Hydroxyhexadecanoic acid; C21n: (+/−)12 (13)-DiHOME; C13n: 4-Acetamidobutyric Acid; C14n: D-Proline; C26n: Uridine; C29n: Corchorifatty acid F; C24n: Prostaglandin A3; C17n: (+/−)9-HpODE; C11n: 7-Methylxanthine; C6n: N-Acetyl-L-phenylalanine; and C30n: Thymidine. Name of root exudates under salt stress: C99p: Apocynin; C113p: (±)-Abscisic acid; C40p: 2-Amino-1,3,4-octadecanetriol; C106p: (11E,15Z)-9,10,13-trihydroxyoctadeca-11,15-dienoic acid; C13p: 8-Hydroxyquinoline; C19p: Indole-3-acetic acid; C36p: 5-OxoETE; C90p: 3-Methylcrotonylglycine; C18p: Acetyl-L-carnitine; C26p: D-(+)-Maltose; C120p: Indole; C27p: 6-Methylquinoline; C47p: WMH; C37p: 13-HPODE; C86p: N-Oleoyl Glycine; C3p: Choline; C83p: Oxymatrine; C50p: 6-Pentyl-2H-pyran-2-one; C109p: N-[1-(4-methoxy-2-oxo-2H-pyran-6-yl)-2-methylbutyl]acetamide; C84p: L-Kynurenine; C75p: Eicosapentaenoic acid; C33p: Palmitoleic Acid; C4p: D-(+)-Proline; C114p: 2-hydroxy-6-[(8Z,11Z)-pentadeca-8,11,14-trien-1-yl] benzoic acid; C94p: 4-(2,3-dihydro-1,4-benzodioxin-6-yl) butanoic acid; C20p: Syringic acid; C52p: Quinoline; C23p: Palmitic Acid; C9p: Carvone; C87p: Stiripentol; C89p: 4-Pyridoxic acid; C72p: Monolaurin; C22p: 1,3-Dihydro-1,3,3-trimethyl-2H-indol-2-ylidene acetaldehyde; C110p: 1-(3,4-dimethoxyphenyl)ethan-1-one oxime; C74p: 5,5-dimethyl-3-morpholinocyclohex-2-en-1-one; C17p: L-Methionine sulfoxide; C100p: 4-acetyl-4-(ethoxycarbonyl) heptanedioic acid; C28p: Acetylcholine; C103p: N-lactoyl-phenylalanine; C30p: Indole-3-acrylic acid; C118p: 3-Amino-4-methylpentanoic acid; C51p: Anthranilic acid; C63p: 4-Methoxycinnamaldehyde; C81p: 3-[4-methyl-1-(2-methylpropanoyl)-3-oxocyclohexyl] butanoic acid; C58p: L-Leucyl-L-alanine Hydrate; C117p: Phenylacetylglycine; C76p: N-Acetyl-L-glutamine; C16p: methyl 4-methyl-2-oxo-2H-pyran-6-carboxylate; C112p: 10b-methyl-1,5,6,10b-tetrahydroimidazo [2,1-a]isoquinolin-2(3H)-one; C111p: N-{[(2R,4S,5R)-5-Ethyl-1-azabicyclo [2.2.2] oct-2-yl]methyl}-2-furamide; C10p: Nicotinamide; C95p: 6-(3-hydroxybutan-2-yl)-5-(hydroxymethyl)-4-methoxy-2H-pyran-2-one; C73p: L-Dopa; C41p: Methyl palmitate; C7p: Pyridoxal; C121p: MAG (18: 4); C1n: Sucrose; C4n: Uridine; C8n: N-[5-(trifluoromethyl)-2-pyridinyl]benzenesulfonamide; C12n: (+/−)9-HpODE; C14n: Decanoic acid; C16n: Prostaglandin A3; C17n: N′1-[1-(2-hydroxyphenyl)ethylidene]-3-methoxybenzene-1-carbohydrazide; C19n: 16-Hydroxyhexadecanoic acid; C21n: Tetradecanedioic acid; C22n: (±)9-HpODE; C25n: Pentadecanoic Acid; C26n: Corchorifatty acid F; and C28n: Gibberellin A7.
Discussion
Drought and salt stress caused significant differences in rhizosphere soil physicochemical properties and root exudates of Nitraria tangutorum
Soil water and salt are important factors affecting soil physicochemical properties and plant growth (Flowers et al., 2010). Our study focused on the rhizosphere soil physicochemical characteristics and N. tangutorum bacterial community assembly strategies under short-term drought and salt stress conditions in a desert steppe. The results showed that under short-term drought stress, rhizosphere soil pH increased significantly with a decrease in SWC, while EC and pH increased significantly under salt stress. Li et al. (2018) and Pan et al. (2021) concluded that gradient changes in SWC and pH affect soil physicochemical properties, particularly the accumulation of TC content. Although the effect of short-term stress on TOC content was not significant, our study found that drought and salt stress reduced the TOC content of the rhizosphere soil of N. tangutorum. Dong et al. (2019) also pointed out that soil TOC is mainly controlled by soil salt concentration, further supporting our conclusions.
Plant roots respond to abiotic stress by secreting exudates, including amino acids, carbohydrate peptides, and phenolic compounds (Mercado-Blanco and Bakker, 2007). Under drought stress, 4-acetyl-4-(ethoxycarbonyl) heptanedioic, N-acetyl-L-glutamine, xanthurenic, and 4-acetamidobutyric acids accumulated in the root exudates of N. tangutorum. Elevated phenolic acid content may be a strategy to cope with prolonged plant growth and stress (Henry et al., 2007). As an important metabolite of plant roots, phenolic acids play an important role in regulating their biological functions (Venugopal et al., 2009). We also found that growth factors such as creatine and nicotinamide accumulate during drought stress. These substances are secondary plant metabolites that play a crucial role in plant defense and adaptation to environmental stresses (Fujii et al., 2012). In our study, the D-proline and sucrose contents decreased significantly after 30 days of drought, differing from previous studies (Gong et al., 2005). Plants improve root osmoregulation through changes in sucrose and polyol metabolism under stress (Ruan, 2014). The decrease may be caused by the longer treatment time in our study than in previous studies. However, after 30 days of salt stress, D-proline content in N. tangutorum root exudates increased substantially, further proving that the difference in the amount and type of plant root exudates was due to different response strategies under different stressful environments (Per et al., 2017).
In our study, rhizosphere soil pH and EC were mostly negatively correlated with N. tangutorum root exudates under drought and salt stress, indicating that the unbalanced absorption and utilization of soil cations and anions by plants under stress resulted in the accumulation of organic and phenolic acids in the root exudates, which in turn had a strong correlation with rhizosphere soil pH (Gregory, 2007). In addition, the root exudates of plants positively correlated with soil TC, TN, TP, and SOM, indicating that root exudates affected plant nutrient uptake, especially in stressful environments. Root exudates have been shown to indirectly affect soil accumulation by secreting carbon-containing organic compounds and low molecular organic acids, which affect the microbial population and enzyme activity related to soil nutrients (Narula et al., 2008). In our study, 3-[4-methyl-1-(2-methylpropanoyl)-3-oxocyclohexyl] butanoic acid, quinoline, L-dopa, and 7-methylpyrimido [4,5-d] pyrimidin-4-amine were strongly correlated with rhizosphere soil TC, TN, and TP content, confirming that root exudates are an important vehicle for material exchange between plant roots and the soil (Santhanam et al., 2015).
Drought and salt stress changed the rhizosphere soil bacterial community structure of Nitraria tangutorum
Changes in soil physicochemical properties significantly affect soil bacterial community structure and diversity (Orozco-Aceves et al., 2017; Huang et al., 2019). However, in the present study, there were no significant differences in the abundance and diversity of N. tangutorum rhizosphere soil bacteria under drought and salt stress compared with those in CK, indicating that the rhizosphere bacterial community structure was stable, consistent with the results of Liu et al. (2021). NMDS analysis showed that the bacterial community structure of N. tangutorum rhizosphere soil was similar under drought and CK (Figure 2), which might be caused by a single species and limited diffusion of the bacterial community. Salt stress changes the composition of the soil bacterial community. Salt induced changes in the N. tangutorum rhizosphere bacterial community more than drought, reflecting the response characteristics of plant rhizosphere microorganisms to salt stress (Sarkar et al., 2018; Tiepo et al., 2018). In the current study, Firmicutes, Bacteroidota, Proteobacteria, and Actinobacteriota were the dominant phyla in the N. tangutorum rhizosphere. Previous studies have indicated that these bacteria are enriched in the rhizosphere of plants (Filion et al., 2004; Sanguin et al., 2006; Lee et al., 2008; Xu et al., 2009; Gottel et al., 2011;). Under drought stress, the relative abundance of Firmicutes increases, probably because the genera of this phylum are single-skinned bacteria with a thick cell wall that has better resistance to water stress (Lennon et al., 2012; Naylor et al., 2017; Cregger et al., 2018; Xu et al., 2018). However, the relative abundance of Proteobacteria and Actinobacteriota increased under salt stress. The sporogenesis ability of Actinobacteriota has been suggested to enable them to remain stable and quiescent in stressful environments, a strategy that may enable them to survive under adverse conditions (Vanessa et al., 2013; Hacquard et al., 2015; Naylor et al., 2017). Furthermore, a lack of nutrients in the plant rhizosphere soil would increase the abundance of Firmicutes (Singh et al., 2007). In our study, the plants were grown in sandy soils with poor fertility, which was exacerbated by drought. In the present study, drought and salt treatments may have caused drastic changes in soil moisture and pH, affecting the composition of microbial communities. The coexistence of microbial species usually depends on metabolic trade-offs, with each species having an adaptive advantage under specific biotic and abiotic conditions (Xu et al., 2018). Bacteroides and Faecalibacterium in N. tangutorum rhizosphere soil, belonging to phylum Bacteroidota, showed similar changes under drought and salt stress. Studies have shown that the relative abundance of bacteria responding to stress consistently changes for some phyla, classes, orders, families, and genera, which may reflect the common functions and life strategies of specific bacterial lineages (Philippot et al., 2010; Amend et al., 2016). Plants or host species growing in the same environment can attract and aggregate different microbial communities in the root zone and rhizosphere (Aleklett et al., 2015; Samad et al., 2017). Changes in plant rhizosphere soil bacteria observed under drought conditions are usually associated only with changes in drought-sensitive bacterial species but not with changes in the overall plant microflora (Naylor and Coleman-Derr, 2018). Therefore, we hypothesized that the stable rhizosphere soil bacterial diversity of N. tangutorum under drought stress is due to the increased abundance of specific bacteria in the rhizosphere soil. Bacterial community network analysis showed that the degree of isolation in the operational taxonomic unit module of the rhizosphere soil core bacterial community of N. tangutorum under drought stress was significantly higher than that in CK (Figure 4).
Salt stress changed the interaction of rhizosphere soil bacteria and the r-and k-strategists of Nitraria tangutorum
Under drought and salt stress, plants can influence the species and quantity of bacterial communities in the rhizosphere through changes in root exudates (Mayak et al., 2004). The rhizosphere soil bacterial community structure of N. tangutorum under drought and salt stress was significantly different. In stressful environments, plant rhizosphere actively release large amounts of bioactive substances, sugars, and organic acids to attract functional bacteria for colonization, thereby changing the structure of the rhizosphere microbial community to adapt to stress (Fierer, 2017; Keswani et al., 2019). In addition, root exudate composition plays a selective role in establishing rhizosphere microbial communities (Wieland et al., 2001; Kowalchuk et al., 2002). Zwetsloot et al. (2020) found that ubiquitous root phenols could alter soil microbial communities. Changes in soil chemical properties and structure caused by rhizosphere metabolic activities allow growth of specific rhizosphere microbial communities (Haichar et al., 2008; Chaparro et al., 2014; Lareen et al., 2016). Our results further confirmed previous conclusions and also found that the root metabolites of plants under different stresses shaped microbial communities with different ecological functions.
For the ecological functions, microbial taxa are often divided into r-and k-strategists based on their growth, reproduction, competition, and adaptation strategies (Fierer et al., 2007; Li et al., 2021). The observed differences in rhizosphere bacterial populations of r-and k-strategists in N. tangutorum under salt stress shed light on the potential microbial mechanism of plant root exudates regulating the interactions among rhizosphere bacteria under stressful environments. Numerous studies have shown that r-strategist bacteria grow and reproduce rapidly in nutrient-rich environments, whereas k-strategist bacteria grow slowly under oligotrophic conditions (Fierer et al., 2007). In the present study, Bacteroidota and Alphaproteobacteria showed r-and k-strategist characteristics, respectively. Under salt stress, plants select suitable bacteria for colonization using different root exudates and then change the bacterial community structure. It has been confirmed that soil bacterial communities are usually limited by the availability of carbon substrates (Raich and Tufekciogul, 2000). In the current study, Bacteroidota and Alphaproteobacteria showed stronger correlations with TC and SOM under salt stress. Therefore, it can be inferred that salt stress reduced soil nutrient availability and inhibited the growth of r-strategist bacteria (Sicardi et al., 2004), whereas the accumulation of unstable carbon sources in low-molecular-weight compounds in N. tangutorum root exudates increased the k-strategist bacterial community (Kielak et al., 2009; Zhang et al., 2018).
The high connectivity of soil bacterial network relationships is considered a rapid response to environmental changes (Zhang et al., 2018). Many studies have shown that the stronger the plant-microbe interactions in the soil, the stronger the network relationships (Zhang et al., 2018). We found that rhizosphere soil bacteria had a closer network relationship under CK, whereas drought stress reduced the network connectivity and complexity of N. tangutorum rhizosphere soil bacteria. Interactions among the rhizosphere soil bacteria of N. tangutorum also decreased under salt stress. Furthermore, Shi et al. (2016) pointed out that the connectivity and complexity of soil microbial networks are important indicators of soil physicochemical properties that determine plant-bacteria interactions (Shi et al., 2016; Li et al., 2018). In our study, the number of nodes and edges in the bacterial network changed with soil moisture and salinity, indicating that these changes were influenced by the soil physicochemical properties. Guan et al. (2021) reported that simple network relationships could negatively affect geochemical functions, especially in salinized soils with unstable bacterial communities. Our study confirms the above conclusion that soil bacterial interactions gradually decrease with increasing soil EC and pH. However, changes in soil physicochemical properties caused by rhizosphere metabolism can shape specific rhizosphere microbial communities (Haichar et al., 2008; Chaparro et al., 2014; Lareen et al., 2016). Our results also confirmed that a change in root exudates caused by soil salinity affects the interaction among rhizosphere soil bacteria.
Conclusion
In conclusion, the rhizosphere soil bacterial community of N. tangutorum was highly responsive to environmental changes (drought and salt stress). Drought and salt stress decreased the nutrient content of the rhizosphere soil of N. tangutorum, while the differential metabolites such as organic acids, growth hormones, and sugars were the active strategies for the root system in response to stress. Meanwhile, the correlation between bacterial community diversity, richness and soil physicochemical properties was enhanced, which drove the bacterial community towards k-strategists. The network analysis also highlighted the effect of environmental changes on rhizosphere bacterial interactions, while differential root metabolites were important factors influencing the r/k categories of bacterial communities. Our analysis further suggests that root exudates play a prominent role as life carriers between plants and the soil environment in responding to environmental changes and assembling the structure of the rhizosphere microbial communities. This study reveals the mechanism of plant–soil-microbe interactions under the action of root exudates, and provides new ideas for studying the response of bacterial communities to stressful environments.
Data availability statement
The datasets presented in this study can be found in online repositories. The names of the repository/repositories and accession number(s) can be found at: https://www.ncbi.nlm.nih.gov/, PRJNA855333.
Author contributions
PK and NS conceived and designed the study. YP, MT, JH, and YZ did running the experiments and data management. JH and YP performed the data mining, statistical analysis, interpretation, and figure and table preparation of the 16S rRNA amplicon sequencing results. YP, PK, JZ, and XL did the manuscript writing and revising. All authors contributed to the article and approved the submitted version.
Funding
This work was supported financially by the National Natural Science Foundation of China (41621001), Key Research and Development Project of Ningxia (2019BEB04018), Ningxia Natural Science Foundation (2022AAC03227), and Innovation Team for Genetic Improvement of Economic Forests Foundation in Ningxia (2022QCXTD04).
Acknowledgments
We would like to thank Editage (www.editage.cn) for English language editing.
Conflict of interest
The authors declare that the research was conducted in the absence of any commercial or financial relationships that could be construed as a potential conflict of interest.
Publisher’s note
All claims expressed in this article are solely those of the authors and do not necessarily represent those of their affiliated organizations, or those of the publisher, the editors and the reviewers. Any product that may be evaluated in this article, or claim that may be made by its manufacturer, is not guaranteed or endorsed by the publisher.
Supplementary material
The Supplementary material for this article can be found online at: https://www.frontiersin.org/articles/10.3389/fpls.2022.997292/full#supplementary-material
Abbreviations
CK, Control samples; SWC, Soil water content; EC, Electrical conductivity; TC, Soil total carbon; TN, Total nitrogen; TP, Total phosphorus; TOC, Soil total organic carbon; NMDS, Non-metric multidimensional scale; QC, Quality control; LC–MS, Liquid chromatography-mass spectrometry; SOM, Soil organic matter.
Footnotes
References
Aleklett, K., Leff, J. W., Fierer, N., and Hart, M. (2015). Wild plant species growing closely connected in a subalpine meadow host distinct root-associated bacterial communities. PeerJ. 3:e804. doi: 10.7717/peerj.804
Amend, A. S., Martiny, A. C., Allison, S. D., Berlemont, R., Goulden, M. L., Lu, Y., et al. (2016). Microbial response to simulated global change is phylogenetically conserved and linked with functional potential. ISME J. 10, 109–118. doi: 10.1038/ismej.2015.96
Badri, D. V., and Vivanco, J. M. (2009). Regulation and function of root exudates. Plant Cell Environ. 32, 666–681. doi: 10.1111/j.1365-3040.2009.01926.x
Baetz, U., and Martinoia, E. (2014). Root exudates: the hidden part of plant defense. Trends Plant Sci. 19, 90–98. doi: 10.1016/j.tplants.2013.11.006
Bahram, M., Harend, H., and Tedersoo, L. (2014). Network perspectives of ectomycorrhizal associations. Fungal Ecol. 7, 70–77. doi: 10.1016/j.funeco.2013.10.003
Banerjee, S., Walder, F., Büchi, L., Meyer, M., Held, A. Y., Gattinger, A., et al. (2019). Agricultural intensification reduces microbial network complexity and the abundance of keystone taxa in roots. ISME J. 13, 1722–1736. doi: 10.1038/s41396-019-0383-2
Bardgett, R. D., and van der Putten, W. H. (2014). Belowground biodiversity and ecosystem functioning. Nature 515, 505–511. doi: 10.1038/nature13855
Berry, D., and Widder, S. (2014). Deciphering microbial interactions and detecting keystone species with co-occurrence networks. Front. Microbiol. 5, 219. doi: 10.3389/fmicb.2014.00219
Bettinelli, M., Beone, G. M., Spezia, S., and Baffi, C. (2000). Determination of heavy metals in soils and sediments by microwave-assisted digestion and inductively coupled plasma optical emission spectrometry analysis. Anal. Chim. Acta 424, 289–296. doi: 10.1016/S0003-2670(00)01123-5
Canarini, A., Kaiser, C., Merchant, A., Richter, A., and Wanek, W. (2019). Root exudation of primary metabolites: mechanisms and their roles in plant responses to environmental stimuli. Front. Plant Sci. 10, 157. doi: 10.3389/fpls.2019.00157
Caporaso, J. G., Lauber, C. L., Walters, W. A., Berg-Lyons, D., Huntley, J., Fierer, N., et al. (2012). Ultra-high-throughput microbial community analysis on the illumina HiSeq and MiSeq platforms. ISME J. 6, 1621–1624. doi: 10.1038/ismej.2012.8
Chaparro, J. M., Badri, D. V., and Vivanco, J. M. (2014). Rhizosphere microbiome assemblage is affected by plant development. ISME J. 8, 790–803. doi: 10.1038/ismej.2013.196
Cregger, M. A., Veach, A. M., Yang, Z. K., Crouch, M. J., Vilgalys, R., Tuskan, G. A., et al. (2018). The Populus holobiont: dissecting the effects of plant niches and genotype on the microbiome. Microbiome 6, 31. doi: 10.1186/s40168-018-0413-8
de Vries, F. T., Williams, A., Stringer, F., Willcocks, R., McEwing, R., Langridge, H., et al. (2019). Changes in root-exudate-induced respiration reveal a novel mechanism through which drought affects ecosystem carbon cycling. New Phytol. 224, 132–145. doi: 10.1111/nph.16001
Delgado-Baquerizo, M., Maestre, F. T., Reich, P. B., Trivedi, P., Osanai, Y., Liu, Y. R., et al. (2016). Carbon content and climate variability drive global soil bacterial diversity patterns. Ecol. Monogr. 86, 373–390. doi: 10.1002/ecm.1216
Dong, X. L., Li, M. Z., Lin, Q. M., Li, G. T., and Zhao, X. R. (2019). Soil Na+ concentration controls salt-affected soil organic matter components in Hetao region China. J. Soils Sediments 19, 1120–1129. doi: 10.1007/s11368-018-2127-8
Duran, P., Thiergart, T., Garrido-Oter, R., Agler, M., Kemen, E., Schulze-Lefert, P., et al. (2018). Microbial Interkingdom interactions in roots promote Arabidopsis survival. Cell 175, 973–983.e14. doi: 10.1016/j.cell.2018.10.020
Fierer, N. (2017). Embracing the unknown: disentangling the complexities of the soil microbiome. Nat. Rev. Microbiol. 15, 579–590. doi: 10.1038/nrmicro.2017.87
Fierer, N., Bradford, M. A., and Jackson, R. B. (2007). Toward an ecological classification of soil bacteria. Ecology 88, 1354–1364. doi: 10.1890/05-1839
Filion, M., Hamelin, R. C., Bernier, L., and St-Arnaud, M. (2004). Molecular profiling of rhizosphere microbial communities associated with healthy and diseased black spruce (Picea mariana) seedlings grown in a nursery. Appl. Environ. Microbiol. 70, 3541–3551. doi: 10.1128/aem.70.6.3541-3551.2004
Flowers, T., Galal, H., and Bromham, L. (2010). Evolution of halophytes: multiple origins of salt tolerance in land plants. Funct. Plant Biol. 37, 604–612. doi: 10.1071/FP09269
Fujii, K., Aoki, M., and Kitayama, K. (2012). Biodegradation of low molecular weight organic acids in rhizosphere soils from a tropical montane rain forest. Soil Biol. Biochem. 47, 142–148. doi: 10.1016/j.soilbio.2011.12.018
Gargallo-Garriga, A., Preece, C., Sardans, J., Oravec, M., Urban, O., and Peñuelas, J. (2018). Root exudate metabolomes change under drought and show limited capacity for recovery. Sci. Rep. 8, 12696. doi: 10.1038/s41598-018-30150-0
Gong, Q., Li, P., Ma, S., Indu Rupassara, S., and Bohnert, H. J. (2005). Salinity stress adaptation competence in the extremophile Thellungiella halophila in comparison with its relative Arabidopsis thaliana. Plant J. 44, 826–839. doi: 10.1111/j.1365-313X.2005.02587.x
Gottel, N. R., Castro, H. F., Kerley, M., Yang, Z., Pelletier, D. A., Podar, M., et al. (2011). Distinct microbial communities within the endosphere and rhizosphere of Populus deltoides roots across contrasting soil types. Appl. Environ. Microbiol. 77, 5934–5944. doi: 10.1128/aem.05255-11
Gregory, P. J. (2007). “The rhizosphere” in Plant Roots: Growth, Activity and Interactions with the Soil. ed. P. J. Gregory (Oxford: Blackwell Publishing), 216–252.
Gu, Y., Bai, Y., Xiang, Q., Yu, X., Zhao, K., Zhang, X., et al. (2018). Degradation shaped bacterial and archaeal communities with predictable taxa and their association patterns in Zoige wetland at Tibet plateau. Sci. Rep. 8, 3884. doi: 10.1038/s41598-018-21874-0
Guan, Y. P., Jiang, N. N., Wu, Y. X., Yang, Z. Z., Bello, A., and Yang, W. (2021). Disentangling the role of salinity-sodicity in shaping soil microbiome along a natural saline-sodic gradient. Sci. Total Environ. 765:142738. doi: 10.1016/j.scitotenv.2020.142738
Gul, S., Whalen, J. K., Thomas, B. W., Sachdeva, V., and Deng, H. (2015). Physico-chemical properties and microbial responses in biochar-amended soils: mechanisms and future directions. Agric. Ecosyst. Environ. 206, 46–59. doi: 10.1016/j.agee.2015.03.015
Guo, H., Cui, Y. N., Pan, Y. Q., Wang, S. M., and Bao, A. K. (2020). Sodium chloride facilitates the Atriplex canescens adaptation to drought stress. Plant Physiol. Biochem. 150, 99–108. doi: 10.1016/j.plaphy.2020.02.018
Guyonnet, J. P., Cantarel, A. A. M., Simon, L., and Haichar, F. E. Z. (2018). Root exudation rate as functional trait involved in plant nutrient-use strategy classification. Ecol. Evol. 8, 8573–8581. doi: 10.1002/ece3.4383
Haas, B. J., Gevers, D., Earl, A. M., Feldgarden, M., Ward, D. V., Giannoukos, G., et al. (2011). Chimeric 16S rRNA sequence formation and detection in sanger and 454-pyrosequenced PCR amplicons. Genome Res. 21, 494–504. doi: 10.1101/gr.112730.110
Hacquard, S., Garrido-Oter, R., González, A., Spaepen, S., Ackermann, G., Lebeis, S., et al. (2015). Microbiota and host nutrition across plant and animal kingdoms. Cell Host Microbe 17, 603–616. doi: 10.1016/j.chom.2015.04.009
Haichar, F. Z., Marol, C., Berge, O., Rangel-Castro, J. I., Prosser, J. I., Balesdent, J., et al. (2008). Plant host habitat and root exudates shape soil bacterial community structure. ISME J. 2, 1221–1230. doi: 10.1038/ismej.2008.80
Henry, A., Doucette, W., Norton, J., and Bugbee, B. (2007). Changes in crested wheatgrass root exudation caused by flood, drought, and nutrient stress. J. Environ. Qual. 36, 904–912. doi: 10.2134/jeq2006.0425sc
Hernandez, D. J., David, A. S., Menges, E. S., Searcy, C. A., and Afkhami, M. E. (2021). Environmental stress destabilizes microbial networks. ISME J. 15, 1722–1734. doi: 10.1038/s41396-020-00882-x
Huang, L., Kou, W., Wu, L., Feinstein, L., Kong, Z. Y., and Ge, G. (2019). Microbial composition and activity of natural, restored, and reclaimed wetland soils: a case study of Poyang Lake Basin, China. Wetlands 39, S113–S123. doi: 10.1007/s13157-018-1020-y
Jenkins, S., Swenson, T. L., Lau, R., Rocha, A. M., Aaring, A., Hazen, T. C., et al. (2017). Construction of viable soil defined media using quantitative metabolomics analysis of soil metabolites. Front. Microbiol. 8, 2618. doi: 10.3389/fmicb.2017.02618
Kang, P., Bao, A. K., Kumar, T., Pan, Y. Q., Bao, Z. L. T., Wang, F., et al. (2016). Assessment of stress tolerance, productivity, and forage quality in T-1 transgenic alfalfa co-overexpressing ZxNHX and ZxVP1-1 from Zygophyllum xanthoxylum. Front. Plant Sci. 7, 1598. doi: 10.3389/fpls.2016.01598
Kang, J. J., Zhao, W. Z., Zhao, M., Zheng, Y., and Yang, F. (2015). NaCl and Na2SiO3 coexistence strengthens growth of the succulent xerophyte Nitraria tangutorum under drought. Plant Growth Regul. 77, 223–232. doi: 10.1007/s10725-015-0055-9
Karlowsky, S., Augusti, A., Ingrisch, J., Akanda, M. K. U., Bahn, M., and Gleixner, G. (2018). Drought-induced accumulation of root exudates supports post-drought recovery of microbes in mountain grassland. Front. Plant Sci. 9, 1593. doi: 10.3389/fpls.2018.01593
Keswani, C., Prakash, O., Bharti, N., Vílchez, J. I., Sansinenea, E., Lally, R. D., et al. (2019). Re-addressing the biosafety issues of plant growth promoting rhizobacteria. Sci. Total Environ. 690, 841–852. doi: 10.1016/j.scitotenv.2019.07.046
Kielak, A., Pijl, A. S., van Veen, J. A., and Kowalchuk, G. A. (2009). Phylogenetic diversity of Acidobacteriota in a former agricultural soil. ISME J. 3, 378–382. doi: 10.1038/ismej.2008.113
Klappenbach, J. A., Dunbar, J. M., and Schmidt, T. M. (2000). rRNA operon copy number reflects ecological strategies of bacteria. Appl. Environ. Microbiol. 66, 1328–1333. doi: 10.1128/aem.66.4.1328-1333.2000
Kong, C. H., Zhang, S. Z., Li, Y. H., Xia, Z. C., Yang, X. F., Meiners, S. J., et al. (2018). Plant neighbor detection and allelochemical response are driven by root-secreted signaling chemicals. Nat. Commun. 9, 3867. doi: 10.1038/s41467-018-06429-1
Kowalchuk, G. A., Buma, D. S., de Boer, W., Klinkhamer, P. G., and van Veen, J. A. (2002). Effects of above-ground plant species composition and diversity on the diversity of soil-borne microorganisms. Antonie Van Leeuwenhoek 81, 509–520. doi: 10.1023/a:1020565523615
Kuczynski, J., Stombaugh, J., Walters, W. A., Gonzalez, A., Caporaso, J. G., and Knight, R. (2012). Using QIIME to analyze 16S rRNA gene sequences from microbial communities. Curr. Protoc. Microbiol. 1, Unit 1E.5. doi: 10.1002/9780471729259.mc01e05s27
Kumar, M., Ji, B., Zengler, K., and Nielsen, J. (2019). Modelling approaches for studying the microbiome. Nat. Microbiol. 4, 1253–1267. doi: 10.1038/s41564-019-0491-9
Langille, M. G., Zaneveld, J., Caporaso, J. G., McDonald, D., Knights, D., Reyes, J. A., et al. (2013). Predictive functional profiling of microbial communities using 16S rRNA marker gene sequences. Nat. Biotechnol. 31, 814–821. doi: 10.1038/nbt.2676
Lareen, A., Burton, F., and Schäfer, P. (2016). Plant root-microbe communication in shaping root microbiomes. Plant Mol. Biol. 90, 575–587. doi: 10.1007/s11103-015-0417-8
Lee, S. H., Ka, J. O., and Cho, J. C. (2008). Members of the phylum Acidobacteriota are dominant and metabolically active in rhizosphere soil. FEMS Microbiol. Lett. 285, 263–269. doi: 10.1111/j.1574-6968.2008.01232.x
Lennon, J. T., Aanderud, Z. T., Lehmkuhl, B. K., and Schoolmaster, D. R. (2012). Mapping the niche space of soil microorganisms using taxonomy and traits. Ecology 93, 1867–1879. doi: 10.1890/11-1745.1
Li, J., Li, C., Kou, Y., Yao, M., He, Z., and Li, X. (2020). Distinct mechanisms shape soil bacterial and fungal co-occurrence networks in a mountain ecosystem. FEMS Microbiol. Ecol. 96, fiaa030. doi: 10.1093/femsec/fiaa030
Li, J. B., Shen, Z. H., Li, C. N., Kou, Y. P., Wang, Y. S., Tu, B., et al. (2018). Stair-step pattern of soil bacterial diversity mainly driven by pH and vegetation types Along the Elevational gradients of Gongga Mountain, China. Front. Microbiol. 9, 569. doi: 10.3389/fmicb.2018.00569
Li, H., Yang, S., Semenov, M., Yao, F., Ye, J., Bu, R., et al. (2021). Temperature sensitivity of SOM decomposition is linked with a K-selected microbial community. Glob. Chang. Biol. 27, 2763–2779. doi: 10.1111/gcb.15593
Liu, W., Zhang, Y., Yuan, X., Xuan, Y., Gao, Y., and Yan, Y. (2016). Exogenous salicylic acid improves salinity tolerance of Nitraria tangutorum. Russ. J. Plant Physiol. 63, 132–142. doi: 10.1134/S1021443716010118
Liu, Q., Zhao, X., Liu, Y., Xie, S., Xing, Y., Dao, J., et al. (2021). Response of sugarcane Rhizosphere bacterial community to drought stress. Front. Microbiol. 12:716196. doi: 10.3389/fmicb.2021.716196
Luo, Q., Sun, L., Hu, X., and Zhou, R. (2014). The variation of root exudates from the hyperaccumulator sedum alfredii under cadmium stress: metabonomics analysis. PLoS One 9:e115581. doi: 10.1371/journal.pone.0115581
Luo, G., Zhou, C., Chen, X., and Li, Y. (2008). A methodology of characterizing status and trend of land changes in oases: a case study of Sangong River watershed, Xinjiang. Chin. J. Environ. Manag. 88, 775–783. doi: 10.1016/j.jenvman.2007.04.003
Ma, B., Wang, H., Dsouza, M., Lou, J., He, Y., Dai, Z., et al. (2016). Geographic patterns of co-occurrence network topological features for soil microbiota at continental scale in eastern China. ISME J. 10, 1891–1901. doi: 10.1038/ismej.2015.261
Ma, B., Wang, Y., Ye, S., Liu, S., Stirling, E., Gilbert, J. A., et al. (2020). Earth microbial co-occurrence network reveals interconnection pattern across microbiomes. Microbiome 8, 82. doi: 10.1186/s40168-020-00857-2
Magoč, T., and Salzberg, S. L. (2011). FLASH: fast length adjustment of short reads to improve genome assemblies. Bioinformatics 27, 2957–2963. doi: 10.1093/bioinformatics/btr507
Mayak, S., Tirosh, T., and Glick, B. R. (2004). Plant growth-promoting bacteria that confer resistance to water stress in tomatoes and peppers. Plant Sci. 166, 525–530. doi: 10.1016/j.plantsci.2003.10.025
Mercado-Blanco, J., and Bakker, P. A. (2007). Interactions between plants and beneficial Pseudomonas spp.: exploiting bacterial traits for crop protection. Antonie Van Leeuwenhoek 92, 367–389. doi: 10.1007/s10482-007-9167-1
Meyer, O. (1994). Functional groups of microorganisms. Biodivers. Ecosyst. Funct. 99, 67–96. doi: 10.1007/978-3-642-58001-7_4
Mommer, L., Hinsinger, P., Prigent-Combaret, C., and Visser, E. J. W. (2016). Advances in the rhizosphere: stretching the interface of life. Plant Soil 407, 1–8. doi: 10.1007/s11104-016-3040-9
Narula, N., Kothe, E., and Behl, R. (2008). Role of root exudates in plant-microbe interactions. J. Appl. Bot. Food Qual. 82, 122–130. doi: 10.1614/IPSM-08-126.1
Naylor, D., and Coleman-Derr, D. (2018). Drought stress and root-associated bacterial communities. Front. Plant Sci. 8, 2223. doi: 10.3389/fpls.2017.02223
Naylor, D., DeGraaf, S., Purdom, E., and Coleman-Derr, D. (2017). Drought and host selection influence bacterial community dynamics in the grass root microbiome. ISME J. 11, 2691–2704. doi: 10.1038/ismej.2017.118
Ni, J. W., Yang, X. Y., Zhu, J. F., Liu, Z. X., Ni, Y. Y., Wu, H. W., et al. (2015). Salinity-induced metabolic profile changes in Nitrariatangutorum Bobr. Suspension cells. Plant Cell Tissue Organ Cult. 122, 239–248. doi: 10.1007/s11240-015-0744-0
Orozco-Aceves, M., Tibbett, M., and Standish, R. J. (2017). Correlation between soil development and native plant growth in forest restoration after surface mining. Ecol. Eng. 106, 209–218. doi: 10.1016/j.ecoleng.2017.06.004
Pan, Y. Q., Guo, H., Wang, S. M., Zhao, B., Zhang, J. L., Ma, Q., et al. (2016). The photosynthesis, Na+/K+ homeostasis and osmotic adjustment of Atriplex canescens in response to salinity. Front. Plant Sci. 7, 848. doi: 10.3389/fpls.2016.00848
Pan, Y. Q., Kang, P., Hu, J. P., and Song, N. P. (2021). Bacterial community demonstrates stronger network connectivity than fungal community in desert-grassland salt marsh. Sci. Total Environ. 798:149118. doi: 10.1016/j.scitotenv.2021.149118
Pascault, N., Ranjard, L., Kaisermann, A., Bachar, D., Christen, R., Terrat, S., et al. (2013). Stimulation of different functional groups of bacteria by various plant residues as a driver of soil priming effect. Ecosystems 16, 810–822. doi: 10.1007/s10021-013-9650-7
Per, T. S., Khan, N. A., Reddy, P. S., Masood, A., Hasanuzzaman, M., Khan, M. I. R., et al. (2017). Approaches in modulating proline metabolism in plants for salt and drought stress tolerance: Phytohormones, mineral nutrients and transgenics. Plant Physiol. Biochem. 115, 126–140. doi: 10.1016/j.plaphy.2017.03.018
Pétriacq, P., Williams, A., Cotton, A., McFarlane, A. E., Rolfe, S. A., and Ton, J. (2017). Metabolite profiling of non-sterile rhizosphere soil. Plant J. 92, 147–162. doi: 10.1111/tpj.13639
Philippot, L., Andersson, S. G. E., Battin, T. J., Prosser, J. I., Schimel, J. P., Whitman, W. B., et al. (2010). The ecological coherence of high bacterial taxonomic ranks. Nat. Rev. Microbiol. 8, 523–529. doi: 10.1038/nrmicro2367
Postma, A., Slabbert, E., Postma, F., and Jacobs, K. (2016). Soil bacterial communities associated with natural and commercial Cyclopia spp. FEMS Microbiol. Ecol. 92, fiw016. doi: 10.1093/femsec/fiw016
Quast, C., Pruesse, E., Yilmaz, P., Gerken, J., Schweer, T., Yarza, P., et al. (2013). The SILVA ribosomal RNA gene database project: improved data processing and web-based tools. Nucleic Acids Res. 41, D590–D596. doi: 10.1093/nar/gks1219
R Core Team (2020). R: A Language and Environment for Statistical Computing. Vienna: R Foundation for Statistical Computing.
Raich, J. W., and Tufekciogul, A. (2000). Vegetation and soil respiration: correlations and controls. Biogeochemistry 48, 71–90. doi: 10.1023/A:1006112000616
Ramette, A. (2010). Multivariate analyses in microbial ecology. FEMS Microbiol. Ecol. 62, 142–160. doi: 10.1111/j.1574-6941.2007.00375.x
Rottjers, L., and Faust, K. (2018). From hairballs to hypotheses-biological insights from microbial networks. FEMS Microbiol. Rev. 42, 761–780. doi: 10.1093/femsre/fuy030
Ruan, Y. L. (2014). Sucrose metabolism: gateway to diverse carbon use and sugar signaling. Annu. Rev. Plant Biol. 65, 33–67. doi: 10.1146/annurev-arplant-050213-040251
Samad, A., Trognitz, F., Compant, S., Antonielli, L., and Sessitsch, A. (2017). Shared and host specific microbiome diversity and functioning of grapevine and accompanying weed plants. Environ. Microbiol. 19, 1407–1424. doi: 10.1111/1462-2920.13618
Sanguin, H., Remenant, B., Dechesne, A., Thioulouse, J., Vogel, T. M., Nesme, X., et al. (2006). Potential of a 16S rRNA-based taxonomic microarray for analyzing the rhizosphere effects of maize on agrobacterium spp. and bacterial communities. Appl. Environ. Microbiol. 72, 4302–4312. doi: 10.1128/aem.02686-05
Santhanam, R., Luu, V. T., Weinhold, A., Goldberg, J., Oh, Y., and Baldwin, I. T. (2015). Native root-associated bacteria rescue a plant from a sudden-wilt disease that emerged during continuous cropping. Proc. Natl. Acad. Sci. U. S. A. 112, E5013–E5020. doi: 10.1073/pnas.1505765112
Sarkar, J., Chakraborty, B., and Chakraborty, U. (2018). Plant growth promoting Rhizobacteria protect wheat plants against temperature stress through antioxidant signalling and reducing chloroplast and membrane injury. J. Plant Growth Regul. 37, 1396–1412. doi: 10.1007/s00344-018-9789-8
Sayer, E. J., Oliver, A. E., Fridley, J. D., Askew, A. P., Mills, R. T., and Grime, J. P. (2017). Links between soil microbial communities and plant traits in a species-rich grassland under long-term climate change. Ecol. Evol. 7, 855–862. doi: 10.1002/ece3.2700
Schleuning, M., Fründ, J., and García, D. (2015). Predicting ecosystem functions from biodiversity and mutualistic networks: an extension of trait-based concepts to plant-animal interactions. Ecography 38, 380–392. doi: 10.1111/ecog.00983
Senechkin, I. V., Speksnijder, A. G., Semenov, A. M., van Bruggen, A. H., and van Overbeek, L. S. (2010). Isolation and partial characterization of bacterial strains on low organic carbon medium from soils fertilized with different organic amendments. Microb. Ecol. 60, 829–839. doi: 10.1007/s00248-010-9670-1
Shi, Y., Delgado-Baquerizo, M., Li, Y., Yang, Y., Zhu, Y. G., Peñuelas, J., et al. (2020). Abundance of kinless hubs within soil microbial networks are associated with high functional potential in agricultural ecosystems. Environ. Int. 142:105869. doi: 10.1016/j.envint.2020.105869
Shi, S., Nuccio, E. E., Shi, Z. J., He, Z., Zhou, J., and Firestone, M. K. (2016). The interconnected rhizosphere: high network complexity dominates rhizosphere assemblages. Ecol. Lett. 19, 926–936. doi: 10.1111/ele.12630
Sicardi, M., Garcı́a-Préchac, F., and Frioni, L. (2004). Soil microbial indicators sensitive to land use conversion from pastures to commercial Eucalyptus grandis (hill ex maiden) plantations in Uruguay. Appl. Soil Ecol. 27, 125–133. doi: 10.1016/j.apsoil.2004.05.004
Singh, B. K., Munro, S., Potts, J. M., and Millard, P. (2007). Influence of grass species and soil type on rhizosphere microbial community structure in grassland soils. Appl. Soil Ecol. 36, 147–155. doi: 10.1016/j.apsoil.2007.01.004
Sokol, N. W., Kuebbing, S. E., Karlsen-Ayala, E., and Bradford, M. A. (2019). Evidence for the primacy of living root inputs, not root or shoot litter, in forming soil organic carbon. New Phytol. 221, 233–246. doi: 10.1111/nph.15361
Su, X., Su, X., Yang, S., Zhou, G., Ni, M., Wang, C., et al. (2020). Drought changed soil organic carbon composition and bacterial carbon metabolizing patterns in a subtropical evergreen forest. Sci. Total Environ. 736:139568. doi: 10.1016/j.scitotenv.2020.139568
Tiepo, A. N., Hertel, M. F., Rocha, S. S., Calzavara, A. K., De Oliveira, A. L. M., Pimenta, J. A., et al. (2018). Enhanced drought tolerance in seedlings of Neotropical tree species inoculated with plant growth-promoting bacteria. Plant Physiol. Biochem. 130, 277–288. doi: 10.1016/j.plaphy.2018.07.021
Toju, H., Peay, K. G., Yamamichi, M., Narisawa, K., Hiruma, K., Naito, K., et al. (2018). Core microbiomes for sustainable agroecosystems. Nat Plants 4, 247–257. doi: 10.1038/s41477-018-0139-4
Vanessa, N. K., Gouvêa, T. R., Duarte, L. O. M., Dini, A. F., Rodrigo, M., Itamar, S. D. M., et al. (2013). Water regime influences bulk soil and rhizosphere of Cereus jamacaru bacterial communities in the Brazilian Caatinga biome. PLoS One 8:e73606. doi: 10.1371/journal.pone.0073606
Venugopal, S. C., Chanda, B., Vaillancourt, L., Kachroo, A., and Kachroo, P. (2009). The common metabolite glycerol-3-phosphate is a novel regulator of plant defense signaling. Plant Signal. Behav. 4, 746–749. doi: 10.4161/psb.4.8.9111
Vives-Peris, V., Molina, L., Segura, A., Gómez-Cadenas, A., and Pérez-Clemente, R. M. (2018). Root exudates from citrus plants subjected to abiotic stress conditions have a positive effect on rhizobacteria. J. Plant Physiol. 228, 208–217. doi: 10.1016/j.jplph.2018.06.003
Wang, W., Akhtar, K., Ren, G., Yang, G., Feng, Y., and Yuan, L. (2019). Impact of straw management on seasonal soil carbon dioxide emissions, soil water content, and temperature in a semi-arid region of China. Sci. Total Environ. 652, 471–482. doi: 10.1016/j.scitotenv.2018.10.207
Wardle, D. A. (2006). The influence of biotic interactions on soil biodiversity. Ecol. Lett. 9, 870–886. doi: 10.1111/j.1461-0248.2006.00931.x
Wieland, G., Neumann, R., and Backhaus, H. (2001). Variation of microbial communities in soil, rhizosphere, and rhizoplane in response to crop species, soil type, and crop development. Appl. Environ. Microbiol. 67, 5849–5854. doi: 10.1128/aem.67.12.5849-5854.2001
Wu, L. K., Lin, W. X., and Lin, X. M. (2014). Advances and perspective in research on plant-soil-microbe interactions mediated by root exudates. Chin. J. Plant Ecol. 38, 298–310. doi: 10.3724/SP.J.1258.2014.00027
Xia, Z. C., Kong, C. H., Chen, L. C., Wang, P., and Wang, S. L. (2016). A broadleaf species enhances an autotoxic conifers growth through belowground chemical interactions. Ecology 97, 2283–2292. doi: 10.1002/ecy.1465
Xiao, Y., Angulo, M. T., Friedman, J., Waldor, M. K., Weiss, S. T., and Liu, Y. Y. (2017). Mapping the ecological networks of microbial communities. Nat. Commun. 8, 2042. doi: 10.1038/s41467-017-02090-2
Xu, L., Naylor, D., Dong, Z., Simmons, T., Pierroz, G., Hixson, K. K., et al. (2018). Drought delays development of the sorghum root microbiome and enriches for monoderm bacteria. Proc. Natl. Acad. Sci. U. S. A. 115, e4284–e4293. doi: 10.1073/pnas.1717308115
Xu, Y., Wang, G., Jin, J., Liu, J., Zhang, Q., and Liu, X. (2009). Bacterial communities in soybean rhizosphere in response to soil type, soybean genotype, and their growth stage. Soil Biol. Biochem. 41, 919–925. doi: 10.1016/j.soilbio.2008.10.027
Yan, Y. Q., Pan, C. H., Du, Y. L., Li, D. Y., and Liu, W. (2018). Exogenous salicylic acid regulates reactive oxygen species metabolism and ascorbate-glutathione cycle in Nitrariatangutorum Bobr. Under salinity stress. Physiol. Mol. Biol. 24, 577–589. doi: 10.1007/s12298-018-0540-5
Ye, J., Joseph, S. D., Ji, M., Nielsen, S., Mitchell, D. R. G., Donne, S., et al. (2017). Chemolithotrophic processes in the bacterial communities on the surface of mineral-enriched biochars. ISME J. 11, 1087–1101. doi: 10.1038/ismej.2016.187
Zhalnina, K., Louie, K. B., Hao, Z., Mansoori, N., da Rocha, U. N., Shi, S., et al. (2018). Dynamic root exudate chemistry and microbial substrate preferences drive patterns in rhizosphere microbial community assembly. Nat. Microbiol. 3, 470–480. doi: 10.1038/s41564-018-0129-3
Zhang, X., Liu, S., Huang, Y., Fu, S., Wang, J., Ming, A., et al. (2018). Tree species mixture inhibits soil organic carbon mineralization accompanied by decreased r-selected bacteria. Plant Soil 431, 203–216. doi: 10.1007/s11104-018-3755-x
Zhou, Y., Tang, N., Huang, L., Zhao, Y., Tang, X., and Wang, K. (2018). Effects of salt stress on plant growth, antioxidant capacity, glandular Trichome density, and volatile exudates of Schizonepeta tenuifolia Briq. Int. J. Mol. Sci. 19, 252. doi: 10.3390/ijms19010252
Keywords: Nitraria tangutorum, drought or salt stress, root exudates, rhizosphere bacteria, co-occurrence network, k-strategists
Citation: Pan Y, Kang P, Tan M, Hu J, Zhang Y, Zhang J, Song N and Li X (2022) Root exudates and rhizosphere soil bacterial relationships of Nitraria tangutorum are linked to k-strategists bacterial community under salt stress. Front. Plant Sci. 13:997292. doi: 10.3389/fpls.2022.997292
Edited by:
Akash Tariq, Xinjiang Institute of Ecology and Geography (CAS), ChinaReviewed by:
Yanfu Bai, Sichuan Agricultural University, ChinaJunhong Zhang, Zhejiang Agriculture and Forestry University, China
Copyright © 2022 Pan, Kang, Tan, Hu, Zhang, Zhang, Song and Li. This is an open-access article distributed under the terms of the Creative Commons Attribution License (CC BY). The use, distribution or reproduction in other forums is permitted, provided the original author(s) and the copyright owner(s) are credited and that the original publication in this journal is cited, in accordance with accepted academic practice. No use, distribution or reproduction is permitted which does not comply with these terms.
*Correspondence: Naiping Song, c29uZ25wQDE2My5jb20=; Xinrong Li, bHhpbnJvbmdAbHpiLmFjLmNu
†These authors have contributed equally to this work and share first authorship