- Department of Grassland Science, College of Animal Science and Technology, Northeast Agricultural University, Harbin, China
In recent years, drought stress caused by global warming has become a major constraint on agriculture. The thiamine thiazole synthase (THI1) is responsible for controlling thiamine production in plants displaying a response to various abiotic stresses. Nonetheless, most of the THI1 activities in plants remain largely unknown. In this study, we extracted MsTHI1 from alfalfa and demonstrated its beneficial impact on improving the resistance of plants to stress conditions. The highest levels of MsTHI1 expression were identified in alfalfa leaves, triggered by exposure to cold, drought, salt, or alkaline conditions. The upregulation of MsTHI1 in drought-stressed transgenic plants resulted in enhanced accumulation of vitamin B1 (VB1), chlorophyll a (Chl a), chlorophyll b (Chl b), soluble protein, higher soil and plant analyzer development (SPAD) value, and the activity of peroxidase (POD), maintained Fv/Fm, and decreased lipid peroxidation. Moreover, overexpression of MsTHI1 upregulated the transcription of THI4, TPK1, RbcX2, Cu/Zn-SOD, CPK13, and CPK32 and downregulated the transcription of TH1 and CPK17 in transgenic alfalfa under drought stress. These results suggested that MsTHI1 enhances drought tolerance by strengthening photosynthesis, regulating the antioxidant defense system, maintaining osmotic homeostasis, and mediating plant signal transduction.
Introduction
Plants must deal with abiotic and biotic stresses in the wild, where they are constantly subjected to unfavorable environmental stresses (Zhu, 2016; Rao et al., 2020). The increased frequency and intensity of extreme weather events due to climate changes make drought stress a significant constraint on agriculture (Rivero et al., 2022). Climate change has made drought an even more substantial problem, dramatically reducing crop yields and quality and limiting plant development (Li et al., 2020). Plants respond to drought conditions by altering photosynthetic, physiological, metabolic, and molecular activities, which in turn causes membrane rearrangement, osmotic pressure aggravation, the buildup of reactive oxygen species (ROS), in addition to functional abnormalities in cellular components (Roo et al., 2020; Yan et al., 2020). As a result, plants have developed sophisticated defense mechanisms to mitigate the detriment of drought conditions, from molecular and physiological to cellular and ecological levels (Xu and Zhou, 2008; Hussain et al., 2018). Rapid genetic enhancements in drought-tolerant crops may be possible if proteins or genes involved in such alterations are identified.
As an enzyme cofactor, thiamine [vitamin B1 (VB1)] is an integral part of the diets of all living species, since it is required for the appropriate metabolic activity of all living things (Strobbe et al., 2022). In plants, the biosynthesis of thiamine is promoted during adaptation responses to persistent abiotic stresses, such as drought, cold, heat, salinity, and oxidative stress (Rapala-Kozik et al., 2008; Yu et al., 2014; Meng et al., 2017; Amjad et al., 2021; Qian et al., 2022). Thiamine can improve the oxidation state of mitochondria, enhance the activities of pyruvate dehydrogenase (PDH), release ROS quickly when stimulated by stress, and activate downstream genes to induce stress resistance in plants. Thiamine thiazole synthase (THI1), also known as 4-methyl-5-hydroxyethyl thiazole phosphate (HET-P) synthase, is a major enzyme in thiamine biosynthesis, and it performs a crucial function in controlling thiamine production in plants (Chen et al., 2022). Nodule expansion and seed development in Lotus japonicus depend on the thiamine synthesis controlled by THI1 (Nagae et al., 2016). Concurrently, THI1 reacted to various abiotic stimuli, which may improve mitochondrial DNA damage tolerance (Rapala-Kozik et al., 2012). The expression of THI was upregulated in Elaeis guineensis (oil palm) under osmotic, salinity, and oxidative stress, and was also found to be predominantly upregulated in the early phase (2–6 h) of the NaCl or sorbitol treatment in Arabidopsis (Rapala-Kozik et al., 2012; Abidin et al., 2016). When temperatures increased, THI1 was more prevalent than usual, while thiamine concentrations were lower than usual in Oryza meridionalis (Scafaro et al., 2010). THI1 plays a vital role in the drought responsiveness in Arabidopsis and the abscisic acid (ABA) signaling in guard cells (Livak and Schmittgen, 2000). To regulate ABA activation of slow-type anion channels and ABA-induced stomatal closure in Arabidopsis, THI1 can inhibit Ca2+-dependent protein kinase (CPK33) activity in a plasma membrane-restricted way (Li et al., 2016).
A significant amount of Medicago sativa, also known as alfalfa, is grown globally as a leguminous feed crop. Alfalfa is essential for the growth of herbivorous animal husbandry and agricultural sustainability due to its high protein content and palatability and symbiotic nitrogen fixation (Yang et al., 2021). The alfalfa acreage has significantly risen in China since the Chinese government has started a strategy to strengthen the milk and alfalfa sectors in 2012 (Fan et al., 2018). Although the deep root structure of alfalfa protects it from drought on the dry and semi-arid ground, the scanty and erratic precipitation of the region has severely hampered crop yields and quality, stifling the growth in the alfalfa business (Zhang and Shi, 2018; Zhao et al., 2019). Enhancing drought resistance of alfalfa requires first learning about its molecular process by which the plant reacts to drought stress. Even though numerous THI1 genes have important activities in other plants, little is known about THI1 in alfalfa. In this investigation, the MsTHI1 was extracted from alfalfa and was characterized. The transcription levels of MsTHI1 in reaction to cold, drought, salt, and alkalinity were tested to identify how MsTHI1 is expressed in response to abiotic stresses. The physiological functions of MsTHI1 under drought stress have also been studied by overexpressing MsTHI1 in Nicotiana benthamiana (tobacco) and alfalfa. In addition, the regulation mechanism was illustrated by quantifying the expression of associated genes involved in the thiamine pathway and stress response. Our findings demonstrated that MsTHI1 upregulation increased drought resistance by boosting photosynthetic activities, decreasing ROS formation, and preserving osmotic stability. This study will improve our knowledge on how alfalfa reacts to droughts, which could have implications for stress-resistance breeding efforts.
Materials and methods
Plant materials and stress treatments
Alfalfa (Medicago sativa L. cv. Longmu 801) seeds were treated with 75% ethanol solution for 30 s, followed by treatment with 10% NaClO solution for 10 min. The seeds were then germinated on moist filter paper in Petri dishes after being cleaned 4–5 times with ultrapure water. When the seedlings were 5 days old, they were transplanted into a pot with nutrient soil, perlite, and vermiculite (1:1:1). They were placed in a growth chamber with 60% humidity and a 16 h photoperiod at 24°C and irrigated with 1/2 Hoagland nutrient solution every 2 days. The seedlings were exposed to stress treatments for 4 weeks at 0 (control), 3, 6, 12, 24, and 48 h. To simulate the effects of drought, salt, and alkaline stress, the alfalfa plants were transplanted into nutritional solutions containing 15% PEG-6000, 150 mM NaCl, and 150 mM NaHCO3, respectively. To carry out the cold treatment, the plants were placed in a growth chamber maintained at 4°C. Leaves, stems, and roots were promptly removed, frozen in liquid nitrogen, and stored at −80°C for subsequent examination. The experiment was performed in triplicate.
Isolation and sequence analysis of MsTHI1 gene
The total RNA from alfalfa leaves was isolated using an Ultrapure RNA kit (CWBIO, Beijing, China). Following the manufacturer's instructions, first-strand complementary DNA (cDNA) was generated using the HiScript II 1st Strand cDNA Synthesis Kit (+gDNA wiper) (Vazyme, Nanjing, China). The coding DNA sequence (CDS) of MsTHI1 was obtained via PCR with degenerate primers (MsTHI1-F/MsTHI1-R, Supplementary Table S1) that were designed based on the MtTHI1 sequence of Medicago truncatula retrieved from the National Center for Biotechnology Information (NCBI). The coding uses a 2×UniqueTM Taq Master Mix (with Dye) (Novogene, Beijing, China) following the manufacturer's instructions. The PCR product was inserted into the pEASY-Blunt Simple Cloning vector using ClonExpress®II One Step Cloning Kit (Vazyme, Nanjing, China) and sequenced by SangonBiotech Co. (Shanghai, China).
DNAMAN software performed multiple comparison determinations (version 9, LynnonBiosoft). The phylogenetic tree was generated using MEGA X software and the neighboring connection method. MEME was employed to examine the conserved motif (http://memesuite.org/). Protparam (http://expasy.org/Proteomics) was used to explore the physical and chemical characteristics of polygenes. The secondary structure of the protein was anticipated by the web application, SPOMA (http://pbil.ibcp.fr). The 3D structure of the MsTHI1 amino acid sequence was predicted using SWISS-MODEL (https://swissmodel.expasy.org/). Using CELLOv.2.5 (http://cello.life.nctu.edu.tw/), the subcellular localization of amino acid sequences in alfalfa was predicted.
Subcellular localization of MsTHI1 protein
The coding region of MsTHI1 without a stop codon was amplified using specific primers (MsTHI1-BamHI-F/MsTHI1-SacI-R, Supplementary Table S1) with BamHI and SacI restriction sites. The amplicons were double-digested with SacI and BamHI, followed by joining with pCAMBIA-1300 to create a fusion plasmid, pCAMBIA1300-MsTHI1-green fluorescent protein (GFP). The MsTHI1 fusion protein was transformed into the epidermal cells of N. benthamiana through the infiltration of Agrobacterium tumefaciens. A confocal laser scanning microscope was used to identify the fluorescence signal after 48 h incubation at 25°C in the dark (Leica TCS SP2 AOBS, Germany).
Quantitative real-time PCR analysis
Quantitative real-time PCR (qPCR) was used to determine how MsTHI1 is expressed in different tissues and how it responds to environmental stress in transgenic alfalfa. Total RNA was removed from each piece of harvested tissue and reverse transcribed into cDNA. The CDS of MsTHI1 was used to make the qPCR primers for MsTHI1. The GAPDH gene was utilized in alfalfa as an internal control (He et al., 2020). In transgenic tobacco plants, the tobacco actin gene (NtActin) was used as a reference gene. Using the online NCBI Primer-BLAST (http://www.ncbi.nlm.nih.gov/tools/primer-blast/), gene-specific primers for thiamine pathway genes were made and are listed in Supplementary Table S1. Three separate biological replicates and three identical reactions were done with each sample. The 2−ΔΔCT comparative method was used to determine how each gene was expressed (Livak and Schmittgen, 2000).
Plant transformation and generation of transgenic plants
For generating transgenic tobacco plants, the gene-specific primers (MsTHI1-PstI-F/MsTHI1-BamHI-R, Supplementary Table S1) of MsTHI1 were inserted into the pCAMBIA1300 vector to make pCAMBIA1300-MsTHI1 expression vector. Afterward, the recombinant vector was put into the A. tumefaciens strain, EHA105. Then, the recombinant vector was introduced into EHA105, and transformed into wild-type (WT) tobacco by the agroinfiltration method (Kaur et al., 2021). A 20 mg·L−1 hygromycin (Hyg) was put into the medium for seed germination to find transgenic lines (T1) that are resistant to Hyg. Utilizing specific primers (Hyg-F/Hyg-R, Supplementary Table S1), a PCR test was used to detect the Hyg gene in homozygous transformants (T2), which was validated by qPCR using specific primers for MsTHI1. NtActin was used as a control within the lab. For more drought stress tests, lines 2 (OV#Nt2), 3 (OV#Nt3), and 7 (OV#Nt7) from T2-generation homozygous lines and WT lines were utilized.
To create transgenic alfalfa plants, the gene-specific primers (MsTHI1-SpeI-F/MsTHI1-XbaI-R, Supplementary Table S1) of MsTHI1 were inserted into the pMDC123 vector to make pMDC123-MsTHI1 expression vector. After that, the recombinant vector was put into A. tumefaciens strain, LB4404, and transformed into the cotyledon nodes of alfalfa using the agroinfiltration method (Sun et al., 2020). Since the pMDC123 vector has a Bar resistance gene that makes it resistant to the herbicide glufosinate, 1.0 mg·L−1 of glufosinate-ammonium was used to pick out the transformants. The shoots grown back were planted in 1/2 Murashige and Skoog (MS) medium. The herbicide-resistant plants were then put through a PCR test to find the Bar gene by employing specific primers (Bar-F/Bar-R, Supplementary Table S1), which was affirmed by qPCR using specific primers for MsTHI1. The GAPDH gene from alfalfa was used as a control for the study. The homozygous lines were then passed on and used in more tests to find how drought affects plants.
Drought tolerance assay
On 1/2-strength MS medium plates, seeds of WT tobacco and transgenic tobacco lines were planted and kept at 4°C for 2 days before being moved to a growth chamber at 25°C for 7 days. After the seeds were germinated, they were moved to a greenhouse and put in plastic containers with vermiculite. Drought resistance tests were done on 3-week-old plants. The WT alfalfa and transgenic alfalfa were spread by cutting. Then, the same WT and transgenic plants were moved into plastic pots with vermiculite and put in a greenhouse. Drought tolerance tests were done on 6-week-old plants. For drought tolerance tests, the plants were irrigated for a week with a nutrient solution with 15% PEG-6000. Afterward, the treated leaves of the plants were picked right away, frozen in liquid nitrogen, and kept at −80°C for subsequent use.
Measurement of physiological and biochemical changes
Stress-treated WT, transgenic plants, and three categories of biological repeats, in addition to the three technical repeats, were set up at each time point. A kit was used to quantify the amount of VB1 based on the manufacturer's recommendations (Comin, Suzhou, China). The accumulation of malondialdehyde (MDA) was determined by a modified thiobarbituric acid (TBA) method as described by Puckette et al. (2007). The relative chlorophyll content [soil and plant analyzer development (SPAD) value] was measured by the SPAD-502 Chlorophyll Meter, and three leaves from each group were used to determine the value. Three measurements were made to find the average SPAD value. Three leaves kept in the dark for 20 min were measured thrice to get an average Fv/Fm value. The amount of superoxide anion radical () was measured by making some changes to the procedure reported by Jiang and Zhang (2001). The nitroblue tetrazolium (NBT) method (Giannopolitis and Ries, 1972) with a few minor changes were used to test the activity of superoxide dismutase (SOD, EC1.15.1.1). The guaiacol method was used to measure the peroxidase activity (POD, EC 1.11.1.7) (Polle et al., 1994). The amount of proline (Pro) was found using spectrophotometry and the ninhydrin method (Zhao et al., 2009). Wellburn and Lichtenthaler (1984) found out the quantity of chlorophyll a (Chl a) and chlorophyll b (Chl b) present in plants. Tietze (1969) used fluorometry to identify reduced glutathione (GSH) in the body. The Bradford method was employed to determine the soluble protein (Bradford, 1976).
Expression analysis of related genes
In WT and transgenic alfalfa, the expression of eight genes associated with the thiamine pathway and stress response was analyzed by employing qPCR; this was done to show how MsTHI1 helps plants deal with drought. Some of these genes, like thiazole biosynthetic enzyme (THIAMIN4, THI4), 4-amino-2-methyl-5-hydroxymethyl pyrimidine phosphate (HMP-P) kinase/thiamin monophosphate (TMP) pyrophosphorylase (TH1), thiamine pyrophosphokinase (TPK1), chaperonin-like RbcX protein 2 (RbcX2), SOD [Cu-Zn] (Cu/Zn-SOD), and calcium-dependent protein kinases (CPK13, CPK17, and CPK32) play critical roles in thiamine synthesis and abiotic stress response. The GAPDH gene was utilized as an internal reference gene, and Supplementary Table S1 lists the primers employed in this study.
Data analysis
All experiments presented in this study were done three times in the same way. For statistical analysis with SPSS 22.0, the Student's t-test and one-way analysis of variance were used to identify significant differences.
Results
Isolation and characteristics of MsTHI1
The MsTHI1 CDS (GenBank accession: MH206189) encoded a protein of 350 amino acids with three putative thiazole synthesis domains, 20 essential amino acids, 28 positively charged amino acid residues (Arg, Lys), and 36 negatively charged amino acid residues (Asp, Glu) (Supplementary Figure S1A). The MsTHI1 has the chemical formula of C1623H2605N441O500S19, the molecular weight (MW) of 36.91 kDa, and a predicted isoelectric point (PI) of 5.68. MsTHI1 protein aqueous solution at 280 nm has an extinction coefficient of 18,575, fat coefficient of 90.3, an average hydrophilic coefficient of 0.112, and an instability coefficient of 33.24, suggesting the stability of the protein. The neighbor-joining approach used to generate the phylogenetic tree showed that MsTHI1 and MtTHI1 were on the same branch and that MsTHI1 was the most similar to MtTHI1 (Supplementary Figure S1B). MsTHI1 protein, as accurately predicted by its three-dimensional structure, consists of 32.86% α-helices, 18.57% β-extension chain, 8.29% β-rotation angle, and 40.29% irregular curls (Supplementary Figure S1C).
Subcellular localization of MsTHI1
To investigate the subcellular distribution of MsTHI1, a GFP-MsTHI1 fusion protein was transiently coexpressed in N. benthamiana leaves and then visualized using laser scanning confocal microscopy. MsTHI1-GFP fluorescence was found in the cell membrane and chloroplasts, as evidenced by the brilliant field under infected leaves, as well as the fusion picture (Figure 1). However, predictions of CELLO 2.5 to find where MsTHI1 is subcellularly localized suggested that it is most likely to be found in chloroplasts (4.275). Therefore, the MsTHI1 protein was primarily found in chloroplasts.
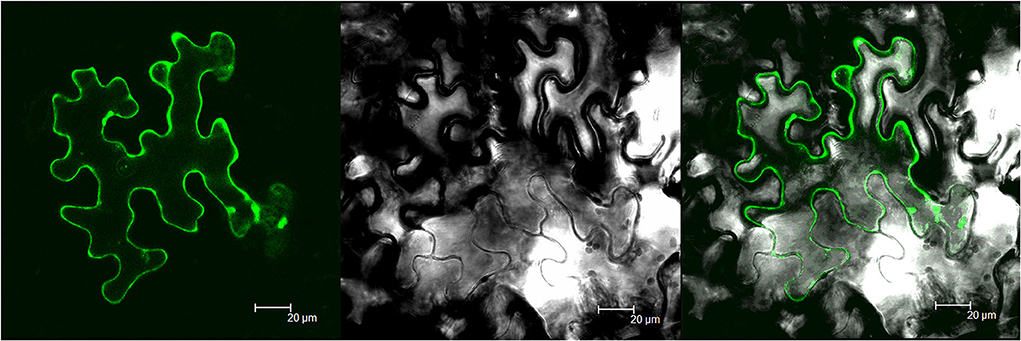
Figure 1. Subcellular localization of MsTHI1. (A) fusion GFP fluorescence. (B) bright field under infected leaves, (C) fusion picture. Scale bar, 20 μm.
MsTHI1 expression patterns
The expression profiles of MsTHI1 in distinct alfalfa tissues and its sensitivities to drought, cold, salt, and alkaline stress were studied through qPCR. The findings demonstrated that the expression pattern of MsTHI1 in alfalfa leaves was more significant than in the roots and stems (Figure 2A). The expression level of MsTHI1 was significantly suppressed during cold stress (Figure 2B). The expression level of MsTHI1 changed comparably with 15% of PEG and 150 mM of NaCl treatment. They peaked at 3 h, dropped at 6 and 12 h, and climbed once more at 24 and 48 h. Contrary to the MsTHI1 upregulation under salt stress, the MsTHI1 expression was significantly suppressed at 6 and 12 h under drought stress. The expression level of MsTHI1 at 150 mM of NaHCO3 peaked at 3 h, followed by a drop from 6 to 48 h. These findings indicated that MsTHI1 expression was tissue-specific in alfalfa and that MsTHI1 might implicate the self-protection mechanisms of alfalfa in response to aggressive ecological scenarios.
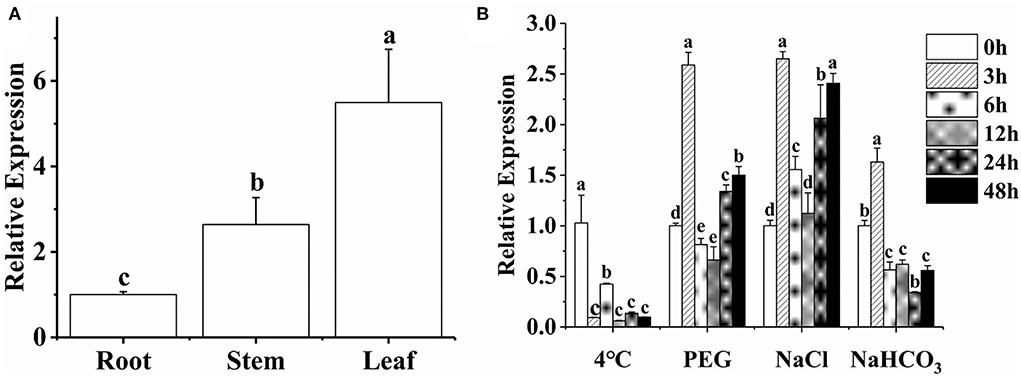
Figure 2. Expression pattern of MsTHI1. (A) Tissue-specific expression of MsTHI in the alfalfa root, stem, and leaf. (B) Expression analysis of MsTHI1 in leaves of alfalfa subjected to cold (4°C), drought (15% PEG), salt (150 mM of NaCl), and alkaline (150 mM of NaHCO3) stresses. The expression levels were normalized in the alfalfa GAPDH gene. Mean ± standard deviations from three biological replicates are shown with error bars. These letters denote variations that are statistically significant (α = 0.05).
The ability of transgenic plants to tolerate drought is enhanced by MsTHI1 upregulation
Transgenic tobacco (T2) and alfalfa plants were created and validated with Hyg and Bar, respectively, through PCR to examine the involvement of MsTHI1 in the plant drought tolerance (Supplementary Figure S2). MsTHI1 transcript levels in OV#Nt2, OV#Nt3, as well as OV#Nt7 transgenic lines were 413.6, 3593.0, and 3014.8 times greater than those in WT tobacco plants, accordingly (Figure 3A). The expression of MsTHI1 was 29.2 and 6.9 times greater in OV#Ms7 and OV#Ms9 transgenic lines, respectively, compared to that in WT alfalfa plants (Figure 3B). No phenotype was observed between MsTHI1-transformed and WT plants under normal control conditions in either transgenic tobacco or transgenic alfalfa (Figures 3C,D). When exposed to dryness for a week, the leaves of both WT tobacco and alfalfa plants wilted, and the growth was significantly suppressed. Despite this, OV#Nt2, OV#Nt3, OV#Nt7, OV#Ms7, and OV#Ms9 continued to develop properly with only a few yellow leaves. The results demonstrated that the drought resistance of transgenic plants was enhanced by overexpressing MsTHI1.
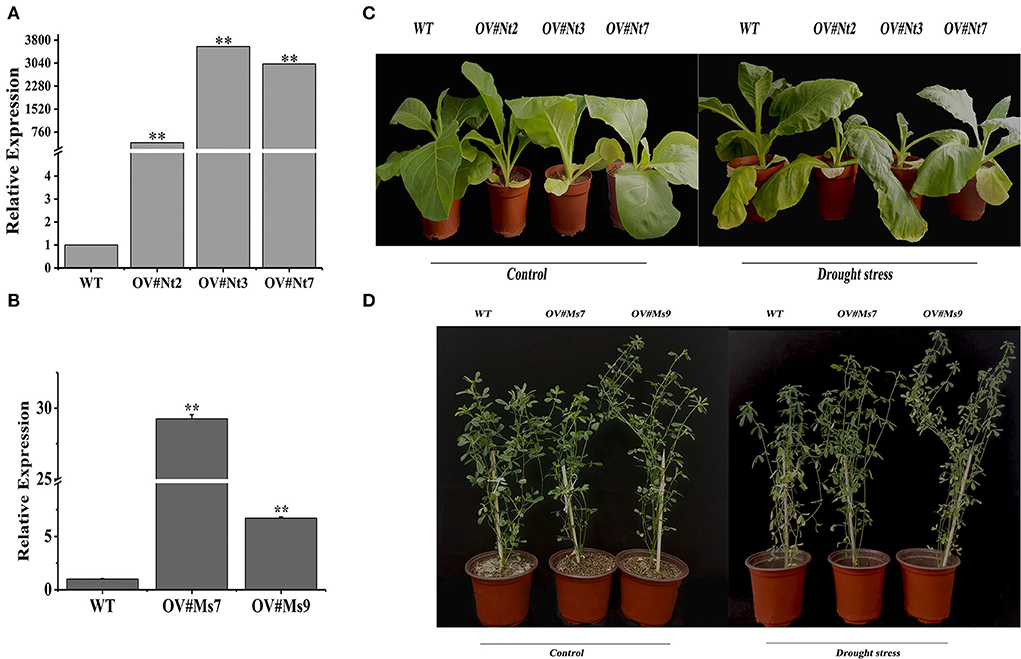
Figure 3. Identification of transformation with MsTHI1 via qPCR and phenotype of transformation with MsTHI1 under control conditions and drought stress. (A) Identifying transgenic tobacco via quantitative real-time PCR (qPCR). (B) Identifying transgenic alfalfa via qPCR. (C) Phenotype of three-week-old transgenic tobacco plants with overexpressed MsTHI1 under control and drought stress for seven days. (D) Phenotype of six-week-old transgenic alfalfa plants with overexpressed MsTHI1 under control and drought stress for seven days. NtActin and alfalfa GAPDH gene were used as the internal reference control for identifying transgenic tobacco and alfalfa, respectively, via qPCR. Mean ± standard deviations from three biological replicates are shown with error bars. “**”: extremely significant difference between WT and transformation line (P < 0.01). WT: wild type; OV#Nt2, OV#Nt3, and OV#Nt7: transgenic tobacco lines 2, 3, and 7, respectively. OV#Ms7 and OV#Ms9: transgenic alfalfa lines 7 and 9, respectively.
Modifications in the physiology of transgenic plants that overexpress MsTHI1 during conditions of drought stress
To better demonstrate the role of MsTHI1 in stress conditions tolerance, the physiological modifications in the transgenic tobacco or even alfalfa plants experiencing environmental stresses were studied. Transgenic tobacco with an increased VB1 concentration was found both in normal and drought conditions due to MsTHI1 upregulation (Figure 4). During normal conditions, MDA levels in WT plants were higher than in OV#Nt2 and OV#Nt3 species. Even though the SPAD value of the transgenic tobacco was lesser than that of the WT plants in the control environment, all plants increased their SPAD value after being subjected to drought treatment, with OV#Nt7 plants showing the most significant increase. During drought conditions, the Fv/Fm ratio decreased more quickly in WT plants than in transgenic tobacco, with OV#Nt2 plants having the highest Fv/Fm ratio (0.79). Following drought stress, the WT and transgenic tobacco showed an increase in concentration and SOD and POD activities. While WT plants increased their level quicker than the transgenic tobacco, the latter eventually caught up. Considering drought stress, there was no significant difference between the WT and transgenic tobacco in free Pro concentration.
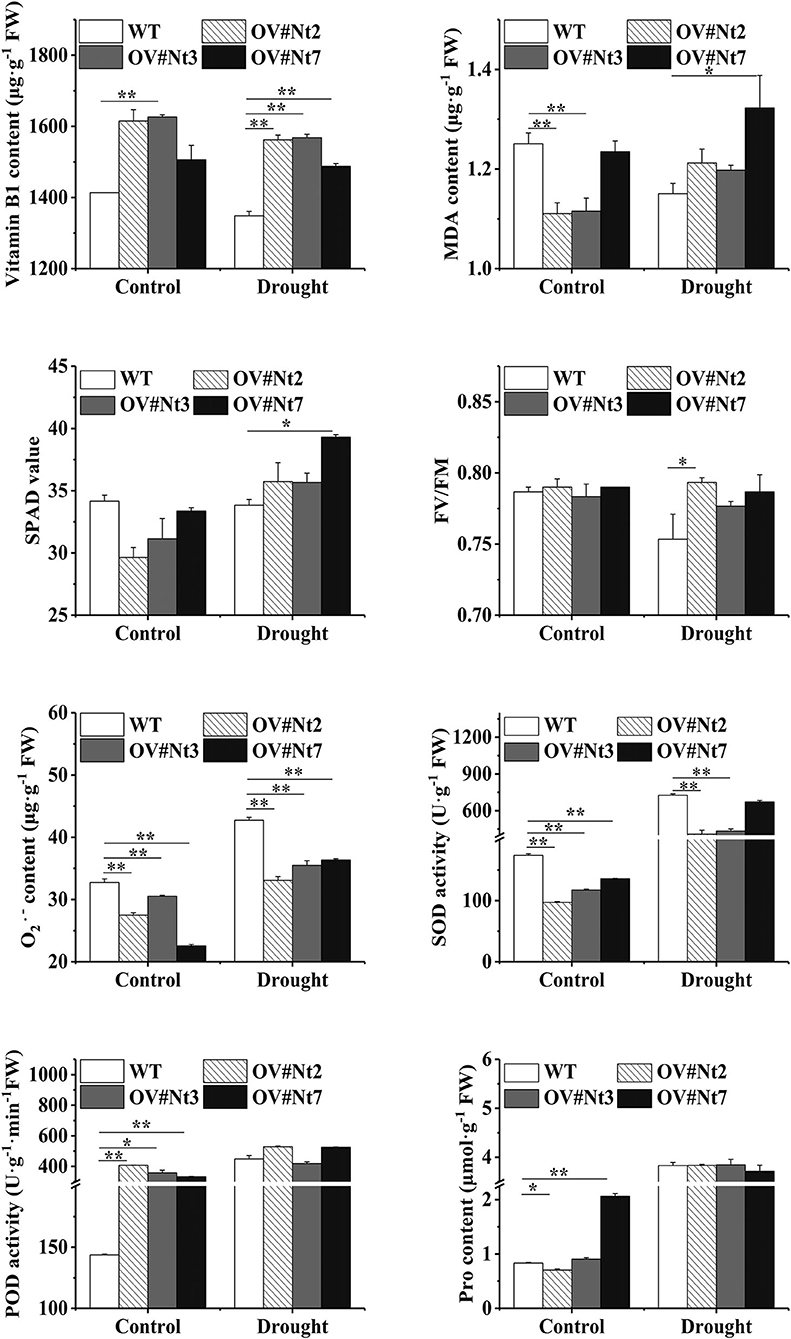
Figure 4. Modifications in the physiology of transgenic tobacco plants that overexpress MsTHI1 during control or under drought stress. Mean ± standard deviations from three biological replicates are displayed with error bars. MDA, malondialdehyde. SPAD, soil and plant analyzer development. Fv/Fm, the PS II primary light energy conversion efficiency. , superoxide anion radical. SOD, superoxide dismutase. POD, peroxidase. Pro, proline. WT, wild type. OV#Nt2, OV#Nt3, and OV#Nt7, transgenic tobacco lines 2, 3, and 7, respectively. “*”, significant difference between WT and transgenic tobacco lines (P < 0.05). “**”, extremely significant difference between WT and transgenic tobacco lines (P < 0.01).
During drought conditions, VB1 levels in transgenic and WT alfalfa decreased, but the transgenic plants maintained a greater VB1 concentration than the WT ones. During drought conditions, MDA levels of transgenic and WT alfalfa were elevated, whereas the MDA level of transgenic alfalfa was lower under control or drought conditions. Chl a and Chl b concentrations were reduced during drought and were increased in MsTHI-transformed plants, mirroring the pattern of VB1. Transgenic and WT alfalfa both elevated their levels in response to drought conditions, whereas the level of transgenic alfalfa remained lower in control and drought conditions. However, the functions of SOD and the concentrations of GSH in transgenic alfalfa plants were significantly decreased compared to WT plants during control and droughts scenarios, confirming the role of VB1 in alfalfa drought tolerance. Transgenic alfalfa and WT plants exhibited statistically significant elevations in POD activity and Pro content in response to drought conditions.
Expression analysis of associated genes in the overexpression of MsTHI1 in transgenic alfalfa under drought stress
Eight associated genes involving THI4, TH1, TPK1, RbcX2, Cu/Zn-SOD, CPK13, CPK17, and CPK32 were assessed for their expression patterns in transgenic and WT alfalfa plants under drought conditions using qPCR. As depicted in Figure 6, drought stress dramatically upregulated the expression of THI4, TPK1, RbcX2, and CPK32 in transgenic and WT alfalfa plants. Intriguingly, during drought stress, TH1 and CPK17 expression levels were reduced in transgenic alfalfa while they were increased in WT alfalfa. During drought conditions, Cu/Zn-SOD expression did not alter much in the transgenic alfalfa, whereas it was significantly upregulated in WT alfalfa. During drought stress, the CPK13 expression was elevated in the transgenic alfalfa but suppressed in WT plants.
Discussion
To support proper metabolic activity, people depend on an adequate thiamin intake from food. Metabolic engineering to boost thiamin production in plants is a promising approach to reducing VB1 deficiency and, by extension, improving worldwide human health (Strobbe et al., 2021). In addition to its established roles in thiamine synthesis and mitochondrial DNA damage resistance, THI1 contributes significantly to plant abiotic response to stress (Rapala-Kozik et al., 2012; Li et al., 2016). Several plant THI1 genes that respond to drought, salinity, heat, and oxidative stress have been identified (Livak and Schmittgen, 2000; Scafaro et al., 2010; Abidin et al., 2016; Mangel et al., 2017; Chen et al., 2022), but no research has been conducted on alfalfa. Here, we report the isolation and functional characterization of a THI1 gene (called MsTHI1) from alfalfa. The protein encoded by MsTHI1 is 350 amino acids long. It has three putative thiazole synthesis domains (Supplementary Figure S1A), all of which bind to 2-carboxylate-4-methyl-5-beta-(ethyladenosine 5'-diphosphate) thiazole, a possible intermediate in the thiazole biosynthesis of Eukaryotes (Godoi et al., 2006). The MsTHI1 protein was localized mainly in the chloroplast of plant cells (Figure 1), as is the case for the translation initiation at the first AUG (start codons) directs translocation of THI1 to chloroplasts, indicating a high requirement for THI1 in chloroplasts (Chabregas et al., 2003). Similar to THI1 expression in Arabidopsis (Teixeira et al., 2005; Dong et al., 2015), the expression level of MsTHI1 in alfalfa leaves was higher than that in the roots and stems (Figure 2A), confirming the tissue-specific expression of THI1. The MsTHI1 was quickly upregulated in alfalfa leaves within 3 h during drought, salt, and alkaline stress, and downregulated under cold stress (Figure 2B), which might contribute to the self-protection mechanisms of alfalfa in response to unfavorable ecological scenarios. Although the expression of MsTHI1 was similar in response to drought and salt, MsTHI1 responses were more sensitive to salt, which may be associated with the characters of the alfalfa cultivar used in the study.
Overexpressing MsTHI1 can increase drought resistance in transgenic plants. Drought stress significantly stunted the development of WT tobacco and alfalfa plants (Figure 3). To better understand the role of MsTHI1 in plants during drought conditions, we studied the physiological and biochemical responses of transgenic plants. VB1 is a vital member of the vitamin B group, and the elevation in the level of VB1 is important in alleviating stressful conditions (Amjad et al., 2021). Our results demonstrated that VB1 levels in transgenic tobacco and alfalfa were always significantly higher than in WT plants. However, VB1 contents diminished in WT and transgenic plants under drought conditions suggesting that MsTHI1 overexpression can counteract drought stress by consuming VB1. The MDA amount was also analyzed as a vital sign of lipid peroxidation in the membrane, reflecting stress-induced molecular damage. Following exposure to drought, transgenic SbWRKY30 Arabidopsis, as well as rice plants, had lower levels of MDA than WT plants (Yang et al., 2020). In this case, MDA levels in MsTHI1-overexpressed alfalfa were considerably lower than in WT alfalfa during control and drought stress, demonstrating a robust ability to reduce membrane damage. However, the MDA content in the transgenic tobacco was higher than that in the WT tobacco after drought stress. The results may reflect the difference in ROS scavenging capacity and plasma membrane damage in alfalfa and tobacco. Balanced photosynthesis under drought is necessary for better survivability and agricultural benefits in terms of biomass and output (Daszkowska-Golec et al., 2019). Leaf SPAD readings were directly correlated with grain output, and most of the high SPAD genotypes sustained hot canopies under drought (Raina et al., 2019). The chlorophyll contents and Fv/Fm, utilized as reliable benchmark indicators in identifying drought-adapted genotypes, were reduced under drought-treated Zea mays (maize) seedlings (Chen et al., 2016). In this study, the SPAD value in OV#Nt7 was substantially higher than that in the WT tobacco under drought stress. Simultaneously, the decline rate of Fv/Fm in the WT tobacco was faster than in transgenic plants during drought conditions. The levels of Chl a and Chl b reduced during drought conditions, but the alfalfa transformed with MsTHI had more significant Chl a and Chl b levels. These results revealed that MsTHI1 upregulation might have a function of controlling chlorophyll content to balance photosynthesis, thus conferring a degree of drought conditions.
Drought stress can produce an immediate generation of ROS. Plants might elicit an antioxidant protective mechanism to scavenge superfluous ROS and protect the cells against oxidative stress during stressful circumstances (Sharma et al., 2012). The antioxidant enzymes, including SOD and POD, and non-enzymatic antioxidants, including GSH, are critical in detoxifying stress-induced ROS. Herein, we noticed that the transgenic tobacco and alfalfa revealed a lower level than WT plants during drought conditions (Figures 4, 5). Nevertheless, SOD activities and GSH concentration in the transgenic alfalfa were similarly lesser than in WT plants. The variations in POD activities depend on the expression pattern of MsTHI1 in transformants. There was no difference in the POD activity between transgenic and WT tobacco under drought stress. Conversely, the concentration of soluble protein fell quickly during drought conditions. Previous findings have indicated that the rise of VB1 by stimulating thiamine metabolism protected cell organelles and decreased the ROS levels in the cells of Taxus Chinensis, and chlorophyll could be employed as an antioxidant (Meng et al., 2017; Agathokleous et al., 2020). Consequently, ROS scavenging in transgenic plants might correlate with MsTHI1 upregulation, which triggered the thiamine metabolism and the aggregation of chlorophyll to decrease the amount of ROS. The soluble protein and free Pro are key osmotic regulators, which can raise the osmotic potential of cells and boost plant tolerance to stressful conditions (Hosseinifard et al., 2022). In the present research, the soluble protein level in transgenic alfalfa was mainly greater than that in WT alfalfa during control or drought settings. Although the level of Pro in transgenic alfalfa was consistently lower than that in WT alfalfa throughout control and drought situations, there was no difference in the level of Pro between transgenic and WT tobacco under drought conditions. These findings demonstrated that enhancing drought tolerance in transgenic plants might link with the buildup of soluble protein during drought environments.
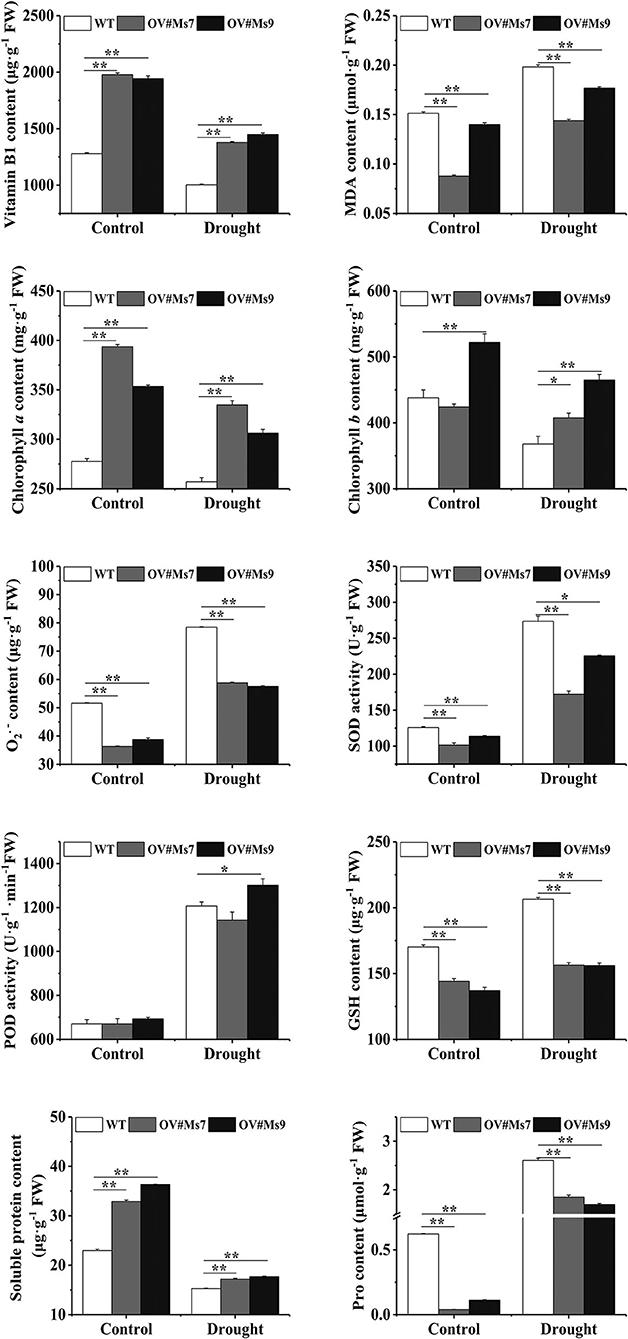
Figure 5. Physiological changes of transgenic alfalfa plants with overexpressing MsTHI1 under control and drought stress. Mean ± standard deviations from three biological replicates are depicted with error bars. MDA, malondialdehyde. superoxide anion radical. SOD, superoxide dismutase. POD, peroxidase. GSH, reduced glutathione. Pro, proline. WT, wild type. OV#Ms7 and OV#Ms9, transgenic alfalfa lines 7 and 9, respectively. “*”, significant difference between WT and transgenic tobacco lines (P < 0.05). “**”, extremely significant difference between WT and transgenic tobacco lines (P < 0.01).
Upregulated MsTHI1 alfalfa responds to drought conditions by upregulating genes involved in the thiamine system and stress response. The suicide enzyme, THI4 is responsible for mediating the production of the thiazole precursor of thiamin (cThz-P), and hence, it must be destroyed and resynthesized (Sun et al., 2019). Verticillium dahliae, a disease of vascular plants, is more resistant to stresses like UV damage and oxidative stress due to VdTHI4 (Hoppenau et al., 2014). TH1, a thiamine phosphate synthase, was upregulated in response to oxidative stress due to its involvement in the phosphorylation of HMP-P from plant thiamine thiazole to HMP-pyrophosphate (HMP-PP) (Strobbe et al., 2021). TPK is a crucial enzyme in the biosynthetic pathways of thiamine, and during environmental stresses, TPK activities were significantly increased in regulating thiamine metabolism in maize seedlings (Rapala-Kozik et al., 2008). During drought conditions, this research found that THI4 and TPK1 transcription levels were elevated in transgenic alfalfa, whereas TH1 levels were declined (Figure 6). RbcX2 can carboxylate Rubisco, which boosts photosynthesis and can also fold proteins to control the accumulation of soluble protein and drought resistance in plants (Liu et al., 2010; Doron et al., 2020). One form of SOD, Cu/Zn-SOD, is fundamental due to its role in ROS elimination and its correlation with drought tolerance in plants (Saed-Moucheshi et al., 2021; Zhang et al., 2021). Both transgenic and WT alfalfa showed an increase in RbcX2 transcription in response to drought stress, while WT alfalfa showed a marked increase in Cu/Zn-SOD transcription. Changes in the content of soluble protein and SOD activity in the drought tolerance experiment of transformants were in line with these findings. Serine/threonine protein kinases, of which CPKs are a subfamily, interact with THI1 to confer resistance to abiotic stressors, such as dehydration, salinity, and low temperature (Atif et al., 2019; Zhang et al., 2020; Chen et al., 2021). Variations in CPK13, CPK17, and CPK32 transcription were observed in the present study. These findings indicated that CPKs might have a role in regulating the response of MsTHI1 to drought stress; however, the mechanisms by which CPKs regulated MsTHI1 expression still require investigation.
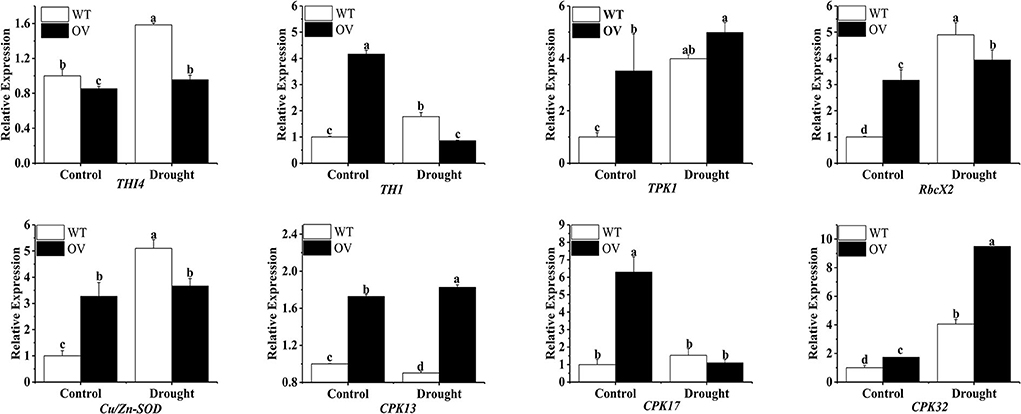
Figure 6. Expression of eight associated genes in transgenic alfalfa plants with overexpressing MsTHI1 under drought stress. WT, wild-type alfalfa. OV, overexpressing MsTHI1 alfalfa. THI4, thiazole biosynthetic enzyme THIAMIN4. TH1, HMP-P kinase/TMP pyrophosphorylase. TPK1, thiamine pyrophosphokinase. RbcX2, chaperonin-like RbcX protein 2. Cu/Zn-SOD, superoxide dismutase [Cu-Zn]. CPK13, CPK17, and CPK32: calcium-dependent protein kinases. Mean ± standard deviations from three biological replicates are shown with error bars. These letters denote statistically significant variations (α = 0.05).
Accordingly, we postulated that MsTHI1 participation in the plant drought tolerance might include a complicated regulating network that includes the physiological and gene expression levels (Figure 7). The overexpression of MsTHI1 can enhance the transcription of THI4, TPK1, RbcX2, Cu/Zn-SOD, CPK13, and CPK32, and decrease the transcription of TH1 and CPK17 to increase the accumulation of VB1, Chl a, Chl b, soluble protein, and POD activities, which helps preserve osmotic balance and strengthens photosynthesis and ROS scavenging.
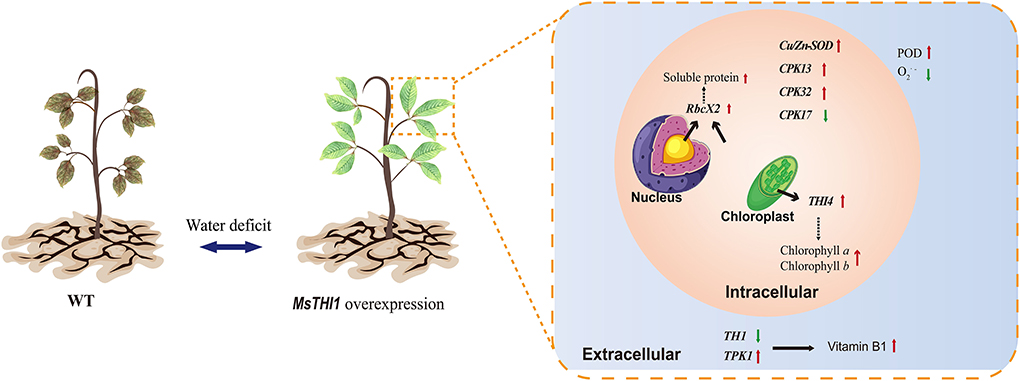
Figure 7. Possible function model of MsTHI1 response to drought stress. The red arrows represent significant increases in physiological signs or genes being overexpressed. Green arrows represent decreased expressions of genes or declines in physiological markers. Dotted lines represent potential regulating pathways. CPK13, CPK 17, and CPK32: calcium-dependent protein kinases. Cu/Zn-SOD, superoxide dismutase [Cu-Zn]. , superoxide anion radical. POD, peroxidase. RbcX2, chaperonin-like RbcX protein 2. TH1, HMP-P kinase/TMP pyrophosphorylase. THI4, thiazole biosynthetic enzyme THIAMIN4. TPK1, thiamine pyrophosphokinase. WT, wild type.
Conclusion
Overall, we could identify the thiamine thiazole synthase, MsTHI1, from alfalfa and determine its beneficial effect on the enhancement of drought stress tolerance. MsTHI1 localized to chloroplasts and alfalfa leaves exhibited excellent expression levels. Simultaneously, exposure to stressful conditions increased MsTHI1. The upregulation of MsTHI1 enhanced drought tolerance in plants and led to an enhancement in photosynthesis by increasing SPAD, maintaining Fv/Fm, and aggregating Chl a and Chl b, reducing the ROS accumulation and lipid peroxidation by elevating VB1 synthesis and POD activity, reducing MDA, and maintaining the balance of osmotic by strengthening the accumulation of soluble protein. Moreover, MsTHI1 mediated signal transduction by upregulating the transcription of THI4, TPK1, RbcX2, Cu/Zn-SOD, CPK13, and CPK32, and downregulating the transcription of TH1 and CPK17 in transgenic alfalfa under drought stress. Our findings shed light on the mechanism by which MsTHI1 alleviates plant drought stress and suggest that it should be incorporated into successful breeding initiatives to enhance the tolerance of alfalfa to drought.
Data availability statement
The datasets presented in this study can be found in online repositories. The names of the repository/repositories and accession number(s) can be found in the article/Supplementary material.
Author contributions
HY, GC, and PZ designed the experiment. HY experimented and wrote the manuscript. ZW, HL, YZ, and MY performed part of the experiment and analyzed and discussed data analysis. GC and PZ supervised the project and revised the manuscript. All authors agree to be accountable for the content of the work, contributed to the article and approved the submitted version.
Funding
This research was funded by the Heilongjiang Provincial Natural Science Foundation of China (YQ2021C019).
Acknowledgments
We would like to thank Prof. Hua Cai from the Northeast Agricultural University for kindly providing the pMDC123 vector and all reviewers for their helpful comments on the manuscript.
Conflict of interest
The authors declare that the research was conducted in the absence of any commercial or financial relationships that could be construed as a potential conflict of interest.
Publisher's note
All claims expressed in this article are solely those of the authors and do not necessarily represent those of their affiliated organizations, or those of the publisher, the editors and the reviewers. Any product that may be evaluated in this article, or claim that may be made by its manufacturer, is not guaranteed or endorsed by the publisher.
Supplementary material
The Supplementary Material for this article can be found online at: https://www.frontiersin.org/articles/10.3389/fpls.2022.992024/full#supplementary-material
Supplementary Figure S1. Alignment of homologous protein sequences. (A). phylogenetic tree generation (B), and three-dimensional modeling of MsTHI1 (C).
Supplementary Figure S2. Identification of transformation with MsTHI via PCR. (A). PCR identification of transgenic tobacco with Hyg. (B). PCR identification of transgenic alfalfa with Bar. M, Trans2K DNA Marker; “-”, negative control; “+”, positive control; WT, wild type; number, transformation line.
Supplementary Table S1. List of primers used in this study.
References
Abidin, A. A. Z., Yee, W. S., Rahman, N. S. A., Idris, Z. H. C., and Yusof, Z. N. B. (2016). Osmotic, oxidative and salinity stresses upregulate the expressions of Thiamine (vitamin B1) biosynthesis genes (THIC and THI1/THI4) in oil palm (Elaeis guineensis). J. Oil Palm Res. 28, 308–319. doi: 10.21894/jopr.2016.2803.07
Agathokleous, E., Feng, Z., and Penuelas, J. (2020). Chlorophyll hormesis: are chlorophylls major components of stress biology in higher plants? Sci. Total Environ. 726, 138637. doi: 10.1016/j.scitotenv.2020.138637
Amjad, S. F., Mansoora, N., Yaseen, S., Kamal, A., Butt, B., Matloob, H., et al. (2021). Combined use of endophytic bacteria and pre-sowing treatment of thiamine mitigates the adverse effects of drought stress in wheat (Triticum aestivum L.) Cultivars. Sustainability 13, 6582. doi: 10.3390/su13126582
Atif, R. M., Shahid, L., Waqas, M., Ali, B., Rashid, M. A. R., Azeem, F., et al. (2019). Insights on Calcium-Dependent Protein Kinases (CPKs) signaling for abiotic stress tolerance in plants. Int. J. Mol. Sci. 20, 5298. doi: 10.3390/ijms20215298
Bradford, M. M. (1976). A rapid and sensitive method for the quantitation of microgram quantities of protein utilizing the principle of protein-dye binding. Anal. Biochem. 72, 248–254. doi: 10.1016/0003-2697(76)90527-3
Chabregas, M. S., Luche, D. D., Van Sluys M-A., Menck, C. F. M., and Silva-Filho M. C. (2003). Differential usage of two in-frame translational start codons regulates subcellular localization of Arabidopsis thaliana THI1. J. Cell Sci. 116, 285–291. doi: 10.1242/jcs.00228
Chen, D. Q., Wang, S. W., Cao, B. B., Cao, D., Leng, G. H., Li, H. B., et al. (2016). Genotypic variation in growth and physiological response to drought stress and re-watering reveals the critical role of recovery in drought adaptation in maize seedlings. Front. Plant Sci. 6, 1241. doi: 10.3389/fpls.2015.01241
Chen, P. F., Liu, P., Zhang, Q. F., Zhao, L., Hao, X. R., Liu, L., et al. (2022). Dynamic physiological and transcriptome changes reveal a potential relationship between the circadian clock and salt stress response in Ulmus pumila. Mol. Genet. Genom. 297, 303–317. doi: 10.1007/s00438-021-01838-2
Chen, X., Ding, Y., Yang, Y., Song, C., Wang, B., Yang, S., et al. (2021). Protein kinases in plant responses to drought, salt, and cold stress. J. Integr. Plant Biol. 63, 53–78. doi: 10.1111/jipb.13061
Daszkowska-Golec, A., Collin, A., Sitko, K., Janiak, A., Kalaji, H. M., and Szarejko, I. (2019). Genetic and physiological dissection of photosynthesis in barley exposed to drought stress. Int. J. Mol. Sci. 20, 6341. doi: 10.3390/ijms20246341
Dong, W, Stockwell, V. O, and Goyer, A. (2015). Enhancement of Thiamin content in arabidopsis thaliana by metabolic engineering. Plant Cell Physiol. 56, 2285–2296. doi: 10.1093/pcp/pcv148
Doron, L., Xu, L., Rachmilevitch, S., and Stern, D. B. (2020). Transgenic overexpression of rubisco subunits and the assembly factor RAF1 are beneficial to recovery from drought stress in maize. Environ. Exp. Botany 177, 104126. doi: 10.1016/j.envexpbot.2020.104126
Fan, Q., Creamer, R., and Li, Y. (2018). Time-course metabolic profiling in alfalfa leaves under Phoma medicaginis infection. PloS ONE 13, e0206641. doi: 10.1371/journal.pone.0206641
Giannopolitis, C. N., and Ries, S. K. (1972). Superoxide dismutase I. Occurrence in higher plants. Plant Physiol. 59, 309–314. doi: 10.1104/pp.59.2.309
Godoi, P. H. C., Galhardo, R. S., Luche, D. D., Van Sluys, M.-A., Menck, C. F. M., and Oliva, G. (2006). Structure of the thiazole biosynthetic enzyme THI1 from Arabidopsis thaliana. J. Biol. Chem. 281, 30957–30966. doi: 10.1074/jbc.M604469200
He, L., Liu, Y., He, H., Liu, Y., Qi, J., Zhang, X., et al. (2020). A molecular framework underlying the compound leaf pattern of Medicago truncatula. Nature Plants 6, 511–521. doi: 10.1038/s41477-020-0642-2
Hoppenau, C. E., Tran, V. T., Kusch, H., Asshauer, K. P., Landesfeind, M., Meinicke, P., et al. (2014). Verticillium dahliae VdTHI4, involved in thiazole biosynthesis, stress response and DNA repair functions, is required for vascular disease induction in tomato. Environ. Exp. Botany 108, 14–22. doi: 10.1016/j.envexpbot.2013.12.015
Hosseinifard, M., Stefaniak, S., Ghorbani Javid, M., Soltani, E., Wojtyla, Ł., and Garnczarska, M. (2022). Contribution of exogenous proline to abiotic stresses tolerance in plants: a review. Int. J. Mol. Sci. 23, 5186. doi: 10.3390/ijms23095186
Hussain, H. A., Hussain, S., Khaliq, A., Ashraf, U., Anjum, S. A., Men, S., et al. (2018). Chilling and drought stresses in crop plants: implications, cross talk, and potential management opportunities. Front. Plant Sci. 9, 393. doi: 10.3389/fpls.2018.00393
Jiang, M., and Zhang, J. (2001). Effect of abscisic acid on active oxygen species, antioxidative defence system and oxidative damage in leaves of maize seedlings. Plant Cell Physiol. 42, 1265–1273. doi: 10.1093/pcp/pce162
Kaur, M., Manchanda, P., Kalia, A., Ahmed, F. K., Nepovimova, E., Kuca, K., et al. (2021). Agroinfiltration Mediated Scalable Transient Gene Expression in Genome Edited Crop Plants. Int. J. Mol. Sci. 22, 10882. doi: 10.3390/ijms221910882
Li, C. L., Wang, M., Wu, X. M., Chen, D. H., Lv, H. J., Shen, J. L., et al. (2016). THI1, a Thiamine Thiazole Synthase, Interacts with Ca2+-Dependent Protein Kinase CPK33 and modulates the S-type anion channels and stomatal closure in Arabidopsis. Plant Physiol. 170, 1090–1104. doi: 10.1104/pp.15.01649
Li, P., Liu, H., Yang, H., Pu, X., Li, C., Huo, H., et al. (2020). Translocation of drought-responsive proteins from the chloroplasts. Cells 9, 259. doi: 10.3390/cells9010259
Liu, C., Young, A. L., Starling-Windhof, A., Bracher, A., Saschenbrecker, S., Rao, B. V., et al. (2010). Coupled chaperone action in folding and assembly of hexadecameric Rubisco. Nature 463, 197–U181. doi: 10.1038/nature08651
Livak, K., and Schmittgen, T. (2000). Analysis of relative gene expression data using real-time quantitative PCR and the 2−ΔΔCt method. Methods 25, 402–408. doi: 10.1006/meth.2001.1262
Mangel, N., Fudge, J. B., Fitzpatrick, T. B., Gruissem, W., and Vanderschuren, H. (2017). Vitamin B-1 diversity and characterization of biosynthesis genes in cassava. J. Exp. Botany 68, 3351–3363. doi: 10.1093/jxb/erx196
Meng, D. L., Yu, X. H., Ma, L. Y., Hu, J., Liang, Y. L., Liu, X. D., et al. (2017). Transcriptomic response of Chinese Yew (Taxus chinensis) to cold stress. Front. Plant Sci. 8, 468. doi: 10.3389/fpls.2017.00468
Nagae, M., Parniske, M., Kawaguchi, M., and Takeda, N. (2016). The thiamine biosynthesis gene THI1 promotes nodule growth and seed maturation. Plant Physiol. 172, 2033–2043. doi: 10.1104/pp.16.01254
Polle, A., Otter, T., and Seifert, F. (1994). Apoplastic Peroxidases and Lignification in Needles of Norway Spruce (Picea abies L.). Plant Physiol. 106, 53–60. doi: 10.1104/pp.106.1.53
Puckette, M. C., Hua, W., and Mahalingam, R. (2007). Physiological and biochemical responses to acute ozone-induced oxidative stress in Medicago truncatula. Plant Physiol. Biochem. 45, 70–79. doi: 10.1016/j.plaphy.2006.12.004
Qian, R. J., Hu, Q. D., Ma, X. H., Zhang, X. L., Ye, Y. J., Liu, H. J., et al. (2022). Comparative transcriptome analysis of heat stress responses of Clematis lanuginosa and Clematis crassifolia. BMC Plant Biol. 22, 138. doi: 10.1186/s12870-022-03497-w
Raina, S. K., Rane, J., Raskar, N., Singh, A. K., Govindasamy, V., Kumar, M., et al. (2019). Physiological traits reveal potential for identification of drought tolerant mungbean Vigna radiata (L.) Wilczek genotypes under moderate soil-moisture deficit. Indian J. Genet. Plant Breed. 79, 427–437. doi: 10.31742/IJGPB.79.2.6
Rao, S., Tian, Y., Xia, X., Li, Y., and Chen, J. (2020). Chromosome doubling mediates superior drought tolerance in Lycium ruthenicum via abscisic acid signaling. Hortic. Res. 7, 40. doi: 10.1038/s41438-020-0260-1
Rapala-Kozik, M., Kowalska, E., and Ostrowska, K. (2008). Modulation of thiamine metabolism in Zea mays seedlings under conditions of abiotic stress. J. Exp. Botany 59, 4133–4143. doi: 10.1093/jxb/ern253
Rapala-Kozik, M., Wolak, N., Kujda, M., and Banas, A. K. (2012). The upregulation of thiamine (vitamin B-1) biosynthesis in Arabidopsis thaliana seedlings under salt and osmotic stress conditions is mediated by abscisic acid at the early stages of this stress response. BMC Plant Biology 12, 2. doi: 10.1186/1471-2229-12-2
Rivero, R. M., Mittler, R., Blumwald, E., and Zandalinas, S. I. (2022). Developing climate-resilient crops: improving plant tolerance to stress combination. Plant J. 109, 373–389. doi: 10.1111/tpj.15483
Roo, L. D., Salomón, R. L., and Steppe, K. (2020). Woody tissue photosynthesis reduces stem CO2 efflux by half and remains unaffected by drought stress in young Populus tremula trees. Plant Cell Environ. 43, 981–991. doi: 10.1111/pce.13711
Saed-Moucheshi, A., Sohrabi, F., Fasihfar, E., Baniasadi, F., Riasat, M., and Mozafari, A. A. (2021). Superoxide dismutase (SOD) as a selection criterion for triticale grain yield under drought stress: a comprehensive study on genomics and expression profiling, bioinformatics, heritability, and phenotypic variability. BMC Plant Biol. 21, 148. doi: 10.1186/s12870-021-02919-5
Scafaro, A. P., Haynes, P. A., and Atwell, B. J. (2010). Physiological and molecular changes in Oryza meridionalis Ng., a heat-tolerant species of wild rice. J. Exp. Botany 61, 191–202. doi: 10.1093/jxb/erp294
Sharma, P., Jha, A. B., Dubey, R. S., and Pessarakli, M. (2012). Reactive oxygen species, oxidative damage, and antioxidative defense mechanism in plants under stressful conditions. J. Botany 2012, 217037. doi: 10.1155/2012/217037
Strobbe, S., Verstraete, J., Fitzpatrick, T. B., Faustino, M., Lourenco, T. F., Oliveira, M. M., et al. (2022). A novel panel of yeast assays for the assessment of thiamin and its biosynthetic intermediates in plant tissues. New Phytologist 234, 748–763. doi: 10.1111/nph.17974
Strobbe, S., Verstraete, J., Stove, C., and Van Der Straeten, D. (2021). Metabolic engineering provides insight into the regulation of thiamin biosynthesis in plants. Plant Physiol. 186, 1832–1847. doi: 10.1093/plphys/kiab198
Sun, J., Sigler, C. L., Beaudoin, G. A. W., Joshi, J., Patterson, J. A., Cho, K. H., et al. (2019). Parts-prospecting for a high-efficiency thiamin thiazole biosynthesis pathway. Plant Physiol. 179, 958–968. doi: 10.1104/pp.18.01085
Sun, N., Song, T., Ma, Z., Dong, L., Zhan, L., Xing, Y., et al. (2020). Overexpression of MsSiR enhances alkali tolerance in alfalfa (Medicago sativa L.) by increasing the glutathione content. Plant Physiol. Biochem. 154, 538–546. doi: 10.1016/j.plaphy.2020.07.001
Teixeira, R. D., Paiva, F. L., Dantas, d,.A. J., Mayumi, K. P., Ribeiro, A.F.C. C., et al. (2005). Functional characterization of the thi1 promoter region from Arabidopsis thaliana. J. Exp. Botany 56, 1797–1804. doi: 10.1093/jxb/eri168
Tietze, F. (1969). Enzymic method for quantitative determination of nanogram amounts of total and oxidized glutathione: applications to mammalian blood and other tissues. Anal. Biochem. 27, 502–522. doi: 10.1016/0003-2697(69)90064-5
Wellburn, A. R., and Lichtenthaler, H. K. (1984). Formulae and Program to Determine Total Carotenoids and Chlorophylls A and B of Leaf Extracts in Different Solvents. Springer Netherlands.
Xu, Z., and Zhou, G. (2008). Responses of leaf stomatal density to water status and its relationship with photosynthesis in a grass. J. Exp. Botany 59, 3317–3325. doi: 10.1093/jxb/ern185
Yan, C., Song, S., Wang, W., Wang, C., Li, H., Wang, F., et al. (2020). Screening diverse soybean genotypes for drought tolerance by membership function value based on multiple traits and drought-tolerant coefficient of yield. BMC Plant Biol. 20, 321–321. doi: 10.1186/s12870-020-02519-9
Yang, M., Duan, X., Wang, Z., Yin, H., Zang, J., Zhu, K., et al. (2021). Overexpression of a Voltage-Dependent Anion-Selective Channel (VDAC) protein-encoding gene, MsVDAC, from Medicago sativa confers cold and drought tolerance to transgenic tobacco. Genes 12, 1706. doi: 10.3390/genes12111706
Yang, Z., Chi, X., Guo, F., Jin, X., Luo, H., Hawar, A., et al. (2020). SbWRKY30 enhances the drought tolerance of plants and regulates a drought stress-responsive gene, SbRD19, in sorghum. J. Plant Physiol. 246, 153142. doi: 10.1016/j.jplph.2020.153142
Yu, J. G., Lee, G. H., Lee, S. C., and Park, Y. D. (2014). Gene expression and phenotypic analyses of transgenic Chinese cabbage over-expressing the cold tolerance gene, BrCSR. Hortic. Environ. Biotechnol. 55, 415–422. doi: 10.1007/s13580-014-0054-1
Zhang, C., and Shi, S. (2018). Physiological and proteomic responses of contrasting Alfalfa (Medicago sativa L.) varieties to PEG-induced osmotic stress. Front. Plant Sci. 9, 242. doi: 10.3389/fpls.2018.00242
Zhang, H., Liu, D., Yang, B., Liu, W.-Z., Mu, B., Song, H., et al. (2020). Arabidopsis CPK6 positively regulates ABA signaling and drought tolerance through phosphorylating ABA-responsive element-binding factors. J. Exp. Botany 71, 188–203. doi: 10.1093/jxb/erz432
Zhang, X., Zhang, L., Chen, Y., Wang, S., Fang, Y., Zhang, X., et al. (2021). Genome-wide identification of the SOD gene family and expression analysis under drought and salt stress in barley. Plant Growth Regul. 94, 49–60. doi: 10.1007/s10725-021-00695-8
Zhao, L., Liu, F., Xu, W., Di, C., Zhou, S., Xue, Y., et al. (2009). Increased expression of OsSPX1 enhances cold/subfreezing tolerance in tobacco and Arabidopsis thaliana. Plant Biotechnol. J. 7, 550–561. doi: 10.1111/j.1467-7652.2009.00423.x
Zhao, Y., Ma, W., Wei, X., Long, Y., Zhao, Y., Su, M., et al. (2019). Identification of exogenous nitric oxide-responsive miRNAs from Alfalfa (Medicago sativa L.) under drought stress by high-throughput sequencing. Genes 11, 30. doi: 10.3390/genes11010030
Keywords: alfalfa, MsTHI1, photosynthesis, antioxidant defense, osmotic homeostasis, drought tolerance
Citation: Yin H, Wang Z, Li H, Zhang Y, Yang M, Cui G and Zhang P (2022) MsTHI1 overexpression improves drought tolerance in transgenic alfalfa (Medicago sativa L.). Front. Plant Sci. 13:992024. doi: 10.3389/fpls.2022.992024
Received: 12 July 2022; Accepted: 08 August 2022;
Published: 08 September 2022.
Edited by:
Linkai Huang, Sichuan Agricultural University, ChinaReviewed by:
Guohui Yu, Nanjing Agricultural University, ChinaZhiqiang Zhang, Inner Mongolia Agricultural University, China
Copyright © 2022 Yin, Wang, Li, Zhang, Yang, Cui and Zhang. This is an open-access article distributed under the terms of the Creative Commons Attribution License (CC BY). The use, distribution or reproduction in other forums is permitted, provided the original author(s) and the copyright owner(s) are credited and that the original publication in this journal is cited, in accordance with accepted academic practice. No use, distribution or reproduction is permitted which does not comply with these terms.
*Correspondence: Pan Zhang, emhhbmdwYW4mI3gwMDA0MDtuZWF1LmVkdS5jbg==; Guowen Cui, Y2d3NjAzJiN4MDAwNDA7MTYzLmNvbQ==