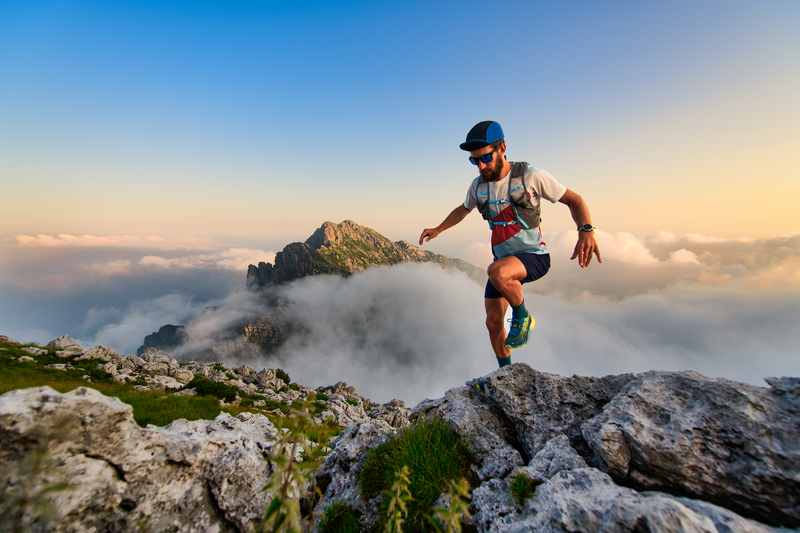
95% of researchers rate our articles as excellent or good
Learn more about the work of our research integrity team to safeguard the quality of each article we publish.
Find out more
REVIEW article
Front. Plant Sci. , 11 August 2022
Sec. Plant Membrane Traffic and Transport
Volume 13 - 2022 | https://doi.org/10.3389/fpls.2022.957995
This article is part of the Research Topic Regulation of Plant Organelle Biogenesis and Trafficking View all 8 articles
Membrane trafficking contributes to distinct protein compositions of organelles and is essential for proper organellar maintenance and functions. The trans-Golgi network (TGN) acts as a sorting station where various cargo proteins are sorted and directed to post-Golgi compartments, such as the multivesicular body or pre-vacuolar compartment, vacuoles, and plasma membrane. The spatial and temporal segregation of cargo proteins within the TGN, which is mediated with different sets of regulators including small GTPases and cargo adaptors, is a fundamental process in the sorting machinery. Recent studies with powerful imaging technologies have suggested that the TGN possesses spatially distinct subdomains or zones for different trafficking pathways. In this review, we will summarize the spatially and dynamically characteristic features of the plant TGN and their relation to cargo protein trafficking.
Membrane trafficking tightly regulates protein localization among organelles and plays a fundamental role in numerous biological processes such as cell growth, development, and stress responses. It is an evolutionarily conserved system among eukaryotes and consists of four fundamental processes: (1) forming transport carriers and sorting cargo proteins on donor organelle membranes; (2) transporting the carriers from the donor to the target organelles; (3) tethering; and (4) fusing them with the target organelle membrane (Fujimoto and Ueda, 2012; Figure 1). These processes are conducted with conserved key regulators and effectors, such as ADP-ribosylation factor/Secretion-associated Ras-related (Arf/Sar) GTPases, coat proteins, RAB GTPases, and soluble N-ethylmaleimide-sensitive factor attachment protein receptors (SNAREs).
Figure 1. Scheme of a single round of membrane trafficking. General process of membrane trafficking involves the vesicle formation and cargo sorting step, transport, tethering, and finally fusion. Some components of post-Golgi membrane trafficking are showed as representative regulators. Arf GTPase involves in the vesicle formation step. Coat proteins are responsible for the cargo protein sorting and the membrane distortion. RAB GTPase tethers the vesicle by interacting its effectors. SNAREs mediate the membrane fusion between the vesicle and the target membrane. This model is based on the AP-1/clathrin- or AP-2/clathrin-coated vesicles and may be different for other vesicles, such as AP-3-, AP-4-, or AP-5-coated vesicles. AP, adaptor protein; ENTH, epsin N-terminal homology domain; MTV1, MODIFIED TRANSPORT TO THE VACUOLE1.
First, Arf/Sar GTPases activated by their guanine-nucleotide exchange factor (GEF) promote the recruitment of coat proteins on the donor membrane. Coat proteins play important roles in cargo protein recognition and membrane distortion (Singh and Jürgens, 2018; Arora and Van Damme, 2021; Law et al., 2022). RAB GTPases are involved in various trafficking events, such as tethering (Saito and Ueda, 2009; Minamino and Ueda, 2019). SNAREs mainly function in the last step and can be divided into two groups: target membrane-localized Q-SNAREs and transport carrier-localized R-SNAREs. A specific combination of three Q-SNAREs and one R-SNARE is thought to mediate the fusion between the target membrane and transport carrier (Uemura and Ueda, 2014).
Biosynthetic trafficking starts in the endoplasmic reticulum (ER) where approximately one-third of proteins are synthesized. In normal anterograde membrane trafficking, the cis cisterna of the Golgi apparatus receives cargo proteins from the ER, whereas the trans cisterna sends them to their destinations, including the plasma membrane (PM) and vacuoles, via the trans Golgi network (TGN; Ito and Boutté, 2020; Figure 2). Therefore, the TGN lies in a branch of different trafficking routes and has long been suggested to be the site for cargo sorting. Recent studies have revealed that the TGN harbors spatially segregated functional subdomains or zones for differentially regulated trafficking routes (Heinze et al., 2020; Shimizu et al., 2021). In this review, we will summarize and discuss the recent discoveries that have been made on TGN-mediated trafficking.
Figure 2. Endomembrane system in plant cells. Plant endomembrane system at least consists of the endoplasmic reticulum (ER), the Golgi apparatus, the trans-Golgi network (TGN), the multivesicular body/pre-vacuolar compartment (MVB/PVC), the vacuole and the plasma membrane (PM). The plant TGN can be further classified into the GA-TGN and the GI-TGN. Putative RAB6-GEF LOT plays a critical role in the TGN biogenesis from the TGNs. Microtubule (MT)-binding protein TGNap1 regulates the GI-TGN biogenesis from the Golgi apparatus. The TGN contains cargo proteins derived from the Golgi apparatus, which are synthesized in the ER, and sorts these cargos for delivery to their destinations. In other words, the plant TGN is a branch point in secretory and vacuolar trafficking. In addition, endocytic vesicles labeled with FM4-64 first reach the GA-TGN. Thus, biosynthetic and endocytic trafficking converge at the GA-TGN. The solid arrows and dashed arrows indicate biosynthetic trafficking pathways and endocytic/recycling trafficking pathways, respectively. TGN-bypassing pathways are not shown.
The TGN is generally recognized as a membranous structure at the trans-side of the Golgi apparatus (Griffiths and Simons, 1986; Ito and Boutté, 2020), which partially corresponds to the compartment previously proposed as the Golgi-associated structure that is a part of the ER and forms Lysosomes (GERL) in the 1970s or termed the partially coated reticulum (PCR) in 1980s (Marty, 1978; Harris and Oparka, 1983; Pesacreta and Lucas, 1984; Staehelin et al., 1990). Morphologically distinct types of vesicles or multiple coat proteins have been found in the TGN, indicating that it is an important site for sorting cargo proteins with different destinations (Griffiths and Simons, 1986; Singh and Jürgens, 2018). Cargo proteins are thought to be transported from the Golgi to the TGN by the process of “cisternal maturation,” which has been well-studied in budding yeast (Glick and Nakano, 2009). According to this model, the trans-most Golgi cisterna is the cargo carrier and matures into the TGN without packing cargo molecules into the nascent transport carrier. Therefore, the TGN plays an essential role in sorting mixtures of biosynthetic cargo proteins with different destinations, which are derived from the Golgi apparatus. In partial support of this hypothesis, a knockout mutant of a Golgi-localized putative GEF for RAB6 (termed the “loss of TGN,” or LOT) was found to impair the biogenesis of the TGN, while the Golgi apparatus became over-stacked and elongated in Arabidopsis (Jia et al., 2018, 2019).
Interestingly, the plant TGN is a unique compartment that also functions as an early endosome where endocytosed molecules are first delivered. In a canonical model based on mammalian studies, endocytosed materials first reached the early endosome, which was physically separated from the TGN. They were then recycled back to the PM or redirected to lysosomes (Rohn et al., 2000; Barlow and Dacks, 2018). In plant cells, however, the lipophilic fluorescent dye FM4-64, which is gradually internalized from the PM by the endocytic machinery, first reached the TGN before other endomembrane organelles (Dettmer et al., 2006; Lam et al., 2007; Chow et al., 2008). Endocytic cargo proteins, such as BRASSINOSTEROID INSENSITIVE1 and REQUIRES HIGH BORON1, have also been reported to pass through the TGN (Viotti et al., 2010). A recent study involving the budding yeast Saccharomyces cerevisiae further reported that endocytosed FM4-64 first colocalized with the TGN marker (Day et al., 2018). These findings suggest that the TGN functions as an early endosome in plants and budding yeast and plays important roles not only in biosynthetic trafficking pathways, but also in endocytic trafficking pathways (Figure 2).
Although the concept of the TGN as the sorting platform in post-Golgi membrane trafficking is widely accepted, the mechanisms by which the TGN directs multiple sorting events remain unknown. To do this, the TGN must pack cargos with different destinations into distinct vesicles and/or sort them into different subdomains or zones. Recent studies have revealed that the TGN harbors at least two distinct domains or zones for different trafficking pathways (Heinze et al., 2020; Shimizu et al., 2021; Figure 3).
Figure 3. Golgi- and TGN-localized coat proteins and R-SNAREs regulate distinct pathways. TGN-localized coat proteins and R-SNAREs exhibit spatially distinct distributions. AP-1 (coat), EPSIN1 (coat), clathrin (coat), and VAMP721 (R-SNARE) comprise a subdomain or zone of the GA-TGN, which is involved in secretory trafficking. On the other hand, AP-4 (coat), MTV1 (coat), and VAMP727 (R-SNARE) comprise another subdomain or zone on the same GA-TGN, which is involved in vacuolar trafficking via the MVB/PVC. It remains controversial whether AP-4-mediated trafficking requires clathrin. The former subdomain or zone matures into the GI-TGN. The GI-TGN produces non-coated secretory and clathrin-coated vesicles. AP-1/clathrin-coated vesicles may play a role in retrograde trafficking from the GI-TGN. AP-3-coated vesicles may be formed at the trans-cisterna of the Golgi rather than the TGN and transport vacuolar cargo proteins, such as VAMP711 and VAMP713, to vacuoles independently of MVB/PVC. ER, endoplasmic reticulum; GA-TGN, Golgi-associated TGN; GI-TGN, Golgi-independent TGN; MVB/PVC, multivesicular body/pre-vacuolar compartment; PM, plasma membrane; AP, adaptor protein; MTV1, MODIFIED TRANSPORT TO THE VACUOLE1; VAMP, vesicle-associated membrane protein.
In a branch of the post-Golgi trafficking pathways, R-SNAREs with different destinations, VAMP721 and VAMP727, localize to the TGN. VAMP721 functions in the trafficking to the PM and cell plate, whereas VAMP727 mainly functions in the trafficking in the vacuolar pathway (Ebine et al., 2008; Kwon et al., 2008; Zhang et al., 2011; El Kasmi et al., 2013). Recently, we used super-resolution confocal live imaging microscopy (SCLIM) to reveal that VAMP721 and VAMP727 were spatially segregated as subdomains or zones, but not vesicles, within a single TGN (Shimizu et al., 2021). The VAMP721-localized subdomains and VAMP727-localized subdomains were proposed as the “secretory-trafficking zone” and “vacuolar-trafficking zone,” respectively. A similar segregation pattern was also seen in multiple coat proteins. The localizations of TGN-resident coat proteins, including adaptor protein complex 1 (AP-1), AP-4, EPSIN1, MODIFIED TRANSPORT TO THE VACUOLE1 (MTV1), and clathrin, have been investigated by SCLIM or high-resolution Airyscan imaging (Heinze et al., 2020; Shimizu et al., 2021). The comprehensive analyses revealed that the secretory-trafficking zone was enriched with VAMP721, AP-1, EPSIN1, and clathrin, whereas the vacuolar-trafficking zone was enriched with VAMP727, AP-4, and MTV1. Thus, secretory and vacuolar cargo proteins may be sorted via the spatially distinct zones within a single TGN.
In addition to its sub-organellar features, the plant TGN is classifiable at the spatiotemporal level. Fast live imaging by spinning disk confocal microscopy and 3D reconstruction by electron tomography have revealed that the plant TGN can dissociate from or associate with the Golgi apparatus (Staehelin and Kang, 2008; Viotti et al., 2010; Kang et al., 2011; Uemura et al., 2014). The canonical and non-canonical modes of the TGN have been termed as the Golgi-associated TGN (GA-TGN) and Golgi-independent TGN (free-TGN/GI-TGN), respectively. The detachment of the GI-TGN from the GA-TGN requires TGNap1, which likely functions as a linker between the TGN and microtubules (Renna et al., 2018). At the component level, the GA-TGN harbors VAMP721, or its close homolog VAMP722, and VAMP727. In contrast to the GA-TGN, the GI-TGN is enriched with VAMP721 and/or VAMP722, but not VAMP727, suggesting that the GI-TGN predominantly mediates secretory trafficking to the PM (Uemura et al., 2019). In the earlier stages (approximately 5 min after uptake), endocytosed FM4-64 colocalizes with the GA-TGN, AP-1, and AP-4, but not the GI-TGN (Uemura et al., 2019; Shimizu et al., 2021). These results highlight the importance of the GA-TGN as a sorting hub for secretory, vacuolar, and endocytic trafficking. The GI-TGN further harbors both AP-1/clathrin-coated and secretory vesicles, and the ratio of secretory vesicles to clathrin-coated vesicles can vary, even within single cells (Kang et al., 2011; Shimizu et al., 2021).
Heterotetrameric AP complexes and clathrin are well-characterized coat proteins and function in post-Golgi membrane trafficking. Five AP complexes (AP-1–AP-5) are derived from a common ancestral complex and are thought to have been present in the last eukaryotic common ancestor (Hirst et al., 2011; Dacks and Robinson, 2017). Hence, they are evolutionarily conserved in eukaryotes. AP complexes typically consist of two large subunits (β and α/γ/δ/ε/ζ), a medium subunit (μ), and a small subunit (σ). They sort membrane-bound or transmembrane cargo proteins by recognizing and binding to sorting motifs or signals, such as the tyrosine motif, dileucine motif, and ubiquitin, which are present in the cytoplasmic domains of cargo proteins (Arora and Van Damme, 2021; Law et al., 2022). Various AP complexes interact with clathrin to form clathrin-coated vesicles. Well-established examples include AP-1/clathrin-coated vesicles (Park et al., 2013) and AP-2/clathrin-coated vesicles (Di Rubbo et al., 2013; Kim et al., 2013; Yamaoka et al., 2013). Each of the AP complexes functions in distinct trafficking pathways in eukaryotes. In Arabidopsis, both AP-1 and AP-4 function in the TGN (Park et al., 2013; Teh et al., 2013; Wang et al., 2013; Fuji et al., 2016).
AP-1 is essential for survival as ap1γ1 ap1γ2 double mutants cannot be obtained (Wang et al., 2014). ap1μ2 (major isoform of AP-1 μ subunits) mutants have retarded growth and are unable to produce progenies (Park et al., 2013). In contrast to AP-4 as described below, AP-1 seems to play roles in a wide range of trafficking pathways and localizes to the TGN secretory-trafficking zone with VAMP721. For example, secretory invertase and GFP are not properly secreted into the extracellular medium, whereas the vacuolar proteins sporamin and βFructosidase4 are unprocessed in ap1m2-1/hap13-1 protoplasts in Arabidopsis. Furthermore, PIN-FORMED2 (PIN2) polar recycling from the brefeldin A (BFA) compartment to the PM or vacuoles is compromised in ap1m2-1/hap13-1 seedlings. BRASSINOSTEROID INSENSITIVE1 recycling from the BFA compartment to the PM is also compromised in ap1m2-1/hap13-1 seedlings (Park et al., 2013; Wang et al., 2013). It has also been reported that the AP-1 μ subunit can bind transmembrane receptors for vacuolar soluble proteins known as vacuolar sorting receptors (VSRs; Park et al., 2013; Gershlick et al., 2014; Nishimura et al., 2016). In addition, the trafficking of cytokinesis-specific SNARE KNOLLE/SYP111 to the cell plate is severely impaired, leading to incomplete cytokinesis (Park et al., 2013; Teh et al., 2013). Severe growth defects in ap1m2-1/hap13-1 mutants can be rescued by expressing functional AP1M2 with the KNOLLE/SYP111 promoter as well as AP1M2 promoter (Teh et al., 2013; Shimada et al., 2018). Interestingly, outermost integument cells of the pKNOLLE:AP1M2-GFP-rescued ap1m2-1/hap13-1 seed lack AP1M2 expression and exhibit reduced mucilage extrusion and its abnormal accumulation in vacuoles. This suggests that AP-1 is also involved in the secretory trafficking of macromolecules and cargo proteins (Shimada et al., 2018). Furthermore, the disruption of AP-1 function has been found to reduce the PM association of AP-2, clathrin, and the clathrin adaptor TPLATE complex. This impairs clathrin-mediated endocytosis and vice versa (Yan et al., 2021). Therefore, AP-1/clathrin-mediated trafficking from the TGN and AP-2/clathrin-mediated trafficking from the PM couple by unknown mechanisms. AP-1 is therefore involved in the secretory, vacuolar, and recycling trafficking pathways, although it remains unclear how AP-1 can manage such complicated sorting events.
Furthermore, we have assessed the role of AP-1 from the spatiotemporal perspective. SCLIM observations have revealed that AP-1- and clathrin-labeled compartments leave the GA-TGN with SYNTAXIN OF PLANTS61 (SYP61) as components of the GI-TGN, while AP-4 seems to stay with the GA-TGN (Shimizu et al., 2021). Electron tomography has further revealed that the GI-TGN harbors clathrin-coated and secretory vesicles (Kang et al., 2011). These findings suggest that AP-1/clathrin-coated vesicles may function in the GI-TGN. Intriguingly, Yan et al. recently reported that the TGN-resident SNAREs SYP41 and SYP61, but not VHAa1, were significantly dissociated from the TGN and mislocalized to the PM in ap1m2/hap13 root cells. This suggests that AP-1 is responsible for maintaining the functional integrity of the TGN (Yan et al., 2021). In support of this hypothesis, ap1m2-1/hap13-1 mutants have been found to exhibit abnormal morphologies in the Golgi and TGN (Park et al., 2013). It has also been reported that SYP4s and SYP61 regulate both secretory and vacuolar trafficking (Bassham et al., 2000; Uemura et al., 2012; Hachez et al., 2014; Lu et al., 2020). These findings indicate the possibility that AP-1/clathrin-coated vesicles may deliver TGN-resident proteins and missorted vacuolar proteins from the GI-TGN to the GA-TGN or Golgi apparatus. Recent studies involving budding yeast have further reported that AP-1 plays a role in the retrograde transport of cargo proteins from mature to young Golgi cisternae (Casler et al., 2019, 2022; Casler and Glick, 2020). It should be noted that, although the mechanisms are unknown, the quantity of GA-TGN-localized proteins seems to be maintained at a relatively stable level, as the fluorescence of TGN markers, including SYP43 and SYP61, seems to be recovered around the GA-TGN region after producing the GI-TGN (Uemura et al., 2014; Shimizu et al., 2021).
In a forward genetic screen in Arabidopsis, AP-4 components were reported to be responsible for the vacuolar sorting of GFP-CT24, which is an artificial cargo protein with β-conglycinin-derived vacuolar targeting signals (Fuji et al., 2007, 2016). The tonoplast proteins molybdate transporter 2 and natural resistance macrophage protein 3 and 4 were missorted to the PM in ap4m mesophyll protoplasts (Müdsam et al., 2018). In addition, the μ subunit of AP-4 and that of AP-1 mentioned above have been found to bind to the tyrosine motifs of VSR1 and VSR2 (Gershlick et al., 2014; Fuji et al., 2016). Given that the putative cargo VAMP727 mainly functions in the multivesicular body (MVB; Ebine et al., 2008) and that the VSR-mediated trafficking pathway relies on the MVB to deliver cargo proteins to vacuoles, AP-4 likely functions as a gatekeeper in the pathway from the TGN to MVB, although it remains controversial where VSR itself travels (Ivanov and Robinson, 2020). MTV1, an accessory protein of AP-4, has also been identified as a responsible gene for the vacuolar trafficking of the artificial cargo protein VAC2 (Sauer et al., 2013). Thus, genetic and biochemical analyses support the role of AP-4 in vacuolar trafficking. The question remains as to whether plant AP-4 is a component of clathrin-coated vesicles in vivo. Coimmunoprecipitation assays have shown that the μ subunit of AP-4 immunoprecipitates clathrin heavy chains (Fuji et al., 2016; Shimizu et al., 2021). However, SCLIM observations of Arabidopsis root cells have shown that AP-4 is segregated from clathrin as opposed to AP-1 (Shimizu et al., 2021). Recently, Dahhan et al. characterized the proteome of purified plant clathrin-coated vesicles and found that AP-4 was enriched in the clathrin-coated vesicle fraction (Dahhan et al., 2022). Thus, it remains controversial whether clathrin is required for plant AP-4-mediated trafficking in vivo. The way in which AP-4 localizes to the TGN also remains unknown. Although the ARF-GEFs BIG1–BIG4 and ARF1 have been shown to recruit AP-1 to the TGN membrane (Richter et al., 2014; Singh et al., 2018), it is unclear which ARF-GEFs and ARFs are required for localizing AP-4 to the TGN.
Several vacuolar proteins are transported to vacuoles independently of MVB-mediated pathways. A striking example is the AP-3-dependent pathway. The vacuolar membrane targeting of VAMP711/VAMP713 and PROTEIN S-ACYL TRANSFERASE10 is mediated by AP-3 independent of the MVB-pathway requiring RAB5 (Ebine et al., 2014; Feng et al., 2018). Studies in mammalian models have reported that AP-3 sorts lysosomal cargo proteins in the TGN and endosomes (Peden et al., 2004; Huang et al., 2019). However, it remains unclear whether plant AP-3 constitutes a distinct zone within the TGN like AP-1 and AP-4 since the localization of AP-3 is not well defined. Fluorescent protein-labeled AP-3 exhibits a relatively high degree of colocalization with the Golgi markers WAVE22/SYP32 and TGN marker VHAa1 (Feng et al., 2017). Studies on mammalian cells and the biochemical interactions of plant AP-3 with clathrin (Zwiewka et al., 2011) may confuse the precise interpretation of AP-3 localization in plant cells. Intriguingly, PROTEIN S-ACYL TRANSFERASE10 seems to be retained in the Golgi but not TGN in ap3δ mutants, suggesting that AP-3 sorts vacuolar cargo proteins at the Golgi apparatus rather than the TGN (Feng et al., 2018). Further investigations on AP-3 localization will contribute to a better understanding why and how vacuolar trafficking is regulated by different components.
The studies on secretory-trafficking and vacuolar-trafficking zones marked with VAMP721 and VAMP727, respectively, appear to be oversimplified. For example, there are two different routes from the TGN to the PM. The most striking discovery is that the polar recycling of PIN1 to the basal PM is regulated by ARF-GEF GNOM, whereas the recycling of apical, lateral, and nonpolar PM proteins is regulated by RABA2a (Geldner et al., 2003; Li et al., 2017). In these studies, BFA and Endosidin16, chemical inhibitors of membrane trafficking, are used as a powerful tool to dissect the different trafficking routes. BFA binds between the GDP-bound ARF/SAR and its GEF, thereby blocking a subset of secretion from the Golgi and/or TGN and early secretory trafficking from the ER to the Golgi (Jackson and Casanova, 2000). Endosidin16 compromises RABA2A-mediated trafficking from the TGN (Li et al., 2017). It should be noted that the establishment and maintenance of PIN polarity are coordinated by endocytosis and polar recycling (Geldner et al., 2003; Men et al., 2008; Kitakura et al., 2011; Glanc et al., 2018), as well as the de novo delivery of PIN2 (Wattelet-Boyer et al., 2016).
Rodriguez-Furlan et al. recently reported that newly synthesized INFLORESCENCE AND ROOT APICES RECEPTOR KINASE (IRK) and KINASE ON THE INSIDE (KOIN) are sorted at the TGN by different machineries and transported to the opposite sides (i.e., outer and inner sides, respectively) of the root endodermal PM. These different de novo pathways have also been demonstrated using BFA and Endosidin16 in combination with or without the protein synthesis inhibitor cycloheximide. Trafficking of IRK is compromised with BFA but not Endosidin16, whereas that of KOIN is sensitive to Endosidin16 but not BFA (Rodriguez-Furlan et al., 2022). Interestingly, the PM targeting of both IRK and KOIN is also compromised with Endosidin2 (Rodriguez-Furlan et al., 2022). Endosidin2 inhibits exocytosis by binding the EXOCYST subunit EXO70A1 that plays important roles in targeted secretion and tethering at the PM (Zhang et al., 2016). These results suggest that IRK and KOIN are sorted into distinct trafficking pathways at the TGN; however, they are regulated by EXOCYST-mediated tethering mechanisms on the way to and/or at the PM. As some cargo proteins are exported from the TGN by different machinery, so some cargo proteins are regulated by distinct tethering and SNARE proteins on the target membrane. Plasma membrane-localized Q-SNAREs SYP121/ PEN1 (PENETRATION1) and SYP122 have partially redundant functions, since syp121 syp122 double mutants display growth defects that are not observed in either single mutant (Collins et al., 2003; Assaad et al., 2004; Zhang et al., 2007). However, mass spectrometry analysis using the syp121 and syp122 mutants revealed that SYP121 and SYP122 mediate the secretion of partially overlapping but distinct sets of distinct cargo proteins (Waghmare et al., 2018). Other studies also show that K+ channels bind selectively to SYP121 but not SYP122 (Honsbein et al., 2009, 2011; Grefen et al., 2010). The RABA2a-dependent and RABE1-EXOCYST-dependent secretory pathways, which coexist in Arabidopsis, have been also proposed (Pang et al., 2022). Given these studies, the secretory-trafficking zone of the TGN may further be classified into the sub-zone level or the single-vesicle level.
It is now clear that the plant TGN is the central hub of secretory, vacuolar, and endocytic trafficking. Accumulating evidence suggests that the TGN can be divided into subdomains or zones where distinct trafficking events are executed. Such subdomain/zone-like segregation has been seen in various TGN-localized proteins including coat proteins, SNAREs, and tethering factors (Bassham et al., 2000; Chow et al., 2008; Gendre et al., 2011, 2013; Boutté et al., 2013; Wattelet-Boyer et al., 2016; Ravikumar et al., 2018; Renna et al., 2018; Heinze et al., 2020; Shimizu et al., 2021). However, it remains poorly understood how these subdomains or zones are organized. Lipid composition of the organelle membrane can regulate protein localization and thus plays an important role in endomembrane trafficking and diverse cellular functions (Bigay and Antonny, 2012; Holthuis and Menon, 2014; Boutté and Jaillais, 2020). Interestingly, it has been reported that α-hydroxylated-very long chain fatty acid-containing sphingolipids are specifically enriched in the immunoisolated SYP61-, but not RABA2a-, localized TGN (Wattelet-Boyer et al., 2016). Pharmacological reduction of very long chain fatty acid-containing sphingolipids disturbs the polar delivery of PIN2 (Wattelet-Boyer et al., 2016). Furthermore, α-hydroxylated-very long chain fatty acids are involved in regulating the quantity of phosphatidylinositol 4-phosphate (PI4P) in the TGN (Ito et al., 2021). In plant cells, PI4P mainly accumulates at the PM and to a lesser extent at the TGN. TGN-localized phosphatidylinositol 4-kinases pi4kβ1 pi4kβ2 double mutants have the abnormal TGN with highly variable sizes of secretory vesicles and exhibit pleiotropic growth defects (Preuss et al., 2006; Kang et al., 2011; Sašek et al., 2014; Antignani et al., 2015; Lin et al., 2019). In Arabidopsis, ARF-GAP VASCULAR NETWORK DEFECTIVE3 and RABA4b effector PLANT U-BOX13 have been shown to bind PI4P and to localize to the TGN (Koizumi et al., 2005; Naramoto et al., 2009; Antignani et al., 2015). It has also been reported that the mammalian AP-1 complex binds PI4P (Wang et al., 2003). Therefore, distinct lipid environments likely serve as platforms for organizing subdomains or zones in the TGN, which will be investigated in the near future. To better understand sub-organellar features, cutting-edge imaging technologies are essential. For example, correlative light and electron microscopy will fill the gaps in the current knowledge on morphological features and fluorescence-based protein localization (Wang and Kang, 2020). In addition, to better understand how cargo proteins are sorted into specific TGN subdomains/zones, we should evaluate the association between the organization of trafficking zones and cargo flow. Because the passage of cargo proteins is transient, it is necessary to establish a pulse-chase-type experimental system. Several methods have been proposed and successfully applied in other organisms, such as retention using a selective hooks system in mammalian cultured cells (Boncompain et al., 2012) and a temperature-controlled system in budding yeast (Kurokawa et al., 2014). However, such systems have not yet been established in plant cells. Given that membrane trafficking appears to be intricately regulated during developmental processes and environmental changes, it is important to investigate how cargo proteins are transported in various conditions.
YS and TU wrote and edited the manuscript and prepared the figure. All authors contributed to the article and approved the submitted version.
This work was supported by Grants-in-Aid for Scientific Research from the Ministry of Education, Culture, Sports, Science and Technology (grant number: 18H04857 to TU), JST (grant number: JPMJCR20E5 to TU), Grant for Basic Science Research Project from Sumitomo Foundation (to TU), and JSPS Research Fellowship for Young Scientists (to YS).
The authors declare that the research was conducted in the absence of any commercial or financial relationships that could be construed as a potential conflict of interest.
All claims expressed in this article are solely those of the authors and do not necessarily represent those of their affiliated organizations, or those of the publisher, the editors and the reviewers. Any product that may be evaluated in this article, or claim that may be made by its manufacturer, is not guaranteed or endorsed by the publisher.
Antignani, V., Klocko, A. L., Bak, G., Chandrasekaran, S. D., Dunivin, T., and Nielsen, E. (2015). Recruitment of PLANT U-BOX13 and the PI4Kβ1/β2 phosphatidylinositol-4 kinases by the small GTPase RabA4B plays important roles during salicylic acid-mediated plant defense signaling in Arabidopsis. Plant Cell 27, 243–261. doi: 10.1105/tpc.114.134262
Arora, D., and Van Damme, D. V. (2021). Motif-based endomembrane trafficking. Plant Physiol. 186, 221–238. doi: 10.1093/plphys/kiab077
Assaad, F. F., Qiu, J. L., Youngs, H., Ehrhardt, D., Zimmerli, L., Kalde, M., et al. (2004). The PEN1 syntaxin defines a novel cellular compartment upon fungal attack and is required for the timely assembly of papillae. Mol. Biol. Cell 15, 5118–5129. doi: 10.1091/mbc.e04-02-0140
Barlow, L. D., and Dacks, J. B. (2018). Seeing the endomembrane system for the trees: evolutionary analysis highlights the importance of plants as models for eukaryotic membrane-trafficking. Semin. Cell Dev. Biol. 80, 142–152. doi: 10.1016/j.semcdb.2017.09.027
Bassham, D. C., Sanderfoot, A. A., Kovaleva, V., Zheng, H., and Raikhel, N. V. (2000). AtVPS45 complex formation at the trans-Golgi network. Mol. Biol. Cell 11, 2251–2265. doi: 10.1091/mbc.11.7.2251
Bigay, J., and Antonny, B. (2012). Curvature, lipid packing, and electrostatics of membrane organelles: defining cellular territories in determining specificity. Dev. Cell 23, 886–895. doi: 10.1016/j.devcel.2012.10.009
Boncompain, G., Divoux, S., Gareil, N., de Forges, H., Lescure, A., Latreche, L., et al. (2012). Synchronization of secretory protein traffic in populations of cells. Nat. Methods 9, 493–498. doi: 10.1038/nmeth.1928
Boutté, Y., and Jaillais, Y. (2020). Metabolic cellular communications: feedback mechanisms between membrane lipid homeostasis and plant development. Dev. Cell 54, 171–182. doi: 10.1016/j.devcel.2020.05.005
Boutté, Y., Jonsson, K., McFarlane, H. E., Johnson, E., Gendre, D., Swarup, R., et al. (2013). ECHIDNA-mediated post-Golgi trafficking of auxin carriers for differential cell elongation. Proc. Natl. Acad. Sci. U. S. A. 110, 16259–16264. doi: 10.1073/pnas.1309057110
Casler, J. C., and Glick, B. S. (2020). A microscopy-based kinetic analysis of yeast vacuolar protein sorting. elife 9:e56844. doi: 10.7554/eLife.56844
Casler, J. C., Johnson, N., Krahn, A. H., Pantazopoulou, A., Day, K. J., and Glick, B. S. (2022). Clathrin adaptors mediate two sequential pathways of intra-Golgi recycling. J. Cell Biol. 221:e202103199. doi: 10.1083/jcb.202103199
Casler, J. C., Papanikou, E., Barrero, J. J., and Glick, B. S. (2019). Maturation-driven transport and AP-1–dependent recycling of a secretory cargo in the Golgi. J. Cell Biol. 218, 1582–1601. doi: 10.1083/jcb.201807195
Chow, C. M., Neto, H., Foucart, C., and Moore, I. (2008). Rab-A2 and Rab-A3 GTPases define a trans-Golgi endosomal membrane domain in Arabidopsis that contributes substantially to the cell plate. Plant Cell 20, 101–123. doi: 10.1105/tpc.107.052001
Collins, N. C., Thordal-Christensen, H., Lipka, V., Bau, S., Kombrink, E., Qiu, J. L., et al. (2003). SNARE-protein-mediated disease resistance at the plant cell wall. Nature 425, 973–977. doi: 10.1038/nature02076
Dacks, J. B., and Robinson, M. S. (2017). Outerwear through the ages: evolutionary cell biology of vesicle coats. Curr. Opin. Cell Biol. 47, 108–116. doi: 10.1016/j.ceb.2017.04.001
Dahhan, D. A., Reynolds, G. D., Cárdenas, J. J., Eeckhout, D., Johnson, A., Yperman, K., et al. (2022). Proteomic characterization of isolated Arabidopsis clathrin-coated vesicles reveals evolutionarily conserved and plant-specific components. Plant Cell 34, 2150–2173. doi: 10.1093/plcell/koac071
Day, K. J., Casler, J. C., and Glick, B. S. (2018). Budding yeast has a minimal endomembrane system. Dev. Cell 44:e4, 56–72. doi: 10.1016/j.devcel.2017.12.014
Dettmer, J., Hong-Hermesdorf, A., Stierhof, Y. D., and Schumacher, K. (2006). Vacuolar H+-ATPase activity is required for endocytic and secretory trafficking in Arabidopsis. Plant Cell 18, 715–730. doi: 10.1105/tpc.105.037978
Di Rubbo, S., Irani, N. G., Kim, S. Y., Xu, Z. Y., Gadeyne, A., Dejonghe, W., et al. (2013). The clathrin adaptor complex AP-2 mediates endocytosis of brassinosteroid insensitive1 in Arabidopsis. Plant Cell 25, 2986–2997. doi: 10.1105/tpc.113.114058
Ebine, K., Inoue, T., Ito, J., Ito, E., Uemura, T., Goh, T., et al. (2014). Plant vacuolar trafficking occurs through distinctly regulated pathways. Curr. Biol. 24, 1375–1382. doi: 10.1016/j.cub.2014.05.004
Ebine, K., Okatani, Y., Uemura, T., Goh, T., Shoda, K., Niihama, M., et al. (2008). A SNARE complex unique to seed plants is required for protein storage vacuole biogenesis and seed development of Arabidopsis thaliana. Plant Cell 20, 3006–3021. doi: 10.1105/tpc.107.057711
El Kasmi, F., Krause, C., Hiller, U., Stierhof, Y. D., Mayer, U., Conner, L., et al. (2013). SNARE complexes of different composition jointly mediate membrane fusion in Arabidopsis cytokinesis. Mol. Biol. Cell 24, 1593–1601. doi: 10.1091/mbc.e13-02-0074
Feng, Q. N., Liang, X., Li, S., and Zhang, Y. (2018). The ADAPTOR PROTEIN-3 complex mediates pollen tube growth by coordinating vacuolar targeting and organization. Plant Physiol. 177, 216–225. doi: 10.1104/pp.17.01722
Feng, Q. N., Song, S. J., Yu, S. X., Wang, J. G., Li, S., and Zhang, Y. (2017). Adaptor protein-3-dependent vacuolar trafficking involves a subpopulation of COPII and HOPS tethering proteins. Plant Physiol. 174, 1609–1620. doi: 10.1104/pp.17.00584
Fuji, K., Shimada, T., Takahashi, H., Tamura, K., Koumoto, Y., Utsumi, S., et al. (2007). Arabidopsis vacuolar sorting mutants (green fluorescent seed) can be identified efficiently by secretion of vacuole-targeted green fluorescent protein in their seeds. Plant Cell 19, 597–609. doi: 10.1105/tpc.106.045997
Fuji, K., Shirakawa, M., Shimono, Y., Kunieda, T., Fukao, Y., Koumoto, Y., et al. (2016). The adaptor complex AP-4 regulates vacuolar protein sorting at the trans-Golgi network by interacting with vacuolar SORTING RECEPTOR1. Plant Physiol. 170, 211–219. doi: 10.1104/pp.15.00869
Fujimoto, M., and Ueda, T. (2012). Conserved and plant-unique mechanisms regulating plant post-Golgi traffic. Front. Plant Sci. 3:197. doi: 10.3389/fpls.2012.00197
Geldner, N., Anders, N., Wolters, H., Keicher, J., Kornberger, W., Muller, P., et al. (2003). The Arabidopsis GNOM ARF-GEF mediates endosomal recycling, auxin transport, and auxin-dependent plant growth. Cell 112, 219–230. doi: 10.1016/S0092-8674(03)00003-5
Gendre, D., McFarlane, H. E., Johnson, E., Mouille, G., Sjödin, A., Oh, J., et al. (2013). Trans-Golgi network localized ECHIDNA/Ypt interacting protein complex is required for the secretion of cell wall polysaccharides in Arabidopsis. Plant Cell 25, 2633–2646. doi: 10.1105/tpc.113.112482
Gendre, D., Oh, J., Boutté, Y., Best, J. G., Samuels, L., Nilsson, R., et al. (2011). Conserved Arabidopsis ECHIDNA protein mediates trans–Golgi-network trafficking and cell elongation. Proc. Natl. Acad. Sci. U. S. A. 108, 8048–8053. doi: 10.1073/pnas.1018371108
Gershlick, D. C., Lousa, C. M., Foresti, O., Lee, A. J., Pereira, E. A., da Silva, L. L. P., et al. (2014). Golgi-dependent transport of vacuolar sorting receptors is regulated by COPII, AP1, and AP4 protein complexes in tobacco. Plant Cell 26, 1308–1329. doi: 10.1105/tpc.113.122226
Glanc, M., Fendrych, M., and Friml, J. (2018). Mechanistic framework for cell-intrinsic re-establishment of PIN2 polarity after cell division. Nat. Plants. 4, 1082–1088. doi: 10.1038/s41477-018-0318-3
Glick, B. S., and Nakano, A. (2009). Membrane traffic within the Golgi apparatus. Annu. Rev. Cell Dev. Biol. 25, 113–132. doi: 10.1146/annurev.cellbio.24.110707.175421
Grefen, C., Chen, Z., Honsbein, A., Donald, N., Hills, A., and Blatt, M. R. (2010). A novel motif essential for SNARE interaction with the K+ channel KC1 and channel gating in Arabidopsis. Plant Cell 22, 3076–3092. doi: 10.1105/tpc.110.077768
Griffiths, G., and Simons, K. (1986). The trans Golgi network: sorting at the exit site of the Golgi complex. Science 234, 438–443. doi: 10.1126/science.2945253
Hachez, C., Laloux, T., Reinhardt, H., Cavez, D., Degand, H., Grefen, C., et al. (2014). Arabidopsis SNAREs SYP61 and SYP121 coordinate the trafficking of plasma membrane aquaporin PIP2;7 to modulate the cell membrane water permeability. Plant Cell 26, 3132–3147. doi: 10.1105/tpc.114.127159
Harris, N., and Oparka, K. J. (1983). Connections between dictyosomes, ER and GERL in cotyledons of mung bean (Vigna radiata L.). Protoplasma 114, 93–102. doi: 10.1007/BF01279872
Heinze, L., Freimuth, N., Rößling, A. K., Hahnke, R., Riebschläger, S., Fröhlich, A., et al. (2020). EPSIN1 and MTV1 define functionally overlapping but molecularly distinct trans-Golgi network subdomains in Arabidopsis. Proc. Natl. Acad. Sci. U. S. A. 117, 25880–25889. doi: 10.1073/pnas.2004822117
Hirst, J., Barlow, L. D., Francisco, G. C., Sahlender, D. A., Seaman, M. N. J., Dacks, J. B., et al. (2011). The fifth adaptor protein complex. PLoS Biol. 9:e1001170. doi: 10.1371/journal.pbio.1001170
Holthuis, J. C. M., and Menon, A. K. (2014). Lipid landscapes and pipelines in membrane homeostasis. Nature 510, 48–57. doi: 10.1038/nature13474
Honsbein, A., Blatt, M. R., and Grefen, C. (2011). A molecular framework for coupling cellular volume and osmotic solute transport control. J. Exp. Bot. 62, 2363–2370. doi: 10.1093/jxb/erq386
Honsbein, A., Sokolovski, S., Grefen, C., Campanoni, P., Pratelli, R., Paneque, M., et al. (2009). A tripartite SNARE-K+ channel complex mediates in channel-dependent K+ nutrition in Arabidopsis. Plant Cell 21, 2859–2877. doi: 10.1105/tpc.109.066118
Huang, Y., Ma, T., Lau, P. K., Wang, J., Zhao, T., Du, S., et al. (2019). Visualization of protein sorting at the trans-Golgi network and endosomes through super-resolution imaging. Front. Cell Dev. Biol. 7:181. doi: 10.3389/fcell.2019.00181
Ito, Y., and Boutté, Y. (2020). Differentiation of trafficking pathways at Golgi entry core compartments and post-Golgi subdomains. Front. Plant Sci. 11:609516. doi: 10.3389/fpls.2020.609516
Ito, Y., Esnay, N., Platre, M. P., Wattelet-Boyer, V., Noack, L. C., Fougère, L., et al. (2021). Sphingolipids mediate polar sorting of PIN2 through phosphoinositide consumption at the trans-Golgi network. Nat. Commun. 12:4267. doi: 10.1038/s41467-021-24548-0
Ivanov, R., and Robinson, D. G. (2020). EMAC, Retromer, and VSRs: do they connect? Protoplasma 257, 1725–1729. doi: 10.1007/s00709-020-01543-8
Jackson, C. L., and Casanova, J. E. (2000). Turning on ARF: the Sec7 family of guanine-nucleotide-exchange factors. Trends Cell Biol. 10, 60–67. doi: 10.1016/S0962-8924(99)01699-2
Jia, P. F., Xue, Y., Li, H. J., and Yang, W. C. (2018). Golgi-localized LOT regulates trans-Golgi network biogenesis and pollen tube growth. Proc. Natl. Acad. Sci. U. S. A. 115, 12307–12312. doi: 10.1073/pnas.1809206115
Jia, P. F., Xue, Y., Li, H. J., and Yang, W. C. (2019). LOT regulates TGN biogenesis and Golgi structure in plants. Plant Signal. Behav. 14:e1573100. doi: 10.1080/15592324.2019.1573100
Kang, B. H., Nielsen, E., Preuss, M. L., Mastronarde, D., and Staehelin, L. A. (2011). Electron tomography of RabA4b- and PI-4Kβ1-labeled trans Golgi network compartments in Arabidopsis. Traffic 12, 313–329. doi: 10.1111/j.1600-0854.2010.01146.x
Kim, S. Y., Xu, Z. Y., Song, K., Kim, D. H., Kang, H., Reichardt, I., et al. (2013). Adaptor protein complex 2-mediated endocytosis is crucial for male reproductive organ development in Arabidopsis. Plant Cell 25, 2970–2985. doi: 10.1105/tpc.113.114264
Kitakura, S., Vanneste, S., Robert, S., Löfke, C., Teichmann, T., Tanaka, H., et al. (2011). Clathrin mediates endocytosis and polar distribution of PIN auxin transporters in Arabidopsis. Plant Cell 23, 1920–1931. doi: 10.1105/tpc.111.083030
Koizumi, K., Naramoto, S., Sawa, S., Yahara, N., Ueda, T., Nakano, A., et al. (2005). VAN3 ARF-GAP-mediated vesicle transport is involved in leaf vascular network formation. Development 132, 1699–1711. doi: 10.1242/dev.01716
Kurokawa, K., Okamoto, M., and Nakano, A. (2014). Contact of cis-Golgi with ER exit sites executes cargo capture and delivery from the ER. Nat. Commun. 5:3653. doi: 10.1038/ncomms4653
Kwon, C., Neu, C., Pajonk, S., Yun, H. S., Lipka, U., Humphry, M., et al. (2008). Co-option of a default secretory pathway for plant immune responses. Nature 451, 835–840. doi: 10.1038/nature06545
Lam, S. K., Siu, C. L., Hillmer, S., Jang, S., An, G., Robinson, D. G., et al. (2007). Rice SCAMP1 defines Clathrin-coated, trans-Golgi–located tubular-vesicular structures as an early endosome in tobacco BY-2 cells. Plant Cell 19, 296–319. doi: 10.1105/tpc.106.045708
Law, K. C., Chung, K. K., and Zhuang, X. (2022). An update on coat protein complexes for vesicle formation in plant post-Golgi trafficking. Front. Plant Sci. 13:826007. doi: 10.3389/fpls.2022.826007
Li, R., Rodriguez-Furlan, C., Wang, J., van de Ven, W., Gao, T., Raikhel, N. V., et al. (2017). Different endomembrane trafficking pathways establish apical and basal polarities. Plant Cell 29, 90–108. doi: 10.1105/tpc.16.00524
Lin, F., Krishnamoorthy, P., Schubert, V., Hause, G., Heilmann, M., and Heilmann, I. (2019). A dual role for cell plate-associated PI4Kβ in endocytosis and phragmoplast dynamics during plant somatic cytokinesis. EMBO J. 38:303. doi: 10.15252/embj.2018100303
Lu, C., Feng, Z., Yuan, F., Han, G., Guo, J., Chen, M., et al. (2020). The SNARE protein LbSYP61 participates in salt secretion in Limonium bicolor. Environ. Exp. Bot. 176:104076. doi: 10.1016/j.envexpbot.2020.104076
Marty, F. (1978). Cytochemical studies on GERL, provacuoles, and vacuoles in root meristematic cells of Euphorbia. Proc. Natl. Acad. Sci. U. S. A. 75, 852–856. doi: 10.1073/pnas.75.2.852
Men, S., Boutté, Y., Ikeda, Y., Li, X., Palme, K., Stierhof, Y. D., et al. (2008). Sterol-dependent endocytosis mediates post-cytokinetic acquisition of PIN2 auxin efflux carrier polarity. Nat. Cell Biol. 10, 237–244. doi: 10.1038/ncb1686
Minamino, N., and Ueda, T. (2019). RAB GTPases and their effectors in plant endosomal transport. Curr. Opin. Plant Biol. 52, 61–68. doi: 10.1016/j.pbi.2019.07.007
Müdsam, C., Wollschläger, P., Sauer, N., and Schneider, S. (2018). Sorting of Arabidopsis NRAMP3 and NRAMP4 depends on adaptor protein complex AP4 and a dileucine-based motif. Traffic 19, 503–521. doi: 10.1111/tra.12567
Naramoto, S., Sawa, S., Koizumi, K., Uemura, T., Ueda, T., Friml, J., et al. (2009). Phosphoinositide-dependent regulation of VAN3 ARF-GAP localization and activity essential for vascular tissue continuity in plants. Development 136, 1529–1538. doi: 10.1242/dev.030098
Nishimura, K., Matsunami, E., Yoshida, S., Kohata, S., Yamauchi, J., Jisaka, M., et al. (2016). The tyrosine-sorting motif of the vacuolar sorting receptor VSR4 from Arabidopsis thaliana, which is involved in the interaction between VSR4 and AP1M2, μ1-adaptin type 2 of clathrin adaptor complex 1 subunits, participates in the post-Golgi sorting of VSR4. Biosci. Biotechnol. Biochem. 80, 694–705. doi: 10.1080/09168451.2015.1116925
Pang, L., Ma, Z., Zhang, X., Huang, Y., Li, R., Miao, Y., et al. (2022). The small GTPase RABA2a recruits SNARE proteins to regulate the secretory pathway in parallel with the exocyst complex in Arabidopsis. Mol. Plant 15, 398–418. doi: 10.1016/j.molp.2021.11.008
Park, M., Song, K., Reichardt, I., Kim, H., Mayer, U., Stierhof, Y. D., et al. (2013). Arabidopsis μ-adaptin subunit AP1M of adaptor protein complex 1 mediates late secretory and vacuolar traffic and is required for growth. Proc. Natl. Acad. Sci. U. S. A. 110, 10318–10323. doi: 10.1073/pnas.1300460110
Peden, A. A., Oorschot, V., Hesser, B. A., Austin, C. D., Scheller, R. H., and Klumperman, J. (2004). Localization of the AP-3 adaptor complex defines a novel endosomal exit site for lysosomal membrane proteins. J. Cell Biol. 164, 1065–1076. doi: 10.1083/jcb.200311064
Pesacreta, T. C., and Lucas, W. J. (1984). Plasma membrane coat and a coated vesicle-associated reticulum of membranes: their structure and possible interrelationship in Chara corallina. J. Cell Biol. 98, 1537–1545. doi: 10.1083/jcb.98.4.1537
Preuss, M. L., Schmitz, A. J., Thole, J. M., Bonner, H. K. S., Otegui, M. S., and Nielsen, E. (2006). A role for the RabA4b effector protein PI-4Kbeta1 in polarized expansion of root hair cells in Arabidopsis thaliana. J. Cell Biol. 172, 991–998. doi: 10.1083/jcb.200508116
Ravikumar, R., Kalbfuß, N., Gendre, D., Steiner, A., Altmann, M., Altmann, S., et al. (2018). Independent yet overlapping pathways ensure the robustness and responsiveness of trans-Golgi network functions in Arabidopsis. Development 145, dev169201. doi: 10.1242/dev.169201
Renna, L., Stefano, G., Slabaugh, E., Wormsbaecher, C., Sulpizio, A., Zienkiewicz, K., et al. (2018). TGNap1 is required for microtubule-dependent homeostasis of a subpopulation of the plant trans-Golgi network. Nat. Commun. 9, 5313. doi: 10.1038/s41467-018-07662-4
Richter, S., Kientz, M., Brumm, S., Nielsen, M. E., Park, M., Gavidia, R., et al. (2014). Delivery of endocytosed proteins to the cell–division plane requires change of pathway from recycling to secretion. elife 3:e02131. doi: 10.7554/eLife.02131
Rodriguez-Furlan, C., Campos, R., Toth, J. N., and Van Norman, J. M. (2022). Distinct mechanisms orchestrate the contra-polarity of IRK and KOIN, two LRR-receptor-kinases controlling root cell division. Nat. Commun. 13:235. doi: 10.1038/s41467-021-27913-1
Rohn, W. M., Rouillé, Y., Waguri, S., and Hoflack, B. (2000). Bi-directional trafficking between the trans-Golgi network and the endosomal/lysosomal system. J. Cell Sci. 113, 2093–2101. doi: 10.1242/jcs.113.12.2093
Saito, C., and Ueda, T. (2009). Chapter 4: functions of RAB and SNARE proteins in plant life. Int. Rev. Cell Mol. Biol. 274, 183–233. doi: 10.1016/S1937-6448(08)02004-2
Sašek, V., Janda, M., Delage, E., Puyaubert, J., Guivarc, H. A., López Maseda, E., et al. (2014). Constitutive salicylic acid accumulation in pi4kIIIβ1β2 Arabidopsis plants stunts rosette but not root growth. New Phytol. 203, 805–816. doi: 10.1111/nph.12822
Sauer, M., Delgadillo, M. O., Zouhar, J., Reynolds, G. D., Pennington, J. G., Jiang, L., et al. (2013). MTV1 and MTV4 encode plant-specific ENTH and ARF GAP proteins that mediate clathrin-dependent trafficking of vacuolar cargo from the trans-Golgi network. Plant Cell 25, 2217–2235. doi: 10.1105/tpc.113.111724
Shimada, T., Kunieda, T., Sumi, S., Koumoto, Y., Tamura, K., Hatano, K., et al. (2018). The AP-1 complex is required for proper mucilage formation in Arabidopsis Seeds. Plant Cell Physiol. 59, 2331–2338. doi: 10.1093/pcp/pcy158
Shimizu, Y., Takagi, J., Ito, E., Ito, Y., Ebine, K., Komatsu, Y., et al. (2021). Cargo sorting zones in the trans-Golgi network visualized by super-resolution confocal live imaging microscopy in plants. Nat. Commun. 12, 1901. doi: 10.1038/s41467-021-22267-0
Singh, M. K., and Jürgens, G. (2018). Specificity of plant membrane trafficking - ARFs, regulators and coat proteins. Semin. Cell Dev. Biol. 80, 85–93. doi: 10.1016/j.semcdb.2017.10.005
Singh, M. K., Richter, S., Beckmann, H., Kientz, M., Stierhof, Y. D., Anders, N., et al. (2018). A single class of ARF GTPase activated by several pathway-specific ARF-GEFs regulates essential membrane traffic in Arabidopsis. PLoS Genet. 14:e1007795. doi: 10.1371/journal.pgen.1007795
Staehelin, L. A., Giddings, T. H. Jr., Kiss, J. Z., and Sack, F. D. (1990). Macromolecular differentiation of Golgi stacks in root tips of Arabidopsis and Nicotiana seedlings as visualized in high pressure frozen and freeze-substituted samples. Protoplasma 157, 75–91. doi: 10.1007/BF01322640
Staehelin, L. A., and Kang, B. H. (2008). Nanoscale architecture of endoplasmic reticulum export sites and of Golgi membranes as determined by electron tomography. Plant Physiol. 147, 1454–1468. doi: 10.1104/pp.108.120618
Teh, O. K., Shimono, Y., Shirakawa, M., Fukao, Y., Tamura, K., Shimada, T., et al. (2013). The AP-1 μ adaptin is required for KNOLLE localization at the cell plate to mediate cytokinesis in Arabidopsis. Plant Cell Physiol. 54, 838–847. doi: 10.1093/pcp/pct048
Uemura, T., Nakano, R. T., Takagi, J., Wang, Y., Kramer, K., Finkemeier, I., et al. (2019). A Golgi-released subpopulation of the trans-Golgi network mediates protein secretion in Arabidopsis. Plant Physiol. 179, 519–532. doi: 10.1104/pp.18.01228
Uemura, T., Suda, Y., Ueda, T., and Nakano, A. (2014). Dynamic behavior of the trans-Golgi network in root tissues of Arabidopsis revealed by super-resolution live imaging. Plant Cell Physiol. 55, 694–703. doi: 10.1093/pcp/pcu010
Uemura, T., and Ueda, T. (2014). Plant vacuolar trafficking driven by RAB and SNARE proteins. Curr. Opin. Plant Biol. 22, 116–121. doi: 10.1016/j.pbi.2014.10.002
Uemura, T., Ueda, T., and Nakano, A. (2012). The physiological role of SYP4 in the salinity and osmotic stress tolerances. Plant Signal. Behav. 7, 1118–1120. doi: 10.4161/psb.21307
Viotti, C., Bubeck, J., Stierhof, Y. D., Krebs, M., Langhans, M., van den Berg, W., et al. (2010). Endocytic and secretory traffic in Arabidopsis merge in the trans-Golgi network/early endosome, an independent and highly dynamic organelle. Plant Cell 22, 1344–1357. doi: 10.1105/tpc.109.072637
Waghmare, S., Lileikyte, E., Karnik, R., Goodman, J. K., Blatt, M. R., and Jones, A. M. E. (2018). SNAREs SYP121 and SYP122 mediate the secretion of distinct cargo subsets. Plant Physiol. 178, 1679–1688. doi: 10.1104/pp.18.00832
Wang, X., Cai, Y., Wang, H., Zeng, Y., Zhuang, X., Li, B., et al. (2014). trans-Golgi network-located AP1 gamma adaptins mediate dileucine motif-directed vacuolar targeting in Arabidopsis. Plant Cell 26, 4102–4118. doi: 10.1105/tpc.114.129759
Wang, P., and Kang, B. H. (2020). Correlative light and electron microscopy imaging of the plant trans-Golgi network. Methods Mol. Biol. 2177, 59–67. doi: 10.1007/978-1-0716-0767-1_6
Wang, J. G., Li, S., Zhao, X. Y., Zhou, L. Z., Huang, G. Q., Feng, C., et al. (2013). HAPLESS13, the Arabidopsis μ1 adaptin, is essential for protein sorting at the trans-Golgi network/early endosome. Plant Physiol. 162, 1897–1910. doi: 10.1104/pp.113.221051
Wang, Y. J., Wang, J., Sun, H. Q., Martinez, M., Sun, Y. X., Macia, E., et al. (2003). Phosphatidylinositol 4 phosphate regulates targeting of clathrin adaptor AP-1 complexes to the Golgi. Cell 114, 299–310. doi: 10.1016/S0092-8674(03)00603-2
Wattelet-Boyer, V., Brocard, L., Jonsson, K., Esnay, N., Joubès, J., Domergue, F., et al. (2016). Enrichment of hydroxylated C24- and C26-acyl-chain sphingolipids mediates PIN2 apical sorting at trans-Golgi network subdomains. Nat. Commun. 7:12788. doi: 10.1038/ncomms12788
Yamaoka, S., Shimono, Y., Shirakawa, M., Fukao, Y., Kawase, T., Hatsugai, N., et al. (2013). Identification and dynamics of Arabidopsis adaptor protein-2 complex and its involvement in floral organ development. Plant Cell 25, 2958–2969. doi: 10.1105/tpc.113.114082
Yan, X., Wang, Y., Xu, M., Dahhan, D. A., Liu, C., Zhang, Y., et al. (2021). Cross-talk between clathrin-dependent post-Golgi trafficking and clathrin-mediated endocytosis in Arabidopsis root cells. Plant Cell 33, 3057–3075. doi: 10.1093/plcell/koab180
Zhang, C., Brown, M. Q., van de Ven, W., Zhang, Z. M., Wu, B., Young, M. C., et al. (2016). Endosidin2 targets conserved exocyst complex subunit EXO70 to inhibit exocytosis. Proc. Natl. Acad. Sci. U. S. A. 113, E41–E50. doi: 10.1073/pnas.1521248112
Zhang, Z., Feechan, A., Pedersen, C., Newman, M. A., Qiu, J. L., Olesen, K. L., et al. (2007). A SNARE-protein has opposing functions in penetration resistance and defence signalling pathways. Plant J. 49, 302–312. doi: 10.1111/j.1365-313X.2006.02961.x
Zhang, L., Zhang, H., Liu, P., Hao, H., Jin, J. B., and Lin, J. (2011). Arabidopsis R-SNARE proteins VAMP721 and VAMP722 are required for cell plate formation. PLoS One 6:e26129. doi: 10.1371/journal.pone.0026129
Keywords: trans-Golgi network, cargo proteins, spatiotemporal, membrane trafficking, RAB GTPase
Citation: Shimizu Y and Uemura T (2022) The sorting of cargo proteins in the plant trans-Golgi network. Front. Plant Sci. 13:957995. doi: 10.3389/fpls.2022.957995
Received: 31 May 2022; Accepted: 20 July 2022;
Published: 11 August 2022.
Edited by:
Jean-Marc Neuhaus, Universitéde Neuchât el, SwitzerlandReviewed by:
Ben Zhang, Shanxi University, ChinaCopyright © 2022 Shimizu and Uemura. This is an open-access article distributed under the terms of the Creative Commons Attribution License (CC BY). The use, distribution or reproduction in other forums is permitted, provided the original author(s) and the copyright owner(s) are credited and that the original publication in this journal is cited, in accordance with accepted academic practice. No use, distribution or reproduction is permitted which does not comply with these terms.
*Correspondence: Tomohiro Uemura, dWVtdXJhLnRvbW9oaXJvQG9jaGEuYWMuanA=
Disclaimer: All claims expressed in this article are solely those of the authors and do not necessarily represent those of their affiliated organizations, or those of the publisher, the editors and the reviewers. Any product that may be evaluated in this article or claim that may be made by its manufacturer is not guaranteed or endorsed by the publisher.
Research integrity at Frontiers
Learn more about the work of our research integrity team to safeguard the quality of each article we publish.