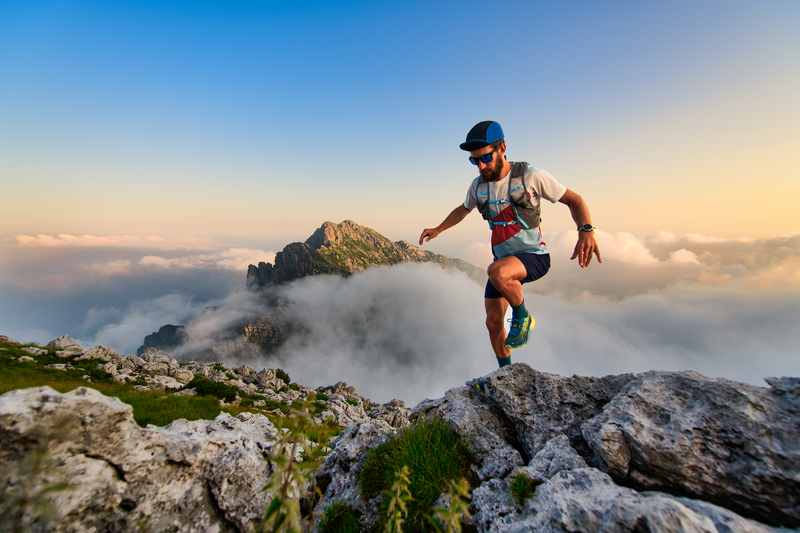
95% of researchers rate our articles as excellent or good
Learn more about the work of our research integrity team to safeguard the quality of each article we publish.
Find out more
ORIGINAL RESEARCH article
Front. Plant Sci. , 19 August 2022
Sec. Plant Bioinformatics
Volume 13 - 2022 | https://doi.org/10.3389/fpls.2022.967546
This article is part of the Research Topic Advances on Genomics and Genetics of Horticultural Crops and their Contribution to Breeding Efforts View all 7 articles
The bZIP gene family is one of the largest transcription factor families and has important roles in plant growth, development, and stress responses. However, bZIP genes in the Solanaceae family have not been extensively investigated. Here, we conducted genome-wide re-annotation in nine Solanaceae species and Arabidopsis thaliana. We annotated 935 bZIP genes, including 107 (11%) that were newly identified. Structural analyses of bZIP genes in the Solanaceae family revealed that the bZIP domain displayed two types of architectures depending on the presence of an additional domain, suggesting that these architectures generate diversified structures and functions. Motif analyses indicated that the two types of bZIP genes had distinct sequences adjacent to the bZIP domain. Phylogenetic analyses suggested that the two types of bZIP genes distinctly evolved and ultimately adapted in different lineages. Transcriptome analyses in pepper (Capsicum annuum) and tomato (Solanum lycopersicum) revealed putative functional diversity between the two types of bZIP genes in response to various abiotic stresses. This study extensively updated bZIP gene family annotations and provided novel evolutionary and functional evidence for the role of bZIP genes in Solanaceae plants. Our findings provide evolutionary and functional characteristics of bZIP genes for a better understanding of their roles in Solanaceae plants.
Plants have evolved sophisticated mechanisms regulated through multiple gene families to maintain optimal growth under changing environments in response to a variety of stresses (Scharf et al., 2012). Transcription factors (TFs) belong to one of the regulator gene families that has a role in regulating transcription by attaching to the cis-element of a target gene promoter region (Mitchell and Tjian, 1989; Talanian et al., 1990). The bZIP TF gene family contains the bZIP DNA-binding domain, which contains 40–80 amino acids that include a basic region, a leucine zipper region, and an interconnecting hinge region (Landschulz et al., 1988; Perez-Rodriguez et al., 2010). The basic region is conserved, consists of 16–18 amino acids, and contains an invariant N-X7-R/K motif for binding to specific DNA sequences with an ACGT core, such as A-box (TACGTA), C-box (GACGTC), and G-box (CACGTG; Kouzarides and Ziff, 1989; Izawa et al., 1993; Deppmann et al., 2004). The leucine zipper region is composed of heptad repeats of leucine or other hydrophobic amino acids, which mediates the dimerization of bZIP protein (Landschulz et al., 1988; Ehlert et al., 2006; Llorca et al., 2015). The bZIP gene family has been identified and studied in plants including Arabidopsis thaliana (Jakoby et al., 2002), Oryza sativa (Nijhawan et al., 2008), Zea mays (Wei et al., 2012), Vitis vinifera (Liu et al., 2014b), and six species of legume (Wang et al., 2015). Functional studies of the bZIP gene family indicate that it has a key role in plant growth, development, and response to biotic/abiotic stresses (Uno et al., 2000; Baena-Gonzalez et al., 2007).
More than 3,000 plant species belong to the Solanaceae family, including many economically important crops such as Capsicum annuum and Solanum lycopersicum (Rigano et al., 2013). Capsicum annuum is a major ingredient in spicy cuisine and provides essential dietary vitamins and minerals (Kim et al., 2019b). Solanum lycopersicum contains many nutrients that promote human health, such as carotenoids and anthocyanins, and is widely used in genomic research because of its small genome size and short generation time (Rigano et al., 2013). Whole-genome sequencing of Solanaceae crops has been completed, thereby providing opportunities to explore the bZIP gene family in individual species of the Solanaceae family, such as S. lycopersicum, Solanum tuberosum, and C. annuum (Li et al., 2015; Gai et al., 2020; Herath and Verchot, 2020; Wang et al., 2021). Despite the availability of Solanaceae genomic resources, comparative genomics and transcriptomics analyses of bZIP genes in the Solanaceae family have not yet been performed.
In this study, we re-annotated and comparative analyzed bZIP genes in nine Solanaceae species along with A. thaliana. We identified 935 bZIP genes, including 107 (11%) updated genes, which were used in our further analysis. The overall structural features of bZIP genes identified two bZIP domain architectures in the Solanaceae family. Extensive motif analyses showed that the bZIP domains of the two types of bZIP genes contained distinct sequence compositions. Phylogenetic analysis indicated that bZIP genes were clustered into 14 subgroups, including 13 subgroups previously known in A. thaliana and 1 newly constructed subgroup with distinct domain architecture. Expression analyses incorporating gene ontology (GO) enrichment data suggested that bZIP genes have diverse functions in pepper (C. annuum) and tomato (S. lycopersicum) under abiotic stress conditions. Our study provided comprehensive information on the structure, expression, and functions of bZIP genes in Solanaceae. These results would be useful for future agricultural studies in Solanaceae crops.
To re-annotate the bZIP gene family in 10 plant species, we downloaded the genomic and transcriptomic data for the following plants: A. thaliana (Lamesch et al., 2012), Nicotiana benthamiana (Bombarely et al., 2012), Petunia inflata (Bombarely et al., 2016), C. annuum (Kim et al., 2014), Capsicum chinense (Kim et al., 2017), Capsicum baccatum (Kim et al., 2017), S. tuberosum (Pham et al., 2020), S. lycopersicum (Fernandez-Pozo et al., 2015), Solanum pennellii (Bolger et al., 2014), and Solanum pimpinellifolium (Wang et al., 2020; Supplementary Table 1). We used TGFam-Finder v1.20 for re-annotation of bZIP genes considering the parameters described previously (Kim et al., 2020). The TSV files including functional domain information were generated by InterProScan 5 (-f tsv-appl Pfam; Jones et al., 2014) and used as “TSV_FOR_DOMAIN_IDENTIFICATION.” The “TARGET_DOMAIN_ID” was set as “PF00170 (bZIP)” according to the Pfam database.1
We newly assigned gene names to re-annotated bZIP genes instead of using locus tag names from the published annotations. We matched gene names to previously annotated names if they had been assigned in previous research, as for bZIP genes in A. thaliana, C. annuum, S. lycopersicum, and S. tuberosum (Jakoby et al., 2002; Li et al., 2015; Gai et al., 2020; Herath and Verchot, 2020; Supplementary Table 2). New names also were assigned to the updated bZIP genes from the other species.
The domain architectures of the updated bZIP genes were analyzed using TSV files generated by InterProScan 5 (-f tsv-appl Pfam; Jones et al., 2014) according to the Pfam database.1 We defined integrated domains (IDs) if bZIP genes had other domain (s) in addition to the bZIP domain (PF00170). To acquire more precise information, we excluded domains with high e-value (>1e − 4) or those that overlapped the bZIP domain.
We extracted the bZIP domain sequences of the 10 plant genomes to determine the amino acid sequence compositions of the bZIP domains. We utilized MAFFT v7.470 (Katoh and Standley, 2013) to align the bZIP domain sequences, and then trimmed the alignment with TrimalAl v1.4 (-gt 0.5; Capella-Gutierrez et al., 2009). WebLogo v2.8.22 (Crooks et al., 2004) was used for the visualization of amino acid sequence composition. We divided the bZIP domain into five compartments based on signature residues. The conservation score of each compartment was determined as the average of the scores of residues within each compartment as calculated by CLC Sequence Viewer software v8.0.
We performed a GO analysis to analyze the putative function (s) of bZIP genes using OmicsBox v1.4.3 BLASTP was used to align bZIP protein sequences to the NCBI non-redundant protein database (nr v5) with e-value cut off 10−3. The results of InterProScan (Jones et al., 2014) results were integrated with the BLAST results. Next, we performed Blast2GO Mapping and Blast2GO Annotation with default parameters. The results of GO analysis were grouped into three categories (biological process, molecular function, and cellular component). We displayed the top five GO terms in the direct GO count of each category in the analysis.
To search conserved motifs of all protein sequences of bZIP genes, we used the MEME v5.1.1 (Bailey et al., 2006) program with the following parameters: -mod zoops, -nmotifs 50, -minw 10, -maxw 50, -objfun se, -markov_order 0. We used MAST v5.1.1 to match protein sequences to set of motifs (Bailey and Gribskov, 1998). We decided the position of conserved motifs manually using sequence alignments and motif compositions. To clarify the motif position of the top five gene structures, we excluded motifs that were repetitively placed at various motif sites.
We performed an enrichment test of motifs using Fisher’s test and Chi-square test functions from the Statistics::R module in R to check whether specific motifs were enriched in genes that contain a bZIP domain only or IDs. p-values were calculated by Monte Carlo test (B = 10,000). p-values < 0.0001 were regarded as highly important for the assured enrichment test.
Multiple sequence alignments of 935 re-annotated bZIP protein sequences were performed using MAFFT v7.470 (Katoh and Standley, 2013). TrimAL v1.4 was used to eliminate ambiguous alignments using the gt 0.5 trimming option (Capella-Gutierrez et al., 2009). The maximum-likelihood phylogenetic tree was generated using IQ-TREE v2.0.6 with JTT + R6 amino acid substitution model and 1,000 ultrafast bootstrap replicates (Minh et al., 2020). To visualize the mid-point rooted tree, we used Interactive Tree of Life (iToL) v6.4 The tree of bZIP genes was clustered into 14 subgroups (13 previously assigned subgroups and one unassigned subgroup) based on domain and motif structures. We named the unassigned clade as the St subgroup because most of the genes in the St subgroup contained the StAR-related lipid-transfer (START) domain.
We investigated the expression profiles of the bZIP gene in C. annuum and S. lycopersicum using C. annuum (Kang et al., 2020) and S. lycopersicum RNA-seq data in leaf under various abiotic stresses (SRR7652567, SRR7652566, SRR7652565, SRR7652564, SRR7652571, SRR7652570, SRR7652569, SRR7652568, SRR7652563, SRR15410554, SRR15410555, SRR15410556, SRR15410551, SRR15410552, SRR15410553, SRR15607561, SRR15607560, SRR15607558, SRR15607557, SRR15607556, and SRR15607555). RNA-seq data in C. annuum were generated under cold, heat, osmotic, and salt stress treatments at different time points (3, 6, 12, 24, and 72 h). RNA-seq data in S. lycopersicum were generated under cold, heat, drought, and salt stress treatments without reference to specific time points. All experiments were performed with three biological repeats. We trimmed the raw FASTQ files using CLC Assembly Cell (CLC Bio, Aarhus, Denmark) to eliminate low-quality data. Filtered data were mapped to the C. annuum and S. lycopersicum to reference genomes using HISAT2 (-dta-x; Kim et al., 2019a). We performed StringTie (-e-B-G; Pertea et al., 2015) to calculate fragment per kilobase of transcript per million mapped reads (FPKM) values of whole genes with the newly annotated bZIP genes in C. annuum and S. lycopersicum. FPKM values were converted to read counts using python scripts (prepDE.py). Differentially expressed genes (DEGs) were identified using DESeq2 in R software with the following criteria: log2FoldChange > 1 or < −1, and adjusted value of p <0.05 (Love et al., 2014).
We performed expressional clustering analysis of all C. annuum DEGs including bZIP DEGs. We grouped all C. annuum DEGs by expression patterns under all abiotic stresses at different time points using the Mfuzz program in R software (Kumar and Futschik, 2007). Four clusters were identified using the k-means algorithm. We also grouped all S. lycopersicum DEGs into upregulated or downregulated groups under each stress regime. Then, we conducted GO annotation in each cluster/group using OmicsBox v1.4.5 We examined the significance of the GO enrichment analysis using Fisher’s exact test (false discovery rate adjusted value of p ≤ 0.01) in each cluster/group.
We re-annotated the bZIP genes in nine Solanaceae species and A. thaliana to construct improved bZIP gene models. We identified 935 bZIP genes in 10 plant genomes (Table 1), and 107 (11%) of these genes were newly annotated. The number of bZIP genes per genome ranged from 69 (C. annuum) to 193 (N. benthamiana), and the number of newly annotated bZIP genes per genome ranged from 1 (S. tuberosum) to 32 (N. benthamiana). We examined domain architectures in the updated genes to explore the genome structure of bZIP genes in the 10 plant genomes. Our analysis revealed that bZIP genes primarily displayed two types of domain architectures: 645 bZIP genes (69%) contained only the bZIP domain (bZIP_only), and 290 bZIP genes (31%) contained additional integrated domains (bZIP_IDs; Figure 1A). The proportion of bZIP genes displaying these two architectures was similar in 8 of the species, whereas N. benthamiana and A. thaliana had slightly higher proportions of the bZIP_only architecture (74%; Supplementary Figure 1). Among bZIP genes displaying the bZIP_IDs architecture, 124 bZIP genes contained the DOG1 (PF14144) domain (42% of bZIP_IDs), which is involved in controlling seed dormancy (Figure 1B; Bentsink et al., 2006). Other less abundant bZIP_IDs domains included MFMR (PF07777), MFMR_assoc (PF16596), START (PF01852), MEKHLA (PF08670), and bZIP_C (PF12498; Figure 1B). These results provide the domain architecture repertoire of bZIP genes in the Solanaceae family and A. thaliana based on our updated annotations.
Figure 1. Characteristics of bZIP genes in Solanaceae. (A) The number of genes with the top five domain architecture repertoires is illustrated in the bar chart. Different domain architectures are shown on the left of the chart; domains are defined by different shaped symbols as defined below the chart. The percentages of the two types of bZIP genes are presented in the pie chart. (B) The bar chart shows the number of genes with integrated domains (top 10). Pfam IDs of the integrated domains are labeled next to the chart. (C) The amino acid sequence of the bZIP domain in 10 species. The height of the amino acid residue indicates the relative frequency of each amino acid at the specific position. The conservation scores of each compartment and residues are displayed as bar plots. The bar colors represent three regions of the bZIP domain: green, basic region; light blue, hinge region; and yellow, leucine zipper region. Red letters below the bar plots represent significantly conserved residues in bZIP genes. (D) Distribution of gene ontology (GO) terms of bZIP genes. The three GO categories are displayed on the right side of the heat map. The top five GO descriptions in each category are shown in the heat map. The colored scale at the bottom right side of the heat map represents the proportion of bZIP genes in each species.
We investigated the amino acid sequence composition of the bZIP domain in 10 plant species. The bZIP domain contains three motif regions (basic, hinge, and leucine zipper regions) that display N-X7-R/K, X9, and L-X6-L amino acid sequences, respectively (Droge-Laser et al., 2018). Our analysis shows that the bZIP domain in Solanaceae with A. thaliana was also clearly separated into three regions with those known signature residues of the bZIP domain covered by two conserved motifs (motif #2 and #1 in order; Figure 1C). This result supports the high accuracy of our updated annotation based on the known signature motifs and sequences in the bZIP domain. Comparative analysis of bZIP domains by species indicated that they were highly conserved (Supplementary Figure 2A), although bZIP domains significantly differed according to the major domain architectures (Supplementary Figure 2B). This result suggests that the distinct sequence compositions of bZIP domains with different domain architectures originated from the independent evolution of each bZIP domain architecture. We divided the bZIP domain into five compartments based on their highly conserved residues (N, R/K, L1, L2, and L3) and calculated the amino acid conservation score for those residues in each compartment (Figure 1C). The conservation scores of the highly conserved residues (N, R/K, L1, L2, and L3) and the second compartment (located between the N and R/K regions) were significantly higher (64%–90%) than those of the first, third, fourth, and fifth compartments (39%, 49%, 31%, and 34%, respectively). These results indicate that this compartment and residues were highly conserved in the Solanaceae family, which was consistent with previous reports (Li et al., 2015, 2021; Gai et al., 2020; Wang et al., 2021).
To characterize the putative function (s) of the updated bZIP genes, we performed GO analysis (Figure 1D). GO terms of 935 bZIP genes were classified into three categories: biological process, molecular function, and cellular component. The overall distributions of GO terms were similar for each species (Figure 1D). The most dominant GO terms in the three categories were “Regulation of transcription” (58%), “DNA-binding transcription factor activity” (95%), and “Nucleus” (56%), respectively. This suggests potential functions of updated bZIP genes as TFs in Solanaceae, as the bZIP gene family contained known TFs involved in plant development (Izawa et al., 1993). Taken together, our results showed that the updated annotation of bZIP genes enabled precise analyses of the domain structure, sequence composition, and putative function of bZIP genes in nine Solanaceae species and A. thaliana.
Motifs that exist outside of the bZIP domain enhance the structural and functional diversity of bZIP genes (Nijhawan et al., 2008; Wei et al., 2012). We surveyed the updated bZIP genes and identified 50 conserved motifs, excluding 20 genes that did not contain any conserved motifs (Supplementary Table 3). We verified that 35 of the 50 motifs were located in 17 specific positions (Figure 2), whereas 15 of the 50 motifs were located in various positions and were excluded from further analysis. These results suggest that bZIP genes in Solanaceae contained a variety of sequence motifs encompassing the bZIP domain, thereby increasing the sequence diversity of bZIP genes as described in previous reports (Nijhawan et al., 2008; Wei et al., 2012; Liu et al., 2014b). We found that other domains in bZIP_IDs (Figure 1A) were located between positions #5 and #6 (MFMR and MFMR_C) or positions #12 and #13 (DOG1, START, MEKHLA, and bZIP_C), suggesting that other motifs occupied conserved locations in bZIP_IDs (Figure 2).
Figure 2. Motif compositions of the top five domain architectures of bZIP genes. The numbers in the bars indicate motif labels as described in Supplementary Table 2. The types of integrated domains are listed below the ID box. The font sizes of the names of integrated domains represent the number of domains. Bar colors represent bZIP_only-enriched, bZIP_IDs-enriched, or neutral (p < 0.0001).
We performed enrichment tests of the 35 motifs in conserved locations to identify motifs that were abundant in bZIP_only or bZIP_IDs (Figure 2). We classified motifs into three groups: motifs enriched in bZIP_only, motifs enriched in bZIP_IDs, and motifs that were not enriched in any type of domain architecture. Our analyses showed that the motif compositions and locations of abundant motifs significantly differed in bZIP_only and bZIP_IDs. These results suggest that bZIP_only and bZIP_IDs distinctly evolved after the emergence of bZIP_IDs through domain integration into bZIP_only. Most of the enriched motifs in bZIP_only and bZIP_IDs were located upstream and downstream of the bZIP domain, respectively (Figure 2). This may indicate that bZIP_only primarily obtained specific sequences upstream of the bZIP domain, whereas bZIP_IDs gained specific downstream motifs through domain integration.
To explore the evolutionary relationships of Solanaceae bZIP genes (bZIP_only and bZIP_IDs), we constructed a phylogenetic tree using the updated bZIP genes in 10 species. We divided them into 14 subgroups, including 13 subgroups that were consistent with those described previously in A. thaliana (Droge-Laser et al., 2018) and 1 newly constructed subgroup (St) that was omitted in the previous phylogenetic tree (Li et al., 2015; Droge-Laser et al., 2018; Gai et al., 2020; Wang et al., 2021; Figure 3A). We found that bZIP_only and bZIP_IDs were distinctly clustered among the subgroups. The dominant bZIP_IDs were enriched in four subgroups: START domain in the St subgroup (81%), MFMR domain in subgroup G (92%), DOG1 domain in subgroup D (93%), and bZIP_C domain in subgroup C (74%). This suggests that those bZIP_IDs evolved through independent copy number expansion, and were finally adopted as individual lineages. We then examined the number of bZIP genes in each subgroup to verify the copy number variation of bZIP genes among different subgroups and among the same subgroups in different species (Figure 3B). The subgroup S containing 21% of the updated bZIP genes was the largest lineage, and I (11%), A (17%), and D (14%) were observed as dominant subgroups in order, suggesting copy number expansion of specific lineages. Including these subgroups, we found a similar proportion of bZIP genes in the 10 plant species belonging to the same subgroup (Figure 3B). This result suggests that the progenitor bZIP genes of Solanaceae emerged in a common ancestor of Solanaceae and Brassicaceae (Correa et al., 2008).
Figure 3. Phylogenetic relationships among bZIP genes and subgroup characteristics. (A) Phylogenetic tree of bZIP genes in the 10 plant species. Different species on the end of the branch are denoted by different colors. The colored branch and colored bars on the outer ring represent individual subgroups. Subgroup names are listed next to the outer rings representing integrated domains in the bracket. (B) The numbers of bZIP genes in subgroups by species are shown in a heatmap. Scale bars on the bottom right side indicate the number of genes. (C) The most abundant motifs adjacent to the bZIP domain are portrayed. Numbers in boxes indicate motif labels. The box colors represent the type of enriched motifs. The top five domain architecture repertoires are shown on the right side as different geometric patterns.
Previous studies reported distinct functions of bZIP genes depending on subgroup-specific motifs in A. thaliana (Choi et al., 2000; Lopez-Molina et al., 2001; Bensmihen et al., 2002). Subgroup A-specific motifs contain casein kinase II (CKII) phosphorylation sites of ABRE-binding factor (ABF) and ABA-responsive element-binding protein (AREB) genes. Both genes function as ABA-dependent TFs to control abiotic stress tolerance, which is a representative function of subgroup A. We investigated the motifs of bZIP genes in each subgroup, excluding motifs of the bZIP domain, to characterize sequences representing each subgroup (Figure 3C). The results showed that the ID-specific subgroups (subgroup C, D, G, and St) included bZIP_IDs-enriched motifs, whereas bZIP genes in other subgroups primarily containing bZIP_only had motifs enriched in bZIP_only or common motifs observed in bZIP_only and bZIP_IDs. Some motifs appeared in a specific group as subgroup-specific motifs; for example, motifs #36, #33, and #23 appeared specifically in subgroups A, I, and S, respectively (Figure 3C). These results and previous studies (Choi et al., 2000; Finkelstein and Lynch, 2000; Lopez-Molina et al., 2001; Bensmihen et al., 2002; Jakoby et al., 2002) suggest that distinct motifs enhanced the structural diversity and divergent functions of bZIP genes in each subgroup. Our analyses revealed the phylogenetic relationships and lineage-specific structural characteristics of bZIP genes in Solanaceae, which will facilitate future genetic and functional studies in agriculturally important crops.
The bZIP genes have crucial roles in response to abiotic stress (Hossain et al., 2010; Liu et al., 2014a). We conducted expression analyses of whole genes of pepper (C. annuum), including the newly identified bZIP genes, to examine the potential functions of bZIP genes under various abiotic stress conditions. The expression profiles were investigated under four stresses (cold, heat, mannitol, and salt) at five time points (3, 6, 12, 24, and 72 h). We detected DEGs under abiotic stresses compared with the untreated control: cold (10,718 DEGs), heat (9,990 DEGs), mannitol (3,548 DEGs), and salt (5,766 DEGs). We identified 29, 26, 12, and 20 bZIP DEGs in pepper in response to cold, heat, mannitol, and salt treatment, respectively (Supplementary Figure 3; Supplementary Table 4). The bZIP DEGs primarily belonged to specific subgroups as follows: A (11), D (10), E (8), G (6), and S (16). This indicates that bZIP genes in these subgroups may have roles in responding to abiotic stresses. Several functional bZIP genes in pepper, such as CabZIP25 (CANN_61) and CaBZ1 (CANN_67), were significantly upregulated under abiotic stress, which was consistent with previous studies (Moon et al., 2015; Gai et al., 2020) and validated the accuracy of our transcriptome analyses. We performed a temporal soft-clustering analysis of whole DEGs in pepper to investigate the expression patterns of bZIP and other genes in pepper (Figure 4A). DEGs were grouped into four distinct clusters (C1–C4) based on expression levels under each abiotic stress. We observed that the bZIP DEGs were abundant in C3 and C4 under cold stress, C1 and C3 under heat stress, C1 and C2 under mannitol stress, and C1 and C4 under salt stress (Figure 4B). This suggests that bZIP genes in these clusters were associated with specific functions under these abiotic stress conditions.
Figure 4. Transcriptome and gene ontology (GO) enrichment analyses of differentially expressed genes under four abiotic treatments in pepper (Capsicum annuum). (A) Expression patterns of whole pepper DEGs and bZIP DEGs during abiotic stress are displayed as four clusters for each abiotic stress. (B) Heatmap depicts the number of bZIP DEGs in each subgroup. The scale colors on the bottom right side of the heat map display the number of genes. (C) The top five GO terms enriched in each major cluster are displayed in dot plots. Shapes represent the GO categories; shape sizes represent the GO term frequencies.
We conducted GO enrichment analysis to predict the potential functions of bZIP genes in pepper using the genes in the selected eight clusters including abundant bZIP DEGs (Figure 4C). When we surveyed which GO terms were enriched in the eight clusters, a variety of functional descriptions were identified and many were distinctly observed in specific clusters (Figure 4C). For example, heat stress C1 contained GO terms in cellular component categories such as “cellular anatomical entity,” “organelle,” and “intracellular organelle.” By contrast, heat stress C3 contained GO terms in biological process categories such as “response to oxidative stress,” “response to temperature stimulus,” and “response to abiotic stimulus.” This result suggests that bZIP genes in pepper were associated with diverse functions under abiotic stresses, and their functions differed among the clusters. We investigated response-related GO terms in each cluster to verify specific functions in response to abiotic stress, as the previous study focused on response-related GO terms under abiotic stress conditions (Liu et al., 2015; Supplementary Figure 4). The repertoires of abiotic stress response-related GO terms were distinct from those of expression clusters. For example, salt stress C1 with abundant bZIP genes in subgroup A (bZIP_only-enriched subgroup) and salt stress C4 with abundant bZIP genes in subgroup D (DOG1-enriched subgroup) had different GO term repertoires such as “response to abscisic acid” and “response to stress,” respectively. CabZIP25 of pepper in subgroup A was highly expressed under salt stress and associated with ABA signaling (GO:0009737), thereby enhancing resistance to stress (Gai et al., 2020). The OsHBP1b of O. sativa in subgroup D promoted salt stress tolerance by altering activation of the ROS scavenging system, and had the child GO term of “response to stress” (GO:0006952; Lakra et al., 2015). The biotic stress-related GO terms (e.g., “response to biotic stimulus,” “response to external biotic stimulus,” and “response to other organisms”) were only enriched in salt stress C4, which was a typical function of bZIP genes in subgroup D (Alves et al., 2013; Fu and Dong, 2013; Droge-Laser et al., 2018). These results represent that bZIP_only and bZIP_IDs were functionally diverse according to the diverse bZIP gene architectures, which resulted from the integration of additional domains. Our analyses provided novel insights into bZIP gene expression and function in pepper, which will facilitate further studies.
We analyzed the expression profiles of whole genes in tomato (S. lycopersicum) along with the newly annotated bZIP genes to predict the function of tomato bZIP genes under abiotic stress. The gene expression profiles were examined using RNA-seq data under four stresses: cold, drought, heat, and salt. A total of 9,251 (cold), 1,174 (drought), 4,632 (heat), and 1,520 (salt) DEGs were identified. We identified 28, 15, 8, and 9 bZIP DEGs in tomato under cold, drought, heat, and salt conditions, respectively (Figure 5A; Supplementary Table 5). Subgroups A (11), D (8), E (7), G (9), and S (10) were observed as dominant subgroups with bZIP DEGs at least under one or more stress conditions, which were the same as those in pepper, suggesting that Solanaceae family members share common subgroups associated with abiotic stress responses. We verified that SlbZIP38 (SLYC_44) expression was downregulated under salt stress, which was consistent with the previous study and validated the accuracy of our expression analyses of tomato bZIP genes (Supplementary Figure 5; Pan et al., 2017). We examined the expression levels of bZIP and other genes in tomato under abiotic stress and classified DEGs as upregulated or downregulated (Figure 5B). The results showed that all bZIP DEGs in subgroup A were upregulated, consistent with previous studies reporting that they were highly expressed under abiotic stress to regulate ABA-dependent signaling (Yoshida et al., 2010; Gai et al., 2020).
Figure 5. Expression analyses and functional predictions of differentially expressed genes under different abiotic stresses in tomato (Solanum lycopersicum). (A) Volcano plot for tomato DEGs under different abiotic stresses. Scattered gray dots represent whole genes in tomato. Red and blue dots depict upregulated and downregulated bZIP DEGs based on 1-fold expression difference (represented by two black vertical lines). (B) Number of bZIP DEGs in each subgroup is displayed in a heatmap. Heatmap values on the bottom right side indicate the number of bZIP DEGs in each subgroup. (C) Dot plots depict the top five GO enrichment results for each group including bZIP genes. Shapes represent the GO categories; shape sizes represent the GO term frequencies.
Next, we conducted a GO enrichment analysis of bZIP gene functions in the eight groups containing bZIP DEGs in tomato (Figure 5C). We observed that several functional descriptions appeared in specific groups, similarly observed in pepper. For example, metabolism-related GO terms such as “metabolic process,” “organic substance metabolic process,” and “primary metabolic process” existed in five groups (cold-downregulated, cold-upregulated, drought-downregulated, drought-upregulated, and heat-downregulated), and binding-related GO terms were enriched in three groups (cold-upregulated, heat-upregulated, and salt-downregulated). These results suggest that bZIP genes were involved in diverse functions under abiotic stresses, and these functions were distinct among the groups. We also examined GO terms related to response and metabolism (GO:0050896 and GO:0008152, respectively) in each group (Supplementary Figure 6). The repertoires of enriched GO terms for response and metabolism were distinct among the groups. GO term repertoires specifically differed between two groups: cold-upregulated with abundant bZIP genes in subgroup G (MFMR-enriched subgroup), and salt-upregulated with many bZIP genes in subgroup A (bZIP_only-enriched subgroup). The cold-upregulated group included abundant metabolism-related GO descriptions, whereas the salt-upregulated group primarily contained response-related GO terms. We did not find functional bZIP genes related to those GO terms in subgroups G and A in Solanaceae genomes including tomato but did verify those functions of bZIP genes in other plant genomes. For example, GBF1 of Z. mays in subgroup G functioned in metabolism (GO:0006355) for cold stress tolerance by binding to a pseudo-palindromic cis-acting element called G-box region of alcohol dehydrogenase-1 (Devetten and Ferl, 1995; Shi et al., 2017). The A. thaliana AtbZIP37 in subgroup A was involved in ABA-dependent signaling under high salinity conditions (GO:0009651), thereby enhancing stress resistance (Yoshida et al., 2010). These results suggest that bZIP_only and bZIP_IDs had diverse functions under different abiotic stress conditions in tomato due to distinct domain architectures.
The bZIP genes are an essential transcription factor that regulates plant growth. We updated the annotation of bZIP genes and performed comprehensive comparative and functional analyses of the updated bZIP genes in nine Solanaceae genomes and A. thaliana. We divided bZIP genes into bZIP_only and bZIP_IDs depending on the domain architectures, and comparatively analyzed the two groups. Our data identified distinct motif compositions in bZIP_only and bZIP_IDs primarily due to the upstream (bZIP_only) or downstream (bZIP_IDs) locations of specific motifs relative to the bZIP domain. Based on the phylogenetic relationship, we found that bZIP_only and bZIP_IDs were clustered into distinct subgroups with unequal copy numbers among the subgroups. These results suggest that bZIP_only and bZIP_IDs underwent unequal copy number expansion in specific subgroups since their initial emergence from ancestral genes. Transcriptome analyses with GO enrichment analysis in pepper (C. annuum) and tomato (S. lycopersicum) revealed the potential functions of bZIP genes interacting with other genes under various abiotic stress conditions. Our data suggested functional diversity and distinct roles for bZIP_only and bZIP_IDs under four abiotic stress conditions. These results may be due to the distinct gene structures resulting from domain integration, which ultimately contributed to the functional diversification of bZIP genes.
Previous studies suggested that gene family analyses using advanced annotation methods could provide novel insights into the structural and functional characteristics of genes (Bayer et al., 2018; Chae et al., 2021; Lee et al., 2021; Guk et al., 2022). We also performed independent annotation updates and comparative analyses of bZIP genes in Solanaceae. In contrast to previous studies of bZIP genes, we focused on an extensive comparison of bZIP genes in Solanaceae to understand bZIP gene characteristics in the Solanaceae family rather than in individual species. Specifically, our data revealed comprehensive structural and expressional differences between two types of domain architectures: bZIP_only and bZIP_IDs, and suggested that those two architectures are involved in diverse functions under abiotic stress conditions. Taken together, our analyses provide novel insights into the structural, evolutionary, and functional features of bZIP genes in the Solanaceae family. These results will facilitate future research in plant bZIP genes.
The datasets presented in this study can be found in online repositories. The names of the repository/repositories and accession number (s) can be found in the article/Supplementary material.
SK designed the study. J-WC, H-EK, and SK performed the re-annotation and comparative analyses and edited and reviewed the final version. J-WC wrote the initial manuscript draft. All authors contributed to the article and approved the submitted version.
This study was supported by a National Research Foundation of Korea (NRF) grant funded by the Korean government (NRF-2022R1C1C1004918) to SK and the Korea Institute of Planning and Evaluation for Technology in Food, Agriculture, and Forestry (IPET) through the Digital Breeding Transformation Technology Development Program funded by Ministry of Agriculture, Food, and Rural Affairs (MAFRA; 322075–3) to SK.
The authors declare that the research was conducted in the absence of any commercial or financial relationships that could be construed as a potential conflict of interest.
All claims expressed in this article are solely those of the authors and do not necessarily represent those of their affiliated organizations, or those of the publisher, the editors and the reviewers. Any product that may be evaluated in this article, or claim that may be made by its manufacturer, is not guaranteed or endorsed by the publisher.
The Supplementary material for this article can be found online at: https://www.frontiersin.org/articles/10.3389/fpls.2022.967546/full#supplementary-material
IDs, Integrated domains; GO, Gene ontology; FPKM, Fragment per kilobase of transcript per million mapped reads; DEGs, Differentially expressed genes; FDR, False discovery rate.
Alves, M. S., Dadalto, S. P., Goncalves, A. B., De Souza, G. B., Barros, V. A., and Fietto, L. G. (2013). Plant bZIP transcription factors responsive to pathogens: a review. Int. J. Mol. Sci. 14, 7815–7828. doi: 10.3390/ijms14047815
Baena-Gonzalez, E., Rolland, F., Thevelein, J. M., and Sheen, J. (2007). A central integrator of transcription networks in plant stress and energy signalling. Nature 448, 938–942. doi: 10.1038/nature06069
Bailey, T. L., and Gribskov, M. (1998). Combining evidence using p-values: application to sequence homology searches. Bioinformatics 14, 48–54. doi: 10.1093/bioinformatics/14.1.48
Bailey, T. L., Williams, N., Misleh, C., and Li, W. W. (2006). MEME: discovering and analyzing DNA and protein sequence motifs. Nucleic Acids Res. 34, W369–W373. doi: 10.1093/nar/gkl198
Bayer, P. E., Edwards, D., and Batley, J. (2018). Bias in resistance gene prediction due to repeat masking. Nat. Plants 4, 762–765. doi: 10.1038/s41477-018-0264-0
Bensmihen, S., Rippa, S., Lambert, G., Jublot, D., Pautot, V., Granier, F., et al. (2002). The homologous ABI5 and EEL transcription factors function antagonistically to fine-tune gene expression during late embryogenesis. Plant Cell 14, 1391–1403. doi: 10.1105/tpc.000869
Bentsink, L., Jowett, J., Hanhart, C. J., and Koornneef, M. (2006). Cloning of DOG1, a quantitative trait locus controlling seed dormancy in Arabidopsis. Proc. Natl. Acad. Sci. 103, 17042–17047. doi: 10.1073/pnas.0607877103
Bolger, A., Scossa, F., Bolger, M. E., Lanz, C., Maumus, F., Tohge, T., et al. (2014). The genome of the stress-tolerant wild tomato species Solanum pennellii. Nat. Genet. 46:1034. doi: 10.1038/ng.3046
Bombarely, A., Moser, M., Amrad, A., Bao, M., Bapaume, L., Barry, C. S., et al. (2016). Insight into the evolution of the Solanaceae from the parental genomes of Petunia hybrida. Nat. Plants 2:2. doi: 10.1038/nplants.2016.74
Bombarely, A., Rosli, H. G., Vrebalov, J., Moffett, P., Mueller, L. A., and Martin, G. B. (2012). A draft genome sequence of Nicotiana benthamiana to enhance molecular plant-microbe biology research. Mol. Plant Microbe Interact. 25, 1523–1530. doi: 10.1094/MPMI-06-12-0148-TA
Capella-Gutierrez, S., Silla-Martinez, J. M., and Gabaldon, T. (2009). trimAl: a tool for automated alignment trimming in large-scale phylogenetic analyses. Bioinformatics 25, 1972–1973. doi: 10.1093/bioinformatics/btp348
Chae, G. Y., Hong, W. J., Jang, M. J., Jung, K. H., and Kim, S. (2021). Recurrent mutations promote widespread structural and functional divergence of MULE-derived genes in plants. Nucleic Acids Res. 49, 11765–11777. doi: 10.1093/nar/gkab932
Choi, H. I., Hong, J. H., Ha, J. O., Kang, J. Y., and Kim, S. Y. (2000). ABFs, a family of ABA-responsive element binding factors. J. Biol. Chem. 275, 1723–1730. doi: 10.1074/jbc.275.3.1723
Correa, L. G. G., Riano-Pachon, D. M., Schrago, C. G., Dos Santos, R. V., Mueller-Roeber, B., and Vincentz, M. (2008). The role of bZIP transcription factors in green plant evolution: adaptive features emerging from four founder genes. PLoS One 3:e2944. doi: 10.1371/journal.pone.0002944
Crooks, G. E., Hon, G., Chandonia, J. M., and Brenner, S. E. (2004). WebLogo: A sequence logo generator. Genome Res. 14, 1188–1190. doi: 10.1101/gr.849004
Deppmann, C. D., Acharya, A., Rishi, V., Wobbes, B., Smeekens, S., Taparowsky, E. J., et al. (2004). Dimerization specificity of all 67 B-ZIP motifs in Arabidopsis thaliana: a comparison to Homo sapiens B-ZIP motifs. Nucleic Acids Res. 32, 3435–3445. doi: 10.1093/nar/gkh653
Devetten, N. C., and Ferl, R. J. (1995). Characterization of a maize G-box binding-factor That is induced by hypoxia. Plant J. 7, 589–601. doi: 10.1046/j.1365-313X.1995.7040589.x
Droge-Laser, W., Snoek, B. L., Snel, B., and Weiste, C. (2018). The Arabidopsis bZIP transcription factor family - an update. Curr. Opin. Plant Biol. 45, 36–49. doi: 10.1016/j.pbi.2018.05.001
Ehlert, A., Weltmeier, F., Wang, X., Mayer, C. S., Smeekens, S., Vicente-Carbajosa, J., et al. (2006). Two-hybrid protein-protein interaction analysis in Arabidopsis protoplasts: establishment of a heterodimerization map of group C and group S bZIP transcription factors. Plant J. 46, 890–900. doi: 10.1111/j.1365-313X.2006.02731.x
Fernandez-Pozo, N., Menda, N., Edwards, J. D., Saha, S., Tecle, I. Y., Strickler, S. R., et al. (2015). The sol genomics network (SGN)-from genotype to phenotype to breeding. Nucleic Acids Res. 43, D1036–D1041. doi: 10.1093/nar/gku1195
Finkelstein, R. R., and Lynch, T. J. (2000). The Arabidopsis abscisic acid response gene ABI5 encodes a basic leucine zipper transcription factor. Plant Cell 12, 599–609. doi: 10.1105/tpc.12.4.599
Fu, Z. Q., and Dong, X. N. (2013). Systemic acquired resistance: turning local infection into global defense. Annu. Rev. Plant Biol. 64, 839–863. doi: 10.1146/annurev-arplant-042811-105606
Gai, W. X., Ma, X., Qiao, Y. M., Shi, B. H., Ul Haq, S., Li, Q. H., et al. (2020). Characterization of the bZIP transcription factor family in pepper (Capsicum annuum L.): CabZIP25 positively modulates the salt tolerance. Front. Plant Sci. 11:139. doi: 10.3389/fpls.2020.00139
Guk, J. Y., Jang, M. J., and Kim, S. (2022). Identification of novel PHD-finger genes in pepper by genomic re-annotation and comparative analyses. BMC Plant Biol. 22:206. doi: 10.1186/s12870-022-03580-2
Herath, V., and Verchot, J. (2020). Insight into the bzip gene family in Solanum tuberosum: genome and transcriptome analysis to understand the roles of gene diversification in spatiotemporal gene expression and function. Int. J. Mol. Sci. 22:253. doi: 10.3390/ijms22010253
Hossain, M. A., Lee, Y., Cho, J. I., Ahn, C. H., Lee, S. K., Jeon, J. S., et al. (2010). The bZIP transcription factor OsABF1 is an ABA responsive element binding factor that enhances abiotic stress signaling in rice. Plant Mol. Biol. 72, 557–566. doi: 10.1007/s11103-009-9592-9
Izawa, T., Foster, R., and Chua, N. H. (1993). Plant Bzip protein-DNA binding-specificity. J. Mol. Biol. 230, 1131–1144. doi: 10.1006/jmbi.1993.1230
Jakoby, M., Weisshaar, B., Droge-Laser, W., Vicente-Carbajosa, J., Tiedemann, J., Kroj, T., et al. (2002). bZIP transcription factors in Arabidopsis. Trends Plant Sci. 7, 106–111. doi: 10.1016/S1360-1385(01)02223-3
Jones, P., Binns, D., Chang, H. Y., Fraser, M., Li, W. Z., Mcanulla, C., et al. (2014). InterProScan 5: genome-scale protein function classification. Bioinformatics 30, 1236–1240. doi: 10.1093/bioinformatics/btu031
Kang, W. H., Sim, Y. M., Koo, N., Nam, J. Y., Lee, J., Kim, N., et al. (2020). Transcriptome profiling of abiotic responses to heat, cold, salt, and osmotic stress of Capsicum annuum L. Sci. Data 7:17. doi: 10.1038/s41597-020-0352-7
Katoh, K., and Standley, D. M. (2013). MAFFT multiple sequence alignment software version 7: improvements in performance and usability. Mol. Biol. Evol. 30, 772–780. doi: 10.1093/molbev/mst010
Kim, S., Cheong, K., Park, J., Kim, M. S., Kim, J., Seo, M. K., et al. (2020). TGFam-finder: a novel solution for target-gene family annotation in plants. New Phytol. 227, 1568–1581. doi: 10.1111/nph.16645
Kim, E. H., Lee, S. Y., Baek, D. Y., Park, S. Y., Lee, S. G., Ryu, T. H., et al. (2019b). A comparison of the nutrient composition and statistical profile in red pepper fruits (capsicums annuum L.) based on genetic and environmental factors. Appl. Biol. Chem. 62:48. doi: 10.1186/s13765-019-0456-y
Kim, D., Paggi, J. M., Park, C., Bennett, C., and Salzberg, S. L. (2019a). Graph-based genome alignment and genotyping with HISAT2 and HISAT-genotype. Nat. Biotechnol. 37:907. doi: 10.1038/s41587-019-0201-4
Kim, S., Park, M., Yeom, S. I., Kim, Y. M., Lee, J. M., Lee, H. A., et al. (2014). Genome sequence of the hot pepper provides insights into the evolution of pungency in capsicum species. Nat. Genet. 46:270. doi: 10.1038/ng.2877
Kim, S., Park, J., Yeom, S. I., Kim, Y. M., Seo, E., Kim, K. T., et al. (2017). New reference genome sequences of hot pepper reveal the massive evolution of plant disease-resistance genes by retroduplication. Genome Biol. 18:210. doi: 10.1186/s13059-017-1341-9
Kouzarides, T., and Ziff, E. (1989). Leucine zippers of Fos, Jun and Gcn4 dictate dimerization specificity and thereby control DNA-binding. Nature 340, 568–571. doi: 10.1038/340568a0
Kumar, L., and Futschik, M. E. (2007). Mfuzz: a software package for soft clustering of microarray data. Bioinformation 2, 5–7. doi: 10.6026/97320630002005
Lakra, N., Nutan, K. K., Das, P., Anwar, K., Singla-Pareek, S. L., and Pareek, A. (2015). A nuclear-localized histone-gene binding protein from rice (OsHBP1b) functions in salinity and drought stress tolerance by maintaining chlorophyll content and improving the antioxidant machinery. Plant Physiol. 176, 36–46. doi: 10.1016/j.jplph.2014.11.005
Lamesch, P., Berardini, T. Z., Li, D. H., Swarbreck, D., Wilks, C., Sasidharan, R., et al. (2012). The Arabidopsis information resource (TAIR): improved gene annotation and new tools. Nucleic Acids Res. 40, D1202–D1210. doi: 10.1093/nar/gkr1090
Landschulz, W. H., Johnson, P. F., and Mcknight, S. L. (1988). The Leucine zipper - a hypothetical structure common to a new class of DNA-binding proteins. Science 240, 1759–1764. doi: 10.1126/science.3289117
Lee, Y. M., Chae, G. Y., Kim, M. K., and Kim, S. (2021). Comparative analysis of re-annotated genes provides insight into evolutionary divergence and expressions of aquaporin family in pepper. Plants 10:10. doi: 10.3390/plants10061039
Li, Z. Y., Chao, J. T., Li, X. X., Li, G. B., Song, D., Guo, Y. F., et al. (2021). Systematic analysis of the bZIP family in tobacco and functional characterization of NtbZIP62 involvement in salt stress. Agronomy 11:148. doi: 10.3390/agronomy11010148
Li, D. Y., Fu, F. Y., Zhang, H. J., and Song, F. M. (2015). Genome-wide systematic characterization of the bZIP transcriptional factor family in tomato (Solanum lycopersicum L.). BMC Genomics 16:771. doi: 10.1186/s12864-015-1990-6
Liu, J. Y., Chen, N. N., Chen, F., Cai, B., Dal Santo, S., Tornielli, G. B., et al. (2014b). Genome-wide analysis and expression profile of the bZIP transcription factor gene family in grapevine (Vitis vinifera). BMC Genomics 15:281. doi: 10.1186/1471-2164-15-281
Liu, C. T., Mao, B. G., Ou, S. J., Wang, W., Liu, L. C., Wu, Y. B., et al. (2014a). OsbZIP71, a bZIP transcription factor, confers salinity and drought tolerance in rice. Plant Mol. Biol. 84, 19–36. doi: 10.1007/s11103-013-0115-3
Liu, Z. S., Xin, M. M., Qin, J. X., Peng, H. R., Ni, Z. F., Yao, Y. Y., et al. (2015). Temporal transcriptome profiling reveals expression partitioning of homeologous genes contributing to heat and drought acclimation in wheat (Triticum aestivum L.). BMC Plant Biol. 15:152. doi: 10.1186/s12870-015-0511-8
Llorca, C. M., Berendzen, K. W., Malik, W. A., Mahn, S., Piepho, H. P., and Zentgraf, U. (2015). The elucidation of the Interactome of 16 Arabidopsis bZIP factors reveals three independent functional networks. PLoS One 10:e0139884. doi: 10.1371/journal.pone.0139884
Lopez-Molina, L., Mongrand, S., and Chua, N. H. (2001). A postgermination developmental arrest checkpoint is mediated by abscisic acid and requires the AB15 transcription factor in Arabidopsis. Proc. Natl. Acad. Sci. 98, 4782–4787. doi: 10.1073/pnas.081594298
Love, M. I., Huber, W., and Anders, S. (2014). Moderated estimation of fold change and dispersion for RNA-seq data with DESeq2. Genome Biol. 15:550. doi: 10.1186/s13059-014-0550-8
Minh, B. Q., Schmidt, H. A., Chernomor, O., Schrempf, D., Woodhams, M. D., Von Haeseler, A., et al. (2020). IQ-TREE 2: new models and efficient methods for phylogenetic inference in the genomic era. Mol. Biol. Evol. 37, 1530–1534. doi: 10.1093/molbev/msaa015
Mitchell, P. J., and Tjian, R. (1989). Transcriptional regulation in mammalian-cells by sequence-specific DNA-binding proteins. Science 245, 371–378. doi: 10.1126/science.2667136
Moon, S. J., Han, S. Y., Kim, D. Y., Yoon, I. S., Shin, D., Byun, M. O., et al. (2015). Ectopic expression of a hot pepper bZIP-like transcription factor in potato enhances drought tolerance without decreasing tuber yield. Plant Mol. Biol. 89, 421–431. doi: 10.1007/s11103-015-0378-y
Nijhawan, A., Jain, M., Tyagi, A. K., and Khurana, J. P. (2008). Genomic survey and gene expression analysis of the basic leucine zipper transcription factor family in rice. Plant Physiol. 146, 333–350. doi: 10.1104/pp.107.112821
Pan, Y. L., Hu, X., Li, C. Y., Xu, X., Su, C. G., Li, J. H., et al. (2017). SlbZIP38, a Tomato bZIP Family Gene Downregulated by Abscisic Acid, Is a Negative Regulator of Drought and Salt Stress Tolerance. Genes 8:8. doi: 10.3390/genes8120402
Perez-Rodriguez, P., Riano-Pachon, D. M., Correa, L. G., Rensing, S. A., Kersten, B., and Mueller-Roeber, B. (2010). PlnTFDB: updated content and new features of the plant transcription factor database. Nucleic Acids Res. 38, D822–D827. doi: 10.1093/nar/gkp805
Pertea, M., Pertea, G. M., Antonescu, C. M., Chang, T. C., Mendell, J. T., and Salzberg, S. L. (2015). StringTie enables improved reconstruction of a transcriptome from RNA-seq reads. Nat. Biotechnol. 33:290. doi: 10.1038/nbt.3122
Pham, G. M., Hamilton, J. P., Wood, J. C., Burke, J. T., Zhao, H., Vaillancourt, B., et al. (2020). Construction of a chromosome-scale long-read reference genome assembly for potato. Gigascience 9:giaa100. doi: 10.1093/gigascience/giaa100
Rigano, M. M., De Guzman, G., Walmsley, A. M., Frusciante, L., and Barone, A. (2013). Production of pharmaceutical proteins in Solanaceae food crops. Int. J. Mol. Sci. 14, 2753–2773. doi: 10.3390/ijms14022753
Scharf, K. D., Berberich, T., Ebersberger, I., and Nover, L. (2012). The plant heat stress transcription factor (Hsf) family: structure, function and evolution. Biochim. Biophys. Acta 1819, 104–119. doi: 10.1016/j.bbagrm.2011.10.002
Shi, H. T., Liu, W., Yao, Y., Wei, Y. X., and Chan, Z. L. (2017). Alcohol dehydrogenase 1 (ADH1) confers both abiotic and biotic stress resistance in Arabidopsis. Plant Sci. 262, 24–31. doi: 10.1016/j.plantsci.2017.05.013
Talanian, R. V., Mcknight, C. J., and Kim, P. S. (1990). Sequence-specific DNA-binding by a short peptide dimer. Science 249, 769–771. doi: 10.1126/science.2389142
Uno, Y., Furihata, T., Abe, H., Yoshida, R., Shinozaki, K., and Yamaguchi-Shinozaki, K. (2000). Arabidopsis basic leucine zipper transcription factors involved in an abscisic acid-dependent signal transduction pathway under drought and high-salinity conditions. Proc. Natl. Acad. Sci. 97, 11632–11637. doi: 10.1073/pnas.190309197
Wang, Z. H., Cheng, K., Wan, L. Y., Yan, L. Y., Jiang, H. F., Liu, S. Y., et al. (2015). Genome-wide analysis of the basic leucine zipper (bZIP) transcription factor gene family in six legume genomes. BMC Genomics 16:1053. doi: 10.1186/s12864-015-2258-x
Wang, X., Gao, L., Jiao, C., Stravoravdis, S., Hosmani, P. S., Saha, S., et al. (2020). Genome of Solanum pimpinellifolium provides insights into structural variants during tomato breeding. Nat. Commun. 11:11. doi: 10.1038/s41467-020-19682-0
Wang, Q., Guo, C., Li, Z. Y., Sun, J. H., Wang, D., Xu, L. T., et al. (2021). Identification and analysis of bZIP family genes in potato and their potential roles in stress responses. Front. Plant Sci. 12:637343. doi: 10.3389/fpls.2021.637343
Wei, K. F., Chen, J., Wang, Y. M., Chen, Y. H., Chen, S. X., Lin, Y. N., et al. (2012). Genome-wide analysis of bZIP-encoding genes in maize. DNA Res. 19, 463–476. doi: 10.1093/dnares/dss026
Yoshida, T., Fujita, Y., Sayama, H., Kidokoro, S., Maruyama, K., Mizoi, J., et al. (2010). AREB1, AREB2, and ABF3 are master transcription factors that cooperatively regulate ABRE-dependent ABA signaling involved in drought stress tolerance and require ABA for full activation. Plant J. 61, 672–685. doi: 10.1111/j.1365-313X.2009.04092.x
Keywords: bZIP, transcription factors, Solanaceae, abiotic stress, integrated domain, re-annotation
Citation: Choi J-W, Kim H-E and Kim S (2022) Two different domain architectures generate structural and functional diversity among bZIP genes in the Solanaceae family. Front. Plant Sci. 13:967546. doi: 10.3389/fpls.2022.967546
Received: 13 June 2022; Accepted: 01 August 2022;
Published: 19 August 2022.
Edited by:
Christos Bazakos, Max Planck Institute for Plant Breeding Research, GermanyReviewed by:
Venura Herath, University of Peradeniya, Sri LankaCopyright © 2022 Choi, Kim and Kim. This is an open-access article distributed under the terms of the Creative Commons Attribution License (CC BY). The use, distribution or reproduction in other forums is permitted, provided the original author(s) and the copyright owner(s) are credited and that the original publication in this journal is cited, in accordance with accepted academic practice. No use, distribution or reproduction is permitted which does not comply with these terms.
*Correspondence: Seungill Kim, a3NpMjIwNEB1b3MuYWMua3I=
Disclaimer: All claims expressed in this article are solely those of the authors and do not necessarily represent those of their affiliated organizations, or those of the publisher, the editors and the reviewers. Any product that may be evaluated in this article or claim that may be made by its manufacturer is not guaranteed or endorsed by the publisher.
Research integrity at Frontiers
Learn more about the work of our research integrity team to safeguard the quality of each article we publish.