- 1State Key Laboratory of Water Resources and Hydropower Engineering Science, Wuhan University, Wuhan, China
- 2State Key Laboratory of Hybrid Rice, Institute for Advanced Studies, Wuhan University, Wuhan, China
With the increasing shortage of land resources and people’s attention to the ecological environment, the application of microbial fertilizer with natural soil microorganisms as the main component has attracted increasing attention in saline agriculture. In this study, two salt-tolerant strains, YL07 (Bacillus atrophaeus) and YL10 (Planococcus soli), were isolated from maize (Zea mays L.) rhizosphere soil with a saturated conductivity (ECe) of 6.13 dS/m and pH of 8.32 (Xinjiang, China). The effects of B. atrophaeus WZYH01 (YL07) and Planococcus soli WZYH02 (YL10) on the growth and development of maize (Zea mays L.) under salt stress (ECe = 5.9 dS/m) were further studied. The results showed that compared with uninoculation, inoculation with B. atrophaeus WZYH01 and Planococcus soli WZYH02 significantly improved maize growth performance, biomass yield, and antioxidant levels under salt stress, and the effect of Planococcus soli WZYH02 was more prominent than the effect of B. atrophaeus WZYH01. Moreover, inoculation with B. atrophaeus WZYH01 and Planococcus soli WZYH02 protected maize from salt stress by regulating plant hormone [IAA and abscisic acid (ABA)] levels and increasing nutrient acquisition. In addition, the tested strains were most efficient for maize growth and health, increasing the content of K+ accompanied by an effective decrease in Na+ in maize tissues. The transcription levels of salt tolerance genes (ZMNHX1, ZMNHX2, ZMHKT, ZMWRKY58, and ZMDREB2A) in inoculated maize were also dramatically higher than the transcription levels of the specified salt tolerance genes in uninoculated maize. In conclusion, B. atrophaeus WZYH01 and Planococcus soli WZYH02 can alleviate the harmful effects of salt stress on crop growth, thereby promoting sustainable agricultural development.
Introduction
Currently, approximately 1,125 million hectares of land worldwide are affected by salinization (Wicke et al., 2011; Dong et al., 2022). In China, approximately 3.67 million hectares of soil, which represents 4.88% of the total available land across the country, is threatened by salt (Zeng et al., 2016; Liu et al., 2020). To increase crop yield in salinized land, a large amount of chemical fertilizer is applied to the soil (Wang L. L. et al., 2018), but the increase in chemical input is not directly proportional to the increase in crop yield (Hawkesford, 2014). Meanwhile, this behavior has also brought serious soil ecological and environmental problems such as secondary salinization, soil consolidation, soil acidification, and microecological imbalance (Hawkesford, 2014; Ma et al., 2021). In addition, the Ministry of Agriculture and Rural Affairs of the People’s Republic of China (2015)1 stated that the use of chemical fertilizer should be greatly reduced and that resource-saving and environmentally friendly modern agricultural development roads should be actively explored. Therefore, it is imperative to reduce the use of chemical fertilizer in agricultural production in saline land.
To overcome this issue, a new biocontrol approach has been developed to protect plants from salt stress in soil by utilizing beneficial microorganisms to achieve eco-friendly sustainable agriculture (Etesami and Maheshwari, 2018; Moreira et al., 2019). Plant growth-promoting rhizobacteria (PGPR) are an important microbial community that has a beneficial impact on plant growth and development. PGPR extensively colonizes plant roots, increases their growth, and reduces plant diseases (Shameer and Prasad, 2018). When combined with roots and other tissues, PGPR improves the nutritional supply of crop plants through several mechanisms. PGPR has direct effects, including nitrogen fixation, phosphorus solubilization, production of NH3, indole acetic acid (IAA), and siderophores, and indirect effects, including antioxidant defense, volatile organic compounds (VOCs), exopolysaccharides (EPS), and osmotic balance mechanisms for improving plant growth and enhancing tolerance against salt stress (Moreira et al., 2016; Abbas et al., 2019; Kumar et al., 2019). Therefore, for decades, people have been studying how soil microorganisms play a role in plant growth to provide an alternative way to reduce the amount of fertilizer.
The application of PGPR to alleviate salinity-induced plant stress has become a promising approach. PGPR-mediated tolerance has been demonstrated in different plant–microbe interactions (Goswami et al., 2014; Li et al., 2020; Tirry et al., 2021). However, the efficiency of PGPR is affected by environmental factors such as climate, weather conditions, soil characteristics (e.g., texture, pH, temperature, and water content), and interaction with soil indigenous microbial flora (Siddikee et al., 2010; Qiu et al., 2019; Khalilpour et al., 2021). van Elsas et al. (1986) also reported that the numbers of Bacillus subtilis decreased rapidly in loamy sand and silt loam, while Pseudomonas fluorescens survived better in silty sand than in loamy loam. In addition, Habibi et al. (2019) found that PGPR isolated from Bala Doshi rice cultivars could promote Bala Doshi rice plant growth more than other rice cultivars, which might be due to the host specificity of Bala Doshi rice cultivars to the obtained strains. Simultaneously, recent studies have increasingly emphasized the benefits of using local microorganisms to enhance plant resistance to biological and abiotic stresses (Marulanda et al., 2009; Banerjee et al., 2017), suggesting that the activities of strains already adapted to the plant environment may increase the chances of inoculum survival and confer a positive effect on plant development under stress (Qiu et al., 2019). Therefore, we suggest screening of PGPR suitable for local soil and climate conditions.
Xinjiang is one of the most important agricultural production areas in the arid and semiarid regions of China (Wang et al., 2012), but 31.1% of the existing arable land in Xinjiang has suffered soil salinization (Liang et al., 2021; Liu Y. et al., 2021). The Statistical Yearbook of Xinjiang (2015) has shown that the annual yields of these three crops (grain, vegetables, and fruits) are approximately 1.50 × 107, 1.93 × 107, and 0.96 × 107 tons, respectively (Liu W. G. et al., 2021). At present, many studies on screening indigenous PGPR have also been carried out in these crops. Han et al. (2021) indicated that Pseudomonas SCPG-7 inoculated from pepper (Capsicum annuum L.) seeds had the ability to promote plant growth by secreting organic acids, alkaline phosphatase, siderophore, and IAA under salt stress in Shihezi, Xinjiang Province. The bacterial strain Klebsiella oxytoca Rs-5 was screened from the salinized soil of cotton in Xinjiang Province and could relieve salt stress and promote cotton seedling growth (Yue et al., 2007). Pseudomonas putida Rs-198 isolated from a cotton alkaline soil in Xinjiang mitigated osmotic stress in cotton seedlings, which led to an improved germination rate, healthy stands, and growth parameters (Yao et al., 2010). Liu et al. (2011) also reported that Bacillus subtilis SL-13 obtained from tomato field soil in Xinjiang Province was proven to promote sprouting and seedling growth in tomatoes. However, in the saline soils of Xinjiang, relatively few studies have been carried out on the screening of maize rhizosphere growth-promoting bacteria, which requires attention from the scientific community.
Compared with PGPR isolated from other soils, PGPR isolated from plants grown under chronically stressful salinity conditions have a stronger ability to survive due to their adaptation to the local environment (Kumar et al., 2017). However, knowledge of the potential of native PGPR isolates and their effects in maize plant growth and physiological characteristics under salt stress is still gravely limited. Therefore, the purpose of this study was to screen indigenous stress-tolerant PGPR with growth-promoting traits and evaluate the effects of PGPR isolates on the growth, physiology, and expression levels of stress-tolerant genes in maize seedlings under salt stress.
Materials and Methods
Plant Material, Growing Conditions, and Treatments
Two strains (YL07 and YL10) were screened from maize rhizosphere soil in Yanqi, Xinjiang province, China (41°91’ N, 86°49’ E, elevation 1,061 m). The screening method is described in detail in the Supplementary Material. Maize seeds were treated with 75% ethanol for 30 s, sterilized with 10% sodium hypochlorite solution for 15 min, and then washed with sterile water 5–6 times. Sterilized seeds were soaked in sterile water for 12 h and then dipped in strain inoculum for 2 h. All the above experiments were carried out in a sterile environment. Thereafter, the treated maize seeds were cultivated in the seedling tray for 7 days, and then, maize seedlings with consistent growth were selected and transplanted into pots containing 1.2 kg sterilized soil (120°C, 30 min, 100 kPa, and sterilized twice) in each pot. Three maize plants were maintained in each pot. The three treatments were as follows: uninoculated strain exposed to 5.9 dS/m salt stress (CK); YL07 strain exposed to 5.9 dS/m salt (YL07); and YL10 strain exposed to 5.9 dS/m salt stress (YL10). Each treatment had five repetitions. The maize was harvested after 31 days of growth.
Growth, Biomass Yield, Physiological Indicators, and Plant Hormones
After 31 days of cultivation, the plants were collected, and the roots were rinsed with sterile water. Plant height was measured and recorded. To obtain plant dry biomass, the plants were dried at 105°C for 30 min and kept at 75 ± 2°C for 24 h to achieve a constant dry weight (DW). The leaf superoxide dismutase (SOD), catalase (CAT), peroxidase (POD), ascorbate peroxidase (APX), glutathione reductase (GR) and reduced glutathione (GSH), soluble sugar, proline, IAA, and abscisic acid (ABA) contents were measured by Qingdao Sci-tech Innovation Quality Testing Co., Ltd2.
Determination of N, K+, Na+, and K+/Na+
After 31 days of cultivation, the maize roots were washed and wiped dry to determine the content of nutrients and ions. Briefly, the maize leaves and roots were ground into fine powder, and then, 0.2 g samples were weighed into digestive tubes with 1 ml of distilled water. Then, 5 ml of H2SO4 was added to the mixture, and 2 ml of hydrogen peroxide was added twice. After the fierce reaction, the mixtures were put on the digestion furnace for digestion, and heating was stopped when the solution turned brown. After cooling slightly, 10 drops of H2O2 were added, heating was continued until the solution was colorless or clear, and heating continued for 5 min to remove excess H2O2. Nutrient nitrogen (N) was determined by the Kjeldahl method as indicated previously (Bremner, 2009). K+ and Na+ were determined by flame photometry according to Wolf (2008). The K+/Na+ ratio was calculated in line with the K+ and Na+ concentrations.
Transcription Analysis
The expression levels of related genes in maize seedlings inoculated with YL07, YL10, and the control treatment under salt stress were determined by quantitative real-time polymerase chain reaction (qRT-PCR). Total RNA was extracted from each maize seedling using TRIzol reagent following the manufacturer’s instructions. The transcription levels of the ion balance-related genes ZmNHX1 and ZmNHX2 and ZmHKT, key transcription factor genes linked to the plant response to abiotic stress ZmDREB2A and ZmWRKY58, and key genes associated with ABA synthesis ZmNCED were measured. The sequences of primers used in qRT-PCR to study the relative expression of genes in maize lines are presented in the Supplementary Table 1. All PCR experiments were performed with SYBR Green Master Mix on a CFX Connect Real-Time PCR Detection System (1855201) with 40 cycles, and an annealing temperature of 55°C was used (in a final volume of 20 μl). The constitutively expressed β-actin gene was used as an internal control. The relative expression levels of the target genes were calculated using the 2–ΔΔCt method. Five mRNA samples from five independent leaf samples (biological replicates) were analyzed.
Data and Statistical Analysis
All the data were statistically analyzed using RStudio (version 4.0.3). The significance of the differences between the control and treated groups was analyzed using one-way ANOVA followed by LSD post hoc comparison tests. Different letters indicate significant differences at the P < 0.05 level. Means and standard errors for all parameters were calculated from at least three replicates. Hierarchical clustering was performed using the “pheatmap” package based on Euclidean distance. Principal component analysis (PCA) was conducted using the “FactoMineR” package. All results were visualized using the “ggplot2” package in RStudio.
Results
Growth and Biomass Yield
In the pot experiments, we recorded the maize growth index, such as plant height and dry biomass, under salt stress. The results showed that the plant height (F = 1.93, P < 0.05) and dry weight (F = 6.33, P < 0.01) were increased significantly by inoculating strains YL07 and YL10 under salt stress conditions. Compared with control plants, the plant height of inoculated strains YL07 and YL10 increased by 12.48 and 21.71%, respectively (Figure 1A), and the dry weight increased by 12.56 and 21.67%, respectively (Figure 1B).
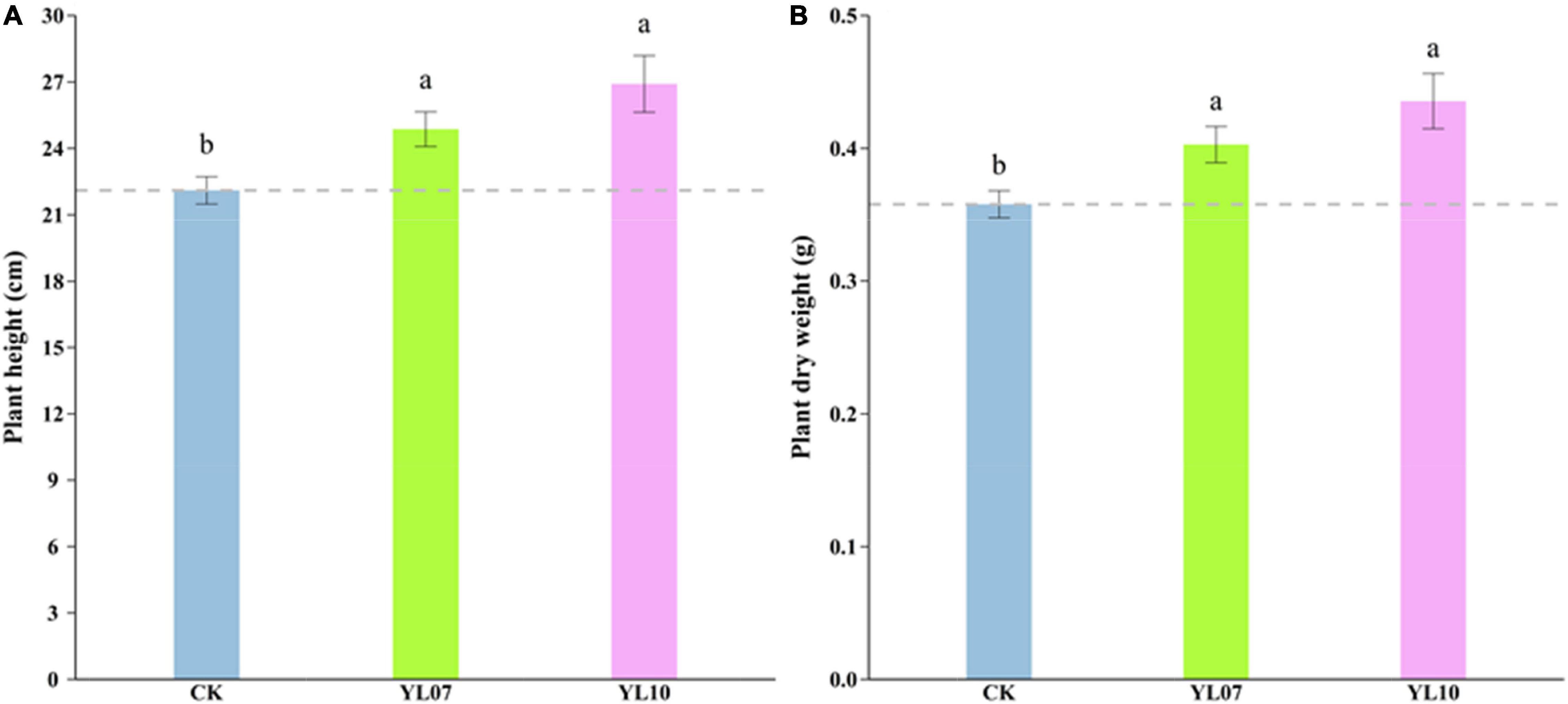
Figure 1. Effect of strains YL07 and YL10 on the plant height (A) and dry weight (B) of maize under NaCl stress. Different letters indicate significant differences at the P < 0.05 level among the different treatments, as determined by LSD post hoc comparison tests.
The Contents of N, K+, Na+, and K+/Na+
To determine the absorption of nutrients and main ions in inoculated YL07 and YL10, the contents of N, K+, and Na+ in maize seedlings were measured. As shown in Table 1, the contents of nutrient N and K+ in roots and shoots increased significantly (P < 0.05) in inoculated with YL07 and YL10, and the contents of shoots were higher than the contents of roots. Nevertheless, the content of Na+ in roots and shoots inoculated with YL07 and YL10 decreased significantly (P < 0.05) in comparison with the control treatment. These results showed that the inoculated YL07 and YL10 strains could promote the absorption of N and K+ and prevent the absorption of Na+. Moreover, the K+/Na+ ratio is an indicator of ion balance in maize plants. As shown in Table 1, after inoculating the YL07 and YL10 strains, the K+/Na+ ratio in maize roots and shoots increased significantly (P < 0.05) compared with the K+/Na+ ratio in the control treatment. This result further reveals that inoculated YL07 and YL10 strains could promote the absorption of K+ and inhibit the absorption of Na+ in maize plants.

Table 1. Effects of YL07 and YL10 strain inoculation on nutrient elements and essential ion absorption by maize seedlings grown in pots under NaCl stress.
Antioxidant Enzymes, GSH, Soluble Sugar, and Proline Content
Salt stress mainly triggers the production of reactive oxygen species (ROS), and a large amount of ROS will cause serious damage to plant cells. Antioxidant enzymes such as SOD, CAT, POD, APX, and GR can remove excess ROS to maintain normal plant physiological activities. As shown in Figure 2, the contents of SOD, CAT, POD, APX, and GR in leaves inoculated with the YL07 and YL10 strains dramatically increased compared to the control treatment, in which SOD, YL07 and YL10 increased by 11.13 and 10.42% (F = 96.08, P < 0.001), respectively; CAT, YL07 and YL10 increased by 17.88 and 21.49% (F = 279.60, P < 0.001); POD, YL07 and YL10 increased by 26.65 and 25.11% (F = 132.10, P < 0.001); APX, YL07 and YL10 increased by 18.07 and 22.10% (F = 153.10, P < 0.001); GR, YL07 and YL10 increased by 23.15 and 25.73% (F = 127.60, P < 0.001), respectively. Similarly, the GSH content of YL07 and YL10 was significantly enhanced by 13.31 and 20.94%, respectively (F = 223.80, P < 0.001), compared to the control treatment (Figure 2F). As shown in Figures 2G,H, soluble sugars and proline showed the same results. The inoculated YL07 and YL10 were also considerably increased compared to the control treatment, in which soluble sugar, YL07 and YL10 increased by 5.24 and 9.26%, respectively (F = 81.51, P < 0.001); proline, YL07 and YL10 increased by 3.07 and 6.71%, respectively (F = 11.96, P < 0.001).
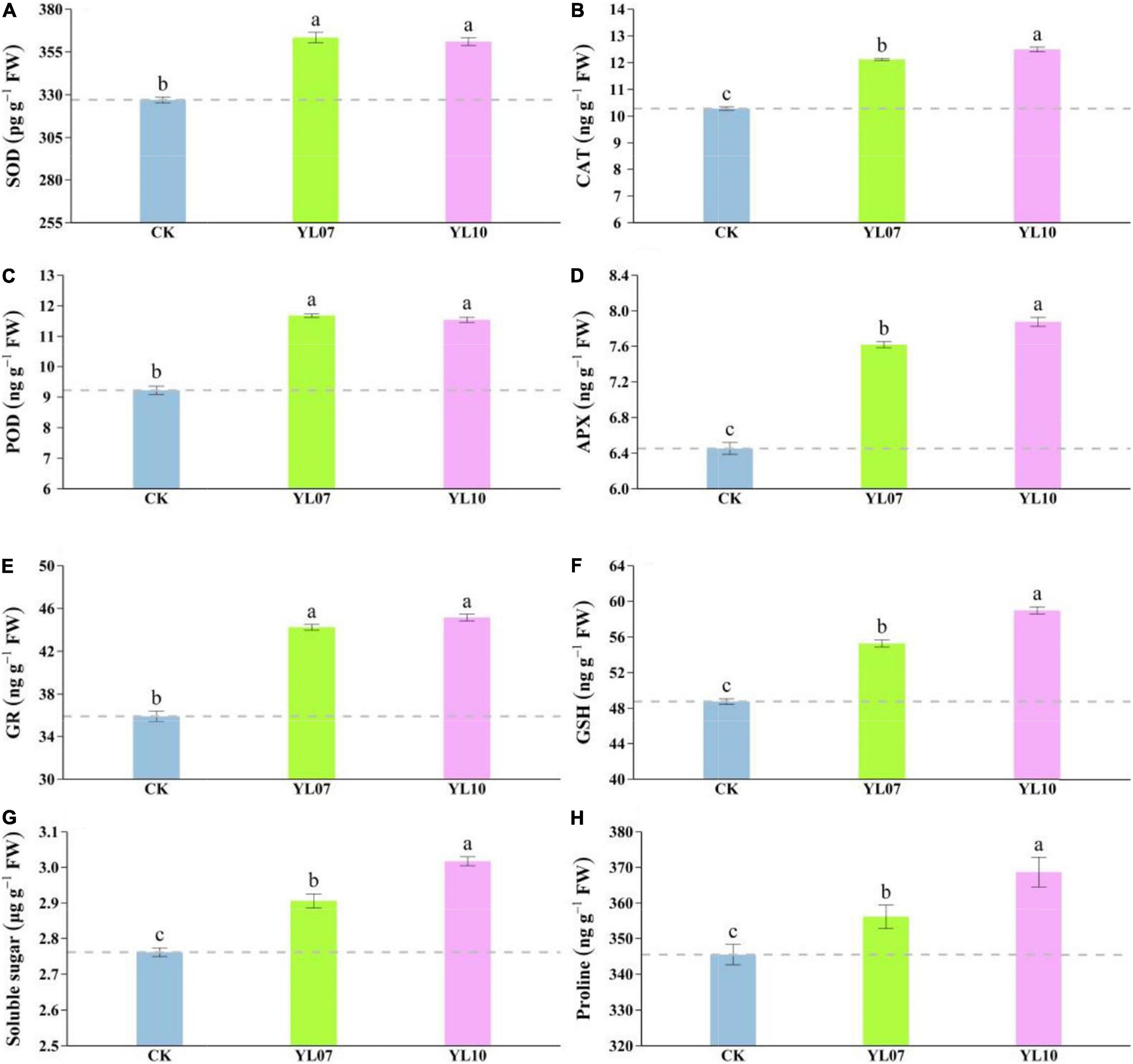
Figure 2. Effect of strains YL07 and YL10 on antioxidant enzymes, including SOD (A), CAT (B), POD (C), APX (D), and GR (E) activities of maize under NaCl stress. Effects of YL07 and YL10 inoculation on GSH (F), soluble sugar (G), and proline (H) contents of maize grown in pots for 31 days under NaCl stress. Different letters indicate significant differences at the P < 0.05 level among the different treatments, as determined by LSD post hoc comparison tests. The content of IAA and ABA in plants.
Under salt stress, the content of plant endogenous hormones regulates a series of physiological and biochemical reactions. As shown in Figure 3, the production of IAA was dramatically enhanced and the level of ABA was reduced in inoculated YL07 and YL10 under NaCl stress. Under inoculation with YL07 and YL10, the IAA content in the leaves of inoculated maize seedlings was significantly (F = 447.40, P < 0.001) increased by 18.46 and 22.96%, respectively, compared to the IAA content in the leaves of uninoculated control seedlings (Figure 3A). In contrast, the ABA content in maize leaves was significantly (F = 158.30, P < 0.001) decreased by 2.55 and 13.67%, respectively, under inoculation with the YL07 and YL10 treatments compared to the control treatments (Figure 3B).
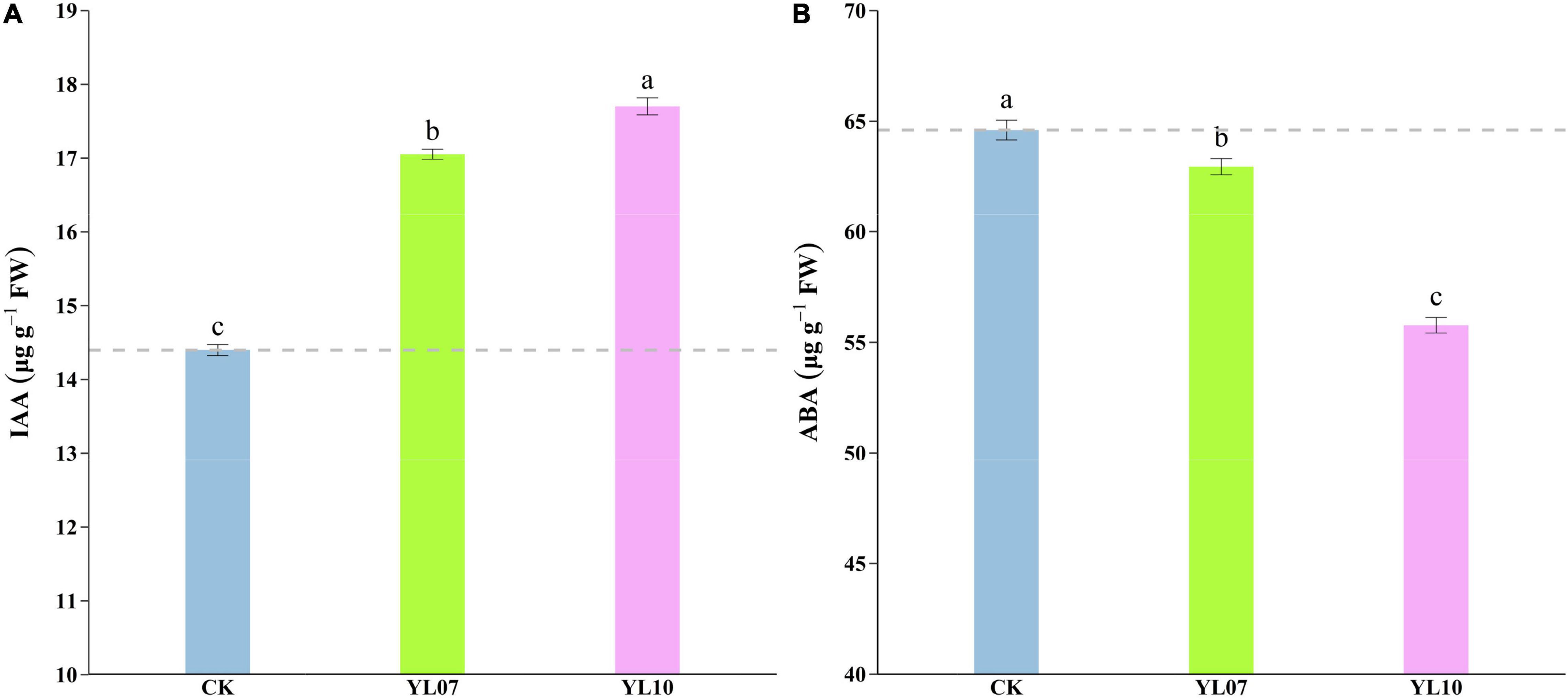
Figure 3. Effect of inoculating with strains YL07 and YL10 on endogenous phytohormone IAA content (A) and ABA content (B) of maize seedlings grown in pots for 31 days under NaCl stress conditions. Different letters indicate significant differences at the P < 0.05 level among the different treatments, as determined by LSD post hoc comparison tests.
Expression of Related Genes in Maize Seedlings
As shown in Figures 4A–C, the transcription levels of the ion balance-related genes ZmNHX1 and ZmNHX2 and ZmHKT in inoculated YL07 and YL10 under NaCl stress were upregulated in comparison with the control treatments, in which ZmNHX1, YL07 and YL10 increased by 465.04 and 742.80% (F = 76.90, P < 0.001), respectively; ZmNHX2, YL07, and YL10 increased by 385.20 and 602.87% (F = 150.60, P < 0.001), respectively; and ZmHKT, YL07, and YL10 increased by 442.57 and 673.70% (F = 68.13, P < 0.001), respectively. Similarly, key transcription gene ZmDREB2A and ZmWRKY58 levels linked to plant response to abiotic stress were also upregulated inoculated with YL07 and YL10 in which ZmDREB2A, YL07 and YL10 increased by 232.13 and 497.66% (F = 82.28, P < 0.001), respectively (Figure 4D); ZmWRKY58, YL07 and YL10 increased by 305.60 and 441.68% (F = 57.79, P < 0.001), respectively (Figure 4E). Moreover, the expression level of the key gene ZmNCED, which is associated with the ABA biosynthesis pathway, indicated that the YL07 and YL10 strains dramatically reduced the ABA content (Figure 4F). Analogously, the expression levels of ZmNCED were significantly decreased by 41.96 and 69.77% (F = 51.92, P < 0.001) under inoculated YL07 and YL10, respectively, compared to the control treatments.
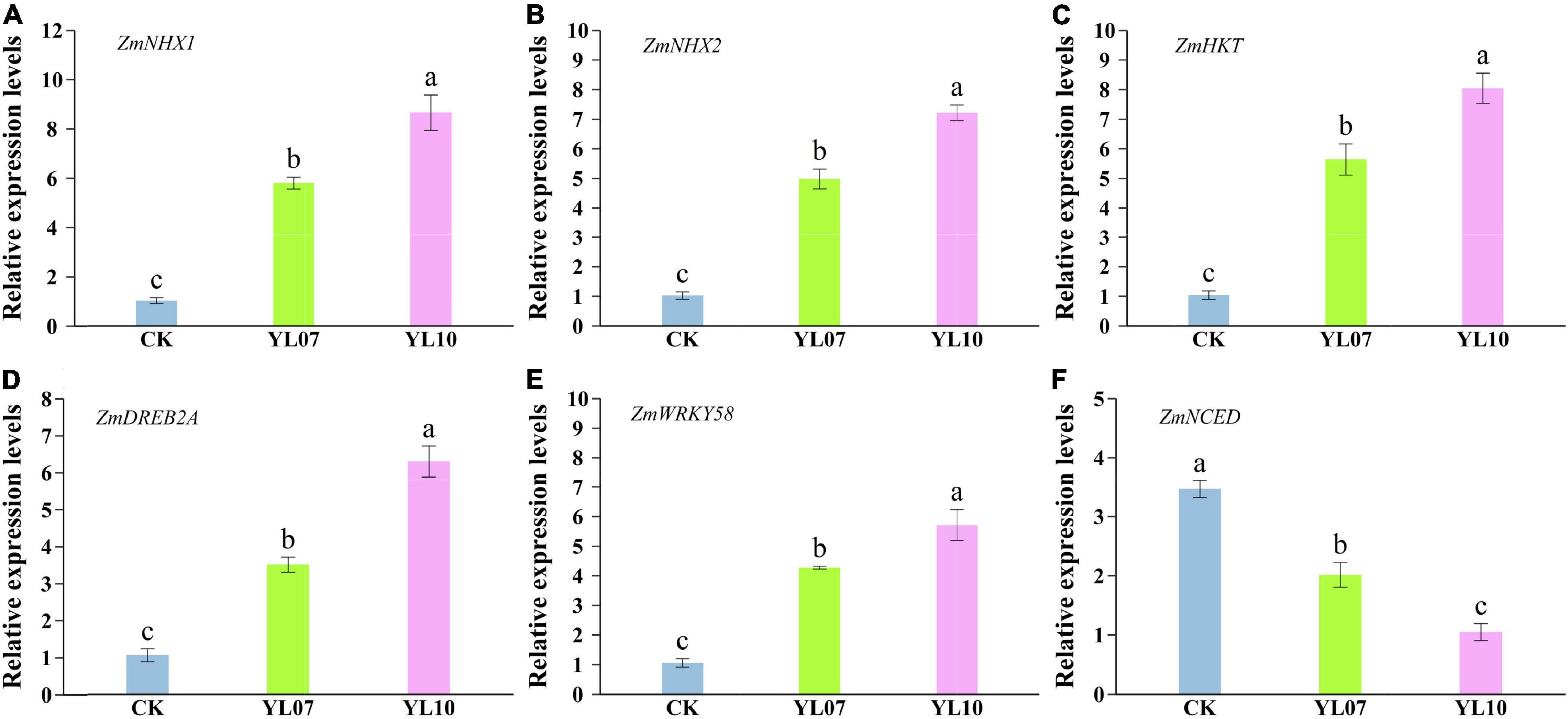
Figure 4. Effect of inoculating with strains YL07 and YL10 on the expression of genes including ZmNHX1 (A), ZmNHX2 (B), ZmHKT (C), ZmDREB2A (D), ZmWRKY58 (E), and ZmNCED (F) related to salt tolerance in maize seedlings under NaCl stress conditions. Different letters indicate significant differences at the P < 0.05 level among the different treatments, as determined by LSD post hoc comparison tests.
Correlation Analysis Between the Studied Parameters
Cluster analysis of all studied parameters revealed that all samples clustered into two major clusters (Figure 5). The changes in the parameters were further observed in the heatmap and indicated that the different treatment groups could be separated based on these parameters. The PCA of all parameters revealed the effects of uninoculated CK and inoculation YL07 and YL10 on maize plants under salt stress (Figure 6). PC1 accounted for 80.1% of the variance, and PC2 accounted for 6.1% of the variance. The CK treatment group was differentiated from the YL07 and YL10 groups, which might be attributed mainly to changes in parameters, including Na+ content, ABA level, and ZmNCED expression level.
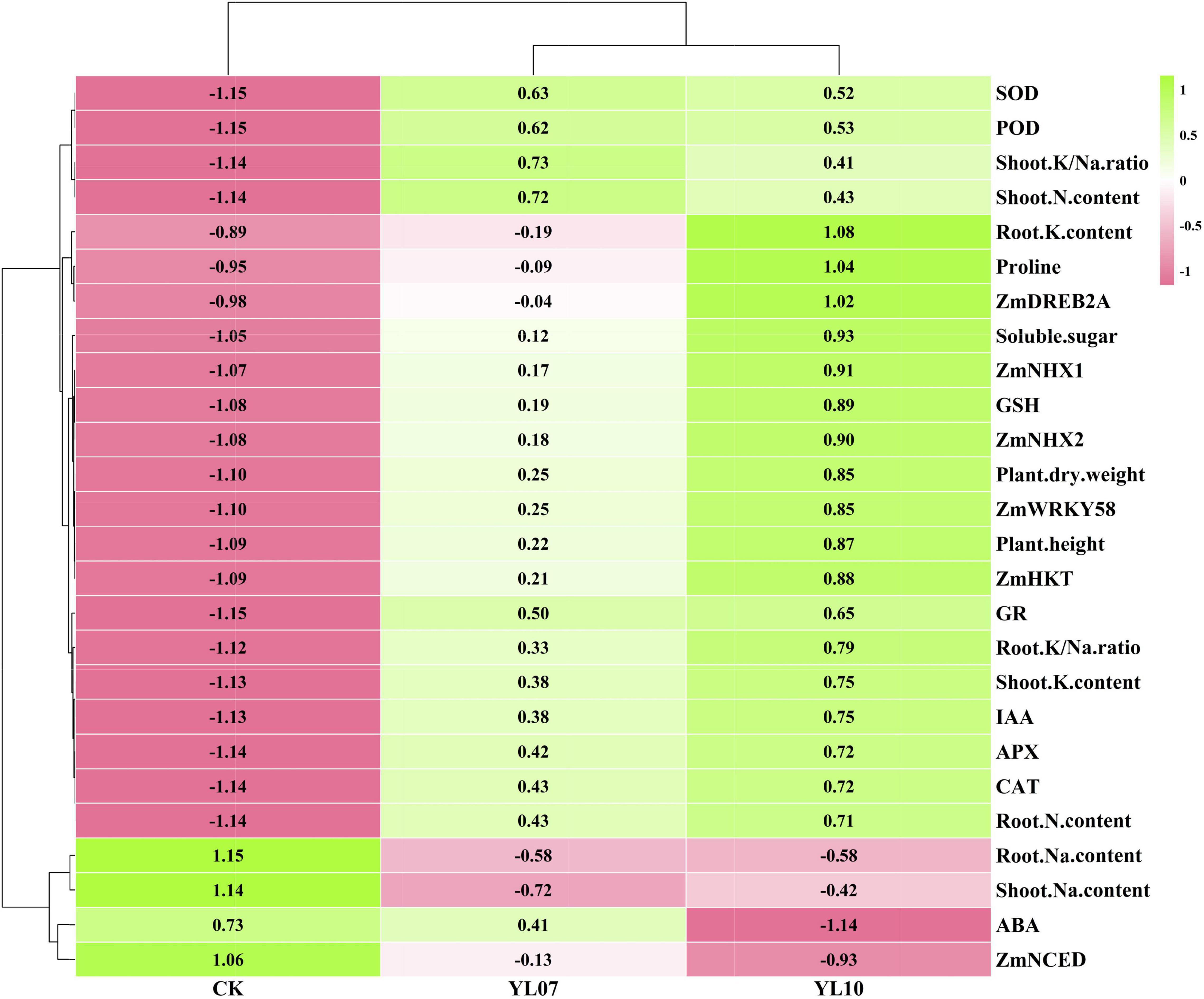
Figure 5. Hierarchical clustering to understand treatment-variable relationships in maize plants under NaCl stress conditions.
Discussion
In previous studies, we found that fungi were more vulnerable to salt than bacteria in Xinjiang saline–alkali land (Hou et al., 2021). Therefore, in this screening of plant growth-promoting rhizobacteria, we focused on bacteria. Second, the previous screening methods were first based on the secretion characteristics of bacteria (Arruda et al., 2013; Pandey and Gupta, 2020; Sultana et al., 2021), but we first started from the germination rate experiment of maize by bacteria. On the one hand, our procedure was more instructive from the perspective of production practice. On the other hand, we found that bacteria secreting promoting substances (such as indole acetic acid, solubilized phosphates, siderophores, and extracellular polysaccharides) might not necessarily promote the growth of crops (Arruda et al., 2013; Wang W. F. et al., 2018). Based on these reasons, combined with the results of the germination experiment and salt tolerance results by bacteria, we comprehensively selected the two kinds of dominant strains, precisely measured the growth-promoting substance secreted by bacteria, and finally thoroughly explained the growth-promoting function of bacteria on maize at the mechanistic level.
Characterization of Plant Growth-Promoting Rhizobacteria Traits Under Salt Stress
In this study, we isolated culturable rhizosphere bacteria from maize rhizosphere soil in saline–alkali land of Xinjiang for the first time and studied their ability in vitro. Two PGPR strains, Bacillus atrophaeus WZYH01 (YL07) and Planococcus soli WZYH02 (YL10), were capable of producing IAA and EPS and solubilizing phosphate effectively (Supplementary Figure 3). Bacillus atrophaeus belongs to the Bacillus genus, which is a gram-positive, aerobic, spore-forming bacterium phenotypically (Nakamura, 1989). Bacillus atrophaeus includes many strains that play an important role in the production of a range of products, including fermented food, bioinsecticides, antibiotics, and other products with commercial applications (Schallmey et al., 2004). A study showed that B. atrophaeus was remarkably resistant to extreme environments (i.e., heat, radiation, toxic chemicals, and pH extremes) (Moir, 2006). Ma et al. (2018) reported that B. atrophaeus strain GQJK17, as a kind of PGPR, had significant effects on inhibiting some soil-borne diseases and promoting the growth of some plants. Bacillus atrophaeus was verified to promote the growth of Zea mays L. and Solanum lycopersicum and had extraordinary activity in root colonization (Huang et al., 2015). The genus Planococcus was first proposed by Migula to adapt to accommodate gram-positive, aerobic bacteria of the family Planococcaceae (Yang et al., 2020). Many Planococcus species have been isolated from a variety of natural environments, including soil samples, marine samples, and extreme environments (Yang et al., 2020). They were highly resistant to the environment, usually salt-tolerant, and could grow at lower temperatures; some special strains could even grow at −15°C (Zhang et al., 2021). For example, Planococcus has also shown the ability to degrade and treat various pollutants, such as phenols and heavy metals (Sun et al., 2014). Planococcus rifietoensis M8T is a salt-tolerant bacterium with potential plant growth-promoting properties that can promote the growth of the wheat by converting ammonia into nitrogen (See-Too et al., 2016). Although the above literature evidence indicates that some species belonging to the Bacillus and Planococcus genera have appropriate potential as PGPR, to our knowledge, this is the first report that B. atrophaeus and Planococcus soli can produce IAA, EPS, and solubilized phosphate under salt stress, which can be used as PGPR to promote maize plant growth under salt stress.
Plant growth-promoting rhizobacteria can effectively improve the tolerance of plants to abiotic stress and then promote plant growth and yield. In our results, we observed that maize inoculated with B. atrophaeus WZYH01 and Planococcus soli WZYH02 showed better growth prospects under salt stress. For example, in the germination experiment, the strains B. atrophaeus WZYH01 and Planococcus soli WZYH02 reduced the adverse effect of salt stress on the maize germination rate compared with the control (Supplementary Figure 1A). Inoculation with B. atrophaeus WZYH01 and Planococcus soli WZYH02 also increased maize root length compared with the root length in the uninoculated treatment (Supplementary Figure 1B). Our research was consistent with a previous study showing that the application of PGPR strains significantly improved the percentage of seed germination under saline conditions (Kaymak et al., 2009).
In the pot experiment, we also found that the strains B. atrophaeus WZYH01 and Planococcus soli WZYH02 had a certain alleviating effect on the growth of maize under salt stress. Compared with the uninoculated control, inoculating strains markedly increased the maize plant height and dry weight (Figure 1). Consistently, Mayak et al. (2004) indicated that salt-tolerant PGPR bacteria could improve the salt tolerance and yield of tomato, corn, and pepper crops in saline soil land.
Plant growth-promoting rhizobacteria traits, such as hormone production, phosphate solubilization, EPS secretion, ACC deaminase synthesis, siderophore production, and nitrogen fixation, had positive effects on promoting plant growth (Etesami and Maheshwari, 2018). This study clearly stated that both B. atrophaeus WZYH01 and Planococcus soli WZYH02, which produce hormone IAA, solubilize phosphorus, and secrete EPS, could improve the maize germination rate, root length, and growth conditions. Therefore, with the inoculation of these two strains, the promoting effect of maize under salt stress might be mediated by these growth-promoting characteristics. The hormone IAA secreted by bacteria was suggested to play an important role in promoting the growth of maize. Kumari et al. (2015) indicated that the higher root length of the inoculated plants might be due to the existence of the IAA hormone secreted by bacteria. Several studies also reported that PGPR strains showed IAA activity, and the interaction between IAA and plants helped to increase root growth under salt stress, which might be an adaptive response to salt stress (Albacete et al., 2008).
Bacterial polysaccharides synthesized and secreted into the external environment may be referred to as exopolysaccharides (Nwodo et al., 2012). EPS produced by PGPR contributed to soil aggregation, water retention, and chelation of metal ions in plants under salinity (Upadhyay et al., 2011). Under salt stress, EPS produced by PGPR could combine with Na+ ions, reduce Na+ content, and help to maintain ion balance in the root zone (Mishra et al., 2021). Liu et al. (2017) found that EPS produced by B. amyloliquefaciens FZB42 could finally reduce the Na+ concentration in plants owing to binding to Na+ content and inhibiting the absorption of Na+. In the current study, B. atrophaeus WZYH01 and Planococcus soli WZYH02 produced a large number of EPS (Supplementary Figure 3), which could help maize plants resist salt stress. A previous study indicated that the formation of EPS and biofilms produced by the PGPR Pseudomonas anguilliseptica SAW 24 significantly improved the growth of Vicia faba L. under salt stress (Mohammed, 2018).
Phosphorus is the second most important key plant nutrient after nitrogen (Nanthakumar and Panneerselvam, 2017). Phosphorus participates in the important functions of plant energy metabolism, structural function, and signal transduction function and is necessary for plant growth and development (Lobo et al., 2019). Most of the phosphorus in the soil was fixed. Therefore, although the soil was rich in inorganic phosphorus and organic phosphorus, plant-available phosphorus was rarely effective. Phosphate solubilizing bacteria (PSB) have the ability to mobilize insoluble phosphate in soil and improve the tolerance of plants to various stresses (Kumar et al., 2018). The available phosphorus is dissolved by phosphate-dissolving microorganisms by producing acids (Alori et al., 2017). In this investigation, B. atrophaeus WZYH01 and Planococcus soli WZYH02 were solubilized phosphorus strains, which could help maize overcome nutrient deficiency and hormone imbalance under salt conditions (Supplementary Figure 3). Consistently, PSB could increase the content of soluble phosphorus in soil solution, promote the root growth of rice seedlings, and increase plant biomass (Panhwar et al., 2013). In addition, cucumber plants inoculated with Cucumis sativus also exhibited a higher P content than uninoculated plants under salinity stress (Kang et al., 2014a).
Effect of Plant Growth-Promoting Rhizobacteria Inoculation on Maize Tolerance to NaCl Stress
Salt stress inhibited plant growth due to the increase in Na+ concentration and the decrease in the K+/Na+ ratio. Na+ exclusion and K+ influx are the most important strategies for plants to alleviate salt stress (Rojas-Tapias et al., 2012). PGPR could increase intracellular K+ levels and maintain the K+/Na+ ratio by removing excess Na+ in plant cells under salt stress (Mishra et al., 2021). We observed that high levels of Na+ in maize roots and shoots were negatively correlated with maize biomass and plant height. In contrast, the K+ content was positively correlated with plant biomass and plant height (Figure 6). Similarly, the results showed that inoculation with the two bacteria resulted in a decrease in Na+ and a significant increase in K+, which led to an increase in the K+/Na+ ratio (Table 1). Consistently, under salt stress, inoculation of PGPR has been reported to be able to avoid excessive accumulation of Na+ in plants and maintain ion homeostasis (Kang et al., 2014b). Moreover, PGPR Azospirillum lipoferum and Azotobacter chroococcum increased the K+ level of maize plants under salt stress, maintained the K+/Na+ ratio, and reduced the Na+ level (Latef et al., 2020).
The main aspects of PGPR-mediated plant salt tolerance include generating reaction mechanisms to concentrate toxicity and establish osmotic equilibrium to avoid plant cell desiccation and flaccidity. As part of this regulatory pathway, Na+/H+ antiporters of tonoplast are encoded by the NHX genes. This progress in understanding the signal transduction pathway for the ion homeostasis and salt resistance of higher plants opens possibilities to establish salt-resistant crop plants (Zorb et al., 2005). PGPR inhibits the absorption of Na+ by changing the composition of the cell wall or cell membrane, increases the electrogenic Na+/H+ ion transporters in plants, and increases the expression of NHX transporters (Kumar Arora et al., 2020). In this study, inoculating two strains, B. atrophaeus WZYH01 and Planococcus soli WZYH02, significantly reduced the level of Na+ and upregulated the expression of ZmNHX and ZmHKT genes in maize under salt stress, indicating that inoculated strains might reduce the content of Na+ in maize by isolating Na+ into vacuoles and excreting Na+ from cells to improve Na+ toxicity and salt tolerance (Figures 4A–C). A previous study indicated that Bacillus promoted the growth of maize under salt stress by upregulating the expression of the plant ion homeostasis-related genes NHX1 and HKT1. Furthermore, Bacillus subtilis reduced Na+ uptake by Arabidopsis thaliana roots by downregulating the high-affinity K+ transporter (HKT1) under salt stress (Zhang et al., 2008). Our results substantiate that the two PGPRs could improve maize salt tolerance by the upregulation of ZmNHX and ZmHKT genes with increased inclusion of Na+ in vacuoles to avoid the accumulation of Na+.
Mechanism of Plant Growth-Promoting Rhizobacteria in Promoting Maize Growth
The plant responds to salt stress conditions by generating reactive oxygen species (ROS), which generate oxidative stain in plants and impair chlorophyll, DNA, protein, and membrane functions (Kaushal and Wani, 2016). Salinity also causes the deleterious effect of osmotic pressure, deterioration of metabolic functions, decreased energy requirements, and disruption of cell divisions as well (Desoky et al., 2019). Whenever possible, plants are developing and/or adopting an antioxidant system to mitigate the destructive effects caused by salinity-induced ROS (Desoky et al., 2020). This system contains many enzymatic and non-enzymatic antioxidants with low molecular weights (Mishra et al., 2021). In the current study, inoculation with B. atrophaeus WZYH01 and Planococcus soli WZYH02 significantly increased the activity of enzymatic antioxidants (SOD, CAT, POD, APX, and GR) and the content of the non-enzymatic antioxidant GSH in maize under salt stress (Figures 2A–F). The above results showed that the two strains could stimulate the defense response of plants to reactive oxygen species detoxification and promote the growth of maize seedlings. Enzymatic and non-enzymatic antioxidant activities may be reduced as PGPR detoxifies ROS through a variety of plant protection mechanisms, such as K+ accumulation and phytohormones secreted by PGPR.
Plant growth-promoting rhizobacteria treatment resulted in a significant increase in proline and soluble sugars contents in salt stress, which acted as an osmoprotectant mechanism (Figures 2G,H). Proline, as a non-enzymatic antioxidant, increases with PGPR inoculation to improve the plant antioxidant system and restore energy compensation in plants. Proline reduces ROS damage and enhances plant tolerance by reducing the detoxification of ROS resulting from salinity stress (Howladar, 2014). Likewise, the accumulation of soluble sugars maintains the harmony between the osmotic quality of the cytosol and the vacuole. However, Desoky et al. (2020) indicated that proline and soluble sugars were significantly reduced by inoculation with PGPRs, which was not consistent with our research. In the present study, B. atrophaeus WZYH01 and Planococcus soli WZYH02 could increase proline and soluble sugar accumulation under salt stress. This also suggested the existence of other osmoprotectants to improve plant resistance to salt stress.
Plant growth-promoting rhizobacteria have been reported to stimulate the plant’s nutrient acquisition machinery by activating nutrient-deficiency-induced transcription factors in addition to auxins and cytokinin secretion (Li et al., 2020). ABA is involved in various physiological processes of plants, including stomatal conductance, seedling growth, and plant responses to environmental stress (Li et al., 2020). Mishra et al. (2021) indicated that inoculation with PGPR could change the expression of ABA and the related gene NCED in plants. PGPR P. fluorescence and P. putida could downregulate the NCED gene of ABA biosynthesis in salt-stressed barley (Zaib et al., 2020). This study was consistent with our research. Bacillus atrophaeus WZYH01 and Planococcus soli WZYH02 showed a lower ABA content and ZmNCED expression (Figures 3B, 4F). DREBs and WRKY transcript factors also play a critical role in enhancing plant salt tolerance (Li et al., 2020). Similar to the situation in wheat, the expression of a DREB2 homolog in maize was found to be activated also by cold despite its induction by drought and salt stress (Nguyen et al., 2009). This study showed an increase in ZmDREB2A and ZmWRKY58 gene expression in B. atrophaeus WZYH01 and Planococcus soli WZYH02-inoculated maize under saline conditions compared to non-inoculated control plants (Figures 4D,E). Therefore, under salt stress, B. atrophaeus WZYH01 and Planococcus soli WZYH02 are involved in changes in plant hormone-related gene expression, which may be involved in the improvement of salt stress and the regulation of plant development.
Conclusion
In this study, two salt-tolerant strains, B. atrophaeus WZYH01 and Planococcus soli WZYH02, were isolated from maize rhizosphere soil in Xinjiang, China. Two strains could tolerate up to 1197 mM NaCl and exhibited three growth-promoting traits: IAA, exopolysaccharide production, and phosphate solubilization. Bacillus atrophaeus WZYH01 and Planococcus soli WZYH02 could protect maize from salt stress, which could be considered the integration of multiple physiological processes, including improving plant mineral nutrition and increasing plant antioxidant capacity through plant hormones (IAA and ABA), and the expression of transporter genes, increasing the K+/Na+ ratio in maize plants. In general, the effect of Planococcus soli WZYH02 was more prominent in the growth index and gene expression than the effect of B. atrophaeus WZYH01. In conclusion, this study showed that B. atrophaeus WZYH01 and Planococcus soli WZYH02 could be used as eco-friendly PGPR based on their potential in promoting and protecting maize plant growth under saline conditions.
Data Availability Statement
The datasets presented in this study can be found in online repositories. The names of the repository/repositories and accession number(s) can be found below: National Center for Biotechnology Information (NCBI) BioProject database under accession numbers MZ919348 and MZ919345.
Author Contributions
YH involved in investigation, data curation, writing—original draft preparation, and visualization. WZ involved in conceptualization, methodology, writing—review and editing, funding acquisition, and supervision. CA took a leading role in writing—review and editing. YL involved in investigation, validation, and formal analysis. ZW and MH designed the methodology and formal analysis. JH involved in funding acquisition and supervision. All authors contributed to the article and approved the submitted version.
Funding
We are grateful for the financial support from the Program of the National Natural Science Foundation of China (NSFC) (grant numbers 51790533, 51879196, and 52179039).
Conflict of Interest
The authors declare that the research was conducted in the absence of any commercial or financial relationships that could be construed as a potential conflict of interest.
Publisher’s Note
All claims expressed in this article are solely those of the authors and do not necessarily represent those of their affiliated organizations, or those of the publisher, the editors and the reviewers. Any product that may be evaluated in this article, or claim that may be made by its manufacturer, is not guaranteed or endorsed by the publisher.
Supplementary Material
The Supplementary Material for this article can be found online at: https://www.frontiersin.org/articles/10.3389/fpls.2022.891372/full#supplementary-material
Footnotes
References
Abbas, R., Rasul, S., Aslam, K., Baber, M., Shahid, M., Mubeen, F., et al. (2019). Halotolerant PGPR: a hope for cultivation of saline soils. J. King Saud Univ. Sci. 31, 1195–1201. doi: 10.1016/j.jksus.2019.02.019
Albacete, A., Ghanem, M. E., Martinez-Andujar, C., Acosta, M., Sanchez-Bravo, J., Martinez, V., et al. (2008). Hormonal changes in relation to biomass partitioning and shoot growth impairment in salinized tomato (Solanum lycopersicum L.) plants. J. Exp. Bot. 59, 4119–4131. doi: 10.1093/jxb/ern251
Alori, E. T., Glick, B. R., and Babalola, O. O. (2017). Microbial phosphorus solubilization and its potential for use in sustainable agriculture. Front. Microbiol. 8:971. doi: 10.3389/fmicb.2017.00971
Arruda, L., Beneduzi, A., Martins, A., Lisboa, B., Lopes, C., Bertolo, F., et al. (2013). Screening of rhizobacteria isolated from maize (Zea mays L.) in Rio Grande do Sul State (South Brazil) and analysis of their potential to improve plant growth. Appl. Soil Ecol. 63, 15–22. doi: 10.1016/j.apsoil.2012.09.001
Banerjee, A., Bareh, D. A., and Joshi, S. R. (2017). Native microorganisms as potent bioinoculants for plant growth promotion in shifting agriculture (Jhum) systems. J. Soil Sci. Plant Nutr. 17, 127–140. doi: 10.4067/s0718-95162017005000010
Bremner, J. M. (2009). Determination of nitrogen in soil by the Kjeldahl method. J. Agric. Sci. 55, 11–33. doi: 10.1017/s0021859600021572
Desoky, E. M., Elrys, A. S., and Rady, M. M. (2019). Integrative moringa and licorice extracts application improves Capsicum annuum fruit yield and declines its contaminant contents on a heavy metals-contaminated saline soil. Ecotoxicol. Environ. Saf. 169, 50–60.
Desoky, E.-S. M., Saad, A. M., El-Saadony, M. T., Merwad, A.-R. M., and Rady, M. M. (2020). Plant growth-promoting rhizobacteria: potential improvement in antioxidant defense system and suppression of oxidative stress for alleviating salinity stress in Triticum aestivum (L.) plants. Biocatal. Agric. Biotechnol. 30:101878.
Dong, Y., Chen, R., Petropoulos, E., Yu, B., Zhang, J., Lin, X., et al. (2022). Interactive effects of salinity and SOM on the ecoenzymatic activities across coastal soils subjected to a saline gradient. Geoderma 406:115519. doi: 10.1016/j.geoderma.2021.115519
Etesami, H., and Maheshwari, D. K. (2018). Use of plant growth promoting rhizobacteria (PGPRs) with multiple plant growth promoting traits in stress agriculture: action mechanisms and future prospects. Ecotox. Environ. Saf. 156, 225–246. doi: 10.1016/j.ecoenv.2018.03.013
Goswami, D., Dhandhukia, P., Patel, P., and Thakker, J. N. (2014). Screening of PGPR from saline desert of Kutch: growth promotion in Arachis hypogea by Bacillus licheniformis A2. Microbiol. Res. 169, 66–75. doi: 10.1016/j.micres.2013.07.004
Habibi, S., Djedidi, S., Ohkama-Ohtsu, N., Sarhadi, W. A., Kojima, K., Rallos, R. V., et al. (2019). Isolation and screening of indigenous plant growth-promoting rhizobacteria from different rice cultivars in afghanistan soils. Microbes Environ. 34, 347–355. doi: 10.1264/jsme2.ME18168
Han, Y. J., Liu, S. X., Chen, F. L., Deng, X. L., Miao, Z., Wu, Z. S., et al. (2021). Characteristics of plant growth-promoting rhizobacteria SCPG-7 and its effect on the growth of Capsicum annuum L. Environ. Sci. Pollut. Res. 28, 11323–11332. doi: 10.1007/s11356-020-11388-6
Hawkesford, M. J. (2014). Reducing the reliance on nitrogen fertilizer for wheat production. J. Cereal Sci. 59, 276–283. doi: 10.1016/j.jcs.2013.12.001
Hou, Y. L., Zeng, W. Z., Hou, M. L., Wang, Z., Luo, Y., Lei, G. Q., et al. (2021). Responses of the soil microbial community to salinity stress in maize fields. Biol. Basel 10:1114. doi: 10.3390/biology10111114
Howladar, S. M. (2014). A novel Moringa oleifera leaf extract can mitigate the stress effects of salinity and cadmium in bean (Phaseolus vulgaris L.) plants. Ecotoxicol. Environ. Saf. 100, 69–75.
Huang, X. F., Zhou, D., Guo, J., Manter, D. K., Reardon, K. F., and Vivanco, J. M. (2015). Bacillus spp. from rainforest soil promote plant growth under limited nitrogen conditions. J. Appl. Microbiol. 118, 672–684. doi: 10.1016/10.1111/jam.1272
Kang, S. M., Khan, A. L., Waqas, M., You, Y. H., Kim, J. H., Kim, J. G., et al. (2014a). Plant growth-promoting rhizobacteria reduce adverse effects of salinity and osmotic stress by regulating phytohormones and antioxidants in Cucumis sativus. J. Plant Interact. 9, 673–682. doi: 10.1080/17429145.2014.894587
Kang, S. M., Radhakrishnan, R., Khan, A. L., Kim, M. J., Park, J. M., Kim, B. R., et al. (2014b). Gibberellin secreting rhizobacterium, Pseudomonas putida H-2-3 modulates the hormonal and stress physiology of soybean to improve the plant growth under saline and drought conditions. Plant Physiol. Biochem. 84, 115–124. doi: 10.1016/j.plaphy.2014.09.001
Kaushal, M., and Wani, S. P. (2016). Rhizobacterial-plant interactions: strategies ensuring plant growth promotion under drought and salinity stress. Agric. Ecosyst. Environ. 231, 68–78. doi: 10.1016/j.agee.2016.06.031
Kaymak, H. C., Guvenc, I., Yarali, F., and Donmez, M. F. (2009). The effects of bio-priming with PGPR on germination of radish (Raphanus sativus L.) seeds under saline conditions. Turk. J. Agric. For. 33, 173–179. doi: 10.3906/tar-0806-30
Khalilpour, M., Mozafari, V., and Abbaszadeh-Dahaji, P. (2021). Tolerance to salinity and drought stresses in pistachio (Pistacia vera L.) seedlings inoculated with indigenous stress-tolerant PGPR isolates. Sci. Hortic. 289:110440. doi: 10.1016/j.scienta.2021.110440
Kumar, A., Kumar, A., and Patel, H. (2018). Role of microbes in phosphorus availability and acquisition by plants. Int. J. Curr. Microbiol. App. Sci. 7, 1344–1347. doi: 10.20546/ijcmas.2018.705.161
Kumar, A., Patel, J. S., Meena, V. S., and Srivastava, R. (2019). Recent advances of PGPR based approaches for stress tolerance in plants for sustainable agriculture. Biocatal. Agric. Biotechnol. 20:101271. doi: 10.1016/j.bcab.2019.101271
Kumar, K., Amaresan, N., and Madhuri, K. (2017). Alleviation of the adverse effect of salinity stress by inoculation of plant growth promoting rhizobacteria isolated from hot humid tropical climate. Ecol. Eng. 102, 361–366. doi: 10.1016/j.ecoleng.2017.02.023
Kumar Arora, N., Fatima, T., Mishra, J., Mishra, I., Verma, S., Verma, R., et al. (2020). Halo-tolerant plant growth promoting rhizobacteria for improving productivity and remediation of saline soils. J. Adv. Res. 26, 69–82. doi: 10.1016/j.jare.2020.07.003
Kumari, S., Vaishnav, A., Jain, S., Varma, A., and Choudhary, D. K. (2015). Bacterial-mediated induction of systemic tolerance to salinity with expression of stress alleviating enzymes in soybean (Glycine max L. Merrill). J. Plant Growth Regul. 34, 558–573. doi: 10.1007/s00344-015-9490-0
Latef, A. A. H. A., Abu Alhmad, M. F., Kordrostami, M., Abo-Baker, A. B. A., and Zakir, A. (2020). Inoculation with Azospirillum lipoferum or Azotobacter chroococcum reinforces maize growth by improving physiological activities under saline conditions. J. Plant Growth Regul. 39, 1293–1306. doi: 10.1007/s00344-020-10065-9
Li, X. Z., Sun, P., Zhang, Y. N., Jin, C., and Guan, C. F. (2020). A novel PGPR strain Kocuria rhizophila Y1 enhances salt stress tolerance in maize by regulating phytohormone levels, nutrient acquisition, redox potential, ion homeostasis, photosynthetic capacity and stress-responsive genes expression. Environ. Exp. Bot. 174:104023. doi: 10.1016/j.envexpbot.2020.104023
Liang, J. P., Li, Y., Si, B. C., Wang, Y. Z., Chen, X. G., Wang, X. F., et al. (2021). Optimizing biochar application to improve soil physical and hydraulic properties in saline-alkali soils. Sci. Total Environ. 771:144802. doi: 10.1016/j.scitotenv.2020.144802
Liu, M. L., Wang, C., Liu, X. L., Lu, Y. C., and Wang, Y. F. (2020). Saline-alkali soil applied with vermicompost and humic acid fertilizer improved macroaggregate microstructure to enhance salt leaching and inhibit nitrogen losses. Appl. Soil Ecol. 156:103705. doi: 10.1016/j.apsoil.2020.103705
Liu, S. F., Hao, H. T., Lu, X., Zhao, X., Wang, Y., Zhang, Y. B., et al. (2017). Transcriptome profiling of genes involved in induced systemic salt tolerance conferred by Bacillus amyloliquefaciens FZB42 in Arabidopsis thaliana. Sci. Rep. 7:10795. doi: 10.1038/s41598-017-11308-8
Liu, W. G., Yang, X. D., Duan, L. C., Naidu, R., Yan, K. H., Liu, Y. J., et al. (2021). Variability in plant trace element uptake across different crops, soil contamination levels and soil properties in the Xinjiang Uygur Autonomous Region of northwest China. Sci. Rep. 11:2064. doi: 10.1038/s41598-021-81764-w
Liu, Y., Ao, C., Zeng, W. Z., Srivastava, A. K., Gaiser, T., Wu, J. W., et al. (2021). Simulating water and salt transport in subsurface pipe drainage systems with HYDRUS-2D. J. Hydrol. 592:125823. doi: 10.1016/j.jhydrol.2020.125823
Liu, Y., Tao, J., Yan, Y. J., Li, B., Li, H., and Li, C. (2011). Biocontrol efficiency of Bacillus subtilis SL-13 and characterization of an antifungal chitinase. Chin. J. Chem. Eng. 19, 128–134. doi: 10.1016/S1004-9541(09)60188-9
Lobo, C. B., Juarez Tomas, M. S., Viruel, E., Ferrero, M. A., and Lucca, M. E. (2019). Development of low-cost formulations of plant growth-promoting bacteria to be used as inoculants in beneficial agricultural technologies. Microbiol. Res. 219, 12–25. doi: 10.1016/j.micres.2018.10.012
Ma, J. J., Wang, C. Q., Wang, H. D., Liu, K., Zhang, T. R., Yao, L. T., et al. (2018). Analysis of the complete genome sequence of Bacillus atrophaeus GQJK17 reveals its biocontrol characteristics as a plant growth-promoting rhizobacterium. Biomed. Res. Int. 2018:9473542. doi: 10.1155/2018/9473542
Ma, T., Zeng, W. Z., Lei, G. Q., Wu, J. W., and Huang, J. S. (2021). Predicting the rooting depth, dynamic root distribution and the yield of sunflower under different soil salinity and nitrogen applications. Ind. Crop Prod. 170:113749. doi: 10.1016/j.indcrop.2021.113749
Marulanda, A., Barea, J. M., and Azcon, R. (2009). Stimulation of plant growth and drought tolerance by native microorganisms (AM Fungi and Bacteria) from dry environments: mechanisms related to bacterial effectiveness. J. Plant Growth Regul. 28, 115–124. doi: 10.1007/s00344-009-9079-6
Mayak, S., Tirosh, T., and Glick, B. R. (2004). Plant growth-promoting bacteria confer resistance in tomato plants to salt stress. Plant Physiol. Biochem. 42, 565–572. doi: 10.1016/j.plaphy.2004.05.009
Mishra, P., Mishra, J., and Arora, N. K. (2021). Plant growth promoting bacteria for combating salinity stress in plants - Recent developments and prospects: a review. Microbiol. Res. 252:126861. doi: 10.1016/j.micres.2021.126861
Mohammed, A. F. (2018). Effectiveness of exopolysaccharides and biofilm forming plant growth promoting rhizobacteria on salinity tolerance of faba bean (Vicia faba L.). Afr. J. Microbiol. Res. 12, 399–404. doi: 10.5897/ajmr2018.8822
Moir, A. (2006). How do spores germinate? J. Appl. Microbiol. 101, 526–530. doi: 10.1111/j.1365-2672.2006.02885.x
Moreira, H., Pereira, S. I. A., Marques, A. P. G. C., Rangel, A. O. S. S., and Castro, P. M. L. (2016). Selection of metal resistant plant growth promoting rhizobacteria for the growth and metal accumulation of energy maize in a mine soil — Effect of the inoculum size. Geoderma 278, 1–11. doi: 10.1016/j.geoderma.2016.05.003
Moreira, H., Pereira, S. I. A., Marques, A. P. G. C., Rangel, A. O. S. S., and Castro, P. M. L. (2019). Effects of soil sterilization and metal spiking in plant growth promoting rhizobacteria selection for phytotechnology purposes. Geoderma 334, 72–81. doi: 10.1016/j.geoderma.2018.07.025
Nakamura, L. K. (1989). Taxonomic relationship of black-pigmented Bacillus subtilis strains and a proposal for Bacillus atrophaeus sp. nov. Int. J. Syst. Bacteriol. 39, 295–300. doi: 10.1099/00207713-39-3-295
Nanthakumar, S., and Panneerselvam, P. (2017). Phosphorus and sulphur releasing pattern and their availability for maize cultivation. J. Cer. Oil. 8, 10–13. doi: 10.5897/jco2016.0152
Nguyen, H. T., Leipner, J., Stamp, P., and Guerra-Peraza, O. (2009). Low temperature stress in maize (Zea mays L.) induces genes involved in photosynthesis and signal transduction as studied by suppression subtractive hybridization. Plant Physiol. Biochem. 47, 116–122.
Nwodo, U. U., Green, E., and Okoh, A. I. (2012). Bacterial exopolysaccharides: functionality and prospects. Int. J. Mol. Sci. 13, 14002–14015. doi: 10.3390/ijms131114002
Pandey, S., and Gupta, S. (2020). Diversity analysis of ACC deaminase producing bacteria associated with rhizosphere of coconut tree (Cocos nucifera L.) grown in Lakshadweep islands of India and their ability to promote plant growth under saline conditions. J. Biotechnol. 324, 183–197. doi: 10.1016/j.jbiotec.2020.10.024
Panhwar, Q. A., Jusop, S., Naher, U. A., Othman, R., and Razi, M. I. (2013). Application of potential phosphate-solubilizing bacteria and organic acids on phosphate solubilization from phosphate rock in aerobic rice. Sci. World J. 2013:272409. doi: 10.1155/2013/272409
Qiu, Z., Egidi, E., Liu, H., Kaur, S., and Singh, B. K. (2019). New frontiers in agriculture productivity: optimised microbial inoculants and in situ microbiome engineering. Biotechnol. Adv. 37:107371. doi: 10.1016/j.biotechadv.2019.03.010
Rojas-Tapias, D., Moreno-Galvan, A., Pardo-Diaz, S., Obando, M., Rivera, D., and Bonilla, R. (2012). Effect of inoculation with plant growth-promoting bacteria (PGPB) on amelioration of saline stress in maize (Zea mays). Appl. Soil Ecol. 61, 264–272. doi: 10.1016/j.apsoil.2012.01.006
Schallmey, M., Singh, A., and Ward, O. P. (2004). Developments in the use of Bacillus species for industrial production. Can. J. Microbiol. 50, 1–17. doi: 10.1139/w03-076
See-Too, W. S., Convey, P., Pearce, D. A., Lim, Y. L., Ee, R., Yin, W. F., et al. (2016). Complete genome of Planococcus rifietoensis M8T, a halotolerant and potentially plant growth promoting bacterium. J. Biotechnol. 221, 114–115. doi: 10.1016/j.jbiotec.2016.01.026
Shameer, S., and Prasad, T. N. V. K. V. (2018). Plant growth promoting rhizobacteria for sustainable agricultural practices with special reference to biotic and abiotic stresses. Plant Growth Regul. 84, 603–615. doi: 10.1007/s10725-017-0365-1
Siddikee, M. A., Chauhan, P. S., Anandham, R., Han, G. H., and Sa, T. (2010). Isolation, characterization, and use for plant growth promotion under salt stress, of ACC deaminase-producing halotolerant bacteria derived from coastal soil. J. Microbiol. Biotechnol. 20, 1577–1584. doi: 10.4014/jmb.1007.07011
Sultana, S., Alam, S., and Karim, M. M. (2021). Screening of siderophore-producing salt-tolerant rhizobacteria suitable for supporting plant growth in saline soils with iron limitation. J. Agric. Food Res. 4:100150. doi: 10.1016/j.jafr.2021.100150
Sun, J. Q., Xu, L., Zhang, Z., Li, Y., Tang, Y. Q., and Wu, X. L. (2014). Diverse bacteria isolated from microtherm oil-production water. Antonie Van Leeuwenhoek 105, 401–411. doi: 10.1007/s10482-013-0088-x
Tirry, N., Kouchou, A., Laghmari, G., Lemjereb, M., Hnadi, H., Amrani, K., et al. (2021). Improved salinity tolerance of Medicago sativa and soil enzyme activities by PGPR. Biocatal. Agric. Biotechnol. 31:101914. doi: 10.1016/j.bcab.2021.101914
Upadhyay, S. K., Singh, J. S., and Singh, D. P. (2011). Exopolysaccharide-producing plant growth-promoting rhizobacteria under salinity condition. Pedosphere 21, 214–222. doi: 10.1016/s1002-0160(11)60120-3
van Elsas, J. D., Dijkstra, A. F., Govaert, J. M., and van Veen, J. A. (1986). Survival of Pseudomonas fluorescens and Bacillus subtilis introduced into two soils of different texture in field microplots. FEMS Microbiol. Ecol. 2, 151–160. doi: 10.1111/j.1574-6968.1986.tb01724.x
Wang, L. L., Palta, J. A., Chen, W., Chen, Y. L., and Deng, X. P. (2018). Nitrogen fertilization improved water-use efficiency of winter wheat through increasing water use during vegetative rather than grain filling. Agric. Water Manage. 197, 41–53. doi: 10.1016/j.agwat.2017.11.010
Wang, R. S., Kang, Y. H., Wan, S. Q., Hu, W., Liu, S. P., Jiang, S. F., et al. (2012). Influence of different amounts of irrigation water on salt leaching and cotton growth under drip irrigation in an arid and saline area. Agric. Water Manage. 110, 109–117. doi: 10.1016/j.agwat.2012.04.005
Wang, W. F., Wu, Z. S., He, Y. H., Huang, Y. Y., Li, X., and Ye, B. C. (2018). Plant growth promotion and alleviation of salinity stress in Capsicum annuum L. by Bacillus isolated from saline soil in Xinjiang. Ecotoxicol. Environ. Saf. 164, 520–529. doi: 10.1016/j.ecoenv.2018.08.070
Wicke, B., Smeets, E., Dornburg, V., Vashev, B., Gaiser, T., Turkenburg, W., et al. (2011). The global technical and economic potential of bioenergy from salt-affected soils. Energy Environ. Sci. 4, 2669–2681. doi: 10.1039/c1ee01029h
Wolf, B. (2008). A comprehensive system of leaf analyses and its use for diagnosing crop nutrient status. Commun. Soil Sci. Plant Anal. 13, 1035–1059. doi: 10.1080/00103628209367332
Yang, R. Q., Zhang, B. L., Wang, J. C., Tai, X. S., Sun, H. L., Zhang, G. S., et al. (2020). Planococcus lenghuensis sp. nov., an oil-degrading bacterium isolated from petroleum-contaminated soil. Antonie van Leeuwenhoek 113, 839–850. doi: 10.1007/s10482-020-01394-6
Yao, L. X., Wu, Z. S., Zheng, Y. Y., Kaleem, I., and Li, C. (2010). Growth promotion and protection against salt stress by Pseudomonas putida Rs-198 on cotton. Eur. J. Soil Biol. 46, 49–54. doi: 10.1016/j.ejsobi.2009.11.002
Yue, H. T., Mo, W. P., Li, C., Zheng, Y. Y., and Li, H. (2007). The salt stress relief and growth promotion effect of Rs-5 on cotton. Plant Soil 297, 139–145. doi: 10.1007/s11104-007-9327-0
Zaib, S., Ahmad, I., and Shakeel, S. N. (2020). Modulation of barley (Hordeum vulgare) defense and hormonal pathways by Pseudomonas species accounted for salinity tolerance. Pak. J. Agric. Sci. 57, 1469–1481. doi: 10.21162/PAKJAS/20.9373
Zeng, W. Z., Xu, C., Wu, J. W., and Huang, J. S. (2016). Sunflower seed yield estimation under the interaction of soil salinity and nitrogen application. Field Crop. Res. 198, 1–15. doi: 10.1016/j.fcr.2016.08.007
Zhang, H., Kim, M. S., Sun, Y., Dowd, S. E., Shi, H., and Pare, P. W. (2008). Soil bacteria confer plant salt tolerance by tissue-specific regulation of the sodium transporter HKT1. Mol. Plant Microbe Interact. 21, 737–744. doi: 10.1094/MPMI-21-6-0737
Zhang, S. Y., Ping, W. W., Xin, Y. H., Xin, D., and Zhang, J. L. (2021). Planococcus soli sp. nov., isolated from antarctic soil. Antonie Van Leeuwenhoek 114, 1107–1115. doi: 10.1007/s10482-021-01581-z
Keywords: maize, PGPR, soil salinity, plant growth promotion, antioxidant
Citation: Hou Y, Zeng W, Ao C, Luo Y, Wang Z, Hou M and Huang J (2022) Bacillus atrophaeus WZYH01 and Planococcus soli WZYH02 Improve Salt Tolerance of Maize (Zea mays L.) in Saline Soil. Front. Plant Sci. 13:891372. doi: 10.3389/fpls.2022.891372
Received: 07 March 2022; Accepted: 08 April 2022;
Published: 06 May 2022.
Edited by:
Jin-Lin Zhang, Lanzhou University, ChinaReviewed by:
Manoj Kumar Solanki, University of Silesia in Katowice, PolandHonghong Wu, Huazhong Agricultural University, China
Copyright © 2022 Hou, Zeng, Ao, Luo, Wang, Hou and Huang. This is an open-access article distributed under the terms of the Creative Commons Attribution License (CC BY). The use, distribution or reproduction in other forums is permitted, provided the original author(s) and the copyright owner(s) are credited and that the original publication in this journal is cited, in accordance with accepted academic practice. No use, distribution or reproduction is permitted which does not comply with these terms.
*Correspondence: Wenzhi Zeng, emVuZ3dlbnpoaTE5ODlAd2h1LmVkdS5jbg==; Chang Ao, YW9jaGFuZ0B3aHUuZWR1LmNu