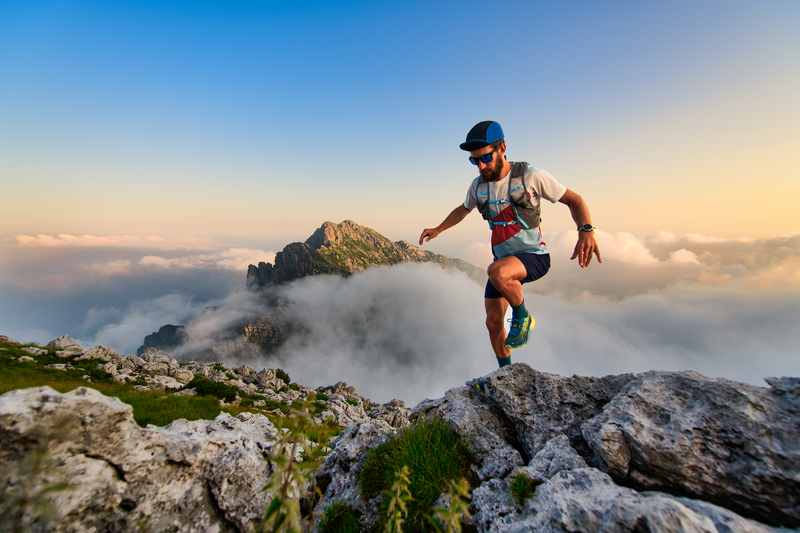
95% of researchers rate our articles as excellent or good
Learn more about the work of our research integrity team to safeguard the quality of each article we publish.
Find out more
ORIGINAL RESEARCH article
Front. Plant Sci. , 29 September 2022
Sec. Plant Bioinformatics
Volume 13 - 2022 | https://doi.org/10.3389/fpls.2022.994679
This article is part of the Research Topic Multi-omics and Computational Biology in Horticultural Plants: From Genotype to Phenotype View all 32 articles
Orchidaceae, with more than 25,000 species, is one of the largest flowering plant families that can successfully colonize wide ecological niches, such as land, trees, or rocks, and its members are divided into epiphytic, terrestrial, and saprophytic types according to their life forms. Cellulose synthase (CesA) and cellulose synthase-like (Csl) genes are key regulators in the synthesis of plant cell wall polysaccharides, which play an important role in the adaptation of orchids to resist abiotic stresses, such as drought and cold. In this study, nine whole-genome sequenced orchid species with three types of life forms were selected; the CesA/Csl gene family was identified; the evolutionary roles and expression patterns of CesA/Csl genes adapted to different life forms and abiotic stresses were investigated. The CesA/Csl genes of nine orchid species were divided into eight subfamilies: CesA and CslA/B/C/D/E/G/H, among which the CslD subfamily had the highest number of genes, followed by CesA, whereas CslB subfamily had the least number of genes. Expansion of the CesA/Csl gene family in orchids mainly occurred in the CslD and CslF subfamilies. Conserved domain analysis revealed that eight subfamilies were conserved with variations in orchids. In total, 17 pairs of CesA/Csl homologous genes underwent positive selection, of which 86%, 14%, and none belonged to the epiphytic, terrestrial, and saprophytic orchids, respectively. The inter-species collinearity analysis showed that the CslD genes expanded in epiphytic orchids. Compared with terrestrial and saprophytic orchids, epiphytic orchids experienced greater strength of positive selection, with expansion events mostly related to the CslD subfamily, which might have resulted in strong adaptability to stress in epiphytes. Experiments on stem expression changes under abiotic stress showed that the CslA might be a key subfamily in response to drought stress for orchids with different life forms, whereas the CslD might be a key subfamily in epiphytic and saprophytic orchids to adapt to freezing stress. This study provides the basic knowledge for the further systematic study of the adaptive evolution of the CesA/Csl superfamily in angiosperms with different life forms, and research on orchid-specific functional genes related to life-history trait evolution.
Stored carbohydrates serve as a carbon and energy resource for land plant growth and against adverse and favorable conditions (Ranwala and Miller, 2008), and have contributed to drought resistance, frost resistance, salt tolerance, and penetration (Mattana et al., 2005; Rosa et al., 2009; Zhang et al., 2016). The cell wall, an important part of plant cells, consists of a basic skeleton of polysaccharides that constitutes the plant’s main carbon sink, which is ultimately maintained by the plant’s ability to fix carbon dioxide through photosynthesis (Lampugnani et al., 2018). Plants overcome the high intracellular osmotic pressure to a certain extent by assembling some photosynthetic carbohydrate products into cell wall polysaccharides, thereby increasing the strength and flexibility of terrestrial plant cell walls, enabling individual cells to withstand enormous swelling pressures, preventing the rupture of membrane and the structure that controls cell growth, and enabling plants to better cope with environmental stresses, such as drought, freezing, and osmosis (Sarkar et al., 2009). Therefore, the synthesis of plant cell wall polysaccharides plays an important role in the growth and adaptive evolution of plants with different life forms living in extreme or harsh natural habitats.
Studies have shown that mannan is one of the main components of the cell wall polysaccharide in plants. For example, mannan accounts for 58.3% of the dry weight of the crude polysaccharide of the orchid Dendrobium officinale (Xing et al., 2015). The key enzymes involved in the biosynthesis of mannan belong to the cellulose synthase (CesA) family (Lerouxel et al., 2006). The CesA family can be subdivided into one cellulose synthase family (CesA) and eight cellulose synthases-like (CslA-CslH), in which each subfamily is involved in regulating different life processes that are involved in cell wall polysaccharide synthesis (Richmond and Somerville, 2000; Suzuki et al., 2006; Little et al., 2018). The main function of CesA is to participate in primary and secondary cell wall synthesis (Hamann et al., 2004; Farrokhi et al., 2006). CslA mainly encodes β-1,4-mannan synthase (Yin et al., 2009). CslC is involved in catalyzing the formation of the xyloglucan skeleton (Kim et al., 2020). CslD is involved in the synthesis of cell wall polysaccharides, mainly xylan and galacturonan (Verhertbruggen et al., 2011; Yang et al., 2020). CslF and CslH mediate β-(1,3;1,4)-D-glucan synthesis (Burton et al., 2006; Doblin et al., 2009). The CslG gene family is generally considered to be involved in the synthesis of cell wall polysaccharides (Richmond and Somerville, 2000). However, the biological functions of CslB and CslE are unclear (Richmond and Somerville, 2000).
Orchidaceae is one of the largest and most widely distributed families of flowering plants with more than 25,000 species, accounting for approximately 10% of flowering plant species (Leitch et al., 2009). Orchids have unique flower morphologies and extraordinary lifestyle diversity and are distributed in almost every habitat on the Earth (Roberts and Dixon, 2008; Givnish et al., 2015, 2016). Orchids can successfully colonize terrestrial, epiphytic, or lithophytic ecological niches, and attach to trees or rocks; they are mainly divided into epiphytic, saprophytic, and terrestrial types, and utilize crassulacean acid metabolism (CAM) for growth under different environmental conditions (Cai et al., 2015; Zhang et al., 2016). Several studies showed that orchids especially those (e.g., Dendrobium genus) with fleshy stems are rich in various types of active polysaccharides in the cell wall, which are related to drought or cold stress adaptation to different environmental conditions (Xing et al., 2015; Jin et al., 2016; Zhang et al., 2016; Wan et al., 2018).
Over the past two decades, the number of sequenced species has increased exponentially with advances in genome-sequencing technology and genome-assembly algorithms. Sequences of more than 1,000 plant genomes have been published, representing more than 790 different species with highly diverse life histories (Marks et al., 2021; Sun et al., 2021). Since the release of the first orchid genome of Phalaenopsis equestris (Cai et al., 2015), other orchids, such as D. officinale (Zhang et al., 2016), D. huoshanense (Han et al., 2020), D. chrysotoxum (Zhang et al., 2021), Apostasia shenzhenica (Zhang et al., 2017), Cymbidium ensifolium (Ai et al., 2021), P. aphrodite (Chao et al., 2018), Vanilla planifolia (Hasing et al., 2020), and Gastrodia elata (Xu et al., 2021) have been sequenced. Among the above nine orchid species, D. officinale, D. huoshanense, D. chrysotoxum, P. equestris, and P. aphrodite are epiphytic; C. ensifolium, V. planifolia, and A. shenzhenica are terrestrial; and G. elata is saprophytic. These species differ in the composition of the cell wall membrane, which synthesizes various types of active polysaccharides by the expression and regulation of related CesA/Csl genes, while their life forms are divergent via adapting to their specific local environmental conditions (Zhang et al., 2016; Lan et al., 2019; Gao et al., 2020; Idris et al., 2021; Xi et al., 2021).
To date, few studies have conducted evolutionary studies of the gene families related to polysaccharide synthesis in plant congeners with different growth types or life forms regarding their adaptation to environmental stress. However, the available whole-genome sequences of orchids with different life forms provided a unique opportunity to identify and study the adaptive evolution of the CesA/Csl superfamily, which is involved in polysaccharide synthesis. Therefore, we used nine whole-genomes sequenced orchid species with three types of life forms (terrestrial, epiphytic, and saprophytic) and the genomes of Oryza sativa and Arabidopsis thaliana to compare the patterns of gene family divergence and construct the evolutionary pathways of the members of the CesA/Csl superfamily among the three types of life forms. Furthermore, the gene structure related to protein motifs and conserved domains, evolutionary selection pressures, gene collinearity among species were systematically analyzed, and the expression profiles detection and qRT-PCR validation in stem tissues under abiotic stress were conducted, to comprehensively study the adaptive evolution of CesA/Csl superfamily in species with different life forms in the orchid family.
Whole-genome and protein sequences were downloaded from the China National Gene Bank (CNGB),1 National Center for Biotechnology Information (NCBI),2 and RGAP3 databases for the studied Orchidaceae species (D. officinale, D. huoshanense, D. chrysotoxum, P. aphrodite, P. equestris, C. ensifolium, G. elata, V. planifolia, and A. shenzhenic) and O. sativa (Supplementary Table 1). Dendrobium officinale annotation was generated using the GETA annotation pipeline (Supplementary Table 1). The corresponding protein sequences of CesA and Csl were obtained from the Arabidopsis information resource (TAIR) database.4 The RNA-seq raw reads of the 10 species were downloaded from the NCBI sequence read archive (SRA) database5 (Supplementary Table 2).
Two methods were applied to identify the gene members in CesA/Csl superfamily in orchid genomes. First, the hidden Markov model (HMM) profiles of two domains Cellulose_synt (PF03552) and zf-UDP (PF14569) retrieved from the Pfam database were used against the protein database of 11 species using HMMER version 3.0, with a threshold of E < 1e-10 (Sun and Buhler, 2007; Finn et al., 2011). Second, the BLASTP (Mahram and Herbordt, 2015) search was performed using Ces/Csl protein sequences of A. thaliana and O. sativa as queries against the protein database of 11 species with the threshold E < 1e-10, and sequences with identity > 50% were retained. We extracted the common protein sequences identified by both hmmsearch and BLASTP searches and submitted them to the PfamScan website6 for domain alignment. Genes with E < 1e-20 and containing PF03552 and PF14569 domains were finally defined as members of the CesA/Csl family (Supplementary Table 3).
The protein sequences of CesA/Csl genes of the 11 species were used for multiple sequence alignment using the MUSCLE software (Edgar, 2004) with default parameters. The BMGE software (Criscuolo and Gribaldo, 2010) was used to filter non-conserved sequences before tree construction. The phylogenetic tree under the optimal model was constructed using IQtree2 (Minh et al., 2020) with the parameter -m MFP. According to the clustering relationships with A. thaliana and O. sativa, the CesA/Csl gene family members in the nine orchid species were classified into subfamilies, and the CesA/Csl family members of each species were renamed according to the classification of subfamilies.
We used the MEME software7 to predict conserved motifs in the protein sequences of each subfamily. The maximum number of motifs was 20 and the other parameters were default values. Using NCBI conserved domains database,8 the “Pfam–18271 PSSMs” database was selected for conserved domain search for each subfamily. Additionally, we used TBtools (Chen et al., 2020a) to display the distribution and regularity of the protein motifs and conserved domains corresponding to each subfamily.
After pairwise matching of the coding sequences (CDS) of the corresponding subfamilies of nine orchids, the KaKs_Calculator (Zhang, 2022) software was used to calculate the ratio of non-synonymous (Ka) and synonymous substitution rates (Ks) of CesA/Csl genes under different selection pressures; Ka/Ks > 1 represents positive selection, Ka/Ks < 1 denotes negative selection, and Ka/Ks = 1 indicates neutral evolution (Yadav et al., 2015). In addition, McscanX (Wang et al., 2012) was used to calculate inter-species CesA/Csl gene collinearity with a BLASTP threshold E < 1e-5. TBtools (Chen et al., 2020a) was used to display the collinearity of the CesA/Csl genes of A. shenzhenic with other orchid species to determine the contraction and expansion of the gene families.
First, we used fastq-dump (Edwards and Edwards, 2019) to convert RNA-seq raw data (Supplementary Table 2) into fastq format and then used Trimmomatic (Bolger et al., 2014) for quality control of the sequences. The parameters were set as follows: ILLUMINACLIP: TruSeq3-SE:2:30:10, LEADING:3, TRAILING:3, SLIDINGWINDOW:4:15, and MINLEN:36. Thereafter, trim_galore9 was used to remove low-quality reads and linkers with the following parameters: –length, 75; quality, 25; stringency, 5. After indexing the genome and transcripts, the data were aligned to the reference genome using HISAT2 (Guo et al., 2022). The featureCounts software (Liao et al., 2014) was used to calculate the count value of the transcriptome data that matched the genome data. We extracted the length of the gene corresponding to the exon in the corresponding annotation file of each species and associated the total number of reads, count value, and gene length file on the mapping to obtain the FPKM gene expression matrix corresponding to each species. Finally, CesA/Csl gene expression levels in each species were represented in a heatmap to summarize the differences.
Dendrobium officinale, C. ensifolium, and G. elata were selected as representative orchid species for the three life forms (epiphytic, terrestrial, and saprophytic). After treatment with drought (20 days) and freezing (0 and 20°C as control), the stem parts were collected and immediately frozen in liquid nitrogen. Total RNA was extracted from each sample using a TIANGEN polysaccharide and polyphenol plant total RNA extraction kit [Tiangen Biotech (Beijing) Co., Ltd.], and cDNA was synthesized using a TaKaRa PrimeScript™ RT reagent kit with a gDNA Eraser (Perfect Real Time) kit. Primers were designed using the Primer Premier 5 software (Lalitha, 2000; Supplementary Table 5). Real-time quantitative reverse transcription polymerase chain reaction (RT-qPCR) was performed using the TB Green Premix Ex Taq™ II kit (Tli RNase H Plus) and the CFX connect™ Real-Time PCR Detection System. The reaction mixture (20 μl) included 10 μl of TB Green Premix Ex Taq II (Tli RNaseH Plus) (2X), 0.5 μl of PCR Forward Primer (10 μM), 0.5 μl of PCR Reverse Primer (10 μM), 2 μl of fivefold diluted cDNA template, and 7 μl of ddH2O. The reactions were performed according to the following cycling profile: Pre-denaturation at 95°C for 3 min; denaturation at 95°C for 10 s, annealing at 60°C for 30 s, and 40 cycles. Then, the temperature was slowly increased from 65 to 95°C for melting curve analysis. Three technical replicates were performed for each sample. Gene expression levels were calculated using the 2-ΔΔCT method as previously described (Livak and Schmittgen, 2001). We used the GraphPad prism8 software (Swift, 1997) to test the normal distribution of the data. Pairwise t-tests were performed to determine whether the differences in expression were significant.
To identify the CesA/Csl genes extensively, we explored nine orchid genomes using the HMM profile of two domains [Cellulose_synt (PF03552) and zf-UDP (PF14569)] and BLASTP searches using 40 CesA/Csl protein sequences of A. thaliana and 45 CesA/Csl protein sequences of O. sativa as the query. A total of 349 CesA/Csl were identified in nine orchid species, with 51, 40, 40, 32, 36, 48, 24, 45, and 33 CesA/Csl genes in D. officinale, D. huoshanense, D. chrysotoxum, P. aphrodite, P. equestris, C. ensifolium, G. elata, V. planifolia, and A. shenzhenica, respectively (Table 1). While 45 and 40 CesA/Csl genes were identified in O. sativa and A. thaliana, separately. Among all the species, saprophytic orchid G. elata had the lowest (24) and epiphytic orchid D. officinale had the highest (51) number of CesA/Csl genes. The numbers of CesA/Csl genes in D. officinale, C. ensifolium, V. planifolia, and O. sativa were higher than that in A. thaliana, while the numbers of CesA/Csl genes in P. aphrodite, P. equestris, A. shenzhenica, and G. elata were less than that in A. thaliana, and the numbers of CesA/Csl genes in D. huoshanense and D. chrysotoxum were the same as that in A. thaliana. Within each type of the epiphytic, terrestrial, and saprophytic orchids, D. officinale (51), C. ensifolium (48), and G. elata (24) had the highest number of CesA/Csl genes, respectively. The results showed that the number distribution of CesA/Csl genes in the nine orchid species is quite varied and divergent within and among different life forms.
To classify and detect the evolutionary relationship among CesA/Csl genes in Orchidaceae, a phylogenetic tree of CesA/Csl genes among nine orchids species was constructed (Figure 1). Based on the clustering relationships with A. thaliana and O. sativa, the typologies of the phylogenetic tree showed that CesA/Csl genes were classified into nine subfamilies: CesA, CslA, CslB, CslC, CslD, CslE, CslF, CslG, and CslH (Table 1). A comparison of subfamilies among different orchid species showed that the number of CesA genes ranged from the highest number in V. planifolia (15) to the lowest number in P. aphrodite (8). D. officinale had the highest number of each member in CslA (10), CslE (4), and CslG (7) subfamilies; while C. ensifolium had the highest number of each member in CslB (4) and CslH (3) subfamilies. CslH was found to be a unique subfamily in grasses (Farrokhi et al., 2006); however, it was identified in orchid species except for D. huoshanense and G. elata. The number of CslD subfamily members was highest (16) in D. huoshanense and lowest (6) in G. elata. Moreover, the CslF subfamily was lost in all orchid species. The distribution and divergence in the number of CesA/Csl subfamily members suggest that the CesA/Csl genes in the nine species with different life forms had undergone different evolutionary processes in Orchidaceae.
Figure 1. Phylogenetic tree of CesA/Csl superfamily for nine orchid species as well as A. thaliana and O. sativa. CesA, CslA, CslB, CslC, CslD, CslE, CslF, CslG, and CslH are nine subfamilies. The different colored blocks represent the distribution of different subfamilies. The numbers on the branches represent the bootstrap value (60–100).
Totally, 20 motifs and seven domains were identified from the orchid CesA/Csl superfamily (Figure 2, Supplementary Figure 1, and Supplementary Table 4). A comparison of the motifs and conserved domains of orchid proteins revealed that the CesA/Csl genes were conserved within different subfamilies but varied among subfamilies. Among all subfamilies, the types of motifs in CesA and CslD were exactly the same, both of which contained motif 1∼15 and motif 17 with the highest number (16) among all the subfamilies. CesA/CslD both contain common conserved domains Cellulose synt, but with unique domain zf-UDP in CesA and unique domain zf-RING_4 in CslD, respectively. Moreover, there were the same number (8) and types of protein motifs in the CslA and CslC subfamily (Supplementary Table 4). Both CslA and CslC uniquely contained motifs 16, 18, 19, and 20, but lacked 12 motifs (1,4,6,7,8,9,10,11,12,13,14,17), which might lead to the major sequence structural differences of CslA/CslC from other subfamilies (Supplementary Table 4). The conserved domains of both CslA and CslC were Glyco_trans_2_3, Glycos_transf_2, Glyco_tranf_GTA_type, and Glyco_tranf_2_3, all of which were conserved domains of Csl (Figure 2). In the CslB subfamily, there was the lowest number of motifs (4) with Cellulose_syn as a conserved domain. The motif distributions of the CslE, CslG, and CslH subfamilies were varied but with a single conserved Cellulose_syn domain. Overall, the CesA and CslD/E/G/H subfamilies that constituted 69.1% (241/349) of CesA/Csl genes were highly conserved, with 12–17 motifs and conserved Cellulose_synt domain. The CslA and CslC subfamilies accounted for 28.9% (101/349) of the CesA/Csl genes, and contained eight common motifs with four conserved domains. By multiple sequence alignments of the common motifs (motif 3 and motif 5) among subfamilies, it showed that the sequence of motif 3 in CslA/CslC had a larger variation compared with those of CesA/CslD, while the sequence of motif 5 in CslA/CslC had higher variations followed by that in CslE/CslG/CslH, while in CslD and CesA, motif 5 has the lowest variation (Figure 2 and Supplementary Figure 1).
Figure 2. Protein motifs, conserved domains, and amino acid sequences of represented motifs (Motif 3 and Motif 5) corresponding eight CesA/Csl subfamilies in nine orchid species. Nine orchids have 20 types of motifs (Motif 1-Motif 20) and six types conserved domains: Cellulose_synt, Glyco_trans_2_3, Glyco_tranf_2_3, Glyco_tranf_2, Glyco_tranf_GTA_type, and zf_UDP.
The evolutionary selection pressure on CesA/Csl genes of the eight subfamilies among the nine orchid species was analyzed. Among all the gene-pairs comparisons, a total of 17 pairs of CesA/Csl genes with the Ka/Ks ratios > 1 (Figure 3). Five, five, one, two, and four gene pairs with Ka/Ks ratios > 1 were found in the CesA, CslD, CslA, CslE, and CslG subfamilies, respectively, indicating that members of these subfamilies underwent positive selection (Table 2). Among the members of the subfamilies subjected to positive selection, the CesA subfamily in D. huoshanense and D. chrysotoxum experienced the stronger positive selection, while the CslD subfamily in D. officinale had the highest number of positive selective genes. Additionally, the CslG subfamily in D. officinale and D. chrysotoxum experienced stronger positive selection. Moreover, the CslA subfamily in V. planifolia experienced positive selection; The positive selection pressure on the CslE subfamily in P. aphrodite was more than that in C. ensifolium and less than in D. chrysotoxum. The other four subfamilies, CslB, CslC, CslF, and CslH, had Ka/Ks ratios < 1, indicating that the members of these four subfamilies might have undergone purification selection. Overall, among the 17 CesA/Csl gene pairs that had undergone positive selection, 86% belonged to epiphytic orchids, 14% belonged to terrestrial orchids, while the saprophytic orchid G. elata did not undergo positive selection.
Figure 3. The collinearity diagram between A. shenzhenica and each of other eight orchid species. Red lines highlight the homologous gene pairs of CesA/Csl genes, and gray lines represent genome-wide collinear gene pairs.
Analysis of the collinearity relationship showed that the six Ces/Csl genes in the A. shenzhenica genome were collineated with eight homologous genes in the D. chrysotoxum genome (Figure 3). The AsCslD1 gene in the A. shenzhenica genome collineated with three CslD genes in the D. chrysotoxum genome. Three Ces/Csl genes in the A. shenzhenica genome had four homologous genes in the D. huoshanense genome. Furthermore, the five Ces/Csl genes of A. shenzhenica had six homologous genes in the D. officinale genome. Among these, the CslD subfamily had expanded into two genes in D. officinale collineated with the AsCslD1 gene in A. shenzhenica. The four Ces/Csl genes of A. shenzhenica had five homologous genes in the C. ensifolium genome, and the CslD subfamily genes in C. ensifolium had expanded into two genes collineated with AsCslD1. Moreover, three Ces/Csl genes in A. shenzhenica shared three homologous genes in the G. elata genome. P. aphrodite and A. shenzhenica had one pair of Ces/Csl homologous genes, while V. planifolia had four pairs of homologous genes with AsCslC1 and AsCslC3 contracted into one VpCslC4 gene. Taking the CesA/Csl genes in A. shenzhenica as the references, among the corresponding homologous genes, two to three CslD subfamily genes had expanded in epiphytic D. chrysotoxum, D. officinale, D. huoshanense, and terrestrial C. ensifolium. However, CesA/Csl genes did not expand in epiphytic P. aphrodite and saprophytic G. elata, suggesting that the expansion and contraction of the CslD subfamily may be related to adaptive evolution to the local environment of each species during the evolution of different life forms in Orchidaceae.
We observed diversified expression patterns of the identified CesA/Csl genes in the stems tissues of eight orchid species, as well as that of Arabidopsis and O. sativa, using the FPKM expression matrix normalized by the column shown in the heat map (Figure 4 and Supplementary Table 2). The CesA genes of the CesA subfamily were strongly higher expressed among all the subfamilies in seven orchid species with epiphytic and terrestrial life forms. However, the expression level of CesA subfamily genes in saprophytic orchid G. elata exhibited weakly higher expression levels among nine subfamilies. The expression levels of genes in CslA (DoCslA4 and DoCslA9) and CslD (DoCslD11 and DoCslD12) subfamilies were higher among nine subfamilies in epiphytic D. officinale. The genes belonging to the CslC and CslG subfamilies did not show obvious upregulation expression patterns among subfamilies in each orchid species except DhCslG1. The GeCslE1 in the CslE subfamily had the highest expression level among subfamilies in the saprophytic orchid G. elata. The genes in CslH subfamily were higher expressed among subfamilies in species P. equestris and V. planifolia. These results indicate that the CesA/Csl gene family has different biased expression patterns among subfamilies in orchid species, which implies that epiphytic, terrestrial, and saprophytic orchids had distinct adaptive expression regulation patterns related to cellulose synthase when adapting to different environments and stresses, of which CesA, CslA, and CslD subfamilies showed the most varied expression levels.
Figure 4. Heatmap of expression profiles of CesA/Csl genes in stem tissues of nine orchid species. The color bar indicates the expression level (normalized FPKM values) of cellulose synthase genes, with the red color indicating a high expression level, while the blue color represents a low expression level. The gray block indicates NA with no gene. The gene expression was normalized by each column respectively. DOF, D. officinal; DCH, D. chrysotoxum; DHU, D. huoshanense; PAP, P. aphrodite; PEQ, P. equestris; ASH, A. shenzhenica; VPL, V. planifolia; GEL, G. elata; OSA, O. sativa; ATH, A. thaliana.
To detect the changes in expression patterns of CesA/Csl genes in orchids with three different life forms under abiotic stress, three species (D. officinale, C. ensifolium, and G. elata) were selected as representatives of epiphytic, terrestrial, and saprophytic orchids, respectively, and the genes of CesA, CslA, and CslD subfamilies were selected to detect the gene expression changes under drought and freezing stresses in each orchid using real-time reverse transcription quantitative PCR (qRT-PCR; Figure 5 and Supplementary Table 5). The results showed that gene expression patterns in stems were either upregulated or downregulated for orchids with different life forms in response to drought and freezing stress (Figure 5). In the epiphytic orchid D. officinale, the expressions of DoCslA2 and DoCesA2 were upregulated under drought stress (t-test, P < 0.05), whereas the DoCslD6 and DoCslA2 were significantly increased under freezing stress (t-test, P < 0.001). The expression of genes CeCslD8, CeCslA2, CeCslA3, CeCesA2 and CeCesA9 in terrestrial orchid C. ensifolium was significantly increased under drought stress (t-test, P < 0.05), whereas CeCesA9 strongly upregulated (t-test, P = 0.0003), and CeCslD1 and CeCslD8 slight downregulated (t-test, P < 0.05) under freezing stress. In the saprophytic orchid G. elata, the expression levels of GeCslD3, GeCslD4, GeCslA1, and GeCesA9 were significantly upregulations under drought stress (t-test, P < 0.001), and expression levels of almost all genes were significantly decreased under freezing stress except GeCslA5 (t-test, P < 0.05).
Figure 5. Histogram of the expression changes of selected genes of D. officinale, C. ensifolium, and G. elata under drought and low-temperature stress validated by qRT-PCR. (A) Gene expression changes of D. officinale under drought stress (S means normal watering, D means drought stress for 20 days, same below). (B) Gene expression changes of D. officinale under freezing stress (20°C, 0°C, and same below). (C) Gene expression changes of C. ensifolium under drought stress. (D) Gene expression changes of C. ensifolium under freezing stress. (E) Gene expression changes of G. elata under drought stress. (F) Gene expression changes of G. elata under freezing stress. T-tests were performed to determine whether expression changes were significant with ****P ≤ 0.0001; ***P ≤ 0.001; **P ≤ 0.01; *P ≤ 0.05.
Orchidaceae is one of the few families of angiosperms that can successfully colonize terrestrial, epiphytic, or lithophytic ecological niches, such as land, trees, or rocks (Roberts and Dixon, 2008; Givnish et al., 2015, 2016). They are mainly divided into terrestrial, epiphytic, and saprophytic types based on their life forms under different environmental conditions (Cai et al., 2015; Zhang et al., 2016). Polysaccharides are the basic skeleton of plant cell walls and form the first line of defense that determines plant development and growth and helps plants resist adverse stress (Lampugnani et al., 2018; Zhang et al., 2020). Several studies showed that orchids especially those (e.g., Dendrobium orchids) with fleshy stems are rich in various types of active polysaccharides that are related to drought or cold stress adaptation (Xing et al., 2015; Jin et al., 2016; Zhang et al., 2016; Wan et al., 2018). The CesA and Csl genes that synthesize the β-1,4-linked glycan backbone of cellulose and hemicellulose polysaccharides encode enzymes related to cellulose and hemicellulose polysaccharides (Daras et al., 2021). Therefore, the systematic study of the CesA and Csl genes superfamily based on nine published orchid genomes and transcriptomes data has great significance for exploring the distribution and adaptive evolution of CesA/Csl genes among orchids with different life forms.
In this study, we identified 349 CesA/Csl genes in nine whole-genome sequenced species with different life forms (epiphytic, terrestrial, and saprophytic) in Orchidaceae. The evolutionary analysis of nine orchid species showed that CesA/Csl gene family members can be divided into eight subfamilies: CesA, and CslA/B/C/D/E/G/H (Figure 1), among which the CslD subfamily was the largest, followed by CesA, and the number of CslB subfamily members was the lowest, indicating that expansion of the CesA/Csl gene family in orchid species mainly occurred in the CslD subfamily, which is similar to the results of Lan et al. (2019). Although CslF and CslH only exist in grasses, the CslF subfamily was not identified in orchids in this study; however, we found that the CslH subfamily evolved in orchids, thereby adding new information to the evolution of CslH in monocots (Farrokhi et al., 2006). The identification of CesA/Csl gene family members in orchids showed that epiphytic orchid D. officinale contained the highest number of CesA/Csl genes (51), followed by terrestrial orchid C. ensifolium (48) and saprophytic orchid G. elata (24). Because D. officinale is an epiphyte and G. elata is a saprophyte, the different numbers of CesA/Csl genes might be related to the different life forms of orchid species. In addition, the distribution of each subfamily greatly differed in different orchid species. The CesA/Csl genes of D. officinale, D. huoshanense, and V. planifolia had expanded in the CslD subfamily. CslD is involved in the synthesis of xylan and galacturonic acid (Vogel, 2008). The synthesis of cellulose or mannan in the tip growth cells is closely related to the synthesis of metabolic components, such as polysaccharides (Park et al., 2011; Yang et al., 2020). In addition, both D. officinale and D. huoshanense are rich in various active polysaccharide ingredients (Jin et al., 2016). The expansion of CslD genes in these species might have played an important role in the synthesis of active polysaccharides for adapting to different environmental stress.
Analysis of protein motifs and conserved domains showed that the motifs and domains were strongly conserved within subfamilies in orchids. The CesA and CslD/E/G/H subfamilies, which have been found in orchid species were highly conserved, contained 12–16 motifs and Cellulose_synt conserved domains, and were involved in the synthesis of UDP and (1,4-β-D-glucosyl)n + 1 (Glaser, 1958). In addition, the CslA and CslC subfamilies contain eight motifs and four conserved domains (Glyco_trans2_3, Glyco_tranf_2_3, Glyco_tranf_2, and Glyco_tranf_GTA_type), all of which belong to glucosyltransferases involved in the biosynthesis of bacterial capsules (Swartley et al., 1998). Research has shown that the structures of genes in CesA and CslD, CslB, CslE, CslG, and CslH subfamilies are very similar (Daras et al., 2021). The members of CslA and CslC uniquely contained four motifs, but lacked 12 motifs, leading to the major structural differences with the other six CesA/Csl subfamilies, which are consistent with the results obtained in Daras et al. (2021).
Selection pressure analysis revealed that among the 17 CesA/Csl gene pairs that had undergone positive selection in orchids, 86%, 14%, and none belonged to epiphytic, terrestrial, and saprophytic orchids, respectively. Positive selection is one of the bases of adaption for the plant population evolution under local specific environmental conditions (Eyre-Walker, 2006). In our study, the evolutionary effects of positive selection on CesA/Csl were the strongest in epiphytic orchids, followed by the terrestrial orchids, and the weakest in the saprophytic orchids. The CesA/Csl genes of epiphytic orchids might have experienced stronger positive selection under drought and cold stress conditions in epiphytic environments than that in other environmental conditions (Roberts and Dixon, 2008; Givnish et al., 2015, 2016).
The inter-species collinearity analysis showed that the CslD subfamily in epiphytic D. chrysotoxum, D. officinale, and D. huoshanense, as well as terrestrial C. ensifolium, but not in terrestrial P. aphrodite and saprophytic G. elata had expanded into two to three genes collineated with AsCslD1 gene in A. shenzhenica, suggesting that the expansion and contraction of the CslD subfamily might be related to adaptive evolution to environmental stress during the evolution of different life forms in Orchidaceae. Since CslD is located on the Golgi membrane and catalyzes the synthesis of 1,4-β-D-glucomannan from GDP glucose and GDP mannose (Huang et al., 2018; Yang et al., 2020). It is conserved in all terrestrial plants and is involved in various aspects of the plant life cycle, including polysaccharide synthesis (Zhang et al., 2016; Yu et al., 2018; Zhan, 2019), responses to environmental stimuli (Song et al., 2019), cell expansion and division (Yang et al., 2016; Peng et al., 2019), as well as plant development (Bernal et al., 2008; Luan et al., 2011; Yoo et al., 2012). Studies have shown that DcCslDs are differentially expressed in roots, stems, and leaves of D. catenatum using qRT-PCR (Xi et al., 2021). The drought-resistant recovery treatment was closely related to the expression level of DcCslD5, and low-temperature stress significantly affected the expression levels of DcCslD1, DcCslD2a, DcCslD2b, DcCslD3a, and DcCslD5 (Xi et al., 2021). In our study, the CslD gene mainly expanded in epiphytic and partly in terrestrial orchids, but not in saprophytic orchids, which indicated that expansion of the CslD gene, which mainly synthesizes polysaccharides, might have promoted the adaptation of epiphytic orchids to extreme environments under positive selection.
Previous studies showed that the expression levels of CesA/Csl genes in the various tissues are differentiation which is also related to the abiotic stress in different species, such as Arabidopsis, rice, and orchids (Hamann et al., 2004; Kilian et al., 2007; Wang et al., 2010; Zhu et al., 2010; Lan et al., 2019; Gao et al., 2020; Xi et al., 2021). Our transcriptome analysis of eight orchid species (Figure 4) suggested that different CesA/Csl subfamilies with distinctly biased expression patterns in stems might be related to their adaptive ability to different environmental stresses. Further qRT-PCR results showed that the effects of both drought and cold stresses on the expression patterns of CesA genes were different in orchids with different life forms (Figure 5). In epiphytic orchid D. officinale, the CesA/Csl genes responded significantly to both drought and low-temperature stress. The CslA and CesA genes positively responded to drought stress, while the CslD and CslA genes positively responded to freezing stress (Figure 5). In terrestrial orchid C. ensifolium, the CslA and CesA genes were significantly upregulated under drought stress, while only the CeCesA9 gene was significantly upregulated under freezing stress. The expression levels of CslD and CslA genes in saprophytic orchids G. elata were significantly upregulated under drought stress, but strongly downregulated under freezing stress, suggesting that saprophytic orchids were sensitive to both drought and cold stress by either up or down regulations of CesA/Csl genes. A previous study showed that the expression of A. thaliana CslA (CslA7 and CslA10) and CslD (CslD2 and CslD3) is induced under drought, cold, and osmotic stresses (Kilian et al., 2007; Zhu et al., 2010). The expression levels of DcCslD5 are upregulated under drought stress in D. catenatum, while DcCslD1, DcCslD2a, DcCslD2b, DcCslD3a, and DcCslD5 are influenced strongly by low temperature (Xi et al., 2021). Gao et al. (2020) showed that the promoter region of CslA genes contains cis-elements related to hormonal regulation, which play an important role in biosynthesis and accumulation of glucomannan that responds to stress in D. catenatum. Under different stress treatments, low temperatures induced the expression of DcCslA5 and inhibited the expression of DcCslA3 (Gao et al., 2020). Combining with previous studies as well as our studies of gene expressions and positive selection, we proposed that the CslA might be a key subfamily in orchids with different life forms in response to drought stress, while the CslD might be a key subfamily in epiphytic and saprophytic orchids to adapt to freezing stress.
In this study, 349 CesA/Csl genes were identified in nine orchid species with different life forms (epiphytic, terrestrial, and saprophytic) in Orchidaceae. The CesA/Csl superfamily in orchids was divided into eight subfamilies: CesA and CslA/B/C/D/E/G/H with conserved motifs and domains. Totally, 17 pairs of CesA/Csl homologous genes underwent positive selection, of which 86%, 14%, and none belonged to the epiphytic, terrestrial, and saprophytic orchids, respectively. Epiphytic orchids experienced greater strength of positive selection, with expansion events mostly related to the CslD subfamily, which might have resulted in strong adaptability to stress in epiphytes. Epiphytic, terrestrial, and saprophytic orchids had different adaptive expression patterns. CslA might be a key subfamily in orchids with different life forms in response to drought stress, whereas the CslD subfamily might be a key subfamily in epiphytic and saprophytic orchids to adapt to freezing stress. The novel findings in the study will provide the basic resource for further research on the adaptive evolution of the CesA/Csl superfamily in angiosperms, as well as the orchid-specific functional genes related to life-history trait evolution.
The datasets presented in this study can be found in online repositories. The names of the repository/repositories and accession number(s) can be found in the article/Supplementary material.
XM conceived the project and designed the experiments. JW, JL, WL, BD, LL, and XL carried out the experiments. JW and XM analyzed the data and wrote the manuscript. QH and KL visualized the experimental results. XM, DQ, BH, and MF revised the manuscript. All authors read and approved the final manuscript.
This work was supported by the National Natural Science Foundation of China (Project No. 31700199) to XM.
The authors declare that the research was conducted in the absence of any commercial or financial relationships that could be construed as a potential conflict of interest.
All claims expressed in this article are solely those of the authors and do not necessarily represent those of their affiliated organizations, or those of the publisher, the editors and the reviewers. Any product that may be evaluated in this article, or claim that may be made by its manufacturer, is not guaranteed or endorsed by the publisher.
The Supplementary Material for this article can be found online at: https://www.frontiersin.org/articles/10.3389/fpls.2022.994679/full#supplementary-material
Ai, Y., Li, Z., Sun, W.-H., Chen, J., Zhang, D., Ma, L., et al. (2021). The Cymbidium genome reveals the evolution of unique morphological traits. Hortic. Res. 8:255. doi: 10.1038/s41438-021-00709-6
Bernal, A. J., Yoo, C.-M., Mutwil, M., Jensen, J. K., Hou, G., Blaukopf, C., et al. (2008). Functional analysis of the cellulose synthase-like genes CSLD1, CSLD2, and CSLD4 in tip-growing Arabidopsis cells. Plant Physiol. 148, 1238–1253. doi: 10.1104/pp.108.121939
Bolger, A. M., Lohse, M., and Usadel, B. (2014). Trimmomatic: A flexible trimmer for Illumina sequence data. Bioinformatics 30, 2114–2120. doi: 10.1093/bioinformatics/btu170
Burton, R. A., Wilson, S. M., Hrmova, M., Harvey, A. J., Shirley, N. J., Medhurst, A., et al. (2006). Cellulose synthase-like CslF genes mediate the synthesis of cell wall (1, 3; 1, 4)-ß-D-glucans. Science 311, 1940–1942. doi: 10.1126/science.1122975
Cai, J., Liu, X., Vanneste, K., Proost, S., Tsai, W.-C., Liu, K.-W., et al. (2015). The genome sequence of the orchid Phalaenopsis equestris. Nat. Genet. 47, 65–72. doi: 10.1038/ng.3149
Chao, Y. T., Chen, W. C., Chen, C. Y., Ho, H. Y., Yeh, C. H., Kuo, Y. T., et al. (2018). Chromosome-level assembly, genetic and physical mapping of Phalaenopsis aphrodite genome provides new insights into species adaptation and resources for orchid breeding. Plant Biotechnol. J. 16, 2027–2041. doi: 10.1111/pbi.12936
Chen, C., Chen, H., Zhang, Y., Thomas, H. R., Frank, M. H., He, Y., et al. (2020a). TBtools: An integrative toolkit developed for interactive analyses of big biological data. Mol. plant. 13, 1194–1202. doi: 10.1016/j.molp.2020.06.009
Criscuolo, A., and Gribaldo, S. (2010). BMGE (Block Mapping and Gathering with Entropy): A new software for selection of phylogenetic informative regions from multiple sequence alignments. BMC Evol. Biol. 10:210. doi: 10.1186/1471-2148-10-210
Daras, G., Templalexis, D., Avgeri, F., Tsitsekian, D., Karamanou, K., and Rigas, S. (2021). Updating insights into the catalytic domain properties of plant cellulose synthase (CesA) and Cellulose synthase-like (Csl) Proteins. Molecules 26:4335. doi: 10.3390/molecules26144335
Doblin, M. S., Pettolino, F. A., Wilson, S. M., Campbell, R., Burton, R. A., Fincher, G. B., et al. (2009). A barley cellulose synthase-like CSLH gene mediates (1, 3; 1, 4)-β-D-glucan synthesis in transgenic Arabidopsis. Proc. Natl. Acad. Sci. 106, 5996–6001. doi: 10.1073/pnas.0902019106
Edgar, R. C. (2004). MUSCLE: Multiple sequence alignment with high accuracy and high throughput. Nucleic Acids Res. 32, 1792–1797. doi: 10.1093/nar/gkh340
Edwards, J. A., and Edwards, R. A. (2019). Fastq-pair: Efficient synchronization of paired-end fastq files. bioRxiv [Preprint]. 2019:552885. doi: 10.1101/552885
Eyre-Walker, A. (2006). The genomic rate of adaptive evolution. Trends Ecol. Evol. 21, 569–575. doi: 10.1016/j.tree.2006.06.015
Farrokhi, N., Burton, R. A., Brownfield, L., Hrmova, M., Wilson, S. M., Bacic, A., et al. (2006). Plant cell wall biosynthesis: Genetic, biochemical and functional genomics approaches to the identification of key genes. Plant Biotechnol. J. 4, 145–167. doi: 10.1111/j.1467-7652.2005.00169.x
Finn, R. D., Clements, J., and Eddy, S. R. (2011). HMMER web server: Interactive sequence similarity searching. Nucleic Acids Res. 39, W29–W37. doi: 10.1093/nar/gkr367
Gao, Y.-Q., Chen, X.-L., Chen, D.-H., Liu, J.-J., and Si, J.-P. (2020). Genome-wide identification and expression analysis of CSLA gene family of Dendrobium catenatum. China J. Chin. Mater. Med. 45, 3120–3127. doi: 10.19540/j.cnki.cjcmm.20200506.103
Givnish, T. J., Spalink, D., Ames, M., Lyon, S. P., Hunter, S. J., Zuluaga, A., et al. (2016). Orchid historical biogeography, diversification, Antarctica and the paradox of orchid dispersal. J. Biogeograph. 43, 1905–1916. doi: 10.1111/jbi.12854
Givnish, T. J., Spalink, D., Ames, M., Lyon, S. P., Hunter, S. J., Zuluaga, A., et al. (2015). Orchid phylogenomics and multiple drivers of their extraordinary diversification. Proc. R. Soc. B Biol. Sci. 282:20151553. doi: 10.1098/rspb.2015.1553
Glaser, L. (1958). The synthesis of cellulose in cell-free extracts of Acetobacter xylinum. J. Biol. Chem. 232, 627–636. doi: 10.1016/S0021-9258(19)77383-9
Guo, J., Gao, J., and Liu, Z. (2022). HISAT2 parallelization method based on spark cluster. J. Phys. Conf. Ser. 2179:012038. doi: 10.1088/1742-6596/2179/1/012038
Hamann, T., Osborne, E., Youngs, H. L., Misson, J., Nussaume, L., and Somerville, C. (2004). Global expression analysis of CESA and CSL genes in Arabidopsis. Cellulose 11, 279–286. doi: 10.1023/B:CELL.0000046340.99925.57
Han, B., Jing, Y., Dai, J., Zheng, T., Gu, F., Zhao, Q., et al. (2020). A Chromosome-level genome assembly of Dendrobium huoshanense using long reads and Hi-C data. Genome Biol. Evol. 12, 2486–2490. doi: 10.1093/gbe/evaa215
Hasing, T., Tang, H., Brym, M., Khazi, F., Huang, T., and Chambers, A. H. (2020). A phased Vanilla planifolia genome enables genetic improvement of flavour and production. Nat. Food. 1, 811–819. doi: 10.1038/s43016-020-00197-2
Huang, Y.-P., He, T.-B., Cuan, X.-D., Wang, X.-J., Hu, J.-M., and Sheng, J. (2018). 1, 4-β-d-Glucomannan from Dendrobium officinale activates NF-êB via TLR4 to regulate the immune response. Molecules 23:2658. doi: 10.3390/molecules23102658
Idris, N. A., Aleamotuá, M., McCurdy, D. W., and Collings, D. A. (2021). The orchid velamen: A model system for studying patterned secondary cell wall development? Plants 10:1358. doi: 10.3390/plants10071358
Jin, Q., Jiao, C., Sun, S., Song, C., Cai, Y., Lin, Y., et al. (2016). Metabolic analysis of medicinal Dendrobium officinale and Dendrobium huoshanense during different growth years. PLoS One. 11:e0146607. doi: 10.1371/journal.pone.0146607
Kilian, J., Whitehead, D., Horak, J., Wanke, D., Weinl, S., Batistic, O., et al. (2007). The AtGenExpress global stress expression data set: Protocols, evaluation and model data analysis of UV-B light, drought and cold stress responses. Plant J. 50, 347–363. doi: 10.1111/j.1365-313X.2007.03052.x
Kim, S.-J., Chandrasekar, B., Rea, A. C., Danhof, L., Zemelis-Durfee, S., Thrower, N., et al. (2020). The synthesis of xyloglucan, an abundant plant cell wall polysaccharide, requires CSLC function. Proc. Natl. Acad. Sci. 117, 20316–20324. doi: 10.1073/pnas.2007245117
Lalitha, S. (2000). Primer premier 5. Biotech. Softw. Int. Rep Comput. Softw. J. Sci. 1, 270–272. doi: 10.1089/152791600459894
Lampugnani, E. R., Khan, G. A., Somssich, M., and Persson, S. (2018). Building a plant cell wall at a glance. J. Cell Sci. 131:jcs207373. doi: 10.1242/jcs.207373
Lan, X., Hu, S., Feng, L., Tian, Y., and He, F. (2019). Bioinformatic analysis of the cellulose synthase supergene family in Dendrobium candidum. J. Hen. Agric. Sci. 48, 49–55.
Leitch, I., Kahandawala, I., Suda, J., Hanson, L., Ingrouille, M. J., Chase, M., et al. (2009). Genome size diversity in orchids: Consequences and evolution. Ann. Bot. 104, 469–481. doi: 10.1093/aob/mcp003
Lerouxel, O., Cavalier, D. M., Liepman, A. H., and Keegstra, K. (2006). Biosynthesis of plant cell wall polysaccharides—a complex process. Curr. Opin. Plant Biol. 9, 621–630. doi: 10.1016/j.pbi.2006.09.009
Liao, Y., Smyth, G. K., and Shi, W. (2014). featureCounts: An efficient general purpose program for assigning sequence reads to genomic features. Bioinformatics 30, 923–930. doi: 10.1093/bioinformatics/btt656
Little, A., Schwerdt, J. G., Shirley, N. J., Khor, S. F., Neumann, K., O’Donovan, L. A., et al. (2018). Revised phylogeny of the cellulose synthase gene superfamily: Insights into cell wall evolution. Plant Physiol. 177, 1124–1141. doi: 10.1104/pp.17.01718
Livak, K. J., and Schmittgen, T. D. (2001). Analysis of relative gene expression data using real-time quantitative PCR and the 2- ΔΔCT method. Methods 25, 402–408. doi: 10.1006/meth.2001.1262
Luan, W., Liu, Y., Zhang, F., Song, Y., Wang, Z., Peng, Y., et al. (2011). OsCD1 encodes a putative member of the cellulose synthase-like D sub-family and is essential for rice plant architecture and growth. Plant Biotechnol. J. 9, 513–524. doi: 10.1111/j.1467-7652.2010.00570.x
Mahram, A., and Herbordt, M. C. (2015). NCBI BLASTP on high-performance reconfigurable computing systems. ACM Trans. Reconfigurable Technol. Syst. (TRETS). 7, 1–20. doi: 10.1145/2629691
Marks, R. A., Hotaling, S., Frandsen, P. B., and VanBuren, R. (2021). Representation and participation across 20 years of plant genome sequencing. Nat. plants 7, 1571–1578. doi: 10.1038/s41477-021-01031-8
Mattana, M., Biazzi, E., Consonni, R., Locatelli, F., Vannini, C., Provera, S., et al. (2005). Overexpression of Osmyb4 enhances compatible solute accumulation and increases stress tolerance of Arabidopsis thaliana. Physiol. Plant 125, 212–223. doi: 10.1111/j.1399-3054.2005.00551.x
Minh, B. Q., Schmidt, H. A., Chernomor, O., Schrempf, D., Woodhams, M. D., Von Haeseler, A., et al. (2020). IQ-TREE 2: New models and efficient methods for phylogenetic inference in the genomic era. Mol. Biol. Evol. 37, 1530–1534. doi: 10.1093/molbev/msaa015
Park, S., Szumlanski, A. L., Gu, F., Guo, F., and Nielsen, E. (2011). A role for CSLD3 during cell-wall synthesis in apical plasma membranes of tip-growing root-hair cells. Nat. Cell Biol. 13, 973–980. doi: 10.1038/ncb2294
Peng, X., Pang, H., Abbas, M., Yan, X., Dai, X., Li, Y., et al. (2019). Characterization of Cellulose synthase-like D (CSLD) family revealed the involvement of PtrCslD5 in root hair formation in Populus trichocarpa. Sci. Rep. 9, 1–9. doi: 10.1038/s41598-018-36529-3
Pérez-Silva, A., Nicolás-García, M., Petit, T., Dijoux, J. B., de los Ángeles Vivar-Vera, M., Besse, P., et al. (2021). Quantification of the aromatic potential of ripe fruit of Vanilla planifolia (Orchidaceae) and several of its closely and distantly related species and hybrids. Eur. Food Res. Technol. 247, 1489–1499. doi: 10.1007/s00217-021-03726-w
Ranwala, A. P., and Miller, W. B. (2008). Analysis of nonstructural carbohydrates in storage organs of 30 ornamental geophytes by high-performance anion-exchange chromatography with pulsed amperometric detection. New Phytol. 180, 421–433. doi: 10.1111/j.1469-8137.2008.02585.x
Richmond, T. A., and Somerville, C. R. (2000). The cellulose synthase superfamily. Plant Physiol. 124, 495–498. doi: 10.1104/pp.124.2.495
Roberts, D. L., and Dixon, K. W. (2008). Orchids. Curr. Biol. 18, R325–R329. doi: 10.1016/j.cub.2008.02.026
Rosa, M., Prado, C., Podazza, G., Interdonato, R., González, J. A., Hilal, M., et al. (2009). Soluble sugars: Metabolism, sensing and abiotic stress: A complex network in the life of plants. Plant Signal. Behav. 4, 388–393. doi: 10.4161/psb.4.5.8294
Sarkar, P., Bosneaga, E., and Auer, M. (2009). Plant cell walls throughout evolution: Towards a molecular understanding of their design principles. J. Exp. Bot. 60, 3615–3635. doi: 10.1093/jxb/erp245
Song, X., Xu, L., Yu, J., Tian, P., Hu, X., Wang, Q., et al. (2019). Genome-wide characterization of the cellulose synthase gene superfamily in Solanum lycopersicum. Gene 688, 71–83. doi: 10.1016/j.gene.2018.11.039Get
Sun, Y., and Buhler, J. (2007). Designing patterns for profile HMM search. Bioinformatics 23, e36–e43. doi: 10.1093/bioinformatics/btl323
Sun, Y., Shang, L., Zhu, Q.-H., Fan, L., and Guo, L. (2021). Twenty years of plant genome sequencing: Achievements and challenges. Trends Plant Sci. 27, 391–401. doi: 10.1016/j.tplants.2021.10.006
Suzuki, S., Li, L., Sun, Y.-H., and Chiang, V. L. (2006). The cellulose synthase gene superfamily and biochemical functions of xylem-specific cellulose synthase-like genes in Populus trichocarpa. Plant Physiol. 142, 1233–1245. doi: 10.1104/pp.106.086678
Swartley, J. S., Liu, L.-J., Miller, Y. K., Martin, L. E., Edupuganti, S., and Stephens, D. S. (1998). Characterization of the gene cassette required for biosynthesis of the (α1 → 6)-linked N-acetyl-d-mannosamine-1-phosphate capsule of serogroup A Neisseria meningitidis. J. Bacteriol. 180, 1533–1539. doi: 10.1128/JB.180.6.1533-1539.1998
Swift, M. L. (1997). GraphPad prism, data analysis, and scientific graphing. J. Chem. Inform. Comput. Sci. 37, 411–412. doi: 10.1021/ci960402j
Verhertbruggen, Y., Yin, L., Oikawa, A., and Scheller, H. V. (2011). Mannan synthase activity in the CSLD family. Plant Signal. Behav. 6, 1620–1623. doi: 10.4161/psb.6.10.17989
Vogel, J. (2008). Unique aspects of the grass cell wall. Curr. Opin. Plant Biol. 11, 301–307. doi: 10.1016/j.pbi.2008.03.002
Wan, X., Zou, L.-H., Zheng, B.-Q., Tian, Y.-Q., and Wang, Y. (2018). Transcriptomic profiling for prolonged drought in Dendrobium catenatum. Sci. Data 5, 1–9. doi: 10.1038/sdata.2018.233
Wang, L., Guo, K., Li, Y., Tu, Y., Hu, H., Wang, B., et al. (2010). Expression profiling and integrative analysis of the CESA/CSL superfamily in rice. BMC Plant Biol. 10:282. doi: 10.1186/1471-2229-10-282
Wang, Y., Tang, H., DeBarry, J. D., Tan, X., Li, J., Wang, X., et al. (2012). MCScanX: A toolkit for detection and evolutionary analysis of gene synteny and collinearity. Nucleic Acids Res. 40, e49–e49. doi: 10.1093/nar/gkr1293
Xi, H., Liu, J., Li, Q., Chen, X., Liu, C., Zhao, Y., et al. (2021). Genome-wide identification of Cellulose-like synthase D gene family in Dendrobium catenatum. Biotechnol. Biotechnol. Equip. 35, 1163–1176. doi: 10.1080/13102818.2021.1941252
Xing, X., Cui, S. W., Nie, S., Phillips, G. O., Goff, H. D., and Wang, Q. (2015). Study on Dendrobium officinale O-acetyl-glucomannan (Dendronan§): Part II. Fine structures of O-acetylated residues. Carbohydr. Polym. 117, 422–433. doi: 10.1016/j.carbpol.2014.08.121
Xu, Y., Lei, Y., Su, Z., Zhao, M., Zhang, J., Shen, G., et al. (2021). A chromosome-scale Gastrodia elata genome and large-scale comparative genomic analysis indicate convergent evolution by gene loss in mycoheterotrophic and parasitic plants. Plant J. 108, 1609–1623. doi: 10.1111/tpj.15528
Yadav, C. B., Bonthala, V. S., Muthamilarasan, M., Pandey, G., Khan, Y., and Prasad, M. (2015). Genome-wide development of transposable elements-based markers in foxtail millet and construction of an integrated database. DNA Res. 22, 79–90. doi: 10.1093/dnares/dsu039
Yang, J., Bak, G., Burgin, T., Barnes, W. J., Mayes, H. B., Peña, M. J., et al. (2020). Biochemical and genetic analysis identify CSLD3 as a beta-1, 4-glucan synthase that functions during plant cell wall synthesis. Plant Cell 32, 1749–1767. doi: 10.1105/tpc.19.00637
Yang, W., Schuster, C., Beahan, C. T., Charoensawan, V., Peaucelle, A., Bacic, A., et al. (2016). Regulation of meristem morphogenesis by cell wall synthases in Arabidopsis. Curr. Biol. 26, 1404–1415. doi: 10.1016/j.cub.2016.04.026
Yin, Y., Huang, J., and Xu, Y. (2009). The cellulose synthase superfamily in fully sequenced plants and algae. BMC Plant Biol. 9:99. doi: 10.1186/1471-2229-9-99
Yoo, C.-M., Quan, L., and Blancaflor, E. B. (2012). Divergence and redundancy in CSLD2 and CSLD3 function during Arabidopsis thaliana root hair and female gametophyte development. Front. Plant Sci. 3:111. doi: 10.3389/fpls.2012.00111
Yu, Z., He, C., da Silva, J. A. T., Luo, J., Yang, Z., and Duan, J. (2018). The GDP-mannose transporter gene (DoGMT) from Dendrobium officinale is critical for mannan biosynthesis in plant growth and development. Plant Sci. 277, 43–54. doi: 10.1016/j.plantsci.2018.07.021
Zhan, Z.-G. (2019). Research progress on genomics, transcriptomics and functional genes of Dendrobium officinale. Chin. Tradit. Herb. Drugs 2019, 3979–3989.
Zhang, G.-Q., Xu, Q., Bian, C., Tsai, W.-C., Yeh, C.-M., Liu, K.-W., et al. (2016). The Dendrobium catenatum Lindl. genome sequence provides insights into polysaccharide synthase, floral development and adaptive evolution. Sci. Rep. 6, 1–10. doi: 10.1038/srep19029
Zhang, G.-Q., Liu, K.-W., Li, Z., Lohaus, R., Hsiao, Y.-Y., Niu, S.-C., et al. (2017). The Apostasia genome and the evolution of orchids. Nature 549, 379–383. doi: 10.1038/nature23897
Zhang, W.-J., Wang, S., Kang C-z, Lv C-g, Zhou, L., Huang, L.-Q., et al. (2020). Pharmacodynamic material basis of traditional Chinese medicine based on biomacromolecules: A review. Plant Methods 16:26. doi: 10.1186/s13007-020-00571-y
Zhang, Y., Zhang, G.-Q., Zhang, D., Liu, X.-D., Xu, X.-Y., Sun, W.-H., et al. (2021). Chromosome-scale assembly of the Dendrobium chrysotoxum genome enhances the understanding of orchid evolution. Hortic. Res. 8:183. doi: 10.1038/s41438-021-00621-z
Zhang, Z. (2022). KaKs_Calculator 3.0: Calculating selective pressure on coding and non-coding sequences. Genom. Proteom. Bioinform. doi: 10.1016/j.gpb.2021.12.002
Keywords: orchids, epiphyte, terrestrial, saprophytic, CesA/Csl, life-history, expression patterns
Citation: Wang J, Li J, Lin W, Deng B, Lin L, Lv X, Hu Q, Liu K, Fatima M, He B, Qiu D and Ma X (2022) Genome-wide identification and adaptive evolution of CesA/Csl superfamily among species with different life forms in Orchidaceae. Front. Plant Sci. 13:994679. doi: 10.3389/fpls.2022.994679
Received: 15 July 2022; Accepted: 26 August 2022;
Published: 29 September 2022.
Edited by:
Liangsheng Zhang, Zhejiang University, ChinaReviewed by:
Shi Yan, Fujian Medical University, ChinaCopyright © 2022 Wang, Li, Lin, Deng, Lin, Lv, Hu, Liu, Fatima, He, Qiu and Ma. This is an open-access article distributed under the terms of the Creative Commons Attribution License (CC BY). The use, distribution or reproduction in other forums is permitted, provided the original author(s) and the copyright owner(s) are credited and that the original publication in this journal is cited, in accordance with accepted academic practice. No use, distribution or reproduction is permitted which does not comply with these terms.
*Correspondence: Dongliang Qiu, cWl1ZGwxOTcwQGZhZnUuZWR1LmNu; Xiaokai Ma, bWFlcXV1c0AxMjYuY29t
†These authors have contributed equally to this work
Disclaimer: All claims expressed in this article are solely those of the authors and do not necessarily represent those of their affiliated organizations, or those of the publisher, the editors and the reviewers. Any product that may be evaluated in this article or claim that may be made by its manufacturer is not guaranteed or endorsed by the publisher.
Research integrity at Frontiers
Learn more about the work of our research integrity team to safeguard the quality of each article we publish.