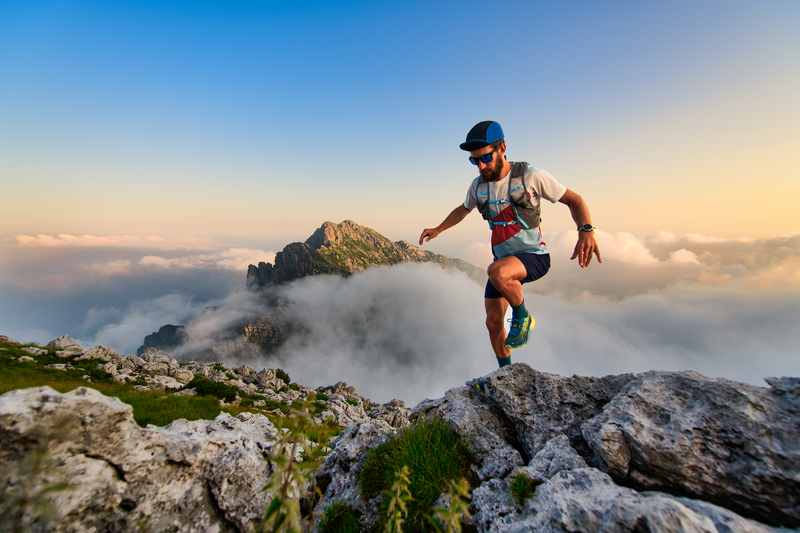
95% of researchers rate our articles as excellent or good
Learn more about the work of our research integrity team to safeguard the quality of each article we publish.
Find out more
REVIEW article
Front. Plant Sci. , 08 September 2022
Sec. Plant Membrane Traffic and Transport
Volume 13 - 2022 | https://doi.org/10.3389/fpls.2022.964059
This article is part of the Research Topic Metal Transport in Plants, Volume II View all 8 articles
Calcium (Ca2+) serves as a ubiquitous second messenger by mediating various signaling pathways and responding to numerous environmental conditions in eukaryotes. Therefore, plant cells have developed complex mechanisms of Ca2+ communication across the membrane, receiving the message from their surroundings and transducing the information into cells and organelles. A wide range of biotic and abiotic stresses cause the increase in [Ca2+]cyt as a result of the Ca2+ influx permitted by membrane-localized Ca2+ permeable cation channels such as CYCLIC NUCLEOTIDE-GATE CHANNELs (CNGCs), and voltage-dependent HYPERPOLARIZATION-ACTIVATED CALCIUM2+ PERMEABLE CHANNELs (HACCs), as well as GLUTAMATE RECEPTOR-LIKE RECEPTORs (GLRs) and TWO-PORE CHANNELs (TPCs). Recently, resistosomes formed by some NUCLEOTIDE-BINDING LEUCINE-RICH REPEAT RECEPTORs (NLRs) are also proposed as a new type of Ca2+ permeable cation channels. On the contrary, some Ca2+ transporting membrane proteins, mainly Ca2+-ATPase and Ca2+/H+ exchangers, are involved in Ca2+ efflux for removal of the excessive [Ca2+]cyt in order to maintain the Ca2+ homeostasis in cells. The Ca2+ efflux mechanisms mediate the wide ranges of cellular activities responding to external and internal stimuli. In this review, we will summarize and discuss the recent discoveries of various membrane proteins involved in Ca2+ influx and efflux which play an essential role in fine-tuning the processing of information for plant responses to abiotic and biotic stresses.
Plants often survive in constantly changing environments that are stressful for their normal growth. To recognize and cope with these stress conditions caused by various biotic and abiotic factors, plants have evolved sophisticated mechanisms to use intracellular signaling molecules as second messengers in alerting cells (Lecourieux et al., 2006; Zhang et al., 2014). The calcium ion (Ca2+) acts as an important secondary signaling molecule and plays critical roles in many biological processes across organisms. Exposure to various sources of abiotic stresses, including heat, metals, salt, wounding, cold, and hypoxia, cause the increase in cytosolic Ca2+ ([Ca2+]cyt). Activation of PATTERN RECOGNITION RECEPTORs (PRRs) by extracellular patterns and NUCLEOTIDE-BINDING LEUCINE-RICH REPEAT (NLR) receptors by cytosolic pathogenic effectors induces PATTERN-TRIGGERED IMMUNITY (PTI) and EFFECTOR-TRIGGERED IMMUNITY (ETI), respectively. Either layer of the immune system also evokes cytosolic Ca2+ signals as a conserved overlapping cell signaling event. The magnitude and pattern of the increases of [Ca2+]cyt vary upon the different stresses (Bose et al., 2011), and the distinct specificities of Ca2+ signals are generally referred to as a Ca2+ signature. Ca2+ channels are involved in the removal of the excessive [Ca2+]cyt and the [Ca2+]cyt removed from cytosol by Ca2+-ATPases is mainly stored in the endoplasmic reticulum (ER).
The advance of genomics and molecular biology has accelerated and enabled genome-wide studies, which have resulted in the identification of various Ca2+ channels and pumps. Recent advances in Ca2+ signal analysis with high-throughput genetics screens has facilitated the validation of the numbers of Ca2+ channels and pumps which are essential contributors to the Ca2+ signature. However, the specific functions of each member of the Ca2+ channels and pumps in stress signaling are only beginning to emerge. In addition, as more research progresses on Ca2+ signaling upon exposure to diverse stresses, additional unanswered questions continued to be revealed. In this review, we discuss the recent studies of Ca2+ channels and pumps which have focused on elucidating their functional roles in plant stresses.
Plant CYCLIC NUCLEOTIDE-GATED CHANNELs (CNGCs), first discovered in barley (Schuurink et al., 1998), are nonspecific Ca2+-permeable cation channels (Sanders et al., 2002; Ali et al., 2007; Jha et al., 2016; Duszyn et al., 2019). They have been predicted as multiple genes in many plant species; Arabidopsis thaliana (Arabidopsis) has 20 CNGCs (Schuurink et al., 1998) and Oryza sativa (rice) has 16 (Nawaz et al., 2014). CNGCs are members of the “P-loop” superfamily of cation channels present in all prokaryotic and eukaryotic cells (Ward et al., 2009). The channel structure is formed by four subunits, each of which has six transmembrane domains including a positively charged transmembrane domain, a pore region (P-loop) between the fifth and sixth domains, and a CYCLIC NUCLEOTIDE BINDING DOMAIN (CNBD; Zelman et al., 2012; Duszyn et al., 2019). The CNBD contains a PHOSPHATE BINDING CASSETTE (PBC) and hinge region with a CALMODULIN-BINDING DOMAIN (CaMBD) at its C-terminus. Most CNGCs have been demonstrated to localize to the plasma membrane (Duszyn et al., 2019). However, a seemingly contradictory findings of mitochondrial, nuclear, and vacuolar membrane localization of CNGCs has been also reported (Duszyn et al., 2019). It is plausible that the contrasting observations of their locations could be caused by extensive formation of different CNGC heterotetramers.
CALMODULIN-BINDING DOMAIN is located in a site overlapping with the C-terminal α-helix of the CNBD, which allows CALMODULIN (CaM) to compete with cyclic nucleotide monophosphate (cGMP/cAMP) as a ligand in allosteric gating of channel conductance (Kaplan et al., 2007). However, in contrast to the previously proposed competitive ligand model, some CNGCs were found to carry more CaM-BINDING SITE (CaMBS) than initially reported (Fischer et al., 2013, 2017; DeFalco et al., 2016a,b). AtCNGC20 binds CaM via “ISOLEUCINE-GLUTAMINE” (IQ) domains adjacent to but not overlapping an α-helix in the CNBD (Fischer et al., 2013). Additionally, AtCNGC12 also contains multiple CaMBSs at cytosolic N and C termini, and apoCaM lacking bound Ca2+ interacts with a conserved IQ domain, while Ca2+/CaM binds additional N- and C-terminal motifs with different affinities (DeFalco et al., 2016a).
Transient but robust changes in intracellular Ca2+ concentration upon pathogen infection have been reported as vital early signaling to induce defense responses (Lecourieux et al., 2006; Zhang et al., 2014). Arabidopsis null mutants, atcngc2 and atcngc4 (also called defense, no death 1 and 2), displayed impaired cell death upon exposure to avirulent bacteria, indicating that AtCNGC2 and AtCNGC4-mediated Ca2+ signaling is critical for plant disease resistance (Yu et al., 1998; Jurkowski et al., 2004) (Figure 1). Additionally, they have nearly identical phenotypes, including constitutively activated defense response, elevated levels of salicylic acid (SA), and increased expression of PATHOGEN RESISTANCE (PR) genes (Clough et al., 2000; Jurkowski et al., 2004). It was reported that AtCNGC2 conducts Ca2+ into cells and the dnd1 mutant (atcngc2) without functional AtCNGC2 lacks this cell membrane Ca2+ current and does not display cell death (Ali et al., 2007). Bimolecular fluorescence complementation analyses in Nicotiana benthamiana support the hypothesis that AtCNGC2 and AtCNGC4 are likely part of the same heterotetrameric channel complex (Chin et al., 2013). Ca2+ accumulation in response to H2O2 were reduced in atcngc2 and atcngc4 mutants (Tian et al., 2019) and PATHOGEN-ASSOCIATED MOLECULAR PATTERN (PAMP)-induced REACTIVE OXYGEN SPECIES (ROS) production was impaired in atcngc2 mutant (Ma et al., 2009), suggesting mutual interplay of Ca2+ channels and ROS signaling in plant immune response. The Arabidopsis gain-of function mutant, CONSTITUTIVE EXPRESSER OF PR GENES 22 (cpr22), was identified as the fusion of two tandemly repeated AtCNGC11 and AtCNGC12 genes (Yoshioka et al., 2001, 2006). The cpr22 mutant shares a similar phenotype with dnd1 and dnd2, displaying elevated levels of SA and increased expression of PR genes, with the exception that is cpr22 induced upon cell death.
Figure 1. Arabidopsis and rice Ca2+ channels and transporters involved in diverse stresses. (A) Schematic summary of Arabidopsis and rice Ca2+ channels involved in abiotic stresses. The Ca2+ channels whose function were validated in Arabidopsis and rice are shown. Cold/freezing, metal, salt, hypoxia, wound, and heat stresses are depicted. When two channels create a complex and function as one unit, they are marked together. (B) Schematic summary of Arabidopsis and rice Ca2+ channels involved in biotic stress and cell death. The calcium channels whose function were validated in Arabidopsis and rice are shown. Transporters belonging to the same family are marked with the same geometric shapes. When two or more channels create a complex and function as one unit, they are marked together.
The rice OsCNGC9 mutant, oscngc9 (cds1, cell death and susceptible to blast 1), displayed a lesion mimic phenotype and impaired resistance to Magnaporthe oryzae (Wang et al., 2019b). Rice receptor-like cytoplasmic kinase 185, inducing PTI, physically interacts with and phosphorylates OsCNGC9 to activate its channel activity and Ca2+ influx (Wang et al., 2019b). The expression patterns of many rice CNGCs were up- or downregulated upon various pathogens, suggesting their functional involvement as defense-related genes. For examples, more than 10 OsCNGC genes were significantly upregulated in rice inoculated with Xanthomonas oryzae pv. oryzae (Xoo) and Pseudomonas fuscovaginae (Nawaz et al., 2014).
Interestingly, the cpr22 mutant of Arabidopsis was temperature sensitive, displaying more intense cell death when grown under low temperature conditions (16°C; Chin et al., 2010; Mosher et al., 2010). The null mutants for atcngc2 (dnd1) and atcngc4 (dnd2) also show temperature-sensitive cell death phenotypes (Yu et al., 1998; Jurkowski et al., 2004; Finka et al., 2012). In addition, atcngc2 mutants displayed enhanced tolerance to heat stress with higher levels of heat response protein that accumulate during the seeding stage (Katano et al., 2018). Genetic evidence identified AtCNGC16 as critical for pollen fertility under stress conditions (Tunc-Ozdemir et al., 2013). Under hot/cold and drought stresses, atcngc16 resulted in a greater than 10-fold stress-dependent reduction in pollen fitness and seed set (Tunc-Ozdemir et al., 2013). Arabidopsis transgenic overexpressing AtCNGC19 or AtCNGC20 displayed salt tolerance and their knockout plants become more sensitive to the salt stress (Oranab et al., 2021). Some AtCNGCs involved in the uptake and transport of heavy metal ions such as Pb2+ and Cd2+. atcngc11, atcngc15, and atcngc19 resulted in reduced Pb2+ and Cd2+ accumulation, while atcngc1 and atcngc13 displayed reduced Pb2+ accumulation but not Cd2+ (Moon et al., 2019). Distinct groups of AtCNGCs appear to have different characteristics regarding their roles in heavy metal uptake. In rice, OsCNGC9 conferred chilling tolerance by regulating cold-induced calcium influx and the activation of cold stress-related genes (Wang et al., 2021). Many OsCNGC genes were also differentially regulated upon exposure to cold stress (Nawaz et al., 2014). For example, OsCNGC6 showed a 192-fold increase, while OsCNGC16 showed a 2-fold decrease in response to cold stress. These findings provide functional evidence that a calcium signaling cascade mediated by CNGCs plays a role in plant acclimatization to diverse abiotic stresses.
Recently, several studies showed that activated NUCLEOTIDE-BINDING LEUCINE-RICH REPEAT RECEPTORs (NLRs) containing a COILED-COIL (CC) domain or a RESISTANCE TO POWDERY MILDEW 8 (RPW8)-like CC domain function as Ca2+ channels to induce cell death and defense responses (Bi et al., 2021; Jacob et al., 2021). CC-NLR and RPW8-like CC-NLR are known as CNL and RNL, respectively. Arabidopsis HOPZ-ACTIVATED RESISTANCE 1 (ZAR1) represents a CNL, which is one of the best structurally characterized sensor NLR recognizing pathogen effectors (Wang et al., 2019a,b). Inactive ZAR1 exists in a preformed complex with RESISTANCE-RELATED KINASE 1 (RKS1; Wang et al., 2015). X. campestris type III secreted effector protein, AvrAC, uridylates receptor-like cytoplasmic kinase PBL2 resulting in PBL2UMP. The PBL2UMP binds to the ZAR1-RKS1 complex, which induces ZAR1 conformational changes to an active pentameric state of ZAR1-RKS1-PBL2UMP complex, called a resistosome (Wang et al., 2019c; Hu et al., 2020). The ZAR1 resistosome, containing an ion-conducting pore constituted by the N-terminal helix α1 domains, penetrates the plasma membrane and forms a calcium-permeable cation channel (Bi et al., 2021). The calcium influx further leads to accumulation of reactive oxygen species, activation of cell death, and defense response. Therefore, ZAR1 functions as both a sensor of pathogens and an executor of immune response as a functional Ca2+ channel (Wang et al., 2019c; Bi et al., 2021; Wan and He, 2021).
Another type of sensor NLR proteins containing a TOLL/INTERLEUKIN-1 RECEPTOR (TIR) domain, TNLs, have been confirmed to possess oligomerization-dependent NICOTINAMIDE ADENINE DINUCLEOTIDE (NAD) HYDROLASE (NADase) activity within their TIR domain (Horsefield et al., 2019; Wan et al., 2019). Tetramerization in the TIR domain of Arabidopsis TNL, RECOGNITION OF PERONOSPORA PARASITICA 1 (RPP1), creates the active site for catalysis after RPP1 recognizes and directly binds Hyaloperonospora arabidopsidis effector, A. thaliana RECOGNIZED 1 (ATR1; Ma et al., 2020; Martin et al., 2020). The oligomerization-dependent NADase activity was also proposed in Nicotiana benthamiana (tobacco) TNL, RECOGNITION OF XANTHOMONAS OUTER PROTEIN Q 1 (XopQ 1; ROQ1), after it interacts with Xanthomonas effector protein, XopQ 1 (Martin et al., 2020). NAD+ and its cleavage products are known to perform many essential cellular functions, including immune signaling and the activation of calcium channels (Lee and Zhao, 2019; Bayless and Nishimura, 2020). In Arabidopsis, TNLs require the redundant helper NLRs which transduce signals downstream from sensor NLRs (Koster et al., 2022). Helper NLRs, ACTIVATED DISEASE RESISTANCE 1 (ADR1), and N REQUIREMENT GENE 1.1 (NRG1.1) subfamilies, belong to the RNL family (Jubic et al., 2019). The mechanisms of how the helper NLRs, ADR1, and NRG1 are activated by TIR domain NADase activity is an active area of research. Auto-active AtNRG1.1 D485V and wild-type AtADR1 induce autonomous cell death in N. benthamiana, functioning as calcium permeable cation channels. They oligomerize, enrich in PM, and induce Ca2+ influx to cause cell death in N. benthamiana and human HeLa cells, and the cell death activity has been shown to be inhibited by Ca2+ channel blockers, LaCl3 and GdCl3 (Jacob et al., 2021). Therefore, it was proposed that TNL activation induces RNL-dependent Ca2+ influx, to initiate cell death and, likely, immune responses (Jacob et al., 2021).
So far, there is no direct evidence that the Ca2+-permeable cation channels consisting of CNLs and RNLs are involved in abiotic stresses. However, the Ca2+ influx caused by NLR activation induces massive transcriptional reprogramming toward abiotic stress response, including apetala2/ethylene responsive factor, basic helix–loop–helix, MYB, WRKY, basic leucine zipper, and CaM-binding transcription activator families (Jacob et al., 2018; Ng et al., 2018). Further validation should be undertaken to determine the possible overlapping of NLR-mediated Ca2+ influx in transcriptional responses between pathogen perception and abiotic stress.
Mammalian IONOTROPIC GLUTAMATE RECEPTORs (iGluRs) are known to mediate the majority of excitatory neurotransmission in the central nervous system (Wisden and Seeburg, 1993). Functionally, iGluRs are GLUTAMATE (Glu)-gated cation channels that are selective for Na+, K+, and Ca2+ ions. Plant GLUTAMATE RECEPTOR-LIKE RECEPTORs (GLRs) have a high similarity to their animal counterparts in respect to their channel structure, ligand binding domain, and amino acid sequence (Lacombe et al., 2001). Arabidopsis has 20 GLRs and most plants possess more than 10 GLRs in their genome (Lacombe et al., 2001; Sanders et al., 2002; Kwaaitaal et al., 2011; Tapken et al., 2013; Demidchik et al., 2018; Qiu et al., 2020). In contrast to the ligand specificity of mammalian iGluRs that are mainly gated by Glu, Arabidopsis GLRs, AtGLR1.4, AtGLR3.3, and AtGLR3.4, are gated by a broad spectrum of amino acids, at least 12 out of 20 proteinogenic amino acids and the triple reduced glutathione (Qi et al., 2006; Tapken et al., 2013; Forde and Roberts, 2014). It is hypothesized that this broader ligand specificity of GLRs is caused by differences in the sequence of their pore regions (Forde and Roberts, 2014). Heterologous expression in Xenopus oocytes revealed that AtGLR1.4, like mammalian iGluRs, functioned as a nonselective Ca2+-permeable cation channel (Tapken et al., 2013), but that AtGLR3.4 was highly selective for Ca2+ (Vincill et al., 2012). Biological functions of plant GLRs have been reported in many aspects including biotic and abiotic stress responses (Meyerhoff et al., 2005; Kang et al., 2006; Kwaaitaal et al., 2011; Vincill et al., 2012; Li et al., 2013; Forde and Roberts, 2014).
Possible involvement of plant GLRs in defense responses was suggested in several early reports. Overexpression of Raphanus Sativus (radish) GLR in Arabidopsis triggered greater Ca2+ influx after glutamate treatment and conferred enhanced resistance to a necrotic fungal pathogen, Botrytis cinerea (Kang et al., 2006). Later, using the experiments with antagonists of mammalian iGluRs, initiation of defense responses upon several PAMPs, FLAGELLIN 22 (flg22), ELONGATION FACTOR TU 18 (elf18), and fungal chitin, involved apoplastic Ca2+ influx via iGluR-like channels, suggesting that GLRs are related to the induction of defense response after PAMP recognition (Kwaaitaal et al., 2011). In addition, loss-of-function atglr3.3 mutants showed decreased expression of defense-related genes and increased susceptibility to the bacterial pathogen Pseudomonas syringae pv tomato DC3000 (Li et al., 2013). Genetic experiments performed with different atglr T-DNA mutants, atglr3.1, atglr3.3, atglr3.4, atglr3.5, and atglr3.7, concluded that the atglr3.3 mutant was more susceptible to H. arabidopsidis and treatment of GLR antagonist compromised resistance (Manzoor et al., 2013).
AtGLR3.4 and AtGLR3.7 are involved in the regulation of seed germination with NaCl stress (Cheng et al., 2016, 2018). Recently, AtGLR3.7 was shown to be phosphorylated by a CALCIUM-DEPENDENT PROTEIN KINASE (CDPK), and its interaction with 14–3-3 proteins participates in the regulation of cytosolic Ca2+ concentration under salt stress (Wang et al., 2019e). The perception of cold stress by the plasma membrane can activate Ca2+ channels and triggers Ca2+-mediated signaling pathways to respond and adapt to cold stress (Ding et al., 2019; Qiu et al., 2020). The expression of AtGLR3.4 was stimulated by exposure to cold, touch, and osmotic stress in an ABA-independent manner, but dependent upon Ca2+ (Meyerhoff et al., 2005). An increase in [Ca2+]cyt triggered by cold treatment was blocked by GLRs antagonists, 6,7-dinitroquinoxaline-2,3-dione and 6-cyano-7-nitroquinoxaline-2,3-dione, suggesting that AtGLR3.4 plays a very important role in the Ca2+-mediated signaling transmission of cold stress. Overexpression of AtGLR1.2 or AtGLR1.3 improved the tolerance of mutants to cold stress by synthesizing endogenous jasmonic acid (JA) and their mutants became more sensitive to cold the stress (Zheng et al., 2018).
In addition to the apoplast, internal cellular compartments such as the vacuole contribute to the rise in [Ca2+]cyt (Xu et al., 2022). In the vacuolar membrane, TWO-PORE CHANNEL (TPC) functions as a nonselective cation channel co-regulated by voltage and Ca2+, and generates a slow vacuolar current (Furuichi et al., 2001; Ye et al., 2021). Genomic analysis indicated that there is a single TPC1 gene in Arabidopsis (Peiter et al., 2005) and rice. Arabidopsis TPC1 is the well-studied plant TPC and is activated by the membrane depolarization and [Ca2+]cyt but inhibited by [Ca2+]vac (Dadacz-Narloch et al., 2011; Schulze et al., 2011). AtTPC1 forms a homodimer, where each subunit consists of two homologous six-transmembrane segment domains, therefore equivalent to a classical voltage-gated ion channel with four voltage-sensing domains and one pore domain (Guo et al., 2016; Ye et al., 2021). There are two EF-hand motifs in the cytosolic linker part of AtTPC1; Ca2+ binding at EF hand 1 appears to play a structural role and Ca2+ binding at EF hand 2 is central for Ca2+ activation (Schulze et al., 2011; Guo et al., 2016).
A few studies provided functional evidence to demonstrate that plant TPCs are implicated in plant immunity. Rice TPC1 (OsTPC1), localized at vacuolar membrane, functions as a Ca2+-permeable cation channel involved in the regulation of growth and development (Kurusu et al., 2004, 2012). A later study reported that OsTPC1 plays a positive role in elicitor-induced defense gene expression, oxidative burst, MAP kinase activation, and cell death (Kurusu et al., 2005). In Arabidopsis, the AtTPC1 gain-of-function mutant fou2 was found to possess enhanced resistance to B. cinerea, accompanied with increased JA accumulation (Bonaventure et al., 2007). However, in a later study, Ca2+ responses in AtTPC1-overexpressing Arabidopsis and attpc1-2 knockout mutants did not display any alteration in the stimulus-induced Ca2+ signals including abiotic stresses and PAMPs, elf18 and flg22 (Ranf et al., 2008). Therefore, it is possible that AtTPC1 is not a major player in the defense response, at least in Arabidopsis (Moeder et al., 2019; Xu et al., 2022). AtTPC1 is also known to be involved in the systemic spread of both the wound- and NaCl-driven systemic [Ca2+]cyt increases (Choi et al., 2014, 2017; Kiep et al., 2015). In the recently proposed systemic communication pathways mediated by Ca2+ and ROS (Johns et al., 2021), AtTPC1 was found to be involved in the release of Ca2+from the vacuole, functioning as a Ca2+ amplifier for the initial increases in [Ca2+]cyt. The resulting elevated [Ca2+]cyt could then further trigger RESPIRATORY BURST OXIDASE HOMOLOG (RBOH) activation for additional production of apoplastic ROS (Choi et al., 2014). A feed-forward loop between Ca2+ and ROS results in the propagation of the signal from cell to cell (Johns et al., 2021), suggesting the potential contribution of AtTPC1 to whole-plant stress tolerance.
The particular calcium conductance mediated by DEPOLARIZATION-ACTIVATED Ca2+ CHANNELs (DACCs) have been found in various plant species including Arabidopsis, tobacco, and Zea mays (maize), although DACCs in plants have not been associated with any gene yet (Demidchik et al., 2018). Several pharmaceutical approaches using the DACC channel blockers such as nifedipine showed that abiotic stresses result in a depolarization of the plasma membranes by DACCs, followed by an increase in [Ca2+]cyt (Crotty et al., 1996; Thion et al., 1998; Lhuissier et al., 2001; Miedema et al., 2001, 2008; Okazaki et al., 2002; Carpaneto et al., 2007; McAinsh and Pittman, 2009; White, 2009; Seifikalhor et al., 2019). DACCs are more responsive to a short and transient Ca2+ influx triggered by exposure to acute stress stimuli such as cold and cadmium stresses (Carpaneto et al., 2007; White, 2009; Wilkins et al., 2016).
In plants, HYPERPOLARIZATION-ACTIVATED Ca2+ CHANNELs (HACCs) were first found in Solanum lycopersicum (tomato) responding to fungal infection (Gelli and Blumwald, 1997; Manohar et al., 2011b). A primary role of HACCs is to a sustain Ca2+ influx such as guard cell signaling and root hair elongation (Miedema et al., 2001, 2008; Mortimer et al., 2008; Swarbreck et al., 2013; Tang and Luan, 2017). In guard cells, HACC activities were confirmed to be activated by ABA and ROS subsequent to an increase in [Ca2+]cyt. Additionally, HACC conductance is further stimulated by extracellular ATP in guard cells and pollen plasma membranes (Demidchik et al., 2009; Wu et al., 2018; Wang et al., 2019d). In the root epidermis, HACC functions downstream of the plasma membrane RBOHC and contributes to net [Ca2+]cyt influx in the root zone (Demidchik et al., 2009, 2011; Shang et al., 2009; Wang et al., 2019d).
Annexins are membrane binding proteins that can form Ca2+-permeable conductance in vitro. Plasma membrane-localized Arabidopsis ANNEXIN1 (AtANN1) mediated Ca2+ influx in root epidermal cells, which is activated by extracellular •OH and H2O2 (Demidchik et al., 2010; Richards et al., 2014). In addition, the AtANN1 mutant, atann1 was found to lack root hairs and •OH-activated Ca2+- and K+-permeable conductance (Demidchik et al., 2010). Furthermore, the atann1 was also found to lack an induction of salt-induced transcripts. Salt stress and cell death often leads to an increase in extracellular •OH. Collectively, these findings support the concept that AtANN1 functions as a Ca2+-permeable transporter link between stress-induced ROS (•OH) and [Ca2+]cyt (Lee et al., 2004; Shang et al., 2009; Laohavisit et al., 2012, 2013; Richards et al., 2014; Kurusu et al., 2015; Wilkins et al., 2016). In addition, AtANN1 would function together with AtCNGC5, AtCNGC6, and AtCNGC9 for root hair growth (Tan et al., 2020), although further experimental evidence is still needed.
Plant cells sense mechanical stimuli in nature, such as touch, gravity, and the stretching of membranes. MECHANOSENSITIVE (MS) ion channels that serve to sense and respond to changes in membrane tension have been known to directly couple mechanical stimuli to ion flux (Peyronnet et al., 2014; Basu and Haswell, 2017). There are a few plant MS channel families that have been reported as Ca2+ channels (Basu and Haswell, 2017). One of them is ECHANOSENSITIVE CHANNEL OF SMALL CONDUCTANCE (MSCS)-LIKE CHANNELs (MSLs). It has been predicted that there are 10 and five MSL genes in Arabidopsis and rice, respectively (Haswell, 2007; Saddhe and Kumar, 2015). Arabidopsis MSL10 was shown to have a plant-specific N-terminal domain which is capable of inducing cell death in a phosphorylation-dependent manner (Veley et al., 2014). A gain-of-function mutation in AtMSL10 triggered cell death and wound-induced hyperaccumulation of JA (Zou et al., 2016). Recently, it has been reported that AtMSL10 functions as a phospho-regulated membrane-based sensor that connects the perception of cell swelling to a downstream signaling cascade and cell death (Basu and Haswell, 2020). Finally, it was revealed that mechanical signals generated during pathogenic invasion are exploited by AtMSL10 (Basu et al., 2021). Overexpression and gain-of-function mutants of AtMSL10 exhibited reduced susceptibility to infection by P. syringae pv. tomato DC3000 and showed an accelerated induction of Arabidopsis PATHOGENESIS-RELATED PROTEIN 1 (AtPR1) expression compared to wild-type plants, indicating that mechanical signals are important.
Another MS channel family functioning as a Ca2+ channel is a MID1-COMPLEMENTING ACTIVITY (MCA), exhibiting 10% identity to yeast MATING-INDUCED DEATH 1 (Mid1). AtMCA1 and AtMCA2 have been identified in Arabidopsis via functional complementation of the yeast mutant mid1 (Yamanaka et al., 2010). MCA proteins share certain structural features, an EF hand-like and a CC motif in the N-terminal region, and two to four putative transmembrane domains and a Cys-rich PLAC8 domain of unknown function in the C-terminal region (Galaviz-Hernandez et al., 2003; Yamanaka et al., 2010; Nishii et al., 2021). In a recent study, the cold-induced [Ca2+]cyt increase in atmca1 and atmca2 mutants was markedly lower than what occurred in wild-types. Importantly, the atmca1/2 double mutant displayed increased cold sensitivity, suggesting that AtMCA1 and AtMCA2 are functionally involved in a cold-induced elevation of [Ca2+]cyt (Mori et al., 2018). In addition, MCAs have been reported to function in diverse cellular responses to different stresses, including osmotic sensing and cell wall damage responses (Nakagawa et al., 2007; Denness et al., 2011; Moeder et al., 2019).
HYPEROSMOLALITY-GATED CALCIUM-PERMEABLE CHANNELs (OSCAs) were first identified as an osmosensors in Arabidopsis (Yuan et al., 2014). After studying mutants with a low intracellular free calcium concentration under high osmotic stress, OSCA was determined to function in the perception of extracellular changes to trigger hyperosmolality-induced [Ca2+]cyt increases. Arabidopsis contains 15 AtOSCAs which possess 9 transmembrane domains, including one cleavable transmembrane domain (Demidchik et al., 2018). Predictive analysis from the rice (O. sativa L. Japonica) genomic database revealed a total of 11 OsOSCAs genes (Li et al., 2015). Due to the complex regulation of Ca2+ signaling and homeostasis, the potential involvement of OSCA in specific aspects of defense regulation has been suggested (Moeder et al., 2019). A recent study revealed that AtOSCA1.3 is rapidly phosphorylated upon perception of PAMP flg22, controlling stomatal closure during immune signaling (Thor, 2019). AtOSCA1.1 is reported to be involved in sensing extracellular changes which results in a triggering of increases in hyperosmolality-induced [Ca2+]cyt (Yuan et al., 2014).
One of the major membrane protein families which are responsible for Ca2+ efflux, Ca2+-ATPases, is high affinity (Km = 0.1–2.0 μM) and low capacity Ca2+ transporter (Garcia Bossi et al., 2020). Its primary role is the termination of Ca2+-mediated signaling. The P-type Ca2+-ATPases are directly activated by ATP and are found in animal, fungi, as well as plants (Geisler et al., 2000a). A primary role of plant Ca2+-ATPases is to maintain ion homeostasis through the pumping of [Ca2+]cyt out of the cytosol. Plant Ca2+-ATPases belong to either the P2a-type Ca2+-ATPases (ECA) or P2b-type AUTOINHIBITED CA2+-ATPASEs (ACAs) which have 10 transmembrane domains (Demidchik et al., 2018; Demidchik and Shabala, 2018). ECAs are mostly localized on endomembranes, but ACAs carrying an N-terminal CaM-regulated autoinhibitory domain have been confirmed to localize on plasma membranes or endomembranes (Geisler et al., 2000b; Huda et al., 2013c). There are 10 ACAs and 4 ECAs in Arabidopsis and 11 ACAs and 3 ECAs in rice (Sze et al., 2000; Geisler et al., 2000b; Baxter et al., 2003; Huda et al., 2013c,d). ACAs are known to exclusively transport Ca2+ but ECAs, however, are capable of transporting Ca2+ and Mn2+ (Baxter et al., 2003). ECAs are similar to mammal SACRO/ENDOPLASMIC RETICULUM CACLIUM ATPASE (SERCA), which are known as transporters of Ca2+, Mn2+, and Zn2+ (Carafoli and Brini, 2000; Wu et al., 2002). Despite this similarity, mammal ECAs are regulated by phospholamban but plant ECAs do not have phospholamban-binding sites (Baxter et al., 2003). ACAs that are similar to mammalian CaM-stimulated ATPases, and plant ACAs were confirmed to be localized on the multiple cellular position different from only plasma membrane-localized animal ACAs. Specifically, AtACA2 is localized on the ER (Harper et al., 1998), AtACA4 is vacuolar (Geisler et al., 2000b; Baxter et al., 2003), and AtACA8 resides in the plasma membrane (Bonza et al., 2000). Genome structure analysis of ACAs determined that they are divided into four subfamily members in plants (Carafoli and Brini, 2000; Sze et al., 2000; Wu et al., 2002). Arabidopsis AtECA1, AtECA2, AtECA4 and rice OsECA1, OsECA2, and OsECA4 belong to subfamily I; and OsECA3 and AtECA3 belong to subfamily II (Baxter et al., 2003).
Abiotic and biotic stress have been known to increase [Ca2+]cyt, which is subsequently followed by the accumulation of ROS, and the excessive [Ca2+]cyt is eventually diminished from cytosol (Beffagna et al., 2005; Lecourieux et al., 2006; Mortimer et al., 2008; Laohavisit et al., 2013; Swarbreck et al., 2013; Shabala et al., 2016; Wilkins et al., 2016; Ahmadi et al., 2018; Ahmed et al., 2018; Demidchik et al., 2018; Demidchik and Shabala, 2018). Many reports have documented the contributions of plant Ca2+-ATPases for the removal of excessive [Ca2+]cyt from the cytosol. The double mutant of vacuolar localized AtACA4 and AtACA11 results in a high frequency of cell death that is suppressed when plants are grown in the presence of more than 15 mM anions by decreasing SA. AtACA8 and AtACA10 were confirmed to function as positive regulators for a PAMP-triggered Ca2+ burst and a double knockout of AtACA4 and AtACA11, ataca4/11 displayed SA-dependent cell death-like lesions. Similarly, tobacco plants which lacked NbCA1 exhibited enhanced cell death in response to the tobacco mosaic virus (Nemchinov et al., 2008; Boursiac et al., 2010; Huda et al., 2013c). The vacuolar ACAs have been shown to mediate a SA-dependent cell death response in plants (Boursiac et al., 2010). An ER-localized AtACA1, AtACA2, and AtACA7 triple mutant, ataca1/2/7, was confirmed to have reduced pollen fertility and smaller rosette size. These genes were suggested to be functionally redundant since each of the three genes could complement the defective phenotype. Similar to the like ataca4/11 mutant, the ataca1/2/7 triple mutant also displays cell death but to a lesser extent. In addition, the expression of NahG encoding salicylate hydroxylase was found to attenuate the cell death of the ataca1/2/7 triple mutant (Resentini et al., 2021). AtACA8, 10, 12, and 13 constitute a complex with an Arabidopsis PRR redundantly and regulate plant immune response via the removal of excessive Ca2+ from the cytosol, and subsequently control the PAMP-triggered Ca2+ signaling (Frei Dit Frey et al., 2012; Yu et al., 2018; Tian et al., 2020). The elevations of [Ca2+]cyt during cell death are essential for defense response, including oxidative burst (Atkinson et al., 1990; Levine et al., 1996; Bose et al., 2011). Cell death has been shown to be inhibited by Ca2+ channel blockers via blocking the elevation of Ca2+ influx resultant from the initiation of cell death. After mimicking cell death, [Ca2+]cyt levels were found to dramatically and rapidly decrease, resulting in the prevention of any damage from oxidative stress. Ca2+-ATPases are known to partially mediate this aforementioned Ca2+ efflux (Atkinson et al., 1990; Levine et al., 1996; Nemchinov et al., 2008; Boursiac et al., 2010; Pottosin et al., 2014). In tobacco, Ca2+-ATPases are involved in the removal of excessive Ca2+ that was caused by Potato Virus X (PVX)-induced acquired resistance (Shabala et al., 2011b). Ca2+ efflux that mediated by Ca2+-ATPases contributed to the process of PVX-induced resistance to oxidative stress in tobacco (Shabala et al., 2011a). These reports support that Ca2+-ATPases play important roles in abiotic and biotic stress through adjusting [Ca2+]cyt levels.
A lack of AtACA2 and AtACA4 resulted in an increase in salt sensitivity but their overexpression led to salt tolerance in yeast Saccharomyces cerevisiae. The function of AtACA2 was shown to restore [Ca2+]cyt that was induced by salt stress in yeast (Schiott et al., 2004; Anil et al., 2008; Seifikalhor et al., 2019). In plants, the expression of AtACA8 and AtACA9, but not AtACA10, were confirmed to be up-regulated by ABA. When exposed to cold stress, contrasting expression patterns were observed with AtACA8 showing increased expression to cold stress, whereas, AtACA10 expression was decreased. The loss-of-function of AtACA8 resulted in a higher Ca2+ accumulation in roots during hypoxia (Schiott et al., 2004; Cerana et al., 2006). The N-terminal modification of AtACA4 was found to be associated with increased salt tolerance (Geisler et al., 2000b). Collectively, these findings suggest that ACAs induced by abiotic stresses are mainly involved in the responses to abiotic stresses via the removal of [Ca2+]cyt which results from these abiotic stresses. AtECA4-mediated recycling of proteins from the endosome to the plasma membrane plays a key role in salt-induced ROS accumulation independent from PAMP flg22-induced ROS accumulation (Lee et al., 2021). ER-localized AtECA1 restored yeast growth on a high Mn2+ and Zn2+ background (Sze et al., 2000). Additionally, AtECA1 also controlled plant growth in Ca2+ deficient or Mn2+ toxic conditions (Wu et al., 2002). Multiple rice ACAs are involved in abiotic stress, including OsACA4 in salt stress (Yamada et al., 2014). In tobacco, overexpression of OsACA6 led to increased abiotic stress tolerance toward drought, cold, Cd, and UV (Huda et al., 2013a; Kamrul Huda et al., 2014; Shukla et al., 2014). The promoter sequence of rice plasma membrane-localized Ca2+-ATPase has several cis-elements which respond to various abiotic stresses including ABA, light, wounding, dehydration, cold and heat (Huda et al., 2013b). The increased Ca2+ fluxes and ROS caused by hyperosmotic and hypoosmotic stress were attenuated by eosin yellow which is a selective inhibitor of plasma membrane Ca2+-ATPases (Beffagna et al., 2005).
Overexpression of Medicago sativa (Alfalfa) ACAs, MsRCI2s resulted in increased tolerance to alkaline and salt stress (Li et al., 2021). Expression of a plasma membrane-localized SOYBEAN CA2+-ATPASE (SCA1) was rapidly induced by NaCl or the fungal elicitor treatment, but not by KCl or mannitol treatment. The regulation of SCA1 activity was shown to be Ca2+-dependent and CaM-binding-dependent (Chung et al., 2000). In chilling-sensitive wheat, a 2°C chilling treatment led to an increase in the [Ca2+]cyt level and a decrease in Ca2+-ATPase activity. On the other hand, in chilling tolerant winter wheat, however, Ca2+ level was restored and Ca2+-ATPase activity was maintained (Jian et al., 1999). Expression of Physcomitrella patens (moss) ACA, PCA1, is localized within small vacuoles, and was shown to be up-regulated by drought, salt, and abscisic acid. A knockout of PCA1 resulted in alteration of the responses to [Ca2+]cyt, which in turn altered the expression of stress-induced genes and interfered with the tolerance response to abiotic stress (Qudeimat et al., 2008). Barley varieties that are tolerant to Al are characterized by increased Ca2+-ATPase activities (Ahmed et al., 2018). Boron-starvation led to an increase in root hair growth, and up-regulated expression of ACAs (AtACA10, AtACA11, AtACA12, and AtACA13) in Arabidopsis roots (Gonzalez-Fontes et al., 2013, 2014; Quiles-Pando et al., 2013; Wilkins et al., 2016). As a consequence of boron deficiency, Ca2+-ATPase activity was induced by NO and functioned to alleviate Fe2+ deficiency in peanut (Song et al., 2018).
CALCIUM/CATION ANTIPORTERs (CaCAs), especially CA2+/H+ EXCHANGERs (CAXs), largely contribute to Ca2+ homeostasis in plant cells and they are activated by the transport of counter cations. Three subfamilies of CaCAs, NA+/CA2+ EXCHANGERs (NCXs), CATION/Ca2+ EXCHANGERs (CCXs) and CAX, were confirmed to exist in land plants (Bose et al., 2011; Emery et al., 2012; Mao et al., 2021). Until recently, it was thought that NCXs existed only in animal cells. However, a bioinformatic genome analysis has suggested that a putative NCX gene exists within the plant genome. Plant CAXs are comprised of 11 transmembrane domains, an acidic motif between sixth and seventh transmembrane domains, a N-terminal autoinhibitory domain, and a Ca2+-specific binding domain (Shigaki and Hirschi, 2006; Manohar et al., 2011a; Demidchik et al., 2018). In Arabidopsis, an NCX-LIKE (AtNCL) protein was confirmed to have Ca2+ binding activity and its expression was upregulated by salt stress. Furthermore, the mutant plant of AtNCL exhibited reduced sensitivity to salt stress (Shigaki and Hirschi, 2006). This finding suggested that AtNCL mediated the Ca2+ homeostasis in the presence of high levels of available Na+ (Wang et al., 2012). The functions of plant CCXs are not well known at this time. Recently, an Arabidopsis AtCCX1 was revealed to be involved in leaf senescence and exhibits an affinity to Ca2+ (Conn et al., 2011; Li et al., 2016). Although AtCCX3, AtCCX4, and AtCCX5 were suggested to have affinity to K+, Na+, or Mn2+, there is no evidence that they have an affinity to Ca2+ (Morris et al., 2008; Zhang et al., 2011; Corso et al., 2018). The ER-localized Arabidopsis CCX2, AtCCX2, has been shown to adjust osmotic stress via a direct control of Ca2+ fluxes between the cytosol and the ER. AtCCX2 knockout plants showed a decreased [Ca2+]cyt and increased ER Ca2+, and exhibited reduced tolerance to osmotic stress (Corso et al., 2018).
CA2+/H+ EXCHANGERs are low affinity (Km = 10–15 μM) and high capacity Ca2+ transport. CAXs restore [Ca2+]cyt levels via removal of [Ca2+]cyt (Sze et al., 2000; Bose et al., 2011). Arabidopsis CAX3, AtCAX3 expression was induced by PAMP flg22 and mechanical wounding (Ecker and Davis, 1987; Hocking et al., 2017). Co-expression of AtCAX1/AtCAX3 was found in mesophyll cells during the defense response and they interact in a homo and hetero combination. Many biotic stress resistance related proteins, including AtPR1 and AtPR2, were up-regulated in the double mutant of AtCAX1 and AtCAX3, atcax1/3. These data suggested that the AtCAX1/AtCAX3 heterodimeric complex plays a role in defense response. Additionally, the AtCAX1/AtCAX3 complex is functionally involved in controlling the opening of stomata for maintaining the Ca2+ response and downstream signaling (Hocking et al., 2017). The atcax1/3 mutant exhibited cell death at leaf tips and was compensated by lower external Ca2+ availability like atcncg2/4. It was suggested that CAXs function in plant disease resistance through the sequestration of Ca2+ to vacuoles (Shigaki et al., 2002; Cheng et al., 2003, 2005; Manohar et al., 2011b; Tian et al., 2020).
Some CAXs are known to be involved in transporting trace metal ions such as Mn2+, Cd2+ as well as Ca2+. CAXs broadly contribute to abiotic stress tolerance in plants, Specifically, in halophytic plants, CAXs mediate salt and heavy metal tolerance (Pittman and Hirschi, 2016). In mesophyll cells, CAXs are involved in stomatal conductance along with Ca2+ homeostasis. When the activities of CAXs were altered, the sensitivity of plants to metal stresses was altered (Bradshaw, 2005; Conn et al., 2011; Pittman and Hirschi, 2016). The double mutant of AtCAX1 and AtCAX3, atcax1/3, showed the alteration of Ca2+ homeostasis, sensitivities to metals and tolerance to phosphate deficiency (Liu et al., 2018). CAXs are involved in not only salt stress, but also other abiotic stresses such as drought, cold, and heat stress. In accordance with these observations, their overexpression in various plant species resulted in an increased tolerance to abiotic stresses as well (Cheng et al., 2003, 2005; Zhao et al., 2009; Shigaki et al., 2010; Conn et al., 2011; Wu et al., 2011). AtCAX1, AtCAX3, Glycine max (soybean) GmCAX1, rice OsCAX4, Malus x domestica (apple) MdCAXs and Gossypium hirsutum (cotton) GhCAX1 were transcriptionally regulated by abiotic stress and are known to function in process of cold acclimation with key roles related to salt, drought and freezing tolerance (Hirschi, 1999; Cheng et al., 2005; Kim et al., 2005; Manohar et al., 2011b; Han et al., 2012; Kamiya et al., 2012; Xu et al., 2013; Mao et al., 2021). The knockout of AtCAX3 led to an increase in salt tolerance, but the AtCAX1 knockout plants exhibited the freezing tolerance. Taken together, these observations suggest that there may be the different CAXs that are in charge of each stress response for maintaining [Ca2+]cyt homeostasis (Bradshaw, 2005; Zhao et al., 2008, 2009; Bose et al., 2011). AtCAX11 performs critical roles for maintaining [Ca2+]cyt homeostasis during hypoxia in roots and resulted in tolerance to water logging stress (Wang et al., 2016). The expression of tonoplast localized OsCCX2 increased under drought and salt stress, and was up-regulated by ABA but downregulated by Ca2+ deficiency. OsCCX2 in Ca2+ sensitive yeast mutant line resulted in the tolerance to zinc, iron and cobalt stress conditions (Yadav et al., 2015). In addition, Arabidopsis CAXs were revealed to be functionally involved in the response to boron deficiency (Quiles-Pando et al., 2013; Gonzalez-Fontes et al., 2014). AtCAX3 contributes to the sequestration of the boron-deficiency induced [Ca2+]cyt to the vacuole (Gonzalez-Fontes et al., 2013; Quiles-Pando et al., 2013).
Heavy metal, cadmium-induced [Ca2+]cyt was predominantly mediated by CAXs. AtCAX1, AtCAX3, and AtCAX4 contribute to Cd transport in a Cd stress condition and led to Cd tolerance (Wu et al., 2011, 2020; Baliardini et al., 2015; Ahmadi et al., 2018; Modareszadeh et al., 2021). However, these results still need to be confirmed to better understand whether they resulted from direct transport of metals by CAXs ions or by an alteration of a stress tolerance pathway by CAXs via maintaining [Ca2+]cyt homeostasis.
In this review, recent findings pertaining to involvement of Ca2+ transport in biotic and abiotic stress was summarized, with a focus on Ca2+ influx and efflux. Overall, up-to-date research on Ca2+ signaling has led to significant progress, which have been enabled by cutting edge genomic tools. With the development of Ca2+ detection methods and recording techniques, long-awaited breakthroughs on Ca2+ signaling in plants will lead to advances in the effective management of plant stress.
Therefore, how these channels and pumps work together or independently to encode the specific Ca2+ signatures to various stress should be intensively explored. Another challenge to be addressed involves the identification and causal interconnection of Ca2+ channels for influx and counteracting Ca2+ pumps for efflux in relation to stress response. Restoration of the basal [Ca2+]cyt levels is essential to terminate Ca2+ signaling and to reload Ca2+ stores. Therefore, sophisticated and coordinated regulation of Ca2+ channels and pumps should be addressed. An arising important question is how the limited numbers of Ca2+ channels encode a large number of Ca2+ signatures in plant cells. Many Ca2+ channel families have extended numbers of members and it is possible that they may also form hetero multimers. Studies on Arabidopsis CNGC and GLR members have provided the evidence that these channels interact with each other as a subunit and assemble into heteromeric functional Ca2+ channels. Therefore, it could be a general rule to generate a large repertoire of Ca2+ channels with heteromeric subunits in plants, encoding a large number of Ca2+ signatures involved in a wide array of biotic and abiotic stress processes.
C-JP and RS wrote the manuscript and drew the diagram. All authors contributed to the article and approved the submitted version.
This work was supported by a National Research Foundation of Korea (NRF) grant funded by the Korea government (MSIT) (NRF-2020R1A2C1007778 to C-JP) and a RIKEN CSRS Innovative Plant Biotechnology Collaboration Project (RIKEN CSRS to RS).
The authors declare that the research was conducted in the absence of any commercial or financial relationships that could be construed as a potential conflict of interest.
All claims expressed in this article are solely those of the authors and do not necessarily represent those of their affiliated organizations, or those of the publisher, the editors and the reviewers. Any product that may be evaluated in this article, or claim that may be made by its manufacturer, is not guaranteed or endorsed by the publisher.
Ahmadi, H., Corso, M., Weber, M., Verbruggen, N., and Clemens, S. (2018). CAX1 suppresses cd-induced generation of reactive oxygen species in Arabidopsis halleri. Plant Cell Environ. 41, 2435–2448. doi: 10.1111/pce.13362
Ahmed, I. M., Nadira, U. A., Qiu, C. W., Cao, F. B., Zhang, G. P., Holford, P., et al. (2018). Tolerance to drought, low pH and Al combined stress in Tibetan wild barley is associated with improvement of ATPase and modulation of antioxidant defense system. Int. J. Mol. Sci. 19:3553. doi: 10.3390/ijms19113553
Ali, R., Ma, W., Lemtiri-Chlieh, F., Tsaltas, D., Leng, Q., von Bodman, S., et al. (2007). Death don’t have no mercy and neither does calcium: Arabidopsis CYCLIC NUCLEOTIDE GATED CHANNEL2 and innate immunity. Plant Cell 19, 1081–1095. doi: 10.1105/tpc.106.045096
Anil, V. S., Rajkumar, P., Kumar, P., and Mathew, M. K. (2008). A plant Ca2+ pump, ACA2, relieves salt hypersensitivity in yeast. Modulation of cytosolic calcium signature and activation of adaptive Na+ homeostasis. J. Biol. Chem. 283, 3497–3506. doi: 10.1074/jbc.M700766200
Atkinson, M. M., Keppler, L. D., Orlandi, E. W., Baker, C. J., and Mischke, C. F. (1990). Involvement of plasma-membrane calcium influx in bacterial induction of the K+/H+ and hypersensitive responses in tobacco. Plant Physiol. 92, 215–221. doi: 10.1104/pp.92.1.215
Baliardini, C., Meyer, C. L., Salis, P., Saumitou-Laprade, P., and Verbruggen, N. (2015). CATION EXCHANGER1 cosegregates with cadmium tolerance in the metal hyperaccumulator Arabidopsis halleri and plays a role in limiting oxidative stress in Arabidopsis Spp. Plant Physiol. 169, 549–559. doi: 10.1104/pp.15.01037
Basu, D., Codjoe, J., Veley, K., and Haswell, E. S. (2021). The mechanosensitive ion channel MSL10 modulates susceptibility to pseudomonas syringae in Arabidopsis thaliana. Mol. Plant-Microbe Interact. 35, 567–582. doi: 10.1094/MPMI-08-21-0207-FI
Basu, D., and Haswell, E. S. (2017). Plant mechanosensitive ion channels: An ocean of possibilities. Curr. Opin. Plant Biol. 40, 43–48. doi: 10.1016/j.pbi.2017.07.002
Basu, D., and Haswell, E. S. (2020). The mechanosensitive ion channel MSL10 potentiates responses to cell swelling in Arabidopsis seedlings. Curr. Biol. 30:e6, 2716–2728.e6. doi: 10.1016/j.cub.2020.05.015
Baxter, I., Tchieu, J., Sussman, M. R., Boutry, M., Palmgren, M. G., Gribskov, M., et al. (2003). Genomic comparison of P-type ATPase ion pumps in Arabidopsis and rice. Plant Physiol. 132, 618–628. doi: 10.1104/pp.103.021923
Bayless, A. M., and Nishimura, M. T. (2020). Enzymatic functions for Toll/Interleukin-1 receptor domain proteins in the plant immune system. Front. Genet. :11:539. doi: 10.3389/fgene.2020.00539
Beffagna, N., Buffoli, B., and Busi, C. (2005). Modulation of reactive oxygen species production during osmotic stress in Arabidopsis thaliana cultured cells: involvement of the plasma membrane Ca2+-ATPase and H+-ATPase. Plant Cell Physiol. 46, 1326–1339. doi: 10.1093/pcp/pci142
Bi, G. Z., Su, M., Li, N., Liang, Y., Dang, S., Xu, J. C., et al. (2021). The ZAR1 resistosome is a calcium-permeable channel triggering plant immune signaling. Cells 184, 3528–3541.e12. doi: 10.1016/j.cell.2021.05.003
Bonaventure, G., Gfeller, A., Proebsting, W. M., Hortensteiner, S., Chetelat, A., Martinoia, E., et al. (2007). A gain-of-function allele of TPC1 activates oxylipin biogenesis after leaf wounding in Arabidopsis. Plant J. 49, 889–898. doi: 10.1111/j.1365-313X.2006.03002.x
Bonza, M. C., Morandini, P., Luoni, L., Geisler, M., Palmgren, M. G., and De Michelis, M. I. (2000). AtACA8 encodes a plasma membrane-localized calcium-ATPase of Arabidopsis with a calmodulin-binding domain at the N terminus. Plant Physiol. 123, 1495–1506. doi: 10.1104/pp.123.4.1495
Bose, J., Pottosin, I. I., Shabala, S. S., Palmgren, M. G., and Shabala, S. (2011). Calcium efflux systems in stress signaling and adaptation in plants. Front. Plant Sci. 2:85. doi: 10.3389/fpls.2011.00085
Boursiac, Y., Lee, S. M., Romanowsky, S., Blank, R., Sladek, C., Chung, W. S., et al. (2010). Disruption of the vacuolar calcium-ATPases in Arabidopsis results in the activation of a salicylic acid-dependent programmed cell death pathway. Plant Physiol. 154, 1158–1171. doi: 10.1104/pp.110.159038
Bradshaw, H. D. Jr. (2005). Mutations in CAX1 produce phenotypes characteristic of plants tolerant to serpentine soils. New Phytol. 167, 81–88. doi: 10.1111/j.1469-8137.2005.01408.x
Carafoli, E., and Brini, M. (2000). Calcium pumps: structural basis for and mechanism of calcium transmembrane transport. Curr. Opin. Chem. Biol. 4, 152–161. doi: 10.1016/S1367-5931(99)00069-1
Carpaneto, A., Ivashikina, N., Levchenko, V., Krol, E., Jeworutzki, E., Zhu, J. K., et al. (2007). Cold transiently activates calcium-permeable channels in Arabidopsis mesophyll cells. Plant Physiol. 143, 487–494. doi: 10.1104/pp.106.090928
Cerana, M., Bonza, M. C., Harris, R., Sanders, D., and De Michelis, M. I. (2006). Abscisic acid stimulates the expression of two isoforms of plasma membrane Ca2+-ATPase in Arabidopsis thaliana seedlings. Plant Biol. 8, 572–578. doi: 10.1055/s-2006-924111
Cheng, N. H., Pittman, J. K., Barkla, B. J., Shigaki, T., and Hirschi, K. D. (2003). The Arabidopsis cax1 mutant exhibits impaired ion homeostasis, development, and hormonal responses and reveals interplay among vacuolar transporters. Plant Cell 15, 347–364. doi: 10.1105/tpc.007385
Cheng, N. H., Pittman, J. K., Shigaki, T., Lachmansingh, J., LeClere, S., Lahner, B., et al. (2005). Functional association of Arabidopsis CAX1 and CAX3 is required for normal growth and ion homeostasis. Plant Physiol. 138, 2048–2060. doi: 10.1104/pp.105.061218
Cheng, Y., Tian, Q. Y., and Zhang, W. H. (2016). Glutamate receptors are involved in mitigating effects of amino acids on seed germination of Arabidopsis thaliana under salt stress. Environ. Exp. Bot. 130, 68–78. doi: 10.1016/j.envexpbot.2016.05.004
Cheng, Y., Zhang, X. X., Sun, T. Y., Tian, Q. Y., and Zhang, W. H. (2018). Glutamate receptor homolog3.4 is involved in regulation of seed germination under salt stress in Arabidopsis. Plant Cell Physiol. 59, 978–988. doi: 10.1093/pcp/pcy034
Chin, K., DeFalco, T. A., Moeder, W., and Yoshioka, K. (2013). The Arabidopsis cyclic nucleotide-gated ion channels AtCNGC2 and AtCNGC4 work in the same signaling pathway to regulate pathogen defense and floral transition. Plant Physiol. 163, 611–624. doi: 10.1104/pp.113.225680
Chin, K., Moeder, W., Abdel-Hamid, H., Shahinas, D., Gupta, D., and Yoshioka, K. (2010). Importance of the alpha C-helix in the cyclic nucleotide binding domain for the stable channel regulation and function of cyclic nucleotide gated ion channels in Arabidopsis. J. Exp. Bot. 61, 2383–2393. doi: 10.1093/jxb/erq072
Choi, W. G., Miller, G., Wallace, I., Harper, J., Mittler, R., and Gilroy, S. (2017). Orchestrating rapid long-distance signaling in plants with Ca2+, ROS and electrical signals. Plant J. 90, 698–707. doi: 10.1111/tpj.13492
Choi, W. G., Toyota, M., Kim, S. H., Hilleary, R., and Gilroy, S. (2014). Salt stress-induced Ca2+ waves are associated with rapid, long-distance root-to-shoot signaling in plants. Proc. Natl. Acad. Sci. U. S. A. 111, 6497–6502. doi: 10.1073/pnas.1319955111
Chung, W. S., Lee, S. H., Kim, J. C., Heo, W. D., Kim, M. C., Park, C. Y., et al. (2000). Identification of a calmodulin-regulated soybean Ca2+-ATPase (SCA1) that is located in the plasma membrane. Plant Cell 12, 1393–1407.
Clough, S. J., Fengler, K. A., Yu, I. C., Lippok, B., Smith, R. K., and Bent, A. F. (2000). The Arabidopsis dnd1 "defense, no death" gene encodes a mutated cyclic nucleotide-gated ion channel. Proc. Natl. Acad. Sci. U. S. A. 97, 9323–9328. doi: 10.1073/pnas.150005697
Conn, S. J., Gilliham, M., Athman, A., Schreiber, A. W., Baumann, U., Moller, I., et al. (2011). Cell-specific vacuolar calcium storage mediated by CAX1 regulates apoplastic calcium concentration, gas exchange, and plant productivity in Arabidopsis. Plant Cell 23, 240–257. doi: 10.1105/tpc.109.072769
Corso, M., Doccula, F. G., de Melo, J. R. F., Costa, A., and Verbruggen, N. (2018). Endoplasmic reticulum-localized CCX2 is required for osmotolerance by regulating ER and cytosolic Ca2+ dynamics in Arabidopsis. Proc. Natl. Acad. Sci. U. S. A. 115, 3966–3971. doi: 10.1073/pnas.1720422115
Crotty, C. M., Wang, M. Y., and Poole, R. J. (1996). A depolarization-activated calcium channel in tobacco protoplasts. Plant Physiol. 111:428.
Dadacz-Narloch, B., Beyhl, D., Larisch, C., Lopez-Sanjurjo, E. J., Reski, R., Kuchitsu, K., et al. (2011). A novel calcium binding site in the slow vacuolar cation channel TPC1 senses luminal calcium levels. Plant Cell 23, 2696–2707. doi: 10.1105/tpc.111.086751
DeFalco, T. A., Marshall, C. B., Munro, K., Kang, H. G., Moeder, W., Ikura, M., et al. (2016a). Multiple calmodulin-binding sites positively and negatively regulate Arabidopsis CYCLIC NUCLEOTIDE-GATED CHANNEL12. Plant Cell 28, 1738–1751. doi: 10.1105/tpc.15.00870
DeFalco, T. A., Moeder, W., and Yoshioka, K. (2016b). Opening the gates: insights into cyclic nucleotide-gated channel-mediated signaling. Trends Plant Sci. 21, 903–906. doi: 10.1016/j.tplants.2016.08.011
Demidchik, V., Cuin, T. A., Svistunenko, D., Smith, S. J., Miller, A. J., Shabala, S., et al. (2010). Arabidopsis root K+-efflux conductance activated by hydroxyl radicals: single-channel properties, genetic basis and involvement in stress-induced cell death. J. Cell Sci. 123, 1468–1479. doi: 10.1242/jcs.064352
Demidchik, V., and Shabala, S. (2018). Mechanisms of cytosolic calcium elevation in plants: the role of ion channels, calcium extrusion systems and NADPH oxidase-mediated 'ROS-Ca2+ Hub'. Funct. Plant Biol. 45, 9–27. doi: 10.1071/FP16420
Demidchik, V., Shabala, S., Isayenkov, S., Cuin, T. A., and Pottosin, I. (2018). Calcium transport across plant membranes: mechanisms and functions. New Phytol. 220, 49–69. doi: 10.1111/nph.15266
Demidchik, V., Shang, Z., Shin, R., Colaco, R., Laohavisit, A., Shabala, S., et al. (2011). Receptor-like activity evoked by extracellular ADP in Arabidopsis root epidermal plasma membrane. Plant Physiol. 156, 1375–1385. doi: 10.1104/pp.111.174722
Demidchik, V., Shang, Z., Shin, R., Thompson, E., Rubio, L., Laohavisit, A., et al. (2009). Plant extracellular ATP signalling by plasma membrane NADPH oxidase and Ca2+ channels. Plant J. 58, 903–913. doi: 10.1111/j.1365-313X.2009.03830.x
Denness, L., McKenna, J. F., Segonzac, C., Wormit, A., Madhou, P., Bennett, M., et al. (2011). Cell wall damage-induced lignin biosynthesis is regulated by a reactive oxygen species- and jasmonic acid-dependent process in Arabidopsis. Plant Physiol. 156, 1364–1374. doi: 10.1104/pp.111.175737
Ding, Y. L., Shi, Y. T., and Yang, S. H. (2019). Advances and challenges in uncovering cold tolerance regulatory mechanisms in plants. New Phytol. 222, 1690–1704. doi: 10.1111/nph.15696
Duszyn, M., Swiezawska, B., Szmidt-Jaworska, A., and Jaworski, K. (2019). Cyclic nucleotide gated channels (CNGCs) in plant signalling-current knowledge and perspectives. J. Plant Physiol. 241:153035. doi: 10.1016/j.jplph.2019.153035
Ecker, J. R., and Davis, R. W. (1987). Plant defense genes are regulated by ethylene. Proc. Natl. Acad. Sci. U. S. A. 84, 5202–5206. doi: 10.1073/pnas.84.15.5202
Emery, L., Whelan, S., Hirschi, K. D., and Pittman, J. K. (2012). Protein phylogenetic analysis of Ca2+/cation antiporters and insights into their evolution in plants. Front. Plant Sci. 3:1. doi: 10.3389/fpls.2012.00001
Finka, A., Cuendet, A. F. H., Maathuis, F. J. M., Saidi, Y., and Goloubinoff, P. (2012). Plasma membrane cyclic nucleotide gated calcium channels control land plant thermal sensing and acquired thermotolerance. Plant Cell 24, 3333–3348. doi: 10.1105/tpc.112.095844
Fischer, C., DeFalco, T. A., Karia, P., Snedden, W. A., Moeder, W., Yoshioka, K., et al. (2017). Calmodulin as a Ca2+ −sensing subunit of Arabidopsis cyclic nucleotide-gated channel complexes. Plant Cell Physiol. 58, 1208–1221. doi: 10.1093/pcp/pcx052
Fischer, C., Kugler, A., Hoth, S., and Dietrich, P. (2013). An IQ domain mediates the interaction with calmodulin in a plant cyclic nucleotide-gated channel. Plant Cell Physiol. 54, 573–584. doi: 10.1093/pcp/pct021
Forde, B. G., and Roberts, M. R. (2014). Glutamate receptor-like channels in plants: a role as amino acid sensors in plant defence? F1000Prime Rep. 6:37. doi: 10.12703/P6-37
Frei Dit Frey, N., Mbengue, M., Kwaaitaal, M., Nitsch, L., Altenbach, D., Haweker, H., et al. (2012). Plasma membrane calcium ATPases are important components of receptor-mediated signaling in plant immune responses and development. Plant Physiol. 159, 798–809. doi: 10.1104/pp.111.192575
Furuichi, T., Cunningham, K. W., and Muto, S. (2001). A putative two pore channel AtTPC1 mediates Ca2+ flux in Arabidopsis leaf cells. Plant Cell Physiol. 42, 900–905. doi: 10.1093/pcp/pce145
Galaviz-Hernandez, C., Stagg, C., de Ridder, G., Tanaka, T. S., Ko, M. S. H., Schlessinger, D., et al. (2003). Plac8 and Plac9, novel placental-enriched genes identified through microarray analysis. Gene 309, 81–89. doi: 10.1016/S0378-1119(03)00508-0
Garcia Bossi, J., Kumar, K., Barberini, M. L., Dominguez, G. D., Rondon Guerrero, Y. D. C., Marino-Buslje, C., et al. (2020). The role of P-type IIA and P-type IIB Ca2+-ATPases in plant development and growth. J. Exp. Bot. 71, 1239–1248. doi: 10.1093/jxb/erz521
Geisler, M., Axelsen, K. B., Harper, J. F., and Palmgren, M. G. (2000a). Molecular aspects of higher plant P-type Ca2+-ATPases. Biochim. Biophys. Acta 1465, 52–78. doi: 10.1016/S0005-2736(00)00131-0
Geisler, M., Frangne, N., Gomes, E., Martinoia, E., and Palmgren, M. G. (2000b). The ACA4 gene of Arabidopsis encodes a vacuolar membrane calcium pump that improves salt tolerance in yeast. Plant Physiol. 124, 1814–1827. doi: 10.1104/pp.124.4.1814
Gelli, A., and Blumwald, E. (1997). Hyperpolarization-activated Ca2+-permeable channels in the plasma membrane of tomato cells. J. Membr. Biol. 155, 35–45. doi: 10.1007/s002329900156
Gonzalez-Fontes, A., Navarro-Gochicoa, M. T., Camacho-Cristobal, J. J., Herrera-Rodriguez, M. B., Quiles-Pando, C., and Rexach, J. (2014). Is Ca2+ involved in the signal transduction pathway of boron deficiency? New hypotheses for sensing boron deprivation. Plant Sci. 217-218, 135–139. doi: 10.1016/j.plantsci.2013.12.011
Gonzalez-Fontes, A., Rexach, J., Quiles-Pando, C., Herrera-Rodriguez, M. B., Camacho-Cristobal, J. J., and Navarro-Gochicoa, M. T. (2013). Transcription factors as potential participants in the signal transduction pathway of boron deficiency. Plant Signal. Behav. 8:e26114. doi: 10.4161/psb.26114
Guo, J. T., Zeng, W. Z., Chen, Q. F., Lee, C., Chen, L. P., Yang, Y., et al. (2016). Structure of the voltage-gated two-pore channel TPC1 from Arabidopsis thaliana. Nature 531, 196–201. doi: 10.1038/nature16446
Han, N., Lan, W. J., He, X., Shao, Q., Wang, B. S., and Zhao, X. J. (2012). Expression of a Suaeda salsa vacuolar H+/Ca2+ transporter gene in Arabidopsis contributes to physiological changes in salinity. Plant Mol. Biol. Report. 30, 470–477. doi: 10.1007/s11105-011-0353-y
Harper, J. F., Hong, B., Hwang, I., Guo, H. Q., Stoddard, R., Huang, J. F., et al. (1998). A novel calmodulin-regulated Ca2+-ATPase (ACA2) from Arabidopsis with an N-terminal autoinhibitory domain. J. Biol. Chem. 273, 1099–1106. doi: 10.1074/jbc.273.2.1099
Haswell, E. S. (2007). MscS-like proteins in plants. Curr. Top. Membr. 58, 329–359. doi: 10.1016/S1063-5823(06)58013-5
Hirschi, K. D. (1999). Expression of Arabidopsis CAX1 in tobacco: altered calcium homeostasis and increased stress sensitivity. Plant Cell 11, 2113–2122. doi: 10.1105/tpc.11.11.2113
Hocking, B., Conn, S. J., Manohar, M., Xu, B., Athman, A., Stancombe, M. A., et al. (2017). Heterodimerization of Arabidopsis calcium/proton exchangers contributes to regulation of guard cell dynamics and plant defense responses. J. Exp. Bot. 68, 4171–4183. doi: 10.1093/jxb/erx209
Horsefield, S., Burdett, H., Zhang, X. X., Manik, M. K., Shi, Y., Chen, J., et al. (2019). NAD+ cleavage activity by animal an plant TIR domains in cell death pathways. Science 365, 793–799. doi: 10.1126/science.aax1911
Hu, M. J., Qi, J. F., Bi, G. Z., and Zhou, J. M. (2020). Bacterial effectors induce oligomerization of immune receptor ZAR1 in vivo. Mol. Plant 13, 793–801. doi: 10.1016/j.molp.2020.03.004
Huda, K. M., Banu, M. S., Garg, B., Tula, S., Tuteja, R., and Tuteja, N. (2013a). OsACA6, a P-type IIB Ca2+ ATPase promotes salinity and drought stress tolerance in tobacco by ROS scavenging and enhancing the expression of stress-responsive genes. Plant J. 76, 997–1015. doi: 10.1111/tpj.12352
Huda, K. M., Banu, M. S., Pathi, K. M., and Tuteja, N. (2013b). Reproductive organ and vascular specific promoter of the rice plasma membrane Ca2+ ATPase mediates environmental stress responses in plants. PLoS One 8:e57803. doi: 10.1371/journal.pone.0057803
Huda, K. M., Banu, M. S., Tuteja, R., and Tuteja, N. (2013c). Global calcium transducer P-type Ca2+-ATPases open new avenues for agriculture by regulating stress signalling. J. Exp. Bot. 64, 3099–3109. doi: 10.1093/jxb/ert182
Huda, K. M., Yadav, S., Akhter Banu, M. S., Trivedi, D. K., and Tuteja, N. (2013d). Genome-wide analysis of plant-type II Ca2+ ATPases gene family from rice and Arabidopsis: potential role in abiotic stresses. Plant Physiol. Biochem. 65, 32–47. doi: 10.1016/j.plaphy.2013.01.002
Jacob, P., Kim, N. H., Wu, F. H., El Kasmr, F., Chi, Y., Walton, W. G., et al. (2021). Plant "helper" immune receptors are Ca2+-permeable nonselective cation channels. Science 373, 420–425. doi: 10.1126/science.abg7917
Jacob, F., Kracher, B., Mine, A., Seyfferth, C., Blanvillain-Baufume, S., Parker, J. E., et al. (2018). A dominant-interfering camta3 mutation compromises primary transcriptional outputs mediated by both cell surface and intracellular immune receptors in Arabidopsis thaliana. New Phytol. 217, 1667–1680. doi: 10.1111/nph.14943
Jha, S. K., Sharma, M., and Pandey, G. K. (2016). Role of cyclic nucleotide gated channels in stress management in plants. Curr. Genomics 17, 315–329. doi: 10.2174/1389202917666160331202125
Jian, L.-C., Li, J.-H., Chen, W.-P., Li, P. H., and Ahlstrand, G. G. (1999). Cytochemical localization of calcium and Ca2+-ATPase activity in plant cells under chilling stress: a comparative study between the chilling-sensitive maize and the chilling-insensitive winter wheat. Plant Cell Physiol. 40, 1061–1071. doi: 10.1093/oxfordjournals.pcp.a029488
Johns, S., Hagihara, T., Toyota, M., and Gilroy, S. (2021). The fast and the furious: rapid long-range signaling in plants. Plant Physiol. 185, 694–706. doi: 10.1093/plphys/kiaa098
Jubic, L. M., Saile, S., Furzer, O. J., El Kasmi, F., and Dangl, J. L. (2019). Help wanted: helper NLRs and plant immune responses. Curr. Opin. Plant Biol. 50, 82–94. doi: 10.1016/j.pbi.2019.03.013
Jurkowski, G. I., Smith, R. K., Yu, I. C., Ham, J. H., Sharma, S. B., Klessig, D. F., et al. (2004). Arabidopsis DND2, a second cyclic nucleotide-gated ion channel gene for which mutation causes the “defense, no death” phenotype. Mol. Plant Micro. Interact. 17, 511–520. doi: 10.1094/MPMI.2004.17.5.511
Kamiya, T., Yamagami, M., Hirai, M. Y., and Fujiwara, T. (2012). Establishment of an in planta magnesium monitoring system using CAX3 promoter-luciferase in Arabidopsis. J. Exp. Bot. 63, 355–363. doi: 10.1093/jxb/err283
Kamrul Huda, K. M., Akhter Banu, M. S., Yadav, S., Sahoo, R. K., Tuteja, R., and Tuteja, N. (2014). Salinity and drought tolerant OsACA6 enhances cold tolerance in transgenic tobacco by interacting with stress-inducible proteins. Plant Physiol. Biochem. 82, 229–238. doi: 10.1016/j.plaphy.2014.06.007
Kang, S., Kim, H. B., Lee, H., Choi, J. Y., Heu, S., Oh, C. J., et al. (2006). Overexpression in Arabidopsis of a plasma membrane-targeting glutamate receptor from small radish increases glutamate-mediated Ca2+ influx and delays fungal infection. Mol. Cell 21, 418–427.
Kaplan, B., Sherman, T., and Fromm, H. (2007). Cyclic nucleotide-gated channels in plants. FEBS Lett. 581, 2237–2246. doi: 10.1016/j.febslet.2007.02.017
Katano, K., Kataoka, R., Fujii, M., and Suzuki, N. (2018). Differences between seedlings and flowers in anti-ROS based heat responses of Arabidopsis plants deficient in cyclic nucleotide gated channel 2. Plant Physiol. Biochem. 123, 288–296. doi: 10.1016/j.plaphy.2017.12.021
Kiep, V., Vadassery, J., Lattke, J., Maass, J. P., Boland, W., Peiter, E., et al. (2015). Systemic cytosolic Ca2+ elevation is activated upon wounding and herbivory in Arabidopsis. New Phytol. 207, 996–1004. doi: 10.1111/nph.13493
Kim, K. M., Park, Y. H., Kim, C. K., Hirschi, K., and Sohn, J. K. (2005). Development of transgenic rice plants overexpressing the Arabidopsis H+/Ca2+ antiporter CAX1 gene. Plant Cell Rep. 23, 678–682. doi: 10.1007/s00299-004-0861-4
Koster, P., DeFalco, T. A., and Zipfel, C. (2022). Ca2+ signals in plant immunity. EMBO J. 41:e110741. doi: 10.15252/embj.2022110741
Kurusu, T., Hamada, H., Koyano, T., and Kuchitsu, K. (2012). Intracellular localization and physiological function of a rice Ca2+-permeable channel OsTPC1. Plant Signal. Behav. 7, 1428–1430. doi: 10.4161/psb.22086
Kurusu, T., Kuchitsu, K., and Tada, Y. (2015). Plant signaling networks involving Ca2+ and Rboh/Nox-mediated ROS production under salinity stress. Front. Plant Sci. 6:427. doi: 10.3389/fpls.2015.00427
Kurusu, T., Sakurai, Y., Miyao, A., Hirochika, H., and Kuchitsu, K. (2004). Identification of a putative voltage-gated Ca2+-permeable channel (OsTPC1) involved in Ca2+ influx and regulation of growth and development in rice. Plant Cell Physiol. 45, 693–702. doi: 10.1093/pcp/pch082
Kurusu, T., Yagala, T., Miyao, A., Hirochika, H., and Kuchitsu, K. (2005). Identification of a putative voltage-gated Ca2+ channel as a key regulator of elicitor-induced hypersensitive cell death and mitogen-activated protein kinase activation in rice. Plant J. 42, 798–809. doi: 10.1111/j.1365-313X.2005.02415.x
Kwaaitaal, M., Huisman, R., Maintz, J., Reinstadler, A., and Panstruga, R. (2011). Ionotropic glutamate receptor (iGluR)-like channels mediate MAMP-induced calcium influx in Arabidopsis thaliana. Biochem. J. 440, 355–373. doi: 10.1042/BJ20111112
Lacombe, B., Becker, D., Hedrich, R., DeSalle, R., Hollmann, M., Kwak, J. M., et al. (2001). The identity of plant glutamate receptors. Science 292, 1486–1487. doi: 10.1126/science.292.5521.1486b
Laohavisit, A., Richards, S. L., Shabala, L., Chen, C., Colaco, R. D., Swarbreck, S. M., et al. (2013). Salinity-induced calcium signaling and root adaptation in Arabidopsis require the calcium regulatory protein annexin1. Plant Physiol. 163, 253–262. doi: 10.1104/pp.113.217810
Laohavisit, A., Shang, Z., Rubio, L., Cuin, T. A., Very, A. A., Wang, A., et al. (2012). Arabidopsis annexin1 mediates the radical-activated plasma membrane Ca2+- and K+-permeable conductance in root cells. Plant Cell 24, 1522–1533. doi: 10.1105/tpc.112.097881
Lecourieux, D., Ranjeva, R., and Pugin, A. (2006). Calcium in plant defence-signalling pathways. New Phytol. 171, 249–269. doi: 10.1111/j.1469-8137.2006.01777.x
Lee, J., Hanh Nguyen, H., Park, Y., Lin, J., and Hwang, I. (2021). Spatial regulation of RBOHD via AtECA4-mediated recycling and clathrin-mediated endocytosis contributes to ROS accumulation during salt stress response but not flg22-induced immune response. Plant J. 109, 816–830. doi: 10.1111/tpj.15593
Lee, S., Lee, E. J., Yang, E. J., Lee, J. E., Park, A. R., Song, W. H., et al. (2004). Proteomic identification of annexins, calcium-dependent membrane binding proteins that mediate osmotic stress and abscisic acid signal transduction in Arabidopsis. Plant Cell 16, 1378–1391. doi: 10.1105/tpc.021683
Lee, H. C., and Zhao, Y. J. (2019). Resolving the topological enigma in Ca2+ signaling by cyclic ADP-ribose and NAADP. J. Biol. Chem. 294, 19831–19843. doi: 10.1074/jbc.REV119.009635
Levine, A., Pennell, R. I., Alvarez, M. E., Palmer, R., and Lamb, C. (1996). Calcium-mediated apoptosis in a plant hypersensitive disease resistance response. Curr. Biol. 6, 427–437. doi: 10.1016/S0960-9822(02)00510-9
Lhuissier, F. G. P., De Ruijter, N. C. A., Sieberer, B. J., Esseling, J. J., and Emons, A. M. C. (2001). Time course of cell biological events evoked in legume root hairs by rhizobium nod factors: state of the art. Ann. Bot. 87, 289–302. doi: 10.1006/anbo.2000.1333
Li, C. X., Song, T. T., Zhan, L. F., Cong, C. L., Xu, H. H., Dong, L., et al. (2021). Overexpression of MsRCI2A, MsRCI2B, and MsRCI2C in alfalfa (Medicago sativa L.) provides different extents of enhanced alkali and salt tolerance due to functional specialization of MsRCI2s. Front. Plant Sci. 12:702195. doi: 10.3389/fpls.2021.702195
Li, Z., Wang, X., Chen, J., Gao, J., Zhou, X., and Kuai, B. (2016). CCX1, a putative cation/Ca2+ exchanger, participates in regulation of reactive oxygen species homeostasis and leaf senescence. Plant Cell Physiol. 57, 2611–2619. doi: 10.1093/pcp/pcw175
Li, F., Wang, J., Ma, C. L., Zhao, Y. X., Wang, Y. C., Hasi, A., et al. (2013). Glutamate receptor-like channel3.3 is involved in mediating glutathione-triggered cytosolic calcium transients, transcriptional changes, and innate immunity responses in Arabidopsis. Plant Physiol. 162, 1497–1509. doi: 10.1104/pp.113.217208
Li, Y., Yuan, F., Wen, Z., Li, Y., Wang, F., Zhu, T., et al. (2015). Genome-wide survey and expression analysis of the OSCA gene family in rice. BMC Plant Biol. 15:261. doi: 10.1186/s12870-015-0653-8
Liu, J., Niu, Y., Zhang, J., Zhou, Y., Ma, Z., and Huang, X. (2018). Ca2+ channels and Ca2+ signals involved in abiotic stress responses in plant cells: recent advances. Plant Cell Tissue Organ Cult. 132, 413–424. doi: 10.1007/s11240-017-1350-0
Ma, S. C., Lapin, D., Liu, L., Sun, Y., Song, W., Zhang, X. X., et al. (2020). Direct pathogen-induced assembly of an NLR immune receptor complex to form a holoenzyme. Science 370:1184. doi: 10.1126/science.abe3069
Ma, W., Qi, Z., Smigel, A., Walker, R. K., Verma, R., and Berkowitz, G. A. (2009). Ca2+, cAMP, and transduction of non-self perception during plant immune responses. Proc. Natl. Acad. Sci. U. S. A. 106, 20995–21000. doi: 10.1073/pnas.0905831106
Manohar, M., Shigaki, T., and Hirschi, K. D. (2011a). Plant cation/H+ exchangers (CAXs): biological functions and genetic manipulations. Plant Biol. 13, 561–569. doi: 10.1111/j.1438-8677.2011.00466.x
Manohar, M., Shigaki, T., Mei, H., Park, S., Marshall, J., Aguilar, J., et al. (2011b). Characterization of Arabidopsis Ca2+/H+ exchanger CAX3. Biochemist 50, 6189–6195. doi: 10.1021/bi2003839
Manzoor, H., Kelloniemi, J., Chiltz, A., Wendehenne, D., Pugin, A., Poinssot, B., et al. (2013). Involvement of the glutamate receptor AtGLR3.3 in plant defense signaling and resistance to Hyaloperonospora arabidopsidis. Plant J. 76, 466–480. doi: 10.1111/tpj.12311
Mao, K., Yang, J., Wang, M., Liu, H., Guo, X., Zhao, S., et al. (2021). Genome-wide analysis of the apple CaCA superfamily reveals that MdCAX proteins are involved in the abiotic stress response as calcium transporters. BMC Plant Biol. 21:81. doi: 10.1186/s12870-021-02866-1
Martin, R., Qi, T., Zhang, H., Liu, F., King, M., Toth, C., et al. (2020). Structure of the activated ROQ1 resistosome directly recognizing the pathogen effector XopQ. Science 370:eabd9993. doi: 10.1126/science.abd9993
McAinsh, M. R., and Pittman, J. K. (2009). Shaping the calcium signature. New Phytol. 181, 275–294. doi: 10.1111/j.1469-8137.2008.02682.x
Meyerhoff, O., Muller, K., Roelfsema, M. R., Latz, A., Lacombe, B., Hedrich, R., et al. (2005). AtGLR3.4, a glutamate receptor channel-like gene is sensitive to touch and cold. Planta 222, 418–427. doi: 10.1007/s00425-005-1551-3
Miedema, H., Bothwell, J. H., Brownlee, C., and Davies, J. M. (2001). Calcium uptake by plant cells--channels and pumps acting in concert. Trends Plant Sci. 6, 514–519. doi: 10.1016/s1360-1385(01)02124-0
Miedema, H., Demidchik, V., Very, A. A., Bothwell, J. H. F., Brownlee, C., and Davies, J. M. (2008). Two voltage-dependent calcium channels co-exist in the apical plasma membrane of Arabidopsis thaliana root hairs. New Phytol. 179, 378–385. doi: 10.1111/j.1469-8137.2008.02465.x
Modareszadeh, M., Bahmani, R., Kim, D., and Hwang, S. (2021). CAX3 (cation/proton exchanger) mediates a cd tolerance by decreasing ROS through Ca elevation in Arabidopsis. Plant Mol. Biol. 105, 115–132. doi: 10.1007/s11103-020-01072-1
Moeder, W., Phan, V., and Yoshioka, K. (2019). Ca2+ to the rescue - Ca2+ channels and signaling in plant immunity. Plant Sci. 279, 19–26. doi: 10.1016/j.plantsci.2018.04.012
Moon, J. Y., Belloeil, C., Ianna, M. L., and Shin, R. (2019). Arabidopsis CNGC family members contribute to heavy metal ion uptake in plants. Int. J. Mol. Sci. 20:413. doi: 10.3390/ijms20020413
Mori, K., Renhu, N., Naito, M., Nakamura, A., Shiba, H., Yamamoto, T., et al. (2018). Ca2+-permeable mechanosensitive channels MCA1 and MCA2 mediate cold-induced cytosolic Ca2+ increase and cold tolerance in Arabidopsis. Sci. Rep. 8:550. doi: 10.1038/s41598-017-17483-y
Morris, J., Tian, H., Park, S., Sreevidya, C. S., Ward, J. M., and Hirschi, K. D. (2008). AtCCX3 is an Arabidopsis endomembrane H+ −dependent K+ transporter. Plant Physiol. 148, 1474–1486. doi: 10.1104/pp.108.118810
Mortimer, J. C., Laohavisit, A., Miedema, H., and Davies, J. M. (2008). Voltage, reactive oxygen species and the influx of calcium. Plant Signal. Behav. 3, 698–699. doi: 10.4161/psb.3.9.6405
Mosher, S., Moeder, W., Nishimura, N., Jikumaru, Y., Joo, S. H., Urquhart, W., et al. (2010). The lesion-mimic mutant cpr22 shows alterations in abscisic acid signaling and abscisic acid insensitivity in a salicylic acid-dependent manner. Plant Physiol. 152, 1901–1913. doi: 10.1104/pp.109.152603
Nakagawa, Y., Katagiri, T., Shinozaki, K., Qi, Z., Tatsumi, H., Furuichi, T., et al. (2007). Arabidopsis plasma membrane protein crucial for Ca2+ influx and touch sensing in roots. Proc. Natl. Acad. Sci. U. S. A. 104, 3639–3644. doi: 10.1073/pnas.0607703104
Nawaz, Z., Kakar, K. U., Saand, M. A., and Shu, Q. Y. (2014). Cyclic nucleotide-gated ion channel gene family in rice, identification, characterization and experimental analysis of expression response to plant hormones, biotic and abiotic stresses. BMC Genomics 15:853. doi: 10.1186/1471-2164-15-853
Nemchinov, L. G., Shabala, L., and Shabala, S. (2008). Calcium efflux as a component of the hypersensitive response of Nicotiana benthamiana to pseudomonas syringae. Plant Cell Physiol. 49, 40–46. doi: 10.1093/pcp/pcm163
Ng, D. W., Abeysinghe, J. K., and Kamali, M. (2018). Regulating the regulators: the control of transcription factors in plant defense signaling. Int. J. Mol. Sci. 19:3737. doi: 10.3390/ijms19123737
Nishii, K., Moller, M., and Iida, H. (2021). Mix and match: patchwork domain evolution of the land plant-specific Ca2+-permeable mechanosensitive channel MCA. PLoS One 16:e0249735. doi: 10.1371/journal.pone.0249735
Okazaki, Y., Ishigami, M., and Iwasaki, N. (2002). Temporal relationship between cytosolic free Ca2+ and membrane potential during hypotonic turgor regulation in a brackish water charophyte Lamprothamnium succinctum. Plant Cell Physiol. 43, 1027–1035. doi: 10.1093/pcp/pcf127
Oranab, S., Ghaffar, A., Kiran, S., Yameen, M., Munir, B., Zulfiqar, S., et al. (2021). Molecular characterization and expression of cyclic nucleotide gated ion channels 19 and 20 in Arabidopsis thaliana for their potential role in salt stress. Saudi. J. Biol. Sci. 28, 5800–5807. doi: 10.1016/j.sjbs.2021.06.027
Peiter, E., Maathuis, F. J., Mills, L. N., Knight, H., Pelloux, J., Hetherington, A. M., et al. (2005). The vacuolar Ca2+-activated channel TPC1 regulates germination and stomatal movement. Nature 434, 404–408. doi: 10.1038/nature03381
Peyronnet, R., Tran, D., Girault, T., and Frachisse, J. M. (2014). Mechanosensitive channels: feeling tension in a world under pressure. Front. Plant Sci. 5:558. doi: 10.3389/fpls.2014.00558
Pittman, J. K., and Hirschi, K. D. (2016). CAX-ing a wide net: Cation/H+ transporters in metal remediation and abiotic stress signalling. Plant Biol. 18, 741–749. doi: 10.1111/plb.12460
Pottosin, I., Velarde-Buendia, A. M., Bose, J., Zepeda-Jazo, I., Shabala, S., and Dobrovinskaya, O. (2014). Cross-talk between reactive oxygen species and polyamines in regulation of ion transport across the plasma membrane: implications for plant adaptive responses. J. Exp. Bot. 65, 1271–1283. doi: 10.1093/jxb/ert423
Qi, Z., Stephens, N. R., and Spalding, E. P. (2006). Calcium entry mediated by GLR3.3, an Arabidopsis glutamate receptor with a broad agonist profile. Plant Physiol. 142, 963–971. doi: 10.1104/pp.106.088989
Qiu, X. M., Sun, Y. Y., Ye, X. Y., and Li, Z. G. (2020). Signaling role of glutamate in plants. Front. Plant Sci. 10:1743. doi: 10.3389/fpls.2019.01743
Qudeimat, E., Faltusz, A. M., Wheeler, G., Lang, D., Holtorf, H., Brownlee, C., et al. (2008). A PIIB-type Ca2+-ATPase is essential for stress adaptation in Physcomitrella patens. Proc. Natl. Acad. Sci. U. S. A. 105, 19555–19560. doi: 10.1073/pnas.0800864105
Quiles-Pando, C., Rexach, J., Navarro-Gochicoa, M. T., Camacho-Cristobal, J. J., Herrera-Rodriguez, M. B., and Gonzalez-Fontes, A. (2013). Boron deficiency increases the levels of cytosolic Ca2+ and expression of Ca2+-related genes in Arabidopsis thaliana roots. Plant Physiol. Biochem. 65, 55–60. doi: 10.1016/j.plaphy.2013.01.004
Ranf, S., Wunnenberg, P., Lee, J., Becker, D., Dunkel, M., Hedrich, R., et al. (2008). Loss of the vacuolar cation channel, AtTPC1, does not impair Ca2+ signals induced by abiotic and biotic stresses. Plant J. 53, 287–299. doi: 10.1111/j.1365-313X.2007.03342.x
Resentini, F., Grenzi, M., Ancora, D., Cademartori, M., Luoni, L., Franco, M., et al. (2021). Simultaneous imaging of ER and cytosolic Ca2+ dynamics reveals long-distance ER Ca2+ waves in plants. Plant Physiol. 187, 603–617. doi: 10.1093/plphys/kiab251
Richards, S. L., Laohavisit, A., Mortimer, J. C., Shabala, L., Swarbreck, S. M., Shabala, S., et al. (2014). Annexin 1 regulates the H2O2-induced calcium signature in Arabidopsis thaliana roots. Plant J. 77, 136–145. doi: 10.1111/tpj.12372
Saddhe, A. A., and Kumar, K. (2015). In silico identification and expression analysis of MscS like gene family in rice. Plant Gene 1, 8–17. doi: 10.1016/j.plgene.2014.12.001
Sanders, D., Pelloux, J., Brownlee, C., and Harper, J. F. (2002). Calcium at the crossroads of signaling. Plant Cell 14, S401–S417. doi: 10.1105/tpc.002899
Schiott, M., Romanowsky, S. M., Baekgaard, L., Jakobsen, M. K., Palmgren, M. G., and Harper, J. F. (2004). A plant plasma membrane Ca2+ pump is required for normal pollen tube growth and fertilization. Proc. Natl. Acad. Sci. U. S. A. 101, 9502–9507. doi: 10.1073/pnas.0401542101
Schulze, C., Sticht, H., Meyerhoff, P., and Dietrich, P. (2011). Differential contribution of EF-hands to the Ca2+-dependent activation in the plant two-pore channel TPC1. Plant J. 68, 424–432. doi: 10.1111/j.1365-313X.2011.04697.x
Schuurink, R. C., Shartzer, S. F., Fath, A., and Jones, R. L. (1998). Characterization of a calmodulin-binding transporter from the plasma membrane of barley aleurone. Proc. Natl. Acad. Sci. U. S. A. 95, 1944–1949. doi: 10.1073/pnas.95.4.1944
Seifikalhor, M., Aliniaeifard, S., Shomali, A., Azad, N., Hassani, B., Lastochkina, O., et al. (2019). Calcium signaling and salt tolerance are diversely entwined in plants. Plant Signal. Behav. 14:1665455. doi: 10.1080/15592324.2019.1665455
Shabala, S., Baekgaard, L., Shabala, L., Fuglsang, A., Babourina, O., Palmgren, M. G., et al. (2011a). Plasma membrane Ca2+ transporters mediate virus-induced acquired resistance to oxidative stress. Plant Cell Environ. 34, 406–417. doi: 10.1111/j.1365-3040.2010.02251.x
Shabala, S., Baekgaard, L., Shabala, L., Fuglsang, A. T., Cuin, T. A., Nemchinov, L. G., et al. (2011b). Endomembrane Ca2+-ATPases play a significant role in virus-induced adaptation to oxidative stress. Plant Signal. Behav. 6, 1053–1056. doi: 10.4161/psb.6.7.15634
Shabala, S., Bose, J., Fuglsang, A. T., and Pottosin, I. (2016). On a quest for stress tolerance genes: membrane transporters in sensing and adapting to hostile soils. J. Exp. Bot. 67, 1015–1031. doi: 10.1093/jxb/erv465
Shang, Z., Laohavisit, A., and Davies, J. M. (2009). Extracellular ATP activates an Arabidopsis plasma membrane Ca2+-permeable conductance. Plant Signal. Behav. 4, 989–991. doi: 10.4161/psb.4.10.9680
Shigaki, T., and Hirschi, K. D. (2006). Diverse functions and molecular properties emerging for CAX cation/H+ exchangers in plants. Plant Biol. 8, 419–429. doi: 10.1055/s-2006-923950
Shigaki, T., Mei, H., Marshall, J., Li, X., Manohar, M., and Hirschi, K. D. (2010). The expression of the open reading frame of Arabidopsis CAX1, but not its cDNA, confers metal tolerance in yeast. Plant Biol. 12, 935–939. doi: 10.1111/j.1438-8677.2010.00368.x
Shigaki, T., Sreevidya, C., and Hirschi, K. D. (2002). Analysis of the Ca2+ domain in the Arabidopsis H+/Ca2+ antiporters CAX1 and CAX3. Plant Mol. Biol. 50, 475–483. doi: 10.1023/A:1019880006606
Shukla, D., Huda, K. M., Banu, M. S., Gill, S. S., Tuteja, R., and Tuteja, N. (2014). OsACA6, a P-type 2B Ca2+ ATPase functions in cadmium stress tolerance in tobacco by reducing the oxidative stress load. Planta 240, 809–824. doi: 10.1007/s00425-014-2133-z
Song, Y. L., Dong, Y. J., Tian, X. Y., Wang, W. W., and He, Z. L. (2018). Mechanisms of exogenous nitric oxide and 24-epibrassinolide alleviating chlorosis of peanut plants under iron deficiency. Pedosphere 28, 926–942. doi: 10.1016/S1002-0160(17)60446-6
Swarbreck, S. M., Colaco, R., and Davies, J. M. (2013). Plant calcium-permeable channels. Plant Physiol. 163, 514–522. doi: 10.1104/pp.113.220855
Sze, H., Liang, F., Hwang, I., Curran, A. C., and Harper, J. F. (2000). Diversity and regulation of plant Ca2+ pumps: insights from expression in yeast. Annu. Rev. Plant Physiol. Plant Mol. Biol. 51, 433–462. doi: 10.1146/annurev.arplant.51.1.433
Tan, Y. Q., Yang, Y., Zhang, A., Fei, C. F., Gu, L. L., Sun, S. J., et al. (2020). Three CNGC family members, CNGC5, CNGC6, and CNGC9, are required for constitutive growth of Arabidopsis root hairs as Ca2+-permeable channels. Plant Commun. 1:100001. doi: 10.1016/j.xplc.2019.100001
Tang, R. J., and Luan, S. (2017). Regulation of calcium and magnesium homeostasis in plants: from transporters to signaling network. Curr. Opin. Plant Biol. 39, 97–105. doi: 10.1016/j.pbi.2017.06.009
Tapken, D., Anschutz, U., Liu, L. H., Huelsken, T., Seebohm, G., Becker, D., et al. (2013). A plant homolog of animal glutamate receptors is an ion channel gated by multiple hydrophobic amino acids. Sci. Signal. 6:ra47. doi: 10.1126/scisignal.2003762
Thion, L., Mazars, C., Nacry, P., Bouchez, D., Moreau, M., Ranjeva, R., et al. (1998). Plasma membrane depolarization-activated calcium channels, stimulated by microtubule-depolymerizing drugs in wild-type Arabidopsis thaliana protoplasts, display constitutively large activities and a longer half-life in ton 2 mutant cells affected in the organization of cortical microtubules. Plant J. 13, 603–610.
Thor, K. (2019). Calcium-nutrient and messenger. Front. Plant Sci. 10:440. doi: 10.3389/fpls.2019.00440
Tian, W., Hou, C., Ren, Z., Wang, C., Zhao, F., Dahlbeck, D., et al. (2019). A calmodulin-gated calcium channel links pathogen patterns to plant immunity. Nature 572, 131–135. doi: 10.1038/s41586-019-1413-y
Tian, W., Wang, C., Gao, Q., Li, L., and Luan, S. (2020). Calcium spikes, waves and oscillations in plant development and biotic interactions. Nat. Planta 6, 750–759. doi: 10.1038/s41477-020-0667-6
Tunc-Ozdemir, M., Tang, C., Ishka, M. R., Brown, E., Groves, N. R., Myers, C. T., et al. (2013). A cyclic nucleotide-gated channel (CNGC16) in pollen is critical for stress tolerance in pollen reproductive development. Plant Physiol. 161, 1010–1020. doi: 10.1104/pp.112.206888
Veley, K. M., Maksaev, G., Frick, E. M., January, E., Kloepper, S. C., and Haswell, E. S. (2014). Arabidopsis MSL10 has a regulated cell death signaling activity that is separable from its mechanosensitive ion channel activity. Plant Cell 26, 3115–3131. doi: 10.1105/tpc.114.128082
Vincill, E. D., Bieck, A. M., and Spalding, E. P. (2012). Ca2+ conduction by an amino acid-gated ion channel related to glutamate receptors. Plant Physiol. 159, 40–46. doi: 10.1104/pp.112.197509
Wan, L., Essuman, K., Anderson, R. G., Sasaki, Y., Monteiro, F., Chung, E. H., et al. (2019). TIR domains of plant immune receptors are NAD+-cleaving enzymes that promote cell death. Science 365, 799–803. doi: 10.1126/science.aax1771
Wan, L., and He, Z. (2021). NADase and now Ca2+ channel, what else to learn about plant NLRs? Stress Biol. 1:7. doi: 10.1007/s44154-021-00007-0
Wang, F., Chen, Z. H., Liu, X., Colmer, T. D., Zhou, M., and Shabala, S. (2016). Tissue-specific root ion profiling reveals essential roles of the CAX and ACA calcium transport systems in response to hypoxia in Arabidopsis. J. Exp. Bot. 67, 3747–3762. doi: 10.1093/jxb/erw034
Wang, J. Z., Hu, M. J., Wang, J., Qi, J. F., Han, Z. F., Wang, G. X., et al. (2019c). Reconstitution and structure of a plant NLR resistosome conferring immunity. Science 364:eaav5870. doi: 10.1126/science.aav5870
Wang, P. H., Lee, C. E., Lin, Y. S., Lee, M. H., Chen, P. Y., Chang, H. C., et al. (2019e). The glutamate receptor-like protein GLR3.7 interacts with 14-3-3ω and participates in salt stress response in Arabidopsis thaliana. Front. Plant Sci. 10:1169. doi: 10.3389/fpls.2019.01169
Wang, P., Li, Z., Wei, J., Zhao, Z., Sun, D., and Cui, S. (2012). A Na+/Ca2+ exchanger-like protein (AtNCL) involved in salt stress in Arabidopsis. J. Biol. Chem. 287, 44062–44070. doi: 10.1074/jbc.M112.351643
Wang, J. C., Liu, X., Zhang, A., Ren, Y. L., Wu, F. Q., Wang, G., et al. (2019b). A cyclic nucleotide-gated channel mediates cytoplasmic calcium elevation and disease resistance in rice. Cell Res. 29, 820–831. doi: 10.1038/s41422-019-0219-7
Wang, J., Ren, Y., Liu, X., Luo, S., Zhang, X., Liu, X., et al. (2021). Transcriptional activation and phosphorylation of OsCNGC9 confer enhanced chilling tolerance in rice. Mol. Plant 14, 315–329. doi: 10.1016/j.molp.2020.11.022
Wang, G., Roux, B., Feng, F., Guy, E., Li, L., Li, N., et al. (2015). The decoy substrate of a pathogen effector and a pseudokinase specify pathogen-induced modified-self recognition and immunity in plants. Cell Host Microbe 18, 285–295. doi: 10.1016/j.chom.2015.08.004
Wang, L., Stacey, G., Leblanc-Fournier, N., Legue, V., Moulia, B., and Davies, J. M. (2019d). Early extracellular ATP signaling in Arabidopsis root epidermis: a multi-conductance process. Front. Plant Sci. 10:1064. doi: 10.3389/fpls.2019.01064
Wang, J., Wang, J., Hu, M., Wu, S., Qi, J., Wang, G., et al. (2019a). Ligand-triggered allosteric ADP release primes a plant NLR complex. Science 364:eaav5868. doi: 10.1126/science.aav5868
Ward, J. M., Maser, P., and Schroeder, J. I. (2009). Plant ion channels: gene families, physiology, and functional genomics analyses. Annu. Rev. Physiol. 71, 59–82. doi: 10.1146/annurev.physiol.010908.163204
White, P. J. (2009). Depolarization-activated calcium channels shape the calcium signatures induced by low-temperature stress. New Phytol. 183, 6–8. doi: 10.1111/j.1469-8137.2009.02857.x
Wilkins, K. A., Matthus, E., Swarbreck, S. M., and Davies, J. M. (2016). Calcium-mediated abiotic stress signaling in roots. Front. Plant Sci. 7:1296. doi: 10.3389/fpls.2016.01296
Wisden, W., and Seeburg, P. H. (1993). Mammalian ionotropic glutamate receptors. Curr. Opin. Neurobiol. 3, 291–298. doi: 10.1016/0959-4388(93)90120-N
Wu, Q., Huang, L., Su, N., Shabala, L., Wang, H., Huang, X., et al. (2020). Calcium-dependent hydrogen peroxide mediates hydrogen-rich eater-reduced cadmium uptake in plant roots. Plant Physiol. 183, 1331–1344. doi: 10.1104/pp.20.00377
Wu, Z., Liang, F., Hong, B., Young, J. C., Sussman, M. R., Harper, J. F., et al. (2002). An endoplasmic reticulum-bound Ca2+/Mn2+ pump, ECA1, supports plant growth and confers tolerance to Mn2+ stress. Plant Physiol. 130, 128–137. doi: 10.1104/pp.004440
Wu, Y., Qin, B., Feng, K., Yan, R., Kang, E., Liu, T., et al. (2018). Extracellular ATP promoted pollen germination and tube growth of Nicotiana tabacum through promoting K+ and Ca2+ absorption. Plant Reprod. 31, 399–410. doi: 10.1007/s00497-018-0341-6
Wu, Q., Shigaki, T., Williams, K. A., Han, J. S., Kim, C. K., Hirschi, K. D., et al. (2011). Expression of an Arabidopsis Ca2+/H+ antiporter CAX1 variant in petunia enhances cadmium tolerance and accumulation. J. Plant Physiol. 168, 167–173. doi: 10.1016/j.jplph.2010.06.005
Xu, G., Moeder, W., Yoshioka, K., and Shan, L. (2022). A tale of many families: calcium channels in plant immunity. Plant Cell 34, 1551–1567. doi: 10.1093/plcell/koac033
Xu, L., Zahid, K. R., He, L. R., Zhang, W. W., He, X., Zhang, X. L., et al. (2013). GhCAX3 gene, a novel Ca2+/H+ exchanger from cotton, confers regulation of cold response and ABA induced signal transduction. PLoS One 8:e66303. doi: 10.1371/journal.pone.0066303
Yadav, A. K., Shankar, A., Jha, S. K., Kanwar, P., Pandey, A., and Pandey, G. K. (2015). A rice tonoplastic calcium exchanger, OsCCX2 mediates Ca2+/cation transport in yeast. Sci. Rep. 5:17117. doi: 10.1038/srep17117
Yamada, N., Theerawitaya, C., Cha-um, S., Kirdmanee, C., and Takabe, T. (2014). Expression and functional analysis of putative vacuolar Ca2+-transporters (CAXs and ACAs) in roots of salt tolerant and sensitive rice cultivars. Protoplasma 251, 1067–1075. doi: 10.1007/s00709-014-0615-2
Yamanaka, T., Nakagawa, Y., Mori, K., Nakano, M., Imamura, T., Kataoka, H., et al. (2010). MCA1 and MCA2 that mediate Ca2+ uptake have distinct and overlapping roles in Arabidopsis. Plant Physiol. 152, 1284–1296. doi: 10.1104/pp.109.147371
Ye, F., Xu, L., Li, X., Zeng, W., Gan, N., Zhao, C., et al. (2021). Voltage-gating and cytosolic Ca2+ activation mechanisms of Arabidopsis two-pore channel AtTPC1. Proc. Natl. Acad. Sci. U. S. A. 118:e2113946118. doi: 10.1073/pnas.2113946118
Yoshioka, K., Kachroo, P., Tsui, F., Sharma, S. B., Shah, J., and Klessig, D. F. (2001). Environmentally sensitive, SA-dependent defense responses in the cpr22 mutant of Arabidopsis. Plant J. 26, 447–459. doi: 10.1046/j.1365-313X.2001.2641039.x
Yoshioka, K., Moeder, W., Kang, H. G., Kachroo, P., Masmoudi, K., Berkowitz, G., et al. (2006). The chimeric Arabidopsis CYCLIC NUCLEOTIDE-GATED ION CHANNEL11/12 activates multiple pathogen resistance responses. Plant Cell 18, 747–763. doi: 10.1105/tpc.105.038786
Yu, I. C., Parker, J., and Bent, A. F. (1998). Gene-for-gene disease resistance without the hypersensitive response in Arabidopsis dnd1 mutant. Proc. Natl. Acad. Sci. U. S. A. 95, 7819–7824. doi: 10.1073/pnas.95.13.7819
Yu, H., Yan, J., Du, X., and Hua, J. (2018). Overlapping and differential roles of plasma membrane calcium ATPases in Arabidopsis growth and environmental responses. J. Exp. Bot. 69, 2693–2703. doi: 10.1093/jxb/ery073
Yuan, F., Yang, H., Xue, Y., Kong, D., Ye, R., Li, C., et al. (2014). OSCA1 mediates osmotic-stress-evoked Ca2+ increases vital for osmosensing in Arabidopsis. Nature 514, 367–371. doi: 10.1038/nature13593
Zelman, A. K., Dawe, A., Gehring, C., and Berkowitz, G. A. (2012). Evolutionary and structural perspectives of plant cyclic nucleotide-gated cation channels. Front. Plant Sci. 3:95. doi: 10.3389/fpls.2012.00095
Zhang, L., Du, L., and Poovaiah, B. W. (2014). Calcium signaling and biotic defense responses in plants. Plant Signal. Behav. 9:e973818. doi: 10.4161/15592324.2014.973818
Zhang, X., Zhang, M., Takano, T., and Liu, S. (2011). Characterization of an AtCCX5 gene from Arabidopsis thaliana that involves in high-affinity K+ uptake and Na+ transport in yeast. Biochem. Biophys. Res. Commun. 414, 96–100. doi: 10.1016/j.bbrc.2011.09.030
Zhao, J., Barkla, B. J., Marshall, J., Pittman, J. K., and Hirschi, K. D. (2008). The Arabidopsis cax3 mutants display altered salt tolerance, pH sensitivity and reduced plasma membrane H+-ATPase activity. Planta 227, 659–669. doi: 10.1007/s00425-007-0648-2
Zhao, J., Shigaki, T., Mei, H., Guo, Y. Q., Cheng, N. H., and Hirschi, K. D. (2009). Interaction between Arabidopsis Ca2+/H+ exchangers CAX1 and CAX3. J. Biol. Chem. 284, 4605–4615. doi: 10.1074/jbc.M804462200
Zheng, Y., Luo, L., Wei, J., Chen, Q., Yang, Y., Hu, X., et al. (2018). The glutamate receptors AtGLR1.2 and AtGLR1.3 increase cold tolerance by regulating jasmonate signaling in Arabidopsis thaliana. Biochem. Biophys. Res. Commun. 506, 895–900. doi: 10.1016/j.bbrc.2018.10.153
Keywords: abiotic stress, biotic stress, calcium, Ca2+ influx, Ca2+ efflux, channels, transporters
Citation: Park C-J and Shin R (2022) Calcium channels and transporters: Roles in response to biotic and abiotic stresses. Front. Plant Sci. 13:964059. doi: 10.3389/fpls.2022.964059
Received: 08 June 2022; Accepted: 22 August 2022;
Published: 08 September 2022.
Edited by:
Tomoko Nozoye, Meiji Gakuin University, JapanCopyright © 2022 Park and Shin. This is an open-access article distributed under the terms of the Creative Commons Attribution License (CC BY). The use, distribution or reproduction in other forums is permitted, provided the original author(s) and the copyright owner(s) are credited and that the original publication in this journal is cited, in accordance with accepted academic practice. No use, distribution or reproduction is permitted which does not comply with these terms.
*Correspondence: Chang-Jin Park, Y2pwYXJrQHNlam9uZy5hYy5rcg==; Ryoung Shin, cnlvdW5nLnNoaW5AcmlrZW4uanA=
Disclaimer: All claims expressed in this article are solely those of the authors and do not necessarily represent those of their affiliated organizations, or those of the publisher, the editors and the reviewers. Any product that may be evaluated in this article or claim that may be made by its manufacturer is not guaranteed or endorsed by the publisher.
Research integrity at Frontiers
Learn more about the work of our research integrity team to safeguard the quality of each article we publish.