- 1College of Life Sciences and Oceanography, Shenzhen University, Shenzhen, China
- 2Guangdong Provincial Key Laboratory for Plant Epigenetics, College of Life Sciences and Oceanography, Shenzhen University, Shenzhen, China
Plants are exposed to various environmental stresses. The sensing of environmental cues and the transduction of stress signals into intracellular signaling are initial events in the cellular signaling network. As a second messenger, Ca2+ links environmental stimuli to different biological processes, such as growth, physiology, and sensing of and response to stress. An increase in intracellular calcium concentrations ([Ca2+]i) is a common event in most stress-induced signal transduction pathways. In recent years, significant progress has been made in research related to the early events of stress signaling in plants, particularly in the identification of primary stress sensors. This review highlights current advances that are beginning to elucidate the mechanisms by which abiotic environmental cues are sensed via Ca2+ signals. Additionally, this review discusses important questions about the integration of the sensing of multiple stress conditions and subsequent signaling responses that need to be addressed in the future.
Introduction
Plants encounter various abiotic stresses in their natural environment, for example, mechanical stimuli, drought, flood, cold, and salinity stress. These adverse conditions often limit plant growth and crop productivity worldwide (Zhang et al., 2018b). To survive, plants, which are sessile organisms, must detect the nature and strength of environmental stimuli, transduce these signals into intracellular signaling and activate appropriate physiological responses (Kissoudis et al., 2014; Zhang et al., 2022). The perception of environmental stress and the subsequent transduction of stress signals are initial events in the adaptation of all organisms to stresses in their environment (Lamers et al., 2020). Therefore, how plants sense abiotic stress signals, transduce them into cellular signaling and subsequently adapt to adverse environment is a fundamental and significant biological question.
Typically, a primary abiotic stress sensor is required to perceive external environmental changes; in response to such changes, the sensor remodels signal transduction pathways and initiates appropriate responses that allow the plant to adapt to the stress condition. Thus, perception of the external environment by a sensor is the earliest step in the conversion of external stimuli into cellular signals by plants.
Calcium (Ca2+), an indispensable second messenger, is considered to be a critical element by which plants modulate a complicated network of signaling pathways to respond to various abiotic stresses (Hetherington and Brownlee, 2004; Pandey et al., 2004; Dodd et al., 2010; Yuan et al., 2014). The increase in intracellular calcium concentrations ([Ca2+]i) is one of the earliest signaling events when plants are challenged with abiotic stimuli, and the resulting Ca2+ signaling regulates many processes in plants, including transcriptional regulation and subsequent physiological as well as developmental responses (Dodd et al., 2010; Kudla et al., 2010; Cao et al., 2017; Aldon et al., 2018). Bioluminescence-based aequorin technology for the detection of [Ca2+]i oscillations has been reported, and this technology uses the aequorin system to detect abiotic stimuli-induced Ca2+ signals (Knight et al., 1991, 1997). The results obtained with this approach have suggested that Ca2+ is an important second messenger for understanding plant-abiotic stimulus interactions (Van Zelm et al., 2020).
In the resting state, [Ca2+]i is maintained within a range of 50–200 nM (Berridge et al., 2000; Mehlmer et al., 2012; Lee and Seo, 2021). However, this concentration increases by approximately 10-fold and reaches millimolar levels upon stimulation (Lee and Seo, 2021). Transient increases in Ca2+ levels are caused by Ca2+ influx from the extracellular space or Ca2+ release from organelles through Ca2+ channels and transporters in membranes (Rentel and Knight, 2004; Kudla et al., 2010; Batistic and Kudla, 2012; Stael et al., 2012). Various stresses activate different Ca2+ channels and trigger [Ca2+]i increase in cells, and these increases exhibit tissue-specific and stress-specific differences in peak amplitude, peak oscillation pattern, and wave propagation. This stress-specific phenomenon is known as a “Ca2+ signature,” which leads to the activation of downstream events, such as stress-responsive gene expression and protein interactions (Jeworutzki et al., 2010; Stael et al., 2012; Steinhorst and Kudla, 2013; Seybold et al., 2014). Despite several potential stress sensors functioning as Ca2+-permeable cation channels (e.g., several ion channels that directly sense membrane tension), the link between abiotic stress sensors and the Ca2+ influx channels that are regulated by these sensors is still largely unknown. Here, we mainly discuss recent findings related to Ca2+ signaling to reveal the mechanisms by which plants sense stressful environment; these findings have advanced our understanding of plant sensory mechanisms.
Mechanical Stimuli
Responses to environmental stress are essential features of plant behavior (Braam, 2005). A fundamental characteristic of plants is their ability to sense and respond to mechanical stimuli, such as touch, gravity, and flexure (Trewavas and Knight, 1994; Braam, 2005; Nakagawa et al., 2007). When Arabidopsis plants that express a Ca2+-sensitive aequorin are exposed to touch and gravistimulation, the recombinant plants emit light immediately (Plieth and Trewavas, 2002; Toyota et al., 2008), suggesting that mechanical stimuli influence membrane-localized Ca2+-permeable channels and immediately trigger a transient increase in [Ca2+]i; this is an early event in the response to mechanical stimuli, and it subsequently influences plant growth and development. In addition, osmotic stress (including both hyperosmotic stress caused by drought and hypoosmotic shock) also influences membrane tension and triggers a [Ca2+]i increase by activating certain Ca2+-permeable channels, allowing plant cells to perceive changes in the physical properties of the membrane (Gong et al., 2020).
To date, five families of mechanosensitive channels have been reported in plants: mechanosensitive channel of small conductance (MscS)-like proteins (MSLs), Mid1-complement activity protein channels (MCAs), two-pore potassium channels (TPKs), Piezo channels (PZO), and reduced hyperosmolality induced [Ca2+]i increase channels (OSCA; Haswell and Meyerowitz, 2006; Nakagawa et al., 2007; Haswell et al., 2008; Coste et al., 2010; Yamanaka et al., 2010; Maathuis, 2011; Yuan et al., 2014).
MCA proteins were identified as PM-localized Ca2+-permeable mechanosensitive ion channels that regulate mechanoresponsive Ca2+ influx (Nakagawa et al., 2007; Figure 1). AtMCA1 and AtMCA2 are two paralogous MCA genes in Arabidopsis (Yamanaka et al., 2010; Nishii et al., 2021). Mca1-knockout seedlings have reduced amplitudes and very slow [Ca2+]i increases (Nakano et al., 2021). Ectopic overexpression of MCA proteins increases Ca2+ uptake in Arabidopsis seedlings as well as in cultured rice cells and enhances the hypo-osmotic shock-induced increase in [Ca2+]i (Nakagawa et al., 2007; Kurusu et al., 2012a,b). In addition, MCA1 was first reported to induce a gravistimulation-induced [Ca2+]i increase under the condition of 1–5 g gravitational acceleration (Nakano et al., 2021). Recently, the mechanisms underlying MCA activation have been elucidated. Yoshimura et al. purified and reconstituted MCA1 and MCA2 into artificial liposomal membranes and examined their Ca2+ permeability properties. They found that a C-terminally truncated form of the MCA2 protein that acts as a Ca2+-permeable and mechanosensitive channel directly senses membrane tension to regulate channel opening (Yoshimura et al., 2021).
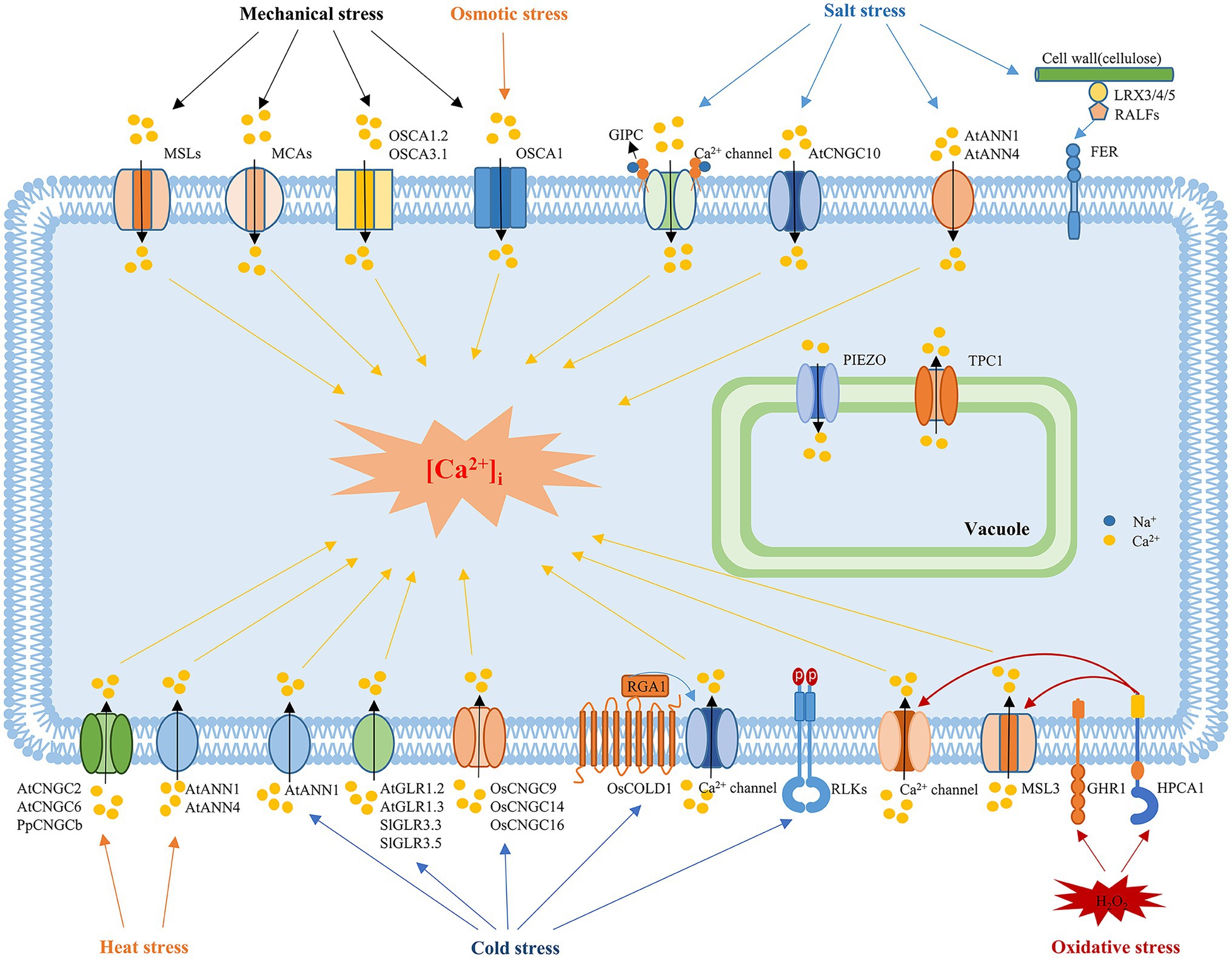
Figure 1. Mechanisms underlying the sensing of abiotic stress in plants. In response to mechanical stimuli, mechanosensitive channels, such as MSLs, MCA, Piezos, and OSCAs, are activated to induce Ca2+ influx. MCAs and OSCA are plasma membrane mechanosensitive cation channels, while Piezo is located in the vacuole membrane. OSCA1 is a plasma membrane protein that functions as a hyperosmolality-gated calcium-permeable channel that induces the initial increase in [Ca2+]i during osmotic stress. Salt stress is sensed by the binding of monovalent cations to the negatively charged GlcA of the GIPC sphingolipids. Upon Na+ binding, the GIPC-gated unknown Ca2+ channel is activated to trigger the Ca2+ influx. ANN1, ANN4, and CNGC10 are also involved in the salt-induced Ca2+ influx. In addition, the cell wall-localized leucine-rich repeat extensins LRX3, LRX4, and LRX5, RALFs and the receptor-like kinase FER also participate in sensing salt stress signals. The vacuolar ion channel TPC1 is involved in systemic salt and Ca2+ signaling. Environmental stresses trigger tissue-specific and stress-specific [Ca2+]i increases, which are known as “Ca2+ signatures.” The crucial protein in sensing cold stress in rice is COLD1. COLD1 interacts with RGA1 in response to cold temperatures, resulting in increased GTPase activity, which activates an unknown calcium influx channel. Plasma membrane-localized RLCK plays a negative role in regulating freezing tolerance. In addition, plant CNGCs, such as CNGC9, CNGC14, and CNGC16 in rice, are involved in cold sensing, while CNGC2 and CNGC6 in Arabidopsis and CNGCb in Physcomitrella mediate the heat-induced [Ca2+]i increase. Glutamate receptors, including GLR1.2 and GLR1.3 in Arabidopsis and GLR3.3 and GLR3.5 in tomato plants, also play roles in cold sensing and tolerance. ANN1 and ANN4 are involved in the heat-induced [Ca2+]i increase and tolerance to heat, while ANN1 is also involved in the cold-induced Ca2+ influx. H2O2 perception causes oxidation of HPCA1, and oxidative modification of HPCA1 might act alone or with a coreceptor to phosphorylate and activate an unknown Ca2+ channel to trigger Ca2+ influx. GHR1, a plasma membrane receptor-like kinase, may also sense H2O2 signals and regulate ABA signaling and stomatal closure in guard cells. MSL3 and TPC1 are involved in systemic ROS and Ca2+ signaling.
Using calcium imaging, Arabidopsis thaliana PIEZO1 has been shown to exhibit the conserved function of the mammalian PIEZO mechanosensitive ion channel, and this channel responds to a mechanical stimulus with transiently increasing Ca2+ levels (Mousavi et al., 2021). Evidence has shown that plant PIEZO1 is expressed in the columella and lateral root cap cells of the root tip, which experience robust mechanical strain during root growth. Deleting PIEZO1 from the whole plant significantly reduced the ability of roots to penetrate denser barriers compared to that of the wild-type plants. The root tips of piezo1 mutant plants exhibited decreased transient changes in Ca2+ levels in response to mechanical stimulation, supporting a role of plant PIEZO1 in root mechanotransduction. Recently, a very interesting finding was reported that plant PIEZO was found to be localized to the vacuole membrane, where it plays an important role in regulating the formation of vacuole morphology and inducing [Ca2+]i oscillation (Radin et al., 2021; Figure 1).
Based on forward genetic screening to isolate EMS-mutagenized aequorin-expressing Arabidopsis seedlings, OSCA1 was the first potential osmosensor to be identified (Yuan et al., 2014). Mutation in OSCA1 results in a decrease in Ca2+ accumulation in guard cells and root cells, as well as a lack of transpiration regulation and reduced root growth in response to osmotic stress. OSCA1 was identified as a hyperosmolality-gated Ca2+-permeable channel, and it is responsible for the initial increase in free Ca2+ concentrations upon the sensing of osmotic stress (Yuan et al., 2014; Figure 1). The activation of these channels has been hypothesized to be caused by mechanical forces on the cell membrane or cell wall generated by osmotic stress (Gong et al., 2020). Phylogenetic analysis demonstrated that Arabidopsis has 15 homologs of OSCA1, suggesting that the sensing of hyperosmotic conditions could be mediated by a redundant family of Ca2+ channels (Liu et al., 2018). Using cryo-electron microscopy (cryo-EM), three groups have separately characterized the structures of OSCA1.1, OSCA1.2, and OSCA3.1, and these groups found that the OSCA proteins belong to a new class of mechanosensitive ion channels with structural similarities to mammalian TMEM16-family proteins (Jojoa-Cruz et al., 2018; Liu et al., 2018; Zhang et al., 2018a; Figure 1).
Salt Stress
Soil salinization is a severely adverse abiotic factor that affects seed germination, crop growth, and productivity (Zhao et al., 2020). In contrast to animals, sodium is not an essential element to most plants, and at high concentrations, it is very detrimental to plant growth. High concentrations of Na+ and Cl− in soil induce both osmotic stress and ionic stress. When exposed to high levels of Na+, large, low-affinity Na+ fluxes occur that are mediated by HKT-type carriers (Garciadeblas et al., 2003; James et al., 2011) and nonselective ion channels (NSCCs), including glutamate receptor-like (GLR) and cyclic nucleotide-gated channels (CNGCs; Amtmann and Sanders, 1999).
By performing a forward genetic screen, an Arabidopsis monocation-induced [Ca2+]i increases 1 (moca1) mutant was isolated successfully, and this mutant exhibits decreased Na+-induced increases in [Ca2+]i and is hypersensitive to growth inhibition by Na+ (Jiang et al., 2019). The lack of MOCA1 activity also impairs the membrane surface potential and, notably, the activity of the Na+/H+ antiporter SOS1, making the mutant plants more sensitive to salt than the wild-type plants. Thus, MOCA1 represents one of the missing links between salt perception and SOS pathway activation. MOCA1 was identified as a glucuronosyltransferase that transfers a negatively charged GlcA to inositol phosphorylceramide (IPC) to form glycosylinositol phosphorylceramide (GIPC) sphingolipids in the external layer of the plasma membrane, and it is essential for NaCl-triggered depolarization of the cell-surface potential (Jiang et al., 2019). The study found that moca1 mutant plants contained lower levels of GIPCs but higher levels of IPCs than wild-type plants (Jiang et al., 2019). Since IPCs do not contain a negatively charged head, there are fewer monovalent cation binding sites on the membranes of moca1 mutant plants than on the membranes of wild-type plants, which is consistent with the previously described strong Na+-binding properties of GIPCs (Markham et al., 2006). Regarding the Na+ perception mechanism, Na+ directly binds to GIPCs and presumably regulates Ca2+ influx channels, providing the molecular basis for Na+ sensing in plants (Figure 1). However, the exact mechanism of GIPC-mediated Ca2+ influx remains unknown as long as the Ca2+ channel involved remains unidentified (Steinhorst and Kudla, 2019). In addition, downstream of GIPCs, the Ca2+ wave speed is altered in the tpc1 (two-pore channel 1) mutant, indicating that TPC1 mediates Ca2+ release from the vacuole and facilitates the propagation of Ca2+ waves (Choi et al., 2014). A putative glycosyltransferase QUA1, required for normal pectin synthesis and cell adhesion, was reported to regulate [Ca2+]i in response to salt stress in Arabidopsis (Zheng et al., 2017).
Luminescence-based detection of [Ca2+]i showed that the Ca2+-permeable transporter AtANNEXIN1 (AtANN1) is required for NaCl-activated Ca2+ influx through the plasma membrane in root epidermal cells. The loss-of-function mutation of AtANN4 also disrupts the salt-induced [Ca2+]i increases in Arabidopsis (Laohavisit et al., 2013; Ma et al., 2019). In addition to AtANNs, CNGC10 was reported to negatively regulate salt tolerance in Arabidopsis (Jin et al., 2015). It would be interesting to investigate whether there is a direct functional association between GIPC and AtANN1, AtANN4, or CGNC10. In addition, the cell wall is involved in salt sensing. A receptor-like kinase FERONIA (FER) possibly perceives salt-induced changes in the cell wall. Mutation of FER results in decreased salt-induced Ca2+ signaling and increased sensitivity to high salinity (Feng et al., 2018; Figure 1). Recent studies have shown that the cell wall-localized leucine-rich repeat extensins LRX3, LRX4, and LRX5 and the secretory peptide RALFs participate in sensing salt stress signals along with FER (Figure 1). However, the mechanism of the initial sensing of salt-induced changes in the cell wall by LRXs remains unknown (Zhao et al., 2018).
Temperature Stress
Temperature is one of the major environmental factors that affect plant growth and development. Extreme temperatures induce a series of biochemical, physiological, and morphological changes in plants and often reduce plant productivity (Cui et al., 2020a). Cold stress decreases the fluidity of cellular membranes and causes membrane rigidification in plant cells, which also disrupts the stability of proteins or protein complexes and decreases the activities of enzymes (Orvar et al., 2000; Zhu, 2016; Gong et al., 2020). To survive under extreme temperatures, plants must be able to perceive temperature signals and transduce them to the downstream signaling pathways, subsequently activating appropriate defense mechanisms (Guo et al., 2018).
Cold sensing is followed by the generation of secondary messengers, including Ca2+ (Yan et al., 2006). After exposure of both plants and animals to cold, [Ca2+]i is increased very rapidly via Ca2+ channels, which is thought to be one of the earliest events in cold stress signaling (Knight et al., 1991; Ding et al., 2019). Cold stress induces transient Ca2+ influx into the cell cytoplasm, and repeated cold treatment can induce repetitive transient Ca2+ influxes (Krebs et al., 2012). In mammals, the transient receptor potential (TRP) superfamily of cation channels is involved in thermosensation (Waszczak et al., 2018). However, no TRP orthologs have been identified in plants. How Ca2+ signals are involved in cold perception in plants is unclear. Ma et al. identified a transmembrane protein named COLD1 (chilling-tolerance-divergence 1), which was the first potential cold sensor to be identified in rice. Overexpression of COLD1 significantly improves survival rates after chilling treatment, whereas mutants with deficiency or decreased expression of COLD1 are sensitive to chilling stress (Ma et al., 2015). These results suggest that COLD1 is an important component of the regulation of chilling tolerance in rice. Further study showed that COLD1 regulates G protein signaling by interacting with rice G protein subunit 1 (RGA1) to increase GTPase activity under conditions of cold stress. Furthermore, COLD1-RGA1 form a complex to trigger a cold-induced increase in [Ca2+]i, leading to the activation of the cold tolerance response (Figure 1). However, the molecular mechanism by which COLD1 senses cold stress and COLD1 activates Ca2+ channels remains unclear. It would be interesting to determine whether COLD1 itself functions as a temperature-regulated membrane ion channel and how COLD1 acts as a cold sensor to trigger cold-induced Ca2+ influx in plants.
In addition, cold can decrease the fluidity of cellular membranes (Zhu, 2016). This change in membrane fluidity may be sensed by membrane-localized proteins, such as Ca2+ channels and receptor-like kinases (RLKs; Zhu, 2016, Gong et al., 2020). Several RLKs have been reported to play critical roles in regulating cold responses. CRLK1 and CRLK2 are two calcium/calmodulin-regulated receptor-like cytoplasmic kinases (RLCKs) that positively regulate cold tolerance (Yang et al., 2010). Recently, a plasma membrane-localized RLCK, cold-responsive protein kinase 1 (CRPK1), was found to play a negative role in regulating cold tolerance (Liu and Howell, 2016; Figure 1). Plant CNGCs are involved in thermal sensing and thermotolerance (Finka et al., 2012). In rice, loss-of-function mutant of OsCNGC9 is defective in cold-induced Ca2+ influx, which make plants more sensitive to cold treatment; these results suggest that OsCNGC9 could positively regulate chilling tolerance (Wang et al., 2021). Additionally, the loss of either OsCNGC14 or OsCNGC16 reduced or abolished the [Ca2+]i escalation by either heat or chilling stress, indicating that OsCNGC14 and OsCNGC16 modulate Ca2+ signaling in response to temperature stress (Cui et al., 2020b; Figure 1). A previous study reported that CNGCb in Physcomitrella and its Arabidopsis ortholog AtCNGC2 mediate heat-induced [Ca2+]i increases, enhancing plant survival at high temperatures (Finka et al., 2012; Figure 1). In addition, AtCNGC6 in Arabidopsis is also involved in heat-induced induced [Ca2+]i increases (Gao et al., 2012; Figure 1). In addition to CNGCs, AtANNs are involved in temperature dependent Ca2+ influx. A recent study reported that loss-of-function mutations in AtANN1 significantly impair cold-induced Ca2+ influx and reduce tolerance to cold stress in Arabidopsis (Liu et al., 2021; Figure 1). Moreover, AtANN1 and AtANN4 are also involved in heat-induced [Ca2+]i increases and consequently heat tolerance in Arabidopsis (Liao et al., 2017; Figure 1). Additionally, GLR could act as sensors and mediators for temperature in plants. Plants with loss-of-function mutations in AtGLR1.2 and AtGLR1.3 are sensitive to cold stress, while AtGLR1.2 and AtGLR1.3 increase cold tolerance by regulating jasmonate signaling in Arabidopsis (Zheng et al., 2018; Figure 1). GLR3.3 and GLR3.5 are involved in cold tolerance in tomato plants (Li et al., 2019; Figure 1).
Oxidative Stress
Reactive oxygen species (ROS) play a key role in plant cell signaling. Plant cells generate various ROS, including hydrogen peroxide (H2O2), which is produced extracellularly in response to external stresses and internal cues. Extracellular hydrogen peroxide (eH2O2) plays a vital role in many physiological processes during the lifecycle of plants, such as root development, pollen tube growth, organ wilting, and responses to biotic and abiotic stress (Waszczak et al., 2018).
It has been reported that the H2O2 signal can be sensed by Guard Cell Hydrogen Peroxide-Resistant1 (GHR1), which regulates ABA signaling and stomatal closure in guard cells (Hua et al., 2012; Figure 1). Additionally, GHR1 is also involved in stomatal responses to high CO2 concentrations, activating guard cell anion channel slow anion channel1 (SLAC1) and stimulating stomatal closure (Horak et al., 2016; Sierla et al., 2018). In addition, the influx of eH2O2 into the cytosol via aquaporin channel proteins has also been reported, but whether and how plants perceive eH2O2 at the cell surface is unknown. Although little is known about the initial sensing of eH2O2, the fact that eH2O2 triggers an influx of Ca2+ is much better defined and is thought to be involved in H2O2 sensing and signaling. Interestingly, Ca2+ was also found to bind respiratory burst oxidase homolog protein D (RBOHD) EF-hands and promotes H2O2 which then subsequently activates Ca2+ channels in response to a range of abiotic stresses (Ogasawara et al., 2008; Miller et al., 2009; Suzuki et al., 2011).
By using a forward genetic screen based on Ca2+ imaging, Wu et al. isolated hydrogen peroxide-induced Ca2+ increase (hpca) mutants in Arabidopsis and showed that HPCA1 encodes a leucine-rich repeat receptor kinase that localizes to the cell surface. It was the first molecular component found to be responsible for sensing eH2O2 (Pei et al., 2000; Wu et al., 2020). The hpca1 mutant did not display growth or developmental phenotypes, but eH2O2- and ABA-insensitive phenotypes were observed in terms of stomatal closure. In addition, the hpca1 mutant exhibits decreased eH2O2-induced Ca2+ influx, indicating that HPCA1-mediated activation of Ca2+ channels is critical for eH2O2 signaling. Further study demonstrated that HPCA1 can be activated by eH2O2 via the covalent modification of its extracellular cysteine residues, which leads to autophosphorylation of HPCA1 and its function as an eH2O2 sensor to induce Ca2+ influx (Figure 1).
A recent research finding suggested that MSL3 could function downstream of HPCA1, indicating that HPCA1 is required for systemic ROS and Ca2+ cell-to-cell signaling and plant acclimation to stress (Fichman et al., 2022). In addition, ROS also stimulate the vacuolar ion channel TPC1 to release Ca2+ from the vacuole, allowing substantial propagation of systemic Ca2+ waves (Evans et al., 2016).
Conclusion and Perspectives
As sessile organisms, plants cannot escape from environmental stress. It is very important for plants to accurately and specifically recognize environmental signals and initiate the correct responses. In the last decade, some major advances have been made in the elucidation of mechanisms underlying abiotic stimulus perception, such as the role of OSCA1 in response to osmotic stress, the role of COLD1 in sensing cold stress, the role of GIPCs as monovalent cation sensors, and the role of HPCA1 as an eH2O2 sensor. At present, many gaps remain in our understanding of plant stress sensing. Identifying stress sensors is an important goal for studying abiotic stress responses in plants, but overcoming genetic redundancy and lethality remains a challenge.
The increase in [Ca2+]i is one of earliest signaling events when plants are challenged with abiotic stimuli. Ca2+ influx occurs within a few seconds after the perception of environmental stress. Different sensors sense specific stressors to directly or indirectly activate Ca2+ channels and mediate the influx of Ca2+. However, the identity of the Ca2+ channel involved in signal sensing and transduction and how environmental signals activate Ca2+ channels remain largely unknown. Therefore, the identification of sensing mechanisms is an indispensable step in the elucidation of cellular signaling pathways that participate in the response to environmental stress and how they lead to appropriate responses. Furthermore, more attention should be given to the functional coordination of Ca2+ channels in different cellular organelles because [Ca2+]i could be complicated by the interplay of PM-localized influx channels and organelle-specific efflux channels.
Molecular genetics methods and various bioimaging techniques have contributed greatly to our current knowledge. With recent advances in bioimaging techniques and genetic methods, utilizing Ca2+ imaging-based forward genetic screens may be an effective way to reveal the mechanisms underlying stress sensing and potential Ca2+ channels in the future. These advances in understanding mechanisms underlying abiotic stimulus perception demonstrate that genetic screens based on “imaging technologies” represent the new standard for discovering new genes involved in important signaling pathways. In addition to bioluminescence approaches, fluorescence-based Ca2+ indicators have potential for single-cell analyzes (Krebs et al., 2012), but they are also mainly used at the tissue level. Fluorescence-based Ca2+ indicators can be broadly categorized into single fluorescent proteins (such as green fluorescent GCaMPs/G-GECOs and red fluorescent R-GECOs; Diao et al., 2018; Vigani and Costa, 2019; Luo et al., 2020) and dual-fluorescent protein indicators (such as FRET-based yellow cameleons; Allen et al., 1999; Walia et al., 2018; Vigani and Costa, 2019). Note that ratiometric Ca2+ indicators can compensate for variations in fluorescence readouts due to differences in expression levels between different cell and tissue types. It is clear that the increasing development of plant Ca2+ indicators should provide a fruitful foundation for novel discoveries.
Although several stress sensors have been reported recently, their physiological functions and biochemical sensing mechanisms remain unclear. Furthermore, because plants are exposed to multiple abiotic stresses simultaneously in the natural environment, determining stress-specific sensing mechanisms at the molecular level is important. Thus, to improve our understanding of abiotic stress resistance in plants, more attention should be given to the perception of multiple abiotic stresses by plants in the future.
In addition to sensing mechanisms, a great deal of responses and crosstalk are observed in downstream signaling pathways, which could lead to additive, synergistic or antagonistic effects, resulting in either enhanced or compromised stress resistance. However, since most current studies have focused on a single stress condition, the mechanisms by which plants respond to combined stresses remains largely unpredictable. Thus, revealing the mechanisms underlying plant perception and responses to multiple stresses at the molecular level will be necessary to gain knowledge about the integration of stress-induced signaling pathways, which is a promising direction for future research. Knowledge of how plants perceive and respond to stress will allow us to answer fundamental questions of how plants convert abiotic stress into electrochemical signals and will allow us to understand important biological processes by which how plants grow and develop to tolerate stressful environment.
Author Contributions
TX, JN, and ZJ conceived the idea and wrote the manuscript. All authors contributed to the article and approved the submitted version.
Funding
This work was supported by grants from Natural Science Foundation of Guangdong Province (2022B1515020040) and Shenzhen Peacock Plan (KQTD2017032715165926).
Conflict of Interest
The authors declare that the research was conducted in the absence of any commercial or financial relationships that could be construed as a potential conflict of interest.
Publisher’s Note
All claims expressed in this article are solely those of the authors and do not necessarily represent those of their affiliated organizations, or those of the publisher, the editors and the reviewers. Any product that may be evaluated in this article, or claim that may be made by its manufacturer, is not guaranteed or endorsed by the publisher.
References
Aldon, D., Mbengue, M., Mazars, C., and Galaud, J. P. (2018). Calcium signalling in plant biotic interactions. Int. J. Mol. Sci. 19, 665–683. doi: 10.3390/ijms19030665
Allen, G. J., Kwak, J. M., Chu, S. P., Llopis, J., Tsien, R. Y., Harper, J. F., et al. (1999). Cameleon calcium indicator reports cytoplasmic calcium dynamics in Arabidopsis guard cells. Plant J. 19, 735–747. doi: 10.1046/j.1365-313x.1999.00574.x
Amtmann, A., and Sanders, D. (1999). Mechanisms of Na(+) uptake by plant cells. Adv. Bot. Res. Inc. Adv. Plant Pathol. 29, 75–112.
Batistic, O., and Kudla, J. (2012). Analysis of calcium signaling pathways in plants. Biochim Biophys. Acta Gen. Subj. 1820, 1283–1293. doi: 10.1016/j.bbagen.2011.10.012
Berridge, M. J., Lipp, P., and Bootman, M. D. (2000). The versatility and universality of calcium signalling. Nat. Rev. Mol. Cell Biol. 1, 11–21. doi: 10.1038/35036035
Braam, J. (2005). In touch: plant responses to mechanical stimuli. New Phytol. 165, 373–389. doi: 10.1111/j.1469-8137.2004.01263.x
Cao, X. Q., Jiang, Z. H., Yi, Y. Y., Yang, Y., Ke, L. P., Pei, Z. M., et al. (2017). Biotic and abiotic stresses activate different Ca(2+) permeable channels in Arabidopsis. Front. Plant Sci. 8:83. doi: 10.3389/fpls.2017.00083
Choi, W. G., Toyota, M., Kim, S. H., Hilleary, R., and Gilroy, S. (2014). Salt stress-induced Ca2+ waves are associated with rapid, long-distance root-to-shoot signaling in plants. Proc. Natl. Acad. Sci. U. S. A. 111, 6497–6502. doi: 10.1073/pnas.1319955111
Coste, B., Mathur, J., Schmidt, M., Earley, T. J., Ranade, S., Petrus, M. J., et al. (2010). Piezo1 and Piezo2 are essential components of distinct mechanically activated cation channels. Science 330, 55–60. doi: 10.1126/science.1193270
Cui, Y., Lu, S., Li, Z., Cheng, J., Hu, P., Zhu, T., et al. (2020a). CYCLIC NUCLEOTIDE-GATED ION CHANNELs 14 and 16 promote tolerance to heat and chilling in rice. Plant Physiol. 183, 1794–1808. doi: 10.1104/pp.20.00591
Cui, Y. M., Lu, S., Li, Z., Cheng, J. W., Hu, P., Zhu, T. Q., et al. (2020b). CYCLIC NUCLEOTIDE-GATED ION CHANNELs 14 and 16 promote tolerance to heat and chilling in Rice(1)([OPEN]). Plant Physiol. 183, 1794–1808. doi: 10.1104/pp.20.00591
Diao, M., Qu, X. L., and Huang, S. J. (2018). Calcium imaging in Arabidopsis pollen cells using G-CaMP5. J. Integr. Plant Biol. 60, 897–906. doi: 10.1111/jipb.12642
Ding, Y., Shi, Y., and Yang, S. (2019). Advances and challenges in uncovering cold tolerance regulatory mechanisms in plants. New Phytol. 222, 1690–1704. doi: 10.1111/nph.15696
Dodd, A. N., Kudla, J., and Sanders, D. (2010). The language of calcium signaling. Annu. Rev. Plant Biol. 61, 593–620. doi: 10.1146/annurev-arplant-070109-104628
Evans, M. J., Choi, W. G., Gilroy, S., and Morris, R. J. (2016). A ROS-assisted calcium wave dependent on the AtRBOHD NADPH oxidase and TPC1 cation channel propagates the systemic response to salt stress. Plant Physiol. 171, 1771–1784. doi: 10.1104/pp.16.00215
Feng, W., Kita, D., Peaucelle, A., Cartwright, H. N., Doan, V., Duan, Q. H., et al. (2018). The FERONIA receptor kinase maintains cell-wall integrity during salt stress through Ca2+ signaling. Curr. Biol. 28, 666–675. doi: 10.1016/j.cub.2018.01.023
Fichman, Y., Zandalinas, S. I., Peck, S., Luan, S., and Mittler, R. (2022). HPCA1 is required for systemic ROS and calcium cell-to-cell signaling and plant acclimation to stress. bioRxiv [Preprint]. doi: 10.1101/2022.03.24.485694
Finka, A., Cuendet, A. F. H., Maathuis, F. J. M., Saidi, Y., and Goloubinoff, P. (2012). Plasma membrane cyclic nucleotide gated calcium channels control land plant thermal sensing and acquired thermotolerance. Plant Cell 24, 3333–3348. doi: 10.1105/tpc.112.095844
Gao, F., Han, X. W., Wu, J. H., Zheng, S. Z., Shang, Z. L., Sun, D. Y., et al. (2012). A heat-activated calcium-permeable channel – Arabidopsis cyclic nucleotide-gated ion channel 6 – is involved in heat shock responses. Plant J. 70, 1056–1069. doi: 10.1111/j.1365-313X.2012.04969.x
Garciadeblas, B., Senn, M. E., Banuelos, M. A., and Rodriguez-Navarro, A. (2003). Sodium transport and HKT transporters: the rice model. Plant J. 34, 788–801. doi: 10.1046/j.1365-313X.2003.01764.x
Gong, Z., Xiong, L., Shi, H., Yang, S., Herrera-Estrella, L. R., Xu, G., et al. (2020). Plant abiotic stress response and nutrient use efficiency. Sci. China Life Sci. 63, 635–674. doi: 10.1007/s11427-020-1683-x
Guo, X., Liu, D., and Chong, K. (2018). Cold signaling in plants: insights into mechanisms and regulation. J. Integr. Plant Biol. 60, 745–756. doi: 10.1111/jipb.12706
Haswell, E. S., and Meyerowitz, E. M. (2006). MscS-like proteins control plastid size and shape in Arabidopsis thaliana. Curr. Biol. 16, 1–11. doi: 10.1016/j.cub.2005.11.044
Haswell, E. S., Peyronnet, R., Barbier-Brygoo, H., Meyerowitz, E. M., and Frachisse, J. M. (2008). Two MscS homologs provide mechanosensitive channel activities in the Arabidopsis root. Curr. Biol. 18, 730–734. doi: 10.1016/j.cub.2008.04.039
Hetherington, A. M., and Brownlee, C. (2004). The generation of Ca2+ signals in plants. Annu. Rev. Plant Biol. 55, 401–427. doi: 10.1146/annurev.arplant.55.031903.141624
Horak, H., Sierla, M., Toldsepp, K., Wang, C., Wang, Y. S., Nuhkat, M., et al. (2016). A dominant mutation in the HT1 kinase uncovers roles of MAP kinases and GHR1 in CO2-induced STOMATAL closure. Plant Cell 28, 2493–2509. doi: 10.1105/tpc.16.00131
Hua, D., Wang, C., He, J., Liao, H., Duan, Y., Zhu, Z., et al. (2012). A plasma membrane receptor kinase, GHR1, mediates abscisic acid- and hydrogen peroxide-regulated stomatal movement in Arabidopsis. Plant Cell 24, 2546–2561. doi: 10.1105/tpc.112.100107
James, R. A., Blake, C., Byrt, C. S., and Munns, R. (2011). Major genes for Na+ exclusion, Nax1 and Nax2 (wheat HKT1;4 and HKT1;5), decrease Na+ accumulation in bread wheat leaves under saline and waterlogged conditions. J. Exp. Bot. 62, 2939–2947. doi: 10.1093/jxb/err003
Jeworutzki, E., Roelfsema, M. R. G., Anschutz, U., Krol, E., Elzenga, J. T. M., Felix, G., et al. (2010). Early signaling through the Arabidopsis pattern recognition receptors FLS2 and EFR involves Ca2+−associated opening of plasma membrane anion channels. Plant J. 62, 367–378. doi: 10.1111/j.1365-313X.2010.04155.x
Jiang, Z., Zhou, X., Tao, M., Yuan, F., Liu, L., Wu, F., et al. (2019). Plant cell-surface GIPC sphingolipids sense salt to trigger Ca(2+) influx. Nature 572, 341–346. doi: 10.1038/s41586-019-1449-z
Jin, Y. K., Jing, W., Zhang, Q., and Zhang, W. H. (2015). Cyclic nucleotide gated channel 10 negatively regulates salt tolerance by mediating Na+ transport in Arabidopsis. J. Plant Res. 128, 211–220. doi: 10.1007/s10265-014-0679-2
Jojoa-Cruz, S., Saotome, K., Murthy, S. E., Tsui, C. C. A., Sansom, M. S. P., Patapoutian, A., et al. (2018). Cryo-EM structure of the mechanically activated ion channel OSCA1.2. eLife 7:e41845. doi: 10.7554/eLife.41845
Kissoudis, C., Van De Wiel, C., Visser, R. G. F., and Van Der Linden, G. (2014). Enhancing crop resilience to combined abiotic and biotic stress through the dissection of physiological and molecular crosstalk. Front. Plant Sci. 5:207. doi: 10.3389/fpls.2014.00207
Knight, M. R., Campbell, A. K., Smith, S. M., and Trewavas, A. J. (1991). Transgenic plant Aequorin reports the effects of touch and cold-shock and elicitors on cytoplasmic calcium. Nature 352, 524–526. doi: 10.1038/352524a0
Knight, H., Trewavas, A. J., and Knight, M. R. (1997). Calcium signalling in Arabidopsis thaliana responding to drought and salinity. Plant J. 12, 1067–1078. doi: 10.1046/j.1365-313X.1997.12051067.x
Krebs, M., Held, K., Binder, A., Hashimoto, K., Den Herder, G., Parniske, M., et al. (2012). FRET-based genetically encoded sensors allow high-resolution live cell imaging of Ca2+dynamics. Plant J. 69, 181–192. doi: 10.1111/j.1365-313X.2011.04780.x
Kudla, J., Batistic, O., and Hashimoto, K. (2010). Calcium signals: the lead currency of plant information processing. Plant Cell 22, 541–563. doi: 10.1105/tpc.109.072686
Kurusu, T., Nishikawa, D., Yamazaki, Y., Gotoh, M., Nakano, M., Hamada, H., et al. (2012a). Plasma membrane protein OsMCA1 is involved in regulation of hypo-osmotic shock-induced Ca2+ influx and modulates generation of reactive oxygen species in cultured rice cells. BMC Plant Biol. 12:11. doi: 10.1186/1471-2229-12-11
Kurusu, T., Yamanaka, T., Nakano, M., Takiguchi, A., Ogasawara, Y., Hayashi, T., et al. (2012b). Involvement of the putative Ca2+−permeable mechanosensitive channels, NtMCA1 and NtMCA2, in Ca2+ uptake, Ca2+−dependent cell proliferation and mechanical stress-induced gene expression in tobacco (Nicotiana tabacum) BY-2 cells. J. Plant Res. 125, 555–568. doi: 10.1007/s10265-011-0462-6
Lamers, J., Van Der Meer, T., and Testerink, C. (2020). How plants sense and respond to stressful environments. Plant Physiol. 182, 1624–1635. doi: 10.1104/pp.19.01464
Laohavisit, A., Richards, S. L., Shabala, L., Chen, C., Colaco, R. D. D. R., Swarbreck, S. M., et al. (2013). Salinity-induced calcium signaling and root adaptation in Arabidopsis require the calcium regulatory protein Annexin 1. Plant Physiol. 163, 253–262. doi: 10.1104/pp.113.217810
Lee, H. J., and Seo, P. J. (2021). Ca(2+)talyzing initial responses to environmental stresses. Trends Plant Sci. 26, 849–870. doi: 10.1016/j.tplants.2021.02.007
Li, H. Z., Jiang, X. C., Lv, X. Z., Ahammed, G. J., Guo, Z. X., Qi, Z. Y., et al. (2019). Tomato GLR3.3 and GLR3.5 mediate cold acclimation-induced chilling tolerance by regulating apoplastic H2O2 production and redox homeostasis. Plant Cell Environ. 42, 3326–3339. doi: 10.1111/pce.13623
Liao, C. C., Zheng, Y., and Guo, Y. (2017). MYB30 transcription factor regulates oxidative and heat stress responses through ANNEXIN-mediated cytosolic calcium signaling in Arabidopsis. New Phytol. 216, 163–177. doi: 10.1111/nph.14679
Liu, Q. B., Ding, Y. L., Shi, Y. T., Ma, L., Wang, Y., Song, C. P., et al. (2021). The calcium transporter Annexin1 mediates cold-induced calcium signaling and freezing tolerance in plants. EMBO J. 40:e104559. doi: 10.15252/embj.2020104559
Liu, J. X., and Howell, S. H. (2016). Managing the protein folding demands in the endoplasmic reticulum of plants. New Phytol. 211, 418–428. doi: 10.1111/nph.13915
Liu, X., Wang, J. W., and Sun, L. F. (2018). Structure of the hyperosmolality-gated calcium-permeable channel OSCA1.2. Nat. Commun. 9:5060. doi: 10.1038/s41467-018-07564-5
Luo, J., Chen, L. L., Huang, F. F., Gao, P., Zhao, H. P., Wang, Y. D., et al. (2020). Intraorganellar calcium imaging in Arabidopsis seedling roots using the GCaMP variants GCaMP6m and R-CEPIA1er. J. Plant Physiol. 246–247:153127. doi: 10.1016/j.jplph.2020.153127
Ma, Y., Dai, X., Xu, Y., Luo, W., Zheng, X., Zeng, D., et al. (2015). COLD1 confers chilling tolerance in rice. Cell 160, 1209–1221. doi: 10.1016/j.cell.2015.01.046
Ma, L., Ye, J. M., Yang, Y. Q., Lin, H. X., Yue, L. L., Luo, J., et al. (2019). The Sos2-Scabp8 complex generates and fine-tunes an AtANN4-dependent calcium signature under salt stress. Dev. Cell 48, 697.e5–709.e5. doi: 10.1016/j.devcel.2019.02.010
Maathuis, F. J. M. (2011). Vacuolar two-pore K+ channels act as vacuolar osmosensors. New Phytol. 191, 84–91. doi: 10.1111/j.1469-8137.2011.03664.x
Markham, J. E., Li, J., Cahoon, E. B., and Jaworski, J. G. (2006). Separation and identification of major plant sphingolipid classes from leaves. J. Biol. Chem. 281, 22684–22694. doi: 10.1074/jbc.M604050200
Mehlmer, N., Parvin, N., Hurst, C. H., Knight, M. R., Teige, M., and Vothknecht, U. C. (2012). A toolset of aequorin expression vectors for in planta studies of subcellular calcium concentrations in Arabidopsis thaliana. J. Exp. Bot. 63, 1751–1761. doi: 10.1093/jxb/err406
Miller, G., Schlauch, K., Tam, R., Cortes, D., Torres, M. A., Shulaev, V., et al. (2009). The plant NADPH oxidase RBOHD mediates rapid systemic signaling in response to diverse stimuli. Sci. Signal. 2:ra45. doi: 10.1126/scisignal.2000448
Mousavi, S. A. R., Dubin, A. E., Zeng, W. Z., Coombs, A. M., Do, K., Ghadiri, D. A., et al. (2021). PIEZO ion channel is required for root mechanotransduction in Arabidopsis thaliana. Proc. Natl. Acad. Sci. U. S. A. 118. doi: 10.1073/pnas.2102188118
Nakagawa, Y., Katagiri, T., Shinozaki, K., Qi, Z., Tatsumi, H., Furuichi, T., et al. (2007). Arabidopsis plasma membrane protein crucial for Ca2+ influx and touch sensing in roots. Proc. Natl. Acad. Sci. U. S. A. 104, 3639–3644. doi: 10.1073/pnas.0607703104
Nakano, M., Furuichi, T., Sokabe, M., Iida, H., and Tatsumi, H. (2021). The gravistimulation-induced very slow Ca2+ increase in Arabidopsis seedlings requires MCA1, a Ca(2+)-permeable mechanosensitive channel. Sci. Rep. 11:227. doi: 10.1038/s41598-020-80733-z
Nishii, K., Moller, M., and Iida, H. (2021). Mix and match: patchwork domain evolution of the land plant-specific Ca2+−permeable mechanosensitive channel MCA. PLoS One 16:e0249735. doi: 10.1371/journal.pone.0249735
Ogasawara, Y., Kaya, H., Hiraoka, G., Yumoto, F., Kimura, S., Kadota, Y., et al. (2008). Synergistic activation of the Arabidopsis NADPH oxidase AtrbohD by Ca2+ and phosphorylation. J. Biol. Chem. 283, 8885–8892. doi: 10.1074/jbc.M708106200
Orvar, B. L., Sangwan, V., Omann, F., and Dhindsa, R. S. (2000). Early steps in cold sensing by plant cells: the role of actin cytoskeleton and membrane fluidity. Plant J. 23, 785–794. doi: 10.1046/j.1365-313x.2000.00845.x
Pandey, G. K., Cheong, Y. H., Kim, K. N., Grant, J. J., Li, L. G., Hung, W., et al. (2004). The calcium sensor calcineurin B-like 9 modulates abscisic acid sensitivity and biosynthesis in Arabidopsis. Plant Cell 16, 1912–1924. doi: 10.1105/tpc.021311
Pei, Z. M., Murata, Y., Benning, G., Thomine, S., Klusener, B., Allen, G. J., et al. (2000). Calcium channels activated by hydrogen peroxide mediate abscisic acid signalling in guard cells. Nature 406, 731–734. doi: 10.1038/35021067
Plieth, C., and Trewavas, A. J. (2002). Reorientation of seedlings in the earth’s gravitational field induces cytosolic calcium transients. Plant Physiol. 129, 786–796. doi: 10.1104/pp.011007
Radin, I., Richardson, R., Coomey, J., Weiner, E., Bascom, C., Li, T., et al. (2021). Plant PIEZO homologs modulate vacuole morphology during tip growth. Sci. Total Environ. 373, 586–590. doi: 10.1126/science.abe6310
Rentel, M. C., and Knight, M. R. (2004). Oxidative stress-induced calcium signaling in Arabidopsis. Plant Physiol. 135, 1471–1479. doi: 10.1104/pp.104.042663
Seybold, H., Trempel, F., Ranf, S., Scheel, D., Romeis, T., and Lee, J. (2014). Ca2+ signalling in plant immune response: from pattern recognition receptors to Ca2+ decoding mechanisms. New Phytol. 204, 782–790. doi: 10.1111/nph.13031
Sierla, M., Horak, H., Overmyer, K., Waszczak, C., Yarmolinsky, D., Maierhofer, T., et al. (2018). The receptor-like Pseudokinase GHR1 is required for stomatal closure. Plant Cell 30, 2813–2837. doi: 10.1105/tpc.18.00441
Stael, S., Wurzinger, B., Mair, A., Mehlmer, N., Vothknecht, U. C., and Teige, M. (2012). Plant organellar calcium signalling: an emerging field. J. Exp. Bot. 63, 1525–1542. doi: 10.1093/jxb/err394
Steinhorst, L., and Kudla, J. (2013). Calcium and reactive oxygen species rule the waves of signaling. Plant Physiol. 163, 471–485. doi: 10.1104/pp.113.222950
Steinhorst, L., and Kudla, J. (2019). How plants perceive salt. Nature 572, 318–320. doi: 10.1038/d41586-019-02289-x
Suzuki, N., Miller, G., Morales, J., Shulaev, V., Torres, M. A., and Mittler, R. (2011). Respiratory burst oxidases: the engines of ROS signaling. Curr. Opin. Plant Biol. 14, 691–699. doi: 10.1016/j.pbi.2011.07.014
Toyota, M., Furuichi, T., Tatsumi, H., and Sokabe, M. (2008). Cytoplasmic calcium increases in response to changes in the gravity vector in hypocotyls and petioles of Arabidopsis seedlings. Plant Physiol. 146, 505–514. doi: 10.1104/pp.107.106450
Trewavas, A. J., and Knight, H. (1994). Mechanical signalling calcium and plant form. Plant Mol. Biol. 26, 1329–1341. doi: 10.1007/BF00016478
Van Zelm, E., Zhang, Y., and Testerink, C. (2020). Salt tolerance mechanisms of plants. Annu. Rev. Plant Biol. 71, 403–433. doi: 10.1146/annurev-arplant-050718-100005
Vigani, G., and Costa, A. (2019). Harnessing the new emerging imaging technologies to uncover the role of Ca2+ signalling in plant nutrient homeostasis. Plant Cell Environ. 42, 2885–2901. doi: 10.1111/pce.13611
Walia, A., Waadt, R., and Jones, A. M. (2018). Genetically encoded biosensors in plants: pathways to discovery. Annu. Rev. Plant Biol. 69, 497–524. doi: 10.1146/annurev-arplant-042817-040104
Wang, J. C., Ren, Y. L., Liu, X., Luo, S., Zhang, X., Liu, X., et al. (2021). Transcriptional activation and phosphorylation of OsCNGC9 confer enhanced chilling tolerance in rice. Mol. Plant 14, 315–329. doi: 10.1016/j.molp.2020.11.022
Waszczak, C., Carmody, M., and Kangasjarvi, J. (2018). Reactive oxygen species in plant signaling. Annu. Rev. Plant Biol. 69, 209–236. doi: 10.1146/annurev-arplant-042817-040322
Wu, F., Chi, Y., Jiang, Z., Xu, Y., Xie, L., Huang, F., et al. (2020). Hydrogen peroxide sensor HPCA1 is an LRR receptor kinase in Arabidopsis. Nature 578, 577–581. doi: 10.1038/s41586-020-2032-3
Yamanaka, T., Nakagawa, Y., Mori, K., Nakano, M., Imamura, T., Kataoka, H., et al. (2010). Mca1 and Mca2 that mediate Ca2+ uptake have distinct and overlapping roles in Arabidopsis. Plant Physiol. 152, 1284–1296. doi: 10.1104/pp.109.147371
Yan, Y., Wei, C. L., Zhang, W. R., Cheng, H. P., and Liu, J. (2006). Cross-talk between calcium and reactive oxygen species signaling. Acta Pharmacol. Sin. 27, 821–826. doi: 10.1111/j.1745-7254.2006.00390.x
Yang, T., Shad Ali, G., Yang, L., Du, L., Reddy, A. S., and Poovaiah, B. W. (2010). Calcium/calmodulin-regulated receptor-like kinase CRLK1 interacts with MEKK1 in plants. Plant Signal. Behav. 5, 991–994. doi: 10.4161/psb.5.8.12225
Yoshimura, K., Iida, K., and Iida, H. (2021). MCAS in Arabidopsis are Ca2+-permeable mechanosensitive channels inherently sensitive to membrane tension. Nat. Commun. 12:6074. doi: 10.1038/s41467-021-26363-z
Yuan, F., Yang, H., Xue, Y., Kong, D., Ye, R., Li, C., et al. (2014). OSCA1 mediates osmotic-stress-evoked Ca2+ increases vital for osmosensing in Arabidopsis. Nature 514, 367–371. doi: 10.1038/nature13593
Zhang, Y., Lv, Y., Jahan, N., Chen, G., Ren, D., and Guo, L. (2018b). Sensing of abiotic stress and ionic stress responses in plants. Int. J. Mol. Sci. 19:3298. doi: 10.3390/ijms19113298
Zhang, M. F., Wang, D. L., Kang, Y. L., Wu, J. X., Yao, F. Q., Pan, C. F., et al. (2018a). Structure of the mechanosensitive OSCA channels. Nat. Struct. Mol. Biol. 25, 850–858. doi: 10.1038/s41594-018-0117-6
Zhang, H., Zhu, J., Gong, Z., and Zhu, J. K. (2022). Abiotic stress responses in plants. Nat. Rev. Genet. 23, 104–119. doi: 10.1038/s41576-021-00413-0
Zhao, C. Z., Zayed, O., Yu, Z. P., Jiang, W., Zhu, P. P., Hsu, C. C., et al. (2018). Leucine-rich repeat extensin proteins regulate plant salt tolerance in Arabidopsis. Proceedings of the National Academy of Sciences of the United States of America 115, 13123–13128. doi: 10.1073/pnas.1816991115
Zhao, C., Zhang, H., Song, C., Zhu, J.-K., and Shabala, S. (2020). Mechanisms of plant responses and adaptation to soil salinity. Innovation 1:100017. doi: 10.1016/j.xinn.2020.100017
Zheng, Y., Liao, C. C., Zhao, S. S., Wang, C. W., and Guo, Y. (2017). The Glycosyltransferase QUA1 regulates chloroplast-associated calcium signaling During salt and drought stress in Arabidopsis. Plant Cell Physiol. 58, 329–341. doi: 10.1093/pcp/pcw192
Zheng, Y., Luo, L. D., Wei, J. J., Chen, Q., Yang, Y. P., Hu, X. Y., et al. (2018). The glutamate receptors AtGLR1.2 and AtGLR1.3 increase cold tolerance by regulating jasmonate signaling in Arabidopsis thaliana. Biochem. Biophys. Res. Commun. 506, 895–900. doi: 10.1016/j.bbrc.2018.10.153
Keywords: abiotic stress, sensing mechanisms, Ca2+ signaling, sensor, transduction
Citation: Xu T, Niu J and Jiang Z (2022) Sensing Mechanisms: Calcium Signaling Mediated Abiotic Stress in Plants. Front. Plant Sci. 13:925863. doi: 10.3389/fpls.2022.925863
Edited by:
Manuel Nieves-Cordones, Center for Edaphology and Applied Biology of Segura (CSIC), SpainCopyright © 2022 Xu, Niu and Jiang. This is an open-access article distributed under the terms of the Creative Commons Attribution License (CC BY). The use, distribution or reproduction in other forums is permitted, provided the original author(s) and the copyright owner(s) are credited and that the original publication in this journal is cited, in accordance with accepted academic practice. No use, distribution or reproduction is permitted which does not comply with these terms.
*Correspondence: Zhonghao Jiang, emgtamlhbmdAc3p1LmVkdS5jbg==