- 1School of Biological Sciences, Seoul National University, Seoul, South Korea
- 2Research Center for Plant Plasticity, Seoul National University, Seoul, South Korea
Proper timing of flowering, a phase transition from vegetative to reproductive development, is crucial for plant fitness. The floral repressor FLOWERING LOCUS C (FLC) is the major determinant of flowering in Arabidopsis thaliana. In rapid-cycling A. thaliana accessions, which bloom rapidly, FLC is constitutively repressed by autonomous pathway (AP) genes, regardless of photoperiod. Diverse AP genes have been identified over the past two decades, and most of them repress FLC through histone modifications. However, the detailed mechanism underlying such modifications remains unclear. Several recent studies have revealed novel mechanisms to control FLC repression in concert with histone modifications. This review summarizes the latest advances in understanding the novel mechanisms by which AP proteins regulate FLC repression, including changes in chromatin architecture, RNA polymerase pausing, and liquid–liquid phase separation- and ncRNA-mediated gene silencing. Furthermore, we discuss how each mechanism is coupled with histone modifications in FLC chromatin.
Introduction
Proper timing of flowering, a phase transition from vegetative to reproductive development, is crucial for plant survival. Consequently, plants have evolved and developed various mechanisms to control flowering time in response to variable environments. Many plants in temperate regions have adopted winter-annual flowering traits that require prolonged cold winter temperatures for flowering in spring when the environment is favorable (Chouard, 1960; Bernier et al., 1993). However, some plants complete their life cycle rapidly, either in spring or fall (Weinig and Schmitt, 2004). For example, Arabidopsis thaliana accessions are classified into winter-annual and rapid-cycling types based on the requirement of long-term winter cold for rapid flowering (Michaels and Amasino, 2000). The underlying genetic difference in flowering traits between the two types is the presence or absence of the FLOWERING LOCUS C (FLC) and FRIGIDA (FRI) genes (Gazzani et al., 2003). FLC, which encodes a MADS-box transcription factor, acts as a potent floral repressor which inhibits the transcription of floral promoters, including FT (encoding florigen) and SUPPRESSOR OF OVEREXPRESSION OF CONSTANS 1 (Lee et al., 2000; Michaels et al., 2005; Helliwell et al., 2006). FRI, a coiled-coil protein which forms part of a super protein complex, acts as a transcriptional activator of FLC (Michaels and Amasino, 1999; Choi et al., 2011; Li et al., 2018).
In rapid cyclers, the autonomous floral-promotion pathway (AP) induces early flowering by repressing FLC expression (Koornneef et al., 1998; Levy and Dean, 1998). Since LUMINIDEPENDENS (LD) was first isolated (Rédei, 1962; Lee et al., 1994), several genes, including FCA, FLD, FLK, FPA, FVE, and FY, have been cloned as AP genes (Macknight et al., 1997; Koornneef et al., 1998; Schomburg et al., 2001; He et al., 2003; Quesada et al., 2003; Simpson et al., 2003; Lim et al., 2004; Mockler et al., 2004). For the past two decades, researchers have investigated the biochemical functions of AP proteins. Reports suggest that a subset of AP proteins catalyze the epigenetic changes in FLC chromatin. Specifically, FVE and FLD constitute histone deacetylation or demethylation complexes, whereby the FLC chromatin turns into a repressive state (Liu et al., 2007; Yu et al., 2016). Additionally, several RNA-binding family proteins, such as FPA, FCA, and FY, indirectly repress FLC by mediating the 3′-end processing of FLC antisense transcript (Simpson et al., 2003; Liu et al., 2007; Hornyik et al., 2010; Liu et al., 2010). However, the function of AP proteins has yet to be completely understood.
Emerging evidence suggests that multiple layers of transcriptional processes determine transcript level (Gressel et al., 2019). In addition to well-known processes (e.g., enhancer- and histone modification-mediated gene regulation), regulatory mechanisms, such as RNA polymerase II (Pol II) pause–release control during transcriptional elongation and alternative polyadenylation during transcriptional termination, are critical gene regulatory processes (Tian and Manley, 2017; Chen et al., 2018). Notably, recent studies have consistently revealed that AP in floral promotion is also involved in such mechanisms. This review summarizes the latest findings on the molecular mechanisms of AP, including the control of chromatin architecture, Pol II pausing, and phase separation with ncRNA-mediated gene silencing.
Architecture of FLC chromatin and AP
The 3D property of chromatin plays a vital role in transcriptional regulation (Dileep and Tsai, 2021; Deng et al., 2022). Although histone modifications such as methylation and acetylation have been the main focus of the studies for transcriptional regulation over the past two decades, studies on how chromatin architecture, such as chromatin loops, R-loops, and DNA topology, controls gene expression have been actively conducted in recent years (Kadauke and Blobel, 2009; Kouzine et al., 2014; Al-Hadid and Yang, 2016). Accordingly, the AP-mediated repression of FLC has been re-examined based on its chromatin architecture.
Chromatin loops, defined as the intergenic or intragenic bending of chromatin, are observed genome-wide in Arabidopsis (Grob et al., 2013; Feng et al., 2014). The 5′-end region of the FLC locus is connected to either the first intron or 3′-end region to form chromatin loops (Crevillén et al., 2013; Kim and Sung, 2017; Li et al., 2018). Importantly, the loop linking the 5′- and 3′-ends of FLC may contribute to FLC activation, possibly through enhancing Pol II recycling (Crevillén et al., 2013; Li et al., 2018). A recent study has identified novel AP members, the GH1-HMGA family proteins, which are involved in regulating this loop (Zhao B. et al., 2021). GH1-HMGA family proteins, also known as HIGH MOBILITY GROUP A4, 5 (HON4, 5), are homologs of human HMGA proteins which bend or unwind local chromatin structure (Ozturk et al., 2014). Similar to other AP mutant lines, the honq (gh1-hmga quadruple) mutant line exhibits increased FLC expression and delayed flowering (Zhao B. et al., 2021). Given that the FLC gene loop in the honq mutant line is increased, it has been suggested that the disruption of gene looping by GH1-HMGA family proteins may repress FLC expression by altering chromatin structures required for effective transcription (Figure 1A; Zhao B. et al., 2021). However, the causal relationship between the chromatin looping and the repression of FLC by GH1-HMGA family proteins should be validated in the future study. In contrast to the GH1-HMGA family proteins, the histone variant H3.3 appears to stabilize FLC looping by binding at both ends of FLC gene (Zhao F. et al., 2021). Importantly, h3.3 knock-down mutants (h3.3kd) consistently show reduced FLC looping and decreased FLC level (Zhao F. et al., 2021). Therefore, it is likely that the opposite effects of GH1-HMGA family proteins and H3.3 for the FLC looping may be associated with their antagonistic function on FLC expression. BAF60, a component of the Arabidopsis SWI/SNF (SWITCH/SUCROSE NON-FERMNETABLE)-type ATP-dependent chromatin remodeling complex, also participates in FLC repression by affecting FLC gene looping (Jégu et al., 2014). It has been shown that the RNA interference lines of BAF60 (BAF60 RNAi) display an increased number of FLC gene loops and upregulated expression of FLC, thereby producing the late-flowering phenotype in long days. This finding suggests that BAF60 plays a negative role in loop formation. Histone modifications including H3K27me3, H3K9Ac, and H2A.Z replacement, are also altered in the BAF60 RNAi lines; thus, the effect of BAF60 on FLC gene looping may be mediated through histone modifications. One caveat is that BAF60 is not a typical AP gene because the BAF60 RNAi lines do not show delayed flowering in short days. The increased FLC level caused by BAF60 RNAi is probably masked by the additional targets of BAF60. Therefore, BAF60 may also be an FLC repressor which acts on gene looping. The functional interdependency between GH1-HMGA family proteins and BAF60 needs further analysis.
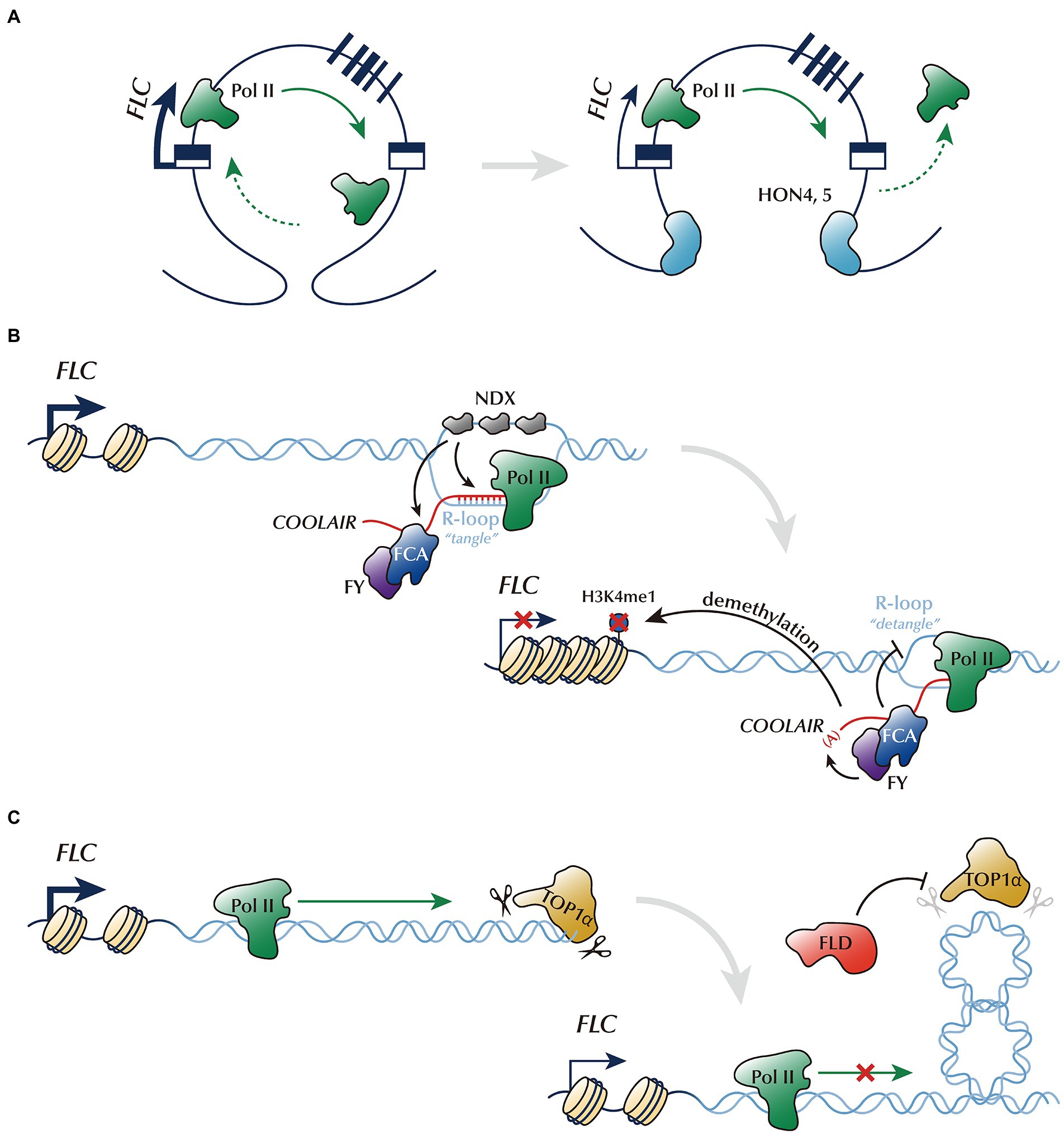
Figure 1. Control of FLC chromatin architecture by the autonomous pathway. (A) Chromatin loop linking the 5′- and 3′-ends of FLC will likely intensify the FLC transcription by promoting Pol II recycling. GH1-HMGA family proteins, HON4 and HON5, disrupt this chromatin looping, and thus reduce FLC expression. (B) NDX stabilizes the R-loop at the FLC 3′-end, where the antisense ncRNA, COOLAIR, is transcribed (tangled). This process probably enhances the binding of FCA/FY onto COOLAIR and inhibits Pol II progression. FCA/FY, in turn, represses FLC expression by promoting the proximal polyadenylation of COOLAIR and resolving the R-loop (detangled). (C) Antagonized function of TOP1α and FLD controlling DNA topology. TOP1α enhances FLC transcription potentially by reducing the torsional stress generated by DNA supercoiling. FLD partially counteracts TOP1α activity.
R-loops are another type of chromatin architecture which are composed of a DNA:RNA hybrid and an associated non-template single-stranded DNA (Al-Hadid and Yang, 2016). R-loops play important roles in gene expression, genome stability, and epigenomic signatures (Gao et al., 2021). FLC chromatin has an R-loop around its 3′-end, where the antisense transcript COOLAIR is transcribed (Sun et al., 2013; Baxter et al., 2021; Xu et al., 2021b). NODULIN HOMEOBOX (NDX) is a potential AP member that reportedly stabilizes this R-loop by binding onto the non-template ssDNA region (Sun et al., 2013). The increased COOLAIR level and the reduction of FCA-COOLAIR interaction in the ndx mutant suggest that R-loop stabilizing processes likely inhibit further transcription of COOLAIR and enhance binding of FCA onto COOLAIR (Xu et al., 2021b). The fca and fy mutants show an increased level of R-loops, suggesting that FCA and its binding partner, FY, act to resolve the R-loops (Figure 1B). Thus, R-loop dynamics, involving the stabilization by NDX and resolution by FCA and FY, result in FLC repression. However, the detailed mechanism by which R-loops participate in FLC transcription warrants further investigation. Furthermore, the loss of m6A methyltransferase (mRNA ADENOSINE METHYLASE, MTA) increases the level of R-loops, indicating that the N6-methyladenosine (m6A) modification of RNA is involved in R-loop resolution (Xu et al., 2021b). MTA interacts with FCA and is a potential AP member, as evidenced by the increased FLC expression in the mta mutant line. Moreover, a follow-up study showed that the resolution of the R-loop by FCA or FY is required for the proper progression of DNA replication fork, suggesting an interplay between DNA replication and transcription (Baxter et al., 2021).
AP is also possibly involved in regulating DNA topology. During transcription, torsional stress generated by DNA supercoiling inhibits proper transcription (Liu and Wang, 1987). Thus, the proper release of supercoiling by topoisomerases is required for transcriptional activation (French et al., 2011). Consistently, DNA topoisomerase I, TOP1α, in Arabidopsis, which binds to FLC chromatin, promotes FLC expression (Gong et al., 2017). Thus, the modulation of DNA topology by TOP1α promotes FLC transcription, possibly through Pol II accommodation. In contrast, the AP protein, FLD, counteracts TOP1α (Inagaki et al., 2021). FLD acts antagonistically to TOP1α for FLC transcription, as evidenced by the partial suppression of the late-flowering phenotype of fld in the top1α fld double mutant line (Gong et al., 2017). In addition, enhanced Pol II enrichment on the FLD-target genes in the fld mutant line is suppressed by top1α (Inagaki et al., 2021). This result suggests that FLD antagonizes the function of TOP1α and FLD is involved in the control of torsional stress on FLC chromatin (Figure 1C). However, the detailed function of FLD needs further elucidation.
FLC repression by 3′-pausing of Pol II
During transcription in Drosophila melanogaster, or in mammalian cells, Pol II is transiently paused before it enters the elongation phase (Adelman and Lis, 2012; Chen et al., 2018). Controlling Pol II pause–release is possibly a core determinant of gene expression, considering that successful Pol II release into the productive elongation phase is required for the complete transcription (Core and Adelman, 2019). In most animal genes, Pol II pauses after transcribing short stretches (approximately 30–50 nts) of RNA from the transcription start site (TSS). Several pause-inducing factors, including DRB sensitivity-inducing factor (DSIF) and negative elongation factor (NELF), are known to stabilize the paused Pol II (Wu et al., 2003; Wu et al., 2005).
In contrast to animals, plants were thought to have different types of Pol II pausing, because they lack NELF proteins (Hetzel et al., 2016). However, Pol II pausing at the 5′-end is also observed in plants, although Pol II is usually stalled near the transcription termination site (TTS) of plant genes according to the studies using Global Run-On sequencing (GRO-seq) and plant native elongating transcript sequencing (plaNET-seq) methods (Hetzel et al., 2016; Zhu et al., 2018; Kindgren et al., 2020). FLC appears to be one of the 3′-paused genes, as it shows the typical characteristics: it has a relatively long gene length, it expresses antisense RNAs, and it is relatively close to its neighbor gene with the same orientation (Yu et al., 2019; Inagaki et al., 2021).
Emerging evidence suggests that some AP genes act as pause-inducing factors which may govern FLC transcription (Figure 2A). For example, a recent study has identified novel AP members, called BORDER (BDR) family genes (Yu et al., 2021). Similar to other AP mutants, the bdr123 triple mutant shows delayed flowering with elevated expression of FLC. In general, BDR proteins localize at the borders of genes close to their neighbor genes (Yu et al., 2019). They are likely to inhibit the progression of Pol II over the gene border, thereby preventing Pol II invasion into the promoters of downstream genes. However, the impediment of Pol II elongation by BDRs may result in decreased transcript accumulation. FLC may also be a target of such an inhibitory mechanism; however, further research is warranted for verification. Notably, the popular AP protein, FPA, is located at the borders of genes, especially at TTS (Yu et al., 2021). In addition, FPA physically interacts with BDR proteins and shares common targets. Therefore, it would be valuable to address whether FPA also promotes 3’ Pol II pausing in a similar manner to BDR, especially around the FLC locus. In addition, there is still uncertainty around whether 3’ Pol II pausing causes FLC gene silencing.
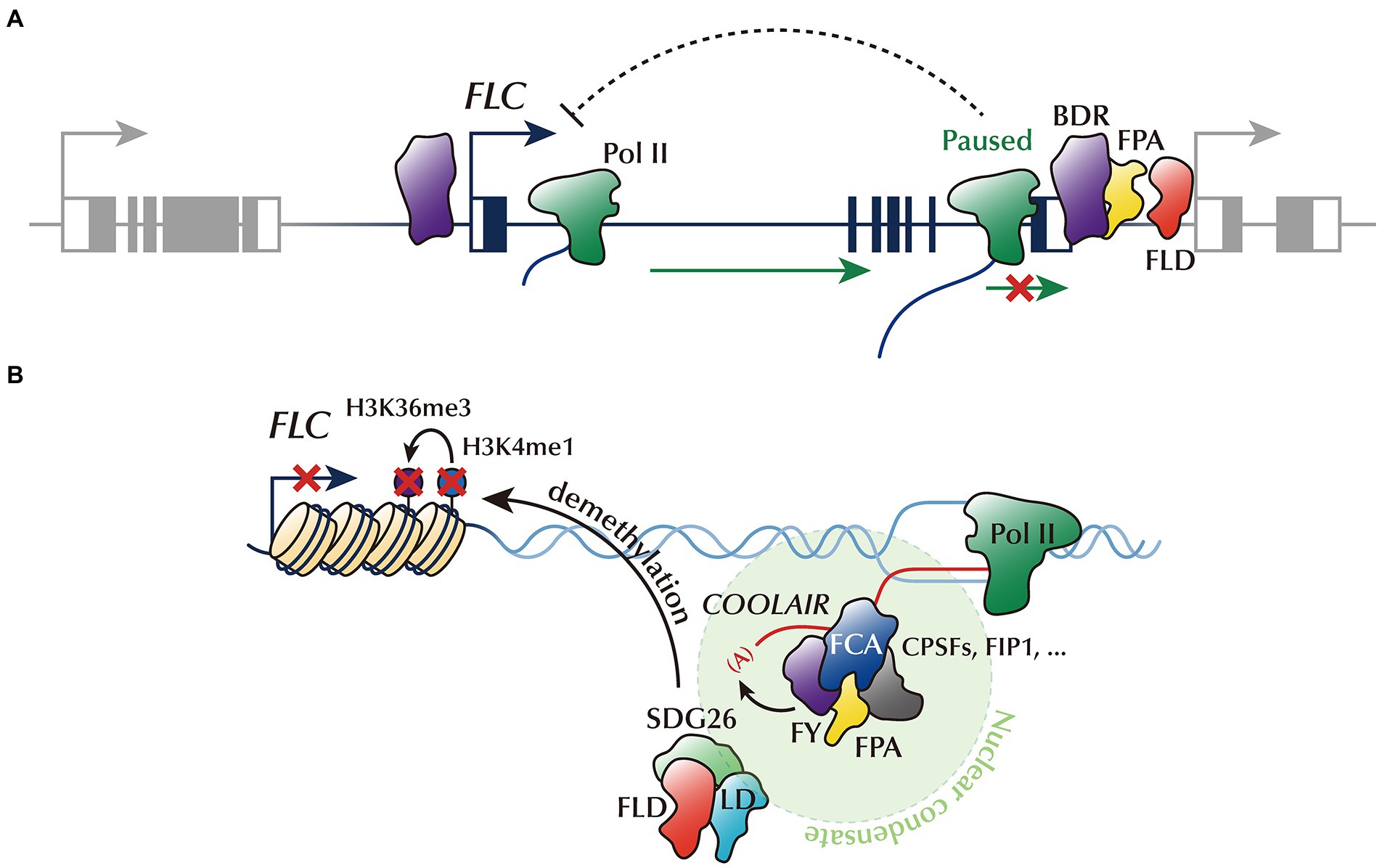
Figure 2. FLC repression by 3′-pausing, phase-separated AP proteins, and ncRNA. (A) AP proteins, BDR, FPA, and FLD, localizing at the gene borders, trigger 3’ Pol II pausing. Impediment of Pol II release into the elongation phase may reduce FLC transcript accumulation. (B) AP proteins, including FCA, FPA, FY, and RNA-processing factors, are condensed into phase-separated nuclear condensates to promote the proximal polyadenylation of COOLAIR. This phase-separated ncRNA-processing machinery transiently interacts with FLD/LD/SDG26, H3K4me1 demethylase complex, thereby removing the active histone marks (H3K4me1 and H3K36me3) from FLC chromatin.
FLD, another major AP component, reportedly modulates Pol II pause–release (Inagaki et al., 2021). FLD is enriched at the TSS and TTS of genes rather than their gene body. Pol II is stalled around the TTS of FLD-targeted genes, and such 3’ Pol II pausing is conspicuously reduced in the fld mutant, indicating that FLD accelerates Pol II pausing. Since FLD occupies the 3′-end regions of FLC (Inagaki et al., 2021), FLC transcription is potentially repressed by FLD-promoted 3’ Pol II pausing. The physical interaction between FLD and LD proteins and similar transcriptome profiles between the fld and ld mutants suggest that FLD and LD cooperatively regulate the transcription (Fang et al., 2020; Inagaki et al., 2021). While the genome-wide function of FLD on 3’ Pol II pausing has been addressed (Inagaki et al., 2021), whether FLD also triggers 3’ Pol II pausing on the FLC locus is yet to be confirmed.
Accumulating evidence from studies using metazoans suggests an interplay between Pol II pausing and chromatin landscape. For instance, a rapid release of Pol II facilitates a broad distribution of active histone marks over the gene body, which is tightly linked with the high expression of the gene (Chen et al., 2015; Tettey et al., 2019). In contrast, Polycomb-group (PcG) proteins that catalyze the deposition of repressive histone marks preferentially target paused promoters (Enderle et al., 2011). Similarly, AP-mediated 3′-pausing at FLC may switch the FLC chromatin state inactive, thus suppressing FLC transcription. Consistent with this idea, 3’ Pol II pausing events triggered by the BDRs and FPA are correlated with the removal of H3K4me3 and the deposition of H3K27me3 (Yu et al., 2021). Furthermore, FLD and LD are likely to remove H3K4me1 from the gene bodies of their targets, suggesting that the AP proteins coordinate transcriptional events with chromatin silencing (Fang et al., 2020; Inagaki et al., 2021). Future research should explore the mechanism by which Pol II pause–release is linked to histone modifications for FLC suppression.
Phase-separated AP proteins- and non-coding RNA-mediated gene silencing
Non-coding RNAs (ncRNAs) are RNAs that are not translated into proteins. They function in transcriptional or post-transcriptional gene regulation, structural organization of nuclear bodies, and genome integrity control (Ponting et al., 2009; Statello et al., 2021). The FLC locus also produces multiple long non-coding RNAs, such as COOLAIR, COLDAIR, and COLDWRAP (Swiezewski et al., 2009; Heo and Sung, 2011; Kim and Sung, 2017), all of which reportedly control dynamic alterations of chromatin state in the FLC locus after long-term cold exposure (Csorba et al., 2014; Kim and Sung, 2017; Kim et al., 2017; Zhao Y. et al., 2021). Among these RNAs, COOLAIR, an antisense transcript produced from the 3′-end of FLC, has been proposed to play a role in the epigenetic control of FLC with the help of AP proteins (Whittaker and Dean, 2017; Wu et al., 2020).
Multiple studies suggest that several AP genes, especially those encoding RNA-processing factors, control the 3′-end processing of COOLAIR (Hornyik et al., 2010; Liu et al., 2010; Marquardt et al., 2014; Wang et al., 2014). Some RNA-processing factors, such as a core spliceosome subunit [PRE-MRNA PROCESSING 8 (PRP8)] and a transcriptional elongation factor [CYCLIN-DEPENDENT KINASE C;2 (CDKC;2)], have been identified as AP members (Marquardt et al., 2014; Wang et al., 2014). The functions of PRP8 and CDKC;2 in FLC repression are dependent on COOLAIR; prp8 or cdkc;2 does not upregulate FLC expression any further if the COOLAIR promoter is replaced with rbcs3B terminator sequence [FLC-TEX in Marquardt et al. (2014) and Wang et al. (2014)]. Consistent with this, previous studies reported that PRP8 and CDKC;2 indirectly affect the expression of FLC by promoting the proximal polyadenylation of COOLAIR (Marquardt et al., 2014; Wang et al., 2014). The major AP genes, FCA, FPA, and FY, have also been proposed to control the processing of COOLAIR. FCA, FPA, and FY reportedly favor the usage of proximal poly(A) site in COOLAIR (Liu et al., 2010). Considering the epistatic interactions between fca, and prp8 or cdkc;2, FCA, and PRP8 or CDKC;2 are thought to share the same genetic pathway to antagonize FLC expression (Marquardt et al., 2014; Wang et al., 2014).
Such COOLAIR-processing machinery is likely to be condensed into phase-separated nuclear bodies, and this process may be a mechanism behind FLC repression. FCA is clustered into nuclear condensates together with FPA, FY, and the subunits of polyadenylation machinery, including cleavage and polyadenylation factor 30 (CPSF30), CPSF100, and FH INTERACTING PROTEIN 1 (FIP1; Fang et al., 2019). FCA is required for the condensation of the polyadenylation machinery and directly associates with COOLAIR transcripts; thus, it likely concentrates the polyadenylation machinery near the COOLAIR to promote the usage of the proximal poly(A) site (Fang et al., 2019; Tian et al., 2019; Xu et al., 2021b). This condensation is enhanced by the prion-like domain (PrLD)-containing protein FLX-LIKE 2 (FLL2), RNA slicer ARGONAUTE 1 (AGO1), and m6A writer complex depositing m6A onto COOLAIR (Fang et al., 2019; Xu et al., 2021a,b).
The phase-separated COOLAIR-processing complex likely controls the FLC chromatin state through FLD. FLD assembles into a complex with LD and SET DOMAIN GROUP 26 (SDG26), which causes the removal of H3K4me1 deposited at FLC chromatin (Fang et al., 2020). This disables SDG8, which binds to H3K4me1 and facilitates the enrichment of H3K36me3, thereby suppressing FLC transcription (Fang et al., 2020). Recent results obtained using cross-linked nuclear immunoprecipitation and mass spectrometry (CLNIP-MS) suggest that a transient and dynamic interaction occurs between SDG26 and the components of the phase-separated poly(A) machinery, such as FCA, FPA, and FY (Figure 2B; Fang et al., 2019; Fang et al., 2020). In addition, AGO1, which is bound to COOLAIR at a proximal exon-intron junction region, also interacts with SDG26 (Xu et al., 2021a). Therefore, the COOLAIR 3′-processing event likely controls the FLC chromatin state through the physical interaction between components of the COOLAIR polyadenylation condensate and the FLD/LD/SDG26 protein complex. Moreover, this phase-separated polyadenylation complex, including FCA and FY, may resolve the COOLAIR-mediated R-loop at the 3′-end of FLC (Xu et al., 2021a,b), as mentioned earlier. Given that this R-loop is also closely connected to the histone modifications in other organisms (Chédin, 2016), this connection may be a missing link between co-transcriptional COOLAIR processing and FLC chromatin silencing. However, the causal relationship between COOLAIR-mediated R-loop processing and FLC chromatin silencing needs further verification (Xu et al., 2021b).
Recent studies have inferred that another clade of ncRNAs, small RNAs (sRNAs), could be associated with FLC repression. For example, AGO1 interacts with sRNA fragments that are complementary to COOLAIR (Xu et al., 2021a). Moreover, DICER-LIKE 1 (DCL1) and DCL3, required for sRNA production, are likely to suppress FLC independently of the FCA-mediated FLC silencing mechanism (Schmitz et al., 2007; Xu et al., 2021a). Therefore, a deeper understanding of the role of sRNAs in AP for flowering should be a focus in future research.
Conclusion
This review summarizes the latest research progress in the autonomous pathway in Arabidopsis. Decades of studies have proposed unique mechanisms for FLC regulation, such as control of Pol II pause–release mechanism, modulation of chromatin architecture, and processing of ncRNA triggered by phase-separated machinery. The studies on such mechanisms are still in their infancy and heavily dependent on genome-wide transcriptome analyses. Thus, a large portion of the current models presented in this review has yet to be validated. Further verification of the proposed mechanisms through biochemical, genetic, and molecular work would be valuable to develop a better understanding of AP. In addition, this pathway has been closely linked with the epigenetic modification of FLC chromatin, particularly in relation to the changes in histone methylation patterns in the AP mutants. However, the detailed mechanism connecting the regulatory function of AP proteins described in this review and the epigenetic silencing of FLC remain largely unknown; thus, further studies are required.
The FRI complex strongly activates FLC expression even in the presence of AP proteins in winter-annual Arabidopsis (Johanson et al., 2000; Choi et al., 2011). This finding suggests that the FLC regulatory mechanisms of AP genes are counteracted by FRI complex. Therefore, there is a need for further studies elucidating the role of the FRI complex in the mechanisms of AP.
Author contributions
JK and MJ drafted the manuscript. MJ prepared the figures. IL reviewed and edited the manuscript. All authors contributed to the article and approved the submitted version.
Funding
This work was supported by the National Research Foundation of Korea (NRF) grant funded by the Korea Government (MSIT; No. NRF-2021R1A5A1032428).
Conflict of interest
The authors declare that the research was conducted in the absence of any commercial or financial relationships that could be construed as a potential conflict of interest.
Publisher’s note
All claims expressed in this article are solely those of the authors and do not necessarily represent those of their affiliated organizations, or those of the publisher, the editors and the reviewers. Any product that may be evaluated in this article, or claim that may be made by its manufacturer, is not guaranteed or endorsed by the publisher.
References
Adelman, K., and Lis, J. T. (2012). Promoter-proximal pausing of RNA polymerase II: emerging roles in metazoans. Nat. Rev. Genet. 13, 720–731. doi: 10.1038/nrg3293
Al-Hadid, Q., and Yang, Y. (2016). R-loop: an emerging regulator of chromatin dynamics. Acta Biochim. Biophys. Sin. Shanghai 48, 623–631. doi: 10.1093/abbs/gmw052
Baxter, C. L., Šviković, S., Sale, J. E., Dean, C., and Costa, S. (2021). The intersection of DNA replication with antisense 3' RNA processing in Arabidopsis FLC chromatin silencing. Proc. Natl. Acad. Sci. U. S. A. 118:e2107483118. doi: 10.1073/pnas.2107483118
Bernier, G., Havelange, A., Houssa, C., Petitjean, A., and Lejeune, P. (1993). Physiological signals that induce flowering. Plant Cell 5, 1147–1155. doi: 10.1105/tpc.5.10.1147
Chédin, F. (2016). Nascent connections: R-loops and chromatin patterning. Trends Genet. 32, 828–838. doi: 10.1016/j.tig.2016.10.002
Chen, F. X., Smith, E. R., and Shilatifard, A. (2018). Born to run: control of transcription elongation by RNA polymerase II. Nat. Rev. Mol. Cell Biol. 19, 464–478. doi: 10.1038/s41580-018-0010-5
Chen, K., Chen, Z., Wu, D., Zhang, L., Lin, X., Su, J., et al. (2015). Broad H3K4me3 is associated with increased transcription elongation and enhancer activity at tumor-suppressor genes. Nat. Genet. 47, 1149–1157. doi: 10.1038/ng.3385
Choi, K., Kim, J., Hwang, H. J., Kim, S., Park, C., Kim, S. Y., et al. (2011). The FRIGIDA complex activates transcription of FLC, a strong flowering repressor in Arabidopsis, by recruiting chromatin modification factors. Plant Cell 23, 289–303. doi: 10.1105/tpc.110.075911
Chouard, P. (1960). Vernalization and its relations to dormancy. Annu. Rev. Plant Physiol. Plant Mol. Biol. 11, 191–238. doi: 10.1146/annurev.pp.11.060160.001203
Core, L., and Adelman, K. (2019). Promoter-proximal pausing of RNA polymerase II: a nexus of gene regulation. Genes Dev. 33, 960–982. doi: 10.1101/gad.325142.119
Crevillén, P., Sonmez, C., Wu, Z., and Dean, C. (2013). A gene loop containing the floral repressor FLC is disrupted in the early phase of vernalization. EMBO J. 32, 140–148. doi: 10.1038/emboj.2012.324
Csorba, T., Questa, J. I., Sun, Q., and Dean, C. (2014). Antisense COOLAIR mediates the coordinated switching of chromatin states at FLC during vernalization. Proc. Natl. Acad. Sci. U. S. A. 111, 16160–16165. doi: 10.1073/pnas.1419030111
Deng, S., Feng, Y., and Pauklin, S. (2022). 3D chromatin architecture and transcription regulation in cancer. J. Hematol. Oncol. 15:49. doi: 10.1186/s13045-022-01271-x
Dileep, V., and Tsai, L. H. (2021). Three-dimensional chromatin organization in brain function and dysfunction. Curr. Opin. Neurobiol. 69, 214–221. doi: 10.1016/j.conb.2021.04.006
Enderle, D., Beisel, C., Stadler, M. B., Gerstung, M., Athri, P., and Paro, R. (2011). Polycomb preferentially targets stalled promoters of coding and noncoding transcripts. Genome Res. 21, 216–226. doi: 10.1101/gr.114348.110
Fang, X., Wang, L., Ishikawa, R., Li, Y., Fiedler, M., Liu, F., et al. (2019). Arabidopsis FLL2 promotes liquid-liquid phase separation of polyadenylation complexes. Nature 569, 265–269. doi: 10.1038/s41586-019-1165-8
Fang, X., Wu, Z., Raitskin, O., Webb, K., Voigt, P., Lu, T., et al. (2020). The 3′ processing of antisense RNAs physically links to chromatin-based transcriptional control. Proc. Natl. Acad. Sci. U. S. A. 117, 15316–15321. doi: 10.1073/pnas.2007268117
Feng, S., Cokus, S. J., Schubert, V., Zhai, J., Pellegrini, M., and Jacobsen, S. E. (2014). Genome-wide hi-C analyses in wild-type and mutants reveal high-resolution chromatin interactions in Arabidopsis. Mol. Cell 55, 694–707. doi: 10.1016/j.molcel.2014.07.008
French, S. L., Sikes, M. L., Hontz, R. D., Osheim, Y. N., Lambert, T. E., El Hage, A., et al. (2011). Distinguishing the roles of topoisomerases I and II in relief of transcription-induced torsional stress in yeast rRNA genes. Mol. Cell. Biol. 31, 482–494. doi: 10.1128/MCB.00589-10
Gao, J., Zhang, P., Li, X., Wu, W., Wei, H., and Zhang, W. (2021). Toward an understanding of the detection and function of R-loops in plants. J. Exp. Bot. 72, 6110–6122. doi: 10.1093/jxb/erab280
Gazzani, S., Gendall, A. R., Lister, C., and Dean, C. (2003). Analysis of the molecular basis of flowering time variation in Arabidopsis accessions. Plant Physiol. 132, 1107–1114. doi: 10.1104/pp.103.021212
Gong, X., Shen, L., Peng, Y. Z., Gan, Y., and Yu, H. (2017). DNA topoisomerase Iα affects the floral transition. Plant Physiol. 173, 642–654. doi: 10.1104/pp.16.01603
Gressel, S., Schwalb, B., and Cramer, P. (2019). The pause-initiation limit restricts transcription activation in human cells. Nat. Commun. 10:3603. doi: 10.1038/s41467-019-11536-8
Grob, S., Schmid, M. W., Luedtke, N. W., Wicker, T., and Grossniklaus, U. (2013). Characterization of chromosomal architecture in Arabidopsis by chromosome conformation capture. Genome Biol. 14:R129. doi: 10.1186/gb-2013-14-11-r129
He, Y., Michaels, S. D., and Amasino, R. M. (2003). Regulation of flowering time by histone acetylation in Arabidopsis. Science 302, 1751–1754. doi: 10.1126/science.1091109
Helliwell, C. A., Wood, C. C., Robertson, M., James Peacock, W., and Dennis, E. S. (2006). The Arabidopsis FLC protein interacts directly in vivo with SOC1 and FT chromatin and is part of a high-molecular-weight protein complex. Plant J. 46, 183–192. doi: 10.1111/j.1365-313X.2006.02686.x
Heo, J. B., and Sung, S. (2011). Vernalization-mediated epigenetic silencing by a long intronic noncoding RNA. Science 331, 76–79. doi: 10.1126/science.1197349
Hetzel, J., Duttke, S. H., Benner, C., and Chory, J. (2016). Nascent RNA sequencing reveals distinct features in plant transcription. Proc. Natl. Acad. Sci. U. S. A. 113, 12316–12321. doi: 10.1073/pnas.1603217113
Hornyik, C., Terzi, L. C., and Simpson, G. G. (2010). The spen family protein FPA controls alternative cleavage and polyadenylation of RNA. Dev. Cell 18, 203–213. doi: 10.1016/j.devcel.2009.12.009
Inagaki, S., Takahashi, M., Takashima, K., Oya, S., and Kakutani, T. (2021). Chromatin-based mechanisms to coordinate convergent overlapping transcription. Nat Plants 7, 295–302. doi: 10.1038/s41477-021-00868-3
Jégu, T., Latrasse, D., Delarue, M., Hirt, H., Domenichini, S., Ariel, F., et al. (2014). The BAF60 subunit of the SWI/SNF chromatin-remodeling complex directly controls the formation of a gene loop at FLOWERING LOCUS C in Arabidopsis. Plant Cell 26, 538–551. doi: 10.1105/tpc.113.114454
Johanson, U., West, J., Lister, C., Michaels, S., Amasino, R., and Dean, C. (2000). Molecular analysis of FRIGIDA, a major determinant of natural variation in Arabidopsis flowering time. Science 290, 344–347. doi: 10.1126/science.290.5490.344
Kadauke, S., and Blobel, G. A. (2009). Chromatin loops in gene regulation. Biochim. Biophys. Acta 1789, 17–25. doi: 10.1016/j.bbagrm.2008.07.002
Kim, D. H., and Sung, S. (2017). Vernalization-triggered intragenic chromatin loop formation by long noncoding RNAs. Dev. Cell 40, 302.e4–312.e4. doi: 10.1016/j.devcel.2016.12.021
Kim, D. H., Xi, Y., and Sung, S. (2017). Modular function of long noncoding RNA, COLDAIR, in the vernalization response. PLoS Genet. 13:e1006939. doi: 10.1371/journal.pgen.1006939
Kindgren, P., Ivanov, M., and Marquardt, S. (2020). Native elongation transcript sequencing reveals temperature dependent dynamics of nascent RNAPII transcription in Arabidopsis. Nucleic Acids Res. 48, 2332–2347. doi: 10.1093/nar/gkz1189
Koornneef, M., Alonso-Blanco, C., Peeters, A. J., and Soppe, W. (1998). Genetic control of flowering time in Arabidopsis. Annu. Rev. Plant Physiol. Plant Mol. Biol. 49, 345–370. doi: 10.1146/annurev.arplant.49.1.345
Kouzine, F., Levens, D., and Baranello, L. (2014). DNA topology and transcription. Nucleus 5, 195–202. doi: 10.4161/nucl.28909
Lee, H., Suh, S. S., Park, E., Cho, E., Ahn, J. H., Kim, S. G., et al. (2000). The AGAMOUS-LIKE 20 MADS domain protein integrates floral inductive pathways in Arabidopsis. Genes Dev. 14, 2366–2376. doi: 10.1101/gad.813600
Lee, I., Aukerman, M. J., Gore, S. L., Lohman, K. N., Michaels, S. D., Weaver, L. M., et al. (1994). Isolation of LUMINIDEPENDENS: a gene involved in the control of flowering time in Arabidopsis. Plant Cell 6, 75–83. doi: 10.1105/tpc.6.1.75
Levy, Y. Y., and Dean, C. (1998). The transition to flowering. Plant Cell 10, 1973–1989. doi: 10.1105/tpc.10.12.1973
Li, Z., Jiang, D., and He, Y. (2018). FRIGIDA establishes a local chromosomal environment for FLOWERING LOCUS C mRNA production. Nat Plants 4, 836–846. doi: 10.1038/s41477-018-0250-6
Lim, M. H., Kim, J., Kim, Y. S., Chung, K. S., Seo, Y. H., Lee, I., et al. (2004). A new Arabidopsis gene, FLK, encodes an RNA binding protein with K homology motifs and regulates flowering time via FLOWERING LOCUS C. Plant Cell 16, 731–740. doi: 10.1105/tpc.019331
Liu, F., Marquardt, S., Lister, C., Swiezewski, S., and Dean, C. (2010). Targeted 3′ processing of antisense transcripts triggers Arabidopsis FLC chromatin silencing. Science 327, 94–97. doi: 10.1126/science.1180278
Liu, F., Quesada, V., Crevillén, P., Bäurle, I., Swiezewski, S., and Dean, C. (2007). The Arabidopsis RNA-binding protein FCA requires a lysine-specific demethylase 1 homolog to downregulate FLC. Mol. Cell 28, 398–407. doi: 10.1016/j.molcel.2007.10.018
Liu, L. F., and Wang, J. C. (1987). Supercoiling of the DNA template during transcription. Proc. Natl. Acad. Sci. U. S. A. 84, 7024–7027. doi: 10.1073/pnas.84.20.7024
Macknight, R., Bancroft, I., Page, T., Lister, C., Schmidt, R., Love, K., et al. (1997). FCA, a gene controlling flowering time in Arabidopsis, encodes a protein containing RNA-binding domains. Cell 89, 737–745. doi: 10.1016/s0092-8674(00)80256-1
Marquardt, S., Raitskin, O., Wu, Z., Liu, F., Sun, Q., and Dean, C. (2014). Functional consequences of splicing of the antisense transcript COOLAIR on FLC transcription. Mol. Cell 54, 156–165. doi: 10.1016/j.molcel.2014.03.026
Michaels, S. D., and Amasino, R. M. (1999). FLOWERING LOCUS C encodes a novel MADS domain protein that acts as a repressor of flowering. Plant Cell 11, 949–956. doi: 10.1105/tpc.11.5.949
Michaels, S. D., and Amasino, R. M. (2000). Memories of winter: Vernalization and the competence to flower. Plant Cell Environ. 23, 1145–1153. doi: 10.1046/j.1365-3040.2000.00643.x
Michaels, S. D., Himelblau, E., Kim, S. Y., Schomburg, F. M., and Amasino, R. M. (2005). Integration of flowering signals in winter-annual Arabidopsis. Plant Physiol. 137, 149–156. doi: 10.1104/pp.104.052811
Mockler, T. C., Yu, X., Shalitin, D., Parikh, D., Michael, T. P., Liou, J., et al. (2004). Regulation of flowering time in Arabidopsis by K homology domain proteins. Proc. Natl. Acad. Sci. U. S. A. 101, 12759–12764. doi: 10.1073/pnas.0404552101
Ozturk, N., Singh, I., Mehta, A., Braun, T., and Barreto, G. (2014). HMGA proteins as modulators of chromatin structure during transcriptional activation. Front. Cell Dev. Biol. 2:5. doi: 10.3389/fcell.2014.00005
Ponting, C. P., Oliver, P. L., and Reik, W. (2009). Evolution and functions of long noncoding RNAs. Cell 136, 629–641. doi: 10.1016/j.cell.2009.02.006
Quesada, V., Macknight, R., Dean, C., and Simpson, G. G. (2003). Autoregulation of FCA pre-mRNA processing controls Arabidopsis flowering time. EMBO J. 22, 3142–3152. doi: 10.1093/emboj/cdg305
Rédei, G. P. (1962). Supervital mutants of Arabidopsis. Genetics 47, 443–460. doi: 10.1093/genetics/47.4.443
Schmitz, R. J., Hong, L., Fitzpatrick, K. E., and Amasino, R. M. (2007). DICER-LIKE 1 and DICER-LIKE 3 redundantly act to promote flowering via repression of FLOWERING LOCUS C in Arabidopsis thaliana. Genetics 176, 1359–1362. doi: 10.1534/genetics.107.070649
Schomburg, F. M., Patton, D. A., Meinke, D. W., and Amasino, R. M. (2001). FPA, a gene involved in floral induction in Arabidopsis, encodes a protein containing RNA-recognition motifs. Plant Cell 13, 1427–1436. doi: 10.1105/tpc.13.6.1427
Simpson, G. G., Dijkwel, P. P., Quesada, V., Henderson, I., and Dean, C. (2003). FY is an RNA 3′ end-processing factor that interacts with FCA to control the Arabidopsis floral transition. Cell 113, 777–787. doi: 10.1016/s0092-8674(03)00425-2
Statello, L., Guo, C. J., Chen, L. L., and Huarte, M. (2021). Gene regulation by long non-coding RNAs and its biological functions. Nat. Rev. Mol. Cell Biol. 22, 96–118. doi: 10.1038/s41580-020-00315-9
Sun, Q., Csorba, T., Skourti-Stathaki, K., Proudfoot, N. J., and Dean, C. (2013). R-loop stabilization represses antisense transcription at the Arabidopsis FLC locus. Science 340, 619–621. doi: 10.1126/science.1234848
Swiezewski, S., Liu, F., Magusin, A., and Dean, C. (2009). Cold-induced silencing by long antisense transcripts of an Arabidopsis Polycomb target. Nature 462, 799–802. doi: 10.1038/nature08618
Tettey, T. T., Gao, X., Shao, W., Li, H., Story, B. A., Chitsazan, A. D., et al. (2019). A role for FACT in RNA polymerase II promoter-proximal pausing. Cell Rep. 27, 3770.e7–3779.e7. doi: 10.1016/j.celrep.2019.05.099
Tian, B., and Manley, J. L. (2017). Alternative polyadenylation of mRNA precursors. Nat. Rev. Mol. Cell Biol. 18, 18–30. doi: 10.1038/nrm.2016.116
Tian, Y., Zheng, H., Zhang, F., Wang, S., Ji, X., Xu, C., et al. (2019). PRC2 recruitment and H3K27me3 deposition at FLC require FCA binding of COOLAIR. Sci. Adv. 5:eaau7246. doi: 10.1126/sciadv.aau7246
Wang, Z. W., Wu, Z., Raitskin, O., Sun, Q., and Dean, C. (2014). Antisense-mediated FLC transcriptional repression requires the P-TEFb transcription elongation factor. Proc. Natl. Acad. Sci. U. S. A. 111, 7468–7473. doi: 10.1073/pnas.1406635111
Weinig, C., and Schmitt, J. (2004). Environmental effects on the expression of quantitative trait loci and implications for phenotypic evolution. Bioscience 54, 627–635. doi: 10.1641/0006-3568(2004)054[0627,EEOTEO]2.0.CO;2
Whittaker, C., and Dean, C. (2017). The FLC locus: a platform for discoveries in epigenetics and adaptation. Annu. Rev. Cell Dev. Biol. 33, 555–575. doi: 10.1146/annurev-cellbio-100616-060546
Wu, C. H., Lee, C., Fan, R., Smith, M. J., Yamaguchi, Y., Handa, H., et al. (2005). Molecular characterization of Drosophila NELF. Nucleic Acids Res. 33, 1269–1279. doi: 10.1093/nar/gki274
Wu, C. H., Yamaguchi, Y., Benjamin, L. R., Horvat-Gordon, M., Washinsky, J., Enerly, E., et al. (2003). NELF and DSIF cause promoter proximal pausing on the hsp70 promoter in Drosophila. Genes Dev. 17, 1402–1414. doi: 10.1101/gad.1091403
Wu, Z., Fang, X., Zhu, D., and Dean, C. (2020). Autonomous pathway: FLOWERING LOCUS C repression through an antisense-mediated chromatin-silencing mechanism. Plant Physiol. 182, 27–37. doi: 10.1104/pp.19.01009
Xu, C., Fang, X., Lu, T., and Dean, C. (2021a). Antagonistic cotranscriptional regulation through ARGONAUTE1 and the THO/TREX complex orchestrates FLC transcriptional output. Proc. Natl. Acad. Sci. U. S. A. 118:e2113757118. doi: 10.1073/pnas.2113757118
Xu, C., Wu, Z., Duan, H. C., Fang, X., Jia, G., and Dean, C. (2021b). R-loop resolution promotes co-transcriptional chromatin silencing. Nat. Commun. 12:1790. doi: 10.1038/s41467-021-22083-6
Yu, C. W., Chang, K. Y., and Wu, K. (2016). Genome-wide analysis of gene regulatory networks of the FVE-HDA6-FLD complex in Arabidopsis. Front. Plant Sci. 7:555. doi: 10.3389/fpls.2016.00555
Yu, X., Martin, P. G. P., and Michaels, S. D. (2019). BORDER proteins protect expression of neighboring genes by promoting 3' pol II pausing in plants. Nat. Commun. 10:4359. doi: 10.1038/s41467-019-12328-w
Yu, X., Martin, P. G. P., Zhang, Y., Trinidad, J. C., Xu, F., Huang, J., et al. (2021). The BORDER family of negative transcription elongation factors regulates flowering time in Arabidopsis. Curr. Biol. 31, 5377.e5–5384.e5. doi: 10.1016/j.cub.2021.09.074
Zhao, B., Xi, Y., Kim, J., and Sung, S. (2021). Chromatin architectural proteins regulate flowering time by precluding gene looping. Sci. Adv. 7:eabg3097. doi: 10.1126/sciadv.abg3097
Zhao, F., Zhang, H., Zhao, T., Li, Z., and Jiang, D. (2021). The histone variant H3.3 promotes the active chromatin state to repress flowering in Arabidopsis. Plant Physiol. 186, 2051–2063. doi: 10.1093/plphys/kiab224
Zhao, Y., Zhu, P., Hepworth, J., Bloomer, R., Antoniou-Kourounioti, R. L., Doughty, J., et al. (2021). Natural temperature fluctuations promote COOLAIR regulation of FLC. Genes Dev. 35, 888–898. doi: 10.1101/gad.348362.121
Keywords: flowering, autonomous pathway, FLOWERING LOCUS C, histone modification, RNA polymerase II pausing, chromatin architecture, non-coding RNA
Citation: Kyung J, Jeon M and Lee I (2022) Recent advances in the chromatin-based mechanism of FLOWERING LOCUS C repression through autonomous pathway genes. Front. Plant Sci. 13:964931. doi: 10.3389/fpls.2022.964931
Edited by:
Ming Luo, South China Botanical Garden (CAS), ChinaReviewed by:
Danhua Jiang, Institute of Genetics and Developmental Biology (CAS), ChinaZhe Wu, Southern University of Science and Technology, China
Copyright © 2022 Kyung, Jeon and Lee. This is an open-access article distributed under the terms of the Creative Commons Attribution License (CC BY). The use, distribution or reproduction in other forums is permitted, provided the original author(s) and the copyright owner(s) are credited and that the original publication in this journal is cited, in accordance with accepted academic practice. No use, distribution or reproduction is permitted which does not comply with these terms.
*Correspondence: Ilha Lee, aWxoYWxlZUBzbnUuYWMua3I=
†These authors have contributed equally to this work