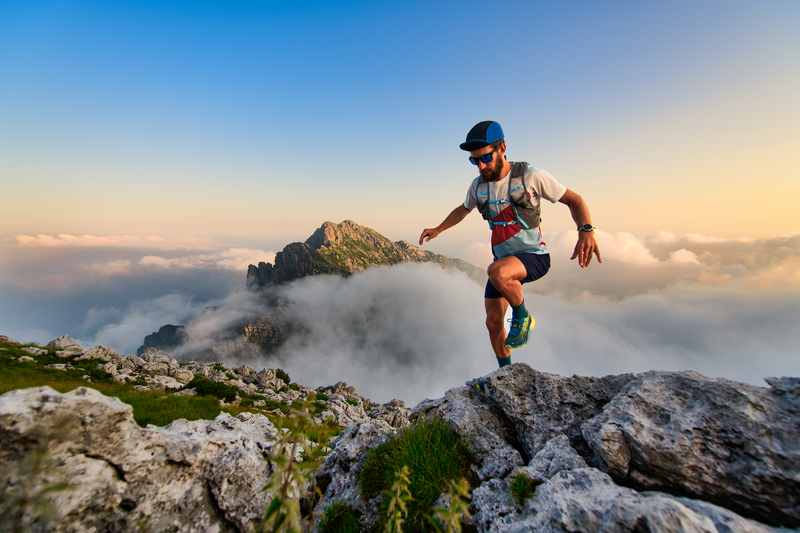
95% of researchers rate our articles as excellent or good
Learn more about the work of our research integrity team to safeguard the quality of each article we publish.
Find out more
MINI REVIEW article
Front. Plant Sci. , 12 August 2022
Sec. Plant Physiology
Volume 13 - 2022 | https://doi.org/10.3389/fpls.2022.968665
This article is part of the Research Topic Ethylene: A key regulatory molecule in plants, Volume II View all 8 articles
To cope with nutrient scarcity, plants generally follow two main complementary strategies. On the one hand, they can slow down growing, mainly shoot growth, to diminish the demand of nutrients. We can call this strategy as “stop growing.” On the other hand, plants can develop different physiological and morphological responses, mainly in their roots, aimed to facilitate the acquisition of nutrients. We can call this second strategy as “searching for nutrients.” Both strategies are compatible and can function simultaneously but the interconnection between them is not yet well-known. In relation to the “stop growing” strategy, it is known that the TOR (Target Of Rapamycin) system is a central regulator of growth in response to nutrients in eukaryotic cells. TOR is a protein complex with kinase activity that promotes protein synthesis and growth while some SnRK (Sucrose non-fermenting 1-Related protein Kinases) and GCN (General Control Non-derepressible) kinases act antagonistically. It is also known that some SnRKs and GCNs are activated by nutrient deficiencies while TOR is active under nutrient sufficiency. In relation to the “searching for nutrients” strategy, it is known that the plant hormone ethylene participates in the activation of many nutrient deficiency responses. In this Mini Review, we discuss the possible role of ethylene as the hub connecting the “stop growing” strategy and the “searching for nutrients” strategy since very recent results also suggest a clear relationship of ethylene with the TOR system.
Terrestrial plants are sessile organisms that have to continuously change their physiology and morphology to adapt to the availability of different nutrients in the soil. To cope with nutrient deficiencies, they can adopt several strategies (Romera et al., 2021), like to stop growth (“stop growing” strategy), to limit the demand of nutrients (Marschner et al., 1996; Shin, 2011; Marzec and Melzer, 2018); to develop physiological and morphological responses (“searching for nutrients” strategy), to facilitate the acquisition of the deficient nutrient(s) (Kobayashi and Nishizawa, 2012; Zhang et al., 2014; García et al., 2015; Romera et al., 2021); to recycle the deficient nutrient(s) from the oldest leaves and organs to the youngest ones (Masclaux-Daubresse et al., 2017; Chen et al., 2019); and to substitute the deficient nutrient(s) by other(s) that can play a similar role (Wakeel et al., 2011; Lambers et al., 2012; Waters et al., 2012). These different strategies are not incompatible between them and can occur simultaneously. This Mini Review will focus on the two first strategies, the “stop growing” strategy and the “searching for nutrients” strategy, whose interrelationship is not yet known. It is clear that, if plants dedicate resources to activate nutrient deficiency responses, they must take away them from other functions, such as growth. In fact, iron (Fe) efficient soybean varieties offer lower yields than the inefficient ones when grown under Fe sufficient conditions (Atwood et al., 2014). The “stop growing” strategy is frequently used by wild plant genotypes adapted to impoverished soils since, in this way, they can limit the demand of nutrients without showing any symptoms of deficiency. In crop plants, however, the “stop growing” strategy can have undesirable side effects because their yield may be compromised. On the other hand, if crop plant genotypes keep growing despite nutrient deficiencies, this can lead to clear symptoms of deficiency. Thus, it is necessary a balance between both kind of strategies, in such a way that crop plants can acquire the nutrients they need by means of the “searching for nutrients” strategy without compromising their growth and yield by the “stop growing” strategy.
In yeasts and animals, growth is to a large degree controlled by the TOR system, which is a protein complex with kinase activity that stimulates protein synthesis and growth when there are enough nutrients and other favorable conditions. In plants, although less studied, the TOR pathway integrates hormonal and nutritional signals to regulate plant growth and development (Robaglia et al., 2012; Barrada et al., 2015; Dobrenel et al., 2016a; Rosenberger and Chen, 2018; Ryabova et al., 2019; Wu et al., 2019; Fu et al., 2020). In relation to the “searching for nutrients” strategy, it is known that plants can develop morphological and physiological responses, mainly in their roots, aimed to facilitate mobilization and acquisition of the deficient nutrient(s) (Zhang et al., 2014; García et al., 2015, 2021a,b; Romera et al., 2021). These responses are activated when plants suffer from a nutrient deficiency and are switched off once the nutrient has been acquired in sufficient quantity. Several hormones and signaling molecules, like ethylene (ET), auxin and nitric oxide (NO), have been implicated as activators of most of them (Romera and Alcántara, 2004; Jung et al., 2009; García et al., 2011, 2015; Lucena et al., 2015; Romera et al., 2016, 2017). Very recently, it has been found that TOR can affect ET synthesis and signaling (Dong et al., 2015; Zhuo et al., 2020; Fu et al., 2021), which indicates that both strategies (“stop growing” and “searching for nutrients”) could be interconnected through TOR and ET (Figure 1).
Figure 1. Working model to explain the possible roles of the TOR/SnRKs/GCNs regulatory system and ethylene (ET) in plant growth and development of nutrient deficiency responses. Under nutrient sufficiency, the TOR kinase is activated leading to an increase of protein synthesis and meristem activity, and consequently to a promotion of plant growth. In this condition, TOR blocks ET synthesis and signaling. Under nutrient deficiency, other kinases, like SnRKs and GCNs, are activated leading to inhibition of TOR, protein synthesis, meristem activity, and growth. In this condition, ET synthesis and signaling is enhanced, which leads to the activation of nutrient deficiency responses, mainly in roots, aimed at acquiring the deficient nutrient from the medium. Based on Robaglia et al. (2012), García et al. (2015, 2021a,b), Lucena et al. (2015), Dobrenel et al. (2016a), Rodríguez et al. (2019), Zhuo et al. (2020), Fu et al. (2021), and Romera et al. (2021).
As a general rule, the growth of plants is related to the quantity of nutrients they receive, in such a way that growth linearly increases when a determined nutrient augments until reaching an optimum and then decreases because of toxicity problems (Marschner, 2012). When nutrients are deficient, a frequent adaptive response of plants is the inhibition of shoot growth, to limit the demand of nutrients (Marschner et al., 1996; Shin, 2011). Root growth is often less inhibited, which increases the root/shoot ratio and consequently the capacity of roots to fulfill the demand of the deficient nutrient (Marschner et al., 1996; Borch et al., 1999; Shin, 2011; Marzec and Melzer, 2018).
Nutritional disorders that reduce growth and yield are generally characterized by specific visible symptoms. However, in some cases plants do not show apparent symptoms upon a nutrient deficiency because they stop growing and suffer a latent deficiency. The effects of the different nutrients on growth are not exactly the same. Some nutrient deficiencies affect more the youngest leaves and the apical meristems, such as calcium (Ca), boron (B), or iron (Fe) deficiency, while other ones affect more the oldest leaves, such as nitrogen (N), phosphorus (P), or potassium (K) deficiency (Marschner, 2012). Similarly, root growth is differently affected depending on the nutrient deficiencies (Gruber et al., 2013; Singh et al., 2018; Bouain et al., 2019).
As previously stated, to cope with nutrient deficiencies plants can develop physiological and morphological responses, mainly in roots, aimed to facilitate the acquisition of the deficient nutrient(s) (Shin, 2011; Kobayashi and Nishizawa, 2012; Zhang et al., 2014; García et al., 2015, 2021a,b; Romera et al., 2021). Following are some of the most frequent physiological and morphological responses.
Physiological responses include acidification of the rhizosphere; increased expression of specific nutrient transporters, both at the root epidermis and in other tissues; increased synthesis and/or release of chelating agents into the medium (to improve mobilization of nutrients in the soil and/or inside the plant); increased expression of transporters associated with the release of different compounds to the medium; enhancement of some root enzymatic activities, like ferric reductase activity and phosphatase activity; and others (Zhang et al., 2014; García et al., 2015, 2021a,b; Lucena et al., 2015, 2019; Romera et al., 2021).
Morphological responses include modifications of the root system architecture, related to primary root elongation and development of lateral roots; development of root hairs; of cluster roots (also named proteoid roots); and of root transfer cells. Most of these root modifications enhance nutrient uptake by increasing the surface of contact of roots with soil (Wang et al., 2014; García et al., 2015). In many cases, both physiological and morphological responses are located in the subapical regions of the roots where they act synergistically (Romera and Alcántara, 2004; Jung et al., 2009; García et al., 2011; Wang et al., 2014; Lucena et al., 2015).
Once the deficient nutrient has been acquired in sufficient quantity, the responses need to be switched off to avoid nutrient toxicity and energy costs. Their regulation is not totally known but several hormones and signaling molecules, such as ET, auxin, cytokinins, and NO, have been involved in their control (Krouk et al., 2011; Iqbal et al., 2013; García et al., 2015; Romera et al., 2017, 2021). ET has been involved in the activation of both physiological and morphological responses, like the acidification response, the expression of nutrient transporters, the release of phenolic compounds and the development of root hairs, to several nutrient deficiencies, such as P, Fe, K, Mg, and B (you can find abundant information about this in the following reviews and in the references therein: Romera and Alcántara, 2004; García et al., 2015; Lucena et al., 2015; Romera et al., 2016, 2017, 2021; Li and Lan, 2017).
Ethylene is synthesized from methionine via a pathway where ACC synthases (encoded by ACS genes) and ACC oxidases (encoded by ACO genes) participate, and where 1-aminocyclopropane-1-carboxylic acid (ACC) is the immediate ET precursor (Sauter et al., 2013). Although ET’s mode of action is not fully understood, a linear canonical signaling pathway has been proposed in Arabidopsis (Binder, 2020):
ET—||ET receptors → CTR1—||EIN2 → EIN3/EILs → ERFs → ET responses
In this pathway, CTR1 is a kinase, EIN2 is a protein located in the endoplasmic reticulum (ER) membrane, and EIN3, EILs and ERFs are transcription factors (Binder, 2020). EIN2 possesses a Nramp-like transmembrane domain and a cytosolic COOH end (CEND; Figure 2) domain (Binder, 2020). In the absence of ET, CTR1 phosphorylates EIN2, preventing the cleavage and translocation of CEND into the nucleus. In the presence of ET, CTR1 is inactivated, resulting in dephosphorylation of EIN2 and its cleavage. CEND is then translocated into the nucleus, where it finally enhances EIN3/EIL1 binding to target genes and transcription activation (Figure 2; Binder, 2020; Angulo et al., 2021).
Figure 2. Working model to explain the possible roles of ethylene (ET) and TOR in the activation of nutrient deficiency responses through EIN2. Left: in the absence of ethylene (ET), which occurs under nutrient sufficiency, the CTR1 kinase phosphorylates EIN2 at Ser645/Ser924, preventing the cleavage and translocation of CEND into the nucleus. In parallel, under nutrient sufficiency, the TOR kinase phosphorylates EIN2 at Thr657, preventing the cleavage and translocation of CEND into the nucleus. Right: under nutrient deficiency, ET production is enhanced and, once perceived by ET receptors, CTR1 is inactivated, thus resulting in dephosphorylation of EIN2 and its cleavage. CEND is then translocated into the nucleus, where results in more EIN3/EIL1 binding to target genes and ultimately transcription activation of ET responsive genes, like those related to nutrient deficiency responses. Similarly, under nutrient deficiency, TOR is inactivated, which could also result in dephosphorylation of EIN2 and its cleavage and translocation into the nucleus. Whether the influence of TOR on EIN2 plays a key role in the activation or deactivation of nutrient deficiency responses deserves further research. ER, Endoplasmic Reticulum; NM, Nuclear Membrane. Based on Angulo et al. (2021) and Fu et al. (2021).
The general control of plant growth has been associated with some protein kinases, like Target Of Rapamycin (TOR), Sucrose non-fermenting 1-Related protein Kinases (SnRK1, SnRK2) and General Control Non-derepressible (GCN) kinases (GCN1, GCN2, GCN20; Robaglia et al., 2012; Barrada et al., 2015; Dobrenel et al., 2016a; Sesma et al., 2017; Rosenberger and Chen, 2018; Shi et al., 2018; Ryabova et al., 2019; Li et al., 2021). Under nutrient sufficiency and other favorable conditions, TOR promotes growth by activating meristems and anabolic processes, such as protein synthesis, and by repressing catabolic processes, such as autophagy (Figure 1; Barrada et al., 2015; Dobrenel et al., 2016a; Sesma et al., 2017; Rosenberger and Chen, 2018; Shi et al., 2018; Chen et al., 2019; Rodríguez et al., 2019; Ryabova et al., 2019; Couso et al., 2020). It should be noted that protein synthesis is one of the most energetically requiring processes (Dong et al., 2017; Romero et al., 2020). In contrast, nutrient deficiency activates other kinases, like SnRKs and GCNs, that act antagonistically to TOR, thus repressing protein synthesis and growth (Figure 1; Robaglia et al., 2012; Barrada et al., 2015; Dobrenel et al., 2016a,b; Wang et al., 2017; Rosenberger and Chen, 2018; Shi et al., 2018; Chen et al., 2019; Rodríguez et al., 2019; Ryabova et al., 2019; Li et al., 2021). The ways TOR, SnRKs, and GCNs regulate protein synthesis is related to their capacity to control processes like ribosome biogenesis and mRNA translation (Echevarría-Zomeño et al., 2013; Dobrenel et al., 2016b; Dong et al., 2017; Sesma et al., 2017; Caballero-Molada et al., 2018; Faus et al., 2018; Shi et al., 2018; Rodríguez et al., 2019; Ryabova et al., 2019; Couso et al., 2020; Romero et al., 2020). For example, GCN2 phosphorylates the eIF2 factor, necessary to initiate mRNA translation, preventing it (Lageix et al., 2008; Sesma et al., 2017; Caballero-Molada et al., 2018; Faus et al., 2018; Romero et al., 2020). In plants, SnRKs can interact and phosphorylate RAPTOR, inhibiting TOR activity (Figure 1; Rodríguez et al., 2019 and references therein; Li et al., 2021 and references therein). However, the direct interaction between TOR and GCN2 is not yet clear in plants (Lageix et al., 2008).
In yeast and mammals, the TOR system comprises two protein complexes (TORC1 and TORC2) while in plants is only present the TORC1 complex, composed of TOR, RAPTOR (“Regulatory Associated Protein of TOR”) and LST8 (“Lethal with Sec Thirteen8”; Robaglia et al., 2012; Salem et al., 2018; Shi et al., 2018; Zhang et al., 2018; Rodríguez et al., 2019; Ryabova et al., 2019; Salem and Giavalisco, 2019). In most plant species, including Arabidopsis, TOR is encoded by a single gene (Menand et al., 2002; Dobrenel et al., 2016a). However, in Arabidopsis there are two RAPTOR genes, RAPTOR1A and RAPTOR1B, and two LST8 genes, LST8-1 and LST8-2, being RAPTOR1B (also named RAPTOR3G) and LST8-1 the most expressed ones (Moreau et al., 2012; Zhang et al., 2018; McCready et al., 2020; Pereyra et al., 2020). In plants, the TOR gene is not expressed in all tissues but in shoot and root meristems where plays a key role in their activity (Menand et al., 2002; Xiong et al., 2013; Li et al., 2017; Ryabova et al., 2019).
The relationship between the TOR system and the responses to nutrient deficiencies has not been deeply studied. However, there are several experimental results suggesting an interconnection between them, both in algae and in higher plants. In Chlamydomonas reinhardtii it has been found that the TORC1 complex activity depends on P availability (Couso et al., 2020). In Arabidopsis, TOR inhibition affects the expression of Fe-related genes as well as the development of root hairs, a typical morphological response to Fe deficiency (Ren et al., 2012). Besides Fe and P, TOR also affects K, S, and N assimilation (Xiong et al., 2013; Salem et al., 2018; Shi et al., 2018; Wu et al., 2019; Fu et al., 2020; Ingargiola et al., 2020). TOR signaling activates transcription of genes involved in sulfur assimilation and transport, like the sulfate transporter SULTR1.2, as well as genes involved in nitrogen assimilation and transport, like the nitrate transporter NRT1.1 (Xiong et al., 2013; Fu et al., 2020). By contrast, some nutrient deficiencies, like sulfur deficiency, affect TOR activity, probably through glucose energy signaling (Dong et al., 2017). Similarly, TOR is inhibited in nitrogen-deprived seedlings, and quickly reactivated upon resupply of either nitrate, ammonium, or amino acids (Liu et al., 2021).
Besides TOR, SnRKs and GCNs have also been related to nutrient stresses. In relation to SnRKs, it has been found that SnRK2.1 plays a key role in the signaling cascade leading to S deprivation responses in Chlamydomonas (Gonzalez-Ballester et al., 2008). In the same way, the Arabidopsis AtSnRK2.2, AtSnRK2.3, and AtSnRK2.4, and the Triticum polonicum TpSnRK2.10 and TpSnRK2.11 have been linked to the expression of several metal-related genes under Cd stress (Kulik et al., 2012; Fan et al., 2014; Wang et al., 2019). In relation to GCNs, there are several results suggesting a role for them on Fe nutrition, most of them in yeast (Caballero-Molada et al., 2018; Faus et al., 2018; Romero et al., 2020). In dicot plants, such as Glycine max and Medicago truncatula, it has also been found a relationship between GCN2 and Fe deficiency, since GCN2 expression is enhanced in both roots and leaves of Fe-deficient plants (Santos et al., 2016). GCN2 activation usually requires the participation of other GCN proteins, like GCN1 and GCN20 (Wang et al., 2017; Faus et al., 2018; Romero et al., 2020). In Arabidopsis, the GCN1 protein is also named ILITHYIA (ILA), and the gcn1 (ila) mutants present chlorosis of youngest leaves and shorter roots, both symptoms related to Fe deficiency (Faus et al., 2018).
As previously stated, ET is a key player in the activation of physiological and morphological responses to several nutrient deficiencies. Moreover, EIN2 and EIN3, two components of the ET transduction pathway, play crucial roles in such an activation (Angulo et al., 2021; García et al., 2021a,b). Consequently, ET is a critical signal for the regulation of nutrient deficiency responses. In addition, ET could have a very close relationship with the TOR/SnRKs/GCNs system: TOR inhibition positively affects ET (Figure 1) by up-regulating ET-synthesis genes, like ACS and ACO genes, and ET-signaling genes, like ERF genes (Dong et al., 2015; Zhuo et al., 2020); SnRK1 affects ET synthesis and signaling (Figure 1; Rodríguez et al., 2019 and references therein; Jamsheer et al., 2021 and references therein); and ACC (ET precursor) up-regulates GCN2 expression in Arabidopsis (Lageix et al., 2008). In general, when TOR activity is inhibited, and consequently growth, genes related to hormones that promote growth, like auxin and brassinosteroids, are repressed, while those that inhibit growth, like ET and abscisic acid (ABA), are up-regulated (Dong et al., 2015; Burkart and Brandizzi, 2021).
Very recently, a close link between TOR and ET has been reported. Fu et al. (2021) have found that EIN2, a critical component of the ET transduction pathway is a target of TOR. TOR is a kinase that could act in parallel to the CTR1 kinase, belonging to the canonical ET signaling pathway (Figure 2; Binder, 2020; Fu et al., 2021). As previously described, in the absence of ET, CTR1 phosphorylates EIN2, preventing the cleavage and translocation of its CEND portion into the nucleus. Similarly, TOR can phosphorylate EIN2 (at other phosphorylation sites than CTR1) and thereby could affect the cleavage and translocation of the CEND portion into the nucleus thus affecting processes downstream EIN2 (Figure 2; Fu et al., 2021). These results and the ones described in the above paragraph suggest a clear interrelationship between ET and the TOR system.
In conclusion, ET can be envisioned as a hub connecting the “searching for nutrients” strategy, since it is a key player in the activation of many nutrient deficiency responses, and the “stop growing” strategy, since its synthesis and signaling is negatively regulated by TOR. Moreover, ET itself can be an inhibitor of growth (Street et al., 2015).
The interrelation between the “stop growing” and the “searching for nutrients” strategy is a very important question for crop plants since, under nutrient deficiencies, they should keep growing to avoid a drastic reduction of their yield. However, this can only be done if they have the ability to develop efficient nutrient deficiency responses. TOR is implicated in the control of plant growth, and ET in the activation of nutrient deficiency responses. Since TOR can negatively affect ET synthesis and signaling, it is tempting to speculate that both strategies (“stop growing” and “searching for nutrients”) should be interrelated through the interdependency of TOR and ET, as suggested in Figures 1, 2. Nonetheless, this interaction has been barely studied and needs further research to answer questions like the following ones: Does the modification of TOR affect the activation of nutrient deficiency responses through EIN2 (Figure 2)? Does the onset of nutrient deficiency responses affect TOR? Do nutrient deficiencies affect TOR in the same way at the early stages of the deficiency and later on? Obviously, the working models depicted in Figures 1, 2 are simplified models because hormones and factors others than TOR and ET can influence growth and nutrient deficiency responses. The understanding of the interrelation between TOR and ET in the control of plant growth and development of nutrient deficiency responses is complex and has just begun.
We apologize to authors whose works were not cited in this review due to manuscript length restrictions.
MG and FR wrote a first draft of the manuscript that was corrected and improved by all the authors. All authors revised the information related to TOR, ET, and nutrient deficiency responses and approved the submitted version.
This work was supported by the European Regional Development Fund from the European Union, the “Ministerio de Economía y Competitividad” (Project RTI2018-097935-B-I00), the “Plan Propio” Universidad de Córdoba (Spain), and the “Junta de Andalucía” (Research Groups AGR115 and BIO159). We acknowledged the financial support from the Spanish Ministry of Science and Innovation, the Spanish State Research Agency, through the Severo Ochoa and María de Maeztu Program for Centers and Units of Excellence in R&D (Ref. CEX2019-000968-M).
The authors declare that the research was conducted in the absence of any commercial or financial relationships that could be construed as a potential conflict of interest.
All claims expressed in this article are solely those of the authors and do not necessarily represent those of their affiliated organizations, or those of the publisher, the editors and the reviewers. Any product that may be evaluated in this article, or claim that may be made by its manufacturer, is not guaranteed or endorsed by the publisher.
Angulo, M., García, M. J., Alcántara, E., Pérez-Vicente, R., and Romera, F. J. (2021). Comparative study of several Fe deficiency responses in the Arabidopsis thaliana ethylene insensitive mutants ein2-1 and ein2-5. Plants 10:262. doi: 10.3390/plants10020262
Atwood, S. E., O’Rourke, J. A., Peiffer, G. A., Yin, T., Majumder, M., Zhang, C., et al. (2014). Replication protein A subunit 3 and the iron efficiency response in soybean. Plant Cell Environ. 37, 213–234. doi: 10.1111/pce.12147
Barrada, A., Montané, M. H., Robaglia, C., and Menand, B. (2015). Spatial regulation of root growth: Placing the plant TOR pathway in a developmental perspective. Int. J. Mol. Sci. 16, 19671–19697. doi: 10.3390/ijms160819671
Binder, B. M. (2020). Ethylene signaling in plants. J. Biol. Chem. 295, 7710–7725. doi: 10.1074/jbc.REV120.010854
Borch, K., Bouma, T. J., Lynch, J. P., and Brown, K. M. (1999). Ethylene: A regulator of root architectural responses to soil phosphorus availability. Plant Cell Environ. 22, 425–431.
Bouain, N., Korte, A., Satbhai, S. B., Nam, H. I., Rhee, S. Y., Busch, W. (2019). Systems genomics approaches provide new insights into Arabidopsis thaliana root growth regulation under combinatorial mineral nutrient limitation. PLoS Genet. 15:e1008392. doi: 10.1371/journal.pgen
Burkart, G. M., and Brandizzi, F. (2021). A tour of TOR complex signaling in plants. Trends Biochem. Sci. 46, 417–428. doi: 10.1016/j.tibs.2020.11.004
Caballero-Molada, M., Planes, M. D., Benlloch, H., Atares, S., Naranjo, M. A., and Serrano, R. (2018). The Gcn2–eIF2α pathway connects iron and amino acid homeostasis in Saccharomyces cerevisiae. Biochem. J. 475, 1523–1534. doi: 10.1042/BCJ20170871
Chen, Q., Shinozaki, D., Luo, J., Pottier, M., Havé, M., Marmagne, A., et al. (2019). Autophagy and nutrients management in plants. Cells 8:1426. doi: 10.3390/cells8111426
Couso, I., Pérez-Pérez, M. E., Ford, M. M., Martínez-Force, E., Hicks, L. M., Umen, J. G., et al. (2020). Phosphorus availability regulates TORC1 signaling via LST8 in Chlamydomonas. Plant Cell 32, 69–80. doi: 10.1105/tpc.19.00179
Dobrenel, T., Caldana, C., Hanson, J., Robaglia, C., Vincentz, M., Veit, B., et al. (2016a). TOR signaling and nutrient sensing. Annu. Rev. Plant Biol. 67, 261–285. doi: 10.1146/annurev-arplant-043014-114648
Dobrenel, T., Mancera-Martinez, E., Forzani, C., Azzopardi, M., Davanture, M., Moreau, M., et al. (2016b). The Arabidopsis TOR kinase specifically regulates the expression of nuclear genes coding for plastidic ribosomal proteins and the phosphorylation of the cytosolic ribosomal protein S6. Front. Plant Sci. 7:1611. doi: 10.3389/fpls.2016.01611
Dong, P., Xiong, F., Que, Y., Wang, K., Yu, L., Li, Z., et al. (2015). Expression profiling and functional analysis reveals that TOR is a key player in regulating photosynthesis and phytohormone signaling pathways in Arabidopsis. Front. Plant Sci. 6:677. doi: 10.3389/fpls.2015.00677
Dong, Y., Silbermann, M., Speiser, A., Forieri, I., Linster, E., Poschet, G., et al. (2017). Sulfur availability regulates plant growth via glucose-TOR signaling. Nat. Commun. 8:1174. doi: 10.1038/s41467-017-01224-w
Echevarría-Zomeño, S., Yángüez, E., Fernández-Bautista, N., Castro-Sanz, A. B., Ferrando, A., and Castellano, M. M. (2013). Regulation of translation initiation under biotic and abiotic stresses. Int. J. Mol. Sci. 14, 4670–4683. doi: 10.3390/ijms14034670
Fan, S. K., Fang, X. Z., Guan, M. Y., Ye, Y. Q., Lin, X. Y., Du, S. T., et al. (2014). Exogenous abscisic acid application decreases cadmium accumulation in Arabidopsis plants, which is associated with the inhibition of IRT1-mediated cadmium uptake. Front. Plant Sci. 5:721. doi: 10.3389/fpls.2014.00721
Faus, I., Niñoles, R., Gadea, J., Llabata, P., Tam, E., Nebauer, S. G., et al. (2018). Arabidopsis ILITHYIA protein is necessary for proper chloroplast biogenesis and root development independent of eIF2α phosphorylation. J. Plant Physiol. 224-225, 173–182. doi: 10.1016/j.jplph.2018.04.003
Fu, L., Liu, Y., Qin, G., Wu, P., Zi, H., Xu, Z., et al. (2021). The TOR–EIN2 axis mediates nuclear signalling to modulate plant growth. Nature 591, 288–292. doi: 10.1038/s41586-021-03310-y
Fu, L., Wang, P., and Xiong, Y. (2020). Target of rapamycin signaling in plant stress responses. Plant Physiol. 182, 1613–1623. doi: 10.1104/pp.19.01214
García, M. J., Angulo, M., García, C., Lucena, C., Alcántara, E., Pérez-Vicente, R., et al. (2021a). Influence of ethylene signaling in the crosstalk between Fe, S, and P deficiency responses in Arabidopsis thaliana. Front. Plant Sci. 12:643585. doi: 10.3389/fpls.2021.643585
García, M. J., Lucena, C., and Romera, F. J. (2021b). Ethylene and nitric oxide involvement in the regulation of Fe and P deficiency responses in dicotyledonous plants. Int. J. Mol. Sci. 22:4904. doi: 10.3390/ijms22094904
García, M. J., Romera, F. J., Lucena, C., Alcántara, E., and Pérez-Vicente, R. (2015). Ethylene and the regulation of physiological and morphological responses to nutrient deficiencies. Plant Physiol. 169, 51–60. doi: 10.1104/pp.15.00708
García, M. J., Suárez, V., Romera, F. J., Alcántara, E., and Pérez-Vicente, R. (2011). A new model involving ethylene, nitric oxide and Fe to explain the regulation of Fe-acquisition genes in Strategy I plants. Plant Physiol. Biochem. 49, 537–544. doi: 10.1016/j.plaphy.2011.01.019
Gonzalez-Ballester, D., Pollock, S. V., Pootakham, W., and Grossman, A. R. (2008). The central role of a SnRK2 kinase in sulfur deprivation responses. Plant Physiol. 147, 216–227. doi: 10.1104/pp.108.116137
Gruber, B. D., Giehl, R. F., Friedel, S., and von Wirén, N. (2013). Plasticity of the Arabidopsis root system under nutrient deficiencies. Plant Physiol. 163, 161–179. doi: 10.1104/pp.113.218453
Ingargiola, C., Turqueto Duarte, G., Robaglia, C., Leprince, A. S., and Meyer, C. (2020). The plant target of rapamycin: A conduc TOR of nutrition and metabolism in photosynthetic organisms. Genes 11:1285. doi: 10.3390/genes11111285
Iqbal, N., Trivellini, A., Masood, A., Ferrante, A., and Khan, N. A. (2013). Current understanding on ethylene signaling in plants: The influence of nutrient availability. Plant Physiol. Biochem. 73, 128–138. doi: 10.1016/j.plaphy.2013.09.011
Jamsheer, M. K., Kumar, M., and Srivastava, V. (2021). SNF1-related protein kinase 1: The many-faced signaling hub regulating developmental plasticity in plants. J. Exp. Bot. 72, 6042–6065. doi: 10.1093/jxb/erab079
Jung, J. Y., Shin, R., and Schachtman, D. P. (2009). Ethylene mediates response and tolerance to potassium deprivation in Arabidopsis. Plant Cell 21, 607–621. doi: 10.1105/tpc.108.063099
Kobayashi, T., and Nishizawa, N. K. (2012). Iron uptake, translocation and regulation in higher plants. Annu. Rev. Plant Biol. 63, 131–152. doi: 10.1146/annurev-arplant-042811-105522
Krouk, G., Ruffel, S., Gutierrez, R. A., Gojon, A., Crawford, N. M., Coruzzi, G. M., et al. (2011). A framework integrating plant growth with hormones and nutrients. Trends Plant Sci. 16, 178–182. doi: 10.1016/j.tplants.2011.02.004
Kulik, A., Anielska-Mazur, A., Bucholc, M., Koen, E., Szymanska, K., Żmienko, A., et al. (2012). Snf1-Related protein Kinases type 2 are involved in plant responses to cadmium stress. Plant Physiol. 160, 868–883. doi: 10.1104/pp.112.194472
Lageix, S., Lanet, E., Pouch-Pélissier, M. N., Espagnol, M. C., Robaglia, C., Deragon, J. M., et al. (2008). Arabidopsis eIF2α kinase GCN2 is essential for growth in stress conditions and is activated by wounding. BMC Plant Biol. 8:134. doi: 10.1186/1471-2229-8-134
Lambers, H., Cawthray, G. R., Giavalisco, P., Kuo, J., Laliberté, E., Pearse, S. J., et al. (2012). Proteaceae from severely phosphorus-impoverished soils extensively replace phospholipids with galactolipids and sulfolipids during leaf development to achieve a high photosynthetic phosphorus-use-efficiency. New Phytol. 196, 1098–1108. doi: 10.1111/j.1469-8137.2012.04285.x
Li, L., Liu, K. H., and Sheen, J. (2021). Dynamic nutrient signaling networks in plants. Annu. Rev. Cell Dev. Biol. 37, 341–367. doi: 10.1146/annurev-cellbio-010521-015047
Li, W., and Lan, P. (2017). The understanding of the plant iron deficiency responses in Strategy I plants and the role of ethylene in this process by omic approaches. Front. Plant Sci. 8:40. doi: 10.3389/fpls.2017.00040
Li, X., Cai, W., Liu, Y., Li, H., Fu, L., Liu, Z., et al. (2017). Differential TOR activation and cell proliferation in Arabidopsis root and shoot apexes. Proc. Natl. Acad. Sci. U.S.A. 114, 2765–2770. doi: 10.1073/pnas.1618782114
Liu, Y., Duan, X., Zhao, X., Ding, W., Wang, Y., and Xiong, Y. (2021). Diverse nitrogen signals activate convergent ROP2-TOR signaling in Arabidopsis. Dev. Cell 56, 1283–1295. doi: 10.1016/j.devcel.2021.03.022
Lucena, C., Porras, R., García, M. J., Alcántara, E., Pérez-Vicente, R., Zamarreño, A. M., et al. (2019). Ethylene and phloem signals are involved in the regulation of responses to Fe and P deficiencies in roots of Strategy I plants. Front. Plant Sci. 10:1237. doi: 10.3389/fpls.2019.01237
Lucena, C., Romera, F. J., García, M. J., Alcántara, E., and Pérez-Vicente, R. (2015). Ethylene participates in the regulation of Fe deficiency responses in Strategy I plants and in rice. Front. Plant Sci. 6:1056. doi: 10.3389/fpls.2015.01056
Marschner, H., Kirkby, E. A., and Cakmak, I. (1996). Effect of mineral nutritional status on shoot-root partitioning of photoassimilates and cycling of mineral nutrients. J. Exp. Bot. 47, 1255–1263. doi: 10.1093/jxb/47.Special_Issue.1255
Marschner, P. (2012). Marschner’s Mineral Nutrition of Higher Plants, 3rd Edn. London: Academic Press.
Marzec, M., and Melzer, M. (2018). Regulation of root development and architecture by strigolactones under optimal and nutrient deficiency conditions. Int. J. Mol. Sci. 19:1887. doi: 10.3390/ijms19071887
Masclaux-Daubresse, C., Chen, Q., and Havé, M. (2017). Regulation of nutrient recycling via autophagy. Curr. Opin. Plant Biol. 39, 8–17. doi: 10.1016/j.pbi.2017.05.001
McCready, K., Spencer, V., and Kim, M. (2020). The importance of TOR kinase in plant development. Front. Plant Sci. 11:16. doi: 10.3389/fpls.2020.00016
Menand, B., Desnos, T., Nussaume, L., Berger, F., Bouchez, D., Meyer, C., et al. (2002). Expression and disruption of the Arabidopsis TOR (target of rapamycin) gene. Proc. Natl. Acad. Sci. U.S.A. 99, 6422–6427. doi: 10.1073/pnas.092141899
Moreau, M., Azzopardi, M., Clément, G., Dobrenel, T., Marchive, C., Renne, C., et al. (2012). Mutations in the Arabidopsis homolog of LST8/GbL, a partner of the target of rapamycin kinase, impair plant growth, flowering, and metabolic adaptation to long days. Plant Cell 24, 463–481. doi: 10.1105/tpc.111.091306
Pereyra, C. M., Aznar, N. R., Rodriguez, M. S., Salerno, G. L., and Martínez-Noël, G. M. A. (2020). Target of rapamycin signaling is tightly and differently regulated in the plant response under distinct abiotic stresses. Planta 251:21. doi: 10.1007/s00425-019-03305-0
Ren, M., Venglat, P., Qiu, S., Feng, L., Cao, Y., Wang, E., et al. (2012). Target of rapamycin signaling regulates metabolism, growth, and life span in Arabidopsis. Plant Cell 24, 4850–4874. doi: 10.1105/tpc.112.107144
Robaglia, C., Thomas, M., and Meyer, C. (2012). Sensing nutrient and energy status by SnRK1 and TOR kinases. Curr. Opin. Plant Biol. 15, 301–307. doi: 10.1016/j.pbi.2012.01.012
Rodríguez, M., Parola, R., Andreola, S., Pereyra, C., and Martínez-Noël, G. (2019). TOR and SnRK1 signaling pathways in plant response to abiotic stresses: Do they always act according to the “yin-yang” model? Plant Sci. 288, 110220. doi: 10.1016/j.plantsci.2019.110220
Romera, F. J., and Alcántara, E. (2004). Ethylene involvement in the regulation of Fe-deficiency stress responses by Strategy I plants. Funct. Plant Biol. 31, 315–328. doi: 10.1071/FP03165
Romera, F. J., Lucena, C., García, M. J., Alcántara, E., Angulo, M., Aparicio, M. A., et al. (2021). “Plant hormones and nutrient deficiency responses,” in Hormones and Plant Response, eds D. Gupta and F. J. Corpas (Dordrecht: Springer), 29–65.
Romera, F. J., Lucena, C., García, M. J., Alcántara, E., and Pérez-Vicente, R. (2017). “The role of ethylene and other signals in the regulation of Fe deficiency responses by dicot plants,” in Stress Signaling in Plants: Genomics and Proteomics Perspectives, Vol. 2, ed. M. Sarwat (Dordrecht: Springer), 277–300.
Romera, F. J., Smith, A. P., and Pérez-Vicente, R. (2016). Editorial: Ethylene’s role in plant mineral nutrition. Front. Plant Sci. 7:911. doi: 10.3389/fpls.2016.00911
Romero, A. M., Ramos-Alonso, L., Alepuz, P., Puig, S., and Martínez-Pastor, M. T. (2020). Global translational repression induced by iron deficiency in yeast depends on the Gcn2/eIF2α pathway. Sci. Rep. 10:233. doi: 10.1038/s41598-019-57132-0
Rosenberger, C. L., and Chen, J. (2018). To grow or not to grow: TOR and SnRK2 coordinate growth and stress response in Arabidopsis. Mol. Cell 69, 3–4. 2017.12.013 doi: 10.1016/j.molcel.2017.12.013
Ryabova, L. A., Robaglia, C., and Meyer, C. (2019). Target of Rapamycin kinase: Central regulatory hub for plant growth and metabolism. J. Exp. Bot. 70, 2211–2216. doi: 10.1093/jxb/erz108
Salem, M. A., and Giavalisco, P. (2019). Mutation in the Arabidopsis regulatory-associated protein TOR 1B (RAPTOR1B) leads to decreased jasmonates levels in leaf tissue. Plant Signal. Behav. 14:e1649567. doi: 10.1080/15592324.2019.1649567
Salem, M. A., Li, Y., Bajdzienko, K., Fisahn, J., Watanabe, M., Hoefgen, R., et al. (2018). RAPTOR controls developmental growth transitions by altering the hormonal and metabolic balance. Plant Physiol. 177, 565–593. doi: 10.1104/pp.17.01711
Santos, C. S., Serrão, I., and Vasconcelos, M. W. (2016). Comparative analysis of iron deficiency chlorosis responses in soybean (Glycine max) and barrel medic (Medicago truncatula). Rev. Cienc. Agrar. 39, 70–81. doi: 10.19084/RCA16090
Sauter, M., Moffatt, B., Saechao, M. C., Hell, R., and Wirtz, M. (2013). Methionine salvage and S-adenosylmethionine: Essential links between sulfur, ethylene and polyamine biosynthesis. Biochem. J. 451, 145–154. doi: 10.1042/BJ20121744
Sesma, A., Castresana, C., and Castellano, M. M. (2017). Regulation of translation by TOR, eIF4E and eIF2α in plants: Current knowledge, challenges and future perspectives. Front. Plant Sci. 8:644. doi: 10.3389/fpls.2017.00644
Shi, L., Wu, Y., and Sheen, J. (2018). TOR signaling in plants: Conservation and innovation. Development 145:dev160887. doi: 10.1242/dev.160887
Shin, R. (2011). Transcriptional regulatory components responding to macronutrient limitation. J. Plant Biol. 54, 286–293. doi: 10.1007/s12374-011-9174-7
Singh, A. P., Fridman, Y., Holland, N., Ackerman-Lavert, M., Zananiri, R., Jaillais, Y., et al. (2018). Interdependent nutrient availability and steroid hormone signals facilitate root growth plasticity. Dev. Cell 46, 59–72. doi: 10.1016/j.devcel.2018.06.002
Street, I. H., Aman, S., Zubo, Y., Ramzan, A., Wang, X., Shakeel, S. N., et al. (2015). Ethylene inhibits cell proliferation of the Arabidopsis root meristem. Plant Physiol. 169, 338–350. doi: 10.1104/pp.15.00415
Wakeel, A., Farooq, M., Qadir, M., and Schubert, S. (2011). Potassium substitution by sodium in plants. Crit. Rev. Plant Sci. 30, 401–413.
Wang, L., Li, H., Zhao, C., Li, S., Kong, L., Wu, W., et al. (2017). The inhibition of protein translation mediated by AtGCN1 is essential for cold tolerance in Arabidopsis thaliana. Plant Cell Environ. 40, 56–68. doi: 10.1111/pce.12826
Wang, R., Wang, C., Yao, Q., Xiao, X., Fan, X., and Sha, L. (2019). The polish wheat (Triticum polonicum L.) TpSnRK2.10 and TpSnRK2.11 meditate the accumulation and the distribution of Cd and Fe in transgenic Arabidopsis plants. BMC Genom. 20:210. doi: 10.1186/s12864-019-5589-1
Wang, Z., Straub, D., Yang, H., Kania, A., Shen, J., Ludewig, U., et al. (2014). The regulatory network of cluster-root function and development in phosphate-deficient white lupin (Lupinus albus) identified by transcriptome sequencing. Physiol. Plant. 151, 323–338. doi: 10.1111/ppl.12187
Waters, B. M., McInturf, S. A., and Stein, R. J. (2012). Rosette iron deficiency transcript and microRNA profiling reveals links between copper and iron homeostasis in Arabidopsis thaliana. J. Exp. Bot. 63, 5903–5918. doi: 10.1093/jxb/ers239
Wu, Y., Shi, L., Li, L., Fu, L., Liu, Y., Xiong, Y., et al. (2019). Integration of nutrient, energy, light, and hormone signalling via TOR in plants. J. Exp. Bot. 70, 2227–2238. doi: 10.1093/jxb/erz028
Xiong, Y., McCormack, M., Li, L., Hall, Q., Xiang, C., and Sheen, J. (2013). Glucose-TOR signalling reprograms the transcriptome and activates meristems. Nature 496, 181–187.
Zhang, Y., Zhang, Y., McFarlane, H. E., Obata, T., Richter, A. S., Lohse, M., et al. (2018). Inhibition of TOR represses nutrient consumption, which improves greening after extended periods of etiolation. Plant Physiol. 178, 101–117. doi: 10.1104/pp.18.00684
Zhang, Z., Liao, H., and ucas, W. J. (2014). Molecular mechanisms underlying phosphate sensing, signaling, and adaptation in plants. J. Integr. Plant Biol. 56, 192–220. doi: 10.1111/jipb.12163
Keywords: ethylene, nutrient deficiency responses, nutrient scarcity, plant growth, target of rapamycin (TOR)
Citation: García MJ, Angulo M, Lucena C, Pérez-Vicente R and Romera FJ (2022) To grow or not to grow under nutrient scarcity: Target of rapamycin-ethylene is the question. Front. Plant Sci. 13:968665. doi: 10.3389/fpls.2022.968665
Received: 14 June 2022; Accepted: 27 July 2022;
Published: 12 August 2022.
Edited by:
Antonio Ferrante, University of Milan, ItalyReviewed by:
Felipe Dos Santos Maraschin, Federal University of Rio Grande do Sul, BrazilCopyright © 2022 García, Angulo, Lucena, Pérez-Vicente and Romera. This is an open-access article distributed under the terms of the Creative Commons Attribution License (CC BY). The use, distribution or reproduction in other forums is permitted, provided the original author(s) and the copyright owner(s) are credited and that the original publication in this journal is cited, in accordance with accepted academic practice. No use, distribution or reproduction is permitted which does not comply with these terms.
*Correspondence: Francisco Javier Romera, YWcxcm9ydWZAdWNvLmVz
Disclaimer: All claims expressed in this article are solely those of the authors and do not necessarily represent those of their affiliated organizations, or those of the publisher, the editors and the reviewers. Any product that may be evaluated in this article or claim that may be made by its manufacturer is not guaranteed or endorsed by the publisher.
Research integrity at Frontiers
Learn more about the work of our research integrity team to safeguard the quality of each article we publish.