- 1State Key Laboratory for Managing Biotic and Chemical Threats to the Quality and Safety of Agro-products, College of Life Sciences, Zhejiang University, Hangzhou, China
- 2State Key Laboratory for Managing Biotic and Chemical Threats to the Quality and Safety of Agro-products, Institute of Plant Protection and Microbiology, Zhejiang Academy of Agricultural Sciences, Hangzhou, China
- 3Institute of Biotechnology, Zhejiang University, Hangzhou, China
The rice blast fungus Magnaporthe oryzae spores differentiate and mature into functional appressoria by sensing the host surface signals. Environmental stimuli are transduced into cells through internalization during appressorium formation, such as in the cAMP-PKA pathway. Here, we describe a novel contribution to how appressoria mature on the surface of a leaf, and its connection to endosomes and the cAMP-PKA pathway. An appressorium membrane-specific protein, Pams1, is required for maintaining endosomal structure, appressorium maturation, and virulence in M. oryzae. During appressorium development, Pams1 was translocated from the cell membrane to the endosomal membrane. Deletion of PAMS1 led to the formation of two types of abnormal appressoria after 8 h post inoculation (hpi): melanized type I had a reduced virulence, while pale type II was dead. Before 8 hpi, Δpams1 formed appressoria that were similar to those of the wild type. After 8 hpi, the appressoria of Δpams1 was differentiated into two types: (1) the cell walls of type I appressoria were melanized, endosomes were larger, and had a different distribution from the wild type and (2) Type II appressoria gradually stopped melanization and began to die. The organelles, including the nucleus, endosomes, mitochondria, and endoplasmic reticula, were degraded, leaving only autophagic body-like vesicles in type II appressoria. The addition of exogenous cAMP to Δpams1 led to the formation of a greater proportion of type I appressoria and a smaller proportion of type II appressoria. Thus, defects in endosomal structure and the cAMP-PKA pathway are among the causes of the defective appressorium maturation and virulence of Δpams1.
Introduction
Plant pathogenic fungi continue to threaten global food security (Fisher et al., 2012). Magnaporthe oryzae (synonym Pyricularia oryzae), the rice blast disease pathogen, causes serious damage to the production of cereal crops, such as rice and wheat (Talbot, 2003; Inoue et al., 2017). M. oryzae infects plants via an appressorium, a specialized infection structure (Howard and Valent, 1996). Spores of M. oryzae fall on the surface of the plant, germinate, produce germ tubes, and differentiate into appressoria (Jiang et al., 2018). Before differentiating into appressoria, conidial germ tubes sense surface hardness (Liu H. et al., 2007), hydrophobic signals (Lee and Dean, 1994), and chemical molecules, for example, cutin monomers (Lee and Dean, 1993; Skamnioti and Gurr, 2007). A mature and functional appressorium is necessary for the infection of a plant by M. oryzae (Chumley and Valent, 1990; deJong et al., 1997; Dagdas et al., 2012). The developmental process of an appressorium can be divided into three stages: (1) In the initiation or differentiation stage, germ tube hooking and swelling occurs, followed by bulbous apex formation (Wilson and Talbot, 2009); (2) The maturation stage consists of melanization of cell walls, the influx of three-celled spore inclusion into a unicellular appressorium, generation of high turgor pressure, and septin ring formation inside the appressorium (Wilson and Talbot, 2009; Jiang et al., 2018); (3) Finally, the penetration stage takes place with the infection peg emerging from an appressorium (Dagdas et al., 2012). During appressorium formation, spore cells die through autophagy and ferroptosis, and intracellular storage is degraded and transported into the appressorium to build new cellular structures and synthesize solutes such as glycerol (Veneault-Fourrey et al., 2006; Liu X. H. et al., 2007; Shen et al., 2020). Blocking autophagy inhibits spore cell death and degradation and reduces appressorial turgor pressure and virulence in M. oryzae (Liu X. H. et al., 2007).
The cAMP-PKA signaling pathway controls the recognition of surface signals during the initiation of an appressorium (Wilson and Talbot, 2009; Li Y. et al., 2017; Jiang et al., 2018). Deletion of CPKA, a catalytic subunit of Protein Kinase A (PKA), resulted in smaller appressoria and reduced virulence in M. oryzae (Xu and Hamer, 1996; Xu et al., 1997), and ΔcpkAΔcpk2, in which two catalytic subunits of PKA were deleted, completely failed to produce appressoria on hydrophobic surfaces (Selvaraj et al., 2017). Pth11 (a G-protein-coupled receptor), Gα (the α-subunit of heterotrimeric G proteins), Mac1 (an adenylate cyclase), and Rgs1 (the regulator of G protein signaling 1) finely regulate cAMP signaling through endosomes (Zhang et al., 2011; Ramanujam et al., 2013; Kou and Naqvi, 2016; Kou et al., 2017). The endocytosis of the plasma membrane is triggered by the activation of cell surface receptors, and the activated receptors along with their bound extracellular material enter the cell interior via endosomes (Scott et al., 2014). Early endosomes are highly dynamic organelles that fuse with themselves or other types of vesicles. The membrane and its activated cell surface receptors return to the plasma membrane via recycling endosomes, while some late endosomes and multivesicular bodies (MVBs) fuse with lysosomes or vacuoles to degrade their contents (Scott et al., 2014). In M. oryzae, the receptor protein Pth11 enters endosomes from the plasma membrane through endocytosis, and several cAMP-PKA signaling pathway proteins such as Mac1, Rgs1, and Gα are located on endosomes (Zhang et al., 2011; Ramanujam et al., 2013; Kou and Naqvi, 2016). Impaired endocytosis reduces appressorium formation and virulence in M. oryzae (Zhang et al., 2011; Ramanujam et al., 2013).
The Pmk1 kinase signaling pathway controls appressorium formation and penetration (Jiang et al., 2018; Ryder et al., 2019), and the Mps1 kinase signaling pathway regulates appressorium penetration (Xu et al., 1998; Jiang et al., 2018). Glucose-ABL1-TOR signaling and cell cycle arrest finely regulate appressorium morphogenesis (Marroquin-Guzman and Wilson, 2015; Marroquin-Guzman et al., 2017). Pmk1-dependent Mst12 and Hox7 transcriptional network control appressorium formation and plant penetration (Oses-Ruiz et al., 2021). Deletion of a transcription factor HOX7 disabled formation of mature appressoria in M. oryzae (Kim et al., 2009; Oses-Ruiz et al., 2021). In a large-scale functional study of 47 Cys2-His2 transcription factors of M. oryzae, we found that Vrf1 is another key transcription factor controlling appressorium maturation and deletion of VRF1 prevents the incipient appressorium from maturing (Cao et al., 2016). Δvrf1 formed an appressorium with similar morphology as the wild type within 6 h post inoculation (hpi). After that point in time, however, the mutant’s appressorium did not develop into a functional hemispherical appressorium, but gradually became a hypha-like structure (Cao et al., 2016). Our recent study showed that Δvrf1 and Δhox7 have a similar mutant phenotype during appressorium formation and that the double knockout mutant of VRF1 and HOX7 (Δvrf1Δhox7) also has a similar mutant phenotype to Δvrf1 and Δhox7 during appressorium formation (Huang et al., 2022). Δvrf1, Δhox7, and Δvrf1Δhox7 failed to form mature appressoria on hydrophobic surfaces but formed hyphal-like structures. Since the virulence of M. oryzae requires mature functional appressoria, the study of the appressorium maturation mechanism can lead to an in-depth understanding of the interaction mechanism between the fungus and rice.
In this study, we identified a novel appressorium membrane-specific protein, Pams1, that is necessary for appressorium morphogenesis and fungal virulence on rice via endosomes and the cAMP-PKA signaling pathway during appressorium maturation in M. oryzae.
Materials and methods
Fungal strains, growth conditions, and DNA/RNA manipulation
Magnaporthe oryzae strain 70–15 and its derivatives (Supplementary Table 1) were cultured on a complete medium (CM) at 25°C under a 16-h light/8-h dark cycle (Talbot et al., 1993). DNA and RNA manipulations were conducted following standard procedures as previously reported (Sambrook and Russell, 2001; Lu et al., 2014). Total RNAs were extracted from fungal samples using the RNeasy Plant Mini Kit (Qiagen, United States). Real-Time Quantitative Reverse Transcription PCR (qRT-PCR) was performed to obtain gene expression levels from three to five biological replicates using 40S and α-ACTIN as reference genes. Expression levels were calculated using the 2–ΔCT method after normalization to the two reference genes. The PCR primers used in this study are listed in Supplementary Table 2.
Deletion and complementation of targeted genes in Magnaporthe oryzae
A PAMS1 deletion mutant was generated using a method described previously (Lu et al., 2014; Yan et al., 2019). The gene-deletion cassette was built in pKO3B using a hygromycin B phosphotransferase gene (HPH) as a selection gene and transformed into M. oryzae strains via Agrobacterium tumefaciens-mediated transformation (ATMT) according to a reported method (Lu et al., 2014; Yan et al., 2019). Transformants were grown on selection media containing 0.5 μM 5-fluoro-2′-deoxyuridine (F2dU) and 200 μg ml–1 hygromycin B. The null mutants were confirmed by PCR to confirm a deleted gene using β-TUBULIN DNA as a positive control and another PCR to detect a targeted recombinational DNA sequence of the resistance gene (Supplementary Figure 1). The copies of transformed gene-deletion cassettes in mutants were identified by quantitative PCR (qPCR) (Lu et al., 2014), and in all the mutants verified in Supplementary Figure 1, the resistance gene was inserted in a single copy (Supplementary Table 3). The mutants were purified by monoconidiation for further experiments. Δpams1 was complemented with the native copy of PAMS1 that was cloned into the EcoRI-XbaI site of pKD5 containing a sulfonylurea resistance gene (SUR) using a fusion enzyme (Vazyme, China) (Li et al., 2012). The gene-rescued transformants were identified on selection plates supplemented with 100 μg ml–1 chlorimuron-ethyl and confirmed at the mRNA level by reverse transcription PCR (RT-PCR) (Supplementary Figure 1).
Phenotypic analysis
Mycelial growth and conidiation of strains were assayed on CM at 25°C for 8 days. For conidial germination (at 4 hpi) and appressorium formation assays (at 4–24 hpi), 25 μl droplets of spore suspensions (1 × 105 conidia ml–1) were placed on plastic coverslips (Thermo Scientific, United States) or hydrophobic polyvinyl chloride (PVC) films and incubated at 22°C in the dark. Appressorium turgor pressure was evaluated through incipient cytorrhysis (cell collapse) assays as described previously (Howard et al., 1991). At 24–48 hpi, appressoria were incubated in a series of glycerol solutions (0.5–2.0 M) for 5 min and then the collapsed appressoria were counted under a microscope. Ten mM 8-Bromoadenosine 3′,5′-cyclic monophosphate sodium salt (8-Bromo-cAMP, Santa Cruz Biotechnology, United States) were used in the appressorium formation assay. The experiments were performed three times with three or five replicates each time. For conidial germination and appressorium formation assays, 110–200 spores or appressoria were counted per replicate.
Plant infection assays
Barley (Hordeum vulgare cv. ZJ-8) or rice (Oryza sativa cv. CO-39) seeds were sterilized with sodium hypochlorite solution for 3–4 min, rinsed repeatedly with water, and then planted in soil at 25°C for 8 days (barley) or 14 days (3–4 leaf stage for rice). For virulence assays with leaf explants of barley, 5-mm mycelium blocks were inoculated on leaves and cultured at 25°C for 4 days. For the spraying assay on rice, 2-week-old rice seedlings (n ≥ 17) were inoculated by spraying with 2.5 ml conidial suspensions (5 × 104 conidia ml–1) containing 0.2% (w/v) gelatin. The disease severity was assessed within a 5-cm section of the infected leaf exhibiting the most serious disease lesions in each seedling at 7 dpi (days post inoculation) until full disease symptoms on the wild type became apparent (Cao et al., 2016). For observations of the invasive process, spore suspensions (1 × 105 conidia ml–1) were inoculated on barley leaves and rice leaf sheaths. Two sets of each plant species were incubated at 25°C, one set for each of two incubation times, 24 and 48 h. Host cells infected by appressoria were observed under a microscope after each incubation time (Cao et al., 2018). The experiments were repeated three times.
Observation of cytoplasm and glycogen
To visualize surviving cytoplasm, cells were stained with 2 μg ml–1 fluorescein diacetate (FDA) (Sigma-Aldrich, United States) for 5–10 min or glycogen inside cytoplasm was stained with solutions containing 60 mg ml–1 KI and 10 mg ml–1 I2 for 2 min (Thines et al., 2000). To label endolysosomes and vacuoles, 7-amino-4-chloromethylcoumarin (CMAC, Invitrogen, United States) solution (10 μM final concentration) was added to spore suspensions, and the spores were inoculated on hydrophobic borosilicate glass coverslips and incubated for 24 hpi. Samples were observed under a fluorescence microscope (Nikon Eclipse Ni, Japan) or a Leica icc50 W microscope (Leica, Germany). The experiments were performed three times with three replicates each time, and 110–200 spores or appressoria were counted per replicate.
Electron microscope observation
Samples were prepared following a standard procedure (Lu et al., 2009). Spore suspensions were inoculated on barley leaves and cultivated for 18 h at 25°C. And the appressoria were covered with a thin layer of 3.5% agar. The appressoria along with leaves were fixed with 2.5% glutaraldehyde in 0.1 M phosphate buffer (pH 7.3) overnight at 4°C, fixed with 1% osmium acid in 0.1 M phosphate buffer (pH 7.3) for 1 h, dehydrated with ethanol and acetone, and embedded in Spurr resin. The ultrathin sections were stained with lead citrate and uranyl acetate solution for 5–10 min and observed with a transmission electron microscope (H-7650, Hitachi, Japan). Five to 10 slices per sample were observed. The experiments were performed two times, and three independent biological samples were prepared each time.
Observation of fluorescence fusion proteins
To build the GFP-PAMS1 infusion gene, the native PAMS1 (containing its own promoter and terminator) along with GFP (that was inserted into the site between amino acids 50 and 51 of Pams1) were fused into the EcoRI-XbaI site of pKD5-GFP containing SUR (Li et al., 2012). The GFP-PAMS1 expression vector was transformed into Δpams1 via ATMT. pKD3-mCherry is a vector containing mCherry and BAR (a bialaphos resistance gene) instead of the GFP and SUR found in pKD5-GFP, and pKD10-mCherry is a vector with the H3 promoter of pKD3-mCherry replaced with the β-TUBULIN promoter. To visualize endosomes, nuclei, ER, mitochondria, and lipid droplets, one of each of the five vectors, pKD3-mCherry-Rab5A (RAB5A was fused into the C-terminus of mCherry), pKD10-H2B-mCherry (H2B fused into the N-terminus of mCherry of pKD10-mCherry), pKD8-Spf1-GFP (Qu et al., 2020), pKD8-Atp1-GFP (Shi et al., 2018), and pKD8-Cap20-GFP (Cao et al., 2018) were transformed into one of each of the wild type, Δpams1, and Δpams1GFP–PAMS1 (Δpams1 expressing GFP-PAMS1) cultures. Appressoria were induced on hydrophobic borosilicate glass coverslips (Thermo Scientific, United States) and observed under a fluorescence microscope and a laser scanning confocal microscope (FV3000, Olympus, Japan). The observation of each sample was repeated at least three times, more than 100 spores or appressoria were observed each time, and dozens of pictures were taken.
Quantification of glycerol contents
Equal volumes of spore suspensions (5 × 105 conidia ml–1) of Δpams1 and the wild type were separately inoculated onto PVC films at 25°C in the dark. At 24 hpi, the glycerol contents in appressoria and culture solutions were each measured using a glycerol assay kit (MAK117, Sigma-Aldrich, United States). Glycerol contents are shown in nmol per 106 appressoria. The experiments were performed three times with three replicates each time.
Bioinformatics analysis
Transmembrane helices in Pams1 were predicted by the TMHMM Server v. 2.01 and NCBI conserved domain data2. Image analysis software (FV31S-SW, FV31S-DT, or Image J) was used to show fluorescence photos or fluorescence intensity distribution plots.
Statistical analysis
Each value shown for each group is the mean ± SD (standard deviation). All experiments were repeated independently at least three times. The P-value was calculated using an unpaired two-tailed Student’s t-test in GraphPad Prism 8. P-values < 0.05 were considered significant, while P-values > 0.05 were considered non-significant.
Results
Identification of an appressorium-specific membrane protein, Pams1, in Magnaporthe oryzae
In our previous study, appressoria formed by a VRF1-deleted mutant cannot develop into mature and functional appressoria (Cao et al., 2016). A total of 913 genes were downregulated by more than four times (FDR < 0.01) in the appressoria of Δvrf1 than in that of the wild type at 5 hpi (Cao et al., 2016). The downregulation of some key genes in Δvrf1 is one of the reasons for the mutant phenotype. To identify genes that may be involved in appressorium maturation, we knocked out 78 of these downregulated genes and found a mutant 11D9 with defects in appressorium maturation, in which MGG_03640 (XP_003716249.1) was deleted (Supplementary Figure 1; Supplementary Table 3). Since the study described below suggested MGG_03640 as a membrane protein functioning specifically at the appressorium stage, here, MGG_03640 was named Pams1 (Pyricularia appressorium membrane-specific protein 1). PAMS1 was downregulated 423.2-, 385.5-, and 457.7-fold in the 5-hpi appressoria of Δvrf1, Δhox7, and Δhox7Δvrf1, respectively (Figure 1A). Quantitative RT-PCR analysis showed that PAMS1 expression was high in appressoria of 4–10 hpi and very low in hyphae and spores, and this temporal distribution pattern was similar to the mRNA expression pattern of VRF1 and HOX7 (Cao et al., 2016; Huang et al., 2022), suggesting that the expression of PAMS1 is developmental stage-dependent (Figure 1B). TMHMM server predicted that Pams1 encodes a membrane protein with 14 transmembrane regions that constitute an major facilitator superfamily (MFS) domain (Figure 1C). Homologs of Pams1, mainly MFS domain, are present in other fungi (Figure 2). However, the homologous peptides in a 195-amino acid sequence of the N-terminus (1–195 aa, Pams1-N) or a 37-amino acid peptide of the C-terminus (691–727 aa, Pams1-C) of Pams1 are only present in Magnaporthe species (Figures 3A,B).
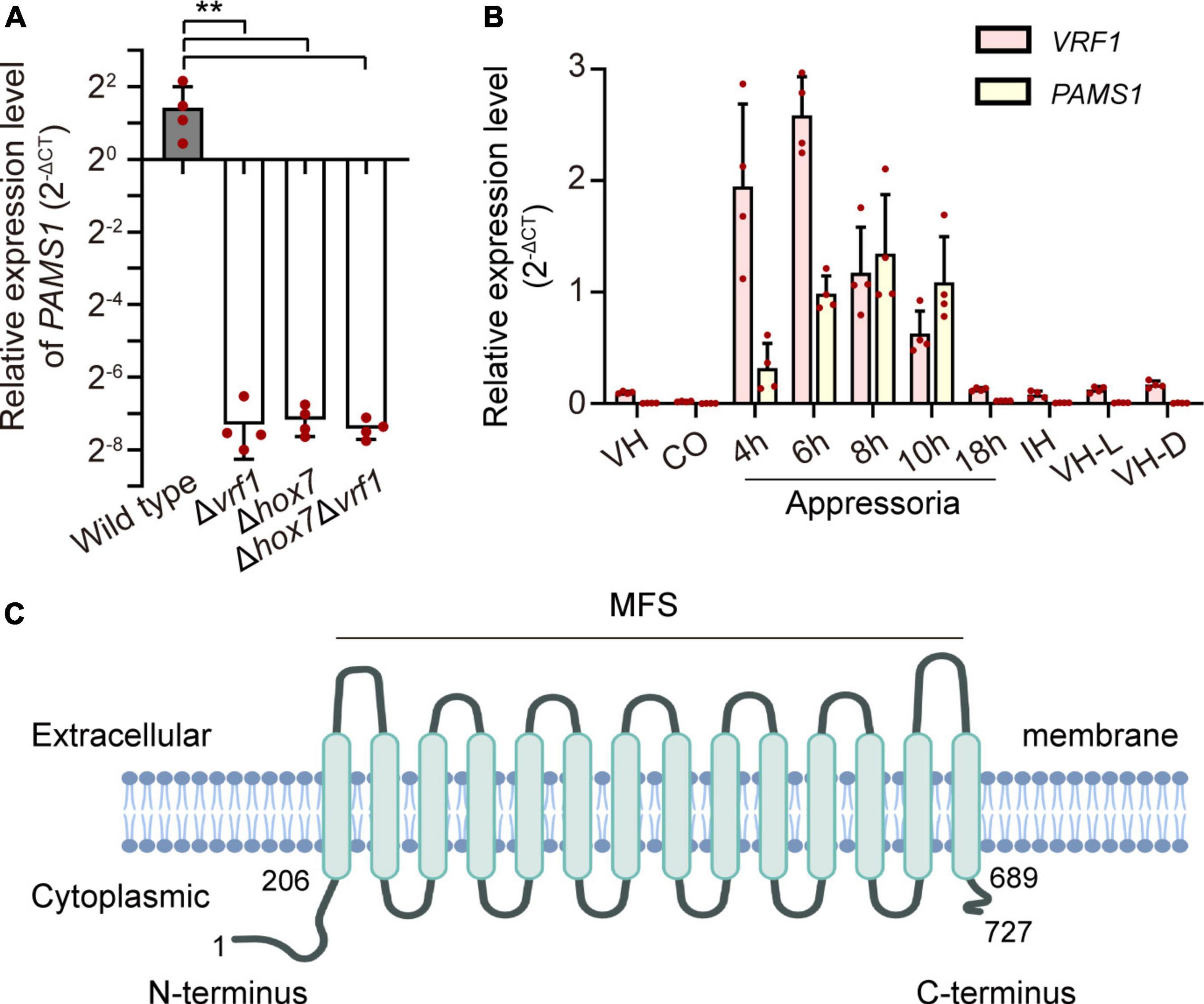
Figure 1. Identification of an appressorium membrane protein Pams1 in M. oryzae. (A) Relative expression levels of PAMS1 in 5-hpi appressoria of the wild type and Δvrf1, Δhox7, and Δhox71Δvrf1 (n = 4). **P < 0.01. (B) Relative transcript levels of PAMS1 and VRF1 in the wild type (n = 4). VH, vegetative hyphae in CM. CO, conidia. 4–18 h, appressoria sampled at 4–18 hpi. IH, invasive hyphae in barley at 2 dpi. VH-L or VH-D, aerial hyphae cultured under light or darkness, respectively. 40S and α-ACTIN are used as reference genes in panels (A,B). (C) Transmembrane helices in Pams1 were predicted by the TMHMM software and NCBI conserved domain data.
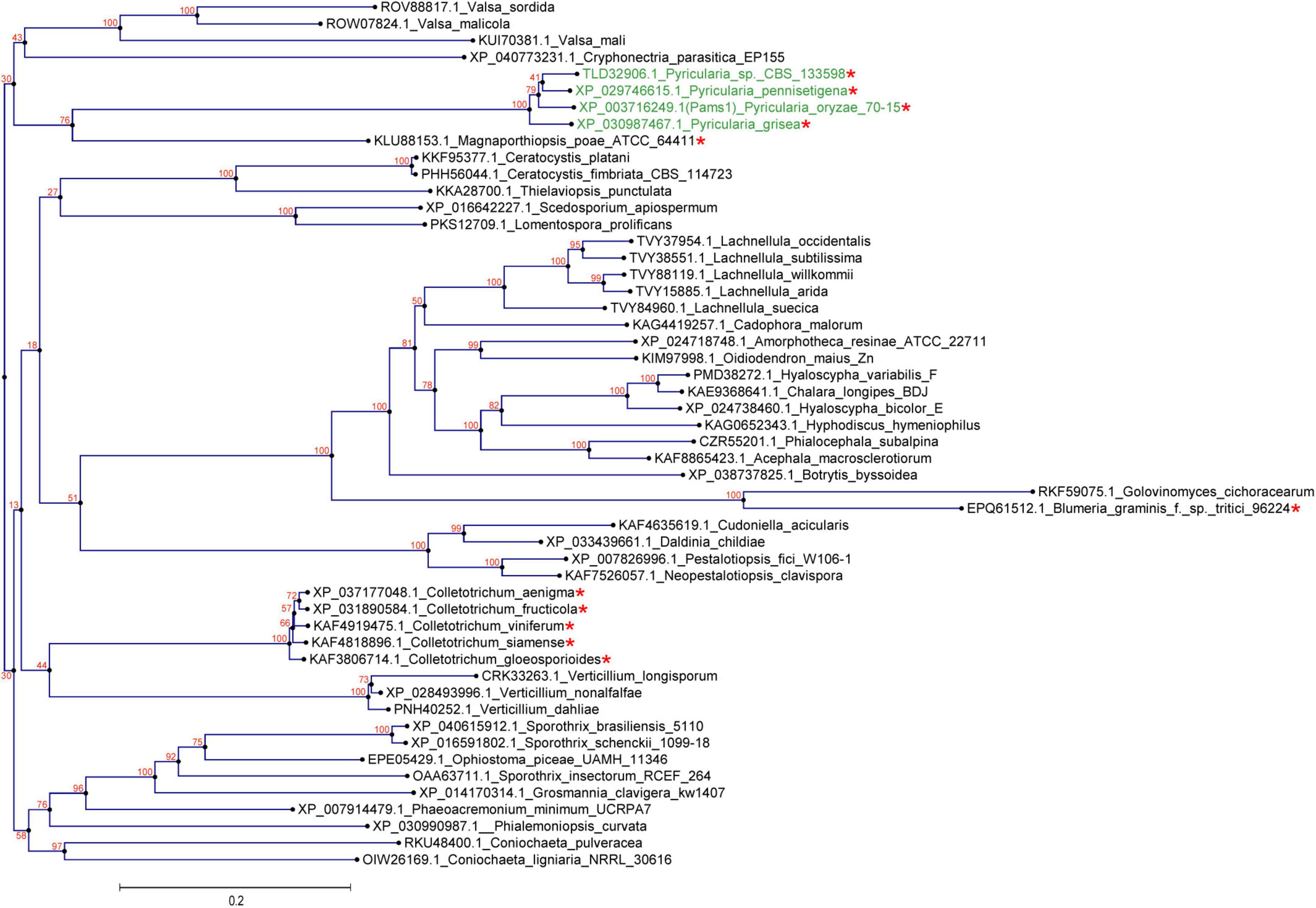
Figure 2. Homologs of Pams1 are present in other fungi. Phylogenetic tree (constructed by the neighbor-joining method) of Pams1 homologs. The proteins used to build this tree were selected from the top 100 homologs of Pams1 with E-values < 9.1E-176 obtained by BLAST search of the NCBI protein database. The top five species with the smallest E-values in a genus were first selected, and then one protein from each species with the smallest E-value was selected. M. oryzae (syn. Pyricularia oryzae) and related three species of Pyricularia (Magnaporthe-like sexual morphs) are indicated in green. Asterisks indicate species that form appressoria or appressoria-like structures. The numbers at branch nodes are the bootstrap values. The scale bar indicates amino acid substitutions per site.
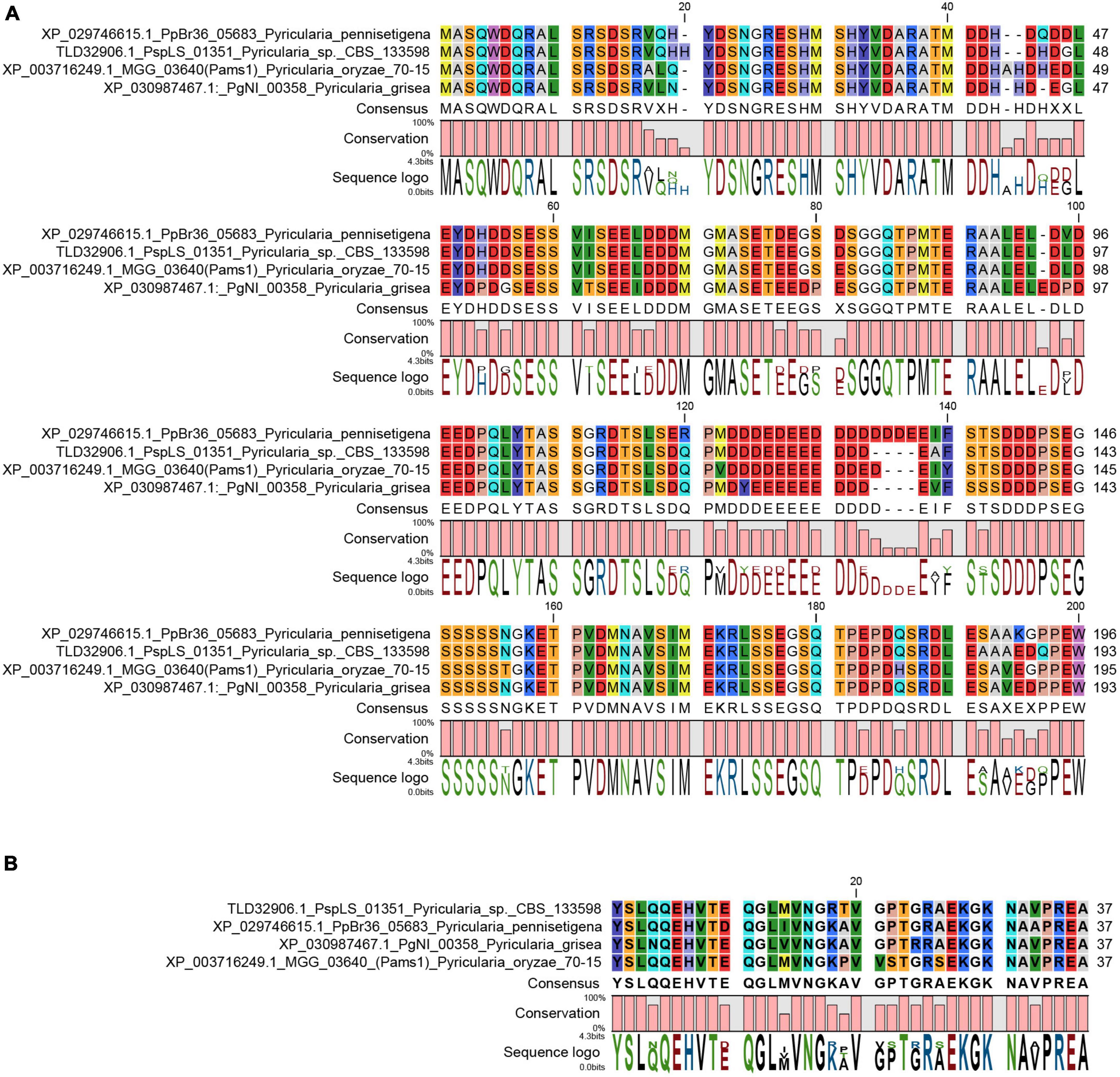
Figure 3. N-terminal and C-terminal peptide sequences of Pams1 were only present in Magnaporthe species. (A,B) Alignment of Pams1-N (A) and Pams1-C (B) homologs (E-value < 1.0) by BLAST search using the respective Pams1-N (1–195 aa) or Pams1-C (691–727 aa) peptide sequences in NCBI.
Pams1 is required for virulence, but not for growth and conidiation
We analyzed the phenotype of Δpams1 in development and virulence. The phenotypic analysis of Δpams1 showed that its colony growth, sporulation, spore germination, and appressorium formation rates were comparable to those of the wild type (Supplementary Figure 2). However, the Δpams1 appressoria formed on plastic coverslips gradually underwent structural deformation after 10 hpi. Finally, two types of abnormal appressoria were formed at 24 hpi (Figure 4). When inoculated on barley leaves with mycelial blocks, the virulence of Δpams1 was greatly reduced (Figure 5A). When sprayed as spore suspensions on rice seedlings, Δpams1 showed significantly reduced virulence as compared to the wild type (Figure 5B). In rice leaf sheath assays, Δpams1 showed reduced host penetration and invasive growth (Figures 5C,D). In Δpams1, melanized type I appressoria displayed a delayed penetration and invasive growth, and pale type II appressoria could not penetrate rice cells (Figure 5C).
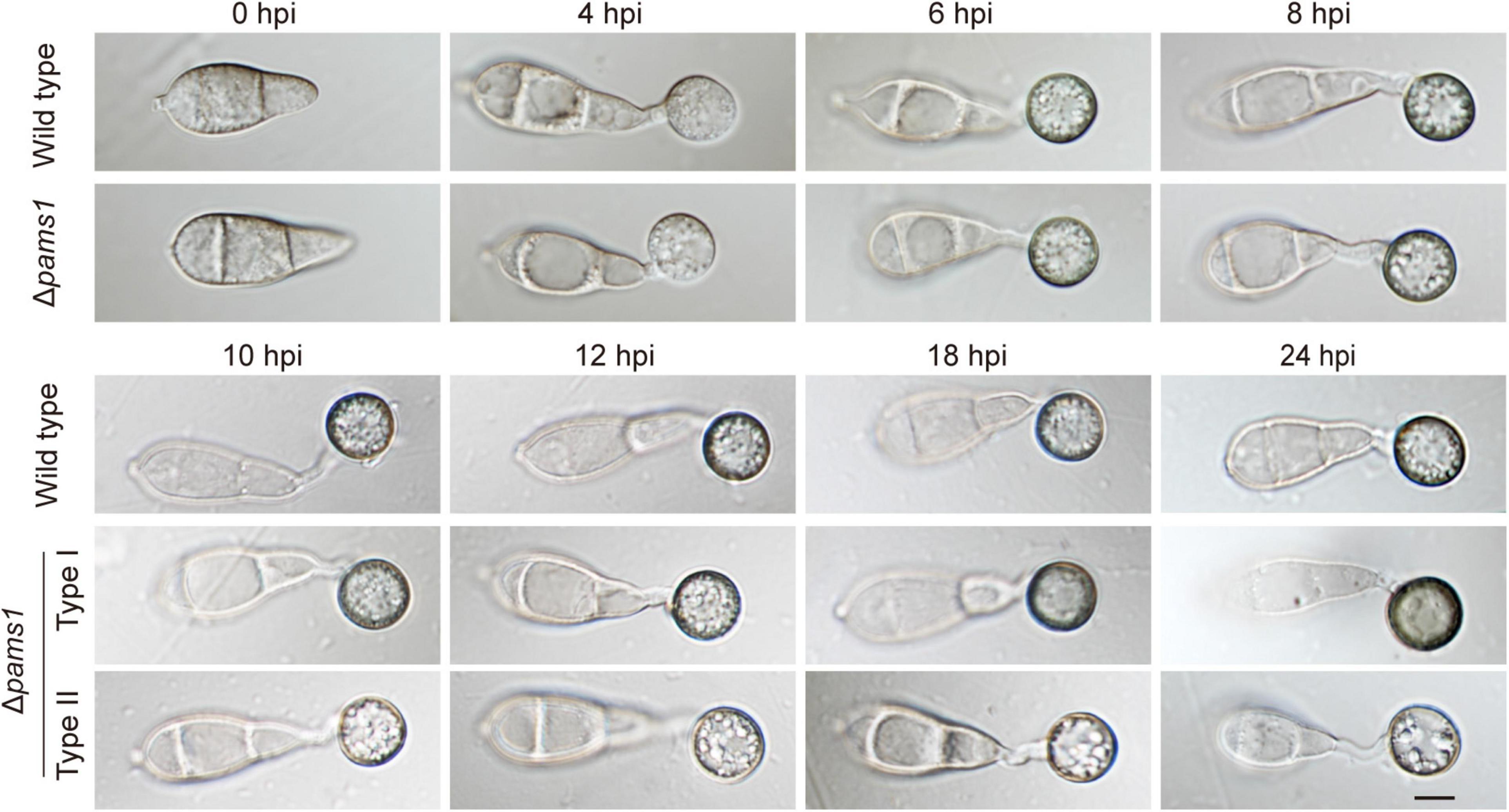
Figure 4. Characteristics of Δpams1 in appressorium formation. Appressoria formed by Δpams1 and the wild type at 0, 4, 6, 8, 10, 12, 18, and 24 hpi. Δpams1 formed two types of abnormal appressoria (types I and II) after 10 hpi. Scale bar, 5 μm.
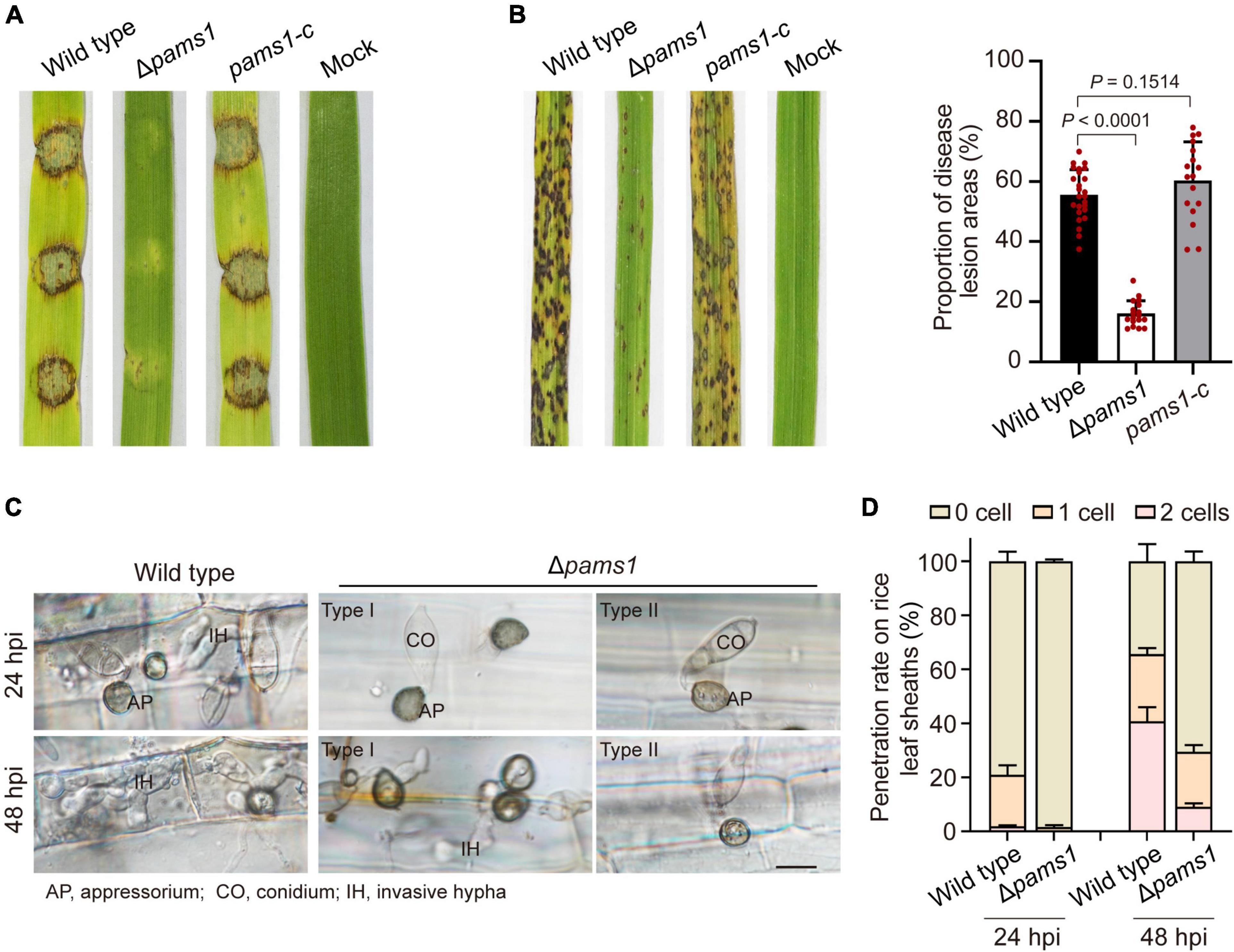
Figure 5. PAMS1 is required for fungal virulence. (A) The 5-mm mycelial blocks were inoculated on leaf explants of barley. Images were taken at 4 dpi. (B) Left, virulence assays on rice by Δpams1, its complementation strain pams1-c and the wild type. A suspension of 5 × 104 conidia per ml was sprayed onto seedlings of the rice cultivar CO-39. Images were taken at 7 dpi. Right, dot plot showing lesion areas calculated from a 5-cm zone of the infected rice leaf exhibiting the most serious disease lesions in each seedling. (C) Host penetration by appressoria and invasive hyphal growth on rice leaf sheaths at 24 and 48 hpi. Type II abnormal appressoria of Δpams1 were unable to invade rice tissue, and the invasion of type I abnormal appressoria into host tissue was delayed until 48 hpi. Scale bar, 10 μm. (D) Percentages of appressoria with invasive hyphae that infected 0 to 3 rice cells (n = 3) at 24 and 48 hpi. At least 150 appressoria were counted per replicate.
Pams1 is required for appressorium maturation
To reveal the structural differences between the two types of appressoria, we carefully assayed the mature appressorium structure of Δpams1 (Figure 6). Δpams1 formed 64.8% melanized type I and 35.2% pale type II abnormal appressoria on plastic coverslips at 24 hpi (Figure 6A). There was no difference in shape between the two types of appressoria, but the cell wall color and internal structure were different (Figures 4, 6B). One (type I) had a black cell wall and fewer but larger-sized intracellular vesicles at 24 hpi relative to the wild type. The other (type II) had a grayish cell wall and fewer intracellular vesicles at 24 hpi when compared with the wild type (Figures 4, 6B). The ultrastructure showed that the density of the melanin layer in the cell wall decreased from the wild type (normal) to type I and type II (abnormal) appressoria at 18 hpi (Figure 6C). Compared with the wild type, the vesicles in type I appressoria were large and branched, and the cytoplasm was deeply stained, while the cytoplasm of type II appressoria was nearly transparent (Figure 6D). The vesicles in type II appressoria were autophagic body-like vesicles (Figures 6D,E).
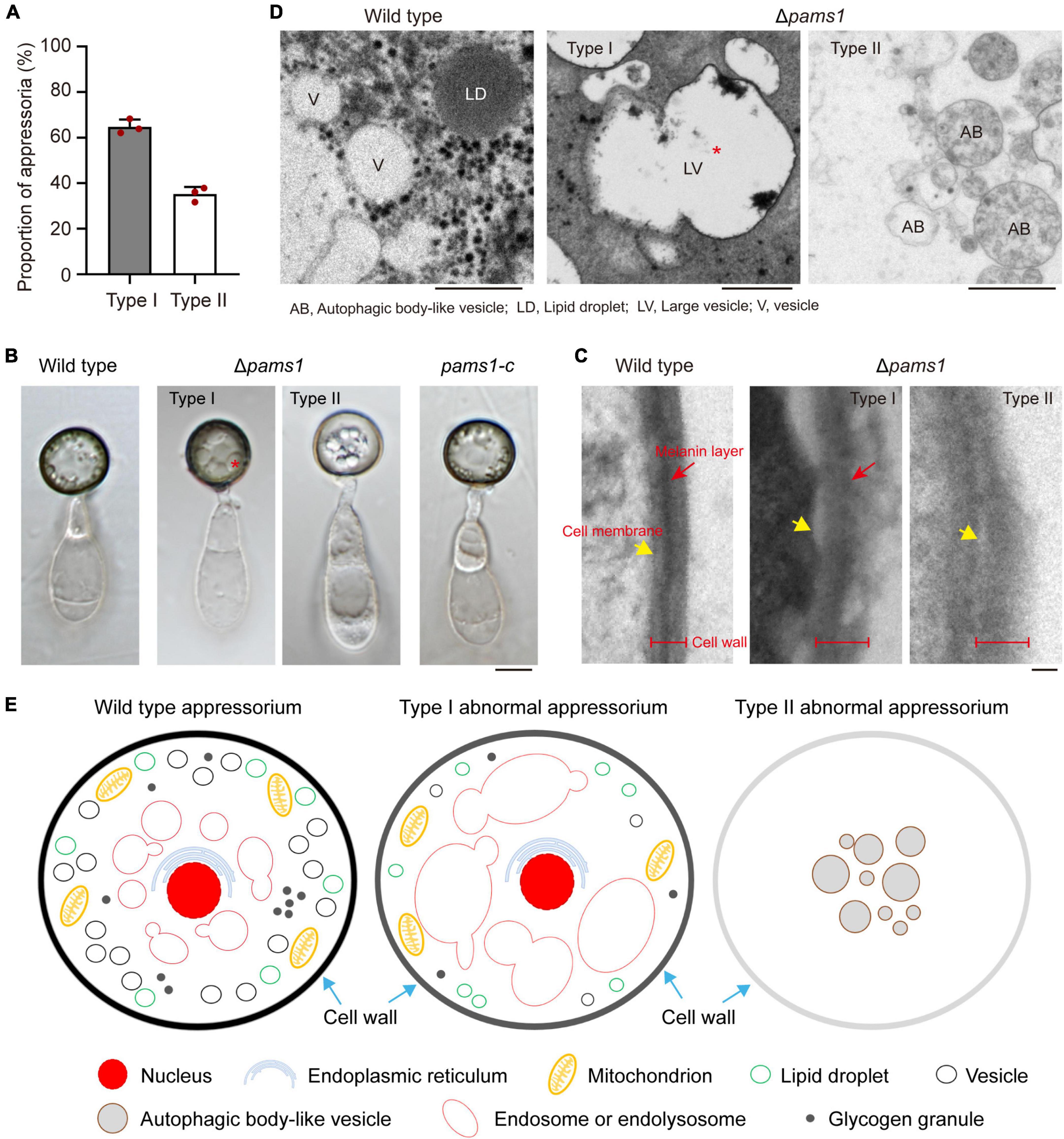
Figure 6. Δpams1 formed two types of abnormal appressoria. (A) Proportions between the two types of abnormal appressoria on plastic coverslips at 24 hpi (n = 3). At least 200 appressoria were counted per replicate. (B) Appressoria of Δpams1, its complementation strain pams1-c, and the wild type at 24 hpi. Δpams1 formed two types of abnormal appressoria (types I and II). Scale bar, 5 μm. (C) Ultrastructure of the appressorial cell wall. Scale bar, 100 nm. (D) Ultrastructure of appressorial vesicles. Scale bars, 1 μm. (E) Schematic diagram of three types of appressoria based on microstructure, ultrastructure, and fluorescently labeled organelles (in Figures 6–8, 10, 11) after 18 hpi. The red stars refer to large vesicles in panels (B,D) are endosomes or endolysosomes.
To understand differences in organelle dynamics during appressorium formation between the wild type and Δpams1, we observed cellular organelles in the germinated spores and developing appressoria on hydrophobic borosilicate glass coverslips by labeling the organelles (Figures 7, 8). At 8 hpi, Δpams1 formed only one type of appressoria that contained endoplasmic reticula, mitochondria, and lipid droplets, which was similar to that in the wild type (Figure 7). At 12 and 24 hpi, the wild type and melanized type I abnormal appressoria had endoplasmic reticula, mitochondria, and lipid droplets in their appressorial cells, while pale type II abnormal appressoria lost normally structured endoplasmic reticula, mitochondria, and lipid droplets (Figure 7). At 24 hpi, the endoplasmic reticula, mitochondria, and lipid droplets in spore cells of the wild type and Δpams1 that formed type I abnormal appressoria had fully degraded or/and migrated into appressoria, while those in the mutant spores that formed type II abnormal appressoria were still present in spore cells (Figure 7). Glycogen granules were transferred from spore cells to an appressorium in a similar manner between the wild type and Δpams1 that formed type I abnormal appressoria during appressorium formation, while glycogen granules were retained in spore cells of Δpams1 that formed type II abnormal appressoria at 24 hpi (Figure 8A). At 8 hpi, the spores and appressoria of the wild type and Δpams1 had one nucleus per cell. At 12 and 24 hpi, the wild type and Δpams1 that formed melanized type I abnormal appressoria had one nucleus in each appressorium, whereas pale type II abnormal appressoria of Δpams1 lost their nuclei (Figures 8B,C). At 24 hpi, the spores of Δpams1 that formed type II abnormal appressoria contained nuclei (Figure 8B). The degradation of the nucleus and other organelles in type II abnormal appressorium after 12 hpi suggests that the appressorium had died.
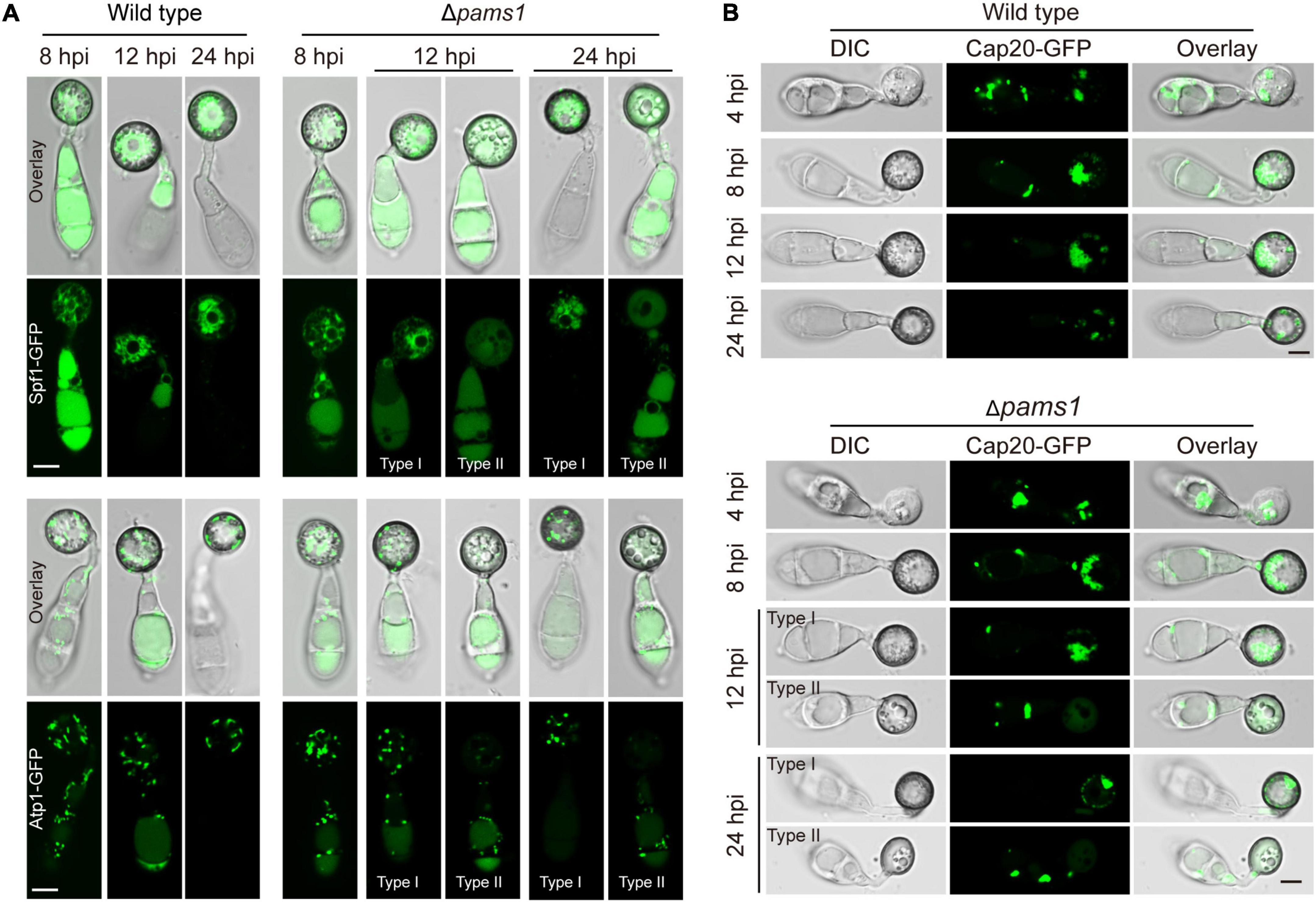
Figure 7. Endoplasmic reticula, mitochondria, and lipid droplets in Δpams1’s appressoria. (A) Distribution of Spf1-GFP-marked endoplasmic reticula and Atp1-GFP-marked mitochondria in appressoria of the wild type and Δpams1 (types I and II abnormal appressoria) at 8, 12, and 24 hpi. (B) Distributions of lipid droplets in spores and appressoria at 4, 8, 12, and 24 hpi. Lipid droplets were marked by Cap20-GFP. Scale bar, 5 μm.
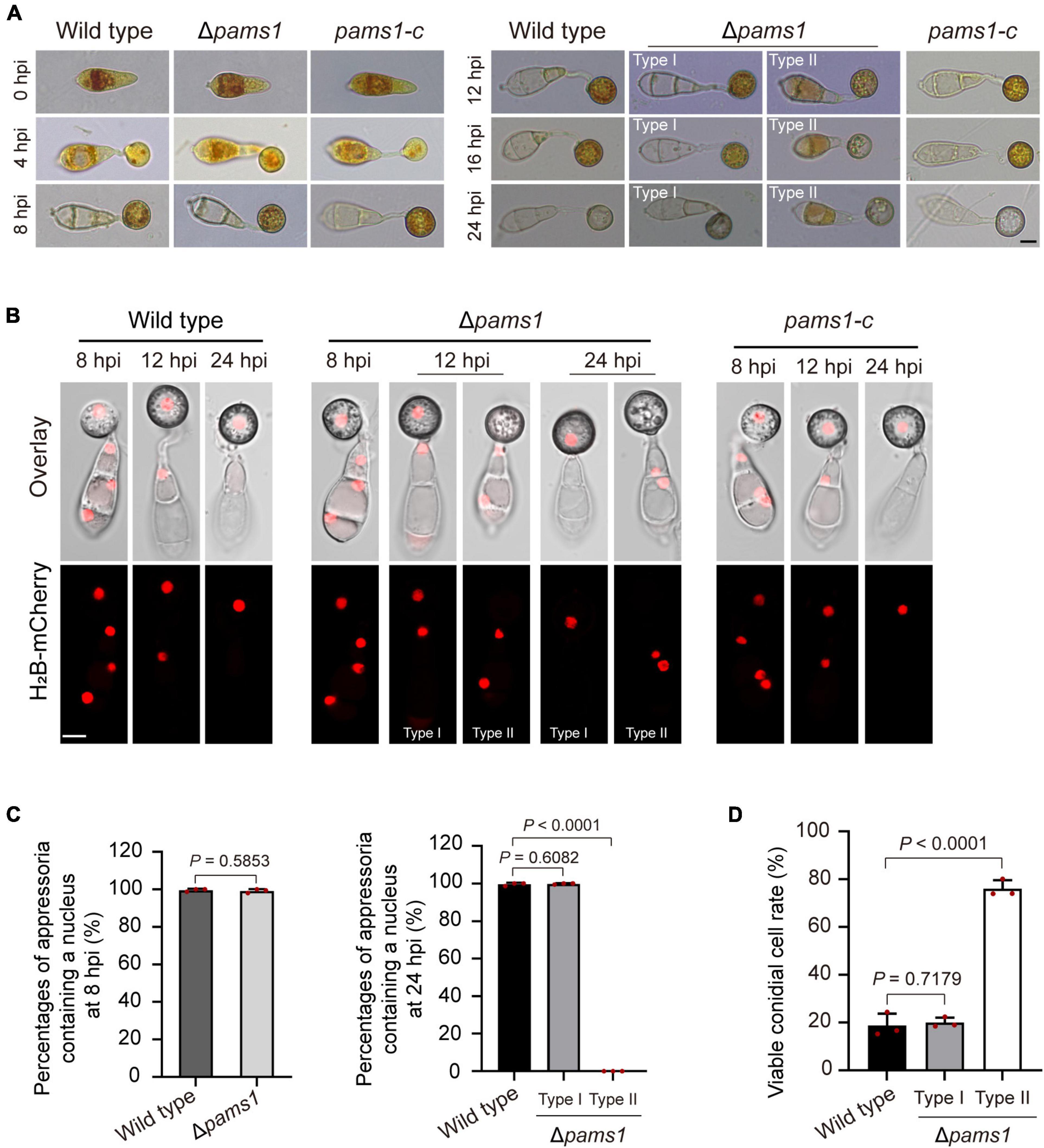
Figure 8. Glycogen granules and nuclei in Δpams1’s appressoria and viable conidial cells in spores that formed appressoria. (A) Distributions of glycogen granules in spores and appressoria at 0, 4, 8, 12, 16, and 24 hpi. Glycogen granules were stained by KI/I2 solution. Glycogen granules in spore cells that formed type II abnormal appressoria were not fully mobilized into appressoria at 24 hpi. (B) Distribution of H2B-mCherry-marked nuclei in appressoria of the wild type, complementation strain pams1-c, and Δpams1 (types I and II abnormal appressoria) at 8, 12, and 24 hpi. (A,B) Scale bars, 5 μm. (C) Percentages of appressoria containing a nucleus at 8 and 24 hpi (n = 3). At least 150 appressoria were counted per replicate. (D) Percentages of viable conidial cells in spores that formed appressoria at 24 hpi (n = 3). The spores were inoculated on plastic coverslips for 24 hpi and stained with FDA. At least 240 conidial cells were counted per replicate.
To further identify the viability of spore cells of Δpams1 at 24 hpi, we stained the germinated spores on hydrophobic borosilicate glass coverslips with FDA. The results displayed that the cytoplasm of 76.06% of the spore cells that formed pale type II malformed appressoria showed green fluorescence (Figure 8D; Supplementary Figure 3), indicating that the spore cells were still viable and the cytoplasm in spore cells had not completely migrated into appressoria. However, the cytoplasm in most spore cells that formed melanized type I malformed appressoria fully mobilized into the appressorial cells, similar to that observed in the wild type. In the pale type II malformed appressoria of Δpams1, many mitochondria, endoplasmic reticula, glycogen granules, lipid droplets, and nuclei were not degraded and migrated from the spore cells into the appressoria (Figures 7, 8).
The appressorial cell wall structure of Δpams1 is severely defective
The ultrastructure of appressorial cell walls showed that the melanin layer was destroyed in Δpams1 (Figure 6C). The melanin layer of the cell wall prevented the escape of high concentrations of glycerol accumulated in an appressorium, resulting in huge intracellular turgor pressure (Chumley and Valent, 1990; deJong et al., 1997; Ryder et al., 2019). To evaluate the effect of a defective melanin layer on appressorial turgor, incipient cytorrhysis assays were conducted to determine turgor pressure of appressoria of Δpams1 in a series of glycerol solutions of varying molarity (Ryder et al., 2019). The proportions of total cell plasmolysis and collapse of Δpams1 appressoria formed on plastic coverslips at 24 hpi were much higher than that of the wild type (Figure 9A), indicating that the turgor pressure in mutant appressoria was lower than that in the wild type. Analysis of the glycerol content at 24 hpi showed that the intracellular glycerol content in the Δpams1 appressoria incubated on hydrophobic PVC films was lower than that in the wild type appressoria, while the amount of extracellular glycerol leaking into the incubation solution from the mutant appressoria was much higher than that from the wild type appressoria (Figure 9B). In addition, the ability of mutant appressorium to adhere to hydrophobic surfaces was not different from that of the wild type. When treated with higher concentrations of a glycerol solution, many appressorial protoplasts of Δpams1 exhibited plasmolysis (Supplementary Figure 4A). Among them, the cell membranes of some melanized type I appressoria were completely separated from the cell wall, forming a spherical protoplast (Figure 9C). The evidence indicates that the appressorial cell wall structure of Δpams1 appressoria is severely defective.
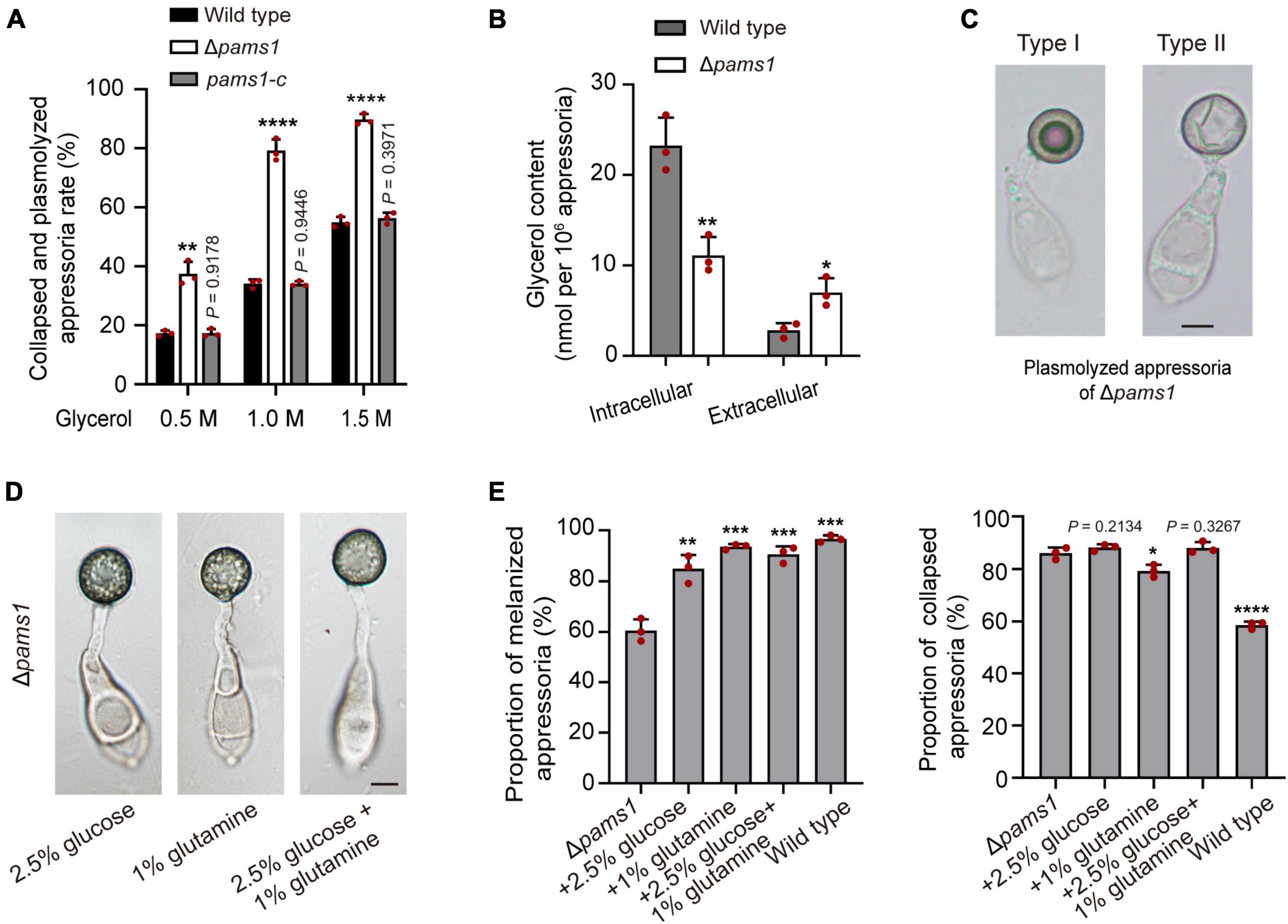
Figure 9. Roles of Pams1 in appressorium cell walls. (A) Incipient cytorrhysis assays for appressorium turgor pressure (n = 3). The appressoria at 24 hpi were incubated in 0.5, 1.0, or 1.5 M of glycerol solutions, and the collapsed and plasmolyzed appressoria were counted under a microscope. At least 140 appressoria were counted per replicate. (B) Intracellular and extracellular glycerol contents of appressoria formed by the wild type and Δpams1 on hydrophobic polyvinyl chloride (PVC) films at 24 hpi (n = 3). (C) Plasmolyzed appressoria (types I and II) formed by Δpams1 at 24 hpi and incubated in a 1.5 M glycerol solution. (D) Appressoria were formed by Δpams1 after the addition of exogenous glucose and/or glutamine at 24 hpi. (E) Addition of exogenous 2.5% glucose and/or 1% glutamine obviously increased the number of melanized appressoria (Left panel) but did not greatly increase appressorium turgor pressure (Right panel) in Δpams1 (n = 3). Appressorium turgor pressure was assayed in a 1.5 M glycerol solution. At least 200 appressoria at 24 hpi were counted per replicate. Unpaired two-tailed Student’s t-test was used for comparisons with non-treated Δpams1 in panel (E) or with the wild type in panels (A,B). *P < 0.05, **P < 0.01, ***P < 0.001, ****P < 0.0001. (C,D) Scale bars, 5 μm.
Exogenous glucose and amino acids partially abolish the Δpams1’s defect in appressorium maturation
To understand whether the death of Δpams1’s type II abnormal appressoria is caused by appressorial nutrient deficiency resulting from disruption of spore cell death, we observed the effect of exogenous nutrients on the appressorium maturation of Δpams1. After adding 2.5% glucose, 1% glutamine, or both simultaneously into spore suspensions, the proportion of melanized appressoria formed by Δpams1 was significantly higher than that without treatment (Figures 9D,E; Supplementary Figure 4B). These melanized appressoria were similar in appearance to the wild type appressoria (Figure 9D; Supplementary Figure 4C). However, the cytoplasm and organelles of spores were still not fully mobilized into the melanized appressoria (Figure 9D; Supplementary Figure 4C), suggesting that the formation of pale type II abnormal appressoria in Δpams1 is related to the unavailability of carbon and nitrogen sources in the mutant appressoria. Under 1.5 M glycerol solution, the percentage of collapsed or plasmolyzed appressoria in Δpams1 with the addition of glucose or both glucose and glutamine was still similar to that without treatment (Figure 9E; Supplementary Figure 4C), indicating that the melanin layer structure of mutant appressoria was still defective.
Loss of Pams1 results in fusion and enlargement of endosomes in appressoria
To explore where and how Pams1 functions, we observed the spatiotemporal localization of Pams1 during appressorium maturation. The GFP-PAMS1 fusion gene under the control of its native promoter was transformed into Δpams1 and could complement the mutant phenotype of Δpams1. GFP-Pams1 expression mainly occurred in appressoria (Figure 10A). Pams1 was initially distributed in membranes of cells and small vesicles at 4–6 hpi, then it was gradually transferred to mid-sized vesicle membranes after 6 hpi, and ultimately coalesced in large-vesicle membranes after 12 hpi (Figure 10A). GFP-Pams1 was co-localized with the endosome marker mCherry-Rab5A in endosome membranes, which indicates that the Pams1-containing intracellular vesicles were endosomes (Figure 10B).
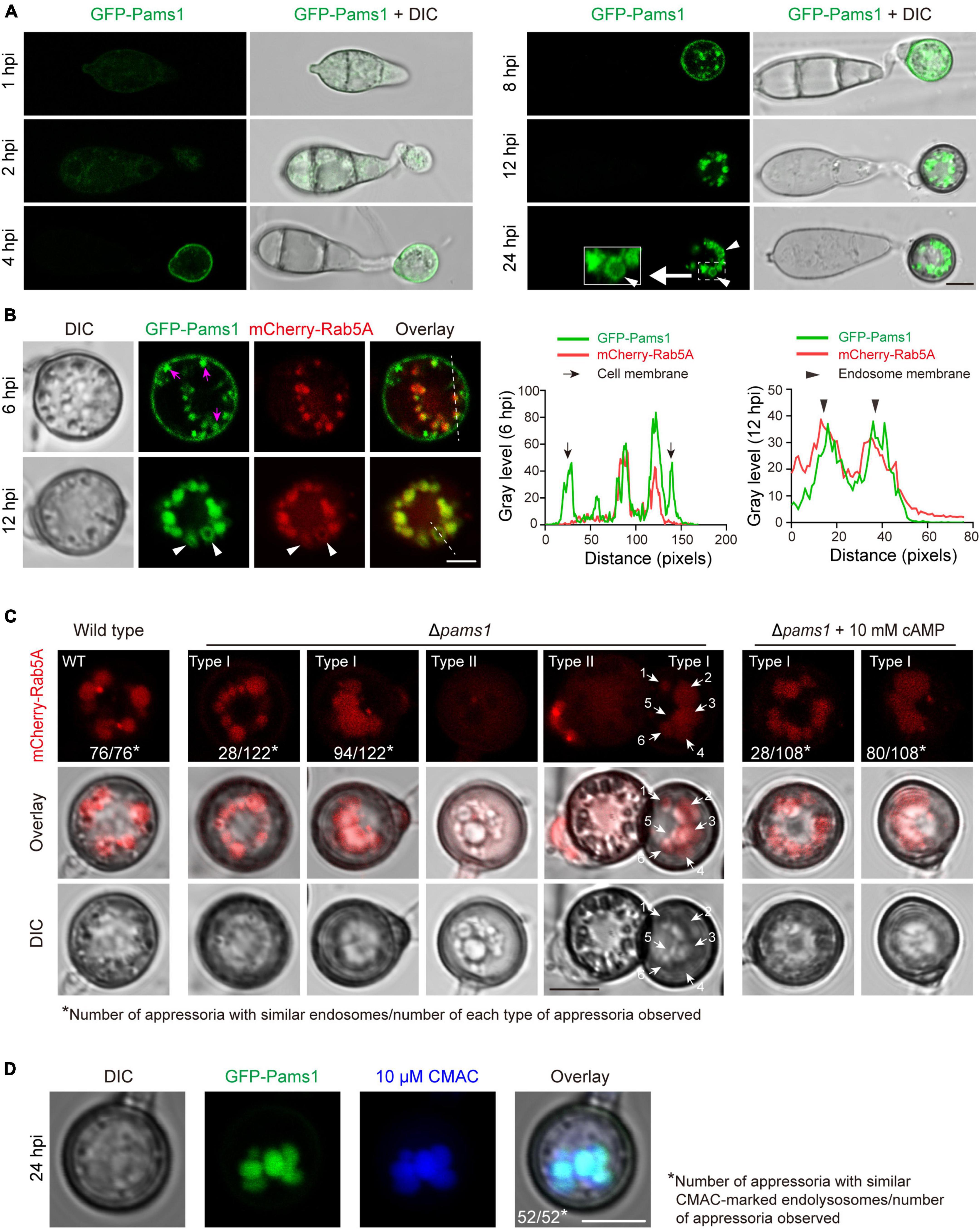
Figure 10. Pams1 is required for the morphology and distribution pattern of endosomes in appressoria. (A) Localization of GFP-Pams1 in spores and developing appressoria at 1–24 hpi. The strain is the complementation strain of Δpams1 constructed by introducing GFP-PAMS1. (B) Left, co-localization of GFP-Pams1 and the endosome marker mCherry-Rab5A in appressoria at 6 and 12 hpi. Right, line-scan graphs showing GFP-Pams1 and mCherry-Rab5A fluorescence density in transverse sections of several endosomes. Pams1 and Rab5A are co-localized in the endosomal membrane. Magenta arrowheads indicate vesicles linked with the cell membrane. The strain is Δpams1 expressing both GFP-PAMS1 and mCherry-RAB5A. White triangular arrowheads indicate endosome membranes (A,B). (C) Endosomes marked by mCherry-Rab5A in the appressoria of the wild type and Δpams1 at 24 hpi. White arrows with numbers refer to six endosomes. (D) Endosomes or endolysosomes were stained by 10 μM CMAC in appressoria of the complementation strain of Δpams1 constructed by introducing GFP-PAMS1. Spore suspensions were added with CMAC solution (final concentration 10 μM), inoculated on hydrophobic borosilicate glass coverslips, and cultured for 24 hpi. Scale bars, 5 μm.
The morphology and distribution patterns of endosomes in Δpams1 were different from those of the wild type. Relative to the wild type, the endosomes of type I abnormal appressoria in Δpams1 were merged into larger vacuoles at 24 hpi. In the wild type, endosomes were circularly arranged in appressoria at 24 hpi. In Δpams1, however, the endosomes of type I abnormal appressoria were arranged circularly or semi-circularly, whereas the endosomes of type II appressoria were disintegrated (Figure 10C). The expression of mCherry-Rab5A slightly increased endosomal size at 12 hpi but not at 24 hpi (Figures 11A,B). However, the size of endosomes of type I abnormal appressoria in Δpams1 was greatly larger than that of the wild type (Figures 10C, 11), meaning that deletion of PAMS1 resulted in increased fusion between endosomes or with other vesicles or vacuoles. After the addition of a vacuolar dye CMAC to the spore suspensions, CMAC co-localized with GFP-Pams1 in appressorial endosomes at 24 hpi (Figure 10D). Thus, these appressorial endosomes at 24 hpi were endolysosomes. Relative to the control (Figure 10A: 24 hpi), however, 24 h of CMAC treatment promoted fusion of endosomes in appressoria (Figure 10D). In the type I appressoria of Δpams1 at 24 hpi, the large vacuoles observed under differential interference contrast (DIC) were co-localized with the fluorescence signal of mCherry-Rab5A (Figure 10C), suggesting that these large vacuoles were merged endolysosomes. These merged large endolysosomes were also seen in Figures 6B,D, marked with red stars in the type I appressoria of Δpams1 at 24 hpi.
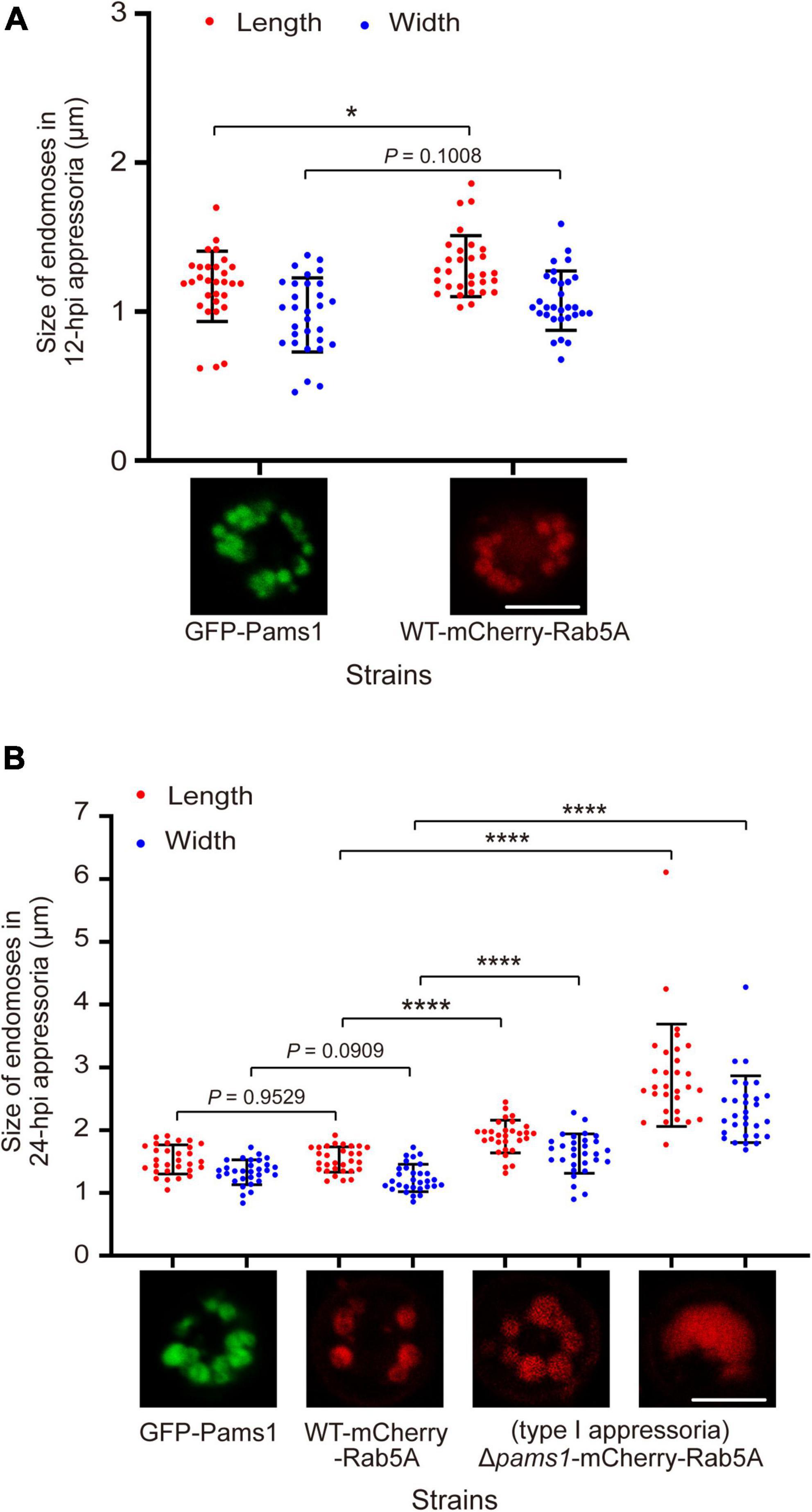
Figure 11. Pams1 prevents excessive fusion of endosomes during appressorium maturation in M. oryzae. (A) Size of 12-hpi appressorial endosomes. (B) Size of 24-hpi appressorial endosomes. The largest three endosomes in each appressorium were selected to measure their size, and a total of 10 appressoria were measured. The strains used here are as follows: GFP-Pams1 (the complementation strain of Δpams1 expressing GFP-PAMS1), WT-mCherry-Rab5A (the wild type strain expressing mCherry-RAB5A), and Δpams1-mCherry-Rab5A (Δpams1 expressing mCherry-RAB5A). Spore suspensions were inoculated on hydrophobic borosilicate glass coverslips and incubated for 12 or 24 hpi. The GFP-Pams1 and WT-mCherry-Rab5A strains formed normal appressoria, and the Δpams1-mCherry-Rab5A strain formed two types of abnormal appressoria, in which only endosomes or endolysosomes in type I appressoria were measured. *p < 0.05, ****p < 0.0001. Scale bars, 5 μm.
Pams1 is involved in the cAMP-PKA pathway during appressorium maturation
Magnaporthe oryzae senses plant surface signals and differentiate them into appressoria via the cAMP-PKA and Pmk1 MAP kinase pathways (Jiang et al., 2018). Among them, the cAMP-PKA signal is transmitted into the cell through the internalization of the cell membrane to form endosomes (Li et al., 2019). To check if the cAMP-PKA pathway was disturbed in Δpams1, we tested the effect of exogenous cAMP on appressorium maturation. After adding 10 mM of cAMP analogs (8-Bromo-cAMP) into spore suspensions, Δpams1 formed a higher proportion of melanized type I abnormal appressoria on plastic coverslips at 24 hpi, as compared to the result in the absence of cAMP treatment (Figures 12A,B). Furthermore, the addition of exogenous cAMP did not eliminate the mutant’s defect in endosomal fusion (Figure 10C).
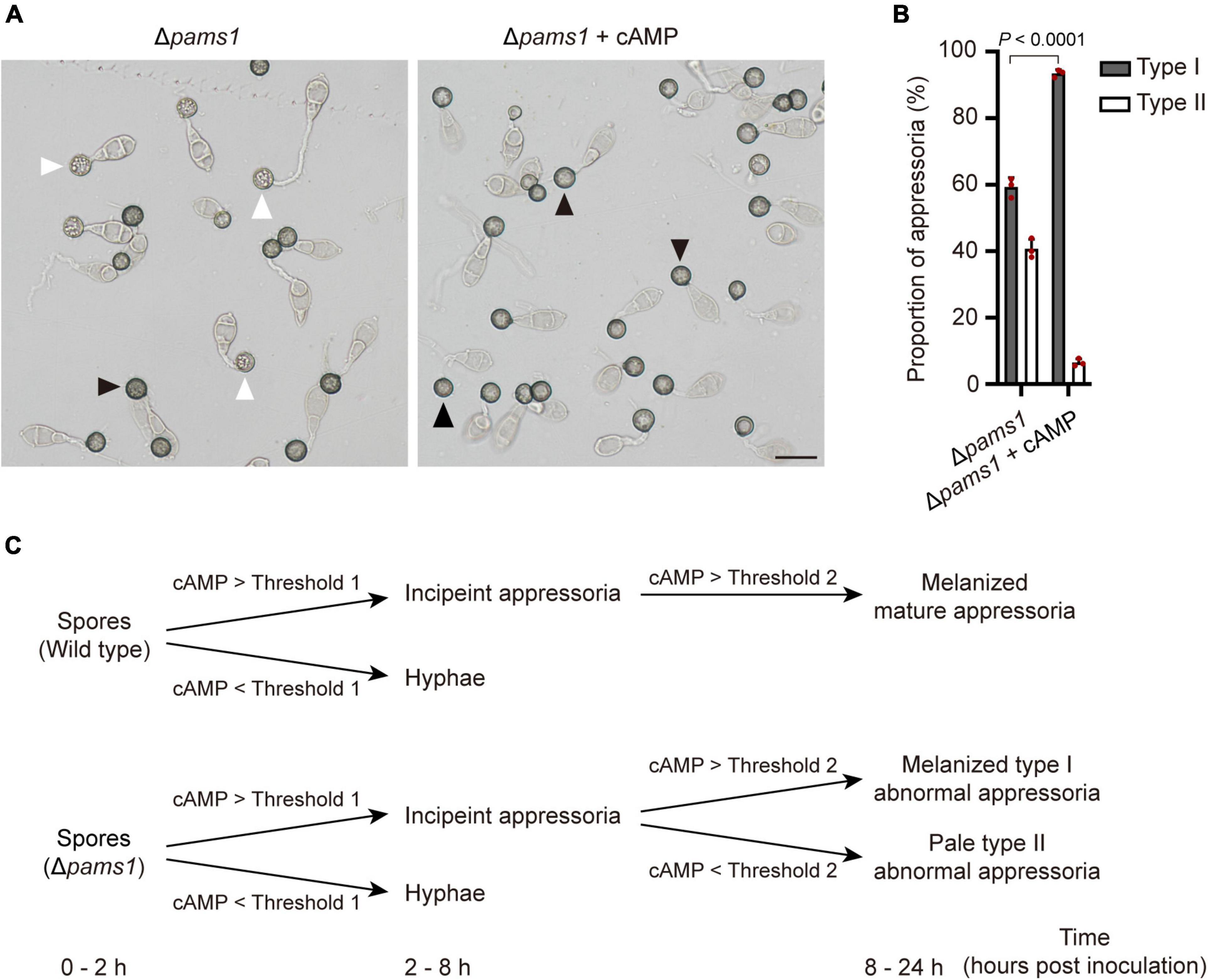
Figure 12. Addition of exogenous cAMP increased the proportion of melanized type I abnormal appressoria formed by Δpams1 on plastic coverslips. (A) Appressoria formed by Δpams1 on plastic coverslips after treatment with 10 mM cAMP at 24 hpi. Black arrows indicate type I abnormal appressoria, and white arrows indicate type II abnormal appressoria. Scale bar, 20 μm. (B) Proportions of types I and II abnormal appressoria formed by Δpams1 on plastic coverslips treated with 10 mM cAMP. The wild type did not form abnormal appressoria on plastic coverslips. At least 110 appressoria were counted per replicate. (C) Schematic illustration of how intracellular cAMP levels affect the differentiation of spores into incipient appressoria and then into mature appressoria in Δpams1. On a membrane surface, if the intracellular cAMP content is higher than threshold 1, the germinated spore of Δpams1 differentiates into an incipient appressorium or it differentiates into a hypha; if the intracellular cAMP of the incipient appressorium is higher than threshold 2, the appressorium continues to develop into a melanized type I abnormal appressorium, otherwise it develops into a pale type II abnormal appressorium.
Discussion
Rice blasts can cause the most serious fungal epidemics in rice production (Talbot, 2003; Inoue et al., 2017). Appressorium formation, penetration, and infective growth mechanisms of M. oryzae have long been the foci of much academic research (Howard and Valent, 1996; Talbot, 2003; Jiang et al., 2018; Ryder et al., 2019; Huang et al., 2022). In this study, we identified an appressorium-specific membrane protein, Pams1, which is required for appressorium maturation and virulence in M. oryzae. PAMS1 was highly expressed in the wild type appressoria; however, it was greatly downregulated in the incipient appressoria of Δvrf1, Δhox7, and Δhox7Δvrf1. Both Vrf1 and Hox7 are transcription factors regulating appressorium maturation in M. oryzae (Kim et al., 2009; Cao et al., 2016; Oses-Ruiz et al., 2021; Huang et al., 2022).
The Δpams1 mutant showed a reduced virulence on barley and rice. This reduced virulence is owing to its decreased appressorial penetration into plant cuticles. Deletion of PAMS1 resulted in the formation of two types of abnormal appressoria which were different from those formed by the wild type in structure. These two types of abnormal appressoria have not been reported in M. oryzae. Appressorium formation is divided into three stages: differentiation, maturation, and penetration (Dagdas et al., 2012; Inoue et al., 2017). The time point at which the Δpams1 mutant differentiated into two types of appressoria (10 hpi) was slightly later than the time when the normal appressoria of the wild type completed expansion and started cell wall melanization (before 8 hpi) (Howard and Valent, 1996; Dean, 1997). Comparing the appressorium morphogenesis and maturation process in the wild type and Δpams1, 8–10 hpi was found as the time point for an appressorium to transit from the differentiation stage to the mature stage.
Abnormal endosome structure and the impaired cAMP-PKA signaling pathway during appressorium maturation are two reasons why Δpams1 forms two types of abnormal appressoria. Several large vesicles were observed in the DIC pictures and the electron microscope pictures of the Δpams1 appressoria at 18 or 24 hpi. These large branched vesicles have structural features of late endosomes and endolysosomes (Kou and Naqvi, 2016). They were co-localized with the endosomal marker protein Rab5A, suggesting that these large vesicles of Δpams1 observed by electron or light microscopy were abnormal endosomes or endolysosomes. In the appressoria of the wild type strain, endosomes were relatively small and not easily distinguishable from other types of vesicles in DIC pictures.
In M. oryzae, spores germinate and sense the induction signals from rice surfaces and synthesize cAMP, which in turn activates PKA kinases and appressorium formation (Jiang et al., 2018). Component members of the cAMP-PKA pathway, such as Pth11, Gα, Rgs1, and Mac1, receive hydrophobic signals and chemical molecules on the plant surface and are internalized from the cell membrane (Zhang et al., 2011; Ramanujam et al., 2013; Kou and Naqvi, 2016; Li et al., 2019). These proteins in the signaling pathway are localized in the endosomal membrane. Endocytosis is required for receptor internalization, signaling activation, and appressorium formation (Li X. et al., 2017). Pams1 was specifically expressed in the appressoria of M. oryzae. At 4–12 hpi, Pams1 was gradually translocated from the cell membrane to the endosomal membrane by endocytosis. Pams1 inhibited excessive fusion between endosomes or with vacuoles during appressorium maturation. The endosome structure of the appressorium was abnormal in Δpams1. Relative to the wild type, Δpams1 formed larger endosomes or endolysosomes at 24-hpi appressoria. We speculate that due to structural defects in the endosomes or endolysosomes, environmental stimuli sensed by the maturing appressorium were not properly transmitted into the cell, resulting in dysfunction of the cAMP-PKA pathway and insufficient cAMP synthesis in Δpams1. In Δpams1, appressorium differentiation was unaffected, but the addition of exogenous cAMP promoted the mutant to form a higher proportion of melanized type I appressoria, suggesting that a fraction of the maturing appressoria did not contain enough cAMP to mature. In other words, cAMP synthesis was defective in these appressoria. The degree of reduction in synthesized cAMP content varied among the maturing appressoria of Δpams1. There may be a threshold of cAMP content below which the incipient appressorium cannot continue to develop into a mature appressorium, but instead enters a process of cell death. However, the threshold for this cAMP content remains unknown. Before 8 hpi, the appressorial cell walls underwent preliminary melanization. After 8 hpi, the appressoria with intracellular cAMP content above the threshold continued to develop and mature, with continued melanization of the cell walls and transportation of the cytoplasm in spore cells to appressorial cells. In contrast, development and cell wall melanization gradually stopped in the appressoria with lower intracellular cAMP content than the threshold. As a result, at 24 hpi, the Δpams1 mutant formed two types of abnormal appressoria: melanized type I appressoria and pale type II appressoria, in which type II appressoria were dead. This means that the formation of two types of appressoria reflects the difference in the degree of reduction in intracellular cAMP levels among the incipient appressoria of Δpams1 (Figure 12C).
In Δpams1, the pale type II appressoria were not viable after 12 hpi, and vesicles in these appressoria were autophagic body-like vesicles (Liu X. H. et al., 2007). During the appressorium formation of M. oryzae, autophagy and ferroptosis are involved in the death process of spore cells (Veneault-Fourrey et al., 2006; Shen et al., 2020). After autophagy or ferroptosis was blocked, the germinated spore cells continued to survive, and their intracellular contents could not be degraded and transported into an appressorium (Veneault-Fourrey et al., 2006; Shen et al., 2020), which is similar to the spore cells that formed pale type II appressoria in Δpams1. However, loss of autophagy and ferroptosis did not cause the death of appressoria themselves (Veneault-Fourrey et al., 2006; Lu et al., 2009; Shen et al., 2020), which is different from that in the pale type II appressoria of Δpams1. Autophagic body-like vesicles appeared in type II appressoria of Δpams1, indicating that the activation of autophagy is involved in the death process of type II appressoria. The addition of an exogenous nitrogen source or carbon source could prevent Δpams1 from forming pale type II appressorium, which means that it reverses the death process or inhibits autophagy of the appressorium. This is consistent with the basic mechanism of autophagy, that is, starvation induces autophagy (Kuma et al., 2004). Defects in the TOR signaling pathway led to the germinated spores forming long tubes and delayed differentiation of irregular appressoria (Marroquin-Guzman et al., 2017; Sun et al., 2019). These irregular appressoria are morphologically different from those formed by Δpams1. The appressoria of Δpams1 were as normal as the wild type before 10 hpi. In mammals, cAMP can promote or inhibit autophagy, depending on the cell type (Grisan et al., 2021). The addition of exogenous cAMP promoted conidial death and prevented appressorial death in Δpams1, implying that cAMP promotes autophagy in germinated conidial cells and inhibits autophagy in developing appressoria in M. oryzae. However, the relationship between Pams1 and the cAMP-PKA pathway, TOR signaling, autophagy, or cell cycle arrest needs to be carefully studied in future.
In summary, we identified the biological function of an appressorium-specifically expressed protein, Pams1. The localization of Pams1 is translocated from the cell membrane to the endosomal membrane before the maturation of an appressorium. Pams1 is involved in the maintenance of the morphology and distribution pattern of endosomes, as well as the function of the cAMP-PKA pathway in a maturing appressorium. PAMS1 is required for appressorium maturation and pathogenicity, but not for colony growth, sporulation, spore germination, and appressorium differentiation in M. oryzae.
Data availability statement
The original contributions presented in this study are included in the article/Supplementary material, further inquiries can be directed to the corresponding author.
Author contributions
JL and JW conceived and designed the study, analyzed the data, and wrote the manuscript. JW, QW, PH, ZH, YQ, and HW performed the experiments with phenotypical and biochemical assays. F-CL, X-HL, and JL contributed to the reagents, plant, and fungal materials. JW, QW, and PH collected the data. All authors contributed to the article and approved the submitted version.
Funding
This work was funded by the National Natural Science Foundation of China (Grant no. 31871908) and the Key R&D Projects of Zhejiang Province, China (Grant no. 2021C02010). This work was also supported by a grant from Organism Interaction from Zhejiang Xianghu Laboratory to F-CL.
Acknowledgments
We thank Xiaoxiao Feng at Agricultural Experiment Station, Zhejiang University for her help in experiment management.
Conflict of interest
The authors declare that the research was conducted in the absence of any commercial or financial relationships that could be construed as a potential conflict of interest.
Publisher’s note
All claims expressed in this article are solely those of the authors and do not necessarily represent those of their affiliated organizations, or those of the publisher, the editors and the reviewers. Any product that may be evaluated in this article, or claim that may be made by its manufacturer, is not guaranteed or endorsed by the publisher.
Supplementary material
The Supplementary Material for this article can be found online at: https://www.frontiersin.org/articles/10.3389/fpls.2022.955254/full#supplementary-material
Footnotes
References
Cao, H., Huang, P., Zhang, L., Shi, Y., Sun, D., Yan, Y., et al. (2016). Characterization of 47 Cys2 -His2 zinc finger proteins required for the development and pathogenicity of the rice blast fungus Magnaporthe oryzae. New Phytol. 211, 1035–1051. doi: 10.1111/nph.13948
Cao, H. J., Huang, P. Y., Yan, Y. X., Shi, Y. K., Dong, B., Liu, X. H., et al. (2018). The basic helix-loop-helix transcription factor Crf1 is required for development and pathogenicity of the rice blast fungus by regulating carbohydrate and lipid metabolism. Environ. Microbiol. 20, 3427–3441. doi: 10.1111/1462-2920.14387
Chumley, F. G., and Valent, B. (1990). Genetic-analysis of melanin-deficient, nonpathogenic mutants of Magnaporthe-grisea. Mol. Plant Microbe Interact. 3, 135–143. doi: 10.1094/MPMI-3-135
Dagdas, Y. F., Yoshino, K., Dagdas, G., Ryder, L. S., Bielska, E., Steinberg, G., et al. (2012). Septin-mediated plant cell invasion by the rice blast fungus, Magnaporthe oryzae. Science 336, 1590–1595. doi: 10.1126/science.1222934
Dean, R. A. (1997). Signal pathways and appressorium morphogenesis. Annu. Rev. Phytopathol. 35, 211–234. doi: 10.1146/annurev.phyto.35.1.211
deJong, J. C., MCCormack, B. J., Smirnoff, N., and Talbot, N. J. (1997). Glycerol generates turgor in rice blast. Nature 389, 244–245. doi: 10.1038/38418
Fisher, M. C., Henk, D. A., Briggs, C. J., Brownstein, J. S., Madoff, L. C., McCraw, S. L., et al. (2012). Emerging fungal threats to animal, plant and ecosystem health. Nature 484, 186–194. doi: 10.1038/nature10947
Grisan, F., Iannucci, L. F., Surdo, N. C., Gerbino, A., Zanin, S., Di Benedetto, G., et al. (2021). PKA compartmentalization links cAMP signaling and autophagy. Cell Death Differ. 28, 2436–2449. doi: 10.1038/s41418-021-00761-8
Howard, R. J., Ferrari, M. A., Roach, D. H., and Money, N. P. (1991). Penetration of hard substrates by a fungus employing enormous turgor pressures. Proc. Natl. Acad. Sci. U.S.A. 88, 11281–11284. doi: 10.1073/pnas.88.24.11281
Howard, R. J., and Valent, B. (1996). Breaking and entering: Host penetration by the fungal rice blast pathogen Magnaporthe grisea. Annu. Rev. Microbiol. 50, 491–512. doi: 10.1146/annurev.micro.50.1.491
Huang, P. Y., Wang, J., Li, Y., Wang, Q., Huang, Z., Qian, H., et al. (2022). Transcription factors Vrf1 and Hox7 coordinately regulate appressorium maturation in the rice blast fungus Magnaporthe oryzae. Microbiol. Res. 263:127141. doi: 10.1016/j.micres.2022.127141
Inoue, Y., Vy, T. T. P., Yoshida, K., Asano, H., Mitsuoka, C., Asuke, S., et al. (2017). Evolution of the wheat blast fungus through functional losses in a host specificity determinant. Science 357, 80–82. doi: 10.1126/science.aam9654
Jiang, C., Zhang, X., Liu, H. Q., and Xu, J. R. (2018). Mitogen-activated protein kinase signaling in plant pathogenic fungi. PLoS Pathog. 14:e1006875. doi: 10.1371/journal.ppat.1006875
Kim, S., Park, S. Y., Kim, K. S., Rho, H. S., Chi, M. H., Choi, J., et al. (2009). Homeobox transcription factors are required for conidiation and appressorium development in the rice blast fungus Magnaporthe oryzae. PLoS Genet. 5:e1000757. doi: 10.1371/journal.pgen.1000757
Kou, Y., and Naqvi, N. I. (2016). Surface sensing and signaling networks in plant pathogenic fungi. Semin. Cell Dev. Biol. 57, 84–92. doi: 10.1016/j.semcdb.2016.04.019
Kou, Y., Tan, Y. H., Ramanujam, R., and Naqvi, N. I. (2017). Structure-function analyses of the Pth11 receptor reveal an important role for CFEM motif and redox regulation in rice blast. New Phytol. 214, 330–342. doi: 10.1111/nph.14347
Kuma, A., Hatano, M., Matsui, M., Yamamoto, A., Nakaya, H., Yoshimori, T., et al. (2004). The role of autophagy during the early neonatal starvation period. Nature 432, 1032–1036. doi: 10.1038/nature03029
Lee, Y. H., and Dean, R. A. (1993). cAMP regulates infection structure formation in the plant-pathogenic fungus Magnaporthe-grisea. Plant Cell 5, 693–700. doi: 10.1105/tpc.5.6.693
Lee, Y. H., and Dean, R. A. (1994). Hydrophobicity of contact surface induces appressorium formation in Magnaporthe-grisea. FEMS Microbiol. Lett. 115, 71–75. doi: 10.1111/j.1574-6968.1994.tb06616.x
Li, H. J., Lu, J. P., Liu, X. H., Zhang, L. L., and Lin, F. C. (2012). Vectors building and usage for gene knockout, protein expression and fluorescent fusion protein in the rice blast fungus. J. Agric. Biotechnol. 20, 94–104.
Li, X., Zhong, K. L., Yin, Z. Y., Hu, J. X., Wang, W. H., Li, L. W., et al. (2019). The seven transmembrane domain protein MoRgs7 functions in surface perception and undergoes coronin MoCrn1-dependent endocytosis in complex with G subunit MoMagA to promote cAMP signaling and appressorium formation in Magnaporthe oryzae. PLoS Pathog. 15:e1007382. doi: 10.1371/journal.ppat.1007382
Li, Y., Zhang, X., Hu, S., Liu, H. Q., and Xu, J. R. (2017). PKA activity is essential for relieving the suppression of hyphal growth and appressorium formation by MoSfl1 in Magnaporthe oryzae. PLoS Genet. 13:e1006954. doi: 10.1371/journal.pgen.1006954
Li, X., Gao, C., Li, L., Liu, M., Yin, Z., Zhang, H., et al. (2017). MoEnd3 regulates appressorium formation and virulence through mediating endocytosis in rice blast fungus Magnaporthe oryzae. PLoS Pathog. 13:e1006449. doi: 10.1371/journal.ppat.1006449
Liu, H., Suresh, A., Willard, F. S., Siderovski, D. P., Lu, S., and Naqvi, N. I. (2007). Rgs1 regulates multiple G alpha subunits in Magnaporthe pathogenesis, asexual growth and thigmotropism. EMBO J. 26, 690–700. doi: 10.1038/sj.emboj.7601536
Liu, X. H., Lu, J. P., Zhang, L., Dong, B., Min, H., and Lin, F. C. (2007). Involvement of a Magnaporthe grisea serine/threonine kinase gene, MgATG1, in appressorium turgor and pathogenesis. Eukaryot. Cell 6, 997–1005. doi: 10.1128/EC.00011-07
Lu, J., Cao, H., Zhang, L., Huang, P., and Lin, F. (2014). Systematic analysis of Zn2Cys6 transcription factors required for development and pathogenicity by high-throughput gene knockout in the rice blast fungus. PLoS Pathog. 10:e1004432. doi: 10.1371/journal.ppat.1004432
Lu, J. P., Liu, X. H., Feng, X. X., Min, H., and Lin, F. C. (2009). An autophagy gene, MgATG5, is required for cell differentiation and pathogenesis in Magnaporthe oryzae. Curr. Genet. 55, 461–473. doi: 10.1007/s00294-009-0259-5
Marroquin-Guzman, M., Sun, G., and Wilson, R. A. (2017). Glucose-ABL1-TOR signaling modulates cell cycle tuning to control terminal appressorial cell differentiation. PLoS Genet. 13:e1006557. doi: 10.1371/journal.pgen.1006557
Marroquin-Guzman, M., and Wilson, R. A. (2015). GATA-dependent glutaminolysis drives appressorium formation in Magnaporthe oryzae by suppressing tor inhibition of cAMP/PKA signaling. PLoS Pathog. 11:e1004851. doi: 10.1371/journal.ppat.1004851
Oses-Ruiz, M., Cruz-Mireles, N., Martin-Urdiroz, M., Soanes, D. M., Eseola, A. B., Tang, B. Z., et al. (2021). Appressorium-mediated plant infection by Magnaporthe oryzae is regulated by a Pmk1-dependent hierarchical transcriptional network. Nat. Microbiol. 6, 1383–1397. doi: 10.1038/s41564-021-00978-w
Qu, Y. M., Wang, J., Zhu, X. M., Dong, B., Liu, X. H., Lu, J. P., et al. (2020). The P5-type ATPase Spf1 is required for development and virulence of the rice blast fungus Pyricularia oryzae. Curr. Genet. 66, 385–395. doi: 10.1007/s00294-019-01030-5
Ramanujam, R., Calvert, M. E., Selvaraj, P., and Naqvi, N. I. (2013). The late endosomal HOPS complex anchors active G-protein signaling essential for pathogenesis in Magnaporthe oryzae. PloS Pathog. 9:e1003527. doi: 10.1371/journal.ppat.1003527
Ryder, L. S., Dagdas, Y. F., Kershaw, M. J., Venkataraman, C., Madzvamuse, A., Yan, X., et al. (2019). A sensor kinase controls turgor-driven plant infection by the rice blast fungus. Nature 574, 423–427. doi: 10.1038/s41586-019-1637-x
Sambrook, J., and Russell, D. W. (2001). Molecular Cloning: A Laboratory Manual, 3nd Edn. New York, NY: Cold Spring Harbor Laboratory Press.
Scott, C. C., Vacca, F., and Gruenberg, J. (2014). Endosome maturation, transport and functions. Semin. Cell Dev. Biol. 31, 2–10. doi: 10.1016/j.semcdb.2014.03.034
Selvaraj, P., Shen, Q., Yang, F., and Naqvi, N. I. (2017). Cpk2, a catalytic subunit of cyclic AMP-PKA, regulates growth and pathogenesis in rice blast. Front. Microbiol. 8:2289. doi: 10.3389/fmicb.2017.02289
Shen, Q., Liang, M. L., Yang, F., Deng, Y. Z., and Naqvi, N. I. (2020). Ferroptosis contributes to developmental cell death in rice blast. New Phytol. 227, 1831–1846. doi: 10.1111/nph.16636
Shi, Y. K., Wang, H., Yan, Y. X., Cao, H. J., Liu, X. H., Lin, F. C., et al. (2018). Glycerol-3-phosphate shuttle is involved in development and virulence in the rice blast fungus Pyricularia oryzae. Front. Plant Sci. 9:687. doi: 10.3389/fpls.2018.00687
Skamnioti, P., and Gurr, S. J. (2007). Magnaporthe grisea cutinase2 mediates appressorium differentiation and host penetration and is required for full virulence. Plant Cell 19, 2674–2689. doi: 10.1105/tpc.107.051219
Sun, G. C., Qi, X. B., and Wilson, R. A. (2019). A feed-forward subnetwork emerging from integrated TOR- and cAMP/PKA-signaling architecture reinforces Magnaporthe oryzae appressorium morphogenesis. Mol. Plant Microbe Interact. 32, 593–607. doi: 10.1094/MPMI-10-18-0287-R
Talbot, N. J. (2003). On the trail of a cereal killer: Exploring the biology of Magnaporthe grisea. Annu. Rev. Microbiol. 57, 177–202. doi: 10.1146/annurev.micro.57.030502.090957
Talbot, N. J., Ebbole, D. J., and Hamer, J. E. (1993). Identification and characterization of Mpg1, a gene involved in pathogenicity from the rice blast fungus Magnaporthe-grisea. Plant Cell 5, 1575–1590. doi: 10.1105/tpc.5.11.1575
Thines, E., Weber, R. W. S., and Talbot, N. J. (2000). MAP kinase and protein kinase A-dependent mobilization of triacylglycerol and glycogen during appressorium turgor generation by Magnaporthe grisea. Plant Cell 12, 1703–1718. doi: 10.1105/tpc.12.9.1703
Veneault-Fourrey, C., Barooah, M., Egan, M., Wakley, G., and Talbot, N. J. (2006). Autophagic fungal cell death is necessary for infection by the rice blast fungus. Science 312, 580–583. doi: 10.1126/science.1124550
Wilson, R. A., and Talbot, N. J. (2009). Under pressure: Investigating the biology of plant infection by Magnaporthe oryzae. Nat. Rev. Microbiol. 7, 185–195. doi: 10.1038/nrmicro2032
Xu, J. R., and Hamer, J. E. (1996). MAP kinase and cAMP signaling regulate infection structure formation and pathogenic growth in the rice blast fungus Magnaporthe grisea. Genes Dev. 10, 2696–2706. doi: 10.1101/gad.10.21.2696
Xu, J. R., Staiger, C. J., and Hamer, J. E. (1998). Inactivation of the mitogen-activated protein kinase Mps1 from the rice blast fungus prevents penetration of host cells but allows activation of plant defense responses. Proc. Natl. Acad. Sci. U.S.A. 95, 12713–12718. doi: 10.1073/pnas.95.21.12713
Xu, J. R., Urban, M., Sweigard, J. A., and Hamer, J. E. (1997). The CPKA gene of Magnaporthe grisea is essential for appressorial penetration. Mol. Plant Microbe Interact. 10, 187–194. doi: 10.1094/MPMI.1997.10.2.187
Yan, Y. X., Wang, H., Zhu, S. Y., Wang, J., Liu, X. H., Lin, F. C., et al. (2019). The methylcitrate cycle is required for development and virulence in the rice blast fungus Pyricularia oryzae. Mol. Plant Microbe Interact. 32, 1148–1161. doi: 10.1094/MPMI-10-18-0292-R
Keywords: Magnaporthe oryzae, appressorium formation, endosome, cAMP, pathogenicity, cell death, cAMP-PKA, endolysosome
Citation: Wang J, Wang Q, Huang P, Qu Y, Huang Z, Wang H, Liu X-H, Lin F-C and Lu J (2022) An appressorium membrane protein, Pams1, controls infection structure maturation and virulence via maintaining endosomal stability in the rice blast fungus. Front. Plant Sci. 13:955254. doi: 10.3389/fpls.2022.955254
Received: 28 May 2022; Accepted: 04 August 2022;
Published: 09 September 2022.
Edited by:
Sabrina Sarrocco, University of Pisa, ItalyReviewed by:
Nicole Donofrio, University of Delaware, United StatesWei Siao, Flanders Institute for Biotechnology, Belgium
Copyright © 2022 Wang, Wang, Huang, Qu, Huang, Wang, Liu, Lin and Lu. This is an open-access article distributed under the terms of the Creative Commons Attribution License (CC BY). The use, distribution or reproduction in other forums is permitted, provided the original author(s) and the copyright owner(s) are credited and that the original publication in this journal is cited, in accordance with accepted academic practice. No use, distribution or reproduction is permitted which does not comply with these terms.
*Correspondence: Jianping Lu, jplu@zju.edu.cn