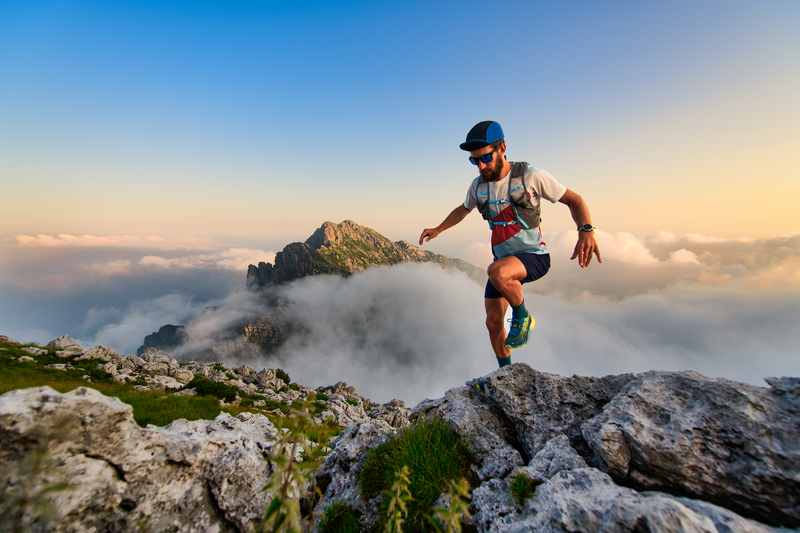
95% of researchers rate our articles as excellent or good
Learn more about the work of our research integrity team to safeguard the quality of each article we publish.
Find out more
REVIEW article
Front. Plant Sci. , 29 August 2022
Sec. Plant Development and EvoDevo
Volume 13 - 2022 | https://doi.org/10.3389/fpls.2022.965000
This article is part of the Research Topic Fruit and Seed Evolution in Angiosperms View all 7 articles
The seed is an evolutionary innovation in the plant kingdom. While human civilization depends heavily on seed production, how the seed trait emerged remains elusive. In this opinion article, a “golden-trio hypothesis” is proposed based on our investigations of LEC1 gene functions in Adiantum capillus-veneris. This hypothesis posits that a “seed program” arose from spatiotemporal integration of three key components: assimilate flow, ABA-mediated stress responses, and stress-induced LEC1 expression. Thus, the evolutionary innovation of seeds should be considered not a simple event resulting from new genes; rather, it represents the outcome of a series of physiological and morphological innovations that emerged prior to and regardless of the origin of the seed program. This new perspective could help us tackle some long-standing questions around the puzzling origin of seeds.
Twenty years ago, one afternoon in early summer, two of the authors of this article discussed evolutionary developmental biology (“evo-devo”) in the corridor linking the main building housing the College of Life Sciences and its annex. One of them was vacationing in Beijing before an upcoming journey to the United Kingdom as a visiting scholar, following a previous collaboration with his colleagues at Australia National University. During this time, evo-devo was starting to become popular in plant biology. In this conversation, they reasoned that committing to such research meant focusing on traits that represent key innovations, such as vascular tissue, seeds, and flowers, in the plant kingdom. Among such key innovations, they preferred to study the origins of seeds. The rationale was simple, that in Arabidopsis, knocking out LEAFY COTYLEDON1 (LEC1) resulted in skipping a “seed program” characterized by assimilate accumulation, desiccation, and dormancy along with the embryo development (Meinke et al., 1994; West et al., 1994; Lotan et al., 1998; Harada, 2001). The lec1 mutant phenotype somehow resembles what is observed in ferns, in which early embryo development continues from zygote division to seedling growth. While one of the authors talked about it for fun, the other took the idea more seriously. Before his departure to the United Kingdom, he asked a graduate student in his lab to perform a sequence comparison among LEC1-related genes from green algae to land plants. This was how the story detailed here started.
A seed is a morphologically complicated structure. It consists of an embryo (together with a distinguishable endosperm in some angiosperms), which is a multicellular structure derived from the newly formed zygote, and a seed coat, a sporophytic tissue derived from the integuments of a maternal ovule. The seed is a hallmark structure that distinguishes spermaphytes (including gymnosperms and angiosperms) from pteridophytes (including ferns and lycophytes) and bryophytes.
Since seeds are a major food resource, the control of seed size and assimilate accumulation have been intensively investigated, as have post-harvest methods for seed storage that maintain freshness and prevent sprouting. The studies on seed development, mostly with angiosperms as the experimental system, can be roughly categorized into two groups: morphological characterization and physiological analysis. Before the 1980s, physiological studies on seed development mainly focused on the analysis of nutritional composition, assimilate accumulation during embryogenesis, and conditions affecting seed dormancy, among others (Harada, 1997). Once forward genetics gained popularity, especially using Arabidopsis as a model, many genes involved in seed development were identified through mutant screens (Koornneef et al., 1984; Koornneef and Meinke, 2010). Given that a “seed” is the final outcome of developmental processes for sporophytic tissues (e.g., megasporangium and integuments of the ovule in Arabidopsis), gametophytic tissues (e.g., megaspore and gametophyte, including embryo sac differentiations, which are enclosed in the megasporangium), and the new generation of sporophytic tissues (via fertilization and embryogenesis), genes identified as being involved in those processes specifically have not been considered “seed genes.” Thus, only genes affecting differentiation steps occurring after the formation of seed-related structures, e.g., integuments and embryos, have been considered seed genes. Among these identified seed genes, in addition to those affecting embryogenesis, endosperm differentiation, and seed coat differentiation, there was a group affecting assimilate accumulation, desiccation, and dormancy, i.e., the biochemical and/or physiological processes that accompany embryo and seed coat morphogenesis. Collectively, these three processes (assimilate accumulation, desiccation, and dormancy) compose seed maturation or what has been called the “seed program” (Wobus and Weber, 1999; Figure 1). LEC1 plays an important role in the processes underlying the seed program (Harada, 2001).
Figure 1. A diagram illustrating the meaning of the “seed program” in the context of the angiosperm life cycle and mainstream study of seed development. Different stages of the angiosperm life cycle are assigned as different research topics, such as vegetative, flower, and seed development, with some overlaps. The sporophyte of the plant, derived from a zygote, is labeled in dark green; the gametophyte is pale green; and the sporophyte of the new generation enclosed within the maternal sporophyte is bright green. The assimilate flow is labeled.
According to phylogenetic analysis of photoautotrophic organisms, the seed is a relatively new trait that emerged around 200 million years ago (Herr, 1995; Taylor et al., 2009), at a time when non-seed plants such as bryophytes and pteridophytes had already occupied land for over 300 million years. Seed origin is therefore a long-standing question to plant biologists: When, from where, and how did the seed trait emerge?
There are diversified hypotheses and terminologies around the origin of seeds—traditionally referred to as the origin of the “seed habit”—based on morphological comparisons and fossil investigations. According to the widely accepted “retention theory” (Herr, 1995; Taylor et al., 2009; Figure 2), a seed is derived from the retention of a megasporangium that differentiated at the tip of a “telome” (a general term describing the diversified shape of branches). Externally, the retained megasporangium is enclosed by surrounding structures that emerged from the telome prior to the megasporangium (Figures 2A–D). These surrounding structures are designated as integuments of the ovule at its early developmental stages and later as the seed coat. Internally, the retained megasporangium can continuously receive nutrition from the rest of the sporophyte (Figure 2E).
Figure 2. A diagram of two events closely related to the “retention” theory regarding seed origin. (A–D) Putative process of integument origin during evolution according to the “telome theory.” (A) Oval-shaped megasporangia are located at the tips of the telomes/branches. (B,C) Several telomes/branches were coopted as surrounding tissues to enclose the dominant/fertile megasporangium. (D) The surrounding tissues merged into a structure designated as the integument (often consisting of two layers in angiosperms; modified from Taylor et al., 2009). (E) Illustration of a stalked ovule of Callospermarion. “m” refers to a megaspore in the megasporangium (indicated by the dotted region) enclosed by the integument with vascular bundles represented by the black strips (modified from Herr, 1995).
In contrast to non-seed plants, e.g., pteridophytes and bryophytes, differentiation of the non-photoautotrophic gametophytes (the haploid multicellular structures derived from spores) in seed plants was physically compressed within the sporangia, for both mega- and micro-sporangia. Within the megasporangium, the egg formed, was fertilized, and then differentiated from a zygote to an embryo. The embryo received its nutritional supply from the sporophytes where the telomes/branches differentiated, rather than from the gametophytes as in bryophytes and pteridophytes.
While it remains elusive how the different parts, such as megasporangium and embryo were integrated into one unit recognized as a seed, it seems that all these parts have counterparts in non-seed plants, based on comparative morphological and paleobotanical studies. However, no clues can be found from such studies on what caused megasporangium retention at the tip of the telome/branch in the first place, how the haploid gametophyte compressed within the megasporangium acquired its nutritional supply from the diploid sporophyte, and under what conditions the enclosed megasporangium together with the embryo detached or abscised from the telome/branch. From this perspective, it is clear that to solve the mystery of seed origin, one must explore new investigation strategies, in addition to those developed in traditional comparative morphology and paleobotany.
The lec1 mutant was first reported in 1992 as a homeotic mutant with cotyledons resembling true leaves (Meinke, 1992). However, the function of the LEC1 gene was soon interpreted as an “embryonic fate” determinant because its overexpression induced somatic embryogenesis rather than seedling formation during tissue culture (Lotan et al., 1998). Alternatively, John Harada has proposed a more sophisticated explanation for the lec1 mutant phenotype (personal communication): If indeed there is a seed maturation program (henceforth referred to as the “seed program”) that encompasses assimilate accumulation, desiccation, and dormancy, there must be a regulatory mechanism to initiate the program and coordinate the associated complex biochemical and physiological processes. Since all processes in the seed program were abnormal in the lec1 mutant, the simplest explanation is that the LEC1 gene plays a key role in initiating and coordinating the seed program (Figure 3). Due to loss of LEC1 function, the seed program cannot be properly turned on and coordinated, resulting in defective assimilate accumulation, desiccation, and dormancy, although embryo morphogenesis remains essentially normal, i.e., there is a functional embryotic shoot and root (Meinke et al., 1994; West et al., 1994). Derepression of the seed program during morphogenesis in lec1 allows primordia committed to the cotyledon in the wild type to differentiate into true leaves with trichomes on the epidermis—the characteristic phenotype of the lec1 mutant that was the inspiration for its name (Meinke, 1992; Lotan et al., 1998).
Figure 3. Cycle of seed maturation and germination in higher plants. Relationship between morphogenesis/embryonic development and seed maturation, suggesting that seed maturation interrupts the morphogenesis process (figure courtesy of J. Harada; modified with the addition of “LEC1” to the left turning point).
While many plant geneticists study angiosperms, two of the authors of this article work in the field of plant evolution. They are quite familiar with the life cycles of both seed and non-seed plants and of their differences. Taking this training background into account, it is understandable that while discussing the lec1 phenotype, they compared the embryogenetic processes of the lec1 mutant to that of ferns, in which morphogenesis continues without desiccation and dormancy (Figure 4). There are substantial differences in embryogenesis in angiosperms and pteridophytes: In the former, the embryogenesis occurs in the embryo sac embedded in the sporophytic ovules; and in the latter, the embryogenesis occurs in the archegonia of photoautotrophic gametophytes independent of sporophytes. However, it was exciting to hypothesize that the seed program originated due to the LEC1 gene, if LEC1 is indeed the key regulator of the seed program. Was this hypothesis experimentally testable? As mentioned above, after their chat in the corridor 20 years ago, one of the authors requested a sequence comparison of LEC1-related genes from green algae to land plants.
Figure 4. A comparison of early development, from zygote to seedling, between angiosperms and ferns. Angiosperms and ferns are represented by Arabidopsis and Adiantum capillus-veneris, respectively. The phenotype of the lec1 mutant during the embryogenesis resembles that of Adiantum capillus-veneris during early development. For a detailed morphological description of Adiantum capillus-veneris, see Li et al. (2013).
The genome sequence comparison revealed that LEC1 belongs to the HAP3 superfamily, together with its closely related non-LEC1 linage (Yang et al., 2005; Figure 5A). While no LEC1-like sequences were found the genomes of algae and bryophytes, LEC1-like sequences were present in the lycophyte Selaginella (highlighted in Figure 5A), as well as in other lycophyte and fern genomes (Xie et al., 2008). Gene expression and genetic complementation analyses excluded the possibility that the LEC1-like sequences identified in the pteridophytes are pseudogenes (Figure 5B) and demonstrated that the LEC1-like sequences are expressed under stress conditions (Figure 5C; Fang et al., 2017; Li et al., 2017).
Figure 5. LEC1-like gene sequence identification, functional complementation, and stress-inducible expression in lycophytes. (A) Phylogeny of HAP3 proteins from different plants, in which the LEC1-type HAP3 (LEC1-like) proteins were identified in the branch labeled “b” (the protein sequences with the prefix “Sm” are from Selaginella moellendorffii and are highlighted by a red rectangle). (B) SsLEC1 (LEC1-like from Seleginella sinensis) can complement the lec1 mutant phenotype in Arabidopsis. (C) Drought-induced expression of the LEC1-like genes in Selaginella sinensis (a) and Adiantum capillus-veneris (b; modified from Xie et al., 2008).
These experimental data led to a perplexing situation: On the one hand, LEC1-like genes were relatively new in the context of land plant phylogeny, as they did not exist before pteridophytes. On the other hand, the original function of LEC1-like genes was unlikely related to a seed program, as the seed trait is absent in Selaginella and Adiantum. However, the LEC1-like genes are expressed during morphogenetic processes of Selaginella and Adiantum and functionally complement Arabidopsis LEC1. If the function of LEC1 was interpreted as a key regulator in the seed program, as most previous studies have claimed, such an interpretation obviously is not applicable to that of its similar sequences found in the pteridophytes. If the function of the LEC1-like gene in pteridophytes is regarded as ancestral, it becomes necessary to explain how the gene was coopted into the seed program, leading to the emergence of the spermatophytes and functioning as a key regulator of the seed program.
While the experimental data disproved a causal relationship between the origin of LEC1 and the origin of the seed program, they generated a new opportunity to solve the seed origin mystery from the perspective of the ancestral function of stress-responsive LEC1-like genes in pteridophytes.
Two important facts about the LEC1-like genes were discovered (Xie et al., 2008): First, the LEC1-like genes emerged in the pteridophytes, prior to the spermatophytes; and second, the LEC1-like genes are induced by stress during pteridophyte morphogenesis. These new facts, together with the known fact that LEC1 is a key regulator of the seed program in Arabidopsis, support the hypothesis that the function of LEC1 as a seed program regulator was derived from the ancestral function of stress-responsive LEC1-like genes in pteridophytes. Now, another question arises: Why did not the expression of LEC1-like genes in the pteridophytes trigger a seed program, even though these sequences were capable of restoring the seed program in the Arabidopsis lec1 mutant?
There are two possible answers to the above question. First, it is possible that the LEC1-like-regulated genes required to organize the seed program regulatory network were absent in the pteridophytes. Second, it is possible that the proper conditions required to induce LEC1-like gene expression were not met during gametophyte development in pteridophytes despite the presence of the LEC1-like-regulated genes in the genome, and therefore no seed program could be activated.
The first possibility could be easily tested by genome comparison if the genome sequences of pteridophytes were available or through experimental analysis. Current information suggests that a lack of downstream genes is less likely the reason for no seed program in pteridophytes (Han et al., 2017; Li et al., 2017). As for the second possibility, while stress-induced expression of LEC1-like genes was found during sporophytic development in both Selaginella and Adiantum, none was detected during gametophytic development (Fang et al., 2017). As gametophytic development is highly sensitive to dehydration and ABA application, it is necessary to find other conditions to test whether LEC1-like genes can be expressed during gametophytic development.
In addition to dehydration and ABA application, hexose sugars were reported to induce LEC1 gene expression during seed development (Tsukagoshi et al., 2007). This finding not only provides a new agent to be examined for its effect on LEC1-like gene expression in pteridophyte gametophytic development, but more significantly, hints to a possible scenario for how the LEC1 gene was coopted as a key regulator of a future seed program, which will be further elaborated below.
As mentioned previously, seeds are the key structures distinguishing spermatophytes from the pteridophytes and bryophytes. While all land plants possess two multicellular structures, diploid sporophytes and haploid gametophytes (Smith et al., 2010; Bai, 2015), the gametophytes of spermatophytes are compressed in megasporangia, which are “retained” at the tip of the telomes/branches where the megasporangia differentiate. It remains elusive how the megasporangia were retained at the tip of the telomes/branches and why the megaspores were not released, after which gametophytic development and embryogenesis continue within the retained megasporangia. However, two facts were unambiguously observed. First, the retained megasporangia were all located at the extremity of the vascular system. This fact suggests that the retained megasporangia enclosed by the integuments are a sink for assimilate flow (Herr, 1995; Taiz et al., 2014). The second fact is that before the floral structure emerged, megasporangia were mostly aerial. Even though megasporangia were also enclosed by integuments in gymnosperms, the combined entities, called ovules, were also aerial (Taylor et al., 2009; Bai, 2017, 2019). This fact suggests that a mechanism to cope with dehydration would have likely evolved during the differentiation of megasporangia.
Taking the above two facts into consideration, a possible scenario emerges: Regardless of how the megasporangia were retained at the tip of telomes/branches and why the megaspores were not released, gametophytic development and embryogenesis continued inside of the megasporangia, which became a sink for assimilate flow originating from the sporophytes. If the megasporangia were indeed a sink, together with the dehydration-proof mechanism (probably mediated with ABA as revealed from numerous studies in angiosperms, Finkelstein and Gibson, 2002; Weber et al., 2005), it is highly possible that LEC1-like gene expression in pteridophytes could be induced by assimilates containing hexoses as well as by the dehydration-proof mechanism (likely mediated by ABA). If the molecular mechanism of LEC1-mediated seed program regulation is common amongst Arabidopsis and other angiosperms, it is difficult to reject the possibility that the induced expression of LEC1-like genes could trigger a similar seed program during the differentiation of megasporangia in pteridophytes or specifically some ancestors of spermatophytes. If the integration of these three components (i.e., assimilate flow, an ABA-mediated dehydration-proof mechanism, and inducible expression of LEC1-like genes) indeed occasionally occurred within the retained megasporangia (possibly already been enclosed by the surrounding structures), perhaps a seed program was eventually established during the evolution of land plants. We describe this scenario for the origin and activation of a seed program in land plants as the “golden-trio hypothesis” (Figure 6).
Figure 6. Diagram of the “golden-trio hypothesis” on the origin of the “seed program.” The “golden trio” refers to three indispensable components for seed program: assimilate flow, ABA-mediated dehydration-proof mechanism, and inductive expression of LEC1-like genes. Interactions among the three components, together with other factors, triggered the seed program overlapping with embryonic development, resulting in emergence of the seed trait.
In the scenario described above, assimilate flow and the ABA-mediated dehydration-proof mechanism were likely indispensable for inducing LEC1-like gene expression to activate the seed program. Given that gametophytic development in pteridophytes is highly sensitive to ABA, at least one of the components required for the activation of the seed program must be lacking in these plants. In addition, gametophytes that develop in lycophytes such as Selaginella are very limited in size and barely self-sustainable (Schulz et al., 2010), and those in ferns such as Adiantum capillus-veneris lack conducting tissue, although the prothallus is self-sustainable. Thus, there is little possibility of extra assimilate accumulation during embryogenesis as storage materials. From this perspective, it is easy to explain why there are LEC1-like genes but no seed program in pteridophytes: The conditions required to induce the expression of LEC1-like genes during gametophytic development were not met.
If the above reasoning stands, one might ask whether artificially applying assimilates during gametophyte development in pteridophytes would induce the expression of the LEC1-like genes and activate a seed program. To answer this question, a new experimental system must be established to meet two criteria: First, gametophytic development in this system must be easy to manipulate under laboratory conditions. Second, it must be possible to effectively assess the biochemical and physiological processes involved in the seed program of the cultivated gametophytes.
Fortunately, Adiantum capillus-veneris was demonstrated as a suitable species for establishing the experimental system (Li et al., 2013). Not only could its gametophytic development be manipulated under laboratory conditions, but its representative gene expression and metabolite accumulation were also possible to analyze during gametophytic development. Using such an experimental system, Fang et al. (2017) showed that sugar treatments can induce Adiantum capillus-veneris LEAFY COTYLEDON1 (AcLEC1) expression and trigger the accumulation of storage products during prothallus (gametophyte) development. While it remains to be determined whether the sugar treatments directly caused the increase in AcLEC1 expression and the accumulation of storage products, these findings provided the first supporting evidence for the golden-trio hypothesis.
While a clear conclusion about seed origin has not yet been reached, studies of pteridophytes have expanded our understanding of the function of LEC1 and LEC1-like genes, particularly their roles in the seed program. These findings also introduced a new perspective on how to approach the questions surrounding seed origin, in addition to traditional comparative morphology and paleobotany.
How could the new findings from the studies on LEC1-like genes in pteridophytes shed light on our understanding of seed origin? While studies demonstrated the existence of functional LEC1-like genes in pteridophytes, their inducible expression during gametophyte development and the existence of LEC1-downstream genes in Adiantum capillus-veneris strongly suggested that seed origin is not an evolutionary innovation that was directly switched on by de novo gene birth. There were at least two innovations for the seed trait to ultimately emerge: One is the retained megasporangia development, and the other is the interwoven biochemical and physiological processes designated as the seed program. For the latter, the findings from pteridophyte studies suggest that while some genes such as LEC1-like are key regulators of the seed program, other pre-existing components are indispensable for the expression of LEC1-like genes during gametophyte development. In short, the emergence of the seed trait as a key evolutionary innovation most likely resulted from complicated interactions and the integration of pre-existing components.
A recently published work provides strong support for that hypothesis; based on the genome sequence and transcriptome analysis of Adiantum capillus-veneris, Fang et al. (2022) determined that AcdLEC1 (previously designated as AcLEC1) is not only expressed during homosporangium development, but also resides at a hub of a regulatory network that is conserved in the Arabidopsis seed program. The work further elucidated the function of AcdLEC1 in pteridophytes, which consistent to the previous finding that the AcdLEC1 expressed in sporophytes under stress conditions (Xie et al., 2008). The similarity of regulatory network between the Adiantum capillus-veneris homosporangium development and Arabidopsis seed program provides a new evidence to what was predicted in the golden-trio hypothesis for origin of seed program.
Integrating the above-mentioned findings from the study of pteridophytes, along with our knowledge on the function of LEC1 genes and the comparative morphology/paleobotany of seed origin, we developed the golden-trio hypothesis to explain how stress-inducible LEC1-like genes were coopted during gametophytic development to become key regulators of the so-called “seed program.” In this hypothesis, retention of megasporangia development at the tip of the telomes/branches was indispensable for seed program emergence. Under such circumstances, assimilate flow and the ABA-mediated dehydration-proof mechanism were present simultaneously and could induce the expression of the LEC1-like genes in developing megasporangia as well as in gametophytes/embryos compressed within the retained megasporangia. Figure 7 compares the life cycle of bryophytes (a), pteridophytes (including lycophytes and ferns; b, c), and spermatophytes (including gymnosperms and angiosperms; d, e). This diagram illustrates the emergence of key components related to seed origin with respect to the phylogeny of land plants.
Figure 7. Life cycle comparison among five major land plant groups and key evolutionary innovations. The five major land plant groups are bryophytes, lycophytes, ferns, gymnosperms, and angiosperms, labeled from A–E, respectively. While the successful development of a seed plant requires integration of multiple evolutionary innovations, these innovations may emerge independently, regardless of seed origin (labeled in round-cornered rectangular, compares to Figure 1).
Traditionally, many evolutionary innovations are included under the topic of “seed origin,” or “origin of seed habit,” such as heterospory, retention of megasporangium, origin of integuments, and even pollen. However, based on the findings from studies on LEC1-like gene function, it seems that the innovations recognized as indispensable for seed origin might not have emerged exclusively for the origin of the seed trait or “seed habit.” Rather, these innovations originally emerged regardless of seed origin and then interacted by chance with other innovations. Among those chance interactions remained a few with adaptive advantages, which were designated as “seed plants.” Wang and Bai (2019) have proposed a possible scenario regarding the transition from homospory to heterospory. Similar adaptive advantages of this transition might underlie the retention of the megasporangia in sporophyte development, which ultimately led to the emergence of the spermatophytes. Based on the conservation of LEC1-centered regulatory networks between Adiantum capillus-veneris homosporangium development and the Arabidopsis seed program (Fang et al., 2022), such LEC1-centered regulatory networks found in Adiantum capillus-veneris might have been available for integration with other evolutionary innovations, resulting in the origin of seeds, which would represent the kind of “tinkering” event suggested by Jacob (1977).
Twenty years have passed since the original idea to explore the role of LEC1 in seed origin. Although the first hypothesis on the relationship between LEC1 and seed origin was proven to be oversimplified, it in principle fell within the scope of classic evo-devo. One lesson should be learned from the study of LEC1-like genes in pteridophytes: There may be a disconnect between the origin of traits and the genes responsible for these traits. Traditionally, genes and their functions were designated by mutant phenotype analysis in model organisms. When a gene was demonstrated as indispensable to a trait, it was inferred that the trait was derived from the origin of that gene. However, with more genomic information available, an increasing number of examples indicate that genes tend to exist prior to the origin of the traits they regulate. These examples include not only the LEC1-like genes, but also genes previously annotated as nerve-specific in sponges that lack a nervous system (Musser et al., 2021). These examples suggest that the original functions of genes might not be what is inferred based on the mutant phenotype in model organisms. Evo-devo researchers should keep in mind that there can be a disconnect between genes and traits pertaining to evolutionary innovation. In conclusion, the findings of the inducible LEC1-like gene expression and assimilate accumulation in Adiantum capillus-veneris gametophyte development provide a new opportunity to explore the mechanisms of seed origin.
All authors listed have made a substantial, direct, and intellectual contribution to the work and approved it for publication.
The studies on the LEC1-like genes were supported by National Natural Science Foundation of China grant no. 30370092 to JY and grant no. 91231105 to G-YR.
We thank Jing-Chu Luo of Peking University for his help in bioinformatics analysis, Zeng-Yan Xie, Xia Li, Yu-Han Fang, Jing-Dan Han, and Yi Zhao for their industrious work in pursuing the experiments on genome sequence analysis of LEC1-like genes in land plants as well as functional analysis in pteridophytes. We thank Dong-Hui Wang for her help in administrative affairs.
The authors declare that the research was conducted in the absence of any commercial or financial relationships that could be construed as a potential conflict of interest.
All claims expressed in this article are solely those of the authors and do not necessarily represent those of their affiliated organizations, or those of the publisher, the editors and the reviewers. Any product that may be evaluated in this article, or claim that may be made by its manufacturer, is not guaranteed or endorsed by the publisher.
Bai, S. N. (2015). The concept of the sexual reproduction cycle and its evolutionary significance. Front. Plant Sci. 6:11. doi: 10.3389/fpls.2015.00011
Bai, S. N. (2017). Reconsideration of plant morphological traits: from a structure-based perspective to a function-based evolutionary perspective. Front. Plant Sci. 8:345. doi: 10.3389/fpls.2017.00345
Bai, S. N. (2019). Plant morphogenesis 123: a renaissance in modern botany? Sci. China Life Sci. 62, 453–466. doi: 10.1007/s11427-018-9457-1
Fang, Y. H., Li, X., Bai, S. N., and Rao, G. Y. (2017). Sugar treatments can induce AcLEAFY COTYLEDON1 expression and trigger the accumulation of storage products during prothallus development of Adiantum capillus-veneris. Front. Plant Sci. 8:541. doi: 10.3389/fpls.2017.00541
Fang, Y. H., Qin, X., Liao, Q. G., Du, R., Luo, X. Z., Zhou, Q., et al. (2022). The genome of homosporous 1 maidenhair fern sheds light on the euphyllophyte evolution and defenses. Nat. Plant (in press).
Finkelstein, R. R., and Gibson, S. I. (2002). ABA and sugar interactions regulating development: cross-talk or voices in a crowd? Curr. Opin. Plant Biol. 5, 26–32. doi: 10.1016/S1369-5266(01)00225-4
Han, J. D., Li, X., Jiang, C. K., Wong, G. K., Rothfels, C. J., and Rao, G. Y. (2017). Evolutionary analysis of the LAFL genes involved in the land plant seed maturation program. Front. Plant Sci. 8:439. doi: 10.3389/fpls.2017.00439
Harada, J. J. (1997). “Seed maturation and control of germination,” in Advances in Cellular and Molecular Biology of Plants, Vol. 4, Cellular and Molecular Biology of Seed Development. eds. B. A. Larkins and I. K. Vasi (Dordrecht: Kluwer Academic Publishers), 545–592. doi: 10.1007/978-94-015-8909-3_15
Harada, J. J. (2001). Role of Arabidopsis LEAFY COTYLEDON genes in seed development. J. Plant Physiol. 158, 405–409. doi: 10.1078/0176-1617-00351
Herr, J. M. (1995). The origin of the ovule. Am. J. Bot. 82, 547–564. doi: 10.1002/j.1537-2197.1995.tb15676.x
Koornneef, M., and Meinke, D. (2010). The development of Arabidopsis as a model plant. Plant J. 61, 909–921. doi: 10.1111/j.1365-313X.2009.04086.x
Koornneef, M., Reuling, G., and Karssen, C. M. (1984). The isolation and characterization of abscisic-acid insensitive mutants of Arabidopsis thaliana. Physiol. Plant. 61, 377–383. doi: 10.1111/j.1399-3054.1984.tb06343.x
Li, X., Fang, Y. H., Yang, J., Bai, S. N., and Rao, G. Y. (2013). Overview of the morphology, anatomy, and ontogeny of Adiantum capillus-veneris: an experimental system to study the development of ferns. J. Syst. Evol. 51, 499–510. doi: 10.1111/jse.12034
Li, X., Han, J.-D., Fang, Y.-H., Bai, S.-N., and Rao, G.-Y. (2017). Expression analyses of embryogenesis-associated genes during somatic embryogenesis of Adiantum capillus-veneris L. in vitro: new insights into the evolution of reproductive organs in land plants. Front. Plant Sci. 8:658. doi: 10.3389/fpls.2017.00658
Lotan, T., Ohto, M., Yee, K. M., West, M. A., Lo, R., Kwong, R. W., et al. (1998). Arabidopsis LEAFY COTYLEDON1 is sufficient to induce embryo development in vegetative cells. Cell 93, 1195–1205. doi: 10.1016/S0092-8674(00)81463-4
Meinke, D. W. (1992). A homoeotic mutant of Arabidopsis thaliana with leafy cotyledons. Science 258, 1647–1650. doi: 10.1126/science.258.5088.1647
Meinke, D. W., Franzmann, L. H., Nickle, T. C., and Yeung, E. C. (1994). Leafy cotyledon mutants of Arabidopsis. Plant Cell 6, 1049–1064. doi: 10.2307/3869884
Musser, J. M., Schippers, K. J., Nickel, M., Mizzon, G., Kohn, A. B., Pape, C., et al. (2021). Profiling cellular diversity in sponges informs animal cell type and nervous system evolution. Science 374, 717–723. doi: 10.1126/science.abj2949
Schulz, C., Little, D. P., Stevenson, D. W., Bauer, D., Moloney, C., and Stützel, T. (2010). An overview of the morphology, anatomy, and life cycle of a new model species: the lycophyte Selaginella apoda (L.) Spring. Int. J. Plant Sci. 171, 693–712. doi: 10.1086/654902
Smith, A. M., Coupland, G., Dolan, L., Harberd, N., Jonse, J., Martin, C., et al. (2010). Plant Biology. New York, NY: Garland Science.
Taiz, L., Zeiger, E., Møller, I. M., and Murphy, A. (2014). Plant Physiology and Development, Sunderland, CT: Sinauer Associates.
Taylor, E. L, Taylor, T. N., and Krings, M. (2009). Paleobotany: The Biology and Evolution of Fossil Plants. Cambridge, MA: Academic Press.
Tsukagoshi, H., Morikami, A., and Nakamura, K. (2007). Two B3 domain transcriptional repressors prevent sugar-inducible expression of seed maturation genes in Arabidopsis seedlings. Proc. Natl. Acad. Sci. U. S. A. 104, 2543–2547. doi: 10.1073/pnas.0607940104
Wang, X., and Bai, S. N. (2019). Key innovations in transition from homospory to heterospory. Plant Sign. Behav. 14:1596010. doi: 10.1080/15592324.2019.1596010
Weber, H., Borisjuk, L., and Wobus, U. (2005). Molecular physiology of legume seed development. Annu. Rev. Plant Biol. 56, 253–279. doi: 10.1146/annurev.arplant.56.032604.144201
West, M., Yee, K. M., Danao, J., Zimmerman, J. L., Fischer, R. L., Goldberg, R. B., et al. (1994). LEAFY COTYLEDON1 is an essential regulator of late embryogenesis and cotyledon identity in Arabidopsis. Plant Cell 6, 1731–1745. doi: 10.2307/3869904
Wobus, U., and Weber, H. (1999). Seed maturation: genetic programmes and control signals. Curr. Opin. Plant Biol. 2, 33–38. doi: 10.1016/S1369-5266(99)80007-7
Xie, Z., Li, X., Glover, B. J., Bai, S. N., Rao, G. Y., Luo, J. C., et al. (2008). Duplication and functional diversification of HAP3 genes leading to the origin of the seed-developmental regulatory gene, LEAFY COTYLEDON1 (LEC1), in nonseed plant genomes. Mol. Biol. Evol. 25, 1581–1592. doi: 10.1093/molbev/msn105
Keywords: seeds, origin, seed program, pteridophytes, “golden-trio hypothesis”
Citation: Bai S-N, Rao G-Y and Yang J (2022) Origins of the seed: The “golden-trio hypothesis”. Front. Plant Sci. 13:965000. doi: 10.3389/fpls.2022.965000
Received: 09 June 2022; Accepted: 05 August 2022;
Published: 29 August 2022.
Edited by:
Alexander P. Sukhorukov, Lomonosov Moscow State University, RussiaReviewed by:
Kazuo Nakashima, Japan International Research Center for Agricultural Sciences, JapanCopyright © 2022 Bai, Rao and Yang. This is an open-access article distributed under the terms of the Creative Commons Attribution License (CC BY). The use, distribution or reproduction in other forums is permitted, provided the original author(s) and the copyright owner(s) are credited and that the original publication in this journal is cited, in accordance with accepted academic practice. No use, distribution or reproduction is permitted which does not comply with these terms.
*Correspondence: Shu-Nong Bai, c2h1bm9uZ2JAcGt1LmVkdS5jbg==
Disclaimer: All claims expressed in this article are solely those of the authors and do not necessarily represent those of their affiliated organizations, or those of the publisher, the editors and the reviewers. Any product that may be evaluated in this article or claim that may be made by its manufacturer is not guaranteed or endorsed by the publisher.
Research integrity at Frontiers
Learn more about the work of our research integrity team to safeguard the quality of each article we publish.