- 1Department of Plant and Soil Sciences, North Mississippi Research and Extension Center, Mississippi State University, Starkville, MS, United States
- 2Department of Biochemistry, Molecular Biology, Entomology, and Plant Pathology, Mississippi State University, Starkville, MS, United States
- 3Institute for Genomics, Biocomputing, and Biotechnology, Mississippi State University, Starkville, MS, United States
Waterlogging induces growth and developmental changes in sensitive crops such as cucumber (Cucumis sativus L.) during early plant development. However, information on the physiological mechanisms underpinning the response of cucumber plants to waterlogging conditions is limited. Here, we investigated the effects of 10-day waterlogging stress on the morphology, photosynthesis, and chlorophyll fluorescence parameters in two cultivars of cucumber seedlings. Waterlogging stress hampered cultivars’ growth, biomass accumulation, and photosynthetic capacity. Both cultivars also developed adventitious roots (ARs) after 10 days of waterlogging (DOW). We observed differential responses in the light- and carbon-dependent reactions of photosynthesis, with an increase in light-dependent reactions. At the same time, carbon assimilation was considerably inhibited by waterlogging. Specifically, the CO2 assimilation rate (A) in leaves was significantly reduced and was caused by a corresponding decrease in stomatal conductance (gs). The downregulation of the maximum rate of Rubisco efficiency (Vcmax) and the maximum rate of photosynthetic electron transport (Jmax) were non-stomatal limiting factors contributing to A reduction. Exposure of cucumber to 10 DOW affected the PSII photochemistry by downregulating the PSII quantum yield (ΦPSII). The redox state of the primary quinone acceptor in the lake model (1-qL), a measure of the regulatory balance of the light reactions, became more oxidized after 10 DOW, indicating enhanced electron sink capacity despite a reduced A. Overall, the results suggest that waterlogging induces alterations in the photochemical apparatus efficiency of cucumber. Thus, developing cultivars that resist inhibition of PSII photochemistry while maintaining carbon metabolism is a potential approach for increasing crops’ tolerance to waterlogged environments.
Introduction
Plants are obligate aerobes and require molecular oxygen to enable cellular respiration and other essential processes. However, due to waterlogging, complete submergence, and poor soil drainage, plants often suffer from hypoxia and anoxia (Fukao and Bailey-Serres, 2004; Bai et al., 2013). Hypoxic conditions are characterized by anaerobic respiration responses, which facilitate energy deficits of up to 37.5% in plants because oxygen diffusion in waterlogged soil is 10,000 times lower than in well-drained soil (Gibbs and Greenway, 2003). Plants experience a series of physiological changes under hypoxia conditions, such as limited gas exchange (Voesenek and Bailey-Serres, 2015), reduced hydraulic conductivity (Tournaire-Roux et al., 2003), changes in gene expression (Xuewen et al., 2014), and increased oxidative damage (Shabala et al., 2014). Collectively, such changes redirect the energetic resources of the plant and prevent them from reaching its true genetic potential. In addition, anaerobic conditions can adversely affect leaf water potential (schildwacht, 1989), enzymatic activity (Hasanuzzaman et al., 2017), nutrient absorption and assimilation (Arduini et al., 2019), plant growth and development (Pan et al., 2021), and ultimately lead to a decline in crop yields.
Waterlogging affects over 16% of the world’s cultivated land (Ploschuk et al., 2018), and over 17 km2 of the land surface is prone to flooding (Voesenek and Sasidharan, 2013). Furthermore, with the rapid climate change, heavy precipitation events are projected to increase by about 7% for every 1°C increase in global warming, leading to increased flood hazard severity (high confidence) (IPCC, 2021). Hence, there is an increasing need to understand the mechanisms of plant tolerance to waterlogging.
Cucumber (Cucumis sativus L.) is one of the world’s most important economic vegetable crops. The current estimated global area for cucumber production is approximately 2.3 million hectares, with an annual output of 87.8 million tons and an average yield of 39.3 t/ha (FAO, 2021). However, cucumbers are sensitive to waterlogging stress due to their shallow root system and high oxygen demand (Xuewen et al., 2014; Xu et al., 2016; Qi et al., 2019). The primary root system of cucumber plants quickly deteriorates under waterlogging due to a lack of oxygen, resulting in energy deficits (Gibbs and Greenway, 2003), limited mineral nutrient uptake and acquisition (Arduini et al., 2019), and a corresponding decline in growth and development (Herzog et al., 2016). Previous studies have shown that the emergence of ARs to promote gas exchange and nutrient absorption is a crucial adaptation mechanism utilized by cucumbers under waterlogging (Qi et al., 2019, 2020). In addition, due to rapidly depleted oxygen under waterlogging, root metabolism changes from aerobic respiration to anaerobic fermentation, while the concentration of CO2 and ethylene continues to increase (Sairam et al., 2009; Pampana et al., 2016). These conditions usually result in a reduction in ATP production from 36 to 2 moles of glucose metabolized (Sousa and Sodek, 2002; Gibbs and Greenway, 2003). The lack of energy at the root system adversely affects hydraulic conductivity, photosynthetic rate, water, and mineral nutrient uptake, all of which are the result of the disruption of aquaporins function (Tournaire-Roux et al., 2003; Tan et al., 2018). Thus, the regulation of the onset and development of ARs represents a critical tool for the survival and adaptation of plants subjected to waterlogging.
The substantial decline in the rate of photosynthesis is another characteristic feature of plants subjected to waterlogging conditions. Previous studies have demonstrated the sensitivity of photosynthesis in many crops, including cucumber (Barickman et al., 2019), squash (Lin et al., 2020), tomato (Bhatt et al., 2015), and watermelon (Yetisir et al., 2006). For instance, within the first day of waterlogging treatment, cucumber plants’ CO2 assimilation rate (A) rate declined rapidly (Kang et al., 2009; Barickman et al., 2019). Thus, even in a short period, the significant reduction in A under waterlogging conditions could lead to a decline in plant energy reserves, indicating the existence of a shared metabolic pattern. Imperatively, the factors affecting the A of plants are primarily divided into stomatal and non-stomatal limitations. Due to limited oxygen under waterlogging conditions, plants close their stomata to maintain plant water status, causing a decline in stomatal conductance (gs) and inhibiting the exchange of CO2 required by the plant’s basic processes (Voesenek and Bailey-Serres, 2015). Kozlowski (1984) attributed the reduction in gs associated with high CO2 concentration during waterlogging to a decrease in root water absorption caused by physical changes in the protoplasm and plasma membrane. Thus, waterlogging impedes the efflux of water, mineral nutrients, and related metabolites (including sucrose, amino acids, proteins, lipids, abscisic acid, etc.) from the root system, resulting in leaf dehydration and stomatal closure. Barickman et al. (2019) reported that a 10-day waterlogging caused a significant reduction in fresh and dry mass (DM) accumulation due to reduce A, gs, and E. Consequently, a reduction in gs eventually leads to a corresponding decrease in A and rate of transpiration (E) (Pedersen et al., 2013).
Other limitations of photosynthesis in waterlogged conditions are the decline in the activity of ribulose-1,5-bisphosphate carboxylase/oxygenase (Rubisco), chloroplast damage, and loss of leaf pigments (Ahmed et al., 2002; Bansal and Srivastava, 2015). These non-stomatal factors severely inhibit A under waterlogging conditions. In addition, waterlogging stress can impair the maximum quantum yield (Fv/Fm) of the photosystem (PSII), leading to photoinhibition of the PSII reaction center (Shao et al., 2013; Zhang et al., 2019). The damage of PSII also affects the photosynthetic electron transport chain and changes the amount of light energy directed to organic synthesis, thereby causing damage to the chlorophyll fluorescence parameters (Guidi et al., 2019). Previous studies have noted critical chlorophyll fluorescence parameters, including photochemical quenching (qP) and electron transport rate (ETR), as valuable evaluation tools for understanding cucumbers’ resilience to waterlogging stress.
Currently, the photosynthesis of cucumber under waterlogging has not been thoroughly studied, especially in the seedling stage. Therefore, more information about the growth, development, and physiological responses is worthy of attention, especially the differences in waterlogging tolerance between cultivars and the time-scale response during waterlogging. The present study evaluated the dynamic changes occurring in cucumber seedlings during a 10-day waterlogging period by determining physiological characteristics such as the growth rate, photosynthesis, and chlorophyll fluorescence. The relative effects of waterlogging stress on carbon assimilation and light reactions were assessed as well. The objective of this study was to explore the waterlogging tolerance of cucumber cultivars using morphological and key physiological parameters that affect carbon assimilation, such as gas exchange and chlorophyll fluorescence parameters. We hypothesized that the carbon assimilation rate of cucumber during waterlogging may be affected by stomatal and non-stomatal factors. This hypothesis was tested in two commercial cucumber cultivars exposed to 10 DOW during early developmental growth stages in a controlled environment.
Materials and methods
Plant material and growth conditions
The study was conducted from 23 March until 14 May 2021 at Mississippi State University’s Vegetable Physiology Greenhouse and Laboratory Facility on the North Mississippi Research and Extension Center (Verona, MS; 34.1651, –8.7206). Two cucumber cultivars, “Straight 8” and “Marketmore,” were selected for this study. Based on a previous study by Barickman et al. (2019), “Straight 8” exhibited apparent sensitivity to waterlogging stress, while “Marketmore” is a known commercial cultivar with tolerance to environmental stress. Thus, both cultivars were used to determine if they might respond differently to waterlogging tolerance. The selected cultivars have similar growth and life cycle duration of 50–70 days. Seeds of both cucumber cultivars (Wax Seed Company, Amory, MS, United States) were grown in a controlled environmental chamber (Model E-41L2; Percival Scientific, Inc., Perry, IA) under the following conditions: relative humidity of 50%; photoperiod of 16/8 h (light/dark); a temperature of 26/22°C (day/night); and light intensity of average 420 μmol m–2 s–1. Similar environmental conditions have previously been used in growing cucumber plants under controlled conditions (Barickman et al., 2019; Qi et al., 2019, 2020). Multiple seeds of each cultivar were sown in 10-cm pots and filled with a soil-less potting mixture (Metro Mix 830, F3B, Sun Gro Horticulture, Agawam, MA, United States). An N-P-K (15-3.9-9.9) controlled-release fertilizer (Osmocote; Scotts Miracle-Gro, Marysville, OH, United States) was applied to the substrate at the rate of 5.93 kg.m–3. At 7 days after sowing (DAS), seedlings were thinned to one plant per pot.
Waterlogging treatments
Fourteen days after germination, uniformly emerged seedlings of cucumber at the second vegetative (V2) leaf stage were subjected to two experimental treatments of waterlogging and control (non-waterlogging) treatments for 10 days. Waterlogging treatments were imposed on cucumber plants by placing six pots of each cucumber cultivar into four replicated 11 L containers (Rubbermaid Inc., Wooster, OH, United States). To simulate waterlogging conditions, each container was filled with enough water to reach 3 cm above the substrate surface. Pots containing cucumber plants under control treatments were watered at optimum field capacity as needed. After 10 days of the treatment (DAT), cucumber plants under waterlogging and control treatments were evaluated for morphological and physiological performance.
Gas exchange measurements
After 10 DAT, photosynthetic and fluorescence parameters were measured on the second most fully expanded trifoliate. The A, gs, intercellular CO2 concentration (Ci), and E were measured in situ with chlorophyll fluorescence at the North Mississippi Research and Extension Center (16.00–20:00 CST) using an LI-6800 portable photosynthesis system (LI-COR, Biosciences, Lincoln, NE, United States). Measurements were allowed to match the chamber environment before the values were recorded. The chamber environment was set to match the growth chamber, with 500 μmol m–2 s–1 of light, 415 ppm of CO2 concentration in the air (Ca), and 50% of relative humidity. Measurements were conducted on six representative cucumber plants of each cultivar subjected to waterlogging and non-waterlogging treatments. The ratio of A/gs was used to calculate intrinsic water use efficiency (WUE) (Martin and Ruiz-Torres, 1992). The internal to external CO2 ratio was calculated using the Ci/Ca relationship, and the stomatal limitation (Ls) was calculated as 1- Ci/Ca.
Chlorophyll fluorescence measurements
The LI-6800 using pulse-amplitude modulated (PAM) fluorometry with a Multiphase Flash Fluorometer (6800-01A, LI-COR Biosciences, Lincoln, NE, United States) was used to measure the chlorophyll fluorescence at 10 DAT. During predawn hours (7.00–10:00 CST), the minimal fluorescence (Fo) was measured on the second-most fully expanded leaf using a measuring light (0.005 μmol m–2 s–1). In dark-adapted leaves, the maximal fluorescence (Fm) was quantified using a 1-s saturating pulse at 8,000 μmol m–2 s–1. The leaves were continuously illuminated for 20 min with an actinic light (1,400 μmol m–2 s–1) to record the steady-state yield of fluorescence (Fs). Maximal light-adapted fluorescence yield (Fm′) was determined by 8,000 μmol m–2 s–1. The actinic light was turned off, and minimal fluorescence yield (Fo′) in the light-adapted state was determined after 5 s of far-red illumination. The difference between the measured values of Fm and Fo is the variable fluorescence (Fv). The chlorophyll fluorescence parameters were calculated using the following formulas (Genty et al., 1989; Maxwell and Johnson, 2000).
where Fv/Fm is the maximal photochemical efficiency of PSII, ΦPSII is the actual photochemical efficiency of PSII, ΦNPQ is the quantum yield for the energy dissipated via Δ pH and xanthophyll-regulated processes, ΦNO is the quantum yield of non-regulated energy dissipated in PSII, and qP and NPQ are photochemical and non-photochemical qP, respectively. ETR was calculated according to Genty et al. (1989).
Measurement of CO2 response
The CO2 response curve (A/Ci) measurements were evaluated using the auto program settings in the LI-6800 at 10 DAT. To measure the steady-state response A/Ci, the leaf chamber settings were fixed at 60% relative humidity, the corresponding light intensity of the growth chamber (500 μmol m–2 s–1), and the temperature was set at 24°C. Using the built-in program on the LI-6800, measurements were taken in a decreasing then ascending bitonic sequence decreasing from ambient (415 ppm) to 50 ppm then to 1,500 ppm, taken measurements at 300, 200, 100, 50, 200, 400, 600, 800, 1,000, 1,200, and 1,500 ppm CO2, with early matching, enabled, and wait times 60–90 s between measurements. A/Ci analyses were performed according to Sharkey et al. (2007), with few changes as portrayed in Olorunwa et al. (2021) using the excel fitting tool 10.0 available at https://landflux.org/tools. The estimated A/Ci response curve was further utilized to calculate the maximum rate of Rubisco carboxylation efficiency (Vcmax) and maximum rate of photosynthetic electron transport (Jmax), according to Sharkey et al. (2007). Representative individual curves were fitted separately, and the extracted parameters were averaged across replicates for each treatment.
Measurement of light curves
For the light response curves of the photosynthetic performance for both cultivars, measurements were taken using the built-in program on the LI-6800 across light intensities of 0, 10, 50, 75, 100, 200, 400, 600, 800, 1,000, 1,200, and 1,500 μmol m–2 s–1. Minimum and maximum wait times were 120–150 s between measurements with early matching enabled. During the measurements, chamber CO2 was held at 415 ppm, temperature at 24 ± 0.5°C, and relative humidity at 60%. The light response curve fitting was done in two parts: first, the optimal equation was determined by plugging the data into the non-rectangular hyperbola-based model of Prioul and Chartier (1977) and identifying the lowest sum of the squares of the errors as described by Lobo et al. (2013). This resulted in the curve by Abe et al. (2009) having the best fit, and various parameters, including light compensation point (Ic), light saturation point (Ik), apparent quantum yield (Φi), and the photosynthetic rate at saturated light (Pmax) were calculated from light response curves using solver function of Microsoft Excel furnished by Lobo et al. (2013).
Morphological performance and plant harvest
Six representative cucumber plants (from each treatment/rep/cultivar) were harvested at the end of the 10-day experiment to obtain morphological performance of the impact of waterlogging stress. Plant’s phenotypic data of leaf number (LN), leaf area (LA), and leaf fresh mass (FM) were evaluated for each treatment combination. LA was measured using the LI-3100 leaf-area meter (Li-Cor Bioscience, Lincoln, NE). Plant component FM was measured from all plants by using a weighing scale. The sample of the plant FM was lyophilized using a FreeZone 2.5 L Freeze Dryer (Labconco Corp., Kansas City, MO, United States) to determine the DM and percent dry mass (%DM).
Statistical analysis
The experiment was a randomized complete block design with two waterlogging treatments, two cucumber cultivars, four replications, and 12 plants in a factorial arrangement. In total, 192 plants (4 replicates × 2 waterlogging treatments × 2 cucumber cultivars × 12 plants) were utilized in this study. SAS (version 9.4; SAS Institute, Cary, NC) was used to perform the statistical analysis of the data. Replicated values of all morphological and photosynthetic parameters measured in this study were analyzed using a two-way analysis of variance of the generalized linear mixed model (PROC GLIMMIX) to determine the effects of waterlogging treatments, cucumber cultivars, and their interactions. Fisher’s protected least significant difference tests at p ≤ 0.05 were employed to test the differences among interactions of cultivars and treatments for measured parameters. The standard errors of the mean were calculated using the pooled error term from the ANOVA table and presented in the figures as error bars. Diagnostic tests, such as Shapiro–Wilk in SAS, were conducted to ensure that treatment variances were statistically equal before pooling. Pearson correlation analysis was utilized to study the relationship between the studied parameters. Graphs were plotted with OriginPro software (version. 9.8.5.204; Origin Lab Corporation, Northampton, MA).
Results
Waterlogging induces morphological changes in cucumber cultivars
Supplementary Table 1 shows no significant interaction between cucumber cultivars and waterlogging treatments for all morphological parameters measured except the DM. However, cultivars and waterlogging treatments independently and significantly affected leaf number (LN), LA, and FM.
As displayed in Figure 1, the growth of “Marketmore” and “Straight 8” was affected at 10 DOW, and “Marketmore” was more tolerant than “Straight 8.” The LN, LA, FM, and DM of “Marketmore” decreased by 8, 8, 14, and 8%, respectively, while the same parameters for “Straight 8” were reduced by 14, 17, 25, and 25%, respectively, compared to those of control (Figure 2). The results also revealed that after 10 DOW the cultivars developed ARs primordia on their hypocotyls compared to the control plants (Figure 1).
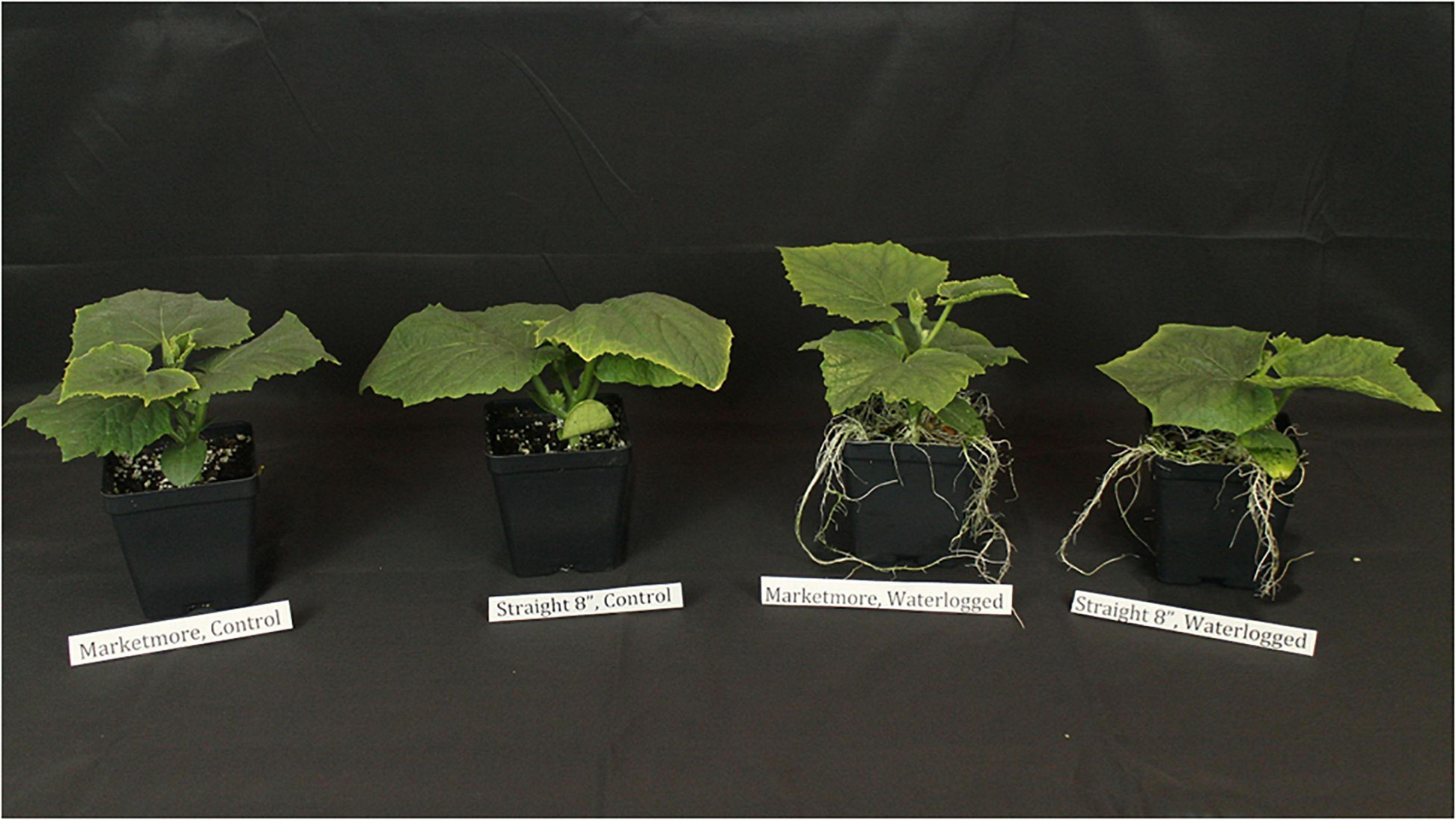
Figure 1. Morphological performance of “Marketmore” and “Straight 8” cucumber cultivars under 10-day control and waterlogging treatments.
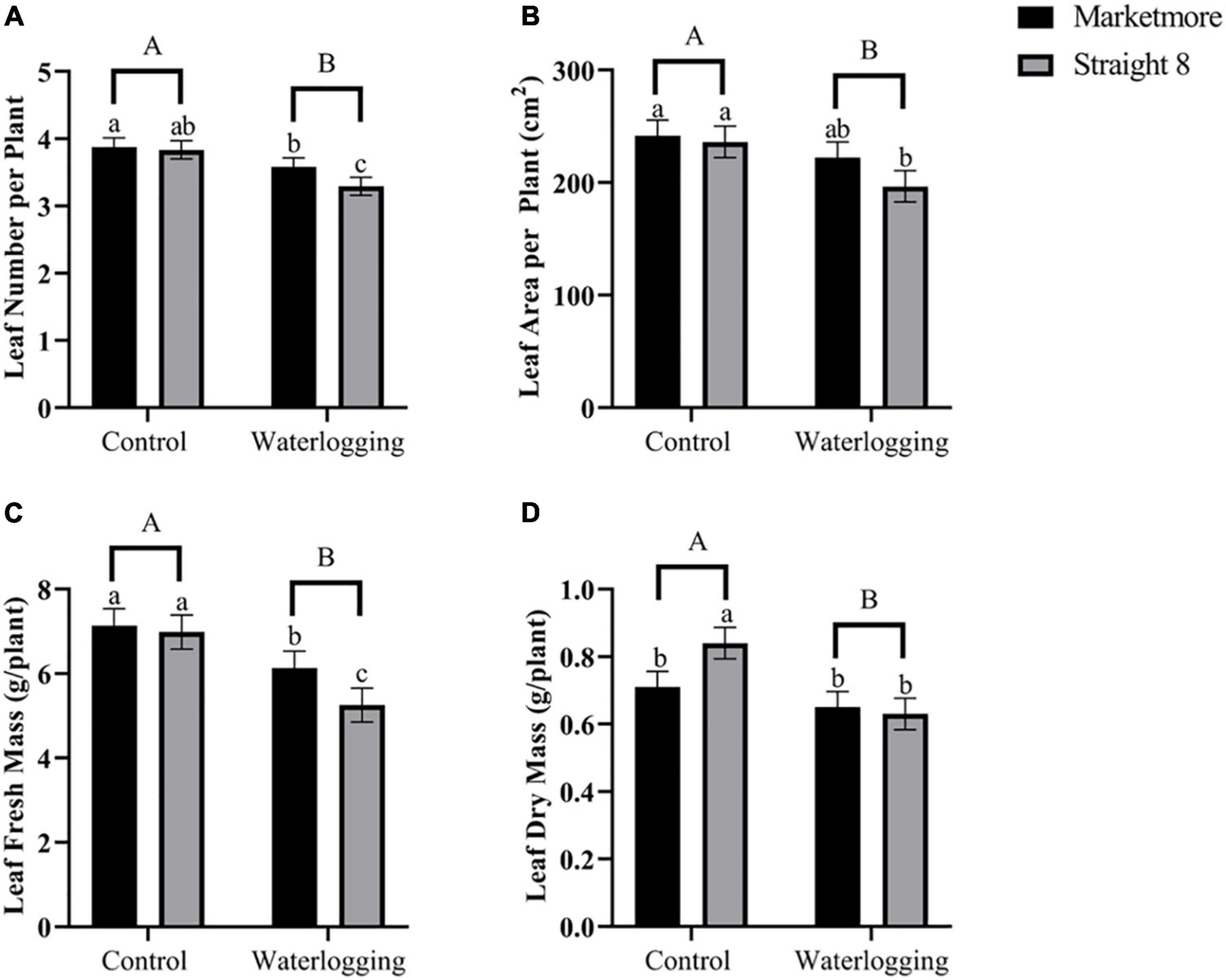
Figure 2. Comparison of (A) mean leaf number (LN), (B) mean leaf area (LA), (C) mean leaf fresh mass (FM), and (D) mean leaf dry mass (DM) of cucumber cultivars (Marketmore and Straight 8) after 10 days of control and waterlogging treatments. Different low case and uppercase letters indicate significant differences between the cultivar’s means and treatments, respectively (P < 0.05) as determined by Fisher’s LSD. The error bar on the vertical bar indicates the standard error of the mean ± 4 replications of each morphological trait. Standard error of the mean, LN = 0.136; LA = 13.928; FM = 0.403; DM = 0.046.
Waterlogging induces changes in the photosynthetic performance of cucumber cultivars
Waterlogging treatments significantly affected all the gas exchange parameters measured between the two selected cultivars, as shown in Supplementary Table 1. The A was observed to be significantly lesser in “Straight 8” (12.46 μmol m–2 s–1) as compared to the “Marketmore,” (13.73 μmol m–2 s–1) at 10 DOW (Figure 3A). However, gs, E, and WUE declined to similar levels in the two cultivars: 22, 14, and 52% in “Marketmore,” and 24, 13, and 40% in “Straight 8,” respectively, when compared to the control treatments (Figure 3). In addition, 10 DOW significantly decreased the Ls of “Marketmore” and “Straight 8” by 43 and 34%, respectively (Figure 3F). Interestingly, after 10 DOW, the Ci of “Marketmore” and “Straight 8” significantly increased by 6 and 4%, respectively, compared to controls (Figure 3D).
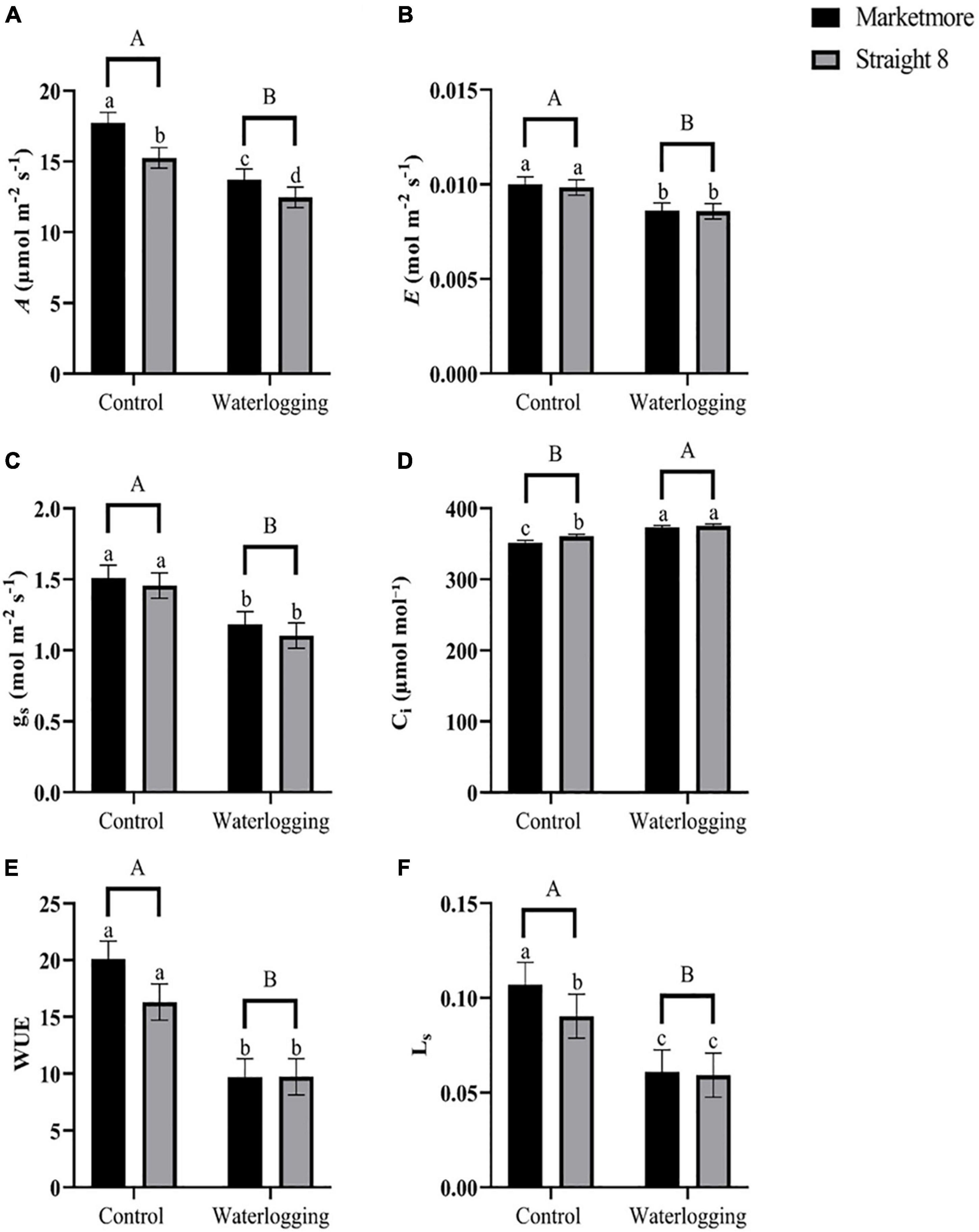
Figure 3. (A) CO2 assimilation rate (A), (B) leaf transpiration rate (E), (C) stomatal conductance (gs), (D) intercellular CO2 concentration (Ci), (E) intrinsic water use efficiency (WUE), and (F) stomatal limitation (Ls) of cucumber cultivars (Marketmore and Straight 8) after 10 days of control and waterlogging treatments. Different low case and uppercase letters indicate significant differences between the cultivar’s means and treatments, respectively (P < 0.05) as determined by Fisher’s LSD. The error bar on the vertical bar indicates the standard error of the mean ± 4 replications of each leaf gas exchange trait. Standard error of the mean, A = 1.23; E = 0.0007; gs = 0.153; Ci = 4.91; WUE = 2.77; Ls = 0.0116.
We used the gas exchange data of cucumber cultivars displayed in Figure 3 to estimate the velocity of Rubisco carboxylation (Vc) and oxygenation (Vo) (Figures 4A,B) to understand whether the increase in carbon loss from photorespiration could explain the decrease in A under waterlogging stress. Both cultivars showed a similar pattern under 10-day waterlogging stress for Vo (Figure 4B). However, waterlogging treatment and cultivar independently and significantly affected Vc (Supplementary Table 1). Compared to the control treatments, waterlogging significantly decreased Vc and Vo in “Marketmore” by 23 and 33%, respectively, and by 19 and 28%, respectively, in “Straight 8” (Figures 4A,B). In addition, cultivars and waterlogging treatments significantly and independently influenced the calculated Vcmax and Jmax from the A/Ci response curve (Figure 5). Under 10 DOW, the Vcmax and Jmax declined by 14 and 15% in “Marketmore” and by 33 and 14% in “Straight 8,” respectively, compared to non-waterlogged plants (Figures 4C,D).
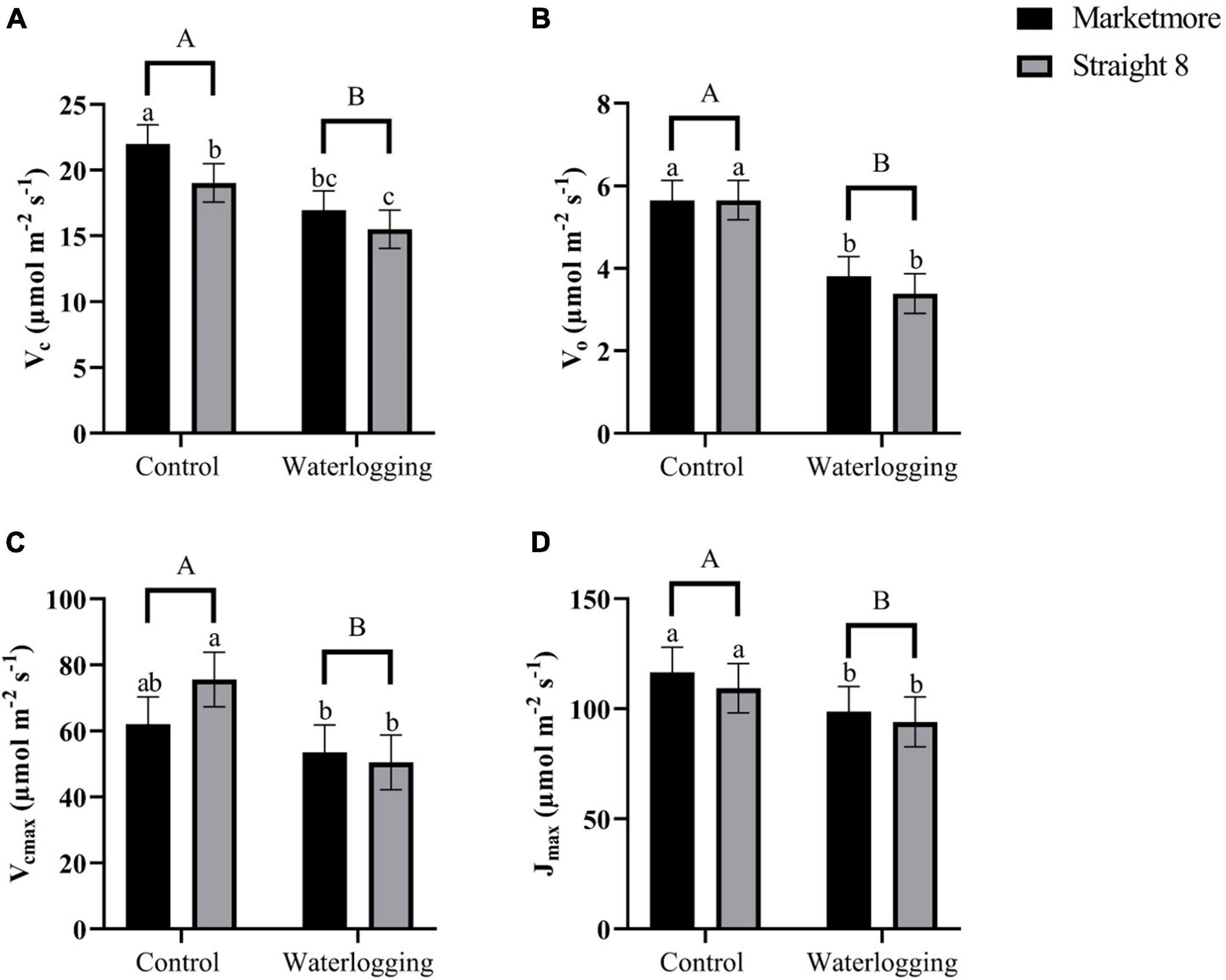
Figure 4. (A) Velocity of rubisco carboxylation (Vc), (B) velocity of rubisco oxygenation (Vo), (C) maximum rate of rubisco carboxylation efficiency (Vcmax), and (D) maximum rate of photosynthetic electron transport (Jmax) of cucumber cultivars (Marketmore and Straight 8) after 10 days of control and waterlogging treatments. Different low case and uppercase letters indicate significant differences between the cultivar’s means and treatments, respectively (P < 0.05) as determined by Fisher’s LSD. The error bar on the vertical bar indicates the standard error of the mean ± 4 replications of each leaf gas exchange trait. Standard error of the mean, Vc = 1.46; Vo = 0.478; Vcmax = 8.24; Jmax = 11.30.
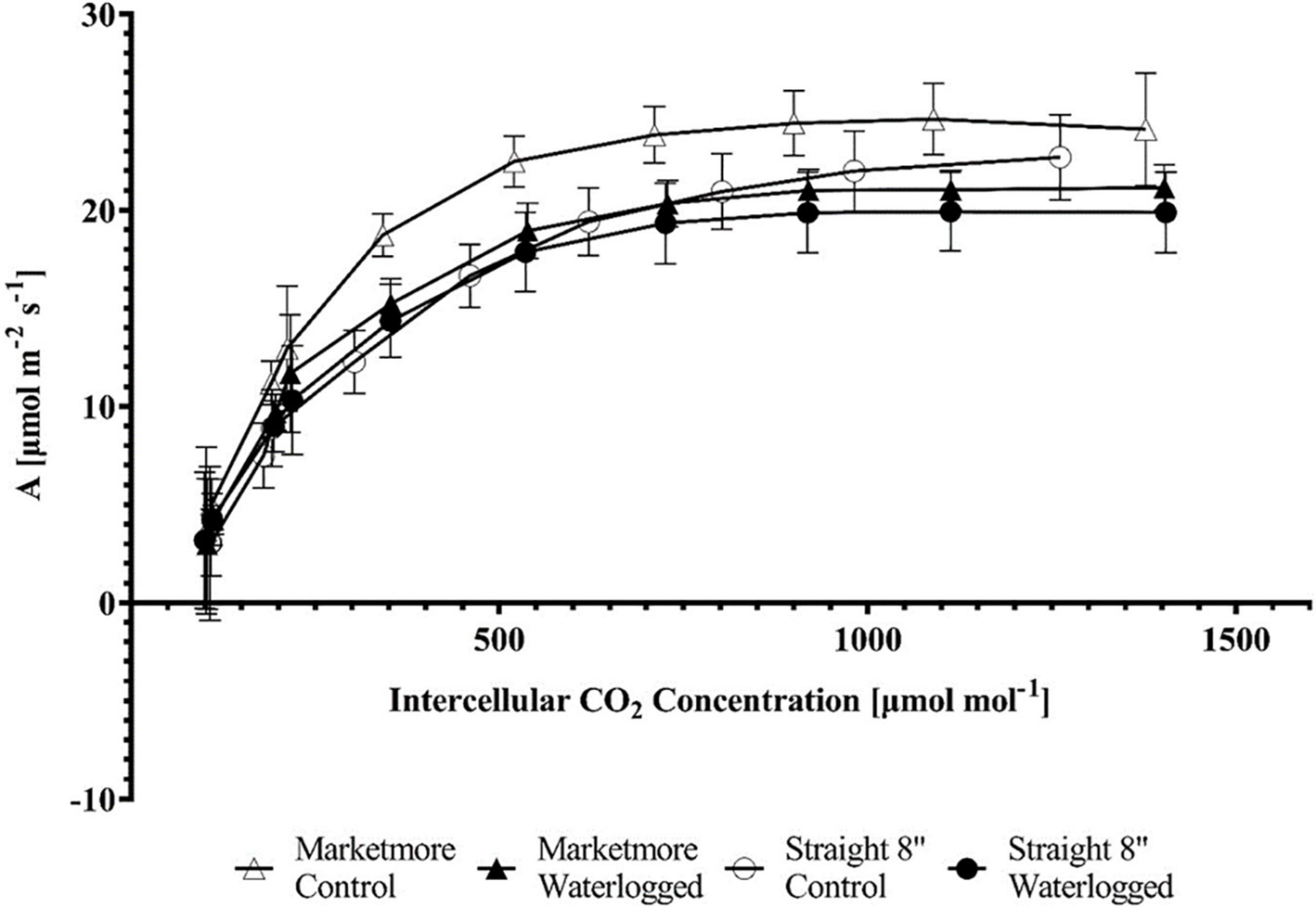
Figure 5. Response of the CO2 assimilation rate (A) to increasing intercellular CO2 concentration (Ci) (A/Ci Curve) in the two cucumber cultivars (Marketmore and Straight 8) after 10 days of control and waterlogging treatments. The vertical bars represent the standard error of the mean (n = 4).
Waterlogging induces changes in the photochemical efficiency of cucumber cultivars
The values of Fv/Fm following 10 DOW showed the maximum photochemical efficiency slightly declined in “Marketmore” and “Straight 8” compared to the control treatments (Figure 6A). Under waterlogged conditions, the Fv′/Fm′ values were found to be comparable for both cultivars (Figure 6B), indicating that PSII was not significantly photoinhibited. In addition, the Fo values of cucumber cultivars under waterlogging decreased by 5 and 6%, respectively, in “Marketmore” and “Straight 8” (Figure 6C). However, there was no significant difference in the values of Fm of both cultivars under waterlogging conditions (Figure 6D). After 10 DOW, the steady state of fluorescence (Fs) decreased more in “Marketmore” than in “Straight 8” (Figure 6E). The redox state of qP, which measures the fraction of open PSII reaction centers, decreased significantly after 10 DOW treatments in both cultivars, compared to the control (Figure 6F).
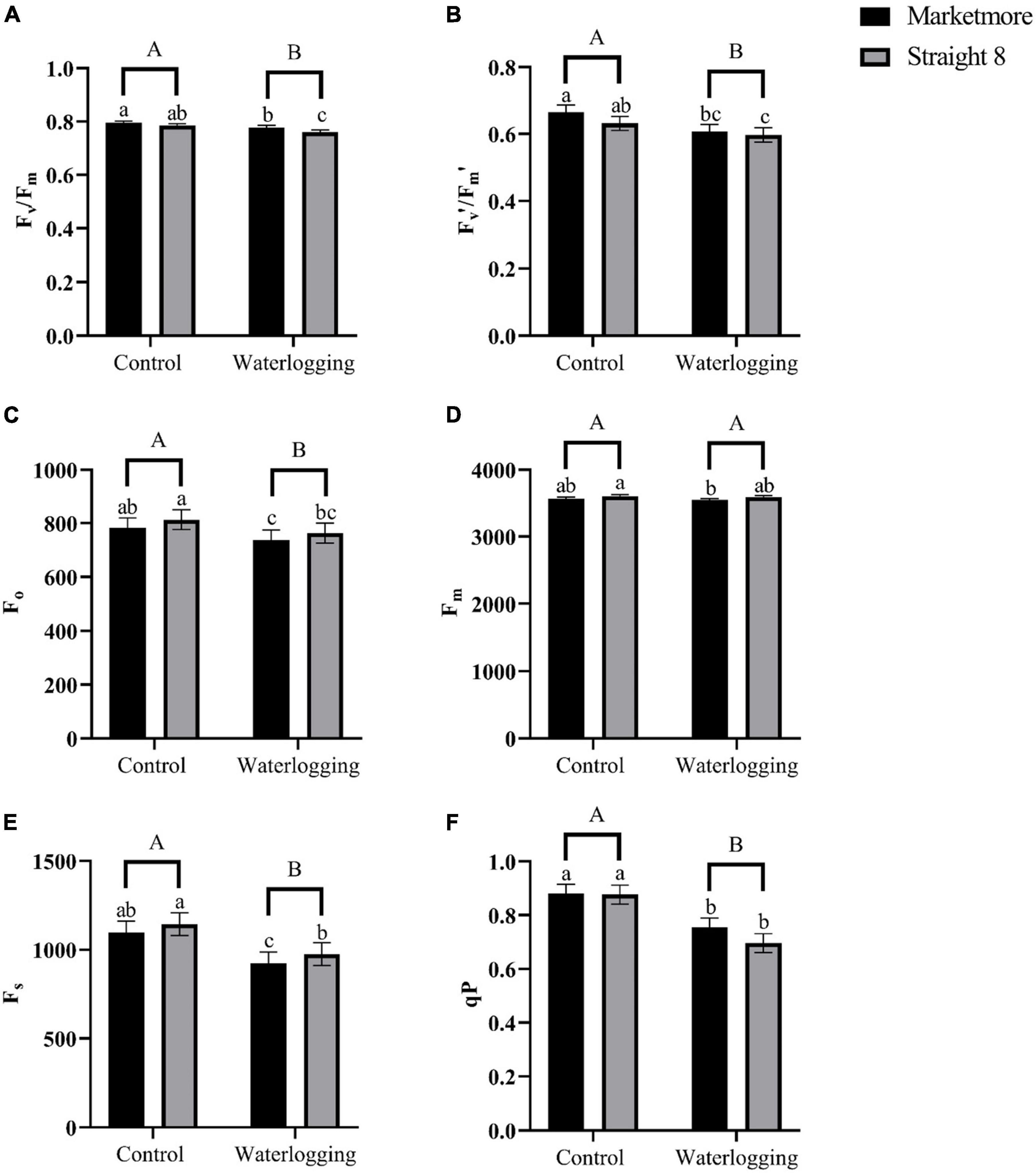
Figure 6. (A) Maximum quantum efficiency of PSII in dark-adapted state (Fv/Fm), (B) maximum quantum efficiency of PSII in the light-adapted state (Fv′/Fm′), (C) initial fluorescence (Fo), (D) maximum fluorescence (Fm), (E) steady-state fluorescence (Fs), and (F) redox state of the plastoquinone pool (qP) of cucumber cultivars (Marketmore and Straight 8) after 10 days of control and waterlogging treatments. Different low case and uppercase letters indicate significant differences between the cultivar’s means and treatments, respectively (P < 0.05) as determined by Fisher’s LSD. The error bar on the vertical bar indicates the standard error of the mean ± 4 replications of each leaf gas exchange trait. Standard error of the mean, Fv/Fm = 0.008; Fv′/Fm′ = 0.021; Fo = 35.95; Fm= 85.43; Fs= 64.09; qP = 0.03.
Contrarily, alterations in NPQ, which reflect heat dissipation using cucumber leaves, significantly increased after 10 days of waterlogging, compared to the control (Figure 7C). At 10 DOW, the ETR at PSII decreased significantly in “Marketmore” and “Straight 8” by 23 and 26%, respectively, compared to the control treatments (Figure 7B). In addition, we estimated the light energy partitioning at PSII, that is, ΦPSII, ΦNPQ, and ΦNO, which sum to one (Kramer et al., 2004). The ΦPSII of “Marketmore” and “Straight 8” under waterlogging experienced a significant decline of 22 and 25% compared to control treatments (Figure 7A). However, the ΦNPQ and ΦNO increased significantly in both cultivars compared to the control (Figures 7D,E). Furthermore, the redox state of QA based on the lake model (1-qL) after 10 DOW treatments became more oxidized in both cucumber cultivars compared to the control plants (Figure 7F).
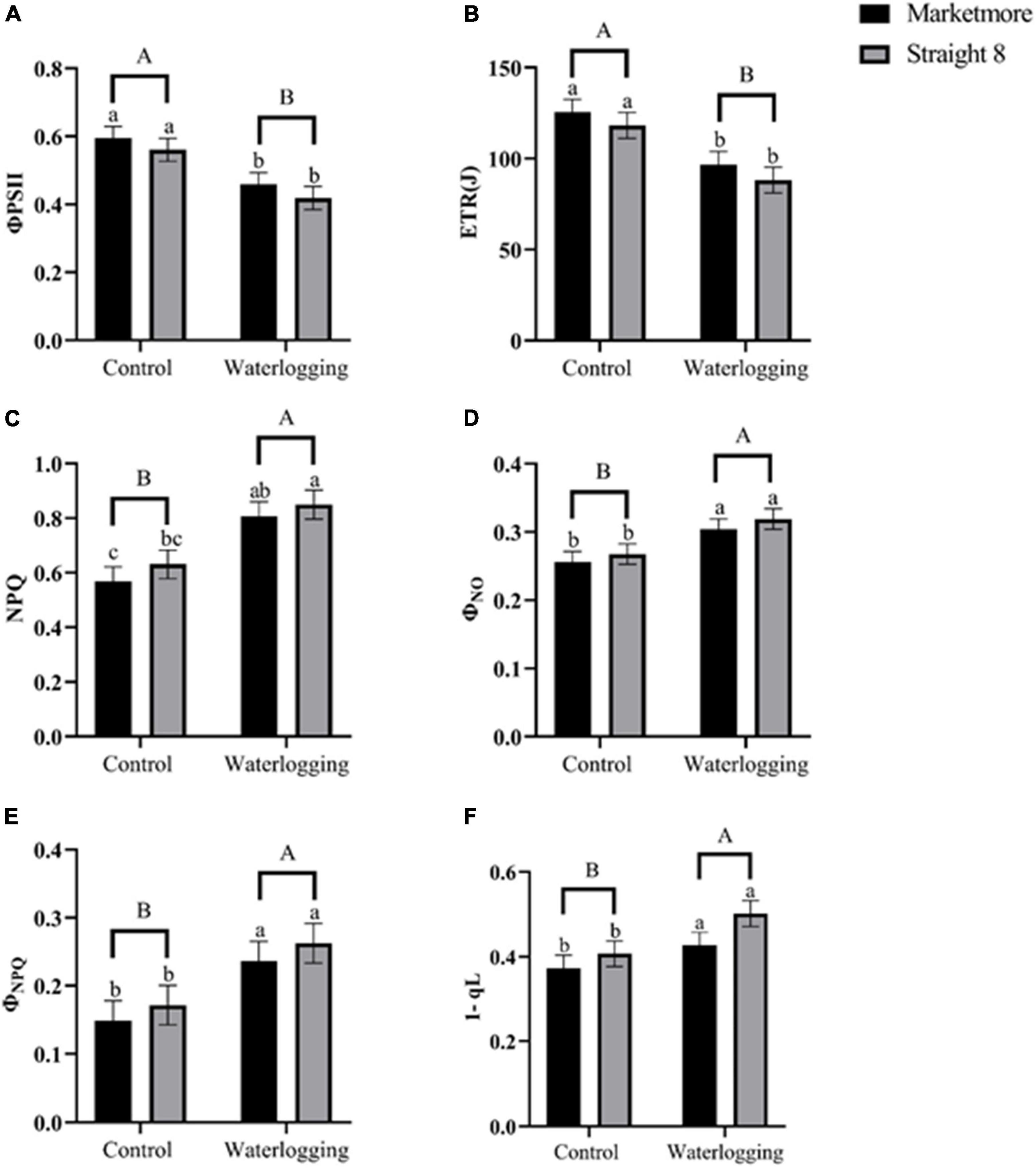
Figure 7. (A) Effective quantum yield of PSII (ΦPSII), (B) electron transport rate (ETR), (C) non-photochemical quenching (NPQ), (D) quantum yield of non-regulated energy dissipated in PSII (ΦNO), (E) quantum yield of regulated non-photochemical energy loss in PSII (ΦNPQ), and (F) redox State of Plastoquinone Pool based on the Lake Model (1-qL) of cucumber cultivars (Marketmore and Straight 8) after 10 days of control and waterlogging treatments. Different low case and uppercase letters indicate significant differences between the cultivar’s means and treatments, respectively (P < 0.05) as determined by Fisher’s LSD. The error bar on the vertical bar indicates the standard error of the mean ± 4 replications of each leaf gas exchange trait. Standard error of the mean, ΦPSII = 0.03; ETR = 7.14; NPQ = 0.09; ΦNO = 0.015; ΦNPQ= 0.029; 1-qL = 0.03.
Waterlogging induces changes in response curves of cucumber cultivars
An A/Ci curve was constructed to understand the biochemical processes of A’s response in cucumbers under waterlogging conditions. The A of the cultivars subjected to waterlogging and control treatments increased with increasing Ci from 0 to 1,500 μmol mol–1 (Figure 5). However, the A was lower under waterlogging when compared to the control conditions. A corresponding response was observed with the light response curve. The A of cucumbers subjected to 10 DOW or control treatments increased with increasing PPFD from 0 to 1,500 μmol m–2 s–1 (Figure 8). According to the requirements of PPFD, cucumber plants clearly showed the maximum photosynthetic rate (Amax) under the light intensity of 1,500 μmol m–2 s–1 under both control and waterlogging treatments. Under control treatment, Amax was 23.62 ± 1.27 μmol m–2 s–1 in “Marketmore” and 19.59 ± 2.67 μmol m–2 s–1 in “Straight 8.” However, Amax was reduced by 8% under waterlogging in “Marketmore” and reduced by 20% under waterlogging in “Straight 8.”
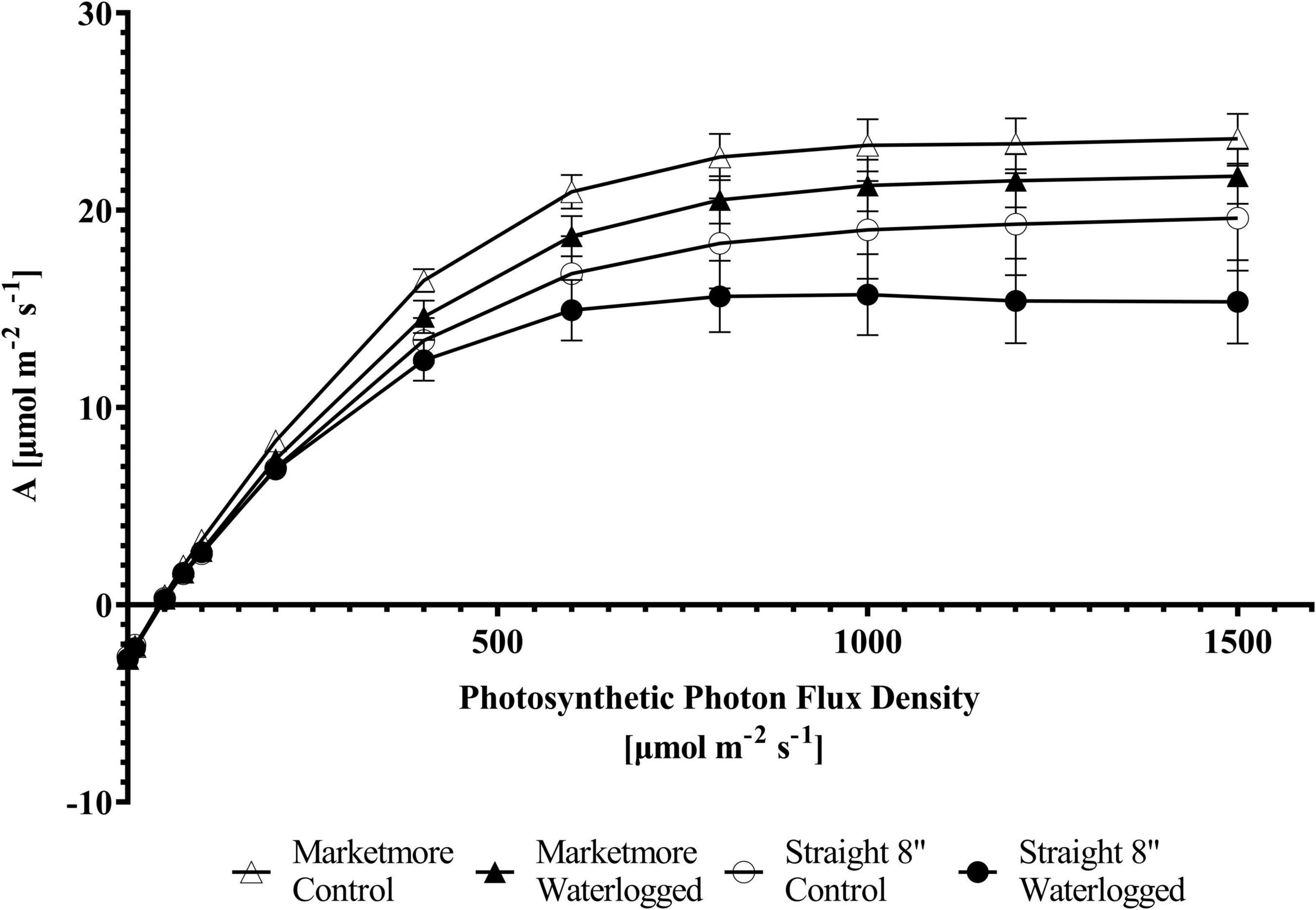
Figure 8. Photosynthetic responses to different light intensities (photosynthetic photon flux density; PPFDs) of the two cucumber cultivars (Marketmore and Straight 8) after 10 days of control and waterlogging treatments. The vertical bars represent the standard error of the mean (n = 4).
Relationships between biomass, gas exchange, and chlorophyll fluorescence parameters
Figure 9 shows the results of a Pearson’s correlation analysis of the biomass, gas exchange, and chlorophyll fluorescence parameters of the “Marketmore” and “Straight 8” under control and waterlogged treatments. The results revealed that the various parameters studied were highly correlated. Except for NPQ, ΦNO, ΦNPQ, and 1-qL, which showed a negative correlation, there was a significant and positive correlation between the biomass yields (FM; DM) of cucumbers with the gas exchange parameters. Similarly, most photosynthetic traits (A, gs, E, WUE) under waterlogging treatments were significantly and positively correlated with Fv/Fm, qP, ETR, and ΦPSII, but negatively associated with NPQ, ΦNO, ΦNPQ, and 1-qL. However, the correlation coefficients of Ci with most parameters were in the range considered moderate to weak. In addition, Vcmax and Jmax followed the same pattern as the photosynthetic traits, but Jmax had a stronger positive correlation compared to Vcmax.
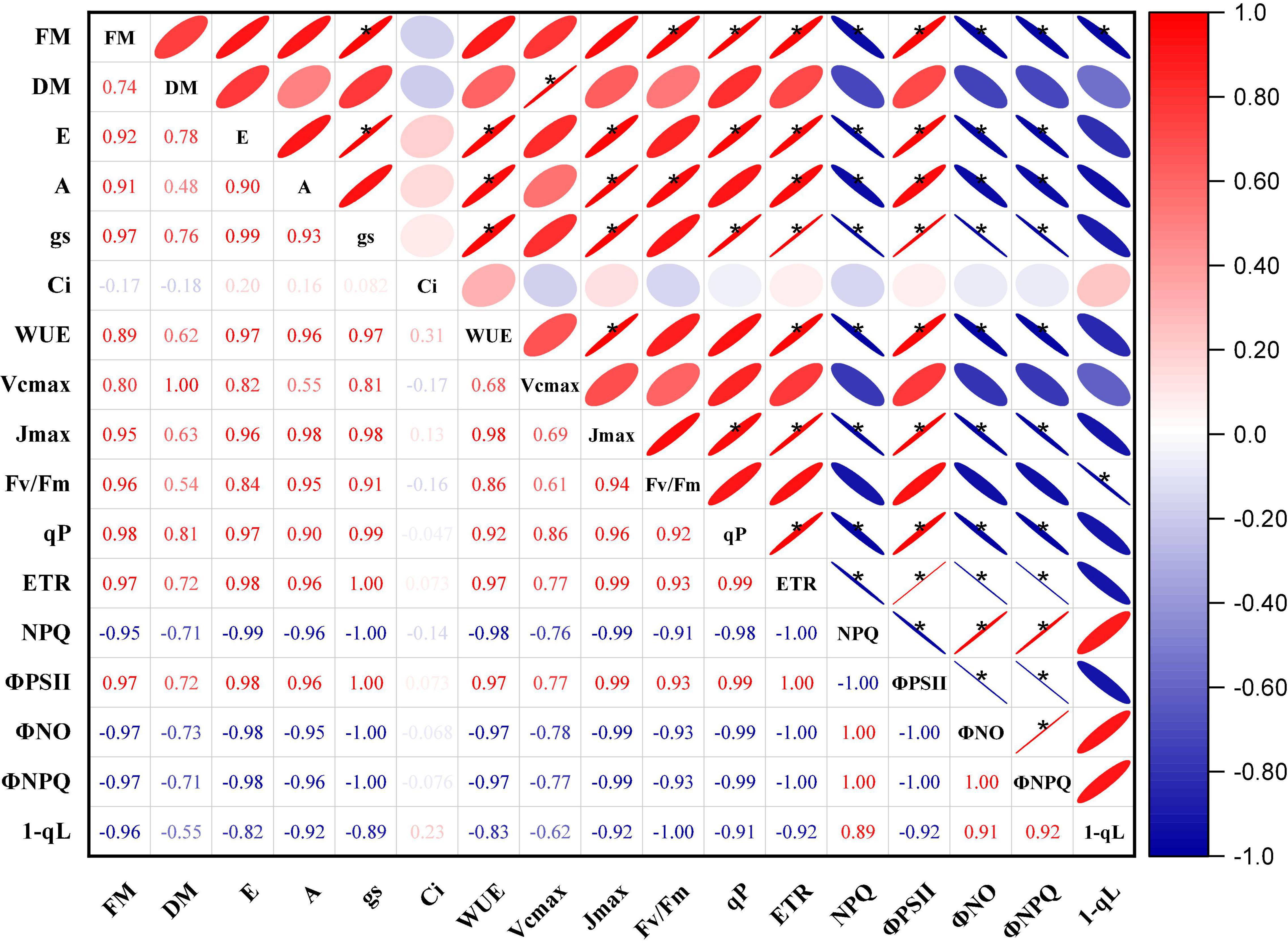
Figure 9. Pearson’s correlation matrix of the changes in biomass, gas exchange, and chlorophyll fluorescence parameters of the two cucumber cultivars under control and waterlogging treatments. Dark color represents strong correlations, and light background color represents weaker correlations. Values close to zero indicate no correlation, and values close to one indicate a strong correlation (positive—red and negative—blue) between two parameters. *Represent correlation coefficient significance levels at P ≤ 0.05.
Discussion
Waterlogging stress imposed on the cucumber plants during the early stages of development results in dramatic changes in plant physiology and biochemistry, with detrimental impacts on morphology and yields. Our previous study on commercially cultivated cucumber plants (“Straight 8”) revealed that the seedling growth stage is extremely sensitive to waterlogging stress (Barickman et al., 2019). We hypothesized that the rapid decline of photosynthetic traits, including A, E, and gs might contribute to the susceptibility of cucumber plants to waterlogging stress. As an adaptive response to hypoxia, the reduction in the photochemical efficiency of PSII and the formation of ARs could mitigate the potential damage to photosynthetic apparatus caused by waterlogging (Smethurst and Shabala, 2003; Olorunwa et al., 2022). With this hypothesis in mind, we evaluated plant growth, photosynthesis, and chlorophyll fluorescence parameters to identify cultivar-specific and differential responses.
We observed a significant decline in LN, LA, FM, and DM of both cultivars after 10 DOW, demonstrating the sensitivity of cucumber plants at the seedling stage to hypoxia. Previous studies revealed that waterlogging induces morphological changes in plants, especially those related to leaf photosynthesis (Zhang et al., 2019; Olorunwa et al., 2022). Analogous findings have been made in previous studies on cucurbits, including cucumber (Barickman et al., 2019), watermelon (Yetisir et al., 2006), and summer squash (Huang et al., 1995). The alterations in the morphology of cucumber plants under waterlogging can be attributed to reduced water and nutrient uptake and transportation due to energy deficits during anaerobic respiration, resulting in restricted cell expansion and leaf growth (Gibbs and Greenway, 2003; Parent et al., 2008).
The effects of waterlogging stress on leaf morphology and biomass are dependent on plant growth stage, duration, and cultivars. Zheng et al. (2021) reported a significant decline in the waterlogging sensitive “Zaojia8424” leaf biomass compared to the waterlogging tolerant cultivar “YL,” which naturally had smaller leaves. Generally, smaller leaves are more beneficial for mitigating waterlogging stress than larger leaves because of their high boundary layer conductance, which prevents heat accumulation (Leigh et al., 2017). In the current study, 10-day waterlogging reduced the leaf biomass and area in “Straight 8” and “Marketmore” cultivars, with no statistical difference between the two cultivars (Figure 1). In addition, ARs developed in the hypocotyls of both cultivars after 10 DOW. Previous studies showed a similar response for other cucurbits during their early developmental growth stages (Huang et al., 1995; Xu et al., 2016; Qi et al., 2019). The ARs of “Marketmore” was more pronounced after 10 DOW when compared to the “Straight 8” cultivar, mainly due to highly aerenchymatous cortical structures (Visser et al., 1996). In addition, after applying 1-methylcyclopropene (ethylene receptor inhibitor), 1-naphthylphthalamic acid (auxin transport inhibitor), or diphenyleneiodonium (NADPH oxidase inhibitor) to waterlogged cucumbers, Qi et al. (2019) observed the formation of ARs. This implies that auxin, ethylene, and ROS production are enhanced under excess water conditions. Also, Vidoz et al. (2010) suggested that ethylene and auxin might interact in forming ARs in waterlogged tomato hypocotyls using hormone-insensitive mutants. Since auxin and ethylene are transported polarly through ATPase-dependent carrier proteins (Visser et al., 1996), waterlogging-induced low levels of ATP cause these hormones to accumulate near the basal stem of waterlogged plants for solute transport, thereby acting as positive regulators for cucumber waterlogging tolerance.
Waterlogging induces significant changes in the photosynthetic performance of C3 crops, especially in sensitive plants like cucurbits (He et al., 2018; Barickman et al., 2019; Lin et al., 2020). This response was evident in the current study as A, gs, and E for the two cultivars after 10 DOW treatments were significantly reduced. The results of the gas exchange analysis of cucumber plants after 10 DOW indicated that the decline in A can be due to both stomatal and non-stomatal factors according to the version of the photosynthesis model by Farquhar et al. (1980). Under waterlogging, the significant decline in A and biomass was mainly due to reduced water potential, which resulted in the rapid closure of the stomata through the effect of abscisic acid on potassium ion regulation of guard cell turgor (Sojka, 1992; Ploschuk et al., 2018). Barickman et al. (2019) opined that restricting A in cucumber plants through stomatal closure under waterlogging could limit the amount of photoassimilate available for the growth and maintenance of the cucumber vegetative and fruit tissues. In agreement with our results, Issarakraisila et al. (2007) found that the A in kale plants was associated with decreased gs after 14 DOW treatments. Although decreased gs is the most common response in plants to waterlogging, the reduction in Ci can also be ascribed to non-stomatal factors. The current study showed that gs decreased in two cultivars after 10 DOW, associated with decreased A and E and increased Ci. Thus, a significant increase in Ci and a decrease in Ls in response to changes in A and decreased gs under stressed conditions suggest an essential contribution of non-stomatal limitation to A (Farquhar and Sharkey, 1982). Previous studies (Yordanova and Popova, 2007; Liu et al., 2014; Pompeiano et al., 2019) in sensitive plants have reported similar findings. In addition, the WUE of both cultivars tested in this study decreased significantly under 10 DOW. Previous research found that waterlogging-sensitive plants have lower WUE and thus gain less carbon per unit of water lost (Meena et al., 2015; Velasco et al., 2019).
In the present study, the reduction in A corresponds to the reduction of Vcmax and Jmax, which are considered to represent the carboxylation and regeneration of Rubisco, respectively. Previous studies have attributed the decrease in Vcmax and Jmax to the decline in the amount of active Rubisco and a reduction in the contribution of ATP during photosynthesis (Flexas et al., 2014). Urban et al. (2008) considered that changes in A response to abiotic stress could be paralleled to the changes in Rubisco activity mediated by Jmax to balance the modification of Vcmax when subjected to stress. In this context, the observed decrease in Vcmax and Jmax for both cultivars after 10 DOW appears to be consistent with a previous study (Ma and Guo, 2014). The A/Ci further demonstrated that waterlogging stress significantly decreased A with increasing Ci. As per the fitting model of photosynthesis employed in this study (Bernacchi et al., 2001; Sharkey et al., 2007), our results indicated that waterlogging impacted the Vcmax more in “Straight 8” when compared to “Marketmore” (33% vs. 14%). Similar results were reported by Ma and Guo (2014) in waterlogging-sensitive cucumber cultivar, and they attributed the significant reduction of Vcmax under waterlogging to chlorophyll degradation. Although we did not measure changes in chlorophyll content in the present study, there is increasing evidence that decreases in A, Vcmax, and Jmax are often associated with the degradation of chlorophyll content in waterlogged plants (Ma and Guo, 2014; Barickman et al., 2019). Primarily, plants subjected to waterlogging stress often experience an increase in the activities of oxidase and chlorophyllase, which are responsible for the degradation of pigments (Muhammad et al., 2021). Jans et al. (2012) suggest that the degradation of plant pigments during waterlogging can be alleviated by reducing the light level capture in a controlled environment. Thus, the downregulation of light utilization efficiency during photosynthesis in a wet environment might be a photoprotective mechanism of cucumber plants.
Moreover, the light response curves revealed that waterlogging declined A in cucumber plants, indicating that light utilization was suppressed under waterlogging. Besides the waterlogging stress, for efficient gas exchanges between cucumber leaves and the environment, the optimum PPFD for both cultivars, irrespective of the origin, was 1,500 μmol m–2 s–1 under the control conditions. However, above the optimum PPFD as depicted in Figure 8, revealed A photoinhibition, signifying that an increased PPFD may further hamper cucumber plants’ growth and developmental processes under waterlogging conditions (Wimalasekera, 2019).
Alterations in chlorophyll fluorescence parameters of waterlogged cucumber plants are an adaptive response to waterlogging stress, notwithstanding the photosynthetic apparatus damage. Most of the chlorophyll fluorescence parameters measured in light- and dark-adapted cucumber leaves were significantly reduced under 10-day waterlogging stress, indicating the sensitivity of cucumber plants to hypoxia stress. Previous studies demonstrated waterlogging causes a significant decline in chlorophyll fluorescence parameters, including Fo, and Fm, which reflect the PSII electron transport capacity in dark-adapted leaves (Smethurst and Shabala, 2003; Rao et al., 2021; Olorunwa et al., 2022). However, in the current study, although Fo decreased, Fm did not change significantly after 10 DOW in cucumber plants. The fact that Fm did not change significantly in response to waterlogging stress could be attributed to the fact that the degree of water stress was not severe enough to severely damage the leaves or alter metabolic pathways in cucumber plants. Moreover, Krause and Weis (1991) noted that healthy leaves had Fv/Fm values between 0.75 and 0.83, and a drop in these values indicated impaired PSII. However, the results of this study showed that the Fv/Fm of both cucumber genotypes ranged from 0.75 to 0.79 under both control and waterlogging treatments. Our current results are consistent with previous studies (Velasco et al., 2019; Lin et al., 2022), indicating that the alterations in leaf PSII at the seedling stage of cucumber were minimal under waterlogging conditions.
Furthermore, we found that the qP of cucumber plants (representing the proportion of excitation energy dissipated at the expense of photochemical utilization) was significantly reduced under waterlogging stress. Corresponding results have been reported by He et al. (2018) in cucumber plants and Zheng et al. (2021) in watermelon at the seedling stage. These studies attributed the decline in qP to either a slower rate of consumption of reductant and ATP produced by non-cyclic electron transport compared to the rate of excitation of open PSII or damage to PSII reaction centers (Urban et al., 2008). Taken together, the evidence from the current and previous studies further supports the fact that waterlogging leads to a decrease in photorespiration and corresponding damage to the PSII reaction centers of sensitive plants, including cucurbits. Conversely, the NPQ of cucumber plants revealed that the excess excitation energy not used during photochemistry increased in response to waterlogging conditions. Increased NPQ is considered a protective mechanism of the photosynthetic apparatus (Demmig-Adams and Adams, 2018). This result is consistent with previous reports (Ma and Guo, 2014; Bhatt et al., 2015; He et al., 2018) that cucurbit and other fruit crops have increased NPQ and decreased ETR under waterlogged conditions.
According to Kramer et al. (2004), the energy absorbed in PSII is divided into three partitions, including ΦPSII, ΦNPQ, and ΦNO. The current study revealed a decline in ΦPSII, and increments in ΦNPQ, and ΦNO in cucumber plants under waterlogging, which corroborates with previous studies (Zhang et al., 2019; Zheng et al., 2021). Since the ΦPSII is a product of qP and Fv′/Fm′ (Genty et al., 1989), the decrease in ΦPSII under waterlogging may be due to qP and Fv′/Fm′ reduction. Moreover, the correlation analysis revealed a positive relationship between ΦPSII, qP, and Fv′/Fm′. Increases in ΦNO in response to waterlogging indicate the lack of photochemical energy conversion and photoprotective regulatory mechanisms, and the excess electron can be used to form singlet oxygen in the chloroplast (Zheng et al., 2021). In both cultivars, an increase in the 1-qL was also demonstrated in response to waterlogging, suggesting that a more significant proportion of the PSII reaction centers were closed during actinic irradiance, thus resulting in a decline in ΦPSII, as denoted by Kramer et al. (2004). The alterations in the qL under waterlogging further indicate that the greater percentage of the energy absorbed in PSII was converted into chemically fixed energy whereas the remaining quanta were dissipated into heat and fluorescence.
Conclusion
Using morphological and physiological processes, we explored the response of cucumber cultivars to waterlogging stress. The light- and carbon-dependent reactions of photosynthesis were shown to be distinct at the photosynthesis level, with the light-dependent reactions increasing, whereas carbon assimilation was considerably impeded. Decreased stomatal conductance, increased CO2 concentration, down-regulated Rubisco carboxylation and regeneration, and absorbed energy dissipated in the form of heat were the main factors that reduced carbon assimilation rate in cucumber plants under waterlogging stress. In addition, the results of this study demonstrated that waterlogging impairs the photoreactions of photosynthesis of cucumber cultivars and that damage could be exacerbated with increasing light intensity.
Overall, our results support the hypothesis that cucumber plants are sensitive to waterlogged conditions, and most effects of waterlogging stress are similar in the two commercial cultivars we analyzed. Notably, the “Marketmore” cultivar appeared more tolerant to waterlogging stress with respect to morphological performance. These observations suggest that cucumber plants can be adapted to waterlogging conditions since they rapidly generate ARs to facilitate gas diffusion and increase plant survival when oxygen concentration is low. However, the newly developed ARs might not contribute to the waterlogging tolerance of cucumbers due to waterlogging-caused inhibition in the carbon metabolism, and PSII reaction centers.
Data availability statement
The raw data supporting the conclusions of this article will be made available by the authors, without undue reservation.
Author contributions
OO, BA, SB, and TB designed the experiments. SB collected physiological and morphological data. OO and BA did the statistical analysis using SAS. OO wrote the first draft of the manuscript. SP, GP, and TB made significant comments and inputs to successive versions of the manuscript. All authors contributed to the article and approved the submitted version.
Funding
This research was based on work that was supported by the USDA-NIFA Hatch Project (accession no. 149210).
Conflict of interest
The authors declare that the research was conducted in the absence of any commercial or financial relationships that could be construed as a potential conflict of interest.
Publisher’s note
All claims expressed in this article are solely those of the authors and do not necessarily represent those of their affiliated organizations, or those of the publisher, the editors and the reviewers. Any product that may be evaluated in this article, or claim that may be made by its manufacturer, is not guaranteed or endorsed by the publisher.
Acknowledgments
We thank Thomas Horgan for his research assistance during data collection at the North Mississippi Research and Extension Center.
Supplementary material
The Supplementary Material for this article can be found online at: https://www.frontiersin.org/articles/10.3389/fpls.2022.896244/full#supplementary-material
References
Abe, M., Yokota, K., Kurashima, A., and Maegawa, M. (2009). High water temperature tolerance in photosynthetic activity of Zostera japonica Ascherson & Graebner seedlings from Ago Bay, Mie Prefecture, central Japan. Fish. Sci. 75, 1117–1123. doi: 10.1007/s12562-009-0141-x
Ahmed, S., Nawata, E., and Sakuratani, T. (2002). Effects of waterlogging at vegetative and reproductive growth stages on photosynthesis, leaf water potential and yield in mungbean. Plant Prod. Sci. 5, 117–123. doi: 10.1626/pps.5.117
Arduini, I., Baldanzi, M., and Pampana, S. (2019). Reduced growth and nitrogen uptake during waterlogging at tillering permanently affect yield components in late sown oats. Front. Plant Sci. 10:1087. doi: 10.3389/fpls.2019.01087
Bai, T., Li, C., Li, C., Liang, D., and Ma, F. (2013). Contrasting hypoxia tolerance and adaptation in Malus species is linked to differences in stomatal behavior and photosynthesis. Physiol. Plant 147, 514–523. doi: 10.1111/j.1399-3054.2012.01683.x
Bansal, R., and Srivastava, J. P. (2015). Effect of waterlogging on photosynthetic and biochemical parameters in pigeonpea. Russ. J. Plant Physiol. 62, 322–327. doi: 10.1134/S1021443715030036
Barickman, T. C., Simpson, C. R., and Sams, C. E. (2019). Waterlogging causes early modification in the physiological performance, carotenoids, chlorophylls, proline, and soluble sugars of cucumber plants. Plants 8:160. doi: 10.3390/plants8060160
Bernacchi, C. J., Singsaas, E. L., Pimentel, C., Portis, A. R. Jr., and Long, S. P. (2001). Improved temperature response functions for models of rubisco-limited photosynthesis. Plant Cell Environ. 24, 253–259. doi: 10.1111/j.1365-3040.2001.00668.x
Bhatt, R. M., Upreti, K. K., Divya, M. H., Bhat, S., Pavithra, C. B., and Sadashiva, A. T. (2015). Interspecific grafting to enhance physiological resilience to flooding stress in tomato (Solanum lycopersicum L.). Sci. Hortic. 182, 8–17. doi: 10.1016/j.scienta.2014.10.043
Demmig-Adams, B., and Adams, W. W. (2018). An integrative approach to photoinhibition and photoprotection of photosynthesis. Environ. Exp. Bot. 154, 1–3. doi: 10.1016/j.envexpbot.2018.05.006
FAO (2021). FAOSTAT Database. Available online at: https://www.fao.org/faostat/en/#data/QCL (accessed November 2, 2021).
Farquhar, G. D., and Sharkey, T. D. (1982). Stomatal conductance and photosynthesis. Annu. Rev. Plant. Physiol. 33, 317–345. doi: 10.1146/annurev.pp.33.060182.001533
Farquhar, G. D., von Caemmerer, S., and Berry, J. A. (1980). A biochemical model of photosynthetic CO2 assimilation in leaves of C3 species. Planta 149, 78–90. doi: 10.1007/BF00386231
Flexas, J., Carriquí, M., Coopman, R. E., Gago, J., Galmés, J., Martorell, S., et al. (2014). Stomatal and mesophyll conductances to CO2 in different plant groups: underrated factors for predicting leaf photosynthesis responses to climate change? Plant Sci. 226, 41–48. doi: 10.1016/j.plantsci.2014.06.011
Fukao, T., and Bailey-Serres, J. (2004). Plant responses to hypoxia – is survival a balancing act? Trends Plant Sci. 9, 449–456. doi: 10.1016/j.tplants.2004.07.005
Genty, B., Briantais, J.-M., and Baker, N. R. (1989). The relationship between the quantum yield of photosynthetic electron transport and quenching of chlorophyll fluorescence. Biochim. Biophys. Acta 990, 87–92. doi: 10.1016/S0304-4165(89)80016-9
Gibbs, J., and Greenway, H. (2003). Review: mechanisms of anoxia tolerance in plants, I. Growth, survival and anaerobic catabolism. Funct. Plant Biol. 30, 1–47. doi: 10.1071/PP98095
Guidi, L., Lo Piccolo, E., and Landi, M. (2019). Chlorophyll fluorescence, photoinhibition and abiotic stress: Does it make any difference the fact to be a C3 or C4 species? Front. Plant Sci. 10:174. doi: 10.3389/fpls.2019.00174
Hasanuzzaman, M., Al Mahmud, J., Nahar, K., Anee, T. I., Inafuku, M., Oku, H., et al. (2017). “Responses, adaptation, and ROS metabolism in plants exposed to waterlogging stress,” in Reactive Oxygen Species and Antioxidant Systems in Plants: Role and Regulation under Abiotic Stress, eds M. I. R. Khan and N. A. Khan (Singapore: Springer Singapore), 257–281. doi: 10.1007/978-981-10-5254-5_10
He, L., Yu, L., Li, B., Du, N., and Guo, S. (2018). The effect of exogenous calcium on cucumber fruit quality, photosynthesis, chlorophyll fluorescence, and fast chlorophyll fluorescence during the fruiting period under hypoxic stress. BMC Plant Biol. 18:180. doi: 10.1186/s12870-018-1393-3
Herzog, M., Striker, G. G., Colmer, T. D., and Pedersen, O. (2016). Mechanisms of waterlogging tolerance in wheat – a review of root and shoot physiology. Plant Cell Environ. 39, 1068–1086. doi: 10.1111/pce.12676
Huang, B., NeSmith, D. S., Bridges, D. C., and Johnson, J. W. (1995). Responses of squash to salinity, waterlogging, and subsequent drainage: II, Root and shoot growth. J. Plant. Nutr. 18, 141–152. doi: 10.1080/01904169509364891
IPCC (2021). Climate Change 2021: The Physical Science Basis. Contribution of Working Group I to the Sixth Assessment Report of the Intergovernmental Panel on Climate Change. Cambridge: Cambridge University Press, doi: 10.1017/9781009157896
Issarakraisila, M., Ma, Q., and Turner, D. W. (2007). Photosynthetic and growth responses of juvenile Chinese kale (Brassica oleracea var. alboglabra) and Caisin (Brassica rapa subsp. parachinensis) to waterlogging and water deficit. Sci. Hortic. 111, 107–113. doi: 10.1016/j.scienta.2006.10.017
Jans, W. W. P., Dibor, L., Verwer, C., Kruijt, B., Tan, S., and Van Der Meer, P. J. (2012). Effects of light and soil flooding on the growth and photosynthesis of ramin (Gonystylus bancanus) seedlings in Malaysia. J. Trop. For. Sci. 54–63.
Kang, Y.-Y., Guo, S.-R., Li, J., and Duan, J.-J. (2009). Effect of root applied 24-epibrassinolide on carbohydrate status and fermentative enzyme activities in cucumber (Cucumis sativus L.) seedlings under hypoxia. Plant Growth Regul. 57, 259–269. doi: 10.1007/s10725-008-9344-x
Kozlowski, T. T. (1984). Plant Responses to Flooding of Soil. BioScience 34, 162–167. doi: 10.2307/1309751
Kramer, D. M., Johnson, G., Kiirats, O., and Edwards, G. E. (2004). New fluorescence parameters for the determination of QA redox state and excitation energy fluxes. Photosyn. Res. 79:209. doi: 10.1023/B:PRES.0000015391.99477.0d
Krause, G. H., and Weis, E. (1991). Chlorophyll Fluorescence and Photosynthesis: the Basics. Annu. Rev. Plant. Physiol. Plant. Mol. Biol. 42, 313–349. doi: 10.1146/annurev.pp.42.060191.001525
Leigh, A., Sevanto, S., Close, J. D., and Nicotra, A. B. (2017). The influence of leaf size and shape on leaf thermal dynamics: Does theory hold up under natural conditions? Plant Cell Environ. 40, 237–248. doi: 10.1111/pce.12857
Lin, H.-H., Lin, K.-H., Huang, M.-Y., and Su, Y.-R. (2020). Use of non-destructive measurements to identify cucurbit species (Cucurbita maxima and Cucurbita moschata) tolerant to waterlogged conditions. Plants 9:1226. doi: 10.3390/plants9091226
Lin, S.-Y., Chen, P.-A., and Zhuang, B.-W. (2022). The stomatal conductance and Fv/Fm as the indicators of stress tolerance of avocado seedlings under short-term waterlogging. Agronomy 12:1084. doi: 10.3390/agronomy12051084
Liu, Z., Cheng, R., Xiao, W., Guo, Q., and Wang, N. (2014). Effect of off-season flooding on growth, photosynthesis, carbohydrate partitioning, and nutrient uptake in Distylium chinense. PLoS One 9:e107636. doi: 10.1371/journal.pone.0107636
Lobo, F. A., de Barros, M. P., Dalmagro, H. J., Dalmolin, ÂC., Pereira, W. E., de Souza, ÉC., et al. (2013). Fitting net photosynthetic light-response curves with Microsoft Excel — a critical look at the models. Photosynthetica 51, 445–456. doi: 10.1007/s11099-013-0045-y
Ma, Y. H., and Guo, S. R. (2014). 24-epibrassinolide improves cucumber photosynthesis under hypoxia by increasing CO2 assimilation and photosystem II efficiency. Photosynthetica 52, 96–104. doi: 10.1007/s11099-014-0010-4
Martin, B., and Ruiz-Torres, N. A. (1992). Effects of water-deficit stress on photosynthesis, its components and component limitations, and on water use efficiency in wheat (Triticum aestivum L.). Plant Physiol. 100:733. doi: 10.1104/pp.100.2.733
Maxwell, K., and Johnson, G. N. (2000). Chlorophyll fluorescence—a practical guide. J. Exp. Bot. 51, 659–668. doi: 10.1093/jexbot/51.345.659
Meena, K. C., Rao, S., Gontia, A. S., Upadhyaya, A., and Rao, S. K. (2015). Physiological performance of pigeon pea [Cajanus cajan (L.) Millsp.] genotypes after recovery from waterlogging. Ind. J. Plant Physiol. 20, 162–166. doi: 10.1007/s40502-015-0146-7
Muhammad, I., Shalmani, A., Ali, M., Yang, Q.-H., Ahmad, H., and Li, F. B. (2021). Mechanisms regulating the dynamics of photosynthesis under abiotic stresses. Front. Plant Sci. 11:615942. doi: 10.3389/fpls.2020.615942
Olorunwa, O. J., Adhikari, B., Shi, A., and Barickman, T. C. (2022). Screening of cowpea (Vigna unguiculata (L.) Walp.) genotypes for waterlogging tolerance using morpho-physiological traits at early growth stage. Plant Sci. 315:111136. doi: 10.1016/j.plantsci.2021.111136
Olorunwa, O. J., Shi, A., and Barickman, T. C. (2021). Varying drought stress induces morpho-physiological changes in cowpea (Vigna unguiculata (L.) genotypes inoculated with Bradyrhizobium japonicum. Plant Stress 2:100033. doi: 10.1016/j.stress.2021.100033
Pampana, S., Masoni, A., and Arduini, I. (2016). Response of cool-season grain legumes to waterlogging at flowering. Can. J. Plant Sci. 96, 597–603. doi: 10.1139/cjps-2015-0268
Pan, J., Sharif, R., Xu, X., and Chen, X. (2021). Mechanisms of waterlogging tolerance in plants: research progress and prospects. Front. Plant Sci. 11:2319. doi: 10.3389/fpls.2020.627331
Parent, C., Capelli, N., Berger, A., Crèvecoeur, M., and Dat, J. F. (2008). An overview of plant responses to soil waterlogging. Plant Stress 2, 20–27.
Pedersen, O., Colmer, T. D., and Sand-Jensen, K. (2013). Underwater photosynthesis of submerged plants – recent advances and methods. Front. Plant Sci. 4:140. doi: 10.3389/fpls.2013.00140
Ploschuk, R. A., Miralles, D. J., Colmer, T. D., Ploschuk, E. L., and Striker, G. G. (2018). Waterlogging of winter crops at early and late stages: impacts on leaf physiology, growth and yield. Front. Plant Sci. 9:1863. doi: 10.3389/fpls.2018.01863
Pompeiano, A., Huarancca Reyes, T., Moles, T. M., Guglielminetti, L., and Scartazza, A. (2019). Photosynthetic and growth responses of Arundo donax L. plantlets under different oxygen deficiency stresses and reoxygenation. Front. Plant Sci. 10:408. doi: 10.3389/fpls.2019.00408
Prioul, J. L., and Chartier, P. (1977). Partitioning of transfer and carboxylation components of intracellular resistance to photosynthetic CO2 fixation: a critical analysis of the methods used. Ann. Bot. 41, 789–800. doi: 10.1093/oxfordjournals.aob.a085354
Qi, X., Li, Q., Ma, X., Qian, C., Wang, H., Ren, N., et al. (2019). Waterlogging-induced adventitious root formation in cucumber is regulated by ethylene and auxin through reactive oxygen species signalling. Plant Cell Environ. 42, 1458–1470. doi: 10.1111/pce.13504
Qi, X., Li, Q., Shen, J., Qian, C., Xu, X., Xu, Q., et al. (2020). Sugar enhances waterlogging-induced adventitious root formation in cucumber by promoting auxin transport and signalling. Plant Cell Environ. 43, 1545–1557. doi: 10.1111/pce.13738
Rao, L., Li, S., and Cui, X. (2021). Leaf morphology and chlorophyll fluorescence characteristics of mulberry seedlings under waterlogging stress. Sci. Rep. 11:13379. doi: 10.1038/s41598-021-92782-z
Sairam, R. K., Kumutha, D., Ezhilmathi, K., Chinnusamy, V., and Meena, R. C. (2009). Waterlogging induced oxidative stress and antioxidant enzyme activities in pigeon pea. Biol. Plant 53, 493–504. doi: 10.1007/s10535-009-0090-3
schildwacht, P. M. (1989). Is a decreased water potential after withholding oxygen to roots the cause of the decline of leaf-elongation rates in Zea mays L. and Phaseolus vulgaris L.? Planta 177, 178–184. doi: 10.1007/BF00392806
Shabala, S., Shabala, L., Barcelo, J., and Poschenrieder, C. (2014). Membrane transporters mediating root signalling and adaptive responses to oxygen deprivation and soil flooding. Plant Cell Environ. 37, 2216–2233. doi: 10.1111/pce.12339
Shao, G. C., Lan, J. J., Yu, S. E., Liu, N., Guo, R. Q., and She, D. L. (2013). Photosynthesis and growth of winter wheat in response to waterlogging at different growth stages. Photosynthetica 51, 429–437. doi: 10.1007/s11099-013-0039-9
Sharkey, T. D., Bernacchi, C. J., Farquhar, G. D., and Singsaas, E. L. (2007). Fitting photosynthetic carbon dioxide response curves for C3 leaves. Plant Cell Environ. 30, 1035–1040. doi: 10.1111/j.1365-3040.2007.01710.x
Smethurst, C. F., and Shabala, S. (2003). Screening methods for waterlogging tolerance in lucerne: comparative analysis of waterlogging effects on chlorophyll fluorescence, photosynthesis, biomass and chlorophyll content. Funct. Plant Biol. 30:335. doi: 10.1071/FP02192
Sousa, C. A. F., and Sodek, L. (2002). The metabolic response of plants to oxygen deficiency. Braz. J. Plant Physiol. 14, 83–94. doi: 10.1590/S1677-04202002000200002
Tan, X., Xu, H., Khan, S., Equiza, M. A., Lee, S. H., Vaziriyeganeh, M., et al. (2018). Plant water transport and aquaporins in oxygen-deprived environments. J. Plant Physiol. 227, 20–30. doi: 10.1016/j.jplph.2018.05.003
Tournaire-Roux, C., Sutka, M., Javot, H., Gout, E., Gerbeau, P., Luu, D.-T., et al. (2003). Cytosolic pH regulates root water transport during anoxic stress through gating of aquaporins. Nature 425, 393–397. doi: 10.1038/nature01853
Urban, L., Jegouzo, L., Damour, G., Vandame, M., and François, C. (2008). Interpreting the decrease in leaf photosynthesis during flowering in mango. Tree Physiol. 28, 1025–1036. doi: 10.1093/treephys/28.7.1025
Velasco, N. F., Ligarreto, G. A., Díaz, H. R., and Fonseca, L. P. M. (2019). Photosynthetic responses and tolerance to root-zone hypoxia stress of five bean cultivars (Phaseolus vulgaris L.). S. Afr. J. Bot. 123, 200–207. doi: 10.1016/j.sajb.2019.02.010
Vidoz, M. L., Loreti, E., Mensuali, A., Alpi, A., and Perata, P. (2010). Hormonal interplay during adventitious root formation in flooded tomato plants. Plant J. 63, 551–562. doi: 10.1111/j.1365-313X.2010.04262.x
Visser, E. J., Cohen, J. D., Barendse, G. W., Blom, C. W., and Voesenek, L. A. (1996). An ethylene-mediated increase in sensitivity to auxin induces adventitious root formation in flooded Rumex palustris Sm. Plant Physiol. 112, 1687–1692. doi: 10.1104/pp.112.4.1687
Voesenek, L. A. C. J., and Bailey-Serres, J. (2015). Flood adaptive traits and processes: an overview. New Phytol. 206, 57–73. doi: 10.1111/nph.13209
Voesenek, L. A. C. J., and Sasidharan, R. (2013). Ethylene – and oxygen signalling – drive plant survival during flooding. Plant Biol. 15, 426–435. doi: 10.1111/plb.12014
Wimalasekera, R. (2019). Effect of light intensity on photosynthesis. Photosynth. Prod. Environ. Stress 65–73.
Xu, X., Ji, J., Ma, X., Xu, Q., Qi, X., and Chen, X. (2016). Comparative proteomic analysis provides insight into the key proteins involved in cucumber (Cucumis sativus L.) adventitious root emergence under waterlogging stress. Front. Plant Sci. 7:1515. doi: 10.3389/fpls.2016.01515
Xuewen, X., Huihui, W., Xiaohua, Q., Qiang, X., and Xuehao, C. (2014). Waterlogging-induced increase in fermentation and related gene expression in the root of cucumber (Cucumis sativus L.). Sci. Hortic. 179, 388–395. doi: 10.1016/j.scienta.2014.10.001
Yetisir, H., Çaliskan, M. E., Soylu, S., and Sakar, M. (2006). Some physiological and growth responses of watermelon [Citrullus lanatus (Thunb.) Matsum. and Nakai] grafted onto Lagenaria siceraria to flooding. Environ. Exp. Bot. 58, 1–8. doi: 10.1016/j.envexpbot.2005.06.010
Yordanova, R. Y., and Popova, L. P. (2007). Flooding-induced changes in photosynthesis and oxidative status in maize plants. Acta Physiol. Plant 29, 535–541. doi: 10.1007/s11738-007-0064-z
Zhang, R. D., Zhou, Y. F., Yue, Z. X., Chen, X. F., Cao, X., Xu, X. X., et al. (2019). Changes in photosynthesis, chloroplast ultrastructure, and antioxidant metabolism in leaves of sorghum under waterlogging stress. Photosynthetica 57, 1076–1083. doi: 10.32615/ps.2019.124
Keywords: leaf gas exchange, photosynthetic quantum efficiency, infrared gas analysis, hypoxia, chlorophyll fluorescence
Citation: Olorunwa OJ, Adhikari B, Brazel S, Popescu SC, Popescu GV and Barickman TC (2022) Short waterlogging events differently affect morphology and photosynthesis of two cucumber (Cucumis sativus L.) cultivars. Front. Plant Sci. 13:896244. doi: 10.3389/fpls.2022.896244
Received: 14 March 2022; Accepted: 28 June 2022;
Published: 22 July 2022.
Edited by:
Silvia Pampana, University of Pisa, ItalyReviewed by:
Elisa Pellegrini, University of Pisa, ItalyMarco Landi, University of Pisa, Italy
Lorenzo Guglielminetti, University of Pisa, Italy
Fernanda Fidalgo, University of Porto, Portugal
Copyright © 2022 Olorunwa, Adhikari, Brazel, Popescu, Popescu and Barickman. This is an open-access article distributed under the terms of the Creative Commons Attribution License (CC BY). The use, distribution or reproduction in other forums is permitted, provided the original author(s) and the copyright owner(s) are credited and that the original publication in this journal is cited, in accordance with accepted academic practice. No use, distribution or reproduction is permitted which does not comply with these terms.
*Correspondence: T. Casey Barickman, dC5jLmJhcmlja21hbkBtc3N0YXRlLmVkdQ==