- 1Department of Neurobiology, Physiology, and Behavior, University of California, Davis, Davis, CA, United States
- 2Department of Integrative Biology, University of California, Berkeley, Berkeley, CA, United States
Macroalgae provide food and habitat to a diversity of organisms in marine systems, so structural damage and breakage of thallus tissue can have important ecological consequences for the composition and dynamics of marine communities. Common sources of macroalgal damage include breakage by hydrodynamic forces imposed by ambient water currents and waves, tissue consumption by herbivores, and injuries due to epibionts. Many macroalgal species have biomechanical designs that minimize damage by these sources, such as flexibly reconfiguring into streamlined shapes in flow, having either strong or extensible tissues that are tough, and having chemical and morphological defenses against herbivores and epibionts. If damage occurs, some macroalgae have tissue properties that prevent cracks from propagating or that facilitate tissue breakage in certain places, allowing the remainder of the thallus to survive. In contrast to these mechanisms of damage control, some macroalgae use breakage to aid dispersal, while others simply complete their reproduction prior to seasonally-predictable periods of damage (e.g., storm seasons). Once damage occurs, macroalgae have a variety of biomechanical responses, including increasing tissue strength, thickening support structures, or altering thallus shape. Thus, macroalgae have myriad biomechanical strategies for preventing, controlling, and responding to structural damage that can occur throughout their lives.
Introduction
Macroalgae play critical roles in marine ecosystems (Steneck et al., 2002; Schiel and Foster, 2015), so damage that alters their size or morphology can have serious ecological consequences. For example, large macroalgae provide more habitat space and resources for the diverse organisms that live on or amongst their fronds than do small seaweeds (Steneck et al., 2002; Graham et al., 2007; Christie et al., 2009). Large, highly-branched macroalgae also shape surrounding benthic communities by intercepting light, changing sedimentation patterns, and scouring nearby organisms off the substratum (Kennelly, 1989; Arkema et al., 2009; Hughes, 2010). Furthermore, aggregations of macroalgae alter ambient water flow (slowing currents, attenuating waves, altering turbulence spectra), thereby protecting organisms and shorelines from hydrodynamic damage (Denny, 2021; Zhu et al., 2021b; Koehl, 2022).
The ecological effects of damage to macroalgae depend on which species are injured, which parts of their thalli are harmed, and the scale of the damage. For instance, herbivores may eat only certain species (e.g., Toth and Pavia, 2002), life stages (e.g., Van Alstyne et al., 2001; Chenelot and Konar, 2007), or specific macroalgal structures (e.g., Fralick et al., 1974), while seasonal storms rip away some species and sizes of macroalgae more than others (Black, 1976; Koehl, 1999, 2022). Minor damage to macroalgae diminishes provision of food and habitat, whereas major damage disrupts community structure (Johnson and Mann, 1986; Chenelot and Konar, 2007; Poore et al., 2014). However, periodic breakage of competitively-dominant macroalgae enhances local biodiversity (Sousa, 1979). Furthermore, broken macroalgae become organic detritus that enriches benthic communities (Duggins and Eckman, 1997; Krumhansl and Scheibling, 2012; de Bettignies et al., 2013b).
We consider damage to macroalgae through the lens of ecological biomechanics. Biomechanics is the study of how biological structures perform mechanical functions. The integration of biomechanics and ecology (“ecological biomechanics,” Koehl, 1999; “ecomechanics,” Denny, 2012; Higham et al., 2021; “mechanical ecology,” Bauer et al., 2020) provides an ideal framework to study macroalgal damage that incorporates sources of injury in the environment, structural design and tissue material properties that resist or compensate for damage, and effects of morphological changes caused by breakage on the performance of the macroalgae in natural habitats, and thus on their survival and reproduction.
Sources of damage
Hydrodynamic forces
Macroalgae encounter currents and waves. This water motion benefits macroalgae by delivering nutrients, removing wastes, and dispersing gametes and spores (Norton et al., 1981; Koehl, 1984, 1999; Denny, 1988; Vogel, 1996; Hurd, 2000). However, moving water also exerts hydrodynamic forces (drag and acceleration reaction) on macroalgae that can damage or dislodge them. Drag is proportional to the square of water velocity relative to a macroalga, its planform area, and the shape it takes in the flow, while acceleration reaction depends on water acceleration relative to a macroalga, its volume and shape (details in Koehl, 1976; Denny et al., 1985; Vogel, 1996). Hydrodynamic forces on macroalgae vary over different time scales (seconds in a wave; hours over a tidal cycle; months as seasonal storm patterns change; Seymour et al., 1989; Gaylord, 1999; Koehl, 2022) and spatial scales (centimeters to meters of substratum rugosity and neighboring organisms; kilometers of coastal topography and orientation; O’Donnell and Denny, 2008; Nickols et al., 2012).
Moving water damages macroalgae in several ways. Macroalgae are broken if the stress (force per cross-sectional area) imposed by hydrodynamic forces exceeds the strength (stress to break) of their tissues, or are dislodged if stress in the holdfast exceeds attachment strength (Figure 1A; e.g., Koehl, 1986). Moving water can tangle long, flexible algal fronds, increasing hydrodynamic forces and breakage (Figure 1B; Koehl and Wainwright, 1977; Friedland and Denny, 1995; Burnett and Koehl, 2018). Fronds can be abraded as waves scrape them against rough substrata (Figure 1C). Wave-born logs and boulders damage macroalgae as they hit or roll across the shore (Dayton, 1971; Sousa, 1979; Shanks and Wright, 1986), and icebergs scrape away macroalgae (Conlan et al., 1998; Ronowicz et al., 2022).
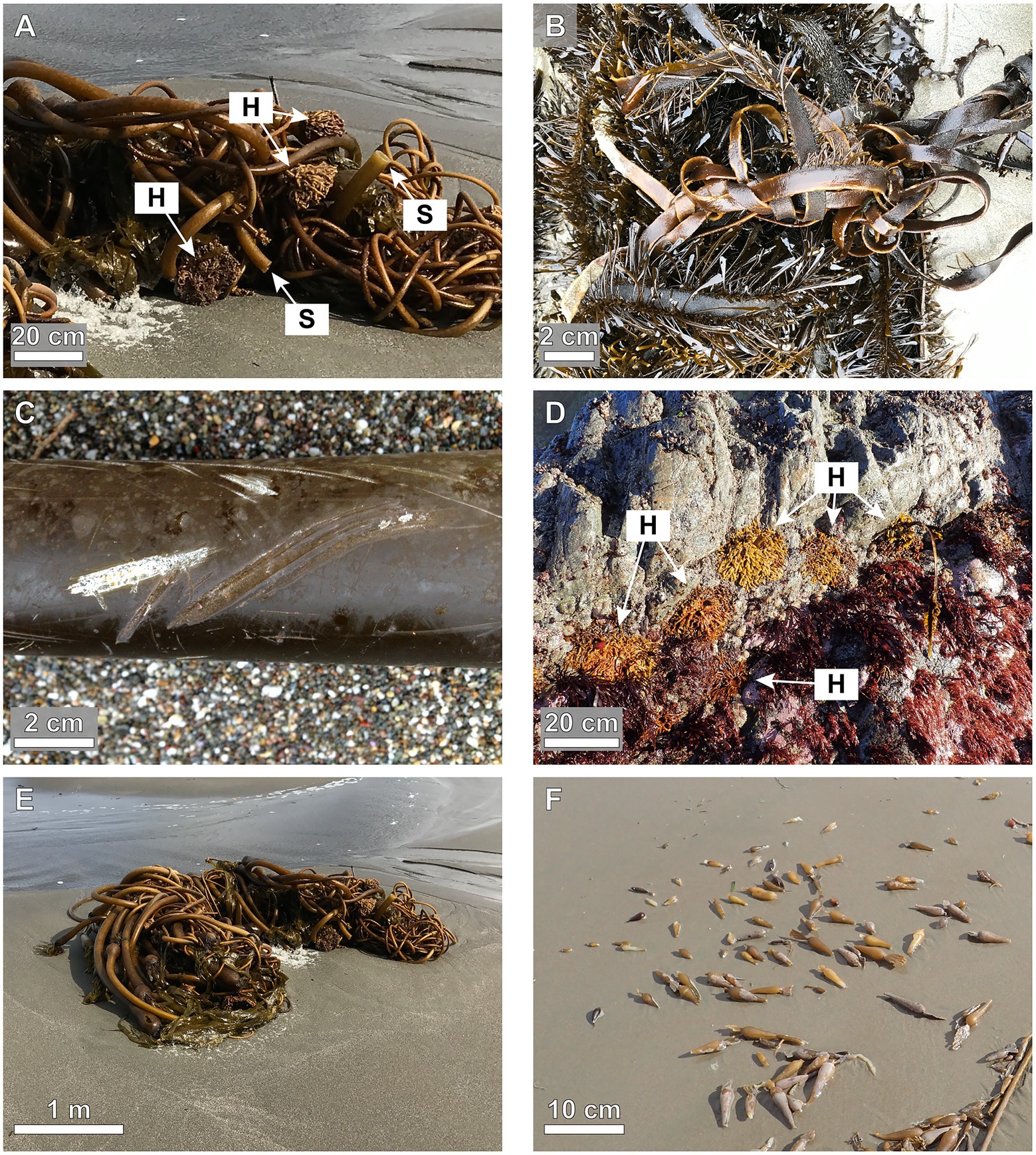
Figure 1. Examples of hydrodynamic damage to macroalgae. (A) Kelp, Nereocystis leutkeana washed up on the shore, showing broken stipes (S) and detached holdfasts (H). (B) Tangled, abraded fronds of kelp, Egregia menziesii. (C) Abrasions on the stipe of a N. leutkeana. (D) Holdfasts of E. menziesii on a rocky shore after stipes have broken away. (E) Beach wrack illustrating the loss of biomass from kelp forests due to hydrodynamic forces on herbivore-damaged N. leutkeana (Koehl and Wainwright, 1977). (F) Gas-filled floats (pneumatocysts) ripped off at their narrow stems from fronds of E. menziesii by moving water and washed ashore.
Consequences of hydrodynamic damage depend on the location of the injury. Dislodgement by holdfast detachment often leads to mortality (Koehl and Wainwright, 1977; Seymour et al., 1989). Stipe breakage removes photosynthetically-active blade tissue (Figure 1D; Santelices et al., 1980; Biedka et al., 1987; Carrington, 1990; Shaughnessy et al., 1996; Bell, 1999; Stewart, 2006b), but does not necessarily cause mortality if drifting thalli survive or if new fronds grow from the holdfast (Lubchenco, 1980; Stewart, 2006b; Loffler et al., 2018; Burnett and Koehl, 2020; Koehl and Daniel, 2022). Biomass loss when blades are damaged is small compared to biomass loss when holdfasts or stipes are broken (Figures 1D,E; Johnson and Mann, 1986; Padilla, 1993; de Bettignies et al., 2013b).
Herbivores
Herbivores, such as limpets and amphipods, damage macroalgae by consuming tissue (Figures 2A,B; Black, 1976; Lowell et al., 1991; de Bettignies et al., 2012). Herbivore bites can lead to further damage by hydrodynamic forces because the cross-sectional area of tissue withstanding those forces is reduced at the bite, so stress is locally higher and can exceed tissue strength (Koehl and Wainwright, 1977; Burnett and Koehl, 2019, 2020). Whether hydrodynamic force on a macroalga causes a crack to propagate across a stipe or blade from a herbivore-inflicted wound depends on the stress-concentration at the crack tip, which is determined by wound shape (Mach et al., 2007; Mach, 2009) sharp cuts inflicted by sea urchins (Koehl and Wainwright, 1977) are more likely to cause breakage than are blunt wounds caused by amphipods and limpets (Black, 1976; Santelices et al., 1980; Gutow et al., 2020). Furthermore, small injuries can enlarge with repeated loading (as in waves), leading to fatigue fracture of a thallus (Mach, 2009). Thus, macroalgal biomass lost due to herbivory is frequently much greater than the tissue consumed by the herbivores (Koehl and Wainwright, 1977; Padilla, 1993).
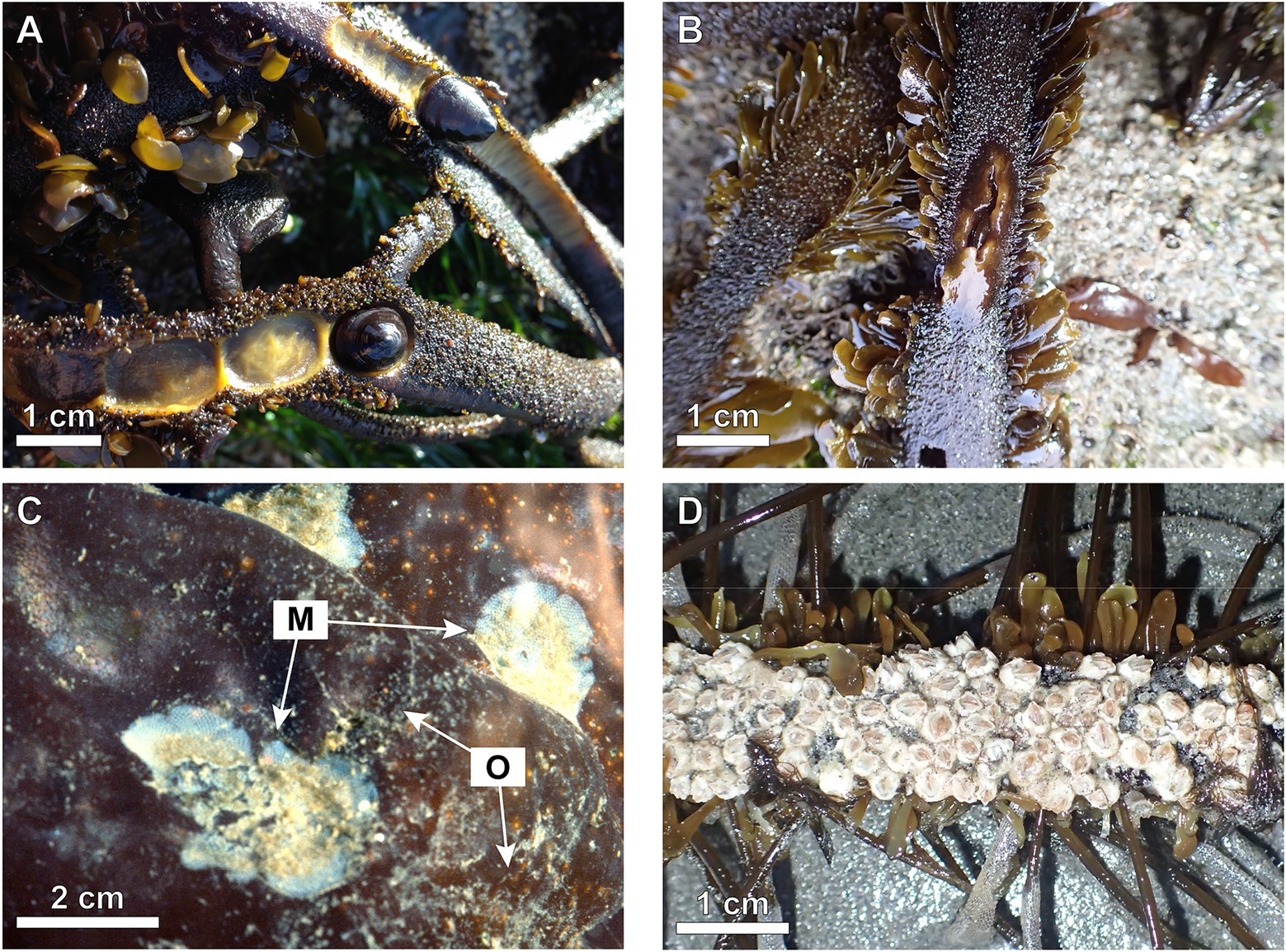
Figure 2. Examples of epibionts on macroalgae. (A) Damage of Egregia menziesii by herbivorous limpets, Discurria insessa. (B) Damage of E. menziesii by a burrowing, herbivorous amphipod. (C) Suspension-feeding encrusting bryozoans, Membranipora membranacea (M), and stoloniferous hydroids, Obelia longissima (O), growing on the blades of the red alga, Mazzaella splendens. (D) Barnacles encrusting a frond of E. menziesii.
Population densities of algae-eating animals and the species composition of herbivore communities are affected by local biological interactions (recruitment, competition, predation) and physical factors (water and air temperature, wave height; e.g., Gunnill, 1984; Paine, 1992; Duggins et al., 2001; de Bettignies et al., 2013b; Burnett et al., 2021). Therefore, the degree and nature of herbivore damage to macroalgae varies geographically and seasonally. Generally herbivores damage macroalgae during months when storm activity is low (thus hydrodynamic forces are small), but macroalgae experience increased breakage at herbivore wounds in subsequent months when storms are frequent (Johnson and Koehl, 1994; de Bettignies et al., 2012, 2013b; Burnett and Koehl, 2020).
Epibionts
Epibionts are organisms living on surfaces of other organisms. Some epibionts on macroalgae are herbivores, but many others do not consume host tissue (see examples in Figures 2C,D; e.g., algae, bryozoans, hydroids, tube worms, barnacles; Koehl and Daniel, 2022). Increased pH under attached epibionts damages host tissues (Wahl, 1989; Harder, 2009), as do anchoring hooks of epibionts (da Gama et al., 2014). Epibionts also damage macroalgae by increasing hydrodynamic forces that their hosts bear by enlarging the structure exposed to ambient flow (Anderson and Martone, 2014), or by stiffening the host, thereby interfering with its reconfiguration by moving water (Koehl and Daniel, 2022).
Damage prevention
Reduction of hydrodynamic forces
Flexibility reduces hydrodynamic forces in several ways. Flexible macroalgae in moving water bend over parallel to the flow and reconfigure into streamlined shapes (blades fold into compact forms; branches and blades collapse together into bundles) that reduce wake size and form drag (Koehl, 1984, 1986, 2022; Koehl and Alberte, 1988; Carrington, 1990; Martone et al., 2012; de Bettignies et al., 2013a; Breitkreutz et al., 2022). Furthermore, flexible macroalgae bent close to the substratum encounter slowed flow in the benthic boundary layer (Koehl, 1984; Stewart, 2004, 2006a). However, flexibility sometimes increases drag if fluttering in flow increases wake size (Koehl and Alberte, 1988; Koehl et al., 2008). Species with fleshy blades are better able to reconfigure in flow than are highly branched species (Boller and Carrington, 2007; Starko et al., 2015), and ruffled blades flutter at greater amplitude and experience higher drag than flat blades (Koehl and Alberte, 1988). Many macroalgae are morphologically plastic and grow into drag-reducing shapes in habitats with rapid flow (Koehl et al., 2008). In kelps, this growth response is triggered by tensile stress (Coleman and Martone, 2020; Koehl and Silk, 2021).
In the oscillatory flow at wave-swept habitats, flexible macroalgae move back and forth with the water motion in waves. When moving with the flow, water velocities and accelerations relative to their surfaces are low, so hydrodynamic forces are small (Koehl, 1984, 1986, 1999, 2022; Burnett and Koehl, 2017). However, when macroalgae reach the end of their tethers, they experience large inertial forces if they were moving rapidly right before being jerked to a halt (Gaylord and Denny, 1997; Denny et al., 1998; Gaylord et al., 2008). Once macroalgae are fully extended, they encounter ambient flow relative to them. Therefore, very long flexible macroalgae in waves may not experience flow past their surfaces or high forces, while shorter macroalgae can reduce hydrodynamic forces if they become fully extended at some point in the wave cycle when water velocities and accelerations are low (Koehl, 1984, 1999, 2022; Wolcott, 2007). Since force on a macroalga in waves depends on its length relative to the distance the water in a wave travels before reversing direction, breakage that shortens a thallus can have profound effects on subsequent damage.
Macroalgae often grow in aggregations (kelp forests, intertidal algal beds). These canopies decrease water speeds, damp wave action, and alter turbulence, so macroalgae in the middle of aggregations experience smaller hydrodynamic forces than isolated macroalgae or those at aggregation edges (Koehl and Alberte, 1988; Johnson, 2001; Gaylord et al., 2007; Zhu et al., 2021a; Koehl, 2022).
Morphological features and tissue mechanical properties that resist damage
Macroalgae avoid breaking in ambient flow if stresses in their tissues due to hydrodynamic forces are lower than their tissue strength (e.g., Koehl and Wainwright, 1977; Johnson and Koehl, 1994). The distribution of mechanical stresses in macroalgae are calculated using engineering structural analysis (Wainwright et al., 1982), which reveals that macroalgae loaded in tension by ambient flow experience much lower stresses for a given force than do seaweeds bent by the flow, and that wider regions of a thallus experience lower local stresses than do narrow ones (Koehl, 1984, 1999).
Whether local stresses in a macroalga cause damage depends on the mechanical properties of its tissues, which are composite materials composed of cells with fiber-reinforced walls (calcified in some species) and polymeric intercellular matrix (Koehl, 1999; Martone, 2006). Tissue mechanical properties (e.g., strength, extensibility, toughness, resilience) are measured using techniques from materials science (Koehl and Wainwright, 1985). One defense against breakage is having tissues strengthened by calcification or by thick fiber-reinforced cell walls aligned with the directions of highest imposed stresses in the thallus (Padilla, 1993; Koehl, 1999; Martone, 2006; Janot and Martone, 2016; Starko et al., 2018). Another defense against breakage for macroalgae exposed to transient high forces is having very extensible, resilient tissues that do not have time to be stretched to breaking extension during a pulse of force, and that bounce back to their unstretched length before the next pulse (Koehl and Wainwright, 1977). Cells in such tissues are separated by a deformable intercellular matrix, and fibers in their thin walls are oriented at high angles relative to their long axes (Koehl and Wainwright, 1977; Koehl, 1999). Both strategies (strength or extensibility) render a macroalga tough (work/volume to break is high; Koehl, 1999).
Macroalgal tissue strength differs between species (Koehl, 2000; Harder et al., 2006; Krumhansl et al., 2015), within species between habitats (Johnson and Koehl, 1994), and within an individual between support, photosynthetic, and reproductive structures (Demes et al., 2013). Tissue mechanical properties also change with age, growth rate, and season (Johnson and Koehl, 1994; Koehl, 1999; Burnett and Koehl, 2019; Sirison and Burnett, 2020; Koehl and Silk, 2021; Millar et al., 2021). Because flow conditions also vary with time, environmental stress factor (ESF) is used to characterize the resistance of a macroalga to breaking at a defined stage in its life. ESF is the ratio of the season-dependent stress required to break a macroalga to the maximum flow-induced stress it experiences in its habitat during that season (Johnson and Koehl, 1994). Many macroalgae have high ESF’s during the calm summer growth and reproduction season, but low ESF’s during winter, as they accumulate damage and experience storms (Johnson and Koehl, 1994; Koehl and Daniel, 2022). Some species develop similar ESF’s in rapid-flow habitats as in calm sites by increasing tissue strength or cross-sectional area of support structures, and/or by growing into low-drag morphologies (Johnson and Koehl, 1994; Sirison and Burnett, 2020; Koehl and Daniel, 2022).
Defenses against herbivores and epibionts
Macroalgae use chemical and mechanical defenses against herbivores and epibionts (Padilla, 1989, 1993; Wahl, 1989; Paul, 1992; Steinberg and De Nys, 2002; Walters et al., 2003; Amsler and Fairhead, 2005; da Gama et al., 2014; Koehl and Daniel, 2022). However, epibionts can avoid defended surfaces by preferentially settling in wounds (Black, 1974).
Several hydrodynamic mechanisms remove epibionts from macroalgae. Flowing water can rip epibionts off macroalgae (Fralick et al., 1974; Duggins et al., 2001; Toth and Pavia, 2002; Chenelot and Konar, 2007; Anderson and Martone, 2014). Some macroalgae enhance this removal by shedding their cuticle or surface cell layers (Wahl, 1989; Wahl et al., 1998; Walters et al., 2003; Harder, 2009). As macroalgae with extensible tissues are stretched and bent by ambient flow, stiff animals (e.g., encrusting bryozoans, calcareous tubeworms) crack and pop off their surfaces (Walters et al., 2003; Koehl and Daniel, 2022). Flexible seaweeds flapping in waves can sweep herbivores off the surrounding substratum (Santelices et al., 1980; Kennelly, 1989; Hughes, 2010). When flow breaks off injured parts of macroalgae infested with herbivores, those animals are removed and cannot damage the remaining thallus (Black, 1976; Wahl, 1989, 2008; Wahl and Hay, 1995).
The role of tissue strength and toughness in herbivore deterrence can be determined if mechanical properties of the tissues attacked by herbivores are measured on the spatial scale of herbivore biting or rasping structures (Padilla, 1985). Studies measuring mechanical properties and chemical deterrents showed that tissue toughness is not always a defense against herbivory (Padilla, 1985, 1989; Martone et al., 2021). Similarly, puncture resistance correlates with reduced grazing rates for some macroalgae (Taylor et al., 2002), but not others (Steinberg, 1985).
Damage management
Controlling patterns of breakage
Macroalgae can reduce tissue loss via structural designs that direct where breakage occurs, and tissue properties that determine how cracks propagate across thalli. For example, some species localize where bending occurs by having joints (narrow regions with flexible tissues; Koehl, 1999; Martone, 2006; Janot and Martone, 2016; Janot et al., 2022). If breakage occurs at such localized regions of high stress, a macroalga can be pruned by ambient flow (Figure 1F) rather than ripped off the shore (Martone, 2006; Martone and Denny, 2008). When cracks propagating through macroalgal tissues are diverted at interfaces between the intercellular matrix and cell walls, more mechanical work is needed to drive the cracks across the structure (Vincent, 2012). Distribution and orientation of fibers and calcification also determines the direction of tears in algae (like rip-stop fabric; Padilla, 1993), for example, causing blades to rip longitudinally without tissue loss.
Growth and healing in response to damage
After damage occurs, some macroalgae increase the strength of tissue around the wound (Lowell et al., 1991; Toth and Pavia, 2006), while others increase the cross-sectional area of the damaged structure (Burnett and Koehl, 2019). Some damaged macroalgae grow new fronds, becoming bushier (Black, 1974; Fox, 2013). Damage that prunes macroalgae to smaller size reduces their danger of washing away in some cases (Black, 1976; Wolcott, 2007; de Bettignies et al., 2012), but not in others (Burnett and Koehl, 2020). However, excessive damage may leave macroalgae less able to heal or grow (Poore et al., 2018), leading to stunted size or death (Toth and Pavia, 2006; O’Brien and Scheibling, 2016; Pfister and Betcher, 2018; Burnett and Koehl, 2020).
Life history strategies that compensate for or utilize damage
Some perennial macroalgae persist in rapid-flow habitats by putting their resources into producing strong thalli (thus growing slowly and delaying reproduction), while other species are successful at such sites by growing rapidly and reproducing before seasonally-predictable storms rip their weak thalli off the shore (Santelices et al., 1980; Johnson and Koehl, 1994; Koehl, 1999; Wolcott, 2007). Some macroalgae with “bad” mechanical designs regrow from perennial holdfasts (Bell, 1999), and some recruit opportunistically year-round (Santelices et al., 1980).
Some macroalgae increase the strength of reproductive tissues so they are not prematurely damaged (Demes et al., 2013), whereas others use damage to enhance reproduction and dispersal. For example, Turbinaria ornata are weaker and more buoyant when reproductive, so hydrodynamic forces break their stipes and they form floating aggregations where they release gametes and are transported to new sites by currents (Stewart, 2006b). Similarly, drifting in ocean currents by broken-off reproductive kelp aids long-distance dispersal (Bernardes Batista et al., 2018; Fraser et al., 2020, 2022).
Discussion
Studying the biomechanics of damage to macroalgae from an ecological perspective reveals some surprises. For example, the assumption that an increase in size leads to larger hydrodynamic forces and greater risk of breakage is not necessarily true for macroalgae in waves. Furthermore, while biomechanical analyses show why certain macroalgae have “bad” engineering designs prone to damage, ecological studies reveal how such breakable organisms survive and reproduce in mechanically-stressful environments. Damage can play positive roles in the survival, reproduction, and dispersal of macroalgae. Moreover, damage to macroalgae that outcompete other organisms for space and light can have positive consequences for the local community, enhancing the diversity, growth rates, and abundance of other organisms (Sousa, 1979; Hughes, 2010; Clements et al., 2018).
There are gaps in our current knowledge of macroalgal damage. Little is known about pathways by which cells recognize damage and initiate repairs, whole-thallus signaling that initiates the formation of new fronds when old ones break, or cellular mechanisms that transduce mechanical stresses experienced in nature into patterns of cell division, enlargement, and cell wall construction. Future research should also explore the ecological biomechanics of damage across a greater diversity of macroalgal species, habitats, and life stages to identify ecological patterns and evolutionary histories of mechanisms of damage prevention and management, and to gain insights about the sensitivities of these processes to environmental stress.
Author contributions
All authors listed have made a substantial, direct, and intellectual contribution to the work, and approved it for publication.
Funding
NB’s research reviewed here was supported by a National Science Foundation Graduate Research Fellowship DGE-0903711, and MK’s research reviewed here was supported in part National Science Foundation Grant OCE-9217338.
Acknowledgments
We thank R. Alberte, E. Carrington, T. Daniel, M. Denny, B. Gaylord, J. Gosline, A. Johnson, R. Paine, W. Silk, H. Stewart, S. Vogel, B. Wolcott, and S. Wainwright for helpful discussions over the years that helped shape our thinking about the issues reviewed here.
Conflict of interest
The authors declare that this paper was written in the absence of any commercial or financial relationships that could be construed as a potential conflict of interest.
Publisher’s note
All claims expressed in this article are solely those of the authors and do not necessarily represent those of their affiliated organizations, or those of the publisher, the editors and the reviewers. Any product that may be evaluated in this article, or claim that may be made by its manufacturer, is not guaranteed or endorsed by the publisher.
References
Amsler, C. D., and Fairhead, V. A. (2005). Defensive and sensory chemical ecology of brown algae. Adv. Bot. Res. 43, 1–91. doi: 10.1016/S0065-2296(05)43001-3
Anderson, L. M., and Martone, P. T. (2014). Biomechanical consequences of epiphytism in intertidal macroalgae. J. Exp. Biol. 217, 1167–1174. doi: 10.1242/jeb.088955
Arkema, K. K., Reed, D. C., and Schroeter, S. C. (2009). Direct and indirect effects of giant kelp determine benthic community structure and dynamics. Ecology 90, 3126–3137. doi: 10.1890/08-1213.1
Bauer, U., Poppinga, S., and Müller, U. K. (2020). Mechanical ecology—taking biomechanics to the field. Integr. Comp. Biol. 60, 820–828. doi: 10.1093/icb/icaa018
Bell, E. C. (1999). Applying flow tank measurements to the surf zone: predicting dislodgment of the Gigartinaceae. Phycol. Res. 47, 159–166. doi: 10.1111/j.1440-1835.1999.tb00296.x
Bernardes Batista, M., Batista Anderson, A., Franzan Sanches, P., Simionatto Polito, P., Lima Silveira, T., Velez-Rubio, G., et al. (2018). Kelps’ long-distance dispersal: role of ecological/oceanographic processes and implications to marine forest conservation. Diversity 10:11. doi: 10.3390/d10010011
Biedka, R., Gosline, J., and De Wreede, R. (1987). Biomechanical analysis of wave-induced mortality in the marine alga Pterygophora californica. Mar. Ecol. Prog. Ser. 36, 163–170. doi: 10.3354/meps036163
Black, R. (1974). Some biological interactions affecting intertidal populations of the kelp Egregia laevigata. Mar. Biol. 28, 189–198. doi: 10.1007/BF00387297
Black, R. (1976). The effects of grazing by the limpet, Acmaea insessa, on the kelp, Egregia laevigata, in the intertidal zone. Ecology 57, 265–277. doi: 10.2307/1934815
Boller, M. L., and Carrington, E. (2007). Interspecific comparison of hydrodynamic performance and structural properties among intertidal macroalgae. J. Exp. Biol. 210, 1874–1884. doi: 10.1242/jeb.02775
Breitkreutz, A., Coleman, L. J. M., and Martone, P. T. (2022). Less than the sum of its parts: blade clustering reduces drag in the bull kelp, Nereocystis luetkeana (Phaeophyceae). J Phycol. 13258. doi: 10.1111/jpy.13258
Burnett, N. P., Armstrong, E. J., Romero, R., Runzel, C., and Tanner, R. L. (2021). Kelp morphology and herbivory are maintained across latitude despite geographic shift in kelp-wounding herbivores. Biol. Bull. 241, 168–184. doi: 10.1086/715039
Burnett, N. P., and Koehl, M. A. R. (2017). Pneumatocysts provide buoyancy with minimal effect on drag for kelp in wave-driven flow. J. Exp. Mar. Biol. Ecol. 497, 1–10. doi: 10.1016/j.jembe.2017.09.003
Burnett, N. P., and Koehl, M. A. R. (2018). Knots and tangles weaken kelp fronds while increasing drag forces and epifauna on the kelp. J. Exp. Mar. Biol. Ecol. 508, 13–20. doi: 10.1016/j.jembe.2018.08.003
Burnett, N. P., and Koehl, M. A. R. (2019). Mechanical properties of the wave-swept kelp Egregia menziesii change with season, growth rate and herbivore wounds. J. Exp. Biol. 222:jeb190595. doi: 10.1242/jeb.190595
Burnett, N. P., and Koehl, M. A. R. (2020). Thallus pruning does not enhance survival or growth of a wave-swept kelp. Mar. Biol. 167:52. doi: 10.1007/s00227-020-3663-5
Carrington, E. (1990). Drag and dislodgment of an intertidal macroalga: consequences of morphological variation in Mastocarpus papillatus Kützing. J. Exp. Mar. Biol. Ecol. 139, 185–200. doi: 10.1016/0022-0981(90)90146-4
Chenelot, H., and Konar, B. (2007). Lacuna vincta (Mollusca, Neotaenioglossa) herbivory on juvenile and adult Nereocystis luetkeana (Heterokontophyta, Laminariales). Hydrobiologia 583, 107–118. doi: 10.1007/s10750-006-0484-6
Christie, H., Norderhaug, K., and Fredriksen, S. (2009). Macrophytes as habitat for fauna. Mar. Ecol. Prog. Ser. 396, 221–233. doi: 10.3354/meps08351
Clements, C., Rasher, D., Hoey, A., Bonito, V., and Hay, M. (2018). Spatial and temporal limits of coral-macroalgal competition: the negative impacts of macroalgal density, proximity, and history of contact. Mar. Ecol. Prog. Ser. 586, 11–20. doi: 10.3354/meps12410
Coleman, L. J. M., and Martone, P. T. (2020). Morphological plasticity in the kelp Nereocystis luetkeana (Phaeophyceae) is sensitive to the magnitude, direction, and location of mechanical loading. J. Phycol. 56, 1414–1427. doi: 10.1111/jpy.13043
Conlan, K., Lenihan, H., Kvitek, R., and Oliver, J. (1998). Ice scour disturbance to benthic communities in the Canadian High Arctic. Mar. Ecol. Prog. Ser. 166, 1–16. doi: 10.3354/meps166001
da Gama, B. A. P., Plouguerné, E., and Pereira, R. C. (2014). The antifouling defence mechanisms of marine macroalgae. Adv. Bot. Res. 71, 413–440. doi: 10.1016/B978-0-12-408062-1.00014-7
Dayton, P. K. (1971). Competition, disturbance, and community organization: the provision and subsequent utilization of space in a rocky intertidal community. Ecol. Monogr. 41, 351–389. doi: 10.2307/1948498
de Bettignies, T., Thomsen, M., and Wernberg, T. (2012). Wounded kelps: patterns and susceptibility to breakage. Aquat. Biol. 17, 223–233. doi: 10.3354/ab00471
de Bettignies, T., Wernberg, T., and Lavery, P. S. (2013a). Size, not morphology, determines hydrodynamic performance of a kelp during peak flow. Mar. Biol. 160, 843–851. doi: 10.1007/s00227-012-2138-8
de Bettignies, T., Wernberg, T., Lavery, P. S., Vanderklift, M. A., and Mohring, M. B. (2013b). Contrasting mechanisms of dislodgement and erosion contribute to production of kelp detritus. Limnol. Oceanogr. 58, 1680–1688. doi: 10.4319/lo.2013.58.5.1680
Demes, K. W., Harley, C. D. G., Anderson, L. M., and Carrington, E. (2013). Shifts in morphological and mechanical traits compensate for performance costs of reproduction in a wave-swept seaweed. J. Ecol. 101, 963–970. doi: 10.1111/1365-2745.12099
Denny, M. (1988). Biology and the Mechanics of the Wave-Swept Environment. Princeton, NJ: Princeton University Press.
Denny, M. (2012). Biophysics, bioenergetics and mechanistic approaches to ecology. J. Exp. Biol. 215:871. doi: 10.1242/jeb.069575
Denny, M. (2021). Wave-energy dissipation: seaweeds and marine plants are ecosystem engineers. Fluids 6:151. doi: 10.3390/fluids6040151
Denny, M. W., Daniel, T. L., and Koehl, M. A. R. (1985). Mechanical limits to size in wave-swept organisms. Ecol. Monogr. 55, 69–102. doi: 10.2307/1942526
Denny, M., Gaylord, B., Helmuth, B., and Daniel, T. (1998). The menace of momentum: dynamic forces on flexible organisms. Limnol. Oceanogr. 43, 955–968. doi: 10.4319/lo.1998.43.5.0955
Duggins, D. O., and Eckman, J. E. (1997). Is kelp detritus a good food for suspension feeders? Effects of kelp species, age and secondary metabolites. Mar. Biol. 128, 489–495. doi: 10.1007/s002270050115
Duggins, D., Eckman, J., Siddon, C., and Klinger, T. (2001). Interactive roles of mesograzers and current flow in survival of kelps. Mar. Ecol. Prog. Ser. 223, 143–155. doi: 10.3354/meps223143
Fox, M. D. (2013). Resource translocation drives δ13 C fractionation during recovery from disturbance in giant kelp, Macrocystis pyrifera. J. Phycol. 49, 811–815. doi: 10.1111/jpy.12099
Fralick, R. A., Turgeon, K. W., and Mathieson, A. C. (1974). Destruction of kelp populations by Lacuna vincta (Montagu). Nautilus 88, 112–114.
Fraser, C. I., Dutoit, L., Morrison, A. K., Pardo, L. M., Smith, S. D. A., Pearman, W. S., et al. (2022). Southern Hemisphere coasts are biologically connected by frequent, long-distance rafting events. Curr. Biol. 32, 3154–3160.e3. doi: 10.1016/j.cub.2022.05.035
Fraser, C. I., Velásquez, M., Nelson, W. A., Macaya, E. C., and Hay, C. H. (2020). The biogeographic importance of buoyancy in macroalgae: a case study of the southern bull-kelp genus Durvillaea (Phaeophyceae), including descriptions of two new species. J. Phycol. 56, 23–36. doi: 10.1111/jpy.12939
Friedland, M. T., and Denny, M. W. (1995). Surviving hydrodynamic forces in a wave-swept environment: consequences of morphology in the feather boa kelp, Egregia menziesii (Turner). J. Exp. Mar. Biol. Ecol. 190, 109–133. doi: 10.1016/0022-0981(95)00038-S
Gaylord, B. (1999). Detailing agents of physical disturbance: wave-induced velocities and accelerations on a rocky shore. J. Exp. Mar. Biol. Ecol. 239, 85–124. doi: 10.1016/S0022-0981(99)00031-3
Gaylord, B., and Denny, M. (1997). Flow and flexibility. I. Effects of size, shape and stiffness in determining wave forces on the stipitate kelps Eisenia arborea and Pterygophora californica. J. Exp. Biol. 200, 3141–3164. doi: 10.1242/jeb.200.24.3141
Gaylord, B., Denny, M. W., and Koehl, M. A. R. (2008). Flow forces on seaweeds: field evidence for roles of impingement and organism inertia. Biol. Bull. 215, 295–308. doi: 10.2307/25470713
Gaylord, B., Rosman, J. H., Reed, D. C., Koseff, J. R., Fram, J., MacIntyre, S., et al. (2007). Spatial patterns of flow and their modification within and around a giant kelp forest. Limnol. Oceanogr. 52, 1838–1852. doi: 10.4319/lo.2007.52.5.1838
Graham, M. H., Vásquez, J. A., and Buschmann, A. H. (2007). Global ecology of the giant kelp Macrocystis: from ecotypes to ecosystems. Oceanogr. Mar. Biol. Ann. Rev. 45, 39–88.
Gunnill, F. C. (1984). Differing distributions of potentially competing amphipods, copepods and gastropods among specimens of the intertidal alga Pelvetia fastigiata. Mar. Biol. 82, 277–291. doi: 10.1007/BF00392408
Gutow, L., Poore, A. G. B., Díaz Poblete, M. A., Villalobos, V., and Thiel, M. (2020). Small burrowing amphipods cause major damage in a large kelp. Proc. R. Soc. B 287:20200330. doi: 10.1098/rspb.2020.0330
Harder, T. (2009). “Marine epibiosis: concepts, ecological consequences and host defence,” in Marine and Industrial Biofouling Springer Series on Biofilms. eds. H.-C. Flemming, P. S. Murthy, R. Venkatesan, and K. Cooksey (Berlin, Heidelberg: Springer), 219–231. doi: 10.1007/978-3-540-69796-1_12
Harder, D. L., Hurd, C. L., and Speck, T. (2006). Comparison of mechanical properties of four large, wave-exposed seaweeds. Am. J. Bot. 93, 1426–1432. doi: 10.3732/ajb.93.10.1426
Higham, T. E., Ferry, L. A., Schmitz, L., Irschick, D. J., Starko, S., Anderson, P. S. L., et al. (2021). Linking ecomechanical models and functional traits to understand phenotypic diversity. Trends Ecol. Evol. 36, 860–873. doi: 10.1016/j.tree.2021.05.009
Hughes, B. B. (2010). Variable effects of a kelp foundation species on rocky intertidal diversity and species interactions in central California. J. Exp. Mar. Biol. Ecol. 393, 90–99. doi: 10.1016/j.jembe.2010.07.003
Hurd, C. L. (2000). Water motion, marine macroalgal physiology, and production. J. Phycol. 36, 453–472. doi: 10.1046/j.1529-8817.2000.99139.x
Janot, K., and Martone, P. T. (2016). Convergence of joint mechanics in independently evolving, articulated coralline algae. J. Exp. Biol. 219, 383–391. doi: 10.1242/jeb.131755
Janot, K. G., Unda, F., Mansfield, S. D., and Martone, P. T. (2022). Evolutionary patterns in chemical composition and biomechanics of articulated coralline algae. Integr. Comp. Biol., icac021. doi: 10.1093/icb/icac021
Johnson, A. S. (2001). Drag, drafting, and mechanical interactions in canopies of the red alga Chondrus crispus. Biol. Bull. 201, 126–135. doi: 10.2307/1543328
Johnson, A., and Koehl, M. (1994). Maintenance of dynamic strain similarity and environmental stress factor in different flow habitats: thallus allometry and material properties of a giant kelp. J. Exp. Biol. 195, 381–410. doi: 10.1242/jeb.195.1.381
Johnson, C. R., and Mann, K. H. (1986). The importance of plant defence abilities to the structure of subtidal seaweed communities: The kelp Laminaria longicruris de la Pylaie survives grazing by the snail Lacuna vincta (Montagu) at high population densities. J. Exp. Mar. Biol. Ecol. 97, 231–267. doi: 10.1016/0022-0981(86)90244-3
Kennelly, S. J. (1989). Effects of kelp canopies on understorey species due to shade and scour. Mar. Ecol. Prog. Ser. 50, 215–224. doi: 10.3354/meps050215
Koehl, M. A. R. (1976). “Mechanical design in Sea Anemones,” in Coelenterate Ecology and Behavior. ed. G. O. Mackie (Boston, MA: Springer), 23–31.
Koehl, M. A. R. (1984). How do benthic organisms withstand moving water? Am. Zool. 24, 57–70. doi: 10.1093/icb/24.1.57
Koehl, M. A. R. (1986). “Seaweeds in moving water: form and mechanical function” in On the Economy of Plant Form and Function. ed. T. J. Givnish (Cambridge: Cambridge University Press), 603–634.
Koehl, M. A. R. (1999). Ecological biomechanics of benthic organisms: life history, mechanical design and temporal patterns of mechanical stress. J. Exp. Biol. 202, 3469–3476. doi: 10.1242/jeb.202.23.3469
Koehl, M. A. R. (2000). “Mechanical design and hydrodynamics of blade-like algae: Chondracanthus exasperatus” in Proceedings of the Third International Plant Biomechanics Conference. eds. H. C. Spatz and T. Speck (Stuttgart: Thieme Verlag), 295–308.
Koehl, M. A. R. (2022). Ecological biomechanics of marine macrophytes. J. Exp. Bot. 73, 1104–1121. doi: 10.1093/jxb/erab536
Koehl, M. A. R., and Alberte, R. S. (1988). Flow, flapping, and photosynthesis of Nereocystis leutkeana: a functional comparison of undulate and flat blade morphologies. Mar. Biol. 99, 435–444. doi: 10.1007/BF02112137
Koehl, M. A. R., and Daniel, T. L. (2022). Hydrodynamic interactions between macroalgae and their epibionts. Front. Mar. Sci. 9:872960. doi: 10.3389/fmars.2022.872960
Koehl, M. A. R., and Silk, W. K. (2021). How kelp in drag lose their ruffles: environmental cues, growth kinematics, and mechanical constraints govern curvature. J. Exp. Bot. 72, 3677–3687. doi: 10.1093/jxb/erab111
Koehl, M. A. R., Silk, W. K., Liang, H., and Mahadevan, L. (2008). How kelp produce blade shapes suited to different flow regimes: a new wrinkle. Integr. Comp. Biol. 48, 834–851. doi: 10.1093/icb/icn069
Koehl, M. A. R., and Wainwright, S. A. (1977). Mechanical adaptations of a giant kelp. Limnol. Oceanogr. 22, 1067–1071. doi: 10.4319/lo.1977.22.6.1067
Koehl, M. A. R., and Wainwright, S. A. (1985). “Biomechanics” in Handbook of Phycological Methods. Ecological Field Methods: Macroalgae. eds. M. L. Littler and D. S. Littler (New York, NY: Cambridge University Press), 292–313.
Krumhansl, K. A., Demes, K. W., Carrington, E., and Harley, C. D. G. (2015). Divergent growth strategies between red algae and kelps influence biomechanical properties. Am. J. Bot. 102, 1938–1944. doi: 10.3732/ajb.1500289
Krumhansl, K., and Scheibling, R. (2012). Production and fate of kelp detritus. Mar. Ecol. Prog. Ser. 467, 281–302. doi: 10.3354/meps09940
Loffler, Z., Graba-Landry, A., Kidgell, J. T., McClure, E. C., Pratchett, M. S., and Hoey, A. S. (2018). Holdfasts of Sargassum swartzii are resistant to herbivory and resilient to damage. Coral Reefs 37, 1075–1084. doi: 10.1007/s00338-018-01745-w
Lowell, R. B., Markham, J. H., and Mann, K. H. (1991). Herbivore-like damage induces increased strength and toughness in a seaweed. Proc. R. Soc. Lond. B 243, 31–38. doi: 10.1098/rspb.1991.0006
Lubchenco, J. (1980). Algal zonation in the New England rocky intertidal community: an experimental analysis. Ecology 61, 333–344. doi: 10.2307/1935192
Mach, K. J. (2009). Mechanical and biological consequences of repetitive loading: crack initiation and fatigue failure in the red macroalga Mazzaella. J. Exp. Biol. 212, 961–976. doi: 10.1242/jeb.026989
Mach, K. J., Nelson, D. V., and Denny, M. W. (2007). Techniques for predicting the lifetimes of wave-swept macroalgae: a primer on fracture mechanics and crack growth. J. Exp. Biol. 210, 2213–2230. doi: 10.1242/jeb.001560
Martone, P. T. (2006). Size, strength and allometry of joints in the articulated coralline Calliarthron. J. Exp. Biol. 209, 1678–1689. doi: 10.1242/jeb.02139
Martone, P. T., and Denny, M. W. (2008). To break a coralline: mechanical constraints on the size and survival of a wave-swept seaweed. J. Exp. Biol. 211, 3433–3441. doi: 10.1242/jeb.020495
Martone, P. T., Kost, L., and Boller, M. (2012). Drag reduction in wave-swept macroalgae: alternative strategies and new predictions. Am. J. Bot. 99, 806–815. doi: 10.3732/ajb.1100541
Martone, P. T., Schipper, S. R., Froese, T., Bretner, J., DeMong, A., and Eastham, T. M. (2021). Calcification does not necessarily protect articulated coralline algae from urchin grazing. J. Exp. Mar. Biol. Ecol. 537:151513. doi: 10.1016/j.jembe.2021.151513
Millar, R., Houghton, J. D. R., and Kregting, L. (2021). The stress and strain of life – how differences in the mechanical properties and cellular composition enable the kelp Laminaria digitata to thrive in different hydrodynamic environments. Mar. Environ. Res. 169:105330. doi: 10.1016/j.marenvres.2021.105330
Nickols, K., Gaylord, B., and Largier, J. (2012). The coastal boundary layer: predictable current structure decreases alongshore transport and alters scales of dispersal. Mar. Ecol. Prog. Ser. 464, 17–35. doi: 10.3354/meps09875
Norton, T. A., Mathieson, A. C., and Neushul, M. (1981). “Morphology and environment” in The Biology of Seaweeds Botanical Monograhs eds. C. S. Lobban and M. J. Wynne (Berkeley: University of California Press), 421–451.
O’Brien, J. M., and Scheibling, R. E. (2016). Nipped in the bud: mesograzer feeding preference contributes to kelp decline. Ecology 97, 1873–1886. doi: 10.1890/15-1728.1
O’Donnell, M. J., and Denny, M. W. (2008). Hydrodynamic forces and surface topography: centimeter-scale spatial variation in wave forces. Limnol. Oceanogr. 53, 579–588. doi: 10.4319/lo.2008.53.2.0579
Padilla, D. K. (1985). Structural resistance of algae to herbivores: a biomechanical approach. Mar. Biol. 90, 103–109. doi: 10.1007/BF00428220
Padilla, D. K. (1989). Algal structure defenses: form and calcification in resistance to tropical limpets. Ecology 70, 835–842. doi: 10.2307/1941352
Padilla, D. K. (1993). Rip stop in marine algae: minimizing the consequences of herbivore damage. Evol. Ecol. 7, 634–644. doi: 10.1007/BF01237826
Paine, R. T. (1992). Food-web analysis through field measurement of per capita interaction strength. Nature 355, 73–75. doi: 10.1038/355073a0
Paul, V. J. (1992). “Seaweed chemical defenses on coral reefs,” in Ecological Roles of Marine Natural Products (Ithaca, NY: Cornell University Press), 24–50.
Pfister, C. A., and Betcher, S. P. (2018). Climate drivers and animal host use determine kelp performance over decadal scales in the kelp Pleurophycus gardneri (Laminariales, Phaeophyceae). J. Phycol. 54, 1–11. doi: 10.1111/jpy.12601
Poore, A. G. B., Gutow, L., Lörz, A.-N., and Thiel, M. (2018). Nest building by a small mesograzer limits blade size of the giant kelp Macrocystis pyrifera. Mar. Biol. 165:184. doi: 10.1007/s00227-018-3444-6
Poore, A. G. B., Gutow, L., Pantoja, J. F., Tala, F., Jofré Madariaga, D., and Thiel, M. (2014). Major consequences of minor damage: impacts of small grazers on fast-growing kelps. Oecologia 174, 789–801. doi: 10.1007/s00442-013-2795-4
Ronowicz, M., Kukliński, P., and Włodarska-Kowalczuk, M. (2022). Morphological variation of kelps (Alaria esculenta, cf. Laminaria digitata, and Saccharina latissima) in an Arctic glacial fjord. Estuar. Coast. Shelf Sci. 268:107802. doi: 10.1016/j.ecss.2022.107802
Santelices, B., Castilla, J. C., Cancino, J., and Schmiede, P. (1980). Comparative ecology of Lessonia nigrescens and Durvillaea antarctica (Phaeophyta) in Central Chile. Mar. Biol. 59, 119–132. doi: 10.1007/BF00405461
Schiel, D. R., and Foster, M. S. (2015). The Biology and Ecology of Giant Kelp Forests. Oakland, CA: University of California Press.
Seymour, R. J., Tegner, M. J., Dayton, P. K., and Parnell, P. E. (1989). Storm wave induced mortality of giant kelp, Macrocystis pyrifera, in Southern California. Estuar. Coast. Shelf Sci. 28, 277–292. doi: 10.1016/0272-7714(89)90018-8
Shanks, A. L., and Wright, W. G. (1986). Adding teeth to wave action: the destructive effects of wave-borne rocks on intertidal organisms. Oecologia 69, 420–428. doi: 10.1007/BF00377065
Shaughnessy, F., De Wreede, R., and Bell, E. (1996). Consequences of morphology and tissue strength to blade survivorship of two closely related Rhodophyta species. Mar. Ecol. Prog. Ser. 136, 257–266. doi: 10.3354/meps136257
Sirison, N., and Burnett, N. P. (2020). Turbinaria ornata (Phaeophyceae) varies size and strength to maintain environmental safety factor across flow regimes. J. Phycol. 56, 233–237. doi: 10.1111/jpy.12933
Sousa, W. P. (1979). Disturbance in marine intertidal boulder fields: the nonequilibrium maintenance of species diversity. Ecology 60:1225. doi: 10.2307/1936969
Starko, S., Claman, B. Z., and Martone, P. T. (2015). Biomechanical consequences of branching in flexible wave-swept macroalgae. New Phytol. 206, 133–140. doi: 10.1111/nph.13182
Starko, S., Mansfield, S. D., and Martone, P. T. (2018). Cell wall chemistry and tissue structure underlie shifts in material properties of a perennial kelp. Eur. J. Phycol. 53, 307–317. doi: 10.1080/09670262.2018.1449013
Steinberg, P. D. (1985). Feeding preferences of Tegula funebralis and chemical defenses of marine brown algae. Ecol. Monogr. 55, 333–349. doi: 10.2307/1942581
Steinberg, P. D., and De Nys, R. (2002). Chemical mediation of colonization of seaweed surfaces. J. Phycol. 38, 621–629. doi: 10.1046/j.1529-8817.2002.02042.x
Steneck, R. S., Graham, M. H., Bourque, B. J., Corbett, D., Erlandson, J. M., Estes, J. A., et al. (2002). Kelp forest ecosystems: biodiversity, stability, resilience and future. Envir. Conserv. 29, 436–459. doi: 10.1017/S0376892902000322
Stewart, H. L. (2004). Hydrodynamic consequences of maintaining an upright posture by different magnitudes of stiffness and buoyancy in the tropical alga Turbinaria ornata. J. Mar. Sys. 49, 157–167. doi: 10.1016/j.jmarsys.2003.05.007
Stewart, H. L. (2006a). Hydrodynamic consequences of flexural stiffness and buoyancy for seaweeds: a study using physical models. J. Exp. Biol. 209, 2170–2181. doi: 10.1242/jeb.02254
Stewart, H. L. (2006b). Ontogenetic changes in buoyancy, breaking strength, extensibility, and reproductive investment in a drifting macroalgal Turbinaria ornata (Phaeophyta). J. Phycol. 42, 43–50. doi: 10.1111/j.1529-8817.2006.00184.x
Taylor, R. B., Sotka, E., and Hay, M. E. (2002). Tissue-specific induction of herbivore resistance: seaweed response to amphipod grazing. Oecologia 132, 68–76. doi: 10.1007/s00442-002-0944-2
Toth, G. B., and Pavia, H. (2002). Intraplant habitat and feeding preference of two gastropod herbivores inhabiting the kelp Laminaria hyperborea. J. Mar. Biol. Assoc. 82, 243–247. doi: 10.1017/S0025315402005416
Toth, G. B., and Pavia, H. (2006). Artificial wounding decreases plant biomass and shoot strength of the brown seaweed Ascophyllum nodosum (Fucales, Phaeophyceae). Mar. Biol. 148, 1193–1199. doi: 10.1007/s00227-005-0167-2
Van Alstyne, K. L., Whitman, S. L., and Ehlig, J. M. (2001). Differences in herbivore preferences, phlorotannin production, and nutritional quality between juvenile and adult tissues from marine brown algae. Mar. Biol. 139, 201–210. doi: 10.1007/s002270000507
Vincent, J. F. V. (2012). Structural Biomaterials. 3rd Edn. Princeton, NJ: Princeton University Press.
Vogel, S. (1996). Life in Moving Fluids: The Physical Biology of Flow. 2. ed., rev.expanded, 2. printing and first paperback printing. Princeton, NJ: Princeton University Press.
Wahl, M. (1989). Marine epibiosis. I. Fouling and antifouling: some basic aspects. Mar. Ecol. Prog. Ser. 58, 175–189. doi: 10.3354/meps058175
Wahl, M. (2008). Ecological lever and interface ecology: epibiosis modulates the interactions between host and environment. Biofouling 24, 427–438. doi: 10.1080/08927010802339772
Wahl, M., and Hay, M. E. (1995). Associational resistance and shared doom: effects of epibiosis on herbivory. Oecologia 102, 329–340. doi: 10.1007/BF00329800
Wahl, M., Kröger, K., and Lenz, M. (1998). Non-toxic protection against epibiosis. Biofouling 12, 205–226. doi: 10.1080/08927019809378355
Wainwright, S. A., Biggs, W. D., Currey, J. D., and Gosline, J. M. (1982). Mechanical Design in Organisms. Princeton, NJ: Princeton University Press.
Walters, L. J., Smith, C. M., and Hadfield, M. G. (2003). Recruitment of sessile marine invertebrates on Hawaiian macrophytes: do pre-settlement or post-settlement processes keep plants free from fouling? Bull. Mar. Sci. 72, 813–839.
Wolcott, B. (2007). Mechanical size limitation and life-history strategy of an intertidal seaweed. Mar. Ecol. Prog. Ser. 338, 1–10. doi: 10.3354/meps338001
Zhu, G., Ebbing, A., Bouma, T. J., and Timmermans, K. R. (2021a). Morphological and physiological plasticity of Saccharina latissima (Phaeophyceae) in response to different hydrodynamic conditions and nutrient availability. J. Appl. Phycol. 33, 2471–2483. doi: 10.1007/s10811-021-02428-w
Keywords: hydrodynamics, material properties, strength, breakage, wounds, herbivory, drag
Citation: Burnett NP and Koehl MAR (2022) Ecological biomechanics of damage to macroalgae. Front. Plant Sci. 13:981904. doi: 10.3389/fpls.2022.981904
Edited by:
Jianping Yu, National Renewable Energy Laboratory (DOE), United StatesReviewed by:
Ergun Taskin, Celal Bayar University, TurkeyCopyright © 2022 Burnett and Koehl. This is an open-access article distributed under the terms of the Creative Commons Attribution License (CC BY). The use, distribution or reproduction in other forums is permitted, provided the original author(s) and the copyright owner(s) are credited and that the original publication in this journal is cited, in accordance with accepted academic practice. No use, distribution or reproduction is permitted which does not comply with these terms.
*Correspondence: Nicholas P. Burnett, YnVybmV0dG5wQGdtYWlsLmNvbQ==