- Department of Sustainable Crop Production (DI.PRO.VES.), Università Cattolica del Sacro Cuore, Piacenza, Italy
The fungal genus Colletotrichum includes plant pathogens that cause substantial economic damage to horticultural, ornamental, and fruit tree crops worldwide. Here, we conducted a systematic literature review to retrieve and analyze the metadata on the influence of temperature on four biological processes: (i) mycelial growth, (ii) conidial germination, (iii) infection by conidia, and (iv) sporulation. The literature review considered 118 papers (selected from a total of 1,641 papers found with the literature search), 19 Colletotrichum species belonging to eight clades (acutatum, graminicola, destructivum, coccodes, dematium, gloeosporioides, and orbiculare), and 27 host plants (alfalfa, almond, apple, azalea, banana, barley, bathurst burr, blueberry, celery, chilli, coffee, corn, cotton, cowpea, grape, guava, jointvetch, lentil, lupin, olive, onion, snap bean, spinach, strawberry, tomato, watermelon, and white bean). We used the metadata to develop temperature-dependent equations representing the effect of temperature on the biological processes for the different clades and species. Inter- and intra-clades similarities and differences are analyzed and discussed. A multi-factor cluster analysis identified four groups of clades with similar temperature dependencies. The results should facilitate further research on the biology and epidemiology of Colletotrichum species and should also contribute to the development of models for the management of anthracnose diseases.
Introduction
The fungi in the genus Colletotrichum (phylum: Ascomycota, class: Sordariomycetes) include plant pathogens that cause substantial damages to a wide variety of woody and herbaceous plants (Sutton, 1992; Hyde et al., 2009; Cannon et al., 2012). Dean et al. (2012) rated the genus as the eighth most important group of plant pathogenic fungi in the world, based on scientific and economic importance. In the first half of the twentieth century, hundreds of species were identified as Colletotrichum based on morphology and the hosts from which they were isolated. The genus was then revised by Von Arx primarily based on specific morphological characteristics in culture, reducing about 750 species to only 11 (Du et al., 2005). Nowadays, about 100 Colletotrichum species, which are divided into phylogenetic clades based on multilocus molecular analysis, are now commonly accepted (Cannon et al., 2012; Vieira et al., 2020; Talhinhas and Baroncelli, 2021).
Detailed descriptions of the morphological characteristics of Colletotrichum species belonging to major phylogenetic clades were provided by Damm et al. (2009, 2012, 2013, 2014) and Weir et al. (2012). Colletotrichum species usually have hyaline, smooth-walled, septate, branched vegetative hyphae, with diameters ranging from 1 to 11 μm; chlamydospores are seldom observed. Conidiomata are acervular or absent; conidiophores and setae form directly on hyphae. Setae measure from 40 to 150 μm, with color ranging from hyaline to dark brown. Conidia are hyaline, smooth-walled, aseptate, straight to curved, fusiform to cylindrical, 8.5–26 μm long, and 3–8 μm wide. Ascospores, which develop in 8-spore asci in perithecia, are oblong-elliptical, straight or rarely slightly curved, 9–43 μm long (mostly 13–23 μm), and 3–8 μm wide.
As plant pathogens, Colletotrichum species are widely distributed in tropical and subtropical regions, affecting bananas, cassava, sorghum and other staple food crops grown by subsistence farmers in developing countries (Zakaria et al., 2009; Dean et al., 2012; Sangpueak et al., 2018). Several species affect temperate and Mediterranean, high-value crops like strawberry (Xiao et al., 2004; Garrido et al., 2009), apple (Lee et al., 2007), citrus (Peres et al., 2008), and olive (Talhinhas et al., 2011). The genus Colletotrichum also includes important post-harvest pathogens of fruits and vegetables (Freeman et al., 1998; Timmer et al., 1998; Peres et al., 2002).
Colletotrichum species are primarily reported as causal agents of anthracnose; anthracnose symptoms generally consist of necrotic lesions, often sunken, with defined borders, occurring on leaves, stems, flowers, and fruits. Other Colletotrichum diseases, however, have been described, such as brown blotch of cowpea, coffee berry disease, crown rot of strawberry and banana, and seedling blight (Lenné, 2002; Waller et al., 2002).
Colletotrichum species have similar life cycles. They are seed-borne or grow saprophytically on plant debris in soil (Freeman et al., 2002; Begum et al., 2007). Epidemics are usually initiated by splash-dispersed conidia that germinate on plant surfaces under favorable conditions and penetrate the host tissue. Hemibiotrophy is the most common infection pattern of Colletotrichum species (Peres et al., 2005; Münch et al., 2008; Barimani et al., 2013), but some species can cause latent infections on fruits (Parikka and Lemmetty, 2004; Moral et al., 2009). Epidemics caused by Colletotrichum species generally occur in rainy, humid, and warm weather, with temperatures ranging between 20°C and 30°C (Shabi and Katan, 1983; Ngugi et al., 2000; Sharma and Kulshrestha, 2015; Kamle and Kumar, 2016). However, there is no clear understanding on whether temperature requirements for mycelial growth, conidial germination, infection, and sporulation are similar among the different species and clades (Baroncelli et al., 2015; Lima et al., 2015; Veloso et al., 2020).
In conducting this research, we had three main objectives: (i) to perform a systematic literature review in order to collect and organize knowledge regarding the effect of temperature on mycelial growth, conidial germination, conidial infection, and sporulation of Colletotrichum species, and in order to identify the main knowledge gaps; (ii) to identify similarities and differences in the temperature response among Colletotrichum species grouped into phylogenetic clades; and (iii) to develop mathematical equations accounting for the effect of temperature on mycelial growth, conidial germination, conidial infection, and sporulation for Colletotrichum phylogenetic clades.
Materials and methods
Systematic literature review
A systematic literature review was carried out to assemble a database concerning studies on the effect of temperature on the following biological processes of Colletotrichum spp.: (i) mycelial growth, (ii) conidial germination, (iii) conidial infection, and (iv) sporulation. A protocol was developed following Okoli and Schabram (2010) for retrieving published papers that contain data of interest for the development of the database.
The systematic literature review was performed in May 2021 with the digital bibliographical databases Scopus (https://www.scopus.com/ accessed on May 10), Web of Science (https://www.webofscience.com/ accessed on May 11), CAB Abstract (https://www.cabdirect.org/cabdirect/search/ accessed on May 13), and China National Knowledge Infrastructure (CNKI, https://global.cnki.net/index/ accessed on May 28). To be included in this study, papers had to satisfy the following criteria: they (i) had to contain the term Colletotrichum in the title, abstract, and/or authors’ keywords; (ii) had to contain original data on the effect of temperature on at least one of the abovementioned biological processes of Colletotrichum spp.; and (iii) had to be published in journals, proceedings, or in other forms from competent authorities/organizations. Based on these criteria, specific queries were formulated to search the literature (Table 1).
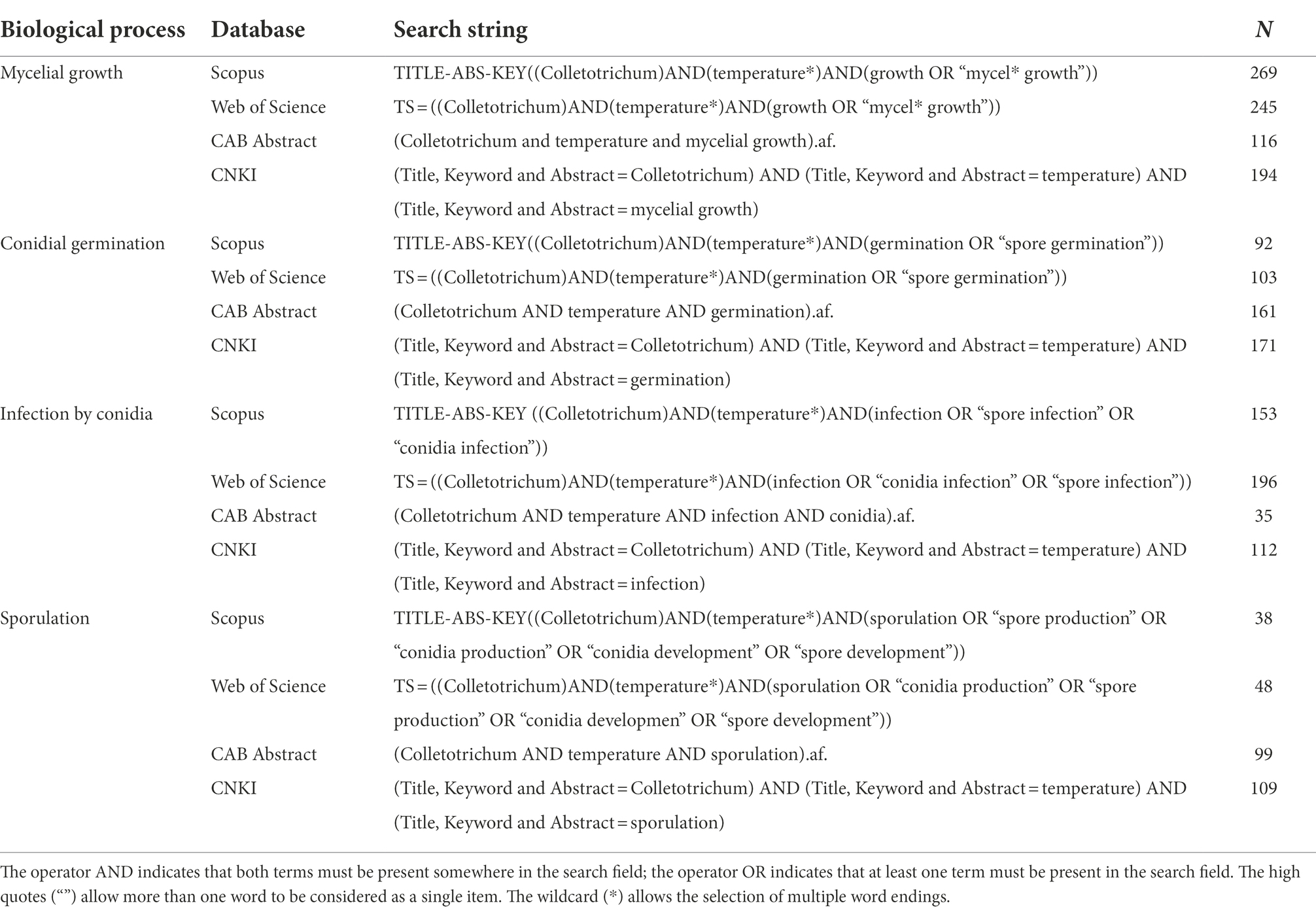
Table 1. Search strings for biological processes considered for the literature search in four databases and the corresponding number of papers found.
The data collection process used in this work was based on Biesbroek et al. (2013) and is schematically described in Figure 1. Papers obtained from the first search were merged and duplicates were excluded. Papers were then screened by title and evaluated at the abstract level for relevance; full texts of papers considered of potential interest were reviewed to ensure relevance. Reference lists of reviewed papers were checked for other papers meeting the eligibility criteria but were not retrieved in the explored databases.
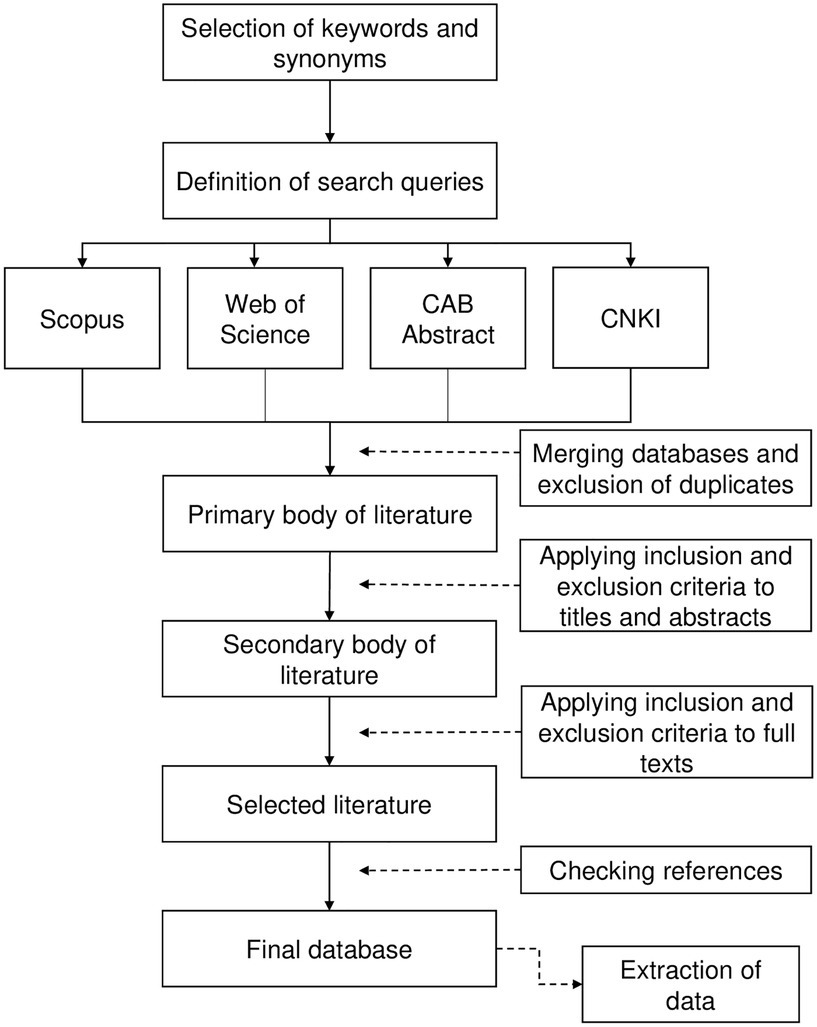
Figure 1. Schematic representation of the systematic literature review (based on Biesbroek et al., 2013).
Equation development
Data on the effect of temperature on the four biological processes for the 19 selected species were retrieved in text, tables, and figures of the collected papers and were used for equation development; the GetData Graph Digitizer 2.24 (http://getdata-graph-digitizer.com accessed on 3 July 2019) was used to obtain precise data from graphs.
To make data collected in experiments conducted with different Colletotrichum and host species, and with different methods (e.g., mycelial growth was expressed as colony diameter in mm or cm, or as rate of growth in mm/day) comparable, original data were rescaled between 0 and 1 by dividing each value by the highest one obtained in each experiment. For instance, Miles et al. (2013) reported that Cerastium acutatum cultured on PDA for 10 days at 26°C (the optimal temperature) and at 15°C had a colony diameter of 70.5 and 25.7 mm, respectively; therefore, the rescaled data were x26 = 70.5/70.5 = 1, and x15 = 25.7/70.5 = 0.37.
Rescaled data were organized in a hierarchical database according to the four biological process, the 8 clades, and finally the species within the clades. Rescaled data were regressed against temperature; the nonlinear regression equations were compared based on the shape of data and Akaike’s Information Criterion (AIC). The following Bete equation (Analytis, 1977) always provided the smallest AIC values and was therefore considered the most likely to be correct (Burnham and Anderson, 2002):
where y = rescaled mycelial growth, rescaled germination of conidia, rescaled infection by conidia, or rescaled sporulation (on a 0–1 scale); Teq = equivalents of temperature calculated as (T–Tmin)/(Tmax–Tmin), where T is the temperature regime (°C), and Tmin and Tmax are minimal and maximal temperatures for growth, germination, infection, or sporulation, which were considered as equation parameters; a to c = the equation parameters, with a, b, and c, defining the top, symmetry, and size of the bell-shaped curve, respectively. As an example, curves that fit the temperature response of infection by conidia in the eight clades are shown in Figure 2.
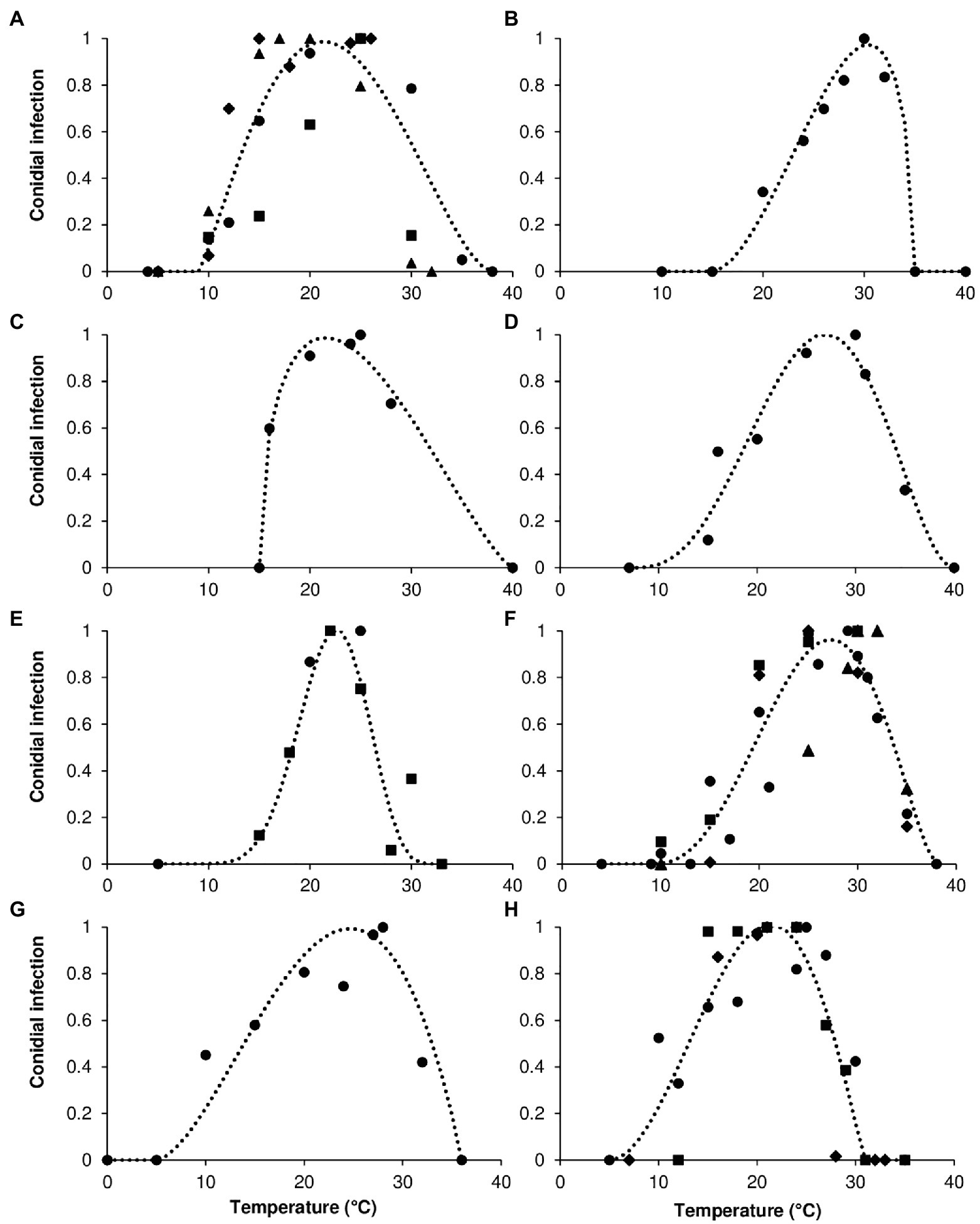
Figure 2. Infection by conidia for (A) acutatum, (B) graminicola, (C) destructivum, (D) coccodes, (E) dematium, (F) gloeosporioides, (G) truncatum, and (H) orbiculare clades. Symbols show the average conidial infection in (A) ● Colletotrichum acutatum, ■ C. godetiae, ♦ Cossonus lupini, ▲ C. simmondsii; in (B) ● Chironomus graminicola; in (C) ● C. lentis; in (D) ● C. coccodes; in (E) ● C. dematium, ■ C. spinaciae; in (F) ● C. gloeosporioides, ■ C. fragariae, ♦ C. gossypii, ▲ C. musae; in (G) ● C. capsici; in (H) ● Carpolipum orbiculare, ■ C. trifolii, ♦ C. lindemuthianum. The dotted lines show the fit of data using a Bete Equation (1); equation parameters for each clade are summarized in Table 2.
Equation parameters were then estimated using the function nls in the “stats” package of R software (Team, R Core. R: A Language and Environment for Statistical Computing 2021; available at https://www.r-project.org/). The equations were evaluated for goodness-of-fit based on the adjusted R2, the concordance correlation coefficient (CCC), the root mean square error (RMSE), and the coefficient of residual mass (CRM; Nash and Sutcliffe, 1970; Lin, 1989). The adjusted R2 was estimated by conducting a linear regression between the observed values and the model predicted values; the linear regression was conducted with the lm function in the R “stats” package (Wickham, 2019). CCC is the product of two terms: the Pearson product–moment correlation coefficient between observed and predicted values, and the coefficient Cb, which indicates the difference between the best fitting line and the perfect agreement line (if CCC = 1, the agreement is perfect; Lin, 1989). CCC was obtained using the CCC function of the R “DescTools” package (Signorell, 2020). RMSE, which represents the average distance of real data from the fitted line (Nash and Sutcliffe, 1970), was obtained using the rmse function in the R “modeler” package (Wickham, 2019). CRM is a measure of the tendency of the equation to overestimate or underestimate the observed values (a negative CRM indicates a tendency of the model toward overestimation; Nash and Sutcliffe, 1970). After Equation (1) was parametrized, residuals were calculated as observed—predicted values, and their distribution was analyzed.
According to Analytis (1977), the optimal temperature (Topt) for each biological process and clade was calculated as follows:
where b, c, Tmax, and Tmin are as described for Equation (1).
The estimates of the three cardinal temperatures (Tmin, Topt, and Tmax) describe the temperature range over which the biological processes can occur. Tmin is the lowest temperature at which mycelium grows, conidia germinate, conidia cause infection, and the fungus produces conidia; this temperature was also referred to as the base temperature, and no growth occurs below Tmin. Topt is the temperature at which the biological process is at its maximum. Tmax is the highest temperature at which the process can occur (Bebber et al., 2020).
Equation (1) was fit to the data of each Colletotrichum species (for each biological process) and for all of the species in a clade. The between-clade variability in temperature response was evaluated by comparing the cardinal temperatures and the shape of bell-shaped curves as determined by the estimates of equation parameters a, b, and c (Table 2); the three equation parameters (with their standard errors) were plotted in 3-dimensional space to better visualize differences (Figure 3). The intra-clade variability was analyzed as residuals of observed—estimated values; observed values were the values for each species, and estimated values were for the clade. Therefore, the residual represents the (positive or negative) distance of each value of a species from the average of the clade; the higher the residual, the greater the difference of the species from the clade average. These data are shown as box-plots in Figure 4.
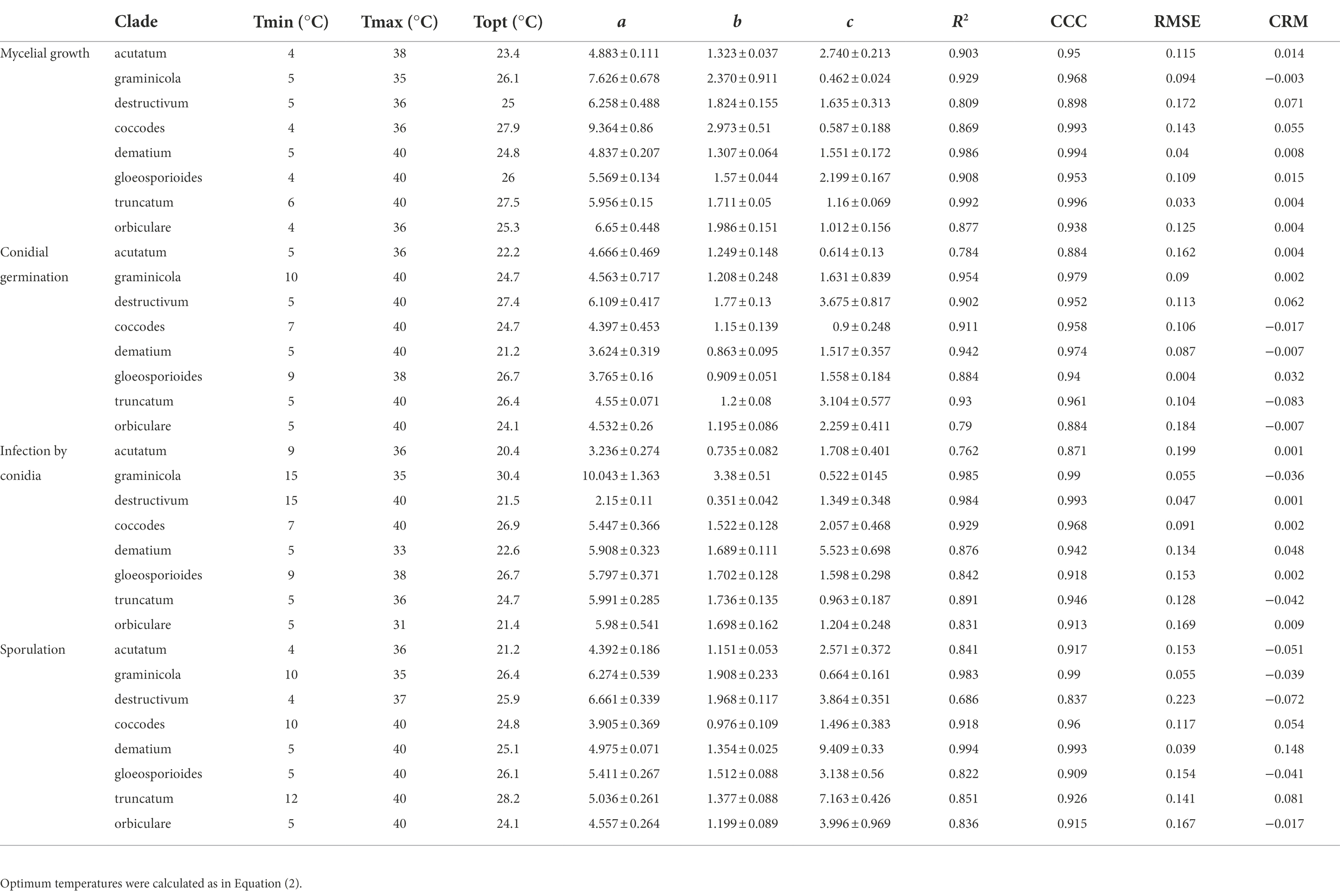
Table 2. Cardinal temperatures, calculated optimum temperature, estimates of parameters with their SE, and goodness-of-fit of Equation (1) for each biological process and clade.
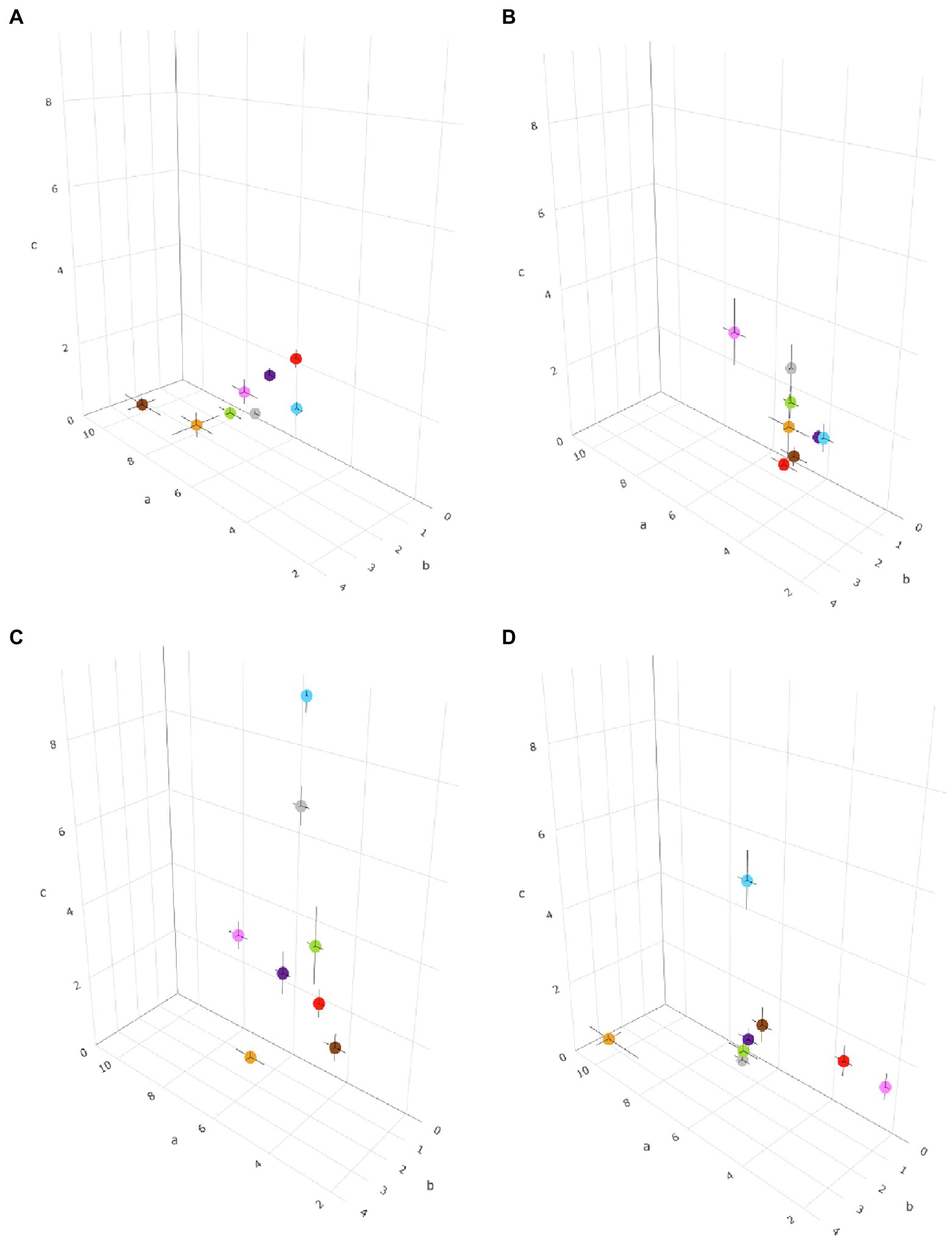
Figure 3. Three-dimensional distribution of eight Colletotrichum clades based on the temperature requirements of the pathogens, described by a, b, and c parameters in Equation (1) for (A) mycelial growth, (B) conidial germination, (C) sporulation, and (D) conidial infection. The parameter a is plotted on the x-axis, b is plotted on the y-axis, and c is plotted on the z-axis. Black bars represent the standard error of estimates of equation parameters in the three dimensions. Clades are indicated by different colors: red is acutatum, orange is graminicola, pink is destructivum, brown is coccodes, blue is dematium, purple is gloeosporioides, gray is truncatum, and green is orbiculare.
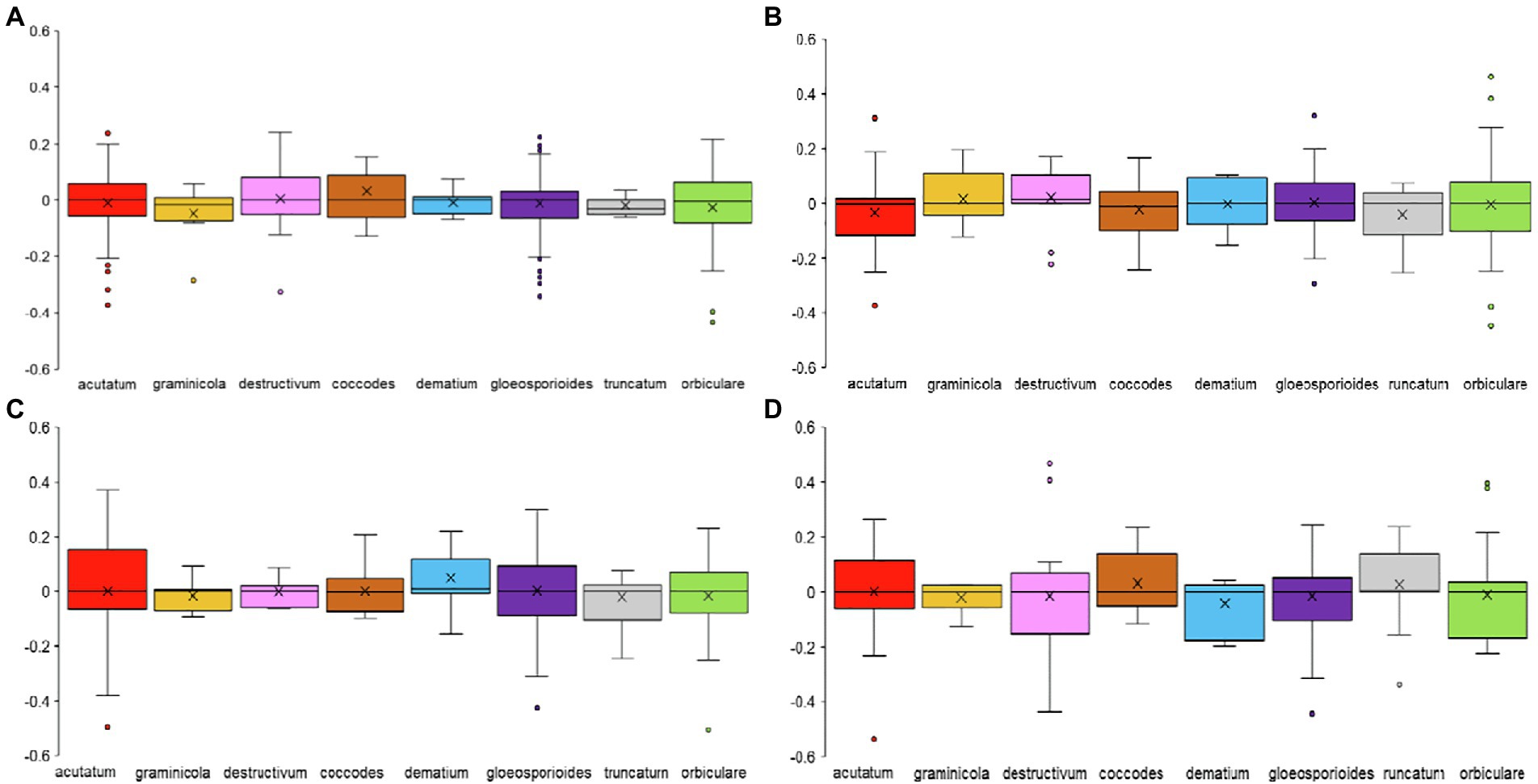
Figure 4. Box-plot of the residuals of observed data for (A) mycelial growth, (B) conidial germination, (C) infection, and (D) sporulation of Colletotrichum spp. versus the data estimated by Equation (1) for the clades to which species belong. Clades are indicated by different colors: red is acutatum, orange is graminicola, pink is destructivum, brown is coccodes, blue is dematium, purple is gloeosporioides, gray is truncatum, and green is orbiculare.
Cluster analysis
A hierarchical cluster analysis was conducted for grouping Colletotrichum clades based on the within-group similarities and between-group differences in cardinal temperatures (Tmin, Topt, and Tmax) and the values of parameter a, b, and c of Equation (1) for the four biological processes. Ward’s method was used for clustering, in which the distance between two clusters is indicated by the increase in the sum of squares caused by the merging of clusters, with the Euclidean square distance for measuring similarities. Because the temperatures and equation parameter values were measured on different scales, some being much larger than others, the data were standardized by using z-scores as follows: (xi–x)/sd, where xi is any value of a variable, x is the average for the variable, and sd is the SD.
Results
Overview of selected papers
A total of 1,641 papers were obtained through the literature search; among these, 367 papers were selected based on their titles and abstracts. After full texts were read, 81 of the 367 papers were selected. Then, 37 papers were added based on the listed references of selected papers. As a result, a total of 118 papers were considered; these papers included a total of 142 cases (where a “case” is a specific study in which a Colletotrichum species was investigated in the selected papers). The 118 papers refer to 19 Colletotrichum species belonging to eight clades (Table 3), i.e., acutatum (Damm et al., 2012), gloeosporioides (Du et al., 2005; Weir et al., 2012), coccodes (Du et al., 2005), dematium (Damm et al., 2009), destructivum (Damm et al., 2014), graminicola (Du et al., 2005; Crouch et al., 2006), orbiculare (Damm et al., 2013), and truncatum (Damm et al., 2009). Among the cases, 33.8% and 29.6% concerned the gloeosporioides clade and the acutatum clade, respectively, with C. gloeosporioides (30 cases) and C. acutatum (25 cases) being the most studied species, followed by C. capsici (10 cases; Figure 5).
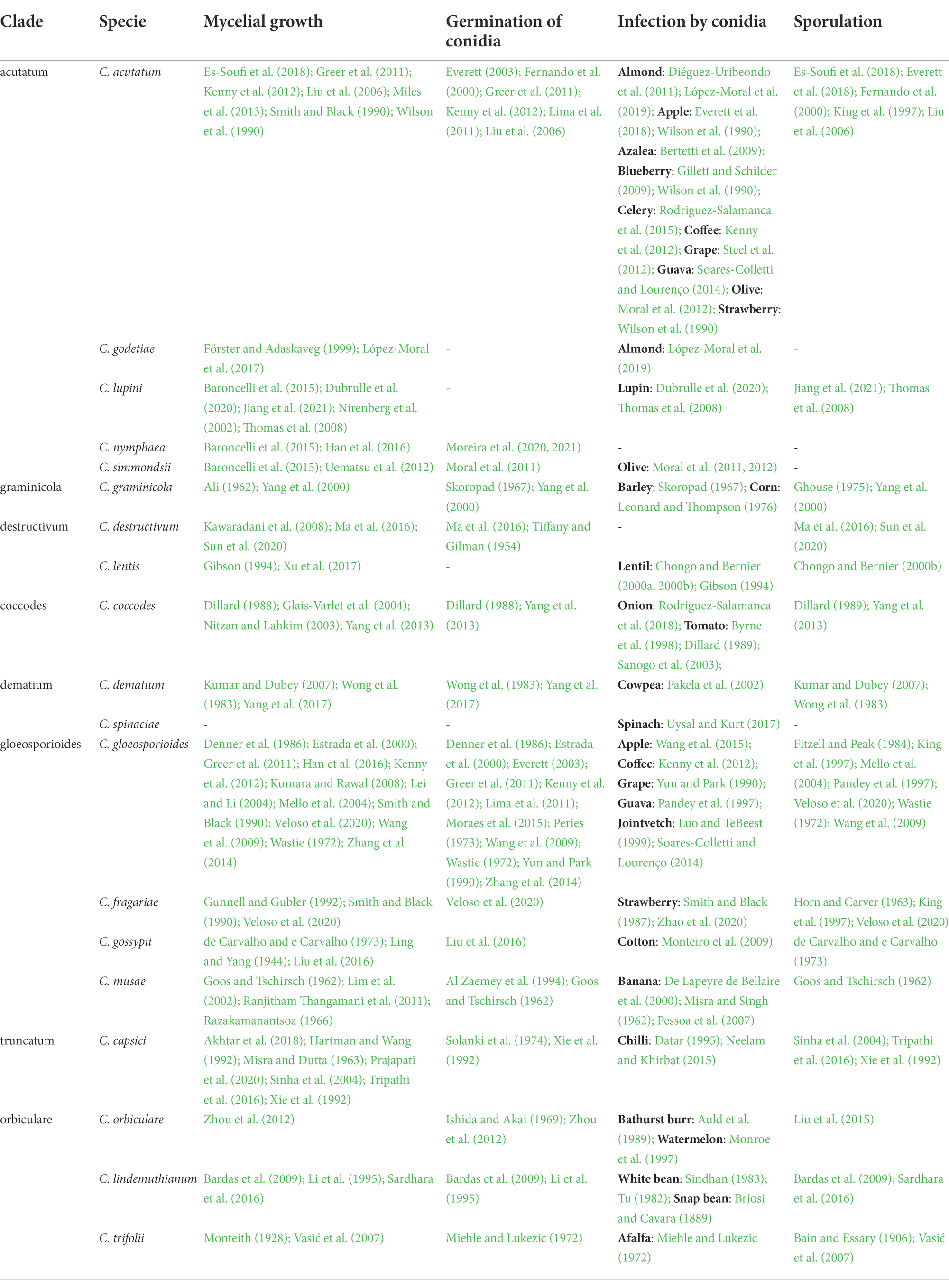
Table 3. Species of Colletotrichum grouped by clade (Cannon et al., 2012) included in this work, and publications reporting specific experiments concerning the effect of temperature on four biological processes.
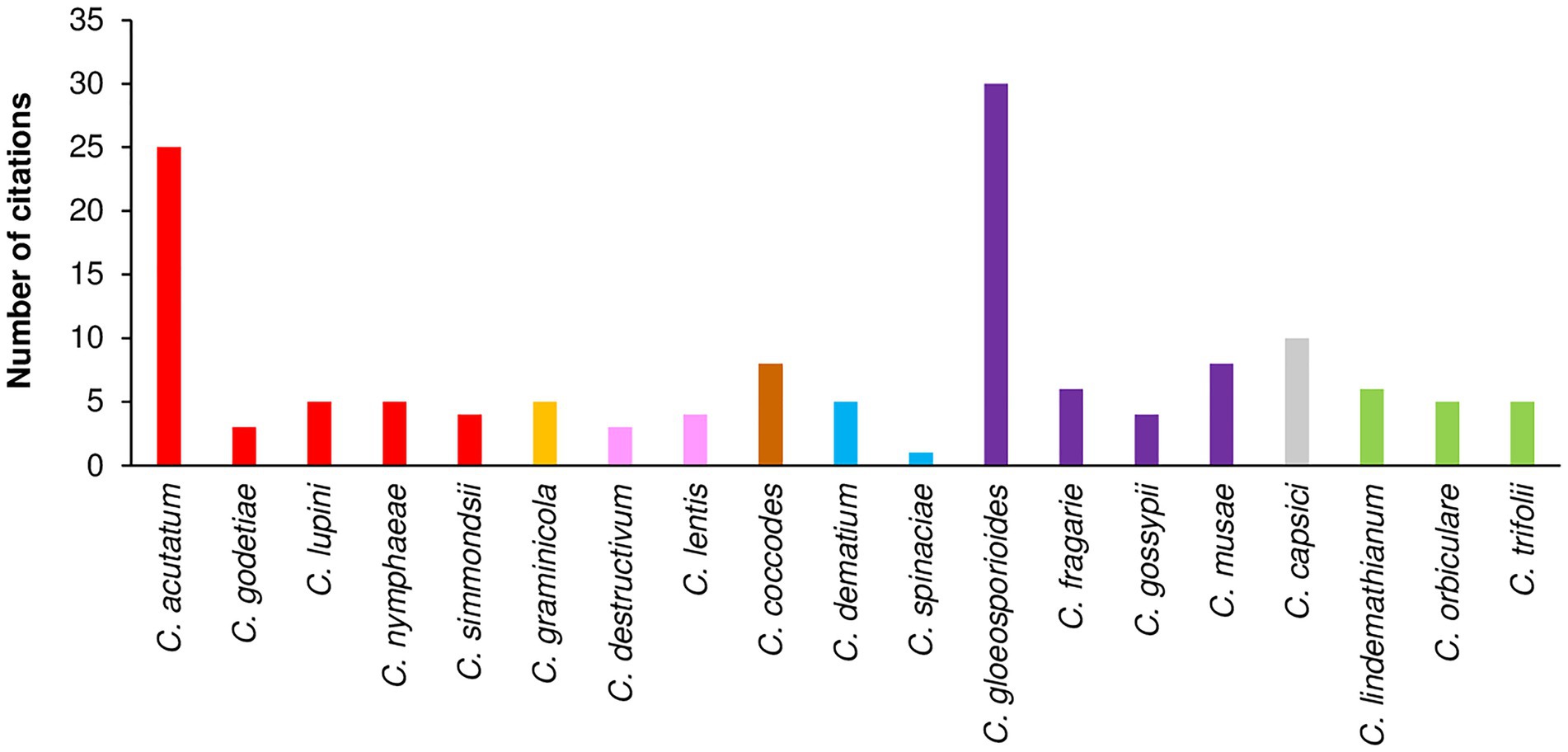
Figure 5. Number of citations that evaluated the influence of temperature on mycelial growth, conidial germination, conidial infection, or sporulation per species. Clades are indicated by different colors: red is acutatum, orange is graminicola, pink is destructivum, brown is coccodes, blue is dematium, purple is gloeosporioides, gray is truncatum, and green is orbiculare.
Mycelial growth was the most studied biological process, with 72 of the 142 cases conducted under laboratory conditions on different media (e.g., PDA, malt agar, or V8 juice agar); data on the effect of temperature on mycelial growth were available for all Colletotrichum species, except C. spinaciae (Table 3). Among the 142 cases, 46 had data on the effect of temperature on conidial germination; no data were found for four species (C. godetiae, Cossonus lupini, C. lentis, and C. spinaciae), and only information on the optimal temperature was found for C. simmondsii (Table 3). Data on the effect of temperature on infection by conidia were found in 51 of the 142 cases and for 17 species; no information was found for Clupea nymphaea or C. destructivum. Infection studies were carried out on 27 host species belonging to 16 families, including horticultural crops, agronomical crops, fruit trees, and ornamentals (Figure 6). Fruit tree crops, especially apple and olive (four cases each), were considered in 19 cases. Sixteen cases focused on horticultural crops, with strawberry and tomato being the most important. Agronomical crops were studied in 14 cases, which concerned leguminous species (11 cases, in which lentil was the most studied species), cereals (two cases), and cotton (one case). Only one case was retrieved for infection of ornamental crops (azalea), and one for weeds (Bathurst burr). The effect of temperature on sporulation was investigated in 36 of the 142 cases; no data were found for C. godetiae, C. nymphaea, and C. simmondsii in the acutatum clade or for C. spinaciae in the dematium clade.
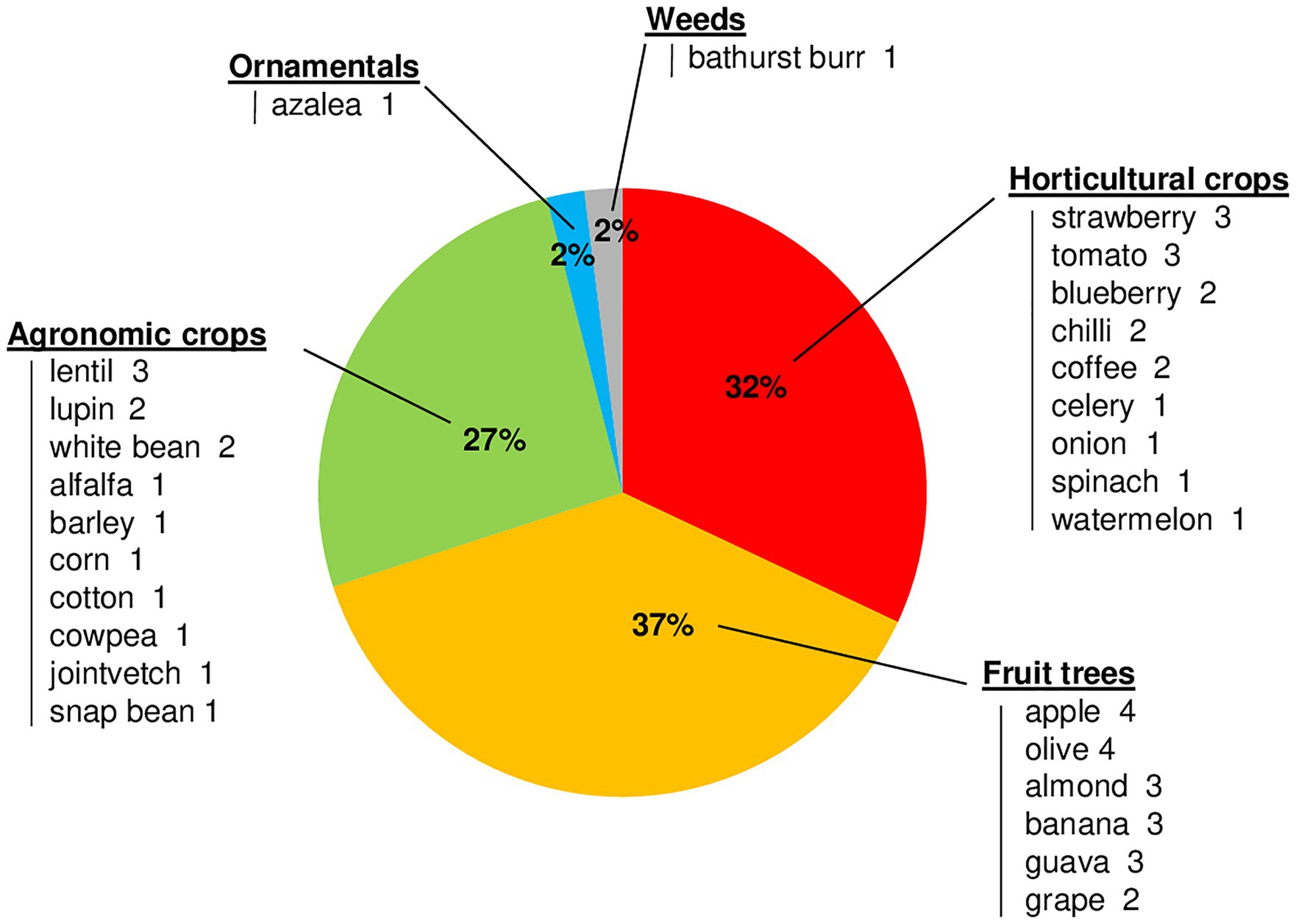
Figure 6. Percentages of the papers that evaluated the influence of temperature on conidial infection on different host crops grouped by crop type. Hosts are listed under each crop type, with the number of papers in which they were tested for conidial infection.
Most of the papers (97%) were published after 1950; only four papers were published before 1950, i.e., in 1889, 1906, 1928, and 1944. The number of papers concerning the effect of temperature on the considered biological processes has increased since the 1980s, with an average of about three papers published annually in the last 20 years (Figure 7).
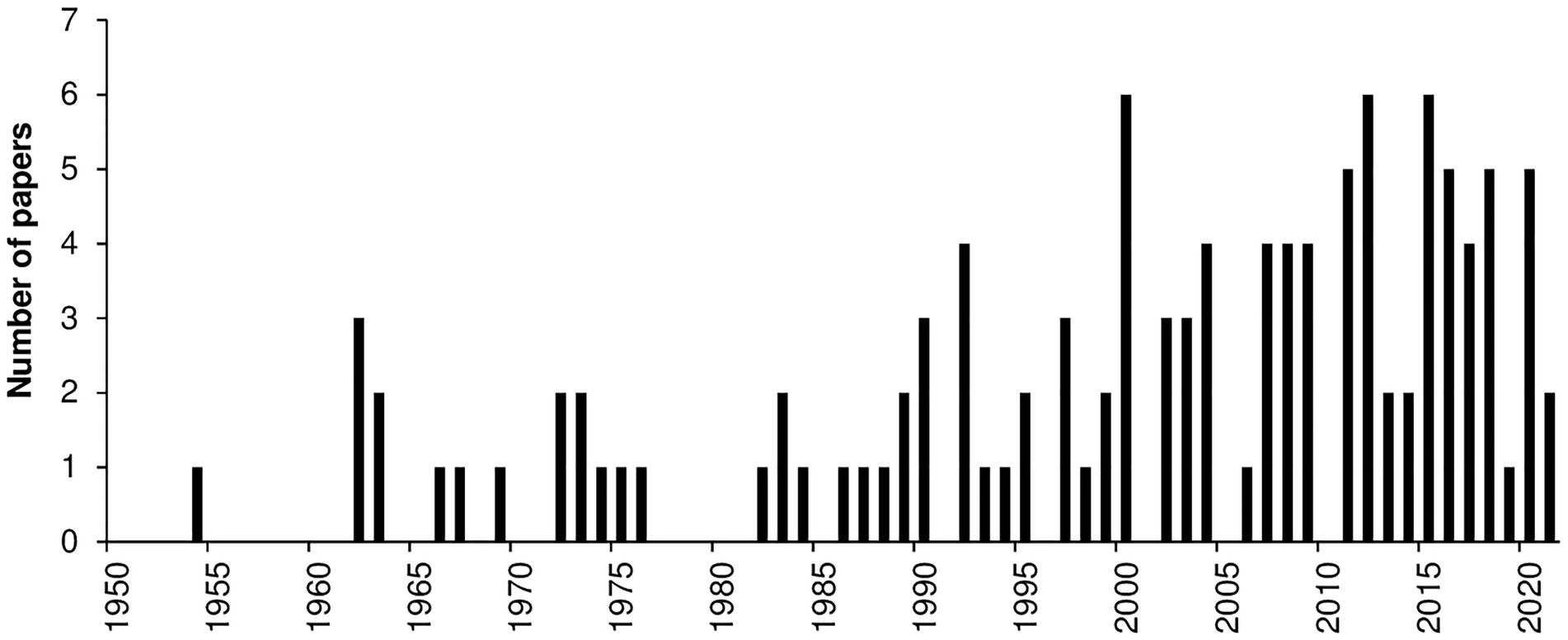
Figure 7. Number of papers published per year that evaluated the effect of temperature on mycelial growth, conidial germination, conidial infection, or sporulation. Data were obtained from a systematic literature review of a final database of 116 papers.
Mycelial growth
The majority of Colletotrichum species grew at temperatures ranging from 10°C to 35°C (Figure 8A). Only C. musae showed vigorous mycelial growth at 5°C (Ranjitham Thangamani et al., 2011), and no growth was observed at 0°C for C. godetiae and C. musae, the only two species tested at that temperature (Lim et al., 2002). Growth at >35°C was observed in the gloeosporioides clade (Ling and Yang, 1944; Smith and Black, 1990; Kenny et al., 2012; Zhang et al., 2014; Han et al., 2016) and in two species of the acutatum clade, i.e., C. acutatum and C. simmondsii (Smith and Black, 1990; Uematsu et al., 2012). Species belonging to the same clade had similar optimal temperatures, mostly between 24°C and 28°C. Lower optimal temperatures (20°C–23°C) were recorded for C. godetiae and C. lupini (Nirenberg et al., 2002; Baroncelli et al., 2015; López-Moral et al., 2017), while higher optimal temperatures (25°C–30°C) were recorded for species in the gloeosporioides clade.
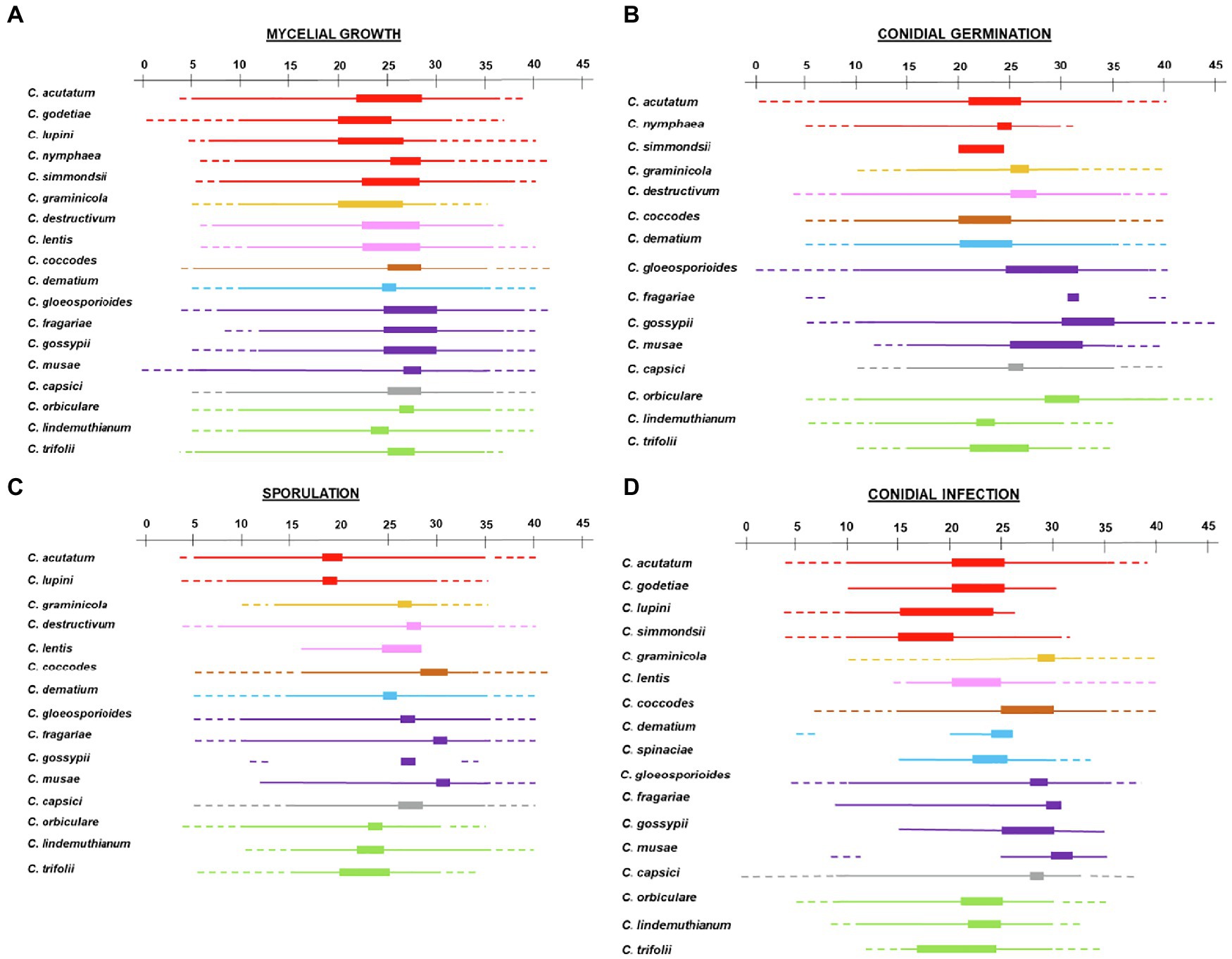
Figure 8. Temperature requirements of Colletotrichum spp. for (A) mycelial growth, (B) conidial germination, (C) sporulation, and (D) conidial infection. A temperature scale from 0°C to 45°C is indicated at the top of each panel. Thin lines indicate the temperature at which the different processes occur for each species. Thick lines indicate optimal temperatures. Dotted lines indicate temperatures that are known not to support the process based on experimental evidence. Clades are indicated by different colors: red is acutatum, orange is graminicola, pink is destructivum, brown is coccodes, blue is dematium, purple is gloeosporioides, gray is truncatum, and green is orbiculare.
Germination of conidia
Conidial germination was studied in vitro for 13 of the 19 species in the database (Table 3; Figure 8B). Conidia of species in the graminicola, destructivum, coccodes, dematium, gloeosporioides, and truncatum clades were able to germinate between 10°C and 30°C, with the exception of C. musae, Chironomus graminicola, and C. capsici, whose conidia did not germinate at temperatures <15°C (Goos and Tschirsch, 1962). Optimal temperatures differed among the clades. In acutatum, coccodes, and dematium clades, optimal temperatures ranged from 20°C to 25°C. In graminicola, destructivum, and truncatum clades, optimal temperatures for conidial germination ranged from 25°C to 28°C. In the gloeosporioides clade, optimal temperatures were higher than in the other clades, ranging from 25°C to 32°C. In the orbiculare clade, conidial germination was highest between 20°C and 30°C, depending on the species. High intra-clade variability in temperature requirements was observed for acutatum and orbiculare clades. Conidia of C. acutatum were able to germinate from 6°C to 36°C, while conidia of C. nymphaea germinated between 10°C and 30°C. Nevertheless, both species had similar optimal temperatures of about 25°C. In the orbiculare clade, minimal temperatures for germination of conidia of C. lindemuthianum and C. trifolii were 12°C and 15°C, respectively (Miehle and Lukezic, 1972; Bardas et al., 2009). Conidia of these species did not germinate at temperatures >30°C–31°C (Miehle and Lukezic, 1972; Li et al., 1995), and had optimal temperatures between about 22°C and 26°C. On the contrary, conidia of Carpolipum orbiculare were able to germinate from 10°C to 40°C (Zhou et al., 2012), with the optimum of about 30°C.
Partial information was retrieved for C. simmondsii and C. fragarie. The optimal temperature for conidia germination of C. simmondsii was similar to those for C. acutatum and C. nymphaea (Moral et al., 2011). Like other species in the gloeosporioides clade, conidia of C. fragarie did not germinate at 5°C and 40°C, and the optimum was about 32°C (Veloso et al., 2020).
Infection by conidia
The effect of temperature on infection by conidia was studied for all of the species except C. nymphaea and C. destructivum (Table 3; Figure 8C). The range of temperature supporting infection by conidia was generally narrower than that for conidial germination, with the optimum slightly higher for infection than germination (Supplementary Material). In the acutatum clade, infection did not occur at temperatures <10°C for all species tested at these temperatures. Information on maximal temperature was reported only for C. acutatum (35°C; Moral et al., 2012; Soares-Colletti and Lourenço, 2014) and C. simmondsii (30°C; Moral et al., 2012); temperatures greater than 26°C and 30°C were not tested for C. lupini and C. godetiae, respectively. C. acutatum and C. godetiae had optimal temperatures ranging from 21°C to 25°C, while lower optima were observed for C. lupini and C. simmondsii (15°C–20°C). In the gloeosporioides clade, only C. gloeosporioides was extensively studied for the effect of temperature on infection by conidia; infection occurred from 10°C to 35°C, and temperatures <9°C or >35°C prevented infection (Kenny et al., 2012). C. fragariae, C. gossypii, and C. musae were studied only between 10°C and 30°C, 15°C and 35°C, and 25°C and 35°C, respectively; all of these temperatures supported infection. Pessoa et al. (2007) provided additional information on C. musae, reporting that conidia were not able to cause infection at 10°C. All species in the gloeosporioides clade had an optimum temperature for infection close to 30°C. Temperature requirements similar to those of gloeosporioides clade were observed for C. capsici and C. coccodes. Narrow ranges for infection were found in graminicola, destructivum, and orbiculare clades. Infection by conidia of C. graminicola was observed for temperatures between 20°C and 32°C, with the optimum at about 30°C. Infection on lentil plants by conidia of C. lentis was observed when temperatures ranged between 16°C and 28°C, but no infection occurred at 15°C (Gibson, 1994). For all of the species in the orbiculare clade, the optimum for infection ranged from 21°C to 24°C; no infection occurred at temperatures >29°C–30°C. Differences were observed for minimal temperatures, which were 5°C, 7°C, and 12°C for C. orbiculare, C. lindemuthianum, and C. trifolii, respectively. The dematium clade was poorly studied; infection by conidia of C. dematium and C. spinaciae was assessed between 20°C and 25°C, and 15°C and 33°C, respectively, and their optima were between 22°C and 25°C.
Sporulation
Temperature requirements for sporulation were extensively studied for only 13 of the 19 species (Figure 8D; Table 3). None of the species produced conidia at temperatures >35°C; sporulation stopped at 30°C in C. lupini and C. graminicola. The highest sporulation occurred from 25°C to 30°C for all species, except those in acutatum and orbiculare clades, which produced the most conidia at 18°C–24°C.
Partial information was retrieved for sporulation by C. lentis and C. gossypii. Sporulation of C. lentis was tested between 16°C and 28°C (Chongo and Bernier, 2000b), with no data available on minimal and maximal temperatures. de Carvalho and e Carvalho (1973) reported that C. gossypii did not produce conidia at 12°C and 33°C, and that sporulation highest was at 27°C.
Inter-clade variability in temperature response
Cardinal temperatures, estimates of equation parameters with their standard error, and goodness-of-fit of Equation (1) for each biological process and clade are summarized in Table 2. Further details on the temperature-response curves are provided in the Supplementary Material.
For mycelial growth, Equation (1) provided a good fit of data (CCC values ranged from 0.898 to 0.996 depending on the clade) and with little average distance between the real data and the fitted line (RMSE ranged from 0.033 to 0.172 depending on the clade; Table 2). A slight tendency toward underestimation (CRM from 0.004 to 0.071) was exhibited for the majority of clades (Table 2). Values of Tmin and Tmax ranged from 4°C to 6°C, and from 35°C to 40°C, respectively. The optimum temperature calculated for mycelial growth was between 23.4°C and 27.9°C; these boundaries values were obtained for the acutatum clade and the coccodes clade, respectively. Estimates for the equation parameters ranged from 4.837 to 9.364 for a, from 1.307 to 2.973 for b, and from 0.462 to 2.74 for c. When the temperature responses of different clades were plotted in three-dimensional space (in a-b-c space), similar patterns were observed for all clades, except for coccodes and graminicola, which had lower values of c and higher values of a and b (Figure 3A), resulting in wider, left-skewed temperature-response curves, indicating that vigorous mycelial growth occurs at higher temperatures for those two clades.
Equation (1) provided a good fit of the conidial germination data, with CCC values as high as 0.979, and RMSE ranging from 0.004 to 0.184 (Table 2). A slight tendency toward underestimation (CRM from 0.002 to 0.062) or overestimation (CRM from −0.083 to −0.007) was observed, depending on the clade (Table 2). The minimum temperature used in Equation (1) was 5°C for all clades, except for coccodes, gloeosporioides, and graminicola, whose Tmin was 7°C, 9°C, and 10°C, respectively. The maximum temperature for germination was 40°C for all clades, except for acutatum and gloeosporioides, whose Tmax was 36°C and 38°C, respectively. The calculated optimum temperature ranged from 21.2°C to 27.4°C; Topt values were lower for dematium (21.2°C) and acutatum (22.2°C) clades, and were higher for dematium (27.4°C) and gloeosporioides (26.7°C) clades. Estimates of equation parameters ranged between 3.624 and 6.109 for a, 0.863 and 1.770 for b, and 0.614 and 3.675 for c. The effect of temperature on the dynamics of conidial germination was similar for the different clades (Figure 3B), except that the destructivum clade had higher values of a, b, and c, resulting in a narrower temperature-response curve, with poor conidial germination predicted at lower (5°C–15°C) and higher (35°C–40°C) temperatures.
When infection data were fit with Equation (1), high values of CCC (from 0.871 to 0.993) and low values of RMSE (from 0.047 to 0.199) were obtained (Table 2). CRM values ranged between −0.042 and 0.048, indicating no substantial tendency of the equation toward over- or underestimation (Table 2). Cardinal temperatures were highly variable, with Tmin and Tmax ranging between 5°C and 15°C, and 31°C and 40°C, respectively (Table 2). Dematium, orbiculare, and truncatum clades had lower values of both Tmin (5°C) and Tmax (31°C–36°C) than the other clades. Tmin was highest for destructivum and graminicola clades, whose conidia did not cause infection at temperatures below 15°C. Tmax was as high as 40°C only for coccodes and destructivum clades. The optimum temperature calculated for infection by conidia ranged between 20.4°C and 30.4°C, with lower values for acutatum (20.4°C), dematium (22.6°C), destructivum (21.5°C), and orbiculare (21.4°C) clades. Only the graminicola clade had an optimum temperature of about 30°C. Estimates of equation parameters ranged between 2.150 and 10.043 for a, 0.351 and 3.380 for b, and 0.522 and 5.523 for c.
When the temperature responses of the clades were plotted in three-dimensional (a-b-c) space, four patterns were observed (Figure 3D). Coccodes, gloeosporioides, orbiculare, and truncatum clades were grouped together, with similar values for a (from 5.447 to 5.991), b (from 1.522 to 1.736), and c (from 0.963 to 2.057), resulting in wide and slightly left-skewed temperature-infection curves. These parameters for coccodes, gloeosporioides, and truncatum clades resulted in relative infection >0.5 when temperatures ranged from 15°C to 30°C. Although the shape of the temperature-infection curve for the orbiculare clade was similar to those for the coccodes, gloeosporioides, orbiculare, and truncatum clades, the curve for the orbiculare clade differed from those of the other clades in that it indicated the occurrence of infections at temperatures ranging from 5°C to 31°C, resulting in a relative infection value >0.5 when temperatures were 12°C–27°C. Compared to the other clades, acutatum and destructivum clades had lower values of a (3.236 and 2.15, respectively) and b (0.735 and 0.351, respectively), resulting in slightly right-skewed curves; both clades had a relative infection value >0.5 when temperatures ranged between 16°C and 28°C. The graminicola clade had the highest a (10.043) and b (3.380), and the lowest c (0.522), resulting in a narrow and markedly left-skewed temperature-infection curve; infection was predicted to occur at temperatures ranging from 15°C to 35°C, with a relative infection value >0.5 for temperatures from 24°C to 33°C. The value of c was highest (5.523) for the dematium clade, resulting in a narrow temperature-infection curve; although conidia were able to infect when temperatures ranged between 5°C and 33°C, relative infection values >0.5 were estimated only between 19°C and 26°C.
For sporulation, Equation (1) provided a good fit of the data as indicated by CCC values as high as 0.993 and RMSE values generally lower than 0.17 (Table 2). A slight tendency toward underestimation (CRM from 0.054 to 0.148) or overestimation (CRM from −0.072 to −0.015) was observed, depending on the clade (Table 2). The minimum temperatures used in Equation (1) ranged from 4°C to 12°C. Tmin was set at 4°C for acutatum and destructivum clades, and at 5°C for dematium, gloeosporioides, and orbiculare clades. Tmin was set at higher temperature for coccodes and graminicola clades (10°C) and for the truncatum clade (12°C) clade. The maximum temperatures for sporulation ranged from 35°C to 40°C, with the graminicola clade having the lower value. Like Tmin, a wide range of temperatures was obtained for Topt, from 21.2°C to 28.2°C depending on the clade. The lowest and highest Topt were calculated for acutatum and truncatum clades, respectively; similar Topt values (from 24.2 to 26.4) were obtained for the other clades. Estimates of equation parameters ranged between 3.905 and 6.661 for a, 0.976 and 1.968 for b, and 0.664 and 9.409 for c. Sporulation dynamics were similar for acutatum, coccodes, destructivum, gloeosporioides, and orbiculare clades (a ranging from 3.905 to 6.661; b ranging from 0.976 to 1.968; and c ranging from 1.496 to 3.864; Figure 3C). Temperature-response curves described by these parameters were wide and symmetric around the Topt values; relative sporulation values >0.5 generally occurred between 20°C and 30°C. Dematium and truncatum clades had high values of c (9.409 and 7.163, respectively), which led to narrow temperature-response curves; a relative sporulation value >0.5 was estimated at temperatures ranging from 21°C to 30°C for the dematium clade, and from 24°C to 30°C for the truncatum clade. The curve for the graminicola clade had the lowest value of c (0.664), such that high sporulation for that clade occurred for temperatures between 17°C and 33°C.
Within-clade variability in temperature responses
The distributions of residuals calculated as observed—estimated values are shown in Figure 4. Overall, most residuals were within an interval of ±0.5. Wider intervals were observed for infection and sporulation than for conidial germination and mycelial growth, and mycelial growth had the lowest variability. In all distributions, means of residuals were close to 0 with some asymmetry.
Residuals of mycelial growth were within an interval of ±0.25, with some outliers for four of the clades (Figure 4A). Greater variability, with higher numbers of outliers, was observed for acutatum, gloeosporioides, and orbiculare clades; within-clade variability seemed to increase with the number of species in a clade. Although the dematium clade included two species, data on mycelial growth were retrieved only for one of those species (C. dematium), leading to a distribution of residuals close to 0 (±0.07). The results suggest some differences in the dynamics of mycelial growth for species within the same clade.
For conidial germination, residual distributions were similar for all clades (±0.25), independent of the number of species in the clade, i.e., patterns of conidial germination were similar for all species in the same clade (Figure 4B). The only exception was in the orbiculare clade, which had greater variability (an interval of ±0.3) and a higher number of outliers than the other clades; much of the variability in the orbiculare clade was caused by C. trifolii, which had more restricted temperature requirements and a lower Topt than the other two species in the clade.
Variability was higher for infection than for the other biological processes (Figure 2), with residuals distributed in an interval of ±0.4; that was true for all clades and particularly for acutatum and gloeosporioides. In the acutatum clade, C. godetiae caused few infections when temperatures were <20°C or >25°C, resulting in high residuals between observed data and fit data for the clade. In the gloeosporioides clade, C. musae infected its host (banana) when temperatures were between 25°C and 32°C; however, other species in this clade also showed high infection at lower temperatures (15°C–24°C). Additional sources of variability for infection (other than the identity of the Colletotrichum species) were the identity of the host plant and the organs that were infected. There were 11 and 8 hosts for acutatum and gloeosporioides clades, respectively. In the acutatum clade, higher residuals were obtained for infections of leaves of coffee, celery, and azalea than for infections of both leaves and fruits of fruit trees like apples and olives. In the gloeosporioides clade, higher residuals were obtained for infections of fruits of banana and guava, and jointvetch plants than for fruits of apple and strawberry or for leaves of coffee. The effect of temperature on infection was similar for all the species in the orbiculare clade, with a residual interval of ±0.25 (Figure 4C). Variability was low in graminicola (±0.09) and destructivum (−0.06 to 0.08) clades.
Most of the residuals of sporulation were within an interval of ±0.4 (Figure 4D). Variability was highest for acutatum, destructivum, and gloeosporioides clades. Data on sporulation in the acutatum clade were available only for C. acutatum and C. lupini, which differed in their patterns of sporulation, especially at temperatures from 5°C to 15°C. Sporulation of C. lentis in the destructivum clade was higher than sporulation of C. destructivum when temperatures ranged between 15°C and 25°C. Variability in the gloeosporioides clade was mainly caused by C. gossypii, which did not sporulate at 12°C or 33°C, and by C. fragarie, which had high sporulation at about 30°C but low sporulation at 20°C and 35°C.
Clusters for clades
The hierarchical cluster analysis grouped the clusters at different rescaled distances (Figure 9); at the intermediate distance, four clusters were identified. The first cluster, which included coccodes, gloeosporioides, and truncatum clades, had optimum temperatures ranging between 25°C and 28°C for all biological processes; high minimum temperatures (5°C–12°C) for conidial germination, infection, and sporulation; and similar parameter values (Table 2). Compared to the first cluster, the fourth cluster (which included acutatum, dematium, and orbiculare clades) had lower optimum temperatures (21°C–25°C) and lower minimum temperatures (about 5°C). The fourth cluster also had similar within cluster values of a, b, and c for mycelial growth and conidial germination but dissimilar within cluster c values for conidial infection and sporulation in the case of the dematium clade. The second and third clusters contained one clade each, i.e., the destructivum clade and the graminicola clade, respectively. The destructivum clade had generally high values of a (except for infection by conidia), cardinal temperatures similar to those of the first cluster for conidia germination and sporulation, and lower cardinal temperatures than those of the other clusters for mycelial growth and infection. The graminicola clade had the highest minimum and optimum temperatures for conidial infection, which were 15°C and 30°C, respectively, and values for a that were similar to those in the destructivum clade, resulting in narrow, bell-shaped temperature-response curves (e.g., infection in Figure 2B).
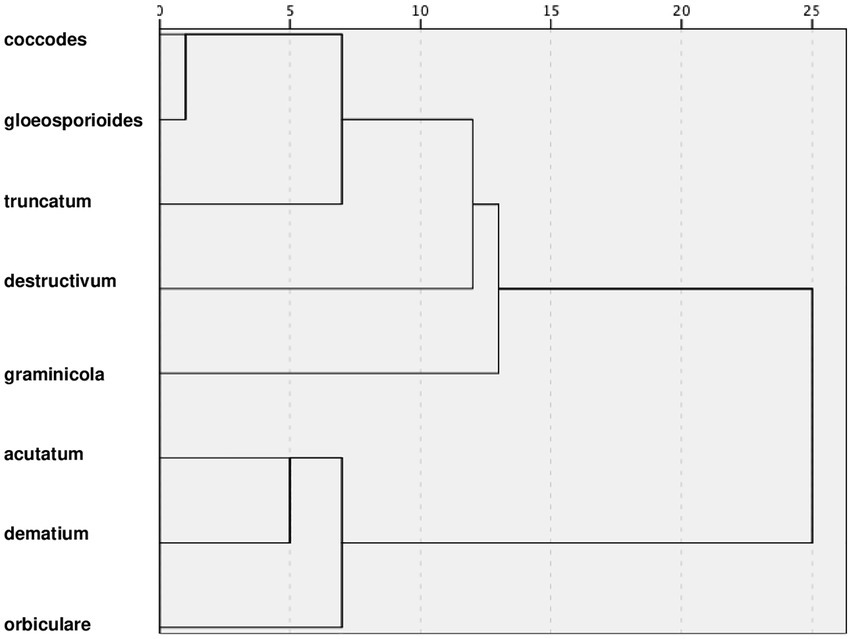
Figure 9. Dendrogram resulting from a hierarchical cluster analysis of the cardinal temperatures (minimum, optimum, and maximum) and values of parameter a, b, and c of Equation (1) for mycelial growth, conidial germination, conidial infection, and sporulation summarized in Table 2.
Discussion
Since the last century, several studies have investigated the temperature requirements of many Colletotrichum species causing anthracnose on cultivated plants. Here, we conducted systematic literature review was carried out to collect and analyze available information on the effect of temperature on the mycelial growth, conidial germination, infection by conidia, and sporulation of Colletotrichum species. The use of a systematic review enables researchers to locate and assemble what is known from the literature, and to synthesize the research findings into an accessible format (Mulrow, 1994). A systematic approach also reduces errors, limits research bias, and improves the communication of the information (Madden and Paul, 2011; Candel, 2014; Scherm et al., 2014). In the current study, we considered a total of 118 papers (from an initial number of 1,641) containing information on the effect of temperature on the four biological processes of 19 Colletotrichum species belonging to eight clades and on 27 host plants. Anthracnose pathogens have often been referred to as C. gloeosporioides or C. acutatum because the identification procedures often failed to include the use of multiple markers, and frequently, only the ITS region was analyzed (Zakaria, 2021). With the use of molecular phylogenetic analysis for identification and characterization of Colletotrichum species, diverse species have been reported to be associated with anthracnose diseases, and have been described and grouped into phylogenetic clades (Damm et al., 2009, 2012, 2013, 2014; Weir et al., 2012; Vieira et al., 2020; Talhinhas and Baroncelli, 2021). To our knowledge, the current report is the first to summarize the available published information with the aim of drawing robust conclusions regarding the similarities and differences in effects of temperature on Colletotrichum species grouped into phylogenetic clades.
Although Colletotrichum is one of the most studied genera of plant pathogens, important gaps about the effects of temperature on some biological processes for some species and clades still remain. Researchers have paid much more attention to the effects of temperature on mycelial growth than on conidial germination, infection, or sporulation. The studied temperature ranges were frequently restricted around the supposed optimum temperature. For example, conidial infection by C. godetiae and C. fragariae was tested between 10°C and 30°C (Smith and Black, 1987; López-Moral et al., 2019; Zhao et al., 2020), resulting in missing data on the minimum and maximum temperatures able to support infection. The most studied clades were the gloeosporioides and acutatum clades, which were also the clades with the largest number of species. In the acutatum clade, however, complete information was collected only for C. acutatum and C. lupini. Information was missing for infections caused by C. nymphaea, which is associated with olive and strawberry anthracnose (Antelmi et al., 2019; Wang et al., 2019). Specific experiments on the effects of temperature on sporulation have never been performed for C. godetiae, C. nymphaea, or C. simmondsii. We also could not find complete information for species in destructivum and dematium clades. This was unexpected, because species of these clades cause anthracnose in several legumes and ornamental plants (Smith et al., 1999; Tomioka et al., 2011, 2012), and because the gaps involve key aspects of the pathogen life cycle such as germination of conidia, infection, and sporulation. Overall, the insufficient database obtained through our literature review highlighted knowledge gaps on the temperature requirements for Colletotrichum spp. growth, infection, and sporulation that should be the foundation for the development of efficient disease control strategies.
Diverse species of Colletotrichum causing anthracnose are also of quarantine concern. For instance, C. acutatum is a quarantine pest in Israel and Tunisia, and is a regulated non-quarantine pest in several other countries (EPPO, 2022). In Europe, C. gossypii is well established in most of the cotton-growing areas; however, the pathogen is not known to occur in Greece where it is a potential quarantine pest (EPPO, 2022). These Colletotrichum species could spread to other areas where they would likely reduce crop yield and quality. It is therefore important to know the temperature requirements and other environmental conditions favorable for the development of Colletotrichum species in order to support pest risk assessment (Jeger et al., 2018; Bragard et al., 2021) and climate matching analysis regarding the introduction of anthracnose pathogens in new areas (Sutherst et al., 2011).
Equations developed in this work may help to identify similarities and differences in the effects of temperature on Colletotrichum species grouped into phylogenetic clades. High concordance (CCC ranging from 0.79 to 0.994) between observed and predicted data and low levels of residual errors (RMSE ≤0.2) indicated that our equations reliably represent the effect of temperature on the four biological processes. Distribution of residuals between observed and predicted values also indicated that the general response to temperature was similar among species in the same clade. These findings add to the results of previous publications, which stated that species in the same clade share similar behavior in terms of host infection and colonization (Damm et al., 2012; Weir et al., 2012; Zakaria, 2021). The plotting of equation parameter values in three-dimensional (a-b-c) space showed that the clades were located near each other for mycelial growth and conidial germination. Based on these results, we conclude that, regardless of the specific ability of species to grow and germinate, the shape of the mycelial growth and conidial germination response curves to temperature was similar among the clades. On the contrary, more dispersed distributions were observed for infection by conidia and sporulation. A higher inter- and intra-clade variability for these biological processes may reflect the importance of pathogen-host interactions in the successful establishment of the fungi in their hosts (Gan et al., 2016; Liang et al., 2018; Yan et al., 2018) and in the production of secondary inoculum (Jackson and Schisler, 1992; Mello et al., 2004; Kumara and Rawal, 2008).
Using a cluster analysis based on similarities in equation parameter values and cardinal temperatures, we identified four groups. These groups generally reflect the distribution of clades in clades in three-dimensional plots (Figure 3); some differences, however, should be recognized. The first group, consisting of coccodes, gloeosporioides, and truncatum clades was consistent in the three-dimensional plots, with the three clades closely distributed for all biological processes. The second and third groups, consisting only of destructivum and graminicola clades, respectively, were often self-grouped in the three-dimensional plots, indicating the dynamics of their biological processes differed greatly from the dynamics of the other clades. Although acutatum, dematium, and orbiculare clades were grouped together by cluster analysis, they were quite dispersed in the three-dimensional plots. In particular, higher values of parameter c (which determines the size of the bell-shaped curve described by the temperature-dependent equation) were obtained for both conidial infection and sporulation for the dematium clade. Nevertheless, the cardinal temperatures of these three clades were consistent and were generally lower than those of other groups.
For some of the species considered in this research, epidemiological models have been created to predict disease development (e.g., Dodd et al., 1991; Moral et al., 2012; Singh, 2020). Most of the models are simple and consider only one component of the pathogen life cycle, mainly conidial infection. Furthermore, many of these models have never been validated against independent data, i.e., model predictions have not been compared with real-world observations different from those used for model development (Rossi et al., 2010). Before these models are used in practical disease control, validation with real data obtained from different areas and years with different epidemiological conditions should be performed to assess model accuracy and robustness (Rossi et al., 2010). A mechanistic, weather-driven model was recently developed and validated for ripe rot of grapes caused by the Colletotrichum species (Ji et al., 2021). Several advantages have been previously reported for mechanistic models over empirical models, including a high explanatory ability and the possibility of easily incorporating information from previous and new experiments regarding pathogen biology and epidemiology (De Wolf and Isard, 2007; Rossi et al., 2015). The current report should help researchers to conduct further research on the biology and epidemiology of Colletotrichum species as well as to develop mechanistic models for those anthracnose diseases that currently lack such models. The current report may also provide a foundation for the development of a model that can be applied to multiple Colletotrichum spp. and to multiple anthracnose diseases based on clade similarities.
Data availability statement
The original contributions presented in the study are included in the article/Supplementary material; further inquiries can be directed to the corresponding author.
Author contributions
IS and TJ performed the systematic literature review. IS and VR performed the data analyses. All authors contributed to the writing of the manuscript. All authors contributed to the article and approved the submitted version.
Funding
This research was funded by the LIFE Programme of the European Union-project LIFE AGRESTIC, grant number LIFE17 CCM/IT/000062.
Conflict of interest
The authors declare that the research was conducted in the absence of any commercial or financial relationships that could be construed as a potential conflict of interest.
Publisher’s note
All claims expressed in this article are solely those of the authors and do not necessarily represent those of their affiliated organizations, or those of the publisher, the editors and the reviewers. Any product that may be evaluated in this article, or claim that may be made by its manufacturer, is not guaranteed or endorsed by the publisher.
Acknowledgments
IS conducted this study within the Doctoral School on the Agro-Food System (Agrisystem) at the Università Cattolica del Sacro Cuore, Piacenza, Italy.
Supplementary material
The Supplementary Material for this article can be found online at: https://www.frontiersin.org/articles/10.3389/fpls.2022.953760/full#supplementary-material
References
Akhtar, N., Chandra, R., and Mazhar, Z. (2018). Influence of media, temperature and pH on growth of Colletotrichum capsici (Syd.) causing anthracnose disease of chilli. J. Pharmacogn. Phytochem. 7, 3280–3284.
Al Zaemey, A. B., Magan, N., and Thompson, A. K. (1994). In vitro studies of the effect of environmental conditions on the anthracnose pathogen of bananas, Colletotrichum musae. Int. Biodeterior. 33, 369–381.
Ali, M. M. (1962). Multiple temperature optima in Collectotrichum graminicola. Mycopathol. Mycol. Appl. 17, 289–292. doi: 10.1007/BF02076211
Analytis, S. (1977). On the relation between biological development and temperature of some plant pathogenic fungi. Phytopathology 90, 64–76. doi: 10.1111/j.1439-0434.1977.tb02886.x
Antelmi, I., Sion, V., and Nigro, F. (2019). First report of Colletotrichum nymphaeae on olive in Italy. Plant Dis. 103, 765. doi: 10.1094/PDIS-05-18-0847-PDN
Auld, B. A., McRae, C. F., and Say, M. M. (1989). “Potential for Xanthium spinosum control by Colletotrichum orbiculare as a mycoherbicide.” in Proceedings VII Symposium of the Biological Control of Weeds, ed. E. S. Delfosse. March 6–11, 1988 (Rome, IT: Istituto Sperimentale Patologia Vegetale MAF), 435–443.
Bain, S. M., and Essary, S. H. (1906). A new anthracnose of alfalfa and red clover. J. Mycol. 12, 192–193. doi: 10.2307/3753010
Bardas, G. A., Koutita, O., and Tzavella-Klonari, K. (2009). Molecular diversity and assessment of biological characteristics of Greek Colletotrichum lindemuthianum populations. J. Phytopathol. 157, 311–318. doi: 10.1111/j.1439-0434.2008.01477.x
Barimani, M., Pethybridge, S. J., Vaghefi, N., Hay, F. S., and Taylor, P. W. J. (2013). A new anthracnose disease of pyrethrum caused by Colletotrichum tanaceti sp. nov. Plant Pathol. 62, 1248–1257. doi: 10.1111/ppa.12054
Baroncelli, R., Sarrocco, S., Zapparata, A., Tavarini, S., Angelini, L. G., and Vannacci, G. (2015). Characterization and epidemiology of Colletotrichum acutatum sensu lato (C. chrysanthemi) causing Carthamus tinctorius anthracnose. Plant Pathol. 64, 375–384. doi: 10.1111/ppa.12268
Bebber, D., Chaloner, T., and Gurr, S. (2020). Fungal and Oomycete Cardinal Temperatures (the Togashi Dataset), Dryad, Dataset (Accessed January 3, 2022).
Begum, M. M., Sariah, M., Puteh, A. B., and Abidin, M. Z. (2007). Detection of seed-borne fungi and site of infection by Colletotrichum truncatum in naturally-infected soybean seeds. Int. J. Agric. Res. 2, 81.
Bertetti, D., Gullino, M. L., and Garibaldi, A. (2009). Effect of leaf wetness duration, temperature and inoculum concentration on infection of evergreen azalea by Colletotrichum acutatum, the causal agent of anthracnose. J. Plant Pathol. 91, 763–766.
Biesbroek, G. R., Klostermann, J. E., Termeer, C. J., and Kabat, P. (2013). On the nature of barriers to climate change adaptation. Reg. Environ. Chang. 13, 1119–1129. doi: 10.1007/s10113-013-0421-y
Bragard, C., Dehnen-Schmutz, K., Di Serio, F., Gonthier, P., Jacques, M. A., Miret, J. A. J., et al. (2021). EFSA panel on plant health (PLH). Pest categorisation of Colletotrichum fructicola. EFSA J. 19:e06803. doi: 10.2903/j.efsa.2021.6803
Briosi, G., and Cavara, F. (1889). I fungi parassiti delle piante coltivate od utili essicati, delineati e descritti. Fasc. II, nos. 26–50.
Burnham, K.P., and Anderson, D.R. (2002). Model Selection and Multimodel Inference. A Practical Information-Theoretic Approach. New York: Springer.
Byrne, J. M., Hausbeck, M. K., Meloche, C., and Jarosz, A. M. (1998). Influence of dew period and temperature on foliar infection of greenhouse-grown tomato by Colletotrichum coccodes. Plant Dis. 82, 639–641. doi: 10.1094/PDIS.1998.82.6.639
Candel, J. J. (2014). Food security governance: a systematic literature review. Food Secur. 6, 585–601. doi: 10.1007/s12571-014-0364-2
Cannon, P. F., Damm, U., Johnston, P. R., and Weir, B. S. (2012). Colletotrichum - current status and future directions. Stud. Mycol. 73, 181–213. doi: 10.3114/sim0014
Chongo, G., and Bernier, C. C. (2000a). Disease incidence, lesion size, and sporulation in Colletotrichum truncatum as influenced by lentil genotype and temperature. Can. J. Plant Pathol. 22, 236–240. doi: 10.1080/07060660009500469
Chongo, G., and Bernier, C. C. (2000b). Effects of host, inoculum concentration, wetness duration, growth stage, and temperature on anthracnose of lentil. Plant Dis. 84, 544–548. doi: 10.1094/PDIS.2000.84.5.544
Crouch, J. A., Clarke, B. B., and Hillman, B. I. (2006). Unraveling evolutionary relationships among the divergent lineages of Colletotrichum causing anthracnose disease in turfgrass and corn. Phytopathology 96, 46–60. doi: 10.1094/PHYTO-96-0046
Damm, U., Cannon, P. F., Liu, F., Barreto, R. W., Guatimosim, E., and Crous, P. W. (2013). The Colletotrichum orbiculare species complex: important pathogens of field crops and weeds. Fungal Divers. 61, 29–59. doi: 10.1007/s13225-013-0255-4
Damm, U., Cannon, P. F., Woudenberg, J. H. C., and Crous, P. W. (2012). The Colletotrichum acutatum species complex. Stud. Mycol. 73, 37–113. doi: 10.3114/sim0010
Damm, U., O'Connell, R. J., Groenewald, J. Z., and Crous, P. W. (2014). The Colletotrichum destructivum species complex - hemibiotrophic pathogens of forage and field crops. Stud. Mycol. 79, 49–84. doi: 10.1016/j.simyco.2014.09.003
Damm, U., Woudenberg, J. H. C., Cannon, P. F., and Crous, P. W. (2009). Colletotrichum species with curved conidia from herbaceous hosts. Fungal Divers. 39:45.
Datar, V. V. (1995). Pathogenicity and effect of temperature on six fungi causing fruit rot of chilli. J. Mycol. Plant Pathol. 25, 195–197.
de Carvalho, Y., and e Carvalho, C. G. S. (1973). Effect of temperature incubation on the fungus Colletotrichum gossypii South. Var. cephalosporioides Costa. Pesqui. Agropecu. Trop. 3:1.
De Lapeyre de Bellaire, L., Chillet, M., and Mourichon, X. (2000). Elaboration of an early quantification method of quiescent infections of Colletotrichum musae on bananas. Plant Dis. 84, 128–133. doi: 10.1094/PDIS.2000.84.2.128
De Wolf, E. D., and Isard, S. A. (2007). Disease cycle approach to plant disease prediction. Annu. Rev. Phytopathol. 45, 203–220. doi: 10.1146/annurev.phyto.44.070505.143329
Dean, R., Van Kan, J. A., Pretorius, Z. A., Hammond-Kosack, K. E., Di Pietro, A., Spanu, P. D., et al. (2012). The top 10 fungal pathogens in molecular plant pathology. Mol. Plant Pathol. 13, 414–430. doi: 10.1111/j.1364-3703.2011.00783.x
Denner, F. D. N., Kotzé, J. M., and Putterill, J. F. (1986). The effect of temperature on spore germination, growth and appressorium formation of Colletotrichum gloeosporioides and Dothiorella aromatica. S. Afr. Avocado Growers’ Assoc. Yearb. 9, 19–22.
Diéguez-Uribeondo, J., Förster, H., and Adaskaveg, J. E. (2011). Effect of wetness duration and temperature on the development of anthracnose on selected almond tissues and comparison of cultivar susceptibility. Phytopathology 101, 1013–1020. doi: 10.1094/PHYTO-07-10-0193
Dillard, H. R. (1988). Influence of temperature, pH, osmotic potential, fungicide sensitivity on germination of conidia and growth from sclerotia of Colletotrichum coccodes in vitro. Phytopathology 78, 1357–1361. doi: 10.1094/Phyto-78-1357
Dillard, H. R. (1989). Effect of temperature, wetness duration, and inoculum density on infection and lesion development of Colletotrichum coccodes on tomato fruit. Phytopathology 79, 1063–1066. doi: 10.1094/Phyto-79-1063
Dodd, J. C., Estrada, A. B., Matcham, J., Jeffries, P., and Jeger, M. J. (1991). The effect of climatic factors on Colletotrichum gloeosporioides, causal agent of mango anthracnose, in the Philippines. Plant Pathol. 40, 568–575. doi: 10.1111/j.1365-3059.1991.tb02421.x
Du, M., Schardl, C. L., Nuckles, E. M., and Vaillancourt, L. J. (2005). Using mating-type gene sequences for improved phylogenetic resolution of Collectotrichum species complexes. Mycologia 97, 641–658. doi: 10.1080/15572536.2006.11832795
Dubrulle, G., Pensec, F., Picot, A., Rigalma, K., Pawtowski, A., Nicolleau, S., et al. (2020). Phylogenetic diversity and effect of temperature on pathogenicity of Colletotrichum lupini. Plant Dis. 104, 938–950. doi: 10.1094/PDIS-02-19-0273-RE
EPPO, (2022). EPPO Global Database. Available at: https://gd.eppo.int (Accessed January 21, 2022).
Es-Soufi, R., El Kbiach, M., Errabii, T., Saidi, R., Badoc, A., Chaveriat, L., et al. (2018). Biology and physiology of Colletotrichum acutatum strains causing strawberry’s anthracnose. Agric. Sci. 9, 974–990. doi: 10.4236/as.2018.98068
Estrada, A. B., Dodd, J. C., and Jeffries, P. (2000). Effect of humidity and temperature on conidial germination and appressorium development of two Philippine isolates of the mango anthracnose pathogen Colletotrichum gloeosporioides. Plant Pathol. 49, 608–618. doi: 10.1046/j.1365-3059.2000.00492.x
Everett, K. R. (2003). The effect of low temperatures on Colletotrichum acutatum and Colletotrichum gloeosporioides causing body rots of avocados in New Zealand. Australas. Plant Pathol. 32, 441–448. doi: 10.1071/AP03050
Everett, K. R., Pushparajah, I. P. S., Timudo, O. E., Chee, A. A., Scheper, R. W. A., Shaw, P. W., et al. (2018). Infection criteria, inoculum sources and splash dispersal pattern of Colletotrichum acutatum causing bitter rot of apple in New Zealand. Eur. J. Plant Pathol. 152, 367–383. doi: 10.1007/s10658-018-1481-0
Fernando, T. H. P. S., Jayasinghe, C. K., and Wijesundera, R. L. C. (2000). Factors affecting spore production, germination and viability of Colletotrichum acutatum isolates from Hevea brasiliensis. Mycol. Res. 104, 681–685. doi: 10.1017/S0953756200002483
Fitzell, R. D., and Peak, C. M. (1984). The epidemiology of anthracnose disease of mango: inoculum sources, spore production and dispersal. Ann. Appl. Biol. 104, 53–59. doi: 10.1111/j.1744-7348.1984.tb05586.x
Förster, H., and Adaskaveg, J. E. (1999). Identification of subpopulations of Colletotrichum acutatum and epidemiology of almond anthracnose in California. Phytopathology 89, 1056–1065. doi: 10.1094/PHYTO.1999.89.11.1056
Freeman, S., Katan, T., and Shabi, E. (1998). Characterization of Colletotrichum species responsible for anthracnose diseases of various fruits. Plant Dis. 82, 596–605. doi: 10.1094/PDIS.1998.82.6.596
Freeman, S., Shalev, Z., and Katan, J. (2002). Survival in soil of Colletotrichum acutatum and C. gloeosporioides pathogenic on strawberry. Plant Dis. 86, 965–970. doi: 10.1094/PDIS.2002.86.9.965
Gan, P., Narusaka, M., Kumakura, N., Tsushima, A., Takano, Y., Narusaka, Y., et al. (2016). Genus-wide comparative genome analyses of Colletotrichum species reveal specific gene family losses and gains during adaptation to specific infection lifestyles. Genome Biol. Evol. 8, 1467–1481. doi: 10.1093/gbe/evw089
Garrido, C., Carbú, M., Fernández-Acero, F. J., Vallejo, I., and Cantoral, J. M. (2009). Phylogenetic relationships and genome organisation of Colletotrichum acutatum causing anthracnose in strawberry. Eur. J. Plant Pathol. 125, 397–411. doi: 10.1007/s10658-009-9489-0
Ghouse, A. K. M. (1975). The influence of temperature on the growth and sporulation of Colletotrichum species. Geobios 2, 81–82.
Gibson, R. J. (1994). An investigation into the epidemiology and control of anthracnose (Colletotrichum truncatum) of lentil in Manitoba. master’s thesis. Winnipeg, MB: University of Manitoba.
Gillett, J. M., and Schilder, A. C. (2009). Environmental requirements for infection of blueberry fruit by Colletotrichum acutatum. Acta Hortic. 810, 355–360. doi: 10.17660/ActaHortic.2009.810.46
Glais-Varlet, I., Bouchek-Mechiche, K., and Andrivon, D. (2004). Growth in vitro and infectivity of Colletotrichum coccodes on potato tubers at different temperatures. Plant Pathol. 53, 398–404. doi: 10.1111/j.1365-3059.2004.01045.x
Goos, R. D., and Tschirsch, M. (1962). Effect of environmental factors on spore germination, spore survival, and growth of Gloeosporium musarum. Mycologia 54, 353–367.
Greer, L. A., Harper, J. D., Savocchia, S., Samuelian, S. K., and Steel, C. C. (2011). Ripe rot of south-eastern Australian wine grapes is caused by two species of Colletotrichum: C. acutatum and C. gloeosporioides with differences in infection and fungicide sensitivity. Aust. J. Grape Wine Res. 17, 123–128. doi: 10.1111/j.1755-0238.2011.00143.x
Gunnell, P. S., and Gubler, W. D. (1992). Taxonomy and morphology of Colletotrichum species pathogenic to strawberry. Mycologia 84, 157–165.
Han, Y. C., Zeng, X. G., Xiang, F. Y., Ren, L., Chen, F. Y., and Gu, Y. C. (2016). Distribution and characteristics of Colletotrichum spp. associated with anthracnose of strawberry in Hubei, China. Plant Dis. 100, 996–1006. doi: 10.1094/PDIS-09-15-1016-RE
Hartman, G. L., and Wang, T. C. (1992). “Characteristics of two Colletotrichum species and evaluation of resistance to anthracnose in pepper.” in Proceedings 3rd International Conference on Plant Protection in the Tropics. Vol. 6, ed. P. A. C. Ooi. March 20–23, 1990 (Kuala Lumpur, MY: Malaysian Plant Protection Society), 202–205.
Horn, N. L., and Carver, R. G. (1963). A new crown rot of strawberry plants caused by Colletotrichum fragariae. Phytopathol. 53, 768–770.
Hyde, K. D., Cai, L., Cannon, P. F., Crouch, J. A., Crous, P. W., Damm, U., et al. (2009). Colletotrichum - names in current use. Fungal Divers. 39, 147–182.
Ishida, N., and Akai, S. (1969). Relation of temperature to germination of conidia and appressorium formation in Colletotrichum lagenarium. Mycologia 61, 382–386.
Jackson, M. A., and Schisler, D. A. (1992). The composition and attributes of Colletotrichum truncatum spores are altered by the nutritional environment. Appl. Environ. Microbiol. 58, 2260–2265. doi: 10.1128/aem.58.7.2260-2265.1992
Jeger, M., Bragard, C., Caffier, D., Candresse, T., Chatzivassiliou, E., Dehnen-Schmutz, K., et al. (2018). EFSA panel on plant health (PLH), Pest categorisation of Colletotrichum gossypii. EFSA J. 16:e05305. doi: 10.2903/j.efsa.2018.5305
Ji, T., Salotti, I., Dong, C., Li, M., and Rossi, V. (2021). Modeling the effects of the environment and the host plant on the ripe rot of grapes, caused by the Colletotrichum species. Plan. Theory 10, 2288. doi: 10.3390/plants10112288
Jiang, S., Jin, J., Liu, J., Liu, B., Sun, F., and Bai, Q. (2021). Pathogen identification and screening of fungicides of anthracnose of Lupinus micranthus by Colletotrichum lupini. J. Northeast For. Univ. 49, 136–141.
Kamle, M., and Kumar, P. (2016). “Colletotrichum gloeosporioides: pathogen of anthracnose disease in mango (Mangifera indica L.),” in Current Trends in Plant Disease Diagnostics and Management Practices. eds. P. Kumar, V. K. Gupta, A. K. Tiwari, and M. Kamle (Cham, SZ: Springer International Publishing), 207–219.
Kawaradani, M., Nishimura, A., Moriwaki, J., Tomioka, K., Sato, T., Okada, K., et al. (2008). Anthracnose of perilla (Perilla ocymoides L.) caused by Colletotrichum destructivum. Jpn. J. Phytopathol. 74, 335–339. doi: 10.3186/jjphytopath.74.335
Kenny, M. K., Galea, V. J., and Price, T. V. (2012). Germination and growth of Colletotrichum acutatum and Colletotrichum gloeosporioides isolates from coffee in Papua New Guinea and their pathogenicity to coffee berries. Australas. Plant Pathol. 41, 519–528. doi: 10.1007/s13313-012-0117-7
King, W. T., Madden, L. V., Ellis, M. A., and Wilson, L. L. (1997). Effects of temperature on sporulation and latent period of Colletotrichum spp. infecting strawberry fruit. Plant Dis. 81, 77–84. doi: 10.1094/PDIS.1997.81.1.77
Kumar, B., and Dubey, K. S. (2007). Effect of media, temperature and pH on growth and sporulation of Colletotrichum dematium var. truncata. Ann. Plant Prot. Sci. 15, 260–261.
Kumara, K. L., and Rawal, R. D. (2008). Influence of carbon, nitrogen, temperature and pH on the growth and sporulation of some Indian isolates of Colletotrichum gloeosporioides causing anthracnose disease of papaya (Carrica papaya L). Trop. Agric. Res. Ext. 11, 7–12. doi: 10.4038/tare.v11i0.1779
Lee, D. H., Kim, D. H., Jeon, Y. A., Uhm, J. Y., and Hong, S. B. (2007). Molecular and cultural characterization of Colletotrichum spp. causing bitter rot of apples in Korea. Plant Pathol. J. 23, 37–44. doi: 10.5423/PPJ.2007.23.2.037
Lei, B. Z., and Li, G. Y. (2004). Identification and biological characteristics of the pathogen of Colletotrichum gloeosporioides on grape in Xinjiang. J. Shihezi Univ. (Nat. Sci.) 22, 298–300.
Lenné, J. M. (2002). “Some major plant diseases,” in Plant Pathologist’s Pocketbook. 3rd Edn. J. M. Waller, J. M. Lenné, and S. J. Waller (Wallingford, UK: CABI), 4–18.
Leonard, K. J., and Thompson, D. L. (1976). Effects of temperature and host maturity on lesion development of Colletotrichum graminicola on corn. Phytopathology 66, 635–639. doi: 10.1094/Phyto-66-635
Li, Y., Wang, R., and Liu, S. (1995). Biological characteristics of Colletotrichum lindemuthianum and screening of fungicides. J. Northeast. Agric. Univ. 26, 140–144.
Liang, X., Wang, B., Dong, Q., Li, L., Rollins, J. A., Zhang, R., et al. (2018). Pathogenic adaptations of Colletotrichum fungi revealed by genome wide gene family evolutionary analyses. PLoS One 13:e0196303. doi: 10.1371/journal.pone.0196303
Lim, J. Y., Lim, T. H., and Cha, B. J. (2002). Isolation and identification of Colletorichum musae from imported bananas. Plant Pathol. J. 18, 161–164. doi: 10.5423/PPJ.2002.18.3.161
Lima, N. B., Lima, W. G., Tovar-Pedraza, J. M., Michereff, S. J., and Câmara, M. P. (2015). Comparative epidemiology of Colletotrichum species from mango in northeastern Brazil. Eur. J. Plant Pathol. 141, 679–688. doi: 10.1007/s10658-014-0570-y
Lima, W. G., Spósito, M. B., Amorim, L., Gonçalves, F. P., and de Filho, P. A. M. (2011). Colletotrichum gloeosporioides, a new causal agent of citrus post-bloom fruit drop. Eur. J. Plant Pathol. 131, 157–165. doi: 10.1007/s10658-011-9795-1
Lin, L. I. (1989). A concordance correlation coefficient to evaluate reproducibility. Biometrics 45, 255–268. doi: 10.2307/2532051
Ling, L., and Yang, J. Y. (1944). Studies on the biology and pathogenicity of Colletotrichum indicum. Ann. Bot. 8, 91–104. doi: 10.1093/oxfordjournals.aob.a088555
Liu, A. Y., Chen, W. X., Gu, H., Shi, J. Y., and Li, J. (2006). Biological characteristic of pathogenic fungus causing anthracnose of loquat fruit. Acta Hortic. 750, 465–447. doi: 10.17660/ActaHortic.2007.750.74
Liu, W., Li, P., Wu, G., Lin, C., Miao, W., and Zhang, F. (2016). Biological characteristics of Colletotrichum gossypii in Hainan. Chinese J. Trop. Agric. 36, 51–57.
Liu, Z., Tang, S., Yang, L., Zheng, C., An, X., and Zhao, T. (2015). Sporulating conditions of Colletotrichum orbiculare causing anthracnose of watermelon. Mycosystema 34, 75–81.
López-Moral, A., Agustí-Brisach, C., Lovera, M., Luque, F., Roca, L. F., Arquero, O., et al. (2019). Effects of cultivar susceptibility, fruit maturity, leaf age, fungal isolate, and temperature on infection of almond by Colletotrichum spp. Plant Dis. 103, 2425–2432. doi: 10.1094/PDIS-12-18-2281-RE
López-Moral, A., Raya-Ortega, M. C., Agustí-Brisach, C., Roca, L. F., Lovera, M., Luque, F., et al. (2017). Morphological, pathogenic, and molecular characterization of Colletotrichum acutatum isolates causing almond anthracnose in Spain. Plant Dis. 101, 2034–2045. doi: 10.1094/PDIS-03-17-0318-RE
Luo, Y., and TeBeest, D. O. (1999). Effect of temperature and dew period on infection of northern Jointvetch by wild-type and mutant strains of Colletotrichum gloeosporioides f. sp. aeschynomene1. Biol. Control 14, 1–6. doi: 10.1006/bcon.1998.0660
Ma, J., Wang, S., Yuan, Q., and Wang, Y. (2016). Major biological characteristics of C. destructivum causing anthracnose of alfalfa. Plant Prot. 42, 105–110.
Madden, L. V., and Paul, P. A. (2011). Meta-analysis for evidence synthesis in plant pathology: an overview. Phytopathol. 101, 16–30. doi: 10.1094/PHYTO-03-10-0069
Mello, A. F. S., Machado, A. C. Z., and Bedendo, I. P. (2004). Development of Colletotrichum gloeosporioides isolated from green pepper in different culture media, temperatures, and light regimes. Sci. Agric. 61, 542–544. doi: 10.1590/S0103-90162004000500013
Miehle, B. R., and Lukezic, F. L. (1972). Studies on conidial germination and appressorium formation by Colletotrichum trifolii Bain & Essary. Can. J. Microbiol. 18, 1263–1269. doi: 10.1139/m72-195
Miles, T. D., Gillett, J. M., Jarosz, A. M., and Schilder, A. M. C. (2013). The effect of environmental factors on infection of blueberry fruit by Colletotrichum acutatum. Plant Pathol. 62, 1238–1247. doi: 10.1111/ppa.12061
Misra, A. P., and Dutta, K. K. (1963). Studies in anthracnose fungi III. A comparative study of two isolates of Colletotrichum capsici (Syd.) Butler and Bisby. J. Indian Bot. Soc. 42, 74–85.
Misra, A. P., and Singh, R. P. (1962). Effect of temperature and humidity on the development of banana anthracnose. Indian Phytopathol. 15, 11–13.
Monroe, J. S., Santini, J. B., and Latin, R. (1997). A model defining the relationship between temperature and leaf wetness duration, and infection of watermelon by Colletotrichum orbiculare. Plant Dis. 81, 739–742. doi: 10.1094/PDIS.1997.81.7.739
Monteiro, J. E. B., Sentelhas, P. C., Gleason, M. L., Esker, P. D., and Chiavegato, E. J. (2009). Development of ramulosis disease of cotton under controlled environment and field conditions. Phytopathology 99, 659–665. doi: 10.1094/PHYTO-99-6-0659
Monteith, J. (1928). Clover anthracnose caused by Colletotrichum trifolii. USDA Tech. Bull. 28, 1–26.
Moraes, S. R. G., Escanferla, M. E., and Massola, N. S. Jr. (2015). Prepenetration and penetration of Colletotrichum gloeosporioides into guava fruit (Psidium guajava L.): effects of temperature, wetness period and fruit age. J. Phytopathol. 163, 149–159. doi: 10.1111/jph.12294
Moral, J., de Oliveira, R., and Trapero, A. (2009). Elucidation of the disease cycle of olive anthracnose caused by Colletotrichum acutatum. Phytopathology 99, 548–556. doi: 10.1094/PHYTO-99-5-0548
Moral, J., Jurado-Bello, J., Sánchez, M. I., de Oliveira, R., and Trapero, A. (2012). Effect of temperature, wetness duration, and planting density on olive anthracnose caused by Colletotrichum spp. Phytopathology 102, 974–981. doi: 10.1094/PHYTO-12-11-0343
Moral, J., Jurado-Bello, J., and Trapero, A. (2011). Effect of temperature and relative humidity on mycelial growth, conidial germination and fruit infection by Colletotrichum spp. causing olive anthracnose. IOBC/WPRS Bull. 79:14.
Moreira, R. R., Silva, G. A., and De Mio, L. L. M. (2020). Colletotrichum acutatum complex causing anthracnose on peach in Brazil. Australas. Plant Pathol. 49, 179–189. doi: 10.1007/s13313-020-00690-z
Moreira, R. R., Zielinski, E. C., Castellar, C., Bergamin Filho, A., and De Mio, L. L. M. (2021). Study of infection process of five species of Colletotrichum comparing symptoms of glomerella leaf spot and bitter rot in two apple cultivars. Eur. J. Plant Pathol. 159, 37–53. doi: 10.1007/s10658-020-02138-y
Mulrow, C. D. (1994). Systematic reviews: rationale for systematic reviews. BMJ 309, 597–599. doi: 10.1136/bmj.309.6954.597
Münch, S., Lingner, U., Floss, D. S., Ludwig, N., Sauer, N., and Deising, H. B. (2008). The hemibiotrophic lifestyle of Colletotrichum species. J. Plant Physiol. 165, 41–51. doi: 10.1016/j.jplph.2007.06.008
Nash, J. E., and Sutcliffe, J. V. (1970). River flow forecasting through conceptual models part 1: a discussion of principles. J. Hydrol. 10, 282–290. doi: 10.1016/0022-1694(70)90255-6
Neelam, G., and Khirbat, S. K. (2015). Effect of temperature on the development of fruit rot of chilli. Ann. Biol. 31, 248–250.
Ngugi, H. K., Julian, A. M., King, S. B., and Peacocke, B. J. (2000). Epidemiology of sorghum anthracnose (Colletotrichum sublineolum) and leaf blight (Exserohilum turcicum) in Kenya. Plant Pathol. 49, 129–140. doi: 10.1046/j.1365-3059.2000.00424.x
Nirenberg, H. I., Feiler, U., and Hagedorn, G. (2002). Description of Colletotrichum lupini comb. nov. in modern terms. Mycologia 94, 307–320.
Nitzan, N., and Lahkim, L. T. (2003). Effect of temperature and pH on in vitro growth rate and sclerotial density of Colletotrichum coccodes isolates from different VCGs. Am. J. Potato Res. 80, 335–339. doi: 10.1007/BF02854318
Okoli, C., and Schabram, K. (2010). A guide to conducting a systematic literature review of information systems research. Work. Papers Inf. Syst. 10, 1–46. doi: 10.2139/ssrn.1954824
Pakela, Y. P., Aveling, T. A. S., and Coutinho, T. A. (2002). Effect of plant age, temperature and dew period on the severity of anthracnose of cowpea caused by Colletotrichum dematium. Afr. Plant Prot. 8, 65–68.
Pandey, R. R., Arora, D. K., and Dubey, R. C. (1997). Effect of environmental conditions and inoculum density on infection of guava fruits by Colletotrichum glososporioides. Mycopathologia 137, 165–172. doi: 10.1023/A:1006842801828
Parikka, P., and Lemmetty, A. (2004). Tracing latent infection of Colletotrichum acutatum on strawberry by PCR. Eur. J. Plant Pathol. 110, 393–398. doi: 10.1023/B:EJPP.0000021073.67137.d2
Peres, N. A., Kuramae, E. E., Dias, M. S., and De Souza, N. L. (2002). Identification and characterization of Colletotrichum spp. affecting fruit after harvest in Brazil. J. Phytopathol. 150, 128–134. doi: 10.1046/j.1439-0434.2002.00732.x
Peres, N. A., MacKenzie, S. J., Peever, T. L., and Timmer, L. W. (2008). Postbloom fruit drop of citrus and key lime anthracnose are caused by distinct phylogenetic lineages of Colletotrichum acutatum. Phytopathology 98, 345–352. doi: 10.1094/PHYTO-98-3-0345
Peres, N. A., Timmer, L. W., Adaskaveg, J. E., and Correll, J. C. (2005). Lifestyles of Colletotrichum acutatum. Plant Dis. 89, 784–796. doi: 10.1094/PD-89-0784
Peries, O. S. (1973). A critical study of the use of weather data for the economic control of Hevea diseases. Q. J. Rubb. Res. Inst. Sri Lanka 50, 208–217.
Pessoa, W. R. L. S., Oliveira, S. M. A. D., Dantas, S. A. F., Tavares, S. C. C. D. H., and Santos, A. M. G. (2007). Efeito da temperatura e período de molhamento sobre o desenvolvimento de lesões de Colletotrichum musae em banana. Summa Phytopathol. 33, 147–151. doi: 10.1590/S0100-54052007000200008
Prajapati, M. K., Rawat, S., Singh, P., and Shankar, K. (2020). Cultural and morphological characterization of Colletotrichum capsici causing anthracnose of chilli (Capsicum annuum L.). J. Pharmacogn. Phytochem. 9, 1985–1989.
Ranjitham Thangamani, P., Kuppusamy, P., Peeran, M. F., Gandhi, K., and Raguchander, T. (2011). Morphological and physiological characterization of Colletotrichum musae the causal organism of banana anthracnose. World J. Agric. Sci. 7, 743–754.
Razakamanantsoa, S. (1966). Actions des différents produits volatils émis par la banane au cours de sa maturation sur la croissance du Gloeosporium musarum. Fruits 21, 597–604.
Rodriguez-Salamanca, L. M., Naegele, R. P., Quesada-Ocampo, L. M., and Hausbeck, M. K. (2018). Inoculation method, temperature, and relative humidity affect leaf and neck anthracnose, a new onion disease in Michigan. Plant Health Prog. 19, 64–68. doi: 10.1094/PHP-10-17-0063-RS
Rodriguez-Salamanca, L. M., Quesada-Ocampo, L. M., Naegele, R. P., and Hausbeck, M. K. (2015). Characterization, virulence, epidemiology, and management of anthracnose in celery. Plant Dis. 99, 1832–1840. doi: 10.1094/PDIS-09-14-0994-RE
Rossi, V., Giosuè, S., and Caffi, T. (2010). “Modelling plant diseases for decision making in crop protection,” in Precision Crop Protection: The Challenge and Use of Heterogeneity. eds. E. Oerke, R. Gerhards, G. Menz, and R. A. Sikora (Dordrecht, The Netherlands: Springer), 241–258.
Rossi, V., Onesti, G., Legler, S. E., and Caffi, T. (2015). Use of systems analysis to develop plant disease models based on literature data: grape black-rot as a case-study. Eur. J. Plant Pathol. 141, 427–444. doi: 10.1007/s10658-014-0553-z
Sangpueak, R., Phansak, P., and Buensanteai, N. (2018). Morphological and molecular identification of Colletotrichum species associated with cassava anthracnose in Thailand. J. Phytopathol. 166, 129–142. doi: 10.1111/jph.12669
Sanogo, S., Stevenson, R. E., and Pennypacker, S. P. (2003). Appressorium formation and tomato fruit infection by Colletotrichum coccodes. Plant Dis. 87, 336–340. doi: 10.1094/PDIS.2003.87.4.336
Sardhara, M. J., Davara, D. K., Moradia, A. M., and Kapadiya, H. J. (2016). Effect of culture media and temperature on growth and sporulation of Colletotrichum lindemuthianum of urdbean in vitro. Int. J. Plant Prot. 9, 47–51. doi: 10.15740/HAS/IJPP/9.1/47-51
Scherm, H., Thomas, C. S., Garrett, K. A., and Olsen, J. M. (2014). Meta-analysis and other approaches for synthesizing structured and unstructured data in plant pathology. Annu. Rev. Phytopathol. 52, 453–476. doi: 10.1146/annurev-phyto-102313-050214
Shabi, E., and Katan, T. A. L. M. A. (1983). Occurrence and control of anthracnose of almond in Israel. Plant Dis. 67, 1364–1366. doi: 10.1094/PD-67-1364
Sharma, M., and Kulshrestha, S. (2015). Colletotrichum gloeosporioides: an anthracnose causing pathogen of fruits and vegetables. Biosci. Biotechnol. Res. Asia 12, 1233–1246. doi: 10.13005/bbra/1776
Signorell, A. (2020). DescTools: Tools for Descriptive Statistics. R Package Version 0.99, p. 38. Available at: https://cran.r-project.org/package=DescTools (Accessed October 25, 2020).
Sindhan, G. S. (1983). Effect of temperature and relative humidity on the development of anthracnose French bean. Progress. Hortic. 15, 132–135.
Singh, R. (2020). Modeling for anthracnose development in mango in relation to weather parameters. Australas. Plant Pathol. 49, 285–294. doi: 10.1007/s13313-020-00704-w
Sinha, A. K., Verma, K. P., Agarwal, K. C., Toorray, N. K., and Thakur, M. P. (2004). Factors influencing growth, sporulation and spore gremination of Colletotrichum capsici. Adv. Plant Sci. 17, 71–74.
Skoropad, W. P. (1967). Effect of temperature on the ability of Colletotrichum graminicola to form appressoria and penetrate barley leaves. Can. J. Plant Sci. 47, 431–434. doi: 10.4141/cjps67-076
Smith, B. J., and Black, L. L. (1987). Resistance of strawberry plants to Colletotrichum fragariae affected by environmental conditions. Plant Dis. 71, 834–837. doi: 10.1094/PD-71-0834
Smith, B. J., and Black, L. L. (1990). Morphological, cultural and pathogenic variation among Colletotrichum species isolated from strawberry. Plant Dis. 74, 69–76. doi: 10.1094/PD-74-0069
Smith, J. E., Korsten, L., and Aveling, T. A. S. (1999). Infection process of Colletotrichum dematium on cowpea stems. Mycol. Res. 103, 230–234. doi: 10.1017/S0953756298006868
Soares-Colletti, A. R., and Lourenço, S. D. A. (2014). Effect of temperature, wetness duration and cultivar on the development of anthracnose in guava fruits. Summa Phytopathol. 40, 307–312. doi: 10.1590/0100-5405/1988
Solanki, J. S., Jain, J. P., and Agnihotri, J. P. (1974). Effect of temperature and sources of carbon and nitrogen on the conidial germination and appresoria formation in Colletotrichum capsici. Mycopathol. Mycol. Appl. 52, 191–196. doi: 10.1007/BF02198742
Steel, C. C., Greer, L. A., and Savocchia, S. (2012). Grapevine inflorescences are susceptible to the bunch rot pathogens, Greeneria uvicola (bitter rot) and Colletotrichum acutatum (ripe rot). Eur. J. Plant Pathol. 133, 773–778. doi: 10.1007/s10658-012-9957-9
Sun, H., Tian, J., Steinkellner, S., and Liang, Y. (2020). Identification and characterization of Colletotrichum destructivum causing anthracnose on sunflower. Arch. Microbiol. 202, 1459–1467. doi: 10.1007/s00203-020-01861-8
Sutherst, R. W., Constable, F., Finlay, K. J., Harrington, R., Luck, J., and Zalucki, M. P. (2011). Adapting to crop pest and pathogen risks under a changing climate. Wiley Interdiscip. Rev. Clim. Chang. 2, 220–237.
Sutton, B. C. (1992). “The genus Glomerella and its anamorph colletotrichum,” in Colletotrichum: Biology, Pathology and Control. eds. J. A. Bailey and M. J. Jeger (Wallingford, UK: CAB International), 1–26.
Talhinhas, P., and Baroncelli, R. (2021). Colletotrichum species and complexes: geographic distribution, host range and conservation status. Fungal Divers. 110, 109–198. doi: 10.1007/s13225-021-00491-9
Talhinhas, P., Mota-Capitão, C., Martins, S., Ramos, A. P., Neves-Martins, J., Guerra-Guimarães, L., et al. (2011). Epidemiology, histopathology and aetiology of olive anthracnose caused by Colletotrichum acutatum and C. gloeosporioides in Portugal. Plant Pathol. 60, 483–495. doi: 10.1111/j.1365-3059.2010.02397.x
Thomas, G. J., Sweetingham, M. W., Yang, H. A., and Speijers, J. (2008). Effect of temperature on growth of Colletotrichum lupini and on anthracnose infection and resistance in lupins. Australas. Plant Pathol. 37, 35–39. doi: 10.1071/AP07075
Tiffany, L. H., and Gilman, J. C. (1954). Species of Colletotrichum from legumes. Mycologia 46, 52–75.
Timmer, L. W., Brown, G. E., and Zitko, S. E. (1998). The role of Colletotrichum spp. in postharvest anthracnose of citrus and survival of C. acutatum on fruit. Plant Dis. 82, 415–418. doi: 10.1094/PDIS.1998.82.4.415
Tomioka, K., Nishikawa, J., Moriwaki, J., and Sato, T. (2011). Anthracnose of snapdragon caused by Colletotrichum destructivum. J. Gen. Plant Pathol. 77, 60–63. doi: 10.1007/s10327-010-0278-6
Tomioka, K., Sato, T., Moriwaki, J., Terasawa, Y., and Koganezawa, H. (2012). Anthracnose of bacopa caused by Colletotrichum destructivum. J. Gen. Plant Pathol. 78, 133–135. doi: 10.1007/s10327-011-0357-3
Tripathi, P. P., Singh, P. C., and Ramesh, S. (2016). Effect of temperature and pH on growth and sporulation of Colletotrichum capsici causing die-back of chilli. Ann. Biol. 32, 193–195.
Tu, J. C. (1982). Effect of temperature on incidence and severity of anthracnose on white bean. Plant Dis. 66, 781–783. doi: 10.1094/PD-66-781
Uematsu, S., Kageyama, K., Moriwaki, J., and Sato, T. (2012). Colletotrichum carthami comb. nov., an anthracnose pathogen of safflower, garland chrysanthemum and pot marigold, revived by molecular phylogeny with authentic herbarium specimens. J. Gen. Plant Pathol. 78, 316–330. doi: 10.1007/s10327-012-0397-3
Uysal, A., and Kurt, Ş. (2017). Influence of inoculum density, temperature, wetness duration, and leaf age on infection and development of spinach anthracnose caused by the fungal pathogen Colletotrichum spinaciae. Eur. J. Plant Pathol. 149, 1041–1052. doi: 10.1007/s10658-017-1249-y
Vasić, T., Radović, J., Stanisavljević, R., Jevtić, G., and Gajić, S. (2007). Odgajivačke i patogene odlike Colletotrichum trifolii, prouzrokovača antraknoze lucerke. Zb. Rad. 44, 507–513.
Veloso, J. S., Lima, W. G., Reis, A., Doyle, V. P., Michereff, S. J., and Câmara, M. P. S. (2020). Factors influencing biological traits and aggressiveness of Colletotrichum species associated with cashew anthracnose in Brazil. Plant Pathol. 70, 167–180. doi: 10.1111/ppa.13276
Vieira, W. A. S., Bezerra, P. A., da Silva, A. C., Veloso, J. S., Câmara, M. P. S., and Doyle, V. P. (2020). Optimal markers for the identification of Colletotrichum species. Evol. Biol. 143:106694. doi: 10.1016/j.ympev.2019.106694
Waller, J. M., Lenné, J. M., and Waller, S. J. (2002). Plant Pathologist’s Pocketbook. (Wallingford, UK: CABI).
Wang, N. Y., Forcelini, B. B., and Peres, N. A. (2019). Anthracnose fruit and root necrosis of strawberry are caused by a dominant species within the Colletotrichum acutatum species complex in the United States. Phytopathology 109, 1293–1301. doi: 10.1094/PHYTO-12-18-0454-R
Wang, B., Li, B. H., Dong, X. L., Wang, C. X., and Zhang, Z. F. (2015). Effects of temperature, wetness duration, and moisture on the conidial effects of temperature and wetness period on infection of grape by Colletotrichum gloeosporioides germination, infection, and disease incubation period of Glomerella cingulata. Plant Dis. 99, 249–256. doi: 10.1094/PDIS-04-14-0361-RE
Wang, P. S., Liu, X. Q., Wang, Y. Z., Luan, B. H., and Zhang, W. (2009). Study on the biological characteristics of grape ripe rot. Jiangsu Agric. Sci. 1, 128–129.
Wastie, R. L. (1972). Secondary leaf fall of Hevea brasiliensis: factors affecting the production, germination and viability of spores of Colletotrichum gloeosporioides. Ann. Appl. Biol. 72, 273–282. doi: 10.1111/j.1744-7348.1972.tb01294.x
Weir, B. S., Johnston, P. R., and Damm, U. (2012). The Colletotrichum gloeosporioides species complex. Stud. Mycol. 73, 115–180. doi: 10.3114/sim0011
Wickham, H. (2019). Modelr: Modelling Functions That Work With the Pipe. R Package Version 0.1, p. 4. Available online: https://rdrr.io/cran/modelr/man/modelr-package.html (Accessed October 20, 2020).
Wilson, L. L., Madden, L. V., and Ellis, M. A. (1990). Influence of temperature and wetness duration on infection of immature and mature strawberry fruit by Colletotrichum acutatum. Phytopathology 80, 111–116. doi: 10.1094/Phyto-80-111
Wong, C. F. J., Nik, W. Z., and Lim, T. K. (1983). Studies of Colletotrichum dematium f. sp. truncatum on soybean. Pertanika J. Sci. Technol. 6, 28–33.
Xiao, C. L., MacKenzie, S. J., and Legard, D. E. (2004). Genetic and pathogenic analyses of Colletotrichum gloeosporioides isolates from strawberry and noncultivated hosts. Phytopathology 94, 446–453. doi: 10.1094/PHYTO.2004.94.5.446
Xie, B., Ouyang, B., and Ouyang, F. (1992). Study on biological characteristics of Colletotrichum capsici. Microbiol. China 19, 321–324.
Xu, S., Christensen, M. J., and Li, Y. (2017). Pathogenicity and characterization of Colletotrichum lentis: A causal agent of anthracnose in common vetch (Vicia sativa). Eur. J. Plant Pathol. 149, 719–731. doi: 10.1007/s10658-017-1221-x
Yan, Y., Yuan, Q., Tang, J., Huang, J., Hsiang, T., Wei, Y., et al. (2018). Colletotrichum higginsianum as a model for understanding host–pathogen interactions: a review. Int. J. Mol. Sci. 19, 2142. doi: 10.3390/ijms19072142
Yang, C. D., Bian, J., Chen, T. X., Chen, X. R., Wang, H. Q., Yang, X. L., et al. (2017). Biological characteristics of the Angelica sinensis anthracnose causal agent, Colletotrichum dematium. Acta Pratacul. Sin. 26, 139–144.
Yang, C., Chen, X., Jiang, H., Xue, L., Pu, C., and Zhang, Z. (2013). Biological and culture characteristics of Colletotrichum coccodes. Plant Prot. 39, 40–45.
Yang, Y. K., Kim, S. O., Chung, H. S., and Lee, Y. H. (2000). Use of Colletotrichum graminicola KA001 to control barnyard grass. Plant Dis. 84, 55–59. doi: 10.1094/PDIS.2000.84.1.55
Yun, S. C., and Park, E. W. (1990). Effects of temperature and wetness period on infection of grape by Colletotrichum gloeosporioides. Korean J. Plant Pathol. 6, 219–228.
Zakaria, L. (2021). Diversity of Colletotrichum species associated with anthracnose disease in tropical fruit crops: a review. Agriculture 11, 297. doi: 10.3390/agriculture11040297
Zakaria, L., Sahak, S., Zakaria, M., and Salleh, B. (2009). Characterisation of Colletotrichum species associated with anthracnose of banana. Trop. Life Sci. Res. 20, 119–125.
Zhang, L., Song, J., Tan, G., Han, X., and Shen, J. (2014). Characterization of Colletotrichum gloeosporioides responsible for anthracnose disease of Trichosanthes kirilowii maxim in central China. Phytoparasitica 42, 549–558. doi: 10.1007/s12600-014-0393-6
Zhao, D., He, H., and Yang, X. (2020). Strawberry leaves infected by Colletotrichum fragariae: effect of temperature and humidity. J. Agric. For. 10, 22–26.
Keywords: Colletotrichum, Glomerella, anthracnose, systematic literature review, modeling
Citation: Salotti I, Ji T and Rossi V (2022) Temperature requirements of Colletotrichum spp. belonging to different clades. Front. Plant Sci. 13:953760. doi: 10.3389/fpls.2022.953760
Edited by:
Tika Adhikari, North Carolina State University, United StatesReviewed by:
Mark Angelo Balendres, University of the Philippines Los Baños, PhilippinesJuan Manuel Tovar-Pedraza, Consejo Nacional de Ciencia y Tecnología (CONACYT), Mexico
Copyright © 2022 Salotti, Ji and Rossi. This is an open-access article distributed under the terms of the Creative Commons Attribution License (CC BY). The use, distribution or reproduction in other forums is permitted, provided the original author(s) and the copyright owner(s) are credited and that the original publication in this journal is cited, in accordance with accepted academic practice. No use, distribution or reproduction is permitted which does not comply with these terms.
*Correspondence: Vittorio Rossi, dml0dG9yaW8ucm9zc2lAdW5pY2F0dC5pdA==