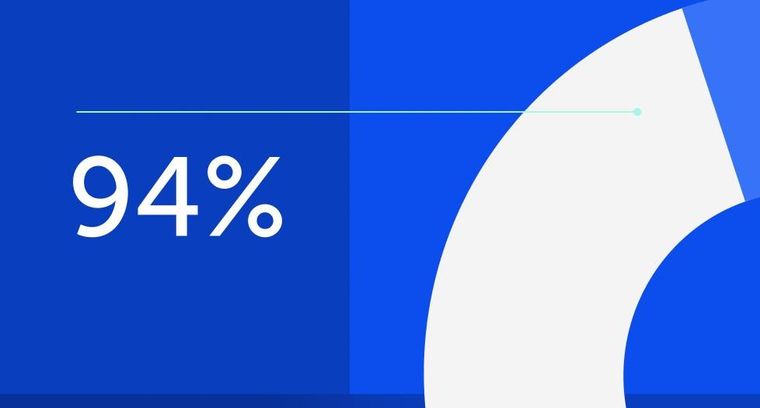
94% of researchers rate our articles as excellent or good
Learn more about the work of our research integrity team to safeguard the quality of each article we publish.
Find out more
REVIEW article
Front. Plant Sci., 01 November 2022
Sec. Plant Abiotic Stress
Volume 13 - 2022 | https://doi.org/10.3389/fpls.2022.994149
This article is part of the Research TopicRising Stars in Plant ROS/Redox Biology Under Abiotic Stress ConditionsView all 7 articles
Nitric oxide (NO), an ancient molecule with multiple roles in plants, has gained momentum and continues to govern plant biosciences-related research. NO, known to be involved in diverse physiological and biological processes, is a central molecule mediating cellular redox homeostasis under abiotic and biotic stresses. NO signaling interacts with various signaling networks to govern the adaptive response mechanism towards stress tolerance. Although diverging views question the role of plants in the current greenhouse gases (GHGs) budget, it is widely accepted that plants contribute, in one way or another, to the release of GHGs (carbon dioxide (CO2), methane (CH4), nitrous oxide (N2O) and ozone (O3)) to the atmosphere, with CH4 and N2O being the most abundant, and occur simultaneously. Studies support that elevated concentrations of GHGs trigger similar signaling pathways to that observed in commonly studied abiotic stresses. In the process, NO plays a forefront role, in which the nitrogen metabolism is tightly related. Regardless of their beneficial roles in plants at a certain level of accumulation, high concentrations of CO2, CH4, and N2O-mediating stress in plants exacerbate the production of reactive oxygen (ROS) and nitrogen (RNS) species. This review assesses and discusses the current knowledge of NO signaling and its interaction with other signaling pathways, here focusing on the reported calcium (Ca2+) and hormonal signaling, under elevated GHGs along with the associated mechanisms underlying GHGs-induced stress in plants.
Nitric oxide (NO) was first described as nitrous air by Joseph Priestly in 1772 (Yu et al., 2014). However, NO production in plants was reported for the first time by Klepper (1979) about 43 years ago. Since then, our understanding of the diverse roles of NO in plant physiology and biology has increased significantly (Kolbert et al., 2019). The discovery of NO as a signaling molecule revealed novel facets of free radicals from their previous portrait as toxic by-products of oxidative metabolism to central regulators of diverse plant metabolic pathways. Unlike in animals where it is well established that a family of NO synthase (NOS) enzymes are the primary source of NO, the presence of such NOS enzyme in plants remains obscure and controversial. Whereas, some lines of evidence reported NOS-like activity in plants (del Rıo et al., 2004; Domingos et al., 2015; Phillips et al., 2018; Singh et al., 2021). During the last two decades, NO has gained momentum due to its multiple roles in plant growth and development (Sanz et al., 2015). As per some evidence (Arc et al., 2013; Signorelli & Considine, 2018), NO attenuates dormancy, while promoting seed germination, in crosstalk with the abscisic acid (ABA) signaling pathway. Similarly, several studies support that NO is a key player in the control of cell proliferation via a functional interaction with cytokinin (Shen et al., 2013). NO also plays an important role in the cell cycle (Correa-Aragunde et al., 2006; Novikova et al., 2017) and auxin-mediated activation of cell division (Ötvös et al., 2005). In the same way, Sánchez-Vicente et al. (2021) indicated that NO altered the pattern of auxin maxim and PIN-FORMED1 (regulates auxin basipetal transport) during shoot development. In addition, it is well established that NO is a key signaling molecule during abiotic or biotic stress conditions in plants (Magalhaes et al., 1999; Hancock & Neill, 2019; Singh et al., 2021). Available data suggest that NO generation in plants occurs by at least eight prominent processes that include enzymatic and nonenzymatic (Khan et al., 2014; Kolbert et al., 2019; Hussain et al., 2022).
Carbon dioxide (CO2), methane (CH4), nitrous oxide (N2O), and ozone (O3) play important roles in plant physiology. CO2 is required for photosynthesis and is fixed to store energy in the form of carbohydrates (Blankenship, 2021). Although the utilization of CO2 can be affected by factors such as light, water, nutrition, humidity and temperature, the atmospheric CO2 concentration has a greater influence. The increase in CO2 level has been shown to result in increased growth rate and biomass production (Ainsworth et al., 2004; Thompson et al., 2017). From another perspective, Sigurdsson (2001) reported variabilities in the response of plants to elevated CO2. Similarly, CH4, previously considered a physiological inert gas, is currently emerging as a signaling molecule that would interact with reactive oxygen (ROS) or nitrogen (RNS) species during abiotic or biotic stress events (Li et al., 2020; Wang et al., 2020). In the same way, N2O, of which the molecular mechanism underlying its production has been widely investigated, was reported to be produced in the mitochondria of plants from NO. In the soil, N2O is formed during the nitrification and denitrification processes (Lenhart et al., 2019; Timilsina et al., 2020a). Meanwhile, O3 causes both beneficial outcomes for plants and the environment (Pasqualini et al., 2009; Wargent & Jordan, 2013; Mukherjee, 2022; Yin et al., 2022), and oxidative stress, which may result in cell death (Riehl Koch et al., 1998; Rao & Davis, 2001). Although CO2, CH4, N2O, and O3 play beneficial roles in plant physiology and biology, these molecules have been identified as potent greenhouse gases (GHGs) (Khalil & Aslam, 2009; Timilsina et al., 2020b; Timilsina et al., 2022). However, in terms of global warming potential (GWP), N2O and CH4 come on top with a GWP of 300 times and 25 times, respectively, greater than that of CO2 in the atmosphere.
Studies show that high amounts of atmospheric CO2 (Niu et al., 2011), CH4 (Li et al., 2020), N2O, and O3 (Sharma & Davis, 1995) trigger various signaling cascades that serve as messengers to activate the adequate defense system to tackle the stress. During these events, plants enhance the production of ROS, such as hydrogen peroxide (H2O2), superoxide anion (), hydroxyl radical (·OH−), singlet oxygen (1O2), and RNS (NO, peroxynitrite (ONOO−), etc.). In the process, NO signaling has proven essential and plays a central role. NO interacts with other signaling pathways, and the results of this interaction confer beneficial outcomes for plants. Generally, ROS and RNS are produced by plants under normal conditions and are harmless at low concentrations. However, upon stress induction by either abiotic stimuli or living organisms, the production of ROS and RNS increases up to the point of causing oxidative or nitro-oxidative stress, which may result in oxidative damage and culminate in cell death. To alleviate the detrimental effects of ROS or RNS overproduction, plants activate antioxidant (enzymatic and non-enzymatic) systems and induce several stress-responsive genes as part of the adaptive response mechanisms toward stress tolerance. This review assesses the current knowledge of the regulatory role of NO in plants under elevated CO2, CH4, N2O, or O3. This work also highlights the crosstalk between NO signaling and the above-mentioned potent GHGs, as well as the causative effects of elevated CO2, CH4, N2O, or O3 on NO production and signaling events. Likewise, we discuss the possible interplay between CO2, CH4, or N2O-induced stress with NO and other stress signaling pathways in plants to maintain a balanced reduction-oxidation status.
In the current era identified as the Anthropocene, CO2 is the most important GHG (considering its emission abundance) emitted globally through human activities (Lashof and Ahuja, 1990; Butler and Montzka, 2016; Liu et al., 2020). Studies investigating NO signaling or biosynthesis in plants increased with climate change, of which the impact on the five critical dimensions of the sustainable development goals (SGDs, also known as the 5Ps: people, planet prosperity, peace and patnership) is no longer to be demonstrated. Like in the event of drought (Xiong et al., 2012; Lau et al., 2021), salinity (Bhardwaj et al., 2021; Fatima et al., 2021), heat stress (Kong et al., 2012; Parankusam et al., 2017), flooding (Khan et al., 2019; Da-Silva and do Amarante, 2022; Park et al., 2022), or heavy metal toxicity (Saxena and Shekhawat, 2013; Cerana and Malerba, 2015), elevated CO2 level triggers NO production and activates NO signaling in plants. Under these conditions, several stress-responsive pathways, including hormonal, Ca2+ (Besson-Bard et al., 2008; Niu et al., 2011) are induced, during which process NO plays a preponderant role. Niu et al. (2011) observed that elevated CO2 caused an increase in carbohydrates production, which in turn activated the auxin or ethylene-related signal transduction pathways that subsequently induced the production of endogenous NO.
Carbon dioxide supplementation enhances the root and shoot growth, resulting in the rapid growth of plants. This could be explained, in part, by the greater uptake of nutrients from soil mediated by the enhanced root development (Yue et al., 2009; Thompson et al., 2017). Under these conditions, available nutrients can be exhausted rapidly in the soil and plants may experience nutrient shortage or deficiency at their advanced growth stage. To sustain an increased growth rate under high CO2 conditions, plants will require higher amounts of inorganic nutrients, including nitrogen. To compensate the gap created due to nutrient deficiency, mineral fertilizers are applied (Wong, 1979; Tissue et al., 1997; Stitt and Krapp, 1999). However, excessive N-rich fertilizer applications cause an increase in CH4 and N2O production (Takeda et al., 2022; Timilsina et al., 2020a; Xu et al., 2016). Nitrogen is the most abundantly used essential macronutrient in agriculture. Nitrogen is available to the plant as nitrate (NO3) and NH4, with NO3 being the major form of nitrogen taken up by plants. The efficiency of nitrogen use by plants is mediated by several genes encoding nitrate reductase (NR) or belonging to five distinct high-, dual-, or low-affinity NO3 transporters protein families, including NRT1, NRT2, chloride channel (CLC), and slow anion channel-associated/slow anion channel-associated homologs (SLAC/SLAH) (Buchanan et al., 2015). In higher plants, NR is the first enzyme and the rate-limiting factor in the NO3 assimilation pathway (Buchanan et al., 2015). NR undergoes changes under elevated CO2 as reported by Stitt and Krapp (1999). As previously reported, elevated CO2 increases the use efficiency of organic nitrogen (NUE) (Wong, 1979; Hocking and Meyer, 1991a; Hocking and Meyer, 1991b; Pettersson et al., 1993; Rogers et al., 1993; McKee and Woodward, 1994). This could be partially explained by the low NO3 and NH4 contents (Purvis et al., 1974; Hocking and Meyer, 1985; Hocking and Meyer, 1991b; Yelle et al., 1987).
As per some evidence, the activity of NR varies across plant species, and different conditions under the influence of NO (Du et al., 2016; Adavi and Sathee, 2019). Thus, plants exposed to elevated CO2 recorded differential patterns, including an increase (Geiger et al., 1998; Constable et al., 2001; Hofmann et al., 2013; Du et al., 2016), a decrease (Stitt and Krapp, 1999; Matt et al., 2001), or unaffected (Agüera et al., 2006; Natali et al., 2009) NR activity. Interestingly, a study conducted by Frungillo et al. (2014) revealed that S-nitrosothiols regulate NO production and storage in plants through the nitrogen assimilation pathway. The authors emphasized that although NO production is mediated by various enzymatic and nonenzymatic pathways (including L-arginine), some amount of NO2 reduced from NO3 in the nitrogen metabolism is transported to the chloroplast. There, NO2 is converted to NH4 for further incorporation into amino acids, as part of the assimilation process. Another fraction of NO2 is converted to NO in the cytosol (Zheng et al., 2013).
In higher plants, S-nitrosoglutathione (GSNO, a stable, mobile, less toxic form of NO) is the major cellular reservoir of NO, and its accumulation is controlled by GSNO reductase (GSNOR1 that negatively regulates the process of protein S-nitrosation, thus controlling endogenous NO levels). GSNOR catalyzes the reduction (irreversible reaction) of GSNO to glutathione disulfide (GSSG, the oxidized glutathione form of GSH) and ammonia (NH3) (Yun et al., 2011; Medina-Rivera et al., 2015; Jahnová et al., 2019; Khajuria et al., 2019; Hurali et al., 2022). It was reported that GSNO exerts an inhibitory effect on NO3 uptake and reduction, which would occur via the inhibition of NR activity. Similarly, GSNOR catalyzes the reaction of NO with GSH (the reduced glutathione) (Feechan et al., 2005; Rustérucci et al., 2007). According to Chaki et al. (2009), the activity of GSNOR helps balance the cellular (RNS) reduction-oxidation (redox) homeostasis under various stressful conditions. Likewise, NO, which is one of the end products of the nitrogen metabolism, inhibits the activity of GSNOR. In turn, the latter prevents the degradation of GSNO. Therefore, building on the above mechanism, Frungillo et al. (2014) suggested that NO feedback regulates its flux via the assimilation of nitrogen by controlling its bioavailability and modulating its own consumption; knowing that high amounts of NO and its derived SNO result from the N assimilation pathway.
Plant hormones are key players in plant growth and development, operating either antagonistically or in synergy with one another. Plant hormones are also well-known for their involvement in signaling events during abiotic or biotic stress. For instance, salicylic acid (SA) signaling regulates plant response to stress (Khan et al., 2015). Other studies demonstrated that the interplay between NO and SA signaling pathways activates appropriate defense mechanisms and enhances resistance to a wide range of plant pathogens (Klessig et al., 2000). According to Li et al. (2019), SA acts upstream of NO under elevated CO2, which in turn mediates the induction of flavonoid biosynthesis in tea (Comellia sinensis L.). The authors observed that SA, NO, and flavonoid contents increased in plants exposed to elevated CO2.
Stomata are epidermal pores through which gas exchange is regulated, including CO2 assimilation. Several studies support that the regulation of stomatal aperture size is achieved through a complex sensory and signaling network (Kim et al., 2010; Merilo et al., 2014). Stomata aperture facilitates more CO2 uptake, which enhances photosynthesis (a process by which CO2 is captured from the atmosphere and is converted to sugar as a source of energy for plant cell, growth and development, as well as plant fitness). It is widely known that plant guard cells are essential for photosynthesis and transpiration, and the stomata aperture is sensitive to environmental stimuli. Recent progress in stress signaling revealed that high levels of CO2-induced stomata closure suggest ROS such as hydrogen peroxide (H2O2), as key factors. ROS are major regulators of stomatal conductance in response to internal or external stress inducers (Sun et al., 2021). Pharmacological and genetic studies showed that NADPH oxidases and cell wall peroxidases-mediated ROS regeneration participate in elevated CO2-induced stomatal closure. Whereas, elevated CO2-mediated inhibition of light-induced stomatal opening would rely on ROS derived from NADPH oxidases, and not from cell wall peroxidases (He et al., 2020). From another perspective, Wang et al. (2015) demonstrated that NR and NOS-like enzymes are involved in CO2-induced NO accumulation in plants, under which conditions the regulation of stomata and photosynthesis are differentially affected. In a converse approach, Cornic (2000) observed the inhibition of photosynthesis by a decrease in stomatal aperture, as a result of a decrease in CO2 acquisition. In the same way, Garcia-Mata and Lamattina (2007) reported that light-induced stomatal opening via calcium (Ca2+) and NO-mediated signaling pathways is inhibited by ABA. The authors suggested that NO and Ca2+ are active components of ABA-induced stomatal closure. Similarly, Chater et al. (2015) suggested that ABA synthesis and signaling are required during elevated CO2-induced stomatal regulation.
Moreover, Hsu et al. (2018) supported that elevated CO2-mediated stomatal closure via an ABA-independent pathway would involve the OST1/SnRK2 (OPEN STOMATA 1/SNF1-related protein kinases 2) kinases family. In addition, studies have suggested that the signaling pathway for elevated CO2-induced stomatal closure shares several elements with but does not overlap with (Hu et al., 2010; Tian et al., 2015), the ABA signaling pathway in guard cells. Such induction of OST1 and its target SLOW-TYPE ANION CHANNEL 1 (SLAC1, also known as one of the five major NO3 transporter protein families), ROS and NO production, enhanced Ca2+ level (Webb et al., 1996; Xue et al., 2011; Shi et al., 2015; Geng et al., 2017). Lines of evidence demonstrated that NO acts as an important secondary messenger in guard cells during stomatal closure (Gayatri et al., 2013). As for Sami et al. (2021), NO enhances the photosynthetic efficiency, among other plant metabolisms. This evidence would be attributed to the increase in biomass, and greater leaf area, causing an increase in productivity (Lawlor and Mitchell, 1991). As for Haworth et al. (2016), elevated CO2 would cause a reduction in photosynthetic physiology in plants. A study conducted by De Souza et al. (2008) supported that elevated CO2 levels (720 ppm) increased photosynthesis in sugarcane plants compared to those grown under ambient (370 ppm) CO2 level. This evidence was supported in several CO2-enrichment studies (Lawlor and Mitchell, 1991). As illustrated in Figure 1, we have proposed a signaling model involving NO and other signaling pathways in plants exposed to high concentrations of CO2.
Figure 1 Illustration of elevated carbon dioxide (CO2)-mediated NO signaling in plants. Unlike other abiotic stresses, elevated CO2 levels have been shown to have both beneficial and detrimental effects on plant growth and development. Beneficial effects are resumed in enhancing the photosynthetic capacity and promotion of growth and development. However, at a certain accumulation level, CO2 induces various signaling cascades, in which NO is said to play a central role. In the process, nitrate reductase and transporter encoding genes are induced, along with the Ca2+ and ABA signaling, ROS and/or RNS signaling, resulting in differential stomatal conductance, enhancement of NO3 uptake and assimilation, and eventually better growth and productivity. Continuous black lines with an arrow indicate positive regulation or induction, while dash red lines with a perpendicular bar denote negative regulation or inhibition in case of over production/accumulation of compounds.
In plants, the cytoskeleton (a structure made up of filamentous proteins responsible for the morphology and intracellular organization of the cell) provides mechanical support to the cell and enables the cell to execute essential functions (Kost and Chua, 2002; Hawkins et al., 2013). The actin cytoskeleton plays a fundamental role in diverse biological processes in plants, such as cell division and expansion, organelle movement, vesicle trafficking, and the establishment of polar cell growth (Kost and Chua, 2002; Paez-Garcia et al., 2018). Drøbak et al. (2004) supported that, in addition to its role in maintaining cell shape and structure, the actin cytoskeleton and its associated elements serve as a key target in various signaling events, as well as a signal transducer. Furthermore, the actin cytoskeleton has been identified as a major target and an effector of various signaling cascades in plants, including Ca2+, mitogen-activated protein kinase/kinase (MAPK/MAPKK) signaling, phytohormone signaling, etc. (Hussey et al., 2006; Wang et al., 2011). Evident findings revealed that the reorganization of cytoskeleton components under stress conditions is regarded as a crucial cell survival response (Wang and Hussey, 2015; Soda et al., 2016a; Sampaio et al., 2022). As indicated in previous paragraphs, high CO2 levels-mediated changes in the stomatal conductance require ABA and ABA signaling (Chater et al., 2015). Stomatal movement is regarded as a means used by plants to increase their adaptability to environmental change. A recent review report discussing the progress on the dynamics of actin filaments and microtubules in the guard cell (Li et al., 2022) revealed that the cytoskeleton is an important factor influencing stomatal conductance. This implication would occur through the change in turgor pressure guard cells. Studies highlight that the shape, structure, and mechanics of guard cells are affected by cytoskeleton-mediated cell division and cell wall synthesis (Panteris et al., 2018; Woolfenden et al., 2018; Muroyama et al., 2020).
Advances in the molecular mechanism underlying NO signaling in plants revealed that NO regulates the function of target proteins through post-translational modifications (PTMs), including tyrosine nitration or S-nitrosation previously referred to as S-nitrosylation (Yemets et al., 2011), thus affecting their activity. Likewise, evidence support that the cytoskeleton is involved in the NO-signaling network (directly or indirectly); while, NO-mediated regulation of cytoskeleton functions occurs through PTMs. Meanwhile, Kasprowicz et al. (2009) found that the organization of the actin cytoskeleton is modulated via NO levels in a cell-type-specific fashion. Findings from diverse studies sustain the existence of a tight association between changes in atmospheric CO2 levels and structural adaptation in plants. Although studies present diverging views on the actual effects of high CO2 levels on photosynthesis, evidence revealed the alteration of plant structure by impairing the rate of cell division, cell expansion, and cell cycling due to high CO2 concentrations. Under these circumstances, metabolic changes could be induced at the cellular level (Masle, 2000; Sharma et al., 2014). Given the role of the cytoskeleton in the stress signaling events as portrayed earlier, coupled with the interplay between NO signaling and the cytoskeleton function in plants, it has become evident that the cytoskeleton plays a crucial role in the adaptive response against abiotic stimuli (Soda et al., 2016b), including elevated CO2.
Depicting the mechanisms underlying plants response to abiotic stress helps improve the understanding of genetic factors associated with stress tolerance in plants. Although investigating the role of each signaling molecule independently provides some useful information on their level of implication in the regulatory mechanisms during abiotic stress events, a genome-wide approach gives new insights on the possible interactions between signaling molecules and their impact at the whole plant level. Generally, signaling pathways do not operate solo. Rather, they are activated along with other signaling networks with which they may establish a certain level of interaction. This interaction may occur in a balanced way since it is a high-energy demanding process consisting of antagonistic or synergetic relationships. This may result in what could be referred to as signaling cascades, allowing the induction or suppression of downstream adaptive response mechanisms. Just like other stress-related conditions where NO plays an active role (Ma et al., 2012; Niu et al., 2017; Sun et al., 2017; Khan et al., 2020), a study conducted by Wang et al. (2013a) revealed crosstalk between NO and Ca2+ signaling (also known as a secondary messenger) during episodes of elevated CO2, which led to enhanced lateral root development. The authors equally indicated that a CO2-mediated increase in NO production triggers the accumulation of cytosolic Ca2+ that acts as a co-factor for NO. In a converse approach, Young et al. (2006) investigated the CO2 signaling in guard cells and indicated that low–high CO2 transitions modulate the cytosolic Ca2+ transient patterns.
Methane (CH4) was previously regarded as a physiologically inert gas but is now emerging as a possible signaling molecule in plants (Li et al., 2020). Some lines of evidence suggest that there could be an interaction between CH4 and ROS, as well as other signaling molecules such as NO, GSH, and hydrogen sulfide (H2S) (Figure 2). Li et al. (2020) indicated that CH4 production occurs through abiotic or biotic pathways. The latter is proposed to be the major pathway of CH4 production through the decomposition of organic compounds as well as microbial activity (Emmanuel and Ague, 2007; Fiebig et al., 2009; Wang et al., 2013b). CH4 exerts a protective effect through the reduction of oxidative stress (Wang, 2014). Similarly, Hu et al. (2018) observed that CH4 delayed post-harvest senescence by re-establishing redox homeostasis. Likewise, Wang et al. (2009) indicated that physically injured plants- and hypoxic conditions-mediated generation of ROS stimulated CH4 emission. These facts nourish the idea that the production of CH4 by plants would be part of a survival strategy during stress conditions. Zhang et al. (2018) exposed Mung bean plants to polyethylene glycol-induced osmotic stress and discovered that NO contributed to CH4-induced osmotic stress tolerance. In a converse approach, Qi et al. (2017) investigated the role of CH4 in inducing the development of adventitious roots in cucumber found that CH4 triggers accumulation NO. The interaction between NO and CH4 has recently been reported (Boros and Keppler, 2019). From another perspective, Zhang et al. (2018) reported that exogenous CH4 triggered NO production under polyethylene glycol-induced osmotic stress, and alleviated the inhibition of seed germination. Therefore, this evidence suggests that CH4-induced abiotic stress tolerance would be NO-dependent, which might involve NR and NOS-like protein.
Figure 2 Methane induces nitric oxide accumulation in plants. High concentration of methane in the atmosphere or within the plant triggers activation of various signaling and biosynthetic pathways, including NO. NO requires Ca2+, among others, as cofactors to govern the adaptive response mechanisms towards abiotic stress in plants. The crosstalk between NO and Ca2+ along with other signaling cascades helps the plant to activate the appropriate defense system to tackle the stress, by inducing related stress-responsive genes and accumulation of various antioxidant systems that detoxify the effect of reactive oxygen (ROS) and nitrogen (RNS) species over accumulation. Persistent over production of ROS and/or RNS leads to oxidative stress/damage that may culminate to cell death and plant necrosis. Continuous black lines with an arrow indicate positive regulation or induction, while dash red line with a perpendicular bar denote negative regulation or inhibition.
Studies aiming at elucidating the unexplored facets of NO in plants discuss the possible interplay between NO and oxygen (O2) availability in plants. It is believed that NH4 and NO3 are the major sources of nitrogen for plants, with NO3 being the most abundant. O2 differentially influences GHGs emission patterns, acting on the activity of specific soil microorganisms, such as methanogens and methanotrophs. In addition, as indicated in previous paragraphs, the influential role of NO on nitrogen assimilation events positions this molecule at the core center of interest in various stress-related studies. It was reported that NO contributes to CH4-mediated induction of osmotic stress tolerance in mung bean (Zhang et al., 2018). Cucumber adventitious rooting was reported to be induced by CH4-rich water via heme oxygenase/carbon monoxide and Ca2+ pathways.
Nitrous oxide (N2O) is a potent GHG with a GWP much higher than CO2 and CH4. N2O is emitted by diffusion from soil or via plant transpiration (Chang et al., 1998). The release of N2O to the atmosphere by plants occurs during the nitrification and denitrification processes. The latter are mediated by soil microbial activities in the soil as well as in the shoot of plants via the action of certain enzymes of the nitrogen metabolism, in which NO takes an active part (Timilsina et al., 2020a). It is established that NO3 is a precursor for N2O formation in both plants (Hakata et al., 2003; Lenhart et al., 2019) and soil (Andrew et al., 2012). In their study, Burlacot et al. (2020) found that the conversion of NO to N2O in Chlamydomonas reinhardtii (Alga) occurs during the photosystem I (PSI)-dependent photoreduction of the photosynthesis. This was similarly observed by Schützenmeister et al. (2020). Meanwhile, Gupta et al. (2005) observed that in higher plants, only root mitochondria, but not leaf mitochondria reduce nitrite to NO. Both N2O and NO are known as NOx, and react with volatile organic compounds and hydroxyl, resulting in organic NO3 and nitric acid (HNO3) formation (Cassia et al., 2018). Unlike in animal and prokaryotic organisms (Arai et al., 2003), the molecular mechanism underlying N2O-induced stress in plants has not been elucidated. Nevertheless, considering the NO-dependent N2O formation, an imbalance in NO synthesis or signaling would affect N2O production in plants.
Like during other abiotic stresses, when plants are exposed to ozone (O3), they undergo various physiological and biochemical changes. In the process, various signaling pathways are activated and act as messengers to trigger an adequate adaptive response mechanism towards stress tolerance, along with the induction or suppression of stress-related genes (Delaney et al., 1994; Sharma and Davis, 1995; Pasqualini et al., 2009). Under the same conditions, antioxidant systems are activated (Nogués et al., 2008; Caregnato et al., 2010), including GSH and ascorbate-GSH cycle (Zhang et al., 2020). The molecular basis of O3-induced stress signals in plants has been widely investigated, of which an SA-dependent signaling pathway was reported to be activated in plants exposed to O3 (Sharma and Davis, 1997). As indicated in previous paragraphs, GSNOR catalyzes the reaction of NO and GSH. A study conducted by Alscher and Hess (1993) sustained that O3 treatment affects the glutathione metabolism in plants. Similarly, Gupta et al. (1991) observed an overtime increase in GSH, GSSG, and total GSH levels in poplar upon O3 treatment. As to Guri (1983), a correlation exists between differential O3 sensitivity and accumulation levels of GSH and the activity GR in tolerant Phaseolus vulgaris cultivars.
Furthermore, a study revealed that dual application of NO and O3 induced a large set of stress-responsive genes, therefore suggesting their possible interplay (Ahlfors et al., 2009a). In the same way, Ahlfors et al. (2009) demonstrated that O3-mediated NO accumulation coincided with the hypersensitive response (HR) in Arabidopsis, followed by O3-mediated induction of SA biosynthesis and signaling pathway genes, and ethylene accumulation. Interestingly, Xu et al. (2012) supported that NO3 reductase is responsible for O3-triggered NO generation and secondary metabolites in Ginkgo biloba plants. Moreover, useful information that would allow enhancing our understanding of the molecular mechanism underlying O3-mediating induction of various signaling pathways and ROS in plants is well summarized by Hasan et al. (2021), who highlighted the role of key protein families associated with the nitrogen metabolism, such as slow anion channel 1 (SLAC1) and that of diverse phytohormone signaling pathways, which regulate stomatal conductance. Likewise, Domingos et al. (2015) highlighted the multidimensional roles of NO in gas signaling in plants. In a recent study, Mukherjee (2022) emphasized the forefront role of NO in mediating O3-induced stress signaling in plants.
Moreover, land plants rely on light for photosynthesis, and leaves serve as light-capturing organs. However, as an undesired corollary, they also absorb damaging light, especially ultraviolet B (UV-B) radiation (280–320 nm waveband of the solar irradiation) (Gupta et al., 2011), a kind of UV light that directly affects plants and microorganisms, and alters the species-specific interactions (Vanhaelewyn et al., 2020), and causes cell death in plants (Nawkar et al., 2013), which was manifested by cell shrinkage, condensation of chromatin in perinuclear areas, and formation of micronuclei in UV-B treated BY-2 tobacco cells (Lytvyn et al., 2010). On the one hand, exposure to UV-B radiation has been shown to impair the genome stability of plants by damaging nucleic acids (Ries et al., 2000; Tanaka et al., 2002). The good news is that the O3 layer in the stratosphere helps absorb UV-B radiation. However, in modern times, exposure to UV-B radiation increases gradually due to the thinning of the protective O3 layer caused by human activities (Andrady et al., 2010). Plants have evolved sophisticated strategies to adapt to the incidence of UV-B light, such as increasing leaf thickness, UV-B reflective properties, and the cellular levels of UV-B absorbing metabolites. In the UV-B signal transduction network, members of the bZIP (basic leucine zipper) transcription factor (TF) family, ELONGATED HYPOCOTYL5 (HY5, a key effector of the UV RESISTANCE LOCUS 8 (UVR8)) and HY5-HOMOLOG (HYH) that mediate several photomorphogenic pathways, and the E3-ubiquitin ligases (COP1) are required for UV-B-induced gene expression (Oravecz et al., 2006; Tohge et al., 2011). Reports indicate that low doses of UV-B induce distinct signaling pathways from the high doses-stress response pathways (Frohnmeyer et al., 1999; Jenkins et al., 2001; Brown et al., 2005). In addition to UV-B radiation, Danon and Gallois (1998) investigated the molecular response of Arabidopsis thaliana plants exposed to UV-C radiation (10–50 kJ/m2), and observed the induction of an oligonucleosomal DNA fragmentation, which characterizes apoptotic-like changes in the nucleus, similar to that observed in human cells (Martin & Cotter, 1991). Reports have shown that UV-C (below 280 nm) is not physiologically relevant to plants because it is effectively intercepted by the earth’s stratosphere (Brash, 1997); however, UV-C radiation yields similar DNA photoproducts to that obtained with UV-B radiation, which reaches the surface of the earth. Thus, several studies employed UV-C radiation to explorer potential DNA damages. In this context, Danon et al. (2004) observed that overexposure of Arabidopsis plants to UV-C radiation induced programmed cell death (PCD), the latter being suggested to be mediated by Caspase-like activities, which in turn would modulate DNA fragmentation. In the process, Caspase inhibitors suppress DNA fragmentation and cell death, where two AtDAD1 and AtDAD2, earlier identified as homologs of Defender against Apoptotic Death-1, are proposed to suppress the onset of DNA fragmentation while supporting an involvement of the endoplasmic reticulum in this form of the plant PCD pathway.
On the other hand, UV radiation triggers the production of free radicals, including ROS and NO (Tossi et al., 2009; Zhang et al., 2011), as well as the counteracting plant defense antioxidants such as ascorbate and glutathione (Jansen et al., 1998; Santa-Cruz et al., 2014). The involvement of NO signaling in mediating plant response to UV-B-induced oxidative stress has been proposed. In this regard, Lytvyn et al. (2016) highlighted the multiple functions of inositol biosynthesis in plants exposed to UV-B. Their study revealed that the response mechanism to NO-dependent oxidative stress induction in Arabidopsis involves the inositol-3-phosphate synthase (IPS1), a key enzyme for biosynthesis of myo-inositol and its derivatives. Other studies showed that the role of ROS are important elements of a wide signaling web that composes with other signaling mediators to activate cellular protective mechanisms in response to UV-B radiation. For instance, Arabidopsis plants treated with the NO scavenger PTIO (2-phenyl-4,4,5,5-tetramethylimidazolin-L-oxyl-3-oxide) and/or with L-NAME (NG-monomethyl-L-arginine), an NOS (NO synthase) inhibitor, suppressed the induction of chalcone synthase (CHS)-encoding gene; therefore suggesting that UV-B-triggered the expression of CHS would require NO (Soheila et al., 2001). One should keep in mind that the presence of NOS in planta remains obscure. In another perspective, Tossi et al. (2014) showed that the Arabidopsis UVR8 photoreceptor regulates plants’ response to UV-B-induced stomatal closure in a NO-dependent manner. Likewise, Wu et al. (2016) revealed that the induction of anthocyanin under UV-B radiation is regulated by the interaction between H2O2, NO and UVR8 in radish. Similarly, Cassia et al. (2019) proposed that UV-B triggers the accumulation of ABA, which increases the production of H2O2 and NO. In the process, the UV-B receptor UVR8 is activated. The latter is stabilized by endogenous NO followed by the induction of HY5 TF. In turn, HY5 TF has the potential to regulate the expression and activity of NR, as well as CHs and chalcone isomerase (CHI) resulting in a downstream increase in flavonoid and anthocyanin contents capable of absorbing UV-B radiation and scavenging ROS.
Nitric oxide (NO) is at the core center of interest in many biosciences and environmental-related research programs, mainly due to its involvement in almost all physiological and biological processes in plants. Since the initial report of NO in plants about four decades ago, our understanding of the molecular mechanism underlying NO biosynthesis and signaling in plants increased during the last two decades, and the physiology of NO has been widely investigated under both normal and stressful conditions. In this work, we assessed and presented the current knowledge on the regulatory network involving NO and its derived molecules in the adaptive response mechanism of plants towards elevated CO2, CH4, N2O, O3 or UV light. This review also gives insights into the interaction of NO with other signaling pathways and highlights the involvement of the nitrogen metabolism and the flavonoid pathway genes in NO-mediated stress signaling during elevated GHGs. These GHGs present both beneficial outcomes and detrimental effects to the plant, depending on their level of accumulation in the atmosphere. Therefore, taking advantage of the current understanding of NO in its diverse dynamic roles in various stressful conditions, NO can be regarded as a game changer in the efforts towards the mitigation of the impact of climate change, while providing a novel path to enhancing the resilience of agricultural and food production systems. In the context of climate change, depicting the molecular basis of NO-mediated plants’ response to elevated CO2, CH4, N2O, or O3 would help elucidate the regulatory mechanisms underlying plants’ response to these GHGs. In addition, exploring the interplay between NO-mediated nutrient acquisition and use efficiency, and the associated defense mechanisms against GHGs would provide more insights towards a paradigm shift for a more resilient agriculture.
NK and B-GM designed the work, conducted the literature mining, and drafted the paper, S-ML, YK, DL, and G-ML contributed to the literature review and manuscript preparation. B-WY and J-HL conceived, revised and edited the manuscript and supervised the project. All authors contributed to the article and approved the submitted version.
This work was supported by the Cooperative Research Program for Agriculture, Science and Technology Development (Project No. PJ01707301) and “2019KoRAA Long-Term Training Program” of the Rural Development Administration, Korea, and the Basic Science Research Program through the National Research Foundation of Korea (NRF) funded by the Ministry of Education (Grant No.2020R1I1A3073247).
The authors declare that the research was conducted in the absence of any commercial or financial relationships that could be construed as a potential conflict of interest.
All claims expressed in this article are solely those of the authors and do not necessarily represent those of their affiliated organizations, or those of the publisher, the editors and the reviewers. Any product that may be evaluated in this article, or claim that may be made by its manufacturer, is not guaranteed or endorsed by the publisher.
Adavi, S. B., Sathee, L. (2019). Elevated CO2-induced production of nitric oxide differentially modulates nitrate assimilation and root growth of wheat seedlings in a nitrate dose-dependent manner. Protolasma 256 (1), 147–159. doi: 10.1007/s00709-018-1285-2
Agüera, E., Ruano, D., Cabello, P., de la Haba, P. (2006). Impact of atmospheric CO2 on growth, photosynthesis and nitrogen metabolism in cucumber (Cucumis sativus l.) plants. J. Plant Physiol. 163 (8), 809–817. doi: 10.1016/j.jplph.2005.08.010
Ahlfors, R., Brosché, M., Kangasjär, J. (2009a). Ozone and nitric oxide interaction in arabidopsis thaliana, a role for ethylene? a role for ethylene? Plant Signal. Behav. 4 (9), 878–879. doi: 10.4161/psb.4.9.9428
Ahlfors, R., Brosché, M., Kollist, H., Kangasjärvi, J. (2009b). Nitric oxide modulates ozone-induced cell death, hormone biosynthesis and gene expression in Arabidopsis thaliana. Plant J. 58 (1), 1–12. doi: 10.1111/j.1365-313X.2008.03756.x
Ainsworth, E. A., Rogers, A., Nelson, R., Long, S. P. (2004). Testing the “source–sink” hypothesis of down-regulation of photosynthesis in elevated [CO2] in the field with single gene substitutions in Glycine max. Agric. For. Meteo. 122 (1-2), 85–94. doi: 10.1016/j.agrformet.2003.09.002
Alscher, R. G., Hess, J. L. (1993). Antioxidants in higher plants Vol. 31 (Florida, USA: CRC Press), 58.
Andrady, A., Aucamp, P. J., Bais, A. F., Ballare, C. L., Björn, L., Bornman, J. F., et al. (2010). Environmental effects of ozone depletion and its interactions with climate change: progress report 2009. Phytochem. Photobiol. Sci. 9 (3), 275–294. doi: 10.1039/b923342n
Andrew, J., Georgios, G., Jules, P., Baggs, M., Richardson, J. (2012). Biological sources and sinks of nitrous oxide and strategies to mitigate emissions. Phil. Trans. R. Soc Biol. Sci. 367 (1593), 1157–1168. doi: 10.1098/rstb.2011.0415
Arai, H., Mizutani, M., Igarashi, Y. (2003). Transcriptional regulation of the NOS genes for nitrous oxide reductase in Pseudomonas aeruginosa. Microbiol 149 (1), 29–36. doi: 10.1099/mic.0.25936-0
Arc, E., Galland, M., Godin, B., Cueff, G., Rajjou, L. (2013). Nitric oxide implication in the control of seed dormancy and germination. Front. Plant Sci. 4. doi: 10.3389/fpls.2013.00346
Besson-Bard, A., Courtois, C., Gauthier, A., Dahan, J., Dobrowolska, G., Jeandroz, S., et al. (2008). Nitric oxide in plants: production and cross-talk with Ca2+ signaling. Mol. Plant 1 (2), 218–228. doi: 10.1093/mp/ssm016
Bhardwaj, S., Kapoor, D., Singh, S., Gautam, V., Dhanjal, D. S., Jan, S., et al. (2021). Nitric oxide: A ubiquitous signal molecule for enhancing plant tolerance to salinity stress and their molecular mechanisms. J. Plant Growth Reg. 40, 1–13. doi: 10.1007/s00344-021-10394-3
Blankenship, R. E. (2021). Molecular mechanisms of photosynthesis (New York, NY, USA: John Wiley & Sons).
Boros, M., Keppler, F. (2019). Methane production and bioactivity-a link to oxido-reductive stress. Front. Physiol. 1244. doi: 10.3389/fphys.2019.01244
Brash, D. E. (1997). Sunlight and the onset of skin cancer. Trend. Genet. 13 (10), 410–414. doi: 10.1016/S0168-9525(97)01246-8
Brown, B. A., Cloix, C., Jiang, G. H., Kaiserli, E., Herzyk, P., Kliebenstein, D. J., et al. (2005). A UV-b-specific signaling component orchestrates plant UV protection. Proceed. Nat. Acad. Sci. 102 (50), 18225–18230. doi: 10.1073/pnas.050718710
Buchanan, B. B., Gruissem, W., Jones, R. L. (2015). Biochemistry and molecular biology of plants (New York, NY, USA: John Wiley & sons).
Burlacot, A., Richaud, P., Gosset, A., Li-Beisson, Y., Peltier, G. (2020). Algal photosynthesis converts nitric oxide into nitrous oxide. Proceed. Nat. Acad. Sci. 117 (5), 2704–2709. doi: 10.1073/pnas.1915276117
Butler, J. H., Montzka, S. A. (2016). The NOAA annual greenhouse gas index (AGGI). NOAA Earth Syst. Res. Lab. 58.
Caregnato, F. F., Clebsch, C. C., Rocha, R. F., Feistauer, L. B., Oliveira, P. L., Divan Junior, A. D., et al. (2010). Ozone exposure differentially affects oxidative stress parameters in distinct Phaseolus vulgaris l. varieties. J. Plant Inter. 5 (2), 111–115. doi: 10.1080/17429140903518455
Cassia, R., Amenta, M., Fernández, M. B., Nocioni, M., Dávila, V. (2019). “The role of nitric oxide in the antioxidant defense of plants exposed to UV-b radiation,” in Reactive oxygen, nitrogen and sulfur species in plant: Production, metabolism, signaling and defense mechanisms (New York, NY, USA:John Wiley & Sons), 555–572. doi: 10.1002/9781119468677
Cassia, R., Nocioni, M., Correa-Aragunde, N., Lamattina, L. (2018). Climate change and the impact of greenhouse gasses: CO2 and NO, friends and foes of plant oxidative stress. Front. Plant Sci. 9. doi: 10.3389/fpls.2018.00273
Cerana, R., Malerba, M. (2015). Role of nitric oxide in heavy metal stress, in nitric oxide action in abiotic stress responses in plants (Cham, Switzerland: Springer), 181–192. doi: 10.1007/978-3-319-17804-2_12
Chaki, M., Fernández-Ocaña, A. M., Valderrama, R., Carreras, A., Esteban, F. J., Luque, F., et al. (2009). Involvement of reactive nitrogen and oxygen species (RNS and ROS) in sunflower–mildew interaction. Plant Cell Physiol. 50 (2), 265–279. doi: 10.1093/pcp/pcn196
Chang, C., Janzen, H., Nakonechny, E., Cho, C. (1998). Nitrous oxide emission through plants. Soil Sci. Soc Am. J. 62 (1), 35–38. doi: 10.2136/sssaj1998.03615995006200010005x
Chater, C., Peng, K., Movahedi, M., Dunn, J. A., Walker, H. J., Liang, Y.-K., et al. (2015). Elevated CO2-induced responses in stomata require ABA and ABA signaling. Curr. Biol. 25 (20), 2709–2716. doi: 10.1016/j.cub.2015.09.013
Constable, J. V., Bassirirad, H., Lussenhop, J., Zerihun, A. (2001). Influence of elevated CO2 and mycorrhizae on nitrogen acquisition: contrasting responses in pinus taeda and liquidambar styraciflua. Tree Physiol. 21 (2–3), 83–91. doi: 10.1093/treephys/21.2-3.83
Cornic, G. (2000). Drought stress inhibits photosynthesis by decreasing stomatal aperture–not by affecting ATP synthesis. Trend. Plant Sci. 5 (5), 187–188. doi: 10.1016/S1360-1385(00)01625-3
Correa-Aragunde, N., Graziano, M., Chevalier, C., Lamattina, L. (2006). Nitric oxide modulates the expression of cell cycle regulatory genes during lateral root formation in tomato. J. Exp. Bot. 57 (3), 581–588. doi: 10.1093/jxb/erj045
Danon, A., Gallois, P. (1998). UV-C radiation induces apoptotic-like changes in Arabidopsis thaliana. FEBS let. 437 (1-2), 131–136. doi: 10.1016/S0014-5793(98)01208-3
Danon, A., Rotari, V. I., Gordon, A., Mailhac, N., Gallois, P. (2004). Ultraviolet-c overexposure induces programmed cell death in arabidopsis, which is mediated by caspase-like activities and which can be suppressed by caspase inhibitors, p35 and defender against apoptotic death. J. Biol. Chem. 279 (1), 779–787. doi: 10.1074/jbc.M304468200
Da-Silva, C. J., do Amarante, L. (2022). Nitric oxide signaling in plants during flooding stress, nitric oxide in plant biology (Cambridge, USA: Academic Press), 241–260. doi: 10.1016/B978-0-12-818797-5.00009-1
Delaney, T. P., Uknes, S., Vernooij, B., Friedrich, L., Weymann, K., Negrotto, D., et al. (1994). A central role of salicylic acid in plant disease resistance. science. Science 266 (5188), 1247–1250. doi: 10.1126/science.266.5188.124
del Rıo, L. A., Corpas, F. J., Barroso, J. B. (2004). Nitric oxide and nitric oxide synthase activity in plants. Phytochem 65 (7), 783–792. doi: 10.1016/j.phytochem.2004.02.001
De Souza, A. P., Gaspar, M., Da Silva, E. A., Ulian, E. C., Waclawovsky, A. J., Nishiyama, M. Y., Jr., et al. (2008). Elevated CO2 increases photosynthesis, biomass and productivity, and modifies gene expression in sugarcane. Plant Cell Envir. 31 (8), 1116–1127. doi: 10.1111/j.1365-3040.2008.01822.x
Domingos, P., Prado, A. M., Wong, A., Gehring, C., Feijo, J. A. (2015). Nitric oxide: a multitasked signaling gas in plants. Mol. Plant 8 (4), 506–520. doi: 10.1016/j.molp.2014.12.010
Drøbak, B., Franklin-Tong, V., Staiger, C. J. N. P. (2004). The role of the actin cytoskeleton in plant cell signaling. New Phytol. 163 (1), 13–30. doi: 10.1111/j.1469-8137.2004.01076.x
Du, S., Zhang, R., Zhang, P., Liu, H., Yan, M., Chen, N., et al. (2016). Elevated CO2-induced production of nitric oxide (NO) by NO synthase differentially affects nitrate reductase activity in arabidopsis plants under different nitrate supplies. J. Exp. Bot. 67 (3), 893–904. doi: 10.1093/jxb/erv506
Emmanuel, S., Ague, J. J. (2007). Implications of present-day abiogenic methane fluxes for the early archean atmosphere. Geophys. Res. Let. 34, L15810. doi: 10.1029/2007GL030532
Fatima, A., Husain, T., Suhel, M., Prasad, S. M., Singh, V. P. (2021). Implication of nitric oxide under salinity stress: The possible interaction with other signaling molecules. J. Plant Growth Reg. 41 (1), 163–177. doi: 10.1007/s00344-020-10255-5
Feechan, A., Kwon, E., Yun, B.-W., Wang, Y., Pallas, J. A., Loake, G. J. (2005). A central role for S-nitrosothiols in plant disease resistance. Proceed. Nat. Acad. Sci. 102 (22), 8054–8059. doi: 10.1073/pnas.050145610
Fiebig, J., Woodland, A. B., D'Alessandro, W., Püttmann, W. (2009). Excess methane in continental hydrothermal emissions is abiogenic. Geology 37 (6), 495–498. doi: 10.1130/G25598A.1
Frohnmeyer, H., Loyall, L., Blatt, M. R., Grabov, A. J. T. P. J. (1999). Millisecond UV-b irradiation evokes prolonged elevation of cytosolic-free Ca2+ and stimulates gene expression in transgenic parsley cell cultures. Plant J. 20 (1), 109–117. doi: 10.1046/j.1365-313X.1999.00584.x
Frungillo, L., Skelly, M. J., Loake, G. J., Spoel, S. H., Salgado, I. J. N. C. (2014). S-nitrosothiols regulate nitric oxide production and storage in plants through the nitrogen assimilation pathway. Nat. Commun. 5 (1), 1–10. doi: 10.1038/ncomms6401
Garcia-Mata, C., Lamattina, L. (2007). Abscisic acid (ABA) inhibits light-induced stomatal opening through calcium-and nitric oxide-mediated signaling pathways. Nitric. Oxide 17 (3–4), 143–151. doi: 10.1016/j.niox.2007.08.001
Gayatri, G., Agurla, S., Raghavendra, A. S. (2013). Nitric oxide in guard cells as an important secondary messenger during stomatal closure. Front. Plant Sci. 4. doi: 10.3389/fpls.2013.00425
Geiger, M., Walch-Liu, P., Engels, C., Harnecker, J., Schulze, E. D., Ludewig, F., et al. (1998). Enhanced carbon dioxide leads to a modified diurnal rhythm of nitrate reductase activity in older plants, and a large stimulation of nitrate reductase activity and higher levels of amino acids in young tobacco plants. Plant Cell Envir. 21 (3), 253–268. doi: 10.1046/j.1365-3040.1998.00277.x
Geng, S., Yu, B., Zhu, N., Dufresne, C., Chen, S. (2017) Metabolomics and proteomics of brassica napus guard cells in response to low CO2. Front. Mol. Biosci. 4. doi: 10.3389/fmolb.2017.00051
Gupta, A. S., Alscher, R. G., McCune, D. (1991). Response of photosynthesis and cellular antioxidants to ozone in populus leaves. Plant Physiol. 96 (2), 650–655. doi: 10.1104/pp.96.2.650
Gupta, K. J., Bauwe, H., Mur, L. A. (2011). Nitric oxide, nitrate reductase and UV-b tolerance. Tree Physiol. 31 (8), 795–797. doi: 10.1093/treephys/tpr080
Gupta, K. J., Stoimenova, M., Kaiser, W. M. (2005). In higher plants, only root mitochondria, but not leaf mitochondria reduce nitrite to NO, in vitro and in situ. J. Exp. Bot. 56 (420), 2601–2609. doi: 10.1093/jxb/eri252
Guri, A. (1983). Variation in glutathione and ascorbic acid content among selected cultivars of Phaseolus vulgaris prior to and after exposure to ozone. Can. J. Plant Sci. 63 (3), 733–737. doi: 10.4141/cjps83-090
Hakata, M., Takahashi, M., Zumft, W., Sakamoto, A., Morikawa, H. (2003). Conversion of the nitrate nitrogen and nitrogen dioxide to nitrous oxides in plants. Acta Biotech. 23 (2-3), 249–257. doi: 10.1002/abio.200390032
Hancock, J. T., Neill, S. (2019). Nitric oxide: its generation and interactions with other reactive signaling compounds. Plants 8 (2), 41. doi: 10.3390/plants8020041
Hasan, M., Rahman, M., Skalicky, M., Alabdallah, N. M., Waseem, M., Jahan, M. S., et al. (2021). Ozone induced stomatal regulations, MAPK and phytohormone signaling in plants. Intl J. Mol. Sci. 22 (12), 6304. doi: 10.3390/ijms22126304
Hawkins, T. L., Sept, D., Mogessie, B., Straube, A., Ross, J. L. (2013). Mechanical properties of doubly stabilized microtubule filaments. Biophys. J. 104 (7), 1517–1528. doi: 10.1016/j.bpj.2013.02.026
Haworth, M., Killi, D., Materassi, A., Raschi, A., Centritto, M. (2016). Impaired stomatal control is associated with reduced photosynthetic physiology in crop species grown at elevated [CO2]. Front. Plant Sci. 7. doi: 10.3389/fpls.2016.01568
He, J., Zhang, R.-X., Kim, D. S., Sun, P., Liu, H., Liu, Z., et al. (2020). ROS of distinct sources and salicylic acid separate elevated CO2-mediated stomatal movements in arabidopsis. Front. Plant Sci. 11. doi: 10.3389/fpls.2020.00542
Hocking, P., Meyer, C. (1985). Responses of noogoora burr (Xanthium occidentale bertol.) to nitrogen supply and carbon dioxide enrichment. Ann. Bot. 55 (6), 835–844. doi: 10.1093/oxfordjournals.aob.a086964
Hocking, P., Meyer, C. (1991a). Effects of CO2 enrichment and nitrogen stress on growth, and partitioning of dry matter and nitrogen in wheat and maize. Funct. Plant Biol. 18 (4), 339–356. doi: 10.1071/PP9910339
Hocking, P., Meyer, C. (1991b). Carbon dioxide enrichment decreases critical nitrate and nitrogen concentrations in wheat. J. Plant Nutr. 14 (6), 571–584. doi: 10.1080/01904169109364225
Hofmann, L. C., Straub, S., Bischof, K. (2013). Elevated CO2 levels affect the activity of nitrate reductase and carbonic anhydrase in the calcifying rhodophyte Corallina officinalis. J. Exp. Bot. 64 (4), 899–908. doi: 10.1093/jxb/ers369
Hsu, P.-K., Takahashi, Y., Munemasa, S., Merilo, E., Laanemets, K., Waadt, R., et al. (2018). Abscisic acid-independent stomatal CO2 signal transduction pathway and convergence of CO2 and ABA signaling downstream of OST1 kinase. Proceed. Nat. Acad. Sci. 115 (42), E9971–E9980. doi: 10.1073/pnas.180920411
Hu, H., Boisson-Dernier, A., Israelsson-Nordström, M., Böhmer, M., Xue, S., Ries, A., et al. (2010). Carbonic anhydrases are upstream regulators of CO2-controlled stomatal movements in guard cells. Nat. Cell Biol. 12 (1), 87–93. doi: 10.1038/ncb2009
Hu, H., Liu, D., Li, P. (2018). Methane delays the senescence and browning in daylily buds by re-established redox homeostasis. J. Sci. Food Agric. 98 (5), 1977–1987. doi: 10.1002/jsfa.8681
Hurali, D. T., Bhurta, R., Tyagi, S., Sathee, L., Sandeep, A. B., Singh, D., et al. (2022). Analysis of NIA and GSNOR family genes and nitric oxide homeostasis in response to wheat-leaf rust interaction. Sci. Rep. 12 (1), 1–16. doi: 10.1038/s41598-021-04696-5
Hussain, A., Imran, Q. M., Shahid, M., Yun, B.-W. (2022). Nitric oxide synthase in the plant kingdom, nitric oxide in plant biology (Cambridge, USA: Academic Press), 43–52. doi: 10.1016/B978-0-12-818797-5.00016-9
Hussey, P. J., Ketelaar, T., Deeks, M. J. (2006). Control of the actin cytoskeleton in plant cell growth. Ann. Rev. Plant Biol. 57 (1), 109–125. doi: 10.1146/annurev.arplant.57.032905.105206
Jahnová, J., Luhová, L., Petřivalský, M. (2019). S-nitrosoglutathione reductase–the master regulator of protein s-nitrosation in plant NO signaling. Plants 8 (2), 48. doi: 10.3390/plants8020048
Jansen, M. A., Gaba, V., Greenberg, B. M. (1998). Higher plants and UV-b radiation: balancing damage, repair and acclimation. Trend. Plant Sci. 3 (4), 131–135. doi: 10.1016/S1360-1385(98)01215-1
Jenkins, G. I., Long, J. C., Wade, H. K., Shenton, M. R., Bibikova, T. N. (2001). UV And blue light signalling: pathways regulating chalcone synthase gene expression in arabidopsis. New Phytol. 151 (1), 121–131. doi: 10.1046/j.1469-8137.2001.00151.x
Kasprowicz, A., Szuba, A., Volkmann, D., Baluška, F., Wojtaszek, P. (2009). Nitric oxide modulates dynamic actin cytoskeleton and vesicle trafficking in a cell type-specific manner in root apices. J. Exp. Bot. 60 (6), 1605–1617. doi: 10.1093/jxb/erp033
Khajuria, A., Bali, S., Sharma, P., Kaur, R., Jasrotia, S., Saini, P., et al. (2019). “S-nitrosoglutathione (GSNO) and plant stress responses,” in Reactive oxygen, nitrogen and sulfur species in plants: Production, metabolism, signaling and defense mechanisms (New York, NY, USA: John Wiley & Sons), 627–644. doi: 10.1002/9781119468677.ch26
Khalil, K., Aslam, M. (2009). Emissions of greenhouse gases from rice agriculture (Portland, Oregon: Portland State University). doi: 10.2172/959124
Khan, M. I. R., Fatma, M., Per, T. S., Anjum, N. A., Khan, N. A. (2015). Salicylic acid-induced abiotic stress tolerance and underlying mechanisms in plants. Front. Plant Sci. 6. doi: 10.3389/fpls.2015.00462
Khan, M. A., Khan, A. L., Imran, Q. M., Asaf, S., Lee, S.-U., Yun, B.-W., et al. (2019). Exogenous application of nitric oxide donors regulates short-term flooding stress in soybean. PeerJ 7, e7741. doi: 10.7717/peerj.7741
Khan, M. N., Mobin, M., Mohammad, F., Corpas, F. J. (2014). Nitric oxide in plants: metabolism and role in stress physiology (Cham, Switzerland: Springer). doi: 10.1007/978-3-319-06710-0
Khan, M. N., Siddiqui, M. H., AlSolami, M. A., Alamri, S., Hu, Y., Ali, H. M., et al. (2020). Crosstalk of hydrogen sulfide and nitric oxide requires calcium to mitigate impaired photosynthesis under cadmium stress by activating defense mechanisms in vigna radiata. Plant Physiol. Biochem. 156, 278–290. doi: 10.1016/j.plaphy.2020.09.017
Kim, T.-H., Böhmer, M., Hu, H., Nishimura, N., Schroeder, J. I. (2010). Guard cell signal transduction network: advances in understanding abscisic acid, CO2, and Ca2+ signaling. Ann. Rev. Plant Biol. 61, 561. doi: 10.1146/annurev-arplant-042809-112226
Klepper, L. (1979). Nitric oxide (NO) and nitrogen dioxide (NO2) emissions from herbicide-treated soybean plants. Atmosph. Envir. 13 (4), 537–542. doi: 10.1016/0004-6981(79)90148-3
Klessig, D. F., Durner, J., Noad, R., Navarre, D. A., Wendehenne, D., Kumar, D., et al. (2000). Nitric oxide and salicylic acid signaling in plant defense. Proceed. Nat. Acad. Sci. 97 (16), 8849–8855. doi: 10.1073/pnas.97.16.8849
Kolbert, Z., Barroso, J. B., Brouquisse, R., Corpas, F. J., Gupta, K. J., Lindermayr, C., et al. (2019). A forty year journey: The generation and roles of NO in plants. Nitric. Oxide 93, 53–70. doi: 10.1016/j.niox.2019.09.006
Kong, W., Huang, C., Chen, Q., Zou, Y., Zhang, J. (2012). Nitric oxide alleviates heat stress-induced oxidative damage in pleurotus eryngii var. tuoliensis. Fung. Genet. Biol. 49 (1), 15–20. doi: 10.1016/j.fgb.2011.12.003
Kost, B., Chua, N.-H. (2002). The plant cytoskeleton: vacuoles and cell walls make the difference. Cell 108 (1), 9–12. doi: 10.1016/S0092-8674(01)00634-1
Lashof, D. A., Ahuja, D. R. (1990). Relative contributions of greenhouse gas emissions to global warming. Nature 344 (6266), 529–531. doi: 10.1038/344529a0
Lau, S.-E., Hamdan, M. F., Pua, T.-L., Saidi, N. B., Tan, B. C. (2021). Plant nitric oxide signaling under drought stress. Plants 10 (2), 360. doi: 10.3390/plants10020360
Lawlor, D., Mitchell, R. (1991). The effects of increasing CO2 on crop photosynthesis and productivity: a review of field studies. Plant Cell Envir. 14 (8), 807–818. doi: 10.1111/j.1365-3040.1991.tb01444.x
Lenhart, K., Behrendt, T., Greiner, S., Steinkamp, J., Well, R., Giesemann, A., et al. (2019). Nitrous oxide effluxes from plants as a potentially important source to the atmosphere. New Phytol. 221 (3), 1398–1408. doi: 10.1111/nph.15455
Liu, X., Wang, X., Licht, G., Licht, S. (2020). Transformation of the greenhouse gas carbon dioxide to graphene. J. CO2 Utiliz. 36, 288–294. doi: 10.1016/j.jcou.2019.11.019
Li, L., Wei, S., Shen, W. (2020). The role of methane in plant physiology: a review. Plant Cell Rep. 39 (2), 171–179. doi: 10.1007/s00299-019-02478-y
Li, X., Zhang, L., Ahammed, G. J., Li, Y.-T., Wei, J.-P., Yan, P., et al. (2019). Salicylic acid acts upstream of nitric oxide in elevated carbon dioxide-induced flavonoid biosynthesis in tea plant (Camellia sinensis l.). Envir. Exp. Bot. 161, 367–374. doi: 10.1016/j.envexpbot.2018.11.012
Li, Y., Zhang, X., Zhang, Y., Ren, H. (2022). Controlling the gate: the functions of the cytoskeleton in stomatal movement. Front. Plant Sci. 13. doi: 10.3389/fpls.2022.849729
Lytvyn, D. I., Raynaud, C., Yemets, A. I., Bergounioux, C., Blume, Y. B. (2016). Involvement of inositol biosynthesis and nitric oxide in the mediation of UV-b induced oxidative stress. Front. Plant Sci. 7. doi: 10.3389/fpls.2016.00430
Lytvyn, D. I., Yemets, A. I., Blume, Y. B. (2010). UV-B overexposure induces programmed cell death in a BY-2 tobacco cell line. Envir. Exp. Bot. 68 (1), 51–57. doi: 10.1016/j.envexpbot.2009.11.004
Magalhaes, J. R., Pedroso, M. C., Durzan, D. (1999). Nitric oxide apoptosis and plant stresses. Physiol. Mol. Biol. Plants 5, 115–125.
Ma, F., Lu, R., Liu, H., Shi, B., Zhang, J., Tan, M., et al. (2012). Nitric oxide-activated calcium/calmodulin-dependent protein kinase regulates the abscisic acid-induced antioxidant defence in maize. J. Exp. Bot. 63 (13), 4835–4847. doi: 10.1093/jxb/ers161
Martin, S. J., Cotter, T. G. (1991). Ultraviolet b irradiation of human leukaemia HL-60 cells in vitro induces apoptosis. Int. J. Rad. Biol. 59 (4), 1001–1016. doi: 10.1080/09553009114550891
Masle, J. (2000). The effects of elevated CO2 concentrations on cell division rates, growth patterns, and blade anatomy in young wheat plants are modulated by factors related to leaf position, vernalization, and genotype. Plant Physiol. 122 (4), 1399–1416. doi: 10.1104/pp.122.4.1399
Matt, P., Geiger, M., Walch-Liu, P., Engels, C., Krapp, A., Stitt, M. (2001). Elevated carbon dioxide increases nitrate uptake and nitrate reductase activity when tobacco is growing on nitrate, but increases ammonium uptake and inhibits nitrate reductase activity when tobacco is growing on ammonium nitrate. Plant Cell Envir. 24 (11), 1119–1137. doi: 10.1046/j.1365-3040.2001.00771.x
McKee, I., Woodward, F. (1994). CO2 enrichment responses of wheat: interactions with temperature, nitrate and phosphate. New Phytol. 127 (3), 447–453. doi: 10.1111/j.1469-8137.1994.tb03962.x
Medina-Rivera, A., Defrance, M., Sand, O., Herrmann, C., Castro-Mondragon, J. A., Delerce, J., et al. (2015). RSAT 2015: regulatory sequence analysis tools. Nucl. Acid. Res. 43 (W1), W50–W56. doi: 10.1093/nar/gkv362
Merilo, E., Jõesaar, I., Brosché, M., Kollist, H. (2014). To open or to close: species-specific stomatal responses to simultaneously applied opposing environmental factors. New Phytol. 202 (2), 499–508. doi: 10.1111/nph.12667
Mukherjee, S. (2022). “Atmospheric nitric oxide (NO) regulates ozone (O3)-induced stress signaling in plants: Ally or foe?,” in Plant stress: Challenges and management in the new decade (Cham, Switzerland: Springer), 89–96. doi: 10.1007/978-3-030-95365-2_5
Muroyama, A., Gong, Y., Bergmann, D. C. (2020). Opposing, polarity-driven nuclear migrations underpin asymmetric divisions to pattern arabidopsis stomata. Curr. Biol. 30 (22), 4467–4475. e4. doi: 10.1016/j.cub.2020.08.100
Natali, S. M., Sañudo-Wilhelmy, S. A., Lerdau, M. T. (2009). Effects of elevated carbon dioxide and nitrogen fertilization on nitrate reductase activity in sweetgum and loblolly pine trees in two temperate forests. Plant Soil 314 (1), 197–210. doi: 10.1007/s11104-008-9718-x
Nawkar, G. M., Maibam, P., Park, J. H., Sahi, V. P., Lee, S. Y., Kang, C. H. (2013). UV-Induced cell death in plants. Intl. J. Mol. Sci. 14 (1), 1608–1628. doi: 10.3390/ijms14011608
Niu, Y. F., Jin, G. L., Chai, R. S., Wang, H., Zhang, Y. S. (2011). Responses of root hair development to elevated CO2. Plant Signal. Behav. 6 (9), 1414–1417. doi: 10.4161/psb.6.9.17302
Niu, L., Yu, J., Liao, W., Yu, J., Zhang, M., Dawuda, M. M. (2017). Calcium and calmodulin are involved in nitric oxide-induced adventitious rooting of cucumber under simulated osmotic stress. Front. Plant Sci. 8. doi: 10.3389/fpls.2017.01684
Nogués, I., Fares, S., Oksanen, E., Loreto, F. (2008). “Ozone effects on the metabolism and the antioxidant system of poplar leaves at different stages of development, photosynthesis,” in Energy from the sun (Dordrecht, The Netherlands: Springer), 1317–1321. doi: 10.1007/978-1-4020-6709-9_284
Novikova, G. V., Mur, L. A., Nosov, A. V., Fomenkov, A. A., Mironov, K. S., Mamaeva, A. S., et al. (2017). Nitric oxide has a concentration-dependent effect on the cell cycle acting via EIN2 in arabidopsis thaliana cultured cells. Front. Plysiol. 8. doi: 10.3389/fphys.2017.00142
Oravecz, A., Baumann, A., Máté, Z., Brzezinska, A., Molinier, J., Oakeley, E. J., et al. (2006). CONSTITUTIVELY PHOTOMORPHOGENIC1 is required for the UV-b response in arabidopsis. Plant Cell 18 (8), 1975–1990. doi: 10.1105/tpc.105.040097
Ötvös, K., Pasternak, T. P., Miskolczi, P., Domoki, M., Dorjgotov, D., Sz˝ cs, A., et al. (2005). Nitric oxide is required for, and promotes auxin-mediated activation of, cell division and embryogenic cell formation but does not influence cell cycle progression in alfalfa cell cultures. Plant J. 43 (6), 849–860. doi: 10.1111/j.1365-313X.2005.02494.x
Paez-Garcia, A., Sparks, J. A., Bang, L., Blancaflor, E. B. (2018). Plant actin cytoskeleton: New functions from old scaffold, concepts in cell biology-history and evolution (Cham, Switzerland: Springer), 103–137. doi: 10.1007/978-3-319-69944-8_6
Panteris, E., Achlati, T., Daras, G., Rigas, S. (2018). Stomatal complex development and f-actin organization in maize leaf epidermis depend on cellulose synthesis. Molecules 23 (6), 1365. doi: 10.3390/molecules23061365
Parankusam, S., Adimulam, S. S., Bhatnagar-Mathur, P., Sharma, K. K. (2017). Nitric oxide (NO) in plant heat stress tolerance: current knowledge and perspectives. Front. Plant Sci. 8. doi: 10.3389/fpls.2017.01582
Park, S.-U., Lee, C.-J., Park, S.-C., Nam, K. J., Lee, K.-L., Kwak, S.-S., et al. (2022). Flooding tolerance in sweet potato (Ipomoea batatas (L.) lam) is mediated by reactive oxygen species and nitric oxide. Antioxidants 11 (5), 878. doi: 10.3390/antiox11050878
Pasqualini, S., Meier, S., Gehring, C., Madeo, L., Fornaciari, M., Romano, B., et al. (2009). Ozone and nitric oxide induce cGMP-dependent and-independent transcription of defence genes in tobacco. New Phytol. 181 (4), 860–870. doi: 10.1111/j.1469-8137.2008.02711.x
Pettersson, R., McDonald, A., Stadenberg, I. (1993). Response of small birch plants (Betula pendula roth.) to elevated CO2 and nitrogen supply. Plant Cell Envir. 16 (9), 1115–1121. doi: 10.1111/j.1365-3040.1996.tb02069.x
Phillips, K., Majola, A., Gokul, A., Keyster, M., Ludidi, N., Egbichi, I. (2018). Inhibition of NOS-like activity in maize alters the expression of genes involved in H2O2 scavenging and glycine betaine biosynthesis. Sci. Rep. 8 (1), 1–9. doi: 10.1038/s41598-018-31131-z
Purvis, A., Peters, D., Hageman, R. (1974). Effect of carbon dioxide on nitrate accumulation and nitrate reductase induction in corn seedlings. Plant Physiol. 53 (6), 934–941. doi: 10.1104/pp.53.6.934
Qi, F., Xiang, Z., Kou, N., Cui, W., Xu, D., Wang, R., et al. (2017). Nitric oxide is involved in methane-induced adventitious root formation in cucumber. Physiol. Planta. 159 (3), 366–377. doi: 10.1111/ppl.12531
Rao, M. V., Davis, K. R. (2001). The physiology of ozone induced cell death. Planta 213 (5), 682–690. doi: 10.1007/s004250100618
Riehl Koch, J., Scherzer, A. J., Eshita, S. M., Davis, K. R. (1998). Ozone sensitivity in hybrid poplar is correlated with a lack of defense-gene activation. Plant Physiol. 118 (4), 1243–1252. doi: 10.1104/pp.118.4.1243
Ries, G., Heller, W., Puchta, H., Sandermann, H., Seidlitz, H. K., Hohn, B. (2000). Elevated UV-b radiation reduces genome stability in plants. Nature 406 (6791), 98–101. doi: 10.1038/35017595
Rogers, G., Payne, L., Milham, P., Conroy, J. (1993). Nitrogen and phosphorus requirements of cotton and wheat under changing atmospheric CO2 concentrations. Plant Soil 155 (1), 231–234. doi: 10.1007/BF00025026
Rustérucci, C., Espunya, M. C., Díaz, M., Chabannes, M., Martínez, M. C. (2007). S-nitrosoglutathione reductase affords protection against pathogens in arabidopsis, both locally and systemically. Plant Physiol. 143 (3), 1282–1292. doi: 10.1104/pp.106.091686
Sami, F., Siddiqui, H., Hayat, S. (2021). Nitric oxide-mediated enhancement in photosynthetic efficiency, ion uptake and carbohydrate metabolism that boosts overall photosynthetic machinery in mustard plants. J. Plant Growth Reg. 40 (3), 1088–1110. doi: 10.1007/s00344-020-10166-5
Sampaio, M., Neves, J., Cardoso, T., Pissarra, J., Pereira, S., Pereira, C. (2022). Coping with abiotic stress in plants–an endomembrane trafficking perspective. Plants 11 (3), 338. doi: 10.3390/plants11030338
Sánchez-Vicente, I., Lechón, T., Fernández-Marcos, M., Sanz, L., Lorenzo, O. (2021). Nitric oxide alters the pattern of auxin maxima and PIN-FORMED1 during shoot development. Front. Plant Sci. 12. doi: 10.3389/fpls.2021.630792
Santa-Cruz, D. M., Pacienza, N. A., Zilli, C. G., Tomaro, M. L., Balestrasse, K. B., Yannarelli, G. G. J. J., et al. (2014). Nitric oxide induces specific isoforms of antioxidant enzymes in soybean leaves subjected to enhanced ultraviolet-b radiation. J. Phytochem. Photobiol. 141, 202–209. doi: 10.1016/j.jphotobiol.2014.09.019
Sanz, L., Albertos, P., Mateos, I., Sánchez-Vicente, I., Lechón, T., Fernández-Marcos, M., et al. (2015). Nitric oxide (NO) and phytohormones crosstalk during early plant development. J. Exp. Bot. 66 (10), 2857–2868. doi: 10.1093/jxb/erv213
Saxena, I., Shekhawat, G. J. N. O. (2013). Nitric oxide (NO) in alleviation of heavy metal induced phytotoxicity and its role in protein nitration. Nitric. Oxide 32, 13–20. doi: 10.1016/j.niox.2013.03.004
Schützenmeister, K., Meurer, K. H., Gronwald, M., Hartmann, A. B., Gansert, D., Jungkunst, H. F. (2020). N2O emissions from plants are reduced under photosynthetic activity. Plant Envir. Inter. 1 (1), 48–56. doi: 10.1002/pei3.10015
Sharma, Y. K., Davis, K. R. (1995). Isolation of a novel arabidopsis ozone-induced cDNA by differential display. plant molecular biology. Plant Mol. Biol. 29 (1), 91–98. doi: 10.1007/BF00019121
Sharma, Y. K., Davis, K. R. (1997). The effects of ozone on antioxidant responses in plants. free radical biology and medicine. Free Rad. Biol. Med. 23 (3), 480–488. doi: 10.1016/S0891-5849(97)00108-1
Sharma, N., Sinha, P. G., Bhatnagar, A. K. (2014). Effect of elevated [CO2] on cell structure and function in seed plants. Clim. Change Envir. Sustain. 2, 69–104. doi: 10.5958/2320-642X.2014.00001.5
Shen, Q., Wang, Y.-T., Tian, H., Guo, F.-Q. (2013). Nitric oxide mediates cytokinin functions in cell proliferation and meristem maintenance in arabidopsis. Mol. Plant 6 (4), 1214–1225. doi: 10.1093/mp/sss148
Shi, K., Li, X., Zhang, H., Zhang, G., Liu, Y., Zhou, Y., et al. (2015). Guard cell hydrogen peroxide and nitric oxide mediate elevated CO2-induced stomatal movement in tomato. New Phytol. 208 (2), 342–353. doi: 10.1111/nph.13621
Signorelli, S., Considine, M. J. (2018). Nitric oxide enables germination by a four-pronged attack on ABA-induced seed dormancy. Front. Plant Sci. 9. doi: 10.3389/fpls.2018.00296
Sigurdsson, B. D. (2001). Elevated [CO2] and nutrient status modified leaf phenology and growth rhythm of young Populus trichocarpa trees in a 3-year field study. Trees 15 (7), 403–413. doi: 10.1007/s004680100121
Singh, V. P., Singh, S., Tripathi, D. K., Romero-Puertas, M. C., Sandalio, L. M. (2021). Nitric oxide in plant biology: An ancient molecule with emerging roles (Academic Press).
Soda, N., Sharan, A., Gupta, B. K., Singla-Pareek, S. L., Pareek, A. J. S. (2016a). Evidence for nuclear interaction of a cytoskeleton protein (OsIFL) with metallothionein and its role in salinity stress tolerance. Sci. Rep. 6 (1), 1–14. doi: 10.1038/srep34762
Soda, N., Singla-Pareek, S. L., Pareek, A. (2016b). Abiotic stress response in plants: Role of cytoskeleton. Abiotic Stress Reponse Plants 6 (1), 1–14. doi: 10.1002/9783527694570.ch6
Soheila, A.-H., John, C. F., Jordan, B., Thomas, B. (2001). Early signaling components in ultraviolet-b responses: distinct roles for different reactive oxygen species and nitric oxide. FEBS let. 489 (2-3), 237–242. doi: 10.1016/S0014-5793(01)02103-2
Stitt, M., Krapp, A. (1999). The interaction between elevated carbon dioxide and nitrogen nutrition: the physiological and molecular background. Plant Cell Envir. 22 (6), 583–621. doi: 10.1046/j.1365-3040.1999.00386.x
Sun, L., Li, Y., Miao, W., Piao, T., Hao, Y., Hao, F.-S. (2017). NADK2 positively modulates abscisic acid-induced stomatal closure by affecting accumulation of H2O2, Ca2+ and nitric oxide in arabidopsis guard cells. Plant Sci. 262, 81–90. doi: 10.1016/j.plantsci.2017.06.003
Sun, C., Zhang, Y., Liu, L., Liu, X., Li, B., Jin, C., et al. (2021). Molecular functions of nitric oxide and its potential applications in horticultural crops. Hortic. Res. 8, 71. doi: 10.1038/s41438-021-00500-7
Takeda, N., Friedl, J., Kirkby, R., Rowlings, D., De Rosa, D., Scheer, C., et al. (2022). Interaction between soil and fertiliser nitrogen drives plant nitrogen uptake and nitrous oxide (N2O) emissions in tropical sugarcane systems. Plant Soil, 477, 647–663. doi: 10.1007/s11104-022-05458-6
Tanaka, A., Sakamoto, A., Ishigaki, Y., Nikaido, O., Sun, G., Hase, Y., et al. (2002). An ultraviolet-b-resistant mutant with enhanced DNA repair in arabidopsis. Plant Physiol. 129 (1), 64–71. doi: 10.1104/pp.010894
Thompson, M., Gamage, D., Hirotsu, N., Martin, A., Seneweera, S. (2017). Effects of elevated carbon dioxide on photosynthesis and carbon partitioning: a perspective on root sugar sensing and hormonal crosstalk. Front. Physiol. 578. doi: 10.3389/fphys.2017.00578
Tian, W., Hou, C., Ren, Z., Pan, Y., Jia, J., Zhang, H., et al. (2015). A molecular pathway for CO2 response in arabidopsis guard cells. Nat. Commun. 6, 6057. doi: 10.1038/ncomms7057
Timilsina, A., Bizimana, F., Pandey, B., Yadav, R. K. P., Dong, W., Hu, C. (2020a). Nitrous oxide emissions from paddies: understanding the role of rice plants. Plants 9 (2), 180. doi: 10.3390/plants9020180
Timilsina, A., Oenema, O., Luo, J., Wang, Y., Dong, W., Pandey, B., et al. (2022). Plants are a natural source of nitrous oxide even in field conditions as explained by 15N site preference. Sci. Tot. Envir. 805, 150262. doi: 10.1016/j.scitotenv.2021.150262
Timilsina, A., Zhang, C., Pandey, B., Bizimana, F., Dong, W., Hu, C. (2020b). Potential pathway of nitrous oxide formation in plants. Front. Plant Sci. 11. doi: 10.3389/fpls.2020.01177
Tissue, D., Thomas, R., Strain, B. (1997). Atmospheric CO2 enrichment increases growth and photosynthesis of Pinus taeda: a 4 year experiment in the field. Plant Cell Envir. 20 (9), 1123–1134. doi: 10.1046/j.1365-3040.1997.d01-140.x
Tohge, T., Kusano, M., Fukushima, A., Saito, K., Fernie, A. R. (2011). Transcriptional and metabolic programs following exposure of plants to UV-b irradiation. Plant Signal. Behav. 6 (12), 1987–1992. doi: 10.4161/psb.6.12.18240
Tossi, V., Lamattina, L., Cassia, R. (2009). An increase in the concentration of abscisic acid is critical for nitric oxide-mediated plant adaptive responses to UV-b irradiation. New Phytol. 181 (4), 871–879. doi: 10.1111/j.1469-8137.2008.02722.x
Tossi, V., Lamattina, L., Jenkins, G. I., Cassia, R. O. (2014). Ultraviolet-b-induced stomatal closure in arabidopsis is regulated by the UV RESISTANCE LOCUS8 photoreceptor in a nitric oxide-dependent mechanism. Plant Physiol. 164 (4), 2220–2230. doi: 10.1104/pp.113.231753
Vanhaelewyn, L., van der Straeten, D., De Coninck, B., Vandenbussche, F. (2020). Ultraviolet radiation from a plant perspective: The plant-microorganism context. Front. Plant Sci. 11, 597642. doi: 10.3389/fpls.2020.597642
Wang, R. J. T. (2014). Gasotransmitters: growing pains and joys. Trend. Biochem. Sci. 39 (5), 227–232. doi: 10.1016/j.tibs.2014.03.003
Wang, Z.-P., Chang, S. X., Chen, H., Han, X.-G. (2013b). Widespread non-microbial methane production by organic compounds and the impact of environmental stresses. Earth Sci. Rev. 127, 193–202. doi: 10.1016/j.earscirev.2013.10.001
Wang, Z.-P., Gulledge, J., Zheng, J.-Q., Liu, W., Li, L.-H., Han, X.-G. (2009). Physical injury stimulates aerobic methane emissions from terrestrial plants. Biogeosci 6 (4), 615–621. doi: 10.5194/bg-6-615-2009
Wang, N., Huang, D., Li, C., Deng, Y., Li, W., Yao, Y., et al. (2020). Regulatory roles of methane in plants. Sci. Hortic. 272, 109492. doi: 10.1016/j.scienta.2020.109492
Wang, P., Hussey, P. J. (2015). Interactions between plant endomembrane systems and the actin cytoskeleton. Front. Plant Sci. 6. doi: 10.3389/fpls.2015.00422
Wang, H., Niu, Y., Chai, R., Liu, M., Zhang, Y. (2013a). Cross–talk between nitric oxide and Ca2+ in elevated CO2-induced lateral root formation. Plant Signal. Behav. 8 (2), 137–144. doi: 10.4161/psb.23106
Wang, H., Xiao, W., Niu, Y., Chai, R., Jin, C., Zhang, Y. (2015). Elevated carbon dioxide induces stomatal closure of arabidopsis thaliana (L.) heynh. through an increased production of nitric oxide. J. Plant Growth Reg. 34 (2), 372–380. doi: 10.1007/s00344-014-9473-6
Wang, C., Zhang, L.-J., Huang, R.-D. (2011). Cytoskeleton and plant salt stress tolerance. Plant Signal. Behav. 6 (1), 29–31. doi: 10.4161/psb.6.1.14202
Wargent, J. J., Jordan, B. R. (2013). From ozone depletion to agriculture: understanding the role of UV radiation in sustainable crop production. New Phytol. 197 (4), 1058–1076. doi: 10.1111/nph.12132
Webb, A. A., McAinsh, M. R., Mansfield, T. A., Hetherington, A. M. (1996). Carbon dioxide induces increases in guard cell cytosolic free calcium. Plant J. 9 (3), 297–304. doi: 10.1046/j.1365-313X.1996.09030297.x
Wong, S. (1979). Elevated atmospheric partial pressure of CO2 and plant growth. Oecologia 44 (1), 68–74. doi: 10.1007/BF00346400
Woolfenden, H. C., Baillie, A. L., Gray, J. E., Hobbs, J. K., Morris, R. J., Fleming, A. J., et al. (2018). Models and mechanisms of stomatal mechanics. Trend. Plant Sci. 23 , 822–832. doi: 10.1016/j.tplants.2018.06.003
Wu, Q., Su, N., Zhang, X., Liu, Y., Cui, J., Liang, Y. (2016). Hydrogen peroxide, nitric oxide and UV RESISTANCE LOCUS8 interact to mediate UV-b-induced anthocyanin biosynthesis in radish sprouts. Sci. Rep. 6 (1), 1–12. doi: 10.1038/srep29164
Xiong, J., Zhang, L., Fu, G., Yang, Y., Zhu, C., Tao, L. (2012). Drought-induced proline accumulation is uninvolved with increased nitric oxide, which alleviates drought stress by decreasing transpiration in rice. J. Plant Res. 125 (1), 155–164. doi: 10.1007/s10265-011-0417-y
Xue, S., Hu, H., Ries, A., Merilo, E., Kollist, H., Schroeder, J. I. (2011). Central functions of bicarbonate in s-type anion channel activation and OST1 protein kinase in CO2 signal transduction in guard cell. EMBO J. 30 (8), 1645–1658. doi: 10.1038/emboj.2011.68
Xu, X., Wu, Z., Dong, Y., Zhou, Z., Xiong, Z. (2016). Effects of nitrogen and biochar amendment on soil methane concentration profiles and diffusion in a rice-wheat annual rotation system. Sci. Rep. 6 (1), 1–13. doi: 10.1038/srep38688
Xu, M., Zhu, Y., Dong, J., Jin, H., Sun, L., Wang, Z., et al. (2012). Ozone induces flavonol production of ginkgo biloba cells dependently on nitrate reductase-mediated nitric oxide signaling. Envir. Exp. Bot. 75, 114–119. doi: 10.1016/j.envexpbot.2011.09.005
Yelle, S., Gosselin, A., Trudel, M. (1987). Effect of atmospheric CO2 concentration and root-zone temperature on growth, mineral nutrition, and nitrate reductase activity of greenhouse tomato. J. Am. Sci. Hortic. Sci. 112 (6), 1036–1040. doi: 10.21273/JASHS.112.6.1036
Yemets, A. I., Krasylenko, Y. A., Lytvyn, D. I., Sheremet, Y. A., Blume, Y. B. (2011). Nitric oxide signalling via cytoskeleton in plants. Plant Sci. 181 (5), 545–554. doi: 10.1016/j.plantsci.2011.04.017
Yin, R., Hao, Z., Zhou, X., Wu, H., Feng, Z., Yuan, X., et al. (2022). Ozone does not diminish the beneficial effects of arbuscular mycorrhizas on Medicago sativa l. in a low phosphorus soil. mycorrhiza. Mycorrhiza 32 (1), 33–43. doi: 10.1007/s00572-021-01059-w
Young, J. J., Mehta, S., Israelsson, M., Godoski, J., Grill, E., Schroeder, J. I. (2006). CO2 signaling in guard cells: calcium sensitivity response modulation, a Ca2+-independent phase, and CO2 insensitivity of the gca2 mutant. Proceed. Nat. Acad. Sci. 103 (19), 7506–7511. doi: 10.1073/pnas.0602225103
Yue, W., Shao-Ting, D., Ling-Ling, L., Huang, L.-D., Ping, F., Xian-Yong, L., et al. (2009). Effect of CO2 elevation on root growth and its relationship with indole acetic acid and ethylene in tomato seedlings. Pedosphere 19 (5), 570–576. doi: 10.1016/S1002-0160(09)60151-X
Yu, M., Lamattina, L., Spoel, S. H., Loake, G. J. (2014). Nitric oxide function in plant biology: a redox cue in deconvolution. New Phytiol. 202 (4), 1142–1156. doi: 10.1111/nph.12739
Yun, B.-W., Feechan, A., Yin, M., Saidi, N. B., Le Bihan, T., Yu, M., et al. (2011). S-nitrosylation of NADPH oxidase regulates cell death in plant immunity. Nature 478 (7368), 264–268. doi: 10.1038/nature10427
Zhang, M., Dong, J.-F., Jin, H.-H., Sun, L.-N., Xu, M.-J. (2011). Ultraviolet-b-induced flavonoid accumulation in betula pendula leaves is dependent upon nitrate reductase-mediated nitric oxide signaling. Tree Physiol. 31 (8), 798–807. doi: 10.1093/treephys/tpr070
Zhang, H., Li, K., Zhang, X., Dong, C., Ji, H., Ke, R., et al. (2020). Effects of ozone treatment on the antioxidant capacity of postharvest strawberry. RSC Adv. 10 (63), 38142–38157. doi: 10.1039/D0RA06448C
Zhang, Y., Su, J., Cheng, D., Wang, R., Mei, Y., Hu, H., et al. (2018). Nitric oxide contributes to methane-induced osmotic stress tolerance in mung bean. BMC Plant Biol. 18 (1), 1–12. doi: 10.1186/s12870-018-1426-y
Keywords: nitric oxide, greenhouse gases, stress signaling, nitrogen metabolism, abiotic stress
Citation: Kabange NR, Mun B-G, Lee S-M, Kwon Y, Lee D, Lee G-M, Yun B-W and Lee J-H (2022) Nitric oxide: A core signaling molecule under elevated GHGs (CO2, CH4, N2O, O3)-mediated abiotic stress in plants. Front. Plant Sci. 13:994149. doi: 10.3389/fpls.2022.994149
Received: 14 July 2022; Accepted: 10 October 2022;
Published: 01 November 2022.
Edited by:
Rosa Rivero, Center for Edaphology and Applied Biology of Segura (CSIC), SpainReviewed by:
Alla Yemets, National Academy of Sciences of Ukraine (NAN Ukraine), UkraineCopyright © 2022 Kabange, Mun, Lee, Kwon, Lee, Lee, Yun and Lee. This is an open-access article distributed under the terms of the Creative Commons Attribution License (CC BY). The use, distribution or reproduction in other forums is permitted, provided the original author(s) and the copyright owner(s) are credited and that the original publication in this journal is cited, in accordance with accepted academic practice. No use, distribution or reproduction is permitted which does not comply with these terms.
*Correspondence: Byung-Wook Yun, Ynd5dW5Aa251LmFjLmty; Jong-Hee Lee, Y2NyaWxqaEBrb3JlYS5rcg==
Disclaimer: All claims expressed in this article are solely those of the authors and do not necessarily represent those of their affiliated organizations, or those of the publisher, the editors and the reviewers. Any product that may be evaluated in this article or claim that may be made by its manufacturer is not guaranteed or endorsed by the publisher.
Research integrity at Frontiers
Learn more about the work of our research integrity team to safeguard the quality of each article we publish.