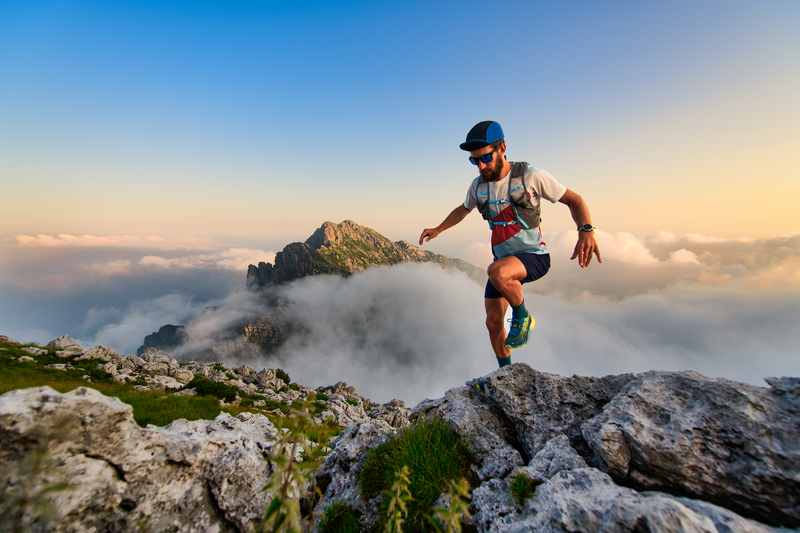
95% of researchers rate our articles as excellent or good
Learn more about the work of our research integrity team to safeguard the quality of each article we publish.
Find out more
ORIGINAL RESEARCH article
Front. Plant Sci. , 12 May 2022
Sec. Functional Plant Ecology
Volume 13 - 2022 | https://doi.org/10.3389/fpls.2022.906071
This article is part of the Research Topic Physiological Ecology of Trees under Environmental Stresses View all 14 articles
Nitric oxide (NO), a bioactive molecule, is often involved in the regulation of physiological and biochemical processes in stressed plants. However, the effects of NO donors on dioecious plants remain unclear. Using a pot experiment, female and male Torreya grandis were used to study the role of sex and NO in salt stress tolerance. In the present study, female and male T. grandis seedlings pretreated with an NO donor (sodium nitroprusside, SNP) were exposed to salt stress, and then leaf relative water content (RWC), photosynthetic pigments, chlorophyll fluorescence parameters, NO and glutathione levels, oxidative damage, and antioxidant enzyme activities were investigated. Female T. grandis plants had better tolerance to salinity, as they were characterized by significantly higher RWC, pigment content, and photochemical activities of photosystem II (PSII) and fewer negative effects associated with higher nitrate reductase (NR) activity and NO content. Pretreatment with an NO donor further increased the endogenous NO content and NR activity of both female and male T. grandis plants compared with salt treatment. Moreover, pretreatment with an NO donor alleviated salt-induced oxidative damage of T. grandis, especially in male plants, as indicated by reduced lipid peroxidation, through an enhanced antioxidant system, including proline and glutathione accumulation, and increased antioxidant enzyme activities. However, the ameliorating effect of the NO donor was not effective in the presence of the NO scavenger (Nω-nitro-L-arginine methyl ester, L-name). In conclusion, enhanced salt tolerance in T. grandis plants is related to nitric oxide levels and the supply of NO donors is an interesting strategy for alleviating the negative effect of salt on T. grandis. Our data provide new evidence to contribute to the current understanding of NO-induced salt stress tolerance.
It has been estimated that up to 70% of plant growth can be impacted by environmental stress, including drought, high salinity, heavy metal exposure, high or low temperatures, and light levels (Bhatnagar-Mathur et al., 2008; Mantri et al., 2012; Li et al., 2020). Salinity is a crucial factor affecting plant growth and metabolic responses throughout the world (Mostofa et al., 2015). In addition to direct ion damage, salt stress can produce secondary damage to plants through the production of reactive oxygen species (ROS) and osmotic stress (Li et al., 2020). When salt content in the soil is significantly higher than the optimal concentration for plant survival, it causes a series of stress responses in plants, including maintenance of osmotic balance and activation of the antioxidant system (Wakeel et al., 2020). In recent years, a great deal of research has been done to explore potential ways to improve saline–alkali land, including the breeding of salt-tolerant varieties, the discovery of salt-tolerant genes, and the application of hormones and growth regulators (Wang et al., 2017; Fang and Xue, 2019).
Current studies have shown that nitric oxide (NO) in plants is mainly produced through two pathways, NO synthase (NOS) and nitrate reductase (NR) pathways (Corpas and Barroso, 2017; Astier et al., 2018), and is further involved in a series of physiological and biochemical reactions, including root morphogenesis (Corpas and Barroso, 2015), seed germination (Kopyra and Gwózdz, 2003), stomatal movement (Laxalt et al., 2016), and abiotic stress regulation (Begara-Morales et al., 2014). Nitric oxide is also involved in the salt tolerance of plant growth. For example, da Silva et al. (2017) reported that salinity-induced accumulation of endogenous NO is associated with modulation of the antioxidant and redox defense systems in Nicotiana tabacum. Ren et al. (2020) reported that pretreatment with NO alleviates salt stress in seed germination and seedling growth of Brassica chinensis by enhancing biochemical parameters and regulating osmolyte accumulation. However, most of the studies focused on vegetables and herbaceous plants (Ren et al., 2020; Valderrama et al., 2021; Wani et al., 2021), and there were few studies on woody plants (Chen et al., 2021).
Dioecious plants, as an important part of the terrestrial ecosystem, account for nearly 6% of angiosperms and play an indispensable role in the cycling stability of the ecosystem (Susanne-S and Ricklefs, 1995). In the process of natural differentiation and evolution, dioecious plants evolve sex specificity and show significant differences in growth, survival, reproductive pattern, spatial distribution, and resource allocation (Todd-E and Bliss, 1989). Many researchers have devoted themselves to studying differences in the growth, survival, spatial distribution, and resource allocation of dioecious plants (Retuerto et al., 2000; Rana and Liu, 2021). On a smaller scale, some scholars have found that male and female individuals of dioecious plants show different physiological, ecological, and biochemical traits under environmental stress (Chen et al., 2018; Wang et al., 2021). For example, drought stress reduces the water content of leaves and stress resistance in female Populus yunnanensis (Zhang et al., 2019). In addition, the mortality rate of female plants was higher than that of male plants, indicating that the drought resistance of male Populus yunnanensis was stronger than that of females (Zhang et al., 2019). However, researchers have found that in some dioecious plants, females are more resistant to stress than males. For example, He et al. (2016) found that female Ginkgo biloba showed superior growth performance and self-protection mechanisms compared with male Ginkgo biloba and also had a higher photosynthetic capacity under drought stress.
Torreya grandis is in a genus of deciduous conifer trees and is valued for its production of nuts and timber in China, but its distribution is limited by its cultivated area and prevailing weather conditions (Hu et al., 2022; Shen et al., 2022). Many studies have investigated bioactive substance synthesis and efficacy (Hu et al., 2022; Song et al., 2022) and the interactions of functional trait × environment interactions, such as the effect of salt stress on the photosynthetic activity of T. grandis (Li et al., 2014). However, comparatively little research has been done to elucidate sex differences in T. grandis under salt stress. Our previous study indicated that T. grandis seedlings showed significant gender differences under drought stress, and female plants had better performance in the process of photosynthesis (Wang et al., 2021). In this study, it was hypothesized that there are sexually different responses to salt, and we also hypothesized that: (1) this difference was related to endogenous NO levels and (2) exogenous NO would improve the salt tolerance of T. grandis through increased endogenous NO content and antioxidant enzyme activities. To test these hypotheses, an NO donor (SNP) and NaCl were added to both male and female T. grandis in this study. The aims of this study were: (1) to analyze the sexually different responses of T. grandis to salt and (2) to evaluate the role of NO in the salt stress tolerance of T. grandis. The photosynthetic pigment contents, chlorophyll fluorescence parameters, leaf relative water content, levels of endogenous NO and glutathione in the leaves, and antioxidant systems were evaluated.
Three-year-old T. grandis seedlings were obtained from the Zhuji Forestry Institute (29°43′N,120°16′E). A total of 72 seedlings with a similar mean ground diameter (10.0 mm) and seedling height (60 cm), split between males (n = 36) and females (n = 36), were used in the salt stress experiment. The trees were grown individually in plastic pots (20-cm diameter × 18-cm deep) in the greenhouse of Jiyang College of Zhejiang A&F University (Zhejiang Province, China; 29°45′N,120°15′E) in late May 2021. The greenhouse environment was controlled under a 16-h/8-h (day/night) photoperiod, with an average temperature of 30/18°С (day/night) and a relative humidity of 70 ± 5%. The plastic pots were placed in trays to prevent NaCl leaching.
Salt treatments were conducted in late June 2021. Both male and female plants were divided into four treatments: CK (control, distilled water), salt (100 mM NaCl), SNP (100 mM NaCl with 0.05 mM sodium nitroprusside [SNP]), and L-name (100 mM NaCl with 0.5 mM SNP and 0.2 mM Nω-nitro-L-arginine methyl ester [L-name]). There were three replications per treatment and three plants per replication. The NO donor (SNP) was sprayed over the surfaces of the T. grandis leaves three days before salt treatment (20 ml SNP per seedling). Dosage of NO was fixed according to previous studies of NO in other plants (Ren et al., 2020; Valderrama et al., 2021; Wani et al., 2021). The NO scavenger used in our study was Nω-nitro-L-arginine methyl ester (L-name) (Suda et al., 2002). To avoid osmotic shock, NaCl solution was gradually added in eight steps to achieve a concentration of 0.2%, which was related to soil weight, according to Li et al. (2014). Irrigation was conducted every three days to maintain the field capacity at 70–75%, and all indexes were measured 60 days after treatment when obvious differences were observed.
Leaves from similar positions within the mid-portion of the main stem were collected from three replicates of each treatment after analyzing the chlorophyll fluorescence parameters. After that, the leaves of each sample were cleaned and immediately used for the analysis of photosynthetic pigments, NO content and enzymatic assays.
First, finely cut leaves (collected from similar positions within the mid-portion of the main stem in each treatment) weighing 0.1 g were mixed and extracted with 8 ml 95% ethanol, according to Arnon (1949). The extraction of chlorophyll was then conducted at 4°C in the dark for 24 h and shaken about three times until the leaf samples were blanched. The absorbance of the samples was measured at 649, 665, and 470 nm using a spectrophotometer (Shimadzu UV-2550, Kyoto, Japan). The chlorophyll concentrations of T. grandis leaves were calculated following Arnon (1949).
The PSII photochemical activity of T. grandis leaves was evaluated after dark adaption for 60 min (based on the previous experiment) using a multifunctional plant efficiency analyzer (Hansatech Instruments, Pentney, UK). The fluorescent parameters, including Fv/Fm (the maximum quantum yield of the primary PSII photochemistry), ABS/RC (the absorbed light energy by the PSII antenna photon flux per active reaction center), DIo/RC (total energy dissipated by the reaction center of PSII), and TRo/RC (total energy used to restore QA by the unit reaction center of PSII), were calculated from the OJIP test curves.
Lipid peroxidation was measured as the amount of malondialdehyde (MDA) determined by the thiobarbituric acid (TBA) reaction, using 0.3 g of the frozen leaf samples per replicate (n = 3) according to Deng et al. (2011). Meanwhile, 10 leaf discs (about 10 mm in diameter) from fully expanded leaves of T. grandis were used in each replicate (n = 3) to determine the relative electrolyte leakage conductivity (REC), according to Li et al. (2014).
The O2·− production rates of T. grandis leaves under salt and NO treatments were assessed by monitoring the nitrite formed from hydroxylamine in the presence of O2·− as described by Wang (1990). Frozen leaf material (1 g) was used for each replicate (n = 3). Similarly, a frozen leaf (1 g) was used in each replicate (n = 3) for the analysis of H2O2 content in T. grandis leaves, following Patterson et al. (1984).
The relative water content (RWC) was measured according to the method of our previous study (Wang et al., 2021). The fresh leaves of each treatment were collected and weighed to obtain the fresh weight (FW). Leaves of T. grandis were then soaked in purified water at 25°С for 4 h to obtain the saturated fresh weight (SW). Subsequently, leaves were placed in a 70°С oven for 6 h to obtain the dry weight (DW). RWC was calculated as follows: RWC = (FW – DW)/(SW – DW) × 100%.
Proline (Pro) content was determined according to Bates et al. (1973). A total of 0.3 g of T. grandis leaves were homogenized in sulfosalicylic acid, and then, 2 ml of acid ninhydrin and 2 ml of glacial acetic acid were added. The samples were heated at 100°C and extracted with toluene, and the free toluene was then quantified at 528 nm using L-proline as a standard.
Fresh leaves (0.3 g) were used in each replicate (n = 3) for the analysis of NO content, according to Cantrel et al. (2011). NO content was calculated using a standard curve of NaNO2 (0–4 μg mL−1) and expressed as μmol g−1 FW. The activity of nitrate reductase (NR; E.C. 1.6.6.1) was determined according to Jaworski (1971). Similarly, a fresh leaf (0.3 g) was used for each replicate (n = 3). The activity of NR was expressed as nmol NO2−1 min−1 mg−1 protein.
Antioxidant enzyme extracts from each treatment were obtained from 0.3 g frozen leaves, according to Yu et al. (2017). The leaves were homogenized at 4°C in 10 ml of 50 mM phosphate buffer solution (pH 7.8) containing 1% polyethylenepyrrole. The homogenate was then centrifuged at 10,000 × g at 4°C for 15 min. The supernatant was collected and used to measure GSH content and enzyme activity. Meanwhile, soluble proteins were determined according to Bradford (1976).
GSH content and glutathione reductase (GR) activity were assayed using kits from the Nanjing Jiancheng Bioengineering Institute (Nanjing, China), following the manufacturer’s specifications. GR activity was expressed as nmol min−1 mg−1 protein. The GSH content was expressed as mg g−1 FW. SOD activity was analyzed following Beauchamp and Fridovich (1971) and was expressed as U g−1 protein. POD activity was estimated according to Ruan et al. (2002) and was expressed as U mg−1 protein. CAT activity was measured following Watanabe et al. (2003) and was expressed as μmol min−1 mg−1 protein. APX activity was measured using the method described by Nakano and Asada (1981) and was expressed as nmol min−1 mg−1 protein.
Two-way analysis of variance (ANOVA), with sex and salt as the main factors and a sex × salt interaction term, was performed using SPSS 19.0 (SPSS Inc., Chicago, IL, USA) to evaluate the effects of sex and salt treatments and was followed by Duncan’s test (p < 0.05). The data are presented as the mean ± standard deviation (SD).
The chlorophyll contents and chlorophyll fluorescence parameters of T. grandis were found to be significantly different under salt treatments (Table 1). Moreover, a significant interaction of sex and salt was observed in the total chlorophyll content, Chl a content, Chl b content, and ratio of Chl a to Chl b of T. grandis (Table 1). Compared with the control, salt treatment significantly inhibited the growth of both T. grandis genders, which was evident from lower pigment contents and Fv/Fm values but higher ABS/RC, DIo/RC, and TRo/RC values (Figures 1, 2). Meanwhile, compared with male plants, female plants grown under salt were characterized by significantly higher Fv/Fm and pigment contents and a smaller Chl a/b ratio (Figures 1, 2). In comparison with salt-treated seedlings, SNP treatment caused an increase in pigment contents and PSII activity in both female and male T. grandis (Figures 1, 2). However, inclusion of NO scavenger (L-name) decreased this effect and again caused a reduction in Fv/Fm and pigment contents and also increased the values of ABS/RC, DIo/RC, and TRo/RC (Figures 1, 2).
Table 1. Summary of significance levels (two-way ANOVA) for the effects of gender, salt, and their interaction on the contents of photosynthetic pigments and fluorescence parameters.
Figure 1. Photosynthetic pigment contents [total chlorophyll content (A), Chl a content (B), Chl b content (C), and ratio of Chl a to Chl b (D)] measured in T. grandis in response to different treatments. CK (control, distilled water), salt (100 mM NaCl), SNP (100 mM NaCl with 0.05 mM SNP), and L-name (100 mM NaCl with 0.5 mM SNP and L-name). Different uppercase letters indicate a significant difference between different genders at p < 0.05, and different lowercase letters indicate significant difference under salt treatment at p < 0.05, according to Duncan’s test.
Figure 2. Chlorophyll fluorescence parameters (Fv/Fm: A; ABS/RC: B, DIo/RC: C, TRo/RC: D) measured in T. grandis in response to different treatments. CK (control, distilled water), salt (100 mM NaCl), SNP (100 mM NaCl with 0.05 mM SNP), and L-name (100 mM NaCl with 0.5 mM SNP and L-name). Different uppercase letters indicate a significant difference between different genders at p < 0.05, and different lowercase letters indicate significant difference under salt treatment at p < 0.05, according to Duncan’s test.
The effects of salt and NO donors on membrane damage of cells were analyzed by determining the MDA content and the relative electrolyte leakage rate, while oxidative stress was investigated in terms of the O2·− production rate and H2O2 content. Values of the MDA content, the relative electrolyte leakage rate, the O2·− production rate, and H2O2 content of T. grandis were all significantly affected by sex, salt, and their interaction (Table 2; p < 0.05). As shown in Figure 3, compared with the control, salt treatment significantly increased the MDA content, relative electrolyte leakage rate, O2·− production rate, and H2O2 content of T. grandis (p < 0.05). Meanwhile, compared with female plants, male plants grown under salt stress were characterized by significantly higher MDA content, relative electrolyte leakage rate, and O2·− production rate but reduced H2O2 content (Figure 3; p < 0.05). However, pretreatment with SNP resulted in significant decreases in these indexes compared with the salt-treated female and male plants (p < 0.05), but this was decreased by the NO scavenger under the L-name treatment.
Table 2. Summary of significance levels (two-way ANOVA) for the effects of gender, salt, and their interaction on RWC, proline content, the level of lipid peroxidation, NO content and NR activity, GSH content and GR activity, and antioxidant enzyme activities.
Figure 3. Lipid peroxidation [MDA content (A), REC (B), O2·- production rate (C), H2O2 content (D)] of T. grandis leaves in response to different treatments. CK (control, distilled water), salt (100 mM NaCl), SNP (100 mM NaCl with 0.05 mM SNP), and L-name (100 mM NaCl with 0.5 mM SNP and L-name). Different uppercase letters indicate a significant difference between different genders at p < 0.05, and different lowercase letters indicate significant difference under salt treatment at p < 0.05, according to Duncan’s test.
The physiological water status and proline content of T. grandis plants are shown in Figure 3. Both the RWC and proline content of T. grandis were significantly affected by sex, salt, and their interaction (Table 2; p < 0.05). Compared with the control, the RWC in salt-treated T. grandis leaves decreased by 9.1% (p < 0.05) in female plants and 19.8% (p < 0.05) in male plants. The NO treatment reduced the effect of salt stress on the RWC decrease in the presence of 0.2% NaCl, and it was significant in the male plants (Figure 4A). In contrast, the inclusion of the NO scavenger (L-name) decreased the effect of SNP on the water status and caused a reduction in RWC; however, these changes were not significant (p > 0.05; Figure 4A). As shown in Figure 4B, the proline content of both female and male T. grandis significantly increased under both salt and SNP treatments compared with that of CK. However, this was significantly decreased by the NO scavenger under the L-name treatment.
Figure 4. Leaf relative water (RWC) content (A) and proline content (B) of T. grandis in response to different treatments. CK (control, distilled water), salt (100 mM NaCl), SNP (100 mM NaCl with 0.05 mM SNP), and L-name (100 mM NaCl with 0.5 mM SNP and L-name). Different uppercase letters indicate a significant difference between different genders at p < 0.05, and different lowercase letters indicate significant difference under salt treatment at p < 0.05, according to Duncan’s test.
Two-way ANOVA showed that both the NR activity and NO content of T. grandis were significantly affected by sex, salt, and their interaction (Table 2; p < 0.05). Compared with male plants, female plants grown under salt were characterized by significantly higher NR activity and endogenous NO content (Figure 5; p < 0.05). As shown in Figure 5, compared with the control, salt treatment significantly increased the NR activity and endogenous NO content of T. grandis by 89.7 and 109.2%, respectively, in female plants and by 45.8 and 40.3%, respectively, in male plants. However, pretreatment with SNP before salt treatment resulted in a further increase in the NR activity (43.2 and 133.6% in female and male plants, respectively) and NO content (45.6 and 99.7% in female and male plants, respectively) in T. grandis leaves compared with the salt-treated plants (p < 0.05; Figure 5). However, the inclusion of the NO scavenger decreased the effect of SNP on NO synthesis, as it was evident that the L-name treatment reduced NR activity by 29.7 and 53.1% and NO content by 25.9 and 50.3% in female and male plants, respectively, compared to salt-stressed plants treated with SNP (p < 0.05; Figure 5).
Figure 5. Nitrate reductase (NR) activity (A) and NO content (B) of T. grandis in response to different treatments. CK (control, distilled water), salt (100 mM NaCl), SNP (100 mM NaCl with 0.05 mM SNP), and L-name (100 mM NaCl with 0.5 mM SNP and L-name). Different uppercase letters indicate a significant difference between different genders at p < 0.05, and different lowercase letters indicate significant difference under salt treatment at p < 0.05, according to Duncan’s test.
Figure 6 illustrates the endogenous GSH content and the activity of the key enzyme involved in GSH biosynthesis under different treatments. Two-way ANOVA showed that both GR activity and GSH content of T. grandis were significantly affected by sex, salt, and their interaction (Table 2; p < 0.05). Compared with female plants, male plants grown under salt stress were characterized by significantly higher GR activity and GSH content (Figure 6; p < 0.05). As shown, compared with the control, salt treatment increased the GR activity and GSH content of T. grandis by 22.5% (p > 0.05) and 40.3% (p < 0.05), respectively, in female plants and by 87.6% (p < 0.05) and 52.1% (p < 0.05), respectively, in male plants. However, pretreatment with an NO donor before salt treatment resulted in a further increase in GR activity (47.1 and 24.6% in females and males, respectively) and GSH content (63.3 and 97.7% in females and males, respectively) in T. grandis leaves relative to the salt-stressed plants (p < 0.05; Figure 6). However, the inclusion of L-name decreased the effect of NO on GSH synthesis.
Figure 6. Glutathione reductase (GR) activity (A) and GSH content (B) of T. grandis in response to different treatments. CK (control, distilled water), salt (100 mM NaCl), SNP (100 mM NaCl with 0.05 mM SNP), and L-name (100 mM NaCl with 0.5 mM SNP and L-name). Different uppercase letters indicate a significant difference between different genders at p < 0.05, and different lowercase letters indicate significant difference under salt treatment at p < 0.05, according to Duncan’s test.
Two-way ANOVA showed that the antioxidant enzyme activities of T. grandis were significantly affected by sex, salt, and their interaction (Table 2; p < 0.05). As shown in Figures 7A–D, the activities of the studied antioxidant enzymes significantly increased under salt treatment compared with the control plants. Compared with male plants, female plants grown under salt stress were characterized by significantly higher SOD and CAT activities but lower POD and APX activities (Figure 7; p < 0.05). Pretreatment with an NO donor before salt treatment further increased SOD (by 26.3% in males), POD (by 45.3 and 53.0% in females and males, respectively), CAT (by 94.8 and 312.8% in females and males, respectively), and APX (by 34.8 and 74.1% in females and males, respectively) activities compared with the salt treatment. However, the inclusion of L-name decreased the effect of NO on antioxidant enzyme activities (Figure 7). Thus, these results suggest that the modulation of antioxidant enzyme activities by an exogenous NO donor might alleviate the ROS-triggered oxidative damage caused by salt stress.
Figure 7. Antioxidant enzyme activities of T. grandis in response to different treatments. CK (control, distilled water), salt (100 mM NaCl), SNP (100 mM NaCl with 0.05 mM SNP), and L-name (100 mM NaCl with 0.5 mM SNP and L-name). Different uppercase letters indicate a significant difference between different genders at p < 0.05, and different lowercase letters indicate significant difference under salt treatment at p < 0.05, according to Duncan’s test.
In recent years, studies have shown that NO is involved in plant morphological development, root growth, photosynthesis, and the regulation of antioxidant enzyme systems, especially when plants are subjected to environmental stress (Valderrama et al., 2021; Wani et al., 2021), but few studies have focused on the effects of NO donors on dioecious plants. This study confirmed our hypothesis that NO is involved in the salt-tolerant growth of both female and male T. grandis. Salt treatment inhibited the growth of female and male T. grandis seedlings, which was indicated by a lower photosynthetic pigment content and decreased Fv/Fm but significantly higher ABS/RC, DIo/RC, and TRo/RC (Figures 1, 2). Additionally, compared with male plants, female plants showed better tolerance to salinity, as they were characterized by significantly higher Fv/Fm and pigment contents and fewer negative effects (Figures 1–3). Our results are similar to those of previous drought tests on T. grandis (Wang et al., 2021), suggesting that female T. grandis are more tolerant of stress. However, a study by Li et al. (2013) on Populus yunnanensis reported that females were more sensitive and suffered from greater negative effects than males under salt stress. Therefore, these studies suggest that sex-linked differences in the growth of dioecious plants are not consistent among species, which can be interpreted as species-specific adaptation. Pretreatment with an NO donor (SNP) caused an increase in pigment contents and PSII activity of both female and male T. grandis; however, inclusion of the NO scavenger (L-name) decreased this effect (Figures 1, 2). Thus, these results suggest that pretreatment with NO donors is a simple and effective measure to reduce the effect of salt on the growth of T. grandis. These findings are consistent with previous reports on saffron (Babaei et al., 2021), Arabidopisis thaliana (Liu et al., 2015), and Glycine max (Vaishnav et al., 2016).
Chlorophyll is an important factor in photosynthesis in plants. In this study, salt and NO treatments significantly affected chlorophyll pigments and their ratios (Figure 1). Decreases in total chlorophyll content of both female and male T. grandis were observed under salt treatment, suggesting that salt stress caused damage to photosynthetic pigments. Similar results were also reported in Mentha spicata and Oryza sativa (Amri et al., 2011; Ounoki et al., 2021). However, compared with salt treatment, NO treatment in both female and male T. grandis resulted insignificantly higher leaf chlorophyll contents, which was inconsistent with that reported in mustard and cotton (Khan et al., 2012; Liu et al., 2013). Changes in the Chl a/b ratio often indicate a change in the ratio between the light-harvesting complexes (LHC) and the reaction center complexes of the photosystem (Venema et al., 2000). The significantly increased Chl a/b ratio in both female and male T. grandis under NO treatment showed that NO might participate in inducing these plants to change the ratio of chlorophyll pigments to maintain a good photoelectron transfer system. This was confirmed by the supply of the NO scavenger (L-name), which reversed the effect of SNP on pigment accumulation and caused a reduction in total chlorophyll, Chl a, and Chl b contents (Figure 2).
Chlorophyll fluorescence parameters are often used to reflect the photosystem changes of plants in the face of environmental stress, and the measurement is convenient, accurate, and sensitive (Pashkovskiy et al., 2018). Generally, plants grown under stress have lower Fv/Fm than non-stressed plants (Baker, 2008), which is consistent with the performance of T. grandis (Figure 2). The significantly higher values of Fv/Fm under treatment with an NO donor compared with those under salt treatment indicated that pretreatment with an NO donor can effectively maintain photochemical efficiency in T. grandis seedlings. The increase in ABS/RC in both female and male T. grandis under salt treatment can be explained by a decrease in the number of active reactive centers (RC) of PSII, which might serve as a defense mechanism to reduce the burden of its systems when salt stress occurs (Strasser et al., 2010). Another effective protective mechanism for plants under salt stress is the dissipation of the absorbed light energy into heat (Baena-Gonzalez and Aro, 2002), which was confirmed by the significantly higher values of non-photochemical quenching (NPQ) per reaction center of PSII (DIo/RC) in T. grandis seedlings under salt stress (Figure 2C). Furthermore, TRo/RC was significantly higher under salt, which indicated that T. grandis seedlings under salt stress had improved the efficiency of the remaining active reaction centers. However, compared with salt-treated seedlings, NO treatment caused an increase in PSII activity in both female and male T. grandis (Figure 2), which is indicated by higher Fv/Fm and lower ABS/RC, DIo/RC, and TRo/RC. However, the inclusion of an NO scavenger (L-name) decreased this effect, caused a reduction in Fv/Fm, and increased the values of ABS/RC, DIo/RC, and TRo/RC (Figure 2). Together, these results confirm that the supply of an NO donor can maintain the photosynthetic capacity of T. grandis plants under salt stress.
When plants are stressed, the photosynthetic electron transport system produces a large number of reactive oxygen species (ROS), including O2·− and H2O2 (You and Chan, 2015). This is consistent with the significantly increased values of O2·− and H2O2 in the leaves of T. grandis under salt stress (Figure 3). Furthermore, the accumulation of ROS could lead to a break in the osmotic balance of plant cell membranes and eventually to membrane lipid peroxidation (Golldack et al., 2014). The increase in MDA content often indicates the occurrence of membrane lipid peroxidation, while the change in membrane permeability leads to an increase in relative conductivity (Li et al., 2014). Compared with female plants, male T. grandis seedlings grown under salt suffered from more negative effects, as they were characterized by a significantly higher MDA content, relative electrolyte leakage rate, and O2·− production rate but decreased H2O2 content (Figure 3; p < 0.05). These results indicated that female T. grandis were more tolerant to environmental stress than male T. grandis, which is consistent with previous results using a drought test (Wang et al., 2021). Our results also showed that the addition of SNP could effectively maintain the balance of intracellular and intracellular permeable substances and the stability of the cell membrane in both male and female T. grandis (Figure 3). This was also confirmed by the application of the NO scavenger (L-name), which again increased these parameters. These results were relatively consistent with the results in Brassica oleracea (Akram et al., 2020), broccoli (Ahmad et al., 2016), and cotton (Dong et al., 2014).
RWC commonly exhibits a decreasing trend under salt treatment (Li et al., 2014). In this study, we found that values of RWC declined under NaCl treatment, and sexual differences were significant, indicating that the leaf water situation of male and female T. grandis are quite different under salt stress. Compared with males, female T. grandis seedlings maintained significantly higher RWC under salt stress (Figure 4A), indicating that female T. grandis seedlings are more tolerant to salt stress than male plants. When plants suffer from salt stress, the accumulation of osmotic substances and proline content in leaf cells is often used as an effective means of alleviating stress. Both male and female T. grandis seedlings showed a higher accumulation of proline under salt stress, while SNP treatment further increased proline content and RWC (Figure 4). In contrast, the inclusion of the NO scavenger (L-name) decreased the effect of SNP on proline accumulation and caused a reduction in RWC, which confirmed that NO might be involved in maintaining the water content in T. grandis. A similar result was reported by Chen et al. (2021), who found that H2S-induced NO alleviated the salt stress of Cyclocarya paliurus by improving proline accumulation.
The synthesis of NO increases when plants are subjected to stress, which has been reflected in this work and in previous research (Valderrama et al., 2021; Wani et al., 2021). Meanwhile, the results of this study showed that the activity of NR and NO content increased simultaneously, indicating that NR is an important enzyme in the synthesis of NO in T. grandis (Corpas and Barroso, 2017). NR-mediated NO production has been reported to be involved in plant physiological responses under stress (Mur et al., 2013). Studies on wheat seedlings have also indicated that the application of NO donors could significantly increase both NR activity and NO content (Khan et al., 2017). Compared with male T. grandis, female T. grandis induced significantly higher NR activity and NO content under salt stress, which to some extent explained the physiological and biochemical differences between male and female T. grandis under environmental stress. However, compared with females, male T. grandis treated with exogenous SNP synthesized more NO (Figure 5B), which may help male T. grandis alleviate the damage caused by salt stress, as they have a lower tolerance. The inclusion of the NO scavenger confirmed the effect of the external SNP supply on endogenous NO synthesis, which was consistent with the results of cotton (Dong et al., 2014) and Zea mays (Kharbech et al., 2020).
One of the mechanisms through which NO participates in plant stress resistance is the activation of the antioxidant system, including the non-enzymatic and enzymatic antioxidant systems (da-Silva, C. J., Modolo, L. V., 2017; Wani et al., 2021). A large number of studies have shown that the content of non-enzymatic substances, such as phenolics and soluble proteins, in salt-treated plants can be significantly increased with the participation of NO donors (Ahmad et al., 2016; da-Silva, C. J., Modolo, L. V., 2017; Chen et al., 2021). The results of this study showed that the GSH content and GR activity of T. grandis seedlings under salt stress were significantly increased after treatment with an NO donor (Figure 6). This result is consistent with previous studies on wheat (Hasanuzzaman et al., 2011), cotton (Liu et al., 2013), and chickpea (Ahmad et al., 2016). Our previous study also showed that NO induced by hydrogen sulfide was involved in the regulation of the salt tolerance of T. grandis, which was related to the massive synthesis of different phenolic antioxidants (Chen et al., 2021). In general, the accumulation of non-enzymatic substances is beneficial for plant cells to cope with osmotic damage caused by stress.
The production of antioxidant enzymes, such as SOD, POD, CAT, and APX, in plants often keeps ROS in a stable state, and these antioxidant systems are activated when ROS are greatly increased under stress (Wakeel et al., 2020). O2·− is dissimulated to H2O2 by SOD, which is then eliminated by CAT and APX, producing H2O and O2 (Lázaro et al., 2013). Female T. grandis under salt stress showed significantly higher SOD and CAT enzyme activities, while male T. grandis showed significantly higher POD and APX enzyme activities (Figure 7). This also explains the different responses of male and female T. grandis to salt stress. Compared with female T. grandis, salt-stressed male T. grandis had significantly higher SOD, POD, CAT, and APX enzyme activities after SNP treatment. This suggests that NO might be more effective in alleviating the salt stress of male T. grandis by improving the activities of antioxidant enzymes. These results also indicated that the increase in the activity of these antioxidant enzymes mediated by SNP might be due to post-translational modifications (PTM), such as persulfidation or S-nitrosation (Singh et al., 2017; Alamri et al., 2020).
Overall, our study showed that female T. grandis showed better tolerance to salinity, as they were characterized by significantly higher RWC, pigment contents, and Fv/Fm and fewer negative effects. This is associated with higher nitrate reductase (NR) activity and NO content. Pretreatment with an NO donor alleviated salt-induced oxidative damage of T. grandis, especially male T. grandis, as indicated by lowered lipid peroxidation through an enhanced antioxidant system, including proline and glutathione accumulation, and increased antioxidant enzyme activities. However, the ameliorating effect of the NO donor was not effective in the presence of an NO scavenger. The present study provides new evidence to contribute to the current understanding of NO-induced salt stress tolerance and the role of NO signaling in dioecious plants.
The raw data supporting the conclusions of this article will be made available by the authors, without undue reservation.
YL is responsible for the whole process of experimenting and writing the paper. SJ provide experimental guidance and amend the manuscript. JK, YT, and DW help to do the experiment. All authors contributed to the article and approved the submitted version.
This work was supported by the National Key Research and Development Project (2019YFE0118900), the National Natural Science Foundation of China (No. 32001305), Jiangsu Province Natural Science Foundation (No. BK20200771), “Pioneer” and “Leading Goose” R&D Program in Universities of Zhejiang (2022), and Jiyang College of Zhejiang A & F University under Grant (No. RQ2020B14; JYKC2117).
The authors declare that the research was conducted in the absence of any commercial or financial relationships that could be construed as a potential conflict of interest.
All claims expressed in this article are solely those of the authors and do not necessarily represent those of their affiliated organizations, or those of the publisher, the editors and the reviewers. Any product that may be evaluated in this article, or claim that may be made by its manufacturer, is not guaranteed or endorsed by the publisher.
We thank LetPub (www.letpub.com) for its linguistic assistance during the preparation of this manuscript.
Ahmad, P., Abdel Latef, A. A., Hashem, A., Abd_Allah, E. F., Gucel, S., and Tran, L. S. P. (2016). Nitric oxide mitigates salt stress by regulating levels of osmolytes and antioxidant enzymes in chickpea. Front. Plant Sci. 7:347. doi: 10.3389/fpls.2016.00347
Akram, N. A., Hafeez, N., Farid-Ul-Haq, M., Ahmad, A., Sadiq, M., and Ashraf, M. (2020). Foliage application and seed priming with nitric oxide causes mitigation of salinity-induced metabolic adversaries in broccoli (Brassica oleracea L.) plants. Acta Physiol. Plant. 42:155. doi: 10.1007/s11738-020-03140-x
Alamri, S., Ali, H. M., Khan, M. I. R., Singh, V. P., and Siddiqui, M. H. (2020). Exogenous nitric oxide requires endogenous hydrogen sulfide to induce the resilience through sulfur assimilation in tomato seedlings under hexavalent chromium toxicity. Plant Physiol. Biochem. 155, 20–34. doi: 10.1016/j.plaphy.2020.07.003
Amri, E., Mirzaei, M., Moradi, M., and Zare, K. (2011). The effects of spermidine and putrescine polyamines on growth of pomegranate (Punica granatum L. cv ‘Rabbab’) in salinity circumstance. Int. J. Plant Physiol. Biochem. 3, 43–49. doi: 10.5897/IJPPB.9000044
Arnon, D. I. (1949). Copper enzymes in isolated chloroplasts. Polyphenoloxidase in Beta vulgaris. Plant Physiol 24, 1–15. doi: 10.1104/pp.24.1.1
Astier, J., Gross, I., and Durner, J. (2018). Nitric oxide production in plants: an update. J. Exp. Bot. 69, 3401–3411. doi: 10.1093/jxb/erx420
Babaei, S., Niknam, V., and Behmanesh, M. (2021). Comparative effects of nitric oxide and salicylic acid on salinity tolerance in saffron (Crocus sativus). Plant Biosyst. 155, 73–82. doi: 10.1080/11263504.2020.1727975
Baena-Gonzalez, E., and Aro, E. M. (2002). Biogenesis, assembly and turnover of photosystem II units. Philos. Trans. R. Soc. Lond. B Biol. Sci. 357, 1451–1460. doi: 10.1098/rstb.2002.1141
Baker, N. R. (2008). Chlorophyll fluorescence: a probe of photosynthesis in vivo. Annu. Rev. Plant Biol. 59, 89–113. doi: 10.1146/annurev.arplant.59.032607.092759
Bates, L. S., Waldren, R. P., and Teare, I. D. (1973). Rapid determination of free proline for water-stress studies. Plant Soil 39, 205–207. doi: 10.1007/BF00018060
Beauchamp, C., and Fridovich, I. (1971). Superoxide dismutase: improved assays and an assay applicable to acrylamidegels. Anal. Biochem. 44, 276–287. doi: 10.1016/0003-2697(71)90370-8
Begara-Morales, J. C., Sánchez-Calvo, B., Luque, F., Leyva-Pérez, M. O., Leterrier, M., Corpas, F. J., et al. (2014). Differential transcriptomic analysis by RNA-Seq of GSNO-responsive genes between Arabidopsis roots and leaves. Plant Cell Physiol. 55, 1080–1095. doi: 10.1093/pcp/pcu044
Bhatnagar-Mathur, P., Vadez, V., and Sharma, K. K. (2008). Transgenic approaches for abiotic stress tolerance in plants: retrospect and prospects. Plant Cell Rep. 27, 411–424. doi: 10.1007/s00299-007-0474-9
Bradford, M. M. (1976). A rapid and sensitive method for the quantitation of microgram quantities of protein utilizing the principle of protein-dye binding. Anal. Biochem. 72, 248–254. doi: 10.1016/0003-2697(76)90527-3
Cantrel, C., Vazquez, T., Puyaubert, J., Rezé, N., Lesch, M., Kaiser, W. M., et al. (2011). Nitric oxide participates in cold-responsive phosphosphingolipid formation and gene expression in Arabidopsis thaliana. New Phytol. 189, 415–427. doi: 10.1111/j.1469-8137.2010.03500.x
Chen, F., Shen, J., Min, D., Ke, L., Tian, X., Korpelainen, H., et al. (2018). Male Populus cathayana than female shows higher photosynthesis and less cellular injury through ABA-induced manganese transporting inhibition under high manganese condition. Trees 32, 255–263. doi: 10.1007/s00468-017-1628-1
Chen, P., Yang, W., Jin, S., and Liu, Y. (2021). Hydrogen sulfide alleviates salinity stress in Cyclocarya paliurus by maintaining chlorophyll fluorescence and regulating nitric oxide level and antioxidant capacity. Plant Physiol. Biochem. 167, 738–747. doi: 10.1016/j.plaphy.2021.09.004
Corpas, F. J., and Barroso, J. B. (2015). Functions of nitric oxide (NO) in roots during development and under adverse stress conditions. Plan. Theory 4, 240–252. doi: 10.3390/plants4020240
Corpas, F. J., and Barroso, J. B. (2017). Nitric oxide synthase-like activity in higher plants. Nitric Oxide 68, 5–6. doi: 10.1016/j.niox.2016.10.009
da Silva, C. J., Batista, F. E. P., and Modolo, L. V. (2017). Salinity-induced accumulation of endogenous H2S and NO is associated with modulation of the antioxidant and redox defense systems in Nicotiana tabacum L. cv. Havana. Plant Sci. 256, 148–159. doi: 10.1016/j.plantsci.2016.12.011
da-Silva, C. J., and Modolo, L. V. (2017). Hydrogen sulfide: a new endogenous player in an old mechanism of plant tolerance to high salinity. Act. Bot. Bras. 32, 150–160. doi: 10.1590/0102-33062017abb0229
Deng, Y., and Shen, S., Chen, CF., Cheng, X., and Zhang, F. (2011). The embryo rescue derived intergeneric hybrid between chrysanthe-mum and Ajania przewalskii shows enhanced cold tolerance. Plant Cell Rep. 30, 2177–2186. doi:doi: 10.1007/s00299-011-1123-x
Dong, Y., Jinc, S., Liu, S., Xu, L., and Kong, J. (2014). Effects of exogenous nitric oxide on growth of cotton seedlings under NaCl stress. J. Soil Sci. Plant Nutr. 14, 1–13. doi: 10.4067/S0718-95162014005000001
Fang, Y. R., and Xue, L. (2019). Research advances in the effect of salt stress on plant chlorophyll fluorescence. Eco. Sci. 38, 225–234. (in Chinese)
Golldack, D., Li, C., Mohan, H., and Probst, N. (2014). Tolerance to drought and salt stress in plants: unraveling the signaling networks. Front. Plant Sci. 5:151. doi: 10.3389/fpls.2014.00151
Hasanuzzaman, M., Hossain, M. A., and Fujita, M. (2011). Nitric oxide modulates antioxidant defense and the methylglyoxal detoxification system and reduces salinity-induced damage of wheat seedlings. Plant Biotechnol. Rep. 5, 353–365. doi: 10.1007/s11816-011-0189-9
He, M., Shi, D., Wei, X., Hu, Y., Wang, T., and Xie, Y. (2016). Gender-related differences in adaptability to drought stress in the dioecious tree Ginkgo biloba. Acta Physiol. Plant. 38, 1–14. doi: 10.1007/s11738-016-2148-0
Hu, Y., Zhang, Z., Hua, B., Tao, L., Chen, W., Gao, Y., et al. (2022). The interaction of temperature and relative humidity affects the main aromatic components in postharvest Torreya grandis nuts. Food Chem. 368:130836. doi: 10.1016/j.foodchem.2021.130836
Jaworski, E. G. (1971). Nitrate reductase assay in intact plant tissues. Biochem. Biophys. Res. Commun. 43, 1274–1279. doi: 10.1016/S0006-291X(71)80010-4
Khan, M. N., Mobin, M., Abbas, Z. K., and Siddiqui, M. H. (2017). Nitric oxide-induced synthesis of hydrogen sulfide alleviates osmotic stress in wheat seedlings through sustaining antioxidant enzymes, osmolyte accumulation and cysteine homeostasis. Nitric Oxide 68, 91–102. doi: 10.1016/j.niox.2017.01.001
Khan, M. N., Siddiqui, M. H., Mohammad, F., and Naeem, M. (2012). Interactive role of nitric oxide and calcium chloride in enhancing tolerance to salt stress. Nitric Oxide 27, 210–218. doi: 10.1016/j.niox.2012.07.005
Kharbech, O., Sakouhi, L., Massoud, M. B., Mur, L. A. J., Corpas, F. J., Djebali, W., et al. (2020). Nitric oxide and hydrogen sulfide protect plasma membrane integrity and mitigate chromium-induced methylglyoxal toxicity in maize seedlings. Plant Physiol. Biochem. 157, 244–255. doi: 10.1016/j.plaphy.2020.10.017
Kopyra, M., and Gwózdz, E. A. (2003). Nitric oxide stimulates seed germination and counteracts the inhibitory effect of heavy metals and salinity on root growth of Lupinus luteus. Plant Physiol. Biochem. 41, 1011–1017. doi: 10.1016/j.plaphy.2003.09.003
Laxalt, A. M., García-Mata, C., and Lamattina, L. (2016). The dual role of nitric oxide in guard cells: promoting and attenuating the ABA and phospholipidderived signals leading to the stomatal closure. Front. Plant Sci. 7:476. doi: 10.3389/fpls.2016.00476
Lázaro, J. J., Jiménez, A., Camejo, D., Martí, M. C., Lázaro-Payo, A., Barranco-Medina, S., et al. (2013). Dissecting the integrative antioxidant and redox systems in plant mitochondria. Effect of stress and S-nitrosylation. Front. Plant Sci. 4:460. doi: 10.3389/fpls.2013.00460
Li, T., Hu, Y., Du, X., Tang, H., Shen, C., and Wu, J. (2014). Salicylic acid alleviates the adverse effects of salt stress in Torreya grandis cv. Merrillii seedlings by activating photosynthesis and enhancing antioxidant systems. PLoS One 9:e109492. doi: 10.1371/journal.pone.0109492
Li, H., Shi, J., Wang, Z., Zhang, W., and Yang, H. (2020). H2S pretreatment mitigates the alkaline salt stress on Malus hupehensis roots by regulating Na+/K+ homeostasis and oxidative stress. Plant Physiol. Biochem. 156, 233–241. doi: 10.1016/j.plaphy.2020.09.009
Li, L., Zhang, Y., Luo, J., Korpelainen, H., and Li, C. (2013). Sex-specific responses of Populus yunnanensis exposed to elevated CO2 and salinity. Physiol. Plant. 147, 477–488. doi: 10.1111/j.1399-3054.2012.01676.x
Liu, S., Dong, Y. J., Xu, L. L., Kong, J., and Bai, X. Y. (2013). Roles of exogenous nitric oxide in regulating ionic equilibrium and moderating oxidative stress in cotton seedlings during salt stress. J. Soil Sci. Plant Nut. 13, 929–941. doi: 10.4067/S0718-95162013005000073
Liu, W., Li, R. J., Han, T. T., Cai, W., Fu, Z. W., and Lu, Y. T. (2015). Salt stress reduces root meristem size by nitric oxide-mediated modulation of auxin accumulation and signaling in Arabidopsis. Plant Physiol. 168, 343–356. doi: 10.1104/pp.15.00030
Mantri, N., Patade, V., Penna, S., Ford, R., and Pang, E. (2012). “Abiotic stress responses in plants: present and future,” in Abiotic Stress Responses in Plants. ed. H. Zhang (New York: Springer), 1–19.
Mostofa, M. G., Saegusa, D., Fujita, M., and Tran, L. S. P. (2015). Hydrogen sulfide regulates salt tolerance in rice by maintaining Na+/K+ balance, mineral homeostasis and oxidative metabolism under excessive salt stress. Front. Plant Sci. 6:1055. doi: 10.3389/fpls.2015.01055
Mur, L. A., Mandon, J., Persijn, S., Cristescu, S. M., Moshkov, I. E., Novikova, G. V., et al. (2013). Nitric oxide in plants: an assessment of the current state of knowledge. AoB Plants 5, 1–17. doi: 10.1093/aobpla/pls052
Nakano, Y., and Asada, K. (1981). Hydrogen peroxide is scavenged by ascorbate-specific peroxidase in spinach chloroplasts. Plant Cell Physiol. 22, 867–880. doi: 10.1093/oxfordjournals.pcp.a076232
Ounoki, R., Ágh, F., Hembrom, R., Ünnep, R., Szögi-Tatár, B., Böszörményi, A., et al. (2021). Salt stress affects plastid ultrastructure and photosynthetic activity but not the essential oil composition in spearmint (Mentha spicata L. var. crispa “Moroccan”). Front. Plant Sci. 12:739467. doi: 10.3389/fpls.2021.739467
Pashkovskiy, P. P., Soshinkova, T. N., Korolkova, D. V., Kartashov, A. V., Zlobin, I. E., and Lyubimov, V. Y. (2018). The effect of light quality on the pro−/antioxidant balance, activity of photosystem II, and expression of lightdependent genes in Eutrema salsugineum callus cells. Photosynth. Res. 136, 199–214. doi: 10.1007/s11120-017-0459-7
Patterson, B. D., MacRae, E. A., and Ferguson, I. B. (1984). Estimation of hydrogen peroxide in plant extracts using titanium (IV). Anal. Biochem. 139, 487–492. doi: 10.1016/0003-2697(84)90039-3
Rana, S., and Liu, Z. (2021). Study on the pattern of vegetative growth in young dioecious trees of Idesia polycarpa maxim. Trees 35, 69–80. doi: 10.1007/s00468-020-02013-7
Ren, Y., Wang, W., He, J., Zhang, L., Wei, Y., and Yang, M. (2020). Nitric oxide alleviates salt stress in seed germination and early seedling growth of pakchoi (Brassica chinensis L.) by enhancing physiological and biochemical parameters. Ecotoxicol. Environ. Saf. 187:109785. doi: 10.1016/j.ecoenv.2019.109785
Retuerto, R., Lema, B. F., Roiloa, S. R., and Obeso, J. R. (2000). Gender, light and water effects in carbon isotope discrimination, and growth rates in the dioecious tree Ilex aquifolium. Funct. Ecol. 14, 529–537. doi: 10.1046/j.1365-2435.2000.t01-1-00454.x
Ruan, H., Shen, W., Ye, M., and Xu, L. (2002). Protective effects of nitric oxide on salt stress-induced oxidative damage to wheat (Triticum aestivum L.) leaves. Chi. Sci. Bull. 47, 677–681. doi: 10.1360/02tb9154
Shen, J., Li, X., Zhu, X., Ding, Z., Huang, X., Chen, X., et al. (2022). Molecular and photosynthetic performance in the yellow leaf mutant of Torreya grandis according to transcriptome sequencing, chlorophyll a fluorescence, and modulated 820 nm reflection. Cell 11:431. doi: 10.3390/cells11030431
Singh, M., Kushwaha, B. K., Singh, S., Kumar, V., Singh, V. P., and Prasad, S. M. (2017). Sulphur alters chromium (VI) toxicity in Solanum melongena seedlings: role of Sulphur assimilation and Sulphur-containing antioxidants. Plant Physiol. Biochem. 112, 183–192. doi: 10.1016/j.plaphy.2016.12.024
Song, L., Meng, X., Yang, L., Ma, Z., Zhou, M., Yu, C., et al. (2022). Identification of key genes and enzymes contributing to nutrition conversion of Torreya grandis nuts during post-ripening process. Food Chem. 384:132454. doi: 10.1016/j.foodchem.2022.132454
Strasser, R. J., Tsimilli-Michael, M., Qiang, S., and Goltsev, V. (2010). Simultaneous in vivo recording of prompt and delayed fluorescence and 820-nm reflection changes during drying and after rehydration of the resurrection plant Haberlea rhodopensis. Biochim. Biophys. Acta 1797, 1313–1326. doi: 10.1016/j.bbabio.2010.03.008
Suda, O., Tsutsui, M., Morishita, T., Tanimoto, A., Horiuchi, M., Tasaki, H., et al. (2002). Long-term treatment with Nω-nitro-L-arginine methyl ester causes arteriosclerotic coronary lesions in endothelial nitric oxide synthase-deficient mice. Circulation 106, 1729–1735. doi: 10.1161/01.CIR.0000029749.16101.44
Susanne-S, R., and Ricklefs, R.-E. (1995). Dioecy and its correlates in the flowering plants. Am. J. Bot. 82, 596–606. doi: 10.1002/j.1537-2197.1995.tb11504.x
Todd-E, D., and Bliss, L.-C. (1989). Patterns of water use and the tissue water relations in the dioecious shrub, Salix arctica: the physiological basis for habitat partitioning between the sexes. Oecologia 79, 332–343. doi: 10.1007/BF00384312
Vaishnav, A., Kumari, S., Jain, S., Varma, A., Tuteja, N., and Choudhary, D. K. (2016). PGPR-mediated expression of salt tolerance gene in soybean through volatiles under sodium nitroprusside. J. Basic Microbiol. 56, 1274–1288. doi: 10.1002/jobm.201600188
Valderrama, R., Chaki, M., Begara-Morales, J. C., Petrivalský, M., and Barroso, J. B. (2021). Nitric oxide in plants. Front. Plant Sci. 12:705157. doi: 10.3389/fpls.2021.705157
Venema, J. H., Villerius, L., and van Hasselt, P. R. (2000). Effect of acclimation to suboptimal temperature on chilling-induced photodamage: comparison between a domestic and a high-altitude wild Lycopersicon species. Plant Sci. 152, 153–163. doi: 10.1016/S0168-9452(99)00228-9
Wakeel, A., Xu, M., and Gan, Y. (2020). Chromium-induced reactive oxygen species accumulation by altering the enzymatic antioxidant system and associated cytotoxic, genotoxic, ultrastructural, and photosynthetic changes in plants. Int. J. Mol. Sci. 21:728. doi: 10.3390/ijms21030728
Wang, A. G. (1990). Quantitative relation between the reaction of hydroxylamine and superoxi-de anion radicals in plants. Plant Physiol. Commun. 26, 55–57.
Wang, Q. Z., Liu, Q., Gao, Y. N., and Liu, X. (2017). Review on the mechanisms of the response to salinity-alkalinity stress in plants. Acta Ecol. Sin. 37, 5565–5577.
Wang, J., Liu, Y., Xu, Y., Chen, W., Han, Y., Wang, G. G., et al. (2021). Sexual differences in gas exchange and chlorophyll fluorescence of Torreya grandis under drought stress. Trees 36, 1–12. doi: 10.1007/s00468-021-02205-9
Wani, K. I., Naeem, M., Castroverde, C. D. M., Kalaji, H. M., Albaqami, M., and Aftab, T. (2021). Molecular mechanisms of nitric oxide (NO) signaling and reactive oxygen species (ROS) homeostasis during abiotic stresses in plants. Int. J. Mol. Sci. 22:9656. doi: 10.3390/ijms22179656
Watanabe, N., Iwamoto, T., Bowen, K. D., Dickinson, D. A., Torres, M., and Forman, H. J. (2003). Bio-effectiveness of tat-catalase conjugate: a potential tool for the identification of H2O2-dependent cellular signal transduction pathways. Biochem. Biophys. Res. Commun. 303, 287–293. doi: 10.1016/S0006-291X(03)00335-8
You, J., and Chan, Z. (2015). ROS regulation during abiotic stress responses in crop plants. Front. Plant Sci. 6:1092. doi: 10.3389/fpls.2015.01092
Yu, W., Liu, Y., Song, L., Jacobs, D. F., Du, X., Ying, Y., et al. (2017). Effect of differential light quality on morphology, photosynthesis, and antioxidant enzyme activity in Camptotheca acuminata seedlings. J. Plant Growth Regul. 36, 148–160. doi: 10.1007/s00344-016-9625-y
Keywords: antioxidant capacity, Torreya grandis, nitric oxide, sexual difference, salt stress
Citation: Liu Y, Jiang Z, Ye Y, Wang D and Jin S (2022) Enhanced Salt Tolerance of Torreya grandis Genders Is Related to Nitric Oxide Level and Antioxidant Capacity. Front. Plant Sci. 13:906071. doi: 10.3389/fpls.2022.906071
Received: 28 March 2022; Accepted: 20 April 2022;
Published: 12 May 2022.
Edited by:
Mirza Hasanuzzaman, Sher-e-Bangla Agricultural University, BangladeshCopyright © 2022 Liu, Jiang, Ye, Wang and Jin. This is an open-access article distributed under the terms of the Creative Commons Attribution License (CC BY). The use, distribution or reproduction in other forums is permitted, provided the original author(s) and the copyright owner(s) are credited and that the original publication in this journal is cited, in accordance with accepted academic practice. No use, distribution or reproduction is permitted which does not comply with these terms.
*Correspondence: Yang Liu, eWFuZ2xpdTg5MTBAemFmdS5lZHUuY24=; dGFvamkubGl1eWFuZ0BxcS5jb20=; Songheng Jin, c2hqaW5AemFmdS5lZHUuY24=
Disclaimer: All claims expressed in this article are solely those of the authors and do not necessarily represent those of their affiliated organizations, or those of the publisher, the editors and the reviewers. Any product that may be evaluated in this article or claim that may be made by its manufacturer is not guaranteed or endorsed by the publisher.
Research integrity at Frontiers
Learn more about the work of our research integrity team to safeguard the quality of each article we publish.