- 1Rice Research Institute, Shenyang Agricultural University, Shenyang, China
- 2College of Agronomy, Jilin Agricultural University, Changchun, China
- 3College of Life Sciences, Northwest A&F University, Yangling, China
- 4College of Life Sciences, Changchun Normal University, Changchun, China
- 5College of Life Sciences, Baicheng Normal University, Baicheng, China
- 6Department of Agronomy, University of Agriculture Faisalabad, Faisalabad, Pakistan
- 7College of Life Sciences, Jilin Normal University, Changchun, China
The soybean is a significant legume crop, providing several vital dietary components. Extreme heat stress negatively affects soybean yield and quality, especially at the germination stage. Continuous change in climatic conditions is threatening the global food supply and food security. Therefore, it is a critical need of time to develop heat-tolerant soybean genotypes. Different molecular techniques have been developed to improve heat stress tolerance in soybean, but until now complete genetic mechanism of soybean is not fully understood. Various molecular methods, like quantitative trait loci (QTL) mapping, genetic engineering, transcription factors (TFs), transcriptome, and clustered regularly interspaced short palindromic repeats (CRISPR), are employed to incorporate heat tolerance in soybean under the extreme conditions of heat stress. These molecular techniques have significantly improved heat stress tolerance in soybean. Besides this, we can also use specific classical breeding approaches and different hormones to reduce the harmful consequences of heat waves on soybean. In future, integrated use of these molecular tools would bring significant results in developing heat tolerance in soybean. In the current review, we have presented a detailed overview of the improvement of heat tolerance in soybean and highlighted future prospective. Further studies are required to investigate different genetic factors governing the heat stress response in soybean. This information would be helpful for future studies focusing on improving heat tolerance in soybean.
Introduction
Abiotic stresses are severe environmental factors affecting crop growth and yield (Yang et al., 2017; Saleem et al., 2020; Zafar-Ul-Hye et al., 2020), undermining global food security (Rasheed et al., 2020a,b,c, 2021a,b), and affected the large area under cultivation (Ashfaq et al., 2021). Heat stress is most disturbing abiotic factor that disturbs plant growth, development, and yield (Mittler and Blumwald, 2010; Suzuki et al., 2014; Nakagawa et al., 2020). For example, extreme heat stress occurred in the United States of America (USA), resulting in a yield loss estimated about 7.1 billion US dollars (Mittler, 2006). Comparable events happening worldwide and caused instability in social and economic aspects and mass migration, and China is also facing extreme heat waves (Figure 1) in many regions (Ault, 2020; Xie et al., 2021). Multiple research studies have shown the effects of heat stress on soybean growth, yield, and quality (Cohen et al., 2021b). In soybean, efforts have been made to develop genotypes with enhanced yield under extreme and prolonged heat waves (Mittler, 2006). Many studies have observed yield loss when soybean was exposed to short-term and long-term heat waves (Hatfield and Dold, 2019; Thomey et al., 2019; Zandalinas et al., 2020) and season-long warming (Ruiz-Vera et al., 2013). Soybean meal is used as the main source of protein in livestock feeding (Consultation, 2004) and harvested on 82 million acres in the USA in 2020 (Ortiz et al., 2022).
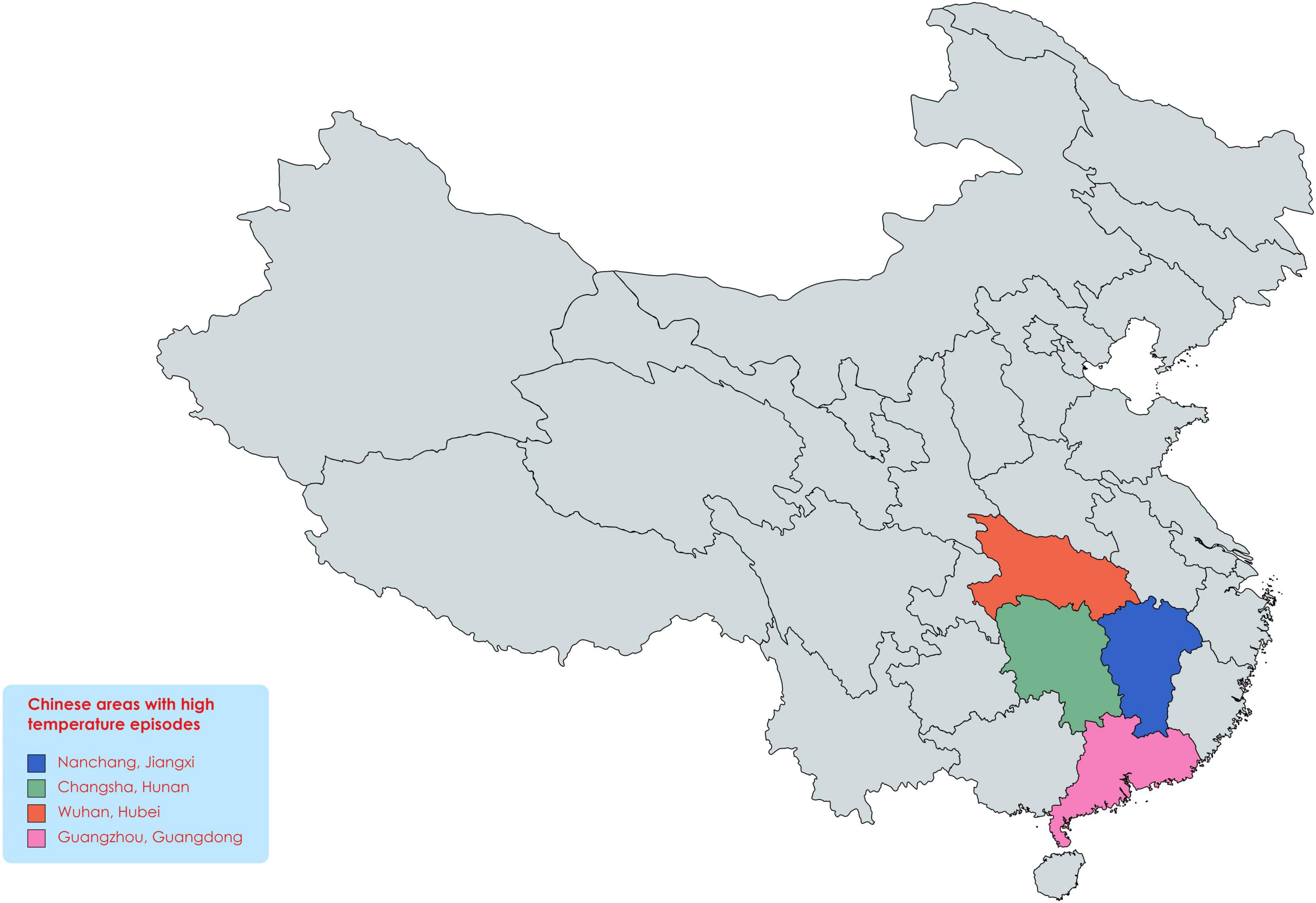
Figure 1. Areas with extreme heatwaves in China. As global warming in rising, the heat waves in these areas continue to increase, which will have devastating effects on crop production in the coming time.
Heat stress significantly affected the soybean seed germination, resulting in poor seed germination (Hamayun et al., 2021; Veas et al., 2021), increased seed vulnerability to pathogen attack, and reduced its economic value (Wang et al., 2018). Climate change is expected to be more severe and increase the severity of heat waves and their impact on seed development during the current century (Chebrolu et al., 2016; Huang et al., 2019). Heat stress affects soybean root growth, alters morphology, and restricts elongation (de Moraes and Gusmao, 2021). Heat stress reduces carbon assimilation, causes stomatal closure and membrane injury, and decreases the activity of several enzymes (Farooq et al., 2009). Heat stress impairs the metabolic pathways (Kosová et al., 2011), which results in altered plant growth (Kosová et al., 2011; Liu et al., 2014). Climate change is expected to increase the incidence and severity of summer heat waves (Balfagón et al., 2022; Ullah et al., 2022), and the impact of heat stress on seed development will become more widespread (Haider et al., 2022) and prolonged during current century (Chebrolu et al., 2016; Sun et al., 2022). Episodes of heat waves are expected to increase in intensity in the coming time due to global warming and climate changes (Balfagón et al., 2019; Ault, 2020; Grossiord et al., 2020). Hence, breeding crops for increased tolerance to heat stress is one of the most critical needs of modern agriculture (Bailey-Serres et al., 2019; Zandalinas et al., 2020). In addition to its effects on growth and biomass, its prolonged period could have a more deadly impact on yield and quality (Cohen et al., 2021b).
Therefore, the presence of heat-tolerant soybean genotypes is vital for global food security, and exploiting genetic variation for heat response could play a significant role in crop development (Ergo et al., 2021; Rustgi et al., 2021). Quantitative trait loci (QTL) mapping is a most powerful technique (Xu et al., 2013; Zhang et al., 2017; Rasheed et al., 2022b), which allows the identification of genomic regions controlling heat tolerance in soybean (Bazzer and Purcell, 2020; Gillman et al., 2021). Soybean plants have been exposed to extreme heat waves, and many significant QTL have been discovered. Soybean exposure to heat waves under controlled conditions would identify novel QTL, which can be cloned and transferred to develop heat-tolerant cultivars. Likewise, genetic engineering plays a crucial role in enhancing heat tolerance in soybean (Rahman et al., 2022; Vital et al., 2022). These techniques have brought fruitful results in molecular biology research (Xu et al., 2019). Likewise, the transcriptome technique has discovered several genes governing the heat tolerance mechanism in soybean (Wang et al., 2018; Katam et al., 2020). CRISPR/Cas9, a novel gene-editing tool, can potentially edit any desired gene of interest in the soybean genome to bring novel variation for heat tolerance (Duan et al., 2021). However, the large-scale application of this tool in soybean needs more studies and research. In the future, using different Cas9 variants would enable any desired mutation in the soybean genome (Kim, 2017; Huang et al., 2019).
Several studies deal with the improvement of soybean heat tolerance; however, complete genetic control of this trait is yet not fully understood. Heat waves are becoming more and more intense every year, creating a significant threat to global food security by reducing crop yields. This review has critically elaborated several classical and molecular techniques to develop heat-tolerant soybean genotypes. The current review highlighted the potential research gaps that should be covered in future research studies. This review would be a potential source of literature for future research studies dealing with heat tolerance in soybean.
Effects of heat stress on soybean growth, physiological and biochemical parameters
Plants face series of harsh environmental factors including biotic and abiotic stresses (Ahmad et al., 2022; Khan et al., 2022). Heat stress negatively affects soybean growth and development. Chebrolu et al. (2016) evaluated soybean heat tolerant and susceptible genotypes under heat stress conditions. Heat stress (42°C) significantly affected the seeds germination (Figure 2) of the heat susceptible genotype, while these parameters remained unaffected in the heat-tolerant genotype. Heat stress affected the seed protein contents and protein storage vacuoles of soybean when plants were exposed to 42°C. Heat stress disturbs the vacuole structure and membrane integrity that stores protein (Krishnan et al., 2020). Likewise, in another study, it was noted that extreme heat stress decreased the contents of salicylic acid (SA) in soybean after exposure for 5–10 days (Khan et al., 2020). Heat stress also affected the activity of different metabolites and amino acids in soybean. Carbohydrates, lipids, proteins, and secondary metabolites were involved under heat stress, and their concentration was reduced when plants were exposed to extreme heat stress (Das et al., 2017). Heat stress negatively affected the seed production in soybean genotypes, ultimately affecting yield. Cohen et al. (2021a) evaluated the soybean genotypes (Magellan and PI 548313) under heat waves to study seed production and yield per plant. Results revealed that PI 548313 yielded more seeds than Magellan under heat stress. These findings indicated that heat stress could affect any plant growth stage and decrease the final yield per plant in soybean (Cohen et al., 2021a). Heat stress damaged the plant leaf and reduced soybean soluble sugar and proline contents when plants were exposed to heat stress for 24 h (Wang et al., 2018).
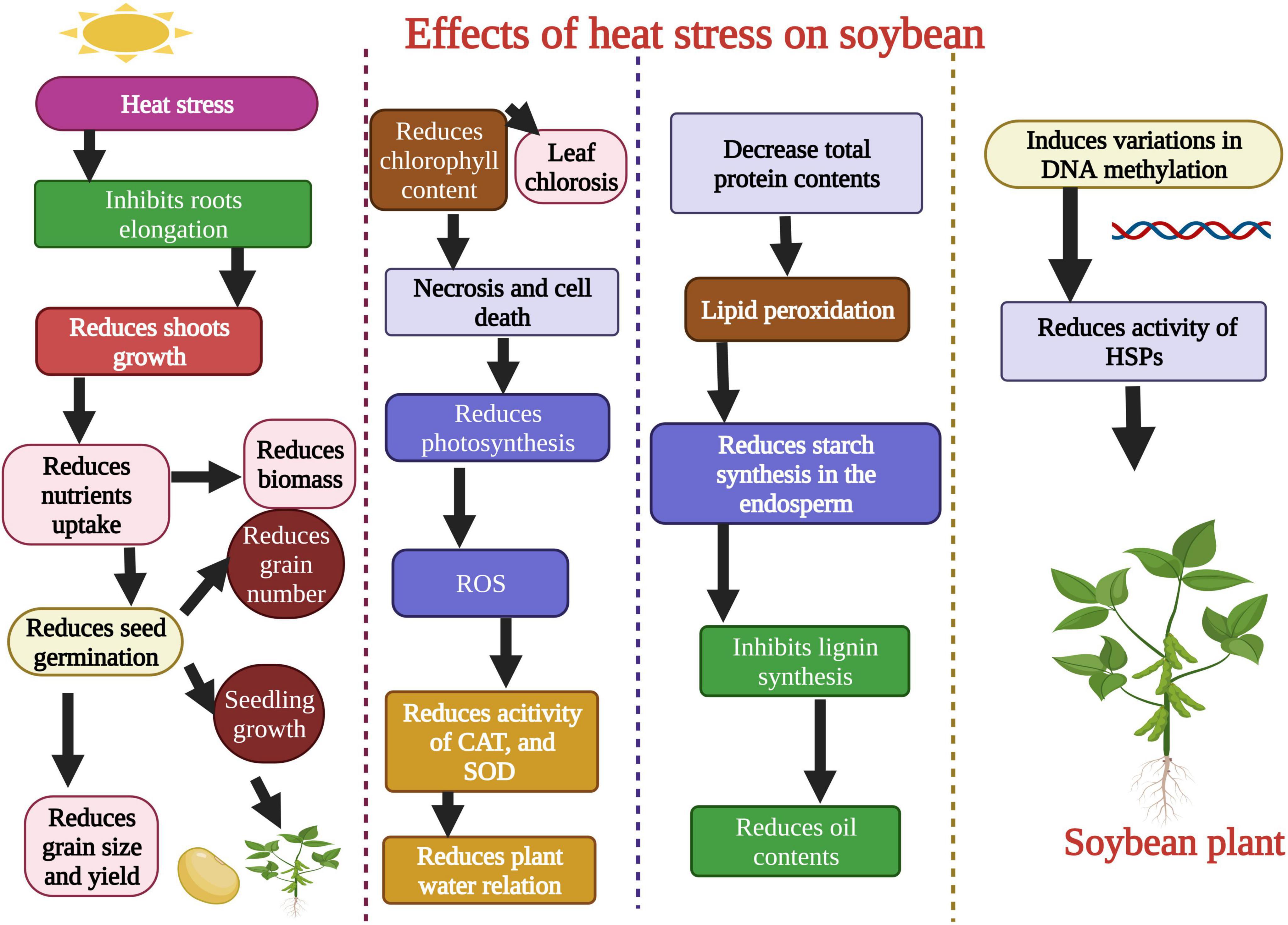
Figure 2. Effects of heat stress on morphological, physiological, biochemical, and molecular level of soybean. Heat stress affects the shoot growth, root growth, reduces seed germination, produces reactive oxygen species (ROS), decreases the activity of antioxidant enzymes, and total protein contents. Heat stress causes changes in DNA methylation and gene’s function. This Figure is created with BioRender.com.
Heat stress affects the soybean root growth and structure (Valdés-López et al., 2016). In another study, heat stress reduced soybean plant chlorophyll content (Figure 2). Heat stress also reduced the accessibility of assimilates to soybean grains and reduced the sink-grain metabolization from young leaves (Ergo et al., 2018). Sharifi et al. (2022) studied the detrimental effects of heat stress on soybean growth, physiology, and biochemical parameters. They found that heat stress reduced the shoot and dry root weight, induced oxidative damage, and decreased the stomatal conductance (Table 1; Sharifi et al., 2022). The soybean yield was also affected under heat stress conditions. High-intensity heat waves reduced the soybean yield by affecting flowering and pod filling stages (Thomey et al., 2019). In another study, Cohen et al. (2021a) studied the detrimental effects of heat stress on soybean reproductive organs like stigma, ovaries, pollens, and flowers, which resulted in lower seed production. Further studies are required for a detailed investigation of the effects of heat stress on the activity of antioxidants, osmolytes, and specific biochemical components of soybean. Heat-induced changes in the genetic material of soybean are critical to understanding to adopt preventive measures.
Molecular mechanism of heat tolerance in soybean
The molecular mechanism of heat tolerance is very complex and governed by multiple genes (Zhang et al., 2022a). Incomplete knowledge of molecular factors underlying heat tolerance in soybean has hindered the research progress (Zhang et al., 2022a). The plant adopts several techniques to cope with heat stress (Katam et al., 2020; Sharifi et al., 2022). Many genes, enzymes, and proteins are crucial in improving soybean heat stress tolerance. Heat stress induced the overexpression of the DREB1 gene family, several dehydrins, and LEA genes, their over-representation between upregulated genes in soybean under heat stress (Kidokoro et al., 2015). Likewise, overexpression of enzymes involved in homeostasis, as well as accumulation of chaperone proteins, mainly heat shock proteins, were observed (Kosová et al., 2011).
Consequently, proteomic analysis under multiple stresses leads to a better understanding of the molecular paths and the molecules related to heat tolerance. Earlier studies also showed the relative abundance of leaf proteins under heat stress conditions in soybean (Ashoub et al., 2015). These studies showed that soybean plants adopt various pathways and mechanisms to cope with high-temperature circumstances. However, some recognized genes and pathways could be employed to improve heat tolerance in soybeans using molecular breeding approaches (Sabagh et al., 2020). Future studies on the molecular mechanism of heat stress tolerance in soybean would identify the different genes and pathways controlling heat tolerance in soybean.
Classical breeding approaches to enhance heat tolerance in soybean
One way to meet future food demands is to develop heat-tolerant soybean cultivars that maintain high yields under heat waves. Heat tolerance is a polygenic trait. It is critical to identify the major contributors to this trait and use molecular markers for breeding using a specific breeding program (Rustgi et al., 2021). A successful breeding program needs significant genetic diversity for a given trait. Crossing closely related individuals can decline genetic variability (Gur et al., 2004). The primary step is the characterization of a shared gene pool, including landraces and wild relatives, which offers the identification of numerous tolerant genes for abiotic tolerance. Wild relatives are opportunistic resources for soybean breeding (Lam et al., 2010; Nawaz et al., 2018). Soybean wild relative Glycine soja is usually considered the uncultivated ancestor of the domesticated soybean (Stupar, 2010). Glycine max and Glycine soja are phenotypically dissimilar in many behaviors, but they readily cross with one another and give rise to productive hybrids for several key features (Stupar, 2010). Comparative genome sequencing of cultivated and wild soybean relatives will enhance our understanding of the limitations of domesticated germplasm and the potential to exploit wild germplasm for breeding programs (Stupar, 2010). The wild soybean genome has more allelic diversity than cultivated soybean. In an earlier study by Lam et al. (2010), resequencing 14 cultivated and 17 wild soybean genomes identified greater allelic diversity (Lam et al., 2010). These studies showed that wild soybean relatives are treasure troves to increase genetic diversity for abiotic stress tolerance by contributing novel alleles (Mammadov et al., 2018).
Significant efforts have been made using conventional breeding to screen heat-tolerant genotypes, which can be used to sustain growth and yield under changing environmental conditions (Jagadish et al., 2008).
Conventional screening techniques for heat tolerance showed promising results in many crop species (Jagadish et al., 2008). Breeding programs were carried out in heat stress areas that endorse crop selection for thermo-tolerant traits. Understanding the genetic and physiological-based heat tolerance mechanisms will help to develop heat tolerant cultivars suitable for sustainable growth under heat waves (Shanmugavel et al., 2020). Alsajri et al. (2019) screened 64 soybean cultivars using certain morphological and physiological traits for heat stress tolerance. They have identified 45A46 (Table 2) high heat tolerant cultivar based on their results. Mass selection, hybridization (Figure 3), pure line selection, and backcrossing methods are widely used for breeding heat-tolerant soybean cultivars. Hybridization is one of the most reliable breeding methods and leads to the development of hundreds of heat-tolerant soybean cultivars (Singh and Nelson, 2015; Mammadov et al., 2018). The soybean line (TN09-239) is an MG5 line developed via four circles of backcrossing (Boehm et al., 2017), and the soybean line (PI587982A) is a plant introduction collected in Sichuan Sheng, China, and currently, USDA-GRIN germplasm collection. PI587982A has been confirmed to have prolonged tolerance to the adverse influences of heat stress during seed filling (Smith et al., 2008; Gillman et al., 2019). Modified breeding techniques would assist in speeding up the development of heat-tolerant soybean cultivars.
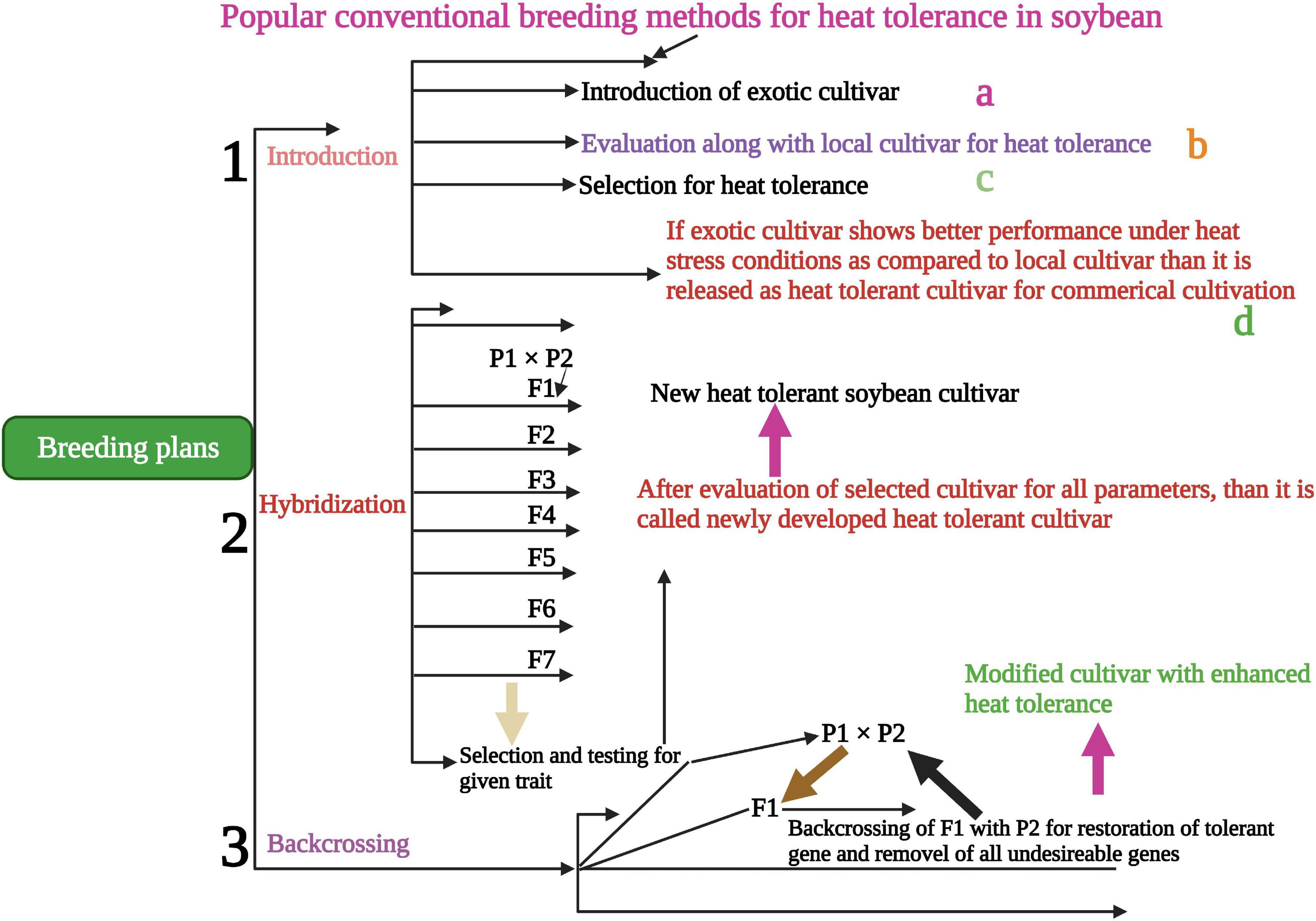
Figure 3. Conventional breeding methods for the development of heat-tolerant soybean cultivars. Hybridization, backcrossing and introduction are powerful traditional ways to develop tolerant soybean cultivars to sustain yield in heat stress conditions. This Figure is created with BioRender.com.
Use of phytohormones for heat tolerance in soybean
Different conventional approaches have been used to enhance heat tolerance in soybean. Earlier reports suggested that certain plant hormones mitigated the toxic effects of heat waves in soybean. The plant has adopted various mechanisms that help to cope with increasing episodes of heat stress. These modifications include leaf orientation, changes in lipid composition in the membrane, activation of the antioxidant defense system, and hormonal regulation (Hasanuzzaman et al., 2013). Heat stress causes ROS overproduction and oxidative damage to DNA, proteins, and lipids (Liu et al., 2019; Haider et al., 2022). Phytohormones control plant growth and development under extreme heat waves. Mineral fertilizers greatly affect the crop growth and production (Amanullah Ilyas et al., 2021; Amjad et al., 2021), so eco-friendly techniques are required to enhance yield under abiotic stresses (Sabagh et al., 2019). A slight change in hormonal concentration affects the cellular dynamics; hence, they have a crucial role in controlling the plant response to heat stress (Ozga et al., 2017; Singh and Meena, 2020). In general, auxin (AX), gibberellins (GA), and cytokinin (CK) involve in the regulation of heat tolerance under heat waves (Ozga et al., 2017). Khan et al. (2020) revealed that heat stress increased the concentration of ABA and SA in soybean, indicating that these phytohormones could increase heat tolerance to sustain growth and yield (Khan et al., 2020).
Ethylene has a negative role in heat tolerance in plants (Rial et al., 2022). Earlier studies reported that heat stress leads to the increase in the production of ethylene contents along with oxidative damage, which lowers the pod setting percentage (Djanaguiraman et al., 2011). Abscisic acid (ABA) and GA are key hormones in regulating seed germination and dormancy. These hormones modify the growth and development of crops during heat stress (Manan and Zhao, 2020). Auxin was applied to soybean seeds which increased the germination percentage by increasing the biosynthesis of ABA (Shuai et al., 2017). However, hormonal content alterations and signaling in soybean seed germination under heat episodes remain unclear and need further studies (Sabagh et al., 2020). Ethylene (ET) is a main gaseous hormone that affects seed germination, growth and development, and fruit production under heat stress (Meena et al., 2020b). This hormone provides resistance to plants against abiotic stresses (Cao et al., 2007; Meena et al., 2020a). Ethylene triggers the expression of genes involved in heat tolerance and affects the osmolytes, which can protect plants under heat stress (Cui et al., 2015).
Further studies are needed to investigate the effect of ethylene on seed germination under adverse environmental conditions (Cui et al., 2015). Leaf senescence in soybean occurs during the reproductive stage and can be increased under heat stress. In a recent study, ethylene role in premature leaf senescence was investigated in soybean. The results identified that heat stress increased ethylene production in soybean leaves. These results showed the prominent role of ethylene in mitigating the adverse effects of heat stress in soybean (Djanaguiraman and Prasad, 2010). However, further studies must critically investigate ethylene role in inducing soybean heat tolerance (Djanaguiraman and Prasad, 2010).
Melatonin (MT) is one of the most significant and vital osmoprotectants, is involved in numerous physiological processes in plants, and has a crucial role in triggering the antioxidant defense response in plants. Therefore, the uses of MT in plants are being assessed by many researchers. Wei et al. (2015) examined the effect of MT on the growth and development of soybean. Results revealed that MT application increased soybean seed size and plant height. Besides this, MT also enhanced the seed and pod numbers, but it did not increase the 100-seed weight under abiotic stresses (Wei et al., 2015). Likewise, MT use could increase the soybean tolerance to heat stress; the evidence shows that contrary environmental circumstances can increase the MT concentration in plants as a defensive response. In recent studies, Imran et al. (2021) revealed the role of MT a vital plant growth hormone, in reducing the harmful effects of heat waves on soybean. Heat stress produced ROS and disturbed plant growth and development. By foliar application of MT, plant growth and photosynthetic pigments (Figure 4) also increased in heat-stressed soybean seedlings.
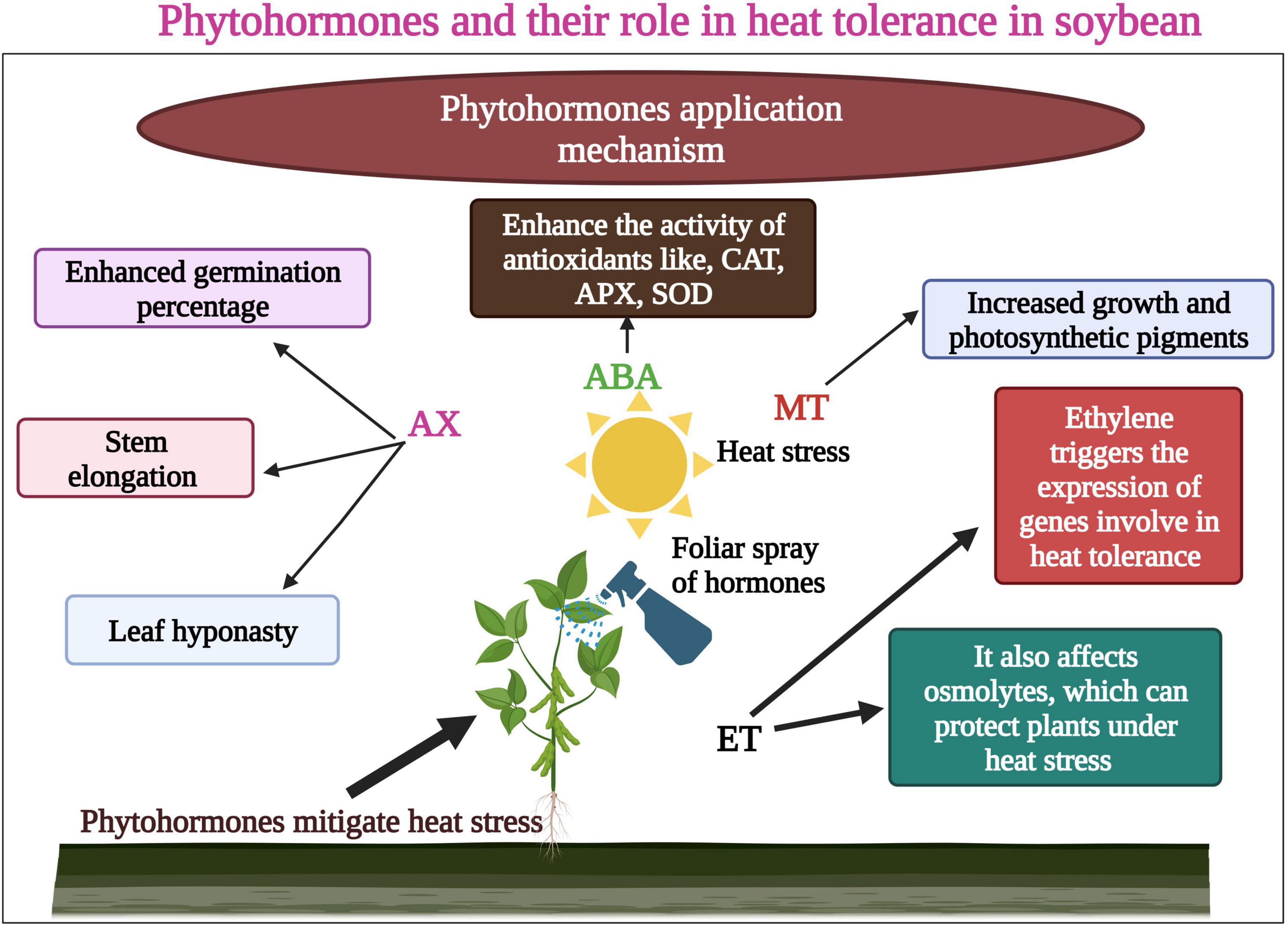
Figure 4. Role of phytohormones in heat tolerance in soybean. Hormones enhance seed germination, stem elongation, and enhanced photosynthetic pigments. Ethylene (ET) affects osmolytes which protect plants under heat stress. ET also enhances the function of genes involved in heat tolerance. This Figure is created with BioRender.com.
Further, MT induced the expression of heat shock proteins and transcription factors, which indicated ROS detoxification (Imran et al., 2021). In another study, Kumar et al. (2021) revealed that MT promotes cell division, isoflavones, and ethylene biosynthesis in soybean under heat stress. The use of phytohormones to mitigate the toxic effects of heat stress in soybean needs further investigation (Kumar et al., 2021). Modified concentrations of hormones at different soybean growth stages under heat stress would help us sustain the soybean growth and yield in changing environments. The hormones and their interactions with the plant at different growth stages are critical to understanding. Further studies are required to investigate the potential role of SA in mitigating the adverse effects of heat stress in soybean.
Other adaptive mechanisms
Under heat stress conditions, the plant adopts various mechanisms like short-term escape and avoidance, changed leaf orientation, membrane lipid functions, cooling, early maturity, and so on for survival (Shanmugavel et al., 2020). The plant also shows different degrees of leaf rolling under heat stress (Sarieva et al., 2010). Increased stomatal activities, a waxy layer, and enhanced xylem vessels are common survival tactics during heat stress. The plant also adopts a long-term tolerance mechanism to survive under heat stress (Srivastava et al., 2012). The use of antioxidants, late embryogenesis, and dehydrins are the main factors used to counter the harmful effects of heat stress. Osmolytes like proline, trehalose, and glycine betaine (GB) play a crucial role in osmotic adjustment, ROS scavenging, and membrane function stabilization under heat stress (Verbruggen and Hermans, 2008; Campobenedetto et al., 2020).
Numerous enzymatic and non-enzymatic antioxidant defense mechanisms are also involved in defense against oxidative stress brought by free radicals (Apel and Hirt, 2004). The action of enzymes involved in the scavenging ROS is temperature specific. Most of the antioxidant enzymes show improved activity with the rise in temperatures. It is also affected by the plant genotype, planting season, and phenological phases (Almeselmani et al., 2006). Campobenedetto et al. (2020) studied the role of lignin-derived biostimulants seed treatment in heat tolerance in soybean. They have observed a dramatic reduction in H2O2 activity associated with a substantial increase in non-protein thiols. These findings suggested the lower oxidative stress in biostimulants treated seed (Campobenedetto et al., 2020). These results offer perceptions of the biostimulants mechanism of action and its uses for seed treatment to enhance heat stress tolerance during seed germination in soybean (Campobenedetto et al., 2020). Nutrients also increase plants growth and yield under abiotic stress conditions (Saud et al., 2017) and increasing plant nutrition can lead to better adaptation under changing environmental conditions. (Ali et al., 2022a,b). The defense role of antioxidant enzymes needs to be improved to help plants withstand heat stress effects. Early maturing soybean should be the primary breeding target in future studies.
Molecular techniques for heat tolerance in soybean
Genome-wide association study (GWAS) also assists in identifying traits-marker associations and knowing the genes controlling heat tolerance in soybean (Longmei et al., 2021). Using genomic studies and phenotypic data can provide information to the crop breeders to make a rapid selection and use advanced breeding tools to develop heat resilient crops. GWAS is one of the most promising tools to identify the QTL underlying particular traits of interest. The genetic control of heat stress analysis via association mapping would accelerate breeding programs (Kulwal et al., 2011). GWAS would identify the genomic regions governing the essential genes by conducting statistical links between traits variation and DNA polymorphism in extensive germplasm collection (Pandey et al., 2014). GWAS has increased the mapping resolution of genes/QTL in many crops (Ma et al., 2012; Liu et al., 2013). GWAS not only simplified to recognition of the marker-trait link but also advanced our knowledge of the genetic construction of complex quantitative characters (Yan et al., 2011). In response to heat stress, many stress-responsive proteins have been identified as heat shock proteins (HSP), indicating the plant tolerance threshold (Wang et al., 2004). Das et al. (2016) identified the 25 heat stress-related protein in soybean and revealed that EF-Tu triggered heat tolerance mechanisms. A higher level of HSPs regulated the heat tolerance mechanisms in soybean (Das et al., 2016). Katam et al. (2020) also studied protein’s response to soybean heat stress. These studies highlighted protein’s based effective heat tolerance mechanism (Katam et al., 2020). Proteins expression significantly changed within 3 h of heat stress in soybean roots. Proteins played significant role in heat tolerance (Valdés-López et al., 2016). Meanwhile, several other multi-omics techniques have great potential to enhance abiotic stress tolerance in crops, mainly using epigenomes in soybean (Chu et al., 2020). These techniques include genomics and microbiome (Liu et al., 2020; Pandian et al., 2020). Cao et al. (2022) presented a detailed review on use of multi-omics techniques for soybean molecular breeding. Machine learning tools play role in understanding the plants and environmental interactions. Hoffman et al. (2020) studied the soybean yield response to low temperature using Random Forest (RF), a diagnostic machine learning tool (Hoffman et al., 2020). Earlier, real time phenotyping framework using machine learning techniques was done in soybean to study the plant stress severity rating (Naik et al., 2017). Although the available data about these novel techniques for heat tolerance in soybean is insufficient, the critical information reported on other crops would assist in applying these powerful tools for developing heat tolerant soybean genotypes.
Identification of quantitative trait loci for heat tolerance in soybean
Due to certain limitations of classical breeding, the development of heat-tolerant soybean genotypes is often limited. Conventional techniques are time-consuming, costly, and cannot fix the desired traits for a long time (Dhungana et al., 2021; Gillman et al., 2021). QTL mapping leads to identifying certain novel QTL controlling heat tolerance in soybean. Climate change may result in prolonged heat stress during germination and growth (Zhang et al., 2022b). The ancestors of modern US high-yielding soybean lack potential resistance to heat stress; however, some plant cultivars can maintain 95% yield and quality under extreme heat stress (Gillman et al., 2021). Gillman et al. (2021) evaluated the backcross recombinant inbred line (BRILs) population for seed emergence and germination produced in six greenhouses. They have found a novel QTL derived from “PI587982A” correlated with significant effects on overall seed germination and emergence. These results revealed that marker-assisted selection (MAS) could be practiced to increase tolerance to heat stress and may lead to the development of cultivars with high-quality seeds in soybean (Gillman et al., 2021). Using a stable mapping population and wild relatives of soybean would be fruitful in identifying novel QTL and potential genes network behind heat tolerance and developing heat-tolerant cultivars (Gillman et al., 2021). The information about QTL mapping for heat tolerance in soybean is limited, strongly emphasizing further studies using diverse mapping populations to identify the genomic regions controlling heat tolerance in soybean (Gillman et al., 2021). The process needs evaluation of soybean in different temperature regimes to validate the potent QTL and use them in MAS to develop heat-tolerant soybean genotypes.
Genetic engineering for heat tolerance in soybean
Genetic engineering leads to the development of several heat-tolerant soybean genotypes (Arce-Paredes et al., 2011). Transgenic soybean expressed transgene in high-temperature regimes and showed a high yield compared to control genotypes. Heat shock proteins are an essential component of plant response to heat stress. Earlier, Huang et al. (2019) investigated the role of the GmHsp90A2 gene in soybean using transgenic lines generated by CRISPR/Cas9 system. Agrobacterium tumefaciens strain EHA105 was used for plant transformation. The T3 transgenic soybean plants with overexpression of GmHsp90A2 exhibited heat tolerance through lower oxidative stress and high chlorophyll contents.
On the other hand, T2 transgenic lines showed high oxidative stress and low chlorophyll contents (Huang et al., 2019). Likewise, in another study, 75 non-redundant AQP encoding genes were recognized in soybean. Results revealed that the AQP gene- GmTIP2;6 was involved in heat tolerance in soybean. This gene enhanced plant growth during heat stress, and these results provided the way to understand the molecular mechanism of GmAQPs in mediating heat stress (Feng et al., 2019). The ortholog of soybean GmGBP1 positively regulated the heat tolerance not only in soybean but also in tobacco. This gene enhanced seed germination during heat stress and contributed to thermal tolerance in soybean (Zhao et al., 2013).
Soybean family protein, GmEF8 enhanced heat tolerance by increasing the proline level in transgenic lines. These results revealed the potential role of the GmEF8 (Figure 5 and Table 3) gene under heat stress. They provided a foundation for further studies to understand the complexities of stress transduction pathways (Zhang et al., 2022a). The soybean gene, Gmdnj1 positively regulated the heat tolerance in transgenic lines. Results showed that Gmdnj1 enhanced heat tolerance by searching misfolded proteins for refolding to sustain the total capacity of cellular roles (Li et al., 2021). Nowadays, transgenic techniques are practiced using efficient transformation vectors. However, available data is insufficient to comprehensively evaluate transgenic lines under extreme heat stress conditions. It would be better to generate a substantial germplasm center and identify the gene for heat tolerance and transfer using Agrobacterium vectors (Li et al., 2021). Transgenic soybean performed best under extreme heat stress conditions; however, further studies are required to identify more potent genes for genetic engineering to develop heat-tolerant genotypes.
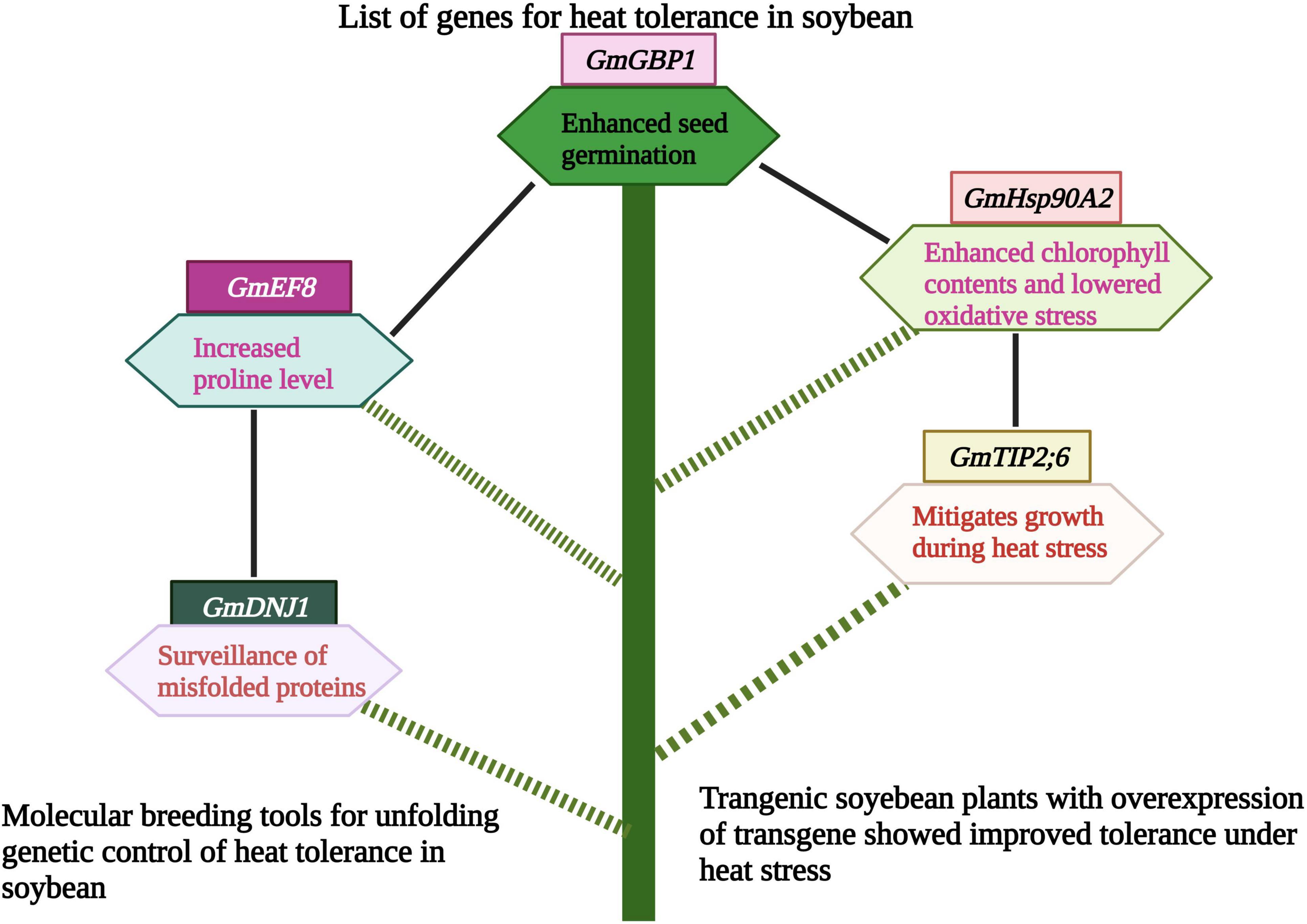
Figure 5. Role of different genes/proteins in heat tolerance in soybean. Heat tolerance genes protect soybean plants by various mechanisms like, increasing proline level, mitigating growth and photosynthesis. This Figure is created with BioRender.com.
CRISPR/Cas9 role in heat tolerance
Soybean tolerance to heat stress is often limited because of the complexity of its genome. With time, the emergence of novel gene-editing tools like CRISPR/Cas9 broke all biological barriers (Ghosh and Dey, 2022; Rasheed et al., 2022a; Razzaq et al., 2022). Increased heat waves threaten food security and damage the soybean crop on large areas (Ahmad et al., 2021; Chennakesavulu et al., 2021; Camerlengo et al., 2022; Pandita, 2022). CRISPR/Ca9 has emerged as a key gene-editing tool for efficient and precise gene-editing of soybean crop; however, little evidence indicates its role in heat tolerance. There are possibilities of large-scale use of this tool in future research studies. Secondly, CRISPR/Cas9 variants would expand its role in increasing soybean tolerance to not only heat stress but also other abiotic stresses. Earlier, CRISPR/Cas9 successfully edited the heat tolerant genes in soybean (Huang et al., 2019). CRISPR/Cas9 edited two genes, GmpPLA-IIε and GmpPLA-IIζ, in response to multiple abiotic stresses. These findings revealed that these genes could also play a key role in heat stress tolerance in soybean. The mutants would be valuable genetic materials to investigate the molecular mechanism governing soybean response to multiple abiotic stresses (Xiao et al., 2021) and hopefully for heat tolerance. Yang et al. (2022) also applied CRISPR/Cas9 to edit the heat shock protein, GmHSP17.9 The resulting mutant plants showed a remarkable variation in nodule number, nodule fresh weight, and nitrogenase activity. These findings reveal an unprecedented molecular mechanism of heat shock protein in nodule development and counter heat stress in soybean. Hopefully, use of CRISPR/Cas9 will increase in soybean to develop heat resilient cultivars. Complete genome sequencing has been completed in soybean, which will be helpful in the future to screen for heat tolerant genes that can be targeted by CRISPR/Cas9.
Transcription factors for heat tolerance in soybean
Transcription factors are proteins that regulate gene expression in soybean (Liu et al., 2022a,b; Min et al., 2022; Yin et al., 2022). Hundreds of TFs have been identified and characterized for heat tolerance in soybean. To respond to heat waves, plants activate heat shock proteins characterized by their highly conserved modular structure and motif. In an earlier study, a potent heat shock TF, GmHsfA1, was identified and distinguished from expressed sequence tag database of soybean by sequence comparison with LpHsfA1 and cloning of complementary DNA (cDNA). Soybean transgenic plants overexpressed GmHsfA1, leading to the activation of GmHsp70 under control conditions and overexpression under high temperatures. The overexpression of GmHsfA1 in transgenic plants enhanced thermotolerance (Zhu et al., 2006).
In the same way, in another study, a novel GmHSFA1was cloned from the soybean genome. Results showed that expression of GmHSFA1 was higher in transgenic lines than in non-transgenic soybean. GmHSFA1 increased thermotolerance in transgenic soybean by activating several genes (Chen et al., 2006). Several abiotic factors restrict the soybean yield, and no soybean genotype with improved tolerance to heat stress is commercially available.
Ribichich et al. (2020) transformed the sunflower TF, HaHB4 into soybean, which confers drought tolerance in Arabidopsis. Results showed that transgenic plants showed greater heat tolerance in field conditions. It was assumed that this TF, HaHB4, could control soybean yield under warm conditions (Ribichich et al., 2020). Soybean TF, DREB1, plays a crucial role in activating gene expression in response to heat stress. 14 DREB1-type TFs were identified from the soybean genome database. The expression of GmDREB1 was enhanced by heat stress. Results suggested that GmDREB1 proteins activate the heat-responsive genes in soybean and increase heat tolerance (Kidokoro et al., 2015). Likewise, heat shock TF GmHsfs also activates heat-responsive genes in soybean. This TF regulates heat shock protein expression in response to extreme heat waves in soybean (Chung et al., 2013). Li et al. (2021) identified a type-I HSP40 gene GmDNJ1 in soybean, which is highly inducible under heat stress. Mutants of Gmdnj1 exhibited reduced chlorophyll contents, oxidative stress, and higher induction of heat-responsive genes. GmDNJ1 plays a key role in plant growth and development under heat stress via the regulation of misfolded proteins (Li et al., 2021). Transcription factor BREB2 in soybean enhanced heat tolerance responses by activating several genes. The regulatory mechanism of DREB2 in soybean is not clear. The novel DREB2 gene, GmDREB2A;2, was highly induced under heat stress (Table 4). This gene has a key role in thermotolerance in soybean and can be used as a candidate gene for future studies (Mizoi et al., 2013).
In soybean, GmEF8 is an elongation factor family protein determined by interaction with GmCBL1, and GmEF8 expression was induced by heat stress. Soybean plants with enhanced tolerance to heat stress showed overexpression of GmEF8 in hairy roots and had a high proline level compared to plants with control hairy roots (Zhang et al., 2022a). Earlier, Song et al. (2017) performed an RNA-seq analysis using two soybean varieties under extreme stress. They analyzed the transcripts and obtained 2,458 differentially expressed genes. In the end, 68 genes were found involved in heat shock proteins under heat stress. As a result, small HSP families were found to be upregulated and expressed in prolonged heat stress conditions. These findings provided valuable information about heat tolerance in soybean (Song et al., 2017). Heat shock TFs play a key role in helping soybean plants in extreme heat stress episodes. In another study, thirty-eight Hsfs were analytically recognized, assembled into three classes and 12 subclasses, and positioned on 15 soybean chromosomes. A1 and A2 subclass showed a high transcription level. Numerous genes were selected for their subcellular localization. Results of subcellular localization showed that GmHsf-04 and GmHsf-34 were positioned in the nucleus. GmHsf-34 gene overexpressed and improved soybean tolerance to heat stresses (Li et al., 2014). Despite the significant progress in enhancing heat tolerance by identifying TFs, the actual and core mechanism of TFs under heat stress is not fully understood. It would be better to expose soybean plants to extreme heat episodes and identify the potential TFs involved in heat tolerance. It would be better to fully characterize the soybean genome (Aleem et al., 2022; Goodman and Kiser, 2022), arrange the gene into groups based on their expression in a cell under abiotic stress and select the genes with high overexpression (Zhang et al., 2022c). Different TFs families, WRKY, bZIP, and NAC should be studied to identify the family members involved in heat tolerance. TFs could be a potential target for genetic engineering and CRISPR/Cas9.
Transcriptome analysis for heat tolerance in soybean
Transcriptome analysis plays a crucial role in identifying stress-responsive genes in soybean (Sun et al., 2021; Chen et al., 2022; Ma et al., 2022; Tariq et al., 2022). Soybean has a complex genetic mechanism for heat tolerance. Knowledge about soybean genetic mechanism of heat tolerance is critical for molecular breeding (Yang et al., 2022). To identify the genetic mechanism of heat stress and open ways for molecular breeding, researchers have conducted several studies to identify the potential gene involvement in heat tolerance in soybean. Wang et al. (2018) used RNA-sequencing to investigate the transcriptional response of soybean toward heat stress. Several genes involved in defense, metabolic pathways, photosynthesis, and morphological responses are expressed differently under heat waves. Results showed 1,468 and 1,220 upregulated and 1,146 and 686 down-regulated genes after heat treatment (8 h and 24 h). These genes are actively involved in defense response. These genes improved heat tolerance in soybean via molecular breeding (Wang et al., 2018). Likewise, in another study, transcriptome analysis was performed to investigate the role of lignin-derived biostimulants seed treatment in enhancing heat tolerance in soybean. RNA-Sequence analysis showed 51 upregulated genes and 828 down-regulated genes in biostimulants treated seed after 24 h of treatment compared to control. Down-regulated genes were involved in heat stress-responsive and hormonal signaling. Genes activation under heat stress improves heat tolerance via molecular breeding (Campobenedetto et al., 2020).
Soybean roots play a crucial role in developing the symbiotic association with microorganisms, but unfortunately, few studies described their possible response to heat stress. Genome-wide transcriptome and proteomic analyses were performed on isolated soybean roots to investigate their response to heat stress. On average, 1,849 and 3,091 genes that were differentially expressed under heat stress were identified. Only ten essential genes were involved in the heat-responsive mechanism in soybean. Besides this, a proteomic analysis was also performed on membrane fractions detached from root hairs. Proteomic analysis identified proteins whose expression was changed after heat stress treatment for 3 h. Most of these identified proteins were actively involved in heat tolerance in soybean (Valdés-López et al., 2016). High-temperature stress affects seed development and maturity and leads to seed deterioration; however, protein involvement is not fully understood. Wang et al. (2012) compared the proteome composition of developing seed, and 42 proteins were identified and belonged to 31 diverse protein species. These proteins involved in 13 cellular response pathways, including protein folding, protein biosynthesis, and carbohydrate metabolism, etc. Such a mechanism allows us to investigate the management techniques to maintain soybean growth under heat stress (Wang et al., 2012).
Many studies reported the role of transcriptomes and proteins in drought stress response in soybean; however, studies about heat tolerance are limited. We emphasize conducting more studies to identify the potential genes governing the heat-responsive pathways in soybean. Exposing plants to varied heat stress levels would be better in analyzing transcriptional gene profiles and investigating their regulatory network. Likewise, the proteomic analysis would show the active proteins in soybean heat tolerance. With the advancement in the molecular field and the discovery of new tools, the speed of molecular breeding would increase and allow us to sustain crop growth under changing environmental conditions.
Conclusions and future research directions
Climatic changes are continuously threatening global food security. Industrialization leads to increased heat waves because of the global warming threats. Heat waves decreased the yield of the crop to a significant level. The continuous expansion of the human population threatens our ability to feed the entire world. Scientists have increased crops yield under changing climatic conditions. Soybean is a significant oilseed crop and part of the human diet and serves as a primary protein source of livestock feed. Crop responses to heat stress involve morphological, physiological, and molecular pathways. In conventional ways, plants use avoidance, escape, and tolerance mechanisms to cope with heat episodes. The difference in protein contents also contributes to heat tolerance in soybean. Variation in stomatal closure and leaf angle is linked with the morpho-physiological type of response. Certain hormones like CK, MT, and ET can help soybean plants counter the harmful effects of heat waves in maintaining yield and quality. The role of other phytohormones is not yet fully understood and needs further studies. This is one of the most potent agronomic approaches to compensate for yield loss in soybean under heat stress conditions. High-yielding soybean varieties have been developed to address food security challenges, and breeders are engaged in bringing genetic variation for heat tolerance in soybean. Conventional breeding methods are time-consuming and costly, so they have limited use in modern agriculture and can be modified for better results.
There are specific challenges in improving heat stress tolerance in soybean. These challenges include lack of complete genetic information, insufficient knowledge about crop nature, genetic erosion, lack of research on wild soybean types for gene identification, and limited use of the latest molecular techniques for heat tolerance. Heat tolerance is a polygenic trait and cannot be fully developed by traditional breeding methods. Hence, molecular techniques like QTL mapping, genetic engineering, CRISPR/Cas9, transcriptome sequencing, and transcription factors identification are reliable genetic approaches to develop heat-tolerant soybean genotypes. Many success stories have been published, indicating the reliability of these methods in understanding the genetic mechanism of heat tolerance in soybean. We recommend the CRISPR/Cas9 as the most promising and reliable tool for targeted editing of heat tolerance genes in soybean, as it can works across all biological boundaries. Unfortunately, QTL mapping for heat tolerance in soybean is limited, and only a few reports have been published. QTL mapping area must be broadened to identify the potential regions involved in heat tolerance in soybean. This review emphasizes using wild relatives to increase genetic diversity for soybean heat tolerance in the future. Transcription and transcriptome analysis played a key role in heat tolerance in soybean; however, there is a big gap in these studies that should be filled. Complete genome sequencing will lead to the detailed characterization of all heat-tolerant genes and their possible use in future breeding plans. By adopting these techniques and combining efforts, soybean production can be maintained under heat stress conditions, leading to a green revolution.
Author contributions
GJ, GY, GuY, and AR prepared the manuscript. ZQ and XZ prepared the figures. AM, ZS, ZoZ, ZnZ, WX, and WJ involved in reviewing and editing. All authors contributed to the article and approved the submitted version.
Funding
This research was funded by the Jilin Province Science and Technology Development Plan Project (20190303075SF).
Acknowledgments
Authors are thankful to WJ for supporting this study.
Conflict of interest
The authors declare that the research was conducted in the absence of any commercial or financial relationships that could be construed as a potential conflict of interest.
Publisher’s note
All claims expressed in this article are solely those of the authors and do not necessarily represent those of their affiliated organizations, or those of the publisher, the editors and the reviewers. Any product that may be evaluated in this article, or claim that may be made by its manufacturer, is not guaranteed or endorsed by the publisher.
References
Ahmad, M., Ali, A., Ullah, Z., Ali, M., Sher, H., Zahoor, M., et al. (2022). Biosynthesized silver nanoparticles using Polygonatum geminiflorum efficiently controls Fusarium wilt disease of tomato. Front. Bioeng. Biotech.
Ahmad, S., Sheng, Z., Jalal, R. S., Tabassum, J., Ahmed, F. K., Hu, S., et al. (2021). “CRISPR–Cas technology towards improvement of abiotic stress tolerance in plants,” in CRISPR and RNAi Systems, eds K. Abd-Elsalam and K. T. Lim (Amsterdam: Elsevier), 755–772. doi: 10.1016/B978-0-12-821910-2.00021-7
Aleem, M., Aleem, S., Sharif, I., Wu, Z., Aleem, M., Tahir, A., et al. (2022). Characterization of SOD and GPX Gene families in the soybeans in response to drought and salinity stresses. Antioxidants 11:460. doi: 10.3390/antiox11030460
Ali, I., Salah, K. B. H., Sher, H., Ali, H., Ullah, Z., Ali, A., et al. (2022a). Drought stress enhances the efficiency of floral dip method of Agrobacterium-mediated transformation in Arabidopsis thaliana. Braz. J. Biol. 84:e259326. doi: 10.1590/1519-6984.259326
Ali, I., Khan, A., Ali, A., Ullah, Z., Li, Y., Khan, N., et al. (2022b). Iron and Zinc micronutrients and soil inoculation of Trichoderma harzianum enhance wheat grain quality and yield. Front. Plant Sci. 13:960948.
Almeselmani, M., Deshmukh, P., Sairam, R. K., Kushwaha, S., and Singh, T. (2006). Protective role of antioxidant enzymes under high temperature stress. Plant Sci. 171, 382–388. doi: 10.1016/j.plantsci.2006.04.009
Alsajri, F. A., Singh, B., Wijewardana, C., Irby, J. T., Gao, W., and Reddy, K. R. (2019). Evaluating soybean cultivars for low-and high-temperature tolerance during the seedling growth stage. Agronomy 9:13. doi: 10.3390/agronomy9010013
Amanullah Ilyas, M., Nabi, H., Khalid, S., Ahmad, M., Muhammad, A., Ullah, S., et al. (2021). Integrated foliar nutrients application improve wheat (Triticum Aestivum L.) productivity under calcareous soils in drylands. Commun. Soil Sci. Plant Analy. 52, 2748–2766. doi: 10.1080/00103624.2021.1956521
Amjad, S. F., Mansoora, N., Din, I. U., Khalid Iqbal, R., Jatoi, G. H., Murtaza, G., et al. (2021). Application of zinc fertilizer and mycorrhizal inoculation on physio-biochemical parameters of wheat grown under water-stressed environment. Sustainability 13:11007. doi: 10.3390/su131911007
Apel, K., and Hirt, H. (2004). Reactive oxygen species: Metabolism, oxidative stress, and signal transduction. Annu. Rev. Plant Biol. 55, 373–399. doi: 10.1146/annurev.arplant.55.031903.141701
Arce-Paredes, P., Mora-Escobedo, R., Luna-Arias, J. P., Mendoza-Hernández, G., and Rojas-Espinosa, O. (2011). “Heat, salinity, and acidity, commonly upregulate A1aB1b proglycinin in soybean embryonic axes,” in Soybean-Biochemistry, Chemistry and Physiology, ed. M. C. N. García (London: InTech Open Access), 402–422. doi: 10.5772/14227
Ashfaq, A., Uzma, Y., Niaz, A., Muhammad, A., Fahad, S., Haider, S., et al. (2021). Toxicity of cadmium and nickel in the context of applied activated carbon biochar for improvement in soil fertility. Saudi Soc. Agric. Sci. 29, 743–750. doi: 10.1016/j.sjbs.2021.09.035
Ashoub, A., Baeumlisberger, M., Neupaertl, M., Karas, M., and Brüggemann, W. (2015). Characterization of common and distinctive adjustments of wild barley leaf proteome under drought acclimation, heat stress and their combination. Plant Mol. Biol. 87, 459–471. doi: 10.1007/s11103-015-0291-4
Ault, T. R. (2020). On the essentials of drought in a changing climate. Science 368, 256–260. doi: 10.1126/science.aaz5492
Bailey-Serres, J., Parker, J. E., Ainsworth, E. A., Oldroyd, G. E., and Schroeder, J. I. (2019). Genetic strategies for improving crop yields. Nature 575, 109–118. doi: 10.1038/s41586-019-1679-0
Balfagón, D., Sengupta, S., Gómez-Cadenas, A., Fritschi, F. B., Azad, R. K., Mittler, R., et al. (2019). Jasmonic acid is required for plant acclimation to a combination of high light and heat stress. Plant Physiol. 181, 1668–1682. doi: 10.1104/pp.19.00956
Balfagón, D., Terán, F., De Oliveira, T. D. R., Santa-Catarina, C., and Gómez-Cadenas, A. (2022). Citrus rootstocks modify scion antioxidant system under drought and heat stress combination. Plant Cell Rep. 41, 593–602. doi: 10.1007/s00299-021-02744-y
Bazzer, S. K., and Purcell, L. C. (2020). Identification of quantitative trait loci associated with canopy temperature in soybean. Sci. Rep. 10:17604. doi: 10.1038/s41598-020-74614-8
Bellaloui, N., Reddy, K. N., and Mengistu, A. (2015). “Drought and heat stress effects on soybean fatty acid composition and oil stability,” in Processing and Impact on Active Components in Food, ed. V. Preedy (Amsterdam: Elsevier), 377–384. doi: 10.1016/B978-0-12-404699-3.00045-7
Bellaloui, N., Smith, J. R., and Mengistu, A. (2017). Seed nutrition and quality, seed coat boron and lignin are influenced by delayed harvest in exotically-derived soybean breeding lines under high heat. Front. Plant Sci. 8:1563. doi: 10.3389/fpls.2017.01563
Boehm, D. J. Jr., Walker, F. R., Bhandari, H. S., Kopsell, D., and Pantalone, V. R. (2017). Seed inorganic phosphorus stability and agronomic performance of two low-phytate soybean lines evaluated across six southeastern US environments. Crop Sci. 57, 2555–2563. doi: 10.2135/cropsci2017.02.0107
Camerlengo, F., Frittelli, A., and Pagliarello, R. (2022). CRISPR towards a Sustainable Agriculture. Encyclopedia 2, 538–558. doi: 10.3390/encyclopedia2010036
Campobenedetto, C., Mannino, G., Agliassa, C., Acquadro, A., Contartese, V., Garabello, C., et al. (2020). Transcriptome analyses and antioxidant activity profiling reveal the role of a lignin-derived biostimulant seed treatment in enhancing heat stress tolerance in soybean. Plants 9:1308. doi: 10.3390/plants9101308
Cao, P., Zhao, Y., Wu, F., Xin, D., Liu, C., Wu, X., et al. (2022). Multi-Omics techniques for soybean molecular breeding. Int. J. Mol. Sci. 23:4994. doi: 10.3390/ijms23094994
Cao, W. H., Liu, J., He, X. J., Mu, R. L., Zhou, H. L., Chen, S. Y., et al. (2007). Modulation of ethylene responses affects plant salt-stress responses. Plant Physiol. 143, 707–719. doi: 10.1104/pp.106.094292
Chebrolu, K. K., Fritschi, F. B., Ye, S., Krishnan, H. B., Smith, J. R., and Gillman, J. D. (2016). Impact of heat stress during seed development on soybean seed metabolome. Metabolomics 12:28. doi: 10.1007/s11306-015-0941-1
Chen, X. J., Ye, C. J., Lü, H. Y., Xu, M. X., Li, W., Zhang, L. M., et al. (2006). Cloning of GmHSFA1 gene and its overexpression leading to enhancement of heat tolerance in transgenic soybean. Yi Chuan Hered. 28, 1411–1420. doi: 10.1360/yc-006-1411
Chen, Z., Zhong, W., Zhou, Y., Ji, P., Wan, Y., Shi, S., et al. (2022). Integrative analysis of metabolome and transcriptome reveals the improvements of seed quality in vegetable soybean (Glycine max (L.) Merr.). Phytochemistry 200:113216. doi: 10.1016/j.phytochem.2022.113216
Chennakesavulu, K., Singh, H., Trivedi, P. K., Jain, M., and Yadav, S. R. (2021). State-of-the-art in CRISPR technology and engineering drought, salinity, and thermo-tolerant crop plants. Plant Cell Rep. 41, 815–831. doi: 10.1007/s00299-021-02681-w
Chu, S., Zhang, X., Yu, K., Lv, L., Sun, C., Liu, X., et al. (2020). Genome-wide analysis reveals dynamic epigenomic differences in soybean response to low-phosphorus stress. Int. J. Mol. Sci. 21:6817. doi: 10.3390/ijms21186817
Chung, E., Kim, K.-M., and Lee, J.-H. (2013). Genome-wide analysis and molecular characterization of heat shock transcription factor family in Glycine max. J. Gen. Gen. 40, 127–135. doi: 10.1016/j.jgg.2012.12.002
Cohen, I., Zandalinas, S. I., Huck, C., Fritschi, F. B., and Mittler, R. (2021b). Meta-analysis of drought and heat stress combination impact on crop yield and yield components. Physiol. Plant. 171, 66–76. doi: 10.1111/ppl.13203
Cohen, I., Zandalinas, S. I., Fritschi, F. B., Sengupta, S., Fichman, Y., Azad, R. K., et al. (2021a). The impact of water deficit and heat stress combination on the molecular response, physiology, and seed production of soybean. Physiol. Plant. 172, 41–52.
Consultation, F. E. (2004). Protein Sources for the Animal Feed Industry. Expert consultation and workshop. Bangkok, 29 April-3 May 2002. Rome: Food and Agriculture Organization of the United Nations.
Cui, M., Lin, Y., Zu, Y., Efferth, T., Li, D., and Tang, Z. (2015). Ethylene increases accumulation of compatible solutes and decreases oxidative stress to improve plant tolerance to water stress in Arabidopsis. J. Plant Biol. 58, 193–201. doi: 10.1007/s12374-014-0302-z
Das, A., Eldakak, M., Paudel, B., Kim, D. W., Hemmati, H., Basu, C., et al. (2016). Leaf proteome analysis reveals prospective drought and heat stress response mechanisms in soybean. BioMed. Res. Int. 2016:6021047. doi: 10.1155/2016/6021047
Das, A., Rushton, P. J., and Rohila, J. S. (2017). Metabolomic profiling of soybeans (Glycine max L.) reveals the importance of sugar and nitrogen metabolism under drought and heat stress. Plants 6:21. doi: 10.3390/plants6020021
de Moraes, M. T., and Gusmao, A. G. (2021). How do water, compaction and heat stresses affect soybean root elongation? A review. Rhizosphere 19:100403. doi: 10.1016/j.rhisph.2021.100403
Dhungana, S. K., Park, J. H., Oh, J. H., Kang, B. K., Seo, J. H., Sung, J. S., et al. (2021). Quantitative trait locus mapping for drought tolerance in soybean recombinant inbred line population. Plants 10:1816. doi: 10.3390/plants10091816
Djanaguiraman, M., and Prasad, P. V. (2010). Ethylene production under high temperature stress causes premature leaf senescence in soybean. Funct. Plant Biol. 37, 1071–1084. doi: 10.1071/FP10089
Djanaguiraman, M., Prasad, P. V., Boyle, D., and Schapaugh, W. (2011). High-temperature stress and soybean leaves: Leaf anatomy and photosynthesis. Crop Sci. 51, 2125–2131. doi: 10.2135/cropsci2010.10.0571
Duan, K., Cheng, Y., Ji, J., Wang, C., Wei, Y., and Wang, Y. (2021). Large chromosomal segment deletions by CRISPR/LbCpf1-mediated multiplex gene editing in soybean. J. Integr. Plant Biol. 63, 1620–1631. doi: 10.1111/jipb.13158
Ergo, V. V., Lascano, R., Vega, C. R., Parola, R., and Carrera, C. S. (2018). Heat and water stressed field-grown soybean: A multivariate study on the relationship between physiological-biochemical traits and yield. Environ. Exp. Bot. 148, 1–11. doi: 10.1016/j.envexpbot.2017.12.023
Ergo, V. V., Veas, R. E., Vega, C. R., Lascano, R., and Carrera, C. S. (2021). Leaf photosynthesis and senescence in heated and droughted field-grown soybean with contrasting seed protein concentration. Plant Physiol. Biochem. 166, 437–447. doi: 10.1016/j.plaphy.2021.06.008
Farooq, M., Wahid, A., Kobayashi, N., Fujita, D., and Basra, S. (2009). Plant drought stress: Effects, mechanisms and management. Agron. Sustain. Dev. 29, 185–212. doi: 10.1051/agro:2008021
Feng, Z. J., Liu, N., Zhang, G. W., Niu, F. G., Xu, S. C., and Gong, Y. M. (2019). Investigation of the AQP family in soybean and the promoter activity of TIP2; 6 in heat stress and hormone responses. Int. J. Mol. Sci. 20:262. doi: 10.3390/ijms20020262
Ghosh, S., and Dey, G. (2022). Biotic and abiotic stress tolerance through CRISPR-Cas mediated genome editing. J. Plant Biochem. Biotech. 31, 227–238. doi: 10.1007/s13562-021-00746-1
Gillman, J. D., Biever, J. J., Ye, S., Spollen, W. G., Givan, S. A., Lyu, Z., et al. (2019). A seed germination transcriptomic study contrasting two soybean genotypes that differ in terms of their tolerance to the deleterious impacts of elevated temperatures during seed fill. BMC Res. Notes 12:522. doi: 10.1186/s13104-019-4559-7
Gillman, J. D., Chebrolu, K., and Smith, J. R. (2021). Quantitative trait locus mapping for resistance to heat-induced seed degradation and low seed phytic acid in soybean. Crop Sci. 61, 2023–2035. doi: 10.1002/csc2.20419
Goodman, R. M., and Kiser, J. (2022). “Biotechnology and its impact on future developments in soybean production and use,” in World Soybean Research Conference III: Proceedings, (Florida, US: CRC Press), 261–271. doi: 10.1201/9780429267932-46
Grossiord, C., Buckley, T. N., Cernusak, L. A., Novick, K. A., Poulter, B., Siegwolf, R. T., et al. (2020). Plant responses to rising vapor pressure deficit. New Phytol. 226, 1550–1566. doi: 10.1111/nph.16485
Gur, A., Zamir, D., and Dangl, J. (2004). Unused natural variation can lift yield barriers in plant breeding. PLoS Biol. 2:e245. doi: 10.1371/journal.pbio.0020245
Haider, S., Raza, A., Iqbal, J., Shaukat, M., and Mahmood, T. (2022). Analyzing the regulatory role of heat shock transcription factors in plant heat stress tolerance: A brief appraisal. Mol. Biol. Rep. 49, 5771–5785. doi: 10.1007/s11033-022-07190-x
Hamayun, M., Hussain, A., Iqbal, A., Khan, S. A., Gul, S., Khan, H., et al. (2021). Penicillium glabrum acted as a heat stress relieving endophyte in soybean and sunflower. Polish J. Environ. Stud. 30, 3099–3110. doi: 10.15244/pjoes/128579
Hasanuzzaman, M., Nahar, K., and Fujita, M. (2013). “Extreme temperature responses, oxidative stress and antioxidant defense in plants,” in Stress-Plant Responses and Applications in Agriculture, eds K. Vahdati and C. Leslie Abiotic (London: Intechopen), 169–205. doi: 10.5772/54833
Hatfield, J. L., and Dold, C. (2019). Water-use efficiency: Advances and challenges in a changing climate. Front. Plant Sci. 10:103. doi: 10.3389/fpls.2019.00103
Hoffman, A., Kemanian, A., and Forest, C. (2020). The response of maize, Sorghum, and soybean yield to growing-phase climate revealed with machine learning. Environ. Res. Lett. 15:094013. doi: 10.1088/1748-9326/ab7b22
Huang, Y., Xuan, H., Yang, C., Guo, N., Wang, H., Zhao, J., et al. (2019). GmHsp90A2 is involved in soybean heat stress as a positive regulator. Plant Sci. 285, 26–33. doi: 10.1016/j.plantsci.2019.04.016
Imran, M., Aaqil Khan, M., Shahzad, R., Bilal, S., Khan, M., Yun, B.-W., et al. (2021). Melatonin ameliorates thermotolerance in soybean seedling through balancing redox homeostasis and modulating antioxidant defense, phytohormones and polyamines biosynthesis. Molecules 26:5116. doi: 10.3390/molecules26175116
Jagadish, S., Craufurd, P., and Wheeler, T. (2008). Phenotyping parents of mapping populations of rice for heat tolerance during anthesis. Crop Sci. 48, 1140–1146. doi: 10.2135/cropsci2007.10.0559
Jumrani, K., and Bhatia, V. S. (2019). Interactive effect of temperature and water stress on physiological and biochemical processes in soybean. Physiol. Mol. Biol. Plants 25, 667–681. doi: 10.1007/s12298-019-00657-5
Katam, R., Shokri, S., Murthy, N., Singh, S. K., Suravajhala, P., Khan, M. N., et al. (2020). Proteomics, physiological, and biochemical analysis of cross tolerance mechanisms in response to heat and water stresses in soybean. PLoS One 15:e0233905. doi: 10.1371/journal.pone.0233905
Khan, M. A., Asaf, S., Khan, A. L., Jan, R., Kang, S. M., Kim, K. M., et al. (2020). Thermotolerance effect of plant growth-promoting Bacillus cereus SA1 on soybean during heat stress. BMC Microbiol. 20:175. doi: 10.1186/s12866-020-01822-7
Khan, S., Bibi, G., Dilbar, S., Iqbal, A., Ahmad, M., Ali, A., et al. (2022). Biosynthesis and characterization of iron oxide nanoparticles from Mentha spicata and screening its combating potential against Phytophthora infestans. Front. Plant Sci. 13:1001499.
Kidokoro, S., Watanabe, K., Ohori, T., Moriwaki, T., Maruyama, K., Mizoi, J., et al. (2015). Soybean DREB 1/CBF-type transcription factors function in heat and drought as well as cold stress-responsive gene expression. Plant J. 81, 505–518. doi: 10.1111/tpj.12746
Kim, H. (2017). CRISPR system-mediated soybean genome editing. J. Kor. Breed. Soc. 2017:27. doi: 10.1016/S0960-8966(17)30253-5
Kosová, K., Vítámvás, P., Prášil, I. T., and Renaut, J. (2011). Plant proteome changes under abiotic stress—contribution of proteomics studies to understanding plant stress response. J. Prot. 74, 1301–1322. doi: 10.1016/j.jprot.2011.02.006
Krishnan, H. B., Kim, W. S., Oehrle, N. W., Smith, J. R., and Gillman, J. D. (2020). Effect of heat stress on seed protein composition and ultrastructure of protein storage vacuoles in the cotyledonary parenchyma cells of soybean genotypes that are either tolerant or sensitive to elevated temperatures. Int. J. Mol. Sci. 21:4775. doi: 10.3390/ijms21134775
Kulwal, P., Thudi, M., and Varshney, R. (2011). “Genomics interventions in crop breeding for sustainable agriculture,” in Encyclopedia of Sustainability Science and Technology, ed. R. E. Meyers (Cham: Springer).
Kumar, G., Saad, K. R., Arya, M., Puthusseri, B., Mahadevappa, P., Shetty, N. P., et al. (2021). The Synergistic role of serotonin and melatonin during temperature stress in promoting cell division, ethylene and isoflavones biosynthesis in Glycine max. Curr. Plant Biol. 26:100206. doi: 10.1016/j.cpb.2021.100206
Lam, H. M., Xu, X., Liu, X., Chen, W., Yang, G., Wong, F. L., et al. (2010). Resequencing of 31 wild and cultivated soybean genomes identifies patterns of genetic diversity and selection. Nat. Gen. 42, 1053–1059. doi: 10.1038/ng.715
Li, K. P., Wong, C. H., Cheng, C. C., Cheng, S. S., Li, M. W., Mansveld, S., et al. (2021). GmDNJ1, a type-I heat shock protein 40 (HSP40), is responsible for both growth and heat tolerance in soybean. Plant Dir. 5:e00298. doi: 10.1002/pld3.298
Li, P. S., Yu, T. F., He, G. H., Chen, M., Zhou, Y. B., Chai, S. C., et al. (2014). Genome-wide analysis of the Hsf family in soybean and functional identification of GmHsf-34 involvement in drought and heat stresses. BMC Gen. 15:1009. doi: 10.1186/1471-2164-15-1009
Lindsey, L., and Thomison, P. (2012). High temperature effects on corn and soybean. CORN Newslet. 2012, 23–26.
Liu, G. T., Ma, L., Duan, W., Wang, B. C., Li, J. H., Xu, H. G., et al. (2014). Differential proteomic analysis of grapevine leaves by iTRAQ reveals responses to heat stress and subsequent recovery. BMC Plant Biol. 14:110. doi: 10.1186/1471-2229-14-110
Liu, H., Brettell, L. E., Qiu, Z., and Singh, B. K. (2020). Microbiome-mediated stress resistance in plants. Trends Plant Sci. 25, 733–743. doi: 10.1016/j.tplants.2020.03.014
Liu, S., Wang, X., Wang, H., Xin, H., Yang, X., Yan, J., et al. (2013). Genome-wide analysis of ZmDREB genes and their association with natural variation in drought tolerance at seedling stage of Zea mays L. PLoS Genet. 9:e1003790. doi: 10.1371/journal.pgen.1003790
Liu, X., Pi, B., Du, Z., Yang, T., Gu, M., Sun, S., et al. (2022a). The transcription factor GmbHLH3 confers Cl-/salt tolerance to soybean by upregulating GmCLC1 expression for maintenance of anion homeostasis. Environ. Exp. Bot. 194:104755. doi: 10.1016/j.envexpbot.2021.104755
Liu, X., Yang, Y., Wang, R., Cui, R., Xu, H., Sun, C., et al. (2022b). GmWRKY46, a WRKY transcription factor, negatively regulates phosphorus tolerance primarily through modifying root morphology in soybean. Plant Sci. 315:111148. doi: 10.1016/j.plantsci.2021.111148
Liu, Y., Li, J., Zhu, Y., Jones, A., Rose, R. J., and Song, Y. (2019). Heat stress in legume seed setting: Effects, causes, and future prospects. Front. Plant Sci. 10:938. doi: 10.3389/fpls.2019.00938
Longmei, N., Gill, G. K., Zaidi, P. H., Kumar, R., Nair, S. K., Hindu, V., et al. (2021). Genome wide association mapping for heat tolerance in sub-tropical maize. BMC Gen. 22:154. doi: 10.1186/s12864-021-07463-y
Ma, Y., Liu, K., Zhang, C., Lin, F., Hu, W., Jiang, Y., et al. (2022). Comparative root transcriptome analysis of two soybean cultivars with different cadmium sensitivities reveals the underlying tolerance mechanisms. Genome 65, 27–42. doi: 10.1139/gen-2021-0048
Ma, Y., Qin, F., and Tran, L. S. P. (2012). Contribution of genomics to gene discovery in plant abiotic stress responses. Mol. Plant 5, 1176–1178. doi: 10.1093/mp/sss085
Mammadov, J., Buyyarapu, R., Guttikonda, S. K., Parliament, K., Abdurakhmonov, I. Y., and Kumpatla, S. P. (2018). Wild relatives of maize, rice, cotton, and soybean: Treasure troves for tolerance to biotic and abiotic stresses. Front. Plant Sci. 9:886. doi: 10.3389/fpls.2018.00886
Manan, S., and Zhao, J. (2020). Role of Glycine max ABSCISIC ACID INSENSITIVE 3 (GmABI3) in lipid biosynthesis and stress tolerance in soybean. Funct. Plant Biol. 48, 171–179. doi: 10.1071/FP19260
Meena, R. S., Lal, R., and Yadav, G. S. (2020b). Long-term impacts of topsoil depth and amendments on soil physical and hydrological properties of an Alfisol in central Ohio. USA. Geoderma 363:114164. doi: 10.1016/j.geoderma.2019.114164
Meena, R. S., Kumar, S., Datta, R., Lal, R., Vijayakumar, V., Brtnicky, M., et al. (2020a). Impact of agrochemicals on soil microbiota and management: A review. Land 9:34.
Min, L., Yang, S., Stress, P. A., Ding, X., Guo, Q., Li, Q., et al. (2022). Reviewed by: High-temperature (HT) is one of the most important environmental factors that negatively impact the yield of some soybean cytoplasmic male sterility (CMS)-based hybrid (F1) combinations. The response of soybean to HT, especially at the male organ development stage, is poorly understood. To investigate the molecular mechanisms of the response from soybean CMS-based F1 male organ to HT, a detailed transcriptomics. Cell. Signal. Netw. Plant Heat Stress Respons. 5.
Mittler, R. (2006). Abiotic stress, the field environment and stress combination. Trends Plant Sci. 11, 15–19. doi: 10.1016/j.tplants.2005.11.002
Mittler, R., and Blumwald, E. (2010). Genetic engineering for modern agriculture: Challenges and perspectives. Ann. Rev. Plant Biol. 61, 443–462. doi: 10.1146/annurev-arplant-042809-112116
Mizoi, J., Ohori, T., Moriwaki, T., Kidokoro, S., Todaka, D., Maruyama, K., et al. (2013). GmDREB2A; 2, a canonical dehydration-responsive element-binding protein2-type transcription factor in soybean, is posttranslationally regulated and mediates dehydration-responsive element-dependent gene expression. Plant Physiol. 161, 346–361. doi: 10.1104/pp.112.204875
Naik, H. S., Zhang, J., Lofquist, A., Assefa, T., Sarkar, S., Ackerman, D., et al. (2017). A real-time phenotyping framework using machine learning for plant stress severity rating in soybean. Plant Methods 13:23. doi: 10.1186/s13007-017-0173-7
Nakagawa, A. C., Ario, N., Tomita, Y., Tanaka, S., Murayama, N., Mizuta, C., et al. (2020). High temperature during soybean seed development differentially alters lipid and protein metabolism. Plant Prod. Sci. 23, 504–512. doi: 10.1080/1343943X.2020.1742581
Nawaz, M. A., Yang, S. H., and Chung, G. (2018). “Wild soybeans: an opportunistic resource for soybean improvement,” in Rediscovery of Landraces as a Resource for the Future, ed. O. Grillo (London: InTechOpen), doi: 10.5772/intechopen.74973
Ortiz, A. C., De Smet, I., Sozzani, R., and Locke, A. M. (2022). Field-grown soybean shows genotypic variation in physiological and seed composition responses to heat stress during seed development. Environ. Exp. Bot. 195:104768. doi: 10.1016/j.envexpbot.2021.104768
Ozga, J. A., Kaur, H., Savada, R. P., and Reinecke, D. M. (2017). Hormonal regulation of reproductive growth under normal and heat-stress conditions in legume and other model crop species. J. Exp. Bot. 68, 1885–1894. doi: 10.1093/jxb/erw464
Pandey, M. K., Upadhyaya, H. D., Rathore, A., Vadez, V., Sheshshayee, M., Sriswathi, M., et al. (2014). Genomewide association studies for 50 agronomic traits in peanut using the ‘reference set’comprising 300 genotypes from 48 countries of the semi-arid tropics of the world. PLoS One 9:e105228. doi: 10.1371/journal.pone.0105228
Pandian, S., Rakkammal, K., Sagina Rency, A., Muthuramalingam, P., Karutha Pandian, S., and Ramesh, M. (2020). “Abiotic stress and applications of omics approaches to develop stress tolerance in agronomic crops,” in Agronomic Crops, ed. M. Hasanuzzaman (Singapore: Springer), 557–578. doi: 10.1007/978-981-15-0025-1_26
Pandita, D. (2022). “CRISPR/Cas-mediated genome editing technologies in plants for stress resilience,” in Antioxidant Defense in Plants, eds T. Aftab and K. R. Hakeem (Singapore: Springer), 13, doi: 10.1007/978-981-16-7981-0_13
Rahman, S. U., Mccoy, E., Raza, G., Ali, Z., Mansoor, S., and Amin, I. (2022). Improvement of soybean; A way forward transition from genetic engineering to new plant breeding technologies. Mol. Biotech. [Epub ahead of print]. doi: 10.1007/s12033-022-00456-6
Rasheed, A., Barqawi, A. A., Mahmood, A., Nawaz, M., Shah, A. N., Bay, D. H., et al. (2022a). CRISPR/Cas9 is a powerful tool for precise genome editing of legume crops: a review. Mol. Biol. Rep. 49, 5595–5609.
Rasheed, A., Mahmood, A., Maqbool, R., Albaqami, M., Sher, A., Sattar, A., et al. (2022b). Key insights to develop drought-resilient soybean: A review. J. King Saud Uni. Sci. 34:102089. doi: 10.1016/j.jksus.2022.102089
Rasheed, A., Fahad, S., Hassan, M. U., Tahir, M. M., Aamer, M., and Wu, Z. (2020a). A review on aluminum toxicity and quantitative trait loci maping in rice (Oryza sative L). App. Ecol. Environ. Res. 18, 3951–3961. doi: 10.1007/s11033-022-07529-4
Rasheed, A., Fahad, S., Hassan, M. U., Tahir, M. M., Aamer, M., and Wu, Z. (2020b). Role of genetic factors in regulating cadmium uptake, transport and accumulation mechanisms and quantitative trait loci mapping in rice. a review. App. Ecol. Environ. Res. 18, 4005–4023. doi: 10.15666/aeer/1803_40054023
Rasheed, A., Hassan, M., Aamer, M., Bian, J., Xu, Z., He, X., et al. (2020c). Iron toxicity, tolerance and quantitative trait loci mapping in rice; a review. App. Ecol. Environ. Res. 18, 7483–7498. doi: 10.15666/aeer/1806_74837498
Rasheed, A., Gill, R. A., Hassan, M. U., Mahmood, A., Qari, S., Zaman, Q. U., et al. (2021a). A Critical review: Recent advancements in the use of CRISPR/Cas9 technology to enhance crops and alleviate global food crises. Curr. Issues Mol. Biol. 43, 1950–1976. doi: 10.3390/cimb43030135
Rasheed, A., Hassan, M. U., Fahad, S., Aamer, M., Batool, M., Ilyas, M., et al. (2021b). “Heavy Metals Stress and Plants Defense Responses,” in Sustainable Soil and Land Management and Climate Change, eds S. Fahad, O. Sonmez, S. Saud, D. Wang, C. Wu, M. Adnan, et al. (Florida, US: CRC Press), 57–82.
Razzaq, M. K., Akhter, M., Ahmad, R. M., Cheema, K. L., Hina, A., Karikari, B., et al. (2022). CRISPR-Cas9 based stress tolerance: New hope for abiotic stress tolerance in chickpea (Cicer arietinum). Mol. Biol. Rep. [Epub ahead of print]. doi: 10.1007/s11033-022-07391-4
Rial, R. C., Merlo, T. C., Santos, P. H. M., Melo, L. F. D., Nazário, C. E. D., and Viana, L. H. (2022). Thermal and oxidative stability of ethyl soybean biodiesel with cagaite (Eugenia dysenterica DC.) leaves extract as additive. J. Ther. Anal. Calorim. 147, 1–7. doi: 10.1007/s10973-022-11267-x
Ribichich, K. F., Chiozza, M., Ávalos-Britez, S., Cabello, J. V., Arce, A. L., Watson, G., et al. (2020). Successful field performance in warm and dry environments of soybean expressing the sunflower transcription factor HB4. J. Exp. Bot. 71, 3142–3156. doi: 10.1093/jxb/eraa064
Ruiz-Vera, U. M., Siebers, M., Gray, S. B., Drag, D. W., Rosenthal, D. M., Kimball, B. A., et al. (2013). Global warming can negate the expected CO2 stimulation in photosynthesis and productivity for soybean grown in the Midwestern United States. Plant Physiol. 162, 410–423. doi: 10.1104/pp.112.211938
Rustgi, S., Kakati, J. P., Jones, Z. T., Zoong Lwe, Z. S., and Narayanan, S. (2021). Heat tolerance as a function of membrane lipid remodeling in the major US oilseed crops (soybean and peanut). J. Plant Biochem. Biotech. 30, 652–667. doi: 10.1007/s13562-021-00729-2
Sabagh, A., Hossain, A., Islam, M. S., Iqbal, M. A., Fahad, S., Ratnasekera, D., et al. (2020). “Consequences and mitigation strategies of heat stress for sustainability of soybean (Glycine max L. Merr.) production under the changing climate,” in Plant Stress Physiology, ed. A. Hossain (London: IntechOpen), doi: 10.5772/intechopen.92098
Sabagh, A. E., Hossain, A., Islam, M. S., Barutçular, C., Ratnasekera, D., and Kumar, N. (2019). Sustainable soybean production and abiotic stress management in saline environments: A critical review. Aust. J. Crop Sci. 13, 228–236. doi: 10.21475/ajcs.19.13.02.p1285
Saleem, M. H., Fahad, S., Khan, S. U., Din, M., Ullah, A., Sabagh, A. E., et al. (2020). Copper-induced oxidative stress, initiation of antioxidants and phytoremediation potential of flax (Linum usitatissimum L.) seedlings grown under the mixing of two different soils of China. Environ. Sci. Poll. Res. 27, 5211–5221. doi: 10.1007/s11356-019-07264-7
Sarieva, G., Kenzhebaeva, S., and Lichtenthaler, H. (2010). Adaptation potential of photosynthesis in wheat cultivars with a capability of leaf rolling under high temperature conditions. Russ. J. Plant Physiol. 57, 28–36. doi: 10.1134/S1021443710010048
Saud, S., Fahad, S., Yajun, C., Ihsan, M. Z., Hammad, H. M., Nasim, W., et al. (2017). Effects of nitrogen supply on water stress and recovery mechanisms in Kentucky bluegrass plants. Front. Plant Sci. 8:983. doi: 10.3389/fpls.2017.00983
Shanmugavel, P., Rajaprakasam, S., Chockalingam, V., Ramasamy, G., Thiyagarajan, K., and Marimuthu, R. (2020). “Breeding mechanisms for high temperature tolerance in crop plants,” in Plant Breeding-Current and Future Views, ed. I. Y. Abdurakhmonov (London: IntechOpen), doi: 10.5772/intechopen.94693
Sharifi, P., Amirnia, R., and Shirani Bidabadi, S. (2022). Role of Silicon in Mediating Heat Shock Tolerance in Soybean. Ges. Pflan. 74, 397–411. doi: 10.1007/s10343-021-00617-8
Shuai, H., Meng, Y., Luo, X., Chen, F., Zhou, W., Dai, Y., et al. (2017). Exogenous auxin represses soybean seed germination through decreasing the gibberellin/abscisic acid (GA/ABA) ratio. Sci. Rep. 7, 1–11. doi: 10.1038/s41598-017-13093-w
Singh, A., and Meena, R. S. (2020). Response of bioregulators and irrigation on plant height of Indian mustard (Brassica juncea L.). J. Oilseed Brassica 11, 9–14.
Singh, R., and Nelson, R. (2015). Intersubgeneric hybridization between Glycine max and G. tomentella: Production of F1, amphidiploid, BC1, BC2, BC3, and fertile soybean plants. Theor. Appl. Genet. 128, 1117–1136. doi: 10.1007/s00122-015-2494-0
Smith, J. R., Mengistu, A., Nelson, R. L., and Paris, R. L. (2008). Identification of soybean accessions with high germinability in high-temperature environments. Crop Sci. 48, 2279–2288. doi: 10.2135/cropsci2008.01.0026
Song, K., Yim, W. C., and Lee, B. M. (2017). Expression of heat shock proteins by heat stress in Soybean. Plant Breed. Biotech. 5, 344–353. doi: 10.9787/PBB.2017.5.4.344
Srivastava, S., Pathak, A. D., Gupta, P. S., Shrivastava, A. K., and Srivastava, A. K. (2012). Hydrogen peroxide-scavenging enzymes impart tolerance to high temperature induced oxidative stress in sugarcane. J. Environ. Biol. 33: 657.
Stupar, R. M. (2010). Into the wild: The soybean genome meets its undomesticated relative. Pro. Nat. Acad. Sci. U. S. A. 107, 21947–21948. doi: 10.1073/pnas.1016809108
Sun, Q., Lu, H., Zhang, Q., Wang, D., Chen, J., Xiao, J., et al. (2021). Transcriptome sequencing of wild soybean revealed gene expression dynamics under low nitrogen stress. J. App. Genet. 62, 389–404. doi: 10.1007/s13353-021-00628-1
Sun, Q., Zhao, Y., Zhang, Y., Chen, S., Ying, Q., Lv, Z., et al. (2022). Heat stress may cause a significant reduction of rice yield in China under future climate scenarios. Sci. Total Environ. 818: 151746. doi: 10.1016/j.scitotenv.2021.151746
Suzuki, N., Rivero, R. M., Shulaev, V., Blumwald, E., and Mittler, R. (2014). Abiotic and biotic stress combinations. New Phytol. 203, 32–43. doi: 10.1111/nph.12797
Tariq, A., Jabeen, Z., Farrakh, S., Noreen, K., Arshad, W., Ahmed, H., et al. (2022). Exploring the genetic potential of Pakistani soybean cultivars through RNA-seq based transcriptome analysis. Mol. Biol. Rep. 49, 2889–2897. doi: 10.1007/s11033-021-07104-3
Thomey, M. L., Slattery, R. A., Köhler, I. H., Bernacchi, C. J., and Ort, D. R. (2019). Yield response of field-grown soybean exposed to heat waves under current and elevated [CO2]. Global Change Biol. 25, 4352–4368. doi: 10.1111/gcb.14796
Ullah, A., Nadeem, F., Nawaz, A., Siddique, K. H., and Farooq, M. (2022). Heat stress effects on the reproductive physiology and yield of wheat. J. Agro. Crop Sci. 208, 1–17. doi: 10.1111/jac.12572
Valdés-López, O., Batek, J., Gomez-Hernandez, N., Nguyen, C. T., Isidra-Arellano, M. C., Zhang, N., et al. (2016). Soybean roots grown under heat stress show global changes in their transcriptional and proteomic profiles. Front. Plant Sci. 7:517. doi: 10.3389/fpls.2016.00517
Veas, R. E., Ergo, V. V., Vega, C. R., Lascano, R. H., Rondanini, D. P., and Carrera, C. S. (2021). Soybean seed growth dynamics exposed to heat and water stress during the filling period under field conditions. J. Agro. Crop Sci. 208, 472–485. doi: 10.1111/jac.12523
Verbruggen, N., and Hermans, C. (2008). Proline accumulation in plants: A review. Amino Acids 35, 753–759. doi: 10.1007/s00726-008-0061-6
Vital, R. G., Müller, C., Freire, F. B. S., Silva, F. B., Batista, P. F., Fuentes, D., et al. (2022). Metabolic, physiological and anatomical responses of soybean plants under water deficit and high temperature condition. Sci. Rep. [Preprint]. doi: 10.21203/rs.3.rs-1317816/v1
Wang, L., Liu, L., Ma, Y., Li, S., Dong, S., and Zu, W. (2018). Transcriptome profilling analysis characterized the gene expression patterns responded to combined drought and heat stresses in soybean. Comput. Biol. Chem. 77, 413–429. doi: 10.1016/j.compbiolchem.2018.09.012
Wang, L., Ma, H., Song, L., Shu, Y., and Gu, W. (2012). Comparative proteomics analysis reveals the mechanism of pre-harvest seed deterioration of soybean under high temperature and humidity stress. J. Prot. 75, 2109–2127. doi: 10.1016/j.jprot.2012.01.007
Wang, W., Vinocur, B., Shoseyov, O., and Altman, A. (2004). Role of plant heat-shock proteins and molecular chaperones in the abiotic stress response. Trends Plant Sci. 9, 244–252. doi: 10.1016/j.tplants.2004.03.006
Wei, W., Li, Q. T., Chu, Y. N., Reiter, R. J., Yu, X. M., Zhu, D. H., et al. (2015). Melatonin enhances plant growth and abiotic stress tolerance in soybean plants. J. Exp. Bot. 66, 695–707. doi: 10.1093/jxb/eru392
Xiao, Y., Karikari, B., Wang, L., Chang, F., and Zhao, T. (2021). Structure characterization and potential role of soybean phospholipases a multigene family in response to multiple abiotic stress uncovered by CRISPR/Cas9 technology. Environ. Exp. Bot. 188:104521. doi: 10.1016/j.envexpbot.2021.104521
Xie, W., Zhou, B., Han, Z., and Xu, Y. (2021). Projected changes in heat waves over China: Ensemble result from RegCM4 downscaling simulations. Int. J. Climatol. 41, 3865–3880. doi: 10.1002/joc.7047
Xu, C., Xia, Z., Huang, Z., Xia, C., Huang, J., Zha, M., et al. (2019). Understanding the physiological and transcriptional mechanism of reproductive stage soybean in response to heat stress. Crop Breed. Genet. Gen. 2:e200004. doi: 10.20900/cbgg20200004
Xu, X., Zeng, L., Tao, Y., Vuong, T., Wan, J., Boerma, R., et al. (2013). Pinpointing genes underlying the quantitative trait loci for root-knot nematode resistance in palaeopolyploid soybean by whole genome resequencing. Proc. Natl. Acad. Sci. U. S. A. 110, 13469–13474. doi: 10.1073/pnas.1222368110
Yan, J., Warburton, M., and Crouch, J. (2011). Association mapping for enhancing maize (Zea mays L.) genetic improvement. Crop Sci. 51, 433–449. doi: 10.2135/cropsci2010.04.0233
Yang, Z., Du, H., Xing, X., Li, W., Kong, Y., Li, X., et al. (2022). A small heat shock protein, GmHSP17. 9, from nodule confers symbiotic nitrogen fixation and seed yield in soybean. Plant Biotech. J. 20, 103–115. doi: 10.1111/pbi.13698
Yang, Z., Zhang, Z., Zhang, T., Fahad, S., Cui, K., Nie, L., et al. (2017). The effect of season-long temperature increases on rice cultivars grown in the central and southern regions of China. Front. Plant Sci. 8:1908. doi: 10.3389/fpls.2017.01908
Yin, X., Du, D., Liang, Z., Han, Z., Nian, H., and Ma, Q. (2022). GsMYB7 encoding a R2R3-type MYB transcription factor enhances the tolerance to aluminum stress in soybean (Glycine Max. L). BMC Gen. 23:529. doi: 10.1186/s12864-022-08744-w
Zafar-Ul-Hye, M., Naeem, M., Danish, S., Fahad, S., Datta, R., Abbas, M., et al. (2020). Alleviation of cadmium adverse effects by improving nutrients uptake in bitter gourd through cadmium tolerant rhizobacteria. Environments 7:54. doi: 10.3390/environments7080054
Zandalinas, S. I., Fichman, Y., Devireddy, A. R., Sengupta, S., Azad, R. K., and Mittler, R. (2020). Systemic signaling during abiotic stress combination in plants. Proc. Natl. Acad. Sci. U. S. A. 117, 13810–13820. doi: 10.1073/pnas.2005077117
Zhang, D., Zhang, H., Chu, S., Li, H., Chi, Y., Triebwasser-Freese, D., et al. (2017). Integrating QTL mapping and transcriptomics identifies candidate genes underlying QTLs associated with soybean tolerance to low-phosphorus stress. Plant Mol. Biol. 93, 137–150. doi: 10.1007/s11103-016-0552-x
Zhang, H. Y., Hou, Z. H., Zhang, Y., Li, Z. Y., Chen, J., Zhou, Y. B., et al. (2022a). A soybean EF-Tu family protein GmEF8, an interactor of GmCBL1, enhances drought and heat tolerance in transgenic Arabidopsis and soybean. Int. J. Biol. Macromol. 205, 462–472. doi: 10.1016/j.ijbiomac.2022.01.165
Zhang, M., Liu, S., Wang, Z., Yuan, Y., Zhang, Z., Liang, Q., et al. (2022b). Progress in soybean functional genomics over the past decade. Plant Biotech. J. 20:256. doi: 10.1111/pbi.13682
Zhang, P. G., Hou, Z. H., Chen, J., Zhou, Y. B., Chen, M., Fang, Z. W., et al. (2022c). The non-specific lipid transfer protein GmLtpI. 3 is involved in drought and salt tolerance in soybean. Environ. Exp. Bot. 196:104823. doi: 10.1016/j.envexpbot.2022.104823
Zhao, L., Wang, Z., Lu, Q., Wang, P., Li, Y., Lv, Q., et al. (2013). Overexpression of a GmGBP1 ortholog of soybean enhances the responses to flowering, stem elongation and heat tolerance in transgenic tobaccos. Plant Mol. Biol. 82, 279–299. doi: 10.1007/s11103-013-0062-z
Keywords: soybean, heat stress, yield, molecular techniques, global warming
Citation: Jianing G, Yuhong G, Yijun G, Rasheed A, Qian Z, Zhiming X, Mahmood A, Shuheng Z, Zhuo Z, Zhuo Z, Xiaoxue W and Jian W (2022) Improvement of heat stress tolerance in soybean (Glycine max L), by using conventional and molecular tools. Front. Plant Sci. 13:993189. doi: 10.3389/fpls.2022.993189
Received: 13 July 2022; Accepted: 23 August 2022;
Published: 26 September 2022.
Edited by:
Iftikhar Ali, State Key Laboratory of Molecular Developmental Biology, Institute of Genetics and Developmental Biology (CAS), ChinaReviewed by:
Tariq Aziz, University of Okara, PakistanGuoqian Yang, Brookhaven National Laboratory (DOE), United States
Copyright © 2022 Jianing, Yuhong, Yijun, Rasheed, Qian, Zhiming, Mahmood, Shuheng, Zhuo, Zhuo, Xiaoxue and Jian. This is an open-access article distributed under the terms of the Creative Commons Attribution License (CC BY). The use, distribution or reproduction in other forums is permitted, provided the original author(s) and the copyright owner(s) are credited and that the original publication in this journal is cited, in accordance with accepted academic practice. No use, distribution or reproduction is permitted which does not comply with these terms.
*Correspondence: Gai Yuhong, Z3loMzIxMDFAMTYzLmNvbQ==; Wang Xiaoxue, d2FuZ3h4QHN5YXUuZWR1LmNu; Wei Jian, MTQ4MDUwNDU5QHFxLmNvbQ==
†These authors have contributed equally to this work