- 1Kunming Institute of Botany, Chinese Academy of Sciences, Kunming, China
- 2University of Chinese Academy of Sciences, Beijing, China
- 3School of Life Sciences, Northwest University, Xi’an, China
Melatonin (MT), an important phytohormone synthesized naturally, was recently used to improve plant resistance against abiotic and biotic stresses. However, the effects of exogenous melatonin on photosynthetic performances have not yet been well clarified. We found that spraying of exogenous melatonin (100 μM) to leaves slightly affected the steady state values of CO2 assimilation rate (AN), stomatal conductance (gs) and mesophyll conductance (gm) under high light in tobacco leaves. However, this exogenous melatonin strongly delayed the induction kinetics of gs and gm, leading to the slower induction speed of AN. During photosynthetic induction, AN is mainly limited by biochemistry in the absence of exogenous melatonin, but by CO2 diffusion conductance in the presence of exogenous melatonin. Therefore, exogenous melatonin can aggravate photosynthetic carbon loss during photosynthetic induction and should be used with care for crop plants grown under natural fluctuating light. Within the first 10 min after transition from low to high light, photosynthetic electron transport rates (ETR) for AN and photorespiration were suppressed in the presence of exogenous melatonin. Meanwhile, an important alternative electron sink, namely water-water cycle, was enhanced to dissipate excess light energy. These results indicate that exogenous melatonin upregulates water-water cycle to facilitate photoprotection. Taking together, this study is the first to demonstrate that exogenous melatonin inhibits dynamic photosynthesis and improves photoprotection in higher plants.
Introduction
Melatonin (MT) is an important hormone synthesized naturally in both plants and animals. Many recent studies have documented that MT is critical in several metabolic processes, including ROS scavenging systems (Siddiqui et al., 2020a,b), secondary metabolism (Farouk and Al-Amri, 2019; Jahan et al., 2020), and modulation of nitrogen metabolism (Qiao et al., 2019; Chen et al., 2021; Meng et al., 2021; Kaya et al., 2022). Therefore, MT plays a significant role in plants to cope with biotic and abiotic stresses (Arnao and Hernández-Ruiz, 2015, 2019, 2020). For example, MT promotes plant growth under harsh environmental conditions such as pollution of harmful elements (Farouk and Al-Amri, 2019; Kaya et al., 2019, 2022; Ahammed et al., 2020; Jahan et al., 2020; Seleiman et al., 2020; Hoque et al., 2021; Li S. et al., 2021; Bhat et al., 2022), heat (Ahammed et al., 2018; Jahan et al., 2019), low temperature (Bajwa et al., 2014; Li et al., 2018; Zhang et al., 2021), salinity (Liang et al., 2015; Qi et al., 2020; Siddiqui et al., 2020a), drought (Sharma and Zheng, 2019; Dai et al., 2020; Imran et al., 2021), high light (Ding et al., 2018; Lee and Back, 2018), ultraviolet radiation (Yao et al., 2021), and herbicides (Park et al., 2013; Giraldo Acosta et al., 2022). Therefore, MT is a plant master regulator with great potential for increasing crop yield in agriculture (Wang et al., 2018; Arnao and Hernández-Ruiz, 2019; Bose and Howlader, 2020). Spraying of melatonin to leaves with a moderate concentration of 100 μM was usually used in previous studies, and the photosynthetic capacity was hardly affected by the spraying of MT (Jahan et al., 2020; Kaya et al., 2022). Naturally, plant growth is not only determined by the photosynthetic capacity but also can be affected by the dynamic photosynthesis under fluctuating light (Adachi et al., 2019; Kimura et al., 2020; Yamori et al., 2020). In nature, fluctuating light can affect plant growth by restricting photosynthesis. However, it is unclear whether the spraying of MT can affect the dynamic photosynthesis in healthy leaves. If the spraying of MT improves photosynthetic induction in crops, it can be used as a potential growth promoter. However, if the dynamic photosynthesis in higher plants is inhibited by the spraying of MT, MT should be used with care to avoid environmental pollution.
Under high light, stomatal conductance (gs) and mesophyll conductance (gm) are elevated to increase CO2 diffusion from air to the sites of Rubisco carboxylation in chloroplasts and thus contribute to the high level of net CO2 assimilation rate (AN) (Oguchi et al., 2003; Xiong et al., 2015, 2018; Ferroni et al., 2021). Under low light, relative low levels of gs and/or gm can satisfy the low AN (Xiong et al., 2018; Qiao et al., 2020; Zhang et al., 2020). Most crop plants cultivated under natural field conditions usually experience dramatic fluctuations of illumination (Pearcy, 1990; Slattery et al., 2018). When light intensity increased abruptly, the low gs and/or gm restricted CO2 diffusion rate and thus made AN to be limited by the low chloroplast CO2 concentration (De Souza et al., 2020; Liu et al., 2022; Sun et al., 2022). Improved stomatal opening or increased gs could significantly accelerate the response speed of AN and thus enhance biomass production in fluctuating light (Kimura et al., 2020; Yamori et al., 2020). Under salinity or nitrogen deficiency conditions, the decreased induction speeds of gs and gm restricted AN during photosynthetic induction, leading to the decline of biomass production under fluctuating light (Zhang et al., 2020; Sun et al., 2022). Therefore, if MT increases the induction speeds of gs and gm, it can be used as a growth promotor for crop plants under natural fluctuating light. In the other hand, if MT decreases the response kinetics of gs and gm under fluctuating light, MT should be used with care to prevent negative effect on plant growth. Therefore, it is necessary to clarify the effects of MT on dynamic changes in gs and gm.
When CO2 assimilation is restricted under environmental stresses, the excess light energy should be finely dissipated harmlessly to avoid photodamage to photosystem I and II (PSI and PSII). For example, fluctuating light causes selective photoinhibition of PSI in angiosperms (Kono et al., 2014; Yamamoto et al., 2016; Huang et al., 2019a; Yamamoto and Shikanai, 2019). When light intensity abruptly increases, electron transport from PSII immediately increases (Sun et al., 2020b; Tan et al., 2021). This rapid change in PSII electron flow is accompanied by much slower kinetics of AN (Yamamoto et al., 2016). The resulting PSI over-reduction produces reactive oxygen species within PSI and thus causes PSI photoinhibition (Yamamoto and Shikanai, 2019). Owing to the key role of PSI in regulation of photosynthetic electron flow, PSI photoinhibition strongly suppresses AN, photoprotection and plant growth (Sejima et al., 2014; Brestic et al., 2015; Zivcak et al., 2015; Lima-Melo et al., 2019; Shimakawa and Miyake, 2019). Under high light, the inhibition of AN increases the electron transfer from PSI to oxygen, resulting in the production of reactive oxygen species in chloroplast stroma (Takahashi and Murata, 2005, 2006). Reactive oxygen species inhibit the de novo synthesis of PSII proteins, primarily the D1 protein at the translation elongation step in psbA expression (Nishiyama et al., 2001, 2005). Under such conditions, the higher rate of PSII photodamage relative to PSII repair accelerates PSII photoinhibition (Murata et al., 2007). If moderate PSII photoinhibition occurred, the oxidation of water at PSII and linear electron flow would be suppressed, restricting regeneration of ATP and NADPH and thus impairing AN and plant growth (Takahashi and Murata, 2008; Huang et al., 2018; Kaya et al., 2022).
Plants have several photoprotective mechanisms to deal with environmental stress (Takahashi and Badger, 2011; Allahverdiyeva et al., 2015; Shikanai and Yamamoto, 2017; Alboresi et al., 2019). In angiosperms, cyclic electron flow plays the key role in protecting PSI and PSII under excess light (Munekage et al., 2002, 2008; Takahashi et al., 2009; Suorsa et al., 2012; Yamamoto and Shikanai, 2019). In addition, water-water cycle can significantly prevent PSI photoinhibition under fluctuating light (Huang et al., 2019b; Sun et al., 2020a; Yang et al., 2020) and protect PSII under high light (Asada, 1999, 2000; Hirotsu et al., 2004; Yi et al., 2014; Huang et al., 2016). During water-water cycle, electrons splitting from water are transported through photosynthetic electron transport chains and ultimately to oxygen. The resulting reactive oxygen species are converted into water by superoxide dismutase (SOD) and ascorbate peroxidase (APX). The operation of water-water cycle can dissipate excess light energy, increase ΔpH formation and balance ATP/NADPH production ratio (Miyake, 2010; Shikanai and Yamamoto, 2017). Consequently, water-water cycle favors photosynthetic regulation when CO2 assimilation is restricted under harsh environmental conditions. As reported in previous studies, exogenous MT can increase the expression of SOD and APX in leaves of higher plants (Kaya et al., 2019; Jahan et al., 2020; Li X. et al., 2021). Because SOD and APX are the two key enzymes in charge of water-water cycle (Asada, 2000), the positive effect of exogenous MT on plant growth under environmental stresses might be related to the enhancement of water-water cycle. However, no study has investigated the effect of exogenous MT on the capacity of water-water cycle.
In the present study, we studied the effect of exogenous MT on dynamic photosynthetic performances in leaves of tobacco. The aims were to (1) understand whether exogenous MT is beneficial or detrimental to dynamic photosynthesis; and (2) explore whether exogenous MT enhances the capacity of water-water cycle. We found that spraying of exogenous MT strongly inhibited the dynamic photosynthesis in healthy leaves of tobacco, suggesting that abuse of MT can restrict the photosynthetic carbon gain under natural fluctuating light. Furthermore, exogenous MT upregulated water-water cycle to favor photoprotection especially when CO2 assimilation was restricted.
Materials and methods
Plant materials and treatments
Tobacco (Nicotiana tabacum cv. K326) plants were grown in an open field with full sunlight. Plants were grown in 19-cm plastic pots with humus soil (the initial soil nitrogen content was 2.1 mg/g). Plants were fertilized with Peters Professional’s water solution (0.15 g N/plant every 2 days) and were watered every day to prevent any nutrient or water stress. After cultivation for 1 month, melatonin solution (MT, 100 μM) or water were sprayed to youngest fully developed leaves. This MT concentration was chosen based on previous studies (Kaya et al., 2019, 2022; Jahan et al., 2020). After spraying twice with the interval of 3 days, photosynthetic measurements were conducted. During the period of treatment, the day/night air temperatures were approximately 30/20 C, the relative air humidity was approximately 60–70%, and the maximum light intensity exposed to leaves was approximately 2,000 μmol photons m–2 s–1.
Gas exchange and chlorophyll fluorescence measurements
Gas exchange and chlorophyll fluorescence were measured using a LI-6400XT coupled with a fluorometer (Li-6400-40; Li-Cor Inc., Lincoln, NE, United States). For all measurements, air temperature was approximately 25°C and the vapor pressure deficit was approximately 1.3 kPa. The flow rate within the chamber was set at 300 mmol air min–1. After pre-illumination at high light (1,500 μmol photons m–2 s–1, 90–10% red-blue light) and 400 μmol CO2 mol–1 air to reach steady-state photosynthesis, leaves were exposed to low light (50 μmol photons m–2 s–1, 90–10% red-blue light) for 5 min to simulate natural shadefleck. Afterward, photosynthetic induction phases were conducted again at high light (1,500 μmol photons m–2 s–1), and the steady-state conditions were achieved after 30 min illumination.
During photosynthesis induction, the steady-state fluorescence (Fs) and the maximum fluorescence (Fm’) were measured for further analysis. Fm′ was measured by application of a saturating white light flash of 8,000 μmol m–2 s–1, and the quantum efficiency of photosystem II (ΦPSII) was calculated as follows (Genty et al., 1989):
The electron transport rate (ETR) through PSII was calculated as
where the PPFD value corresponded to the light intensity stated above, the typical value 0.45 was assumed for the product of α × β (Kaiser et al., 2017).
Estimation of mesophyll conductance, chloroplast CO2 concentration, and maximum velocity of rubisco for carboxylation
Based on the combination of gas exchange and ETR, gm is calculated (Harley et al., 1992):
where AN represents the area-based net CO2 assimilation rate and Γ* represents the CO2 compensation point in the absence of respiration (Farquhar et al., 1980; von Caemmerer and Evans, 2015). The average Γ* for C3 species at 25°C, 41.2 μmol/mol (Hermida-Carrera et al., 2016), was used in this study. In the current study, the day respiration rate (Rd) was calculated as half of the dark respiration rate as measured after dark adaptation for 10 min (Carriquí et al., 2015).
Based on the estimated gm, the chloroplast CO2 concentration (Cc) was calculated (Long and Bernacchi, 2003; Warren and Dreyer, 2006):
The maximum velocity of Rubisco for carboxylation (Vcmax) at steady-state conditions was calculated with following equation (Farquhar et al., 1980; Eyland et al., 2021):
where Km is the effective the Rubisco Michaelis–Menten constant for CO2 under 21% O2, and the average value for C3 species at 25°C, 529.4 μmol mol–1 (Hermida-Carrera et al., 2016; Eyland et al., 2021), was used in this study.
Quantitative limitation analysis of assimilation rate
In general, photosynthesis can be limited by stomatal conductance, mesophyll conductance, and biochemical capacity. The relative photosynthetic limitations ls, lm, and lb represent the relative importance of stomatal conductance, mesophyll conductance, and biochemical capacity, respectively, in determining the observed value of AN. The values of ls, lm, and lb were calculated using the following equations (Grassi and Magnani, 2005):
where the total CO2 diffusion conductance (gtot) was calculated as 1/gtot = 1/ gs +1/gm (Grassi and Magnani, 2005), and the slope of the AN vs. Cc response curve (∂AN/∂Cc) was calculated according to the method of Xiong et al. (2018).
Analysis of photosynthetic electron transport
From gas exchange parameters, the ETR for Rubisco carboxylation and oxygenation (JG) was calculated as follows (Zivcak et al., 2013; Walker et al., 2014):
The alternative electron sink (JA) was calculated by subtracting JG from ETR:
Because JG represents the ETR for NADPH production, it was further divided into the two components devoted to RuBP carboxylation (JC) or RuBP oxygenation (JO) (Valentini et al., 1995):
where JC indicates the rate of electron flow consumed by the Calvin-Benson cycle, and JC indicates the rate of electron flow consumed by photorespiration.
Statistical analysis
All data are displayed as mean values of five leaves from five independent plants. T-test was used to determine whether significant differences existed between different treatments (α = 0.05).
Results
Exogenous melatonin affects gas exchange during photosynthetic induction
The changing kinetics of AN, gs, and gm during photosynthetic induction were measured by transitioning from low light (50 μmol photons m–2 s–1) to high light (1,500 μmol photons m–2 s–1) (Figure 1). The initial values of AN at low light were 1.8 and 0.7 μmol photons m–2 s–1 in CK and MT-treated leaves, respectively. After this photosynthetic induction for 1 min, AN rapidly increased to 16.7 μmol photons m–2 s–1 in CK leaves but just increased to 9.6 μmol photons m–2 s–1 in the MT-treated leaves (Figure 1A). After this photosynthetic induction for 5 and 10 min, AN in CK leaves increased to 18.9 and 20.7 μmol photons m–2 s–1, respectively (Figure 1A). By comparison, AN in MT-treated leaves increased to 10.3 and 15.2 μmol photons m–2 s–1, respectively (Figure 1A). Therefore, the induction of AN after transition from low light was largely delayed by the application of exogenous melatonin. After illumination at high light for 30 min, AN reached 22.9 and 20.9 μmol photons m–2 s–1 in CK and MT-treated leaves, respectively (Figure 1A), indicating that exogenous melatonin just slightly affected the steady-state AN in tobacco leaves.
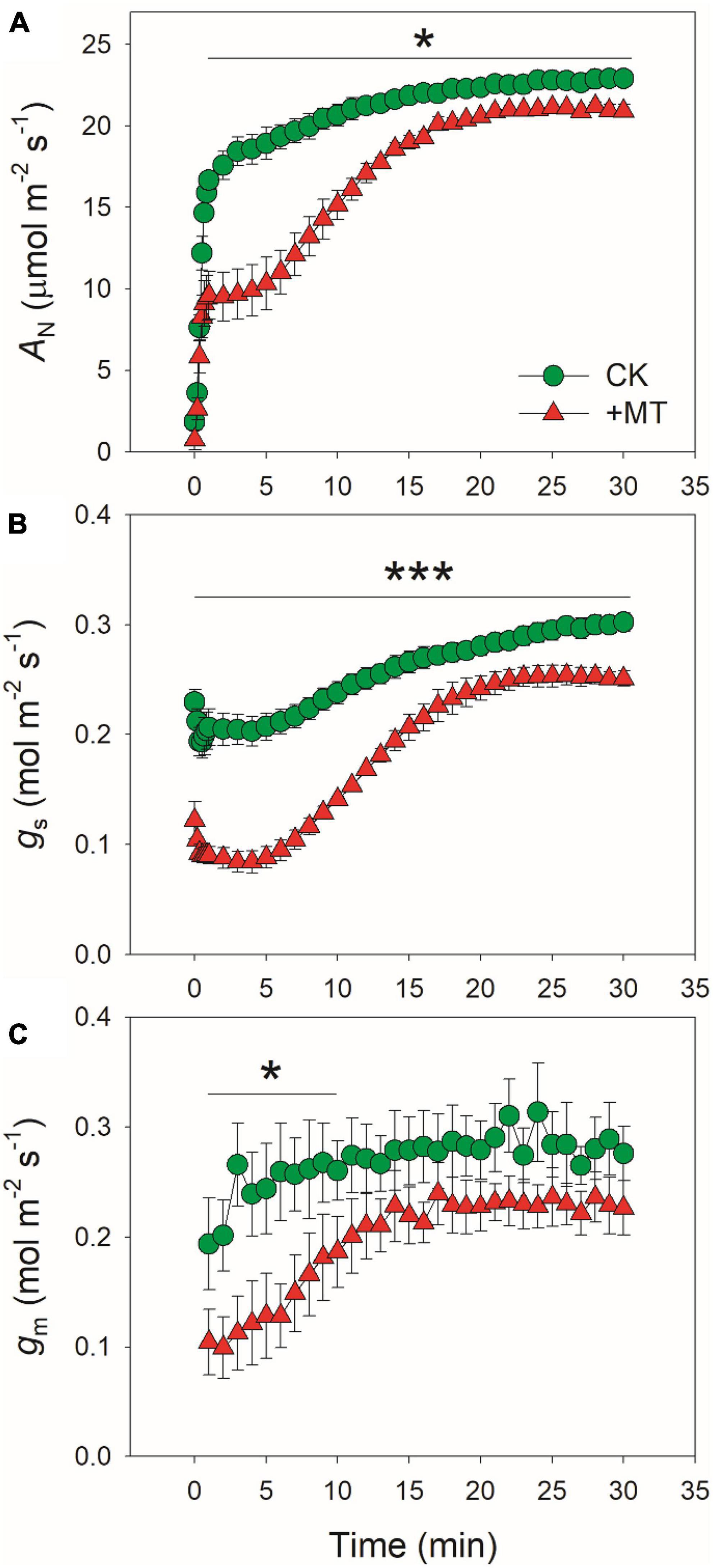
Figure 1. Effects of exogenous melatonin (MT, 100 μM) on the induction response of net CO2 assimilation rate [AN, (A)], stomatal conductance [gs, (B)], and mesophyll conductance [gm, (C)] after transition from 50 to 1500 μmol photons m−2 s−1. Values are means ± SE (n = 5). Asterisk indicates a significant difference between CK and MT-treated leaves.
Because the induction kinetics of AN under fluctuating light is largely affected by gs and gm, we further analyzed the effects of exogenous melatonin on the changing kinetics of gs and gm during photosynthetic induction. Under low light, gs was much lower in the MT-treated leaves when compared with the CK leaves (Figure 1B). Within the first 5 min after photosynthetic induction, gs in CK leaves was two-fold than that in the MT-treated leaves (Figure 1B). After photosynthetic induction for 10 min, gs reached 0.24 and 0.14 mol m–2 s–1 in CK and MT-treated leaves, respectively (Figure 1B). Consistently, the transpiration rate within the first minutes after light increased was also lower in the MT-treated leaves than CK leaves (Supplementary Figure 1). Therefore, exogenous melatonin not only lowered gs under low light but also delayed the stomatal opening under fluctuating light. After photosynthetic induction for 30 min, the values for gs were 0.30 and 0.25 mol m–2 s–1 in CK and MT-treated leaves, respectively (Figure 1B), suggesting the slight effect of exogenous melatonin on steady-state gs. Similar to the performance of gs, the MT-treated leaves showed significantly lower gm than CK leaves within the first 5 min after transition to high light (Figure 1C). However, the steady-state value of gm was just slightly affected by the application of exogenous melatonin (Figure 1C).
After standardization against the maximum values after 30 min photosynthetic induction at high light, the relative changes in AN, gs, and gm after transition from low to high were analyzed (Figure 2). The time required to reach 80% of the maximum AN was approximately 3 min in CK leaves, which was much shorter than that in the MT-treated leaves (12 min) (Figure 2A). Similarly, the time required to reach 70% of the maximum gs was much lower in CK leaves (6 min) than in the MT-treated leaves (13 min) (Figure 2B). The increase in relative gm was faster than gs in both the CK and MT-treated leaves. However, the time required to reach 90% of the maximum gm was much lower in CK leaves (3 min) than in the MT-treated leaves (12 min) (Figure 2C). These results indicated that the induction speeds of AN, gs, and gm during photosynthetic induction were largely delayed upon the application of exogenous melatonin.
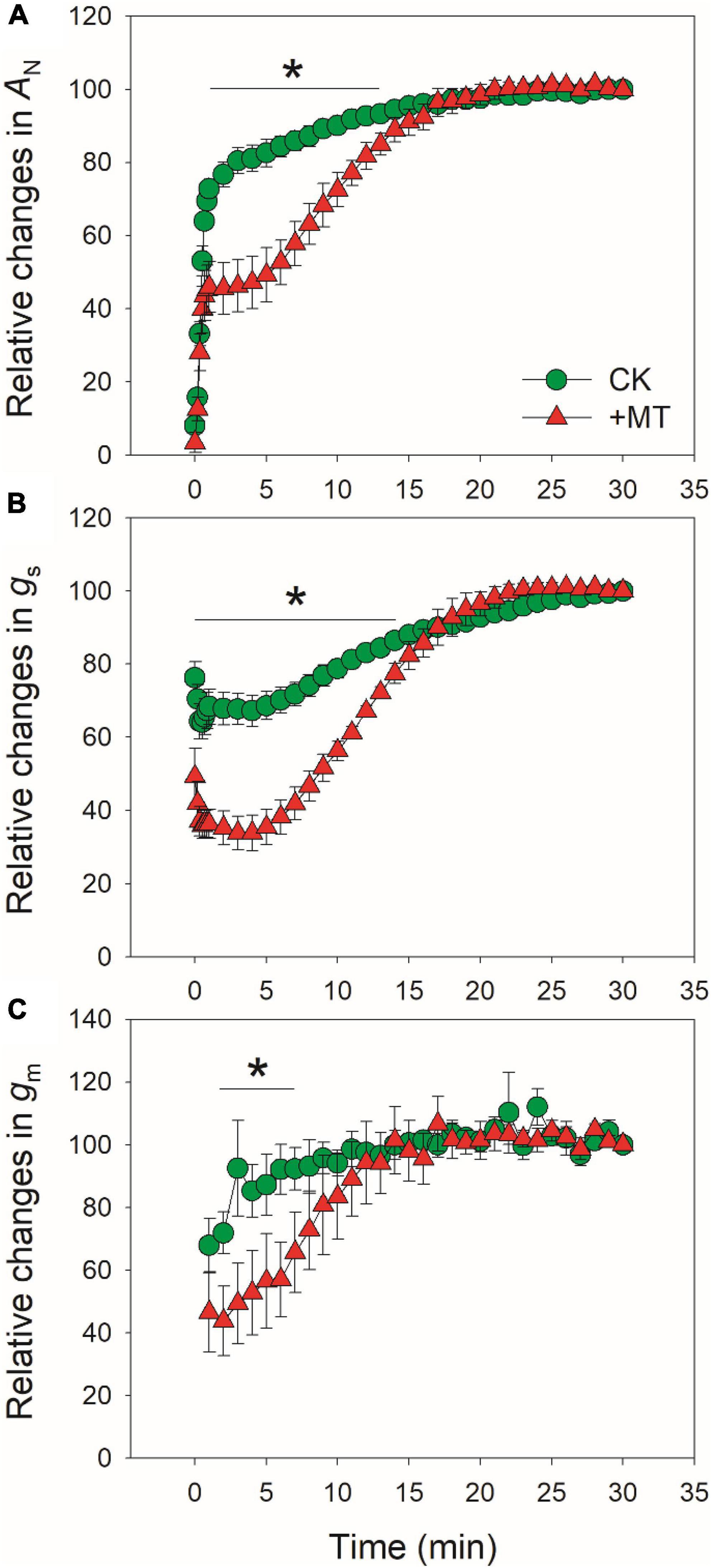
Figure 2. Effects of exogenous melatonin (MT, 100μM) on the relative changes in AN (A), gs (B), and gm (C) after transition from 50 to 1500 μmol photons m−2 s−1. Values are means ± SE (n = 5). Asterisk indicates a significant difference between CK and MT-treated leaves.
Exogenous melatonin alters photosynthetic limitations during photosynthetic induction
Because CO2 diffusion conductance determines photosynthesis through affecting intercellular (Ci) and chloroplast CO2 concentration (Cc), we calculated the response kinetics of Ci and Cc using AN, gs and gm. During the initial 10 min after transition to high light, Ci and Cc were much lower in the MT-treated leaves when compared with CK leaves (Figures 3A,B). Therefore, the delayed induction kinetics of gs and gm in the MT-treated leaves led to the lowering of Cc under fluctuating light. Furthermore, the maximum velocity of Rubisco carboxylation (Vcmax) was inhibited by the exogenous melatonin (Figure 3C), suggesting that the activation state of Rubisco was also decreased by the exogenous melatonin. During photosynthetic induction, the relative limitations of AN by gs (ls), gm (lm), and biochemical factors (lb) changed slightly in CK plants (Figure 4). By comparison, ls gradually decreased and lb gradually increased in the MT-treated leaves. As shown in Figure 4D, the value of (ls + lm)/lb was almost lower than 1.0 in CK leaves, indicating that lb was the major limiting factor of AN after transition from low to high light. In contrast, the value of (ls + lm)/lb in the MT-treated leaves was higher than 1.0 within the initial 10 min of photosynthetic induction (Figure 4D), pointing out that during this period AN was mainly limited by diffusional conductance. Therefore, exogenous melatonin altered the relative limitations of AN during photosynthetic induction. This conclusion was further supported by the ratios of Vcmax and ETR to gross CO2 assimilation rate (AN + Rd). During photosynthetic induction, Vcmax/(AN + Rd) and ETR/(AN + Rd) were maintained stable in CK leaves (Figure 5). However, the MT-treated leaves had higher values of Vcmax/(AN + Rd) and ETR/(AN + Rd) during the initial 10 min of photosynthetic induction (Figure 5). After fully photosynthetic induction, the CK and MT-treated leaves showed similar values of Vcmax/(AN + Rd) and ETR/(AN + Rd) (Figure 5). These results indicated that during photosynthetic induction the limitations of Rubisco activity and electron flow imposed to AN were lowered in the MT-treated leaves compared with CK leaves.
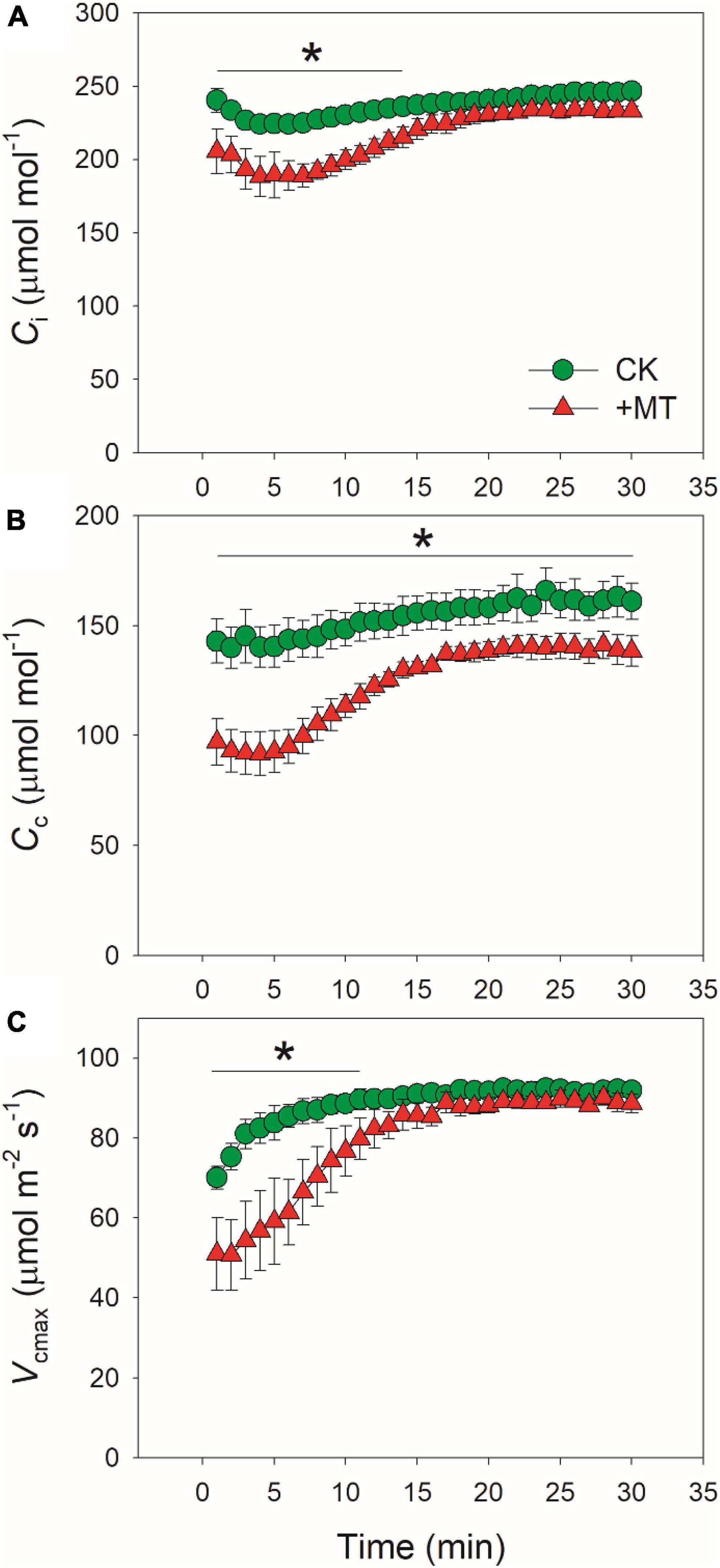
Figure 3. Effects of exogenous melatonin (MT, 100 μM) on the induction response of intercellular CO2 concentration [Ci, (A)], chloroplast CO2 concentration [Cc, (B)], and the maximum velocity of Rubisco for carboxylation [Vcmax, (C)] after transition from 50 to 1500 mol photons m−2 s−1. Values are means ± SE (n = 5). Asterisk indicates a significant difference between CK and MT-treated leaves.
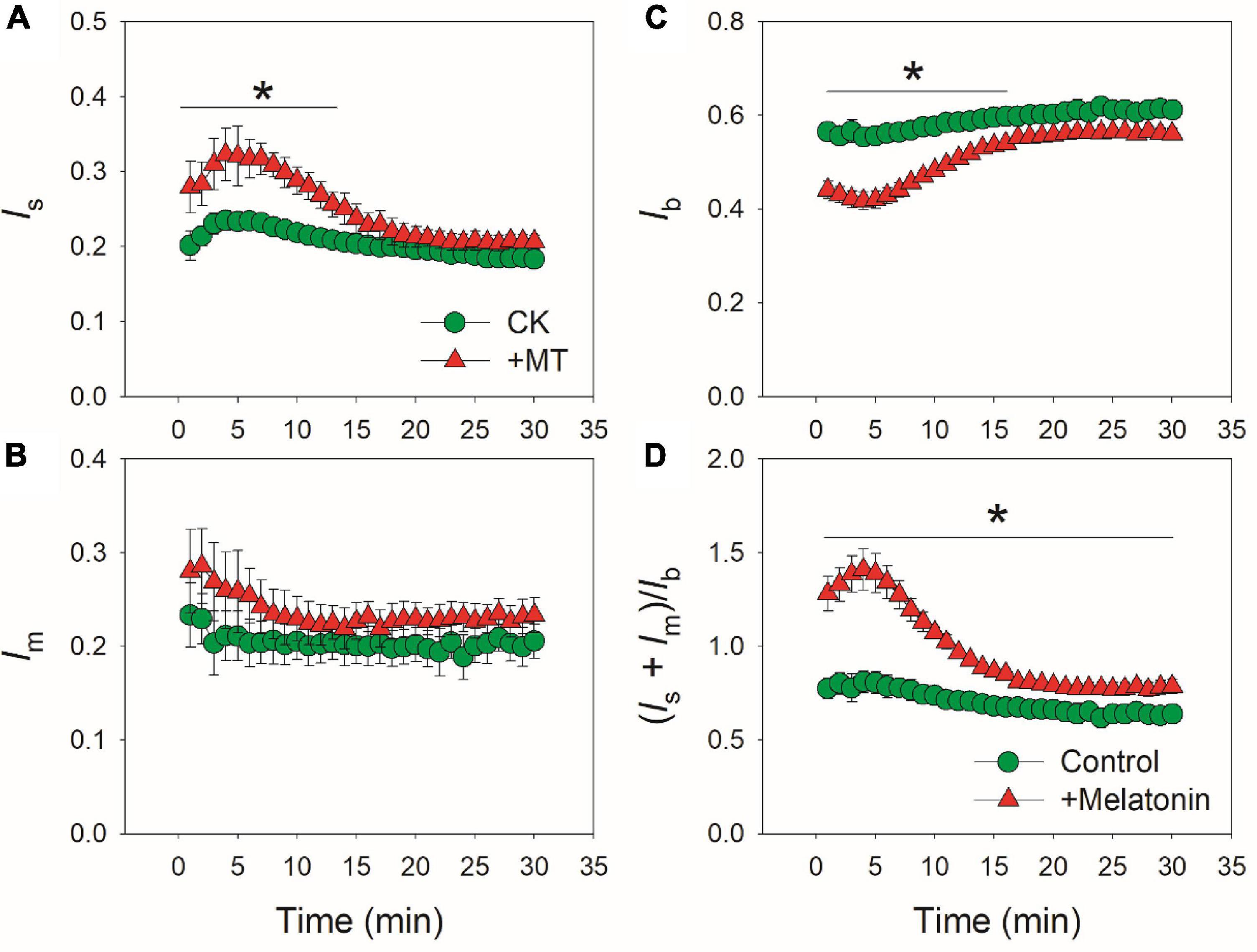
Figure 4. Effects of exogenous melatonin (MT, 100 μM) on the induction response of the relative limitations of gs [ls, (A)], gm [lm, (B)], biochemical factors [lb, (C)] and the ratio of (ls + lm)/lb (D) imposed to photosynthesis after transition from 50 to 1500 μmol photons m−2 s−1. Values are means ± SE (n = 5). Asterisk indicates a significant difference between CK and MT-treated leaves.
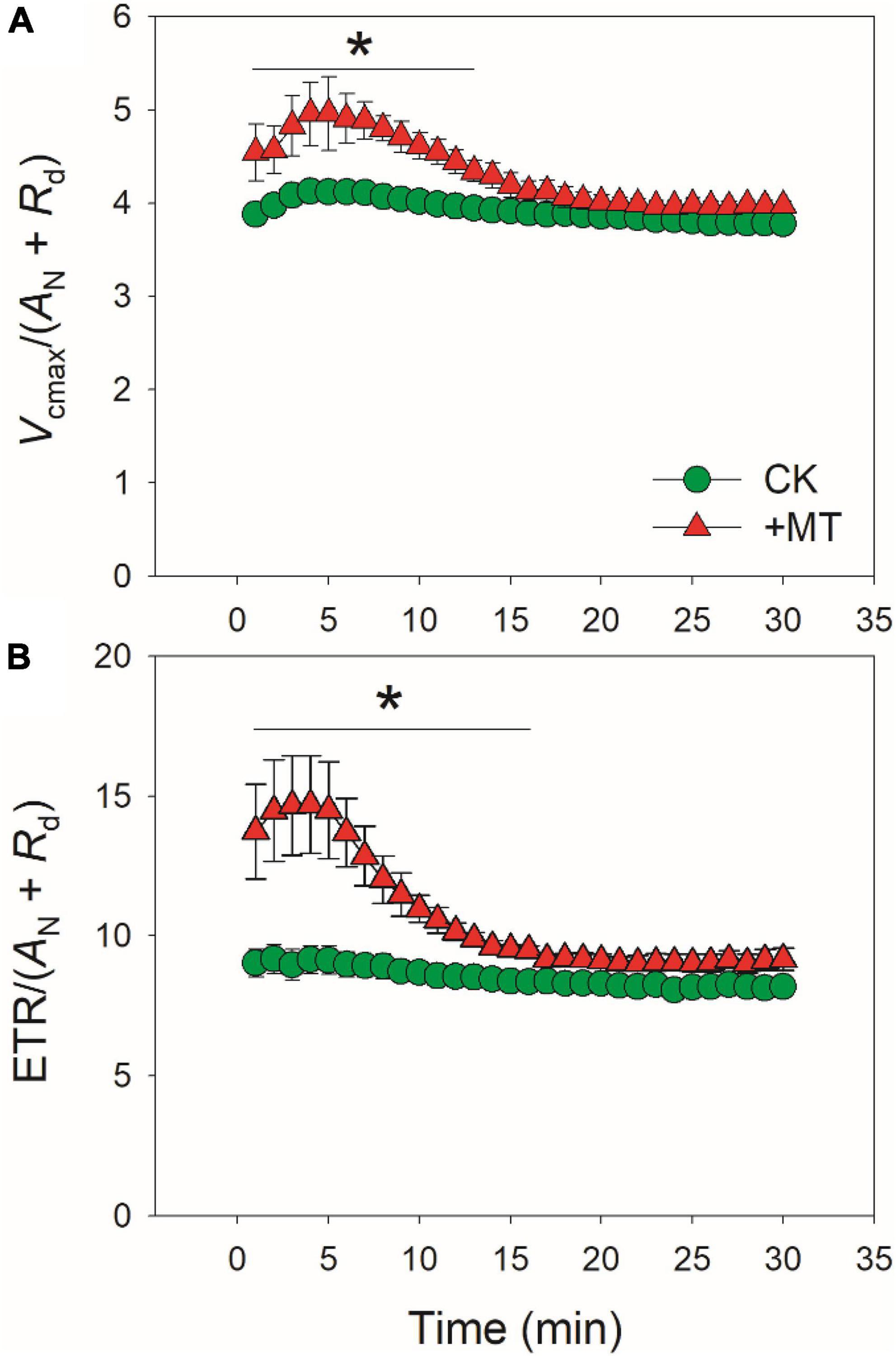
Figure 5. Effects of exogenous melatonin (MT, 100 μM) on the induction response of the values of Vcmax/(AN + Rd) (A) and ETR/(AN + Rd) (B) after transition from 50 to 1500 μmol photons m−2 s−1. Values are means ± SE (n = 5). Asterisk indicates a significant difference between CK and MT-treated leaves.
Exogenous melatonin enhances the capacity of alternative electron sinks
When CO2 was restricted under fluctuating light, alternative electron sinks might protect photosynthetic apparatus against photoinhibition. We analyzed the response kinetics of total PSII ETR, ETR for Rubisco carboxylation (JC), for Rubisco oxygenation (JO), and for alternative sinks (JA) (Figure 6). After transition from low to high light, CK and MT-treated leaves showed similar values of ETR (Figure 6A). However, the MT-treated leaves showed much lower JC and JO during the initial phase of photosynthetic induction (Figures 6B,C). Concomitantly, JA was increased in the MT-treated leaves (Figure 6D). The maximum JA in CK and the MT-treated leaves were 48.6 and 74.5 μmol electrons m–2 s–1, respectively. During photosynthetic induction, JA in the MT-treated leaves was maintained at high levels in the initial 6 min but subsequently decreased gradually. By comparison, JA in CK leaves was maintained stable. Therefore, the MT-treated leaves had a higher JA to compensate for the restriction of JC and JO during the initial phase of photosynthetic induction. After fully photosynthetic induction for 30 min, CK and the MT-treated leaves showed similar ETR. However, a higher JA was observed in the MT-treated leaves. These results strongly indicated that exogenous melatonin enhanced the capacity of JA without altering the total ETR.
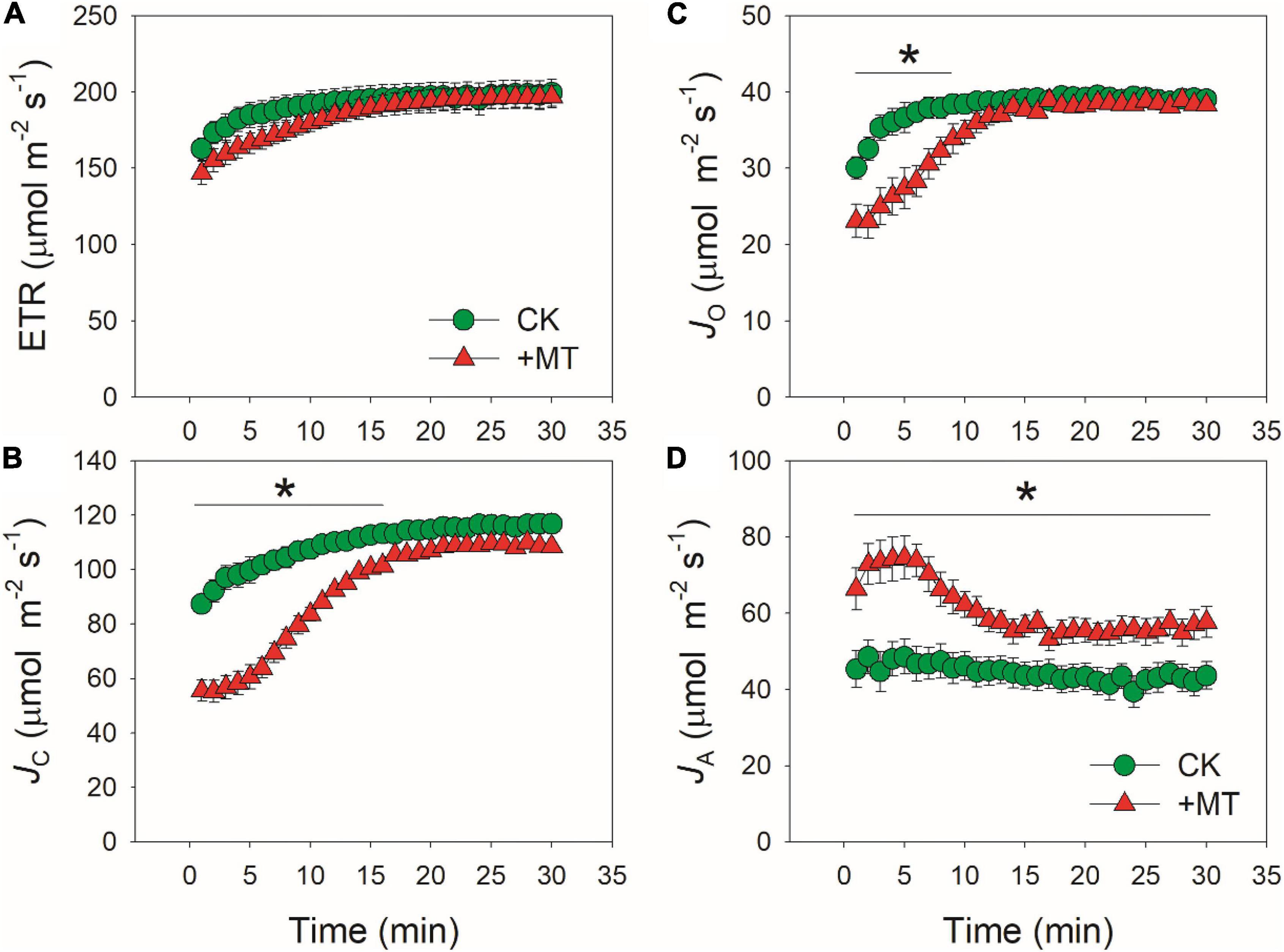
Figure 6. Effects of exogenous melatonin (MT, 100 μM) on the induction response of total electron transport rate (ETR) (A), electron flow for Rubisco carboxylation (JC) (B), electron flow for Rubisco oxygenation (JO) (C), and alternative electron sinks (JA) (D) after transition from 50 to 1500 μmol photons m−2 s−1. Values are means ± SE (n = 5). Asterisk indicates a significant difference between CK and MT-treated leaves.
Discussion
Recently, melatonin has been used as a plant master regulator for improving resistance to abiotic stresses (Wang et al., 2018; Arnao and Hernández-Ruiz, 2019). Generally, exogenous melatonin has the potential to modulate oxidative activity, nitrogen metabolism, secondary metabolism under these stresses, leading to the improvement of plant growth under abiotic and biotic stresses (Kaya et al., 2019, 2022; Ahammed et al., 2020; Jahan et al., 2020; Yao et al., 2021). Spraying of melatonin to the leaves is one of the most popular methods used in agriculture (Kaya et al., 2019, 2022; Jahan et al., 2020). This measure gives rise a question that whether exogenous melatonin has side effects on photosynthesis on healthy leaves. Furthermore, in view of evolutionally story of plants, it is surprising that why melatonin is not highly expressed in wild plants to enhance their resistance to environmental stresses. A possible explanation is that the content of melatonin in leaves should be controlled to a moderate level to avoid side effect on photosynthesis (Arnao and Hernández-Ruiz, 2015, 2019). However, the effects of exogenous melatonin on photosynthesis in higher plants have not yet been well known.
Under natural field conditions, plants usually experience fluctuations of light intensity on timescales of seconds, minutes, and hours owing to cloud, wind, and shading from upper leaves (Valladares et al., 1997; Slattery et al., 2018). In this study, we investigated the effects of exogenous melatonin on gas exchange and photosynthetic electron flow in tobacco plants grown under natural fluctuating light conditions. We found that the maximum AN at 1,500 μmol photons m–2 s–1 was similar between the CK and MT-treated leaves (Figure 1A), indicating that the spraying of moderate concentration of melatonin (100 μM) to the leaves hardly affected the steady-state photosynthetic capacity in tobacco. However, exogenous melatonin strongly affected photosynthesis during the photosynthetic induction (Figure 1A). For example, after transitioning from 50 to 1500 μmol photons m–2 s–1 for 1 min, AN increased to 16.7 μmol CO2 m–2 s–1 in CK leaves but just increased to 9.6 μmol CO2 m–2 s–1 in the MT-treated leaves. During prolonged illumination at high light for 10 min, AN increased to 20.7 μmol CO2 m–2 s–1 in CK leaves but just increased to 15.2 μmol CO2 m–2 s–1 in the MT-treated leaves. Therefore, during the initial 10 min of photosynthetic induction, exogenous melatonin strongly decreased the photosynthetic carbon gain of tobacco leaves. Recent studies have documented that the rate of photosynthetic induction is an important factor affecting carbon gain and plant growth when plants grown under natural and artificial fluctuating light (Kaiser et al., 2020; Kimura et al., 2020; Yamori et al., 2020). Accelerated induction speed of AN significantly enhanced biomass production in Arabidopsis thaliana and rice under fluctuating light (Kimura et al., 2020; Sakoda et al., 2020; Yamori et al., 2020). In tomato (Lycopersicon esculentum) plants treated with moderate salinity (80 mM NaCl), the induction speed of AN was lowered, impairing plant growth and reducing biomass production under fluctuating light (Zhang et al., 2020). Therefore, spraying of exogenous melatonin to leaves might impair the plant growth of crops cultivated under natural fluctuating light conditions.
The induction speed of AN can be affected by diffusional conductance (gs and gm) and biochemical factors (Vcamx and ETR) (Kaiser et al., 2017, 2020; Acevedo-Siaca et al., 2020; De Souza et al., 2020; Sakoda et al., 2021; Liu et al., 2022). We found that the MT-treated leaves displayed much lower gs during initial 10 min of photosynthetic induction (Figure 1B), and gs required more time to reach the maximum value in the MT-treated leaves compared with CK leaves (Figure 2B). Furthermore, induction speed of gm was also delayed in the MT-treated leaves (Figures 1C, 2C). Such lowering of gs and gm decreased Ci and Cc during the initial phase of photosynthetic induction (Figure 3). Although the induction speed of Vcmax was lowered by exogenous melatonin (Figure 3C), the MT-treated leaves showed higher values of Vcmax/(AN + Rd) during the initial phase of photosynthetic induction (Figure 5A), suggesting that exogenous melatonin did not increase the limitation of Vcmax imposed to photosynthesis. Similarly, the MT-treated leaves showed higher values of ETR/(AN + Rd) during the initial phase after transition to high light (Figure 5B), indicating that the limitation of ETR imposed to photosynthesis was decreased in the MT-leaves. After quantitative analysis of relative photosynthetic limitations, we found that during the initial 10 min of photosynthetic induction, AN was mainly limited by diffusional conductance in the WT-treated leaves but was mainly limited by biochemical factors in CK plants (Figure 4). This altered relative photosynthetic limitation by exogenous melatonin was largely caused by the increased limitation of gs imposed on AN. Therefore, the inhibition effect of exogenous melatonin on AN during photosynthetic induction was primarily caused by the decreased induction speed of gs.
Previous studies have reported that exogenous melatonin can affect the expression of antioxidant systems, such as SOD and APX (Kaya et al., 2019; Jahan et al., 2020; Siddiqui et al., 2020a,b). As we know, SOD and APX are two critical antioxidant enzymes participating in an important alternative electron sink, water-water cycle (Asada, 1999, 2000; Miyake, 2010). Furthermore, the inhibition of photosynthesis requires water-water cycle to dissipate excess light energy, which is essential for protecting photosynthetic apparatus against photoinhibition (Makino et al., 2002; Hirotsu et al., 2004, 2005). However, it is unclear whether exogenous melatonin can enhance the capacity of water-water cycle to favor photoprotection. We found that the MT-treated leaves displayed much higher alternative electron sinks when ETRs for Rubisco carboxylation and oxygenation were restricted during photosynthetic induction (Figure 6). This result strongly suggested the enhancement of water-water cycle in the MT-treated leaves, because most of alternative electron flow in higher plants was accounted for the electron flux to oxygen (Asada et al., 2000; Zivcak et al., 2013; Yang et al., 2020; Ferroni et al., 2021; Sun et al., 2021). Therefore, the upregulation of water-water cycle is an important reason for why exogenous MT can strengthen photoprotection when CO2 is restricted under environmental stresses.
Within the first seconds after light intensity abruptly increases, plants cannot build up an enough ΔpH to fine-tune PSI redox state (Huang et al., 2019a,b). The resulting PSI over-reduction induces PSI photoinhibition under fluctuating light (Suorsa et al., 2012; Yamamoto and Shikanai, 2019). Furthermore, a decreased gs could aggravate the extent of PSI over-reduction under fluctuating light (Li T. Y. et al., 2021). Upon a sudden transitioning from low to high light, alternative electron sinks can rapidly consume the reducing power in PSI and thus prevents PSI over-reduction (Gerotto et al., 2016; Jokel et al., 2018; Storti et al., 2019, 2020). Recent studies have found that water-water cycle can protect PSI under fluctuating light more efficiently than cyclic electron flow (Huang et al., 2019b; Sun et al., 2020b; Yang et al., 2020). Consequently, PSI is tolerant to photoinhibition under fluctuating light in higher plants with high capacity of water-water cycle, such as in Camellia species (Huang et al., 2019b; Sun et al., 2020b), Bryophyllum pinnatum (Yang et al., 2019), Dendrobium officinale (Yang et al., 2020, 2021), Vanilla planifolia (Wang et al., 2022). Therefore, the enhancement of water-water cycle in the MT-treated leaves can facilitate PSI photoinhibition under fluctuating light. In addition, water-water cycle can dissipate excess excitation energy and helps the formation of ΔpH, both of which are critical for photoprotection for PSII especially when CO2 assimilation is restricted (Miyake, 2010; Yi et al., 2014; Cai et al., 2017). Because water-water cycle generates ATP without reducing NADP+ and thus increases the ATP/NADPH production ratio (Miyake, 2010; Huang et al., 2016), the enhancement of water-water cycle in the MT-treated leaves can regulate the energy balancing when CO2 fixation is restricted. Taking together, up-regulation of water-water cycle in the MT-treated leaves has important physiological functions in photosynthetic regulation under environmental stresses.
Conclusion
Although melatonin has many positive effects on plant tolerance under environmental stresses, we here for the first time documented that the spraying of moderate melatonin content (100 μM) to healthy tobacco leaves strongly inhibited photosynthesis during photosynthetic induction. In particular, exogenous melatonin delayed the induction speed of gs after transition from low to high light. Therefore, gs is the primary target of the delay effect of exogenous melatonin on photosynthesis. Furthermore, we found that the capacity of water-water cycle was enhanced in the MT-treated leaves. When photosynthesis was restricted, water-water cycle facilitated photoprotection and photosynthetic regulation in the MT-treated leaves. Therefore, exogenous melatonin has large effects on gas exchange and photoprotection in plants grown under fluctuating light.
Data availability statement
The raw data supporting the conclusions of this article will be made available by the authors, without undue reservation.
Author contributions
Y-JY and WH designed the study. HS, X-QW, and Z-LZ performed the photosynthetic measurements. HS, Y-JY, and WH performed the data analysis. WH wrote the first draft of the manuscript, which was extensively edited by all authors.
Funding
This work was supported by the National Natural Science Foundation of China (31971412 and 32171505), by Postdoctoral Research Funding Projects of Yunnan Province, and by Postdoctoral Directional Training Foundation of Yunnan Province.
Conflict of interest
The authors declare that the research was conducted in the absence of any commercial or financial relationships that could be construed as a potential conflict of interest.
Publisher’s note
All claims expressed in this article are solely those of the authors and do not necessarily represent those of their affiliated organizations, or those of the publisher, the editors and the reviewers. Any product that may be evaluated in this article, or claim that may be made by its manufacturer, is not guaranteed or endorsed by the publisher.
Supplementary material
The Supplementary Material for this article can be found online at: https://www.frontiersin.org/articles/10.3389/fpls.2022.917784/full#supplementary-material
Supplementary Figure 1 | Effects of exogenous melatonin (MT, 100 μM) on the kinetics of transpiration rate after transition from 50 to 1,500 μmol photons m–2 s–1. Values are means ± SE (n = 5). Asterisk indicates a significant difference between CK and MT-treated leaves.
References
Acevedo-Siaca, L. G., Coe, R., Wang, Y., Kromdijk, J., Quick, W. P., and Long, S. P. (2020). Variation in photosynthetic induction between rice accessions and its potential for improving productivity. New Phytol. 27, 1097–1108. doi: 10.1111/nph.16454
Adachi, S., Tanaka, Y., Miyagi, A., Kashima, M., Tezuka, A., Toya, Y., et al. (2019). High-yielding rice Takanari has superior photosynthetic response to a commercial rice Koshihikari under fluctuating light. J. Exp. Bot. 70, 5287–5297. doi: 10.1093/jxb/erz304
Ahammed, G. J., Wu, M., Wang, Y., Yan, Y., Mao, Q., Ren, J., et al. (2020). Melatonin alleviates iron stress by improving iron homeostasis, antioxidant defense and secondary metabolism in cucumber. Sci. Hortic. (Amsterdam) 265:109205. doi: 10.1016/j.scienta.2020.109205
Ahammed, G. J., Xu, W., Liu, A., and Chen, S. (2018). COMT1 silencing aggravates heat stress-induced reduction in photosynthesis by decreasing chlorophyll content, photosystem II activity, and electron transport efficiency in tomato. Front. Plant Sci. 9:998. doi: 10.3389/fpls.2018.00998
Alboresi, A., Storti, M., and Morosinotto, T. (2019). Balancing protection and efficiency in the regulation of photosynthetic electron transport across plant evolution. New Phytol. 221, 105–109. doi: 10.1111/nph.15372
Allahverdiyeva, Y., Suorsa, M., Tikkanen, M., and Aro, E. M. (2015). Photoprotection of photosystems in fluctuating light intensities. J. Exp. Bot. 66, 2427–2436. doi: 10.1093/jxb/eru463
Arnao, M. B., and Hernández-Ruiz, J. (2015). Functions of melatonin in plants: a review. J. Pineal Res. 59, 133–150. doi: 10.1111/jpi.12253
Arnao, M. B., and Hernández-Ruiz, J. (2019). Melatonin: a new plant hormone and/or a plant master regulator? Trends Plant Sci. 24, 38–48. doi: 10.1016/j.tplants.2018.10.010
Arnao, M., and Hernández-Ruiz, J. (2020). Is phytomelatonin a new plant hormone? Agronomy 10:95. doi: 10.3390/agronomy10010095
Asada, K. (1999). The water-water cycle in chloroplasts: scavenging of active oxygens and dissipation of excess photons. Annu. Rev. Plant Physiol. Plant Mol. Biol. 50, 601–639. doi: 10.1146/annurev.arplant.50.1.601
Asada, K. (2000). The water-water cycle as alternative photon and electron sinks. Philos. Trans. R. Soc. Lond. B. Biol. Sci. 355, 1419–1431. doi: 10.1098/rstb.2000.0703
Asada, K., Allen, J., Foyer, C. H., and Matthijs, H. C. P. (2000). The water-water cycle as alternative photon and electron sinks. Philos. Trans. R. Soc. B Biol. Sci. 355, 1419–1431.
Bajwa, V. S., Shukla, M. R., Sherif, S. M., Murch, S. J., and Saxena, P. K. (2014). Role of melatonin in alleviating cold stress in Arabidopsis thaliana. J. Pineal Res. 56, 238–245. doi: 10.1111/jpi.12115
Bhat, J. A., Faizan, M., Bhat, M. A., Huang, F., Yu, D., Ahmad, A., et al. (2022). Defense interplay of the zinc-oxide nanoparticles and melatonin in alleviating the arsenic stress in soybean (Glycine max L.). Chemosphere 288:132471. doi: 10.1016/j.chemosphere.2021.132471
Bose, S. K., and Howlader, P. (2020). Melatonin plays multifunctional role in horticultural crops against environmental stresses: a review. Environ. Exp. Bot. 176:104063. doi: 10.1016/j.envexpbot.2020.104063
Brestic, M., Zivcak, M., Kunderlikova, K., Sytar, O., Shao, H., Kalaji, H. M., et al. (2015). Low PSI content limits the photoprotection of PSI and PSII in early growth stages of chlorophyll b-deficient wheat mutant lines. Photosynth. Res. 125, 151–166. doi: 10.1007/s11120-015-0093-1
Cai, Y.-F., Yang, Q.-Y., Li, S.-F., Wang, J.-H., and Huang, W. (2017). The water-water cycle is a major electron sink in Camellia species when CO2 assimilation is restricted. J. Photochem. Photobiol. B Biol. 168, 59–66. doi: 10.1016/j.jphotobiol.2017.01.024
Carriquí, M., Cabrera, H. M., Conesa, M., Coopman, R. E., Douthe, C., Gago, J., et al. (2015). Diffusional limitations explain the lower photosynthetic capacity of ferns as compared with angiosperms in a common garden study. Plant Cell Environ. 38, 448–460. doi: 10.1111/pce.12402
Chen, Z., Cao, X., and Niu, J. (2021). Effects of melatonin on morphological characteristics, mineral nutrition, nitrogen metabolism, and energy status in alfalfa under high-nitrate stress. Front. Plant Sci. 12:694179. doi: 10.3389/fpls.2021.694179
Dai, L., Li, J., Harmens, H., Zheng, X., and Zhang, C. (2020). Melatonin enhances drought resistance by regulating leaf stomatal behaviour, root growth and catalase activity in two contrasting rapeseed (Brassica napus L.) genotypes. Plant Physiol. Biochem. 149, 86–95. doi: 10.1016/j.plaphy.2020.01.039
De Souza, A. P., Wang, Y., Orr, D. J., Carmo-Silva, E., and Long, S. P. (2020). Photosynthesis across African cassava germplasm is limited by Rubisco and mesophyll conductance at steady state, but by stomatal conductance in fluctuating light. New Phytol. 225, 2498–2512. doi: 10.1111/nph.16142
Ding, W., Zhao, Y., Xu, J.-W., Zhao, P., Li, T., Ma, H., et al. (2018). Melatonin: a multifunctional molecule that triggers defense responses against high light and nitrogen starvation stress in Haematococcus pluvialis. J. Agric. Food Chem. 66, 7701–7711. doi: 10.1021/acs.jafc.8b02178
Eyland, D., van Wesemael, J., Lawson, T., and Carpentier, S. (2021). The impact of slow stomatal kinetics on photosynthesis and water use efficiency under fluctuating light. Plant Physiol. 186, 998–1012. doi: 10.1093/PLPHYS/KIAB114
Farouk, S., and Al-Amri, S. M. (2019). Exogenous melatonin-mediated modulation of arsenic tolerance with improved accretion of secondary metabolite production, activating antioxidant capacity and improved chloroplast ultrastructure in rosemary herb. Ecotoxicol. Environ. Saf. 180, 333–347. doi: 10.1016/j.ecoenv.2019.05.021
Farquhar, G. D., von Caemmerer, S., and Berry, J. A. (1980). A biochemical model of photosynthetic CO2 assimilation in leaves of C3 species. Planta 149, 78–90. doi: 10.1007/BF00386231
Ferroni, L., Brestič, M., Živčak, M., Cantelli, R., and Pancaldi, S. (2021). Increased photosynthesis from a deep-shade to high-light regime occurs by enhanced CO2 diffusion into the leaf of Selaginella martensii. Plant Physiol. Biochem. 160, 143–154. doi: 10.1016/j.plaphy.2021.01.012
Genty, B., Briantais, J.-M., and Baker, N. R. (1989). The relationship between the quantum yield of photosynthetic electron transport and quenching of chlorophyll fluorescence. Biochim. Biophys. Acta Gen. Subj. 990, 87–92. doi: 10.1016/S0304-4165(89)80016-9
Gerotto, C., Alboresi, A., Meneghesso, A., Jokel, M., Suorsa, M., Aro, E.-M., et al. (2016). Flavodiiron proteins act as safety valve for electrons in Physcomitrella patens. Proc. Natl. Acad. Sci. U.S.A. 113, 12322–12327. doi: 10.1073/pnas.1606685113
Giraldo Acosta, M., Cano, A., Hernández-Ruiz, J., and Arnao, M. B. (2022). Melatonin as a Possible natural safener in crops. Plants 11:890. doi: 10.3390/plants11070890
Grassi, G., and Magnani, F. (2005). Stomatal, mesophyll conductance and biochemical limitations to photosynthesis as affected by drought and leaf ontogeny in ash and oak trees. Plant Cell Environ. 28, 834–849. doi: 10.1111/j.1365-3040.2005.01333.x
Harley, P. C., Loreto, F., Di Marco, G., and Sharkey, T. D. (1992). Theoretical considerations when estimating the mesophyll conductance to CO2 flux by analysis of the response of photosynthesis to CO2. Plant Physiol. 98, 1429–1436. doi: 10.1104/pp.98.4.1429
Hermida-Carrera, C., Kapralov, M. V., and Galmés, J. (2016). Rubisco Catalytic properties and temperature response in crops. Plant Physiol. 171, 2549–2561. doi: 10.1104/pp.16.01846
Hirotsu, N., Makino, A., Ushio, A., and Mae, T. (2004). Changes in the thermal dissipation and the electron flow in the water-water cycle in rice grown under conditions of physiologically low temperature. Plant Cell Physiol. 45, 635–644. doi: 10.1093/pcp/pch075
Hirotsu, N., Makino, A., Yokota, S., and Mae, T. (2005). The photosynthetic properties of rice leaves treated with low temperature and high irradiance. Plant Cell Physiol. 46, 1377–1383. doi: 10.1093/pcp/pci149
Hoque, M. N., Tahjib-Ul-Arif, M., Hannan, A., Sultana, N., Akhter, S., Hasanuzzaman, M., et al. (2021). Melatonin modulates plant tolerance to heavy metal stress: morphological responses to molecular mechanisms. Int. J. Mol. Sci. 22, 1–24. doi: 10.3390/ijms222111445
Huang, W., Yang, Y.-J., and Zhang, S.-B. (2019a). Photoinhibition of photosystem I under fluctuating light is linked to the insufficient ΔpH upon a sudden transition from low to high light. Environ. Exp. Bot. 160, 112–119. doi: 10.1016/j.envexpbot.2019.01.012
Huang, W., Yang, Y. J., and Zhang, S. B. (2019b). The role of water-water cycle in regulating the redox state of photosystem I under fluctuating light. Biochim. Biophys. Acta Bioenerg. 1860, 383–390. doi: 10.1016/j.bbabio.2019.03.007
Huang, W., Yang, Y.-J., Hu, H., and Zhang, S.-B. (2016). Response of the water–water cycle to the change in photorespiration in tobacco. J. Photochem. Photobiol. B Biol. 157, 97–104. doi: 10.1016/j.jphotobiol.2016.02.006
Huang, W., Zhang, S.-B., and Liu, T. (2018). Moderate photoinhibition of photosystem II significantly affects linear electron flow in the shade-demanding plant Panax notoginseng. Front. Plant Sci. 9:637. doi: 10.3389/fpls.2018.00637
Imran, M., Shazad, R., Bilal, S., Imran, Q. M., Khan, M., Kang, S. M., et al. (2021). Exogenous Melatonin mediates the regulation of endogenous nitric oxide in Glycine max L. to reduce effects of drought stress. Environ. Exp. Bot. 188:104511. doi: 10.1016/j.envexpbot.2021.104511
Jahan, M. S., Guo, S., Baloch, A. R., Sun, J., Shu, S., Wang, Y., et al. (2020). Melatonin alleviates nickel phytotoxicity by improving photosynthesis, secondary metabolism and oxidative stress tolerance in tomato seedlings. Ecotoxicol. Environ. Saf. 197:110593. doi: 10.1016/j.ecoenv.2020.110593
Jahan, M. S., Shu, S., Wang, Y., Chen, Z., He, M., Tao, M., et al. (2019). Melatonin alleviates heat-induced damage of tomato seedlings by balancing redox homeostasis and modulating polyamine and nitric oxide biosynthesis. BMC Plant Biol. 19:414. doi: 10.1186/s12870-019-1992-7
Jokel, M., Johnson, X., Peltier, G., Aro, E. M., and Allahverdiyeva, Y. (2018). Hunting the main player enabling Chlamydomonas reinhardtii growth under fluctuating light. Plant J. 94, 822–835. doi: 10.1111/tpj.13897
Kaiser, E., Kromdijk, J., Harbinson, J., Heuvelink, E., and Marcelis, L. F. M. (2017). Photosynthetic induction and its diffusional, carboxylation and electron transport processes as affected by CO2 partial pressure, temperature, air humidity and blue irradiance. Ann. Bot. 119, 191–205. doi: 10.1093/aob/mcw226
Kaiser, E., Morales, A., Harbinson, J., Heuvelink, E., and Marcelis, L. F. M. (2020). High stomatal conductance in the tomato flacca mutant allows for faster photosynthetic induction. Front. Plant Sci. 11:1317. doi: 10.3389/fpls.2020.01317
Kaya, C., Okant, M., Ugurlar, F., Alyemeni, M. N., Ashraf, M., and Ahmad, P. (2019). Melatonin-mediated nitric oxide improves tolerance to cadmium toxicity by reducing oxidative stress in wheat plants. Chemosphere 225, 627–638. doi: 10.1016/j.chemosphere.2019.03.026
Kaya, C., Sarıoglu, A., Ashraf, M., Alyemeni, M. N., and Ahmad, P. (2022). The combined supplementation of melatonin and salicylic acid effectively detoxifies arsenic toxicity by modulating phytochelatins and nitrogen metabolism in pepper plants. Environ. Pollut. 297:118727. doi: 10.1016/j.envpol.2021.118727
Kimura, H., Hashimoto-Sugimoto, M., Iba, K., Terashima, I., and Yamori, W. (2020). Improved stomatal opening enhances photosynthetic rate and biomass production in fluctuating light. J. Exp. Bot. 71, 2339–2350. doi: 10.1093/jxb/eraa090
Kono, M., Noguchi, K., and Terashima, I. (2014). Roles of the cyclic electron flow around PSI (CEF-PSI) and O2-dependent alternative pathways in regulation of the photosynthetic electron flow in short-term fluctuating light in Arabidopsis thaliana. Plant Cell Physiol. 55, 990–1004. doi: 10.1093/pcp/pcu033
Lee, H. Y., and Back, K. (2018). Melatonin induction and its role in high light stress tolerance in Arabidopsis thaliana. J. Pineal Res. 65:e12504. doi: 10.1111/jpi.12504
Li, S., Guo, J., Wang, T., Gong, L., Liu, F., Brestic, M., et al. (2021). Melatonin reduces nanoplastic uptake, translocation, and toxicity in wheat. J. Pineal Res. 71:e12761. doi: 10.1111/jpi.12761
Li, T. Y., Shi, Q., Sun, H., Yue, M., Zhang, S.-B., and Huang, W. (2021). diurnal response of photosystem i to fluctuating light is affected by stomatal conductance. Cells 10:3128. doi: 10.3390/cells10113128
Li, X., Ahammed, G. J., Zhang, X.-N., Zhang, L., Yan, P., Zhang, L.-P., et al. (2021). Melatonin-mediated regulation of anthocyanin biosynthesis and antioxidant defense confer tolerance to arsenic stress in Camellia sinensis L. J. Hazard. Mater. 403:123922. doi: 10.1016/j.jhazmat.2020.123922
Li, X., Brestic, M., Tan, D.-X., Zivcak, M., Zhu, X., Liu, S., et al. (2018). Melatonin alleviates low PS I-limited carbon assimilation under elevated CO 2 and enhances the cold tolerance of offspring in chlorophyll b -deficient mutant wheat. J. Pineal Res. 64:e12453. doi: 10.1111/jpi.12453
Liang, C., Zheng, G., Li, W., Wang, Y., Hu, B., Wang, H., et al. (2015). Melatonin delays leaf senescence and enhances salt stress tolerance in rice. J. Pineal Res. 59, 91–101. doi: 10.1111/jpi.12243
Lima-Melo, Y., Gollan, P. J., Tikkanen, M., Silveira, J. A. G., and Aro, E. M. (2019). Consequences of photosystem-I damage and repair on photosynthesis and carbon use in Arabidopsis thaliana. Plant J. 97, 1061–1072. doi: 10.1111/tpj.14177
Liu, T., Barbour, M. M., Yu, D., Rao, S., and Song, X. (2022). Mesophyll conductance exerts a significant limitation on photosynthesis during light induction. New Phytol. 233, 360–372. doi: 10.1111/nph.17757
Long, S. P., and Bernacchi, C. J. (2003). Gas exchange measurements, what can they tell us about the underlying limitations to photosynthesis? Procedures and sources of error. J. Exp. Bot. 54, 2393–2401. doi: 10.1093/jxb/erg262
Makino, A., Miyake, C., and Yokota, A. (2002). Physiological functions of the water-water cycle (Mehler reaction) and the cyclic electron flow around PSI in rice leaves. Plant Cell Physiol. 43, 1017–1026. doi: 10.1093/pcp/pcf124
Meng, S., Wang, X., Bian, Z., Li, Z., Yang, F., Wang, S., et al. (2021). Melatonin enhances nitrogen metabolism and haustorium development in hemiparasite Santalum album Linn. Environ. Exp. Bot. 186:104460. doi: 10.1016/j.envexpbot.2021.104460
Miyake, C. (2010). Alternative electron flows (water-water cycle and cyclic electron flow around PSI) in photosynthesis: molecular mechanisms and physiological functions. Plant Cell Physiol. 51, 1951–1963. doi: 10.1093/pcp/pcq173
Munekage, Y. N., Genty, B., and Peltier, G. (2008). Effect of PGR5 impairment on photosynthesis and growth in Arabidopsis thaliana. Plant Cell Physiol. 49, 1688–1698. doi: 10.1093/pcp/pcn140
Munekage, Y., Hojo, M., Meurer, J., Endo, T., Tasaka, M., and Shikanai, T. (2002). PGR5 is involved in cyclic electron flow around photosystem I and is essential for photoprotection in Arabidopsis. Cell 110, 361–371. doi: 10.1016/S0092-8674(02)00867-X
Murata, N., Takahashi, S., Nishiyama, Y., and Allakhverdiev, S. I. (2007). Photoinhibition of photosystem II under environmental stress. Biochim. Biophys. Acta Bioenerg. 1767, 414–421. doi: 10.1016/j.bbabio.2006.11.019
Nishiyama, Y., Allakhverdiev, S. I., and Murata, N. (2005). Inhibition of the repair of photosystem II by oxidative stress in cyanobacteria. Photosynth. Res. 84, 1–7. doi: 10.1007/s11120-004-6434-0
Nishiyama, Y., Yamamoto, H., Allakhverdiev, S. I., Inaba, M., Yokota, A., and Murata, N. (2001). Oxidative stress inhibits the repair of photodamage to the photosynthetic machinery. EMBO J. 20, 5587–5594. doi: 10.1093/emboj/20.20.5587
Oguchi, R., Hikosaka, K., and Hirose, T. (2003). Does the photosynthetic light-acclimation need change in leaf anatomy? Plant Cell Environ. 26, 505–512. doi: 10.1046/j.1365-3040.2003.00981.x
Park, S., Lee, D.-E., Jang, H., Byeon, Y., Kim, Y.-S., and Back, K. (2013). Melatonin-rich transgenic rice plants exhibit resistance to herbicide-induced oxidative stress. J. Pineal Res. 54, 258–263. doi: 10.1111/j.1600-079X.2012.01029.x
Pearcy, R. W. (1990). Sunflecks and photosynthesis in plant canopies. Annu. Rev. Plant Physiol. Plant Mol. Biol. 41, 421–453. doi: 10.1146/annurev.pp.41.060190.002225
Qi, C., Zhang, H., Liu, Y., Wang, X., Dong, D., Yuan, X., et al. (2020). CsSNAT positively regulates salt tolerance and growth of cucumber by promoting melatonin biosynthesis. Environ. Exp. Bot. 175:104036. doi: 10.1016/j.envexpbot.2020.104036
Qiao, M.-Y., Zhang, Y.-J., Liu, L.-A., Shi, L., Ma, Q.-H., Chow, W. S., et al. (2020). Do rapid photosynthetic responses protect maize leaves against photoinhibition under fluctuating light? Photosynth. Res. 149, 57–68. doi: 10.1007/s11120-020-00780-5
Qiao, Y., Yin, L., Wang, B., Ke, Q., Deng, X., and Wang, S. (2019). Melatonin promotes plant growth by increasing nitrogen uptake and assimilation under nitrogen deficient condition in winter wheat. Plant Physiol. Biochem. 139, 342–349. doi: 10.1016/j.plaphy.2019.03.037
Sakoda, K., Yamori, W., Groszmann, M., and Evans, J. R. (2021). Stomatal, mesophyll conductance, and biochemical limitations to photosynthesis during induction. Plant Physiol. 185, 146–160. doi: 10.1093/plphys/kiaa011
Sakoda, K., Yamori, W., Shimada, T., Sugano, S. S., Hara-Nishimura, I., and Tanaka, Y. (2020). Higher stomatal density improves photosynthetic induction and biomass production in Arabidopsis under fluctuating light. Front. Plant Sci. 11:1308. doi: 10.3389/fpls.2020.589603
Sejima, T., Takagi, D., Fukayama, H., Makino, A., and Miyake, C. (2014). Repetitive short-pulse light mainly inactivates photosystem i in sunflower leaves. Plant Cell Physiol. 55, 1184–1193. doi: 10.1093/pcp/pcu061
Seleiman, M. F., Ali, S., Refay, Y., Rizwan, M., Alhammad, B. A., and El-Hendawy, S. E. (2020). Chromium resistant microbes and melatonin reduced Cr uptake and toxicity, improved physio-biochemical traits and yield of wheat in contaminated soil. Chemosphere 250:126239. doi: 10.1016/j.chemosphere.2020.126239
Sharma, A., and Zheng, B. (2019). Melatonin mediated regulation of drought stress: physiological and molecular aspects. Plants 8:190. doi: 10.3390/plants8070190
Shikanai, T., and Yamamoto, H. (2017). Contribution of cyclic and pseudo-cyclic electron transport to the formation of proton motive force in chloroplasts. Mol. Plant 10, 20–29. doi: 10.1016/j.molp.2016.08.004
Shimakawa, G., and Miyake, C. (2019). What quantity of photosystem I is optimum for safe photosynthesis? Plant Physiol. 179, 1479–1485. doi: 10.1104/pp.18.01493
Siddiqui, M. H., Alamri, S., Alsubaie, Q. D., and Ali, H. M. (2020a). Melatonin and gibberellic acid promote growth and chlorophyll biosynthesis by regulating antioxidant and methylglyoxal detoxification system in tomato seedlings under salinity. J. Plant Growth Regul. 39, 1488–1502. doi: 10.1007/s00344-020-10122-3
Siddiqui, M. H., Alamri, S., Nasir Khan, M., Corpas, F. J., Al-Amri, A. A., Alsubaie, Q. D., et al. (2020b). Melatonin and calcium function synergistically to promote the resilience through ROS metabolism under arsenic-induced stress. J. Hazard. Mater. 398:122882. doi: 10.1016/j.jhazmat.2020.122882
Slattery, R. A., Walker, B. J., Weber, A. P. M., and Ort, D. R. (2018). The impacts of fluctuating light on crop performance. Plant Physiol. 176, 990–1003. doi: 10.1104/pp.17.01234
Storti, M., Alboresi, A., Gerotto, C., Aro, E., Finazzi, G., and Morosinotto, T. (2019). Role of cyclic and pseudo-cyclic electron transport in response to dynamic light changes in Physcomitrella patens. Plant. Cell Environ. 42, 1590–1602. doi: 10.1111/pce.13493
Storti, M., Segalla, A., Mellon, M., Alboresi, A., and Morosinotto, T. (2020). Regulation of electron transport is essential for photosystem I stability and plant growth. New Phytol. 228, 1316–1326. doi: 10.1111/nph.16643
Sun, H., Shi, Q., Zhang, S.-B., and Huang, W. (2021). Coordination of cyclic electron flow and water–water Cycle facilitates photoprotection under fluctuating light and temperature stress in the epiphytic orchid Dendrobium officinale. Plants 10:606. doi: 10.3390/plants10030606
Sun, H., Zhang, S.-B., Liu, T., and Huang, W. (2020b). Decreased photosystem II activity facilitates acclimation to fluctuating light in the understory plant Paris polyphylla. Biochim. Biophys. Acta Bioenerg. 1861:148135. doi: 10.1016/j.bbabio.2019.148135
Sun, H., Yang, Y.-J., and Huang, W. (2020a). The water-water cycle is more effective in regulating redox state of photosystem I under fluctuating light than cyclic electron transport. Biochim. Biophys. Acta Bioenerg. 1861:148235. doi: 10.1016/j.bbabio.2020.148235
Sun, H., Zhang, Y.-Q., Zhang, S., and Huang, W. (2022). Photosynthetic induction under fluctuating light is affected by leaf nitrogen content in tomato. Front. Plant Sci. 13:835571. doi: 10.3389/fpls.2022.835571
Suorsa, M., Jarvi, S., Grieco, M., Nurmi, M., Pietrzykowska, M., Rantala, M., et al. (2012). PROTON GRADIENT REGULATION5 is essential for proper acclimation of Arabidopsis photosystem I to naturally and artificially fluctuating light conditions. Plant Cell 24, 2934–2948. doi: 10.1105/tpc.112.097162
Takahashi, S., and Badger, M. R. (2011). Photoprotection in plants: a new light on photosystem II damage. Trends Plant Sci. 16, 53–60. doi: 10.1016/j.tplants.2010.10.001
Takahashi, S., and Murata, N. (2005). Interruption of the Calvin cycle inhibits the repair of photosystem II from photodamage. Biochim. Biophys. Acta Bioenerg. 1708, 352–361. doi: 10.1016/j.bbabio.2005.04.003
Takahashi, S., and Murata, N. (2006). Glycerate-3-phosphate, produced by CO2 fixation in the Calvin cycle, is critical for the synthesis of the D1 protein of photosystem II. Biochim. Biophys. Acta Bioenerg. 1757, 198–205. doi: 10.1016/j.bbabio.2006.02.002
Takahashi, S., and Murata, N. (2008). How do environmental stresses accelerate photoinhibition? Trends Plant Sci. 13, 178–182. doi: 10.1016/j.tplants.2008.01.005
Takahashi, S., Milward, S. E., Fan, D.-Y., Chow, W. S., and Badger, M. R. (2009). How does cyclic electron flow alleviate photoinhibition in Arabidopsis? Plant Physiol. 149, 1560–1567. doi: 10.1104/pp.108.134122
Tan, S.-L., Huang, J.-L., Zhang, F.-P., Zhang, S.-B., and Huang, W. (2021). Photosystem I photoinhibition induced by fluctuating light depends on background low light irradiance. Environ. Exp. Bot. 181:104298. doi: 10.1016/j.envexpbot.2020.104298
Valentini, R., Epron, D., Angelis, P. D. E., Matteucci, G., and Dreyer, E. (1995). In situ estimation of net CO2 assimilation, photosynthetic electron flow and photorespiration in Turkey oak (Q. cerris L.) leaves: diurnal cycles under different levels of water supply. Plant Cell Environ. 18, 631–640. doi: 10.1111/j.1365-3040.1995.tb00564.x
Valladares, F., Allen, M. T., and Pearcy, R. W. (1997). Photosynthetic responses to dynamic light under field conditions in six tropical rainforest shrubs occurring along a light gradient. Oecologia 111, 505–514. doi: 10.1007/s004420050264
von Caemmerer, S., and Evans, J. R. (2015). Temperature responses of mesophyll conductance differ greatly between species. Plant Cell Environ. 38, 629–637. doi: 10.1111/pce.12449
Walker, B. J., Strand, D. D., Kramer, D. M., and Cousins, A. B. (2014). The response of cyclic electron flow around photosystem I to changes in photorespiration and nitrate assimilation. Plant Physiol. 165, 453–462. doi: 10.1104/pp.114.238238
Wang, H., Wang, X.-Q., Zeng, Z.-L., Yu, H., and Huang, W. (2022). Photosynthesis under fluctuating light in the CAM plant Vanilla planifolia. Plant Sci. 317:111207. doi: 10.1016/j.plantsci.2022.111207
Wang, Y., Reiter, R. J., and Chan, Z. (2018). Phytomelatonin: a universal abiotic stress regulator. J. Exp. Bot. 69, 963–974. doi: 10.1093/jxb/erx473
Warren, C. R., and Dreyer, E. (2006). Temperature response of photosynthesis and internal conductance to CO2: results from two independent approaches. J. Exp. Bot. 57, 3057–3067. doi: 10.1093/jxb/erl067
Xiong, D., Douthe, C., and Flexas, J. (2018). Differential coordination of stomatal conductance, mesophyll conductance, and leaf hydraulic conductance in response to changing light across species. Plant Cell Environ. 41, 436–450. doi: 10.1111/pce.13111
Xiong, D., Liu, X., Liu, L., Douthe, C., Li, Y., Peng, S., et al. (2015). Rapid responses of mesophyll conductance to changes of CO2 concentration, temperature and irradiance are affected by N supplements in rice. Plant Cell Environ. 38, 2541–2550. doi: 10.1111/pce.12558
Yamamoto, H., and Shikanai, T. (2019). PGR5-dependent cyclic electron flow protects photosystem I under fluctuating light at donor and acceptor sides. Plant Physiol. 179, 588–600. doi: 10.1104/pp.18.01343
Yamamoto, H., Takahashi, S., Badger, M. R., and Shikanai, T. (2016). Artificial remodelling of alternative electron flow by flavodiiron proteins in Arabidopsis. Nat. Plants 2:16012. doi: 10.1038/nplants.2016.12
Yamori, W., Kusumi, K., Iba, K., and Terashima, I. (2020). Increased stomatal conductance induces rapid changes to photosynthetic rate in response to naturally fluctuating light conditions in rice. Plant. Cell Environ. 43, 1230–1240. doi: 10.1111/pce.13725
Yang, Y.-J., Tan, S.-L., Huang, J.-L., Zhang, S.-B., and Huang, W. (2020). The water-water cycle facilitates photosynthetic regulation under fluctuating light in the epiphytic orchid Dendrobium officinale. Environ. Exp. Bot. 180:104238. doi: 10.1016/j.envexpbot.2020.104238
Yang, Y.-J., Tan, S.-L., Sun, H., Huang, J.-L., Huang, W., and Zhang, S.-B. (2021). Photosystem I is tolerant to fluctuating light under moderate heat stress in two orchids Dendrobium officinale and Bletilla striata. Plant Sci. 303:110795. doi: 10.1016/j.plantsci.2020.110795
Yang, Y.-J., Zhang, S.-B., and Huang, W. (2019). Photosynthetic regulation under fluctuating light in young and mature leaves of the CAM plant Bryophyllum pinnatum. Biochim. Biophys. Acta Bioenerg. 1860, 469–477. doi: 10.1016/j.bbabio.2019.04.006
Yao, J., Ma, Z., Ma, Y., Zhu, Y., Lei, M., Hao, C., et al. (2021). Role of melatonin in UV-B signaling pathway and UV-B stress resistance in <scp> Arabidopsis thaliana </scp>. Plant. Cell Environ. 44, 114–129. doi: 10.1111/pce.13879
Yi, X. P., Zhang, Y. L., Yao, H. S., Zhang, X. J., Luo, H. H., Gou, L., et al. (2014). Alternative electron sinks are crucial for conferring photoprotection in field-grown cotton under water deficit during flowering and boll setting stages. Funct. Plant Biol. 41, 737–747. doi: 10.1071/FP13269
Zhang, H., Liu, L., Wang, Z., Feng, G., Gao, Q., and Li, X. (2021). Induction of low temperature tolerance in wheat by pre-soaking and parental treatment with melatonin. Molecules 26:1192. doi: 10.3390/molecules26041192
Zhang, Y., Kaiser, E., Marcelis, L. F. M., Yang, Q., and Li, T. (2020). Salt stress and fluctuating light have separate effects on photosynthetic acclimation, but interactively affect biomass. Plant. Cell Environ. 43, 2192–2206. doi: 10.1111/pce.13810
Zivcak, M., Brestic, M., Balatova, Z., Drevenakova, P., Olsovska, K., Kalaji, H. M., et al. (2013). Photosynthetic electron transport and specific photoprotective responses in wheat leaves under drought stress. Photosynth. Res. 117, 529–546. doi: 10.1007/s11120-013-9885-3
Keywords: melatonin, photosynthesis, fluctuating light, stomatal conductance, mesophyll conductance, photoprotection
Citation: Sun H, Wang X-Q, Zeng Z-L, Yang Y-J and Huang W (2022) Exogenous melatonin strongly affects dynamic photosynthesis and enhances water-water cycle in tobacco. Front. Plant Sci. 13:917784. doi: 10.3389/fpls.2022.917784
Received: 11 April 2022; Accepted: 11 July 2022;
Published: 03 August 2022.
Edited by:
Marian Brestic, Slovak University of Agriculture, SlovakiaReviewed by:
Stefan Timm, University of Rostock, GermanyXiangnan Li, Northeast Institute of Geography and Agroecology (CAS), China
Copyright © 2022 Sun, Wang, Zeng, Yang and Huang. This is an open-access article distributed under the terms of the Creative Commons Attribution License (CC BY). The use, distribution or reproduction in other forums is permitted, provided the original author(s) and the copyright owner(s) are credited and that the original publication in this journal is cited, in accordance with accepted academic practice. No use, distribution or reproduction is permitted which does not comply with these terms.
*Correspondence: Ying-Jie Yang, eWFuZ3lpbmdqaWVAbWFpbC5raWIuYWMuY24=; Wei Huang, aHVhbmd3ZWlAbWFpbC5raWIuYWMuY24=