- 1Institute of Environment and Sustainable Development in Agriculture, Chinese Academy of Agricultural Sciences, Beijing, China
- 2National Institute for Genomics and Advanced Biotechnology, Islamabad, Pakistan
- 3Institute of Soil Science, Pir Mehr Ali Shah-Arid Agriculture University, Rawalpindi, Pakistan
- 4Department of Plant Breeding and Genetics, The University of Haripur, Haripur, Pakistan
- 5Pakistan Agricultural Research Council, Islamabad, Pakistan
- 6Department of Biotechnology, Chonnam National University, Yeosu, South Korea
The abundance and structural composition of nitrogen (N) transformation-related microbial communities under certain environmental conditions provide sufficient information about N cycle under different soil conditions. This study aims to explore the major challenge of low N use efficiency (NUE) and N dynamics in aerobic rice systems and reveal the agronomic-adjustive measures to increase NUE through insights into the ecophysiology of ammonia oxidizers. Water-saving practices, like alternate wetting and drying (AWD), dry direct seeded rice (DDSR), wet direct seeding, and saturated soil culture (SSC), have been evaluated in lowland rice; however, only few studies have been conducted on N dynamics in aerobic rice systems. Biological ammonia oxidation is majorly conducted by two types of microorganisms, ammonia-oxidizing archaea (AOA) and ammonia-oxidizing bacteria (AOB). This review focuses on how diversified are ammonia oxidizers (AOA and AOB), whose factors affect their activities and abundance under different soil conditions. It summarizes findings on pathways of N cycle, rationalize recent research on ammonia oxidizers in N-cycle, and thereby suggests adjustive agronomic measures to reduce N losses. This review also suggests that variations in soil properties significantly impact the structural composition and abundance of ammonia oxidizers. Nitrification inhibitors (NIs) especially nitrapyrin, reduce the nitrification rate and inhibit the abundance of bacterial amoA without impacting archaeal amoA. In contrast, some NIs confine the hydrolysis of synthetic N and, therefore, keep low NH4+-N concentrations that exhibit no or very slight impact on ammonia oxidizers. Variations in soil properties are more influential in the community structure and abundance of ammonia oxidizers than application of synthetic N fertilizers and NIs. Biological nitrification inhibitors (BNIs) are natural bioactive compounds released from roots of certain plant species, such as sorghum, and could be commercialized to suppress the capacity of nitrifying soil microbes. Mixed application of synthetic and organic N fertilizers enhances NUE and plant N-uptake by reducing ammonia N losses. High salt concentration promotes community abundance while limiting the diversity of AOB and vice versa for AOA, whereas AOA have lower rate for potential nitrification than AOB, and denitrification accounts for higher N2 production. Archaeal abundance, diversity, and structural composition change along an elevation gradient and mainly depend on various soil factors, such as soil saturation, availability of NH4+, and organic matter contents. Microbial abundance and structural analyses revealed that the structural composition of AOA was not highly responsive to changes in soil conditions or N amendment. Further studies are suggested to cultivate AOA and AOB in controlled-environment experiments to understand the mechanisms of AOA and AOB under different conditions. Together, this evaluation will better facilitate the projections and interpretations of ammonia oxidizer community structural composition with provision of a strong basis to establish robust testable hypotheses on the competitiveness between AOB and AOA. Moreover, after this evaluation, managing soils agronomically for potential utilization of metabolic functions of ammonia oxidizers would be easier.
Introduction
Human-based activities and climate change have fiercely shifted the global nitrogen (N)-cycle with increased amount of reactive N in the biosphere. Shifts in N cycle have reasonably resulted from interactions of N pools with crops and soil under changed climatic conditions, which are subjected to global warming and extreme stress events (Fowler et al., 2013). Enhanced use of synthetic and industrially manufactured N fertilizers in terrestrial habitats, and N2 fixation due to interactions of symbiotic microorganisms and plants have exceeded the natural N-inputs added to anthropic N inputs (Fowler et al., 2013; Zhang et al., 2019a,b). The N use efficiency (NUE) of synthetic N fertilizers in agriculture remain fairly low; ordinarily, 50% or even less of the N fertilizer applied is taken up by plants (Millar et al., 2014). Conventional rice systems need several transformational approaches that reduce water consumption to balance the economic, environmental, and production status under future threats of scarce availability of freshwater resources. Transformational modifications (aerobic rice systems) in conventional rice sustain yield but at the cost of higher N loss. Therefore, comprehensive understanding and comprehension of recent advances in the N cycling of aerobic rice systems under irrigated conditions is necessary to intensify a sustainable rice yield while reducing N loss and environmental hazards (Awan et al., 2014; Javed et al., 2021; Uzair et al., 2022). The ecophysiology, abundance, and activities of soil microbes involved in N cycling processes change under different crop production systems. Therefore, explication of diversity and functions of soil microbes that are dominant in N cycling require a comprehensive understanding of the ecophysiology of key ammonia-oxidizing microorganisms and thereby suggest agronomic adjustive measures to enhance NUE (Wang et al., 2019). Classification and evaluation of essential considerations like N-fertilizer management (Snyder, 2016) especially for nitrification (explanation in later parts) may enhance NUE while reducing greenhouse gas (GHG) emission and N loss and the transport of reactive N, avoiding environmental pollution (Shiozaki et al., 2016; Smith et al., 2016). Ammonia-oxidizing bacteria (AOB) and ammonia-oxidizing archaea (AOA) are among the dominant microbes playing crucial roles in N cycle (Sauder et al., 2017; Wang H. et al., 2017). The current knowledge of the ecophysiology of vital ammonia-oxidizing microbes has been primarily based on pure isolates, but studies under natural conditions are rare (Tourna et al., 2011). Therefore, the present study is aimed to provide a deep insight into the ecophysiology of major soil ammonia oxidizers (AOA and AOB); understanding the major pathways of ammonia oxidation and mechanisms of how active ammonia oxidizers interactively impacts N cycling and plant N-uptake, thereby recommending adjustive measures to limit the N-losses.
The first part of this article reviews the importance of bringing transformations (aerobic rice systems) in conventional rice systems and how N-cycle occurs under aerobic rice systems with emphasis on the importance of reducing N loss. Then, it moves toward elaboration of important reactions in N-cycle viz. ammonification, nitrification, denitrification, and volatilization and leaching. Later, it expands the understanding toward the ecophysiology, abundance, diversification, and activities of microbial communities in aerobic rice systems and how their growth and activities are affected by various environmental factors like soil temperature, pH, NH4+ availability, soil dissolved oxygen, and moisture content. The later parts of the article review the pathways of action of AOA and AOB, and thereby recommend several soil, crop, and fertilizer adjustive management strategies to optimize the abundance and activities of ammonia oxidizers.
Climate Change and Rice Production
Concrete bodies of evidence have revealed that earth mean surface temperature has been warming since the 19th century. Based on the temperature change evidence observed over the last century, it has been observed that global average temperature has increased by 1.09°C since the middle of the 19th century according to the IPCC AR6 WGI report, and that most of the temperature change was noticed in the last three decades of the 19th century (IPCC, 2015). Climate change occurs because of three major factors: natural factors, human-induced factors, such as GHGs and methane (CH4) emission, and land use shifts. Human-induced factors enhanced the levels of atmospheric carbon dioxide (CO2) from 284 ppm in 1832 to 410 ppm in 2013 (Hoffman et al., 2014), which ultimately increased the temperature and caused global warming. It is likely that climate change will produce severe changes in temperature, precipitation frequency and patterns, and extreme stress events. Undergoing this projection, it is anticipated that global warming patterns will not be even across the globe, and that arid and oceanic parts will be under more threats (Solomon et al., 2007). Meanwhile, it has also been revealed that average earth temperature will rise more slowly than projected with climatic models because of higher absorption of CO2 by oceans (Balmaseda et al., 2013). Moreover, due to unevenness and irregular climatic changes, there are uncertainties regarding precipitation patterns, intensity, and frequency, specifically over tropical regions, due the inefficacy of models to demonstrate an accurate hydrological cycle (Lorenz et al., 2012).
Climate change has threatened global rice production especially in major rice-cultivating Asian countries. There is an important linkage between rice production and climate change as the former contributes to global climate warming because of higher rate of CH4 and other GHG emission, while rice systems are getting impacted by climate change. Rice production is required to be increased by 40% by the end of 2030 because of scarcity of resources to meet the world’s growing population staple food demands (FAO, 2016), which may exacerbate the problem of environmental hazards because of increased CH4 and GHG emissions. Globally, China and India are the most densely populated countries sharing 20 and 28.5% of total global rice area, respectively (Hwalla et al., 2016) and, therefore, have a large share in CH4 emission. Comparing with the area division, 90 and 46% of rice production in China and India, respective, is irrigated (Jain et al., 2000; Pathak et al., 2005). Rice, during flooding conditions, acts as one of the main sources of CH4 (Oelbermann, 2014). Various transformed rice production practices, including aerobic rice systems, have been introduced, are resource-use efficient, and can sustain grain yield but may create environmental hazards (N oxide emission) because of higher N loss although reduce the emission of CH4.
The emission rate of GHGs from rice soil is greatly dependent on soil organic matter (OM) status, land use changes, cropping intensity, irrigation management practices, soil microbial abundance and their functioning, soil properties, and environmental variables. Normally, GHG emission, especially CH4, is higher in flooded rice systems. However, the transformation of conventional rice systems to aerobic rice systems may fulfill future food demands by sustaining grain yield under scarcity of inputs but at the cost of higher N loss, such as nitrous oxide (N2O), through coupled nitrification-denitrification processes under an alternate wetting and drying (AWD) irrigation system (Huang and Gerber, 2015). Therefore, understanding the shifts in microbial abundance and activities and pathways of N-cycle under aerobic rice systems is necessary to reduce N loss undergoing several agronomic management measures enhancing NUE, thereby reducing environmental hazards.
Need for Transformation of Conventional Rice Systems to Aerobic Rice Systems
Scarcity of fresh water resources is bringing changes in irrigated rice systems, such as to switch toward a water-use efficient direct-seeded rice system accompanying AWD irrigation management (Joshi et al., 2013). This necessarily requires the identification of the most suitable cultivars with high yield and resource use efficiency features under different nutrient and water management regimes (Nie et al., 2012; Kumar et al., 2022). More than 75% of global rice comes from 79 million ha of irrigated rice areas across the globe, and it has been predicted that 17 million ha of irrigated rice in Asia will experience “physical water scarcity” and that 22 million ha will be subjected to “economic water scarcity” (Tuong and Bouman, 2003). By the end of 2050s, agricultural output must increase by 70% to feed over nine billion people mostly living in urban areas across the globe (FAO, 2017). Considering this challenge, the required increase in agricultural output has to be achieved with diminishing resources of water, land, and energy. Scarcity of resources has driven the focus on improving the general eco-efficiencies of agricultural systems. Eco-efficiency is about achieving more with less, i.e., more agricultural outputs per unit application of inputs, both in terms of quantity and quality (Keating et al., 2010). The overall aim of this concept is to increase the productivity of low-input systems (rainfed upland rice) and the sustainability of high-input systems (irrigated lowland rice) through transformational technologies (aerobic rice systems) (Bouman et al., 2005, 2006). The aerobic rice system is a new resource-use efficient, initiated by the International Rice Research Institute (IRRI) and is an alternative to the conventional rice system for high production under non-flooded, non-puddled, and unsaturated soil conditions. The aerobic rice system is considered as highly receptive to nutrient availability, can be rainfed or irrigated, and occasionally tolerates flooding (Bouman and Tuong, 2001), and this system has been successfully adopted in countries like China, Brazil, and many other Asian rice-producing countries (Kato and Katsura, 2014). Therefore, it can be an alternative to the conventional rice system with typically high resource-use efficiencies and profitability for farmers (Kadiyala et al., 2012; Djaman et al., 2018).
N Use Dynamics Under Aerobic Rice Systems
Currently, global rice systems are suffering from higher N loss next to water, which shares key roles in vegetative growth, and grain, and reduces evaporative water loss (Jia et al., 2019). Rice grown under aerobic soil conditions utilizes NO3–-N, which minimizes ammonia (NH3) volatilization (Froes de Borja Reis et al., 2018). However, the AWD irrigation management of aerobic rice systems causes decomposition of OM, which is subjected to N loss in the form of NH3, and when the soil becomes dry, rapid coupled processes of nitrification-denitrification lead to more losses of N in the form of N2O (Sahrawat, 2010). Relative to lowland rice, the abundant form of N in aerobic rice systems is NO3–, which is subjected to lower NH3 volatilization loss, the prevailing form of N loss in lowland rice (Sahrawat, 2010, 2012). Intermittent soil wetting and drying lead to high ammonification of N from decomposition of OM under dry conditions, followed by NH3 loss during flooding conditions. Then, the soil becomes dry; again, ammonification followed by nitrification-denitrification processes lead to production of N2 and N-oxides (Cui et al., 2018). In aerobic rice systems, production response mechanisms in terms of apparent N recovery to total N applied and agronomic N-use efficiency are strongly affected by water supply (Fuhrmann et al., 2018), crop management practices, and microbial activities. Net productivity, N uptake, and biomass production can be enhanced with more N supply but with higher N loss and GHG emission, because the quantity of N available was higher than its uptake (Zhou et al., 2017; Huang et al., 2022). Therefore, for widespread adaptation of aerobic rice systems, understanding the shifts in N use dynamics under aerobic soil conditions is necessary, thereby reducing N loss and serious environmental hazards through agronomic management (Nie et al., 2009).
Importance of Increasing N Use Efficiency
Policy experts and scientists involved in climate change issues contend that N2O, one of the major GHG and the third ozone depletion agent, demands more focused consideration from agricultural and climate science research collaborations (Kanter et al., 2013). Human-induced activities have significantly impacted agricultural systems by increased use of synthetic inputs and changed the major biogeochemical processes especially N cycling, and contribute to high GHG emission. Agriculture is one of the major sources responsible for contribution in two-thirds of anthropogenic emissions directly or indirectly (Wang et al., 2013). Hence, agricultural and nonagricultural GHG emissions (energy generation sector, industry, and transport) increased the atmospheric concentration of N2O from 275 ppb in 1950 to 328 ppb in 2010 (Butler and Montzka, 2015). However, N2O is one of the major causes of GHG emission that aggravates climatic changes; its emission is also impacted by climate change. Rise in atmospheric temperature, changes in precipitation patterns and soil water contents, and increase in CO2 concentration directly impact N cycling, because N2O emission rate is dependent on these factors, several indirect impacts on N cycling because of variations in environmental components, which stimulate changes in CO2 concentrations and plant N uptake. The increase in temperature induced by climatic changes increases the rate of decomposition of soil OMs (Zhu et al., 2019; Khan et al., 2021), releasing the more mineral form of N into the soil medium and ultimately ensuring the more availability of N for nitrification and denitrification pathways (Fowler et al., 2015). Functions of soil oxygen levels are nitrification and denitrification that are inversely proportional to overall water contents in the soil. Meanwhile, soil water contents are a function of soil texture, balance between evapotranspiration and precipitation, and topography. Therefore, the impacts of increase in temperature and changes in precipitation patterns could change the overall N cycle through impacts on rates of nitrification and denitrification.
Additionally, it has been evidenced that increase in atmospheric CO2 concentrations indirectly impacts N cycle through shifts in N2O emission (Zaehle, 2013). Limited crop performance has been observed under N deficiency during elevated CO2 conditions, depending on the ability of plant N-uptake. Moreover, elevated CO2 can reduce soil N-availability because of probable increase in microbial growth, leading to enhancement of inorganic N in the soil. Under elevated CO2 conditions, higher carbon fertilization causes increase in photosynthesis while decreasing transpiration because of increase in plant N uptake, which ultimately decreases N2O emission (Venterea et al., 2012; Xu-Ri et al., 2012). The effect of continuity and magnitude of carbon fertilization mainly depends on the access of plants to acquire the available mineral N. Limited N availability is a major issue especially for natural ecosystems where main sources of N inputs are not able to maintain increased plant N demands stimulated by higher carbon fertilization (Zaehle, 2013). However, in agricultural systems that are major sources of anthropogenic N loss, N is commonly not a major concern because of excessive amendment and ensured availability of N from synthetic N fertilizers and organic manure. Therefore, in several regions, N may not limit the yield because of higher carbon fertilization under elevated CO2 conditions, which improves the N and water use efficiencies (Ueyama et al., 2013). If some copping systems can increase yields because of higher carbon fertilization, this could then decrease the excessive availability of soil N than required by plants, ultimately potentially reducing N losses.
Description of N Cycle
Ammonification
The second step of N mineralization is referred to as ammonification where reduced organic N (NH2) is converted into gaseous ammonia or reduced inorganic (NH4+) as end product. Various species of microbes, including bacteria and archaea, are involved in ammonification to derive metabolic energy by oxidation of organic N to NH4+. NH4+ is the readily available form of N for assimilation and incorporation into amino acids or other metabolic processes. If NH4+-N is not readily taken up or existing in excess than the metabolic requirements of microorganisms, it will be lost to the environment (Rehman et al., 2016). The rice plant preferably uptakes the NH4+ form of N rather than NO3– (Coskun et al., 2017a). Organic s usage in aerobic rice systems is one the best N management measures to increase NUE (Awan et al., 2014), where the N derived from organic sources is converted to NH4+ by biological N mineralization. The rate of ammonification in aerobic rice systems is less than that of other agricultural field crops with higher O2 depletion during flooding conditions (Belder et al., 2005).
Nitrification
Nitrification is the conversion of NH4+ to NO3– (Barth et al., 2020) via nitrite (NO2–) by biological oxidation (Black et al., 2019). In rice soils, NH4+ is readily converted to NO3–, which accumulates in the soil solution to make its concentrations higher. Conversion of NH4+ ions to NO3– via NO2– specifies the movement of N through negatively charged soil particles that determines the fate of N in the soil (Skiba et al., 2011). Generally, the nitrification process is divided into two pathways, In the first step, also termed as autotrophic nitrification, autotrophic nitrifiers obtain their required energy by oxidizing NH4+ to NO2– (Jia and Conrad, 2009; Li et al., 2018c). In the second step, also known as heterotrophic nitrification, heterotrophs convert the inorganic and organic N forms (NO2–) to NO3– without gaining any required energy. However, the share of autotrophic nitrification is larger than that of heterotrophic nitrification in N cycle (Hayatsu et al., 2017). The first step is usually performed by AOB, but this operation can also be performed by AOA (He et al., 2018). NO3–-N is more likely than NH4+ to move to plant roots via mass flow, thereby leaching down from the root zone during soil flooding conditions if not readily taken up or being lost because of denitrification during dry conditions of soil (Farquharson, 2016).
Recent advances in soil research have shown that AOA abundance is higher than that of AOB, but that AOB contribution in nutrification is more than that of AOA in rice soils (Banning et al., 2015). Nonetheless, AOB and AOA communities increased greatly in the rice rhizosphere after the application of a synthetic urea fertilizer (Liu X. et al., 2016; Liu Y. et al., 2016). The process of nitrification either with AOB or AOA is shown below in the reaction form, whereas a general complete description of N cycle internally in soil with all major steps, viz. (1) nitrification, (2) ammonification, (3) NH4+ immobilization, (4) NO3– immobilization, (5) dissimilatory NO3– reduction to NH3 (DNRA) (Palacin-Lizarbe et al., 2019), (6) heterotrophic nitrification, and (7) monomers uptake by plants, is shown in Figure 1. The general description of N cycle involves how a set of biogeochemical processes converts inorganic and organic N into various forms consecutively from the atmosphere to the soil to living organisms and then back to the atmosphere (Figure 1). N undergoes various procedures of transformations aiming to keep a balance in the ecosystem. N transformations help plants to synthesize chlorophyll from N compounds. During N cycle, ammonification occurs during which bacteria assist in the decomposition process of plants and animal matters, which indirectly facilitates in cleaning up the environment.
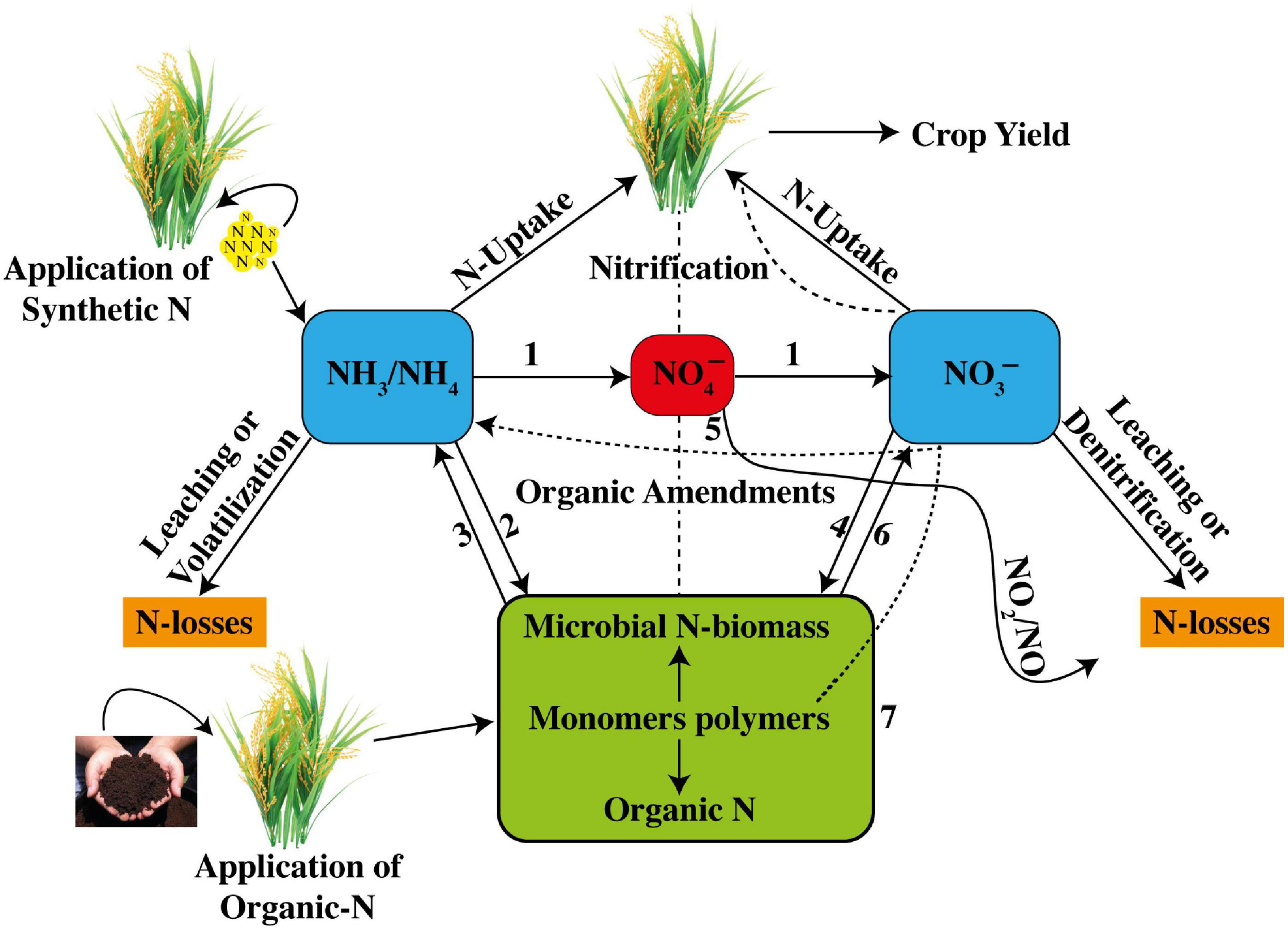
Figure 1. Internal soil N-cycling is comprised of nitrification, ammonification, NH4+-immobilization, NO3–-immobilization, dissimilatory NO3– reduction to NH3 (DNRA), heterotrophic nitrification, and plant N uptake (taking reference of Norton and Ouyang, 2019).
Latterly, certain species of Nitrospira bacteria have been observed in different paddy soils to mediate the entire two-step reaction of NH4+ to NO3– in an organism.
During the second step of nitrification, NO2–-oxidation is switched by nitrite-oxidizing bacteria (NOB) belonging to the genera Nitrobacter, Nitrospina, Nitrococcus, and Nitrospira (Pajares and Bohannan, 2016). Certain species of AOA, AOB, NOB, and Comammox (abbreviation of complete ammonia, and the name ascribed to a group of microorganisms that convert NH4+ into NO2– and then into NO3– via the complete process of nitrification) microorganisms avail their required energy from nitrification pathways by oxidizing inorganic N compounds as they are chemolithoautotrophs (Kuypers et al., 2018). Chemolithoautotrophic species of archaea and bacteria are the dominant microorganisms during nitrification in aerobic rice systems. These microbes are actively dependent on oxidation of NH4+ and/or NO2– for the required energy for their proper growth. Table 1 presents the information about multiple archaeal and bacterial strains and their ecophysiological roles in N cycle.
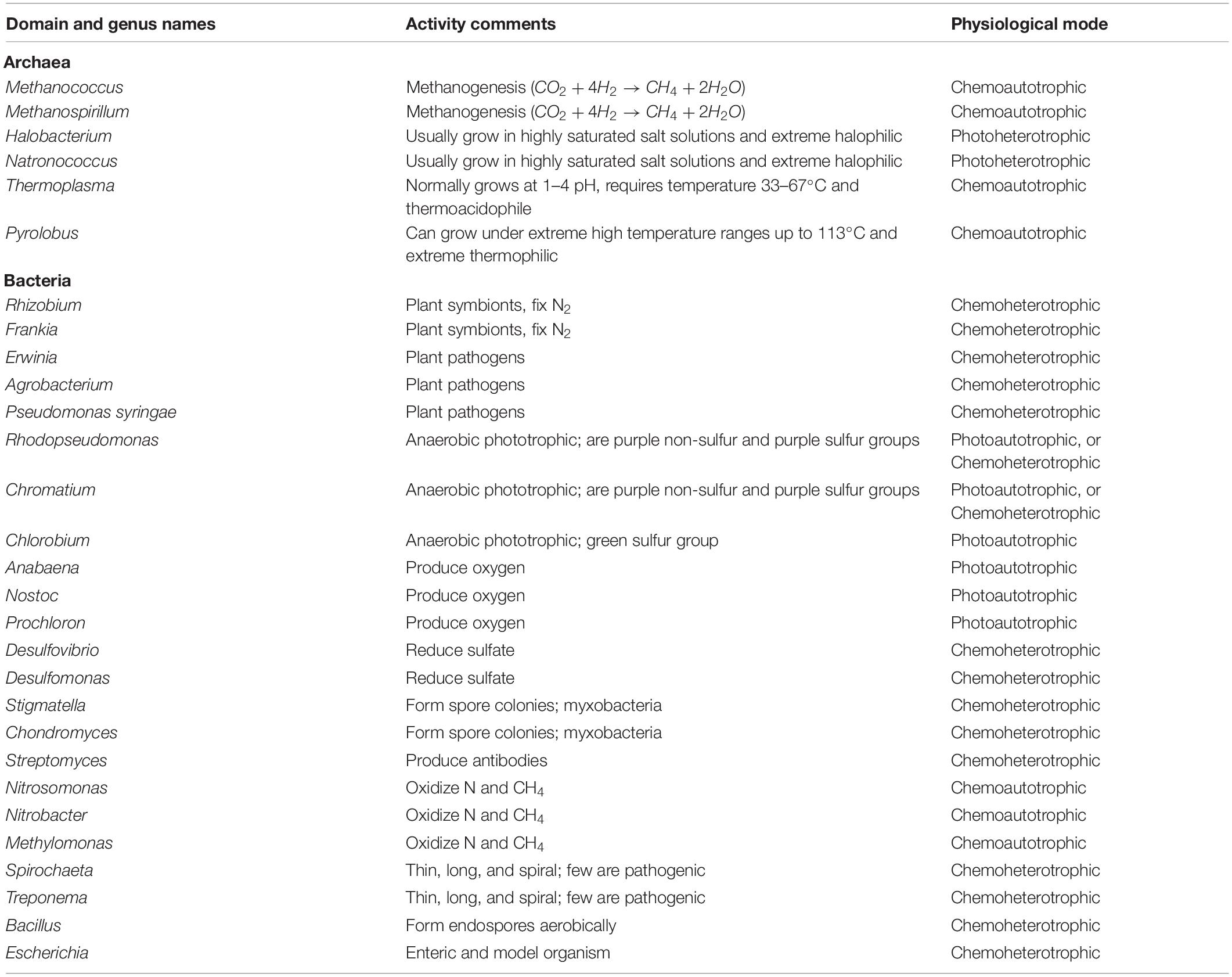
Table 1. Details of some archaeal and bacterial strains, their phylum names, and ecophysiological activities.
Denitrification
The microbial respiratory reduction process, in which the NO3– and NO2– forms of N are converted into gaseous forms of N like NO, N2O, and N2, is shown below in the reaction form. Reaction 1 corresponds to complete steps of reducing NO3– to N2 and showcases the intermediate products, whereas reaction 2 represents the complete redox reaction of denitrification showing reduction to end product. Denitrification is primarily mediated by denitrifying microbes, such as Pseudomonas, Thiobacillus, Bacillus, and Alcaligenes. The process of denitrification involves both heterotrophic (Paracoccus denitrificans and various Pseudomonas) and autotrophic denitrifiers (Thiobacillus denitrificans). Under flooded conditions, oxides of N serve as alternate electron acceptors to oxygen. Gaseous N2O can also serve as an alternative electron acceptor, but non-denitrifiers have the ability to reduce gaseous N2O (Bueno et al., 2015). Denitrification ability is infrequently dispersed among various groups of bacteria and archaea based on their taxonomic features (Nishizawa et al., 2013). Reduction of NO3– to NO2– is mediated by the nitrate reductase enzyme, and conversion of NO2– to NO is mediated by nitrite reductase enzyme, while its reduction to N2O is conducted in the presence of nitric oxide reductase.
Culture-based findings have shown that soil denitrifying bacteria population in rice soil ranges between 103 g–1 and 105 g–1 but how diverse they are is still unknown (Ishii et al., 2011; Maeda et al., 2011). Recently, microorganisms involved in nitrification in rice soils have been identified more clearly by crossing functional measures, such as stable isotope probing (SIP) (Yoshida et al., 2012), denitrification functional gene quantification, and functional single cell (FSC) isolation processes. In SIP analysis, succinate is used as an electron donor for denitrification process, which enhances denitrification than fermentation, and DNRA (Albina et al., 2019). A clone library analysis by closely full-length 16S rRNA gene sequencing has demonstrated that Burkholderiales, Rhodocyclales, and novel Betaproteobacteria, intimately related to Rhodocyclales, were prevalent in clones obtained, an SIP analysis has revealed that bacteria belonging to Rhodocyclales and Burkholderiales with closely shared features were dominant members of a 13C-succinate-assimilating community (Saito et al., 2008), and a comparative 16S rRNA gene analysis depicted that bacteria belonging to the genera Herbaspirillum and Rhodocyclales were abundant in rice soils under denitrification-prompting environments (Ishii et al., 2009).
Volatilization and Leaching
Inorganic N in aerobic rice systems is lost under AWD conditions because of leaching and volatilization. On acidic soils, ammonia volatilization is usually insignificant but under alkaline or calcareous soil conditions, N loss may occur (Ishii et al., 2011). Temperature, pH, and CO2 concentration are the major factors influencing ammonia volatilization (Buresh et al., 2008). Ammonium ions have the ability to be adsorbed into soil clay particles, contrary to NO3– and NO2–, which can easily be transported into the soil-water environment and, subsequently, can lead to eutrophication (Buresh et al., 2008). Therefore, during flooding conditions in aerobic rice systems, NO3– and NO2– are lost because of leaching. Compared with lowland rice systems, however, only a small proportion of NO3– is leached down because of strong denitrification pathways. Groundwater samples from a flooded rice field were extracted in Japan, and it was observed that a 0.9% proportion of the samples were contaminated with NO3– at ≤10 mg-N L–1, and that nearly a 36% proportion of the samples were contaminated with >10 mg-N L–1 (Kumazawa, 2002). Moreover, it was concluded that the concentration of NO3– in groundwater reached or even exceeded the unacceptable level. An overview representation of ammonification, nitrification, and denitrification of N cycle in rice soils is shown in Figure 1.
Ecophysiology and Relative Abundance of Ammonia-Oxidizing Archaea and Ammonia-Oxidizing Bacteria
Ammonia-oxidizing bacteria are terrestrial microbes, and they consist of two genera, Nitrosospira and Nitrosomonas; each of them is comprised of not less than four sub-clusters. Nitrosospira spp. are generally found in soil environments, whereas Nitrosomonas spp. are widespread in N-rich systems like sewage areas and lakes (Wang et al., 2010; Ke and Lu, 2012). It has been found that the habitat difference for the abundance of AOB species is associated with their physiological characteristics (Norton, 2014). Nistrosomonas spp. are termed as r-strategists with a comparatively fast growth rates but low substrate affinities, whereas Nitrosospira spp. are considered as k-strategists (Li et al., 2007) with relatively slow and decreased growth rates but high substrate affinities, which can decrease the pollutants’ concentration to low levels. More importantly, K-strategists can also play crucial roles in the biodegradation process of fractious organic pollutants. Fluctuations in substrate availability favor the potential activities of Nitrosospira spp., whereas Nitrosomonas spp. are better suited under soil conditions with high substrate availability.
All currently known AOA species belong to the phylum Thaumarchaeota in the domain Archaea. AOA exhibit the diagnostic archaeal ammonia monooxygenase (amoA) large subunit gene, which encodes the alpha subunit of ammonia monooxygenase and helps to produce the lipid biomarker thaumarchaeol. The amoA gene has been widely exploited and utilized as a molecular biomarker to study AOA and AOB distributions and dispersion in various environments, and it is used as a marker of the nitrification process. Ammonia monooxygenase (AMO), a membrane-bound enzyme, is considered as an important functional protein for ammonia oxidation and can oxidize NH4+ in AOA and AOB. Because of its capacity to encode the active site of ammonia monooxygenase, the amoA gene can oxidize ammonia and generate energy for the successive oxidation reaction. The distribution of the archaeal amoA gene has shown that the abundance and diversity of archaeal communities associated with soil vary with soil depth and conditions, such as pH, oxygen level, moisture content, salt concentration, and availability of NH4+ ions (Zhang et al., 2011; Sintes et al., 2013). The subclassification of AOA into the phylum Thaumarchaeota forms a separate group of AOA species showing their better adaptation and optimum activities under decreased ammonia concentration in soil. Moreover, an aerobic and mesophilic ammonia-oxidizing archaeon named Nitrososphaera viennensis has been found, which utilizes different trophic modes to gain energy for optimum growth (Beam et al., 2014; Stieglmeier et al., 2014). The ecological importance of AOA is still unclear under different environmental conditions (Shen et al., 2012; Huang et al., 2016), but under marine environments they are believed to be dominant, sharing 70% of total oxidized ammonia (Pitcher et al., 2011). There remain many confusions and uncertainties in findings of previous studies on whether AOA are more important in ammonia oxidation (Pitcher et al., 2011; Zhang et al., 2012) than AOB (Shen et al., 2012). However, it has been indicated that AOA abundance was higher at low NH4+ concentrations, and that high NH4+ availability favors AOB community abundance (Xia et al., 2011; Pester et al., 2012).
Ammonia-oxidizing archaea and AOB are the major ammonia oxidizers that contribute to ammonia oxidation in agricultural soils, but their relative abundance and share in ammonia oxidation are still challenging to predict (Zhao et al., 2019, 2020), because their abundance and activities are affected under contrasting soil conditions (Prosser and Nicol, 2012). In rice soil usually fertilized with inorganic fertilizers, the top soil layer was found abundant with 80 times more amoA (described in later part) copies, which can rise up to 700 times at a 30-cm soil depth (Leininger et al., 2006; Bertagnolli et al., 2016; Han et al., 2018). The ecospecies of NO2+ and NH4+ oxidizers in soil govern the rate of nitrification providing substrate excess are ensured (Taylor et al., 2012). Soil microbes, depending on soil conditions, show their potential receptiveness to the addition of synthetic N fertilizers because of temporary increase in substrate availability. A positive correlation between AOB abundance and nitrification rates was observed under high NH4+ concentration, but there was no or slight correlation for AOA (Ouyang et al., 2016). AOA community abundance showed a strong positive correlation with nitrification rates under acidic soil conditions (Sterngren et al., 2015), because acidic soil with no addition of synthetic N fertilizers reveals lower nitrification rates (Marusenko et al., 2013, 2015). However, recently, it was noticed that synthetic N fertilizers, when added to soil, had a positive and strong correlation with abundance of soil microbes (Ouyang et al., 2018).
Pathways of Ammonia Oxidation in Ammonia-Oxidizing Archaea and Ammonia-Oxidizing Bacteria
Ammonia-Oxidizing Bacteria
The rate-limiting reaction in nitrification is ammonia oxidation, which is considered as the main contributor in balancing the relative proportions of NH4+ and NO3– (Urakawa et al., 2006). Microbes responsible for this process, including AOA, AOB, and Comammox, have an enzyme named AMO (Koch et al., 2019), which is the key enzyme in oxidation of NH4+ (Simon and Klotz, 2013). It catalyzes the conversion of ammonia into hydroxylamine (NH2OH, then converted into by hydroxylamine oxide reductase) (Vajrala et al., 2013). This membrane-bound enzyme is composed of three subunits, produced, and coded by the genes amoA, amoB, and amoC that are arranged as the amoCAB operon (Figure 2). Two polypeptide subunits of AMO are coded for by adjacent genes, amoA and amoB, which are anteceded by a third gene, amoC. amoCAB operon clusters are present in the form of multiple copies in nitrifying bacteria of the β-subdivision. Considering the homology of amoA and amoC with other monooxygenases and hydrophobicity projections, they are supposed to the intrinsic part of membrane proteins, whereas amoB seemingly accompanies a copper-catalyzed active site (Balasubramanian et al., 2010). Later, the AMO-based end product NH2OH is converted into by the hydroxylamine oxidoreductase (HAO) enzyme (Bollmann et al., 2013; French and Bollmann, 2015) presented in Figure 2. In contrast to AMO, HAO contains ferrous heme P460 in the active site; therefore, it has no susceptibility to inhibition by copper chelators. However, the NH2OH obligate intermediate model was postulated which demonstrated that NO is an active and essential intermediate product during the oxidation of NH2OH to in ammonia-oxidizing archaea rather than participating directly in the oxidation of NH3 to NH2OH. This phenomenon subjected towards the introduction of “NH2OH/NO obligate model”. Therefore, NO would not be considered as a byproduct of NH2OH oxidation but is preferably required in producing NO2 (Liu et al., 2017). This, however, implicates the presence of an anonymous enzyme leading that conversion (Caranto and Lancaster, 2017).
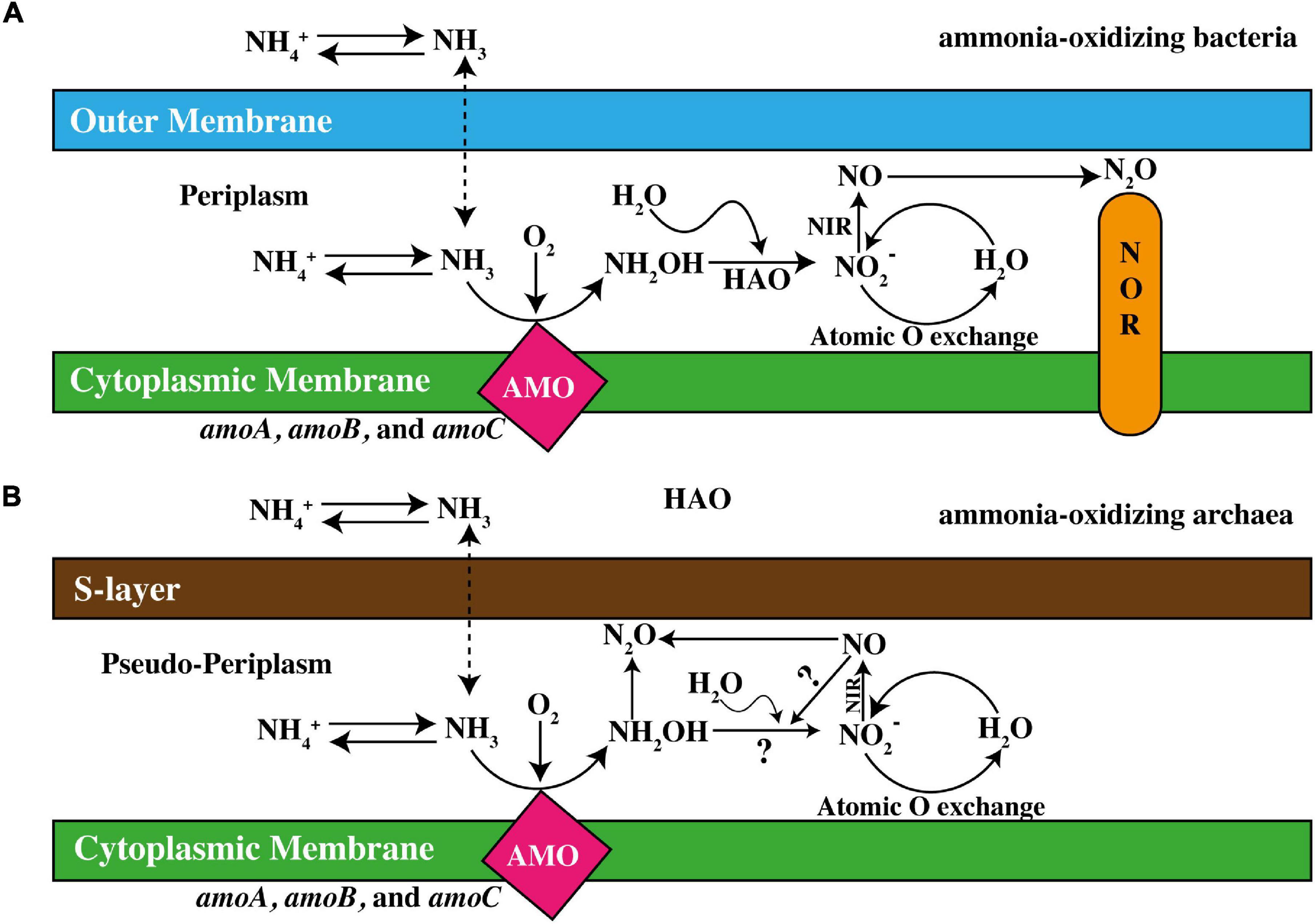
Figure 2. Demonstration of ammonia-oxidation in (A) ammonia-oxidizing bacteria (AOB) and (B) ammonia-oxidizing archaea (AOA). HAO, hydroxylamine dehydrogenase; NIR, nitrite reductase; NOR, nitric oxide reductase (adapted from Yin et al., 2018).
Ammonia-Oxidizing Archaea
Ammonia oxidation pathways conducted by AOA is less understood (Schleper and Nicol, 2010) in comparison to AOB, but genomic analyses have shown that AOA are occupied with well-defined homologs of genes encoding three subunits of AMO (Stahl and de la Torre, 2012; Kerou et al., 2016). Additionally, the AMO in AOA is accompanying a gene named amoX and potentially encodes a fourth AMO subunit (Bartossek et al., 2012). Archaea, are devoid of the active bacterial amoB site, which intimates different ammonia oxidation pathways (Tolar et al., 2017). Possibly, another active site in the amoX or amoC subunit may be responsible for the AMO activity in archaea, the earlier probably less likely due to its transmembrane prophecy (Li et al., 2018b). Even with the nonexistence of HAO homologs, intermediate production and consumption of NH2OH exist during archaeal ammonia oxidation, (Kozlowski et al., 2016; Sauder et al., 2018), as shown in Figure 2. Alternatively, various oxidoreductase enzymes have been found contributing in bacterial HAO as P460, and due to their cytoplasmic nature owing no signal peptide for periplasmic secretions such as of energy-efficiency concerns, NH2OH production take place in the periplasm (Lawton et al., 2013).
A functionally multifaceted cofactor is found in all archaeal genomes assisting in various low-potential 2-electron redox reactions and could be responsible for HAO activities (Zhalnina et al., 2014). For proper transport of required electrons in archaeal NH2OH oxidation, production of NO is probably necessary (Kozlowski et al., 2016; Hink et al., 2018), which is accomplished through a nitrite reductase enzyme (Spang et al., 2012). A schematic illustration of ammonia-oxidation pathways in AOA and AOB is given in Figure 2.
Interactive Mechanisms of N Cycle, Plant N Uptake, and Microbial Activities
Plant N uptake preference for NH4+ or NO3– could employ varying effects on soil microbes (Thion et al., 2016; Li et al., 2017). Generally, the N-transformation in soil is stimulated by the release of carbon by plants into the rhizosphere either as root exudates or direct release to mycorrhizal communities (Meier et al., 2017). Interactive processes in the rhizosphere reduce the rate of nitrification by microbial assimilation of NH4+ (Hawkes et al., 2007). Nitrification can be inhibited by nitrification inhibitors (NIs) either released by roots or added artificially (Sun et al., 2016; Coskun et al., 2017b).
Pure cultures of Nitrosomonas spp. have been noticed with weak competition for NH4+ uptake relative to heterotrophic bacteria spp. (Laanbroek and Bär-Gilissen, 2002). Rice grown under different soil conditions has shown very high nitrification rates than microbial NH4+ assimilation, specifying that soil nitrifiers are powerful and responsive microbes for NH4+ (Bottomley et al., 2012; Inselsbacher and Näsholm, 2012). Soil microbes having a heterotrophic mode of activities can also assimilate NO3– when the soil contains high OM and carbon contents. The competition for NH4+ is determined by balance between soil carbon and NH4+ availability, whereas interactions between different soil microbial communities involved in the food web of soil also bridge several reactions in N cycle (Jiang et al., 2014; Trap et al., 2015). Interestingly, it has been found that the community structural composition of AOB entirely got changed because of the presence of bacterivorous nematodes (Xiao et al., 2010). Moreover, the presence of bacterivorous nematodes considerably reduces AOB abundance but encourages AOA community abundance (Zhu et al., 2018). In a study conducted on rice systems grown under wastewater application conditions have shown that Nitrospira spp. are consumed by bacterial predators (Zhang et al., 2019b). Complex interactions among soil, plants, and microbes involved in the soil food web are indispensable to nutrient cycling processes, such as soil organic and inorganic N. In the root zone within a soil environment, the interaction between microbes and the root system implicates a range of microbial activities that contribute to C and N dispersal through diffusion and mass flow into the soil composition.
Factors Affecting the Activities of Ammonia Oxidizers
Changes in NH4+ Concentration
Nitrogen, in the form NH4+, functions as the common substrate of AOA and AOB; therefore, ammonia concentration in soil influences the growth and activity of nitrifying microbes. AOA are highly responsive to NH4+ compared to AOB (Chen et al., 2017), consequently making low inhibitory level for AOA (Yin et al., 2018). By increasing NH4+ concentration, the amoA gene of AOA was decreased, which stipulated that low NH4+ concentration favors the growth and activity of AOA (Sauder et al., 2012) and vice versa for AOB (He et al., 2018; Wan et al., 2019). It has been observed that the per cell rates for NH4+-oxidation and the half-saturation constant for AOA activities were significantly lower than those for AOB (Ouyang et al., 2017). For example, an increase of 100 mg/L in NH4+ concentration did not produce any significant differences in AOB abundance, but AOA abundance was reduced (Bellucci et al., 2011). In summary, synthetic N fertilizers and high availability of NH4+ promptly encourage AOB community abundance and activities, whereas AOA activities, abundance, and their response to NH4+ were not affected by high amendment of synthetic N fertilizers and availability of the NH4+ substrate (Wang et al., 2022). AOB communities dominantly contribute by their roles in nitrification pathways under nonlimiting NH4+ whereas AOA dominate nitrification at low NH4+ concentration in the soil. Understanding and interpretation of different response mechanisms of AOA and AOB to the NH4+ substrate can permit ammonia oxidizers to be indulged into the simulation as dynamic elements that drive N-fluxes.
Changes in Soil pH
Soil pH is affected by factors, such as land use changes, soil burning, and accumulation of acids (Malik et al., 2018). Soil microbial populations and their relative activities in biogeochemical processes are affected by changes in soil pH (Bernhard et al., 2010). Among soil microbial communities, especially ammonia oxidizers, changes in soil pH greatly influence bacterial growth and activities (Zebarth et al., 2015), and depict the relative abundance of soil ammonia oxidizers (Prosser and Nicol, 2012). Soil pH is one of the principal factors commanding the rates and end-product accumulations of nitrification (Kyveryga and Blackmer, 2018). Ammonia and nitrite oxidation rates are enhanced under neutral or slight alkaline soil conditions, therefore higher N-loss occurs because of accumulation of NO3–. Increase in acidification of arable soil encourages high rate of nitrification and leaching of NO3– (Schroder et al., 2011). AOB cultures showed no tolerance to acidic conditions, and their rates of nitrification were fiercely decreased with decrease in soil pH (Zhang et al., 2017). Under application of synthetic N-fertilizers, such as urea substrates, the heterotrophic mode of nitrification has been observed under acidic soil conditions (Burton and Prosser, 2001). Ammonia oxidation in acidic soil via AOA has been under great consideration since the actual roles of AOA in N cycle have been disclosed, but the literature is still ambiguous with high uncertainties (Afzal et al., 2019). However, the low-pH soil has low intensity of nitrification relative to high pH soil (Shen et al., 2012) where ammonia availability decides the pH of soil (Lu et al., 2012). Moreover, the presence of toxic and heavy metals in soil makes it less fertile, thereby impacting the activity and growth of AOA and AOB. This can be attributed to soil microbes with high tolerance to low-pH soil, and microbes with no tolerance to high acidity are only operative in basic soils (Shen et al., 2012). Recently, it was confirmed that the most active and abundant ammonia-oxidizing microbes are AOA, although AOB were also found but with different response mechanisms (Huang et al., 2016). Physiological and microcosm studies have shown the mechanisms by which changes in soil pH impact the abundance, activities, and growth of microbial functional groups (Verhamme et al., 2011).
Changes in Soil Temperature
Soil microbial activities are highly reactive to modulation of soil temperature (Naher et al., 2019). Generally, microbial growth and activities are increased under high temperature conditions at a typical temperature range (Heinze et al., 2016). The responsiveness and preferential depletion in the mineral soil of mineral-associated OM under warming is highly dependent on the availability and type of the substrate (Hill et al., 2015). The fate and quantity of available N to the rice plant is determined by soil temperature (Thangarajan et al., 2015). The biological transformation of N is greatly influenced by soil temperature (Daebeler et al., 2018), as nitrifiers are highly responsive to changes in temperature (Osborne et al., 2016) where the rate of nitrification also fluctuates with variations in temperature (Ramanathan et al., 2017).
The nitrification response to varying soil temperature depicted that the optimum temperature required for nitrification is environment-specific (Taylor et al., 2017). Across the range of global ecosystems, the community abundance and diversity of AOB were correspondent with temperature change (Wessén et al., 2011); however, the soil with relatively high dominance of AOA has been observed under the optimum temperature range required to conduct nitrification pathways (Alves et al., 2019). AOB had an optimum soil temperature range of 25–30°C (Hayatsu et al., 2017), whereas a type of soil with AOA dominance was noticed in a winter temperature range in Arctic regions (Alves et al., 2013), which evidenced that AOA communities with high tolerance to acidity are well-adapted to low temperature conditions (Herbold et al., 2017). In contrast, a different study has reported that archaeal population and archaeal ammonia oxidation activities were increased under warmer soil conditions (Tourna et al., 2008). In high-altitude regions, the prevalence of cold periods influences the yearly mineralization of N (Amoo and Babalola, 2017). Some proportions of microorganism communities remained active in unfrozen water films in high-altitude regions to keep a satisfactory level of microbial activity (Schütt et al., 2014). However, it has been noticed that intensive microbial activities stop when soil temperature becomes 5°C (Nottingham et al., 2019). In contrast, optimum activities of nitrifying bacteria and archaea (Santoro and Casciotti, 2011) were observed in the temperature range of 4–8°C (Schütt, 2015). A typical temperature range of 20–36°C for optimized activities of ammonia oxidizers has already been demonstrated (Thangarajan et al., 2015), but for nitrifiers, enhanced activities were noticed specifically at 25°C (Sahrawat, 2008).
The occurrence of soil biological processes (pathways of N-cycle) and climate change are closely correlated with each other in many aspects, as climate variability causes prevalence of biotic and abiotic stresses with adverse impacts on biological processes (Cheng et al., 2015). It has been observed in several climatic regions with high temperature that stress increased the nitrification rates even by up to 50°C (Ihara et al., 2014). The synchronization between interactions of OM and temperature response function may impact the N mineralization process (Takriti et al., 2018). Moreover, it has been observed that temperature impacts the biological activities in N cycle differently compared to inorganic and organic N amendments (Amoo and Babalola, 2017).
Changes in Oxygen Levels
Nitrification requires oxygen as a necessary substrate, and different responses of soil microbes to oxygen (AOA > AOB) considerably impact nitrification, which also changes with fluctuations in soil available ammonium, water, and pH. Under flooded conditions, the high oxygen response of AOA makes them more competitive over AOB (Sheng et al., 2016). Higher abundance of AOA was seen under hypoxia and low dissolved oxygen concentrations (Zhalnina et al., 2012), which predicted the simultaneous occurrence of nitrification and denitrification processes (Lian et al., 2014). It has also predicted that inhibition of nitrite-oxidizing activities may be conducted by the coupled activity of AOA and denitrifying bacteria (Jang et al., 2019), where N may be assimilated through the simultaneous presence of nitrifiers and denitrifiers (Li et al., 2018a). A real-time PCR quantitative analysis showed the coexistence of microbial communities that include AOA, AOB, NOB, and anammox bacteria (anaerobic AOB have a unique mode of metabolic activities and are able to oxidize NH4+ and reduce NO2– or NO3– to produce N2 gas) under low oxygen (Yapsakli et al., 2011). N assimilation through mutual activities of soil microbes involved in ammonia oxidation and denitrification (anammox bacteria) can efficiently be performed by regulating dissolved oxygen concentration to optimize microbial community abundance.
Changes in Soil Water Contents
Soil water availability greatly influences microbial activities, as accessibility of water changes the activity of microbial enzymes (Yan et al., 2015). Nitrification rate is influenced by variation in soil moisture contents, which impact the substrate accessibility of oxygen and NH4+ by diffusion and through direct effects of dehydration under low water potential. N mineralization is also impacted by changes in soil water availability, as it shifts the required substrate availability to microbes by impacting their growth and internal metabolism (Han et al., 2012). Controlled increase in water contents increases nitrification rate, but submergence of water reduces nitrification rate, as flooding leads to limited oxygen supply (Norton and Stark, 2011). During dry conditions, thin water layers between successive soil particles inhibit the movement of substrates to microbes (Yan et al., 2015). Production of NO3– and availability of soil water are positively interlinked, because water serves as the medium for substrate transportation (Wang J. et al., 2017). Microbial cell growth, metabolism, and abundance get adversely affected under high available NH4+ and higher solute concentration in different soil regimes; therefore, microbial activities become limited because of low water potential (Hu et al., 2015). Moreover, the abundance of the amoA gene is directly linked with the availability of soil water contents.
Changes in Organic Matter Contents
Microbial communities responsible for ammonia oxidation are also influenced by changes in soil OM contents (Gao et al., 2016). Generally, AOB are usually recognized as autotrophs, but it is very difficult and still uncertain to distinguish their mode of action either as autotrophic or mixotrophic. It has been found that high OM significantly inhibited the growth of certain AOA strains, i.e., Nitrosopumilus maritimus and Nitrosocaldus yellowstonii (Rusch, 2013). It has been discovered that increase in OM content encourages the growth of AOA strains having a mixotrophic mode of growth and activity (Qin et al., 2014). Genome sequencing has proven that various AOA strains had carbon utilization mechanisms namely 3-hydroxypropionic acid/4-hydroxybutyric acid cycle named as autotrophic metabolism. Moreover, tricarboxylic acid cycle was named as heterotrophic metabolism, which consequently demonstrated that AOA strains are equipped with both the potential autotrophic and heterotrophic modes of metabolisms (Beman et al., 2008). Comparing the structural composition of soil microbial communities, it has been assessed that there may exist a nexus of metabolic mechanisms in AOA with diversified metabolic characteristics at different carbon levels, eventually depicting different ammonia oxidation capacities of ammonia oxidizers (AOA and AOB).
Changes in Bioavailability of Nutrients
Microbial activity is reduced under limited availability of required substrates and is a significant feature in nitrification activity. Production of NO3– from ammonia oxidation pathways is conducted by either chemoautotrophic or heterotrophic nitrifiers (bacteria, fungi, and archaea) (Pajares and Bohannan, 2016). The contribution of microbes in biological processes can be estimated through the C/N ratio of the required substrates. The bioavailability of carbon is intensified under higher C/N ratio stimulating the growth of microbes (heterotrophs) that inhibit the activities and growth of autotrophs. Substrates required for nitrification can be taken from inorganic and organic sources. N-containing compounds play the role of a substrate, as the N from organic sources has been observed to be used as substrates in N cycle on acidic soils (Zhang et al., 2014).
The substrates required in N cycle determine the type of vegetation in any climatic region (Stein and Klotz, 2016). The growth, abundance, and activities of microbial communities in N cycle are affected by plant population (Bosco et al., 2015). The bioavailability of nutrients has an impact more than other factors, because addition of any required substrate to soil and uncommon changes in soil pH may occur (Zhang et al., 2016). Low NH4+ level may have concerns with reduction in growth and activities of AOA (Norton and Ouyang, 2019). During N cycle, the concentrations of required NH4+ substrates vary for AOA and AOB (Leininger et al., 2006). AOA are presumed to work well under low soil fertility and oligotrophic conditions (Liu et al., 2018), whereas AOB work appropriately under opposite conditions (Erguder et al., 2009; Daebeler et al., 2012). Meanwhile, AOB survive better under high concentration of NH4+, whereas AOA thrive superiorly at low level of NH4+ (Sterngren et al., 2015). A hypothetical illustration of variations in responses of AOA and AOB to changes in environmental factors is shown in Figure 3.
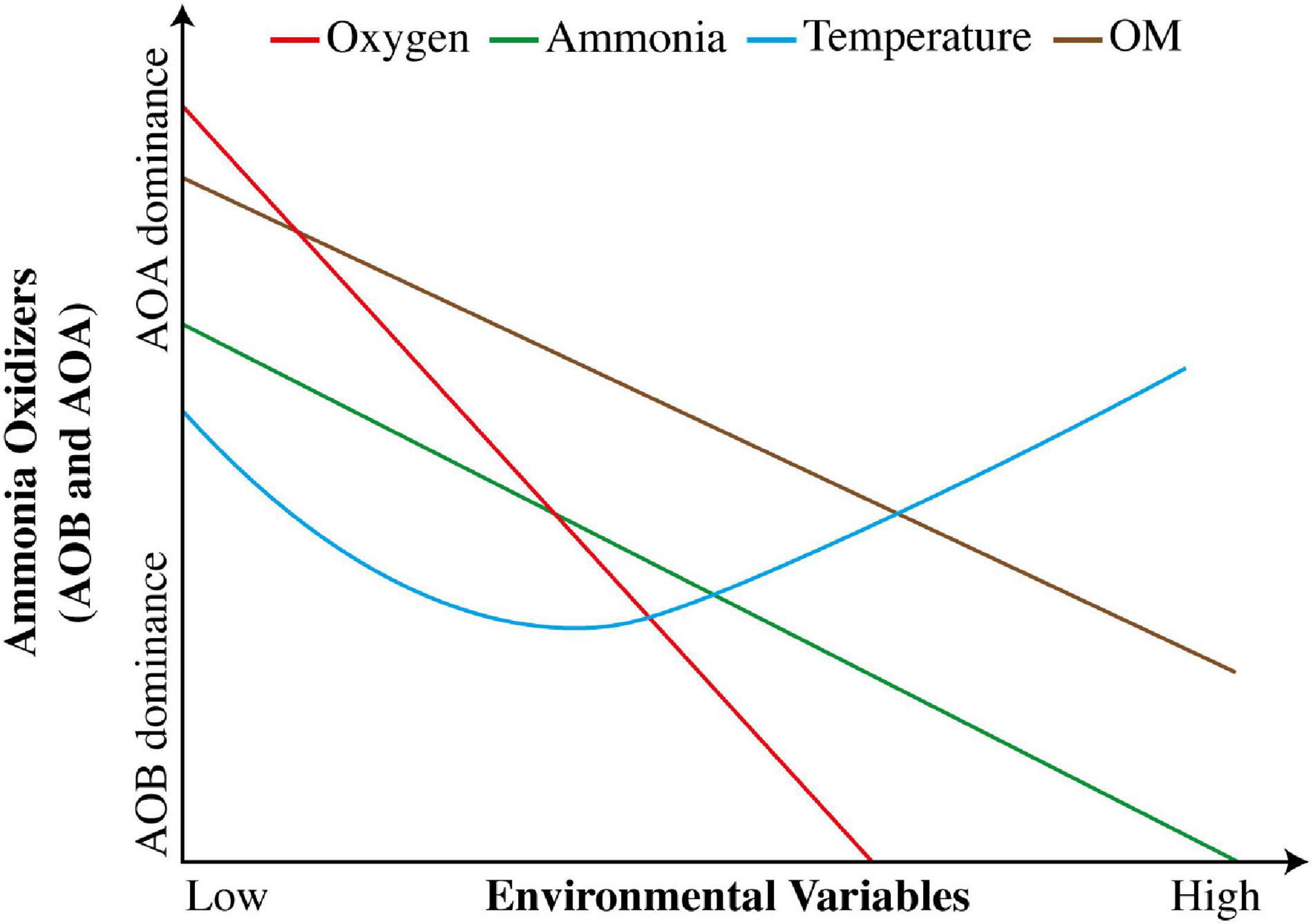
Figure 3. Hypothetical variation in responses of AOA and AOB to changes in environmental factors (adapted from Ouyang et al., 2017).
Agronomic Management Measures
Increasing N Use Efficiency and Development of Nitrification Inhibition Assays
Among rice-producing countries, China is the top intensive user of N fertilizers for higher rice production to meet its national food demands. However, the country should have adopted management measures, such as use of controlled-release fertilizers, NIs, N-splitting, basal and deep placement of N fertilizers, and optimizing the rate and timing of fertilizer application to increase NUE (Xia et al., 2017). The application of N fertilizers based on soil requirements can be effective to minimize N-losses due to leaching, volatilization, and runoff in aerobic rice systems, thereby enhancing NUE and reducing environmental hazards. Splitting and coating of total required N along with use of NIs can establish economic benefits and help in meeting food demands under scarce resource availability and selective conditions (Nelson et al., 2014). Soil management practices, such as zero or no tillage, crop rotation, soil mulching, and crop residue amendment, can improve soil health and nutrient availability, resultantly making aerobic rice systems more resilient (Zhang et al., 2015). Moreover, soil and N fertilizer management practices can ensure environmental sustainability with enhanced NUE and sustainable production in aerobic rice system same as the conventional.
Inhibition of Nitrification Through Nitrification Inhibitors
Nitrification inhibitors reduce the speed of microbial conversion pathways of NH4+-N to NO3–-N, reducing the risk of possible N-losses by leaching or volatilization, thereby increasing NUE (Menéndez et al., 2012). Many synthetic NIs target the ammonia monooxygenase enzyme often acting as a competitive suicide substrate (acetylene) (Ruser and Schulz, 2015). Irrigation management strategies through the AWD technique in aerobic rice systems cause coupled nitrification-denitrification pathways, which are subjected to high N losses. Therefore, to reduce N loss and avoid the negative impacts of nitrification, various Nis can be commercially manufactured and widely used. Most recurrently used commercial NIs include dicyandiamide (DCD), nitrapyrin, and 3,4-dimethylpyrazole phosphate (DMPP) (Suter et al., 2010) that act as metal chelators to bind copper (Cu) on the active site of the amoB subunit (Ruser and Schulz, 2015). Urease inhibitors, i.e., N-(n-butyl) thiophosphoric triamide (NBPT), are used to decrease synthetic urea hydrolysis and volatilization. Moreover, nitrapyrin can be used as an alternative amoB substrate, consequently producing an end product that irremediably stops ammonia oxidation. It has been evidenced that in a Nitrosomonas europaea probe, using either ammonia or hydroxylamine as a substrate, nitrapyrin and DCD were evidenced to deploy the inhibition of AMO, not HAO (Zakir et al., 2008; Pariasca Tanaka et al., 2010). Amazingly, nitrapyrin is equipped with a very weak nitrification inhibition capacity toward AOB (Shen et al., 2013). It has also been found that application of NIs (including urease) significantly reduced the leaching loss of inorganic N by 48%, N2O emission by 44%, and NO emission by 24% (Qiao et al., 2015) and that it increased agricultural production by 7.5% and NUE by 12.9% (Abalos et al., 2014; Recio et al., 2018).
The strength of NIs under different soil conditions is impacted by temperature fluctuations and plays a key role for their efficient inhibition activities (Guardia et al., 2018a). For optimum balance between GHG emission and N oxides, improved NUE and crop yield preferably require the use of Nis of organic sources in aerobic rice systems (Recio et al., 2018). Nevertheless, NI use under field conditions may increase environmental pollution because of high potential of NH3 emission (Qiao et al., 2015). Many potential NIs have been discovered, but some concerns restrict their widespread application under field conditions; usually, they are equipped with alkynes functional groups potentially hindering ammonia oxidation (Taylor et al., 2015). Acetylene is known as a general NI with a short-chain alkyne group because of its ability to bind the amoA subunit instead of amoB (Bennett et al., 2016). The nitrous oxide scavenging observed among NO scavengers that showed their receptibility with AOA was proposed to block the necessary electron transport to parallel bacterial HAO (Hu et al., 2013). Mixed application of NIs is suggested to target different levels of ammonia oxidation pathways, which can reduce the possibility of resistance development (Coskun et al., 2017b). Moreover, commercial chemical NIs are not recommended in highly certified and organically produced cropping systems; therefore, organic alternatives for such NIs are necessary to control nitrification (Opoku et al., 2014).
Nitrification Inhibition Through Biological Nitrification Inhibitors
Certain plants produce root exudations, which act as NIs when exposed to NH4+ substrates; this process is termed biological nitrification inhibition (BNI), and compounds involved in this process are known as BNIs (Byrnes et al., 2017; Nuñez et al., 2018). Rice rotation with crops producing BNIs potentially enable cash and catch crops to block the activities of HAO and AMO (Subbarao et al., 2009). Root exudations of BNIs are better and beneficial than synthetic NIs (Tesfamariam et al., 2014), as their impacts are short-term and dependent on soil conditions (Ruser and Schulz, 2015). Notably, methyl 3-(4-hydroxyphenyl) propionate (MHPP) is one of the BNIs that stimulates root branching and increases the potential of N hunting because of a dual kind of functionality (Meena et al., 2014). Some BNIs have been extracted from leguminous crops in tropical regions, i.e., Sorghum bicolor has been observed to have nitrification inhibition abilities (Subbarao et al., 2013, 2015). Root exudates containing BNIs are also stable under different soil conditions, and the production of BNIs is mainly restricted to the rhizosphere; hence, the modes of action of these compounds could be more efficient. Brachiaria humidicola is a tropical pasture grass and one of the well-understood BNI-containing plant species with capacities to grow under highly acidic soil and humid environmental conditions, and it produces the biochemical BNI compound known as brachialactone. Blending of such leguminous and non-leguminous crops into rice crop rotation may help in increasing the soil capacity to retain N and soil N-pools. Transferring the BNI traits of green manure crops into cereal crops will potentially increase NUE with some trade-offs to production.
Nitrification Management Under Changing Climate
Modern rice production systems require plenty of management measures to reduce N losses under climate changes, which have been going for centuries. The aim of reducing N losses in modern production systems is seemingly difficult considering the technical, management, and socioeconomic consequences. Soil specificity-based management strategies are necessarily required to limit the residence time of N in soil while promoting the maintenance of N retention capacity of soil. Limiting the field fallowing after harvest, use of mulches and cover crops, green manure amendments, and crop rotation practices are beneficial practices to reduce N losses, allowing soil to retain more N (Figure 4). Now, it is well-understood that N-cycle is impacted by climate change, which brings certain changes in nitrification pathways (Robertson et al., 2013), such as changing notification rates and its tendency and subjectivity, toward higher N losses by leaching and volatilization (Li, 2007). As described earlier, nitrification pathways are totally dependent on availability of soil NH4+ substrates, moisture content, temperature, pH, and textural conditions (He et al., 2019). Moreover, trait-based models have shown that during nitrification, changes in activities and abundance of AOA, AOB, and NOB communities can occur because of climate change (Bouskill et al., 2012; Bowles et al., 2018). However, fertilizer application management strategies, inputting synthetic NIs and BNIs, and management of irrigational water are totally dependent on farmer choice. Therefore, such management should necessarily be applied in modeling research on soil nitrification to recommend modifications on local scales in these practices under climate change.
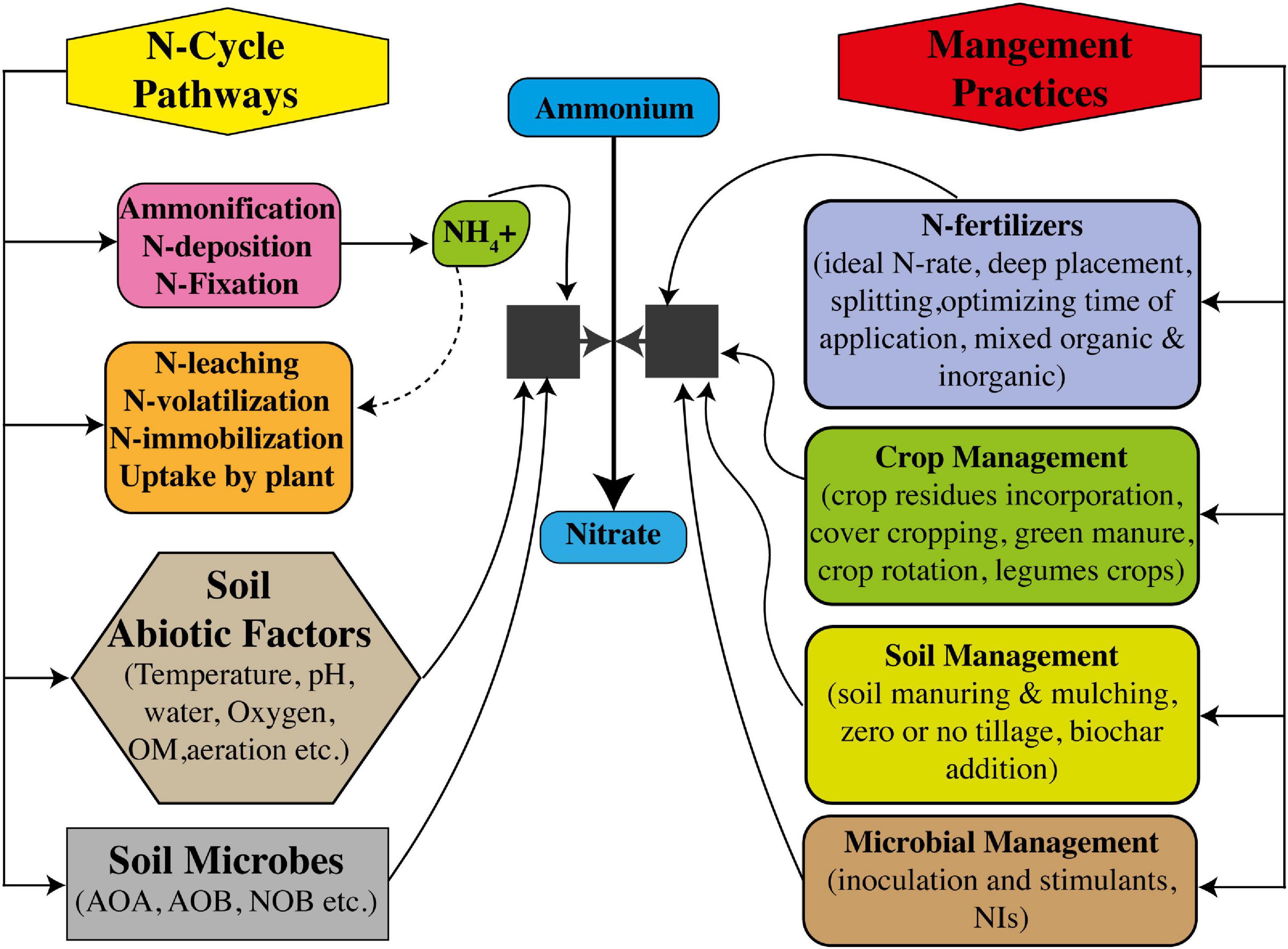
Figure 4. Interactive control of nitrification to increase nitrogen use efficiency (NUE) through soil microbes and management measures of soil, crop, and N fertilizers.
Optimizing Time of N Fertilizer Application
Conventionally, application of N fertilizers can be performed before planting or in splits where the quantity can be maintained based on growth stage requirements. The plant N uptake and NUE of different N fertilizers can be enhanced by ensuring the application of N fertilizers as per crop growth stage requirements rather than prior to crop planting (Morris et al., 2018). Splitting of N fertilizers and timing of application should match the specific plant growth stage after proper soil testing where the crop is grown. Side-dressing of N fertilizers is one of the best fertilizer management measures that can increase plant N-uptake and NUE by reducing N2O production by 60% (Guardia et al., 2018b). There are vast areas globally where it is more convenient to apply anhydrous NH3 during dry soil conditions aiming to retain higher soil N with reduced nitrification rates. Under dry soil conditions, there are limited economic benefits of N fertilizer application; therefore, it is recommended to apply N fertilizers when temperature and moisture contents are in optimum ranges to delay nitrification. Application of Nis along with synthetic N fertilizers in aerobic rice systems under the AWD system to delay nitrification can greatly increase NUE.
Ensuring Better Assimilation and Utilization of Available N
The availability of N nutrients in both organic forms, such as urea and free amino acids, and inorganic forms like NH4+ and NO3– under different soil conditions greatly differs and impacts plant growth, and physiological, metabolic, and root morphological features. Soil available N can change nitrification rates and NO3– accumulation under high competition conditions for N uptake. The N retention capacity of soil and plant roots in organic forms greatly depends on soil properties and plant root morphological characteristics. Paradoxically, when nitrification is happening, low nitrification rates can be observed because of high NO3– uptake by plants and microbes (Norton and Stark, 2011). Aiming to increases the direct N uptake in plants (by substantial ammonification) with suppression of nitrification, a wide range of N conservation measures have been developed for agricultural systems. Fundamentally, these essential measures limit N cycling with increase in organic N accumulation in soil. These essential measures include rice crop rotation with cover crops and legumes, synthetic N fertilizer management, and OM incorporation, which support the subsistence of rice plant roots, improve plant N uptake, and decrease N losses (He et al., 2019).
Modifying and Strengthening N Cycle
Aerobic rice systems with intensive amendments of inorganic N fertilizers share soil N cycling with higher nitrification rates. Generally, NO3– is considered as the dominant form of N right after the application of synthetic N-fertilizers. It is well-known that the quantity of soil available N increases after fixation and ammonification depending on N fertilizer source, and rice systems with reduced rates of nitrification are recommended to limit N losses and improve NUE. To ensure higher and sustainable grain yield in aerobic rice systems, it is necessary to ensure the sufficient availability of soil N in either of the preferable plant-available forms, i.e., as NO3– or NH4+, during the crop-growing season (Javed et al., 2022). As a result of OM mineralization, these available forms of mineral N compounds are unremittingly released in soil solution and available to plants. The release of mineral N compounds as with OM turnover, also termed as “N-delivery,” is majorly dependent on the climatic, soil, and cultivation features. Although there were large-scale and diversified research studies conducted in the past, so far, there have been uncertainties in estimation of available mineralization rates under aerobic rice systems. Therefore, fertilizer management strategies are necessary to bring modifications in overall N cycling, which can ensure the availability of preferable form of N for plant uptake.
Diversification of microbial communities having functions in N cycling can also favor the soil N retaining capacity. For better assimilation of applied N, intensification and modifications in N cycle are necessary with incorporation of high carbon-containing organic fertilizers particularly green manure, biochar, and compost (Paustian et al., 2016). Moreover, direct inoculation of N fixing and ammonification encouraging bacteria in N cycling of intensified aerobic rice systems can also improve the assimilation of soil-amended N (Hu and He, 2018). It has been depicted that use of inorganic N sources makes N cycling easier but at the cost of high N losses due to higher nitrification rates, whereas amendment of organic sources favors N fixation and ammonification and ultimately increases soil N availability for plants. Therefore, a simplified aerobic rice cropping system with less nitrification rate by proper selection of either organic or inorganic N fertilizer sources is necessary to increase the overall NUE (Norton and Ouyang, 2019), as shown in Figures 5A,B.
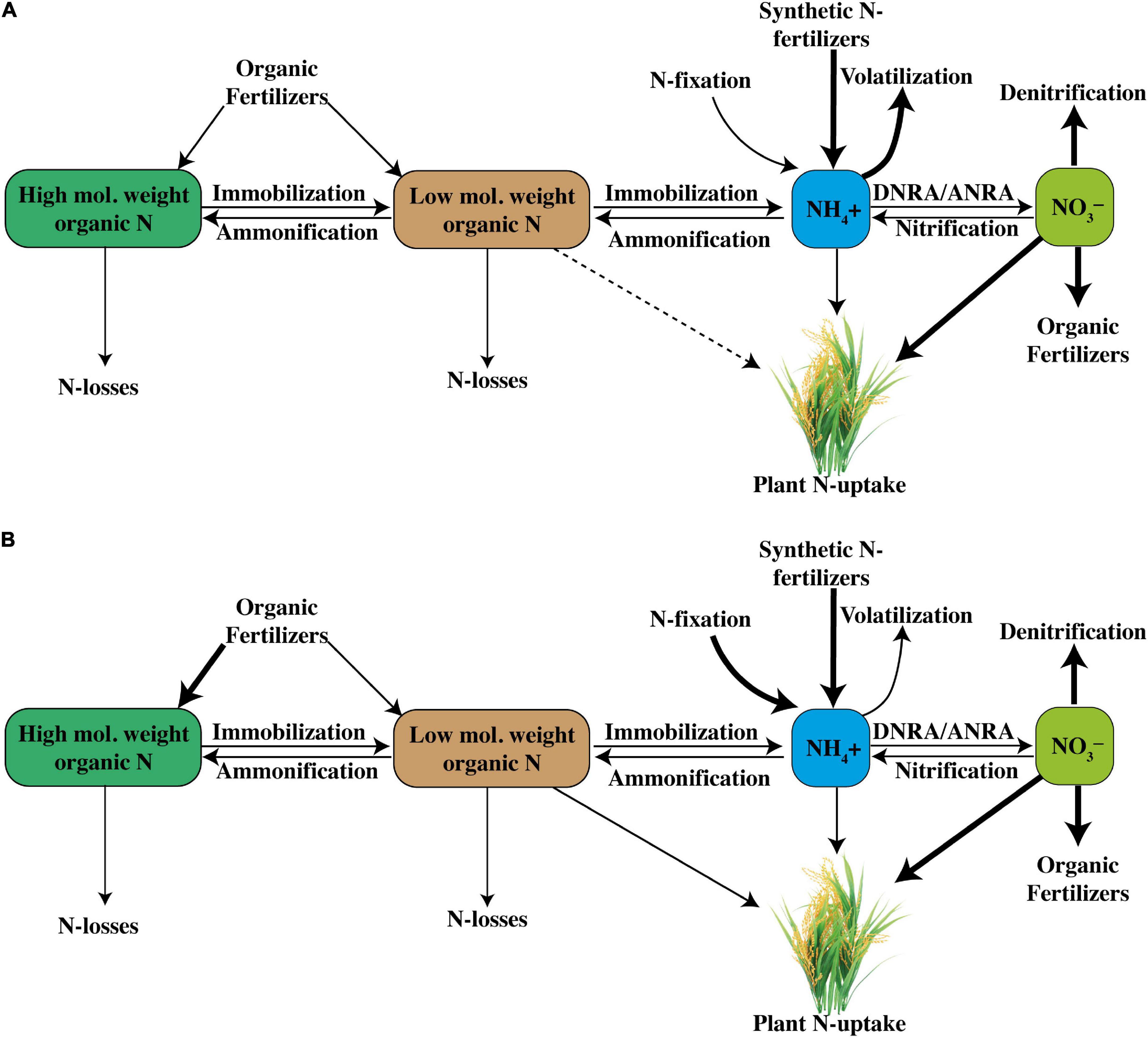
Figure 5. Conjectural comparison of N-cycle under a (A) rice system with higher nitrification rates and a (B) rice system with relatively low nitrification rates (DNRA, dissimilatory NO3– reduction to NH4+; and ANRA, assimilatory NO3– reduction to NH4+).
Conclusion
Importance of Aerobic Rice Systems
Globally, rice systems are faced with the challenges of having higher yields with decreased irrigational water. The sustainability of the flooded rice system is being threatened by scarcity of inputs, such as irrigational water, labor, and energy. Aerobic rice systems can be a valid option to sustain future rice yield under scarce freshwater resources through proper agronomic management measures. One of the main benefits of aerobic rice systems over the conventional is reduction in use of resources, such as irrigations with higher water productivity and less labor cost. Low yield in aerobic rice systems suggests the need to undertake necessary measures in the management of N losses and weeds. A big yield gap between aerobic and conventional rice systems can be reduced under favorable conditions if coupled with proper agronomic management practices in minimizing weed invasion and N losses. If desired soil chemical and physical properties are satisfied, timely sowing, proper stand establishment, and higher grain yield can be ensured under aerobic rice systems unlike under the conventional rice system where land preparation is more laborious.
An aerobic rice system is a production setup where rice is grown under well-drained, non-saturated, and non-puddled soil conditions. Resource use efficiency is very high because of less use of irrigational water during land preparation, no transplantation requirements, and less labor costs from sowing to harvesting. Cultivars that are cultivated in aerobic system must have better yield performance traits of lowland conditions and better drought tolerance characteristics of upland conditions with required root traits. Physiological traits in terms of protein and chlorophyll contents, flowering, relative water contents, osmotic adjustments under drought stress, and enzyme activities are the major attributes that play major role in widespread adaptation of aerobic rice systems. Low NUE is one of the major problems for yield decline and adaptation of aerobic rice systems due to changes in soil nutrient status and difference in microbial activities. Aerobic rice systems undertaking agronomic management measures of applied N fertilizers to improve the abundance and activities of microbial communities important in N cycle may lead to sustained grain yield. This will also help in environmental sustainability in the scenario of climate warming by reducing GHG emission, which is an added benefit of this system.
Key Findings of the Review Article
Nitrogen loss is one of the major issues in both conventional and aerobic rice systems, although in the latter losses are relatively very high. The conventional rice system needs some transformational approaches, such as aerobic rice systems, because of the future projected scarcity of resources. However, the higher rates of N losses in aerobic rice systems threaten the wider adaptation of these transformed rice systems and cause environmental hazards. For widespread adaptation of aerobic rice systems, reducing N losses is necessary by adjustments in microbial communities involved in N cycle via deep insights into pathways and their ecophysiology. Therefore, this study was designed to investigate the ecophysiology of soil microbial communities involved in N-cycle, their diversification, abundance, and activities, and how their activities are affected under different soil conditions. This study discusses the recent discovery of AOA, which widens the knowledge of N cycle and shattered the conventional perspectives for the past 10 decades that ammonia oxidation is only controlled by AOB. Hence, the N cycle in aerobic rice systems requires reconsiderations, as some recent novel developments in N cycling have depicted changes in activities and dominance of AOA rather than AOB under different environments. The conjoint, competitive, and inhibitive microbial interactions need further exploration in conventional and aerobic rice systems to increase overall NUE. This study discussed the mechanisms of how highly active ammonia oxidizers interactively impacting N-cycle and plant N uptake. After understanding the interactive pathways, this study narrowed down several adjustive management measures to limit N-losses that may enhance NUE in aerobic rice system, reducing GHG emissions and environmental hazards.
Ammonia is predominantly found in nature and considered as a substrate to produce NO3–. AOA and AOB are primary ammonia oxidizers that engage in conversion of NH4+ to NO3–, which is the most preferable form to be utilized by the rice plant. This article suggests management measures for fertilizers, soils and crops to adjust the functioning of AOA and AOB and better assimilate soil available N. It also discusses measures to control nitrification rates through NIs and BNIs, measures to enhance NH4+ substrate availability, managing the time, type, and application methods of N-fertilizers, and other measures for ensuring better plant N assimilation and utilization.
Few Open Questions and Future Thrust
Currently, the most important point to be taken is the biochemistry of archaeal ammonia oxidizers and, therefore, its implications for habitat separation from AOB. The estimation of substrate ranges required for AOA and AOB as well as the involvement of substrate range in losses of N can fundamentally help in the understanding of the impacts of archaeal and bacterial ammonia oxidizers on soil biochemical processes. Another issue currently considered not sufficient is the relationship between AOA and AOB and nitrite oxidizers. The mutual dependency of nitrite oxidizers on AOA and AOB is one of the typical examples of the metabolic association of three functional groups of microbes. Therefore, insufficient knowledge of co-dependence of AOA and AOB on nitrite oxidizers is potentially unfortunate. Moreover, the evaluation of spatial and temporal co-occurrence of these communities under different soil conditions is still unstudied. Further research studies are suggested to conduct several cultivation-based experiments to cultivate AOA and AOB under more strictly controlled conditions. It will be helpful for better understanding of the response mechanisms of AOA and AOB to environmental variability under conditions that will allow AOA and AOB for better utilization of their metabolic functions under natural conditions.
Author Contributions
MF, ZM, and MU conceived the idea and collected the relevant literature. MF and MU visualized the figures. MF, ZM, MY, MK, SF, SY, and MU helped in writing the original draft. All authors carefully read, revised, and approved the article for submission.
Funding
The publication of this study was supported by a National Research Foundation (NRF) of Korea grant funded by the Korean Government (MSIT, NRF-2021R1F1A1055482).
Conflict of Interest
The authors declare that the research was conducted in the absence of any commercial or financial relationships that could be construed as a potential conflict of interest.
Publisher’s Note
All claims expressed in this article are solely those of the authors and do not necessarily represent those of their affiliated organizations, or those of the publisher, the editors and the reviewers. Any product that may be evaluated in this article, or claim that may be made by its manufacturer, is not guaranteed or endorsed by the publisher.
Acknowledgments
We acknowledge the research facilities provided by the National Institute for Genomics and Advanced Biotechnology (NIGAB).
References
Abalos, D., Jeffery, S., Sanz-Cobena, A., Guardia, G., and Vallejo, A. (2014). Meta-analysis of the effect of urease and nitrification inhibitors on crop productivity and nitrogen use efficiency. Agric. Ecosyst. Environ. 189, 136–144. doi: 10.1016/j.agee.2014.03.036
Afzal, M., Yu, M., Tang, C., Zhang, L., Muhammad, N., Zhao, H., et al. (2019). The negative impact of cadmium on nitrogen transformation processes in a paddy soil is greater under non-flooding than flooding conditions. Environ. Int. 129, 451–460. doi: 10.1016/J.ENVINT.2019.05.058
Albina, P., Durban, N., Bertron, A., Albrecht, A., Robinet, J.-C., and Erable, B. (2019). Influence of hydrogen electron donor, alkaline pH, and high nitrate concentrations on microbial denitrification: a review. Int. J. Mol. Sci. 20:5163. doi: 10.3390/ijms20205163
Alves, R. J. E., Kerou, M., Zappe, A., Bittner, R., Abby, S. S., Schmidt, H. A., et al. (2019). Ammonia oxidation by the arctic terrestrial thaumarchaeote Candidatus Nitrosocosmicus arcticus is stimulated by increasing temperatures. Front. Microbiol. 10:1571. doi: 10.3389/fmicb.2019.01571
Alves, R. J. E., Wanek, W., Zappe, A., Richter, A., Svenning, M. M., Schleper, C., et al. (2013). Nitrification rates in Arctic soils are associated with functionally distinct populations of ammonia-oxidizing archaea. ISME J. 7, 1620–1631. doi: 10.1038/ismej.2013.35
Amoo, A. E., and Babalola, O. O. (2017). Ammonia-oxidizing microorganisms: key players in the promotion of plant growth. J. soil Sci. Plant Nutr. 17, 935–947. doi: 10.4067/S0718-95162017000400008
Awan, M. I., Bastiaans, L., van Oort, P., Ahmad, R., Ashraf, M. Y., and Meinke, H. (2014). Nitrogen use and crop performance of rice under aerobic conditions in a semiarid subtropical environment. Agron. J. 106, 199–211. doi: 10.2134/agronj2013.0262
Balasubramanian, R., Smith, S. M., Rawat, S., Yatsunyk, L. A., Stemmler, T. L., and Rosenzweig, A. C. (2010). Oxidation of methane by a biological dicopper centre. Nature 465, 115–119. doi: 10.1038/nature08992
Balmaseda, M. A., Trenberth, K. E., and Källén, E. (2013). Distinctive climate signals in reanalysis of global ocean heat content. Geophys. Res. Lett. 40, 1754–1759. doi: 10.1002/grl.50382
Banning, N. C., Maccarone, L. D., Fisk, L. M., and Murphy, D. V. (2015). Ammonia-oxidising bacteria not archaea dominate nitrification activity in semi-arid agricultural soil. Sci. Rep. 5:11146. doi: 10.1038/srep11146
Barth, G., Otto, R., Almeida, R. F., Cardoso, E. J. B. N., Cantarella, H., Vitti, G. C., et al. (2020). Conversion of ammonium to nitrate and abundance of ammonium-oxidizing-microorganism in tropical soils with nitrification inhibitor. Sci. Agric. 77:e20180370. doi: 10.1590/1678-992x-2018-0370
Bartossek, R., Spang, A., Weidler, G., Lanzen, A., and Schleper, C. (2012). Metagenomic analysis of ammonia-oxidizing archaea affiliated with the soil group. Front. Microbiol. 3:208. doi: 10.3389/fmicb.2012.00208
Beam, J. P., Jay, Z. J., Kozubal, M. A., and Inskeep, W. P. (2014). Niche specialization of novel Thaumarchaeota to oxic and hypoxic acidic geothermal springs of Yellowstone National Park. ISME J. 8, 938–951. doi: 10.1038/ismej.2013.193
Belder, P., Bouman, B. A. M., Spiertz, J. H. J., Peng, S., Castañeda, A. R., and Visperas, R. M. (2005). Crop performance, nitrogen and water use in flooded and aerobic rice. Plant Soil 273, 167–182. doi: 10.2307/24125208
Bellucci, M., Ofiţeru, I. D., Graham, D. W., Head, I. M., and Curtis, T. P. (2011). Low-dissolved-oxygen nitrifying systems exploit ammonia-oxidizing bacteria with unusually high yields. Appl. Environ. Microbiol. 77, 7787–7796. doi: 10.1128/AEM.00330-11
Beman, J. M., Popp, B. N., and Francis, C. A. (2008). Molecular and biogeochemical evidence for ammonia oxidation by marine Crenarchaeota in the Gulf of California. ISME J. 2, 429–441. doi: 10.1038/ismej.2007.118
Bennett, K., Sadler, N. C., Wright, A. T., Yeager, C., and Hyman, M. R. (2016). Activity-based protein profiling of ammonia monooxygenase in Nitrosomonas europaea. Appl. Environ. Microbiol. 82, 2270–2279. doi: 10.1128/AEM.03556-15
Bernhard, A. E., Landry, Z. C., Blevins, A., de la Torre, J. R., Giblin, A. E., and Stahl, D. A. (2010). Abundance of ammonia-oxidizing archaea and bacteria along an estuarine salinity gradient in relation to potential nitrification rates. Appl. Environ. Microbiol. 76, 1285–1289. doi: 10.1128/AEM.02018-09
Bertagnolli, A. D., McCalmont, D., Meinhardt, K. A., Fransen, S. C., Strand, S., Brown, S., et al. (2016). Agricultural land usage transforms nitrifier population ecology. Environ. Microbiol. 18, 1918–1929. doi: 10.1111/1462-2920.13114
Black, E. M., Chimenti, M. S., and Just, C. L. (2019). Metagenomic analysis of nitrogen-cycling genes in upper Mississippi river sediment with mussel assemblages. Microbiol. Open 8:e00739. doi: 10.1002/mbo3.739
Bollmann, A., Sedlacek, C. J., Norton, J., Laanbroek, H. J., Suwa, Y., Stein, L. Y., et al. (2013). Complete genome sequence of Nitrosomonas sp. Is79, an ammonia oxidizing bacterium adapted to low ammonium concentrations. Stand. Genomic Sci. 73, 469–482. doi: 10.4056/SIGS.3517166
Bosco, T., Bertiller, M. B., and Carrera, A. L. (2015). Micro-environmental conditions affect grass and shrub seedling emergence in denuded areas of the arid Patagonian Monte, Argentina. Flora Morphol. Distrib. Funct. Ecol. Plants 210, 66–71. doi: 10.1016/j.flora.2014.10.001
Bottomley, P. J., Taylor, A. E., and Myrold, D. D. (2012). A consideration of the relative contributions of different microbial subpopulations to the soil N cycle. Front. Microbiol. 3:373. doi: 10.3389/fmicb.2012.00373
Bouman, B. A., and Tuong, T. (2001). Field water management to save water and increase its productivity in irrigated lowland rice. Agric. Water Manage. 49, 11–30. doi: 10.1016/S0378-3774(00)00128-1
Bouman, B. A. M., Peng, S., Castañeda, A. R., and Visperas, R. M. (2005). Yield and water use of irrigated tropical aerobic rice systems. Agric. Water Manage. 74, 87–105. doi: 10.1016/j.agwat.2004.11.007
Bouman, B. A. M., Yang, X., Wang, H., Wang, Z., Zhao, J., and Chen, B. (2006). Performance of aerobic rice varieties under irrigated conditions in North China. Field Crop. Res. 97, 53–65. doi: 10.1016/J.FCR.2005.08.015
Bouskill, N. J., Tang, J., Riley, W. J., and Brodie, E. L. (2012). Trait-based representation of biological nitrification: model development, testing, and predicted community composition. Front. Microbiol. 3:364. doi: 10.3389/fmicb.2012.00364
Bowles, T. M., Atallah, S. S., Campbell, E. E., Gaudin, A. C. M., Wieder, W. R., and Grandy, A. S. (2018). Addressing agricultural nitrogen losses in a changing climate. Nat. Sustain. 1, 399–408. doi: 10.1038/s41893-018-0106-0
Bueno, E., Mania, D., Frostegard, Ç, Bedmar, E. J., Bakken, L. R., and Delgado, M. J. (2015). Anoxic growth of Ensifer meliloti 1021 by N2O-reduction, a potential mitigation strategy. Front. Microbiol. 6:537. doi: 10.3389/fmicb.2015.00537
Buresh, R. J., Reddy, K. R., and van Kessel, C. (2008). “Nitrogen transformations in submerged soils,” in Nitrogen in Agricultural Systems. Agronomy Monograph 49, eds J. S. Schepers and Raun, W. R. (ASA: Madison, WI), 401–436. doi: 10.2134/agronmonogr49.c11
Burton, S. A. Q., and Prosser, J. I. (2001). Autotrophic ammonia oxidation at low pH through urea hydrolysis. Appl. Environ. Microbiol. 67, 2952–2957. doi: 10.1128/AEM.67.7.2952-2957.2001
Butler, J. H., and Montzka, S. A. (2015). The NOAA Annual Greenhouse Gas Index (AGGI). Available online at: http://www.esrl.noaa.gov/gmd/aggi/ (accessed March 25, 2022).
Byrnes, R. C., Nùñez, J., Arenas, L., Rao, I., Trujillo, C., Alvarez, C., et al. (2017). Biological nitrification inhibition by Brachiaria grasses mitigates soil nitrous oxide emissions from bovine urine patches. Soil Biol. Biochem. 107, 156–163. doi: 10.1016/J.SOILBIO.2016.12.029
Caranto, J. D., and Lancaster, K. M. (2017). Nitric oxide is an obligate bacterial nitrification intermediate produced by hydroxylamine oxidoreductase. Proc. Natl. Acad. Sci. U.S.A. 114, 8217–8222. doi: 10.1073/pnas.1704504114
Chen, H., Yue, Y., Jin, W., Zhou, X., Wang, Q., Gao, S. H., et al. (2017). Enrichment and characteristics of ammonia-oxidizing archaea in wastewater treatment process. Chem. Eng. J. 323, 465–472. doi: 10.1016/j.cej.2017.04.130
Cheng, Y., Wang, J., Zhang, J. B., Wang, S. Q., and Cai, Z. C. (2015). The different temperature sensitivity of gross N transformations between the coniferous and broad-leaved forests in subtropical China. Soil Sci. Plant Nutr. 61, 506–515. doi: 10.1080/00380768.2014.994187
Coskun, D., Britto, D. T., Shi, W., and Kronzucker, H. J. (2017a). How plant root exudates shape the nitrogen cycle. Trends Plant Sci. 22, 661–673. doi: 10.1016/j.tplants.2017.05.004
Coskun, D., Britto, D. T., Shi, W., and Kronzucker, H. J. (2017b). Nitrogen transformations in modern agriculture and the role of biological nitrification inhibition. Nat. Plants 3:17074. doi: 10.1038/nplants.2017.74
Cui, Y., Zhang, W., Lin, X., Xu, S., Xu, J., and Li, Z. (2018). Simultaneous improvement and genetic dissection of drought tolerance using selected breeding populations of rice. Front. Plant Sci. 9:320. doi: 10.3389/fpls.2018.00320
Daebeler, A., Abell, G. C. J., Bodelier, P. L. E., Bodrossy, L., Frampton, D. M. F., Hefting, M. M., et al. (2012). Archaeal dominated ammonia-oxidizing communities in Icelandic grassland soils are moderately affected by long-term N fertilization and geothermal heating. Front. Microbiol. 3:352. doi: 10.3389/fmicb.2012.00352
Daebeler, A., Herbold, C. W., Vierheilig, J., Sedlacek, C. J., Pjevac, P., Albertsen, M., et al. (2018). Cultivation and genomic analysis of “Candidatus Nitrosocaldus islandicus,” an obligately thermophilic, ammonia-oxidizing thaumarchaeon from a hot spring biofilm in Graendalur Valley, Iceland. Front. Microbiol. 9:193. doi: 10.3389/fmicb.2018.00193
Djaman, K., Mel, V. C., Diop, L., Sow, A., El-Namaky, R., Manneh, B., et al. (2018). Effects of alternatewetting and drying irrigation regime and nitrogen fertilizer on yield and nitrogen use efficiency of irrigated rice in the Sahel. Water 10:711. doi: 10.3390/w10060711
Erguder, T. H., Boon, N., Wittebolle, L., Marzorati, M., and Verstraete, W. (2009). Environmental factors shaping the ecological niches of ammonia-oxidizing archaea. FEMS Microbiol. Rev. 33, 855–869. doi: 10.1111/j.1574-6976.2009.00179.x
FAO (2016). OECD-FAO Agricultural Outlook 2017-2026 SpECiAl FOCuS: SOuthEASt ASiA. Available online at: https://www.oecd-ilibrary.org/docserver/agr_outlook-2017-en.pdf?expires=1525270117&id=id&accname=guest&checksum=9D11B4396CD77C729311E7ACDFAFB1F8 (accessed March 29, 2022).
FAO (2017). The Future of Food and Agriculture: Trends and Challenges. Rome: FAO. doi: 10.4161/chan.4.6.12871
Farquharson, R. (2016). Nitrification rates and associated nitrous oxide emissions from agricultural soils – a synopsis. Soil Res. 54, 469–480. doi: 10.1071/SR15304
Fowler, D., Coyle, M., Skiba, U., Sutton, M. A., Cape, J. N., Reis, S., et al. (2013). The global nitrogen cycle in the twenty-first century. Philos. Trans. R. Soc. B Biol. Sci. 368:20130164. doi: 10.1098/rstb.2013.0164
Fowler, D., Steadman, C. E., Stevenson, D., Coyle, M., Rees, R. M., Skiba, U. M., et al. (2015). Effects of global change during the 21st century onthe nitrogen cycle. Atmos. Chem. Phys. 15, 13849–13893. doi: 10.5194/ACP-15-13849-2015
French, E., and Bollmann, A. (2015). Freshwater ammonia-oxidizing archaea retain amoA mRNA and 16S rRNA during ammonia starvation. Life 5, 1396–1404. doi: 10.3390/life5021396
Froes de Borja Reis, A. F., Estevam Munhoz de Almeida, R., Cocco Lago, B., Trivelin, P. C., Linquist, B., et al. (2018). Aerobic rice system improves water productivity, nitrogen recovery and crop performance in Brazilian weathered lowland soil. Field Crop. Res. 218, 59–68. doi: 10.1016/j.fcr.2018.01.002
Fuhrmann, I., He, Y., Lehndorff, E., Brüggemann, N., Amelung, W., Wassmann, R., et al. (2018). Nitrogen fertilizer fate after introducing maize and upland-rice into continuous paddy rice cropping systems. Agric. Ecosyst. Environ. 258, 162–171. doi: 10.1016/J.AGEE.2018.02.021
Gao, J., Fan, X., Wu, G., Li, T., and Pan, K. (2016). Changes of abundance and diversity of ammonia-oxidizing archaea (AOA) and bacteria (AOB) in three nitrifying bioreactors with different ammonia concentrations. Desalin. Water Treat. 57, 21463–21475. doi: 10.1080/19443994.2015.1123196
Guardia, G., Marsden, K. A., Vallejo, A., Jones, D. L., and Chadwick, D. R. (2018a). Determining the influence of environmental and edaphic factors on the fate of the nitrification inhibitors DCD and DMPP in soil. Sci. Total Environ. 624, 1202–1212. doi: 10.1016/j.scitotenv.2017.12.250
Guardia, G., Sanz-Cobena, A., Sanchez-Martín, L., Fuertes-Mendizábal, T., González-Murua, C., Álvarez, J. M., et al. (2018b). Urea-based fertilization strategies to reduce yield-scaled N oxides and enhance bread-making quality in a rainfed Mediterranean wheat crop. Agric. Ecosyst. Environ. 265, 421–431. doi: 10.1016/J.AGEE.2018.06.033
Han, S., Li, X., Luo, X., Wen, S., Chen, W., and Huang, Q. (2018). Nitrite-oxidizing bacteria community composition and diversity are influenced by fertilizer regimes, but are independent of the soil aggregate in acidic subtropical red soil. Front. Microbiol. 9:885. doi: 10.3389/fmicb.2018.00885
Han, W.-Y., Xu, J.-M., Yi, X.-Y., and Lin, Y.-D. (2012). Net and gross nitrification in tea soils of varying productivity and their adjacent forest and vegetable soils. Soil Sci. Plant Nutr. 58, 173–182. doi: 10.1080/00380768.2012.664783
Hawkes, C. V., DeAngelis, K. M., and Firestone, M. K. (2007). “Root interactions with soil microbial communities and processes,” in The Rhizosphere, eds Z. G. Cardon and Whitbeck, J. L. (Cambridge, MA: Academic Press), 1–29. doi: 10.1016/B978-012088775-0/50003-3
Hayatsu, M., Tago, K., Uchiyama, I., Toyoda, A., Wang, Y., Shimomura, Y., et al. (2017). An acid-tolerant ammonia-oxidizing γ-proteobacterium from soil. ISME J. 11, 1130–1141. doi: 10.1038/ismej.2016.191
He, H., Zhen, Y., Mi, T., Fu, L., and Yu, Z. (2018). Ammonia-oxidizing archaea and bacteria differentially contribute to ammonia oxidation in sediments from adjacent waters of Rushan Bay, China. Front. Microbiol. 9:116. doi: 10.3389/fmicb.2018.00116
He, W., Dutta, B., Grant, B. B., Chantigny, M. H., Hunt, D., Bittman, S., et al. (2019). Assessing the effects of manure application rate and timing on nitrous oxide emissions from managed grasslands under contrasting climate in Canada. Sci. Total Environ. 716:135374. doi: 10.1016/J.SCITOTENV.2019.135374
Heinze, J., Gensch, S., Weber, E., and Joshi, J. (2016). Soil temperature modifies effects of soil biota on plant growth. J. Plant Ecol. 10:rtw097. doi: 10.1093/jpe/rtw097
Herbold, C. W., Lehtovirta-Morley, L. E., Jung, M.-Y., Jehmlich, N., Hausmann, B., Han, P., et al. (2017). Ammonia-oxidising archaea living at low pH: insights from comparative genomics. Environ. Microbiol. 19, 4939–4952. doi: 10.1111/1462-2920.13971
Hill, P. W., Garnett, M. H., Farrar, J., Iqbal, Z., Khalid, M., Soleman, N., et al. (2015). Living roots magnify the response of soil organic carbon decomposition to temperature in temperate grassland. Glob. Change Biol. 21, 1368–1375. doi: 10.1111/gcb.12784
Hink, L., Gubry-Rangin, C., Nicol, G. W., and Prosser, J. I. (2018). The consequences of niche and physiological differentiation of archaeal and bacterial ammonia oxidisers for nitrous oxide emissions. ISME J. 12, 1084–1093. doi: 10.1038/s41396-017-0025-5
Hoffman, F. M., Randerson, J. T., Arora, V. K., Bao, Q., Cadule, P., Ji, D., et al. (2014). Causes and implications of persistent atmospheric carbon dioxide biases in Earth System Models. J. Geophys. Res. Biogeosci. 119, 141–162. doi: 10.1002/2013JG002381
Hu, H.-W., and He, J.-Z. (2018). Manipulating the soil microbiome for improved nitrogen management. Microbiol. Aust. 39, 24–27. doi: 10.1071/ma18007
Hu, H.-W., Zhang, L.-M., Yuan, C.-L., Zheng, Y., Wang, J.-T., Chen, D., et al. (2015). The large-scale distribution of ammonia oxidizers in paddy soils is driven by soil pH, geographic distance, and climatic factors. Front. Microbiol. 6:938. doi: 10.3389/fmicb.2015.00938
Hu, Z., Lotti, T., van Loosdrecht, M., and Kartal, B. (2013). Nitrogen removal with the anaerobic ammonium oxidation process. Biotechnol. Lett. 35, 1145–1154. doi: 10.1007/s10529-013-1196-4
Huang, P., Shen, F., Abbas, A., Wang, H., Du, Y., Du, D., et al. (2022). Effects of different nitrogen forms and competitive treatments on the growth and antioxidant system of Wedelia trilobata and Wedelia chinensis under high nitrogen concentrations. Front. Plant Sci. 13:851099. doi: 10.3389/fpls.2022.851099
Huang, R., Zhao, D., Zeng, J., Luo, J., Shen, F., Cao, X., et al. (2016). Abundance and community composition of ammonia oxidizers in rhizosphere sediment of two submerged macrophytes. J. Freshw. Ecol. 31, 407–419. doi: 10.1080/02705060.2016.1187208
Huang, Y., and Gerber, S. (2015). Global soil nitrous oxide emissions in a dynamic carbon-nitrogen model. Biogeosciences 12, 6405–6427. doi: 10.5194/bg-12-6405-2015
Hwalla, N., El Labban, S., and Bahn, R. A. (2016). Nutrition security is an integral component of food security. Front. Life Sci. 9, 167–172. doi: 10.1080/21553769.2016.1209133
Ihara, H., Kato, N., Takahashi, S., and Nagaoka, K. (2014). Effect of soil solarization on subsequent nitrification activity at elevated temperatures. Soil Sci. Plant Nutr. 60, 824–831. doi: 10.1080/00380768.2014.947233
Inselsbacher, E., and Näsholm, T. (2012). The below-ground perspective of forest plants: soil provides mainly organic nitrogen for plants and mycorrhizal fungi. New Phytol. 195, 329–334. doi: 10.1111/j.1469-8137.2012.04169.x
IPCC (2015). “Agriculture, forestry and other land use (AFOLU),” in Climate Change 2014: Mitigation of Climate Change. Contribution of Working Group III to the Fifth Assessment Report of the Intergovernmental Panel on Climate Change, eds O. Edenhofer, R. Pichs-Madruga, Y. Sokona, E. Farahani, S. Kadner, K. Seyboth, et al. (Cambridge: Cambridge University Press), 811–922. doi: 10.1017/cbo9781107415416.017
Ishii, S., Ikeda, S., Minamisawa, K., and Senoo, K. (2011). Nitrogen cycling in rice paddy environments: past achievements and future challenges. Microbes Environ. 26, 282–292. doi: 10.1264/jsme2.ME11293
Ishii, S., Yamamoto, M., Kikuchi, M., Oshima, K., Hattori, M., Otsuka, S., et al. (2009). Microbial populations responsive to denitrification-inducing conditions in rice paddy soil, as revealed by comparative 16S rRNA gene analysis. Appl. Environ. Microbiol. 75, 7070–7078. doi: 10.1128/AEM.01481-09
Jain, M. C., Kumar, S., Wassmann, R., Mitra, S., Singh, S. D., Singh, J. P., et al. (2000). Methane emissions from irrigated rice fields in northern India (New Delhi). Nutr. Cycl. Agroecosyst. 58, 75–83. doi: 10.1023/A:1009882216720
Jang, J., Anderson, E. L., Venterea, R. T., Sadowsky, M. J., Rosen, C. J., Feyereisen, G. W., et al. (2019). Denitrifying bacteria active in woodchip bioreactors at low-temperature conditions. Front. Microbiol. 10:635. doi: 10.3389/fmicb.2019.00635
Javed, T., Afzal, I., and Mauro, R. P. (2021). Seed coating in direct seeded rice: an innovative and sustainable approach to enhance grain yield and weed management under submerged conditions. Sustainability 13: 2190.
Javed, T., Singhal, R., Shabbir, R., Kumar, P., Shah, A. N., Jinger, D., et al. (2022). Recent advances in agronomic and physio-molecular approaches for improving nitrogen use efficiency in crop plants. Front. Plant Sci. 13:877544. doi: 10.3389/fpls.2022.877544
Jia, Y., Wang, J., Qu, Z., Zou, D., Sha, H., Liu, H., et al. (2019). Effects of low water temperature during reproductive growth on photosynthetic production and nitrogen accumulation in rice. Field Crop. Res. 242:107587. doi: 10.1016/J.FCR.2019.107587
Jia, Z., and Conrad, R. (2009). Bacteria rather than archaea dominate microbial ammonia oxidation in an agricultural soil. Environ. Microbiol. 11, 1658–1671. doi: 10.1111/j.1462-2920.2009.01891.x
Jiang, Y., Jin, C., and Sun, B. (2014). Soil aggregate stratification of nematodes and ammonia oxidizers affects nitrification in an acid soil. Environ. Microbiol. 16, 3083–3094. doi: 10.1111/1462-2920.12339
Joshi, E., Kumar, D., Lal, B., Nepalia, V., Gautam, P., and Vyas, A. (2013). Management of direct seeded rice for enhanced resource – use efficiency. Plant Knowl. J. 2, 119–134.
Kadiyala, M. D. M., Mylavarapu, R. S., Li, Y. C., Reddy, G. B., and Reddy, M. D. (2012). Impact of aerobic rice cultivation on growth, yield, and water productivity of rice–maize rotation in semiarid tropics. Agron. J. 104, 1757–1765. doi: 10.2134/agronj2012.0148
Kanter, D., Mauzerall, D. L., Ravishankara, A. R., Daniel, J. S., Portmann, R. W., Grabiel, P. M., et al. (2013). A post-Kyoto partner: considering the stratospheric ozone regime as a tool to manage nitrous oxide. Proc. Natl. Acad. Sci. U.S.A. 110, 4451–4457. doi: 10.1073/PNAS.1222231110/-/DCSUPPLEMENTAL
Kato, Y., and Katsura, K. (2014). Rice adaptation to aerobic soils: physiological considerations and implications for agronomy. Plant Prod. Sci. 17, 1–12. doi: 10.1626/pps.17.1
Ke, X., and Lu, Y. (2012). Adaptation of ammonia-oxidizing microorganisms to environment shift of paddy field soil. FEMS Microbiol. Ecol. 80, 87–97. doi: 10.1111/j.1574-6941.2011.01271.x
Keating, B. A., Carberry, P. S., Bindraban, P. S., Asseng, S., Meinke, H., and Dixon, J. (2010). Eco-efficient agriculture: concepts, challenges, and opportunities. Crop Sci. 50, S109–S119. doi: 10.2135/cropsci2009.10.0594
Kerou, M., Offre, P., Valledor, L., Abby, S. S., Melcher, M., Nagler, M., et al. (2016). Proteomics and comparative genomics of Nitrososphaera viennensis reveal the core genome and adaptations of archaeal ammonia oxidizers. Proc. Natl. Acad. Sci. U.S.A. 113, E7937–E7946. doi: 10.1073/pnas.1601212113
Khan, N. U., Khan, A. A., Goheer, M. A., Shafique, I., Hussain, S., Hussain, S., et al. (2021). Effect of zero and minimum tillage on cotton productivity and soil characteristics under different nitrogen application rates. Sustainability 13:13753.
Koch, H., van Kessel, M. A. H. J., and Lücker, S. (2019). Complete nitrification: insights into the ecophysiology of comammox Nitrospira. Appl. Microbiol. Biotechnol. 103, 177–189. doi: 10.1007/s00253-018-9486-3
Kozlowski, J. A., Stieglmeier, M., Schleper, C., Klotz, M. G., and Stein, L. Y. (2016). Pathways and key intermediates required for obligate aerobic ammonia-dependent chemolithotrophy in bacteria and Thaumarchaeota. ISME J. 10, 1836–1845. doi: 10.1038/ismej.2016.2
Kumar, R., Pareek, N., Kumar, U., Javed, T., Alhuqail, A., Rathore, V., et al. (2022). Coupling effects of nitrogen and irrigation levels on growth attributes, nitrogen use efficiency, and economics of cotton. Front. Plant Sci. 13:890181. doi: 10.3389/fpls.2022.890181
Kumazawa, K. (2002). Nitrogen fertilization and nitrate pollution in groundwater in Japan: present status and measures for sustainable agriculture. Nutr. Cycl. Agroecosyst. 63, 129–137. doi: 10.1023/A:1021198721003
Kuypers, M. M. M., Marchant, H. K., and Kartal, B. (2018). The microbial nitrogen-cycling network. Nat. Rev. Microbiol. 16, 263–276. doi: 10.1038/nrmicro.2018.9
Kyveryga, P. M., and Blackmer, A. M. (2018). “Effects of soil pH on nitrification and losses of fall-applied anhydrous ammonia,” in Proceedings of the Integrated Crop Management Conference (Ames, IA: IOWA State University), 545–551. doi: 10.31274/icm-180809-709
Laanbroek, H. J., and Bär-Gilissen, M. J. (2002). Weakened activity of starved ammonia-oxidizing bacteria by the presence of pre-activated Nitrobacter winogradskyi. Microbes Environ. 17, 122–127. doi: 10.1264/jsme2.17.122
Lawton, T. J., Bowen, K. E., Sayavedra-Soto, L. A., Arp, D. J., and Rosenzweig, A. C. (2013). Characterization of a nitrite reductase involved in nitrifier denitrification. J. Biol. Chem. 288, 25575–25583. doi: 10.1074/jbc.M113.484543
Leininger, S., Urich, T., Schloter, M., Schwark, L., Qi, J., Nicol, G. W., et al. (2006). Archaea predominate among ammonia-oxidizing prokaryotes in soils. Nature 442, 806–809. doi: 10.1038/nature04983
Li, B., Irvin, S., and Baker, K. (2007). The variation of nitrifying bacterial population sizes in a sequencing batch reactor (SBR) treating low, mid, high concentrated synthetic wastewater. J. Environ. Eng. Sci. 6, 651–663. doi: 10.1139/S07-008
Li, C. (2007). Quantifying greenhouse gas emissions from soils: scientific basis and modeling approach. Soil Sci. Plant Nutr. 53, 344–352. doi: 10.1111/j.1747-0765.2007.00133.x
Li, Y., Chapman, S. J., Nicol, G. W., and Yao, H. (2018c). Nitrification and nitrifiers in acidic soils. Soil Biol. Biochem. 116, 290–301. doi: 10.1016/j.soilbio.2017.10.023
Li, P.-N., Herrmann, J., Tolar, B. B., Poitevin, F., Ramdasi, R., Bargar, J. R., et al. (2018b). Nutrient transport suggests an evolutionary basis for charged archaeal surface layer proteins. ISME J. 12, 2389–2402. doi: 10.1038/s41396-018-0191-0
Li, M., Du, C., Liu, J., Quan, X., Lan, M., and Li, B. (2018a). Mathematical modeling on the nitrogen removal inside the membrane-aerated biofilm dominated by ammonia-oxidizing archaea (AOA): effects of temperature, aeration pressure and COD/N ratio. Chem. Eng. J. 338, 680–687. doi: 10.1016/j.cej.2018.01.040
Li, Y., Huang, L., Zhang, H., Wang, M., and Liang, Z. (2017). Assessment of ammonia volatilization losses and nitrogen utilization during the rice growing season in alkaline salt-affected soils. Sustainability 9:132. doi: 10.3390/su9010132
Lian, Y., Xu, M., Zhong, Y., Yang, Y., Chen, F., and Guo, J. (2014). Ammonia oxidizers in a pilot-scale multilayer rapid infiltration system for domestic wastewater treatment. PLoS One 9:e114723. doi: 10.1371/journal.pone.0114723
Liu, J., Yu, Z., Yao, Q., Sui, Y., Shi, Y., Chu, H., et al. (2018). Ammonia-oxidizing archaea show more distinct biogeographic distribution patterns than ammonia-oxidizing bacteria across the black soil zone of Northeast China. Front. Microbiol. 9:171. doi: 10.3389/fmicb.2018.00171
Liu, S., Han, P., Hink, L., Prosser, J. I., Wagner, M., and Brüggemann, N. (2017). Abiotic conversion of extracellular NH2 OH contributes to N2O emission during ammonia oxidation. Environ. Sci. Technol. 51, 13122–13132. doi: 10.1021/acs.est.7b02360
Liu, X., Wang, H., Zhou, J., Hu, F., Zhu, D., Chen, Z., et al. (2016). Effect of N fertilization pattern on rice yield, N use efficiency and fertilizer-N fate in the Yangtze River Basin, China. PLoS One 11:e0166002. doi: 10.1371/journal.pone.0166002
Liu, Y., Liu, Y., Zhou, H., Li, L., Zheng, J., Zhang, X., et al. (2016). Abundance, composition and activity of denitrifier communities in metal polluted paddy soils. Sci. Rep. 6:19086. doi: 10.1038/srep19086
Lorenz, C., Kunstmann, H., Lorenz, C., and Kunstmann, H. (2012). The hydrological cycle in three state-of-the-art reanalyses: intercomparison and performance analysis. J. Hydrometeorol. 13, 1397–1420. doi: 10.1175/JHM-D-11-088.1
Lu, L., Han, W., Zhang, J., Wu, Y., Wang, B., Lin, X., et al. (2012). Nitrification of archaeal ammonia oxidizers in acid soils is supported by hydrolysis of urea. ISME J. 6, 1978–1984. doi: 10.1038/ismej.2012.45
Maeda, K., Hanajima, D., Toyoda, S., Yoshida, N., Morioka, R., and Osada, T. (2011). Microbiology of nitrogen cycle in animal manure compost. Microb. Biotechnol. 4, 700–709. doi: 10.1111/j.1751-7915.2010.00236.x
Malik, A. A., Puissant, J., Buckeridge, K. M., Goodall, T., Jehmlich, N., Chowdhury, S., et al. (2018). Land use driven change in soil pH affects microbial carbon cycling processes. Nat. Commun. 9:3591. doi: 10.1038/s41467-018-05980-1
Marusenko, Y., Bates, S. T., Anderson, I., Johnson, S. L., Soule, T., and Garcia-Pichel, F. (2013). Ammonia-oxidizing archaea and bacteria are structured by geography in biological soil crusts across North American arid lands. Ecol. Process 2:9. doi: 10.1186/2192-1709-2-9
Marusenko, Y., Garcia-Pichel, F., and Hall, S. J. (2015). Ammonia-oxidizing archaea respond positively to inorganic nitrogen addition in desert soils. FEMS Microbiol. Ecol. 91, 1–11. doi: 10.1093/femsec/fiu023
Meena, H. M., Sachdev, M. S., Manjaiah, K. M., and Dotaniya, M. L. (2014). Nitrification inhibition potential of Brachiaria humidicola. Natl. Acad. Sci. Lett. 37, 113–116. doi: 10.1007/s40009-013-0216-1
Meier, I. C., Finzi, A. C., and Phillips, R. P. (2017). Root exudates increase N availability by stimulating microbial turnover of fast-cycling N pools. Soil Biol. Biochem. 106, 119–128. doi: 10.1016/j.soilbio.2016.12.004
Menéndez, S., Barrena, I., Setien, I., González-Murua, C., and Estavillo, J. M. (2012). Efficiency of nitrification inhibitor DMPP to reduce nitrous oxide emissions under different temperature and moisture conditions. Soil Biol. Biochem. 53, 82–89. doi: 10.1016/j.soilbio.2012.04.026
Millar, N., Doll, J. E., and Robertson, G. P. (2014). Management of Nitrogen Fertilizer To Reduce Nitrous Oxide (N2O) Emissions From Field Crops – Climate Change and Agriculture Fact Sheet Series. Available online at: http://www.multisward.eu/multisward/Media/fichiers/Delivrables/2014/D3.6 (accessed April 2, 2022).
Morris, T. F., Murrell, T. S., Beegle, D. B., Camberato, J. J., Ferguson, R. B., Grove, J., et al. (2018). Strengths and limitations of nitrogen rate recommendations for corn and opportunities for improvement. Agron. J. 110, 1–37. doi: 10.2134/agronj2017.02.0112
Naher, U. A., Sarker, I. U., Jahan, A., Maniruzzaman, M. D., Choudhury, A. K., Kalra, N., et al. (2019). Nutrient mineralization and soil biology as influenced by temperature and fertilizer management practices. Sains Malays. 48, 735–744. doi: 10.17576/jsm-2019-4804-05
Nelson, K., Motavalli, P., and Nathan, M. (2014). Nitrogen fertilizer sources and application timing affects wheat and inter-seeded red clover yields on claypan soils. Agronomy 4, 497–513. doi: 10.3390/agronomy4040497
Nie, L., Peng, S., Bouman, B. A. M., Huang, J., Cui, K., Visperas, R. M., et al. (2009). Alleviating soil sickness caused by aerobic monocropping: responses of aerobic rice to various nitrogen sources. Soil Sci. Plant Nutr. 55, 150–159. doi: 10.1111/j.1747-0765.2008.00338.x
Nie, L., Peng, S., Chen, M., Shah, F., Huang, J., Cui, K., et al. (2012). Aerobic rice for water-saving agriculture. a review. Agron. Sustain. Dev. 32, 411–418. doi: 10.1007/s13593-011-0055-8
Nishizawa, T., Uei, Y., Tago, K., Isobe, K., Otsuka, S., and Senoo, K. (2013). Taxonomic composition of denitrifying bacterial isolates is different among three rice paddy field soils in Japan. Soil Sci. Plant Nutr. 59, 305–310. doi: 10.1080/00380768.2013.773256
Norton, J. M. (2014). “Diversity and environmental distribution of ammonia-oxidizing bacteria,” in Nitrification, eds B. B. Ward, D. J. Arp, and M. G. Klotz (Hoboken, NJ: Wiley), 39–55. doi: 10.1128/9781555817145.ch3
Norton, J., and Ouyang, Y. (2019). Controls and adaptive management of nitrification in agricultural soils. Front. Microbiol. 10:1931. doi: 10.3389/fmicb.2019.01931
Norton, J. M., and Stark, J. M. (2011). Regulation and measurement of nitrification in terrestrial systems. Methods Enzymol. 486, 343–368. doi: 10.1016/B978-0-12-381294-0.00015-8
Nottingham, A. T., Bååth, E., Reischke, S., Salinas, N., and Meir, P. (2019). Adaptation of soil microbial growth to temperature: using a tropical elevation gradient to predict future changes. Glob. Change Biol. 25, 827–838. doi: 10.1111/gcb.14502
Nuñez, J., Arevalo, A., Karwat, H., Egenolf, K., Miles, J., Chirinda, N., et al. (2018). Biological nitrification inhibition activity in a soil-grown biparental population of the forage grass, Brachiaria humidicola. Plant Soil 426, 401–411. doi: 10.1007/s11104-018-3626-5
Oelbermann, M. (2014). Sustainable Agroecosystems in Climate Change Mitigation. Wageningen: Wageningen Academic Publishers. doi: 10.3920/978-90-8686-788-2
Opoku, A., Chaves, B., and De Neve, S. (2014). Neem seed oil: a potent nitrification inhibitor to control nitrate leaching after incorporation of crop residues. Biol. Agric. Hortic. 30, 145–152. doi: 10.1080/01448765.2014.885394
Osborne, B. B., Baron, J. S., and Wallenstein, M. D. (2016). Moisture and temperature controls on nitrification differ among ammonia oxidizer communities from three alpine soil habitats. Front. Earth Sci. 10, 1–12. doi: 10.1007/s11707-015-0556-x
Ouyang, Y., Evans, S. E., Friesen, M. L., and Tiemann, L. K. (2018). Effect of nitrogen fertilization on the abundance of nitrogen cycling genes in agricultural soils: a meta-analysis of field studies. Soil Biol. Biochem. 127, 71–78. doi: 10.1016/j.soilbio.2018.08.024
Ouyang, Y., Norton, J. M., and Stark, J. M. (2017). Ammonium availability and temperature control contributions of ammonia oxidizing bacteria and archaea to nitrification in an agricultural soil. Soil Biol. Biochem. 113, 161–172. doi: 10.1016/j.soilbio.2017.06.010
Ouyang, Y., Norton, J. M., Stark, J. M., Reeve, J. R., and Habteselassie, M. Y. (2016). Ammonia-oxidizing bacteria are more responsive than archaea to nitrogen source in an agricultural soil. Soil Biol. Biochem. 96, 4–15. doi: 10.1016/j.soilbio.2016.01.012
Pajares, S., and Bohannan, B. J. M. (2016). Ecology of nitrogen fixing, nitrifying, and denitrifying microorganisms in tropical forest soils. Front. Microbiol. 7:1045. doi: 10.3389/fmicb.2016.01045
Palacin-Lizarbe, C., Camarero, L., Hallin, S., Jones, C. M., Cáliz, J., Casamayor, E. O., et al. (2019). The DNRA-denitrification dichotomy differentiates nitrogen transformation pathways in mountain lake benthic habitats. Front. Microbiol. 10:1229. doi: 10.3389/fmicb.2019.01229
Pariasca Tanaka, J., Nardi, P., and Wissuwa, M. (2010). Nitrification inhibition activity, a novel trait in root exudates of rice. AoB Plants 2010:plq014. doi: 10.1093/aobpla/plq014
Pathak, H., Li, C., and Wassmann, R. (2005). Greenhouse gas emissions from Indian rice fields: calibration and upscaling using the DNDC model. Biogeosci. Discuss. 2, 77–102. doi: 10.5194/bgd-2-77-2005
Paustian, K., Lehmann, J., Ogle, S., Reay, D., Robertson, G. P., and Smith, P. (2016). Climate-smart soils. Nature 532, 49–57. doi: 10.1038/nature17174
Pester, M., Rattei, T., Flechl, S., Gröngröft, A., Richter, A., Overmann, J., et al. (2012). amoA-based consensus phylogeny of ammonia-oxidizing archaea and deep sequencing of amoA genes from soils of four different geographic regions. Environ. Microbiol. 14, 525–539. doi: 10.1111/j.1462-2920.2011.02666.x
Pitcher, A., Villanueva, L., Hopmans, E. C., Schouten, S., Reichart, G.-J., and Sinninghe Damsté, J. S. (2011). Niche segregation of ammonia-oxidizing archaea and anammox bacteria in the Arabian Sea oxygen minimum zone. ISME J. 5, 1896–1904. doi: 10.1038/ismej.2011.60
Prosser, J. I., and Nicol, G. W. (2012). Archaeal and bacterial ammonia-oxidisers in soil: the quest for niche specialisation and differentiation. Trends Microbiol. 20, 523–531. doi: 10.1016/j.tim.2012.08.001
Qiao, C., Liu, L., Hu, S., Compton, J. E., Greaver, T. L., and Li, Q. (2015). How inhibiting nitrification affects nitrogen cycle and reduces environmental impacts of anthropogenic nitrogen input. Glob. Change Biol. 21, 1249–1257. doi: 10.1111/gcb.12802
Qin, W., Amin, S. A., Martens-Habbena, W., Walker, C. B., Urakawa, H., Devol, A. H., et al. (2014). Marine ammonia-oxidizing archaeal isolates display obligate mixotrophy and wide ecotypic variation. Proc. Natl. Acad. Sci. U.S.A. 111, 12504–12509. doi: 10.1073/pnas.1324115111
Ramanathan, B., Boddicker, A. M., Roane, T. M., and Mosier, A. C. (2017). Nitrifier gene abundance and diversity in sediments impacted by acid mine drainage. Front. Microbiol. 8:2136. doi: 10.3389/fmicb.2017.02136
Recio, J., Vallejo, A., Le-Noë, J., Garnier, J., García-Marco, S., Álvarez, J. M., et al. (2018). The effect of nitrification inhibitors on NH3 and N2O emissions in highly N fertilized irrigated Mediterranean cropping systems. Sci. Total Environ. 636, 427–436. doi: 10.1016/J.SCITOTENV.2018.04.294
Rehman, H., Basra, S. M. A., Farooq, M., and Ge, T. (2016). “Nitrogen splitting; a strategy to improve total nitrogen uptake, apparent recovery and grain yield of direct seeded aerobic rice,” in Proceedings of the 2016 International Nitrogen Initiative Conference, “Solutions to Improve Nitrogen Use Efficiency for the World”, 4–8 December 2016, Melbourne, VIC, 2–5.
Robertson, G. P., Bruulsema, T. W., Gehl, R. J., Kanter, D., Mauzerall, D. L., Rotz, C. A., et al. (2013). Nitrogen-climate interactions in US agriculture. Biogeochemistry 114, 41–70. doi: 10.1007/s10533-012-9802-4
Rusch, A. (2013). Molecular tools for the detection of nitrogen cycling archaea. Archaea 2013:676450. doi: 10.1155/2013/676450
Ruser, R., and Schulz, R. (2015). The effect of nitrification inhibitors on the nitrous oxide (N2O) release from agricultural soils-a review. J. Plant Nutr. Soil Sci. 178, 171–188. doi: 10.1002/jpln.201400251
Sahrawat, K. L. (2008). Factors affecting nitrification in soils. Commun. Soil Sci. Plant Anal. 39, 1436–1446. doi: 10.1080/00103620802004235
Sahrawat, K. L. (2010). Nitrogen mineralization in lowland rice soils: the role of organic matter quantity and quality. Arch. Agron. Soil Sci. 56, 337–353. doi: 10.1080/03650340903093158
Sahrawat, K. L. (2012). Soil fertility in flooded and non-flooded irrigated rice systems. Arch. Agron. Soil Sci. 58, 423–436. doi: 10.1080/03650340.2010.522993
Saito, T., Ishii, S., Otsuka, S., Nishiyama, M., and Senoo, K. (2008). Identification of novel betaproteobacteria in a succinate-assimilating population in denitrifying rice paddy soil by using stable isotope probing. Microbes Environ. 23, 192–200. doi: 10.1264/jsme2.23.192
Santoro, A. E., and Casciotti, K. L. (2011). Enrichment and characterization of ammonia-oxidizing archaea from the open ocean: phylogeny, physiology and stable isotope fractionation. ISME J. 5, 1796–1808. doi: 10.1038/ismej.2011.58
Sauder, L. A., Albertsen, M., Engel, K., Schwarz, J., Nielsen, P. H., Wagner, M., et al. (2017). Cultivation and characterization of Candidatus Nitrosocosmicus exaquare, an ammonia-oxidizing archaeon from a municipal wastewater treatment system. ISME J. 11, 1142–1157. doi: 10.1038/ismej.2016.192
Sauder, L. A., Engel, K., Lo, C. C., Chain, P., and Neufeld, J. D. (2018). “Candidatus Nitrosotenuis aquarius,” an ammonia-oxidizing archaeon from a freshwater aquarium biofilter. Appl. Environ. Microbiol. 84:e1430-18. doi: 10.1128/AEM.01430-18
Sauder, L. A., Peterse, F., Schouten, S., and Neufeld, J. D. (2012). Low-ammonia niche of ammonia-oxidizing archaea in rotating biological contactors of a municipal wastewater treatment plant. Environ. Microbiol. 14, 2589–2600. doi: 10.1111/j.1462-2920.2012.02786.x
Schleper, C., and Nicol, G. W. (2010). Ammonia-oxidising archaea – physiology, ecology and evolution. Adv. Microb. Physiol. 57, 1–41. doi: 10.1016/B978-0-12-381045-8.00001-1
Schroder, J. L., Zhang, H., Girma, K., Raun, W. R., Penn, C. J., and Payton, M. E. (2011). Soil acidification from long-term use of nitrogen fertilizers on winter wheat. Soil Sci. Soc. Am. J. 75:957. doi: 10.2136/sssaj2010.0187
Schütt, M. (2015). Carbon and Nitrogen Mineralization in Temperate Forest Soils at Low Temperatures. Ph.D. dissertation. Bayreuth: Faculty of Biology/Chemistry/Earth Sciences at the University of Bayreuth.
Schütt, M., Borken, W., Spott, O., Stange, C. F., and Matzner, E. (2014). Temperature sensitivity of C and N mineralization in temperate forest soils at low temperatures. Soil Biol. Biochem. 69, 320–327. doi: 10.1016/j.soilbio.2013.11.014
Shen, J.-P., Zhang, L.-M., Di, H. J., and He, J.-Z. (2012). A review of ammonia-oxidizing bacteria and archaea in Chinese soils. Front. Microbiol. 3:296. doi: 10.3389/fmicb.2012.00296
Shen T., Stieglmeier M., Dai J., Urich T., and Schleper C. (2013) Responses of the terrestrial ammonia-oxidizing archaeon Ca. Nitrososphaera viennensis and the ammonia-oxidizing bacterium Nitrosospira multiformis to nitrification inhibitors. FEMS Microbiol Lett. 344, 121–9. doi: 10.1111/1574-6968.12164 [Epub ahead of print].
Sheng, P., Yu, Y., Tian, X., Wang, D., Zhang, Z., and Ding, J. (2016). Diversity of ammonia-oxidising bacteria and archaea in seven different estuarine sediments from Poyang Lake. Mar. Freshw. Res. 67, 1897–1905. doi: 10.1071/MF15129
Shiozaki, T., Ijichi, M., Isobe, K., Hashihama, F., Nakamura, K., Ehama, M., et al. (2016). Nitrification and its influence on biogeochemical cycles from the equatorial Pacific to the Arctic Ocean. ISME J. 10, 2184–2197. doi: 10.1038/ismej.2016.18
Simon, J., and Klotz, M. G. (2013). Diversity and evolution of bioenergetic systems involved in microbial nitrogen compound transformations. Biochim. Biophys. Acta 1827, 114–135. doi: 10.1016/J.BBABIO.2012.07.005
Sintes, E., Bergauer, K., De Corte, D., Yokokawa, T., and Herndl, G. J. (2013). Archaeal amoA gene diversity points to distinct biogeography of ammonia-oxidizing Crenarchaeota in the ocean. Environ. Microbiol. 15, 1647–1658. doi: 10.1111/j.1462-2920.2012.02801.x
Skiba, M. W., George, T. S., Baggs, E. M., and Daniell, T. J. (2011). Plant influence on nitrification. Biochem. Soc. Trans. 39, 275–278. doi: 10.1042/BST0390275
Smith, J. M., Damashek, J., Chavez, F. P., and Francis, C. A. (2016). Factors influencing nitrification rates and the abundance and transcriptional activity of ammonia-oxidizing microorganisms in the dark northeast Pacific Ocean. Limnol. Oceanogr. 61, 596–609. doi: 10.1002/lno.10235
Snyder, C. S. (2016). “Enhanced nitrogen fertilizer technologies support the ‘4R’ concept to optimize crop production and minimize environmental losses aim of this presentation and paper highlight opportunities to improve crop recovery of applied fertilizer N and to reduce,” in Proc. 2016 Int. Nitrogen Initiat. Conf. “Solutions to Improv. nitrogen use Effic. World”, 4 – 8 December 2016, Melbourne, VIC, 4–8.
Solomon, S., Qin, D., Manning, M., Chen, Z., Marquis, M., Averyt, K. B., et al. (2007). Global Climate Projections, 1–8. Available online at: https://philpapers.org/rec/SOLGCP (accessed May 14, 2019).
Spang, A., Poehlein, A., Offre, P., Zumbrägel, S., Haider, S., Rychlik, N., et al. (2012). The genome of the ammonia-oxidizing Candidatus Nitrososphaera gargensis: insights into metabolic versatility and environmental adaptations. Environ. Microbiol. 14, 3122–3145. doi: 10.1111/j.1462-2920.2012.02893.x
Stahl, D. A., and de la Torre, J. R. (2012). Physiology and diversity of ammonia-oxidizing archaea. Annu. Rev. Microbiol. 66, 83–101. doi: 10.1146/annurev-micro-092611-150128
Stein, L. Y., and Klotz, M. G. (2016). The nitrogen cycle. Curr. Biol. 26, R94–R98. doi: 10.1016/J.CUB.2015.12.021
Sterngren, A. E., Hallin, S., and Bengtson, P. (2015). Archaeal ammonia oxidizers dominate in numbers, but bacteria drive gross nitrification in n-amended grassland soil. Front. Microbiol. 6:1350. doi: 10.3389/fmicb.2015.01350
Stieglmeier, M., Klingl, A., Alves, R. J. E., Rittmann, S. K.-M. R., Melcher, M., Leisch, N., et al. (2014). Nitrososphaera viennensis gen. nov., sp. nov., an aerobic and mesophilic, ammonia-oxidizing archaeon from soil and a member of the archaeal phylum Thaumarchaeota. Int. J. Syst. Evol. Microbiol. 64, 2738–2752. doi: 10.1099/ijs.0.063172-0
Subbarao, G. V., Nakahara, K., Hurtado, M. P., Ono, H., Moreta, D. E., Salcedo, A. F., et al. (2009). Evidence for biological nitrification inhibition in Brachiaria pastures. Proc. Natl. Acad. Sci. U.S.A. 106, 17302–17307. doi: 10.1073/pnas.0903694106
Subbarao, G. V., Nakahara, K., Ishikawa, T., Ono, H., Yoshida, M., Yoshihashi, T., et al. (2013). Biological nitrification inhibition (BNI) activity in sorghum and its characterization. Plant Soil 366, 243–259. doi: 10.1007/s11104-012-1419-9
Subbarao, G. V., Yoshihashi, T., Worthington, M., Nakahara, K., Ando, Y., Sahrawat, K. L., et al. (2015). Suppression of soil nitrification by plants. Plant Sci. 233, 155–164. doi: 10.1016/j.plantsci.2015.01.012
Sun, L., Lu, Y., Yu, F., Kronzucker, H. J., and Shi, W. (2016). Biological nitrification inhibition by rice root exudates and its relationship with nitrogen-use efficiency. New Phytol. 212, 646–656. doi: 10.1111/nph.14057
Suter, H., Chen, D. A., Li, H., Edis, R., and Walker, C. (2010). “Comparison of the ability of the nitrification inhibitors DCD and DMPP to reduce nitrification and N2O emissions from nitrogen fertilisers,” in Proceedings of the 19th World Congr. Soil Sci. Soil Solut. a Chang. World, Brisbane, QLD, 84–87.
Takriti, M., Wild, B., Schnecker, J., Mooshammer, M., Knoltsch, A., Lashchinskiy, N., et al. (2018). Soil organic matter quality exerts a stronger control than stoichiometry on microbial substrate use efficiency along a latitudinal transect. Soil Biol. Biochem. 121, 212–220. doi: 10.1016/J.SOILBIO.2018.02.022
Taylor, A. E., Giguere, A. T., Zoebelein, C. M., Myrold, D. D., and Bottomley, P. J. (2017). Modeling of soil nitrification responses to temperature reveals thermodynamic differences between ammonia-oxidizing activity of archaea and bacteria. ISME J. 11, 896–908. doi: 10.1038/ismej.2016.179
Taylor, A. E., Taylor, K., Tennigkeit, B., Palatinszky, M., Stieglmeier, M., Myrold, D. D., et al. (2015). Inhibitory Effects of C 2 to C 10 1-Alkynes on ammonia oxidation in two Nitrososphaera species. Appl. Environ. Microbiol. 81, 1942–1948. doi: 10.1128/AEM.03688-14
Taylor, A. E., Zeglin, L. H., Wanzek, T. A., Myrold, D. D., and Bottomley, P. J. (2012). Dynamics of ammonia-oxidizing archaea and bacteria populations and contributions to soil nitrification potentials. ISME J. 6, 2024–2032. doi: 10.1038/ismej.2012.51
Tesfamariam, T., Yoshinaga, H., Deshpande, S. P., Rao, P. S., Sahrawat, K. L., Ando, Y., et al. (2014). Biological nitrification inhibition in sorghum: the role of sorgoleone production. Plant Soil 379, 325–335. doi: 10.2307/42952834
Thangarajan, R., Bolan, N. S., Naidu, R., and Surapaneni, A. (2015). Effects of temperature and amendments on nitrogen mineralization in selected Australian soils. Environ. Sci. Pollut. Res. 22, 8843–8854. doi: 10.1007/s11356-013-2191-y
Thion, C. E., Poirel, J. D., Cornulier, T., De Vries, F. T., Bardgett, R. D., and Prosser, J. I. (2016). Plant nitrogen-use strategy as a driver of rhizosphere archaeal and bacterial ammonia oxidiser abundance. FEMS Microbiol. Ecol. 92:fiw091. doi: 10.1093/femsec/fiw091
Tolar, B. B., Herrmann, J., Bargar, J. R., van den Bedem, H., Wakatsuki, S., and Francis, C. A. (2017). Integrated structural biology and molecular ecology of N-cycling enzymes from ammonia-oxidizing archaea. Environ. Microbiol. Rep. 9, 484–491. doi: 10.1111/1758-2229.12567
Tourna, M., Freitag, T. E., Nicol, G. W., and Prosser, J. I. (2008). Growth, activity and temperature responses of ammonia-oxidizing archaea and bacteria in soil microcosms. Environ. Microbiol. 10, 1357–1364. doi: 10.1111/j.1462-2920.2007.01563.x
Tourna, M., Stieglmeier, M., Spang, A., Konneke, M., Schintlmeister, A., Urich, T., et al. (2011). Nitrososphaera viennensis, an ammonia oxidizing archaeon from soil. Proc. Natl. Acad. Sci. U.S.A. 108, 8420–8425. doi: 10.1073/pnas.1013488108
Trap, J., Bonkowski, M., Plassard, C., Trap, J., Bonkowski, M., Plassard, C., et al. (2015). Ecological importance of soil bacterivores for ecosystem functions. Plant Soil 398, 1–24.
Tuong, T. P., and Bouman, B. A. M. (2003). “Rice production in water scarce environments,” in Water Productivity in Agriculture: Limits and Opportunities for Improvement, eds J. W. Kijne, R. Barker, and D. Molden (Wallingford: CABI Publishing), 53–67.
Ueyama, M., Ichii, K., Kobayashi, H., Babacar, A. S. S., Faye, W., Webber, H., et al. (2013). Regional disparities in the CO2 fertilization effect and implications for crop yields. Environ. Res. Lett. 8:014054. doi: 10.1088/1748-9326/8/1/014054
Urakawa, H., Maki, H., Kawabata, S., Fujiwara, T., Ando, H., Kawai, T., et al. (2006). Abundance and population structure of ammonia-oxidizing bacteria that inhabit canal sediments receiving effluents from municipal wastewater treatment plants. Appl. Environ. Microbiol. 72, 6845–6850. doi: 10.1128/AEM.00807-06
Uzair, M., Patil, S. B., Zhang, H., Kumar, A., Mkumbwa, H., Zafar, S. A., et al. (2022). Screening direct seeding-related traits by using an improved mesocotyl elongation assay and association between seedling and maturity traits in rice. Agronomy 12:975.
Vajrala, N., Martens-Habbena, W., Sayavedra-Soto, L. A., Schauer, A., Bottomley, P. J., Stahl, D. A., et al. (2013). Hydroxylamine as an intermediate in ammonia oxidation by globally abundant marine archaea. Proc. Natl. Acad. Sci. U.S.A. 110, 1006–1011. doi: 10.1073/pnas.1214272110
Venterea, R. T., Halvorson, A. D., Kitchen, N., Liebig, M. A., Cavigelli, M. A., Del Grosso, S. J., et al. (2012). Challenges and opportunities for mitigating nitrous oxide emissions from fertilized cropping systems. Front. Ecol. Environ. 10, 562–570. doi: 10.1890/120062
Verhamme, D. T., Prosser, J. I., and Nicol, G. W. (2011). Ammonia concentration determines differential growth of ammonia-oxidising archaea and bacteria in soil microcosms. ISME J. 5, 1067–1071. doi: 10.1038/ismej.2010.191
Wan, Y., Ruan, X., Wang, J., and Shi, X. (2019). Spatial and seasonal variations in the abundance of nitrogen-transforming genes and the microbial community structure in freshwater lakes with different trophic statuses. Int. J. Environ. Res. Public Health 16:2298. doi: 10.3390/ijerph16132298
Wang, H., Yu, L., Zhang, Z., Liu, W., Chen, L., Cao, G., et al. (2017). Molecular mechanisms of water table lowering and nitrogen deposition in affecting greenhouse gas emissions from a Tibetan alpine wetland. Glob. Change Biol. 23, 815–829. doi: 10.1111/gcb.13467
Wang, J., Hussain, S., Sun, X., Zhang, P., Javed, T., Dessoky, E. S., et al. (2022). Effects of nitrogen application rate under straw incorporation on photosynthesis, productivity and nitrogen use efficiency in winter wheat. Front. Plant Sci. 13:862088. doi: 10.3389/fpls.2022.862088
Wang, J., Kan, J., Zhang, X., Xia, Z., Zhang, X., Qian, G., et al. (2017). Archaea dominate the ammonia-oxidizing community in deep-sea sediments of the Eastern Indian ocean—from the equator to the Bay of Bengal. Front. Microbiol. 8:415. doi: 10.3389/fmicb.2017.00415
Wang, W., Su, Y., Wang, B., Wang, Y., Zhuang, L., and Zhu, G. (2019). Spatiotemporal shifts of ammonia-oxidizing archaea abundance and structure during the restoration of a multiple pond and plant-bed/ditch wetland. Sci. Total Environ. 684, 629–640. doi: 10.1016/J.SCITOTENV.2019.04.415
Wang, X., Wen, X., Criddle, C., Wells, G., Zhang, J., and Zhao, Y. (2010). Community analysis of ammonia-oxidizing bacteria in activated sludge of eight wastewater treatment systems. J. Environ. Sci. 22, 627–634. doi: 10.1016/S1001-0742(09)60155-8
Wang, Y.-F., Feng, Y.-Y., Ma, X., and Gu, J.-D. (2013). Seasonal dynamics of ammonia/ammonium-oxidizing prokaryotes in oxic and anoxic wetland sediments of subtropical coastal mangrove. Appl. Microbiol. Biotechnol. 97, 7919–7934. doi: 10.1007/s00253-012-4510-5
Wessén, E., Söderström, M., Stenberg, M., Bru, D., Hellman, M., Welsh, A., et al. (2011). Spatial distribution of ammonia-oxidizing bacteria and archaea across a 44-hectare farm related to ecosystem functioning. ISME J. 5, 1213–1225. doi: 10.1038/ismej.2010.206
Xia, L., Lam, S. K., Chen, D., Wang, J., Tang, Q., and Yan, X. (2017). Can knowledge-based N management produce more staple grain with lower greenhouse gas emission and reactive nitrogen pollution? A meta-analysis. Glob. Change Biol. 23, 1917–1925. doi: 10.1111/gcb.13455
Xia, W., Zhang, C., Zeng, X., Feng, Y., Weng, J., Lin, X., et al. (2011). Autotrophic growth of nitrifying community in an agricultural soil. ISME J. 5, 1226–1236. doi: 10.1038/ismej.2011.5
Xiao, H., Griffiths, B., Chen, X., Liu, M., Jiao, J., Hu, F., et al. (2010). Influence of bacterial-feeding nematodes on nitrification and the ammonia-oxidizing bacteria (AOB) community composition. Appl. Soil Ecol. 45, 131–137. doi: 10.1016/j.apsoil.2010.03.011
Xu-Ri, Prentice, I. C., Spahni, R., and Niu, H. S. (2012). Modelling terrestrial nitrous oxide emissions and implications for climate feedback. New Phytol. 196, 472–488. doi: 10.1111/J.1469-8137.2012.04269.X
Yan, N., Marschner, P., Cao, W., Zuo, C., and Qin, W. (2015). Influence of salinity and water content on soil microorganisms. Int. Soil Water Conserv. Res. 3, 316–323. doi: 10.1016/J.ISWCR.2015.11.003
Yapsakli, K., Aliyazicioglu, C., and Mertoglu, B. (2011). Identification and quantitative evaluation of nitrogen-converting organisms in a full-scale leachate treatment plant. J. Environ. Manage. 92, 714–723. doi: 10.1016/j.jenvman.2010.10.017
Yin, Z., Bi, X., and Xu, C. (2018). Ammonia-oxidizing archaea (AOA) play with ammonia-oxidizing bacteria (AOB) in nitrogen removal from wastewater. Archaea 2018:8429145. doi: 10.1155/2018/8429145
Yoshida, M., Ishii, S., Fujii, D., Otsuka, S., and Senoo, K. (2012). Identification of active denitrifiers in rice paddy soil by DNA- and RNA-based analyses. Microbes Environ. 27, 456–461. doi: 10.1264/jsme2.me12076
Zaehle, S. (2013). Terrestrial nitrogen–carbon cycle interactions at the global scale. Philos. Trans. R. Soc. B Biol. Sci. 368:20130125. doi: 10.1098/RSTB.2013.0125
Zakir, H. A. K. M., Subbarao, G. V., Pearse, S. J., Gopalakrishnan, S., Ito, O., Ishikawa, T., et al. (2008). Detection, isolation and characterization of a root-exuded compound, methyl 3-(4-hydroxyphenyl) propionate, responsible for biological nitrification inhibition by sorghum (Sorghum bicolor). New Phytol. 180, 442–451. doi: 10.1111/j.1469-8137.2008.02576.x
Zebarth, B. J., Forge, T. A., Goyer, C., and Brin, L. D. (2015). Effect of soil acidification on nitrification in soil. Can. J. Soil Sci. 95, 359–363. doi: 10.4141/cjss-2015-040
Zhalnina, K., de Quadros, P. D., Camargo, F. A. O., and Triplett, E. W. (2012). Drivers of archaeal ammonia-oxidizing communities in soil. Front. Microbiol. 3:210. doi: 10.3389/fmicb.2012.00210
Zhalnina, K. V., Dias, R., Leonard, M. T., Dorr de Quadros, P., Camargo, F. A. O., Drew, J. C., et al. (2014). Genome Sequence of Candidatus Nitrososphaera evergladensis from Group I.1b enriched from everglades soil reveals novel genomic features of the ammonia-oxidizing archaea. PLoS One 9:e101648. doi: 10.1371/journal.pone.0101648
Zhang, J., Sun, W., Zhong, W., and Cai, Z. (2014). The substrate is an important factor in controlling the significance of heterotrophic nitrification in acidic forest soils. Soil Biol. Biochem. 76, 143–148. doi: 10.1016/j.soilbio.2014.05.001
Zhang, L.-M., Hu, H.-W., Shen, J.-P., and He, J.-Z. (2012). Ammonia-oxidizing archaea have more important role than ammonia-oxidizing bacteria in ammonia oxidation of strongly acidic soils. ISME J. 6, 1032–1045. doi: 10.1038/ismej.2011.168
Zhang, M.-M., Alves, R. J. E., Zhang, D.-D., Han, L.-L., He, J.-Z., and Zhang, L.-M. (2017). Time-dependent shifts in populations and activity of bacterial and archaeal ammonia oxidizers in response to liming in acidic soils. Soil Biol. Biochem. 112, 77–89. doi: 10.1016/J.SOILBIO.2017.05.001
Zhang, S., Song, W., Wemheuer, B., Reveillaud, J., Webster, N., and Thomas, T. (2019a). Comparative genomics reveals ecological and evolutionary insights into sponge-associated Thaumarchaeota. mSystems 4:e00288-19. doi: 10.1128/mSystems.00288-19
Zhang, S., Zheng, Q., Noll, L., Hu, Y., and Wanek, W. (2019b). Environmental effects on soil microbial nitrogen use efficiency are controlled by allocation of organic nitrogen to microbial growth and regulate gross N mineralization. Soil Biol. Biochem. 135, 304–315. doi: 10.1016/j.soilbio.2019.05.019
Zhang, T., Ye, L., Tong, A. H. Y., Shao, M.-F., and Lok, S. (2011). Ammonia-oxidizing archaea and ammonia-oxidizing bacteria in six full-scale wastewater treatment bioreactors. Appl. Microbiol. Biotechnol. 91, 1215–1225. doi: 10.1007/s00253-011-3408-y
Zhang, X., Davidson, E. A., Mauzerall, D. L., Searchinger, T. D., Dumas, P., and Shen, Y. (2015). Managing nitrogen for sustainable development. Nature 528, 51–59. doi: 10.1038/nature15743
Zhang, Y., Zhang, S., Wang, R., Cai, J., Zhang, Y., Li, H., et al. (2016). Impacts of fertilization practices on pH and the pH buffering capacity of calcareous soil. Soil Sci. Plant Nutr. 62, 432–439. doi: 10.1080/00380768.2016.1226685
Zhao, H., Li, X., and Jiang, Y. (2019). Response of nitrogen losses to excessive nitrogen fertilizer application in intensive greenhouse vegetable production. Sustainability 11:1513. doi: 10.3390/su11061513
Zhao, J., Bello, M. O., Meng, Y., Prosser, J. I., and Gubry-Rangin, C. (2020). Selective inhibition of ammonia oxidising archaea by simvastatin stimulates growth of ammonia oxidising bacteria. Soil Biol. Biochem. 141:107673. doi: 10.1016/J.SOILBIO.2019.107673
Zhou, Q., Ju, C., Wang, Z., Zhang, H., Liu, L., Yang, J., et al. (2017). Grain yield and water use efficiency of super rice under soil water deficit and alternate wetting and drying irrigation. J. Integr. Agric. 16, 1028–1043. doi: 10.1016/S2095-3119(16)61506-X
Zhu, T., Yang, C., Wang, J., Zeng, S., Liu, M., Yang, J., et al. (2018). Bacterivore nematodes stimulate soil gross N transformation rates depending on their species. Biol. Fertil. Soils 54, 107–118. doi: 10.1007/s00374-017-1244-7
Keywords: aerobic rice system, N-cycle, ammonia-oxidizers, nitrification-inhibitors, agronomic adjustive measures
Citation: Farooq MS, Uzair M, Maqbool Z, Fiaz S, Yousuf M, Yang SH and Khan MR (2022) Improving Nitrogen Use Efficiency in Aerobic Rice Based on Insights Into the Ecophysiology of Archaeal and Bacterial Ammonia Oxidizers. Front. Plant Sci. 13:913204. doi: 10.3389/fpls.2022.913204
Received: 05 April 2022; Accepted: 16 May 2022;
Published: 13 June 2022.
Edited by:
Adnan Noor Shah, Khwaja Fareed University of Engineering and Information Technology (KFUEIT), PakistanReviewed by:
Talha Javed, Fujian Agriculture and Forestry University, ChinaRoberta Pastorelli, Council for Agricultural Research and Economics (CREA), Italy
Umair Ashraf, University of Education Lahore, Pakistan
Copyright © 2022 Farooq, Uzair, Maqbool, Fiaz, Yousuf, Yang and Khan. This is an open-access article distributed under the terms of the Creative Commons Attribution License (CC BY). The use, distribution or reproduction in other forums is permitted, provided the original author(s) and the copyright owner(s) are credited and that the original publication in this journal is cited, in accordance with accepted academic practice. No use, distribution or reproduction is permitted which does not comply with these terms.
*Correspondence: Seung Hwan Yang, eW1pY2hpZ2FuQGpudS5hYy5rcg==; Muhammad Ramzan Khan, bXJraGFuQHBhcmMuZ292LnBr
†These authors have contributed equally to this work