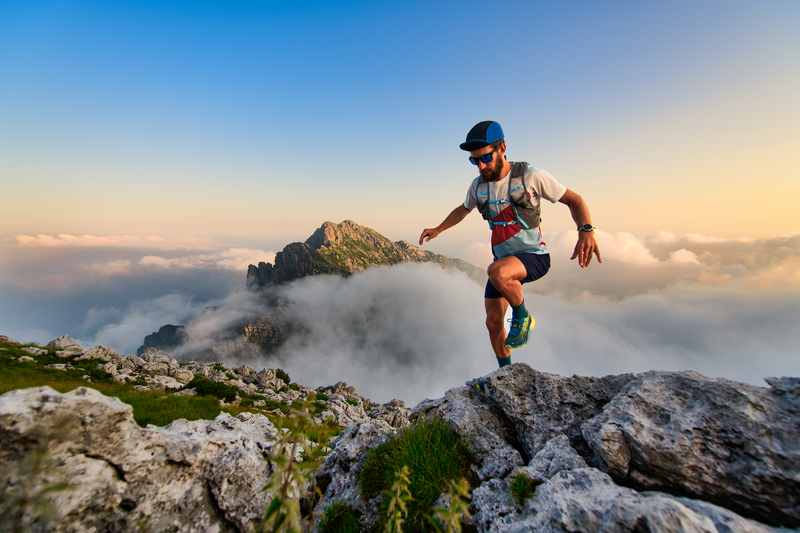
95% of researchers rate our articles as excellent or good
Learn more about the work of our research integrity team to safeguard the quality of each article we publish.
Find out more
ORIGINAL RESEARCH article
Front. Plant Sci. , 20 September 2022
Sec. Aquatic Photosynthetic Organisms
Volume 13 - 2022 | https://doi.org/10.3389/fpls.2022.981602
This article is part of the Research Topic Carbohydrates in Algae: Metabolism, Regulation, Structures, Evolution and Applications View all 5 articles
Alginate (alginic acid) is a linear polysaccharide, wherein (1→4)-linked β-D-mannuronic acid and its C5 epimer, α-L-guluronic acid, are arranged in varying sequences. Alginate lyases catalyze the depolymerization of alginate, thereby cleaving the (1→4) glycosidic linkages between the monomers by a β-elimination mechanism, to yield unsaturated 4-deoxy-L-erythro-hex-4-enopyranosyluronic acid (Δ) at the non-reducing end of resulting oligosaccharides (α-L-erythro configuration) or, depending on the enzyme, the unsaturated monosaccharide itself. In solution, the released free unsaturated monomer product is further hydrated in a spontaneous (keto-enol tautomerization) process to form two cyclic stereoisomers. In this study, two alginate lyase genes, designated alyRm3 and alyRm4, from the marine thermophilic bacterium Rhodothermus marinus (strain MAT378), were cloned and expressed in Escherichia coli. The recombinant enzymes were characterized, and their substrate specificity and product structures determined. AlyRm3 (PL39) and AlyRm4 (PL17) are among the most thermophilic and thermostable alginate lyases described to date with temperature optimum of activity at ∼75 and 81°C, respectively. The pH optimum of activity of AlyRm3 is ∼5.5 and AlyRm4 at pH 6.5. Detailed NMR analysis of the incubation products demonstrated that AlyRm3 is an endolytic lyase, while AlyRm4 is an exolytic lyase, cleaving monomers from the non-reducing end of oligo/poly-alginates.
Alginate (alginic acid) is the most abundant carbohydrate in several types of macroalgae, including marine brown seaweed (Phaeophyceae), and a major constituent of the algal cell wall and intracellular material (approximately 22–44% of the total cell dry weight) (Senturk Parreidt et al., 2018). It is a linear anionic polymer (molecular mass range 10–600 kDa) composed of (1→4)-linked β-D-mannopyranosyluronic acid (β-D-ManpA = M; 4C1 conformation) and (1→4)-linked α-L-gulopyranosyluronic acid (α-L-GulpA = G; 1C4 conformation) units (Figure 1), arranged in varying sequences, i.e., block-regions of both homo- (M- or G-blocks) and hetero- (MG- or GM-blocks) polyuronic acid sequences (Penman and Sanderson, 1972; Gacesa, 1992; Pawar and Edgar, 2012). The constituting monomers β-D-ManpA and α-L-GulpA are C-5 epimers. Alginate oligosaccharides, derived from brown seaweeds, are generally recognized as safe (GRAS) and non-toxic, and are widely used in the food, cosmetics, biochemical, and pharmaceutical industries due to their stabilizing, dehydrating, viscosifying, and gelling (with Ca2+) properties (Wong et al., 2000; Draget and Taylor, 2011; Zhang et al., 2021). Moreover, alginate oligosaccharides were demonstrated to have prebiotic activity (Wang et al., 2006). Alginate oligosaccharides and their derivatives have also been attracting attention due to their anti-inflammatory and anti-coagulant activities, and anti-tumor effects (Lee and Mooney, 2012; Chen et al., 2017; Xing et al., 2020). The constituting monomeric products of the lyase reactions may also be considered as important catalytic products as they can be converted into value-added chemicals, e.g., 2-keto-3-deoxy-gluconate (KDG). The application potential and the importance of the catalytic products of alginate is reviewed by Zhu and Yin (2015).
Figure 1. Structures of β-D-mannopyranosyluronic acid, α-L-gulopyranosyluronic acid, and 4-deoxy-α-L-erythro-hex-4-enopyranosyluronic acid units, as present in (enzymatically degraded) alginate.
The enzymes, capable of catalyzing the depolymerization of alginic acid by splitting the glycosidic bonds through a β-elimination reaction, are generally known as alginate lyases (also called alginases, alginate depolymerases, transeliminases) (Gacesa, 1987; Wong et al., 2000; Kim et al., 2011; Ertesvåg, 2015). Three types of alginate-depolymerizing enzymes have been defined, i.e., poly(α-L-guluronate) lyase (EC 4.2.2.11), poly(β-D-mannuronate) lyase (EC 4.2.2.3), and bifunctional alginate lyase (Garron and Cygler, 2010; Zhu and Yin, 2015). Additionally, alginate lyases can have either endo- or exo-cleaving specificity. Cleavage of the R1-(1→4)-β-D-ManpA-R2 or R1-(1→4)-α-L-GulpA-R2 glycosidic linkages (R1 and R2 = M- and/or G-containing saccharide) in the alginate polymers leads to a C-4,5 double bond in the D-mannuronate and/or the L-guluronate residue, yielding in both cases Δ-R2 with the new non-reducing terminal residue 4-deoxy-α-L-erythro-hex-4-enopyranosyluronic acid, designated by the symbol Δ (Figure 1; Gacesa, 1987, 1992).
A large number of alginate lyases, from sources ranging from various types of soil and marine bacteria, algae, and fungi to marine animals, have been characterized (Wong et al., 2000). In the Carbohydrate-Active Enzymes (CAZY) database (Drula et al., 2022),1 alginate lyases are assigned to different polysaccharide lyase (PL) families, based on amino acid sequence similarities. Several bacterial alginate lyase genes have been identified, cloned, and sequenced. Both native and recombinantly expressed enzymes have been characterized, giving insight into alginate lyase structure and function (Kim et al., 2009, 2011, 2012; Tøndervik et al., 2010; Hamza et al., 2011; Park et al., 2012). Few thermophilic alginate lyases have been described. A structure-function analysis of an alginate lyase from the marine thermophile Defluviitalea phaphyphila was recently reported. The enzyme, which was affiliated with a new PL family, PL39, had temperature optimum of activity at 65°C and pH optimum of activity at 5.8 (Wang et al., 2018; Ji et al., 2019). Another alginate lyase from Nitratiruptor sp. SB155-2, affiliated with family PL7, had a temperature optimum of activity at 70°C and pH optimum 6 (Inoue et al., 2016). Further, a recently described thermophilic exolytic alginate lyase of PL family 17, derived from an Arctic Mid-Ocean Ridge (AMOR) metagenomics data set, had pH optimum of activity at pH 6 and retained 100% activity after a 24-h incubation at 60°C (Arntzen et al., 2021).
Alginate lyases have been used for production of defined alginate oligomers and for protoplasting seaweed (Inoue et al., 2007; Chen et al., 2018). Their application for degradation of alginate polymers produced by Pseudomonas aeruginosa in cystic fibrosis patients has been studied (Mrsny et al., 1994; Alipour et al., 2009). Alginate lyases may also be used for the degradation of algal biomass in the production of biofuels or renewable commodity compounds (Jones and Mayfield, 2012; Wargacki et al., 2012; Doshi et al., 2016; Li et al., 2016, 2020). For utilization in those various industrial processes, robust alginate lyases which can function at extreme circumstances, such as elevated temperatures, may be of interest. Thermostability enables purification of recombinant enzymes from a mesophilic host by heat precipitation of the host’s proteins, which consequently lowers downstream production costs. Temperature is a major factor in defining the application range of an enzymatic activity, but optimum temperature of an industrial process is dependent on both substrate and products. High temperature (50–80°C) is optimal for polysaccharide degradation as it increases solubility, reduces viscosity of polysaccharides and facilitates enzymatic access. Thermostable enzymes have longer shelf lives and will tolerate prolonged reaction times and harsh conditions in bioconversion processes. Thermophilic enzymes may also streamline the integration of degradation processes with prior pre-treatment of seaweeds which is optimally carried out at elevated temperatures. Thermophilic and thermostable enzymes reduce the need for cooling from often high pre-processing temperatures of algal biomass that would be required for mesophilic enzymatic alginate degrading processes. High temperature also prevents contamination by spoilage bacteria (Turner et al., 2007).
The genome of the marine thermophile bacterium Rhodothermus marinus (strain MAT378) contains four alginate lyase genes, designated alyRm1 to alyRm4. Two of the genes, encoding alginate lyases AlyRm1 and AlyRm2 belong to the CAZY polysaccharide lyase (PL) family PL6 subclass 2 and have been shown to function as endo-poly-MG lyases (Mathieu et al., 2016). This report describes the second two thermophilic alginate lyases, AlyRm3 and AlyRm4, their substrate specificity and products. AlyRm4 belongs to the PL17 and AlyRm3 belongs to a newly described polysaccharide lyase family, PL39. The encoding genes were identified in Rhodothermus marinus, a Gram-negative, aerobic, marine thermophile belonging to a sister phylum to Bacteroidetes, Rhodothermaeota (Munoz et al., 2016). The species which has been isolated from marine habitats around the world in the proximity of hot spring vents, is a known producer of various robust polysaccharide-degrading enzymes (Bjornsdottir et al., 2006; Ara et al., 2020). The thermophilic R. marinus alginate lyases with complementing activity add to the previously described activity range of alginate lyases and may have valuable industrial application properties.
The encoding genes alyRm3 and alyRm4 were amplified by PCR, cloned into expression vector and expressed in E. coli. The resulting recombinant enzymes, AlyRm3 and AlyRm4, were characterized with regard to thermostability, and temperature and pH optimum of activity and subsequently investigated in more detail for their depolymerizing activity on mannuronan (M-block alginate), guluronan (G-block alginate), and low-viscosity alginate from Macrocystis pyrifera. The complete reaction mixtures and the products, isolated by size-exclusion chromatography (SEC), were analyzed by TLC, MALDI-TOF-MS, GLC-EI-MS, and one-/two-dimensional 1H and 13C NMR spectroscopy.
R. marinus strain MAT378/R-21 is a close relative of the type strain R. marinus DSM4252. Both strains were originally isolated from a shallow submarine hot spring in SW Iceland (Alfredsson et al., 1988). The MAT378 strain was cultivated in RSC selective medium (Bjornsdottir et al., 2007), containing 0.2% soluble starch (Merck). Pure high-molecular-weight DNA and plasmid DNA was isolated using the MasterPure Gram Positive DNA Purification kit (Lucigen) according to manufacturer’s instructions and used for genome sequencing. The genome was originally sequenced by Sanger sequencing with approximately 5x coverage. With the emergence of high throughput sequencing, the genome was re-sequenced. Libraries were prepared using the Nextera Flex and Nextera MatePair methods (Illumina) and sequenced on the Illumina MiSeq platform. The generated sequence reads were assembled by using SPAdes assembler (Bankevich et al., 2012) and annotated by using the RAST annotation server (Overbeek et al., 2014).
Putative alginate lyase encoding genes were identified by the NCBI BLAST program (non-redundant protein sequences database). Sequence Alignments were performed using the EBI ClustalW2—Multiple Sequence Alignment tool.2 Further protein domain prediction and classification into families was done using InterPro (EMBL-EBI) and SMART (EMBL) databases. Signal peptides were predicted using the SignalP-4.0 Server (CBS).
The putative alginase lyase genes, designated alyRm3 and alyRm4, were amplified from the presented genome of strain MAT378 without the predicted signal peptide sequences (aa 1–17 and 1–22, respectively). For heterologous expression in E. coli, all the alginate lyases genes were modified with an N-terminal hexa-histidine tag. Primers were designed as shown in Supplementary Table 1 to amplify the coding regions of the respective genes and introducing the restriction sites BamHI or BglII at the 5′ ends and a HindIII site behind the stop codons. The amplifications were performed using standard PCR conditions and a proofreading polymerase, the fragments cut with the corresponding restriction enzymes and inserted into the L-rhamnose inducible expression vector pJOE5751. The later contains a His6-eGFP fusion under control of the rhaPBAD promoter. The single BamHI and HindIII restriction sites in the vector allowed the replacement of the eGFP by the alginate lyase genes and fusion to the 5′ His6-tag. E. coli JM109 carrying the respective recombinant plasmids were cultivated in LB medium (200 mL), containing 100 μg/mL ampicillin. For expression of the genes, the cultures were grown at 37°C till cell density reached OD600 of 0.6 then induced by adding of 0.1% rhamnose and further grown for 4 h at 30°C. The cells were harvested by centrifugation at 4,500 × g for 20 min at 4°C, washed, resuspended in 10 mM potassium phosphate buffer, pH 6.5 and disrupted by passing them twice through a French press cell. After centrifugation (13,000 × g for 15 min at 4°C), the supernatants of the crude cell extracts and the cell pellets were analyzed by SDS-PAGE.
The purifications of recombinant alginate lyases proteins were performed by immobilized metal affinity chromatography (IMAC). The supernatant of the respective crude cell extract, containing approximately 25 mg E. coli protein, was applied onto 2 mL Talon® metal affinity resin (Clontech) in a column using gravity flow. The resin was washed with 10 mL of washing buffer (50 mM potassium phosphate, 300 mM NaCl, 5 mM imidazole, pH 7.0). Bound protein was eluted with 3 mL of elution buffer (50 mM potassium phosphate, 300 mM NaCl, 150 mM imidazole, pH 7.0). (His)6-alginate lyase-containing fractions were combined and applied onto an NAP10 column (GE Healthcare), equilibrated with 50 mM potassium phosphate, 300 mM NaCl, pH 7.0 to remove imidazole and stored at 4°C.
Protein concentration was determined using Bradford reagent (Bio-Rad) and BSA standards for preparation of a standard curve. Sodium dodecyl sulfate polyacrylamide gel electrophoresis (SDS-PAGE) was performed using the method of Laemmli (1970), standard gels and a protein standard (Fermentas). Gels were stained using Coomassie Brilliant Blue R-250 (Sigma).
Sodium M- and G-block alginates with a broad range of degrees of polymerization (DP) [M-block (ALG500), averaged DP 20, M/G ratio > 4; G-block (ALG300), averaged DP 20, G/M ratio > 3] were purchased from Elicityl OligotechR (Crolles, France). Low-viscosity sodium alginate from Macrocystis pyrifera, Kelp (Brookfield Viscosity 100–300 cps; 2% in H2O at 25°C) was purchased from Sigma-Aldrich (St. Louis, MO, United States).
The temperature optimum and stability, pH optimum, and impact of NaCl concentration (salinity) were determined for each enzyme using the M. pyrifera alginate as substrate and applying the 3,5-dinitrosalicyclic acid (DNS) method for determination of reducing sugar (Miller, 1959). Generally, the reactions were run not more than 5 min of incubation time to minimize color formation from degradation products at temperatures above 50°C, which otherwise affected the results of the DNS assay. The reaction mixture consisted of 10 μL appropriate diluted purified enzyme, 80 μL 1.25% alginic acid and 10 μL 0.5 M potassium phosphate or acetate buffer (pH 5.5 and 6.5 for AlyRm3 and AlyRm4, respectively) with a final concentration of 50 mM. The temperature optimum experiments were performed at a range from 25 to 100°C. The temperature stability was determined by estimating the temperature at which 50% enzyme activity was measured after 30 min of incubation (T1/2 temperature). I.e., the pure enzymes (1 μM) were incubated in 0.1 M potassium buffer, pH 5.5 and 6.5 for AlyRm3 and AlyRm4, respectively, during 30 min at temperatures over a range from 40 to 95°C. Following incubation, the enzyme was cooled, and the remaining activity measured at 60°C, applying DNS assay. The effect of pH on enzyme activity was tested with purified enzymes using various buffers at 0.5 M: sodium acetate (pH 4.5–5.5), sodium/potassium phosphate (pH 6.0–8.0), and Tris-HCl (pH 8.5–9.5) under standard DNS assay conditions. The effect of NaCl on the alginate lyase activity of the purified enzymes was tested in 0.1 M potassium phosphate buffer at pH 7.0 for AlyRm4 and at pH 5.5 for AlyRm3, including different concentration of NaCl. All reactions were done in triplicates. The percent activity was determined from the absorption units subtracting the blank.
Preparative quantities of incubation mixtures [3 mL solutions, containing 300 μL (0.7 U/mL) enzyme (AlyRm3 or AlyRm4), 300 μL 0.5 M phosphate buffer, pH 5.5, and 2,400 μL alginate substrate (12.5 mg/mL; M-block, G-block, or M. pyrifera alginate), incubated for 24 h at 40°C] were fractionated by size-exclusion chromatography (SEC) on Bio-Gel P-2 (isolation of dimers), P-4 (isolation of trimers) or P-6 (isolation of tetramers) columns (90 × 1 cm; Bio-Rad, Richmond, CA). Elutions, with UV detection at 235 nm, were carried out with 0.2 M NH4HCO3 at room temperature and a flow rate of 12 mL/h, and 10 min-fractions were collected and lyophilized. Depending on the oligomer series (DP) according to MALDI-TOF-MS, some fractions were pooled for further separation on adequate Bio-Gel columns.
Carbohydrate samples were spotted in 1 cm lines on TLC sheets (Merck Kieselgel 60 F254, 20 × 20 cm), which were developed with 1-butanol: acetic acid: water = 2:1:1 (v/v/v). Bands were visualized by orcinol/sulfuric acid staining (10 min for 100°C).
The distribution of the different oligomers in M-block and G-block (1.5 mg/mL, 25 μL) were analyzed on a Dionex DX500 workstation (Dionex, Amsterdam, Netherlands), equipped with an ED40 pulsed amperometric detection (PAD) system. The separation of oligosaccharides was carried out on a Ionpac AS4A column (250 × 5 mm; Dionex) by using a linear gradient of 25–300 mM sodium acetate in 100 mM NaOH (1 mL/min).
MALDI-TOF-MS experiments were performed using an Axima™ mass spectrometer (Shimadzu Kratos Inc., Manchester, United Kingdom), equipped with a nitrogen laser (337 nm, 3 ns pulse width). Negative-ion mode spectra were recorded using the reflector mode at a resolution of 5,000 Full Width at Half Maximum (FWHM) and delayed extraction (450 ns). The accelerating voltage was 19 kV with a grid voltage of 75.2%; the mirror voltage ratio was 1.12, and the acquisition mass range was 200–6,000 Da. Samples were prepared by mixing on the target 0.5 μL sample solutions with 0.5 μL aqueous 10% 2,5-dihydroxybenzoic acid as matrix solution.
Reaction mixtures, obtained after incubation of M-block alginate, G-block alginate, and M. pyrifera alginate substrates with AlyRm4 were lyophilized, and after trimethylsilylation [pyridine: hexamethyldisilazane: trimethylchlorosilane = 5:1:1 (v/v/v), 30 min at room temperature] analyzed by GLC-EI-MS. Screenings were carried out on a GCMS-QP2010 Plus instrument (Shimadzu Kratos Inc.) using an EC-1 column (30 m × 0.25 mm; Alltech/Grace, Deerfield, IL) and a temperature program of 140–250°C at 8°C/min (Kamerling and Gerwig, 2007).
Resolution-enhanced one-/two-dimensional (1D/2D) 500-MHz 1H/13C NMR spectra were recorded in D2O at pD 7.0 on a Varian Inova Spectrometer (NMR Center, University of Groningen) or on a Bruker DRX-500 Spectrometer (Bijvoet Center, Department of NMR Spectroscopy, Utrecht University) at probe temperatures of 300K or 334K. Before analysis, samples were exchanged twice in D2O (99.9 atom% D, Cambridge Isotope Laboratories, Inc., Andover, MA) with intermediate lyophilization, and then dissolved in 0.6 mL D2O. Suppression of the HOD signal using the Bruker instrument was achieved by applying a WEFT (water eliminated Fourier transform) pulse sequence for 1D experiments and by a pre-saturation of 1 s during the relaxation delay in 2D experiments. Standard “Presat” solvent signal suppression (WET1D pulse) was used in case of 1D experiments on the Varian instrument. The 2D gCOSY and TOCSY spectra were recorded using an MLEV-17 mixing sequence with spin-lock times of 20-200 ms. Natural abundance 2D 13C-1H HSQC experiments (1H frequency 500.0821 MHz, 13C frequency 125.7552 MHz) were recorded without decoupling during acquisition of the 1H Free Induction Decay (FID). All spectra were processed using MestReNova 5.3 (Mestrelabs Research SL, Santiago de Compostella, Spain). Chemical shifts (δ) are expressed in ppm by reference to internal acetone (δ 2.225 for 1H and δ 31.08 for 13C).
An alginate polysaccharide utilization locus (Alg-PUL) was identified following sequencing, assembly and annotation of the Rhodothermus marinus strain MAT378 genome as described in section “Materials and methods”. Structurally identical locus was identified in the reference strain, DSM4252, following publication of the genome sequence (Nolan et al., 2009). The locus contains three alginate lyase genes, designated alyRm1 (PL6), alyRm3 (PL39), and alyRm4 (PL17) (Figure 2). An additional alginate lyase gene alyRm2 (PL6) was identified outside the locus. The Alg-PUL also contained genes encoding catabolic enzymes, kdgF and kdgR, which activity has been verified (unpublished results), necessary for downstream funneling of final alginate degradation products into the Entner Douderoff pathway (unpublished data) as well as kdgA encoding putative 4-hydroxy-2-oxoglutarate aldolase; kdgK encoding putative 2-dehydro-3-deoxygluconate kinase. Relevant transporter genes and four hypothetical genes were also detected in the locus (Figure 2). The corresponding Alg-PUL genome sequence has been submitted to GeneBank with the accession number ON640824. The enzymes in Rhodothermus marinus DSM 4252 homologous to AlyRm4 and the AlyRm3 amino acid sequences have the GenBank numbers ACY48056.1 and ACY48059.1, respectively. Both have been annotated as Heparinase II/III family protein.
Figure 2. Alginate polysaccharide utilization locus (Alg-PUL) in R. marinus MAT378 containing the alginate lyase genes alyRm1, alyRm3 and alyRm4. Annotation of the genes from left: kdgA (642 nt) encoding putative 4-hydroxy-2-oxoglutarate aldolase; kdgK (1,026 nt) encoding putative 2- dehydro-3-deoxygluconate kinase; tonB (2,805) TonB dependent receptor; hypA (2,181 nt) encoding T9SS type A sorting domain-containing protein; hypB (1,056 nt) encoding PorV/PorQ family protein; hypC (1,398 nt) encoding hypothetical protein; alyRm1 (1,743 nt) encoding alginase; alyRm4 (2,253 nt) encoding exo-alginase; kdgR (759 nt) encoding 4-hydroxy-2-oxoglutarate reductase; kdgF (357 nt) encoding cupin domain-containing protein. The kdgR (759 nt) and kdgF (366 nt) have been verified as genes encoding 4-deoxy-L-erythro-5-hexosulose uronate (DEHU) reductase and an enzyme catalyzing linearization of unsaturated uronates from alginate, respectively; alyRm3 (2,673 nt) encoding endo-alginase; transporter gene (1,278 nt) encoding predicted mannuronate transporter; hypD (2,844 nt) encoding PD40 domain-containing protein. The colors of arrows indicate the functional role. Blue arrows; enzyme involved in degradation of alginate and production of uronic acids; Orange arrows: transport; Green arrows: enzymes for Entner Douderoff (ED) glycolytic processing of uronic acids. Gray arrows: hypothetical genes.
The predicted gene alyRm3 ORF was 2,613 nt, encoding an 870 amino acid long polypeptide. Its calculated MW is 96,623 Da and pI 5.21. A signal sequence was predicted with cleavage site after Gln-17, a heparinase II/III-like protein domain (aa 374–518) and C-terminal associated with sorting to the outer membrane and covalent modification (Muth et al., 1998). A C-terminal sorting is also found in AlyRm1 PL6 alginate lyase encoded from same cluster indicating that both enzymes are extracellular. AlyRm3 can be assigned to a newly described polysaccharide lyase enzymes in the CAZY database, i.e., family PL39 (Ji et al., 2019). Closest relatives are found various lineages of the phylum of Rhodothermaeota, homologs having 50–100% aa identity depending on source species relatedness. AlyRm3 homologs are found in other R. marinus strains, e.g., the type strain DSM 4252. It is not found in Rhodothermus bifrosti, isolated from a terrestrial hot spring. The highest sequence similarity of AlyRm3, outside the genus was found with a heparinase II/III family protein from e.g., Rhodocaloribacter litoris (66% aa identity, GenBank: WP_166976759.1). Homologs are found in other phyla albeit with distinctly lower sequence similarity including a homolog from the clostridia species Defluviitalea saccharophila with 38% amino acid identity but with similar activity on alginate.
The predicted gene alyRm4, encoding AlyRm4, consists of 2,226 nt, which translates into a 742 aa polypeptide with MW of 83,633 Da and pI 6.06. A hydrophobic sequence was detected at the N-terminal, but the C- terminal sorting domain is missing indicating that the enzyme is located in the periplasmic space. Like AlyRm3, the enzyme contains a heparinase II/III-like protein domain (aa 386–539). AlyRm4 belongs to family PL17 of polysaccharide lyases and shows highest identity to homologous proteins in Rhodothermaeota and various species from other phyla including e.g., Rhodocaloribacter litoris WP166976762, 68% identity and Vibrionales bacterium SWAT-3 (45% aa identity, GenBank: ZP_01815528.1). Other thermophilic strains containing a PL17 sequence are Rhodocaloribacter litoris, Spirochaeta thermophila, Merioribacter roseus, and the Paenibacillus strain Y412MC10.
The encoding sequences of the mature enzymes were amplified by PCR and inserted into the L-rhamnose inducible expression vector pJOE5751 with a N-terminal fusion to a hexa-histidine sequence allowing production of His6-fusion proteins. Rhamnose induction of the E. coli JM109, harboring the respective plasmids, resulted in the production of high amounts of the recombinant proteins as identified by SDS-PAGE (Supplementary Figure 1). Compared to the uninduced crude extracts, a prominent protein band of the size of ∼95 kDa for AlyRm3 and ∼81 kDa for AlyRm4, respectively, indicated a tight regulation and high expression level. Recombinantly expressed alginate lyases were purified by IMAC to homogeneity as judged by SDS-PAGE (Supplementary Figure 1).
The recombinant alginate lyases AlyRm3 and AlyRm4 were active on alginic acid substrates but not on other substrates tested such as chondroitin and chitin (data not shown). The optimal pH, temperature optimum of activity and temperature stability are summarized in Table 1.
The enzymes are thermostable with temperature optima at 75° and 81°C for AlyRm3 and AlyRm4, respectively, measured at set conditions. The T1/2 (50% activity after 30 min incubation in buffer) was 77°C for AlyRm3 and 78°C for AlyRm4. The pH optimum of activity of AlyRm3 was 5.5. The pH activity range of AlyRm4 was 6.0–7.5 (>70%) with optimum at pH 6.5. As the thermophilic alginate lyases are derived from marine bacterium, the optimum salinity concentration was studied. Both enzymes were relatively stable at NaCl concentrations up to 1M (Supplementary Figures 2, 3).
In order to determine the substrate-depolymerizing specificity of the AlyRm3 enzyme, incubation experiments (0–24 h, pH 5.5, 40°C) were performed with M-block alginate, G-block alginate, and low-viscosity M. pyrifera alginate as substrates. Calculated by the peak integrals of specific protons in the 1H NMR spectrum, the low-viscosity M. pyrifera alginate contained an M:G ratio of 61:39. The enzymatic depolymerization reactions were followed by TLC, MALDI-TOF-MS, and 1H NMR spectroscopy.
As shown in the TLC analysis of the 24-h incubations (Figure 3), the three different substrates were depolymerized into mainly di-, tri-, and tetrasaccharides with a 4,5-unsaturated non-reducing-end uronic acid (Δ), indicating that the enzyme can cleave M-M, G-G, M-G, and G-M linkages via a β-elimination mechanism, which was confirmed by 1H NMR spectroscopy of isolated products (see below). Note that the starting materials have completely disappeared.
Figure 3. TLC analysis of the oligosaccharide mixtures generated after a 24-h incubation of (A) M-block alginate, (B) G-block alginate, and (C) low-viscosity M. pyrifera alginate with the AlyRm3 enzyme. Positions of isolated and identified products with non-reducing terminal 4-deoxy-α-L-erythro-hex-4-enopyranosyluronic acid (Δ) units are included (NMR analysis). Non-assigned bands are expected to stem from saturated oligosaccharides (MALDI-TOF-MS analysis). As the samples were run with several other samples, the 0-h control lanes and the lanes with the digested alginate samples were spliced for a clearer presentation.
The negative-ion mode MALDI-TOF mass spectra of the three oligosaccharide mixtures (spectra not shown), obtained after a 24-h incubation, showed [M-H]– peaks at m/z 351.0, 526.8, and 703.0, corresponding to di-, tri-, and tetrasaccharides, respectively, each with a 4,5-unsaturated non-reducing-end uronic acid (Δ). Furthermore, weak [M-H]– peaks at m/z 369.2, 544.9, and 721.0 were also observed, in agreement with the presence of saturated di-, tri-, and tetrasaccharides, respectively. In the case of the G-block alginate incubation, additional small [M-H]– peaks at m/z 879.0, 897.0, and 1073.3 were seen, corresponding with ΔGGGG, GGGGG, and GGGGGG, respectively.
To facilitate the NMR analysis of the oligosaccharide mixtures obtained after a 24-h incubation, generated unsaturated di-, tri-, and tetrasaccharides were isolated via fractionations on Bio-Gel P-2, P-4, and P-6, respectively. The 1D 1H NMR spectra of ΔM, ΔMM, ΔMMM, ΔG, and ΔGG are presented in Supplementary Figure 4. For detailed 1H and 13C assignments, gCOSY, TOCSY (mixing times 20, 50, 150, and 200 ms), and HSQC experiments were carried out. As an example, the 2D TOCSY (150 ms) and HSQC spectra of ΔM are presented in Supplementary Figure 5. The 1H/13C NMR chemical shift data of ΔG, ΔGG, ΔM, and ΔMM have been collected in Table 2. Note the specific Δ H-1 and Δ H-4 chemical shifts in case of Δ-M-R and Δ-G-R (R = M- and/or G-containing saccharide). Furthermore, it is of importance to note that the pH (pD) can influence chemical shifts, in particular the H-5 proton. Besides our own generated NMR chemical shift database, also previously published NMR data on alginate poly- and oligosaccharides were taken into account for resonance assignments (Grasdalen et al., 1979, 1981; Grasdalen, 1983; Steginsky et al., 1992; Heyraud et al., 1996; McIntyre et al., 1996; Zhang et al., 2004; Holtan et al., 2006; Li et al., 2011; Lundqvist et al., 2012; Hu et al., 2013).
The 500-MHz 1H NMR spectra at t = 0 h and t = 24 h of the M-block alginate incubation are presented in Figure 4, and those of the G-block alginate incubation in Figure 5. The average DPs (∼DPs) of the commercial M- and G-block alginates were checked by 1H NMR spectroscopy via integration (I) of the anomeric 1H signals in the respective spectra [∼DPM–block = (IM1 + IMred1α+β)/IMred1α+β and ∼DPG–block = (IG1 + IGred1α+β)/IGred1α+β], yielding ∼DPM–block 22 and ∼DPG–block 25 (Figures 4A, 5A). The HPAEC-PAD profiles presented in Supplementary Figure 6, give an impression of the degree of polymerization or chain length distribution in M-block and G-block alginate. As is evident from the changes in the NMR profiles, going from Figure 4A to Figure 4B and from Figure 5A to Figure 5B, the enzyme is clearly acting as a lyase for both poly-M and poly-G homopolymeric regions. The formation of ΔM from the M-block alginate is demonstrated by the characteristic Δ H-4 resonances at δ 5.82 (coded Δ 4Mα) and δ 5.77 (coded Δ 4Mβ) (ratio ∼ 3:1), whereas the intense signal at δ 5.76 reflects the presence of ΔM(M)n (coded Δ 4MM) (Figure 4B and Table 2; compare Supplementary Figure 4). Note that the MALDI-TOF-MS analysis showed mainly ΔM, ΔMM, and ΔMMM as end products (see above). For the G-block alginate, the appearance of Δ H-4 signals at δ 5.92 (coded Δ 4G) and δ 5.86 (coded Δ 4GG) indicated the presence of ΔG and ΔG(G)n, respectively (Figure 5B and Table 2; compare Supplementary Figure 4). Remarkably, the reducing end residue G (coded G red) has mainly β configuration, while the reducing end residue M (coded M red) showed a configuration ratio of α/β = 2:1. Noteworthy, MALDI-TOF-MS analysis showed mainly ΔG, ΔGG, and ΔGGG as end products.
Figure 4. 1D 500-MHz 1H NMR spectra (300K, D2O) of (A) the M-block alginate substrate before addition of the AlyRm3 enzyme, and (B) the oligosaccharide mixture generated after a 24-h incubation of the M-block alginate substrate with AlyRm3. M 1 to M 5 mean H-1 to H-5 of internal M residues; M red1α and M red1β mean H-1 of the reducing end α-M and β-M residue, respectively; Δ 4Mα/Δ 1Mα, Δ 4Mβ/Δ 1Mβ, and Δ 4MM/Δ 1MM mean H-4/H-1 of Δ in ΔMα, ΔMβ, and ΔM(M)n, respectively (for δ values, see Table 2).
Figure 5. 1D 500-MHz 1H NMR spectra (300K, D2O) of (A) the G-block alginate substrate before addition of the AlyRm3 enzyme, and (B) the oligosaccharide mixture generated after a 24-h incubation of the G-block alginate substrate with AlyRm3. Assignment according to Chen et al. (2018). G 1 to G 5 mean H-1 to H-5 of internal G residues; G red1α and G red1β mean H-1 of the reducing end α-G and β-G residue, respectively; Δ 4G/Δ 1G and Δ 4GG/Δ 1GG mean H-4/H-1 of Δ in ΔGβ and ΔG(G)n, respectively (for δ values, see Table 2).
Making use of the NMR data obtained above for the M- and G-block alginate/oligosaccharide, the depolymerization of the low-viscosity alginate from M. pyrifera with the AlyRm3 enzyme was studied in more detail. The 1H NMR spectrum of the starting material, recorded at 334K instead of 300K to increase solubility and sharper resonance peak recovery (Figure 6A), showed in the δ 4.4-5.1 region the anomeric proton signals. The GulpA H-1 signals resonate at δ∼5.03 [(G or M)G 1G + (G or M)G 1M] and the ManpA H-1 signals at δ 4.70 (M 1G) and δ 4.66 (M 1M). The GulpA H-5 signals are present at δ 4.47 (GG 5G) with a shoulder at the right side for MG 5G (δ 4.43) and at δ 4.73 (MG 5M) with a shoulder at the left side for GG 5M (δ 4.76). Note that a preceding G residue (GG 5G vs. MG 5G and GG 5M vs. MG 5M) leads to a slightly higher chemical shift for G H-5, and that M H-1 in the M-G diad resonates at a slightly higher chemical shift than in the M-M diad (Grasdalen et al., 1979; Grasdalen, 1983). The ManpA H-5 signals resonate in the bulk region at δ∼3.75 (compare Figure 4). The M/G ratio was calculated from the relative integral areas (I) in the anomeric region, as described by Grasdalen et al. (1979), Grasdalen (1983) and Zhang et al. (2004). Hereto, the integration limits applied in the calculations of the area of the peak regions A, B and C were set to 4.97–5.10 ppm, 4.60–4.80 ppm, and 4.40–4.50 ppm, respectively (Figure 6A). Calculation of the percentage G and M monads in the M. pyrifera alginate, using the formula FG = IA /IB + IC, resulted in 39% G and 61% M, and therefore a M/G ratio of 1.6. In a similar way, the percentage GG diads followed from the formula FGG = IC /IB + IC, yielding 20% GG. As FG = FGG + FGM, FM = FMM + FMG, and FGM = FMG, the other diads comprise 19% GM, 19% MG, and 42% MM.
Figure 6. 1D 1H NMR spectra of (A) (334K, D2O) M. pyrifera alginate substrate before addition of the AlyRm3 enzyme, and (B) (300K, D2O) the oligosaccharide mixture generated after a 24-h incubation of the alginate with the AlyRm3 enzyme. For the coding system in panel (A), see text; for the coding system in panel (B), see Figures 4, 5. M 1Mredα and M 1Mredβ/M 1M mean M H-1 of internal M-residues.
The oligosaccharide mixture obtained after a 24-h incubation of the M. pyrifera alginate with the AlyRm3 enzyme was subjected to NMR analyses, and the assignments of resonance peaks were obtained by 2D TOCSY and HSQC experiments. The 1D 1H NMR spectrum (Figure 6B) showed anomeric signals for both reducing-end G and M residues. The G red1β resonance at δ 4.88 (J1,2 = 8.5 Hz) reflects a lyase activity of AlyRm3 on GM and/or GG diads, whereas the M red1α and M red1β resonances at δ 5.25 and δ 4.93, respectively, demonstrate such an activity on MM and/or MG diads (note that the M red1α signal at δ 5.25 overlapped the very minor G red1α resonance) (Table 2). Non-reducing-end Δ residues, formed via β-elimination from both M and G residues during the lyase cleavage reaction, were traced by their H-4 signals, thereby giving information about neighboring uronic acid residues. The Δ H-4 signals detected at δ 5.92 (Δ 4G) and δ 5.86 (Δ 4GG) indicate the presence of ΔG and ΔGGR -type oligosaccharides, respectively, whereas the Δ H-4 signals seen at δ 5.82/5.77 (Δ 4Mα/Δ 4Mβ) and δ 5.76 (Δ 4MM) revealed the presence of ΔM and ΔMMR -type oligosaccharides, respectively (R = M- and/or G-containing saccharide) (Table 2). The presence of Δ 4GM (δ 5.87) and Δ 4MG (δ 5.78) signals (Heyraud et al., 1996) could not be positively observed, due to partial signal overlap in the δ 5.75–5.95 region. Furthermore, the G H-5 signals (δ∼4.75) in the 1H NMR spectrum of the alginate substrate stemming from R MG 5MR and R GG 5MR parts (Figure 6A) have disappeared in the 24-h incubation mixture (Figure 6B). Therefore, the signal at δ 5.04 belongs to H-1 of internal G residues (G 1). The anomeric protons stemming from M residues next to the reducing end (e.g., ΔM M and ΔMM M) are observed at δ 4.70 (M 1Mredα) and δ 4.65 (M 1Mredβ), due to the α/β configuration of the reducing-end M residue. TLC (Figure 3) and MALDI-TOF-MS analyses supported the presence of ΔM, ΔMM, ΔMMM, ΔG, ΔGG, and ΔGGG in the product mixture after the 24-h incubation of M. pyrifera alginate with AlyRm3.
The Δ, G and M distribution (F) in the AlyRm3 lyase-degraded M. pyrifera alginate (original distribution: 39% G and 61% M; see above) was calculated by integration (I) of specific NMR peaks, using the following equations: FG=IGred 1α+IGred 1β+IG 1, FM=IMred 1α+IMred 1β+ IM 1Mredα+IM 1Mredβ and FΔ=IΔ 1G+IΔ 1GG+IΔ 1Mα+IΔ 1Mβ+ IΔ 1MM (or FΔ=IΔ 4G+IΔ 4GG+IΔ 4Mα+IΔ 4Mβ+Δ 4MM). This resulted in 49% Δ, 9% G, and 42% M.
The larger decrease of G residues (39% → 9%) compared to the decrease of M residues (61% → 42%) might indicate a higher conversion of G units into non-reducing Δ residues. This could mean a preferential cleavage between R M-GR and/or R G-GR (R = M- and/or G-containing saccharide) by the AlyRm3 enzyme, despite the majority of M-M bonds in M. pyrifera alginate. The integrals of the M red1α/β signals (δ 5.25/4.93) predominated over those of the G red1α/β signals (δ 5.23/4.88), indicating a preferred splitting of the R M-GMR bonds over the R G-GMR bonds.
Moreover, the integrals ratio 4:1 of (Δ 4Mα + Δ 4Mβ + Δ 4MM) and (Δ 4G + Δ 4GG) supported the preferential cleavage between R M-GMR over R M-GGR, and eventually R G-GMR over R M-GGR (R = M- and/or G-containing saccharide). However, a higher rate of cleavage of R M-GMR bonds (yielding ΔG at the non-reducing ends and Mred at the reducing ends) is also possible, in view of the low presence of only 20% GG in M. pyrifera alginate (see above). Nevertheless, the results from the incubation of the M-block alginate (∼DP 22) with AlyRm3 (Figure 4) showed the enzyme’s capability of cleaving M-M bonds (yielding oligosaccharides having ΔM at the non-reducing ends and Mred at the reducing ends). The average degree of polymerization (DPn) of the products from the AlyRm3-degraded alginate substrate was estimated to be 2.3, using the following equation: DPn=(IΔ1G+IΔ1M+IM1+IG1+IMred+IGred)/[IΔ 1G+IΔ 1M+IMred+IGred)/2] (Ertesvåg et al., 1998). This is in reasonable agreement with the products, ranging from disaccharides to tetrasaccharides, as observed by TLC and MALDI-TOF-MS analysis.
The different observations indicated that within 24-h AlyRm3 can completely depolymerize M-block alginate (∼DP 22) into mainly ΔM, ΔMM, and ΔMMM (di-, tri-, and tetrasaccharides) and G-block alginate (∼DP 25) into ΔG, ΔGG, and ΔGGG (di-, tri-, and tetra-saccharides). So, for both M- and G-block alginates the high content of di-, tri-, and tetrasaccharides seems to indicate that the smallest substrate of the AlyRm3 is a tetrasaccharide, acting as a minimal recognition pattern. As deduced from the results of the incubations of AlyRm3 with M. pyrifera alginate, for alternating GMGM blocks, depolymerization mainly occurs into ΔGR due to the preferential cleaving of the R M-GMR bond (R = GMGMG-saccharide). So far, the following degradation pattern of alginic acid by AlyRm3 lyase can be proposed:
…M↓GMM↓GG↓GGG↓GGGG↓GM↓MMM↓MMMM↓ GM↓GM↓GMM↓M…
…M, ΔMM, ΔG, ΔGG, ΔGGG, ΔM, ΔMM, ΔMMM, ΔM, ΔM, ΔMM, Δ…
In a similar way, as described for the AlyRm3 enzyme, for the determination of the substrate specificity of the AlyRm4 enzyme, incubation experiments (0–24 h, pH 5.5, 40°C) were performed with M-block alginate (∼DP 22), G-block alginate (∼DP 25), and low-viscosity M. pyrifera alginate (M:G ratio of 61:39) as substrates. The enzymatic depolymerization reactions were followed by TLC and 1H NMR spectroscopy.
TLC analysis of the 24-h incubations revealed that the enzyme can almost completely depolymerize the M-block and M. pyrifera alginates into monomers, as shown by the intense decrease of the spot at the origin on the TLC plate (Figures 7A,C). The G-block alginate, however, was only partially degraded into monomers after 24-h, as demonstrated by the left-over spot at the origin on the TLC plate (Figure 7B). Interestingly, no intermediate oligosaccharide bands could be detected, suggesting an exclusive exolytic activity of AlyRm4 on the three substrates. The assignment of the spots (Figure 7) correlated with isolated and identified products (see below).
Figure 7. TLC analysis of the saccharide mixtures generated after a 24-h incubation of (A) M-block alginate, (B) G-block alginate, and (C) low-viscosity M. pyrifera alginate with the AlyRm4 enzyme.
The product mixtures, obtained after the 24-h incubations of the M-block, G-block, and M. pyrifera alginates, were further studied by 1H NMR spectroscopy (Supplementary Figure 7). In all three cases, the typical signals reflecting the presence of 4,5-unsaturated uronic acids (e.g., the Δ H-4 resonances in the δ 5.7–5.9 region) were absent, indicating, when compared with the AlyRm3 product mixtures (see Figures 4–6), a different depolymerization mechanism exists for AlyRm4.
Inspection of the 1D 1H NMR spectra of the product mixture of the three alginates (Supplementary Figure 7) showed only minor signals of residual free or reducing M (M red1α, δ 5.22; M red1β, δ 4.90) and/or free or reducing G (G red1α, δ 5.23; G red1β, δ 4.88). However, in the case of the G-block alginate incubation, intense signals (G1, δ 5.03; G2, δ 3.90; G3, δ 3.99; G4, δ 4.10; G5, δ 4.45) of remaining polymeric/oligomeric material were visible (Supplementary Figure 7B), supporting the TLC indications by the residual spot at the origin (Figure 7). To generate information about the origin of the intense signals, coded A and B in each NMR spectrum, the product mixture of the M. pyrifera alginate incubation was fractionated on Bio-Gel P-2, yielding two fractions denoted I and II. Fraction I contained free ManpA (M) and free GulpA (G) (NMR analysis; spectrum not shown), which could be explained as derived from non-reducing ends of different DP chains of the alginate substrate. Fraction II was studied by 1D/2D NMR spectroscopy (Figure 8 and Supplementary Figure 8) and GLC-EI-MS (Supplementary Figure 9), showing that the fraction contained two saturated cyclic monomers identical with 4-deoxy-L-erythro-5-hexosulose uronic acid [2,4,5,6-tetrahydroxy-pentahydro-pyran-2-carboxylic acid/1,2,3,5-tetrahydro-4H-pyran-5-carboxylc acid (TPC)]. As depicted in Figure 9, it is suggested that free Δ (4-deoxy-L-erythro-hex-4-enopyranosyluronic acid), released from alginic acid via an exolytic AlyRm4-catalyzed β-elimination reaction, is converted into its open chain form, which is further hydrated in a spontaneous process in aqueous solution to form the cyclic hemiacetal stereoisomers (Enquist-Newman et al., 2014). Endolytic activity is excluded because oligomers with Δ in a non-reducing position were not detected, which means that AlyRm4 cleaves monomers one by one from the reducing end. In this context, it should be noted that many lyases catalyze the hydration of bound terminal Δ units, with subsequent rearrangements, resulting in glycosidic bond cleavage (Enquist-Newman et al., 2014; Hobbs et al., 2016). If the enzyme would have a reductase activity, then the released Δ is non-enzymatically converted into 4-deoxy-L-erythro-5-hexosulose uronic acid (DEHU), then reduced to 2-keto-3-deoxy-D-gluconate (KDG), and further metabolized through the Entner-Douderoff pathway (Preiss and Ashwell, 1962a,b). However, in our case, the hypothesis that AlyRm4 has reductase activity can be excluded. The proton at C4 is added in a later spontaneous process (keto-enol tautomerization) in solution.
Figure 8. 1D 500-MHz 1H NMR spectrum (300K, D2O) of Bio-Gel P-2 fraction II (cyclic stereoisomers A and B), obtained after 24-h of incubation of alginic acid with AlyRm4. Assigned according to Enquist-Newman et al. (2014). For 2D TOCSY and HSQC spectra, see Supplementary Figure 8. A1 means H-1 of structure A (red), etc., B1 means H-1 of structure B (green), etc. (for structures, see Figure 9).
Figure 9. Conversion of 4-deoxy-L-erythro-hex-4-enopyranosyluronic acid into two cyclic hemiacetal structures in an aqueous solution.
Note that, in theory, any stereochemical combination at C1 and C5 can take place during the ring closure process, yielding four products. As is clear from the 1H NMR spectrum (Figure 8), only two sets of ring protons are seen in a peak ratio of 1.0:0.8, indicating the presence of two main cyclic structures A (H1: δ 5.01, J1,2 3.5 Hz) and B (H1: δ 4.98, J1,2 6.0 Hz) in equilibrium. The full 1H and 13C NMR data of both stereoisomers A and B are presented in Table 3, and the TOCSY (200 ms) and HSQC spectra in Supplementary Figure 8.
Table 3. 1H and 13C chemical shiftsa and coupling constantsb of saturated cyclic structures A and B (Figure 9), generated by the incubation of M-block, G-block, and M. pyrifera alginates with AlyRm4, recorded in D2O at 300K.
GLC-EI-MS analysis of the incubation mixture of the M. pyrifera alginate (24 h, 40°C), after direct lyophilization and trimethylsilylation, showed a major GLC peak (Rt 10.2 min) with a shoulder of which the EI-MS fragmentation patterns were in agreement with the trimethylsilylated (TMS) proposed six-membered structures A and B (MW 554 Da) (Supplementary Figure 9). Both stereoisomers could not be separated on the GLC column under the conditions used. The presence of phosphate buffer was reflected by the presence of the dominant GLC peak of trimethylsilylated phosphate [characteristic ions at m/z 314 (M) and 299 (M minus CH3)]. Surprisingly, a series of additional small peaks at the beginning of the gas chromatogram gave mass spectra that could be correlated with small organic acids products, such as glyceric acid, malic acid, (hydroxylated) glutaric acids and others (Supplementary Table 2 and Supplementary Figure 10). When the incubation of the M. pyrifera alginate with the AlyRm4 enzyme was performed at 65°C instead of 40°C, the GLC-EI-MS analysis revealed an increase of organic acid formation. It is suggested that the intermediate open chain 4-deoxy-L-erythro-hexosulose uronic acid (DEHU) in Figure 9 is the precursor of these non-enzymatic generation of organic acid products. The formation of high amounts of dicarboxylic acids was promoted by increasing the incubation temperature (data not shown). However, it still remains unclear how these products are generated and further study is in progress.
Finally, the 1H NMR spectrum of the G-block alginate, after a 24-h incubation with AlyRm4 at pH 5.5 and 40°C, showed the uncomplete depolymerization of the G-block by a broad signal of anomeric protons of internal G residues (G1) at δ 5.03, together with minor signals at δ 5.23 (G red1α) and δ 4.87 (G red1β) of Gα/β of non-degraded G-block alginate (Supplementary Figure 7B). Nevertheless, an exolytic activity of AlyRm4 is demonstrated by the presence of the signals belonging to the cyclic, saturated stereoisomers A and B, stemming from released reducing ends initially present at different length chains of the substrate (∼DP25).
Taking together, the absence of Δ in all three incubations with AlyRm4 clearly demonstrates that the lyase activity occurs only from the reducing end. Even when the G-block alginate was not completely degraded by the AlyRm4 enzyme, unsaturated oligomers with a Δ unit at the non-reducing end (e.g., ΔGGG) were not detected. Evidently, the free unsaturated monomers, released via β-elimination, are immediately converted to the two cyclic saturated monomers (A and B, Figure 9) and, eventually, into small organic acids, depending on the incubation temperature. As observed so far, all substrates were degraded, suggesting that the AlyRm4 enzyme has a broad substrate tolerance and can cleave M-M, M-G, G-M and G-G bonds, with a higher specificity/depolymerizing rate for MM homopolymeric sequences than for GG homopolymeric sequences. From the obtained analysis data, it can be concluded that the AlyRm4 lyase completely depolymerizes alginic acid into unsaturated monomers, which are mostly converted into cyclic monomers A and B and small organic acids, under the used conditions at elevated temperature:
Based on sequence similarities, alginate lyases have been assigned to the polysaccharide lyase families PL5, PL6, PL7, PL14, PL15, PL17, and PL183 (Garron and Cygler, 2010; Zhu and Yin, 2015; Mathieu et al., 2016; Drula et al., 2022). Most bacterial alginate lyases, according to their primary structure, belong to the polysaccharide lyase families PL5 and PL7, and their molecular masses range from 25 to > 60 kDa (Lombard et al., 2010). The enzymes are generally classified according to their dominant typical cleaving action as poly-M lyase [(1→4)-β-D-mannuronan lyase; EC 4.2.2.3; PL5 (PL15 and PL17)] and poly-G lyase [(1→4)-α-L-guluronan lyase; EC 4.2.2.11; PL7] (Wong et al., 2000; Yamasaki et al., 2005; Kim et al., 2011). Furthermore, their three-dimensional arrangements allow grouping them into three structural classes, displaying either an α/α6 helix-barrel fold (Yoon et al., 1999) or a β-sandwich (jelly-roll) fold (Osawa et al., 2005). Several alginate lyases have been cloned and characterized from various sources (Zhu and Yin, 2015). In case of the alginate degrading thermophilic bacterium R. marinus strain MAT378/R-21, the (recombinant) AlyRm4 enzyme belongs to the PL17 polysaccharide lyase family, whereas the (recombinant) AlyRm3 enzyme belongs to the recently defined family PL39 (Ji et al., 2019).
Compared to other reported thermophilic alginate lyases, the recombinant AlyRm3 and AlyRm4 are highly thermostable, with T1/2, in absence of substrate, (50% activity after 30 min incubation in buffer) of 77°C and 78°C for AlyRm3 and AlyRm4, respectively. This makes them among the most thermostable alginates lyases that have been described to date, along with AMOR_PL17A, derived from an Arctic Mid-Ocean Ridge (AMOR) metagenomics data set (Arntzen et al., 2021).
Temperature and pH optimum, stability and optimum salinity determinations were carried out applying DNS assays, measuring the release of reducing sugars quantified as glucose equivalents. At higher temperatures (above 55°C), a dark brown color was formed in the reaction solutions and formation of dicarboxylic acids was detected. The DNS assay was adopted accordingly by limiting the time of the enzyme reaction. However, due to the color formation at high temperatures, measuring the specific enzyme activities close to temperature optimum applying DNS assay was considered imprecise, and not reported here.
The thermophilic AlyRm3 and AlyRm4 alginate lyases differ in depolymerizing activity, but together they cover a wide activity range. Our results showed that the endolytic AlyRm3 enzyme can cleave any of the four types of bonds (M-M, G-G, M-G, and G-M), with a slight preference for M-M bonds, and completely degrade alginate into unsaturated di-, tri-, and tetrasaccharides. Interestingly, the exolytic AlyRm4 lyase appears to cleave any of the two reducing end types (G and M) from alginate chains, releasing the unsaturated monosaccharide 4-deoxy-L-erythro-5-hexosulose uronic acid (DEHU), Δ, which spontaneously converts into cyclic monomers. Increasing the incubation temperature resulted in the formation of carboxyl organic acid products (Aoyagi et al., 2008). It must be noted that poly-MG lyases, showing exolytic activity, are very rarely found in nature.
The enzymes, described in this report, can be used as biocatalyst for saccharification of alginate, since they can efficiently degrade polyM, polyG, polyMG blocks, alginate oligosaccharides, and alginate to produce specific molecules with defined properties optimally suited for a given application (“tailor-made alginate”). The enzymatic degradation of alginate can be either selective or complete depending on the choice of enzymes. For instance, the recombinant AlyRm3 converted alginate into unsaturated di-, tri- and tetrasaccharides, while AlyRm4 depolymerized ∼95% of alginate into monomers. High efficiency of the reactions could be explained by the fact that the incubations were performed at elevated temperatures, where the solubility of alginate increases and viscosity decreases, facilitating enzymatic access. Robust alginate lyases, active in such extreme circumstances, may be of great interest for biofuel and chemical industries.
The two alginate lyases described in this report are highly thermostable. Detailed NMR analysis of the incubation products demonstrated that AlyRm3 is an endolytic lyase, while AlyRm4, as an exolytic lyase, cleaves monomers from the non-reducing end of oligo/poly-alginates. The two enzymes can be used separately for selective partial degradation of alginate, or in combination for complete degradation of alginate for production of monomer residues. Their application in industrial processes, where higher processing temperatures are required or preferred, is of substantial interest.
The data presented in the study are deposited in the GenBank repository, accession number ON640824.
All authors listed have made a substantial, direct, and intellectual contribution to the work, and approved it for publication.
This work was supported by EU FP7 grant 265992, AMYLOMICS; BBI grant MACROCASCADE 720755 and the BlueBio Cofund consortium grant, MARIKAT, Grant Icelandic Technology Development Fund 2011273-0611.
The authors declare that the research was conducted in the absence of any commercial or financial relationships that could be construed as a potential conflict of interest.
All claims expressed in this article are solely those of the authors and do not necessarily represent those of their affiliated organizations, or those of the publisher, the editors and the reviewers. Any product that may be evaluated in this article, or claim that may be made by its manufacturer, is not guaranteed or endorsed by the publisher.
The Supplementary Material for this article can be found online at: https://www.frontiersin.org/articles/10.3389/fpls.2022.981602/full#supplementary-material
1D and 2D, one- and two-dimensional; DP, degree of polymerization; G, α -L-guluronic acid; g-COSY, gradient correlation spectroscopy; GLC-EI-MS, gas-liquid chromatography—electron impact mass spectrometry; GRAS, generally recognized as safe; HPAEC-PAD, high pH anion-exchange chromatography with pulsed amperometric detection; HSQC, 1H detected heteronuclear single quantum coherence spectroscopy; M, β -D-mannuronic acid; MALDI-TOF-MS, matrix-assisted laser desorption time-of-flight mass spectrometry; MLEV, composite pulse devised by M. Levitt; NMR, nuclear magnetic resonance; SEC, size-exclusion chromatography; TLC, thin-layer chromatography; TOCSY, total correlation spectroscopy; WEFT, water eliminated Fourier transform.
Alfredsson, G. A., Kristjansson, J. K., Hjorleifsdottir, S., and Stetter, K. O. (1988). Rhodothermus marinus, gen-nov, sp-nov, a thermophilic, halophilic bacterium from submarine hot springs in iceland. J. Gen. Microbiol. 134, 299–306. doi: 10.1099/00221287-134-2-299
Alipour, M., Suntres, Z. E., and Omri, A. (2009). Importance of DNase and alginate lyase for enhancing free and liposome encapsulated aminoglycoside activity against Pseudomonas aeruginosa. J. Antimicrob. Chemother. 64, 317–325. doi: 10.1093/jac/dkp165
Aoyagi, H., Ishii, H., Ugwu, C. U., and Tanaka, H. (2008). Effect of heat-generated product from uronic acids on the physiological activities of microbial cells and its application. Bioresour. Technol. 99, 4534–4538. doi: 10.1016/j.biortech.2007.08.036
Ara, K. Z. G., Månberger, A., Gabriško, M., Linares-Pastén, J. A., Jasilionis, A., Friðjónsson, ÓH., et al. (2020). Characterization and diversity of the complete set of GH family 3 enzymes from Rhodothermus marinus DSM 4253. Sci. Rep. 10:1329. doi: 10.1038/s41598-020-58015-5
Arntzen, M. Ø, Pedersen, B., Klau, L. J., Stokke, R., Oftebro, M., Antonsen, S. G., et al. (2021). Alginate degradation: Insights obtained through characterization of a thermophilic exolytic alginate lyase. Appl. Environ. Microbiol. 87:e02399–20. doi: 10.1128/AEM.02399-20
Bankevich, A., Nurk, S., Antipov, D., Gurevich, A. A., Dvorkin, M., and Kulikov, A. S. (2012). SPAdes: A new genome assembly algorithm and its applications to single-cell sequencing. J. Comput. Biol. 19, 455–477. doi: 10.1089/cmb.2012.0021
Bjornsdottir, S. H., Blondal, T., Hreggvidsson, G. O., Eggertsson, G., Petursdottir, S., Hjorleifsdottir, S., et al. (2006). Rhodothermus marinus: Physiology and molecular biology. Extremophiles 10, 1–16. doi: 10.1007/s00792-005-0466-z
Bjornsdottir, S. H., Fridjonsson, O. H., Kristjansson, J. K., and Eggertsson, G. (2007). Cloning and expression of heterologous genes in Rhodothermus marinus. Extremophiles 11, 283–293. doi: 10.1007/s00792-006-0037-y
Chen, J., Hu, Y., Zhang, L., Wang, Y., Wang, S., and Zhang, Y. (2017). Alginate oligosaccharide DP5 exhibits antitumor effects in osteosarcoma patients following surgery. Front. Pharmacol. 8:623. doi: 10.3389/fphar.2017.00623
Chen, Y., Dou, W., Li, H., Shi, J., and Xu, Z. (2018). The alginate lyase lyase from Isotericola halotolerans CGMCC 5336 as a new tool for the production of alginate oligosaccharides with guluronic acid as reducing end. Carbohydr. Res. 470, 36–41. doi: 10.1016/j.carres.2018.06.005
Doshi, A., Pascoe, S., Coglan, L., and Rainey, T. J. (2016). Economic and policy issues in the production of algae-based biofuels: A review. Renew. Sust. Energ. Rev. 64, 329–337. doi: 10.1016/j.rser.2016.06.027
Draget, K. I., and Taylor, C. (2011). Chemical, physical and biological properties of alginates and their biomedical implications. Food Hydrocoll. 25, 251–256. doi: 10.1016/j.foodhyd.2009.10.007
Drula, E., Garron, M.-L., Dogan, S., Lombard, V., Henrissat, B., and Terrapon, N. (2022) The carbohydrate-active enzyme database: functions and literature. Nucleic Acids Res. 50, D571–D577. doi: 10.1093/nar/gkab1045
Enquist-Newman, M., Faust, A. M. E., Bravo, D. D., Santos, C. N. S., Raisner, R. M., Hanel, A., et al. (2014). Efficient ethanol production from brown macroalgae sugars by a synthetic yeast platform. Nature 505, 239–243. doi: 10.1038/nature12771
Ertesvåg, H. (2015). Alginate-modifying enzymes: Biological roles and biotechnological uses. Front. Microbiol. 6:523. doi: 10.3389/fmicb.2015.00523
Ertesvåg, H., Erlien, F., Skjåk-Bræk, G., Rehm, B. H. A., and Valla, S. (1998). Biochemical properties and substrate specificities of a recombinantly produced Azotobacter vinelandii alginate lyase. J. Bacteriol. 180, 3779–3784. doi: 10.1128/JB.180.15
Gacesa, P. (1987). Alginate-modifying enzymes: A proposed unified mechanism of action for the lyases and epimerases. FEBS Lett. 212, 199–202. doi: 10.1016/0014-5793(87)81344-3
Gacesa, P. (1992). Enzymic degradation of alginates. Int. J. Biochem. 24, 545–552. doi: 10.1016/0020-711X(92)90325-U
Garron, M. L., and Cygler, M. (2010). Structural and mechanistic classification of uronic acid-containing polysaccharide lyases. Glycobiology 20, 1547–1573. doi: 10.1093/glycob/cwq122
Grasdalen, H. (1983). High-field, 1H-n.m.r. spectroscopy of alginate: Sequential structure and linkage conformations. Carbohydr. Res. 118, 255–260. doi: 10.1016/0008-6215(83)88053-7
Grasdalen, H., Larsen, B., and Smidsrød, O. (1979). A p.m.r. study of the composition and sequence of uronate residues in alginates. Carbohydr. Res. 68, 23–31. doi: 10.1016/S0008-6215(00)84051-3
Grasdalen, H., Larsen, B., and Smidsrød, O. (1981). 13C-n.m.r. studies of monomeric composition and sequence in alginate. Carbohydr. Res. 89, 179–191. doi: 10.1016/S0008-6215(00)85243-X
Hamza, A., Piao, Y. L., Kim, M.-S., Choi, C. H., Zhan, C.-G., and Cho, H. (2011). Insight into the binding of the wild type and mutated alginate lyase (AlyVI) with its substrate: A computational and experimental study. Biochim. Biophys. Acta 1814, 1739–1747. doi: 10.1016/j.bbapap.2011.08.018
Heyraud, A., Gey, C., Leonard, C., Rochas, C., Girond, S., and Kloareg, B. (1996). NMR spectroscopy analysis of oligoguluronates and oligomannuronates prepared by acid or enzymatic hydrolysis of homopolymeric blocks of alginic acid: Application to the determination of the substrate specificity of Haliotis tuberculata alginate lyase. Carbohydr. Res. 289, 11–23. doi: 10.1016/0008-6215(96)00060-2
Hobbs, J. K., Lee, S. M., Robb, M., Hof, F., Barr, C., Abe, K. T., et al. (2016). KdgF, the missing link in the microbial metabolism of uronate sugars from pectin and alginate. Proc. Natl. Acad. Sci. U.S.A. 113, 6188–6193. doi: 10.1073/pnas.1524214113
Holtan, S., Zhang, Q., Strand, W. I., and Skjåk-Bræk, G. (2006). Characterization of the hydrolysis mechanism of polyalternating alginate in weak acid and assignment of the resulting MG oligosaccharides by NMR spectroscopy and ESI-mass spectrometry. Biomacromolecules 7, 2108–2121. doi: 10.1021/bm050984q
Hu, T., Li, C., Zhao, X., Li, G., Yu, G., and Guan, H. (2013). Preparation and characterization of guluronic acid oligosaccharides degraded by a rapid microwave irradiation method. Carbohydr. Res. 373, 53–58. doi: 10.1016/j.carres.2013.03.014
Inoue, A., Anraku, M., Nakagawa, S., and Ojima, T. (2016). Discovery of a novel alginate lyase from Nitratiruptor sp. SB155-2 thriving at deep-sea hydrothermal vents and identification of the residues responsible for its heat stability. J. Biol. Chem. 291, 15551–15563. doi: 10.1074/jbc.M115.713230
Inoue, A., Kagaya, M., and Ojima, T. (2007). “Preparation of protoplasts from Laminaria japonica using native and recombinant abalone alginate lyases,” in Nineteenth International Seaweed Symposium. Developments in Applied Phycology, Vol. 2, eds M. A. Borowitzka, A. T. Critchley, S. Kraan, A. Peters, K. Sjøtun, and M. Notoya (Dordrecht: Springer), doi: 10.1007/978-1-4020-9619-8_23
Ji, S., Dix, S. R., Aziz, A. A., Sedelnikova, S. E., Baker, P. J., Rafferty, J. B., et al. (2019). The molecular basis of endolytic activity of a multidomain alginate lyase from Defluviitalea phaphyphila, a representative of a new lyase family, PL39. J. Biol. Chem. 294, 18077–18091. doi: 10.1074/jbc.RA119.010716
Jones, C. S., and Mayfield, S. P. (2012). Algae biofuels: Versatility for the future of bioenergy. Curr. Opin. Biotechnol. 23, 346–351. doi: 10.1016/j.copbio.2011.10.013
Kamerling, J. P., and Gerwig, G. J. (2007). “Strategies for the structural analysis of carbohydrates,” in Comprehensive Glycoscience – From Chemistry to Systems Biology, Vol. 2, eds J. P. Kamerling, G.-J. Boons, Y. C. Lee, A. Suzuki, N. Taniguchi, and A. G. J. Voragen (Amsterdam: Elsevier Ltd), 1–68.
Kim, D. E., Lee, E. Y., and Kim, H. S. (2009). Cloning and characterization of alginate lyase from a marine bacterium Streptomyces sp. ALG-5. Mar. Biotechnol. 11, 10–16. doi: 10.1007/s10126-008-9114-9
Kim, H. S., Lee, C.-G., and Lee, E. Y. (2011). Alginate lyase: Structure, property, and application. Biotechnol. Bioprocess Eng. 16, 843–851. doi: 10.1007/s12257-011-0352-8
Kim, H. T., Chung, J. H., Wang, D., Lee, J., Woo, H. C., Choi, I. G., et al. (2012). Depolymerization of alginate into a monomeric sugar acid using Alg17C, an exo-oligoalginate lyase cloned from Saccharophagus degradans 2-40. Appl. Microbiol. Biotechnol. 93, 2233–2239. doi: 10.1007/s00253-012-3882-x
Laemmli, U. K. (1970). Cleavage of structural proteins during the assembly of the head of bacteriophage T4. Nature 227, 680–685. doi: 10.1038/227680a0
Lee, K. Y., and Mooney, D. J. (2012). Alginate: Properties and biomedical applications. Prog. Polym. Sci. 37, 106–126. doi: 10.1016/j.progpolymsci.2011.06.003
Li, L., Jiang, X., Guan, H., and Wang, P. (2011). Preparation, purification and characterization of alginate oligosaccharides degraded by alginate lyase from Pseudomonas sp. HZJ 216. Carbohydr. Res. 346, 794–800. doi: 10.1016/j.carres.2011.01.023
Li, S., Wang, L., Han, F., Gong, Q., and Yu, W. (2016). Cloning and Characterization of the first polysaccharide lyase family 6 oligoalginate lyase from marine Shewanella sp. Kz7. J. Biochem. 159, 77–86. doi: 10.1093/jb/mvv076
Li, S., Wang, L., Jung, S., Lee, B. S., He, N., and Lee, M.-S. (2020). Biochemical characterization of a new oligoalginate lyase and its biotechnological application in Laminaria japonica degradation. Front. Microbiol. 11:316. doi: 10.3389/fmicb.2020.00316
Lombard, V., Bernard, T., Rancurel, C., Brumer, H., Coutinho, P. M., and Henrissat, B. (2010). A hierarchical classification of polysaccharide lyases for glycogenomics. Biochem. J. 432, 437–444. doi: 10.1042/BJ20101185
Lundqvist, L. C. E., Jam, M., Barbeyron, T., Czjek, M., and Sandström, C. (2012). Substrate specificity of the recombinant alginate lyase from the marine bacteria Pseudomonas alginovora. Carbohydr. Res. 352, 44–50. doi: 10.1016/j.carres.2012.02.014
Mathieu, S., Henrissat, B., Labre, F., Skjak-Braek, G., and Helbert, W. (2016). Functional exploration of polysaccharide lyase family PL6. PLoS One:11:e0159415. doi: 10.1371/journal.pone.0159415
McIntyre, D. D., Ceri, H., and Vogel, H. J. (1996). Nuclear magnetic resonance studies of the heteropolysaccharides alginate, gum arabic and gum xanthan. Starch 48, 285–291. doi: 10.1002/star.19960480711
Miller, G. L. (1959). Use of dinitrosalicylic acid reagent for determination of reducing sugar. Anal. Chem. 31, 426–428. doi: 10.1021/ac60147a030
Mrsny, R. J., Lazazzera, B. A., Daugherty, A. L., Schiller, N. L., and Patapoff, T. W. (1994). Addition of a bacterial alginate lyase to purulent CF sputum in vitro can result in the disruption of alginate and modification of sputum viscoelasticity. Pulm. Pharmacol. 7, 357–366. doi: 10.1006/pulp.1994.1042
Munoz, R., Rosselló-Móra, R., and Amann, R. (2016). Revised phylogeny of Bacteroidetes and proposal of sixteen new taxa and two new combinations including Rhodothermaeota phyl. nov. Syst. Appl. Microbiol. 39, 281–296. doi: 10.1016/j.syapm.2016.04.004
Muth, T. R., Ahn, J., and Caplan, M. J. (1998). Identification of sorting determinants in the C-terminal cytoplasmic tails of the gamma-aminobutyric acid transporters GAT-2 and GAT-3. J. Biol. Chem. 273, 25616–25627. doi: 10.1074/jbc.273.40.25616
Nolan, M., Tindall, B. J., Pomrenke, H., Lapidus, A., Copeland, A., Glavina Del Rio, T., et al. (2009). Complete genome sequence of Rhodothermus marinus type strain (R-10). Stand. Genomic. Sci. 1, 283–290. doi: 10.4056/sigs.46736
Osawa, T., Matsubara, Y., Muramatsu, T., Kimura, M., and Kakuta, Y. (2005). Crystal structure of the alginate (poly α-L-guluronate) lyase from Corynebacterium sp. at 1.2 Å resolution. J. Mol. Biol. 345, 1111–1118. doi: 10.1016/j.jmb.2004.10.081
Overbeek, R., Olson, R., Pusch, G. D., Olsen, G. J., Davis, J. J., Disz, T., et al. (2014). The SEED and the Rapid Annotation of microbial genomes using Subsystems Technology (RAST). Nucleic Acids Res. 42, D206–D214. doi: 10.1093/nar/gkt1226
Park, H. H., Kam, N., Lee, E. Y., and Kim, H. S. (2012). Cloning and characterization of a novel oligoalginate lyase from a newly isolated bacterium Sphingomonas sp. MJ-3. Mar. Biotechnol. 14, 189–202. doi: 10.1007/s10126-011-9402-7
Pawar, S. N., and Edgar, K. J. (2012). Alginate derivatization: A review of chemistry, properties and applications. Biomaterials 33, 3279–3305. doi: 10.1016/j.biomaterials.2012.01.007
Penman, A., and Sanderson, G. R. (1972). A method for the determination of uronic acid sequence in alginates. Carbohydr. Res. 25, 273–282. doi: 10.1016/s0008-6215(00)81637-7
Preiss, J., and Ashwell, G. (1962a). Alginic acid metabolism in bacteria. I. Enzymatic formation of unsaturated oligosaccharides and 4-deoxy-L-erythro-5-hexoseulose uronic acid. J. Biol. Chem. 237, 309–316. doi: 10.1016/S0021-9258(18)93920-7
Preiss, J., and Ashwell, G. (1962b). Alginic acid metabolism in bacteria. II. The enzymatic reduction of 4-deoxy-L-erythro-5-hexoseulose uronic acid to 2-keto-3-deoxy-D-gluconic acid. J. Biol. Chem. 237, 317–321. doi: 10.1016/S0021-9258(18)93921-9
Senturk Parreidt, T., Muller, K., and Schmid, M. (2018). Alginate-based edible films and coatings for food packaging applications. Foods 7:E170. doi: 10.3390/foods7100170
Steginsky, C. A., Beale, J. M., Floss, H. G., and Mayer, R. M. (1992). Structural determination of alginic acid and the effects of calcium binding as determined by high-field n.m.r. Carbohydr. Res. 225, 11–26. doi: 10.1016/0008-6215(92)80036-z
Turner, P., Mamo, G., and Karlsson, E. N. (2007). Potential and utilization of thermophiles and thermostable enzymes in biorefining. Microb. Cell Fact. 6:9. doi: 10.1186/1475-2859-6-9
Tøndervik, A., Klinkenberg, G., Aarstad, O. A., Drabløs, F., Ertesvåg, H., Ellingsen, T. E., et al. (2010). Isolation of mutant alginate lyases with cleavage specificity for di-guluronic acid linkages. J. Biol. Chem. 285, 35284–35292. doi: 10.1074/jbc.M110.162800
Wang, B., Ji, S.-Q., Ma, X.-Q., Lu, M., Wang, L.-S., and Li, F.-L. (2018). Substitution of one calcium binding amino acid strengthens substrate binding in a thermophilic alginate lyase. FEBS Lett. 592, 369–379. doi: 10.1002/1873-3468.12965
Wang, Y., Han, F., Hu, B., Li, J., and Yu, W. (2006). In vivo prebiotic properties of alginate oligosaccharides prepared through enzymatic hydrolysis of alginate. Nutr. Res. 26, 597–603. doi: 10.1016/j.nutres.2006.09.015
Wargacki, A. J., Leonard, E., Win, M. N., Regitsky, D. D., Santos, C. N. S., Kim, P. B., et al. (2012). An engineered microbial platform for direct biofuel production from brown macroalgae. Science 335, 308–313. doi: 10.1126/science.1214547
Wong, T. Y., Preston, L. A., and Schiller, N. L. (2000). Alginate lyase: Review of major sources and enzyme characteristics, structure-function analysis, biological roles, and applications. Annu. Rev. Microbiol. 54, 289–340. doi: 10.1146/annurev.micro.54.1.289
Xing, M., Cao, Q., Wang, Y., Xiao, H., Zhao, J., Zhang, Q., et al. (2020). Advances in research on the bioactivity of alginate oligosaccharides. Mar. Drugs 18:144. doi: 10.3390/md18030144
Yamasaki, M., Ogura, K., Hashimoto, W., Mikami, B., and Murata, K. (2005). A structural basis for depolymerization of alginate by polysaccharide lyase family-7. J. Mol. Biol. 352, 11–21. doi: 10.1016/j.jmb.2005.06.075
Yoon, H.-J., Mikami, B., Hashimoto, W., and Murata, K. (1999). Crystal structure of alginate lyase A1- III from Sphingomonas species A1 at 1.78 Å resolution. J. Mol. Biol. 290, 505–514. doi: 10.1006/jmbi.1999.2883
Zhang, H., Cheng, J., and Ao, Q. (2021). Preparation of alginate-based biomaterials and their applications in biomedicine. Mar. Drugs 19:264. doi: 10.3390/md19050264
Zhang, Z., Yu, G., Guan, H., Zhao, X., Du, Y., and Jiang, X. (2004). Preparation and structure elucidation of alginate oligosaccharides degraded by alginate lyase from Vibrio sp. 510. Carbohydr. Res. 339, 1475–1481. doi: 10.1016/j.carres.2004.03.010
Keywords: alginate oligosaccharides, thermophilic alginate lyase, guluronic acid, mannuronic acid, Rhodothermus marinus, NMR spectroscopy
Citation: Dobruchowska JM, Bjornsdottir B, Fridjonsson OH, Altenbuchner J, Watzlawick H, Gerwig GJ, Dijkhuizen L, Kamerling JP and Hreggvidsson GO (2022) Enzymatic depolymerization of alginate by two novel thermostable alginate lyases from Rhodothermus marinus. Front. Plant Sci. 13:981602. doi: 10.3389/fpls.2022.981602
Received: 29 June 2022; Accepted: 17 August 2022;
Published: 20 September 2022.
Edited by:
Ugo Cenci, Lille University of Science and Technology, FranceReviewed by:
Rufeng Wang, Shanghai University of Traditional Chinese Medicine, ChinaCopyright © 2022 Dobruchowska, Bjornsdottir, Fridjonsson, Altenbuchner, Watzlawick, Gerwig, Dijkhuizen, Kamerling and Hreggvidsson. This is an open-access article distributed under the terms of the Creative Commons Attribution License (CC BY). The use, distribution or reproduction in other forums is permitted, provided the original author(s) and the copyright owner(s) are credited and that the original publication in this journal is cited, in accordance with accepted academic practice. No use, distribution or reproduction is permitted which does not comply with these terms.
*Correspondence: Gudmundur O. Hreggvidsson, Z3VkbXVuZG9AbWF0aXMuaXM=
†Present address: Lubbert Dijkhuizen, CarbExplore Research BV, Groningen, Netherlands Justyna M. Dobruchowska, Chemical Biology and Drug Discovery, Utrecht University, Utrecht, Netherlands
‡These authors have contributed equally to this work
Disclaimer: All claims expressed in this article are solely those of the authors and do not necessarily represent those of their affiliated organizations, or those of the publisher, the editors and the reviewers. Any product that may be evaluated in this article or claim that may be made by its manufacturer is not guaranteed or endorsed by the publisher.
Research integrity at Frontiers
Learn more about the work of our research integrity team to safeguard the quality of each article we publish.