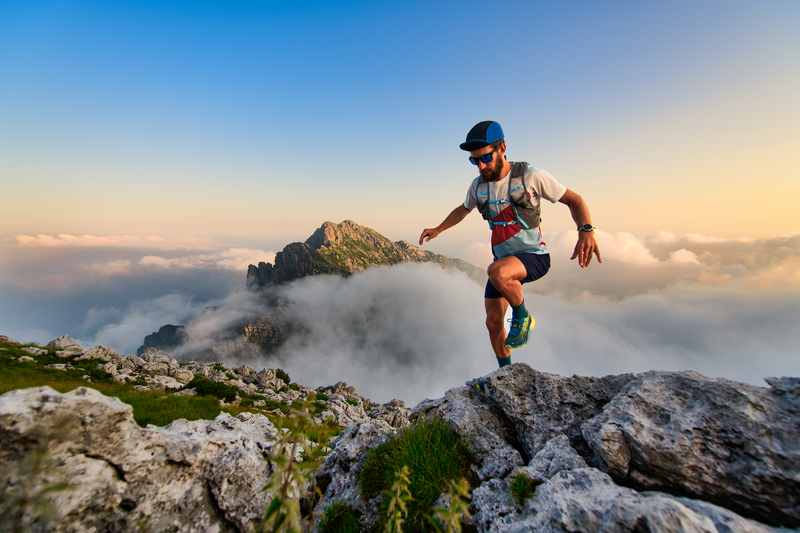
95% of researchers rate our articles as excellent or good
Learn more about the work of our research integrity team to safeguard the quality of each article we publish.
Find out more
REVIEW article
Front. Plant Sci. , 02 November 2022
Sec. Plant Abiotic Stress
Volume 13 - 2022 | https://doi.org/10.3389/fpls.2022.946717
This article is part of the Research Topic Nanofertilizers and Abiotic Stress Tolerance in Plants View all 9 articles
Plants are subjected to a wide range of abiotic stresses, such as heat, cold, drought, salinity, flooding, and heavy metals. Generally, abiotic stresses have adverse impacts on plant growth and development which affects agricultural productivity, causing food security problems, and resulting in economic losses. To reduce the negative effects of environmental stress on crop plants, novel technologies, such as nanotechnology, have emerged. Implementing nanotechnology in modern agriculture can also help improve the efficiency of water usage, prevent plant diseases, ensure food security, reduce environmental pollution, and enhance sustainability. In this regard, nanoparticles (NPs) can help combat nutrient deficiencies, promote stress tolerance, and improve the yield and quality of crops. This can be achieved by stimulating the activity of certain enzymes, increasing the contents (e.g., chlorophyll) and efficiency of photosynthesis, and controlling plant pathogens. The use of nanoscale agrochemicals, including nanopesticides, nanoherbicides, and nanofertilizers, has recently acquired increasing interest as potential plant-enhancing technologies. This review acknowledges the positive impacts of NPs in sustainable agriculture, and highlights their adverse effects on the environment, health, and food chain. Here, the role and scope of NPs as a practical tool to enhance yield and mitigate the detrimental effects of abiotic stresses in crops are described. The future perspective of nanoparticles in agriculture has also been discussed.
Abiotic stress factors, that can affect modern agricultural productivity worldwide include high or low temperature, waterlogging, drought, salinity, heavy metals (HMs), and ultraviolet (UV) radiation (Seleiman et al., 2020a; Badawy et al., 2021). Plant response to abiotic stress involves alterations in various morphological, physiological, and biochemical processes depending on the crop type, stress type, and time of exposure (Semida et al., 2014; Desoky et al., 2020a; Rady et al., 2021; Abd El-Mageed et al., 2022; Shaaban et al., 2022). As such, sustainable agriculture and yield productivity can improve the quality of soil, water, and other resources required by plants (Badal et al., 2013; Saxena et al., 2016; Desoky et al., 2020b). To meet the increasing global food demand, researchers are striving to ameliorate the detrimental effects of abiotic stresses, enhance crop yield and food production, and achieve sustainability and food security. Indeed, for addressing these urgent global concerns, researchers must continue developing innovative technologies or solutions.
Nanotechnology is a fascinating and rapidly developing branch of research that has led to various innovations (El-Saadony et al., 2020, 2021a; Abd El-Ghany et al., 2021). In particular, nanotechnology can help provide effective solutions to agriculture-related problems and achieve a sustainable and secure future for agriculture (Seleiman et al., 2021b). Nanotechnology has gained tremendous attention in recent years owing to its wide range of applications in medicine, drug delivery, energy, poultry production, and the agrifood sector (Seleiman et al., 2020a; Yousry et al., 2020; Salem et al., 2021). In agriculture, nanotechnology is mainly utilized in the application of nanofertilizers and nanopesticides to track products and nutrient levels for enhancing growth and productivity and increasing plant resistance to insect pests and microbial diseases (Shang et al., 2019; Bhatt et al., 2020).
Nanoparticles (NPs) are tiny materials 1–100 nm in size (Khan and Upadhyaya, 2019). In contrast to their larger sized equivalents, NPs possess certain unique and diverse physicochemical properties. For instance, NPs have a large surface area-to-volume ratio, high adsorption efficacy, and increased connecting and working efficiencies owing to their extremely small size (Nel et al., 2006; Dubchak et al., 2010). Thus, NPs have been integrated into disease management strategies as bactericides/fungicides/pesticides to enhance plant health. NPs can also serve as macro and micro-nanofertilizers in plants to alleviate nutrient deficiency symptoms and supplement essential elements. Various biological, physical, and chemical techniques can be used for NPs synthesis (Singh et al., 2016).
In agriculture and agrifood business, NPs can be applied in the form of nanosensors, nanofertilizers, nanoherbicides/nanopesticides, and nanoremediators (Figure 1; Elsakhawy et al., 2018; El-Saadony et al., 2021b). However, the mechanisms of underlying how NPs interact with plants have not been completely elucidated (Saxena et al., 2016; Khan et al., 2019). Therefore, this review highlights the current knowledge and potential uses of NPs that are widely used in agriculture, along with their effects on plants for better crop improvement and sustainable agriculture.
With the increasing world population, abiotic stress conditions are increasingly affecting crop production (Figure 2). During stress, physiological and biochemical changes occur in plant cells that can adversely affect plant growth, development, and productivity (Al-Ashkar et al., 2019; Seleiman et al., 2020b,2021a; Taha et al., 2021). Species and varieties bred to tolerate these challenges along with nanotechnology and other climate-sensitive agricultural technologies could be the most efficient adaptation strategy to cope with climate and abiotic stress factors, thereby achieving sustainable production (Kumari et al., 2022).
Figure 2. Abiotic stress factors and their negative effects on plants. ROS, reactive oxygen species; WUE, water use efficiency; HM, heavy metals.
Major abiotic stressors that limit crop returns globally include heat stress and drought (Abd El-Mageed et al., 2019; Batool et al., 2020; Semida et al., 2020; Rasheed et al., 2021). Drought induces many morphological, physiological, molecular, and metabolic changes in plants which are relatively significant. Plants regulate their stomatal conductance to control the amount of water lost, and optimize CO2 assimilation to avoid photosynthetic inhibition, allowing them to resist water stress (Faraji and Sepehri, 2020). Under arid conditions, phenolics, flavonoids and antioxidant enzymes are affected to a large extent. Root-sourced signals are transported via the xylem to leaves, thus, affecting the cellular status in drought-stressed plants (Afshari et al., 2021). Turgor loss can also be observed in plants under drought stress. If dehydration is severe, the protoplasm may become rigid, consequently altering the cellular metabolism and inhibiting plant growth. Drought can severely disrupt cellular metabolism, ion accumulation, membrane structure integrity, and protein structures in plants. Therefore, leaf growth, photosynthetic rates and enzymatic activities are reduced. Drought can also induce the excessive generation of reactive oxygen species (ROS) in plants; and thereby results in oxidative stress (Cruz de Carvalho, 2008). In addition, salinity and HMs stress are also considered among the environmental factors that limit crop yield in many countries (Abd El-Mageed et al., 2018; Ye et al., 2019; Sofy et al., 2020; Taha et al., 2020; Dustgeer et al., 2021; Khan et al., 2021; Seleiman et al., 2022).
Salinity is a type of abiotic stress that is widespread and responsible for considerably decreasing plant growth. Soil salinity inhibits seed germination owing to the low osmotic potential generated around the seeds, which prevents water uptake (Tavakkoli et al., 2010; Seleiman et al., 2020c; Alkharabsheh et al., 2021; Taha et al., 2021). Sodium chloride (NaCl)-induced oxidative stress in legumes, considerably inhibits growth, decreases seed nutrient quality and lowers nodulation (Hernandez et al., 2000; Ahmad et al., 2008). Plants can employ various antioxidant defense mechanisms, both enzymatic and non-enzymatic, to reduce the effect of oxidative stress associated with salinity. Ascorbate and carotenoids are critical non-enzymatic defense mechanisms against salinity, whereas proline (Pro) is a known osmoregulatory stress-related compound (Anoop and Gupta, 2003).
Plant growth and development benefit from essential elements, such as cobalt (Co), copper (Cu), iron (Fe), manganese (Mn), molybdenum (Mo), nickel (Ni), zinc (Zn), and non-essential elements such as cadmium (Cd), chromium (Cr), lead (Pb), and mercury However, all HMs are highly toxic to plants at high concentrations (White and Pongrac, 2017). The toxic levels of HMs adversely affect various metabolic processes. This may include, but not be limited to, degradation or displacement of protein structures resulting from the development of bonds between the HMs and sulfhydryl groups (Hall, 2002); disruption of cytoplasmic membrane integrity (Farid et al., 2013), and suppression of photosynthesis, respiration, and enzymatic actions (Hossain et al., 2012).
NPs are microscopic particles that can enter the cell through aboveground plant organs (cuticle, epidermis, stomata, hydathodes, or other openings) or underground organs (root tips, cortex, lateral root, wounds, or other openings). The physiological and morphological effects of NPs vary according to plant species, development period, development agents, application method, dose, and exposure time (Dietz and Herth, 2011; Rizwan et al., 2017). According to the mass flow/pressure flow hypothesis, NPs that enter through the stomata are carried within the plant by the phloem and are transported via pressure differences between the leaves and roots (Turgeon, 2010). The route through which NPs enter the plants alters many plant processes, including germination, antioxidant activity, macro and micronutrients, chlorophyll content, chloroplast number, and photosynthesis (Cinisli et al., 2019). In Arabidopsis, NPs application altered intraroot signals by affecting ethylene production (Syu et al., 2014). NPs can penetrate the cell membrane and the cell wall in order to be transported to the epidermis, xylem, central cylinder, and leaves (Tripathi et al., 2017a). Before reaching the central cylinder, NPs are passively transported in the endodermis (Judy et al., 2012). NPs are transported via the active route through osmotic pressure, capillary forces, cell wall pores, and plasmodesmata in plant roots or via the symplastic route (Usman et al., 2020). In general, NPs can bind to carrier proteins via ion channels, aquaporin, and endocytosis, as well as disrupt the plasma membrane to induce the formation of pores for crossing into the cells. The passage of NPs through the cell wall relies on their pore size. Small-sized NPs simply pass through the cell wall (Fleischer et al., 1999), while larger NPs pass through the hydathodes, stigma, and stomata (Hossain et al., 2016). NPs are transported via the stomata when their dimensions are < 15–40 nm (Eichert et al., 2008). Such NPs can act as a substitute for the vascular cambium, in the stomata and be transferred to various plant compartments through the phloem (Tripathi et al., 2017a). The NPs that are widely used in agriculture and their role in enhancing crop tolerance to abiotic stress (Figure 3) are summarized in Table 1. In seed coating, NPs enter through parenchymatous intercellular spaces in the seed coat in which aquaporins play an important role in controlling NPs entry (Abu-Hamdah et al., 2004; Lee et al., 2010).
Figure 3. Effects of nanoparticles on plant health, growth performance and physiological parameters. WUE, water use efficiency.
Table 1. Most commonly used nanoparticles in agriculture and their impacts in enhancing crop tolerance to abiotic stress.
With the emergence of new nanotechnological applications, the use of nanomaterials with a high surface area-to-volume rate has increased. The functions and usage of nanomaterials differ according to the size and structure of NPs (Tunca, 2015). When nanomaterials are used as a biofertilizer, plants are provided with nutrients slowly, small amounts are sufficient (in contrast to chemical fertilizers), and the environmental risks caused by chemical fertilizers are minimized (Cinisli et al., 2019; Usman et al., 2020). The chemical pesticides and fertilizers adversely affect ecosystems and human health, particularly when large doses are used to increase plant yield. Therefore, it has become desirable to replace conventional pesticides and fertilizers with nanopesticides and nanofertilizers, to reduce the use of chemical fertilizers, increase plant yield, and support agricultural development (Bratovcic et al., 2021). Thus, these nanopesticides and nanofertilizers are now receiving increasing research attention (Kah, 2015). Nanofertilizers vary in size (30–40 nm), pass through the stomata, bind to different ions, and release nutrients (Bal, 2019; Cinisli et al., 2019). In general, nanofertilizers affect plant growth and metabolism by improving soil quality and plant growth performance, increasing growth hormone production and enhancing resistance to biotic and abiotic stresses (Cinisli et al., 2019; Saad et al., 2021; El-Ashry et al., 2022; Khairy et al., 2022). Nanopesticides can be produced using physical, chemical, or biological methods. Nanopesticides and nanoformulations, including those incorporating silver (Ag), Cu, silica (SiO2), and zinc oxide (ZnO), exhibit an improved range of pesticide efficacy compared with conventional pesticides; thus, nanopesticides positively influence the control of plant pests and diseases (Chhipa, 2017). Chitosan-metal oxide NPs have been used to ensure that the fertilizers applied to plants are taken up more effectively. The application of chitosan increases the enzyme activity of nitrate reductase, glutamine synthetase, and protease during N metabolism, thereby affecting plant growth and development (Bal, 2019). In peanut and corn plants, ZnO NPs increase the germination percentage and improve seedling development (Prasad et al., 2012; Singh et al., 2017). In addition, treatment with 2000 mg L–1 of 60-nm aluminum (Al) NPs for 5 days reduced the root length of corn seedlings and did not exert any adverse effects on Lolium perenne, Raphanus sativus, Cucumis sativus, Brassica napus, and Lactuca sativa (Yang and Watts, 2005).
Application of 2000 mg L–1 of Zn NPs considerably hindered root development in maize and stopped the root growth of Brassica oleracea, C. sativus, Daucus carota, and Glycine max (Lin and Zhing, 2007). Few experiments have addressed the influence of NPs on seed germination and seedling growth. However, the application of NPs on seeds generally increases seed germination, seedling development, seedling viability, and emergence rate (Abbasi Khalaki et al., 2021). Seed germination, root and shoot length, and fresh weight (FW) and dry weight (DW) values of Agropyron elongatum were positively affected by SiO2 NPs application (Azimi et al., 2014). Ag NPs have been shown to increase the germination level, length of the roots and shoots, FW and DW, average germination time, and vitality indices in Thymus kotschyanus (Abbasi Khalaki et al., 2016). Similarly, Ag NPs have been found to increase the germination rate in Pennisetum glaucum (Parveen and Rao, 2015) and Festuca ovina (Abbasi Khalaki et al., 2019a). However, Ag NPs can reportedly adversely affect the germination of Brassica nigra (Amooaghaie et al., 2015) as well as the shoot length of Medicago sativa, the root length and shoot DW of Ocimum basilicum, and the shoot and root length of Hordeum vulgare, Linum usitatissimum, and L. perenne (El-Temsah and Joner, 2010; Ramezani et al., 2014; Yosefzaei et al., 2016).
In Onobrychis sativa, SiO2 NPs increased shoot length, whereas titanium dioxide (TiO2) NPs increased germination time and percentage (Moameri et al., 2018a). Iron oxide (Fe2O3) NPs increased the germination of L. perenne (Wang H. et al., 2011). In addition, FeO NPs reduced the mycorrhizal biomass and the shoot and root length of Trifolium repens (Feng et al., 2013), Satureja hortensis (Peyvandi et al., 2011a), H. vulgare, and L. perenne (El-Temsah and Joner, 2010). Studies have shown that TiO2 NPs can positively affect the germination of Foeniculum vulgare, and Petroselinum crispum (Dehkourdi and Mosavi, 2013; Feizi et al., 2013). Ag NPs increased the shoot length and chlorophyll content of Brassica juncea and Sorghum bicolor (Namasivayam and Chitrakala, 2011; Sharma et al., 2012b). The root development of T. kotschyanus and Alopecurus textilis was positively affected by SiO2 NPs application (Abbasi Khalaki et al., 2019a,b). Similarly, SiO2 application to M. sativa increased plant height, tiller count, yield, FW, and DW, chlorophyll content, and carotenoid levels (Ma and Yamaji, 2006; Zmeeva et al., 2017). Govorov and Carmeli (2007) reported that SiO2 NPs increase leaf FW and DW as well as chlorophyll content in O. basilicum, and also negatively affect shoot and root growth in S. bicolor, Stipa hohenackeriana, and Secale montanum (Lee et al., 2012; Moameri et al., 2018b; Moameri and Abbasi Khalaki, 2019). ZnO NPs increase biomass, root and shoot length, and chlorophyll content in many plant species (Peyvandi et al., 2011b; Raliya and Tarafdar, 2013; Najafi Disfani et al., 2017; García-López et al., 2018; Yuan et al., 2018). In addition, TiO2 NPs increased the essential oil content and yield of medicinal plants (Ahmad et al., 2018; Fazeli-Nasab et al., 2018). The application of copper oxide (CuO) NPs adversely affects the morphology, physiology, and biochemistry of H. vulgare, L. perenne, M. sativa, and Triticum aestivum (Lee et al., 2008; Atha et al., 2012; Ramezani et al., 2014; Shaw et al., 2014; Hong et al., 2016).
NPs application is important to mitigate the abiotic stress effects of salinity on plants. At the germination stage, the use of Ag NPs in Lathyrus sativus under salt stress improves germination percentage, shoot and root length, and seedling FW and DW; thus, this enhanced osmotic regulation and reduced the negative effects associated with salinity (Hojjat, 2019). Noman et al. (2020) found that applying Cu NPs to the soil reduced oxidative stress in wheat and significantly increased plant development and yield. The use of NPs in wheat not only enhances plant development but also improves germination performance under salt-stress conditions (Shi et al., 2016). Preapplication of Ag NPs to wheat seeds alters antioxidant enzyme activities, reduces oxidative damage, and elevates salt-stress tolerance in such plants (Kashyap et al., 2015). In addition, ZnO NPs are known to increase the DW of sunflowers under salt stress (Torabian et al., 2016). CeO NPs (100 and 200 mg kg–1) was found to enhance the physiological responses of B. napus under salt stress (100 mM NaCl). CeO NPs are also known to boost plant biomass in salt-stressed canola (Rossi et al., 2016). The application of Ag NPs to basil seeds under salt-stress conditions increases seed germination (Darvishzadeh et al., 2015; Hojjat and Kamyab, 2017). Ag NPs applied to S. hortensis increase plant resistance to salt stress while reducing salt-stress–induced effects on germination percentage and plant shoot length (Nejatzadeh, 2021). Furthermore, the use of Ag NPs in salt-stressed cumin plants substantially improves plant salt resistance (Ekhtiyari and Moraghebi, 2012). Finally, Askary et al. (2017) reported that Fe3O4 NPs protects mint plants from oxidative stress caused by increased NaCl content.
Drought is considered a major abiotic stress that can drastically limit crop production (Al-Ashkar et al., 2021; Roy et al., 2021). NPs application is an efficient method for alleviating the impact of drought on plants by increasing antioxidant enzyme activity, improving phytohormone levels, and affecting physiological properties. The use of analcite NPs in soil under hot, dry conditions has been shown to promote germination and plant growth in wheat (Hossain et al., 2021). In addition, the use of ZnO NPs in soybean seeds under arid conditions increases the germination percentage of the seeds (Sedghi et al., 2013). Under drought stress, the use of Cu and Zn NPs in wheat plants increases their antioxidant enzyme activity and relative moisture content, decreases thiobarbituric acid levels, affects reagent precipitation, stabilizes photosynthetic pigment levels in leaves, and reduces the effects of stress (Taran et al., 2017; Semida et al., 2021). In response to drought stress, SiO2 NPs application can increase shoot length and relative water content (RWC) in barley, while reducing superoxide radical formation and membrane damage (Turgeon, 2010).
Jaberzadeh et al. (2013) have reported that foliar usage of TiO2 NPs in wheat is effective to overcome the yield reduction caused by drought stress. Furthermore, the application of Cu NPs to maize increased leaf water content, plant biomass, and anthocyanin, chlorophyll, and carotenoid contents under arid conditions (van Nguyen et al., 2022). Ashkavand et al. (2015) reported that SiO2 NPs applied to hawthorn grown under drought stress reduced photosynthesis and stomatal conductivity. However, silicon (Si) NPs have been reported to ameliorate the effects of drought stress in bananas (Mahmoud et al., 2020). Under moderate drought conditions, foliar application of Si NPs to coriander resulted in optimum antioxidant capacity and essential oil yield (Afshari et al., 2021). Shallan et al. (2016) have reported that foliar application of SiO2 and TiO2 NPs can reduce the negative effects of drought stress on cotton plants under arid conditions. In chickpea plants, the application of Si NPs to the soil reduces the negative effects of drought by increasing the relative moisture content in the plants (Gunes et al., 2007). Si- and selenium (Se)-NPs can reportedly help in enhancing growth, improving ion selectivity in roots, and increasing the yield of rice under saline conditions (Badawy et al., 2021). Although drought stress increases the adverse effects of Cd in wheat, the application of ZnO NPs can reduce both Cd and drought stress (Khan et al., 2019).
Under HMs stress conditions, soil or foliar applications of NPs can eliminate the adverse effects of stress, improve plant development and photosynthesis, and reduce oxidative stress-induced toxicity. Therefore, the application of NPs contributes to in the remediation of HMs-contaminated environments. Under HMs stress conditions, the application of NPs to plants reduces the concentration of HMs in the soil, regulates the expression of HMs transfer genes in plants, increases the activity of plant antioxidant systems, improves physiological functions, and stimulates the production of protective substances such as root secretions, phytochelatin, and organic acids (Rui, 2021). The application of Si NPs on maize plants under arsenic (As) stress reduced the total chlorophyll, carotenoid content, and total protein content; in addition to mitigating the adverse effects of As stress on maximum quantum efficiency, photochemical quenching, and non-photochemical quenching of FS II (Tripathi et al., 2016). Soil application of TiO2 NPs can effectively limit Cd toxicity by enhancing the physiological parameters and photosynthetic rate in soybean plants; therefore, TiO2 NPs are vital to mitigate the effects of HMs-induced oxidative stress (Singh and Lee, 2016). When treated with SiO2 NPs, the activities of enzymes, such as ascorbate peroxidase (APX) and superoxide dismutase (SOD), increased; whereas the effects of oxidative stress were reduced in pea seedlings under Cr stress (Tripathi et al., 2015b). Furthermore, de Sousa et al. (2019) revealed that Si NPs can reduce Al toxicity by activating the antioxidant defense mechanism in maize plants. Konate et al. (2017) found that Fe3O4 NPs protected wheat against Cd-induced oxidative stress. Foliar applications of Se NPs to Chinese cabbage under Cd stress increased the biomass, plant height, leaf chlorophyll content, SOD levels, and plasma glutathione peroxidase (GPX) content, whereas the Cd and malondialdehyde (MDA) contents of the leaves were reduced (Zhang, 2019). Similarly, Si NPs alleviate the effect of Cd stress in rice (Wang et al., 2015). The combined use of foliar ZnO NPs and soil biochar in plants was found to be more effective against Cd stress (Rizwan et al., 2019a). Similarly, the coapplication of Fe NPs and biochar reduced the effects of Cd stress in rice (Hussain et al., 2019c). The use of FeO NPs in Cd-stressed wheat reduced the leaf electrolyte leakage ratio and Cd content in grains, while improving the antioxidant enzyme action and DW of the plants. Foliar application of Fe NPs is preferable over soil usage. Rahmatizadeh et al. (2019) also found that 20 mg L–1 of Fe3O4 NPs reduced Cd accumulation and improved Cd toxicity by increasing nutrient uptake in tomato plants.
Agrochemicals can be released in a controlled manner, and macromolecules can be delivered selectively. By incorporating nanoscale transporters and chemicals, the efficient use of fertilizers and pesticides can be improved, resulting in a reduction in the amount used without compromising the yield of crops. In contrast, commercial fertilizers, provide fewer benefits to plants because of their larger particle size and reduced solubility. In addition, repeated chemical fertilizer application result in a toxic build-up of HMs that disrupts the ecological balance in the soil. In addition, excessive application of chemical fertilizer can contribute to soil pollution due to leaching or being not fully utilized by plants; thus, the remaining is converted into insoluble salts in the soil.
Nanoagrochemicals play an important role in enhancing nutrient use efficiency and water quality management for sustainable agriculture. However, bioaccumulation and long-term exposure of NPs to plants may have a negative impact on edible plants and food chains (Rajput et al., 2020). According to Staroń et al. (2020), NPs can be taken up and deposited in the edible tissues of crop plants. The accumulation of NPs or metal ions in their natural state can disrupt plant physiological activities; affect the integrity of cellular and sub-cellular organelle organizations; and modify the content of proteins, lipids, and nucleic acids by creating hydroxyl radicals (Cota-Ruiz et al., 2018; Rajput et al., 2020). Overall, the wide-ranging applications of NPs may generate a slew of difficulties from an ecological, ethical, health, and safety standpoint (Rajput et al., 2018).
Until now, the potential negative effects of NPs on human health have been speculative and unsubstantiated (Staroń et al., 2020). By developing various NPs as new tools for the agriculture industry, nanotechnology has grown in popularity. There is an urgent need to increase our knowledge and understanding of the specific benefits and drawbacks associated with the use of NPs. The advancement of nanotechnology has resulted in significant amounts of manufactured NPs in the agroenvironment. Although this technology has numerous advantages, researchers and experts are concerned about the unsafe disposal of NPs in large quantities (several hundred tons) each year (Rajput et al., 2020).
The existence of NPs in a various controlled objects (atmospheric air, water objects, soils, hydrobionts, algae, fungi, tissues of land plants/animals) is recommended (Rajput et al., 2020). In comparison with other sources, the fate and movement of NPs in soil have undergone very little research. Simultaneously, the soil offers fundamental nutrients to food crops, which can also operate as NPs collector sink (Rajput et al., 2020). The current review sheds light on the potential impact of NPs on the environment, health, and food security.
Si-based materials and their oxides are found abundantly in the soil. Plants naturally contain high levels of Si (1–10%) as well. Si in plants is found in the form of amorphous silica (SiO2⋅nH2O) in the cell wall, providing it with strength and solidity, in addition to contributing to polyphenols and pectins as a reactant (Bhatt and Sharma, 2018). These substances are also active during plant defense and development. Because of their application in multiple agricultural fields, it has been reported that Si-based NPs can ameliorate abiotic stresses (Jeelani et al., 2020). However, little is known about the mechanisms by which Si alleviates stresses in plants (Ma, 2004; Liang et al., 2007; Datnoff et al., 2009). Si particles and Si NPs can increase tolerance to abiotic stress, nutrient element homeostasis, stimulation of antioxidant enzymes, and improved absorption, immobilization, and partition of metal ions (Liang et al., 2007; Monica and Cremonini, 2009; Qados, 2015). SiO2 NPs considerably enhance germination, development, and yield in plants under stress. This can be attributed to the uptake of these NPs via roots leading to the development of a thin layer in the cell wall helping plants to tolerate various stresses (DeRosa et al., 2010; Siddiqui et al., 2014). SiO2 NPs also increase the efficiency of water translocation, increase turgor pressure, and enhance relative water inclusion in leaves and water usage effectiveness in plants (Rawson et al., 1988; Wang and Naser, 1994). Sharifi-Rad et al. (2014) found that various concentrations of SiO2 NPs significantly promoted maize growth and affected different developmental stages. SiO2 NPs can also be involved in the regulation of protein and phenolics, which are important for the growth and development of Zea mays (Suriyaprabha et al., 2012). In addition, they found that a relatively high level of Si accumulated in roots would boost drought tolerance in maize.
Precipitated Si NPs within plant tissues are capable of increasing the expression of essential biochemical elements, improving development, and enhancing yield factors in maize (Suriyaprabha et al., 2012). Furthermore, the improved action of the enzymatic system, the build-up of nutrients, free Pro, amino acids, and water absorption are positive effects of NPs that improve stress tolerance in crops (Wang et al., 2015; Shalaby et al., 2016; Shojaei et al., 2019). Importantly, Si NPs can also increase plant tolerance to drought stress. Ashkavand et al. (2015) observed enhanced drought tolerance as well as retention of critical biochemical and physiological attributes in hawthorn seedlings following the application of SiO2 NPs under different levels of drought stress. Pretreatment with SiO2 NPs positively influences the photosynthetic rates, stomatal conductance, and augmented xylem water potential in hawthorn seedlings under drought stress. Large dosages of SiO2 NPs supplied with irrigation water before drought treatments mitigate drought stress effects on growth, and biochemical and physiological parameters of Prunus mahleb (Tripathi et al., 2015b). Improved drought tolerance, evident by an improvement in root development and retention of the photosynthetic ratio, was also reported in two cultivars of S. bicolor with varying drought sensitivities after the application of Si NPs. Thus, increase in drought resistance occurred regardless of the cultivar sensitivity to drought stress (Hattori et al., 2005).
Pei et al. (2010) noted that the use of an appropriate concentration of sodium silicate (i.e., 1.0 mM) could moderately diminish the negative effects of drought stress in wheat. In the same study, there was partial promotion of shoot development and chlorophyll content when Si was supplied. This also helped retain leaf water potential and reduced membrane lipid peroxidation in stressed plants (Pei et al., 2010). Under drought stress, Si deposition in plant cells could help reduce the transpiration ratio, and enhance the photosynthesis mechanism (Ali et al., 2012; Siddiqui et al., 2014). Thus, the effects of drought stress can be mitigated by various Si/SiO2 NPs applications in various plant species (Zargar et al., 2010). The improved performance of such NPs can be attributed to the increased absorption and/or penetration into plant tissues; however, the exact mechanisms are not yet understood (Ashkavand et al., 2015). Shallan et al. (2016) have reported that foliar sprays of TiO2 NPs (50 mg L–1) or SiO2 NPs (3200 mg L–1) increase the drought tolerance of cotton plants. In addition, Si can help plants acclimatize to various ecological stresses (Rastogi et al., 2019). Salinity stress restrains crop yield because of Na+ ion toxicity in approximately 23% of planted areas worldwide (Onaga and Wydra, 2016). However, the application of Si NPs and Si fertilizer under salinity stress has positive impacts on the physiological and morphological indices of vegetative characteristics in O. basilicum. This is evident from the remarkable enhancement in the developmental index, chlorophyll content, and Pro concentration. This, may be because of the involvement of NPs and Si fertilizers with increasing tolerance to salinity stress in plants (Kalteh et al., 2014). The use of SiO2 NPs has also been shown to enhance developmental parameters, chlorophyll content, Pro accumulation, and upregulation of antioxidant enzyme activity in tomato and squash plants under salinity stress (Haghighi et al., 2012; Siddiqui et al., 2014).
The application of SiO2 NPs improves not only seed germination and early seedling development but also other related characteristics in lentil genotypes under salinity stress. Thus, SiO2 NPs boost salt toxicity protection in plants (Sabaghnia and Janmohammadi, 2014). SiO2 NPs can also mitigate stress by reducing Na+ ion concentration, resulting in improved crop development, production, and survival under salinity stress (Savvas et al., 2009). The application of SiO2 NPs also increases FW in maize in response to salinity stress (Gao et al., 2006). Si NPs can improve plant development by reducing osmotic potential and Na+ toxicity associated with high salt stress (Raven, 1983). It has been reported that SiO2 NPs form a layer in the root cell wall that enables plants to tolerate several stresses (e.g., salinity) (DeRosa et al., 2010; Abdel Latef et al., 2018).
Wang et al. (2010) and Wang X. et al. (2011) and others have documented the capability of Si and SiO2 NPs in reducing the harmful effects of salt on plant development. Because of their small size, uptaking SiO2 NPs can be performed more efficiently than uptaking micro-SiO2, -Na2SiO3, or -H4SiO4 when added to maize roots and seeds (Suriyaprabha et al., 2012). The particles were subsequently used by plants to improve growth by affecting xylem humidity, water translocation, and increasing turgor pressure; which in turn, improves the RWC and water use efficiency (WUE). The enhancement of plant germination and developmental traits by SiO2 NPs may be associated with an enhanced K/Na ratio, which reduces Na+ uptake (Alsaeedi et al., 2018), and increases the expression of antioxidant enzymes (Torabian et al., 2016; Farhangi-Abriz and Torabian, 2018). According to Almutairi (2016a), it has been found strong interactions between the enhancement of seed germination and growth in tomato-stressed plants with high salt and the increased expression of salt tolerance genes when Si NPs are applied. In contrast to no treatment of Si NPs, Capsicum annuum plants showed increased growth when irrigated with saline water upon the application of Si treatments (Tantawy et al., 2015).
Several studies have demonstrated that nano-Si is effective in detoxifying HMs or reducing their toxic effects while promoting plant development under HMs stress (Shen et al., 2014; Keller et al., 2015). For instance, the toxicity of Cr in pea seedlings was alleviated by supplementing the growth media with Si NPs, which reduced oxidative stress by decreasing the precipitation of Cr and increasing antioxidant mechanisms (Tripathi et al., 2015a,b). In addition, Cui et al. (2020) have reported that SiO2 NPs application can reduce oxidative stress in As-exposed rice cell lines. Similarly, the foliar application of 2.5 mM nano-Si can markedly increase tolerance to Cd stress in rice through the regulation of Cd precipitation (Wang et al., 2015). Si NPs have also been shown to alleviate toxicity caused by Pb, Cu, Zn, and Cd HMs, and their use may be more effective at reducing HMs accumulation compared with traditional strategies (Wang et al., 2016). Liu and Lal (2015) demonstrated that Si NPs are more effective than bulk Si in reducing the detrimental effects of Pb on rice development. Si NPs prevent Pb movement from the rice roots to the shoots and reduce Pb precipitation in grains, especially in high-Pb-precipitating cultivars and in soils with high levels of Pb contamination. Si NPs can also reduce and chelate active HMs ions, stimulate antioxidant systems, enhance the complexation and coprecipitation of toxic metals with Si, and produce fundamental changes in plants by controlling the expression of metal transport genes. However, these processes are dependent on plant genotypes, plant species, metal elements, developmental requirements, and the duration of stress enforced. Therefore, Si-mediated reductions in metal toxicity might be generalized with caution (Adrees et al., 2015). According to studies conducted by Tripathi et al. (2015b), Si NPs are linked with mitigating the toxicity effects of Cr in Pisum sativum seedlings. Cr stress induces toxicity; however, Si NPs can protect pea seedlings from Cr (VI)-induced phytotoxicity by reducing Cr precipitation, enhancing the antioxidant defense system, and alleviating oxidative stress. Tripathi et al. (2016) have also evaluated the effects of Si NPs on alleviating As toxicity in a maize cultivars and hybrids. Hydroponic trials have shown that these NPs can considerably reduce As toxicity by increasing the levels of metabolites of the ascorbate–glutathione cycle, and decreasing the levels of oxidative stress indicators, resulting in reduced As precipitation in the Si NP-treated cultivars and hybrids. It has been hypothesized that Si NPs can be more effective than bulk Si particles for balancing ROS production and ameliorating ROS-mediated damage in treated plants. It has also been reported that Si NPs are more effective than Si in protecting plants against UV-B stress. In general, Si NPs may protect plants by activating their antioxidant defense mechanism and regulating ROS-induced oxidative stress (Tripathi et al., 2017b).
Ag NPs are widely used in the agricultural sector, particularly in crop enhancement, food packaging, coating of domestic products, and pesticides. Their use in electronics, drug delivery, and biological-tagging medicine is also relatively common (Bechert et al., 1999; Davies, 2008; Korkin and Rosei, 2008; Jo et al., 2009; Ahamed et al., 2010; Kim et al., 2012). Ag is toxic when used in high concentrations; however, when reduced to a nanosize of 25–50 nm, it has unique properties compared with bulk Ag (Bhatt et al., 2020). Owing to these unique features, Ag NPs can be applied to enhance the vigor of plants and boost their overall development, productivity, and photosynthetic rate (Sharma et al., 2012a; Hatami and Ghorbanpour, 2013; Vannini et al., 2013; Shelar and Chavan, 2015). Ag NPs can also be used as antimicrobial substances to manage diseases on plants (Lamsal et al., 2011). The effect of different concentrations of chemically produced Ag NPs was investigated in B. juncea seedlings, specifically on the development and antioxidant status of the plants. Ag NPs were capable of improving growth and inducing the activity of specific antioxidant enzymes, which reduced ROS levels, improved overall antioxidant status, and reduced Pro and MDA levels. The growth-improving effect of Ag NPs in plants under stress is concentration-dependent; where a 50-ppm dose was ideal to improve growth (Sharma et al., 2012b). In another study on tomatoes, Ag NPs-treated seeds germinated earlier than those treated with deionized water; however, seed germination was inhibited when higher concentrations of Ag NPs were applied (Karami Mehrian et al., 2016).
Ag NPs may also play a role in the expression of stress genes. For instance, the up- and down-regulation of certain genes by Ag NPs was observed in microarray analysis: upregulated genes were mostly associated with responses to metal toxicity and oxidative stress, whereas downregulated genes were associated with responses to microbes and hormonal stimuli (Banerjee and Kole, 2016). Such responses may be associated plant defense mechanisms under adverse conditions; however, additional studies are required to elucidate the signaling cascades and genes controlled by Ag NPs and other NPs in various plant species.
The effects of Ag NPs on the hydraulic conductivity of the plant stem during drought stress have been studied; however, such NPs might also be capable of entering plant cells and tissues and impairing regular cellular activities (Tripathi et al., 2017a). Hojjat and Ganjali (2016) found that Ag NPs can alleviate the effect of drought stress effects in lentil (Lens culinaris). MahdiNezhad et al. (2018) reported that Ag NPs can reduce the levels of antioxidant enzymes in plants under drought stress; thus, this reduction can be attributed to the reduced antioxidant metabolism. NPs may be directly involved in the elimination of ROS, which reduces the levels of antioxidant enzymes. Seghatoleslami et al. (2015) reported the effects of Ag NPs on the yields and WUE of drought-stressed Carum copticum using a magnetic field.
Ag NPs application is useful to reduce the effect of salinity stress–induced toxicity. This has been demonstrated in studies on the germination of tomato, fennel, and cumin plants treated with Ag NPs; thus, enhancing germination, improving developmental performance, and mitigating the negative effects of salt stress (Ekhtiyari and Moraghebi, 2011; Ekhtiyari et al., 2011; Almutairi, 2016b). Positive effects of different concentrations of Ag NPs suspension have been reported on the germination and development of Solanum lycopersicum under salinity stress (Delfani et al., 2014). In the same study, AREB, P5CS, MAPK2, and CRK1 were induced and TAS14, ZFHD1, and DDF2 were repressed when salt-stressed plants were exposed to Ag NPs. A comparative study of the toxicity revealed that Ag NPs or AgNO3 had negative effects on C. sativus seedlings grown at higher concentrations; however, Ag NPs were less toxic than AgNO3 and had the potential to improve C. sativus yield (Cañas et al., 2008). The role of Ag NPs in relieving salt stress in wheat and B. juncea has been assessed, and Ag NPs were found to efficiently alleviate the effects of salinity stress (Sharma et al., 2012b; Mohamed et al., 2017; Abou-Zeid and Ismail, 2018). Ag NPs at 50 and 75 mg L–1 concentrations can protect plants from heat stress and improve their development (Iqbal et al., 2019).
TiO2 is a typical oxide of titanium. As a metal, titanium is abundant in the Earth’s crust as well as found in plant and animal tissues. TiO2 and nano-TiO2 serve as UV blockers in sunscreens because they diminish the adverse effects of UV radiation. In addition, TiO2 NPs have photocatalytic sterilizing properties and can undergo redox reactions when subjected to light, resulting in the formation of superoxide anion radicals and hydroxide (Hong et al., 2005). Photosterilization by TiO2 NPs can promote photosynthesis and improve plant growth. The potential effects of TiO2 NPs on the photochemical responses of chloroplasts in spinach (Spinacia oleracea) were evaluated (Hong et al., 2005). TiO2 NPs treatment was found to improve the activities of SOD, catalase (CAT), and peroxidase (POD), decrease the accumulation of reactive oxygen free radicals and MDA levels, and maintain the stability of the membrane structure of chloroplast under the light. TiO2 NPs also play a role in plant biochemical processes, morphophysiological characteristics, and reactions to various stresses (Mishra et al., 2014). In S. oleracea, TiO2 NPs can increase antioxidant stress tolerance through decreasing superoxide radical precipitation, reducing stress indicator (H2O2 and MDA) levels, and stimulating antioxidant enzyme activities within the plants during the photochemical interactions in chloroplasts (Lei et al., 2008).
In spinach plants, nano-anatase TiO2 treatment markedly increased photosynthesis, electron transmission, photoreduction activity of photosystem II, oxygen evolution, and photophosphorylation of chloroplasts under visible and UV light illumination (Lei et al., 2007, 2008). In addition, the effects of TiO2 NPs on plant growth have been associated with enhanced photosynthetic rate and nitrogen metabolism (Yang et al., 2006). The photocatalytic degradation of pesticides by TiO2 has been demonstrated as a possible water remediation process (Lee et al., 2003). Moreover, TiO2 NPs increase plant water uptake and nitrogen use and stimulate antioxidant activity in canola (Mahmoodzadeh et al., 2013) and wheat (Jaberzadeh et al., 2013).
Several studies have confirmed the TiO2 NPs-mediated improvement of plant development. For instance, Changmei et al. (2002) found that TiO2 and SiO2 NPs positively affect seed germination and growth of G. max (Changmei et al., 2002). In addition, onion seedlings treated with TiO2 NPs increased the enzymatic activity of SOD, amylase, CAT, and POD (Laware and Raskar, 2014). Mohammadi et al. (2016) explored the potential effects of different concentrations of TiO2 NPs against drought stress in Dracocephalum moldavica. Foliar application of these NPs at higher concentrations (40 ppm) can reportedly alleviate the detrimental effects of drought stress by adjusting the level of antioxidant enzymes and oxidative stress indicators. TiO2 NPs have been reported to increase Rubisco activase activity, chlorophyll formation, and the photosynthetic ratio and plant dry mass (Gao et al., 2008). In Vigna unguiculata, seed yield increases with foliar application of NPs and TiO2. Thus, this could be attributed to the increase in photosynthetic rates (Owolade and Ogunleti, 2008). The activity of the antioxidant enzymes (POD and CAT) increases in response to TiO2 NPs; therefore, MDA precipitation also decreases (Ahmad et al., 2019). The ability of TiO2 NPs to alleviate the adverse effects of drought stress has been investigated in several studies. For instance, the foliar application of TiO2 NPs can promote growth and increase the yield of wheat under drought stress when TiO2 NPs (0.02%) has been used (Jaberzadeh et al., 2013).
TiO2 NPs also improved the ability of plants to capture sunlight in maize plants. Under drought stress, TiO2 NPs can affect the pigment formation, the transformation of light energy to the active electron, and chemical activity, thus, enhancing photosynthetic effectiveness in maize (Akbari et al., 2014). In a similar study, the effects of nano-TiO2 and -SiO2 on the biochemical components and productivity yield of drought-stressed cotton plants have also been tested (Shallan et al., 2016). In their findings, the pretreatment with nano-TiO2 or -SiO2 can improve the pigment content, antioxidant enzyme activity, and antioxidant capacity, and increase the yield of these plants. The optimum concentrations required to reduce the destructive effects of drought stress in cotton plants were 50 and 3200 ppm for nano-TiO2 and -SiO2, respectively. Foliar application of these NPs have also increased drought tolerance in cotton plants. Similar results have been obtained in drought-stressed L. usitatissimum treated with TiO2 NPs (Aghdam et al., 2016). The drought-stressed D. moldavica treated with TiO2 exhibited increased levels of Pro and considerably reduced levels of H2O2 and MDA compared with nontreated plants (Mohammadi et al., 2014). Thus, suggesting that TiO2 NPs can ameliorate stress-induced damage. TiO2 NPs significantly induced the antioxidant enzyme activity, and Pro and soluble sugar content, which in turn promoted osmotic balance in plant cells and growth recovery in plants (Abdel Latef et al., 2018). O. basilicum can tolerate drought stress owing to the combined effects of gibberellin and TiO2 (Hatami, 2017). Overall, the application of nano-TiO2 can alleviate stresses of HMs, light, cold, and heat.
Singh and Lee (2016) have shown that the application of TiO2 NPs can reduce Cd toxicity and enhance the photosynthetic rate in soybean (Singh and Lee, 2016). TiO2 NPs also play an important role in alleviating light stress. When subjected to light, these NPs catalyzed the redox reaction, thereby generating superoxide anion radicals and hydroxide (Khan and Siddiqui, 2018). The addition of TiO2 NPs reduced the impact of heat stress by controlling stomatal opening (Qi et al., 2013). TiO2 NPs also positively affect plant growth and development. The positive effects of NPs, including TiO2-NPs, include enhancement of the carboxylation of Rubisco (Gao et al., 2006), light absorption capabilities of chloroplasts (Ze et al., 2011), electron transport rates, and prevention of ROS formation (Giraldo et al., 2014). The use of nano-TiO2 increases the expression level of genes encoding Rubisco- and chlorophyll-binding proteins (Hasanpour et al., 2015) as well as the activity of antioxidant enzymes (Mohammadi et al., 2014); thus maintaining stable contents of chlorophyll and carotenoids, and improving tolerance to cold conditions. Furthermore, TiO2 NPs positively affect susceptible (ILC 533) and resistant (Sel 11439) genotypes of chickpea under cold stress. Under such stressful conditions, TiO2 dramatically reduced membrane damage indices, such as ion leakage index and MDA levels, resulting in reduced damage to the membrane (Mohammadi et al., 2013). TiO2 treatments can also hinder oxidative damage in chickpea and reduce membrane damage under cold stress; suggesting that TiO2 NPs can ameliorate the redox status under heat exposure (Mohammadi et al., 2014). It has been proposed that TiO2 NPs improves tolerance to cold stress by enhancing the mechanisms of protection and reducing the levels of injuries in chickpea plants. Future studies may confirm the effectiveness and mechanisms of TiO2 NPs in improving the tolerance of crops to cold stress.
In plants, Zn is a critical micronutrient that regulates metabolic processes and facilitates development (Adhikary et al., 2010; Vitti et al., 2014). Zn also plays an important role in the survival of plants under adverse conditions. Plants use Zn in small amounts; therefore, accessibility of Zn at the nano level ensures that suitable amounts are transported to the plant while avoiding Zn toxicity in the plants and soil. ZnO is an ecofriendly compound that can be used as a “green” element (Pandey et al., 2010). Given its functions in maintaining membrane integrity, retaining the potassium content of cells, and the plant–water relationship, ZnO plays a major role in stomatal regulation (Khan et al., 2004). In a study on chickpeas, Zn deficiency decreased their ability to modulate osmotic pressure under drought stress (Khan et al., 2004). Auxin production can also be affected by Zn via the induction of tryptophan synthesis as a precursor for the production of indole acetic acid, which helps in root development and drought tolerance in plants (Waraich et al., 2011). The uptake of Zn can be improved when it is nano-sized; thus, the functions of Zn can be achieved more efficiently when using Zn NPs. The application of Zn NPs enhances radicle development in germinated seeds, and higher Zn content in grains; thereby improving seed survival and tolerance to environmental stresses, especially in Zn-deficient regions (Cakmak et al., 1996; Degenhardt and Gimmler, 2000; Cakmak, 2008).
Several studies have described the effects of Zn-based NPs on plant development and yield. The use of ZnO NPs, at appropriate concentrations, enhanced biomass production, seed germination, and seedling development in chickpeas, in contrast to the use of bulk ZnSO4. ZnO NPs can elevate auxin levels, and thus, promote plant development (Pandey et al., 2010; Burman et al., 2013). In another study, the stimulating effect of zinc sulfide (ZnS) NPs on the growth of B. juncea has been assessed (Nayan et al., 2016). They showed that chlorophyll content, sugar content, and plant biomass, increased significantly after the application of these NPs, and that the growth-stimulating effects were probably associated with improvements in the plant antioxidant system after ZnS NPs treatment. Furthermore, lower concentrations of ZnS NPs were more effective than higher concentrations in improving plant growth. Similar results have been reported in wheat plants treated with ZnO NPs at 66 mg L–1 (Awasthi et al., 2017). ZnO NPs can mediate the increases in photosynthetic pigments and a concomitant reduction in lipid peroxidation in soil-grown Coriandrum sativum plants (Bhatt et al., 2020). Thus, the ZnO-mediated NPs increases the photosynthetic pigments which may help plants cope with stressful conditions by stabilizing ROS generation. Sedghi et al. (2013) have reported that the germination ratio in soybean was potentially augmented by ZnO NPs under water-deficient conditions. Under drought stress, the applied ZnO NPs facilitate the rapid use of seed reservoirs for seedling development and reduced the effects of such stress (Sedghi et al., 2013).
Drought tolerance was also improved by the enhancement of antioxidant enzyme activity in wheat plants via ZnO NPs application (Yang et al., 2018). Both Cu NPs and Zn NPs can improve wheat plant tolerance to drought stress by enhancing the action of antioxidant enzymes and stabilizing the content of photosynthetic pigments (Taran et al., 2017). Seghatoleslami and Forutani (2015) have shown that biomass and WUE have been improved by ZnO NPs in plants under water stress, whereas plants provided with full irrigation achieved strong growth and yield with bulk ZnO treatment. Dimkpa et al. (2017, 2019) have noticed that a composite of ZnO, boric oxide, and CuO NPs can alleviate drought stress in G. max. Under drought stress, shoot development and grain production were enhanced by 33 and 36%, respectively, using these NPs; thus, crop productivity and uptake of P and N can be enhanced by the addition of micronutrient NPs. These findings are in agreement with those reported in another study in which ZnO NPs were demonstrated to mitigate drought-induced damage to sorghum productivity, grain fortification, and nutrient acquisition (Dimkpa et al., 2019).
Yang et al. (2018) found that remodeling of root shape by ZnO and CuO NPs could alter drought tolerance in T. aestivum plants colonized by Pseudomonas chlororaphis O6 (PcO6), a beneficial bacterial species. Zn NPs enhanced the formation of lateral roots, whereas Cu NPs stimulated the propagation of elongated root hairs close to the root tip in wheat seedlings. In the same study, the use of these NPs generally increased the expression of genes related to drought tolerance. In shoots, the expression of other genes, such as those associated with metal stress, increased, and this was consistent with the increases in Cu and Zn concentrations. Thus, plants that were subjected to CuO or ZnO NPs showed cross-protection to multiple challenges, including metal, and drought stress. Despite improvements in root hair formation and production of lateral roots caused by Cu NPs and Zn NPs, respectively, the decreased root length may be the reason for the reduction in water accessibility. In Arabidopsis and mustard, the increased lignification because of CuO may alter the water flow and restrict cell wall expansion. Lignification in the cell wall is a plant response that is associated with drought stress and water flow impairment; thus, this may also occur by the binding of Cu ions with cell wall pectins (Nair and Chung, 2014).
When exposed to CuO NPs, anthocyanin and Pro levels increased under water deficient stress. On the addition of CuO NPs, the precipitation of ROS improved in the roots of wheat. The increased levels of ROS and abscisic acid (ABA) due to drought stress may cause transcriptional changes, resulting in subsequent stress tolerance (Dimkpa et al., 2012). Alharby et al. (2016) have investigated the metabolic response of S. lycopersicum to ZnO NPs under salinity stress; and they found that the NPs can reverse the adverse effects of salinity stress by regulating tolerance-related proteins/enzymes, mainly through the upregulation of SOD and GPX gene expression. These results are consistent with those of Haripriya et al. (2018), who found that a foliar spray of ZnO NPs mitigates salinity stress effects in finger millet. ZnO NPs treatment in soil-grown sorghum can also improve drought-stress tolerance through the translocation of grain N and the restoration of total N content (Dimkpa et al., 2019). In contrast, ZnO NPs at concentrations ≥ 10 mg L–1 resulted in oxidative stress in tomato plants cultivated in 1/2 Murashige and Skoog media (Rédei, 2008). The differences in results could be attributed to the variation in ZnO NPs, levels of NPs used, plant development media used, and possible variation in plant liability to ZnO NPs.
ZnO NPs also reduced HMs stress by decreasing the uptake of HMs by plants; thus protecting plants from HMs toxicity (Baybordi, 2005; Venkatachalam et al., 2017). The symptoms of oxidative stress caused by Cd and Pb toxicity can be improved by ZnO NPs treatment. In addition, ZnO NPs can improve the total soluble protein and photosynthetic pigment levels, while reducing lipid peroxidation in developing seedlings of Leucaena leucocephala (Venkatachalam et al., 2017). When a foliar spray of ZnO NPs was applied to maize leaves, Cd absorption and Cd-induced oxidative stress were reduced (Rizwan et al., 2019c). Torabian et al. (2016) reported an increase in plant growth, photosynthetic index, and chlorophyll content and a decrease in the Na content in sunflower leaves supplied with ZnO NPs. Similarly, wheat plants treated with CuO/ZnO NPs showed improved growth, which could possibly due to the lower solubility of CuO NPs (Fathi et al., 2017). Taken together, these findings indicated that the application of Zn-based NPs enhanced plant stress tolerance.
Nanotechnology has several other possible applications and can play an important role in agriculture, forestry, energy production, food processing, environmental management as well as in ensuring water quality and utilizing waste resources (Prasad et al., 2017; Kim et al., 2018). The extensive scope of nanotechnology and its wide range of applications has led to advancements in the agricultural sector (Kim et al., 2018; Shang et al., 2019). Over the last two decades, the use of nanotechnology in agriculture has been supported by research and practical applications at the academic and industrial levels (Shang et al., 2019).
In particular, nanotechnology has been applied to increase crop production. In addition, nanotechnology has been used to produce nanofertilizers and nanoencapsulated nutrients, which are considered promising methods for achieving site-targeted and regulated delivery of nutrients to plants, thereby improving crop production and yield via “precision agriculture.” Nanoformulations of agrochemicals, such as nanopesticides and nanofertilizers, substantially reduce micronutrient losses of fertilizers through volatilization and leaching, enhance effective phytoavailability, feed plants gradually in a controlled manner, and eventually reduce environmental hazards caused by the excessive use of traditional fertilizers (Solanki et al., 2015; Shang et al., 2019; Zulfiqar et al., 2019). Nanofertilizers can be produced using Cu, SiO2, Zn, TiO2, and polymeric NPs as dendrimers acting as nanocarriers (Paramo et al., 2020).
Studies have shown that nanofertilizers can help crop productivity by improving stress tolerance as well as promoting plant germination, growth, and physiological processes. However, nanofertilizers have some drawbacks that have restricted their extensive application (Zulfiqar et al., 2019). Nanosensors have been reported as another application of nanotechnology that can improve crop quality and yield, while ensuring an output of high-quality and healthy food products. Novel nanosensors are primarily applied in crop safety for the detection and management of phytopathogens and for measuring and monitoring the uses, penetration, and residues of agrochemicals as well as environmental pollution (Ion et al., 2010; Chen et al., 2016; Prasad et al., 2017; Paramo et al., 2020). Their use has advanced the human management of soil and plant health, improved food quality, and protection, optimized packaging methods, and enhanced soil monitoring and crop conditions (Kim et al., 2018; Shang et al., 2019). Other agronomic uses of nanotechnology include the use of nanodevices in plant genetic engineering, postharvest management, and plant disease diagnostics (Ion et al., 2010; Prasad et al., 2017). Nanobiotechnology includes the use of novel methods to genetically modify and engineer crop programs that boost agricultural productivity, food safety, and processing capacity while promoting agricultural sustainability.
Different methods for the application of NPs in agriculture are shown in Figure 4. The application of nanomaterials enable efficient plant transformation for crop improvement (Anderson et al., 2016; Shafiee-Jood and Cai, 2016). Given their unique properties of small size, multiple binding sites and large surface area, nanomaterials are excellent nanocarriers of bioactive molecules (e.g., plasmid DNA and double-stranded RNA) (de Oliveira et al., 2014; Anderson et al., 2016; Shafiee-Jood and Cai, 2016; Kim et al., 2018; Zhao et al., 2020). Engineered NPs can also be used to increase crop safety and detect pesticide residues (Kim et al., 2018). Moreover, nanotechnology has become a common method used by engineers and designers to enhance and improve soil properties. Nano clay–polymer composites and nano-zeolites can be used in the soil to improve its moisture content, increase water-retention capacity, and slow water release during the cultivation season, and nanomagnets have been used to expel soil contaminants (Vundavalli et al., 2015; Prasad et al., 2017; Kim et al., 2018).
The application of nanofertilizers can also help reduce soil toxicity caused by an accumulation of chemical substances applied to the soil, while also acting as an alternative means of enhancing resource-use efficiency (DeRosa et al., 2010; Nair et al., 2010; Jalil and Ansari, 2019). In addition, nanosensors are now widely used in agriculture for soil analysis, water management and transmission, environmental monitoring of pollution in soils and water, and pesticide and nutrient drop (Ion et al., 2010). Various sensors based on nanodetection technology, including biosensors, optical sensors, electrochemical sensors, and other instruments, are important tools for identifying HMs at trace levels (Ion et al., 2010; Prasad et al., 2017; Singhal et al., 2022).
Although NPs have a wide range of applications in agriculture, the majority of NPs are hazardous to plants when present in high concentrations. The uptake, accumulation and interference of NPs with key metabolic processes in different plant tissues may have positive or negative effects on plants, depending on their dosage, movement, characteristics, and reactivity.
High concentrations of NPs can penetrate plant cells and cross the plasma membrane; thus, this may interfere with key cellular activities (Mazumdar and Ahmed, 2011; Mirzajani et al., 2013). NPs can reach plant tissues through the root system or above-ground parts such as root junctions and wounds. As a carrier, NPs must pass through several physiological barriers until they are taken up by the plant and translocated. Plant cell walls, which are made up of cellulose, allow small NPs, ranging between 5 and 20 nm in size, to pass through into the plant cells (Dietz and Herth, 2011).
Some NPs have been shown to develop larger pores in the cell wall to enter the cell (Navarro et al., 2008; Kurepa et al., 2010). NPs can be transferred to other plant tissues via the apoplastic and symplastic pathways (Etxeberria et al., 2006; Ma et al., 2010). Wong et al. (2016) suggested a lipid exchange mechanism for NPs transport into plant cells. The size, magnitude, and zeta potentials of NPs are important to determine their delivery in plant cells.
NPs may affect plant metabolism by delivering micronutrients (Liu and Lal, 2015), gene regulation (Nair and Chung, 2014), and interfering with several oxidative processes in plants (Hossain et al., 2015). Excessive contents of NPs can generate ROS; thus, interfering with the oxidative mechanism; while other pathways have yet to be deciphered. The NPs can disrupt the electron transport chain in mitochondria and chloroplast, causing an oxidative burst and an increase in ROS levels (Pakrashi et al., 2014; Cvjetko et al., 2017). The rate of carbon fixation is reduced in response to stressful conditions; thus, this increases photoinhibition, potentially leading to the overproduction of superoxide anion radicals and H2O2 in the photosystem (Foyer and Noctor, 2005). When ROS is generated as a result of NPs, all biological components are affected causing protein changes, lipid peroxidation, and DNA damage (Van Breusegem and Dat, 2006).
Several studies have found an increase in lipid peroxidation and DNA damage in plants while interacting with NPs (Atha et al., 2012; Saha and Dutta Gupta, 2017). The increase in ROS levels can cause apoptosis or necrosis, resulting in plant cell death (Faisal et al., 2013). Despite its destructive nature, ROS play a role in biological activities, including stress tolerance (Sharma et al., 2012a). The balance between ROS generation and scavenging determines whether ROS has a destructive or signaling function. The cells have developed a robust antioxidant mechanism to precisely control the quantity of ROS. Enzymatic (SOD, CAT, and guaiacol peroxidase) and non-enzymatic (ascorbate, glutathione, carotenoids, tocopherols, and phenolics) antioxidants are attributed to defense mechanisms in plants (Sharma et al., 2012b). Several studies have demonstrated that plants exposed to NPs produce more antioxidant molecules (Jiang et al., 2014; Costa and Sharma, 2016). Plant stress response signaling can also be influenced by phytohormones (Mengiste et al., 2010; O’Brien and Benková, 2013; Sham et al., 2019).
Plant hormones are endogenous molecules involved in the regulation of plant development and stress tolerance (Sham et al., 2017). In response to abiotic stresses, different hormonal pathways can be activated or suppressed (Kwak et al., 2006; O’Brien and Benková, 2013). In red pepper (Capsicum annuum), cytokinin levels increased in response to AgNPs stress; while in cotton (Gossypium sp.), a decrease in the levels of auxins and ABA in response to CuO NPs was detected. This suggests that NPs affect plant hormonal balance and plant metabolism.
Several studies have demonstrated that NPs can also affect the content and activity of photosynthetic pigments in plants (Perreault et al., 2014; Tripathi et al., 2017c). High concentrations of NPs have a negative impact on photosynthesis, resulting in growth retardation or death in plants (Tripathi et al., 2017c).
Nanobiotechnology has the potential to improve stress tolerance, stress sensing/detection, targeted delivery and controlled release of agrochemicals, transgenic events, and seed nanopriming in plants (Wu and Li, 2022). Such nanomaterials free of HMs and high dispersibility can be developed for agricultural use. Future research on evaluating the biological effects of nanozymes i.e., Mn3O4 NPs in plants under stress conditions should be on top of our priorities. Mechanisms underlying nanopriming-induced seed germination, breaking seed dormancy, and their interactions with seeds have to be investigated. Understanding how NPs improve plant stress tolerance will enable researchers to design tailor-made nanomaterials targeting agricultural challenges. In addition, nanomaterials have no doubt a bright future ahead, especially when it comes to their functionality in plants. For example, Santana et al. (2020) have developed a targeted delivery approach using nanomaterials to convert chloroplasts into “chloroplast factory” for better plant photosynthesis under low light conditions. The use of nanomaterials for CRISPR-Cas genome editing in cargo delivery (Demirer et al., 2021) will increase the efficiency of genetic engineering to enhance plant stress tolerance. Developing policies and regulations could help manage biosafety hazards associated with the use of nanomaterials in agriculture. We believe that nanomaterials will play a crucial role in the future of agriculture.
To achieve sustainable agriculture, the research community must identify appropriate ecofriendly solutions that address abiotic-stress–induced loss of crop yield (Figure 3). Nanotechnology is an innovative and effective means of promoting crop yield and quality, enhancing the farming sector, and managing global food demand. The potential role played by several NPs in alleviating abiotic stress-induced damage and improving plant development and crop yield is under intense investigation. NPs, such as TiO2, SiO2, and Ag NPs, can reduce the negative effects of abiotic stress by activating plant defense mechanisms via the induction of ROS production and phytotoxicity. NPs, given their small size, can also easily penetrate plant tissues, after which they positively influence plant morphological, physiological, and biochemical processes, promote plant development, and improve crop productivity in plants under various abiotic stresses. Moreover, NPs have a large surface area that improves the absorption and delivery of various targeted nutrients. Nevertheless, the applications of NPs in crop improvement and sustainable agriculture are still at an early stage of development, and the current research in the field is insufficient and, to some extent, inconsistent (Rajput et al., 2021). Therefore, additional investigations must explore the following issues, which will help limit the undesirable effects of NPs on ecosystems and crops: (a) the reaction of NPs with plants and metabolic process at the molecular and cellular levels, and optimization of NPs size and level before practical application in the field; (b) the effects of NPs and their possible toxicities in different plant species; (c) the impact of NPs on gene regulation and expression in plants under various abiotic stresses; (d) the behavior and fate of NPs in plants and the environment; (e) the effects of soil properties and different plant species on the efficiency of NPs; (f) the classification of NPs as stress initiators or stress in activators; and (g) the combined effects of NPs with other active ingredients and biotic stresses in plants.
All authors listed have made a substantial, direct, and intellectual contribution to the work, and approved it for publication.
This project was funded by Abu Dhabi Education and Knowledge (Grant No. 21S105) to KE-T and Khalifa Center for Genetic Engineering and Biotechnology-UAEU (Grant No. 31R286) to SA.
The authors would like to thank their respective institutions for their support. KE-T would also like to thank the library at Murdoch University, Australia, for providing the valuable online resources and comprehensive databases.
The authors declare that the research was conducted in the absence of any commercial or financial relationships that could be construed as a potential conflict of interest.
All claims expressed in this article are solely those of the authors and do not necessarily represent those of their affiliated organizations, or those of the publisher, the editors and the reviewers. Any product that may be evaluated in this article, or claim that may be made by its manufacturer, is not guaranteed or endorsed by the publisher.
Abbasi Khalaki, M., Ghorbani, A., and Dadjou, F. (2019a). Influence of nanopriming on Festuca ovina seed germination and early seedling traits under drought stress in laboratory condition. Ecopersia 7, 133–139.
Abbasi Khalaki, M., Ghorbani, A., Esmali Ouri, A., Shokouhian, A. A., and Jafari, A. A. (2019b). Varying the vegetative and morphological traits of Thymus kotschyanus L. submitted to potassium silicate nanoparticles, superabsorbent hydrogel, effective microorganisms and animal manure. J. Biosci. 35, 115–125. doi: 10.14393/BJ-v35n1a2019-41832
Abbasi Khalaki, M., Ghorbani, A., and Moameri, M. (2016). Effects of silica and silver nanoparticles on seed germination traits of Thymus kotschyanus in laboratory conditions. J. Rangel. Sci. 6, 221–231.
Abbasi Khalaki, M., Moameri, M., Asgari Lajayer, B., and Astatkie, T. (2021). Influence of nanopriming on seed germination and plant growth of forage and medicinal plants. Plant Growth Regul. 93, 13–28. doi: 10.1007/s10725-020-00670-9
Abd El-Ghany, W. A., Shaalan, M., and Salem, H. M. (2021). Nanoparticles applications in poultry production: an updated review. Worlds Poult. Sci. J. 77, 1001–1025. doi: 10.1080/00439339.2021.1960235
Abd El-Mageed, T. A., El-Sherif, A. M. A., Abd El-Mageed, S. A., and Abdou, N. M. (2019). A novel compost alleviate drought stress for sugar beet production grown in Cd-contaminated saline soil. Agric. Water Manag. 226:105831. doi: 10.1016/j.agwat.2019.105831
Abd El-Mageed, T. A., Rady, M. O. A., Abd El-Wahed, M. H., Abd El-Mageed, S. A., Omran, W. M., Aljuaid, B. S., et al. (2022). Consecutive seasonal effect on yield and water productivity of drip deficit irrigated sorghum in saline soils. Saudi J. Biol. Sci. 29, 2683–2690. doi: 10.1016/j.sjbs.2021.12.045
Abd El-Mageed, T. A., Semida, W. M., Taha, R. S., and Rady, M. M. (2018). Effect of summer-fall deficit irrigation on morpho physiological, anatomical responses, fruit yield and water use efficiency of cucumber under salt affected soil. Sci. Hortic 237, 148–155. doi: 10.1016/j.scienta.2018.04.014
Abdel Latef, A. A. H., Alhmad, M. F. A., and Abdelfattah, K. E. (2017). The possible roles of priming with ZnO nanoparticles in mitigation of salinity stress in lupine (Lupinus termis) plants. J. Plant Growth Regul. 36, 60–70. doi: 10.1007/s00344-016-9618-x
Abdel Latef, A. A. H., Srivastava, A. K., El Sadek, M. S. A., Kordrostami, M., and Tran, L. S. P. (2018). Titanium dioxide nanoparticles improve growth and enhance tolerance of broad bean plants under saline soil conditions. Land Degrad. Dev. 29, 1065–1073. doi: 10.1002/ldr.2780
Abou-Zeid, H., and Ismail, G. (2018). The role of priming with biosynthesized silver nanoparticles in the response of Triticum aestivum L to salt stress. Egypt. J. Bot. 58, 73–85. doi: 10.21608/ejbo.2017.1873.1128
Abu-Hamdah, R., Cho, W. J., Cho, S. J., Jeremic, A., Kelly, M., Ilie, A. E., et al. (2004). Regulation of the water channel aquaporin-1: isolation and reconstitution of the regulatory complex. Cell Biol. Int. 28, 7–17. doi: 10.1016/j.cellbi.2003.11.003
Adhikary, B. H., Shrestha, J., and Baral, B. R. (2010). Effects of micronutrients on growth and productivity of maize in acidic soil. Int. Res. J. Basic Appl. Sci. 1, 8–15.
Adrees, M., Ali, S., Rizwan, M., Zia-Ur-Rehman, M., Ibrahim, M., Abbas, F., et al. (2015). Mechanisms of silicon-mediated alleviation of heavy metal toxicity in plants: a review. Ecotoxicol. Environ. Saf. 119, 186–197. doi: 10.1016/j.ecoenv.2015.05.011
Adrees, M., Khan, Z. S., Ali, S., Hafeez, M., Khalid, S., Rehman, M. Z. U., et al. (2020). Simultaneous mitigation of cadmium and drought stress in wheat by soil application of iron nanoparticles. Chemosphere 238:124681. doi: 10.1016/j.chemosphere.2019.124681
Afshari, M., Pazoki, A., and Sadeghipour, O. (2021). Foliar-applied silicon and its nanoparticles stimulates physio-chemical changes to improve growth, yield and active constituents of coriander (Coriandrum sativum L.) essential oil under different irrigation regimes. Silicon 13, 4177–4188. doi: 10.1007/s12633-021-01101-8
Aghdam, M. T. B., Mohammadi, H., and Ghorbanpour, M. (2016). Effects of nanoparticulate anatase titanium dioxide on physiological and biochemical performance of Linum usitatissimum (Linaceae) under well-watered and drought stress conditions. Braz. J. Bot. 39, 139–146. doi: 10.1007/s40415-015-0227-x
Ahamed, M., AlSalhi, M. S., and Siddiqui, M. K. J. (2010). Silver nanoparticle applications and human health. Clin. Chim. Acta 411, 1841–1848. doi: 10.1016/j.cca.2010.08.016
Ahmad, B., Shabbir, A., Jaleel, H., Khan, M. M., and Sadiq, Y. (2018). Efficacy of titanium dioxide nanoparticles in modulating photosynthesis, peltate glandular trichomes and essential oil production and quality in Mentha piperita L. Curr Plant Biol. 13, 6–15. doi: 10.1016/j.cpb.2018.04.002
Ahmad, J., Ali, A. A., Baig, M. A., Iqbal, M., Haq, I., and Qureshi, M. I. (2019). “Role of phytochelatins in cadmium stress tolerance in plants,” in Cadmium Toxicity and Tolerance in Plants, eds M. Hasanuzzaman, M. N. V. Prasad, and M. Fujita (Cambridge, MA: Academic Press), 185–212.
Ahmad, P., Alyemeni, M. N., Al-Huqail, A. A., Alqahtani, M. A., Wijaya, L., Ashraf, M., et al. (2020). Zinc oxide nanoparticles application alleviates arsenic (As) toxicity in soybean plants by restricting the uptake of as and modulating key biochemical attributes, antioxidant enzymes, ascorbate-glutathione cycle and glyoxalase system. Plants 9:825. doi: 10.3390/plants90708252020
Ahmad, P., John, R., Sarwat, M., and Umar, S. (2008). Responses of proline, lipid peroxidation and antioxidative enzymes in two varieties of Pisum sativum L. under salt stress. Int. J. Plant Prod. 2, 353–366. doi: 10.22069/ijpp.2012.626
Ahmed, T., Noman, M., Manzoor, N., Shahid, M., Abdullah, M., Ali, L., et al. (2021). Nanoparticle-based amelioration of drought stress and cadmium toxicity in rice via triggering the stress responsive genetic mechanisms and nutrient acquisition. Ecotoxicol. Environ. Saf. 209:111829. doi: 10.1016/j.ecoenv.2020.111829
Akbari, G. A., Morteza, E., Moaveni, P., Alahdadi, I., Bihamta, M. R., and Hasanloo, T. (2014). Pigments apparatus and anthocyanins reactions of borage to irrigation, Methylalcohol and titanium dioxide. Int. J. Biosci. 4, 192–208. doi: 10.12692/ijb/4.7.192-208
Al-Ashkar, I., Alderfasi, A., El-Hendawy, S., Al-Suhaibani, N., El-Kafafi, S., and Seleiman, M. F. (2019). Detecting salt tolerance in doubled haploid wheat lines. Agronomy 9:211. doi: 10.3390/agronomy9040211
Al-Ashkar, I., Al-Suhaibani, N., Abdella, K., Sallam, M., Alotaibi, M., and Seleiman, M. F. (2021). Combining genetic and multidimensional analyses to identify interpretive traits related to water shortage tolerance as an indirect selection tool for detecting genotypes of drought tolerance in wheat breeding. Plants 10:931. doi: 10.3390/plants10050931
Alharby, H. F., Metwali, E. M., Fuller, M. P., and Aldhebiani, A. Y. (2016). The alteration of mRNA expression of SOD and GPX genes, and proteins in tomato (Lycopersicon esculentum Mill) under stress of NaCl and/or ZnO nanoparticles. Saudi J. Biol. Sci. 23, 773–781. doi: 10.1016/j.sjbs.2016.04.012
Ali, A., Basra, S. M. A., Hussain, S., Iqbal, J., Haji, M. A. A., Bukhsh, A., et al. (2012). Salt stress alleviation in field crops through nutritional supplementation of silicon. Pak. J. Nutr. 11, 735–753. doi: 10.3923/pjn.2012.735.753
Ali, S., Rizwan, M., Hussain, A., Ur Rehman, M. Z., Ali, B., Yousaf, B., et al. (2019). Silicon nanoparticles enhanced the growth and reduced the cadmium accumulation in grains of wheat (Triticum aestivum L.). Plant Physiol. Biochem. 140, 1–8. doi: 10.1016/j.plaphy.2019.04.041
Alkharabsheh, H. M., Seleiman, M. F., Hewedy, O. A., Battaglia, M. L., Jalal, R. S., Alhammad, B. A., et al. (2021). Field crop responses and management strategies to mitigate soil salinity in modern agriculture: a review. Agronomy 11:2299. doi: 10.3390/agronomy11112299
Almutairi, Z. M. (2016a). Effect of nano-silicon application on the expression of salt tolerance genes in germinating tomato (Solanum lycopersicum L.) seedlings under salt stress. Plant Omics 9, 106–114.
Almutairi, Z. M. (2016b). Influence of silver nano-particles on the salt resistance of tomato (Solanum lycopersicum) during germination. Int. J. Agric. Biol. 18, 449–457. doi: 10.17957/ijab/15.0114
Alsaeedi, A., El-Ramady, H., Alshaal, T., El-Garawani, M., Elhawat, N., and Al-Otaibi, A. (2018). Exogenous nanosilica improves germination and growth of cucumber by maintaining K+/Na+ ratio under elevated Na+ stress. Plant Physiol. Biochem. 125, 164–171. doi: 10.1016/j.plaphy.2018.02.006
Alsaeedi, A., El-Ramady, H., Alshaal, T., El-Garawany, M., Elhawat, N., and Al-Otaibi, A. (2019). Silica nanoparticles boost growth and productivity of cucumber under water deficit and salinity stresses by balancing nutrients uptake. Plant Physiol. Biochem. 139, 1–10. doi: 10.1016/j.plaphy.2019.03.008
Alsaeedi, A. H., El-Ramady, H., Alshaal, T., El-Garawani, M., Elhawat, N., and Almohsen, M. (2017). Engineered silica nanoparticles alleviate the detrimental effects of Na+ stress on germination and growth of common bean (Phaseolus vulgaris). Environ. Sci. Pollut. Res. 24, 21917–21928. doi: 10.1007/s11356-017-9847-y
Amini, S., Maali-Amiri, R., Mohammadi, R., and Kazemi-Shahandashti, S. S. (2017). cDNAAFLP analysis of transcripts induced in chickpea plants by TiO2 nanoparticles during cold stress. Plant Physiol. Biochem. 111, 39–49. doi: 10.1016/j.plaphy.2016.11.011
Amooaghaie, R., Tabatabaei, F., and Ahadi, A. M. (2015). Role of hematin and sodium nitroprusside in regulating Brassica nigra seed germination under nanosilver and silver nitrate stresses. Ecotoxicol. Environ. Saf. 113, 259–270. doi: 10.1016/j.ecoenv.2014.12.017
Anderson, J. A., Gipmans, M., Hurst, S., Layton, R., Nehra, N., Pickett, J., et al. (2016). Emerging agricultural biotechnologies for sustainable agriculture and food security. J. Agric. Food Chem. 64, 383–393. doi: 10.1021/acs.jafc.5b04543
Anoop, N., and Gupta, A. K. (2003). Transgenic indica rice cv IR-50 overexpressing Vigna aconitifolia Δ1-pyrroline -5- carboxylate synthetase cDNA shows tolerance to high salt. J. Plant Biochem. Biotechnol. 12, 109–116. doi: 10.1007/bf03263170
Ashkavand, P., Tabari, M., Zarafshar, M., Tomášková, I., and Struve, D. (2015). Effect of SiO2 nanoparticles on drought resistance in hawthorn seedlings. For. Res. Pap. 76, 350–359.
Ashkavand, P., Zarafshar, M., Tabari, M., Mirzaie, J., Nikpour, A., Bordbar, S. K., et al. (2018). Application of SiO2 nanoparticles as pretreatment alleviates the impact of drought on the physiological performance of Prunus mahaleb (Rosaceae). Bol. Soc. Argent. Bot. 53, 207–219. doi: 10.31055/1851.2372.v53.n2.20578
Ashour, H. A., and Abdel Wahab, M. (2017). Response of Jatropha integerrima plants irrigated with different levels of saline water to nano silicon and gypsum. J. Agric. Sci. 5, 136–160. doi: 10.5296/jas.v5i4.12170
Askary, M., Talebi, S. M., Amini, F., and Bangan, A. D. B. (2017). Effects of iron nanoparticles on Mentha piperita L. under salinity stress. Biologija 63, 65–67. doi: 10.6001/biologija.v63i1.3476
Atha, D. H., Wang, H., Petersen, E. J., Cleveland, D., Holbrook, R. D., Jaruga, P., et al. (2012). Copper oxide nanoparticle mediated DNA damage in terrestrial plant models. Environ. Sci. Technol. 46, 1819–1827. doi: 10.1021/es202660k
Avestan, S., Ghasemnezhad, M., Esfahani, M., and Byrt, C. S. (2019). Application of nano-silicon dioxide improves salt stress tolerance in strawberry plants. Agronomy 9:246. doi: 10.3390/agronomy9050246
Awasthi, A., Bansal, S., Jangir, L. K., Awasthi, G., Awasthi, K. K., and Awasthi, K. (2017). Effect of ZnO nanoparticles on germination of Triticum aestivum seeds. Macromol. Symp. 376:1700043. doi: 10.1002/masy.201700043
Azimi, R., Borzelabad, M. J., Feizi, H., and Azimi, A. (2014). Interaction of SiO2 nanoparticles with seed prechilling on germination and early seedling growth of tall wheatgrass (Agropyron elongatum L.). Pol. J. Chem. Technol. 16, 25–29. doi: 10.2478/pjct-2014-0045
Badal, E., Abd El-Mageed, T. A., Buesa, I., Guerra, D., Bonet, L., and Intrigliolo, D. S. (2013). Moderate plant water stress reduces fruit drop of “Rojo Brillante” persimmon (Diospyros kaki) in a Mediterranean climate. Agric. Water Manage. 119, 154–160. doi: 10.1016/j.agwat.2012.12.020
Badawy, S. A., Zayed, B. A., Bassiouni, S. M. A., Mahdi, A. H. A., Majrashi, A., Ali, E. F., et al. (2021). Influence of nano silicon and nano selenium on root characters, growth, ion selectivity, yield, and yield components of rice (Oryza sativa L.) under salinity conditions. Plants 10:1657. doi: 10.3390/plants10081657
Bal, A. (2019). Effect of Foliar Application of Nanoparticles of Chitosan, Iron Oxide and Chitosan-Iron Oxide Complex on Secondary Metabolites of Hypericum Triquetrifolium Turra. [Master’s Thesis]. Diyarbakir: Dicle University.
Banerjee, J., and Kole, C. (2016). “Plant nanotechnology: an overview on concepts, strategies, and tools,” in Plant Nanotechnology, eds C. Kole, D. S. Kumar, and M. V. Khodakovskaya (Switzerland: Springer), 1–14.
Batool, T., Ali, S., Seleiman, M. F., Naveed, N. H., Ali, A., Ahmed, K., et al. (2020). Plant growth promoting rhizobacteria alleviates drought stress in potato in response to suppressive oxidative stress and antioxidant enzymes activities. Sci. Rep. 10:16975. doi: 10.1038/s41598-020-73489-z
Baybordi, A. (2005). Effect of zinc, iron, manganese and copper on wheat quality under salt stress conditions. J. Water Soil 140, 150–170.
Bechert, T., Böswald, M., Lugauer, S., Regenfus, A., Greil, J., and Guggenbichler, J. P. (1999). The Erlanger silver catheter: in vitro results for antimicrobial activity. Infection 27, S24–S29. doi: 10.1007/bf02561613
Behboudi, F., Tahmasebi Sarvestani, Z., Kassaee, M. Z., Modares Sanavi, S. A. M., Sorooshzadeh, A., and Ahmadi, S. B. (2018). Evaluation of chitosan nanoparticles effects on yield and yield components of barley (Hordeum vulgare L.) under late season drought stress. J. Water Environ. Nanotechnol. 3, 22–39. doi: 10.22090/jwent.2018.01.003
Behboudi, F., Tahmasebi-Sarvestani, Z., Kassaee, M. Z., Modarres-Sanavy, S. A. M., Sorooshzadeh, A., and Mokhtassi-Bidgoli, A. (2019). Evaluation of chitosan nanoparticles effects with two application methods on wheat under drought stress. J. Plant Nutr. 42, 1439–1451. doi: 10.1080/01904167.2019.1617308
Bhatt, D., Bhatt, M. D., Nath, M., Dudhat, R., Sharma, M., and Bisht, D. S. (2020). “Application of nanoparticles in overcoming different environmental stresses,” in Protective Chemical Agents in The Amelioration of Plant Abiotic Stress: Biochemical and Molecular Perspectives, eds A. Roychoudhury and D. K. Tripathi (Hoboken: Wiley-Blackwell), 635–654.
Bhatt, D., and Sharma, G. (2018). Role of silicon in counteracting abiotic and biotic plant stresses. IJCS 6, 1434–1442.
Bratovcic, A., Hikal, W. M., Said-Al Ahl, H. A., Tkachenko, K. G., Baeshen, R. S., Sabra, A. S., et al. (2021). Nanopesticides and nanofertilizers and agricultural development: scopes, advances and applications. Open Ecol. J. 11, 301–316. doi: 10.4236/oje.2021.114022
Burman, U., Saini, M., and Kumar, P. (2013). Effect of zinc oxide nanoparticles on growth and antioxidant system of chickpea seedlings. Toxicol. Environ. Chem. 95, 605–612. doi: 10.1080/02772248.2013.803796
Cakmak, I. (2008). Enrichment of cereal grains with zinc: agronomic or genetic biofortification? Plant Soil 302, 1–17. doi: 10.1007/s11104-007-9466-3
Cakmak, I., Yilmaz, A., Kalayci, M., Ekiz, H., Torun, B., Ereno, B., et al. (1996). Zinc deficiency as a critical problem in wheat production in Central Anatolia. Plant Soil 180, 165–172. doi: 10.1007/bf00015299
Cañas, J. E., Long, M., Nations, S., Vadan, R., Dai, L., Luo, M., et al. (2008). Effects of functionalized and nonfunctionalized single walled carbon nanotubes on root elongation of select crop species. Environ. Toxicol. Chem. 27, 1922–1931. doi: 10.1897/08-117.1
Changmei, L., Chaoying, Z., Junqiang, W., Guorong, W., and Mingxuan, T. (2002). Research of the effect of nanometer materials on germination and growth enhancement of Glycine max and its mechanism. Soybean Sci. 21, 168–171.
Chen, Y. W., Lee, H. V., Juan, J. C., and Phang, S. M. (2016). Production of new cellulose nanomaterial from red algae marine biomass Gelidium elegans. Carbohydr. Polym. 151, 1210–1219. doi: 10.1016/j.carbpol.2016.06.083
Chhipa, H. (2017). Nanofertilizers and nanopesticides for agriculture. Environ. Chem. Lett. 15, 15–22. doi: 10.1007/s10311-016-0600-4
Cinisli, K. T., Uçar, S. M., and Dikbaş, N. (2019). Use of nanomaterials in agriculture. Yuzuncu Yil Univ. J. Agric. Sci. 29, 817–831. doi: 10.29133/yyutbd.595658
Costa, M. V. J. D., and Sharma, P. K. (2016). Effect of copper oxide nanoparticles on growth, morphology, photosynthesis, and antioxidant response in Oryza sativa. Photosynthetica 54, 110–119. doi: 10.1007/s11099-015-0167-5
Cota-Ruiz, K., Delgado-Rios, M., Martínez-Martínez, A., Núñez-Gastelum, J. A., Peralta-Videa, J. R., and Gardea-Torresdey, J. L. (2018). Current findings on terrestrial plants–Engineered nanomaterial interactions: are plants capable of phytoremediating nanomaterials from soil? Curr. Opin. Environ. Sci. Health 6, 9–15.
Cruz de Carvalho, M. H. (2008). Drought stress and reactive oxygen species: production, scavenging and signaling. Plant Signal. Behav. 3, 156–165. doi: 10.4161/psb.3.3.5536
Cui, J., Li, Y., Jin, Q., and Li, F. (2020). Silica nanoparticles inhibit arsenic uptake into rice suspension cells via improving pectin synthesis and the mechanical force of the cell wall. Environ. Sci. Nano 7, 162–171. doi: 10.1039/c9en01035a
Cvjetko, P., Milošic, A., Domijan, A. M., Vinkovic-Vrcek, I., Tolic, S., Peharec-Štefanic, P., et al. (2017). Toxicity of silver ions and differently coate ’ d silver nanoparticles in Allium cepa roots. Ecotoxicol. Environ. Saf. 137, 18–28. doi: 10.1016/j.ecoenv.2016.11.009
Darvishzadeh, F., Najatzadeh, F., and Iranbakhsh, A. R. (2015). Effect of silver nanoparticles on salinity tolerance of basil plant in germination stages under laboratory conditions. J. Cell. Biotechnol. Mol. 20, 63–70.
Datnoff, L. E., Rodrigues, F. A., and Seebold, K. W. (2009). “Silicon and plant disease,” in Mineral Nutrition and Plant Disease, eds L. E. Datnoff, W. H. Elmer, and D. M. Huber (Saint Paul: American Phytopathological Society), 233–246.
Davies, J. C. (2008). Nanotechnology Oversight: An Agenda for the New Administration. The Project on Emerging Nanotechnologies. Washington, DC: Project on Emerging Nanotechnologies.
Dawood, M. F., Abeed, A. H., and Aldaby, E. E. (2019). Titanium dioxide nanoparticles model growth kinetic traits of some wheat cultivars under different water regimes. Plant Physiol. Rep. 24, 129–140. doi: 10.1007/s40502-019-0437-5
de Oliveira, J. L., Campos, E. V. R., Bakshi, M., Abhilash, P. C., and Fraceto, L. F. (2014). Application of nanotechnology for the encapsulation of botanical insecticides for sustainable agriculture: prospects and promises. Biotechnol. Adv. 32, 1550–1561. doi: 10.1016/j.biotechadv.2014.10.010
de Sousa, A., Saleh, A. M., Habeeb, T. H., Hassan, Y. M., Zrieq, R., Wadaan, M. A. M., et al. (2019). Silicon dioxide nanoparticles ameliorate the phytotoxic hazards of aluminum in maize grown on acidic soil. Sci. Total Environ. 693:133636. doi: 10.1016/j.scitotenv.2019.133636
Degenhardt, B., and Gimmler, H. (2000). Cell wall adaptations to multiple environmental stresses in maize roots. J. Exp. Bot. 51, 595–603. doi: 10.1093/jexbot/51.344.595
Dehkourdi, E. H., and Mosavi, M. (2013). Effect of anatase nanoparticles (TiO2) on parsley seed germination (Petroselinum crispum) in vitro. Biol. Trace Elem. Res. 155, 283–286. doi: 10.1007/s12011-013-9788-3
Delfani, M., Baradarn Firouzabadi, M., Farrokhi, N., and Makarian, H. (2014). Some physiological responses of black-eyed pea to iron and magnesium nanofertilizers. Commun. Soil Sci. Plant Anal. 45, 530–540. doi: 10.1080/00103624.2013.863911
Demirer, G. S., Silva, T. N., Jackson, C. T., Thomas, J. B., Ehrhardt, W., Rhee, S. Y., et al. (2021). Nanotechnology to advance CRISPR–Cas genetic engineering of plants. Nat. Nanotechnol. 16, 243–250.
DeRosa, M. C., Monreal, C., Schnitzer, M., Walsh, R., and Sultan, Y. (2010). Nanotechnology in fertilizers. Nat. Nanotechnol. 5:91.
Desoky, E. S. M., Merwad, A. R. M., Semida, W. M., Ibrahim, S. A., El-Saadony, M. T., and Rady, M. M. (2020a). Heavy metals-resistant bacteria (HM-RB): potential bioremediators of heavy metals-stressed Spinacia oleracea plant. Ecotoxicol. Environ. Saf. 198:110685.
Desoky, E. S. M., Saad, A. M., El-Saadony, M. T., Merwad, A. R. M., and Rady, M. M. (2020b). Plant growth-promoting rhizobacteria: potential improvement in antioxidant defense system and suppression of oxidative stress for alleviating salinity stress in Triticum aestivum (L.) plants. Biocatal. Agric. Biotechnol. 30:101878.
Dimkpa, C. O., McLean, J. E., Latta, D. E., Manangón, E., Britt, D. W., Johnson, W. P., et al. (2012). CuO and ZnO nanoparticles: phytotoxicity, metal speciation, and induction of oxidative stress in sand-grown wheat. J. Nanopart. Res. 14:1125.
Dimkpa, C. O., Singh, U., Bindraban, P. S., Elmer, W. H., Gardea-Torresdey, J. L., and White, J. C. (2019). Zinc oxide nanoparticles alleviate drought-induced alterations in sorghum performance, nutrient acquisition, and grain fortification. Sci. Total Environ. 688, 926–934.
Dimkpa, C. O., White, J. C., Elmer, W. H., and Gardea-Torresdey, J. (2017). Nanoparticle and ionic Zn promote nutrient loading of sorghum grain under low NPK fertilization. J. Agric. Food Chem. 65, 8552–8559. doi: 10.1021/acs.jafc.7b02961
Du, W., Yang, J., Peng, Q., Liang, X., and Mao, H. (2019). Comparison study of zinc nanoparticles and zinc sulphate on wheat growth: from toxicity and zinc biofortification. Chemosphere 227, 109–116. doi: 10.1016/j.chemosphere.2019.03.168
Dubchak, S., Ogar, A., Mietelski, J. W., and Turnau, K. (2010). Influence of silver and titanium nanoparticles on arbuscular mycorrhiza colonization and accumulation of radiocaesium in Helianthus annuus. Span. J. Agric. Res. 8, 103–108. doi: 10.5424/sjar/201008S1-1228
Dustgeer, Z., Seleiman, M. F., Khan, I., Chattha, M. U., Ali, E., Alhammad, B., et al. (2021). Glycine-betaine induced salinity tolerance in maize by regulating the physiological attributes, antioxidant defense system and ionic homeostasis. Not. Bot. Horti. Agrobot. Cluj Napoca 49:12248.
Eichert, T., Kurtz, A., Steiner, U., and Goldbach, H. E. (2008). Size exclusion limits and lateral heterogeneity of the stomatal foliar uptake pathway for aqueous solutes and watersuspended nanoparticles. Physiol. Plant. 134, 151–160. doi: 10.1111/j.1399-3054.2008.01135.x
Ekhtiyari, R., Mohebbi, H., and Mansouri, M. (2011). Effect of nanosilver particles on salinity tolerance of fennel plants in early growth under laboratory conditions. J. Plant Biotechnol. 7, 55–62.
Ekhtiyari, R., and Moraghebi, F. (2011). The study of the effects of nano silver technology on salinity tolerance of cumin seed (Cuminum cyminum L.). Plant Ecosyst. 25, 99–107.
Ekhtiyari, R., and Moraghebi, F. (2012). Effect of nanosilver particles on salinity tolerance of cumin (Cuminum cyminum L.). J. Plant Biotechnol. 25, 99–107.
El-Ashry, R. M., El-Saadony, M. T., El-Sobki, A. E., El-Tahan, A. M., Al-Otaibi, S., El-Shehawi, A. M., et al. (2022). Biological silicon nanoparticles maximize the efficiency of nematicides against biotic stress induced by Meloidogyne incognita in eggplant. Saudi J. Biol. Sci. 29, 920–932.
El-Saadony, M. T., Abd El-Hack, M. E., Taha, A. E., Fouda, M., Ajarem, J. S., N Maodaa, S., et al. (2020). Ecofriendly synthesis and insecticidal application of copper nanoparticles against the storage pest Tribolium castaneum. Nanomaterials 10:587. doi: 10.3390/nano10030587
El-Saadony, M. T., Sitohy, M. Z., Ramadan, M. F., and Saad, A. M. (2021a). Green nanotechnology for preserving and enriching yogurt with biologically available iron (II). Innov. Food Sci. Emerg. Technol. 69:102645. doi: 10.1016/j.ifset.2021.102645
El-Saadony, M. T., ALmoshadak, A. S., Shafi, M. E., Albaqami, N. M., Saad, A. M., El-Tahan, A. M., et al. (2021b). Vital roles of sustainable nano-fertilizers in improving plant quality and quantity-an updated review Saudi J. Biol. Sci. 28, 7349–7359. doi: 10.1016/j.sjbs.2021.08.032
El-Saadony, M. T., Desoky, E. S. M., Saad, A. M., Eid, R. S., Selem, E., and Elrys, A. S. (2021c). Biological silicon nanoparticles improve Phaseolus vulgaris L. yield and minimize its contaminant contents on a heavy metals-contaminated saline soil. J. Environ. Sci. 106, 1–14. doi: 10.1016/j.jes.2021.01.012
El-Saadony, M. T., Saad, A. M., Najjar, A. A., Alzahrani, S. O., Alkhatib, F. M., Shafi, M. E., et al. (2021d). The use of biological selenium nanoparticles to suppress Triticum aestivum L. crown and root rot diseases induced by Fusarium species and improve yield under drought and heat stress. Saudi J. Biol. Sci. 28, 4461–4471. doi: 10.1016/j.sjbs.2021.04.043
Elsakhawy, T., Omara, A. E. D., Alshaal, T., and El-Ramady, H. (2018). Nanomaterials and plant abiotic stress in agroecosystems. EBSS 2, 73–94. doi: 10.21608/jenvbs.2018.3897.1030
Elsheery, N. I., Helaly, M. N., El-Hoseiny, H. M., and Alam-Eldein, S. M. (2020). Zinc oxide and silicone nanoparticles to improve the resistance mechanism and annual productivity of salt-stressed mango trees. Agronomy 10:558. doi: 10.3390/agronomy10040558
El-Temsah, Y. S., and Joner, E. J. (2010). Impact of Fe and Ag nanoparticles on seed germination and differences in bioavailability during exposure in aqueous suspension and soil. Environ. Toxicol. 27, 42–49. doi: 10.1002/tox.20610
Etxeberria, E., Gonzalez, P., Pozueta-Romero, J., and Romero, J. P. (2006). Fluid phase endocytic uptake of artificial nano-spheres and fluorescent quantum dots by sycamore cultured cells: evidence for the distribution of solutes to different intracellular compartments. Plant Signal. Behav. 1, 196–200. doi: 10.4161/psb.1.4.3142
Faisal, M., Saquib, Q., Alatar, A. A., Al-Khedhairy, A. A., Hegazy, A. K., and Musarrat, J. (2013). Phytotoxic hazards of NiO-nanoparticles in tomato: a study on mechanism of cell death. J. Hazard. Mater. 250–251, 318–332. doi: 10.1016/j.jhazmat.2013.01.063
Faizan, M., Bhat, J. A., Chen, C., Alyemeni, M. N., Wijaya, L., Ahmad, P., et al. (2021). Zinc oxide nanoparticles (Zno-NPs) induce salt tolerance by improving the antioxidant system and photosynthetic machinery in tomato. Plant Physiol. Biochem. 161, 122–130. doi: 10.1016/j.plaphy.2021.02.002
Faraji, J., and Sepehri, A. (2020). Exogenous nitric oxide improves the protective effects of TiO2 nanoparticles on growth, antioxidant system, and photosynthetic performance of wheat seedlings under drought stress. J. Soil. Sci. Plant Nutr. 20, 703–714. doi: 10.1007/s42729-019-00158-0
Farhangi-Abriz, S., and Torabian, S. (2018). Nano-silicon alters antioxidant activities of soybean seedlings under salt toxicity. Protoplasma 255, 953–962. doi: 10.1007/s00709-017-1202-0
Farid, M., Shakoor, M. B., Ehsan, S., Ali, S., Zubair, M., and Hanif, M. A. (2013). Morphological, physiological and biochemical responses of different plant species to Cd stress. Int. J. Chem. Biochem. Sci. 3, 53–60.
Fathi, A., Zahedi, M., Torabian, S., and Khoshgoftar, A. (2017). Response of wheat genotypes to foliar spray of ZnO and Fe2O3 nanoparticles under salt stress. J. Plant Nutr. 40, 1376–1385. doi: 10.1080/01904167.2016.1262418
Fazeli-Nasab, B., Sirousmehr, A. R., and Azad, H. (2018). Effect of titanium dioxide nanoparticles on essential oil quantity and quality in Thymus vulgaris under water deficit. J. Med. Plants Products 2, 125–133. doi: 10.22092/JMPB.2018.118140
Feizi, H., Kamali, M., Jafari, L., and Rezvani Moghaddam, P. (2013). Phytotoxicity and stimulatory impacts of nanosized and bulk titanium dioxide on fennel (Foeniculum vulgare Mill). Chemosphere 91, 506–511. doi: 10.1016/j.chemosphere.2012.12.012
Feng, Y., Cui, X., He, S., Dong, G., Chen, M., Wang, J., et al. (2013). The role of metal nanoparticles in influencing arbuscular mycorrhizal fungi effects on plant growth. Environ. Sci. Technol. 47, 9496–9504. doi: 10.1021/es402109n
Fleischer, A., O’Neill, M. A., and Ehwald, R. (1999). The pore size of non-graminaceous plant cell walls is rapidly decreased by borate ester crosslinking of the pectic polysaccharide rhamnogalacturonan II. Plant Physiol. 12, 829–838. doi: 10.1104/pp.121.3.829
Foyer, C. H., and Noctor, G. (2005). Redox homeostasis and antioxidant signaling: a metabolic interface between stress perception and physiological responses. Plant Cell 17, 1866–1875. doi: 10.1105/tpc.105.033589
Gao, F., Liu, C., Qu, C., Zheng, L., Yang, F., Su, M., et al. (2008). Was improvement of spinach growth by nano-TiO2 treatment related to the changes of Rubisco activase? Biometals 21, 211–217. doi: 10.1007/s10534-007-9110-y
Gao, X., Zou, C., Wang, L., and Zhang, F. (2006). Silicon decreases transpiration rate and conductance from stomata of maize plants. J. Plant Nutr. 29, 1637–1647. doi: 10.1080/01904160600851494
García-López, J. I., Zavala-García, F., Olivares-Sáenz, E., Lira-Saldívar, R. H., Díaz Barriga-Castro, E., Ruiz-Torres, N. A., et al. (2018). Zinc oxide nanoparticles boosts phenolic compounds and antioxidant activity of Capsicum annuum L. during germination. Agronomy 8:215. doi: 10.3390/agronomy8100215
Ghorbanpour, M. (2015). Major essential oil constituents, total phenolics and flavonoids content and antioxidant activity of Salvia officinalis plant in response to nano-titanium dioxide. Ind. J. Plant Physiol. 20, 249–256. doi: 10.1007/s40502-015-0170-7
Ghorbanpour, M., Hatami, M., and Hatami, M. (2015). Activating antioxidant enzymes, hyoscyamine and scopolamine biosynthesis of Hyoscyamus niger L. plants with nanosized titanium dioxide and bulk application. Acta Agric. Slov. 105, 23–32. doi: 10.14720/aas.2015.105.1.03
Giraldo, J. P., Landry, M. P., Faltermeier, S. M., McNicholas, T. P., Iverson, N. M., Boghossian, A. A., et al. (2014). Plant nanobionics approach to augment photosynthesis and biochemical sensing. Nat. Mater. 13, 400–408. doi: 10.1038/nmat3890
Gohari, G., Mohammadi, A., Akbari, A., Panahirad, S., Dadpour, M. R., Fotopoulos, V., et al. (2020). Titanium dioxide nanoparticles (TiO2 NPs) promote growth and ameliorate salinity stress effects on essential oil profile and biochemical attributes of Dracocephalum moldavica. Sci. Rep. 10:912. doi: 10.1038/s41598-020-57794-1
Govorov, A. O., and Carmeli, I. (2007). Hybrid structures composed of photosynthetic system and metal nanoparticles: plasmon enhancement effect. Nano Lett. 7, 620–625. doi: 10.1021/nl062528t
Gunes, A., Pilbeam, D. J., Inal, A., Bagci, E. G., and Coban, S. (2007). Influence of silicon on antioxidant mechanisms and lipid peroxidation in chickpea (Cicer arietinum L.) cultivars under drought stress. J. Plant Interact. 2, 105–113. doi: 10.1080/17429140701529399
Gupta, S. D., Agarwal, A., and Pradhan, S. (2018). Phytostimulatory effect of silver nanoparticles (AgNPs) on rice seedling growth: an insight from antioxidative enzyme activities and gene expression patterns. Ecotoxicol. Environ. Saf. 161, 624–633. doi: 10.1016/j.ecoenv.2018.06.023
Haghighi, M., Afifipour, Z., and Mozafarian, M. (2012). The effect of N-Si on tomato seed germination under salinity levels. J. Biol. Environ. Sci. 6, 87–90.
Haghighi, M., and Pessarakli, M. (2013). Influence of silicon and nano-silicon on salinity tolerance of cherry tomatoes (Solanum lycopersicum L.) at early growth stage. Sci. Hortic. 161, 111–117. doi: 10.1016/j.scienta.2013.06.034
Haghighi, M., and Pourkhaloee, A. (2013). Nanoparticles in agricultural soils: their risks and benefits for seed germination. Minerva Biotecnol. 25, 123–132.
Hall, J. L. (2002). Cellular mechanisms for heavy metal detoxification and tolerance. J. Exp. Bot. 53, 1–11. doi: 10.1093/jexbot/53.366.1
Haripriya, P., Stella, P. M., and Anusuya, S. (2018). Foliar spray of zinc oxide nanoparticles improves salt tolerance in finger millet crops under glasshouse condition. Sciol. Biotechnol. 1, 20–29.
Hasanpour, H., Maali-Amir, R., and Zeinali, H. (2015). Effect of TiO2 nanoparticles on metabolic limitations to photosynthesis under cold in chickpea. Russ. J. Plant Physiol. 62, 779–787. doi: 10.1134/S1021443715060096
Hatami, M. (2017). Toxicity assessment of multi-walled carbon nanotubes on Cucurbita pepo L. under well-watered and water-stressed conditions. Ecotoxicol. Environ. Saf. 142, 274–283.
Hatami, M., and Ghorbanpour, M. (2013). Effect of nanosilver on physiological performance of Pelargonium plants exposed to dark storage. J. Hortic. Res. 21, 15–20. doi: 10.2478/johr-2013-0003
Hatami, M., and Ghorbanpour, M. (2014). Defense enzyme activities and biochemical variations of Pelargonium zonale in response to nanosilver application and dark storage. Turk. J. Biol. 38, 130–139. doi: 10.3906/biy-1304-64
Hattori, T., Inanaga, S., Araki, H., An, P., Morita, S., Luxová, M., et al. (2005). Application of silicon enhanced drought tolerance in Sorghum bicolor. Physiol. Plant. 123, 459–466. doi: 10.1111/j.1399-3054.2005.00481.x
Hernandez, J. A., Jimenez, A., Mullineaux, P., and Seviela, F. (2000). Tolerance of pea (Pisum sativum L.) to long-term salt stress is associated to induction of antioxidant defences. Plant Cell Environ. 23, 853–862. doi: 10.1046/j.1365-3040.2000.00602.x
Hojjat, S. S. (2019). Effect of interaction between Ag nanoparticles and salinity on germination stages of Lathyrus sativus L. J. Environ. Soil Sci. 2, 186–191. doi: 10.32474/oajess.2019.02.000132
Hojjat, S. S., and Ganjali, A. (2016). The effect of silver nanoparticle on lentil seed germination under drought stress. Intl. J. Farm. Alli. Sci. 5, 208–212.
Hojjat, S. S., and Kamyab, M. (2017). The effect of silver nanoparticle on fenugreek seed germination under salinity levels. Russ. Agric. Sci. 43, 61–65. doi: 10.3103/S1068367417010189
Hong, F., Yang, F., Liu, C., Gao, Q., Wan, Z., Gu, F., et al. (2005). Influences of nano-TiO2 on the chloroplast aging of spinach under light. Biol. Trace Elem. Res. 104, 249–260. doi: 10.1385/BTER:104:3:249
Hong, J., Rico, C. M., Zhao, L., Adeleye, A. S., Keller, A. A., Peralta-Videa, J. R., et al. (2016). Toxic effects of copper-based nanoparticles or compounds to lettuce (Lactuca sativa) and alfalfa (Medicago sativa). Environ. Sci. Process. Impacts 17, 177–185. doi: 10.1039/c4em00551a
Hossain, A., Skalicky, M., Brestic, M., Maitra, S., Ashraful Alam, M., Syed, M. S., et al. (2021). Consequences and mitigation strategies of abiotic stresses in wheat (Triticum aestivum L.) under the changing climate. Agronomy 11:241. doi: 10.3390/agronomy11020241
Hossain, M. A., Piyatida, P., da Silva, J. A. T., and Fujita, M. (2012). Molecular mechanism of heavy metal toxicity and tolerance in plants: central role of glutathione in detoxification of reactive oxygen species and methylglyoxal and in heavy metal chelation. J. Bot. 2012:872875. doi: 10.1155/2012/872875
Hossain, Z., Mustafa, G., and Komatsu, S. (2015). Plant responses to nanoparticle stress. Int. J. Mol. Sci. 16, 26644–26653. doi: 10.3390/ijms161125980
Hossain, Z., Mustafa, G., Sakata, K., and Komatsu, S. (2016). Insights into the proteomic response of soybean towards Al2O3, ZnO, and Ag nanoparticles stress. J. Hazard Mater. 304, 291–305. doi: 10.1016/j.jhazmat.2015.10.071
Hussain, A., Oves, M., Alajmi, M. F., Hussain, I., Amir, S., Ahmed, J., et al. (2019a). Biogenesis of ZnO nanoparticles using Pandanus odorifer leaf extract: anticancer and antimicrobial activities. RSC Adv. 9, 15357–15369. doi: 10.1039/c9ra01659g
Hussain, A., Rizwan, M., Ali, Q., and Ali, S. (2019b). Seed priming with silicon nanoparticles improved the biomass and yield while reduced the oxidative stress and cadmium concentration in wheat grains. Environ. Sci. Pollut. Res. 26, 7579–7588. doi: 10.1007/s11356-019-04210-5
Hussain, A., Ali, S., Rizwan, M., Rehman, M., Qayyum, M. F., and Wang, H. (2019c). Responses of wheat (Triticum aestivum) plants grown in a Cd contaminated soil to the application of iron oxide nanoparticles. Ecotoxicol. Environ. Saf. 173, 156–164. doi: 10.1016/j.ecoenv.2019.01.118
Ion, A. C., Ion, I., Culetu, A., and Gherase, D. (2010). Carbon-based nanomaterials. Environmental applications. Romania. Univ. Politehn. Bucharest 38, 129–132.
Iqbal, M., Raja, N. I., Mashwani, Z., Hussain, M., Muhammad, E., and Yasmeen, F. (2019). Effect of silver nanoparticles on growth of wheat under heat stress. Iran. J. Sci. Technol. Trans. A Sci. 43, 387–395. doi: 10.1007/s40995-017-0417-4
Jaberzadeh, A., Moaveni, P., Tohidi Moghadam, H. R., and Zahedi, H. (2013). Influence of bulk and nanoparticles titanium foliar application on some agronomic traits, seed gluten and starch contents of wheat subjected to water deficit stress. Not. Bot. Horti. Agrobot. 41, 201–207. doi: 10.15835/nbha4119093
Jalil, S. U., and Ansari, M. I. (2019). “Nanoparticles and abiotic stress tolerance in plants: synthesis, action, and signaling mechanisms,” in Plant Signaling Molecules Role and Regulation Under Stressful Environments, eds I. S. Khan, S. P. Reddy, A. Ferrante, and A. N. Khan (Duxford: UK-Woodhead Publishing), 549–561.
Janmohammadi, M., Amanzadeh, T., Sabaghnia, N., and Ion, V. (2016). Effect of nano-silicon foliar application on safflower growth under organic and inorganic fertilizer regimes. Bot. Lith. 22, 53–64. doi: 10.1515/botlit-2016-0005
Jeelani, P. G., Mulay, P., Venkat, R., and Ramalingam, C. (2020). Multifaceted application of silica nanoparticles. A review. Silicon 12, 1337–1354. doi: 10.1007/s12633-019-00229-y
Jiang, H. S., Qiu, X. N., Li, G. B., Li, W., and Yin, L. Y. (2014). Silver nanoparticles induced accumulation of reactive oxygen species and alteration of antioxidant systems in the aquatic plant Spirodela polyrhiza. Environ. Toxicol. Chem. 33, 1398–1405. doi: 10.1002/etc.2577
Jo, Y.-K., Kim, B. H., and Jung, G. (2009). Antifungal activity of silver ions and nanoparticles on phytopathogenic fungi. Plant Dis. 93, 1037–1043. doi: 10.1094/PDIS-93-10-1037
Judy, J. D., Unrine, J. M., Rao, W., Wirick, S., and Bertsch, P. M. (2012). Bioavailability of gold nanomaterials to plants: importance of particle size and surface coating. Environ. Sci. Technol. 46, 8467–8474. doi: 10.1021/es3019397
Kah, M. (2015). Nanopesticides and nanofertilizers: emerging contaminants or opportunities for risk mitigation? Front. Chem. 3:64. doi: 10.3389/fchem.2015.00064
Kalteh, M., Taj, A. Z., Shahram, A., Maryam, M. A., and Alireza, F. N. (2014). Effect of silica nanoparticles on basil (Ocimum basilicum) under salinity stress. J. Chem. Health Risks. 4, 49–55.
Karami Mehrian, S., Heidari, R., Rahmani, F., and Najafi, S. (2016). Effect of chemical synthesis silver nanoparticles on germination indices and seedlings growth in seven varieties of Lycopersicon esculentum Mill (tomato) plants. J. Clust. Sci. 27, 327–340. doi: 10.1007/s10876-015-0932-4
Kardavan Ghabel, V., and Karamian, R. (2020). Effects of TiO2 nanoparticles and spermine on antioxidant responses of Glycyrrhiza glabra L. to cold stress. Acta Bot. Croat. 79, 137–147. doi: 10.37427/botcro-2020-025
Kashyap, P. L., Xiang, X., and Heiden, P. (2015). Chitosan nanoparticle based delivery systems for sustainable agriculture. Int. J. Biol. Macromol. 77, 36–51. doi: 10.1016/j.ijbiomac.2015.02.039
Katiyar, P., Yadu, B., Korram, J., Satnami, M. L., Kumar, M., and Keshavkant, S. (2020). Titanium nanoparticles attenuates arsenic toxicity by up-regulating expressions of defensive genes in Vigna radiata L. J. Environ. Sci. 92, 18–27. doi: 10.1016/j.jes.2020.02.013
Kazemipour, S., Hashemabadi, D., and Kaviani, B. (2013). Effect of silver nanoparticles on the vase life and quality of cut chrysanthemum (Chrysanthemum morifolium L.) flower. Eur. J. Exp. Biol. 3, 298–302.
Keller, C., Rizwan, M., Davidian, J.-C., Pokrovsky, O. S., Bovet, N., Chaurand, P., et al. (2015). Effect of silicon on wheat seedlings (Triticum turgidum L.) grown in hydroponics and exposed to 0 to 30 μM Cu. Planta 241, 847–860. doi: 10.1007/s00425-014-2220-1
Khairy, A. M., Tohamy, M. R., Zayed, M. A., Mahmoud, S. F., El-Tahan, A. M., El-Saadony, M. T., et al. (2022). Eco-friendly application of nano-chitosan for controlling potato and tomato bacterial wilt. Saudi J. Biol. Sci. 29, 2199–2209. doi: 10.1016/j.sjbs.2021.11.041
Khan, H. R., McDonald, G. K., and Rengel, Z. (2004). Zinc fertilization and water stress affects plant water relations, stomatal conductance and osmotic adjustment in chickpea (Cicer arientinum L.). Plant Soil 267, 271–284. doi: 10.1007/s11104-005-0120-7
Khan, I., Seleiman, M. F., Chattha, M. U., Jalal, R. S., Mahmood, F., Hassan, F. A., et al. (2021). Enhancing antioxidant defense system of mung bean with a salicylic acid exogenous application to mitigate cadmium toxicity. Not. Bot. Horti. Agrobot. Cluj Napoca 49:12303.
Khan, M., and Siddiqui, Z. A. (2018). Zinc oxide nanoparticles for the management of Ralstonia solanacearum, Phomopsis vexans and Meloidogyne incognita incited disease complex of eggplant. Indian Phytopathol. 71, 355–364. doi: 10.1007/s42360-018-0064-5
Khan, Z., and Upadhyaya, H. (2019). “Impact of nanoparticles on abiotic stress responses in plants: an overview,” in Nanomaterials in Plants, Algae and Microorganisms, eds D. K. Tripathi, P. Ahmad, S. Sharma, D. K. Chauhan, and N. K. Dubey (Cambridge, MA: Academic Press), 305–322.
Khan, Z. S., Rizwan, M., Hafeez, M., Ali, S., Javed, M. R., and Adrees, M. (2019). The accumulation of cadmium in wheat (Triticum aestivum) as influenced by zinc oxide nanoparticles and soil moisture conditions. Environ. Sci. Pollut. Res. 26, 19859–19870. doi: 10.1007/s11356-019-05333-5
Kiapour, H., Moaveni, P., Habibi, D., and Sani, B. (2015). Evaluation of the application of gibbrellic acid and titanium dioxide nanoparticles under drought stress on some traits of basil (Ocimum basilicum L.). Int. J. Agron. Agric. Res. 6, 138–150.
Kim, D.-Y., Kadam, A., Shinde, S., Saratale, R. G., Patra, J., and Ghodake, G. (2018). Recent developments in nanotechnology transforming the agricultural sector: a transition replete with opportunities. J. Sci. Food Agric. 98, 849–864. doi: 10.1002/jsfa.8749
Kim, J. H., Oh, Y., Yoon, H., Hwang, I., and Chang, Y.-S. (2015). Iron nanoparticle-induced activation of plasma membrane H+-ATPase promotes stomatal opening in Arabidopsis thaliana. Environ. Sci. Technol. 49, 1113–1119. doi: 10.1021/es504375t
Kim, S. W., Jung, J. H., Lamsal, K., Kim, Y. S., Min, J. S., and Lee, Y. S. (2012). Antifungal effects of silver nanoparticles (AgNPs) against various plant pathogenic fungi. Mycobiology 40, 53–58. doi: 10.5941/myco.2012.40.1.053
Kohan-Baghkheirati, E., and Geisler-Lee, J. (2015). Gene expression, protein function and pathways of Arabidopsis thaliana responding to silver nanoparticles in comparison to silver ions, cold, salt, drought, and heat. Nanomaterials 5, 436–467. doi: 10.3390/nano5020436
Konate, A., He, X., Zhang, Z., Ma, Y., Zhang, P., Alugongo, G. M., et al. (2017). Magnetic (Fe3O4) nanoparticles reduce heavy metals uptake and mitigate their toxicity in wheat seedling. Sustainability 9:790. doi: 10.3390/su9050790
Korkin, A., and Rosei, F. (2008). Nanoelectronics and Photonics: From Atoms to Materials, Devices, and Architectures. New York, NY: Springer. 453 p.
Kumari, S., Khanna, R. R., Nazir, F., Albaqami, M., Chhillar, H., Wahid, I., et al. (2022). Bio-synthesized nanoparticles in developing plant abiotic stress resilience: a new boon for sustainable approach. Int. J. Mol. Sci. 23:4452. doi: 10.3390/ijms23084452
Kurepa, J., Paunesku, T., Vogt, S., Arora, H., Rabatic, B. M., Lu, J., et al. (2010). Uptake and distribution of ultrasmall anatase TiO2 Alizarin red S nanoconjugates in Arabidopsis thaliana. Nano Lett. 10, 2296–2302. doi: 10.1021/nl903518f
Kwak, J. M., Nguyen, V., and Schroeder, J. I. (2006). The role of reactive oxygen species in hormonal responses. Plant Physiol. 141, 323–329. doi: 10.1104/pp.106.079004
Lamsal, K., Kim, S. W., Jung, J. H., Kim, Y. S., Kim, K. S., and Lee, Y. S. (2011). Application of silver nanoparticles for the control of Colletotrichum species in vitro and pepper anthracnose disease in field. Mycobiology 39, 194–199. doi: 10.5941/myco.2011.39.3.194
Laware, S. L., and Raskar, S. (2014). Effect of titanium dioxide nanoparticles on hydrolytic and antioxidant enzymes during seed germination in onion. Int. J. Curr. Microbiol. App. Sci. 3, 749–760.
Lee, C. W., Mahendra, S., Zodrow, K., Li, D., Tsai, Y. C., Braam, J., et al. (2010). Developmental phytotoxicity of metal oxide nanoparticles to Arabidopsis thaliana. Environ. Toxicol. Chem. 29, 669–675. doi: 10.1002/etc.58
Lee, D. J., Senseman, S. A., Sciumbato, A. S., Jung, S.-C., and Krutz, L. J. (2003). The effect of titanium dioxide alumina beads on the photocatalytic degradation of picloram in water. J. Agric. Food Chem. 51, 2659–2664. doi: 10.1021/jf026232u
Lee, W. M., An, Y. J., Yoon, H., and Kweon, H. S. (2008). Toxicity and bioavailability of copper nanoparticles to the terrestrial plants mung bean (Phaseolus radiatus) and wheat (Triticum aestivum): plant agar test for water-insoluble nanoparticles. Environ. Toxicol. Chem. 27, 1915–1921. doi: 10.1897/07-481.1
Lee, W. M., Kwak, J. I., and An, Y. J. (2012). Effect of silver nanoparticles in crop plants Phaseolus radiatus and Sorghum bicolor: media effect on phytotoxicity. Chemosphere 86, 491–499. doi: 10.1016/j.chemosphere.2011.10.013
Lei, Z., Mingyu, S., Chao, L., Liang, C., Hao, H., Xiao, W., et al. (2007). Effects of nanoanatase TiO2 on photosynthesis of spinach chloroplasts under different light illumination. Biol. Trace Elem. Res. 119, 68–76. doi: 10.1007/s12011-007-0047-3
Lei, Z., Mingyu, S., Xiao, W., Chao, L., Chunxiang, Q., Liang, C., et al. (2008). Antioxidant stress is promoted by nano-anatase in spinach chloroplasts under UV-B radiation. Biol. Trace Elem. Res. 121, 69–79. doi: 10.1007/s12011-007-8028-0
Li, Y., Zhu, N., Liang, X., Bai, X., Zheng, L., Zhao, J., et al. (2020). Silica nanoparticles alleviate mercury toxicity via immobilization and inactivation of Hg(ii) in soybean (Glycine max). Environ. Sci. Nano 7, 1807–1817. doi: 10.1039/d0en00091d
Li, Z., and Huang, J. (2014). Effects of nanoparticle hydroxyapatite on growth and antioxidant system in pakchoi (Brassica chinensis L.) from cadmium-contaminated soil. J. Nanomater. 2014:470962. doi: 10.1155/2014/470962
Liang, Y., Sun, W., Zhu, Y. G., and Christie, P. (2007). Mechanisms of silicon-mediated alleviation of abiotic stresses in higher plants: a review. Environ Pollut. 147, 422–428. doi: 10.1016/j.envpol.2006.06.008
Lin, D., and Zhing, B. (2007). Phytotoxicity of nanoparticles: inhibition of seed germination and root growth. Environ. Pollut. 150, 243–250. doi: 10.1016/j.envpol.2007.01.016
Liu, R., and Lal, R. (2015). Potentials of engineered nanoparticles as fertilizers for increasing agronomic productions. Sci. Total Environ. 514, 131–139. doi: 10.1016/j.scitotenv.2015.01.104
Lu, L., Huang, M., Huang, Y., Corvini, P. F.-X., Ji, R., and Zhao, L. (2020). Mn3O4 nanozymes boost endogenous antioxidant metabolites in cucumber (Cucumis sativus) plant and enhance resistance to salinity stress. Environ. Sci. Nano 7, 1692–1703. doi: 10.1039/d0en00214c
Ma, J. F. (2004). Role of silicon in enhancing the resistance of plants to biotic and abiotic stresses. Soil Sci. Plant Nutr. 50, 11–18. doi: 10.1080/00380768.2004.10408447
Ma, J. F., and Yamaji, N. (2006). Silicon uptake and accumulation in higher plants. Trends Plant Sci. 11, 392–397. doi: 10.1016/j.tplants.2006.06.007
Ma, X., Geisler-Lee, J., Deng, Y., and Kolmakov, A. (2010). Interactions between engineered nanoparticles (ENPs) and plants: phytotoxicity, uptake and accumulation. Sci. Total Environ. 408, 3053–3061. doi: 10.1016/j.scitotenv.2010.03.031
Madhavi, V., Prasad, T., Reddy, A. V. B., and Madhavi, G. (2013). Plant growth promoting potential of nano-bioremediation under Cr (VI) stress. Int. J. Nanotechnol. Appl. 3, 1–10.
Mahmoodzadeh, H., Nabavi, M., and Kashefi, H. (2013). Effect of nanoscale titanium dioxide particles on the germination and growth of canola (Brassica napus). J. Ornament. Plants 3, 25–32.
Mahmoud, L. M., Dutt, M., Shalan, A. M., El-Kady, M. E., El-Boray, M. S., Shabana, Y. M., et al. (2020). Silicon nanoparticles mitigate oxidative stress of in vitro-derived banana (Musa acuminata ‘Grand Nain’) under simulated water deficit or salinity stress. S. Afr. J. Bot. 132, 155–163. doi: 10.1016/j.sajb.2020.04.027
Manzoor, N., Ahmed, T., Noman, M., Shahid, M., Nazir, M. M., Ali, L., et al. (2021). Iron oxide nanoparticles ameliorated the cadmium and salinity stresses in wheat plants, facilitating photosynthetic pigments and restricting cadmium uptake. Sci. Total Environ. 769:145221. doi: 10.1016/j.scitotenv.2021.145221
Maswada, H. F., Djanaguiraman, M., and Prasad, P. V. V. (2018). Seed treatment with nano-iron (III) oxide enhances germination, seeding growth and salinity tolerance of sorghum. J. Agro. Crop Sci. 204, 577–587. doi: 10.1111/jac.12280
Mazumdar, H., and Ahmed, G. U. (2011). Phytotoxicity effect of silver nanoparticles on Oryza sativa. Int. J. ChemTech. Res. 3, 1494–1500.
Mahdi Nezhad, N., Mousavi, H., Fakheri, B., and Heidari, F. (2018). The effect of some nanoparticles on the activity of antioxidant enzymes and parthenolide yield of feverfew plant (Tanacetum parthenium L.) under water deficit stress. Env. Stresses Crop Sci. 11, 917–929. doi: 10.22077/ESCS.2018.1051.1210
Mengiste, T., Laluk, K., and AbuQamar, S. (2010). “Mechanisms of induced resistance against B. cinerea,” in Post-harvest Pathology, Plant Pathology in the 21st Century, eds D. Prusky and M. L. Gullino (Dordrecht: Springer), 13–30.
Mirzajani, F., Askari, H., Hamzelou, S., Farzaneh, M., and Ghassempour, A. (2013). Effect of silver nanoparticles on Oryza sativa L. and its rhizosphere bacteria. Ecotoxicol. Environ. Saf. 88, 48–54. doi: 10.1016/j.ecoenv.2012.10.018
Mishra, V., Mishra, R. K., Dikshit, A., and Pandey, A. C. (2014). “Interactions of nanoparticles with plants: an emerging prospective in the agriculture industry,” in Emerging Technologies and Management of Crop Stress Tolerance, Vol. 1, eds P. Ahmad and S. Rasool (San Diego, CA: Academic press), 159–180.
Moameri, M., and Abbasi Khalaki, M. (2019). Capability of Secale montanum trusted for phytoremediation of lead and cadmium in soils amended with nano-silica and municipal solid waste compost. Environ. Sci. Pollut. Res. 26, 24315–24322. doi: 10.1007/s11356-017-0544-7
Moameri, M., Abbasi Khalaki, M., and Ghorbani, A. (2018a). Effects of nanopriming and biopriming on growth characteristics of Onobrychis sativa Lam. under laboratory conditions. Rangelands 12, 101–111.
Moameri, M., Jafari, M., Tavili, A., Motasharezadeh, B., Zare Chahouki, M. A., and Madrid Diaz, F. (2018b). Investigating lead and zinc uptake and accumulation by Stipa hohenackeriana trin and rupr in field and pot experiments. Biosci. J. 34, 138–150. doi: 10.14393/BJ-v34n1a2018-37238
Mohamed, A., Qayyum, M. F., Abdel-Hadi, A., Rehman, R. A., Ali, S., and Rizwan, M. (2017). Interactive effect of salinity and silver nanoparticles on photosynthetic and biochemical parameters of wheat. Arch. Agron. Soil Sci. 63, 1736–1747. doi: 10.1080/03650340.2017.1300256
Mohammadi, H., Esmailpour, M., and Gheranpaye, A. (2016). Effects of TiO2 nanoparticles and water-deficit stress on morpho-physiological characteristics of dragonhead (Dracocephalum moldavica L.) plants. Acta Agric. Slov. 107, 385–396. doi: 10.14720/aas.2016.107.2.11
Mohammadi, R., Maali-Amiri, R., and Abbasi, A. (2013). Effect of TiO2 nanoparticles on chickpea response to cold stress. Biol. Trace Elem. Res. 152, 403–410. doi: 10.1007/s12011-013-9631-x
Mohammadi, R., Maali-Amiri, R., and Mantri, N. L. (2014). Effect of TiO2 nanoparticles on oxidative damage and antioxidant defense systems in chickpea seedlings during cold stress. Russ. J. Plant Physiol. 61, 768–775. doi: 10.1134/S1021443714050124
Monica, R. C., and Cremonini, R. (2009). Nanoparticles and higher plants. Caryologia 62, 161–165. doi: 10.1080/00087114.2004.10589681
Moradbeygi, H., Jamei, R., Heidari, R., and Darvishzadeh, R. (2020a). Fe2O3 nanoparticles induced biochemical responses and expression of genes involved in rosmarinic acid biosynthesis pathway in Moldavian balm under salinity stress. Physiol. Plantarum. 169, 555–570. doi: 10.1111/ppl.13077
Moradbeygi, H., Jamei, R., Heidari, R., and Darvishzadeh, R. (2020b). Investigating the enzymatic and non-enzymatic antioxidant defense by applying iron oxide nanoparticles in Dracocephalum moldavica L. plant under salinity stress. Sci. Hortic. 272:109537. doi: 10.1016/j.scienta.2020.109537
Mozafari, A., Ghadakchi Asl, A., and Ghaderi, N. (2018a). Grape response to salinity stress and role of iron nanoparticle and potassium silicate to mitigate salt induced damage under in vitro conditions. Physiol. Mol. Biol. Plants. 24, 25–35. doi: 10.1007/s12298-017-0488-x
Mozafari, A., Havas, F., and Ghaderi, N. (2018b). Application of iron nanoparticles and salicylic acid in in vitro culture of strawberries (Fragaria× ananassa Duch.) to cope with drought stress. Plant Cell Tissue Organ. Cult. 132, 511–523. doi: 10.1007/s11240-017-1347-8
Mukherjee, A., Peralta-Videa, J. R., Bandyopadhyay, S., Rico, C. M., Zhao, L., and Gardea-Torresdey, J. L. (2014). Physiological effects of nanoparticulate ZnO in green peas (Pisum sativum L.) cultivated in soil. Metallomics 6, 132–138. doi: 10.1039/c3mt00064h
Mustafa, G., and Komatsu, S. (2016). Insights into the response of soybean mitochondrial proteins to various sizes of aluminum oxide nanoparticles under flooding stress. J. Proteome Res. 15, 4464–4475. doi: 10.1021/acs.jproteome.6b00572
Mustafa, G., Sakata, K., Hossain, Z., and Komatsu, S. (2015). Proteomic study on the effects of silver nanoparticles on soybean under flooding stress. J. Proteomics 122, 100–118. doi: 10.1016/j.jprot.2015.03.030
Nair, P. M. G., and Chung, I. M. (2014). Impact of copper oxide nanoparticles exposure on Arabidopsis thaliana growth, root system development, root lignificaion, and molecular level changes. Environ. Sci. Pollut. Res. 21, 12709–12722. doi: 10.1007/s11356-014-3210-3
Nair, P. M. G., and Chung, I. M. (2017). Regulation of morphological, molecular and nutrient status in Arabidopsis thaliana seedlings in response to ZnO nanoparticles and Zn ion exposure. Sci. Total Environ. 575, 187–198. doi: 10.1016/j.scitotenv.2016.10.017
Nair, R., Varghese, S. H., Nair, B. G., Maekawa, T., Yoshida, Y., and Kumar, D. S. (2010). Nanoparticulate material delivery to plants. Plant Sci. 179, 154–163. doi: 10.1016/j.plantsci.2010.04.012
Najafi Disfani, M., Mikhak, A., Kassaeec, M. Z., and Magharid, A. H. (2017). Effects of nano Fe/SiO2 fertilizers on germination and growth of barley and maize. Arch. Agron. Soil Sci. 63, 817–826. doi: 10.1080/03650340.2016.1239016
Namasivayam, S., and Chitrakala, K. (2011). Ecotoxicological effect of Lecanicillium lecanii (Ascomycota: Hypocreales) based silver nanoparticles on growth parameters of economically important plants. J. Biopestic. 4, 97–101.
Navarro, E., Piccapietra, F., Wagner, B., Marconi, F., Kaegi, R., Odzak, N., et al. (2008). Toxicity of silver nanoparticles to Chlamydomonas reinhardtii. Environ. Sci. Technol. 42, 8959–8964. doi: 10.1021/es801785m
Nayan, R., Rawat, M., Negi, B., Pande, A., and Arora, S. (2016). Zinc sulfide nanoparticle mediated alterations in growth and anti-oxidant status of Brassica juncea. Biologia 71, 896–902. doi: 10.1515/biolog-2016-0107
Nejatzadeh, F. (2021). Effect of silver nanoparticles on salt tolerance of Satureja hortensis L. during in vitro and in vivo germination tests. Heliyon 7:e05981. doi: 10.1016/j.heliyon.2021.e05981
Nel, A., Xia, T., Mädler, L., and Li, N. (2006). Toxic potential of materials at the nanolevel. Science 311, 622–627. doi: 10.1126/science.1114397
Noman, M., Shahid, M., Ahmed, T., Tahir, M., Naqqash, T., Muhammad, S., et al. (2020). Green copper nanoparticles from a native Klebsiella pneumoniae strain alleviated oxidative stress impairment of wheat plants by reducing the chromium bioavailability and increasing the growth. Ecotoxicol. Environ. Saf. 192:110303. doi: 10.1016/j.ecoenv.2020.110303
Noohpisheh, Z., Amiri, H., Mohammadi, A., and Farhadi, S. (2021). Effect of the foliar application of zinc oxide nanoparticles on some biochemical and physiological parameters of Trigonella foenum-graecum under salinity stress. Plant Biosyst. 155, 267–280. doi: 10.1080/11263504.2020.1739160
O’Brien, J. A., and Benková, E. (2013). Cytokinin cross-talking during biotic and abiotic stress responses. Front. Plant Sci. 4:451. doi: 10.3389/fpls.2013.00451
Onaga, G., and Wydra, K. (2016). “Advances in plant tolerance to abiotic stresses,” in Plant Genomics, ed. I. Y. Abdurakhmonov (London: IntechOpen), 229–272.
Owolade, O., and Ogunleti, D. (2008). Effects of titanium dioxide on the diseases, development and yield of edible cowpea. J. Plant Prot. Res. 48, 329–335. doi: 10.2478/v10045-008-0042-5
Pakrashi, S., Jain, N., Dalai, S., Jayakumar, J., Chandrasekaran, P. T., Raichur, A. M., et al. (2014). In vivo genotoxicity assessment of titanium dioxide nanoparticles by Allium cepa root tip assay at high exposure concentrations. PLoS One 9:e87789. doi: 10.1371/journal.pone.0087789
Pallavi Mehta, C. M., Srivastava, R., Arora, S., and Sharma, A. K. (2016). Impact assessment of silver nanoparticles on plant growth and soil bacterial diversity. 3 Biotech 6:254. doi: 10.1007/s13205-016-0567-7
Palmqvist, N. G. M., Seisenbaeva, G. A., Svedlindh, P., and Kessler, V. G. (2017). Maghemite nanoparticles acts as nanozymes, improving growth and abiotic stress tolerance in Brassica napus. Nanoscale Res. Lett. 12:631. doi: 10.1186/s11671-017-2404-2
Panda, S. (2017). Physiological impact of Zinc nanoparticle on germination of rice (Oryza sativa L) seed. J. Plant Sci Phytopathol. 1, 62–70.
Pandey, A. C., Sanjay, S. S., and Yadav, R. S. (2010). Application of ZnO nanoparticles in influencing the growth rate of Cicer arietinum. J. Exp. Nanosci. 5, 488–497. doi: 10.1080/17458081003649648
Paramo, L. A., Feregrino-Pérez, A. A., Guevara, R., Mendoza, S., and Esquivel, K. (2020). Nanoparticles in agroindustry: applications, toxicity, challenges, and trends. Nanomaterials 10:1654. doi: 10.3390/nano10091654
Parveen, A., and Rao, S. (2015). Effect of nanosilver on seed germination and seedling growth in Pennisetum glaucum. J. Clust. Sci. 26, 693–701. doi: 10.1007/s10876-014-0728-y
Pei, Z. F., Ming, D. F., Liu, D., Wan, G. L., Geng, X. X., Gong, H. J., et al. (2010). Silicon improves the tolerance to water-deficit stress induced by polyethylene glycol in wheat (Triticum aestivum L.) seedlings. J. Plant Growth Regul. 29, 106–115. doi: 10.1007/s00344-009-9120-9
Perreault, F., Samadani, M., and Dewez, D. (2014). Effect of soluble copper released from copper oxide nanoparticles solubilisation on growth and photosynthetic processes of Lemna gibba L. Nanotoxicology 8, 374–382. doi: 10.3109/17435390.2013.789936
Peyvandi, M., Kamali Jamakani, Z., and Mirza, M. (2011a). Comparison of nano Fe chelate with Fe chelate effect on growth parameters and antioxidant enzymes activity of Satureja hortensis. New Cell Mol. Biotech. 2, 2–32.
Peyvandi, M., Parandeh, H., and Mirza, M. (2011b). Comparison of nano Fe chelate with Fe chelate effect on growth parameters and antioxidant enzymes activity of Ocimum basilicum. New Cell Mol. Biotech. J. 1, 89–98.
Prasad, R., Bhattacharyya, A., and Nguyen, Q. D. (2017). Nanotechnology in sustainable agriculture: recent developments, challenges, and perspectives. Front. Microbiol. 8:1014. doi: 10.3389/fmicb.2017.01014
Prasad, T. N. V. K. V., Sudhakar, P., Sreenivasulu, Y., Latha, P., Munaswamy, V., Reddy, K. R., et al. (2012). Effect of nanoscale zinc oxide particles on the germination, growth and yield of peanut. J. Plant Nutr. 35, 905–927. doi: 10.1080/01904167.2012.663443
Prazak, R., Swieciło, A., Krzepiłko, A., Michałek, S., and Arczewska, M. (2020). Impact of Ag nanoparticles on seed germination and seedling growth of green beans in normal and chill temperatures. Agriculture 10:312. doi: 10.3390/agriculture10080312
Qados, A. M. A. (2015). Mechanism of nanosilicon-mediated alleviation of salinity stress in faba bean (Vicia faba L.) plants. Am. J. Exp. Agric. 7, 78–95. doi: 10.9734/ajea/2015/15110
Qados, A. M. A., and Moftah, A. E. (2015). Influence of silicon and nano-silicon on germination, growth and yield of faba bean (Vicia faba L.) under salt stress conditions. J. Exp. Agric. Int. 5, 509–524. doi: 10.9734/ajea/2015/14109
Qi, M., Liu, Y., and Li, T. (2013). Nano-TiO2 improve the photosynthesis of tomato leaves under mild heat stress. Biol. Trace Elem. Res. 156, 323–328. doi: 10.1007/s12011-013-9833-2
Rady, M. M., Boriek, S. H. K., Abd El-Mageed, T. A., Seif El-Yazal, M. A., Ali, E. F., Hassan, F. A. S., et al. (2021). Exogenous gibberellic acid or dilute bee honey boosts drought stress tolerance in Vicia faba by rebalancing osmoprotectants, antioxidants, nutrients, and phytohormones. Plants 10:748. doi: 10.3390/plants10040748
Rahmatizadeh, R., Javad Arvin, S. M., Jamei, R., Mozaffari, H., and Nejhad, F. R. (2019). Response of tomato plants to interaction effects of magnetic (Fe3O4) nanoparticles and cadmium stress. J. Plant Interact. 14, 474–481. doi: 10.1080/17429145.2019.1626922
Rajput, V., Minkina, T., Ahmed, B., Sushkova, S., Singh, R., Soldatov, M., et al. (2020). Interaction of copper-based nanoparticles to soil, terrestrial, and aquatic systems: critical review of the state of the science and future perspectives. Rev. Environ. Contamin. Toxicol. 252, 51–96.
Rajput, V., Minkina, T., Fedorenko, A., Sushkova, S., Mandzhieva, S., Lysenko, V., et al. (2018). Toxicity of copper oxide nanoparticles on spring barley (Hordeum sativum distichum). Sci. Total Environ. 645, 1103–1113.
Rajput, V. D., Minkina, T., Kumari, A., Singh, V. K., Verma, K. K., Mandzhieva, S., et al. (2021). Coping with the challenges of abiotic stress in plants: new dimensions in the field application of nanoparticles. Plants 10:1221. doi: 10.3390/plants10061221
Raliya, R., and Tarafdar, J. C. (2013). ZnO nanoparticle biosynthesis and its effect on phosphorous-mobilizing enzyme secretion and gum contents in clusterbean (Cyamopsis tetragonoloba L.). Agric. Res. 2, 48–57. doi: 10.1007/s40003-012-0049-z
Ramezani, F., Shayanfar, A., Tavakkol Afshari, R., and Rezaee, K. (2014). Effects of silver, nickel, zinc and zinc-copper nanoparticles on germination, seedling establishment and enzyme activity of alfalfa (Medicago sativa) seed. Iran J. Field Crop Sci. 45, 107–118. doi: 10.22059/ijfcs.2014.51038
Rasheed, A., Seleiman, M. F., Nawaz, M., Mahmood, A., Anwar, M. R., Ayub, M. A., et al. (2021). Agronomic and genetic approaches for enhancing tolerance to heat stress in rice: a review. Not. Bot. Horti. Agrobot. Cluj Napoca 49:12501.
Rastogi, A., Tripathi, D. K., Yadav, S., Chauhan, D. K., Živèák, M., Ghorbanpour, M., et al. (2019). Application of silicon nanoparticles in agriculture. 3 Biotech 9:90. doi: 10.1007/s13205-019-1626-7
Raven, J. A. (1983). The transport and function of silicon in plants. Biol. Rev. 58, 179–207. doi: 10.1111/j.1469-185X.1983.tb00385.x
Rawson, H. M., Long, M. J., and Munns, R. (1988). Growth and development in NaCl-treated plants. I. leaf Na+ and Cl– concentrations do not determine gas exchange of leaf blades in barley. Funct. Plant Biol. 15, 519–527. doi: 10.1071/PP9880519
Rédei, G. P. (2008). Encyclopedia of Genetics, Genomics, Proteomics, and Informatics, 3rd Edn. Dordrecht: Springer. 2201 p.
Rezvani, N., Sorooshzadeh, A., and Farhadi, N. (2012). Effect of nano-silver on growth of saffron in flooding stress. World Acad. Sci. Eng. Technol. 6, 517–522.
Rizwan, M., Ali, S., Qayyum, M. F., Ok, Y. S., Adrees, M., Ibrahim, M., et al. (2017). Effect of metal and metal oxide nanoparticles on growth and physiology of globally important food crops: a critical review. J. Hazard. Mater. 322, 2–16. doi: 10.1016/j.jhazmat.2016.05.061
Rizwan, M., Ali, S., Zia Ur Rehman, M., Adrees, M., Arshad, M., Qayyum, M. F., et al. (2019a). Alleviation of cadmium accumulation in maize (Zea mays L.) by foliar spray of zinc oxide nanoparticles and biochar to contaminated soil. Environ. Pollut. 248, 358–367. doi: 10.1016/j.envpol.2019.02.031
Rizwan, M., Noureen, S., Ali, S., Anwar, S., Rehman, M. Z. U., Qayyum, M. F., et al. (2019b). Influence of biochar amendment and foliar application of iron oxide nanoparticles on growth, photosynthesis, and cadmium accumulation in rice biomass. J. Soils Sediments 19, 3749–3759. doi: 10.1007/s11368-019-02327-1
Rizwan, M., Ali, S., Ali, B., Adrees, M., Arshad, M., Hussain, A., et al. (2019c). Zinc and iron oxide nanoparticles improved the plant growth and reduced the oxidative stress and cadmium concentration in wheat. Chemosphere 214, 269–277. doi: 10.1016/j.chemosphere.2018.09.120
Rossi, L., Fedenia, L. N., Sharifan, H., Ma, X., and Lombardini, L. (2019). Effects of foliar application of zinc sulfate and zinc nanoparticles in coffee (Coffea arabica L.) plants. Plant Physiol. Biochem. 135, 160–166. doi: 10.1016/j.plaphy.2018.12.005
Rossi, L., Zhang, W., Lombardini, L., and Ma, X. (2016). The impact of cerium oxide nanoparticles on the salt stress responses of Brassica napus L. Environ. Pollut. 219, 28–36. doi: 10.1016/j.envpol.2016.09.060
Roy, R., Núñez-Delgado, A., Sultana, S., Wang, J., Munir, A., Battaglia, M. L., et al. (2021). Additions of optimum water spent mushroom compost and wood biochar to improve the growth performance of Althaea rosea in drought-prone coal-mined spoils. J. Environ. Manag. 295:113076. doi: 10.1016/j.jenvman.2021.113076
Rui, Y. (2021). Nanoparticles Alleviate Heavy Metals Stress. Available online at: https://encyclopedia.pub/7093, (accessed June 2, 2021).
Saad, A. M., El-Saadony, M. T., El-Tahan, A. M., Sayed, S., Moustafa, M. A., Taha, A. E., et al. (2021). Polyphenolic extracts from pomegranate and watermelon wastes as substrate to fabricate sustainable silver nanoparticles with larvicidal effect against Spodoptera littoralis. Saudi J. Biol. Sci. 28, 5674–5683. doi: 10.1016/j.sjbs.2021.06.011
Sabaghnia, N., and Janmohammadi, M. (2014). Effect of nano-silicon particles application on salinity tolerance in early growth of some lentil genotypes. Ann. UMCS Biol. 69, 39–55. doi: 10.1515/umcsbio-2015-0004
Sadak, M. S. (2019). Impact of silver nanoparticles on plant growth, some biochemical aspects, and yield of fenugreek plant (Trigonella foenum-graecum). Bull. Natl. Res. Cent. 43, 1–6. doi: 10.1186/s42269-019-0077-y
Saha, N., and Dutta Gupta, S. (2017). Low-dose toxicity of biogenic silver nanoparticles fabricated by Swertia chirata on root tips and flower buds of Allium cepa. J. Hazard. Mater. 330, 18–28. doi: 10.1016/j.jhazmat.2017.01.021
Salem, H. M., Ismael, E., and Shaalan, M. (2021). Evaluation of the effects of silver nanoparticles against experimentally induced necrotic enteritis in broiler chickens. Int. J. Nanomedicine 16, 6783–6796. doi: 10.2147/IJN.S319708
Santana, I., Wu, H., Hu, P., and Giraldo, J. P. (2020). Targeted delivery of nanomaterials with chemical cargoes in plants enabled by a biorecognition motif. Nat. Commun. 11:2045.
Savvas, D., Giotis, D., Chatzieustratiou, E., Bakea, M., and Patakioutas, G. (2009). Silicon supply in soilless cultivations of zucchini alleviates stress induced by salinity and powdery mildew infections. Environ. Exp. Bot. 65, 11–17. doi: 10.1016/j.envexpbot.2008.07.004
Saxena, R., Tomar, R. S., and Kumar, M. (2016). Exploring nanobiotechnology to mitigate abiotic stress in crop plants. J. Pharm Sci. Res. 8, 974–980.
Sedghi, M., Mitra, H., and Sahar, T. (2013). Effect of nano zinc oxide on the germination of soybean seeds under drought stress. Ann. West Univ. Timiş. Ser. Biol. 16, 73–78.
Seghatoleslami, M., Feizi, H., Mousavi, G., and Berahmand, A. (2015). Effect of magnetic field and silver nanoparticles on yield and water use efficiency of Carum copticum under water stress conditions. Pol. J. Chem. Technol. 17, 110–114. doi: 10.1515/pjct-2015-0016
Seghatoleslami, M. J., and Forutani, R. (2015). Yield and water use efficiency of sunflower as affected by nano ZnO and water stress. J. Adv. Agric. Technol. 2, 34–37.
Seleiman, M. F., Alotaibi, M. A., Alhammad, B. A., Alharbi, B. M., Refay, Y., and Badawy, S. A. (2020a). Effects of ZnO nanoparticles and biochar of rice straw and cow manure on characteristics of contaminated soil and sunflower productivity, oil quality, and heavy metals uptake. Agronomy 10:790. doi: 10.3390/agronomy10060790
Seleiman, M. F., Santanen, A., and Mäkelä, P. (2020b). Recycling sludge on cropland as fertilizer-Advantages and risks. Resour. Conserv. Recycle 155:104647. doi: 10.1016/j.resconrec.2019.104647
Seleiman, M. F., Semida, W. M., Rady, M. M., Mohamed, G. F., Hemida, K. A., Alhammad, B. A., et al. (2020c). Sequential application of antioxidants rectifies ion imbalance and strengthens antioxidant systems in salt-stressed cucumber. Plants 9:1783. doi: 10.3390/plants9121783
Seleiman, M. F., Al-Suhaibani, N., Ali, N., Akmal, M., Alotaibi, M., Refay, Y., et al. (2021a). Drought stress impacts on plants and different approaches to alleviate its adverse effects. Plants 10:259. doi: 10.3390/plants10020259
Seleiman, M. F., Almutairi, K. F., Alotaibi, M., Shami, A., Alhammad, B. A., and Battaglia, M. L. (2021b). Nano-fertilization as an emerging fertilization technique: why can modern agriculture benefit from its use? Plants 10:2. doi: 10.3390/plants10010002
Seleiman, M. F., Aslam, M. T., Alhammad, B. A., Hassan, M. U., Maqbool, R., Chattha, M. U., et al. (2022). Salinity stress in wheat: effects, mechanisms and management strategies. Phyton 91:667. doi: 10.32604/phyton.2022.017365
Semida, W. M., Abd El-Mageed, T. A., and Howladar, S. M. (2014). A novel organo-mineral fertilizer can alleviate negative effects of salinity stress for eggplant production on reclaimed saline calcareous soil. Acta Hortic. 1034, 493–499.
Semida, W. M., Abdelkhalik, A., Mohamed, G. F., Abd El-Mageed, T. A., Abd El-Mageed, S. A., Rady, M. M., et al. (2021). Foliar application of zinc oxide nanoparticles promotes drought stress tolerance in eggplant (Solanum melongena L.). Plants 10:421. doi: 10.3390/plants10020421
Semida, W. M., Abdelkhalik, A. A., Rady, M. O. A., Marey, R. A., and Abd El-Mageed, T. A. (2020). Exogenously applied proline enhances growth and productivity of drought stressed onion by improving photosynthetic efficiency, water use efficiency and up-regulating osmoprotectants. Sci. Hortic. 272:109580. doi: 10.1016/j.scienta.2020.109580
Shaaban, A., Al-Elwany, O. A., Abdou, N. M., Hemida, K. A., El-Sherif, A., Abdel-Razek, M. A., et al. (2022). Filter mud enhanced yield and soil properties of water-stressed Lupinus termis L. in saline calcareous soil. J. Soil Sci. Plant Nutr. 22, 1572–1588. doi: 10.1007/s42729-021-00755-y
Shafiee-Jood, M., and Cai, X. (2016). Reducing food loss and waste to enhance food security and environmental sustainability. Environ. Sci. Technol. 50, 8432–8443. doi: 10.1021/acs.est.6b01993
Shah, T., Latif, S., Saeed, F., Ali, I., Ullah, S., Abdullah Alsahli, A., et al. (2021). Priming with titanium dioxide nanoparticles enhances seed vigor, leaf water status, and antioxidant enzyme activities in maize (Zea mays L.) under salinity stress. J. King Saud. Univ. Sci. 33:101207. doi: 10.1016/j.jksus.2020.10.004
Shalaby, T. A., Bayoumi, Y., Abdalla, N., Taha, H., Alshaal, T., Shehata, S., et al. (2016). “Nanoparticles, soils, plants and sustainable agriculture,” in Nanoscience in Food and Agriculture 1, eds S. Ranjan, N. Dasgupta, and E. Lichtfouse (Switzerland: Springer), 283–312.
Shallan, M. A., Hassan, H. M., Namich, A. A., and Ibrahim, A. A. (2016). Biochemical and physiological effects of TiO2 and SiO2 nanoparticles on cotton plant under drought stress. Res. J. Pharm. Biol. Chem. Sci. 7, 1540–1551.
Sham, A., Al-Ashram, H., Whitely, K., El-Tarabily, K. A., Iratni, R., and AbuQamar, S. F. (2019). Metatranscriptomic analysis of multiple environmental stresses identifies RAP2.4 gene associated with Arabidopsis immunity to Botrytis cinerea. Sci. Rep. 9:17010. doi: 10.1038/s41598-019-53694-1
Sham, A., Moustafa, K., Al-Shamisi, S., Alyan, S., Iratni, R., and AbuQamar, S. F. (2017). Microarray analysis of Arabidopsis WRKY33 mutants in response to the necrotrophic fungus Botrytis cinerea. PLoS One 12:e0172343. doi: 10.1371/journal.pone.0172343
Shang, Y., Hasan, M., Ahammed, G. J., Li, M., Yin, H., and Zhou, J. (2019). Applications of nanotechnology in plant growth and crop protection: a review. Molecules 24:2558. doi: 10.3390/molecules24142558
Sharifi-Rad, J., Karimi, J., Mohsenzadeh, S., Sharifi-Rad, M., and Moradgholi, J. (2014). Evaluating SiO2 nanoparticles effects on developmental characteristic and photosynthetic pigment contents of Zea mays L. Bull. Environ. Pharm. Life Sci. 3, 194–201.
Sharma, P., Bhatt, D., Zaidi, M. G. H., Saradhi, P. P., Khanna, P. K., and Arora, S. (2012a). Silver nanoparticle-mediated enhancement in growth and antioxidant status of Brassica juncea. Appl. Biochem. Biotechnol. 167, 2225–2233. doi: 10.1007/s12010-012-9759-8
Sharma, P., Jha, A. B., Dubey, R. S., and Pessarakli, M. (2012b). Reactive oxygen species, oxidative damage, and antioxidative defense mechanism in plants under stressful conditions. J. Bot. 2012:217037. doi: 10.1155/2012/217037
Shaw, A. K., Ghosh, S., Kalaji, H. M., Bosa, K., Brestic, M., Zivcak, M., et al. (2014). Nano-CuO stress induced modulation of antioxidative defense and photosynthetic performance of Syrian barley (Hordeum vulgare L.). Environ. Exp. Bot. 102, 37–47. doi: 10.1016/j.envexpbot.2014.02.016
Shelar, G. B., and Chavan, A. M. (2015). Myco-synthesis of silver nanoparticles from Trichoderma harzianum and its impact on germination status of oil seed. Biolife 3, 109–113. doi: 10.13140/RG.2.2.17609.16484
Shen, X., Xiao, X., Dong, Z., and Chen, Y. (2014). Silicon effects on antioxidative enzymes and lipid peroxidation in leaves and roots of peanut under aluminum stress. Acta Physiol. Plant 36, 3063–3069. doi: 10.1007/s11738-014-1676-8
Shi, Y., Zhang, Y., Han, W., Feng, R., Hu, Y., Guo, J., et al. (2016). Silicon enhances water stress tolerance by improving root hydraulic conductance in Solanum lycopersicum L. Front. Plant Sci. 7:196. doi: 10.3389/fpls.2016.00196
Shojaei, T. R., Salleh, M. A. M., Tabatabaei, M., Mobli, H., Aghbashlo, M., Rashid, S. A., et al. (2019). “Applications of nanotechnology and carbon nanoparticles in agriculture,” in Synthesis, Technology and Applications of Carbon Nanomaterials, eds S. Abdul Rashid, R. N. I. R. Othman, and M. Z. Hussein (Amsterdam: Elsevier), 247–277.
Siddiqui, M. H., Al Whaibi, M. H., Faisal, M., and Al Sahli, A. A. (2014). Nano silicon dioxide mitigates the adverse effects of salt stress on Cucurbita pepo L. Environ. Toxicol. Chem. 33, 2429–2437. doi: 10.1002/etc.2697
Silva, S., Ribeiro, T. P., Santos, C., Pinto, D. C., and Silva, A. M. (2020). TiO2 nanoparticles induced sugar impairments and metabolic pathway shift towards amino acid metabolism in wheat. J. Hazard. Mater. 399:122982. doi: 10.1016/j.jhazmat.2020.122982
Singh, J., and Lee, B. K. (2016). Influence of nano-TiO2 particles on the bioaccumulation of Cd in soybean plants (Glycine max): a possible mechanism for the removal of Cd from the contaminated soil. J. Environ. Manage. 170, 88–96. doi: 10.1016/j.jenvman.2016.01.015
Singh, M. D., Jayadeva, H. M., and Chirag Gautam Mohan, M. H. (2017). Effects of nano zinc oxide particles on seedling growth of maize (Zea mays L.) in germinating paper test. Int. J. Microbiol. Res. 9, 897–898.
Singh, S., Tripathi, D. K., Dubey, N. K., and Chauhan, D. K. (2016). Effects of nano-materials on seed germination and seedling growth: striking the slight balance between the concepts and controversies. Mater. Focus 5, 195–201. doi: 10.1166/mat.2016.1329
Singhal, J., Verma, S., and Kumar, S. (2022). The physio-chemical properties and applications of 2D nanomaterials in agricultural and environmental sustainability. Sci. Total Environ. 837:155669. doi: 10.1016/j.scitotenv.2022.155669
Sofy, M. R., Seleiman, M. F., Alhammad, B. A., Alharbi, B. M., and Mohamed, H. I. (2020). Minimizing adverse effects of Pb on maize plants by combined treatment with jasmonic, salicylic acids and proline. Agronomy 10:699. doi: 10.3390/agronomy10050699
Solanki, P., Bhargava, A., Chhipa, H., Jain, N., and Panwar, J. (2015). “Nano-fertilizers and their smart delivery system,” in Nanotechnologies in Food and Agriculture, eds M. Rai, C. Ribeiro, L. Mattoso, and N. Duran (Switzerland: Springer), 81–101.
Song, G., Gao, Y., Wu, H., Hou, W., Zhang, C., and Ma, H. (2012). Physiological effect of anatase TiO2 nanoparticles on Lemna minor. Environ. Toxicol. Chem. 31, 2147–2152. doi: 10.1002/etc.1933
Staroń, A., Długosz, O., Pulit-Prociak, J., and Banach, M. (2020). Analysis of the exposure of organisms to the action of nanomaterials. Materials 13:349.
Sun, L., Song, F., Guo, J., Zhu, X., Liu, S., Liu, F., et al. (2020). Nano-ZnO-induced drought tolerance is associated with melatonin synthesis and metabolism in maize. Int. J. Mol. Sci. 21:782. doi: 10.3390/ijms21030782
Suriyaprabha, R., Karunakaran, G., Yuvakkumar, R., Prabu, P., Rajendran, V., and Kannan, N. (2012). Growth and physiological responses of maize (Zea mays L.) to porous silica nanoparticles in soil. J. Nanopart. Res. 14:1294. doi: 10.1007/s11051-012-1294-6
Syu, Y. Y., Hung, J. H., Chen, J. C., and Chuang, H. W. (2014). Impacts of size and shape of silver nanoparticles on Arabidopsis plant growth and gene expression. Plant Physiol. Biochem. 83, 57–64. doi: 10.1016/j.plaphy.2014.07.010
Taha, R., Seleiman, M. F., Alotaibi, M., Alhammad, B. A., Rady, M. M., and Mahdi, A. H. A. (2020). Exogenous potassium treatments elevate salt tolerance and performances of Glycine max L. by boosting antioxidant defense system under actual saline field conditions. Agronomy 10:1741. doi: 10.3390/agronomy10111741
Taha, R. S., Seleiman, M. F., Shami, A., Alhammad, B. A., and Mahdi, A. H. A. (2021). Integrated application of selenium and silicon enhances growth and anatomical structure, antioxidant defense system and yield of wheat grown in salt-stressed soil. Plants 10:1040. doi: 10.3390/plants10061040
Tantawy, A. S., Salama, Y. A. M., El-Nemr, M. A., and Abdel-Mawgoud, A. M. R. (2015). Nano silicon application improves salinity tolerance of sweet pepper plants. Int. J. ChemTech Res. 8, 11–17.
Tarafdar, J. C., Raliya, R., Mahawar, H., and Rathore, I. (2014). Development of zinc nanofertilizer to enhance crop production in pearl millet (Pennisetum americanum). Agric. Res. 3, 257–262. doi: 10.1007/s40003-014-0113-y
Taran, N., Storozhenko, V., Svietlova, N., Batsmanova, L., Shvartau, V., and Kovalenko, M. (2017). Effect of zinc and copper nanoparticles on drought resistance of wheat seedlings. Nanoscale Res. Lett. 12:60. doi: 10.1186/s11671-017-1839-9
Tavakkoli, E., Rengasamy, P., and McDonald, G. (2010). High concentrations of Na+ and Cl- ions in soil solution have simultaneous detrimental effects on growth of faba bean under salinity stress. J. Exp. Bot. 61, 4449–4459. doi: 10.1093/jxb/erq251
Tighe-Neira, R., Reyes-Díaz, M., Nunes-Nesi, A., Recio, G., Carmona, E., Corgne, A., et al. (2020). Titanium dioxide nanoparticles provoke transient increase in photosynthetic performance and differential response in antioxidant system in Raphanus sativus L. Sci. Hortic. 269:109418. doi: 10.1016/j.scienta.2020.109418
Tirani, M. M., Haghjou, M. M., and Ismaili, A. (2019). Hydroponic grown tobacco plants respond to zinc oxide nanoparticles and bulk exposures by morphological, physiological and anatomical adjustments. Funct. Plant Biol. 46, 360–375. doi: 10.1071/fp18076
Torabian, S., Zahedi, M., and Khoshgoftar, A. H. (2016). Effects of foliar spray of two kinds of zinc oxide on the growth and ion concentration of sunflower cultivars under salt stress. J. Plant Nutr. 39, 172–180. doi: 10.1080/01904167.2015.1009107
Tripathi, D. K., Singh, S., Singh, S., Mishra, S., Chauhan, D. K., and Dubey, N. K. (2015a). Micronutrients and their diverse role in agricultural crops: advances and future prospective. Acta Physiol. Plant. 37:139. doi: 10.1007/s11738-015-1870-3
Tripathi, D. K., Singh, V. P., Prasad, S. M., Chauhan, D. K., and Dubey, N. K. (2015b). Silicon nanoparticles (SiNp) alleviate chromium (VI) phytotoxicity in Pisum sativum (L.) seedlings. Plant Physiol. Biochem. 96, 189–198. doi: 10.1016/j.plaphy.2015.07.026
Tripathi, D. K., Singh, S., Singh, S., Pandey, R., Singh, V. P., Sharma, N. C., et al. (2017a). An overview on manufactured nanoparticles in plants: uptake, translocation, accumulation and phytotoxicity. Plant Physiol. Biochem. 110, 2–12. doi: 10.1016/j.plaphy.2016.07.030
Tripathi, D. K., Singh, S., Singh, V. P., Prasad, S. M., Dubey, N. K., and Chauhan, D. K. (2017b). Silicon nanoparticles more effectively alleviated UV-B stress than silicon in wheat (Triticum aestivum) seedlings. Plant Physiol. Biochem. 110, 70–81. doi: 10.1016/j.plaphy.2016.06.026
Tripathi, D. K., Singh, S., Singh, S., Srivastava, P. K., Singh, V. P., Singh, S., et al. (2017c). Nitric oxide alleviates silver nanoparticles (AgNps)-induced phytotoxicity in Pisum sativum seedlings. Plant Physiol. Biochem. 110, 167–177. doi: 10.1016/j.plaphy.2016.06.015
Tripathi, D. K., Singh, S., Singh, V. P., Prasad, S. M., Chauhan, D. K., and Dubey, N. K. (2016). Silicon nanoparticles more efficiently alleviate arsenate toxicity than silicon in maize cultiver and hybrid differing in arsenate tolerance. Front. Environ. Sci. 4:46. doi: 10.3389/fenvs.2016.00046
Tunca, E. (2015). Nanoparticles as the base of nanotechnology and phytoremediation of nanoparticles. Ordu Univ. J. Sci. Technol. 5, 23–34.
Turgeon, R. (2010). The puzzle of phloem pressure. Plant Physiol. 154, 578–581. doi: 10.1104/pp.110.161679
Usman, M., Farooq, M., Wakeel, A., Nawaz, A., Cheema, S. A., Rehman, H., et al. (2020). Nanotechnology in agriculture: current status, challenges and future opportunities. Sci. Total Environ. 721:137778. doi: 10.1016/j.scitotenv.2020.137778
Van Breusegem, F., and Dat, J. F. (2006). Reactive oxygen species in plant cell death. Plant Physiol. 141, 384–390. doi: 10.1104/pp.106.078295
van Nguyen, D., Nguyen, H. M., Le, N. T., Nguyen, K. H., Nguyen, H. T., Le, H. M., et al. (2022). Copper nanoparticle application enhances plant growth and grain yield in maize under drought stress conditions. J. Plant Growth Regul. 41, 364–375. doi: 10.1007/s00344-021-10301-w
Vannini, C., Domingo, G., Onelli, E., Prinsi, B., Marsoni, M., Espen, L., et al. (2013). Morphological and proteomic responses of Eruca sativa exposed to silver nanoparticles or silver nitrate. PLoS One 8:e68752. doi: 10.1371/journal.pone.0068752
Venkatachalam, P., Jayaraj, M., Manikandan, R., Geetha, N., Rene, E. R., Sharma, N. C., et al. (2017). Zinc oxide nanoparticles (ZnONPs) alleviate heavy metal-induced toxicity in Leucaena leucocephala seedlings: a physiochemical analysis. Plant Physiol. Biochem. 110, 59–69. doi: 10.1016/j.plaphy.2016.08.022
Vitti, A., Nuzzaci, M., Scopa, A., Tataranni, G., Tamburrino, I., and Sofo, A. (2014). Hormonal response and root architecture in Arabidopsis thaliana subjected to heavy metals. Int. J. Plant Biol. 5:5226. doi: 10.4081/pb.2014.5226
Vundavalli, R., Vundavalli, S., Nakka, M., and Rao, D. S. (2015). Biodegradable nano-hydrogels in agricultural farming-alternative source for water resources. Proc. Mater. Sci. 10, 548–554. doi: 10.1016/j.mspro.2015.06.005
Wahid, I., Kumari, S., Ahmad, R., Hussain, S. J., Alamri, S., Siddiqui, M. H., et al. (2020). Silver nanoparticle regulates salt tolerance in wheat through changes in ABA concentration, ion homeostasis, and defense systems. Biomolecules 10:1506. doi: 10.3390/biom10111506
Wang, H., Kou, X., Pei, Z., Xiao, J. Q., Shani, X., and Xing, B. (2011). Physiological effects of magnetite (Fe3O4) nanoparticles on perennial ryegrass (Lolium perenne L.) and pumpkin (Cucurbita mixta) plants. Nanotoxicology 5, 30–42. doi: 10.3109/17435390.2010.489206
Wang, J., and Naser, N. (1994). Improved performance of carbon paste amperometric biosensors through the incorporation of fumed silica. Electroanalysis 6, 571–575. doi: 10.1002/elan.1140060707
Wang, S., Wang, F., and Gao, S. (2015). Foliar application with nano-silicon alleviates Cd toxicity in rice seedlings. Environ. Sci. Pollut. Res. 22, 2837–2845. doi: 10.1007/s11356-014-3525-0
Wang, S., Wang, F., Gao, S., and Wang, X. (2016). Heavy metal accumulation in different rice cultivars as influenced by foliar application of nano-silicon. Water Air Soil Pollut. 227:228. doi: 10.1007/s11270-016-2928-6
Wang, X., Ou-yang, C., Fan, Z. R., Gao, S., Chen, F., and Tang, L. (2010). Effects of exogenous silicon on seed germination and antioxidant enzyme activities of Momordica charantia under salt stress. J. Anim. Plant Sci. 6, 700–708.
Wang, X., Wei, Z., Liu, D., and Zhao, G. (2011). Effects of NaCl and silicon on activities of antioxidative enzymes in roots, shoots and leaves of alfalfa. Afr. J. Biotechnol. 10, 545–549. doi: 10.5897/AJB10.1353
Waraich, E. A., Ahmad, R., and Ashraf, M. Y. (2011). Role of mineral nutrition in alleviation of drought stress in plants. Aust. J. Crop Sci. 5:764.
White, P. J., and Pongrac, P. (2017). “Heavy-metal toxicity in plants,” in Plant Stress Physiology, ed. S. Shabala (Wallingford: CABI), 300–331.
Wong, M. H., Misra, R. P., Giraldo, J. P., Kwak, S. Y., Son, Y., Landry, M. P., et al. (2016). Lipid exchange envelope penetration (LEEP) of nanoparticles for plant engineering: a universal localization mechanism. Nano Lett. 16, 1161–1172. doi: 10.1021/acs.nanolett.5b04467
Wu, H., and Li, Z. (2022). Recent advances in nano-enabled agriculture for improving plant performance. Crop J. 10, 1–12.
Wu, H., Shabala, L., Shabala, S., Shabala, S., and Giraldo, J. P. (2018). Hydroxyl radical scavenging by cerium oxide nanoparticles improves Arabidopsis salinity tolerance by enhancing leaf mesophyll potassium retention. Environ. Sci. Nano 5, 1567–1583. doi: 10.1039/c8en00323h
Yan, S., Wu, F., Zhou, S., Yang, J., Tang, X., and Ye, W. (2021). Zinc oxide nanoparticles alleviate the arsenic toxicity and decrease them accumulation of arsenic in rice (Oryza sativa L.). BMC Plant Biol. 21:150. doi: 10.1186/s12870-021-02929-3
Yang, F., Hong, F., You, W., Liu, C., Gao, F., Wu, C., et al. (2006). Influence of nano-anatase TiO2 on the nitrogen metabolism of growing spinach. Biol. Trace Elem. Res. 110, 179–190.
Yang, K. Y., Doxey, S., McLean, J. E., Britt, D., Watson, A., Al Qassy, D., et al. (2018). Remodeling of root morphology by CuO and ZnO nanoparticles: effects on drought tolerance for plants colonized by a beneficial pseudomonad. Botany 96, 175–186.
Yang, L., and Watts, D. J. (2005). Particle surface characteristics may play an important role in phytotoxicity of alumina nanoparticles. Toxicol Lett. 158, 122–132. doi: 10.1016/j.toxlet.2005.03.003
Ye, Y., Cota-Ruiz, K., Hernandez-Viezcas, J. A., Valdes, C., Medina-Velo, I. A., Turley, R. S., et al. (2020). Manganese nanoparticles control salinity-modulated molecular responses in Capsicum annuum L. through priming: a sustainable approach for agriculture. ACS Sustain. Chem. Eng. 8, 1427–1436. doi: 10.1021/acssuschemeng.9b05615
Ye, Y., Medina-Velo, I. A., Cota-Ruiz, K., MorenoOlivas, F., and Gardea-Torresdey, J. (2019). Can abiotic stresses in plants be alleviated by manganese nanoparticles or compounds? Ecotoxicol. Environ. Saf. 184:109671. doi: 10.1016/j.ecoenv.2019.109671
Yosefzaei, F., Poorakbar, L., and Farhadi, K. (2016). The effect of silver nanoparticles on morphological and physiological indexes of Ocimum basilicum L. Iranian J. Plant Physiol. Biochem. 1, 63–73.
Yousry, C., Zikry, P. M., Salem, H. M., Basalious, E. B., and El-Gazayerly, O. N. (2020). Integrated nanovesicular/self-nanoemulsifying system (INV/SNES) for enhanced dual ocular drug delivery: statistical optimization, in vitro and in vivo evaluation. Drug Deliv. Transl. Res. 10, 801–814. doi: 10.1007/s13346-020-00716-5
Yuan, J., Chen, Y., Li, H., Lu, J., Zhao, H., Liu, M. M., et al. (2018). New insights into the cellular responses to iron nanoparticles in Capsicum annuum. Sci. Rep. 8, 1–9. doi: 10.1038/s41598-017-18055-w
Zargar, S. M., Nazir, M., Kumar Agrawal, G., Kim, D. W., and Rakwal, R. (2010). Silicon in plant tolerance against environmental stressors: towards crop improvement using omics approaches. Curr. Proteomics 7, 135–143. doi: 10.2174/157016410791330507
Ze, Y., Liu, C., Wang, L., Hong, M., and Hong, F. (2011). The regulation of TiO2 nanoparticles on the expression of light-harvesting complex II and photosynthesis of chloroplasts of Arabidopsis thaliana. Biol. Trace Elem. Res. 143, 1131–1141. doi: 10.1007/s12011-010-8901-0
Zhang, H., Du, W., Peralta-Videa, J. R., Gardea-Torresdey, J. L., White, J. C., Keller, A., et al. (2018). Metabolomics reveals how cucumber (Cucumis sativus) reprograms metabolites to cope with silver ions and silver nanoparticle-induced oxidative stress. Environ. Sci. Technol. 52, 8016–8026. doi: 10.1021/acs.est.8b02440
Zhang, S. (2019). Mechanism of Migration and Transformation of Nano Selenium and Mitigates Cadmium Stress in Plants. [master’s thesis]. Jinan: Shandong University.
Zhang, Y., Liu, N., Wang, W., Sun, J., and Zhu, L. (2020). Photosynthesis and related metabolic mechanism of promoted rice (Oryza sativa L.) growth by TiO2 nanoparticles. Front. Environ. Sci. Eng. 14:103. doi: 10.1007/s11783-020-1282-5
Zhao, L., Lu, L., Wang, A., Zhang, H., Huang, M., Wu, H., et al. (2020). Nano-biotechnology in agriculture: use of nanomaterials to promote plant growth and stress tolerance. J. Agric. Food Chem. 68, 1935–1947. doi: 10.1021/acs.jafc.9b06615
Zmeeva, O. N., Daibova, E. B., Proskurina, L. D., Petrova, L. V., Kolomiets, N. E., Svetlichnyi, V. A., et al. (2017). Effects of silicon dioxide nanoparticles on biological and physiological characteristics of Medicago sativa L. nothosubsp. varia (Martyn) in natural agroclimatic conditions of the subtaiga zone in Western Siberia. BioNanoSci 7, 672–679. doi: 10.1007/s12668-017-0395-1
Keywords: abiotic stress, crop yield, modern agriculture, nanoparticles, nanotechnology, plant performance
Citation: El-Saadony MT, Saad AM, Soliman SM, Salem HM, Desoky E-SM, Babalghith AO, El-Tahan AM, Ibrahim OM, Ebrahim AAM, Abd El-Mageed TA, Elrys AS, Elbadawi AA, El-Tarabily KA and AbuQamar SF (2022) Role of Nanoparticles in Enhancing Crop Tolerance to Abiotic Stress: A Comprehensive Review. Front. Plant Sci. 13:946717. doi: 10.3389/fpls.2022.946717
Received: 17 May 2022; Accepted: 22 June 2022;
Published: 02 November 2022.
Edited by:
Mahmoud F. Seleiman, King Saud University, Saudi ArabiaReviewed by:
Shimaa Badawy, Kafrelsheikh University, EgyptCopyright © 2022 El-Saadony, Saad, Soliman, Salem, Desoky, Babalghith, El-Tahan, Ibrahim, Ebrahim, Abd El-Mageed, Elrys, Elbadawi, El-Tarabily and AbuQamar. This is an open-access article distributed under the terms of the Creative Commons Attribution License (CC BY). The use, distribution or reproduction in other forums is permitted, provided the original author(s) and the copyright owner(s) are credited and that the original publication in this journal is cited, in accordance with accepted academic practice. No use, distribution or reproduction is permitted which does not comply with these terms.
*Correspondence: Khaled A. El-Tarabily, a3RhcmFiaWx5QHVhZXUuYWMuYWU=; Synan F. AbuQamar, c2FidXFhbWFyQHVhZXUuYWMuYWU=
Disclaimer: All claims expressed in this article are solely those of the authors and do not necessarily represent those of their affiliated organizations, or those of the publisher, the editors and the reviewers. Any product that may be evaluated in this article or claim that may be made by its manufacturer is not guaranteed or endorsed by the publisher.
Research integrity at Frontiers
Learn more about the work of our research integrity team to safeguard the quality of each article we publish.