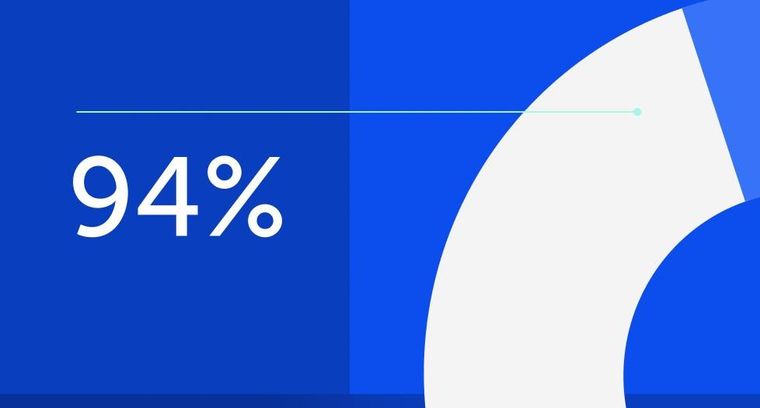
94% of researchers rate our articles as excellent or good
Learn more about the work of our research integrity team to safeguard the quality of each article we publish.
Find out more
REVIEW article
Front. Plant Sci., 14 September 2022
Sec. Plant Abiotic Stress
Volume 13 - 2022 | https://doi.org/10.3389/fpls.2022.972856
This article is part of the Research TopicAbiotic Stress Adaptation and Tolerance Mechanisms in Crop PlantsView all 40 articles
Heavy metal (HM) stress is threatening agricultural crops, ecological systems, and human health worldwide. HM toxicity adversely affects plant growth, physiological processes, and crop productivity by disturbing cellular ionic balance, metabolic balance, cell membrane integrity, and protein and enzyme activities. Plants under HM stress intrinsically develop mechanisms to counter the adversities of HM but not prevent them. However, the exogenous application of abscisic acid (ABA) is a strategy for boosting the tolerance capacity of plants against HM toxicity by improving osmolyte accumulation and antioxidant machinery. ABA is an essential plant growth regulator that modulates various plant growth and metabolic processes, including seed development and germination, vegetative growth, stomatal regulation, flowering, and leaf senescence under diverse environmental conditions. This review summarizes ABA biosynthesis, signaling, transport, and catabolism in plant tissues and the adverse effects of HM stress on crop plants. Moreover, we describe the role of ABA in mitigating HM stress and elucidating the interplay of ABA with other plant growth regulators.
Plants encounter a plethora of environmental stresses classified as biotic or abiotic stresses. Among abiotic stresses, heavy metal (HM) stress is a severe ecological restraint with detrimental effects on plant growth, metabolic processes, and crop productivity (Ghori et al., 2019). Agrarian soils in different parts of the world are slightly/moderately contaminated with HM, which is concerning due to their harmful effect on human health, ecosystem function, and global economic growth (Shahid et al., 2015). HM toxicity hampers normal plant functioning by producing excessive reactive oxygen species (ROS), repressing respiration, photosynthesis, and vascular and enzymatic activities, and disrupting ion homeostasis (He et al., 2021). Plants have complex inherent biological mechanisms to counter the adversities of HM but fail to mitigate them at toxic levels (Chandrakar et al., 2020). However, plant growth regulators (PGRs) play a crucial role in strengthening the tolerance mechanisms of plants against HM stress (Hasanuzzaman et al., 2018; Singh et al., 2020). PGRs are small chemical messengers that control various phenological characteristics, physio-biochemical, and developmental aspects in plants in a concentration of the molecular inducer-dependent and plant development stage-dependent manner (Vimala, 1985). The major PGR classes include abscisic acid (ABA), auxins, brassinosteroid (BR), cytokinins (CK), ethylene (ET), gibberellic acid (GA3), jasmonic acid (JA), salicylic acid (SA), nitric oxide (NO), and polyamines (PAs). The roles of PGRs in enhancing endurance against abiotic stresses, including HM, are well established (Saini et al., 2021; Shah et al., 2021, 2022; Kumar et al., 2022). This review focuses on the role of ABA in mitigating HM stress at the metabolic, transport, and crosstalk with other PGRs levels.
Abscisic acid is a small sesquiterpene molecule with many crucial functions in plant phenological and developmental processes, including seed dormancy and development, vegetative growth, flowering, stomatal movement, lipid and storage protein synthesis, and leaf senescence (Chen et al., 2020). Under HM stress, ABA modulates various physiological, biochemical, and molecular processes in plants (Vishwakarma et al., 2017). Exogenous ABA application can enhance plant tolerance to toxic metal stress. For example, 10 and 20 μM foliar ABA spray alleviated cadmium (Cd) inhibitory effects in Lactuca sativa L. by increasing its growth, chlorophyll content, photosynthetic efficiency, catalase activity, and proline and protein contents (Tang et al., 2020). Deng et al. (2021) reported that 5 μM ABA application mitigated the Cd-induced damage in roots of Malus hupehensis (Pamp.) by decreasing cell death and hydrogen peroxide and malondialdehyde contents. Further, 10 μM foliar ABA treatment ameliorated Cd toxicity in Vigna radiata L. by increasing chlorophyll content, osmolyte concentration, and antioxidant defense machinery (Leng et al., 2021).
It is well-accepted that ABA plays a vital role in improving morphological features, developmental aspects, and yield and quality parameters, and ameliorating the adversities of HM stress in crop plants. This review characterizes ABA biosynthesis, signaling, transport, and catabolism in plants and summarizes the impact of HM stress on crop growth, photosynthesis, and yield. Toxic metal and metalloids evolved with the evolution of green plants, accumulating in edible parts. Hence, plants evolved mechanisms to uptake, exclude, and mitigate the impact of unrequired or toxic metals. ABA is one such PGR evolved to mitigate HM stress.
Abscisic acid is a 15-carbon terpenoid molecule identified in the 1960s and recognized as a growth-inhibiting compound (abscisin II) associated with bud dormancy in maple and fruit abscission in cotton (Schwartz and Zeevaart, 2010). Later work revealed that ABA plays an important role in several developmental and physio-biochemical processes and regulates abiotic stress responses in plants (Sah et al., 2016). ABA is synthesized in all cells with chloroplasts or amyloplasts and found in major organs and tissues (Taiz et al., 2015). ABA biosynthesis starts in the chloroplast and ends in the cytoplasm (Seo and Koshiba, 2002). The isopentenyl diphosphate (IPP) is formed in plastids from pyruvic acid and glyceraldehyde 3-phosphate (Figure 1) and converted into geranylgeranyl diphosphate (GGPP) through the methylerythritol 4-phosphate (MEP) pathway. GGPP then forms phytoene (C40), which is converted into lycopene and β-carotene, and finally zeaxanthin. The conversion of zeaxanthin to all-trans-violaxanthin (C40) is mediated by the enzyme zeaxanthin epoxidase (ZEP). The all-trans-violaxanthin (C40) is then modified into 9-cis-neoxanthin (C40) and divided into xanthoxin (C15) and a C25 metabolite by 9-cis-epoxycarotenoid dioxygenase enzyme (NCED). This reaction is a rate-limiting step, and NCED is a crucial enzyme in ABA biosynthesis. Xanthoxin is transferred to the cytoplasm, where it is transformed into ABA-aldehyde by an enzyme that belongs to a short-chain dehydrogenase/reductase family. ABA-aldehyde is then converted into ABA with the reaction catalyzed by ABA-aldehyde oxidase (AAO) (Milborrow, 2001; Xiong and Zhu, 2003; Nambara and Marion-Poll, 2005; Mehrotra et al., 2014; Cardoso et al., 2020). Various enzymes, encoded by different genes, are involved in ABA biosynthesis. For instance, Arabidopsis aldehyde oxidases genes (AAO3) encode the AAO enzyme (Seo et al., 2000). The ABA2 gene encodes the ZEP enzyme to enhance its activity. Increased upregulation of ABA2 mRNA in genetically altered plants had a positive role in delaying germination and increasing ABA levels in mature seeds of transgenic plants (Frey et al., 1999). In Arabidopsis thaliana L., ABA biosynthesis is controlled by a small family of five NCED genes (AtNCED2, 3, 5, 6, and 9) located in plastids. Moreover, there is a differential contribution of NCEDs to ABA synthesis during Arabidopsis thaliana L. growth and development; for instance, NCED2 and 3 have high expression levels in roots and leaves but low expression levels for seed development, and NCED5, 6 and 9 have increased expression levels during later maturation stages. Further, NCED expression is controlled by the transcription factor MYB96, with an essential role in seed dormancy (Tan et al., 2003; Lee et al., 2015; Ma et al., 2018). ATAF1 (Arabidopsis thaliana activating factor 1) is a transcription factor that regulates Arabidopsis thaliana L. development and environmental stress responses. ATAF1 promotes NCED3 expression that controls ABA biosynthesis (Jensen et al., 2013). HM stress affects plant ABA biosynthesis and varies between species and the type and level of HM stress. HM affect ABA biosynthesis at lower concentrations but inhibit it at higher levels. Copper (Cu) suppressed the transcription of ABA biosynthetic genes (StABA1 and StNCED1, AAO3) in Solanum tuberosum L., decreasing ABA content (Liu et al., 2020). Cu stress significantly increased ABA contents in roots, shoots, and leaves of Helianthus annuus L. seedlings (Zengin and Kirbag, 2007). Hou et al. (2010) reported that aluminum (Al) enhanced endogenous ABA production in roots and leaves of Glycine max. L. Exposure to cadmium (Cd) stress significantly increased leaf ABA concentration in Phaseolus vulgaris L. but inhibited ABA accumulation in dry excised leaves (Poschenrieder et al., 1989). Cd stress increased endogenous ABA levels in the roots of a non-hyperaccumulating ecotype of Sedum alfredii due to upregulated expression of ABA biosynthesis genes (SaABA2, SaNCED) (Tao et al., 2019). Further, Cd stress increased ABA content more in roots than old leaves of Boehmeria nivea L., with the lowest levels in new leaves. Long-time treatment of high Cd concentrations decreased endogenous ABA biosynthesis (Chen et al., 2021). HM compete with other metal ions and depolarize membrane-inducing Ca2+ efflux from organelles, initiating a Ca2+ signaling cascade of events that trigger the upregulation of ZEP, NCED, and MCSU genes (Ahmad et al., 2010).
Figure 1. Abscisic acid (ABA) biosynthesis in plant cells. Endoplasmic reticulum (ER). The precursor of ABA in plants is (C40) β-carotene. Isopentenyl pyrophosphate (IPP) converts C5 to C15 and continues to C40. β-carotene converts C40 to xanthoxin (C15) in plastids and cytosol. Zeaxanthin epoxidase (ZEP), neoxanthin synthase (NYS), 9-cis-epoxycarotenoid dioxygenase (NCEDs), phasic acid (PA), dihydrophaseic acid (DPA), PA reductase (PAR), DPA-4-β-D-glucoside (DPAG), UDP-glucosyltransferase (UGT) encoded by (UGT71C5), cytochrome P450 monooxygenase (P450) encoded by (CYP707As), glycosyltransferase (GT), β-glucosidase encoded by (AtBG1 and AtBG2), ABA-glucose ester (ABA-GE), abscisic aldehyde oxidase (AAO3), and MCSU, molybdenum cofactor sulfurase.
Abscisic acid is produced primarily in vascular tissue with its transportation among cells, tissues, and organs essential for regulating plant physio-biochemical processes under varying environmental responses (Kuromori et al., 2010; Saradadevi et al., 2016). ABA exists in anionic (ABA−) and protonated (ABAH) forms in plants. It is a weak acid, diffusing the bio-membrane passively when in protonated form (Ng et al., 2014; Chen et al., 2020). However, active ABA translocation across the plasma membrane can be mediated by transporters, including ATP-binding cassette (ABC) transporter proteins, nitrate or di/tri-peptide transporters [NRT1/PTR family proteins (NPF)], multidrug and toxic compound extrusion (MATE)-type/DTX transporters, and AWPM-19 family proteins (OsPM1). These transporters were first identified in Oryza sativa L. (Kanno et al., 2012; Zhang et al., 2014; Merilo et al., 2015). In eukaryotes, ABC transporters are categorized into sub-families from ABCA to ABCH. About 129 ABC transporters of ABA have been identified in Arabidopsis thaliana L. (Kang et al., 2010). Many ABCG members are suggested to play a role in ABA transportation. The AtABCG25 transporter, located in the plasma membrane of root vascular bundles and close to leaf vascular veins, exports ABA from vascular tissue to different plant parts (Kuromori et al., 2010; Andolfo et al., 2015). The AtABCG40 transporter mediates ABA import into plant cells, and its loss of function in guard cells delays stomatal closure by limiting ABA uptake (Kang et al., 2010). The AtABCG22 transporter may participate in stomatal regulation by promoting ABA uptake (Kuromori et al., 2011). AtABCG25 and AtABCG31 regulate ABA export from the endosperm to multiple sites in plants, whereas AtABCG30 and AtABCG40 import ABA into the embryo, controlling seed germination (Kang et al., 2015). AtDTX50 (DTX/MATE-type transporter) is localized in vascular tissue and guard cells, participating in ABA efflux in Arabidopsis thaliana L. and exogenous application of ABA upregulated AtDTX50 gene expression (Zhang et al., 2014). Recently, Shimizu et al. (2021) detected two Arabidopsis NPF4.6 and NPF5.1 transporters in vascular tissues, guard cells, and leaf cell tissues that act as ABA importers to regulate stomatal movement by controlling ABA uptake. Studies have suggested that lower levels of HM contamination trigger ABA to increase inside plant cells, which is continuously transported throughout the plant, regulating plant defensive responses. However, under extreme HM stress, ABA synthesis and transport decreases due to blockages in the vascular transportation route. Under such conditions, exogenous supply of ABA can alleviate these perturbations.
Catabolism and biosynthesis rates determine ABA hormone levels and are essential for modulating plant growth and metabolic processes in response to varying environmental cues (Kitahata et al., 2005). Endogenous ABA levels change with varying ecological conditions. Under favorable environmental conditions, ABA is metabolized into an inactive form through hydroxylation and conjugation pathways (Ng et al., 2014). In the hydroxylation pathway, ABA is hydroxylated by the oxidation of its three methyl groups in the ring structure (C-7′, C-8′, and C-9′). C-8′ is the major catabolic route, in which 8′-hydroxy-ABA is unstable and spontaneously converted into phaseic acid (PA) and dihydro-phaseic acid (DPA), catalyzed by cytochrome P450 type enzyme (CYP707A), an important enzyme in ABA metabolism (Saito et al., 2004; Baron et al., 2012; Rodriguez, 2016; Seo and Marion-Poll, 2019). CYP707A gene expression in guard cells and vascular tissue controls ABA metabolism and function under diverse conditions (Okamoto et al., 2009). During seed development and germination, members of the CYP707A gene family (CYP707A1 to CYP707A4) help decrease endogenous ABA levels (Zheng et al., 2018). The inactivation of ABA is also mediated by the conjugation of ABA with glucose to form ABA glucose ester (ABA-GE) catalyzed by uridine diphosphate glycosyltransferases (UGTs). ABA-GE (inactive ABA product) is formed in the cytoplasm and stored in the vacuoles (Priest et al., 2006; Zhu et al., 2011; Chen et al., 2020). However, under abiotic stress, ABA-GE is converted back into free ABA through enzyme-catalyzed hydrolysis regulated by beta-glycosidase homolog 1 and 2 (BG1 and BG2) enzymes (Xu et al., 2012). Therefore, ABA catabolism significantly controls ABA levels under normal and adverse conditions. In summary, HM toxicity decreases endogenous ABA levels by inhibiting the expression of genes involved in ABA biosynthesis and thus halting the ABA-mediated physiological effect.
Understanding the mechanism of action of ABA is essential for plants to enhance their biological functions under unfavorable climatic conditions (Ali et al., 2020). Three main components are involved in ABA signaling: pyrabactin resistance (PYR)/pyrabactin resistance-like (PYL)/regulatory component of ABA receptor (RCAR), protein phosphatase 2C (PP2C; negative regulators), and sucrose non-fermenting (SNF1) related protein kinase 2 (SnRK2; positive regulators) (Ye et al., 2017). When ABA is present, PYR/PYL/RCAR-PP2C starts forming a complex structure that inhibits the negative effect of PP2C and further activators SnRK2 function for the phosphorylation of downstream proteins, including transcription factors to promote ABA-responsive gene expression (Yoshida et al., 2014). Figure 2 is a diagrammatic representation of the mechanism of action of ABA. In addition, HM contamination decreases endogenous ABA content and thus stopped ABA modulated processes due to the non-availability of ABA in cells.
Figure 2. Schematic representation of the mechanism of action of abscisic acid (ABA). The core component of ABA signaling includes pyrabactin resistance 1 (PYR1)/PYR-Like (PYL), regulatory component of ABA receptors (RCARs), clade a protein phosphatases PP2C, ABA insensitive 1/2 ABI 1/2, hypersensitive to ABA1/2, HBA1/2, and sucrose non-fermenting-1-related protein kinase 2 family members (SnRK2s).
Heavy metals are naturally occurring elements with high atomic weight and density, including some micro-nutrients crucial for plant growth and development. However, excess HM concentrations adversely affect plant growth and survival. The main HM-comprising metals and metalloids, such as cadmium (Cd), lead (Pb), arsenic (As), mercury (Hg), silver (Ag), aluminum (Al), and chromium (Cr), do not play vital roles in plant growth and development but their toxic levels disturb plant development and metabolism (Gill, 2014; Keyster et al., 2020). HM are released and accumulated in soils from natural sources and anthropogenic activities, including industrialization, smelting, extensive mining, fertilizer application, polluted water irrigation, fossil fuel combustion, and vehicle emitted gases and pollutants (Khan et al., 2017; Lajayer et al., 2019). Elevated HM levels increase ROS generation, causing metabolic imbalance, disturbing ion homeostasis, disorganizing the antioxidant defense system, disrupting protein structure, pigment synthesis, enzyme activities and membrane integrity, increasing lipid peroxidation, and thus decreasing plant growth and productivity (Emamverdian et al., 2015; Jalmi et al., 2018). HM stress adversely affects crop growth, physiological processes, and production (Table 1).
Table 1. Toxic effects of heavy metal (HM) stress on plant growth, physiology, and yield parameters.
Various studies have reported that HM contamination hinders crop plant growth features by altering root and shoot length morphology and anatomy and reducing plant biomass. For instance, 900 μM Cd and 1500 μM Pb reduced root and shoot lengths of Brassica juncea L. seedlings (John et al., 2009). In another Brassica juncea L. study, Cr, Pb, Cd, and Hg application at 100, 200, 10, and 50 mg kg–1 soil, respectively, decreased root and stem dry weights (Sheetal et al., 2016). Two Zea mays L. genotypes with contrasting root systems exhibited differential growth responses to moderate Cd stress (20 mg kg–1 soil) with less growth reduction in small-rooted genotypes than large-rooted genotypes (An et al., 2022a,b).
Heavy metal stress severely impacts physiological processes, including the electron transport system, enzymatic activities, nutrient uptake, photosynthetic pigments, and the photosystem. Moreover, high HM levels induce toxic ROS production, causing cellular damage by inactivating proteins and enzymes, increasing lipid peroxidation, damaging nucleic acids, and disrupting ion homeostasis and metabolic balance, leading to plant death (Goyal et al., 2020). In addition, HM decrease photosynthetic pigment content, negatively affect photosystems I and II, reduce transpiration rate, stomatal conductance, and water use efficiency, and disturb plant water relations and cellular nutrient balance (Małkowski et al., 2019). Studies have suggested that soil contaminated with HM alters overall plant physiology. For instance, a pre-sowing seed treatment with 100 μM Cd decreased the protein content in roots and peroxide activity in leaves of Cicer arietinum L. (Bavi et al., 2011). Brassica juncea L. grown in soil contaminated with Cr, Pb, Cd, and Hg at 100, 200, 10, and 50 mg kg–1 soil, respectively, had reduced photosynthetic rates and chlorophyll a and b contents (Sheetal et al., 2016). Soil-applied Pb (400, 800, and 1200 ppm) reduced photosynthetic pigments, antioxidant enzyme activities, increased hydrogen peroxide content, and lipid peroxidation and affected protein and proline content in Oryza sativa L. (Ashraf et al., 2017). Tamarix usneoides L. seedlings treated with 18 mg Cd kg–1 Cd had decreased chlorophyll contents and photosynthetic rates (Mayonde et al., 2021).
Heavy metal toxicity induces deleterious effects on various physiological and biochemical processes, decreasing crop productivity (Shahid et al., 2015). Soil-applied As (30 mg kg–1) significantly decreased the number of panicles and filled grains per pot and total grain yield in Oryza sativa L. (Rahman et al., 2007). Soil incubated with 150 mg kg–1 Cr (VI) markedly decreased the yield of Hordeum vulgare L., but significantly increased the yield and ammonia nitrogen content of Zea mays L. (Wyszkowski and Radziemska, 2010). Arduini et al. (2014) reported that soil supplemented with 4.5 mg kg–1 Cd reduced plant height, biomass, and vegetative growth but increased dry matter and grain Cd accumulation in Triticum durum. Further, soil-applied Pb (400, 800, and 1,200 ppm) decreased tiller number per pot, grain number per panicle, 1000-grain weight, and grain yield in Oryza sativa L. Arduini et al. (2014). In another study, soil application of 100 mg kg–1 Cd significantly decreased pod number per plant, seed number per pod, seed weight, and seed yield in Brassica juncea L. (Irfan et al., 2015). Moderate Cd stress significantly reduced the grain yield of Zea mays L., especially under low nitrogen supply (An et al., 2022b). These studies suggest that HM stress impedes growth, physiological processes, and yield attributes, decreasing overall crop production.
Abscisic acid is a prominent stress hormone that regulates numerous physiological processes and helps plants cope with the adverse impacts of HM stress (Shen et al., 2017). ABA regulates toxic metal translocation from roots to shoots. It closes stomata and reduces the transpiration rate, limiting the long-distance transfer of HM (Saradadevi et al., 2017; Hu et al., 2020). ABA enhances plant biomass, physiological processes, antioxidant defense system, and osmolyte accumulation, reducing the effects of HM stress. The activation of genes involved in ABA production increases endogenous ABA concentration in some plant species under Cd stress, including Oryza sativa L., Solanum tuberosum L., Brassica napus L., Triticum aestivum L., and others (Hsu and Kao, 2003; Stroiński et al., 2013; Shen et al., 2017; Hu et al., 2020). Exogenous ABA supply decreased transpiration rate and Cd concentration and increased Cd tolerance in Oryza sativa L. seedlings (Hsu and Kao, 2003). Further, exogenous ABA supplementation reduced the deleterious effects of Cd stress in Brassica napus L. by decreasing internal Cd accumulation and malondialdehyde content and enhancing fresh weight (Meng et al., 2009). According to Stroiński et al. (2013), Solanum tuberosum L. seedlings treated with 0.1 mM ABA increased StPCS1 content, the expression of genes coding 9-cis-epoxycarotenoid dioxygenase 1 and basic leucine zipper, phytochelatins synthase activity in roots, and the endogenous ABA level. In Arabidopsis thaliana L., Fan et al. (2014) and Pan et al. (2020) revealed that ABA reduced Cd absorption by inhibiting the process of iron-regulated transporter 1 (IRT1) and relieving Cd-induced toxicity. In Populus euphratica L. cells, ABA alleviated 100 μM Cd stress by improving antioxidant enzyme activity that scavenged H2O2 to prevent Cd from entering H2O2-induced Ca2+-permeable channels (Han et al., 2016). ABA application at 20 μM L–1 improved Cd extraction and phytoremediation activity in Solanum photeinocarpum L. (Wang et al., 2016). The JrVHAG1gene functions as a Cd stress response regulator in the ABA signaling pathway and transcription regulation network of MYB in plants (Xu et al., 2018). Vitis vinifera L. seedlings treated with 10 μM ABA reduced Zn stress by decreasing Zn uptake and accumulation in roots and promoting the expression of the ZIP gene and detoxification-related genes in leaves and roots (Song et al., 2019). ABA-treated Populus alba L. seedlings had less Pb toxicity and increased expression of some genes involved in Pb transport and detoxification, including NRAMP1.4, ABCG40, PCS1.1, FRD3.1, and ABCC1.1 (Shi et al., 2019). In Lactuca sativa L. seedlings, exogenously applied ABA decreased Cd absorption and relieved Cd toxicity (Dawuda et al., 2020). In Sedum alfredii L. seedlings, exogenously applied ABA (0.2 mg L–1) alleviated Cd stress by increasing endogenous ABA content through enhanced ABA synthetase activity. It also promoted the expression of HsfA4c in roots and NAS and HMA4 in shoots to improve Cd resistance (Lu et al., 2020). Exogenous ABA application minimized the damage of excess Zn by increasing endogenous ABA concentration, proline accumulation, and ascorbate peroxidase (APX) activity and inhibiting lipoxygenase (LOX) activity (Li and Song, 2020). ABA could also be involved in upregulating the APX gene and proline synthesis genes and downregulating the LOX gene in Arabidopsis thaliana L. (Li and Song, 2020). Exogenous ABA application at 40 and 60 μM L–1 ameliorated Cd and Pb toxic effects by improving total chlorophyll content and antioxidant capacity in Sedum alfredii L. and Hylotelephium spectabile L. (Cheng et al., 2022). Seed priming with ABA also reduced HM toxicity in plants by increasing glutathione content, non-protein thiol and cysteine, and phytochelatins synthesis (Saha et al., 2021). ABA reduced Zn stress damage by enhancing proline content and antioxidant enzyme activities in Robinia pseudoacacia L. (Lou et al., 2021). ABA also regulated Cd hyperaccumulation in Sedum alfredii by lowering aquaporin expression in roots, the number of xylem vessels in stems, and stomatal size and density in leaves (Tao et al., 2021). Chen et al. (2022) suggested that exogenously applied ABA improved the phytoremediation efficiency of Sedum alfredii grown in Cd, Pb, and Zn contaminated soil. Figure 3 illustrates the role of exogenously applied ABA in plants. Figure 4 and Table 2 overview HM sequestration in plant cells mediated by ABA.
Figure 3. The effect of exogenous application of abscisic acid (ABA) on plant growth and physiological processes.
Figure 4. Diagram showing the sequestration mechanism of heavy metals (HMs) in plant cells and the role of abscisic acid (ABA) in mitigating HM stress. ROS (reactive oxygen species), PM (plasma membrane), CM (chloroplast membrane), PCs (phytochelatins), MTs (metallothioneins), and PAL (phenylalanine ammonia-lyase).
Abscisic acid and auxin regulate several facets of plant growth and metabolism, mainly in a contrary manner (Sun and Li, 2014). For example, for seed germination and root growth, ABA-induced expression of Auxin Response Factor 2 (ARF2) and arf2 mutants enhanced ABA sensitivity in Arabidopsis thaliana L. (Wang et al., 2011). ABA reduced cell division and altered auxin distribution more in arf2 mutants than wild species. Exogenous ABA application to the arf2 mutant reduced root length (Wang et al., 2011, 2013). ABA can also restrict primary root development by controlling cell proliferation in root tips (Wang et al., 2011). ABA slows seedling growth by increasing auxin signaling (Rock and Sun, 2005). The gene expression network containing the transcription factor ABA insensitive three regulates seed dormancy mediated by ABA and auxin (ABI3). Auxin controls ABI3 gene expression during seed germination by selecting auxin response factors 10 and 16 (Wang et al., 2011; Liu et al., 2013). Auxin is required for seed dormancy while ABA suppresses seed germination, indicating that auxin and ABA have an interacting role in seed dormancy. Auxin acts in two directions in ABA modulated processes by (1) activating the ABA response and (2) improving biological ABA synthesis. However, endogenous and exogenous indoleacetic acid (IAA) inhibited seed germination in radical protrusions experiments, showing that ABA and auxin work together to prevent seed germination (Liu et al., 2013; Matilla, 2020). In the absence of ABA, an equal amount of IAA did not limit seed germination, suggesting that inhibition of seed germination mediated through auxin depends on ABA. In addition, basipetal transport of auxin increased with osmotically induced ABA by increasing AUX1 (Auxin Influx Transporter) and PIN2 (Auxin Efflux Transporter) (Xu et al., 2013; Wu et al., 2020). As a result, auxin and ABA communicate via the auxin response pathway to control plant growth and development.
Many aspects of plant development, such as seed dormancy and maturation, main root growth, and flowering are controlled by the antagonistic interactions of ABA and gibberellins (GA). ABA inhibits seed germination by promoting seed dormancy, whereas GA stimulates seed germination and promotes seedling growth (Yuan et al., 2011). Sustaining germination and dormancy requires balancing ABA and GA production and catabolism (White et al., 2000; Bicalho et al., 2015). In Arabidopsis thaliana L., exogenous ABA supply decreased primary root development, whereas GA promoted root growth (Ubeda-Tomás et al., 2008; Zhang et al., 2010; Shu et al., 2018). Similarly, ABA and brassinosteroids (BR) coordinate antagonistically to modulate plant physiological functions. It is well-known that ABA inhibits seed germination while BR promotes seed germination (Saini et al., 2015). Further, ABA has a regulatory effect on BR signaling. ABA components like AB11 and ABA12 interplay with BR signaling components such as BIN2 and BR11 receptors (Yang et al., 2016). The interaction of ABA and GA3 hormones with Pb and Zn may increase total phenolic content; however, their interaction with Cd prevents an increase in phenolic compounds (Ahmad et al., 2015). ABA is also involved in the interaction between BR and CKs, as it suppresses BR synthesis during metal toxicity (Zhang S. et al., 2009). However, the molecular interactions between ABA and GA and ABA and BR for regulating plant physiological processes need further investigation.
Abscisic acid and cytokinin interact to regulate the stress response. The enzyme cytokinin oxidase encoded by the multigenic family cytokinin oxidase (CKX) has several members responsible for cytokinin breakdown (Avalbaev et al., 2012; Sirko et al., 2021). For instance, ABA decreased the expression of CKX genes in Arabidopsis thaliana L. Under stress conditions, cytokinin receptor kinases (AHK2 and AHK3) negatively controlled ABA and the expression of genes responsible for osmotic stress, while mutants with cytokinin deficiency had increased survival rates (Werner et al., 2006; Tran et al., 2007).
Abscisic acid and ethylene work together to control stomatal closure, with ABA controlling the increase in ethylene concentration in leaves (Chen et al., 2013), while ethylene blocks the ABA signaling pathway and leads to slow stomatal closure (Harrison, 2012; Arc et al., 2013). ABA inhibits the expression of the ethylene biosynthesis gene (ACS5) that encodes an enzyme for determining the rate-limiting step of ethylene biosynthesis. ABA also positively controls ethylene response factor 11 (ERF11) behavior through the long hypocotyl5 (HY5) replication factor, an important molecular contact between ABA and ethylene synthesis that represses ACS5 gene expression. The regulation of YF-AtERF11 expression is crucial for ABA-controlled ethylene biosynthesis (Li et al., 2011; Corbineau et al., 2014). Exogenous SA mitigated Cd stress in Triticum aestivum L. by increasing GSH content, resulting in metal detoxification and scavenging ROS induced by HM-triggered ethylene production. SA supplementation increased ABA levels in wheat seedlings under Cd stress, which was attributed to de novo ABA biosynthesis. Further, endogenous ABA-controlled SA-mediated changes in the concentration of dehydrin proteins under HM stress demonstrated the protective mechanism of SA in Triticum aestivum L. (Kovács et al., 2014; Shakirova et al., 2016).
A complex interaction between ABA and the jasmonate (JA) signaling pathway controls plant protection and gene expression. Exogenous ABA application reduces JA-induced transcription of defense genes. In contrast, mutations in the ABA synthesis genes aba1 and aba2 caused the overexpression of JA-responsive genes (Anderson et al., 2004). Several components of ABA and the JA-ethylene signaling pathway interact antagonistically to control the expression of several stress-responsive genes in response to abiotic and biotic stressors (Anderson et al., 2004; Proietti et al., 2018). Most JA signaling pathway components in Arabidopsis thaliana L. are regulated by the transcription factor MYC2. Furthermore, overexpression of the MYC2 gene in genetically engineered plants has been linked to increased ABA sensitivity and activation of numerous ABA-induced genes. In contrast, Myc2 mutants with Ds mutations are less susceptible to ABA and have lower gene expression (Chen et al., 2011). As a result, MYC2 in Arabidopsis thaliana L. is an integrative hub for controlling JA and ABA signals (Sun and Li, 2014).
Abscisic acid and nitric oxide (NO) may interact to regulate plant growth and physiological processes, including seed germination, root growth, stomatal movement, and antioxidant enzyme activities (Hancock et al., 2011; Prakash et al., 2019). Increasing ABA levels in tobacco by increasing the expression of the 9-cis-epoxycarotenoid dioxygenase gene (SgNCED1) increased H2O2 and NO levels in mesophyll and guard cells, increasing the replication and activity of many antioxidant enzymes (Zhang Y. et al., 2009). The breakdown of seed dormancy in Arabidopsis thaliana L. caused rapid ABA depletion due to rapid NO accumulation, with the opposite effect during seed germination (Liu et al., 2009; Signorelli and Considine, 2018). Moreover, in guard cells, ABA-induced endogenous H2O2 production increased NO production to control stomatal movement (Bright et al., 2006; Saradadevi et al., 2014). Furthermore, exogenous NO application alleviated the inhibitory effect of ABA on leaf senescence in Oryza sativa L. (Hung and Kao, 2003). ABA and NO modulate metabolic plant functions in response to environmental stress by improving osmolyte synthesis and the antioxidant defense system (Iqbal et al., 2021, 2022). During HM stress, NO may interact with ABA to weaken HM stress. NO reduced the As-induced upregulation of ABA content in Vicia faba L., reducing As toxicity (Mohamed et al., 2016). Sadeghipour (2017) revealed that exogenous application of NO to Vigna unguiculata L. reduced ABA content under Pb stress, enhancing Pb tolerance. Wu et al. (2018) reported that molybdenum (Mo) induced ABA synthesis in Triticum aestivum L. by upregulating aldehyde oxidase activity. The NO acting downstream of ABA was involved in oxidative stress induced by Mo, implying an intermediate linkage in the relationship between ABA and NO.
As a result, ABA is a crucial plant growth regulator that coordinates with other phytohormones to control plant growth and development under adverse environmental conditions. Further research is needed on the molecular mechanisms behind complex signaling networks of ABA and other hormones.
Heavy metal toxicity is a severe environmental restraint that hampers plant growth and productivity of food crops. PGRs are small signaling molecules that regulate many physiological and molecular processes in plants. Exogenous application of PGRs is an efficient approach to ameliorating the deleterious effects of HM and improving plant morphological characteristics, physio-biochemical processes, and production. As a vital PGR, ABA controls many developmental and metabolic functions of plants. This review revealed that exogenous ABA application alleviates the toxic effects of HM in plants by improving osmolyte synthesis, antioxidant machinery, phytochelatins synthesis, and enzymatic activities. It is also well-known that ABA has a significant role in providing abiotic stress tolerance in plants and improving plant growth and production. ABA application through the soil, foliar spray, seed priming, and culture media positively enhances crop productivity and thus could be used to improve the growth and yield of economically important crops. ABA plays a crucial role in mitigating HM stress by strengthening the tolerance mechanism of plants. The available literature on the role of ABA in HM stress mitigation offers a brief insight into morphological and physio-biochemical functioning and modulation in plants under HM stress. Thus, more research is needed to explore the molecular mechanisms of HM sequestration regulated through ABA to (1) enhance our understanding of ABA homeostasis during the plant life cycle, (2) identify crosstalk mechanisms between ABA and other PGRs under HM and other abiotic stresses and (3) explore the dynamic role of ABA in crop plants using novel genomic, molecular, and proteomic approaches.
SK and SHS wrote and drafted the review article. YV, HSJ, PA, YC, and KHMS revised and edited the manuscript. All authors contributed to the article and approved the submitted version.
The authors declare that the research was conducted in the absence of any commercial or financial relationships that could be construed as a potential conflict of interest.
All claims expressed in this article are solely those of the authors and do not necessarily represent those of their affiliated organizations, or those of the publisher, the editors and the reviewers. Any product that may be evaluated in this article, or claim that may be made by its manufacturer, is not guaranteed or endorsed by the publisher.
Aqeel, M., Khali, N., Tufail, A., Ahmad, R. Z., Akhter, M. S., Luqman, M., et al. (2021). Elucidating the distinct interactive impact of cadmium and nickel on growth, photosynthesis, metal-homeostasis, and yield responses of mung bean (Vigna radiata L.) varieties. Environ. Sci. and Pollut. Res. 28, 27376–27390. doi: 10.1007/s11356-021-12579-5
Ahmad, A., Hadi, F., and Ali, N. (2015). Effective phytoextraction of cadmium (Cd) with increasing concentration of total phenolics and free proline in Cannabis sativa (L) plant under various treatments of fertilizers, plant growth regulators and sodium salt. Int. J. Phytoremediation 17, 56–65. doi: 10.1080/15226514.2.13.828018
Ahmad, P., Jaleel, C. A., Salem, M. A., Nabi, G., and Sharma, S. (2010). Roles of enzymatic and nonenzymatic antioxidants in plants during abiotic stress. Crit. Rev. Biotechnol. 30, 161–175. doi: 10.3109/07388550903524243
Ali, A., Pardo, J. M., and Yun, D. J. (2020). Desensitization of ABA-signaling: The swing from activation to degradation. Front. Plant Sci 11:379. doi: 10.3389/fpls.2020.00379
An, T., Gao, Y., Kuang, Q., Wu, Y., uz Zaman, Q., Zhang, Y., et al. (2022a). Effect of silicon on morpho-physiological attributes, yield and cadmium accumulation in two maize genotypes with contrasting root system size and health risk assessment. Plant Soil 1–18. doi: 10.1007/s11104-022-05384-7
An, T., Wu, Y., Xu, B., Zhang, S., Deng, X., Zhang, Y., et al. (2022b). Nitrogen supply improved plant growth and Cd translocation in maize at the silking and physiological maturity under moderate Cd stress. Ecotoxicol Environ. Saf. 230:113137. doi: 10.1016/j.ecoenv.2021.113137
Anderson, J. P., Badruzsaufari, E., Schenk, P. M., Manners, J. M., Desmond, O. J., Ehlert, C., et al. (2004). Antagonistic interaction between abscisic acid and jasmonate-ethylene signaling pathways modulates defense gene expression and disease resistance in Arabidopsis. Plant Cell. 16, 3460–3479. doi: 10.1105/tpc.104.025833
Andolfo, G., Ruocco, M., Di Donato, A., Frusciante, L., Lorito, M., Scala, F., et al. (2015). Genetic variability and evolutionary diversification of membrane ABC transporters in plants. BMC Plant Biol. 15:1–15. doi: 10.1186/s12870-014-0323-2
Arc, E., Sechet, J., Corbineau, F., Rajjou, L., and Marion-Poll, A. (2013). ABA crosstalk with ethylene and nitric oxide in seed dormancy and germination. Front. Plant Sci. 4:63. doi: 10.3389/fpls.2013.00063
Arduini, I., Masoni, A., Mariotti, M., Pampana, S., and Ercoli, L. (2014). Cadmium uptake and translocation in durum wheat varieties differing in grain-Cd accumulation. Plant Soil Environ. 60, 43–49. doi: 10.17221/416/2013-pse
Ashraf, U., Kanu, A. S., Deng, Q., Mo, Z., Pan, S., Tian, H., et al. (2017). Lead (Pb) toxicity; physio-biochemical mechanisms, grain yield, quality, and Pb distribution proportions in scented rice. Front. Plant Sci. 8:259. doi: 10.3389/fpls.2017.00259
Avalbaev, A. M., Somov, K. A., Yuldashev, R. A., and Shakirova, F. M. (2012). Cytokinin oxidase is key enzyme of cytokinin degradation. Biochemistry (Moscow). 77, 1354–1361. doi: 10.1134/s0006297912120024
Baron, K. N., Schroeder, D. F., and Stasolla, C. (2012). Transcriptional response of abscisic acid (ABA) metabolism and transport to cold and heat stress applied at the reproductive stage of development in Arabidopsis thaliana. Plant Sci. 188, 48–59. doi: 10.1016/j.plantsci.2012.03.001
Bavi, K., Kholdebarin, B., and Moradshahi, A. (2011). Effect of cadmium on growth, protein content and peroxidase activity in pea plants. Pak. J. Bot. 43, 1467–1470.
Bicalho, E. M., Pintó-Marijuan, M., Morales, M., Müller, M., Munné-Bosch, S., and Garcia, Q. S. (2015). Control of macaw palm seed germination by the gibberellin/abscisic acid balance. Plant Biol. 17, 990–996. doi: 10.1111/plb.12332
Bright, J., Desikan, R., Hancock, J. T., Weir, I. S., and Neill, S. J. (2006). ABA-induced NO generation and stomatal closure in Arabidopsis are dependent on H2O2 synthesis. Plant J. 45, 113–122. doi: 10.1111/j.1365-313x.2005.02615.x
Cardoso, A. A., Gori, A., Da-Silva, C. J., and Brunetti, C. (2020). Abscisic acid biosynthesis and signaling in plants: Key targets to improve water use efficiency and drought tolerance. Applied Sci. 10:6322. doi: 10.3390/app10186322
Chandrakar, V., Yadu, B., Xalxo, R., Kumar, M., and Keshavkant, S. (2020). “Mechanisms of plant adaptation and tolerance to metal/metalloid toxicity,” in Plant ecophysiology and adaptation under climate change: Mechanisms and perspectives II, (Singapore: Springer), 107–135. doi: 10.1007/978-981-15-2172-0_6
Chen, K., Chen, P., Qiu, X., Chen, J., Gao, G., Wang, X., et al. (2021). Regulating role of abscisic acid on cadmium enrichment in ramie (Boehmeria nivea L.). Sci. Rep. 11, 1–10. doi: 10.1038/s41598-021-00322-6
Chen, L. I. N., Dodd, I. C., Davies, W. J., and Wilkinson, S. (2013). Ethylene limits abscisic acid-or soil drying-induced stomatal closure in aged wheat leaves. Plant Cell Environ. 36, 1850–1859. doi: 10.1111/pce.12094
Chen, Q., Sun, J., Zhai, Q., Zhou, W., Qi, L., Xu, L., et al. (2011). The basic helix-loop-helix transcription factor MYC2 directly represses PLETHORA expression during jasmonate-mediated modulation of the root stem cell niche in Arabidopsis. Plant Cell. 23, 3335–3352. doi: 10.1105/tpc.111.089870
Chen, T. T., Liu, F. F., Xiao, D. W., Jiang, X. Y., Li, P., Zhao, S. M., et al. (2020). The Arabidopsis UDP-glycosyltransferase75B1, conjugates abscisic acid and affects plant response to abiotic stresses. Plant Mol. Biol. 102, 389–401. doi: 10.1007/s11103-019-00953-4
Chen, Z., Liu, Q., Chen, S., Zhang, S., Wang, M., Munir, M. A. M., et al. (2022). Roles of exogenous plant growth regulators on phytoextraction of Cd/Pb/Zn by Sedum alfredii Hance in contaminated soils. Environ. Pollut. 293:118510. doi: 10.1016/j.envpol.2021.118510
Cheng, L., Pu, L., Li, A., Zhu, X., Zhao, P., Xu, X., et al. (2022). Implication of exogenous abscisic acid (ABA) application on phytoremediation: Plants grown in co-contaminated soil. Environ. Sci. Pollut. Res. 29, 8684–8693. doi: 10.1007/s11356-021-16241-y
Corbineau, F., Xia, Q., Bailly, C., and El-Maarouf-Bouteau, H. (2014). Ethylene, a key factor in the regulation of seed dormancy. Front. Plant Sci. 5:539. doi: 10.3389/fpls.2014.00539
Da-lin, L., Kai-qi, H., Jing-jing, M., Wei-wei, Q., Xiu-ping, W., and Shu-pan, Z. (2011). Effects of cadmium on the growth and physiological characteristics of sorghum plants. Afr. J. Biotechnol. 10, 15770–15776. doi: 10.5897/AJB11.848
Dawuda, M. M., Liao, W., Hu, L., Yu, J., Xie, J., Calderón-Urrea, A., et al. (2020). Foliar application of abscisic acid mitigates cadmium stress and increases food safety of cadmium-sensitive lettuce (Lactuca sativa L.) genotype. Peer. J. 8:e9270. doi: 10.7717/peerj.9270
Deng, B., Zhang, W., and Yang, H. (2021). Abscisic acid decreases cell death in Malus hupehensis Rehd. Under Cd stress by reducing root Cd 2+ influx and leaf transpiration. J. Plant Growth Regul. 40, 1–8. doi: 10.1007/s00344-021-10327-0
Emamverdian, A., Ding, Y., Mokhberdoran, F., and Xie, Y. (2015). Heavy metal stress and some mechanisms of plant defense response. Sci. World J. 2015:756120. doi: 10.1155/2015/756120
Fan, S. K., Fang, X. Z., Guan, M. Y., Ye, Y. Q., Lin, X. Y., Du, S. T., et al. (2014). Exogenous abscisic acid application decreases cadmium accumulation in Arabidopsis plants, which is associated with the inhibition of IRT1-mediated cadmium uptake. Front. Plant Sci. 5:721. doi: 10.3389/fpls.2014.00721
Frey, A., Audran, C., Marin, E., Sotta, B., and Marion-Poll, A. (1999). Engineering seed dormancy by the modification of zeaxanthin epoxidase gene expression. Plant Mol. Biol. 39, 1267–1274. doi: 10.1023/A:100614502563
Ghani, A. (2010). Toxic effects of heavy metals on plant growth and metal accumulation in maize (Zea mays L.). Iran. J. Toxicol. 325–334.
Ghori, N. H., Ghori, T., Hayat, M. Q., Imadi, S. R., Gul, A., Altay, V., et al. (2019). Heavy metal stress and responses in plants. Int. J. Environ. Sci. Technol. 16, 1807–1828. doi: 10.1007/s13762-019-02215-8
Giannakoula, A., Therios, I., and Chatzissavvidis, C. (2021). Effect of lead and copper on photosynthetic apparatus in citrus (Citrus aurantium L.) plants. The role of antioxidants in oxidative damage as a response to heavy metal stress. Plants 10:155. doi: 10.3390/plants10010155
Goyal, D., Yadav, A., Prasad, M., Singh, T. B., Shrivastav, P., Ali, A., et al. (2020). “Effect of heavy metals on plant growth: An overview,” in Contaminants in agriculture, (Cham: Springer), 79–101. doi: 10.1007/978-3-030-41552-5_4
Han, Y., Wang, S., Zhao, N., Deng, S., Zhao, C., Li, N., et al. (2016). Exogenous abscisic acid alleviates cadmium toxicity by restricting Cd 2+ influx in Populus euphratica cells. J. Plant Growth Regul. 35, 827–837. doi: 10.1007/s00344-016-9585-2
Hancock, J. T., Neill, S. J., and Wilson, I. D. (2011). Nitric oxide and ABA in the control of plant function. Plant Sci. 181, 555–559. doi: 10.1016/j.plantsci.2011.03.017
Harrison, M. A. (2012). “Cross-talk between phytohormone signaling pathways under both optimal and stressful environmental conditions,” in Phytohormones and abiotic stress tolerance in plants, (Berlin: Springer), 49–76.
Hasanuzzaman, M., Bhuyan, M. H. M., Nahar, K., Hossain, M., Mahmud, J. A., Hossen, M., et al. (2018). Potassium: A vital regulator of plant responses and tolerance to abiotic stresses. Agronomy. 8:31. doi: 10.3390/agronomy8030031
He, G., Tian, W., Qin, L., Meng, L., Wu, D., Huang, Y., et al. (2021). Identification of novel heavy metal detoxification proteins in Solanum tuberosum: Insights to improve food security protection from metal ion stress. Sci. Total Environ. 779:146197. doi: 10.1016/j.scitotenv.2021.146197
Hou, N., You, J., Pang, J., Xu, M., Chen, G., and Yang, Z. (2010). The accumulation and transport of abscisic acid insoybean (Glycine max L.) under aluminum stress. Plant Soil 330, 127–137. doi: 10.1007/s11104-009-0184-x
Hsu, Y. T., and Kao, C. H. (2003). Role of abscisic acid in cadmium tolerance of rice (Oryza sativa L.) seedlings. Plant Cell Environ. 26, 867–874. doi: 10.1046/j.1365-3040.2003.01018.x
Hu, B., Den, F., Chen, G., Chen, X., Gao, W., Long, L., et al. (2020). Evolution of abscisic acid signaling for stress responses to toxic metals and metalloids. Front. Plant Sci. 11:909. doi: 10.3389/fpls.2020.00909
Huihui, Z., Xin, L., Zisong, X., Yue, W., Zhiyuan, T., Meijun, A., et al. (2020). Toxic effects of heavy metals Pb and Cd on mulberry (Morus alba L.) seedling leaves: Photosynthetic function and reactive oxygen species (ROS) metabolism responses. Ecotoxicol. Environ. Saf. 195:110469. doi: 10.1016/j.ecoenv.2020.110469
Hung, K. T., and Kao, C. H. (2003). Nitric oxide counteracts the senescence of rice leaves induced by abscisic acid. J. Plant Physiol. 160, 871–879. doi: 10.1078/0176-1617-01118
Iqbal, N., Sehar, Z., Fatma, M., Umar, S., Sofo, A., and Khan, N. A. (2022). Nitric oxide and abscisic acid mediate heat stress tolerance through regulation of osmolytes and antioxidants to protect photosynthesis and growth in wheat plants. Antioxidants 11:372. doi: 10.3390/antiox11020372
Iqbal, N., Umar, S., Khan, N. A., and Corpas, F. J. (2021). Crosstalk between abscisic acid and nitric oxide under heat stress: Exploring new vantage points. Plant Cell Rep. 40, 1429–1450. doi: 10.1007/s00299-021-02695-4
Irfan, M., Hasan, S. A., Hayat, S., and Ahmad, A. (2015). Photosynthetic variation and yield attributes of two mustard varieties against cadmium phytotoxicity. Cogent Food Agric. 1:1106186. doi: 10.1080/23311932.2015.1106186
Jalmi, S. K., Bhagat, P. K., Verma, D., Noryang, S., Tayyeba, S., Singh, K., et al. (2018). Traversing the links between heavy metal stress and plant signaling. Front. Plant Sci. 9:12. doi: 10.3389/fpls.2018.00012
Jensen, M. K., Lindemose, S., De Mas, F., Reimer, J. J., Nielsen, M., Perera, V., et al. (2013). ATAF1 transcription factor directly regulates abscisic acid biosynthetic gene NCED3 in Arabidopsis thaliana. FEBS Open Bio. 3, 321–327. doi: 10.1016/j.fob.2013.07.006
John, R., Ahmad, P., Gadgil, K., and Sharma, S. (2009). Heavy metal toxicity: Effect on plant growth, biochemical parameters and metal accumulation by Brassica juncea L. Int. J. Plant Prod. 3, 1735–8043.
Kamran, M., Danish, M., Saleem, M. H., Malik, Z., Parveen, A., Abbasi, G. H., et al. (2021). Application of abscisic acid and 6-benzylaminopurine modulated morpho-physiological and antioxidative defense responses of tomato (Solanum lycopersicum L.) by minimizing cobalt uptake. Chemosphere 263:128169. doi: 10.1016/j.chemosphere.2020.128169
Kang, J., Hwang, J. U., Lee, M., Kim, Y. Y., Assmann, S. M., Martinoia, E., et al. (2010). PDR-type ABC transporter mediates cellular uptake of the phytohormone abscisic acid. Proc. Natl. Acad. Sci 107, 2355–2360. doi: 10.1073/pnas.0909222107
Kang, J., Yim, S., Choi, H., Kim, A., Lee, K. P., Lopez-Molin, L., et al. (2015). Abscisic acid transporters cooperate to control seed germination. Nat. Commun. 6, 1–10. doi: 10.1038/ncomms9113
Kanno, Y., Hanada, A., Chiba, Y., Ichikawa, T., Nakazawa, M., Matsui, M., et al. (2012). Identification of an abscisic acid transporter by functional screening using the receptor complex as a sensor. Proc. Natl. Acad. Sci. 109, 9653–9658. doi: 10.1073/pnas.1203567109
Keyster, M., Niekerk, L. A., Basson, G., Carelse, M., Bakare, O., Ludidi, N., et al. (2020). Decoding heavy metal stress signalling in plants: Towards improved food security and safety. Plants. 9:1781. doi: 10.3390/plants9121781
Khan, M. A., Khan, S., Khan, A., and Alam, M. (2017). Soil contamination with cadmium, consequences and remediation using organic amendments. Sci. Total Environ. 601, 1591–1605. doi: 10.1016/j.scitotenv.2017.06.030
Kitahata, N., Saito, S., Miyazawa, Y., Umezawa, T., Shimada, Y., Min, Y. K., et al. (2005). Chemical regulation of abscisic acid catabolism in plants by cytochrome P450 inhibitors. Bioorg. Med. Chem. 13, 4491–4498. doi: 10.1016/j.bmc.2005.04.036
Kovács, V., Gondor, O. K., Szalai, G., Darkó, É, Majláth, I., Janda, T., et al. (2014). Synthesis and role of salicylic acid in wheat varieties with different levels of cadmium tolerance. J. Hazard. Mater. 280, 12–19. doi: 10.1016/j.jhazmat.2014.07.048
Kumar, S., Ahanger, M. A., Alshaya, H., Jan, B. L., and Yerramilli, V. (2022). Salicylic acid mitigates salt induced toxicity through the modifications of biochemical attributes and some key antioxidants in Capsicum annuum. Saudi J. Biol. Sci. 29, 1337–1347. doi: 10.1016/j.sjbs.2022.01.028
Kuromori, T., Miyaji, T., Yabuuchi, H., Shimizu, H., Sugimoto, E., Kamiya, A., et al. (2010). ABC transporter AtABCG25 is involved in abscisic acid transport and responses. Proc. Natl. Acad. Sci. U.S.A. 107, 2361–2366. doi: 10.1073/pnas.0912516107
Kuromori, T., Sugimoto, E., and Shinozaki, K. (2011). Arabidopsis mutants of AtABCG22, an ABC transporter gene, increase water transpiration and drought susceptibility. Plant J. 67, 885–894. doi: 10.1111/j.1365-313x.2011.04641.x
Lajayer, B. A., Moghadam, N. K., Maghsoodi, M. R., Ghorbanpour, M., and Kariman, K. (2019). Phytoextraction of heavy metals from contaminated soil, water and atmosphere using ornamental plants: Mechanisms and efficiency improvement strategies. Environ. Sci. Pollut. Res. 26, 8468–8484. doi: 10.1007/s11356-019-04241-y
Lee, H. G., Lee, K., and Seo, P. (2015). The Arabidopsis MYB96 transcription factor plays a role in seed dormancy. Plant Mol. Biol. 87, 371–381. doi: 10.1007/s11103-015-0283-4
Leng, Y., Li, Y., Ma, Y. H., He, L. F., and Li, S. W. (2021). Abscisic acid modulates differential physiological and biochemical responses of roots, stems, and leaves in mung bean seedlings to cadmium stress. Environ. Sci. Pollut. Res. 28, 6030–6043. doi: 10.1007/s11356-020-10843-8
Li, X. S., and Song, L. L. (2020). The role of ABA in the responses of wild-type and abscisic acid mutants of Arabidopsis thaliana to excess zinc. Acta Physiol. Plant. 42, 1–8. doi: 10.1007/s11738-020-03067-3
Li, Z., Zhang, L., Yu, Y., Quan, R., Zhang, Z., Zhang, H., et al. (2011). The ethylene response factor AtERF11 that is transcriptionally modulated by the bZIP transcription factor HY5 is a crucial repressor for ethylene biosynthesis in Arabidopsis. The Plant J. 68, 88–99. doi: 10.1111/j.1365-313x.2011.04670.x
Liu, H. F., Xue, X. J., Yu, Y., Xu, M. M., Lu, C. C., Meng, X. L., et al. (2020). Copper ions suppress abscisic acid biosynthesis to enhance defence against Phytophthora infestans in potato. Mol. Plant Pathol. 21, 636–651. doi: 10.1111/mpp.12919
Liu, X., Zhang, H., Zhao, Y., Feng, Z., Li, Q., Yan, H. Q., et al. (2013). Auxin controls seed dormancy through stimulation of abscisic acid signaling by inducing ARF-mediated ABI3 activation in Arabidopsis. Proc. Natl Acad. Sci. U.S.A. 110, 15485–15490. doi: 10.1073/pnas.1304651110
Liu, Y., Shi, L., Ye, N., Liu, R., Jia, W., and Zhang, J. (2009). Nitric oxide-induced rapid decrease of abscisic acid concentration is required in breaking seed dormancy in Arabidopsis. New Phytologist. 183, 1030–1042. doi: 10.1111/j.1469-8137.2009.02899.x
Lou, X., Zhang, X., Zhang, Y., and Tang, M. (2021). The synergy of arbuscular mycorrhizal fungi and exogenous abscisic acid benefits Robinia pseudoacacia L. growth through altering the distribution of Zn and endogenous abscisic acid. J. Fungi. 7:671. doi: 10.3390/jof7080671
Lu, Q., Chen, S., Li, Y., Zheng, F., He, B., and Gu, M. (2020). Exogenous abscisic acid (ABA) promotes cadmium (Cd) accumulation in Sedum alfredii hance by regulating the expression of Cd stress response genes. Environ. Sci. Pollut. Res. 27, 8719–8731. doi: 10.1007/s11356-019-07512-w
Ma, Y., Cao, J., He, J., Chen, Q., Li, X., and Yang, Y. (2018). Molecular mechanism for the regulation of ABA homeostasis during plant development and stress responses. Int. J. Mol. Sci. 19:3643. doi: 10.3390/ijms19113643
Małkowski, E., Sitko, K., Zieleźnik-Rusinowska, P., Gieroń, Ż, and Szopiński, M. (2019). Heavy metal toxicity: Physiological implications of metal toxicity in plants. In Plant metallomics and functional omics. Cham: Springer, 253–301. doi: 10.1007/978-3-030-19103-010
Matilla, A. J. (2020). Auxin: Hormonal signal required for seed development and dormancy. Plants. 9:705. doi: 10.3390/plants9060705
Mayonde, S., Cron, G. V., Glennon, K. L., and Byrne, M. J. (2021). Effects of cadmium toxicity on the physiology and growth of a halophytic plant, Tamarix usneoides (E. Mey. ex Bunge). Int. J. Phytoremediation 23, 130–138. doi: 10.1080/15226514.2020.1801573
Mehrotra, R., Bhalothia, P., Bansal, P., Basantani, M. K., Bharti, V., and Mehrotra, S. (2014). Abscisic acid and abiotic stress tolerance–Different tiers of regulation. J. Plant Physiol. 171, 486–496. doi: 10.1016/j.jplph.2013.12.007
Meng, H., Hua, S., Shamsi, I. H., Jilani, G., Li, Y., and Jiang, L. (2009). Cadmium-induced stress on the seed germination and seedling growth of Brassica napus L., and its alleviation through exogenous plant growth regulators. Plant Growth Regul. 58, 47–59. doi: 10.1007/s10725-008-9351-y
Merilo, E., Jalakas, P., Laanemets, K., Mohammadi, O., Hõrak, H., Kollist, H., et al. (2015). Abscisic acid transport and homeostasis in the context of stomatal regulation. Mol. Plant. 8, 1321–1333. doi: 10.1016/j.molp.2015.06.006
Milborrow, B. V. (2001). The pathway of biosynthesis of abscisic acid in vascular plants: A review of the present state of knowledge of ABA biosynthesis. J. Exp. Bot 52, 1145–1164. doi: 10.1093/jexbot/52.359.1145
Mohamed, H. I., Latif, H. H., and Hanafy, R. S. (2016). Influence of nitric oxide application on some biochemical aspects, endogenous hormones, minerals and phenolic compounds of Vicia faba plant grown under arsenic stress. Gesunde Pflanzen 68, 99–107. doi: 10.1007/s10343-016-0363-7
Nambara, E., and Marion-Poll, A. (2005). Abscisic acid biosynthesis and catabolism. Annu. Rev. Plant Biol. 56, 165–185. doi: 10.1146/annurev.arplant.56.0326040144046
Ng, L. M., Melcher, K., The, B. T., and Xu, H. E. (2014). Abscisic acid perception and signaling: Structural mechanisms and applications. Acta Pharmacol. Sin. 35, 567–584. doi: 10.1038/aps.2014.5
Okamoto, M., Tanaka, Y., Abrams, S. R., Kamiya, Y., Seki, M., and Nambara, E. (2009). High humidity induces abscisic acid 8′-hydroxylase in stomata and vasculature to regulate local and systemic abscisic acid responses in Arabidopsis. Plant Physiol. 149, 825–834. doi: 10.1104/pp.108.130823
Pan, W., You, Y., Shentu, J. L., Weng, Y. N., Wang, S. T., Xu, Q. R., et al. (2020). Abscisic acid (ABA)-importing transporter 1 (AIT1) contributes to the inhibition of Cd accumulation via exogenous ABA application in Arabidopsis. J. Hazard Mater 391:122189. doi: 10.1016/j.jhazmat.2020.122189
Poschenrieder, C., Gunse, B., and Barcelo, J. (1989). Influence of cadmium on water relations, stomatal resistance, and abscisic acid content in expanding bean leaves. Plant Physiol. 90, 1365–1371. doi: 10.1104/pp.90.4.1365
Prakash, V., Singh, V. P., Tripathi, D. K., Sharma, S., and Corpas, F. J. (2019). Crosstalk between nitric oxide (NO) and abscisic acid (ABA) signalling molecules in higher plants. Environ. Exp. Bot. 161, 41–49. doi: 10.1016/j.envexpbot.2018.10.033
Priest, D. M., Ambrose, S. J., Vaistij, F. E., Elias, L., Higgins, G. S., Ross, A. R., et al. (2006). Use of the glucosyltransferase UGT71B6 to disturb abscisic acid homeostasis in Arabidopsis thaliana. The Plant J. 46, 492–502. doi: 10.1111/j.1365-313X.2006.02701.x
Proietti, S., Caarl, L., Coolen, S., Van Pelt, J. A., Van Wees, S. C., and Pieterse, C. M. (2018). Genome-wide association study reveals novel players in defense hormone crosstalk in Arabidopsis. Plant Cell Environ. 41, 2342–2356. doi: 10.1111/pce.13357
Rahman, M. A., Hasegawa, H., Rahman, M. M., Islam, M. N., Miah, M. M., and Tasmen, A. (2007). Effect of arsenic on photosynthesis, growth and yield of five widely cultivated rice (Oryza sativa L.) varieties in Bangladesh. Chemosphere 67, 1072–1079. doi: 10.1016/j.chemosphere.2006.11.061
Rock, C. D., and Sun, X. (2005). Crosstalk between ABA and auxin signaling pathways in roots of Arabidopsis thaliana (L.) Heynh. Planta 222, 98–106. doi: 10.1007/s00425-005-1521-9
Rodriguez, P. L. (2016). Abscisic acid catabolism generates phaseic acid, a molecule able to activate a subset of ABA receptors. Mol. Plant. 9, 1448–1450. doi: 10.1016/j.molp.2016.09.009
Sadeghipour, O. (2017). Nitric oxide increases Pb tolerance by lowering Pb uptake and translocation as well as phytohormonal changes in cowpea (Vigna unguiculata (L.) Walp.). Sains Malaysiana 46, 189–195. doi: 10.17576/jms-2017-4602-02
Sah, S. K., Reddy, K. R., and Li, J. (2016). Abscisic acid and abiotic stress tolerance in crop plants. Front. Plant Sci. 7:571. doi: 10.3389/fpls.2016.00571
Saha, I., Hasanuzzaman, M., and Adak, M. K. (2021). Abscisic acid priming regulates arsenite toxicity in two contrasting rice (Oryza sativa L.) genotypes through differential functioning of sub1A quantitative trait loci. Environ. Pollut. 287:117586. doi: 10.1016/j.envpol.2021.117586
Saini, S., Kaur, N., and Pati, P. K. (2021). Phytohormones: Key players in the modulation of heavy metal stress tolerance in plants. Ecotoxicol. Environ. Saf. 223:112578. doi: 10.1016/j.ecoenv.2021.112578
Saini, S., Sharma, I., and Pati, P. K. (2015). Versatile roles of brassinosteroid in plants in the context of its homeostasis, signaling and crosstalks. Front. Plant Sci. 6:950. doi: 10.3389/fpls.2015.00950
Saito, S., Hirai, N., Matsumoto, C., Ohigashi, H., Ohta, D., Sakata, K., et al. (2004). Arabidopsis CYP707A s encode (+)-abscisic acid 8′-hydroxylase, a key enzyme in the oxidative catabolism of abscisic acid. Plant Physiol. 134, 1439–1449. doi: 10.1104/pp.103.037614
Saradadevi, R., Bramley, H., Palta, J. A., and Siddique, K. H. M. (2016). Stomatal behaviour under terminal drought affects post-anthesis water use in wheat. Funct. Plant Biol. 44, 279–289. doi: 10.1071/FP16078
Saradadevi, R., Bramley, H., Siddique, K. H. M., Edwards, E., and Palta, J. A. (2014). Reprint of “Contrasting stomatal regulation and leaf ABA concentrations in wheat genotypes when split root systems were exposed to terminal drought”. Field Crops Res. 165, 5–14. doi: 10.1016/j.fcr.2014.02.004
Saradadevi, R., Palta, J. A., and Siddique, K. H. M. (2017). ABA-mediated stomatal response in regulating water use during the development of terminal drought in wheat. Front. Plant Sci. 8:1251. doi: 10.3389/fpls.2017.01251
Schwartz, S. H., and Zeevaart, J. A. (2010). Abscisic acid biosynthesis and metabolism. In Plant hormones. Dordrecht: Springer, 137–155.
Seo, M., and Koshiba, T. (2002). Complex regulation of ABA biosynthesis in plants. Trends Plant Sci. 7, 41–48. doi: 10.1016/s1360-1385(01)02187-2
Seo, M., and Marion-Poll, A. (2019). “Abscisic acid metabolism and transport,” in Advances in Botanical Research, Vol. 92, (Cambridge, MA: Academic Press), doi: 10.1046/j.1365-313x.2000.00812.x
Seo, M., Peeters, A. J., Koiwai, H., Oritani, T., Marion-Poll, A., Zeevaart, J. A., et al. (2000). The Arabidopsis aldehyde oxidase 3 (AAO3) gene product catalyzes the final step in abscisic acid biosynthesis in leaves. Proc. Natl. Acad. Sci. U.S.A. 97, 12908–12913. doi: 10.1073/pnas.22042697
Shah, S. H., Islam, S., and Mohammad, F. (2022). Sulphur as a dynamic mineral element for plants: A review. J. Soil Sci. Plant Nutr. 22, 1–26. doi: 10.1007/s42729-022-00798-9
Shah, S. H., Islam, S., Parrey, Z. A., and Mohammad, F. (2021). Role of exogenously applied plant growth regulators in growth and development of edible oilseed crops under variable environmental conditions: A review. J. Soil Sci. Plant Nutr. 21, 1–25. doi: 10.1007/s42729-021-00606-w
Shahid, M., Khalid, S., Abbas, G., Shahid, N., Nadeem, M., Sabir, M., et al. (2015). Heavy metal stress and crop productivity. In crop production and global environmental issues. Cham: Springer, 1–25. doi: 10.1007/978-3-319-23162-4_1
Shakirova, F. M., Allagulova, C. R., Maslennikova, D. R., Klyuchnikova, E. O., Avalbaev, A. M., and Bezrukova, M. V. (2016). Salicylic acid-induced protection against cadmium toxicity in wheat plants. Environ. Exp. Bot. 122, 19–28. doi: 10.1016/j.envexpbot.2015.08.002
Sheetal, K. R., Singh, S. D., Anand, A., and Prasad, S. (2016). Heavy metal accumulation and effects on growth, biomass and physiological processes in mustard. Indian J. Plant Physiol. 21, 219–223. doi: 10.1007/s40502-016-0221-8
Shen, G., Niu, J., and Deng, Z. (2017). Abscisic acid treatment alleviates cadmium toxicity in purple flowering stalk (Brassica campestris L. ssp. chinensis var. purpurea Hort.) seedlings. Plant Physiol. Biochem. 118, 471–478. doi: 10.1016/j.plaphy.2017.07.018
Shi, W. G., Liu, W., Yu, W., Zhang, Y., Ding, S., Li, H., et al. (2019). Abscisic acid enhances lead translocation from the roots to the leaves and alleviates its toxicity in Populus× canescens. J. Hazard. Mater. 362, 275–285. doi: 10.1016/j.jhazmat.2018.09.024
Shimizu, T., Kanno, Y., Suzuki, H., Watanabe, S., and Seo, M. (2021). Arabidopsis NPF4. 6 and NPF5. 1 control leaf stomatal aperture by regulating abscisic acid transport. Genes 12:885. doi: 10.3390/genes12060885
Shu, J., He, Q., Zheng, H., Liao, M. A., Kuang, N., and Huang, Z. (2021). Abscisic acid improves the ability of accumulator plant Perilla frutescens to perform phytoremediation in cadmium-contaminated soils. Int. J. Environ. Anal. Chem 18, 1–10. doi: 10.1080/03067319.2021.1890058
Shu, K., Zhou, W., Chen, F., Luo, X., and Yang, W. (2018). Abscisic acid and gibberellins antagonistically mediate plant development and abiotic stress responses. Front. Plant Sci. 9:416. doi: 10.3389/fpls.2018.00416
Signorelli, S., and Considine, M. J. (2018). Nitric oxide enables germination by a four-pronged attack on ABA-induced seed dormancy. Front. Plant Sci. 9:296. doi: 10.3389/fpls.2018.00296
Singh, A. D., Sharma, P., Kohli, S. K., Kumar, P., Singh, R., Arora, P., et al. (2020). “Role of plant growth regulators (PGRs) in mitigation of heavy metal phytotoxicity in plants,” in Cellular and molecular phytotoxicity of heavy metals, (Cham: Springer), 263–304. doi: 10.1007/978-3-030-45975-8_14
Sirko, A., Wawrzyńska, A., Brzywczy, J., and Sieńko, M. (2021). Control of ABA signaling and crosstalk with other hormones by the selective degradation of pathway components. Int. J. Mol. Sci. 22:4638. doi: 10.3390/ijms22094638
Song, C., Yan, Y., Rosado, A., Zhang, Z., and Castellarin, S. D. (2019). ABA alleviates uptake and accumulation of zinc in grapevine (Vitis vinifera L.) by inducing expression of ZIP and detoxification-related genes. Front. Plant Sci 10:872. doi: 10.3389/fpls.2019.00872
Stroiński, A., Giżewska, K., and Zielezińska, M. (2013). Abscisic acid is required in transduction of cadmium signal to potato roots. Biol. Plant. 57, 121–127. doi: 10.1007/s10535-012-0135-x
Sun, J., and Li, C. (2014). “Cross talk of signaling pathways between ABA and other phytohormones,” in Abscisic acid: Metabolism, transport and signaling, (Dordrecht: Springer), 243–253.
Sunita, K., Lokesh, B., Anita, D., Swarna, S., and Suneja, A. (2015). Effect of cadmium on growth, nitrogen metabolism, photosynthesis and yield of soybean. Int. J. Trop. Agric. 33, 993–1001.
Taiz, L., Zeiger, E., Møller, I. M., and Murphy, A. (2015). Plant physiology and development, No. Ed. 6 Edn. Sunderland: Sinauer Associates Incorporated.
Tan, B. C., Joseph, L. M., Deng, W. T., Liu, L., Li, Q. B., Cline, K., et al. (2003). Molecular characterization of the Arabidopsis 9-cis epoxy carotenoid dioxygenase gene family. Plant J. 35, 44–56. doi: 10.1046/j.1365-313X.2003.01786.x
Tang, Y., Wang, L., Xie, Y., Yu, X., Li, H., Lin, L., et al. (2020). Effects of exogenous abscisic acid on the growth and cadmium accumulation of lettuce under cadmium-stress conditions. Int. J. Environ. Anal. Chem. 100, 720–731. doi: 10.1080/03067319.2019.1639686
Tao, Q., Jupa, R., Dong, Q., Yang, X., Liu, Y., Li, B., et al. (2021). Abscisic acid-mediated modifications in water transport continuum are involved in cadmium hyperaccumulation in Sedum alfredii. Chemosphere. 268:129339. doi: 10.1016/j.chemosphere.2020.129339
Tao, Q., Jupa, R., Liu, Y., Luo, J., Li, J., Kováč, J., et al. (2019). Abscisic acid-mediated modifications of radial apoplastic transport pathway play a key role in cadmium uptake in hyperaccumulator Sedum alfredii. Plant Cell Environ. 42, 1425–1440. doi: 10.1111/pce.13506
Tran, L. S. P., Urao, T., Qin, F., Maruyama, K., Kakimoto, T., Shinozaki, K., et al. (2007). Functional analysis of AHK1/ATHK1 and cytokinin receptor histidine kinases in response to abscisic acid, drought, and salt stress in Arabidopsis. Proc. Natl. Acad. Sci. U.S.A. 104, 20623–20628. doi: 10.1073/pnas.0706547105
Ubeda-Tomás, S., Swarup, R., Coates, J., Swarup, K., Laplaze, L., Beemster, G. T., et al. (2008). Root growth in Arabidopsis requires gibberellin/DELLA signalling in the endodermis. Nat. Cell Biol. 10, 625–628. doi: 10.1038/ncb1726
Vimala, Y. (1985). Change in the activity of certain enzyme during natural and induced loss of seed viability. J. Indian Bot. Soc. 63, 61b–68b.
Vishwakarma, K., Upadhyay, N., Kumar, N., Yadav, G., Singh, J., Mishra, R. K., et al. (2017). Abscisic acid signaling and abiotic stress tolerance in plants: A review on current knowledge and future prospects. Front. Plant Sci. 8:161. doi: 10.3389/fpls.2017.00161
Wang, J., Lin, L., Luo, L., Liao, M. A., Lv, X., Wang, Z., et al. (2016). The effects of abscisic acid (ABA) addition on cadmium accumulation of two ecotypes of Solanum photeinocarpum. Environ. Monit. Assess. 188, 1–8. doi: 10.1007/s10661-016-5194-6
Wang, L., Hua, D., He, J., Duan, Y., Chen, Z., Hong, X., et al. (2011). Auxin Response Factor2 (ARF2) and its regulated homeodomain gene HB33 mediate abscisic acid response in Arabidopsis. PLoS Genet. 7:e1002172. doi: 10.1371/journal.pgen.1002172
Wang, Y., Li, L., Ye, T., Lu, Y., Chen, X., and Wu, Y. (2013). The inhibitory effect of ABA on floral transition is mediated by ABI5 in Arabidopsis. J. Exp. Bot. 64, 675–684. doi: 10.1093/jxb/ers361
Wani, P. A., Khan, M. S., and Zaidi, A. (2007). Impact of heavy metal toxicity on plant growth, symbiosis, seed yield and nitrogen and metal uptake in chickpea. Aust. J. Exp. Agric. 47, 712–720. doi: 10.1071/EA05369
Werner, T., Köllmer, I., Bartrina, I., and Holst Schmülling, T. (2006). New insights into the biology of cytokinin degradation. Plant Biol. 8, 371–381. doi: 10.1055/s-2006-923928
White, C. N., Proebsting, W. M., Hedden, P., and Rivin, C. J. (2000). Gibberellins and seed development in maize. I. Evidence that gibberellin/abscisic acid balance governs germination versus maturation pathways. Plant Physiol. 122, 1081–1088. doi: 10.1104/pp.122.4.1081
Wu, M., Wu, J., and Gan, Y. (2020). The new insight of auxin functions: Transition from seed dormancy to germination and floral opening in plants. Plant Growth Regul. 91, 169–174. doi: 10.1007/s10725-020-00608-1
Wu, S., Hu, C., Tan, Q., Zhao, X., Xu, S., Xia, Y., et al. (2018). Nitric oxide acts downstream of abscisic acid in molybdenum-induced oxidative tolerance in wheat. Plant Cell Rep. 37, 599–610. doi: 10.1007/s00299-018-2254-0
Wyszkowski, M., and Radziemska, M. (2010). Effects of chromium (III and VI) on spring barley and maize biomass yield and content of nitrogenous compounds. J Toxicol Environ Health. 73, 1274–1282. doi: 10.1080/15287394.2010.492016
Xiong, L., and Zhu, J. K. (2003). Regulation of abscisic acid biosynthesis. Plant Physiol. 133, 29–36. doi: 10.1104/pp.103.025395
Xu, W., Jia, L., Shi, W., Liang, J., Zhou, F., Li, Q., et al. (2013). Abscisic acid accumulation modulates auxin transport in the root tip to enhance proton secretion for maintaining root growth under moderate water stress. New Phytol. 197, 139–150. doi: 10.1111/nph.12004
Xu, Z. Y., Lee, K. H., Dong, T., Jeong, J. C., Jin, J. B., Kanno, Y., et al. (2012). A vacuolar β-glucosidase homolog that possesses glucose-conjugated abscisic acid hydrolyzing activity plays an important role in osmotic stress responses in Arabidopsis. Plant Cell 24, 2184–2199. doi: 10.1105/tpc.112.095935
Xu, Z., Ge, Y., Zhang, W., Zhao, Y., and Yang, G. (2018). The walnut JrVHAG1 gene is involved in cadmium stress response through ABA-signal pathway and MYB transcription regulation. BMC Plant Biol. 18:1–13. doi: 10.1186/s12870-018-1231-7
Yang, X., Bai, Y., Shang, J., Xin, R., and Tang, W. (2016). The antagonistic regulation of abscisic acid-inhibited root growth by brassinosteroids is partially mediated via direct suppression of abscisic acid insensitive 5 expression by brassinazole resistant 1. Plant Cell Environ. 39, 1994–2003. doi: 10.1111/pce.12763
Ye, Y., Zhou, L., Liu, X., Liu, H., Li, D., Cao, M., et al. (2017). A novel chemical inhibitor of ABA signaling targets all ABA receptors. Plant Physiol. 173, 2356–2369. doi: 10.1104/pp.16.01862
Yildirim, E., Ekinci, M., Turan, M., Güleray, A., Selda, ÖR. S., Dursun, A., et al. (2019). Impact of cadmium and lead heavy metal stress on plant growth and physiology of rocket (Eruca sativa L.). Kahramanmaraş Sütçü Ýmam Üniversitesi Tarım ve Doğa Dergisi 22, 843–850. doi: 10.18016/ksutarimdoga.vi.548626
Yoshida, T., Mogami, J., and Yamaguchi-Shinozaki, K. (2014). ABA-dependent and ABA-independent signaling in response to osmotic stress in plants. Curr. Opin. Plant Biol. 21, 133–139. doi: 10.1016/j.pbi.2014.07.009
Yuan, K., Rashotte, A. M., and Wysocka-Diller, J. W. (2011). ABA and GA signaling pathways interact and regulate seed germination and seedling development under salt stress. Acta Physiol. Plant. 33, 261–271. doi: 10.1007/s11738-010-0542-6
Zengin, F. K., and Kirbag, S. (2007). Effects of copper on chlorophyll, proline, protein and abscisic acid level of sunflower (Helianthus annuus L.) seedlings. J Environ. Biol. 28:561.
Zhang, H., Han, W., De Smet, I., Talboys, P., Loya, R., Hassan, A., et al. (2010). ABA promotes quiescence of the quiescent center and suppresses stem cell differentiation in the Arabidopsis primary root meristem. Plant J. 64, 764–774. doi: 10.1111/j.1365-313X.2010.04367.x
Zhang, H., Zhu, H., Pan, Y., Yu, Y., Luan, S., and Li, L. (2014). A DTX/MATE-type transporter facilitates abscisic acid efflux and modulates ABA sensitivity and drought tolerance in Arabidopsis. Mol. Plant. 7, 1522–1532. doi: 10.1093/mp/ssu063
Zhang, S., Cai, Z., and Wang, X. (2009). The primary signaling outputs of brassinosteroids are regulated by abscisic acid signaling. Proc. Natl. Acad. Sci. U.S.A. 106, 4543–4548. doi: 10.1073/pnas.0900349106
Zhang, Y., Tan, J., Guo, Z., Lu, S., He, S., Shu, W., et al. (2009). Increased abscisic acid levels in transgenic tobacco over-expressing 9 cis-epoxycarotenoid dioxygenase influence H2O2 and NO production and antioxidant defenses. Plant Cell Environ. 32, 509–519. doi: 10.1111/j.1365-3040.2009.01945.x
Zhao, H., Guan, J., Liang, Q., Zhang, X., Hu, H., and Zhang, J. (2021). Effects of cadmium stress on growth and physiological characteristics of sassafras seedlings. Sci. Rep. 11, 1–11. doi: 10.1038/s41598-021-89322-0
Zheng, C., Acheampong, A. K., Shi, Z., Mugzech, A., Halaly-Basha, T., Shaya, F., et al. (2018). Abscisic acid catabolism enhances dormancy release of grapevine buds. Plant Cell Environ. 41, 2490–2503. doi: 10.1111/pce.13371
Keywords: abscisic acid, heavy metal, growth, physiological processes, antioxidant, productivity
Citation: Kumar S, Shah SH, Vimala Y, Jatav HS, Ahmad P, Chen Y and Siddique KHM (2022) Abscisic acid: Metabolism, transport, crosstalk with other plant growth regulators, and its role in heavy metal stress mitigation. Front. Plant Sci. 13:972856. doi: 10.3389/fpls.2022.972856
Received: 19 June 2022; Accepted: 17 August 2022;
Published: 14 September 2022.
Edited by:
Mirza Hasanuzzaman, Sher-e-Bangla Agricultural University, BangladeshReviewed by:
Wen-Cheng Liu, Henan University, ChinaCopyright © 2022 Kumar, Shah, Vimala, Jatav, Ahmad, Chen and Siddique. This is an open-access article distributed under the terms of the Creative Commons Attribution License (CC BY). The use, distribution or reproduction in other forums is permitted, provided the original author(s) and the copyright owner(s) are credited and that the original publication in this journal is cited, in accordance with accepted academic practice. No use, distribution or reproduction is permitted which does not comply with these terms.
*Correspondence: Yerramilli Vimala, yvimala17@gmail.com; Kadambot H. M. Siddique, kadambot.siddique@uwa.edu.au
†These authors have contributed equally to this work
Disclaimer: All claims expressed in this article are solely those of the authors and do not necessarily represent those of their affiliated organizations, or those of the publisher, the editors and the reviewers. Any product that may be evaluated in this article or claim that may be made by its manufacturer is not guaranteed or endorsed by the publisher.
Research integrity at Frontiers
Learn more about the work of our research integrity team to safeguard the quality of each article we publish.