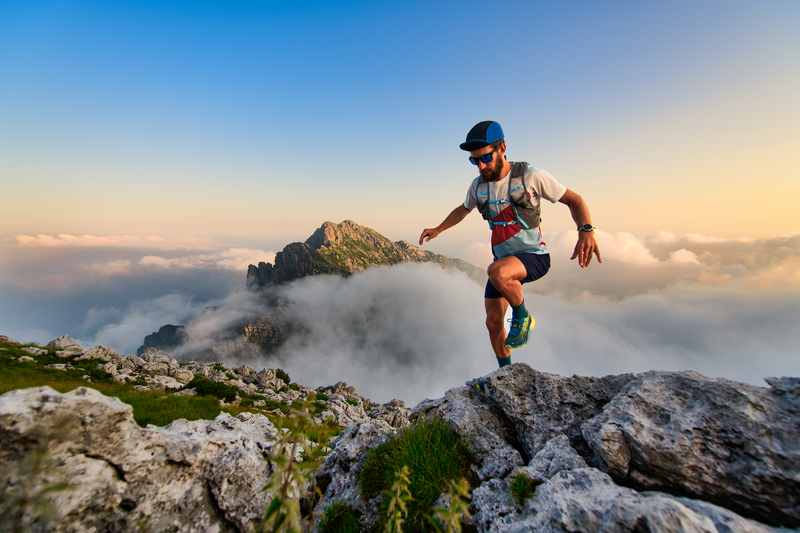
94% of researchers rate our articles as excellent or good
Learn more about the work of our research integrity team to safeguard the quality of each article we publish.
Find out more
ORIGINAL RESEARCH article
Front. Plant Sci. , 28 November 2022
Sec. Plant Physiology
Volume 13 - 2022 | https://doi.org/10.3389/fpls.2022.986688
This article is part of the Research Topic Advances in Plant Hormone Research in the Face of a Changing Environment View all 6 articles
Silicon is a beneficial element for plant growth, as well as for improving plant resistance to multiple biotic and abiotic stresses. Gummosis is a common harmful disease in peach and is induced by many factors. However, the effect of silicon on gummosis of peach has not been determined yet. In this study, we reported that application of silicon significantly reduced gummosis by regulating biosynthesis of ethylene and polyamines in peach. Ethylene promoted the development of gummosis by inducing the expression of genes encoding cell wall degrading enzymes. While application of different types of polyamines, including spermidine and spermine, dramatically inhibited the occurrence of gummosis. Moreover, polyamines inhibited the ethylene biosynthesis by down-regulating expression of ethylene biosynthetic gene PpACS1 (1-aminocyclopropane -1-carboxylic acid synthase), as well as the enzymatic activity of ACS. We further found that application of silicon significantly restricted the development of gummosis in peach. Exogenous silicon dramatically inhibited expression of PpACS1 and the enzymatic activity of its product to reduce ethylene biosynthesis. Simultaneously, the activity of S-adenosylmethionine decarboxylase, a key enzyme in ployamines biosynthesis, was increased by 9.85% under silicon treatment, resulting in elevated accumulation of polyamines. Thus, our data proved that application of silicon restricted gummosis development by activating ployamines biosynthesis and inhibiting ethylene synthesis in peach.
Gummosis is a harmful disease that can be induced by a variety of factors. Gummosis is a broad defence response to abiotic and biotic stresses, accompanied by a series of complex physiological and biochemical reactions (Li et al., 2015; Zhang et al., 2022). The disease is common in cultivation of peach, cherry, plum, and rose (Saniewski et al., 1998; Saniewski et al., 2001; Adesemoye et al., 2014), and has been reported in many countries, including China, the United States, Japan, and so on (Wang et al., 2011; Adesemoye et al., 2014; Ezra et al., 2017). Excessive gum formation seriously weakens tree vigour, destroys the branches and fruits, resulting in decreased yield and quality of fruit. Gummosis involves a process of accumulation and penetration of polysaccharides, and the formation of gum is due to the degradation of cell wall. The gum comes from polysaccharides in the cell wall and is produced in the cambium (Simas-Tosin et al., 2010; Vincent et al., 2010; Li et al., 2014a). The resulting changes in cell wall structure are mainly due to the degradation of pectin and cellulose and changes in other cell wall components. Gum is formed through the hydrolysis of cell wall substances, which is closely related to the activities of hydrolases such as polygalacturonase (PG), pectin-methylesterase (PME) and β-galactosidese (β-Gal) (Vorwerk et al., 2004).
There are many factors that cause gummosis, which mainly include pathogen infection, stress, freezing damage, pest damage, and mechanical wounds (Li et al., 2014a). In addition, plant hormones such as ethylene and jasmonic acid are important factors which exacerbate cell senescence and lysis and accelerate gum production (Miyamoto et al., 2010; Li et al., 2014b; Li et al., 2015). Studies have shown that ethylene promotes the production of gum in plant under stress conditions. Exogenous ethephon induces gum formation in cherry (Miyamoto et al., 2019), hyacinth (Miyamoto et al., 2015), and peach (Li et al., 2015). In addition, concurrent application of ethephon and methyl-jasmonate enhances gummosis in peach (Li et al., 2015), cherry (Miyamoto et al., 2019) and Prunus mume (Carolina and Kusumoto, 2020). Ethylene is widely present in various organs of plants, and its synthesis is a methionine cycle with two key enzymes, ACC (1-aminocyclopropane-1-carboxylic acid) synthase (ACS) and ACC oxidase (ACO). ACS catalyses the synthesis of ACC from S-adenosylmethionine (SAM), and ACO catalyses the synthesis of ethylene from ACC. ACS and ACO are encoded by a multigene family, and transcription is regulated by multiple factors (Ye et al., 2017; Yuan et al., 2019).
Polyamines are essential aliphatic compounds that have multiple functions in plant growth and development and as wll as in cell homeostasis (Bregoli et al., 2006; Leonardo et al., 2010). They are involved in protective stress responses, and the level of polyamine biosynthesis is significantly increased under stress conditions (Franchin et al., 2007). SAM is catalytically decarboxylated by SAM decarboxylase (SAMDC). In addition to serving as a methyl donor, decarboxylated SAM is also a common precursor for the synthesis of polyamines and ethylene, so SAMDC is considered being a regulator of two synthetic pathways. Silicon was shown to enhance the expression of the SAMDC gene, which encodes a key enzyme in the biosynthesis of polyamines, thereby promoting polyamine synthesis and inhibiting ethylene synthesis (Yin et al., 2016). Ethylene and polyamines are synthesized in a competitive relationship, and polyamines share a common substrate, SAM, with ethylene and produce a common product, 5’-methylthioadenosine (Pandey et al., 2000). They have opposite functions, ethylene as a pro-senescence, pro-ripening regulator while polyamines as pro-growth, anti-senescence regulators, have drawn more attention to them (Harpaz-Saad et al., 2012).
Silicon is beneficial to plant growth, especially under stress conditions (Ma and Yamaji, 2006; Safoora et al., 2018). It has been shown to alleviate various abiotic stresses, including heavy metal, salinity, drought, etc. (Gong and Chen, 2012; Bharwana et al., 2013) and biotic stresses, including as pests and diseases (Rodrigues et al., 2004; Liang et al., 2010; Shirani and Zheng, 2018). Moreover, application of silicon improves plant disease resistance. Studies have found that silicon improved plant resistance to rice blast, bacterial blight, and cucumber and melon powdery mildew (Guo et al., 2005; Yu et al., 2009; Wen, 2016). Silicon also activates the plant inducible defence system through physiological and biochemical defence mechanisms to enhance resistance to diseases (Ning and Liang, 2014). Silicon was reported to increase the levels of polyamines, such as free and bound putrescine (Put), by promoting the polyamines biosynthetic genes in sorghum tissues under salt stress (Yin et al., 2019). The study also found that silicon induced the expression of genes related to plant defence and interacted with disease resistance signalling molecules (such as salicylic acid, jasmonic acid, and ethylene) in signal transduction (Chain et al., 2010). Specifically, silicon induces the expression of genes that are involved in cell wall structural modification, allergic response, synthesis of hormones and antibacterial complexes, and pathogenesis-related proteins (Bélanger et al., 2003; Fauteux et al., 2006).
Silicon is beneficial for improving plant disease resistance, but there is no research on whether it can enhance peach gummosis resistance. And it is not clear whether silicon can inhibit the occurrence of peach gummosis by regulating the synthesis of ethylene and polyamines. In this study, we found that silicon inhibited peach gummosis. To better understand the mechanism of gummosis inhibited by silicon in peach, we investigated the factors that influence gummosis caused by ethylene and analyzed the expression patterns of related genes during progression of gummosis inhibited by silicon in peach. We found that application of silicon restricted gummosis development by activating ployamines biosynthesis and inhibiting ethylene synthesis in peach.
Throughout the experiments, shoots were collected from 3-year-old Prunus persica (L.) ‘Spring snow’ plants. The plants were grafted onto wild peach rootstocks and grown in the experimental field of Shandong Agricultural University in Taian. The shoots were cut into 12.0-cm-long segments and surface-sterilized with 75% alcohol for 10 s, followed by rinsing three times with sterile water. The shoot segments were wounded at intervals of 0.5 cm on the midpoint using a sterilized needle. Treatments with silicon, ethephon and various reagents were performed as follows: Na2SiO3 (0.6 mmol/L), ethephon (1.0%, w/w), Put(0.1 mmol/L), Spermidine (Spd) (0.1 mmol/L), Spermine (Spm) (0.1 mmol/L), D-Arginine (D-Arg) (10.0 mg/L), ACC (50 mg/L), Aviglycine (AVG) (0.5 mmol/L) and Methylglyoxal bis-guanyl hydrazine (MGBG) (0.5 mmol/L) were applied individually or together on selected peach shoots; as a control, water was sprayed on the shoots. The shoot segments were separately placed in an upright position in 2000 mL plastic bottles that contained 200 mL of sterilized water, and the bottles were covered with transparent plastic film to maintain humidity. The water in the bottles was refreshed daily, and the isolated shoots were cultivated in an incubator with a humidity of > 70% at 35°C and a photoperiod of 12 hours of light (20,000 lux). Each treatment was made up of 30 peach segments, and six independent segments were obtained in the same way from the treated and control groups for sample collection. The treatment was continued for 5 days. Gum formation was described according to Saniewski et al. (1998). Samples were taken at 0 h, 6 h, 1 day, 2 days and 4 days after treatment, and the tissue within 0.5-1.0 cm from the wound point was collected from the segments. The samples were promptly frozen in liquid nitrogen and then kept at a temperature of -80°C for later use.
The test material was 3-year-old Prunus persica (L.) ‘Spring snow’ plants. The current year’s shoots of uniform growth were selected, and samples were taken on the third day after treatment with ethephon (1.0%, w/w). The control was treated with clean water.
The experimental process of sequencing was carried out by HuaDa Gene (http://www.genomics.cn/index). For this work, the Illumina Gene Expression Sample Prep Kit and Solexa Sequencing Chip (flow cell) were used, and the main instruments were an Illumina Cluster Station and Illumina HiSeq TM 2000 System.
Six peach shoots subjected to different treatments were selected and cultivated in a closed beaker for 3 h. One millilitre of gas from the beaker’s headspace was sampled after incubation. A gas chromatograph (GC-2014C, Shimadzu, Japan) equipped with a GDX-502 column and a flame ionization detector was used to measure ETH production (FID). The injection temperature was 120°C, and the column temperature was 70°C. N2was used as the carrier gas at a rate of 40 mL min-1.
Two millilitres of 85% ethanol was used to extract 0.5 g (fresh weight) of plant material. Following drying of the extracted supernatant, 1.0 mL of chloroform and 1.0 mL of distilled water were added separately. Then, HgCl2 and NaOCl/saturated NaOH (2:1) were added to the aqueous phase. After incubation and intermittent agitation, 1 mL of the air in the headspace was extracted with a gas syringe and injected into a gas chromatograph (GC-2014C, Shimadzu, Japan). The aqueous phase was hydrolysed in 6.0 M HCl at 100°C for 3 h to quantify malonyl-ACC (MACC, conjugated ACC).
High-performance liquid chromatography was used to examine the polyamine content (HPLC: LC-1290, Agilent, America). Plant materials (1.0 g) were pulverized and homogenized in 5.0 mL of 5.0% HClO4 and extracted overnight at room temperature on a shaker. The collected supernatant was used to determine the amount of free polyamines after centrifugation. To measure the bound polyamines (conjugated polyamines and macromolecules), the residue of the plant extract was washed with 5.0% HClO4 and then hydrolysed in 6.0 M HCl at 110°C for 15 h. The filtered hydrolysate was evaporated to dryness, and the residue was dissolved in 5% HClO4 for quantification of the bound polyamines.
An enzyme-linked immunosorbent assay kit (Meibiao Biology, Jiangsu Meibiao Biology Technology Company Limited) was used to detect the enzymatic activity of ACS, ACO, PG, PME, β-Gal and SAMDC. Plant samples (0.5 g) were ground in liquid nitrogen, 5.0 mL of PBS (pH 7.4) was added, and the samples were fully homogenized by a homogenizer. The samples were centrifuged at 3000 rpm for approximately 20 minutes, and the supernatant was collected. Samples were added according to the instructions and detected with a microplate reader, and then the enzyme activity was calculated according to the standard curve.
According to the manufacturer’s instructions, an RNAprep Pure Plant Kit (Tiangen, Beijing, China) was used to isolate total RNA from 0.5 g of sample. The PrimeScriptTM RT Kit (Takara, Japan) was used to generate first-strand cDNA according to the manufacturer’s instructions. On a QuantStudio® 3 real-time PCR instrument (Thermo, USA), we performed three biological and three technical duplicate RT-qPCRs using SYBR® Premix Ex TaqTM (Takara, Japan), with PpActin as the reference gene, according to the manufacturer’s recommendations for all reagents. The 2−ΔΔCT approach was used to calculate the relative expression levels. (Primers are shown in Table 1)
The biological and biochemical data are presented as the means ± standard errors (SEs). The significance of the differences between samples was assessed by Duncan’s multiple range tests using IBM SPSS Statistics version 20.0.
We found that treatment with needle wounding induced the gum production on peach shoots, while no gum was found in control treatment (Figure S1A). However, co-treatment with wounding and 1% ethephon significantly promoted the production of gum compared with that in wounding treatment (Figures S1B, C). These data suggested that ethylene was a promoting factor for gummosis in peach.
To determine the mechanism of how ethylene facilitated gummosis on peach shoots, we constructed a digital gene expression profile library from ethephon-treated and untreated peach shoots. Data analysis showed that many genes were induced or inhibited by more than 2-fold in the bark tissue of peach shoots 3 d after ethephon treatment (Figure S2A). There were 2.13% of tags in ethephon-treated samples having signatures upregulated by more than 5-fold, and 2.74% in CK when the variation in signatures of differentially expressed genes between ethephon and CK was within 6-fold (Figure S2B). According to the screening criteria for differential gene expression, 1362 genes were upregulated and 3005 genes were downregulated under the ethephon treatment (Figure S2C). Among the differentially expressed genes, three kinds of key enzymes that were related to cell wall degradation were identified. Among them, two polygalacturonase genes (ppa007271m, ppa008474m) and three pectinesterase genes (ppa003578m, ppa003852m, ppa003639m) were significantly induced by ethephon. Especially for ppa003578m (pectinesterase) and ppa007271m (polygalacturonase), which were upregulated by 12.176- and 6.620-fold, respectively (Table 2).
As shown in Figure 1A, following silicon treatment, the gum on the peach shoots was significantly smaller than that of the control, while a large amount of gum was produced at the wound of the shoots treated with exogenous ethephon. The gum formation ratio under different treatments showed that the gum appeared earlier and accumulated more in ethephon-treated shoots. The gum formation ratio was 24.44% higher than that of control after 5 d. In contrast, silicon inhibited the production of gum on shoots, the gum formation ratio was reduced by 30.30% compared with control after 4 d (Figures 1B, C). Importantly, a similar result was observed for ethylene synthesis under different treatments (Figure 1D). Silicon inhibited ethylene synthesis in shoots, and the ethylene synthesis rate was reduced by 71.66% compared with ethylene-treated shoots after 24 h of treatment. In electron microscopy analysis, we observed that silicon treatment alleviated the cell damage compared with that of control. Specifically, under silicon treatment, the structure of cell walls and cellular organelles were more intact (Figure 1E).
Figure 1 Silicon inhibits gum formation in peach shoots. (A) Photograph of gum formation in peach shoots treated with silicon (0.6 mmol/L) or ethephon (1%, w/w) after 5 days. (B) Gum formation score of shoots treated with silicon or ethephon after 5 days. The relative levels of gum production were graded based on visual observation, and the scores were as follows: − no gum; + to ++++, increasing degrees (amounts) of gum production (+, trace; ++++, high). (C) Gum formation rates of peach shoots treated with silicon or ethephon. (D) Ethylene synthesis rate of shoots treated with silicon or ethephon. (E) Electron microscopy image of the cell structure after treatment with water and silicon for 5 days (the picture on the right is the marked position of the picture on the left). Data represent the means of three replicates ± SEs, and different letters indicate significant differences (P < 0.05).
Transcriptome analysis showed that cell wall-degrading enzymes were closely related to peach gummosis. We thus used an enzyme-linked immunosorbent assay kit to analyse the enzyme activity of PG, PME and β-Gal and screened the cell wall-degrading enzyme synthesis genes ppa007271m (PG), ppa003578m (PME) and ppa001382m (β-Gal), which are significantly regulated by ethylene, for RT–qPCR analysis. The results showed that enzymatic activity of these three enzymes, while silicon founctioned adversely. In particular, the PG and PME enzymatic activity were significantly inhibited by silicon (Figures 2A–C). 24 h after treatment, the activity of PG and PME under silicon treatment decreased by 11.61% and 10.16% compared with the control, respectively. The expression of ppa007271m, ppa003578m and ppa001382m in peach shoots were significantly upregulated with the increasing degree of gum formation (Figures 2D–F). The expression levels of ppa007271m and ppa003578m were significantly upregulated by ethephon, while they were all downregulated by silicon, especially after 24 h of treatment.
Figure 2 Silicon inhibits cell wall-degrading enzyme activity in peach shoots. Enzyme activity of PG (A), PME (B) and β-Gal (C) in peach shoots. Relative expression of ppa007271m (D), ppa003578m (E) and ppa001382m (F) in peach shoots. The peach shoots were treated with water, Na2SiO3 (0.6 mmol/L) or ethephon (1%, w/w), and samples were taken at different times to determine the enzyme activity and relative gene expression. Bars with different letters indicate significant differences (p < 0.05, Duncan’s multiple range tests).
Given that ethylene synthesis is closely involved in the inhibitory effect of silicon on the gummosis of peach shoots, we next determined the effect of silicon on ethylene biosynthesis. Under silicon treatment, the enzymatic activity of ethylene synthase ACS (Figure 3A) and the relative expression levels of PpACS1 (Figure 3C) and PpACS2 (Figure 3D) were all decreased. Compared with the control, they decreased by 7.40%, 39.94% and 19.63% 24 hours after treatment. However, silicon had no effect on the enzymatic activity and gene expression level of ACO (Figures 3B, E). Silicon treatment reduced the content of total ACC and free ACC in shoots by 21.72% and 41.67% compared to the control after 24 hours treatment, which was contrary to the results of exogenous ethephon treatment (Figures 3F, G). After exogenous ACC treatment, ethylene synthesis was promoted in shoots and that the inhibitory effect of silicon on ethylene synthesis was alleviated by ACC (Figure 3H). These results indicated that the effect of silicon on ethylene synthesis in peach shoots was achieved through the ACC pathway.
Figure 3 Silicon inhibits ethylene synthesis in peach shoots. Enzyme activity of ACS (A) and ACO (B) in peach shoots under different treatments. Relative expression of PpACS1 (C), PpACS2 (D) and PpACO (E) in peach shoots under different treatments. Total ACC content (F) and free ACC content (G) of peach shoots treated with different fluids. (H) Ethylene synthesis rate of peach shoots treated with exogenous ACC. Bars with different letters indicate significant differences (p < 0.05, Duncan’s multiple range tests).
In order to investigate the effect of polyamines on ethylene synthesis, Put, Spd, Spm and D-Arg (Putrescine synthesis inhibitor) were used to treat peach shoots. As shown in Figure 4A, silicon, Spd and Spm inhibited gum formation, as well as the ethylene biosynthesis on peach shoots. After 24 h treatment, compared with the control, the ethylene synthesis rate was reduced by 41.90%, 50.60% and 61.09%, respectively. However, Put had no inhibitory effect on ethylene synthesis in peach shoots, and accelerated the production of gum on shoots (Figure 4B). The total ACC and free ACC levels, as well as the ACS activity, were reduced under treatment of silicon, Spd and Spm after 24 hours-after-treatment (Figures 4C, D and S3A). The expression of PpACS1 was downregulated by silicon, Spd and Spm throughout the whole process. The expression of PpACS1 under treatment of silicon, Spd, and Spm was reduced by 59.10%, 64.76% and 52.43% compared with the control, respectively, after 24 h treatment (Figure S3B). While the expression of PpACS2 was downregulated by silicon, but not Spm and Spd (Figure S3C).
Figure 4 Spd and Spm inhibit ethylene synthesis in peach shoots. (A) Photograph of gum formation in peach shoots treated with water, Na2SiO3 (0.6 mmol/L), Put (0.1 mmol/L), Spd (0.1 mmol/L), Spm (0.1 mmol/L) or Na2SiO3 +D-Arg (10 mg/L) after 5 days. (B) Ethylene synthesis rates of differentially treated peach shoots. Total ACC content (C) and free ACC content (D) of peach shoots treated with different fluids. Bars with different letters indicate significant differences (p < 0.05, Duncan’s multiple range tests).
The ethylene and polyamine synthesis pathways are considered competitive because they share the same precursor, methionine. Under AVG (an ACC synthesis inhibitor) and MGBG (a Spd and Spm synthesis inhibitor) treatment, the synthesis of ethylene and ACC showed opposite results. AVG inhibited the synthesis of ethylene and ACC, while MGBG had the opposite effect. Silicon inhibited ethylene and ACC synthesis as AVG, but the inhibitory effect of silicon was disappeared when peach shoots were co-treated with silicon and MGBG (Figures 5A–C). Moreover, silicon treatment increased the content of Spd (17.74%) and Spm (20.27%), but not Put, in peach shoots. AVG increased the content of Spm and Spd, while MGBG functioned adversely. The promoting effect of silicon on the Spm and Spd content was also enhanced by AVG but inhibited by MGBG (Figures 5D–F).
Figure 5 Effect of silicon on ACC and polyamine content. (A) Ethylene synthesis rate of differentially treated peach shoots. Total ACC content (B) and free ACC content (C) of peach shoots treated with different fluids.The Put content (D), Spd content (E) and Spd content (F) in peach shoots under different treatments.The peach shoots were treated with water, Na2SiO3 (0.6 mmol/L), AVG (0.5 mmol/L) and MGBG (0.5 mmol/L) individually or in combination, and samples were taken for analysis at 0h, 24h, and 48h after treatment. Bars with different letters indicate significant differences (p < 0.05, Duncan’s multiple range tests).
In peach shoots, treatment of AVG inhibited the enzymatic activity of ACS, while MGBG inhibited the enzyme activity of SAMDC. Silicon increased the enzyme activity of SAMDC and inhibited ACS enzyme activity. Compared to control, the enzyme activity of ACS was reduced by 11.50%, while that of SAMDC was increased by 9.85% after silicon treatment (Figures S4A, B). While the inhibitory effect of silicon on ACS activity was alleviated upon addittion of MGBG. The expression of PpACS1 and PpACS2 were downregulated by silicon and AVG, and both of them were upregulated by MGBG. However, the upregulation of gene expression by silicon was weakened by MGBG (Figures S4C, D). The expression of the PpSAMDC was upregulated 12.68-fold compared to control after silicon treatment, and this effect was enhanced by AVG but attenuated by MGBG (Figure S4E). This series of results shows that silicon inhibits the synthesis of ACC by promoting the synthesis of Spm and Spd, thereby inhibiting the production of ethylene.
Gummosis is a process of gum formation, accumulation and exudation that occurs in a variety of plants, especially in the family Rosaceae (Miyamoto et al., 2010). Research on plant gummosis found that hormones are important factors affecting its pathogenesis (Miyamoto et al., 2015; Agnieszka et al., 2019). Since all the abiotic and biotic environmental stress factors, such as pathogen infection, stress, pest damage, etc., are considered being mediated by the action of ethylene produced in plant tissues. And exogenous ethylene or ethephon induces gummosis, so ethylene is considered to be the common factor for gummosis (Li et al., 2014b; Li et al., 2015). Our study found that the mechanical damage site of peach shoots was a high-incidence area for gummosis disease, and exogenous ethephon treatment accelerated the rate of gummosis and increased the gum quantity. The process of gummosis is caused by the destruction of the cell wall structure to form gum ducts, and the formation of gum ducts is a process of cell wall degradation (Gedalovich and Fahn, 1985; Agnieszka et al., 2019). By using radioactive labelling of polysaccharides in the cell wall of gum duct epithelial cells of cherry trees, it was found that the gum came from the polysaccharides in the cell wall (Stosser et al., 1978). There are many kinds of cell wall degrading enzymes that are important factors of cell wall degradation. Among which PME, PG and β-GAL are closely related to peach gummosis (Wei et al., 2010). Transcriptome analysis of peach shoots treated with exogenous ethephon showed that ethylene affected the expression of PME, PG and β-GAL-encoding genes, and the expression of ppa003578m, ppa003852m, ppa003639m and ppa007271m was significantly upregulated by ethylene. After ethephon treatment, the activity of PME, PG and β-GAL were increased in the gummosis-affected region of peach shoots, indicating that the degradation of the cell wall was aggravated and the formation of gum was accelerated. In addition, ethylene promotes the formation of gum ducts in Prunus mume and increases the length and area of gum ducts (Carolina and Kusumoto, 2020). The main reason of peach gummosis is an excessive synthesis of endogenous ethylene.
Silicon is a beneficial element for plant growth and has been shown to improve plant disease resistance (Zeyen, 2002; Sathe et al., 2021).We also found that silicon played an important role in inhibiting peach gummosis, which reduced its incidence. Silicon effectively inhibited the damage to the cells in the gummosis-affected area of the peach shoots and reduced the degradation of the cell wall. On one hand, the strengthening effect of silicon on the cell wall was due to the accumulation of silicon in the shoots. Which promoted the enhancement of cell silicification, enhanced the performance of the cell wall, and formed a physical defence barrier (Cai et al., 2010; Law and Exley, 2011). On the other hand, silicon inhibited ethylene synthase activity and gene expression, preventing massive ethylene synthesis, and reduced the activity of cell wall-degrading enzymes in peach shoots. The regulatory effect of ethylene synthesis by silicon has yet been verified in rice, tobacco and other plants. The inhibitory effect of silicon on rice blast was attributed to silicon inhibiting the expression of ethylene synthesis genes in rice. Rice treated with silicon and subjected to wounding had a lowered ethylene content (Kim et al., 2015; Souri et al., 2020). Silicon can alleviate the salt stress of tobacco suspension cells, but this alleviation must involve the participation of ethylene (Liang et al., 2015).These conclusions also verify our hypothesis. Microarray analysis revealed a stimulatory role of silicon in activating the ethylene signaling pathway in plants infected with pathogens and improving plant immunity (Tripathi et al., 2020). These evidence suggest that silicon inhibits gummosis not only through physical defence but also by inhibiting ethylene synthesis.
Ethylene is synthesized excessively in plants under stress from diseases, insect pests, or mechanical damage (Morgan and Drew, 2010). The immediate precursor of ethylene synthesis is ACC, and its synthesis is regulated by a variety of factors (Kacperska and Maria, 2010). We found that silicon inhibited ethylene synthesis, but exogenous ACC weakens the inhibitory effect of silicon on ethylene synthesis, so silicon reduced ethylene production by inhibiting ACC synthesis. ACS is a key enzyme in ACC synthesis, and the expression of its key synthesis gene PpACS1 was regulated by silicon. This is consistent with Yin’s results, indicating that silicon mediates the ACC synthesis process (Harpaz-Saad et al., 2012; Yin et al., 2014). Polyamines play a variety of roles in plant growth and development, as well as in cell homeostasis (Bregoli et al., 2006; Pottosin and Shabala, 2014; Nader et al., 2020). Polyamine biosynthesis levels are dramatically enhanced under increased stress and are involved in stress defence responses (Francois et al., 2005; Takahashi et al., 2009; Ashraf et al., 2011). Similarly, we found thatthe polyamine levels increased with silicon application. Silicon increased the synthesis of Spd and Spm by upregulating the expression of PpSAMDC and increasing the activity of SAMDC (Ding et al., 2015; Yin et al., 2016). But the enhancement of silicon was blocked by MGBG. SAMDC has a short half-life and is a rate-limiting enzyme in the production of Spd and Spm. Overexpression of SAMDC increases polyamine levels and strengthens resistance (Roy and Wu, 2002), and this is consistent with our results. Polyamines working as stress signalling regulators can induce plant defence responses and enhance resistance (Kuznetsov and Shevyakova, 2010; Singh et al., 2015). Silicon increased the polyamine content, and this may also be a mechanism to inhibit the occurrence of gummosis. Polyamines may be involved in silica precipitation, as long-chain polyamines were discovered to be involved in the mediation of silica precipitation from a silicic acid solution in diatoms (Kröger et al., 2000; Shi et al., 2013). The interaction between silicon and polyamines would have a beneficial effect on the precipitation of silicon on the cell wall and induce plant defence responses.
The enzyme ACS catalyses the conversion of SAM to ACC, which is a critical regulatory step in the biosynthesis of ethylene (Harpaz-Saad et al., 2012). Previous studies had found that polyamines reduced ethylene release by inhibiting ACC synthesis, thereby increasing sorghum’s salt resistance (Yin et al., 2016). After the mutation of the LeACS6 in tomato, reduced ACS enzyme activity, ethylene synthesis was blocked (Shi et al., 2003). In this experiment, silicon increased the Spd and Spm levels in peach shoots, and RT-qPCR showed that the expression of PpSAMDC was upregulated. Furthermore, Spd and Spm inhibited ethylene synthesis by reducing the enzyme activity and gene expressions of ACS, and the levels of total ACC and free ACC were decreased. AVG can promoted the synthesis of polyamines, which had the same effect as silicon. This is consistent with previous research results. However, when silicon and MGBG were used together, the synthesis of Spd and Spm was blocked, and the inhibitory effect of silicon on ACC synthesis was weakened. The results for the enzyme activity and relative gene expressions of ACS and SAMDC supported this conclusion. Ethylene and polyamines have a competitive interaction, implying that silicon inhibits the synthesis of ACC by promoting the synthesis of Spd and Spm, thus inhibiting ethylene synthesis. Decreased accumulation of ACC as well as ethylene can alleviate gummosis.
In conclusion, ethylene is involved in peach gummosis; it enhances the enzymatic activity of cell wall-degrading and promotes gummosis. Silicon upregulated the expression of PpSAMDC, promoted the accumulation of Spd and Spm. Simultaneously, polyamine reduced the expression of PpACS1 and the enzyme activity of ACS, then reduced the ethylene synthesis. (Figure 6). So silicon restricted gummosis development by activating ployamines biosynthesis and inhibiting ethylene synthesis in peach. However, the specific molecular mechanism of silicon regulating the synthesis of polyamines and ethylene to inhibit peach gums needs further study. For example, it is unclear how silicon regulates the expression and synthesis of polyamine and ethylene synthesis genes and the specific mechanism of polyamine and ethylene inhibiting gumming.
Figure 6 Silicon-mediated polyamine inhibition of ethylene synthesis to inhibit gummosis. Target induction is depicted by solid arrows, whereas inhibition is depicted by blocked lines. Red indicates upregulation, and green indicates downregulation. (Si: silicon; SAMDC: S-adenosine methionine decarboxylase; ACS: ACC synthase; Spd: spermidine; Spm: spermine).
The data presented in the study are deposited in online repositories. The data presented in the study are deposited in the NCBI repository, accession number PRJNA899649.
HG: Data curation, writing-original draft. XW: Conceptualization, data curation. XY: Investigation, methodology. MS: Conceptualization, investigation. JL: Investigation. FP: Conceptualization, methodology, project administration, supervision, writing-review, editing. YX: Conceptualization, methodology, supervision, writing – review, editing. All authors contributed to the article and approved the submitted version.
This work was supported by the National Key Research and Development Program of China (2020YFD1000203) and the earmarked fund for China Agriculture Research System (No. CARS-30-2-02)
The authors declare that the research was conducted in the absence of any commercial or financial relationships that could be construed as a potential conflict of interest.
All claims expressed in this article are solely those of the authors and do not necessarily represent those of their affiliated organizations, or those of the publisher, the editors and the reviewers. Any product that may be evaluated in this article, or claim that may be made by its manufacturer, is not guaranteed or endorsed by the publisher.
The Supplementary Material for this article can be found online at: https://www.frontiersin.org/articles/10.3389/fpls.2022.986688/full#supplementary-material
Adesemoye, A. O., Mayorquin, J. S., Wang, D. H., Twizeyimana, M., Lynch, S. C., Eskalen, A., et al. (2014). Identification of species of botryosphaeriaceae causing bot gummosis in citrus in California. Plant Dis. 98, 55–61. doi: 10.1094/pdis-05-13-0492-re
Agnieszka, M., Justyna, G.K., Urszula, K., Kensuke, M., Junichi, U., Marian, S, et al. (2019). Histological analysis of methyl jasmonate-induced gummosis in petiole of culinary rhubarb (Rheum rhabarbarum l.)-ScienceDirect. Scientia Hortic. 254, 172–177. doi: 10.1016/j.scienta.2019.05.001
Ashraf, M., Akram, N. A., Al-Qurainy, F., Foolad, M. R. (2011). Drought tolerance: Role of organic osmolytes, growth regulators, and mineral nutrients. Adv. Agron. 111 (6), 249–296. doi: 10.1142/S0129167X98000282
Bélanger, R. R., Benhamou, N., Menzies, J. G. (2003). Cytological evidence of an active role of silicon in wheat resistance to powdery mildew (blumeria graminis f. sp. tritici). Phytopathology 93 (4), 402–412. doi: 10.1094/PHYTO.2003.93.4.402
Bharwana, S. A., Ali, S., Farooq, M. A., Iqbal, N., Abbas, F., Msa, A., et al. (2013). Alleviation of lead toxicity by silicon is related to elevated photosynthesis, antioxidant enzymes suppressed lead uptake and oxidative stress in cotton. J. Bioremediation Biodegradation 4, 187. doi: 10.4172/2155-6199.1000187
Bregoli, A. M., Ziosi, V., Biondi, S., Claudio, B., Costa, G., Torrigiani, P., et al. (2006). A comparison between intact fruit and fruit explants to study the effect of polyamines and aminoethoxyvinylglycine (AVG) on fruit ripening in peach and nectarine (Prunus persica l Batch). Afterharvest Biol. Technol. 42, 31–40. doi: 10.1016/j.afterharvbio.2006.05.009
Cai, K. Z., Gao, D., Luo, S. M., Zeng, R. S., Yang, J. Y., Zhu, X. Y., et al. (2010). Physiological and cytological mechanisms of silicon-induced resistance in rice against blast disease. Physiologia Plantarum 134 (2), 324–333. doi: 10.1111/j.1399-3054.2008.01140.x
Carolina, A., Kusumoto, D. (2020). Gum duct formation mediated by various concentrations of ethephon and methyl jasmonate treatments in Cerasus x yedoensis, prunus mume and Liquidambar styraciflua. IAWA J. 41, 98–108. doi: 10.1163/22941932-00002105
Chain, F., Cote-Beaulieu, C., Belzile, F., Menzies, J. G., Bélanger, R. R. (2010). A comprehensive transcriptomic analysis of the effect of silicon on wheat plants under control and pathogen stress conditions. Mol. Plant Microbe. Interact. 22 (11), 1323. doi: 10.1094/MPMI-22-11-1323
Ding, C. C., Chen, T., Yang, Y., Liu, S., Yan, K., Yue, X. L., et al. (2015). Molecular cloning and characterization of an s-adenosylmethionine synthetase gene from chorispora bungeana. Gene 572 (2), 205–213. doi: 10.1016/j.gene.2015.07.062
Ezra, D., Hershcovich, M., Shtienberg, D. (2017). Insights into the etiology of gummosis syndrome of deciduous fruit trees in Israel and its impact on tree productivity. Plant Dis. 101, 1354–1361. doi: 10.1094/PDIS-12-16-1836-RE
Fauteux, F., Chain, F., Belzile, F., Menzies, J. G., Belanger, R. R. (2006). The protective role of silicon in the arabidopsis-powdery mildew pathosystem. Proc. Natl. Acad. Sci. 103 (46), 17554–17559. doi: 10.1073/pnas.0606330103
Franchin, C., Fossati, T., Pasquini, E., Lingua, G., Biondi, S. (2007). High concentrations of zinc and copper induce differential polyamine responses in micropropagated white poplar (Populus alba). Physiol. Plant 130, 77–90. doi: 10.1111/j.1399-3054.2007.00886.x
Francois, F., Wilfried, B., James, M., Richard, B. (2005). Silicon and plant disease resistance against pathogenic fungi. FEMS Microbiol. Lett. 249 (1), 1–6. doi: 10.1515/hppj-2016-0001
Gedalovich, E., Fahn, A. (1985). Development and ultrastructure of gum ducts in citrus plants formed as a result of brown-rot gummosis. Protoplasma 127, 73–81. doi: 10.1007/BF01273703
Gong, H. J., Chen, K. M. (2012). The regulatory role of silicon on water relations, photosynthetic gas exchange, and carboxylation activities of wheat leaves in field drought conditions. Acta Physiologiae Plantarum 34 (4), 1589–1594. doi: 10.1007/s11738-012-0954-6
Guo, Y., Chen, D., Yang, B., Hua, Z., Li, Z. (2005). Inhibitive effect of silicon compounds on powdery mildew of muskmelon. J. Fruit Sci. 01, 35–39. doi: 10.13925/j.cnki.gsxb.2005.01.009
Harpaz-Saad, S., Mee Yoon, G., Mattoo, A., Kieber, J. J. (2012). The formation of acc and competition between polyamines and ethylene for sam. Annu. Plant Rev. 44, 53–81. doi: 10.1002/9781118223086.ch3
Kacperska, A., Maria, K. Z. (2010). Formation of stress ethylene depends both on ACC synthesis and on the activity of free radical-generating system. Physiologia Plantarum 77 (2), 231–237. doi: 10.1111/j.1399-3054.1989.tb04974.x
Kim, Y. H., Khan, A. L., Lee, I. J. (2015). Silicon: a duo synergy for regulating crop growth and hormonal signaling under abiotic stress conditions. Crit. Rev. Biotechnol. 36, 1099–1109. doi: 10.3109/07388551.2015.1084265
Kröger, N., Deutzmann, R., Bergsdorf, C., Sumper, M. (2000). Species-specific polyamines from diatoms control silica morphology. Proc. NationalAcademy Sci. United States America 97, 14133–14138. doi: 10.1073/pnas.260496497
Kuznetsov, V. V., Shevyakova, N. I. (2010). Polyamines and plant adaptation to saline environments (Berlin Heidelberg: Springer), 261–298.
Law, C., Exley, C. (2011). New insight into silica deposition in horsetail (Equisetum arvense). BMC Plant Biol. 11, 112. doi: 10.1186/1471-2229-11-112
Leonardo, L. L., Ribeiro, D. M., Catarina, C. S., Barros, R. S., Floh, E. I. S., Otoni, W. C. (2010). Ethylene and polyamine interactions in morphogenesis of Passiflora cincinnata: effects of ethylene biosynthesis and action modulators, as well as ethylene scavengers. Plant Growth Regul. 62, 9–19. doi: 10.1007/s10725-010-9478-5
Liang, Y. C., Sun, W. C., Si, J., Rmheld, V. (2010). Effects of foliar- and root-applied silicon on the enhancement of induced resistance to powdery mildew in Cucumis sativus. Plant Pathol. 54 (5), 678–685. doi: 10.1111/j.1365-3059.2005.01246.x
Liang, X. L., Wang, H. H., Hu, Y. F., Mao, L. N., Sun, L. L. (2015). Silicon does not mitigate cell death in cultured tobacco BY-2 cells subjected to salinity without ethylene emission. Plant Cell Rep. 34 (2), 331–343. doi: 10.1007/s00299-014-1712-6
Li, M. J., Liu, M. Y., Peng, F. T., Fang, l. (2015). Influence factors and gene expression patterns during meja-induced gummosis in peach. J. Plant Physiol. 182, 49–61. doi: 10.1016/j.jplph.2015.03.019
Li, Z., Wang, Y. T., Gao, L., Wang, F., Ye, J. L., Li, G. H., et al. (2014a). Biochemical changes and defence responses during the development of peach gummosis caused by lasiodiplodia theobromae. Eur. J. Plant Pathol. 138 (1), 195–207. doi: 10.1007/s10658-013-0322-4
Li, Z., Zhu, W., Fan, Y. C., Ye, J. L., Li, G. H. (2014b). Effects of pre- and after-treatment with ethephon on gum formation of peach gummosis caused by lasiodiplodia theobromae. Plant Pathol. 63, 1306–1315. doi: 10.1111/ppa.12214
Ma, J. F., Yamaji, N. (2006). Silicon uptake and accumulation in higher plants: A review of abiotic stress series. Trends Plant Sci. 11 (8), 392–397. doi: 10.1016/j.tplants.2006.06.007
Miyamoto, K., Kotake, T., Boncela, A. J., Marian, S., Junichi, U. (2015). Hormonal regulation of gummosis and composition of gums from bulbs of hyacinth (Hyacinthus orientalis). J. Plant Physiol. 174, 1–4. doi: 10.1016/j.jplph.2014.10.007
Miyamoto, K., Kotake, T., Sasamoto, M., Saniewski, M., Ueda, J. (2010). Gummosis in grape hyacinth (Muscari armeniacum) bulbs: hormonal regulation and chemical composition of gums. J. Plant Res. 123 (3), 363–370. doi: 10.1007/s10265-009-0273-1
Miyamoto, K., Saniewski, M., Ueda, J. (2019). Gummosis and leaf abscission in yoshino cherry (Prunus yedoensis): relevance to hormonal regulation and chemical composition of gums. ActaHortic 1235, 467–474. doi: 10.17660/ActaHortic.2019.1235.65
Morgan, P. W., Drew, M. C. (2010). Ethylene and plant responses to stress. Physiologia Plantarum 100 (3), 620–630. doi: 10.1016/j.plaphy.2019.12.033
Nader, A., Khosh-Khui, M., Salehi, H., Razi, H., Moghadam, A. (2020). Role of genes and metabolites involved in polyamines synthesis pathways and nitric oxide synthase in stomatal closure on Rosa damascena Mill. Under drought stress. Plant Physiol. Biochem. 148, 53–61. doi: 10.1016/j.plaphy.2019.12.033
Ning, D. F., Liang, Y. C. (2014). Silicon-mediated plant disease resistance: advance and perspectives. J. Plant Nutr. Fertilizer 20 (05), 1280–1287. doi: 10.11674/zwyf.2014.0525
Pandey, S., Ranade, S. A., Nagar, P. K., Kumar, N. (2000). Role of polyamines and ethylene as modulators of plant senescence. J. Biosciences. 25, 291–299. doi: 10.1007/BF02703938
Pottosin, I., Shabala, S. (2014). Polyamines control of cation transport across plant membranes: implications for ion homeostasis and abiotic stress signaling. Front. Plant Sci. 5. doi: 10.3389/fpls.2014.00154
Rodrigues, F. A., Mcnally, D. J., Datnoff, L. E., Jones, J. B., Bélanger, R. R. (2004). Silicon enhances the accumulation of diterpenoid phytoalexins in rice: a potential mechanism for blast resistance. Phytopathology 94 (2), 177–183. doi: 10.1094/PHYTO.2004.94.2.177
Roy, M., Wu, R. (2002). Overexpression of s-adenosylmethionine decarboxylase gene in rice increases polyamine level and enhances sodium chloride-stress. Plant Sci. 163, 987–992. doi: 10.1016/S0168-9452(02)00272-8
Safoora, D., Cyrus, G., Bahram, B., Mahdi, G., Siamak, S. (2018). Effect of silicon on growth and development of strawberry under water deficit conditions. Hortic. Plant J. 4 (6), 226–232. doi: 10.1016/j.hpj.2018.09.004
Saniewski, M., Miyamoto, K., Ueda, J. (1998). Methyl jasmonate induces gums and stimulates anthocyanin accumulation in peach shoots. J. Plant Growth Regul. 17, 121–124. doi: 10.5586/aa.2001.020
Saniewski, M., Ueda, J., Horbowicz, M., Miyamoto, K., Puchalski, J (2001). Gum in apricot (Prunus armeniaca L.) shoots induced by methyl jasmonate. Acta Agrobot 54, 27–34. doi: 10.5586/aa.2001.020
Sathe, A. P., Kumar, A., Mandlik, R., Raturi, G., Sonah, H. (2021). Role of silicon in elevating resistance against sheath blight and blast diseases in rice (Oryza sativa l.). Plant Physiol. Biochem. 3, 128–139. doi: 10.1016/j.plaphy.2021.05.045
Shirani, B. S., Zheng, S. (2018). ). banana fusarium wilt (Fusarium oxysporum f. sp. cubense) control and resistance, in the context of developing wilt-resistant bananas within sustainable production systems. Hortic. Plant J. 4 (5), 208–218. doi: 10.1016/j.hpj.2018.08.001
Shi, J. Y., Yao, Q. Z., Li, X. M., Zhou, G. T., Fu, S. Q., Bansal, V., et al. (2013). Formation of asymmetrical structured silica controlled by a phase separation process and implication for biosilicification. PloS One 8, e61164. doi: 10.1371/journal.pone.0061164
Shi, Y., Yu, Q. H., Wang., L., Luo, Y. (2003). Obtaining and phenotype of transantisense ACS precessing tomato regeneration plants. Acta Agriculturae Boreali-occidentalis Sin. 01, 40–42. doi: 10.1016/S0891-0618(02)00103-5
Simas-Tosin, F. F., Barraza, R. R., Petkowicz, C. L. O., Silveira, J. L. M., Sassaki, G. L. (2010). Rheological and structural characteristics of peach tree gum exudate. Food Hydrocolloid 24 (5), 486–493. doi: 10.1016/j.foodhyd.2009.12.010
Singh, M., Kumar, J., Singh, S., Singh, V. P. (2015). Prasad SM. roles of osmoprotectants in improving salinity and drought tolerance in plants: a review. Rev. Environ. Sci. Biotechnol. 14, 407–426. doi: 10.1007/s11157-015-9372-8
Souri, Z., Khanna, K., Karimi, N., Ahmad, P. (2020). Silicon and plants: Current knowledge and future prospects. J. Plant Growth Regul. 40 (3), 906–925. doi: 10.1007/s00344-020-10172-7
Stosser, R. (1978). Histochemische nachweis einiger enzyme im zusammenhang mit der gummibildung bei susskirschen. Angewandte Botanik 2, 363–369. doi: 10.1093/aob/mcp259
Takahashi, T (2009). Polyamines: ubiquitous polycations with unique roles in growth and stress responses. Ann. Bot 105, 1–6. doi: 10.1093/aob/mcp259
Tripathi, D. K., Vishwakarma, K., Singh, V. P., Prakash, V., Sharma, S., Muneer, S., et al. (2020). Silicon crosstalk with reactive oxygen species, phytohormones and other signaling molecules. J. Hazardous Materials 3894 (20), 32811–32819. doi: 10.1016/j.jhazmat.2020.124820
Vincent, F., Molin, D. D., Weiner, R. M., Bourne, Y., Henrissat, B. (2010). Structure of a polyisoprenoid binding domain from saccharophagus degradans implicated in plant cell wall breakdown. FEBS Lett. 584 (8), 1577–1584. doi: 10.1016/j.febslet.2010.03.015
Vorwerk, S., Somerville, S., Somerville, C. (2004). The role of plant cell wall polysaccharide composition in disease resistance. Trends Plant Scienc 9 (4), 203–209. doi: 10.1016/j.tplants.2004.02.005
Wang, F., Zhao, L., Li, G., Huang, J., Hsiang, T. (2011). Identification and characterization of botryosphaeria spp. causing gummosis of peach trees in hubei province, central China. Plant Dis. 95, 1378–1384. doi: 10.1094/PDIS-12-10-0893
Wei, J., Ma, F., Shi, S., Qi, X., Zhu, X., Yuan, J., et al. (2010). Changes and afterharvest regulation of activity and gene expression of enzymes related to cell wall degradation in ripening apple fruit. Afterharvest Biology& Technol. 56 (2), 147–154. doi: 10.1016/j.afterharvbio.2009.12.003
Wen, S. (2016). Research progress on resistance mechanism to rice diseases by silicon. Modern Agric. Sci. Technol. 14, 134–135. doi: 10.07-5739201614-0134-02
Ye, X., Zheng, X. B., Zhai, D. H., Song, W., Tan, B., Li, J. D., et al. (2017). Expression patterns of ACS and ACO gene families and ethylene production in rachis and berry of grapes. Hortscience A Publ. Am. Soc. Hortic. Sci. 52 (3), 413–422. doi: 10.21273/hortsci11050-16
Yin, J. L., Jia, J. H., Lian, Z. Y., Hu, Y. H., Guo, J., Huo, G. Q., et al. (2019). Silicon enhances the salt tolerance of cucumber through increasing polyamine accumulation and decreasing oxidative damage. Ecotoxicology Environ. Saf. 169, 8–17. doi: 10.1016/j.ecoenv.2018.10.105
Yin, L. N., Wang, S., Liu, P., Wang, W., Cao, D., Deng, X., et al. (2014). Silicon-mediated changes in polyamine and 1-aminocyclopropane -1-carboxylic acid are involved in silicon-induced drought resistance in Sorghum bicolor l. Plant Physiol. Biochem. 80, 268–277. doi: 10.1016/j.plaphy.2014.04.014
Yin, L. N., Wang, S. W., Tanaka, K., Fujihara, S., Itai, A., Den, X. P., et al. (2016). Silicon-mediated changes in polyamines participate in Si-induced salt tolerance in Sorghum bicolor l. Plant Cell Environ. 39, 245–258. doi: 10.1111/pce.12521
Yuan, H., Yue, P., Bu, H., Han, D., Wang, A. (2019). Genome-wide analysis of ACO and ACS genes in pear (Pyrus ussuriensis). In Vitro Cell. Dev. Biol. Plant 56 (3), 193–199. doi: 10.1007/s11627-019-10009-3
Yu, L., Huang, Y., Du, X. Y., Li, R. J. (2009). Effect analysis of adding silicon fertilizer on the resistance of rice to rice blast. J. Anhui Agric. Sci. 37 (23), 11044–11046. doi: 10.13989/j.cnki.0517-6611
Zeyen, R. (2002). Silicon in plant cell defenses against cereal powdery mildew disease. Abstract Second Silicon Agric. Conf. 2, 15–21.
Keywords: silicon, ethylene, gummosis, polyamine, peach
Citation: Gao H, Wu X, Yang X, Sun M, Liang J, Xiao Y and Peng F (2022) Silicon inhibits gummosis by promoting polyamine synthesis and repressing ethylene biosynthesis in peach. Front. Plant Sci. 13:986688. doi: 10.3389/fpls.2022.986688
Received: 05 July 2022; Accepted: 25 October 2022;
Published: 28 November 2022.
Edited by:
Maren Müller, University of Barcelona, SpainReviewed by:
Tian Zhong Li, China Agricultural University, ChinaCopyright © 2022 Gao, Wu, Yang, Sun, Liang, Xiao and Peng. This is an open-access article distributed under the terms of the Creative Commons Attribution License (CC BY). The use, distribution or reproduction in other forums is permitted, provided the original author(s) and the copyright owner(s) are credited and that the original publication in this journal is cited, in accordance with accepted academic practice. No use, distribution or reproduction is permitted which does not comply with these terms.
*Correspondence: Yuansong Xiao, eXN4aWFvQHNkYXUuZWR1LmNu; Futian Peng, cGZ0QHNkYXUuZWR1LmNu
Disclaimer: All claims expressed in this article are solely those of the authors and do not necessarily represent those of their affiliated organizations, or those of the publisher, the editors and the reviewers. Any product that may be evaluated in this article or claim that may be made by its manufacturer is not guaranteed or endorsed by the publisher.
Research integrity at Frontiers
Learn more about the work of our research integrity team to safeguard the quality of each article we publish.