- 1Key Laboratory of National Forestry and Grassland Administration for Orchid Conservation and Utilization at College of Landscape Architecture, Fujian Agriculture and Forestry University, Fuzhou, China
- 2College of Biological Sciences and Technology, Beijing Forestry University, Beijing, China
The establishment of lateral organs and subsequent plant architecture involves factors intrinsic to the stem apical meristem (SAM) from which they are derived. KNOTTED1-LIKE HOMEOBOX (KNOX) genes are a family of plant-specific homeobox transcription factors that especially act in determining stem cell fate in SAM. Although KNOXs have been studied in many land plants for decades, there is a dearth of knowledge on KNOX’s role in Orchidaceae, the largest and most diverse lineage of flowering plants. In this study, a total of 32 putative KNOX genes were identified in the genomes of five orchid species and further designated into two classes (Class I and Class II) based on phylogenetic relationships. Sequence analysis showed that most orchid KNOX proteins retain four conserved domains (KNOX1, KNOX2, ELK, and Homeobox_KN). Comparative analysis of gene structure showed that the exon–intron structure is conserved in the same clade but most orchids exhibited longer intron, which may be a unique feature of Orchidaceae. Cis-elements identified in the promoter region of orchid KNOXs were found mostly enriched in a function of light responsiveness, followed by MeJA and ABA responsiveness, indicative of their roles in modulating light and phytohormones. Collinear analysis unraveled a one-to-one correspondence among KNOXs in orchids, and all KNOX genes experienced strong purifying selection, indicating the conservation of this gene family has been reinforced across the Orchidaceae lineage. Expression profiles based on transcriptomic data and real-time reverse transcription–quantitative PCR (RT-qPCR) revealed a stem-specific expression of KNOX Class I genes and a broader expression pattern of Class II genes. Taken together, our results provided a comprehensive analysis to uncover the underlying function of KNOX genes in Orchidaceae.
Introduction
Homeoproteins identified in almost all eukaryotic lineages are categorized into two superclasses, Three Amino Acid Length Extension (TALE) and non-TALE (Lee et al., 2008). KNOTTED1-like homeobox (KNOX) genes are one of the TALE superclasses in plants, encoding transcription factors that regulate stem-cell specification, particularly at shoot apical meristem (SAM). KNOX proteins are characterized by four conserved domains: TALE-type homeodomain (Homeobox_KN) at C-terminal; MEINOX domain includes KNOX1 and KNOX2 at N-terminal; and an ELK domain located upstream of homeodomain (Meng et al., 2020). KNOX1 and KNOX2 domains have been previously shown to participate in protein–protein interactions (Reiser et al., 2000). The ELK domain could act as a nuclear localization signal for transcriptional repression, and the homeodomain may function in the recognition of promoter sequences in downstream genes (Bueno et al., 2020). On the basis of the homeodomain similarity, intron, expression patterns, and phylogeny, this small gene family is split into three classes: Class I, Class II, and a newly discovered Class M (Magnani and Hake, 2008). Representatives of Class I and Class II genes are present in bryophytes and all flowering plants, which exhibit conserved but opposing roles (Frangedakis et al., 2017).
Recent studies have shed new light on the function of KNOX genes in several plants including gymnosperms such as Pinus pinaster (Bueno et al., 2020), monocots such as rice (Chen et al., 2021) and Lilium tsingtauense (Zhou et al., 2022), dicots such as Malus pumila (Jia et al., 2021a), while extensive research has only been done in Arabidopsis thaliana (Furumizu et al., 2015; Qin et al., 2020). In A. thaliana, Class I subfamily consists of four members, SHOOT MERISTEMLESS (STM), KNAT1, KNAT2, and KNAT6 (Hake et al., 2004). These genes are specifically expressed in SAM, which harbors pluripotent stem cells to maintain an indeterminate state but switched off in determinate lateral organs. STM functions in shoot apical meristem, maintaining floral meristem and carpel formation with stm mutants fail to specify and maintain a SAM (Scofield et al., 2007). KNAT1, KNAT2, and KNAT6 act redundantly with STM in concert to regulate stem cell maintenance, carpel formation, and embryonic SAM boundaries, respectively (Hay and Tsiantis, 2009). The loss of function of these genes results in irregular floral structure, shortened internodes, and reduced apical dominance (Venglat et al., 2002). Class II KNOX genes include KNAT3, KNAT4, KNAT5, and KNAT7, by contrast, have a broader expression in differentiating and mature tissues. Genetic analyses demonstrate that Class II KNOX genes promote differentiation of all aerial organs whilst suppressing meristematic capability, suggesting that they act antagonistically with Class I genes, but their functions remain largely unknown due to the paucity of studies and extensive genetic redundancy (Furumizu et al., 2015; Wang et al., 2020). KNATM, the only member of Class M found in some eudicots (Jie et al., 2015), is featured by the absence of ELK–homeodomain region and has a function in leaf proximal–distal patterning (Magnani and Hake, 2008).
Organ initiation from meristems under differing regulating mechanisms could give rise to dramatically distinct morphologies and KNOX genes, with no doubt having essential roles in these processes (Hay and Tsiantis, 2010; Sluis and Hake, 2015). With approximately 750 genera and 28,000 species, Orchidaceae represents one of the largest, most widespread, and species-rich families of angiosperm lineages (Chase et al., 2015). Orchids are also one of the most prestigious, horticulturally significant plants owing to their unique morphology and diversity. Despite extensive studies of KNOX genes that have been done within model plants, little is known about the features of KNOX genes in Orchidaceae. As high-quality, chromosomal-level orchid genomes have emerged recently, the opportunity arises to conduct the systematic study of the orchid KNOX family and investigate its expression in meristems during development and its association with plant architecture. In this study, we perform genome-wide identification, comparative and expression analyses of KNOX genes in five orchids, Apostasia shenzhenica (Apostasioideae, the primitive subfamily of Orchidaceae), Phalaenopsis equestris, Cymbidium ensifolium, Cymbidium goeringii, and Dendrobium chrysotoxum (Epidendroideae, the advanced subfamily with most diverse species) to illustrate the characteristics of KNOX genes during the evolution of orchids. The results could provide novel insights into the fundamental mechanisms underlying the organ morphology evolution and diversification of orchids and other flowering plants.
Materials and Methods
Data Sources
Genome sequences, annotation files, and raw data of transcriptome from different tissues of A. shenzhenica (accession number: PRJNA310678), P. equestris (accession number: PRJNA53913), C. goeringii (accession number: PRJNA749652), and D. chrysotoxum (accession number: PRJNA664445) were downloaded from the National Center for Biotechnology Information (NCBI), and data for C. ensifolium (accession number: PRJCA005355) was downloaded from the National Genomics Data Center (NGDC).1 KNOX proteins of A. thaliana and Oryza sativa were retrieved from TAIR2 and Phytozome,3 respectively.
Identification and Physicochemical Properties of KNOX Genes
A local BLASTp search was conducted using A. thaliana KNOX proteins as the query. Four conserved domains of KNOX: PF05920 (Homeobox KN domain), PF03790 (KNOX1 domain), PF03791 (KNOX2 domain), and PF03789 (ELK domain) were downloaded from the online database4 (Finn et al., 2010) to perform HMMER search (default parameters). Truncated and redundant proteins were manually removed after combining the BLASTp and HMMER results. NCBI Batch CD Search Tool5 was used for verifying the presence of the KNOX domain in candidate orchid KNOXs. The completed protein sequences of orchid KNOXs can be found in Supplementary Data Sheet. The physicochemical properties of KNOX genes were predicted by ExPASy database (Artimo et al., 2012). Subcellular localization was predicted by Plant-mPloc (Chou and Shen, 2010).
Phylogenetic Analysis
The KNOX protein sequences of A. thaliana, O. sativa, A. shenzhenica, P. equestris, C. ensifolium, C. goeringii, and D. chrysotoxum were aligned with MAFFT (Rozewicki et al., 2019). The maximum likelihood (ML) method was adopted for constructing a phylogenetic tree using RAxML on the CIPRES Science Gateway web server (RAxML-HPC2 on XSEDE; Miller et al., 2015) under the Protein CAT model and GTR matrix with 1,000 bootstrap iterations. The output phylogenetic tree file was polished using Evolview (He et al., 2016).
Motif and Gene Structure Analysis
GSDS6 (Hu et al., 2015) was used for analyzing KNOX gene structure. Conserved motifs in KNOX sequences were identified using MEME online tool7 (Bailey et al., 2009) with default parameters. Motif and gene structure of KNOX genes were visualized by TBtools (Chen et al., 2020).
Protein Tertiary Structure Prediction
Protein tertiary structure prediction of orchid KNOXs was performed and visualized by SWISS-MODEL (Schwede et al., 2003).8 The tertiary structure was colored by rainbow order representing N to C terminus. The secondary structure was predicted by the SOPMA program9 (Geourjon and Deléage, 1995).
Prediction of Cis-Acting Elements
A total of 2,000 bp upstream of all orchid KNOXs were extracted by TBtools (Chen et al., 2020). The online software PlantCARE (Lescot et al., 2002)10 was employed to identify and annotate the cis-acting elements found in the promoter regions. Cis-acting element numbers and responsive functions were visualized using TBtools (Chen et al., 2020).
Collinearity and Selective Pressure
Given the chromosome-level genome assembly of C. ensifolium, C. goeringii, and D. chrysotoxum, genomic fasta files were merged pairwise to construct a database and query for BLASTp. The merged blast files and modified gff3 files of three species were examined using MCscanX (Wang et al., 2012) to identify the collinear blocks of KNOX genes between D. chrysotoxum and C. goeringii, C. goeringii and C. ensifolium. Dual_synteny_plotter tool of MCscanX (JCVI kit) was used to visualize the collinearity results. To assess the selection pressure of orchid KNOXs, gene pairs with similarities greater than 60% were identified by multiple sequence alignment using DNAman (Lynnon Corporation, Canada) with default parameters. Tbtools (Chen et al., 2020) was further used to calculate Ka (non-synonymous substitutions per site), Ks (synonymous substitutions per site), and Ka/Ks (evolutionary constraint) values.
Expression Analysis
For transcriptomic analysis, RSEM (Li and Dewey, 2011) was used for transcript quantification and calculating the fragment per kilobase of transcript per million mapped reads (FPKM) value for each gene. Heatmaps using FPKM matrix were generated using tbtools (Chen et al., 2020).
To verify the expression pattern of KNOX genes, tender leaves, fully opened flowers, and mature pseudobulbs (stems) were sampled from P. equestris, C. ensifolium, C. goeringii, and D. chrysotoxum that were planted in Fujian Agriculture and Forestry University for RT-PCR analysis. Each tissue type was sampled in three replicates. Total RNA of these tissues was extracted using the RNAsimple Plant Kit. The RNA concentration for each tissue was in a range of 93–456 ng/μl with A260/280 value range from 1.92 to 2.15, indicating the extracted RNA is of high quality. Primer3Plus online tool11 was used to design specific PCR primers. Gene-specific primers for four selected genes and their corresponding internal reference genes are listed in Supplementary Table 1. Vazyme/R223 and Yeasen/11202ES03 kits were used for cDNA synthesis and qPCR, respectively. RT-qPCR was performed to verify the specific expression of Class I genes in the stem using STM homologs in orchids (JL001208, GL17614, Peq022474, Maker101342). All experiments were performed in three biological replicates with three technical replicates. The relative gene expression was calculated using the 2–ΔΔCT method.
Gene Ontology Analysis of Orchid KNOXs
EggNOG-mapper v212 was used to search against the eggNOG5.0 database (Huerta-Cepas et al., 2019) for gene ontology (GO) functional annotation. Orthology was predicted by sequence alignment, and bit-score or E-value setting was used to filter the low quality of orthology assignments; and functional classification was obtained based on the GO annotation terms associated with the proteins involved in known biological processes.
Results
Identification and Protein Features of Orchid KNOXs
A total of 32 putative KNOX genes (five in A. shenzhenica, six in P. equestris, five in C. ensifolium, nine in C. goeringii, and seven in D. chrysotoxum) were identified concerning four conserved domains characteristic of KNOX proteins, as previously detailed in the “Materials and Methods” section. These KNOX sequences varied considerably in the number of amino acids (aa), ranging from 85 to 928 with the molecular weight (MW) of which within a range of 9.81 to 102.35 kDa (Table 1). In addition, the deduced grand average of hydrophilic (GRAVY) values was all negative in five orchids’ KNOX proteins, suggestive of strong hydrophilicity. Except for four genes (C. goeringii (GL09754), P. equestris (Peq027115), A. shenzhenica (Ash015432 and Ash008116)) that exhibited isoelectric points (pI) higher than eight, all other members were weakly acidic (ranging from 4.87 to 7.09). Most of them have the instability index (II) over 40, indicating that these proteins are unstable (Gasteiger et al., 2005). The results from subcellular location predictions showed that all KNOX proteins were located in nucleus, implying they may function on nucleus similar to most transcription factors (Table 1).
Phylogeny and Classification of KNOX Genes
The phylogenetic tree constructed by A. thaliana, O. sativa, A. shenzhenica, P. equestris, C. ensifolium, C. goeringii, and D. chrysotoxum has divided the KNOX genes into three clades, Class I, Class II, and Class III (Class M) (Figure 1), which is consistent with the studies have been done in Arabidopsis, rice, and Populus (Xiong et al., 2018). Among which, Class I was further divided into three subclades, designated as IA, IB, and IC, having eight, five, and 12 members in orchids, respectively. Class II comprises two subclades: IIA and IIB, including five and two members in orchids, respectively. No member of Class M (KNATM) was found in orchids, in accordance with a recent study that KNATM was exclusively found in eudicots (Jie et al., 2015).
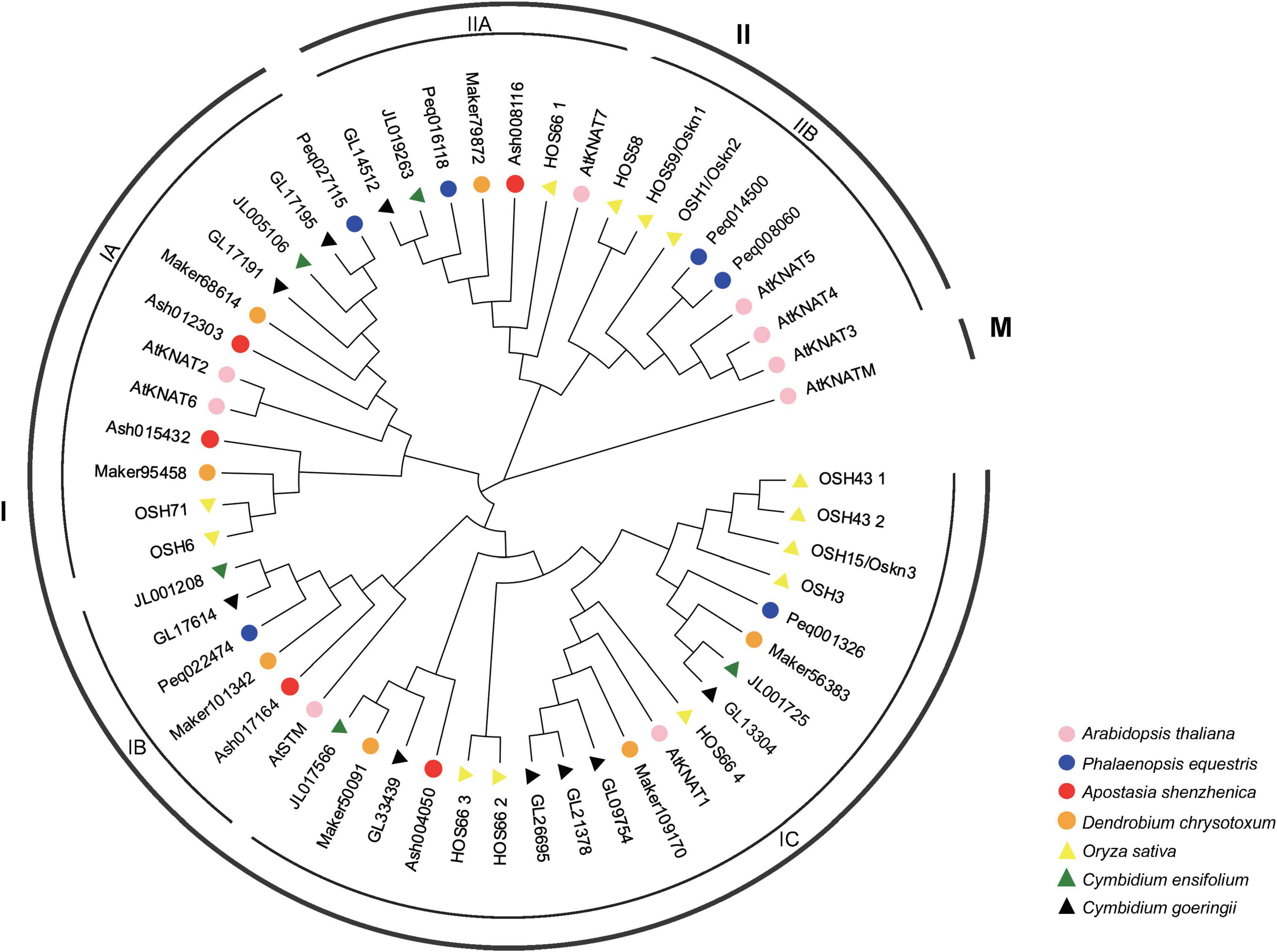
Figure 1. Phylogenetic tree of KNOX genes based on the KNOX protein sequences of seven plant species. The KNOX gene family was classified into three classes: Class I, Class II, and Class M with Class I divided into three subclades: IA, IB, and IC, and two subclades, IIA and IIB in Class II. KNOX protein sequences of all species are available in Supplementary Data Sheet.
Collinearity and Evolutionary Analysis
The collinear relationship among the KNOX genes of D. chrysotoxum, C. goeringii, and C. ensifolium was examined to find the potential events during KNOXs evolution in orchids. The collinear analysis demonstrated a one-to-one correspondence among all KNOX genes in three orchids, suggesting less reshuffling of KNOX orthologous and substantial genomic rearrangements after the lineages of Dendrobium and Cymbidium diverged (Figure 2). In addition, we examined the gene location on the chromosome for C. goeringii which contained the highest number of KNOX genes to look for the potential duplication event. The result showed that a small-scale tandem duplication might lead to the increasing members of KNOXs in C. goeringii (Supplementary Figure 1).
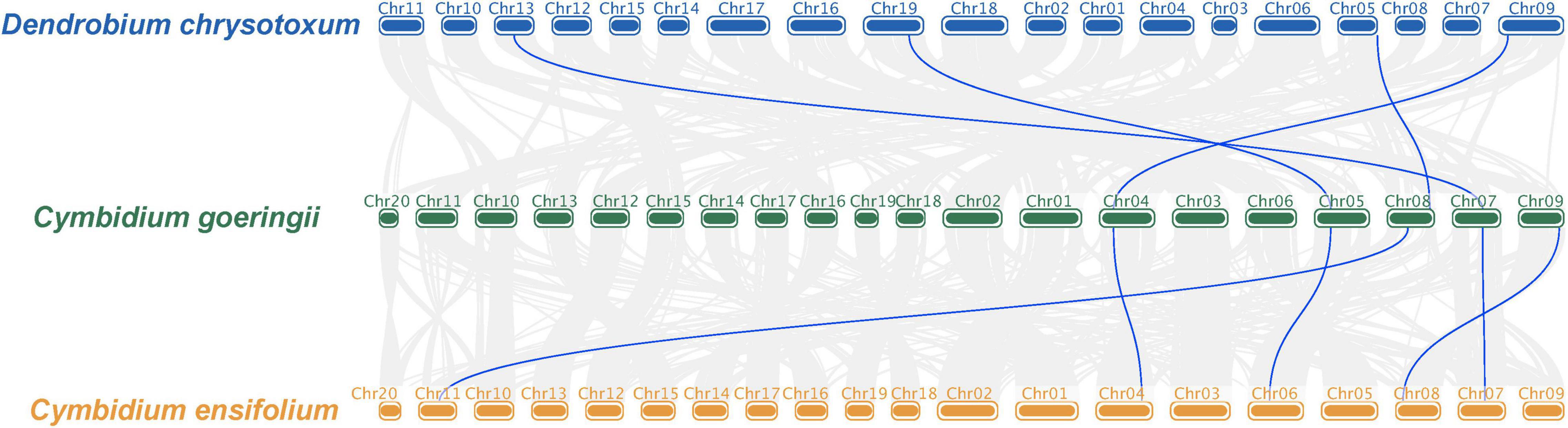
Figure 2. The collinearity of KNOX genes between D. chrysotoxum and C. goeringii, C. goeringii and C. ensifolium. The collinear analysis demonstrated a one-to-one correspondence among all KNOX genes in three orchids.
For selection pressure analysis, 38 gene pairs were selected based on sequence similarity for calculating the ratio of the number of non-synonymous substitutions per non-synonymous site (Ka) to the number of synonymous substitutions per synonymous site (Ks). The results showed that the Ka/Ks ratios of all KNOX genes were less than one, of which most values were below 0.4, indicating that all orchid KNOXs experienced strong purifying selection (Supplementary Table 2; Zhang et al., 2006).
Motif and Gene Structure Analysis
Motifs of KNOX proteins in A. thaliana, rice, and five orchids were examined using the online analysis tool MEME, and 20 motifs were set as upper bound (Figure 3A). The number of KNOX motifs ranged from three (AtKNATM) to 14 (GL13304). Motif 4, motif 2, motif 5, and motif 1 encoded the KNOX1, KNOX2, ELK, and Homeobox domains, respectively. Although most orchid KNOX proteins contained these four conserved protein domains (Figures 3B,D), motif structure differs in each subclade. For instance, motifs 9, 14, and 17 were only present in Class II. Motif 19 was specifically distributed in C. goeringii and D. chrysotoxum and motif 20 was only present in D. chrysotoxum, C. goeringii, and C. ensifolium.
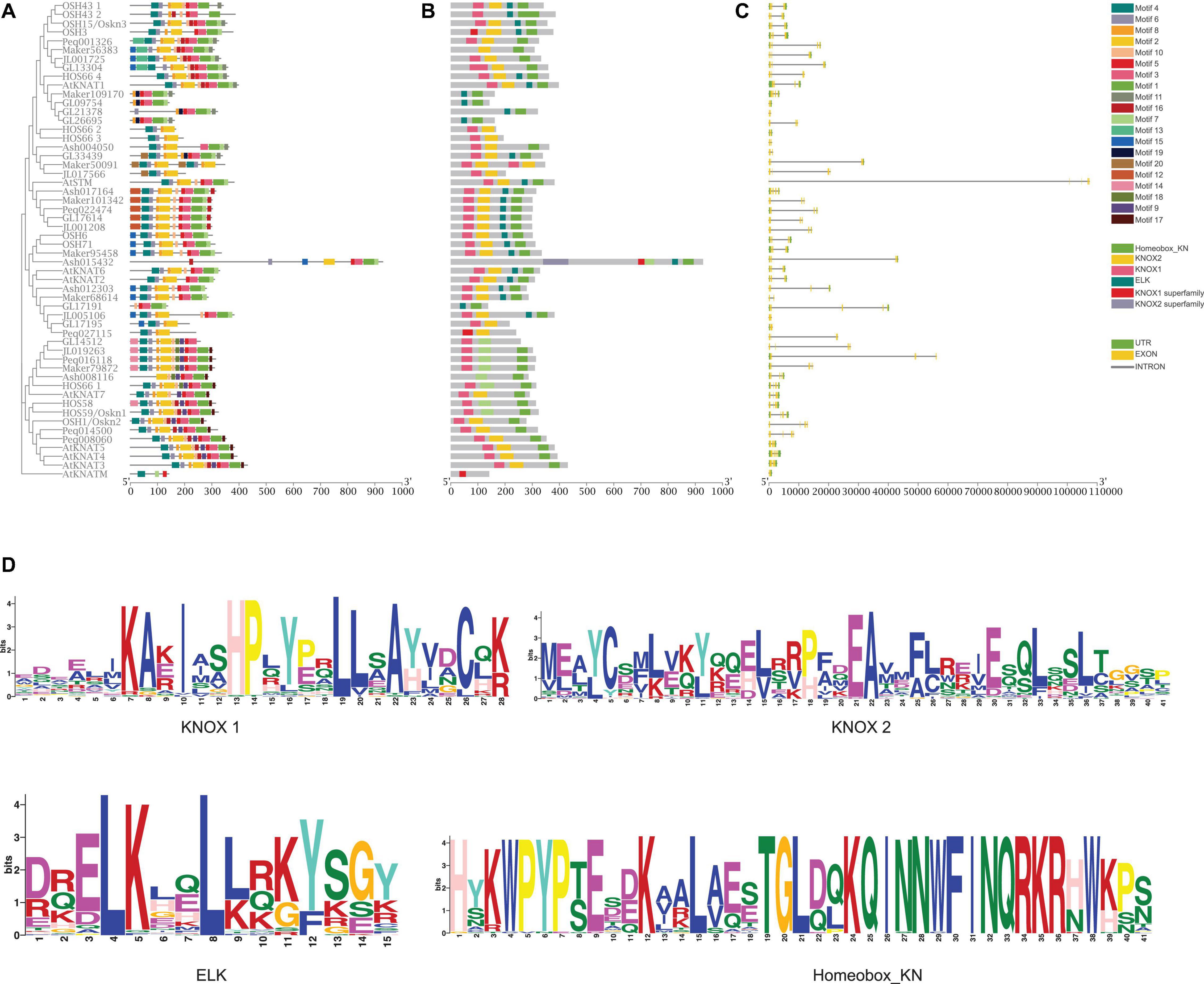
Figure 3. Gene structure, conserved motifs, conserved domains of KNOX genes. (A) Predicted motifs with the phylogenetic tree of orchid KNOXs. (B) Conserved KNOX domains. (C) Gene structure of KNOXs, with green blocks, yellow blocks, and gray lines representing upstream or downstream-untranslated regions (UTR), exons, and introns, respectively. (D) Sequence logo of Motif 4, motif 2, motif 5, and motif 1, which encoded the KNOX1, KNOX2, ELK, and Homeobox domains, respectively.
To further explore the characteristics of KNOX genes in orchids, intron–exon structure was analyzed (Figure 3C). The results showed that the orchid KNOX family is composed of 1–9 exons and 1–4 introns. Although the similarity in gene structure was found in each subclade, orchid KNOX genes have a high degree of variance in intron length and exon numbers in comparison with A. thaliana and rice. In general, most Class I genes exhibited longer intron length than Class II genes. Notably, in Class IB, orchid KNOX genes displayed a significant shortening intron length than AtSTM, whereas in other clades, most orchid KNOXs have longer intron than A. thaliana and rice (Figure 3C), which might be a unique feature of Orchidaceae.
Protein Tertiary Structure Prediction
The tertiary structures of most KNOXs in orchids were highly conserved, characterized by three helices, among which helices I and II were connected by a loop structure, helices II and helices III formed a helix–turn–helix motif (Figure 4). With the exception, GL14512 (Class IIA) showed a merged helix without loop or turn, and the other two helices GL17195 (Class IA) and JL017655 (Class IC) had four helices, and Maker50091 (Class IC) exhibited very short three helices. The secondary structure prediction indicated that all orchid KNOX proteins composed of α-helix (Hh), random coils (Cc), extended strands (Ed), and β-turns (Tt), the mean of which account for 48.00%, 7.02%, 4.98%, and 40.00% of the protein structure, respectively (Supplementary Table 3).
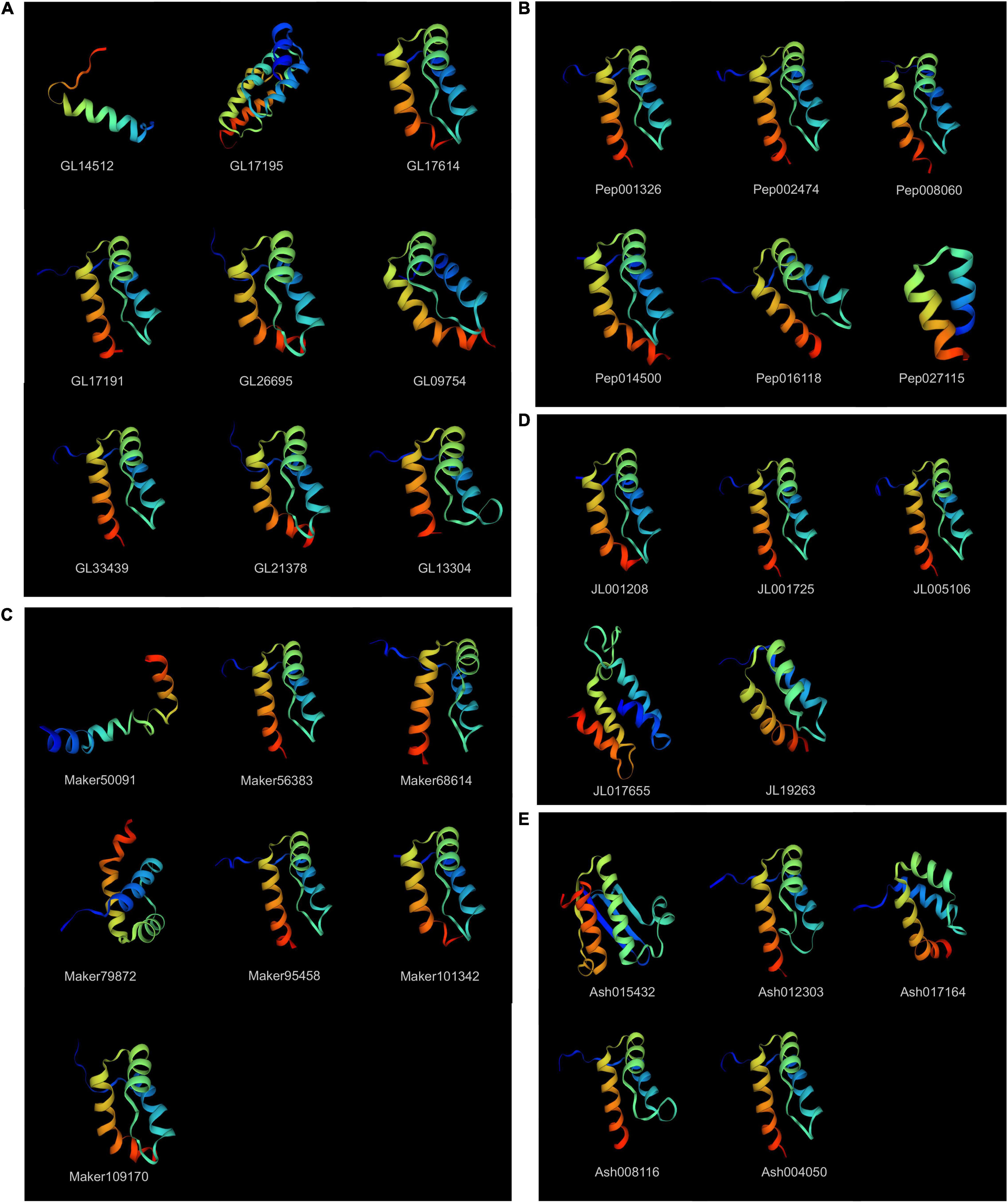
Figure 4. Protein tertiary structure of KNOX genes. (A) C. goeringii. (B) P. equestris. (C) D. chrysotoxum. (D) C. ensifolium. (E) A. shenzhenica. The tertiary structures were colored by rainbow order, representing N to C terminus.
Cis-Acting Regulatory Elements Analysis
To investigate the regulatory functions of KNOXs, the 2,000 bp promoter regions of orchid KNOX genes were retrieved for the identification of putative cis-elements. A total of 797 cis-acting elements attributing to 25 types and 15 responsive functions were identified (Figure 5 and Supplementary Table 4). Among these elements, TATA-box made up the most common elements (62.62%), followed by CAAT-box (15.04%) (Supplementary Table 5). Cis-element functions included phytohormone responsiveness for gibberellin, auxin, methyl jasmonate (MeJA), salicylic acid, and abscisic acid (ABA); stress responsiveness such as drought, anoxic, anaerobic, low-temperature; and growth and development elements as light response and circadian control (Figure 5). Each KNOX gene contained multiple types of elements with light responsiveness as the most occurring element function, supporting the key roles of light that mediate KNOX function during plant development (Figure 5). MeJA-responsive element, followed by ABA-responsiveness, constituting the second and third most abundant type (Supplementary Table 4), respectively, were also widely distributed in most orchid KNOXs, implying they may exert function in modulating these two phytohormones. In particular, we also found 14 elements that are related to meristem expression and meristem-specific activation, which is consistent with the instrumental roles of KNOX in the maintenance of meristem homeostasis.
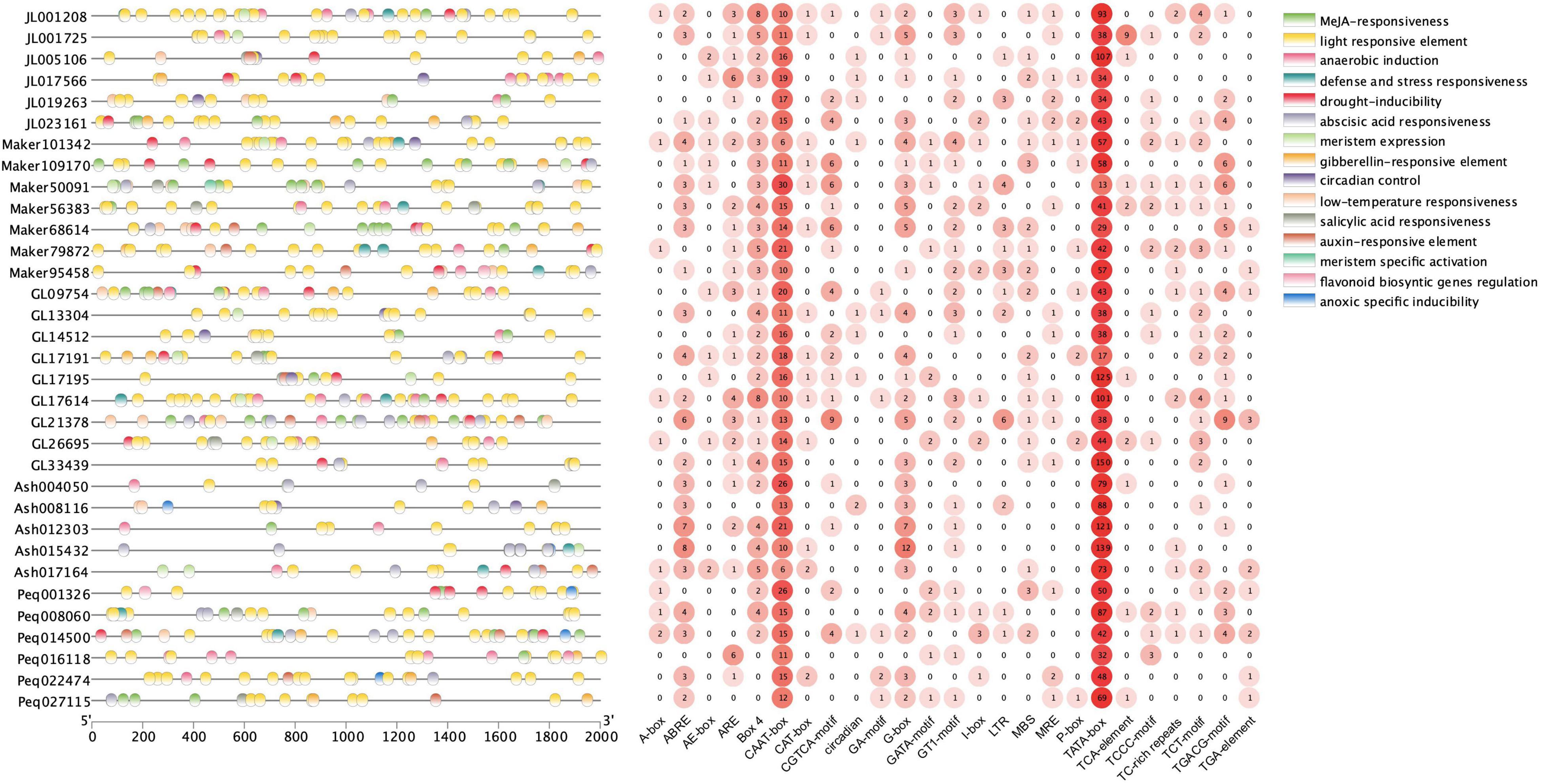
Figure 5. Cis-acting elements in the promoter regions of KNOX genes. Elements with similar regulatory functions are displayed in the same color. Numbers of each type of element are shown on the right side.
Expression Analysis
Expression analysis was conducted based on five orchids’ transcriptome data of various tissues including different flower segments, leaves, pseudobulbs (stem), root, and seed. The expression profile showed that Class II KNOX genes were expressed broadly in differentiating tissues and mature organs, while the expression of Class I genes was more confined to less differentiated tissues with SAM-like pseudobulbs (stem) (Figure 6). For instance, in C. goeringii, Class I genes such as GL21378, GL33439, GL17614, and GL17195 exhibited an exclusive expression in the stem (Figure 6A). In addition, Ash017164, JL001208, and Peq022474, which were homologs to STM have high expression levels in stem, inflorescence, pedicels, and seeds that bear numerous meristematic regions, indicating the Class I KNOX genes’ function in meristem maintenance.
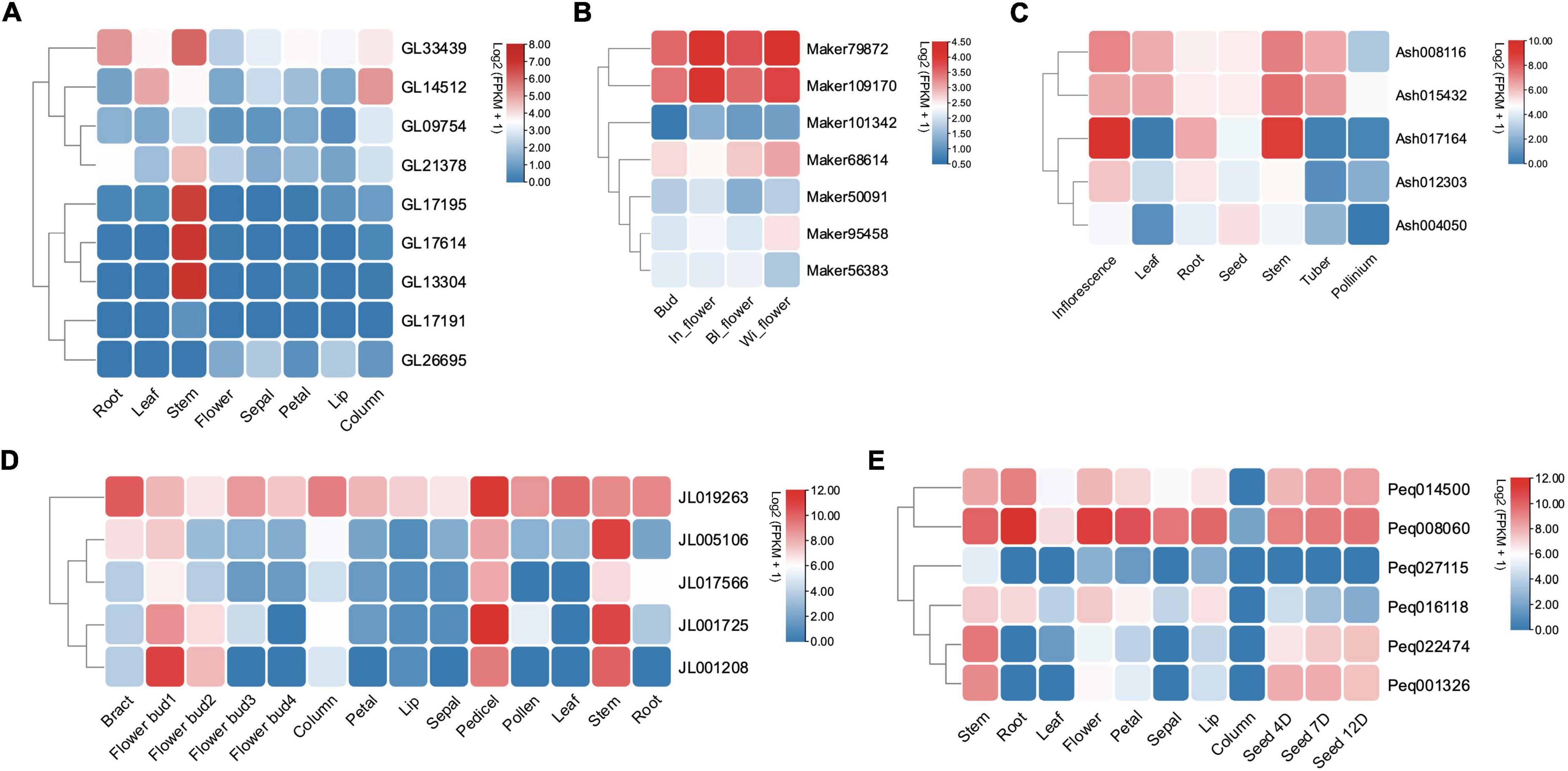
Figure 6. The expression profile of KNOX genes among different tissues in five orchids. (A) C. goeringii. (B) D. chrysotoxum. In_flower, initial opened flower; BI_flower, fully opened flower; Wi_flower, withered flower. (C) A. shenzhenica. (D) C. ensifolium. Flower bud1, flower bud at an early stage; Flower bud2, flower bud at the middle stage; Flower bud3, flower bud at a late stage. (E) P. equestris. Seed 4D, 4 day’s seed; Seed 7D, 7 day’s seed; Seed 12D, 12 day’s seed.
Whereas Class II genes such as JL019263 and Peq008060 were highly expressed in almost all vegetative and reproductive tissues (Figures 6B–E), demonstrating a widespread expression and their possible role in promoting tissue differentiation. STM has an early origin compared to other members of Class I (Furumizu et al., 2015; Frangedakis et al., 2017), and has been shown to positively regulate KNAT1 and KNAT2, with KNAT6 performing redundant function with STM in SAM maintenance (Scofield et al., 2014; Bueno et al., 2020). To further investigate the specific roles of Class I KNOX genes, gene expression of STM homologs in C. goeringii, P. equestris, C. ensifolium, and D. chrysotoxum was analyzed in their leaves, flowers, and stems by RT-qPCR (Figure 7 and Supplementary Table 1). In all examined orchids, the STM homologs showed extremely high expression in stems but were barely detected in flowers and leaves, further verifying that Class I genes have a tissue-specific expression in the stem. As an early diverging Class I KNOX member, the high expression of STM could also explain the concomitant elevated expression of other Class I members in the stem (Figure 6). A critical next step will be the functional analysis of these two classes to unravel the underlying roles of KNOXs in orchids.
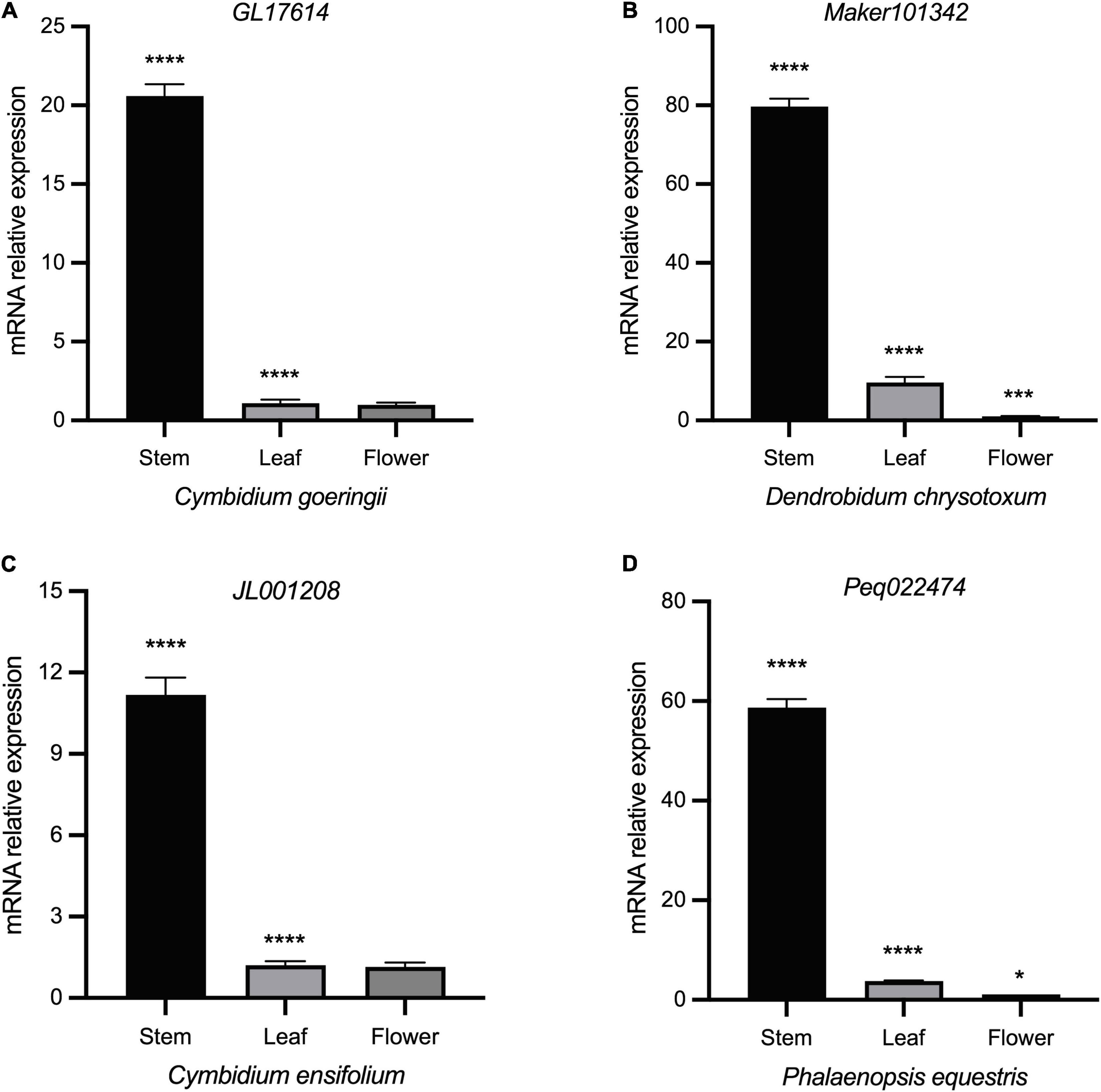
Figure 7. Expression profiles of different tissues of STM homologs in four orchids by RT-qPCR. (A) C. goeringii. (B) D. chrysotoxum. (C) C. ensifolium. (D) P. equestris. ANOVA multiple comparisons were performed with star marks *, ***, and **** representing adjusted p < 0.05, p < 0.001, and p < 0.0001, respectively (Supplementary Table 6).
Gene Ontology Analysis of Orchid KNOXs
Gene ontology analysis was performed to delineate gene functional classifications of orchid KNOXs and investigate the important biological processes they might be involved in. As a result, GO terms “regulation of transcription,” “nucleus,” and “DNA binding” constitute the greatest number of genes for GO ontologies “Biological Process,” “Cellular Component,” and “Molecular Function,” respectively (Figure 8 and Supplementary Table 7). The results match the fact that KNOX is a premier regulator that is associated with numerous downstream transcriptional networks and functions mostly in the nucleus (Table 1). Several other terms such as “regulation of secondary cell wall biogenesis” and “hormone activity” are also consistent with the KNOX’s function reported in previous studies (Tabata et al., 2010; Wang et al., 2020).
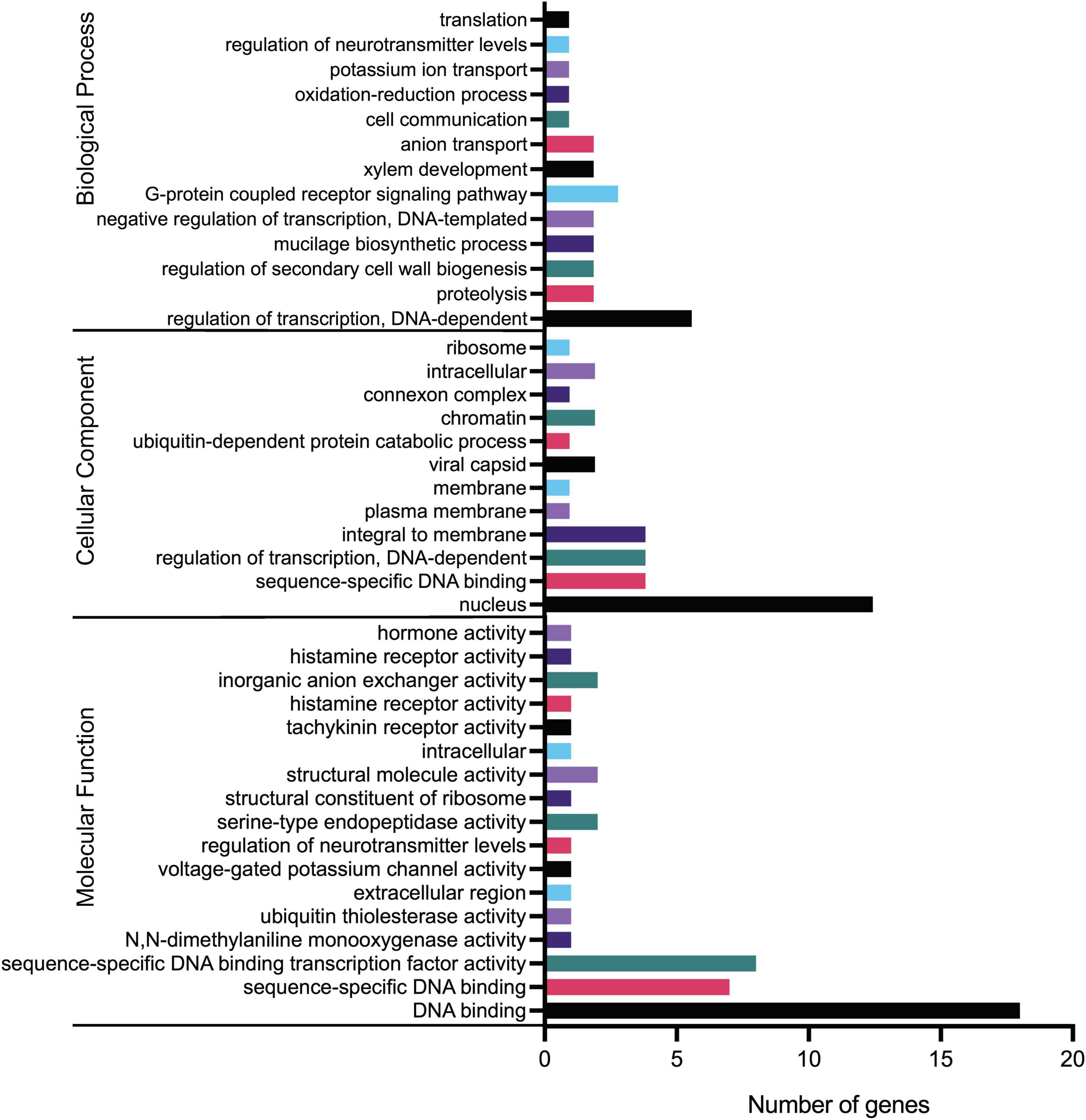
Figure 8. Gene ontology (GO) terms of orchid KNOX genes. Annotation details for each gene are listed in Supplementary Table 7.
Discussion
The primary architecture of plants derives from the SAM, producing leaves, internodes, and axillary buds. As the key regulator functioning in the maintenance of meristematic potentials, KNOX gene family is closely linked to lateral organ morphogenesis. In this study, we identified 32 KNOX genes in five orchids and classified them into two classes: Class I and Class II based on phylogenetic relationship, with no Class M member has been identified, owning to this clade is exclusive to some eudicot species (Jie et al., 2015; Bueno et al., 2020). Class IC, represented by Arabidopsis genes KNAT1, has the greatest number of orchid KNOX homologs, in which D. chrysotoxum, C. ensifolium, and C. goeringii contained more than one member (Figure 1). Evolutionary novelty is thought to be driven by gene duplication, which is rife in angiosperms (Van de Peer et al., 2017). A study based on 48 species revealed that the number of KNOX genes among angiosperms spanning from four to 28, while the duplicated homologs to KNAT1 clade were only observed in Gmax glyma (dicots) and O. sativa (monocots) (Jie et al., 2015). Orchids exhibited a small number of KNOX genes (5–9), the genus-specific duplication in Class IC, therefore, may underlie the possible neo-functionalization of their roles in contributing to important innovations of plant architecture in Orchidaceae.
Variation in the gene structure of a gene family among different species has been largely uncovered thanks to the development of whole-genome sequencing. Given the conserved nature of gene structure within the same clade, we found that orchid KNOXs have a similar exon–intron structure compared to A. thaliana and O. sativa, except that most orchids exhibited longer intron length (Figure 3C). Long intron has been reported in almost all sequenced orchid species (Cai et al., 2014; Zhang et al., 2016, 2017; Yuan et al., 2018; Ai et al., 2021), which might be a unique feature of Orchidaceae. Longer introns are favored in the course of gene evolution because they promote the efficiency of natural selection by increasing the recombination between two adjacent exons (Jo and Choi, 2015), which may account for the marvelous richness of orchids.
Gene expression at the promoter region is primarily regulated by the cis-acting elements situated upstream of the transcriptional start site (Hernandez-Garcia and Finer, 2014). In this study, we identified diverse function types of regulatory elements in orchid KNOXs’ promoter regions, which were further categorized as phytohormone responsive, stress-responsive, and growth and development elements (Figure 5). Our results showed that a large proportion of cis-elements are acting in light responsiveness, indicative of environmental cues such as light could exert an impact on KNOX’s function. In addition, many elements are responsive to JA, GA, ABA, and auxin. Indeed, KNOXs have important functions in modulating these phytohormones. It has been reported that auxin response factor genes positively regulate JA biosynthesis in floral organs via the suppression of Class 1 KNOX genes (Tabata et al., 2010). In leaf, KNOX genes are repressed to maintain a low cytokinin (CK) to high gibberellin (GA) ratio, thus switching SAM from an indeterminate state to leaf-specific growth (Ichihashi and Tsukaya, 2015). Meanwhile, Class II KNOX genes play a crucial role in regulating ABA signaling during organ development (Jia et al., 2021b). The diverse functions of cis-elements reflect the multiple roles of KNOX genes involved in plant growth and development.
Intra-genomic comparisons between D. chrysotoxum, C. goeringii, and C. ensifolium’s chromosomes showed that their KNOX genes were in a one-to-one correspondence, supporting no duplication events have occurred in orchid KNOXs (Figure 2). Moreover, C. goeringii and C. ensifolium have a nearly one-to-one syntenic relationship between their chromosomes, demonstrating there were no obvious inter-chromosomal structure variations after the two species diverged. The Ka/Ks value indicated that all orchid KNOXs undergo strong purifying selection (Supplementary Table 2), which is critical to eliminating newly arising deleterious mutations thus maintaining biological function (Cvijović et al., 2018), indicating the conservation of this gene family has been reinforced across the Orchidaceae lineage.
Neo-functionalization of transcription factors can be achieved through changes in expression pattern or function alteration (Furumizu et al., 2015). In angiosperms, Class I and II KNOX genes play contrary roles in plant growth and development, with Class I and Class II members functioning in meristems maintenance and differentiation of all aerial organs, respectively. It is clear from our study that Class II KNOX genes have a widespread expression in differentiated tissues, while the expression of Class I genes was more restricted to the stem that contains many meristematic regions (Figures 6, 7). It is suggested that the diversified expression pattern of Class II genes may be due to the neofunctionalization during the gene duplication event of an ancestral KNOX gene (Furumizu et al., 2015). The opposing activities between Class I and Class II KNOX genes may underlie the molecular mechanism of key innovations and modification of plant architecture via elaboration of transcriptional networks. Characterization of the loss/gain-of-function mutants and physiological experiments on overexpressed phenotypes may illuminate more undetected roles of Class II KNOX genes.
Conclusion
Members of the KNOX gene family are versatile effectors regulating SAM maintenance and hormonal signaling thus influencing many facets of plant growth and development. A total of 32 orchid KNOX genes were identified and assigned to two subclasses by phylogenetic analysis with more members found in Class I. Motif, gene structure, and protein tertiary prediction suggested that orchid KNOXs were conserved among different species and a small scale tandem duplication gave rise to more members in C. goeringii. We showed that the specific functions of cis-element in meristem expression and activation are acting in concert with the pivotal role of KNOXs in SAM. In addition, the expression patterns generated by transcriptomic and RT-qPCR data supported a tissue-specific expression of Class I genes in the stem where a pool of pluripotent stem cells was generated. Our study presents a comprehensive analysis for uncovering the function and expression pattern of KNOX genes in Orchidaceae. These results build a foundation for further understanding of how KNOX genes have been co-opted in regulating various forms of lateral organs and shed light on the plasticity of plant architecture. A critical next step will be the functional analysis of KNOX in non-model plants to characterize the additional role of KNOX in the context of land plant evolution.
Data Availability Statement
The original contributions presented in the study are included in the article/Supplementary Material, further inquiries can be directed to the corresponding authors.
Author Contributions
W-LY and Z-JL conceived and designed the research. DZ and SL performed the data analysis and wrote the manuscript. All authors contributed to the article and approved the submitted version.
Funding
This work was supported by the National Key Research and Development Program of China (2019YFD1000400).
Conflict of Interest
The authors declare that the research was conducted in the absence of any commercial or financial relationships that could be construed as a potential conflict of interest.
Publisher’s Note
All claims expressed in this article are solely those of the authors and do not necessarily represent those of their affiliated organizations, or those of the publisher, the editors and the reviewers. Any product that may be evaluated in this article, or claim that may be made by its manufacturer, is not guaranteed or endorsed by the publisher.
Supplementary Material
The Supplementary Material for this article can be found online at: https://www.frontiersin.org/articles/10.3389/fpls.2022.901089/full#supplementary-material
Footnotes
- ^ https://bigd.big.ac.cn/
- ^ http://www.arabidopsis.org
- ^ https://phytozome-next.jgi.doe.gov/
- ^ http://pfam.xfam.org/
- ^ https://www.ncbi.nlm.nih.gov/Structure/bwrpsb/bwrpsb.cgi
- ^ http://gsds.gao-lab.org/
- ^ http://meme-suite.org/tools/meme
- ^ https://swissmodel.expasy.org/interactive
- ^ https://npsa-prabi.ibcp.fr/
- ^ http://bioinformatics.psb.ugent.be/webtools/plantcare/html/
- ^ http://www.primer3plus.com/cgi-bin/dev/primer3plus.cgi
- ^ http://eggnog-mapper.embl.de/
References
Ai, Y., Sun, W. H., Chen, J., Zhang, D., Ma, L., Zhang, Q. H., et al. (2021). The Cymbidium genome reveals the evolution of unique morphological traits. Hort. Res. 8:255. doi: 10.1038/s41438-021-00683-z
Artimo, P., Jonnalagedda, M., Arnold, K., Baratin, D., Csardi, G., De Castro, E., et al. (2012). ExPASy: SIB bioinformatics resource portal. Nucleic Acids Res. 40, 597–603. doi: 10.1093/nar/gks400
Bailey, T. L., Boden, M., Buske, F. A., Frith, M., Grant, C. E., Clementi, L., et al. (2009). MEME suite: tools for motif discovery and searching. Nucleic Acids Res. 37, W202–W208. doi: 10.1093/nar/gkp335
Bueno, N., Alvarez, J. M., and Ordás, R. J. (2020). Characterization of the knotted1-like homeobox (KNOX) gene family in Pinus pinaster Ait. Plant Sci. 301:110691. doi: 10.1016/j.plantsci.2020.110691
Cai, J., Vanneste, K., Proost, S., Tsai, W. C., Liu, K. W., and Chen, L. J. (2014). The genome sequence of the orchid Phalaenopsis equestris. Nat. Genet. 47, 65–72. doi: 10.1038/ng.3149
Chase, M. W., Cameron, K. M., Freudenstein, J. V., Pridgeon, A. M., Salazar, G., Berg, C. V. D., et al. (2015). An updated classification of Orchidaceae. Bot. J. Linn. Soc. 177, 151–174. doi: 10.1111/boj.12234
Chen, C. J., Chen, H., Zhang, Y., Thomas, H., Frank, M. H., He, Y. H., et al. (2020). TBtools: an integrative toolkit developed for interactive analyses of big biological data. Mol. Plant 13, 1194–1202. doi: 10.1016/j.molp.2020.06.009
Chen, H., Yu, H., Jiang, W., Li, H., Wu, T., Chu, J., et al. (2021). Overexpression of ovate family protein 22 confers multiple morphological changes and represses gibberellin and brassinosteroid signalings in transgenic rice. Plant Sci. 304:110734. doi: 10.1016/j.plantsci.2020.110734
Chou, K. C., and Shen, H. B. (2010). Plant-mPLoc: a top-down strategy to augment the power for predicting plant protein subcellular localization. PLoS One 5:e11335. doi: 10.1371/journal.pone.0011335
Cvijović, I., Good, B. H., and Desai, M. M. (2018). The effect of strong purifying selection on genetic diversity. Genetics 209, 1235–1278. doi: 10.1534/genetics.118.301058
Finn, R. D., Mistry, J., Tate, J., Coggill, P., Heger, A., Joanne, E., et al. (2010). The Pfam protein families database. Nucleic Acids Res. 38, D211–D222. doi: 10.1093/nar/gkp985
Frangedakis, E., Saint-Marcoux, D., Moody, L. A., Rabbinowitsch, E., and Langdale, J. A. (2017). Nonreciprocal complementation of KNOX gene function in land plants. New Phytol. 216, 591–604. doi: 10.1111/nph.14318
Furumizu, C., Alvarez, J. P., Sakakibara, K., Bowman, J. L., and Qu, L. J. (2015). Antagonistic roles for KNOX1 and KNOX2 genes in patterning the land plant body plan following an ancient gene duplication. PLoS Genetics 11:e1004980. doi: 10.1371/journal.pgen.1004980
Gasteiger, E., Hoogland, C., Gattiker, A., Duvaud, S., Wilkins, M., Appel, R., et al. (2005). “Protein identification and analysis tools on the ExPASy server,” in The Proteomics Protocols Handbook, ed. J. M. Walker (Totowa, NJ: Humana Press). doi: 10.1385/1-59259-584-7:531
Geourjon, C., and Deléage, G. (1995). SOPMA: significant improvements in protein secondary structure prediction by consensus prediction from multiple alignments. Comput. Appl. Biosci. 11, 681–684. doi: 10.1093/bioinformatics/11.6.681
Hake, S., Smith, H., Holtan, H., Magnani, E., and Ramirez, J. (2004). The role of knox genes in plant development. Rev. Cell Dev. Biol. 20, 125–151. doi: 10.1146/annurev.cellbio.20.031803.093824
Hay, A., and Tsiantis, M. (2009). A KNOX family TALE. Curr. Opin. Plant Biol. 12, 593–598. doi: 10.1016/j.pbi.2009.06.006
Hay, A., and Tsiantis, M. (2010). KNOX genes: versatile regulators of plant development and diversity. Development 137, 3153–3165. doi: 10.1242/dev.030049
He, Z., Zhang, H., Gao, S., Lercher, M. J., Chen, W. H., and Hu, S. (2016). Evolview v2: an online visualization and management tool for customized and annotated phylogenetic trees. Nucleic Acids Res. 44, W236–W241. doi: 10.1093/nar/gkw370
Hernandez-Garcia, C. M., and Finer, J. J. (2014). Identification and validation of promoters and cis-acting regulatory elements. Plant Sci. 217, 109–119. doi: 10.1016/j.plantsci.2013.12.007
Hu, B., Jin, J., Guo, A. Y., Zhang, H., Luo, J., and Gao, G. (2015). GSDS 2.0: an upgraded gene feature visualization server. Bioinformatics 31, 1296–1297. doi: 10.1093/bioinformatics/btu817
Huerta-Cepas, J., Szklarczyk, D., Heller, D., Hernández-Plaza, A., Forslund, S. K., Cook, H., et al. (2019). eggNOG 5.0: a hierarchical, functionally and phylogenetically annotated orthology resource based on 5090 organisms and 2502 viruses. Nucleic Acids Res. 47, D309–D314. doi: 10.1093/nar/gky1085
Ichihashi, Y., and Tsukaya, H. (2015). Behavior of leaf meristems and their modification. Front. Plant Sci. 6:1060. doi: 10.3389/fpls.2015.01060
Jia, P., Xing, L., Zhang, C., Chen, H., Li, Y., Zhang, D., et al. (2021a). MdKNOX15, a class I knotted-like transcription factor of apple, controls flowering and plant height by regulating GA levels through promoting the MdGA2ox7 transcription. Environ. Exp. Bot. 185:104411. doi: 10.1016/j.envexpbot.2021.104411
Jia, P., Xing, L., Zhang, C., Zhang, D., Ma, J., Zhao, C., et al. (2021b). MdKNOX19, a class II knotted-like transcription factor of apple, plays roles in ABA signalling/sensitivity by targeting ABI5 during organ development. Plant Sci. 302:110701. doi: 10.1016/j.plantsci.2020.110701
Jie, G., Xue, Y., Wei, Z., Tiange, L., and Tore, S. (2015). Evolution, diversification, and expression of knox proteins in plants. Front. Plant Sci. 6:882. doi: 10.3389/fpls.2015.00882
Jo, B. S., and Choi, S. S. (2015). Introns: the functional benefits of introns in genomes. Genomics Informatics 13, 112–118. doi: 10.5808/GI.2015.13.4.112
Lee, J. H., Lin, H., Joo, S., and Goodenough, U. (2008). Early sexual origins of homeoprotein heterodimerization and evolution of the plant knox/bell family. Cell 133, 829–840. doi: 10.1016/j.cell.2008.04.028
Lescot, M., Déhais, P., Thijs, G., Marchal, K., Moreau, Y., Van de Peer, Y., et al. (2002). PlantCARE, a database of plant cis-acting regulatory elements and a portal to tools for in silico analysis of promoter sequences. Nucleic Acids Res. 30, 325–327. doi: 10.1093/nar/30.1.325
Li, B., and Dewey, C. N. (2011). RSEM: accurate transcript quantification from RNA-Seq data with or without a reference genome. BMC Bioinformatics 12:323. doi: 10.1186/1471-2105-12-323
Magnani, E., and Hake, S. (2008). KNOX lost the OX: the Arabidopsis KNATM gene defines a novel class of KNOX transcriptional regulators missing the homeodomain. Plant Cell 20, 875–887. doi: 10.1105/tpc.108.058495
Meng, L., Liu, X., He, C., Xu, B., Li, Y., and Hu, Y. (2020). Functional divergence and adaptive selection of KNOX gene family in plants. Open Life Sci. 15, 346–363. doi: 10.1515/biol-2020-0036
Miller, M. A., Schwartz, T., Pickett, B. E., He, S., Klem, E. B., Scheuermann, R. H., et al. (2015). A RESTful API for access to phylogenetic tools via the CIPRES science gateway. Evol. Bioinform. 11, 43–48. doi: 10.4137/EBO.S21501
Qin, W., Yin, Q., Chen, J., Zhao, X., Yue, F., He, J., et al. (2020). The class II KNOX transcription factors KNAT3 and KNAT7 synergistically regulate monolignol biosynthesis in Arabidopsis. J. Exp. Bot. 71, 5469–5483. doi: 10.1093/jxb/eraa266
Reiser, L., Sánchez-Baracaldo, P., and Hake, S. (2000). Knots in the family tree: evolutionary relationships and functions of knox homeobox genes. Plant Mol. Biol. 42, 151–166. doi: 10.1023/A:1006384122567
Rozewicki, J., Li, S., Amada, K. M., Standley, D. M., and Katoh, K. (2019). MAFFT-DASH: integrated protein sequence and structural alignment. Nucleic Acids Res. 47, W5–W10. doi: 10.1093/nar/gkz342
Schwede, T., Kopp, J., Guex, N., and Peitsch, M. C. (2003). SWISS-MODEL: an automated protein homology-modeling server. Nucleic Acids Res. 31, 3381–3385. doi: 10.1093/nar/gkg520
Scofield, S., Dewitte, W., and Murray, J. (2007). The KNOX gene SHOOT MERISTEMLESS is required for the development of reproductive meristematic tissues in Arabidopsis. Plant J. 50, 767–781. doi: 10.4161/psb.3.4.5194
Scofield, S., Dewitte, W., and Murray, J. A. H. (2014). STM sustains stem cell function in the Arabidopsis shoot apical meristem and controls KNOX gene expression independently of the transcriptional repressor AS1. Plant Signal. Behav. 9:e28934. doi: 10.4161/psb.28934
Sluis, A., and Hake, S. (2015). Organogenesis in plants: initiation and elaboration of leaves. Trends Genet. 31, 300–306. doi: 10.1016/j.tig.2015.04.004
Tabata, R., Ikezaki, M., Fujibe, T., Aida, M., Tian, C. E., Ueno, Y., et al. (2010). Arabidopsis auxin response factor6 and 8 regulate jasmonic acid biosynthesis and floral organ development via repression of class 1 KNOX genes. Plant Cell Physiol. 51, 164–175. doi: 10.1093/pcp/pcp176
Van de Peer, Y., Mizrachi, E., and Marchal, K. (2017). The evolutionary significance of polyploidy. Nat. Rev. Genet. 18, 411–424. doi: 10.1038/nrg.2017.26
Venglat, S. P., Dumonceaux, T., Rozwadowski, K., Parnell, L., and Datla, R. (2002). The homeobox gene BREVIPEDICELLUS is a key regulator of inflorescence architecture in Arabidopsis. Proc. Natl. Acad. Sci. U S A. 99, 4730–4735. doi: 10.1073/pnas.072626099
Wang, S., Yamaguchi, M., Grienenberger, E., Martone, P. T., Samuels, A. L., and Mansfield, S. D. (2020). The Class II KNOX genes KNAT3 and KNAT7 work cooperatively to influence deposition of secondary cell walls that provide mechanical support to Arabidopsis stems. Plant J. 101, 293–309. doi: 10.1111/tpj.14541
Wang, Y. P., Tang, H. B., Debarry, J. D., Tan, X., Li, J. P., Wang, X. Y., et al. (2012). MCScanX: a toolkit for detection and evolutionary analysis of gene synteny and collinearity. Nucleic Acids Res. 40:e49. doi: 10.1093/nar/gkr1293
Xiong, H. Z., Shi, A. N., Wu, D. X., Weng, Y. J., Qin, J., Ravelombola, W. S., et al. (2018). Genome-Wide identification, classification and evolutionary expansion of KNOX gene family in rice (Oryza sativa) and populus (Populus trichocarpa). Am. J. Plant Sci. 9, 1071–1092. doi: 10.4236/ajps.2018.96082
Yuan, Y., Jin, X., Liu, J., Zhao, X., Zhou, J., Wang, X., et al. (2018). The Gastrodia elata genome provides insights into plant adaptation to heterotrophy. Nat. Commun. 9:1615. doi: 10.1038/s41467-018-03423-5
Zhang, G. Q., Liu, K. W., Li, Z., Lohaus, R., Hsiao, Y. Y., Niu, S. C., et al. (2017). The Apostasia genome and the evolution of orchids. Nature 549, 379–383. doi: 10.1038/nature23897
Zhang, G. Q., Xu, Q., Tsai, W. C., Yeh, C. M., Liu, K. W., Yoshida, K., et al. (2016). The Dendrobium catenatum Lindl. genome sequence provides insights into polysaccharide synthase, floral development and adaptive evolution. Sci. Rep. 6:19029. doi: 10.1038/srep19029
Zhang, Z., Li, J., Zhao, X. Q., Wang, J., Wong, G. K. S., and Yu, J. (2006). KaKs_calculator: calculating Ka and Ks through model selection and model averaging. Genomics Proteom. Bioinform. 4, 259–263. doi: 10.1016/s1672-0229(07)60007-2
Keywords: Orchidaceae, KNOX gene family, stem apical meristem, organ shape, expression pattern
Citation: Zhang D, Lan S, Yin W-L and Liu Z-J (2022) Genome-Wide Identification and Expression Pattern Analysis of KNOX Gene Family in Orchidaceae. Front. Plant Sci. 13:901089. doi: 10.3389/fpls.2022.901089
Received: 21 March 2022; Accepted: 19 April 2022;
Published: 27 May 2022.
Edited by:
Ran Xu, Hainan University, ChinaReviewed by:
Jing Xu, Xi’an University, ChinaKai Zhao, Fujian Normal University, China
Xiaokang Zhuo, University of Florida, United States
Copyright © 2022 Zhang, Lan, Yin and Liu. This is an open-access article distributed under the terms of the Creative Commons Attribution License (CC BY). The use, distribution or reproduction in other forums is permitted, provided the original author(s) and the copyright owner(s) are credited and that the original publication in this journal is cited, in accordance with accepted academic practice. No use, distribution or reproduction is permitted which does not comply with these terms.
*Correspondence: Wei-Lun Yin, eWlud2xAYmpmdS5lZHUuY24=; Zhong-Jian Liu, empsaXVAZmFmdS5lZHUuY24=