- 1Laboratory of Synthetic Biology, Department of Genetics and Morphology, Institute of Biological Science, University of Brasília (UnB), Brasília, Distrito Federal, Brazil
- 2School of Medicine, Federal University of Juiz de Fora (UFJF), Juiz de Fora, Minas Gerais, Brazil
As one of synthetic biology’s foundations, biocircuits are a strategy of genetic parts assembling to recognize a signal and to produce a desirable output to interfere with a biological function. In this review, we revisited the progress in the biocircuits technology basis and its mandatory elements, such as the characterization and assembly of functional parts. Furthermore, for a successful implementation, the transcriptional control systems are a relevant point, and the computational tools help to predict the best combinations among the biological parts planned to be used to achieve the desirable phenotype. However, many challenges are involved in delivering and stabilizing the synthetic structures. Some research experiences, such as the golden crops, biosensors, and artificial photosynthetic structures, can indicate the positive and limiting aspects of the practice. Finally, we envision that the modulatory structural feature and the possibility of finer gene regulation through biocircuits can contribute to the complex design of synthetic chromosomes aiming to develop plants and algae with new or improved functions.
Introduction
Photosynthetic organisms, such as plants and algae, have been discussed as promising candidates to overcome the existing challenges in the different areas of bioeconomy, food and feed, environment, and health. However, efficient methods for multiple genetic and metabolic engineering are essential to access this potential. In this sense, synthetic biology tools could contribute towards this goal.
Genetic circuits combine, in a network manner, genetic parts in several switches that are system units able to perceive an input, process the information and generate an output (Andres et al., 2019). Endogenous biocircuits have been known since the 60s, once Monod and Jacob recognized the resemblance of the gene expression control in the lactose and tryptophan operons with electric circuits (Monod and Jacob, 1961). Nonetheless, it was not until the 2000s that scientists developed the first synthetic genetic circuits (Elowitz and Leibler, 2000; Gardner et al., 2000). Since then, this synthetic biology strategy has been used to produce photosynthetic organisms, such as plants and algae, with desirable traits (Rasala et al., 2012; de Lange et al., 2018).
Earlier than synthetic biocircuits, the first construct with a centromere, an origin of replication, a selectable marker, and telomeres was transformed and maintained in yeast (Murray and Szostak, 1983). From then on, artificial chromosomes have been proposed as a platform for introducing genes of interest to developing organisms with new or improved functions. In plants, minichromosomes, engineered through telomere-mediated truncation (Yu et al., 2006, 2007), can be envisioned as the ground foundation for synthetic chromosome development in these organisms (Birchler, 2015). In this regard, these synthetic structures could be discussed as a safe landing platform to introduce genes finely regulated through synthetic circuits to obtain plants and algae expressing intended phenotypes.
In this review, we will focus on presenting a brief history of biocircuits, the requirements and challenges for their widespread use, and the achievements completed in plants and eukaryotic algae (Figure 1). We will also discuss the perspective of this synthetic biology tool to provide a more precise gene expression control system for artificial chromosomes and how all these advances will contribute to the development of functions of interest (Figure 1).
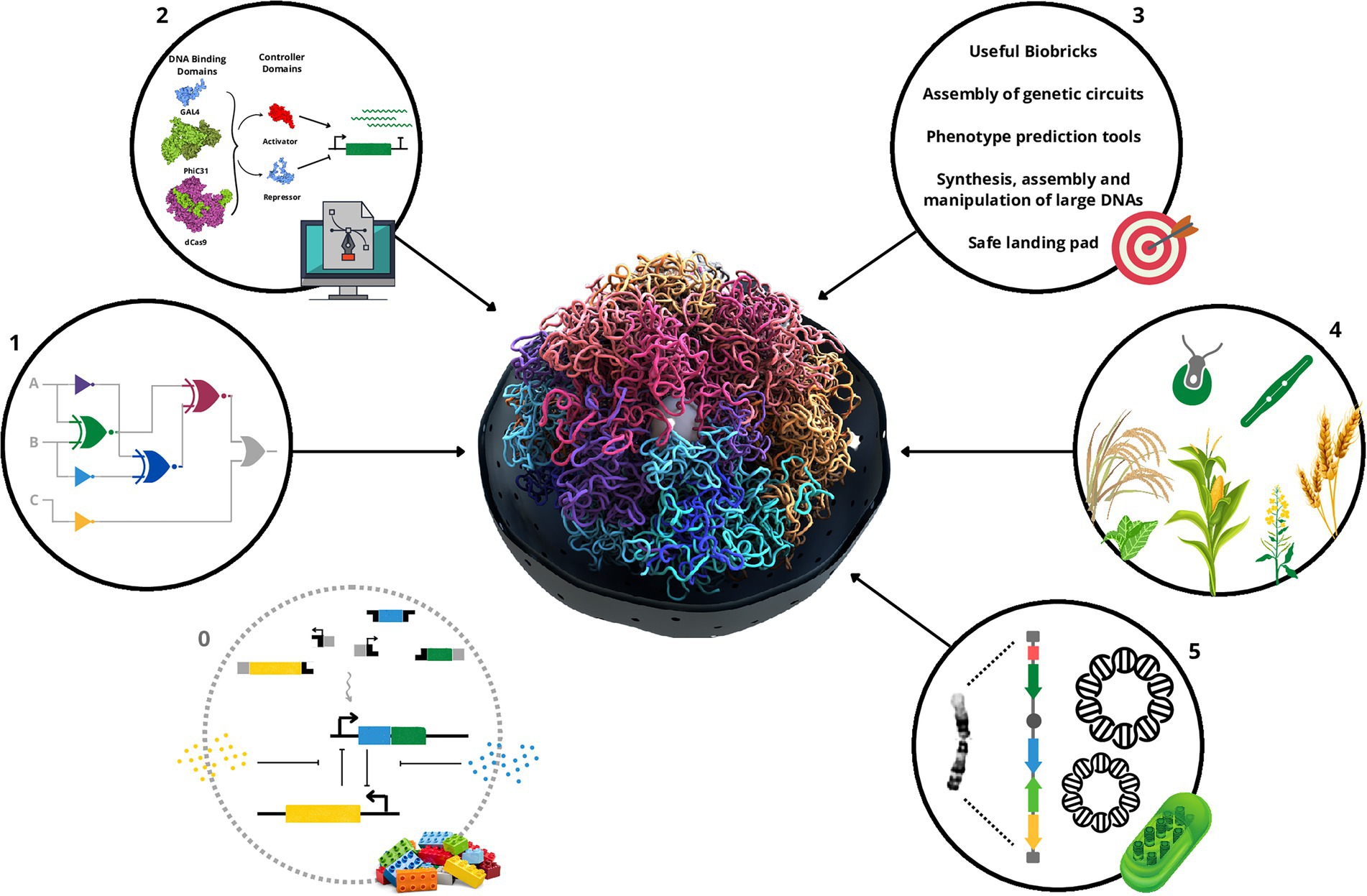
Figure 1. Schematic summary of the important steps, challenges, achievements, and prospective usage in synthetic chromosomes for the biocircuits in plants and algae. First, a repository of reliable, functional, orthogonal, scalable biological parts (0) is essential to the assembly of biocircuits (1). Also, tools that allow fine control of the expression of genes that compose the biocircuits with computational methods that can use the biological parts to assemble genetic circuits and predict the phenotype from the genotype are very welcome (2). Those together with the need of a repository for usable biological parts, tools that allow synthesis, assembly and manipulation of large DNAs, and safe harbors for the genetic circuits represent the main challenges in this area (3). Nevertheless, high vitamin A Golden crops, biosensors, and trans-kingdom genetic circuit plants, in addition to biofabric algae have been developed (4). As a perspective, biocircuits could greatly contribute to the control of gene expression once artificial/synthetic chromosomes become the landing platform for plants and algae development with improved or new functions (5). The 3D design of the molecules in the number 2 scheme was generated by Illustrate (Goodsell et al., 2019).
Genetic circuits foundation and development
After Jacob and Monod proposed that biological elements are connected in regulatory circuits (Monod and Jacob, 1961), other researchers extended these ideas. In this process, an expansion was to evaluate genes as on–off devices, linking the genetic control expression to Boolean algebra functions (Kauffman, 1969; Thomas, 1973; Sugita, 1975). In Boolean terms, the expression of a gene can be seen as binary variables, assuming 1 when the gene is expressed and 0 when it is not. This proposal is valuable for the design of genetic logic gates whose outputs obey their specific truth tables (Wieland and Fussenegger, 2012; Miyamoto et al., 2013).
Emerging from the theoretical studies, the first synthetic circuit was the genetic toggle switch planned to work as a binary on–off gene state in Escherichia coli (Gardner et al., 2000). This system was composed of two inducible promoters controlling the expression of each other’s repressor protein, being one of the genes cistronically followed by the coding sequence of the reporter green fluorescence protein (GFP). Thus, an oscillatory reporter expression was expected after coordinated induction. In the same year, Elowitz and Leibler also designed and constructed a synthetic oscillatory network using repressor proteins (Elowitz and Leibler, 2000), with a similar rationale and extended complexity. The model works as an extension of the first prototypical oscillator, the Goodwin oscillator (Goodwin, 1965). This system consists of elements that regulate themselves as a negative feedback loop. However, this repressilator exhibited undesirable noise behavior and, as the toggle switch, stability was affected by stochastic features of the regulatory components.
As the complexity of synthetic circuits increases, the functional description of biological parts and standardization of assembly methods could help to minimize unpredicted outcomes and facilitate genetic constructions. The Registry of Standard Biological Parts (RSBP), created for this purpose (Endy, 2005; Cameron et al., 2014), catalogs and stores biological genetic parts, such as promoters, terminators, and protein-coding sequences. The standardized BioBrick format allows an assembly approach based on low-frequency restriction enzymes of E. coli (Knight, 2003). Currently, the International Genetically Engineered Machine (iGEM) Foundation is responsible for the public record of these parts. The institution also promotes a student competition for synthetic biology development with projects that have a significant source of approaches to test genetic circuits in several organisms, including plants and algae.
Since 2010, the iGEM teams that aimed at plant studies have been developing projects of biosensors, bioremediation, expression of proteins of interest or compounds with pharmaceutical properties, and CRISPRs systems. Due to the technical challenges, time-consuming required to work with plants, and the competition’s scheduled time, most projects are not fully accomplished. However, it is achievable to highlight substantial contributions to the synthetic biology of plants. Among these are the toolkit of parts available and the perception structures characterized, like some inducible promoters or those responsive to stress conditions. An extensive repository of these and other parts is available.1
Gibson assembly (Gibson et al., 2009), founded on PCR-overlap, and Golden Gate assembly (Engler et al., 2008, 2009), founded on type IIS restriction enzymes, are other proposed methodologies, based on one tube reaction. The Golden Gate approach has received considerable interest in plant synthetic biology for providing the joining of several parts at once into a receptor plasmid with relative simplicity. This cloning technique has evolved into modular assemblies enabling to engineer multiple transcriptional units and consists of the aims of MoClo (Engler et al., 2014) and GoldenBraid (Sarrion-Perdigones et al., 2011) strategies. The dissemination of these techniques raised once again the need to standardize the parts used. Thus, researchers defined, in a collective effort, standards for fusion sites of genetic parts for cloning and assembly methods using type IIs enzymes. This guidance, called Common Syntax for eukaryotic parts assembly (Patron et al., 2015), was adopted by iGEM for the so-designated phytobricks. The MoClo and Golden Braid groups developed specific toolkits for plants, which are presented in Table 1. The DBTL (design, build, test and learn) methodology used to establish these kits provides a range of parts that can be easily changed in a construction and contributes to modules’ functionality information for the future development of plant circuits.
The genetic network construction tests are mainly conducted in plant chassis, such as Nicotiana tabacum, Nicotiana benthamiana and Arabidopsis thaliana, especially by transient assays performed using leaves agroinfiltration and BY-2 cells (tobacco) or protoplasts transformation. However, the moss Physcomitrium patens and the liverwort Marchantia polymorpha have also emerged as synthetic biology investigation chassis. Those organisms have completely sequenced genomes, a short life cycle, set laboratory cultivation, and good heterologous protein production. These advantageous features have allowed the specification of some genetic parts and toolkits (Delmans et al., 2017; Reski et al., 2018; Sauret-Güeto et al., 2020).
For a long time, eukaryotic microalgae have been arousing interest regarding their physiology, photosynthetic metabolism, and biotechnological applications, such as biofuels production. Although algae research has been developing molecular tools, the poor expression of heterologous genes from its nuclear genome is a relevant limitation towards this goal, considering the model organism Chlamydomonas reinhardtii (Scaife and Smith, 2016). However, some strategies are overcoming these hurdles: use of specific promoters, mutated strains with altered chromatin condensation, intron sequences, subcellular targeting, adoption of more efficient transformation methodologies, and codon optimization (Lauersen et al., 2015; Scaife and Smith, 2016; Vavitsas et al., 2019). The green alga C. reinhardtii, as well as the diatom Phaeodactylum tricornutum, have been the most explored species as chassis organisms. In this sense, some research groups have developed toolkits aiming to improve the parts’ availability and characterization efforts (Siaut et al., 2007; Scaife and Smith, 2016; Crozet et al., 2018; Butler et al., 2020). Also, as an example, iGEM groups that developed such toolkits are presented at Table 1.
Circuit design and control
The design of a synthetic genetic circuit demands fulfilling several criteria. Although the synthesis of short DNA sequences has become cheaper and more accessible, making it easier for the modular assembly of parts, there are still limitations, such as the maximum fragment size sustained by a plasmid and the incapability of synthesizing high-quality long DNA fragments. There are also drawbacks to the delivery and manipulation of these long sequences and a lack of knowledge of epigenetic chromosomal interactions and mechanisms that control the genome’s three-dimensional structure (Ostrov et al., 2019). Additionally, there are specific needs for synthetic circuit design, as the engineered circuits’ ability to function and integrate into biological systems in a predictable manner, but not suffering undesired endogenous interference or vice-versa. Therefore, synthetic circuits need to use fully characterized biological parts that are independent, reliable, orthogonal, tunable, composable and scalable (Lucks et al., 2008). In this sense, part sequences usually come from other organisms or are artificially designed as hybrid sequences.
Essential elements for creating more effective genetic circuits are precise and efficient gene control expression systems. For plants, following these premises, chemically inducible promoters have been applied using mechanisms from E. coli tetracycline-regulated de-repression, Aspergillus nidulans ethanol induction, and systems of animal steroid receptors activation (Gatz and Quail, 1988; Schena et al., 1991; Caddick et al., 1998).
Furthermore, the search for new transcriptional controllers has guided the design of synthetic regulators. Some research groups fused DNA-binding domains, such as the yeast GAL4 or the bacterium LexA, with the transactivating domain of the herpes viral protein VP16 or with A. thaliana repressor domains. In some cases, a steroid receptor was fused to control the system induction or repression (Weinmann et al., 1994; Aoyama and Chua, 1997; Zuo et al., 2000; Schaumberg et al., 2016). Additionally, an expanded library of synthetic promoters with variable strengths using a range of cis and trans-regulatory elements to control a plant’s minimal promoter expression was built (Belcher et al., 2020). The Neurospora crassa Q-system was also used as transcriptional gene control. The synthetic structure adjusted three components of the original cluster, a transcriptional activator, a repressor, and the inducing molecule quinic acid (Persad et al., 2020). Already in the GB3.0 system (Vazquez-Vilar et al., 2017), the authors developed two transcription control mechanisms. The first uses phiC31 integrase fused to activation (Gal4 or VP64) or repression (Arabidopsis BRD) domains, and the second is based on transcriptional modules from flavonoids biosynthesis, using the Rosea 1 and Delila regulators.
It is worth mentioning the development of optogenetically regulated controllers for plants: the red light responsive split transcriptional system, based on phytochrome B (PhyB) and phytochrome-interacting factor 6 (PIF6) connection (Müller et al., 2014); and the green-light sensitive Thermus thermophilus CarH-CarO (transcription factor-operator) dependent on the stability of coenzyme AdoB12 (Chatelle et al., 2018). Yet, recently, promoters controlled by hormone-activated Cas9-based repressors (HACRs) in response to three plant hormones: auxin, gibberellin, and jasmonate (Khakhar et al., 2018), that unlocks the system by degron disruption, were developed. Besides this, synthetic promoters were built based on dCas9: VP64 and specific gRNAs, with their binding sites positioned upstream of a minimal promoter. It was validated by N. benthamiana infiltration and A. thaliana transgenic plants. The system used an inducible promoter ethylene-responsive to drive gRNAs expression and verified the transcriptional controller’s orthogonality in a multiplex test (Kar et al., 2022).
It is important to note that the biological parts described in some synthetic transcriptional complexes do not comply with all criteria of the biological circuits design principles because they depend on endogenous components. Nevertheless, the above systems allow the genetic toggle switch construction in which genes can exist in two stable states switching from one to another and interacting to form genetic circuits. Post-transcriptional and translational control systems, mainly RNA-based tools, such as RNA interference, microRNAs, ribozymes, or aptamers, were also developed. A comprehensive list of these tools is described elsewhere (Andres et al., 2019).
Another approach to control gene expression is using site-specific recombinases and placing their recognition sites flanking target parts to be rotated. For instance, a recent study showed the functionality of six serine integrases (Ints) to perform the 180° rotation of coding and promoter sequences of the designed genetic switches, thereby controlling the GFP reporter expression (Gomide et al., 2020). A further study used the phiC31 integrase and its cognate protein, the recombination directionality factor (RDF), to switch between activated or deactivated states of the reporter genes by inversion of regulatory parts in N. benthamiana (Bernabé-Orts et al., 2020).
All the above mentioned expression control systems open up uncountable combinatorial possibilities for the genetic circuits designed for plants. One can also oversee that agricultural applications are under interest to activate synthetic genetic networks with identified promoters responsive to biotic and abiotic stress (Singhal et al., 2016; Muthusamy et al., 2017) or synthesized to switch on with fertilization (Crawford et al., 2010).
On the other hand, there was a delay in algae tools development that allow for controlled nuclear gene expression. In C. reinhardtii, until recently, successful results had not been reached, despite testing several endogenous, chimeric, and viral promoters for gene expression (Wang et al., 2012). Nevertheless, some studies could improve transcriptional control, such as the fusion of the HSP70A-RBCS2 promoters that increased endogenous and exogenous nuclear gene expression levels (Schroda et al., 2000). Additionally, Scranton and collaborators identified a range of cis-motifs in highly expressed nuclear genes from C. reinhhardtii and generated a set of novel functional synthetic algal promoters (Scranton et al., 2016). Inducible and repressible promoters were also available. Among them, it is worth listing: the metal-responsive CYC6 promoter, induced by nickel and repressed by copper ions (Ferrante et al., 2008); the METE promoter, repressible by vitamin B12 (Helliwell et al., 2014); the sulfur starvation-induced promoter of LHCBM9 (Sawyer et al., 2015); an light-inducible promoter from Dunaliella sp. (Baek et al., 2016); the salt-inducible promoter from C.reinhardtii GPDH3 gene (Beltran-Aguilar et al., 2019); an alcohol-inducible promoter from A. nidulans (Lee et al., 2018); and the promoters inducible by digoxin and β-estradiol, that were previously effective for other eukaryotic organisms and adapted for P. tricornutum (Kassaw et al., 2022).
Navarro and Baulcombe extended the gene expression control models for C. reinhardtii using fluorescent models to characterize miRNAs for a post-transcriptional switching-off regulation approach (Navarro and Baulcombe, 2019). In that same algae, Mehrshahi and collaborators described another RNA-based tool for controlling gene expression (Mehrshahi et al., 2020). From the endogenous THI4, they developed novel riboswitches that respond to different ligands and can be used to design synthetic genetic circuits (Mehrshahi et al., 2020). Even though the essential toolkits available for synthetic research advancement in algae are still lagging compared to plants, these works represent substantial steps in this direction.
Computational prediction tools that allow the automated design of the synthetic circuits are primordial for plants and algae, particularly advancing from genotype to phenotype prediction. The availability of characterized biological parts, standard assembly approaches, collections of parts, and improvement of testing systems enabled the conception of computer-aided tools. Nevertheless, it must consider the limitations of plant and algae engineering regarding the efficiency and time-consuming of the existing transformation methods. The synthetic circuit tests in simple model chassis also require caution, especially in plants, since these systems’ behavior might not be the same between the testing platforms and the final organism. With all these considerations, some tools and databases are available for the choice and assembly assistance of the experimental genetic parts. More information about these platforms is in Table 1.
Examples of engineered genetic circuits and their biotechnological applications
Over the years, the expansion of synthetic biologic toolkits has allowed the construction of some genetic circuits in plants that generate predictable outputs after input processing in a network manner (Table 1). Considering that the ultimate goal in these organisms is crop improvement, some of the examples of engineered genetic circuits will focus on them. Until now, most of the synthetic genetic circuits developed in crops relate to synthetic metabolic pathways. Some of these pathways aimed to increase carotenoid content, the vitamin A precursors (de Lange et al., 2018). The deficiency of this vitamin results in blindness and increases infectious diseases being a prominent concern in parts of the developing world. These systems had, as input signals, plant and bacterial encoding enzyme genes (phytoene synthase, phytoene desaturase, carotenoid desaturase, lycopene β-cyclase, and/or β-carotene ketolase) presented in several combinatorial manners. Therefore, this approach could overcome the bottlenecks of the carotenoid biosynthesis network in crops such as rice (Paine et al., 2005), potato (Diretto et al., 2007), maize (Zhu et al., 2008), and wheat (Wang et al., 2014), creating high-content carotenoid plants, so-called golden crops. Nonetheless, after years of carotenoid pathway studies, some information on this multi-enzyme complex system still lacks a better understanding of improved metabolic engineering circuit design (Shumskaya and Wurtzel, 2013). Likewise, consumer acceptance of those engineered crops needs to be addressed, as the Golden Rice still awaits further exploration (de Lange et al., 2018).
Concerning examples of complex genetic circuits for plants, there is still a handful of those described in the literature. One of the first circuits fully designed was built to be a plant biosensor capable of detecting 2,4,6-trinitrotoluene (TNT; Antunes et al., 2011). This plant detection system was based on bacterial chemotactic components adapted for plants. The periplasmic binding protein (PBP) was redesigned to recognize TNT as a ligand in the plant cell apoplast. Once connection occurs, the protein remodeling allows the linkage with the chimeric transmembrane transduction signaling protein, Trg-PhoR, whose activation induces the PhoB-VP64. This transcription factor has an affinity for the synthetic promoter PlantPho, which controls a response signal that promotes the loss of the leaves’ green color. The named de-greening circuit was detailed elsewhere (Antunes et al., 2006) and consists of genes that inhibit chlorophyll synthesis and initiate its degradation. Although this work was an important example of complex plant-engineered genetic circuits with biotechnological applications, there were drawbacks and further adjustments will be needed for this plant system to be used as means of detection of contaminants, explosives, or other chemical agents. Soon after, following the same rationale of using plants as biosensors, Czarnecka and collaborators developed a mechanism for plants to detect human bacterial pathogens (Czarnecka et al., 2012). In this work, the strategy relied on a biological genetic switch, based on a transcriptional autofeedback loop. The input signal was the bacterial flagellin that allowed plant protoplasts to amplify their endogenous defense response to pathogens, which would ultimately lead to an output signal of a deteriorated plant, inhibiting its commercialization.
Yet, there were two outstanding advancements in plant synthetic biology research. The first one was the establishment of a transkingdom genetic circuit of plant-dependent synthesis and signaling of rhizopine to control bacteria in the rhizosphere (Geddes et al., 2019). Rhizopines are rare molecules in nature synthesized by a few rhizobia species in legume nodules during N2-fixing symbiosis. This groundbreaking work creates plant control possibilities for specific soil microbiota members to perform essential tasks for crop improvement, such as Nitrogen (N2) fixation and nutrient solubilization. N2 fixation has been a central concern because the chemical nitrogenous fertilizers, which constituted the base for the green revolution, are a limited resource. Its use also results in high water pollution levels and the eutrophication of lakes and rivers (Bano and Sheikh, 2016). The second exciting work was the development of the so-called cyber-spinach. In this study, the researchers used microfluidics to obtain an artificial chloroplast by encapsulating, operating spinach photosynthetic membranes, and combining it with an improved laboratory-designed enzyme pathway, the CETCH cycle version 7.0 (Miller et al., 2020). The light inputs drive the reactions network for the CO2 conversion, leading to the multicarbon compound glycolate as output. This artificial photosynthetic pathway is more efficient than the natural one having potential biotechnological applications. Despite this, several questions remain, such as the system compatibility with the living cell machinery, its long-time lifespan, and the scalability of an economical operation. Focusing on both techniques’ progression, their respective research groups have been improving some of the biological components of those systems (Scheffen et al., 2021; Haskett et al., 2022). Nevertheless, these works can be viewed as the founder stone for several research applications.
Notably, thorough genetic biocircuits following the Boolean logic gates concepts have just been accomplished for plants. Based on CRISPRi (interference), the assembled gates inhibit the transcription initiation using a dCas9 guided by sgRNAs to a target promoter (Khan et al., 2022 – preprint). The second system has constant output signals by circuits working under recombinases control instead of the transitory condition in the previous work. This research used the recombinases Flp, Cre, and B3 to control a luciferase output by excising the promoter, coding, or terminator sequence. Thus, it obeys Boolean logic gates control in Arabidopsis protoplasts or roots from transgenic plants (Lloyd et al., 2022). Another observed progress was for a result with direct interference in plant development. The work tested synthetic transcription regulators compounding Boolean genetic gates to activate or repress gene expression. Significantly, some of the circuits could successfully control root development in A. thaliana transgenic plants (Brophy et al., 2022 – preprint).
In algae, the advancements of synthetic biology toolkits are paving the way for studies aiming to produce important chemical molecules in these organisms (Table 1). Exemplifying, Rasala and collaborators showed that xylanase, an important industrial enzyme, could achieve a relevant augment in C. reinhardtii cell lysates. In this study, C. reinhardtii xyn1 gene expression was coupled with the virus 2A self-cleavage peptide and with an antibiotic selection gene (Rasala et al., 2012). Likewise, as described above for plants, in algae, the synthetic genetic circuits initially developed relate to synthetic metabolic pathways. For decades, it has been an effort toward metabolic engineering of algae to produce chemically diverse isoprenoid molecules due to their importance in medicine, agriculture, cosmetics, biofuels and several other applications (Wang et al., 2012; Wichmann et al., 2018; Butler et al., 2020). Wichmann and collaborators combined a heterologous overexpression of bisabolene synthase genes and repression of squalene synthase gene expression through microRNA, leading to improvement of bisabolene productivity, which is the sesquiterpene biodiesel precursor (Wichmann et al., 2018). However, the authors argue that the capacity for bisabolene production is still below the industrial demands, and improvement in genetic transformation tools is still needed. Further, P. tricornutum has a raised potential as a candidate for terpenoid heterologous synthesis. The photosynthetic background of enzymes and precursors possibly favors the synthesis of these compounds. Thus, geraniol was synthesized in this diatom microalgae and high productivity was studied between genome insertion and episomal expression (Fabris et al., 2020; George et al., 2020).
Perspectives in circuit design to gene regulation in plants and algae artificial chromosomes
Before discussing how genetic circuits could contribute to the control of gene expression in artificial chromosomes, it is essential to define them and to report advancements in this area in plant and eukaryotic algae. Artificial chromosomes are chemically synthesized DNA molecules with a centromere, origins of replication, telomeric regions, and other regulatory parts, mimicking a natural chromosome’s behavior (Murray and Szostak, 1983). Initially aiming to investigate the structural requirements of natural chromosomes within the cell cycle, artificial chromosomes currently have numerous functions, promises, and challenges. It is worth highlighting their importance in genetic essentiality studies (Hutchison et al., 2016) and for constituting safe harbors for targeting transgenes (Kuroiwa et al., 2000; Gaeta et al., 2013). Additionally to being, in the future, the basis for highly adjustable and specialized chassis organisms creation for the biotechnology industry. The first independent artificial chromosomes were constructed in the unicellular organisms Saccharomyces cerevisiae (Murray and Szostak, 1983) and E. coli (O’Connor et al., 1989), known as Yeast Artificial Chromosomes (YACs) and Bacterial Artificial Chromosomes (BACs), respectively. They played a critical role in the eukaryotic genome sequencing and characterization, including in the Human Genome Project (Lander et al., 2001; Venter et al., 2001). With the advancement of DNA synthesis technology and the improvement of genomics, groups and consortia have assembled synthetic chromosomes and genomes. They are similar or reduced to their natural equivalent and capable of replacing them, as in the case of Mycoplasma (Gibson et al., 2010; Hutchison et al., 2016), S. cerevisiae (Annaluru et al., 2014; Richardson et al., 2017), and E. coli (Fredens et al., 2019; Kurokawa and Ying, 2019). In multicellular organisms, the recent advances are in artificial minichromosome approaches, such as those used in human cells, Human Artificial Chromosomes (HACs; Farr et al., 1995; Harrington et al., 1997; Ikeno et al., 1998), and plant, Plant Artificial chromosomes (PACs; Yu et al., 2006, 2007).
Plants and eukaryotic algae are underrepresented in advances in this area. The formation and function of plant centromeres still present significant challenges that limit bottom-up approaches (Carlson et al., 2007; Ananiev et al., 2009), such as the complex epigenetic influence and species-specific sequence repetitions (Han et al., 2006, 2018; Birchler and Han, 2009). Using top-down methods, the telomere-mediated truncation technique (Farr et al., 1992), for instance, has already been used to create artificial minichromosomes in several cultivars and models such as maize (Yu et al., 2006, 2007), rice (Xu et al., 2012), barley (Kapusi et al., 2012), wheat (Yuan et al., 2017), A. thaliana (Nelson et al., 2011; Teo et al., 2011; Murata et al., 2013), and Brassica napus (Yan et al., 2017). In algae, advances are even timider, consisting mainly of the phases that precede the creation of synthetic chromosomes. Highlights include the assembly of a few genomic chromosomes or the genome of algal organelles in host organisms, such as the two P. tricornutum chromosomes assembled in S. cerevisiae (Karas et al., 2013), the assembly of the chloroplast genome of the green alga C. reinhardtii in yeast and transformed into C. reinhardtii (O’Neill et al., 2012), and the synthetic mitochondrial genome of Thalassiosira pseudonana cloned in yeast and E. coli (Cochrane et al., 2020), respectively.
Artificial chromosomes can be ideal Synthetic Biology tools, working as safe harbors for exogenous gene insertion that compose biosynthetic pathways of molecules of interest. Furthermore, the building of new genetic circuits could contribute to refining their expression control. However, it will be necessary to overcome challenges, such as the manipulation difficulties of large DNA fragments (Ostrov et al., 2019) and the low meiotic transmission rate in some organisms (Han et al., 2007; Masonbrink et al., 2012), to establish these chromosomes as a robust approach.
Discussion
As strategies from Synthetic Biology advance, the use of biocircuits to optimize plants and algae for diverse purposes is obviously aimed, and, indeed, substantial advancements have already been achieved. However, intrinsic functional variation of biological compounds often results in synthetic systems performing below expectations. Thus, most researchers still prefer widely used biological parts with well-established functionalities, for example, some constitutive promoters, such as CaMV35S. Nevertheless, such components have limitations on their use in more expanded and regulated arrangements. The functional consolidation of the new parts deposited in current databases by the next generation of research will be fundamental for more effective biocircuits achievements in these species.
Noticeably, technical issues still hamper studies of systems designed for plants and algae considering other model organisms. For example, Cello, a software developed and employed to design 45 successful genetic circuits in E. coli, was also recently used by Chen and coworkers to build functional biocircuits in yeast, taking advantage of the overwhelming knowledge available to these organisms (Nielsen et al., 2016; Chen et al., 2020). Additionally, transient assays are a more straightforward way to test the operability of circuit components and get rapid answers. Nonetheless, even for this approach type, different from microorganisms or organisms with established cell lines, the time for sampled organisms’ preparation is slower and the transformant phenotypes usually exhibit a wide range of variation. Besides, the adaptation needed for analytical protocols and executions is time-consuming. Kits, facility services, and high throughput methods are also less available. Thus, transposing the built system to stable insertion in a final interest organism represents additional difficulties, including the cost–benefit assessment for the investment in the technology.
Many of the genetic constructions desired for plants and algae demand improvement in the knowledge regarding carbon and photosynthetic metabolism. Likewise, it is necessary a better comprehension of the genome organization and its relationship with the exogenous DNA sequences integrated to compound the biocircuit. Finally, understanding the functional relationships in metabolic networks of sequenced genes is still needed in many pathways.
All these challenges emphasize the importance of establishing new genetic parts and regulatory constructs capable of functioning satisfactorily not only in the model chassis but also in the final target organisms. Computational tools can, therefore, make an important contribution to this challenging expansion of viable biological components for construction of functional new genetic circuits. Furthermore, a perspective that also could contribute to mitigating stochastic effects in the biocircuits is direct the designed constructions to genomic safe harbors. Besides a better knowledge of possible safe harbors in the endogenous chromosomes, the synthetic chromosomes are promising structures to insert complex networks to be expressed, once challenges of stabilization and meiotic division are overcome.
Author contributions
CMC and MSG conceptualized the mini-review. MSG, CMC and MCL designed the table. MCL designed the figure. All the authors contributed to the writing and the final revision of this manuscript.
Conflict of interest
The authors declare that the research was conducted in the absence of any commercial or financial relationships that could be construed as a potential conflict of interest.
Publisher’s note
All claims expressed in this article are solely those of the authors and do not necessarily represent those of their affiliated organizations, or those of the publisher, the editors and the reviewers. Any product that may be evaluated in this article, or claim that may be made by its manufacturer, is not guaranteed or endorsed by the publisher.
Footnotes
References
Ananiev, E. V., Wu, C., Chamberlin, M. A., Svitashev, S., Schwartz, C., Gordon-Kamm, W., et al. (2009). Artificial chromosome formation in maize (Zea mays L.). Chromosoma 118, 157–177. doi: 10.1007/s00412-008-0191-3
Andres, J., Blomeier, T., and Zurbriggen, M. D. (2019). Synthetic switches and regulatory circuits in plants. Plant Physiol. 179, 862–884. doi: 10.1104/pp.18.01362
Annaluru, N., Muller, H., Mitchell, L. A., Ramalingam, S., Stracquadanio, G., et al. (2014). Total synthesis of a functional designer eukaryotic chromosome. Science 344, 55–58. doi: 10.1126/science.1249252
Antunes, M. S., Ha, S.-B., Tewari-Singh, N., Morey, K. J., Trofka, A. M., Kugrens, P., et al. (2006). A synthetic de-greening gene circuit provides a reporting system that is remotely detectable and has a re-set capacity. Plant Biotechnol. J. 4, 605–622. doi: 10.1111/j.1467-7652.2006.00205.x
Antunes, M. S., Morey, K. J., Smith, J. J., Albrecht, K. D., Bowen, T. A., Zdunek, J. K., et al. (2011). Programmable ligand detection system in plants through a synthetic signal transduction pathway. PLoS One 6:e16292. doi: 10.1371/journal.pone.0016292
Aoyama, T., and Chua, N. H. (1997). A glucocorticoid-mediated transcriptional induction system in transgenic plants. Plant J. 11, 605–612. doi: 10.1046/j.1365-313X.1997.11030605.x
Baek, K., Lee, Y., Nam, O., Park, S., Sim, S. J., and Jin, E. (2016). Introducing Dunaliella LIP promoter containing light-inducible motifs improves transgenic expression in Chlamydomonas reinhardtii. Biotechnol. J. 11, 384–392. doi: 10.1002/biot.201500269
Bano, S., and Sheikh, M. (2016). Biological nitrogen fixation to improve plant growth and productivity. Int. J. Agric. Innov. Res. 4, 597–599.
Bartley, B., Beal, J., Clancy, K., Misirli, G., Roehner, N., Oberortner, E., et al. (2015). Synthetic biology open language (SBOL) version 2.0.0. J. Integr. Bioinform. 12:272. doi: 10.2390/biecoll-jib-2015-272
Belcher, M. S., Vuu, K. M., Zhou, A., Mansoori, N., Agosto Ramos, A., Thompson, M. G., et al. (2020). Design of orthogonal regulatory systems for modulating gene expression in plants. Nat. Chem. Biol. 16, 857–865. doi: 10.1038/s41589-020-0547-4
Beltran-Aguilar, A. G., Peraza-Echeverria, S., López-Ochoa, L. A., Borges-Argáez, I. C., and Herrera-Valencia, V. A. (2019). A novel salt-inducible CrGPDH3 promoter of the microalga Chlamydomonas reinhardtii for transgene overexpression. Appl. Microbiol. Biotechnol. 103, 3487–3499. doi: 10.1007/s00253-019-09733-y
Bernabé-Orts, J. M., Quijano-Rubio, A., Vazquez-Vilar, M., Mancheño-Bonillo, J., Moles-Casas, V., Selma, S., et al. (2020). A memory switch for plant synthetic biology based on the phage ϕC31 integration system. Nucleic Acids Res. 48, 3379–3394. doi: 10.1093/nar/gkaa104
Birchler, J. A. (2015). Promises and pitfalls of synthetic chromosomes in plants. Trends Biotechnol. 33, 189–194. doi: 10.1016/j.tibtech.2014.12.010
Birchler, J. A., and Han, F. (2009). Maize centromeres: structure, function, epigenetics. Annu. Rev. Genet. 43, 287–303. doi: 10.1146/annurev-genet-102108-134834
Brophy, J. A. N., Magallon, K. J., Kniazev, K., and Dinneny, J. R. (2022). Synthetic genetic circuits enable reprogramming of plant roots. bioRxiv. doi: 10.1101/2022.02.02.478917
Butler, T., Kapoore, R. V., and Vaidyanathan, S. (2020). Phaeodactylum tricornutum: a diatom cell factory. Trends Biotechnol. 38, 606–622. doi: 10.1016/j.tibtech.2019.12.023
Caddick, M. X., Greenland, A. J., Jepson, L., Krause, K.-P., Qu, N., Riddell, K. V., et al. (1998). An ethanol inducible gene switch for plants used to manipulate carbon metabolism. Nat. Biotechnol. 16, 177–180. doi: 10.1038/nbt0298-177
Cameron, D. E., Bashor, C. J., and Collins, J. J. (2014). A brief history of synthetic biology. Nat. Rev. Microbiol. 12, 381–390. doi: 10.1038/nrmicro3239
Carlson, S. R., Rudgers, G. W., Zieler, H., Mach, J. M., Luo, S., Grunden, E., et al. (2007). Meiotic transmission of an in vitro-assembled autonomous maize minichromosome. PLoS Genet. 3, 1965–1974. doi: 10.1371/journal.pgen.0030179
Chatelle, C., Ochoa-Fernandez, R., Engesser, R., Schneider, N., Beyer, H. M., Jones, A. R., et al. (2018). A green-light-responsive system for the control of transgene expression in mammalian and plant cells. ACS Synth. Biol. 7, 1349–1358. doi: 10.1021/acssynbio.7b00450
Chen, Y., Zhang, S., Young, E. M., Jones, T. S., Densmore, D., and Voigt, C. A. (2020). Genetic circuit design automation for yeast. Nat. Microbiol. 5, 1349–1360. doi: 10.1038/s41564-020-0757-2
Cochrane, R. R., Brumwell, S. L., Shrestha, A., Giguere, D. J., Hamadache, S., Gloor, G. B., et al. (2020). Cloning of thalassiosira pseudonana’s mitochondrial genome in saccharomyces cerevisiae and escherichia coli. Biology 9, 1–15. doi: 10.3390/biology9110358
Crawford, N., Wang, R., Guan, P., and Chen, M. (2010). Nitrate-responsive promoter. Worldwide applications No WO2011028929A2. WIPO - World Intellectual Property Organization (Patent Cooperation Treaty - PCT).
Crozet, P., Navarro, F. J., Willmund, F., Mehrshahi, P., Bakowski, K., Lauersen, K. J., et al. (2018). Birth of a photosynthetic chassis: a MoClo toolkit enabling synthetic biology in the microalga Chlamydomonas reinhardtii. ACS Synth. Biol. 7, 2074–2086. doi: 10.1021/acssynbio.8b00251
Czarnecka, E., Verner, F. L., and Gurley, W. B. (2012). A strategy for building an amplified transcriptional switch to detect bacterial contamination of plants. Plant Mol. Biol. 78, 59–75. doi: 10.1007/s11103-011-9845-2
Dasika, M. S., and Maranas, C. D. (2008). OptCircuit: An optimization based method for computational design of genetic circuits. BMC Syst. Biol. 2:24. doi: 10.1186/1752-0509-2-24
de Lange, O., Klavins, E., and Nemhauser, J. (2018). Synthetic genetic circuits in crop plants. Curr. Opin. Biotechnol. 49, 16–22. doi: 10.1016/j.copbio.2017.07.003
Delmans, M., Pollak, B., and Haseloff, J. (2017). MarpoDB: an open registry for Marchantia polymorpha genetic parts. Plant Cell Physiol. 58:e5. doi: 10.1093/pcp/pcw201
Diretto, G., Al-Babili, S., Tavazza, R., Papacchioli, V., Beyer, P., and Giuliano, G. (2007). Metabolic engineering of potato carotenoid content through tuber-specific overexpression of a bacterial mini-pathway. PLoS One 2, 1–8. doi: 10.1371/journal.pone.0000350
Elowitz, M. B., and Leibler, S. (2000). A synthetic oscillatory network of transcriptional regulators. Nature 403, 335–338. doi: 10.1038/35002125
Engler, C., Gruetzner, R., Kandzia, R., and Marillonnet, S. (2009). Golden Gate shuffling: a one-pot DNA shuffling method based on type IIs restriction enzymes. PLoS One 4:e5553. doi: 10.1371/journal.pone.0005553
Engler, C., Kandzia, R., and Marillonnet, S. (2008). A one pot, one step, precision cloning method with high throughput capability. PLoS One 3:e3647. doi: 10.1371/journal.pone.0003647
Engler, C., Youles, M., Gruetzner, R., Ehnert, T.-M., Werner, S., Jones, J. D. G., et al. (2014). A golden gate modular cloning toolbox for plants. ACS Synth. Biol. 3, 839–843. doi: 10.1021/sb4001504
Estornell, L. H., Orzáez, D., López-Peña, L., Pineda, B., Antón, M. T., Moreno, V., et al. (2009). A multisite gateway-based toolkit for targeted gene expression and hairpin RNA silencing in tomato fruits. Plant Biotechnol. J. 7, 298–309. doi: 10.1111/j.1467-7652.2009.00402.x
Fabris, M., George, J., Kuzhiumparambil, U., Lawson, C. A., Jaramillo-Madrid, A. C., Abbriano, R. M., et al. (2020). Extrachromosomal genetic engineering of the marine diatom Phaeodactylum tricornutum enables the heterologous production of monoterpenoids. ACS Synth. Biol. 9, 598–612. doi: 10.1021/acssynbio.9b00455
Farr, C. J., Bayne, R. A. L., Kipling, D., Mills, W., Critcher, R., and Cooke, H. J. (1995). Generation of a human X-derived minichromosome using telomere-associated chromosome fragmentation. EMBO J. 14, 5444–5454. doi: 10.1002/j.1460-2075.1995.tb00228.x
Farr, C. J., Stevanovic, M., Thomson, E. J., Goodfellow, P. N., and Cooke, H. J. (1992). Telomere-associated chromosome fragmentation: applications in genome manipulation and analysis. Nat. Genet. 2, 275–282. doi: 10.1038/ng1292-275
Ferrante, P., Catalanotti, C., Bonente, G., and Giuliano, G. (2008). An optimized, chemically regulated gene expression system for Chlamydomonas. PLoS One 3:e3200. doi: 10.1371/journal.pone.0003200
Fredens, J., Wang, K., de la Torre, D., Funke, L. F. H., Robertson, W. E., Christova, Y., et al. (2019). Total synthesis of Escherichia coli with a recoded genome. Nature 569, 514–518. doi: 10.1038/s41586-019-1192-5
Gaeta, R. T., Masonbrink, R. E., Zhao, C., Sanyal, A., Krishnaswamy, L., and Birchler, J. A. (2013). In vivo modification of a maize engineered minichromosome. Chromosoma 122, 221–232. doi: 10.1007/s00412-013-0403-3
Gantner, J., Ordon, J., Ilse, T., Kretschmer, C., Gruetzner, R., Löfke, C., et al. (2018). Peripheral infrastructure vectors and an extended set of plant parts for the modular cloning system. PLoS One 13:e0197185. doi: 10.1371/journal.pone.0197185
Gardner, T. S., Cantor, C. R., and Collins, J. J. (2000). Construction of a genetic toggle switch in Escherichia coli. Nature 403, 339–342. doi: 10.1038/35002131
Gatz, C., and Quail, P. H. (1988). Tn10-encoded tet repressor can regulate an operator-containing plant promoter. Proc. Natl. Acad. Sci. U. S. A. 85, 1394–1397. doi: 10.1073/pnas.85.5.1394
Geddes, B. A., Paramasivan, P., Joffrin, A., Thompson, A. L., Christensen, K., Jorrin, B., et al. (2019). Engineering transkingdom signalling in plants to control gene expression in rhizosphere bacteria. Nat. Commun. 10:3430. doi: 10.1038/s41467-019-10882-x
George, J., Kahlke, T., Abbriano, R. M., Kuzhiumparambil, U., Ralph, P. J., and Fabris, M. (2020). Metabolic engineering strategies in diatoms reveal unique phenotypes and genetic configurations with implications for algal genetics and synthetic biology. Front. Bioeng. Biotechnol. 8, 1–19. doi: 10.3389/fbioe.2020.00513
Gibson, D. G., Glass, J. I., Lartigue, C., Noskov, V. N., Chuang, R.-Y., Algire, M. A., et al. (2010). Creation of a bacterial cell controlled by a chemically synthesized genome. Science 329, 52–56. doi: 10.1126/science.1190719
Gibson, D. G., Young, L., Chuang, R.-Y., Venter, J. C., Hutchison, C. A., and Smith, H. O. (2009). Enzymatic assembly of DNA molecules up to several hundred kilobases. Nat. Methods 6, 343–345. doi: 10.1038/nmeth.1318
Gomide, M. S., Sales, T. T., Barros, L. R. C., Limia, C. G., de Oliveira, M. A., Florentino, L. H., et al. (2020). Genetic switches designed for eukaryotic cells and controlled by serine integrases. Commun. Biol. 3:255. doi: 10.1038/s42003-020-0971-8
Goodsell, D. S., Autin, L., and Olson, A. J. (2019). Illustrate: software for biomolecular illustration. Structure 27, 1716–1720.e1. doi: 10.1016/j.str.2019.08.011
Goodwin, B. C. (1965). Oscillatory behavior in enzymatic control processes. Adv. Enzym. Regul. 3, 425–437. doi: 10.1016/0065-2571(65)90067-1
Hahn, F., Korolev, A., Sanjurjo Loures, L., and Nekrasov, V. (2020). A modular cloning toolkit for genome editing in plants. BMC Plant Biol. 20:179. doi: 10.1186/s12870-020-02388-2
Han, F., Gao, Z., Yu, W., and Birchler, J. A. (2007). Minichromosome analysis of chromosome pairing, disjunction, and sister chromatid cohesion in maize. Plant Cell 19, 3853–3863. doi: 10.1105/tpc.107.055905
Han, F., Lamb, J. C., and Birchler, J. A. (2006). High frequency of centromere inactivation resulting in stable dicentric chromosomes of maize. Proc. Natl. Acad. Sci. U. S. A. 103, 3238–3243. doi: 10.1073/pnas.0509650103
Han, F., Lamb, J. C., McCaw, M. E., Gao, Z., Zhang, B., Swyers, N. C., et al. (2018). Meiotic studies on combinations of chromosomes with different sized centromeres in maize. Front. Plant Sci. 9:785. doi: 10.3389/fpls.2018.00785
Harrington, J. J., Van Bokkelen, G., Mays, R. W., Gustashaw, K., and Willard, H. F. (1997). Formation of de novo centromeres and construction of first-generation human artificial microchromosomes. Nat. Genet. 15, 345–355. doi: 10.1038/ng0497-345
Haskett, T. L., Paramasivan, P., Mendes, M. D., Green, P., Geddes, B. A., Knights, H. E., et al. (2022). Engineered plant control of associative nitrogen fixation. Proc. Natl. Acad. Sci. U. S. A. 119:e2117465119. doi: 10.1073/pnas.2117465119
Helliwell, K. E., Scaife, M. A., Sasso, S., Araujo, A. P. U., Purton, S., and Smith, A. G. (2014). Unraveling vitamin B 12-responsive gene regulation in algae. Plant Physiol. 165, 388–397. doi: 10.1104/pp.113.234369
Hutchison, C. A., Chuang, R.-Y., Noskov, V. N., Assad-Garcia, N., Deerinck, T. J., Ellisman, M. H., et al. (2016). Design and synthesis of a minimal bacterial genome. Science 351:aad6253–aad6253. doi: 10.1126/science.aad6253
Ikeno, M., Grimes, B., Okazaki, T., Nakano, M., Saitoh, K., Hoshino, H., et al. (1998). Construction of YAC–based mammalian artificial chromosomes. Nat. Biotechnol. 16, 431–439. doi: 10.1038/nbt0598-431
Jones, T. S., Oliveira, S. M. D., Myers, C. J., Voigt, C. A., and Densmore, D. (2022). Genetic circuit design automation with cello 2.0. Nat. Protoc. 17, 1097–1113. doi: 10.1038/s41596-021-00675-2
Kapusi, E., Ma, L., Teo, C. H., Hensel, G., Himmelbach, A., Schubert, I., et al. (2012). Telomere-mediated truncation of barley chromosomes. Chromosoma 121, 181–190. doi: 10.1007/s00412-011-0351-8
Kar, S., Bordiya, Y., Rodriguez, N., Kim, J., Gardner, E. C., Gollihar, J. D., et al. (2022). Orthogonal control of gene expression in plants using synthetic promoters and CRISPR-based transcription factors. Plant Methods 18:42. doi: 10.1186/s13007-022-00867-1
Karas, B. J., Molparia, B., Jablanovic, J., Hermann, W. J., Lin, Y. C., Dupont, C. L., et al. (2013). Assembly of eukaryotic algal chromosomes in yeast. J. Biol. Eng. 7, 1–12. doi: 10.1186/1754-1611-7-30
Kassaw, T. K., Paton, A. J., and Peers, G. (2022). Episome-based gene expression modulation platform in the model diatom Phaeodactylum tricornutum. ACS Synth. Biol. 11, 191–204. doi: 10.1021/acssynbio.1c00367
Kauffman, S. A. (1969). Metabolic stability and epigenesis in randomly constructed genetic nets. J. Theor. Biol. 22, 437–467. doi: 10.1016/0022-5193(69)90015-0
Khakhar, A., Leydon, A. R., Lemmex, A. C., Klavins, E., and Nemhauser, J. L. (2018). Synthetic hormone-responsive transcription factors can monitor and re-program plant development. elife 7, 1–16. doi: 10.7554/eLife.34702
Khan, M. A., Herring, G., Oliva, M., Fourie, E., Zhu, J. Y., Johnston, B., et al. (2022). CRISPRi-based circuits for genetic computation in plants. bioRxiv. doi: 10.1101/2022.07.01.498372
Knight, T. (2003). Idempotent Vector Design for Standard Assembly of Biobricks. Available at: http://hdl.handle.net/1721.1/21168 (Assessed May 12, 2022).
Kuroiwa, Y., Tomizuka, K., Shinohara, T., Kazuki, Y., Yoshida, H., Ohguma, A., et al. (2000). Manipulation of human minichromosomes to carry greater than megabase-sized chromosome inserts. Nat. Biotechnol. 18, 1086–1090. doi: 10.1038/80287
Kurokawa, M., and Ying, B.-W. (2019). Experimental challenges for reduced genomes: the cell model Escherichia coli. Microorganisms 8:3. doi: 10.3390/microorganisms8010003
Lander, E. S., Linton, L. M., Birren, B., Nusbaum, C., Zody, M. C., Baldwin, J., et al. (2001). Initial sequencing and analysis of the human genome. Nature 409, 860–921. doi: 10.1038/35057062
Lauersen, K. J., Kruse, O., and Mussgnug, J. H. (2015). Targeted expression of nuclear transgenes in Chlamydomonas reinhardtii with a versatile, modular vector toolkit. Appl. Microbiol. Biotechnol. 99, 3491–3503. doi: 10.1007/s00253-014-6354-7
Lee, S., Lee, Y. J., Choi, S., Park, S.-B., Tran, Q.-G., Heo, J., et al. (2018). Development of an alcohol-inducible gene expression system for recombinant protein expression in Chlamydomonas reinhardtii. J. Appl. Phycol. 30, 2297–2304. doi: 10.1007/s10811-018-1480-8
Lloyd, J. P. B., Ly, F., Gong, P., Pflueger, J., Swain, T., Pflueger, C., et al. (2022). Synthetic memory circuits for stable cell reprogramming in plants. Nat. Biotechnol. 1–11. doi: 10.1038/s41587-022-01383-2
Lucks, J. B., Qi, L., Whitaker, W. R., and Arkin, A. P. (2008). Toward scalable parts families for predictable design of biological circuits. Curr. Opin. Microbiol. 11, 567–573. doi: 10.1016/j.mib.2008.10.002
Masonbrink, R. E., Gaeta, R. T., and Birchler, J. A. (2012). Multiple maize minichromosomes in meiosis. Chromosom. Res. 20, 395–402. doi: 10.1007/s10577-012-9283-2
McLaughlin, J. A., Beal, J., Mısırlı, G., Grünberg, R., Bartley, B. A., Scott-Brown, J., et al. (2020). The synthetic biology open language (SBOL) version 3: simplified data exchange for bioengineering. Front. Bioeng. Biotechnol. 8, 1–15. doi: 10.3389/fbioe.2020.01009
Mehrshahi, P., Nguyen, G. T. D. T., Gorchs Rovira, A., Sayer, A., Llavero-Pasquina, M., Lim Huei Sin, M., et al. (2020). Development of novel Riboswitches for synthetic biology in the Green alga Chlamydomonas. ACS Synth. Biol. 9, 1406–1417. doi: 10.1021/acssynbio.0c00082
Miller, T. E., Beneyton, T., Schwander, T., Diehl, C., Girault, M., McLean, R., et al. (2020). Light-powered CO2 fixation in a chloroplast mimic with natural and synthetic parts. Science 368, 649–654. doi: 10.1126/science.aaz6802
Miyamoto, T., Razavi, S., DeRose, R., and Inoue, T. (2013). Synthesizing biomolecule-based Boolean logic gates. ACS Synth. Biol. 2, 72–82. doi: 10.1021/sb3001112
Monod, J., and Jacob, F. (1961). General conclusions: teleonomic mechanisms in cellular metabolism, growth, and differentiation. Cold Spring Harb. Symp. Quant. Biol. 26, 389–401. doi: 10.1101/SQB.1961.026.01.048
Müller, K., Siegel, D., Rodriguez Jahnke, F., Gerrer, K., Wend, S., Decker, E. L., et al. (2014). A red light-controlled synthetic gene expression switch for plant systems. Mol. BioSyst. 10, 1679–1688. doi: 10.1039/C3MB70579J
Murata, M., Shibata, F., Hironaka, A., Kashihara, K., Fujimoto, S., Yokota, E., et al. (2013). Generation of an artificial ring chromosome in Arabidopsis by Cre/LoxP-mediated recombination. Plant J. 74, 363–371. doi: 10.1111/tpj.12128
Murray, A. W., and Szostak, J. W. (1983). Construction of artificial chromosomes in yeast. Nature 305, 189–193. doi: 10.1038/305189a0
Muthusamy, S. K., Sivalingam, P., Sridha, J., Singh, D., Haldhar, S., and Kaushal, P. (2017). Biotic stress inducible promoters in crop plants-a review. J. Agric. Ecol. 4, 14–24. doi: 10.53911/JAE.2017.4202
Navarro, F. J., and Baulcombe, D. C. (2019). MiRNA-mediated regulation of synthetic gene circuits in the green alga Chlamydomonas reinhardtii. ACS Synth. Biol. 8, 358–370. doi: 10.1021/acssynbio.8b00393
Nelson, A. D., Lamb, J. C., Kobrossly, P. S., and Shippen, D. E. (2011). Parameters affecting telomere-mediated chromosomal truncation in Arabidopsis. Plant Cell 23, 2263–2272. doi: 10.1105/tpc.111.086017
Nguyen, T., Jones, T. S., Fontanarrosa, P., Mante, J. V., Zundel, Z., Densmore, D., et al. (2019). Design of asynchronous genetic circuits. Proc. IEEE 107, 1356–1368. doi: 10.1109/JPROC.2019.2916057
Nielsen, A. A. K., Der, B. S., Shin, J., Vaidyanathan, P., Paralanov, V., Strychalski, E. A., et al. (2016). Genetic circuit design automation. Science 352:aac7341. doi: 10.1126/science.aac7341
O’Connor, M., Peifer, M., and Bender, W. (1989). Construction of large DNA segments in Escherichia coli. Science 244, 1307–1312. doi: 10.1126/science.2660262
O’Neill, B. M., Mikkelson, K. L., Gutierrez, N. M., Cunningham, J. L., Wolff, K. L., Szyjka, S. J., et al. (2012). An exogenous chloroplast genome for complex sequence manipulation in algae. Nucleic Acids Res. 40, 2782–2792. doi: 10.1093/nar/gkr1008
Ostrov, N., Beal, J., Ellis, T., Gordon, D. B., Karas, B. J., Lee, H. H., et al. (2019). Technological challenges and milestones for writing genomes. Science 366, 310–312. doi: 10.1126/science.aay0339
Paine, J. A., Shipton, C. A., Chaggar, S., Howells, R. M., Kennedy, M. J., Vernon, G., et al. (2005). Improving the nutritional value of Golden Rice through increased pro-vitamin a content. Nat. Biotechnol. 23, 482–487. doi: 10.1038/nbt1082
Patron, N. J., Orzaez, D., Marillonnet, S., Warzecha, H., Matthewman, C., Youles, M., et al. (2015). Standards for plant synthetic biology: a common syntax for exchange of DNA parts. New Phytol. 208, 13–19. doi: 10.1111/nph.13532
Persad, R., Reuter, D. N., Dice, L. T., Nguyen, M.-A., Rigoulot, S. B., Layton, J. S., et al. (2020). The Q-system as a synthetic transcriptional regulator in plants. Front. Plant Sci. 11, 1–12. doi: 10.3389/fpls.2020.00245
Rasala, B. A., Lee, P. A., Shen, Z., Briggs, S. P., Mendez, M., and Mayfield, S. P. (2012). Robust expression and secretion of Xylanase1 in Chlamydomonas reinhardtii by fusion to a selection gene and processing with the FMDV 2A peptide. PLoS One 7:e43349. doi: 10.1371/journal.pone.0043349
Reski, R., Bae, H., and Simonsen, H. T. (2018). Physcomitrella patens, a versatile synthetic biology chassis. Plant Cell Rep. 37, 1409–1417. doi: 10.1007/s00299-018-2293-6
Richardson, S. M., Mitchell, L. A., Stracquadanio, G., Yang, K., Dymond, J. S., DiCarlo, J. E., et al. (2017). Design of a synthetic yeast genome. Science 355, 1040–1044. doi: 10.1126/science.aaf4557
Roehner, N., and Myers, C. J. (2014). Directed acyclic graph-based technology mapping of genetic circuit models. ACS Synth. Biol. 3, 543–555. doi: 10.1021/sb400135t
Sarrion-Perdigones, A., Falconi, E. E., Zandalinas, S. I., Juárez, P., Fernández-del-Carmen, A., Granell, A., et al. (2011). GoldenBraid: an iterative cloning system for standardized assembly of reusable genetic modules. PLoS One 6:e21622. doi: 10.1371/journal.pone.0021622
Sarrion-Perdigones, A., Vazquez-Vilar, M., Palaci, J., Castelijns, B., Forment, J., Ziarsolo, P., et al. (2013). GoldenBraid 2.0: a comprehensive DNA assembly framework for plant synthetic biology. Plant Physiol. 162, 1618–1631. doi: 10.1104/pp.113.217661
Sauret-Güeto, S., Frangedakis, E., Silvestri, L., Rebmann, M., Tomaselli, M., Markel, K., et al. (2020). Systematic tools for reprogramming plant gene expression in a simple model, Marchantia polymorpha. ACS Synth. Biol. 9, 864–882. doi: 10.1021/acssynbio.9b00511
Sawyer, A. L., Hankamer, B. D., and Ross, I. L. (2015). Sulphur responsiveness of the Chlamydomonas reinhardtii LHCBM9 promoter. Planta 241, 1287–1302. doi: 10.1007/s00425-015-2249-9
Scaife, M. A., and Smith, A. G. (2016). Towards developing algal synthetic biology. Biochem. Soc. Trans. 44, 716–722. doi: 10.1042/BST20160061
Schaumberg, K. A., Antunes, M. S., Kassaw, T. K., Xu, W., Zalewski, C. S., Medford, J. I., et al. (2016). Quantitative characterization of genetic parts and circuits for plant synthetic biology. Nat. Methods 13, 94–100. doi: 10.1038/nmeth.3659
Scheffen, M., Marchal, D. G., Beneyton, T., Schuller, S. K., Klose, M., Diehl, C., et al. (2021). A new-to-nature carboxylation module to improve natural and synthetic CO2 fixation. Nat. Catal. 4, 105–115. doi: 10.1038/s41929-020-00557-y
Schena, M., Lloyd, A. M., and Davis, R. W. (1991). A steroid-inducible gene expression system for plant cells. Proc. Natl. Acad. Sci. U. S. A. 88, 10421–10425. doi: 10.1073/pnas.88.23.10421
Schroda, M., Blocker, D., and Beck, C. F. (2000). The HSP70A promoter as a tool for the improved expression of transgenes in Chlamydomonas. Plant J. 21, 121–131. doi: 10.1046/j.1365-313x.2000.00652.x
Scranton, M. A., Ostrand, J. T., Georgianna, D. R., Lofgren, S. M., Li, D., Ellis, R. C., et al. (2016). Synthetic promoters capable of driving robust nuclear gene expression in the green alga Chlamydomonas reinhardtii. Algal Res. 15, 135–142. doi: 10.1016/j.algal.2016.02.011
Shumskaya, M., and Wurtzel, E. T. (2013). The carotenoid biosynthetic pathway: thinking in all dimensions. Plant Sci. 208, 58–63. doi: 10.1016/j.plantsci.2013.03.012
Siaut, M., Heijde, M., Mangogna, M., Montsant, A., Coesel, S., Allen, A., et al. (2007). Molecular toolbox for studying diatom biology in Phaeodactylum tricornutum. Gene 406, 23–35. doi: 10.1016/j.gene.2007.05.022
Singhal, P., Jan, A. T., Azam, M., and Haq, Q. M. R. (2016). Plant abiotic stress: a prospective strategy of exploiting promoters as alternative to overcome the escalating burden. Front. Life Sci. 9, 52–63. doi: 10.1080/21553769.2015.1077478
Sugita, M. (1975). Functional analysis of chemical systems in vivo using a logical circuit equivalent. J. Theor. Biol. 53, 223–237. doi: 10.1016/0022-5193(75)90113-7
Teo, C. H., Ma, L., Kapusi, E., Hensel, G., Kumlehn, J., Schubert, I., et al. (2011). Induction of telomere-mediated chromosomal truncation and stability of truncated chromosomes in Arabidopsis thaliana. Plant J. 68, 28–39. doi: 10.1111/j.1365-313X.2011.04662.x
Thomas, R. (1973). Boolean formalization of genetic control circuits. J. Theor. Biol. 42, 563–585. doi: 10.1016/0022-5193(73)90247-6
Vavitsas, K., Crozet, P., Vinde, M. H., Davies, F., Lemaire, S. D., and Vickers, C. E. (2019). The synthetic biology toolkit for photosynthetic microorganisms. Plant Physiol. 181, 14–27. doi: 10.1104/pp.19.00345
Vazquez-Vilar, M., Quijano-Rubio, A., Fernandez-del-Carmen, A., Sarrion-Perdigones, A., Ochoa-Fernandez, R., Ziarsolo, P., et al. (2017). GB3.0: a platform for plant bio-design that connects functional DNA elements with associated biological data. Nucleic Acids Res. 45, gkw1326–gkw2209. doi: 10.1093/nar/gkw1326
Venter, J. C., Adams, M. D., Myers, E. W., Li, P. W., Mural, R. J., Sutton, G. G., et al. (2001). The sequence of the human genome. Science 291, 1304–1351. doi: 10.1126/science.1058040
Wang, B., Wang, J., Zhang, W., and Meldrum, D. R. (2012). Application of synthetic biology in cyanobacteria and algae. Front. Microbiol. 3, 1–15. doi: 10.3389/fmicb.2012.00344
Wang, C., Zeng, J., Li, Y., Hu, W., Chen, L., Miao, Y., et al. (2014). Enrichment of provitamin a content in wheat (Triticum aestivum L.) by introduction of the bacterial carotenoid biosynthetic genes CrtB and CrtI. J. Exp. Bot. 65, 2545–2556. doi: 10.1093/jxb/eru138
Weeding, E., Houle, J., and Kaznessis, Y. N. (2010). SynBioSS designer: a web-based tool for the automated generation of kinetic models for synthetic biological constructs. Brief. Bioinform. 11, 394–402. doi: 10.1093/bib/bbq002
Weinmann, P., Gossen, M., Hillen, W., Bujard, H., and Gatz, C. (1994). A chimeric transactivator allows tetracycline-responsive gene expression in whole plants. Plant J. 5, 559–569. doi: 10.1046/j.1365-313X.1994.5040559.x
Wichmann, J., Baier, T., Wentnagel, E., Lauersen, K. J., and Kruse, O. (2018). Tailored carbon partitioning for phototrophic production of (E)-α-bisabolene from the green microalga Chlamydomonas reinhardtii. Metab. Eng. 45, 211–222. doi: 10.1016/j.ymben.2017.12.010
Wieland, M., and Fussenegger, M. (2012). Engineering molecular circuits using synthetic biology in mammalian cells. Annu. Rev. Chem. Biomol. Eng. 3, 209–234. doi: 10.1146/annurev-chembioeng-061010-114145
Xu, C., Cheng, Z., and Yu, W. (2012). Construction of rice mini-chromosomes by telomere-mediated chromosomal truncation. Plant J. 70, 1070–1079. doi: 10.1111/j.1365-313X.2012.04916.x
Yan, X., Li, C., Yang, J., Wang, L., Jiang, C., and Wei, W. (2017). Induction of telomere-mediated chromosomal truncation and behavior of truncated chromosomes in Brassica napus. Plant J. 91, 700–713. doi: 10.1111/tpj.13598
Yang, K., Stracquadanio, G., Luo, J., Boeke, J. D., and Bader, J. S. (2016). BioPartsBuilder: a synthetic biology tool for combinatorial assembly of biological parts. Bioinformatics 32, 937–939. doi: 10.1093/bioinformatics/btv664
Yu, W., Han, F., Gao, Z., Vega, J. M., and Birchler, J. A. (2007). Construction and behavior of engineered minichromosomes in maize. Proc. Natl. Acad. Sci. U. S. A 104, 8924–8929. doi: 10.1073/pnas.0700932104
Yu, W., Lamb, J. C., Han, F., and Birchler, J. A. (2006). Telomere-mediated chromosomal truncation in maize. Proc. Natl. Acad. Sci. U. S. A. 103, 17331–17336. doi: 10.1073/pnas.0605750103
Yuan, J., Shi, Q., Guo, X., Liu, Y., Su, H., Guo, X., et al. (2017). Site-specific transfer of chromosomal segments and genes in wheat engineered chromosomes. J. Genet. Genomics 44, 531–539. doi: 10.1016/j.jgg.2017.08.005
Zhu, C., Naqvi, S., Breitenbach, J., Sandmann, G., Christou, P., and Capell, T. (2008). Combinatorial genetic transformation generates a library of metabolic phenotypes for the carotenoid pathway in maize. Proc. Natl. Acad. Sci. U. S. A. 105, 18232–18237. doi: 10.1073/pnas.0809737105
Keywords: biocircuits, plants, eukaryotic algae, synthetic chromosomes, synthetic biology
Citation: Gomide MS, Leitão MC and Coelho CM (2022) Biocircuits in plants and eukaryotic algae. Front. Plant Sci. 13:982959. doi: 10.3389/fpls.2022.982959
Edited by:
Weichang Yu, Shenzhen University, ChinaReviewed by:
Vivek Ambastha, Washington University in St. Louis, United StatesJiangxin Wang, Shenzhen University, China
Copyright © 2022 Gomide, Leitão and Coelho. This is an open-access article distributed under the terms of the Creative Commons Attribution License (CC BY). The use, distribution or reproduction in other forums is permitted, provided the original author(s) and the copyright owner(s) are credited and that the original publication in this journal is cited, in accordance with accepted academic practice. No use, distribution or reproduction is permitted which does not comply with these terms.
*Correspondence: Cíntia Marques Coelho, Y2ludGlhY29lbGhvbUB1bmIuYnI=