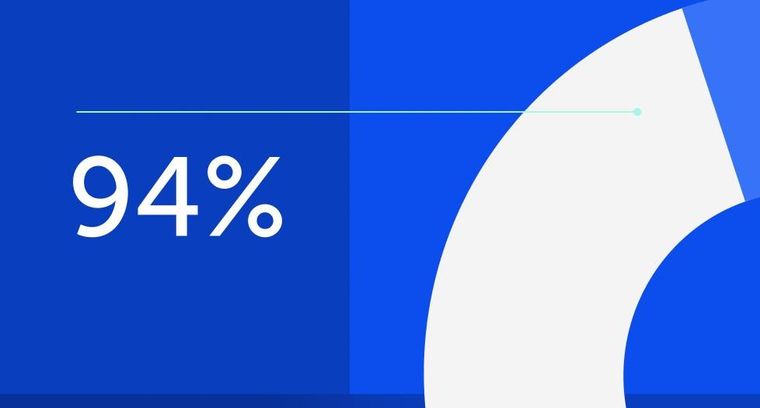
94% of researchers rate our articles as excellent or good
Learn more about the work of our research integrity team to safeguard the quality of each article we publish.
Find out more
ORIGINAL RESEARCH article
Front. Plant Sci., 28 April 2022
Sec. Plant Abiotic Stress
Volume 13 - 2022 | https://doi.org/10.3389/fpls.2022.891117
This article is part of the Research TopicMechanisms of Plant-Aluminum Interactions in Acidic Soils, Volume IIView all 9 articles
Chinese fir (Cunninghamia lanceolata), which is an important coniferous tree species in China, is mainly planted in acidic soils with toxic aluminum (Al) levels. However, the consequences of Al toxicity and its resistance mechanism in Chinese fir remain largely uncharacterized. In this study, the Al-induced modification and possible role of cell wall in regulating Al tolerance in Chinese fir were investigated by using seedlings with contrasting Al tolerance, namely, Al-sensitive (YX02) and Al-resistant (YX01) genotypes. The results in present work showed that Al treatment resulted in a dose- and time-dependent inhibition of root growth and oxidative damage in both genotypes, but more in YX02 than in YX01. The severe oxidative damage observed in YX02 under Al stress was found to correlate with lower antioxidant enzyme activities as compared with YX01. The greater root growth inhibition observed in YX02 compared with YX01 was associated with a higher accumulation of Al in pectin and hemicllulose 1 (HC1) fraction because of the higher pectin and HC1 contents and the lower degree of pectin demethylation due to enhanced pectin methylesterase activity in YX02, which ultimately enhanced cell wall binding capacity for Al in YX02. Taken together, our results suggested that enhancement of antioxidant enzyme activities and cell wall modification-induced Al exclusion are the two mechanisms responsible for the Al tolerance of Chinese fir.
Acidic soils (pH < 5.5) are prevalent in many regions worldwide, with approximately 30% of the world’s ice-free land affected by excessive acidity (Brunner and Sperisen, 2013). Recently, the soil acidification problem has become more seriously due to misuse or excessive use of fertilizers as well as acid deposition (von-Uexkull and Mutert, 1995; Duan et al., 2016). Under acidic soil condition, plants may suffer from various toxicities, including aluminum (Al), iron (Fe), proton (H+) (Kochian et al., 2005; de Wit et al., 2010; Fang et al., 2016; Rao et al., 2016). Since Al ion (Al3+) is reactive, it can interact with multiple cell components (Singh et al., 2017). These interactions result in inhibited of cell division and expansion, decreased plasma membrane integrity, and disrupted signaling cascades, and consequent the root elongation and uptake of water and nutrient elements were inhibited (Matsumoto, 2000; Kochian et al., 2015), which ultimately lead to crop yield losses. Therefore, Al toxicity is recognized as the most serious constraining factors affecting crop production in acidic soils. To deal with Al toxicity, plants have evolved a number of strategies, which can be broadly ascribed to external and internal resistance mechanisms (Horst et al., 2010). The external mechanisms is achieved by secreting chelating agents, such as organic acids, Pi, or phenolic compounds from the root apex and the formation of non-toxic Al-chelating complexes in the rhizosphere to exclude Al from the roots (Kochian et al., 2015). In contrast, the internal mechanisms mainly involve the chelation of Al with organic substances in cells and then sequestration them into vacuoles (Wang et al., 2010, 2017; Chen et al., 2012; Zhou et al., 2014), or an enhancement of the cellular antioxidant capacities (Sun et al., 2014, 2015).
In addition to the above-mentioned mechanisms, recent evidence demonstrated that Al-induced alterations in cell wall properties and the associated Al exclusion are also critical for regulating plant Al tolerance (Zheng, 2014). For instance, Zhu et al. (2019a) showed that exogenous application of putrescine can significantly alleviate Al toxicity in rice (Oryza sativa) by lowering the Al content in the root cell wall via decreased pectin content and pectin methylesterases (PME) activity. The results of another recent study indicated that boron can reduce the apoplastic Al accumulation in trifoliate orange by decreasing the un-methylated pectin and hemicellulose (HC) contents and increasing pectin methylation, thereby decreasing the Al content in roots to prevent Al toxicity (Riaz et al., 2019). Moreover, a comparison of pea (Pisum sativum L.) cultivars with contrasting susceptibilities to Al toxicity revealed that the pectin content and PME activity are often greater in roots of Al-sensitive plants as compared with Al-resistant ones under Al stress (Li et al., 2016). Similar results also observed in buckwheat (Fagopyrum tataricum), it has been found that the higher retention of Al in roots of Al-sensitive cultivar “Liuku2” always accompanied with higher low-methyl-ester pectin content and PME activity (Yang et al., 2011a). In addition to pectin, there is accumulating evidence showed that the cell wall HC is also vital for regulating plant Al tolerance (Yang et al., 2011b; Zhu et al., 2017; Li et al., 2020). More specifically, Yang et al. (2008) found a negative correlation between HC content and Al accumulation in rice roots, the lower HC content could lead to a greater Al exclusion from roots in Al-resistant rice cultivar. Similarly, defects in the synthesis of xyloglucan, which is major component of HC, in the Arabidopsis mutant xth31 considerably enhances plant Al-tolerance by decreasing the HC content and inhibiting the accumulation of Al in the roots (Zhu et al., 2012b). The results of another study suggest that the O-acetylation of the xyloglucan in HC may affect the Al-binding capacity of HC, which affects Al sensitivity (Zhu et al., 2014). Therefore, these results imply that decreasing the cell wall Al content by modifying cell wall components and properties is also a major mechanism employed by plants under Al stress.
Presently, most of the studies related to Al toxicity in plants have focused on herbaceous species, with relatively few studies investigated the role of the cell wall in regulating Al toxicity in woody plant species, especially in coniferous trees (Ryder et al., 2003; Prabagar et al., 2011). Different plant species as well as diverse genotypes within the same species may respond differently to Al stress. Therefore, whether Al-induced cell wall modifications are critical for regulating the Al tolerance of coniferous trees remains far from clear. Chinese fir is an important coniferous tree species in southern China (Ma et al., 2007). Because of its substantial economical value, Chinese fir is now the primary tree species used for afforestation in China, wherein it is grown on more than over 24% of the artificial forest area (Zhang et al., 2004). However, most of the Chinese fir trees were planted in iron-rich aluminized acidic soils, making Al toxicity a major factor limiting tree growth and yield (Luo et al., 2018a,b). Unfortunately, the effects of Al toxicity and the associated defense responses of Chinese fir remain largely unknown. In this study, the Al-induced immobilization of Al in the cell wall and its underlying mechanisms were investigated in the root tips of two Chinese fir genotypes differentially tolerant to Al stress. Evidence presented in this work suggest that enhancing of antioxidant enzyme activities and the cell wall modification-induced Al exclusion from roots under Al stress are the two mechanisms employed by Chinese fir to increase its Al tolerance. To our knowledge, this is the first report to reveal the contribution of the cell wall to the Al resistance mechanism of Chinese fir.
This study was using two Chinese fir genotypes that differ regarding their Al tolerance [YX01 (Al-resistant) and YX02 (Al-sensitive)]. The seeds of Chinese fir were provided by the Chinese Fir Engineering Technology Research Center of National Forestry and Grassland Administration. The seeds were first rinsed with water (three times) to eliminate the empty and aborted seeds and other particles. the remaining seeds were surface-sterilized with a 0.3% potassium permanganate solution for 30 min and then washed with ultrapure water for six times. The sterilized seeds were soaked in warm water (50°C) and allowed to cool down to room temperature (25°C) for 24 h. The soaked seeds were germinated and the resulting seedlings were grown in 3 mM CaCl2 solution on cardboard filter paper for 30 days. Uniformly growing seedlings were selected and pre-cultured in full-strength nutrient solution (SNS) (pH adjusted to 5.5) in a culture incubator for another 30 days. The composition of the nutrient solution was described in our previous report (Ye et al., 2019). The seedlings were placed in a controlled environment growth room as previously described (Ye et al., 2019). After pre-culturing, the uniformly growing Chinese fir seedlings were transferred to different treatment solutions: 1/2 SNS (CK) or 1/2 SNS supplemented with 1 mM AlCl3 (Al treatment) and treated for 16 days. During the treatment period, the solutions pH was adjusted to 4.5. Additionally, the solutions were aerated with an air pump and refreshed every 2 days.
Two-month-old Chinese fir seedlings were cultured in the control (1/2 SNS, pH = 4.5) or Al treatment (1/2 SNS with 1 mM AlCl3, pH = 4.5) solutions for 16 days. At the start and end of the treatment period, the lengths of the primary roots of Chinese fir seedlings were measured with a ruler. The relative root elongation (%) of the seedlings was calculated by comparing the root elongation in the Al treatment solution with that in control solution.
Membrane lipid peroxidation was determined as described by Zhu et al. (2012a). Briefly, the content of malondialdehyde (MDA) was determined by thiobarbituric acid reaction.
The treated root tips (0-1 cm) of Chinese fir were harvested, after which the H2O2 content was determined according to the colorimetrical method as described by Jin et al. (2013). Briefly, the root tips of Chinese fir were ground in a mortar containing with ice-cold acetone and quartz sand. The ground material was centrifuged (12000 rpm for 10 min at 4°C) and the pelleted residue was discarded. A 1-ml aliquot of the supernatant was transferred into a 10-ml test tube, to which 1 ml 5% titanium sulfate solution and 2 ml 17 M ammonia solution were added into the test tube for forming precipitated. The precipitated material was collected and rinsed with ice-cold acetone for 3 times, and then dissolved in 5 ml 2 M H2SO4. The absorbance of the solution as well as a blank control solution was measured at 410 nm, after which the hydrogen peroxide content was calculated by the difference in the absorbance of the two solutions.
The root activity of Chinese fir was analyzed using triphenyl tetrazolium chloride (TTC) method. In brief, after treatment the roots of Chinese fir seedlings were rinsed with deionized water, then root tips (0.2 g) were collected and placed in 50-ml centrifuge tubes, 5 ml 0.4% TTC reagent and 5 ml 67 mM phosphatic buffer solution (pH = 7.0) were added to the tubes, which were then incubated at 37°C for 2 h in the darkness. The reaction was stopped by adding 2 ml of H2SO4 (1 M) into the tubes. After that the roots were transferred into mortar ground into homogenized and extracted with 5 ml ethyl acetate, the residue were rinsed with ethyl acetate for three times, then the red supernatant were pooled into 20 ml volumetric flask. Solution absorbance was detected at 485 nm against blanks. The blank solution was prepared using the same reagents, but order in which the reagents were added differed (i.e., H2SO4 added first and then the roots and the other reagents).
The preparation of cell wall was according to Ye et al. (2015). In brief, after treatment, the roots (0-10 mm) of Chinese fir was collected and rinsed with ultrapure water before they were ground in liquid nitrogen. Next, 10 mL of ethanol (75%) was added to the ground material, which was then incubated for 20 min. The sample was centrifuged at 10,000 × g for 20 min. The pelleted material was rinsed with acetone (for 15 min), then with methanol: chloroform = 1:1, v/v (for 15 min), at last rinsed with methanol (for 15 min). The final residues comprising the cell wall was collected, lyophilized, and stored in a refrigerator until analyzed. The pectin, hemicellulose 1 (HC1), and hemicellulose 2 (HC2) were fractionated as described in Yang et al. (2011b). The uronic acid content in the pectin, HC1, and HC2 was assayed by using a known galacturonic acid (GalA) calibration standard curve as described in previous (Blumenkrantz and Asboe-Hansen, 1973).
The Al contents in the root tips as well as in the cell wall and its fractions were analyzed as described by Yang et al. (2011b) and Zhu et al. (2017). Briefly, the Al contents in root tips (0–1 cm), cell wall and its fraction were extracted with 2 M HCl for 24 h. After extraction, the extract solutions were centrifuged at 15,000 × g for 10 min. The Al contents of the supernatants were determined by inductively coupled plasma optical emission spectrometry (ICP-OES).
At the end of Al treatment, root tips of Chinese fir were collected and extracted with 2 mL buffer (50 mM PBS, pH 7.0, 1 mM EDTA and 1% PVP) after homogenized with liquid nitrogen. The extract was centrifuged at 12,000 × g for 10 min under 4°C, then the supernatant was used for enzyme activity analysis as described by Sun et al. (2014, 2015).
For pectin methylesterase (PME) activity analysis, the root tips (0-10 mm) of Chinese fir were extracted with buffer solution (100 mM Tris and 1M NaCl, pH 7.5) for 1 h at 4°C. After a centrifugation at 15,000 × g for 15 min at 4°C, the pelleted material was discarded and the PME activity of the supernatant was measured as described by Zhu et al. (2012a).
The degree of pectin methylation was determined by calculating the molar ratio of methanol content to uronic acid content as described in Ye et al. (2015). Methanol content released from pectin was measured according to Ye et al. (2015), namely, pectin methyl ester in pectin fraction was hydrolyzed by adding 10 mL of 1 M KOH into 5 mL pectin solutions in a centrifuge tube (50 mL), and incubated for 40 min (25°C). Then the pectin hydrolysates solutions were neutralized with H3PO4 to give 20 mL volume. Isopyknic alcohol oxidase (1 UN⋅mL–1) solution was then added into above solutions and kept at 25°C for 15 min. Finally, fluoral-P (2 mL) was added and incubated at 60°C for 15 min before cooled down to 25°C and determined at 412 nm by spectrophotometer. The uronic acid content in the pectin was determined as described above.
Cell wall extraction was described as previous. Cell wall from CK and Al treatment were used for Al adsorption kinetics experiment. Before the experiment, the cell wall from Al-treated sample was reactivated by two 24-h treatments with 2 M HCl. After rinsing with deionized water, the reactivated cell wall was freeze-dried for further used. The cell wall Al adsorption kinetics experiment were carried out according to Zheng et al. (2004). In brief, the adsorption solution (0.5 mM CaCl2 and 20 μM AlCl3, pH 4.5) was run through a column filled with 20 mg of cell wall at a speed of 3 mL⋅15 min–1 by fraction collector, and then Al content in eluate was detected by ICP-OES.
Data were analyzed using the DPS software (version 9.5). Specifically, the data were subjected to a one-way analysis of variance and the means of each treatment were compared by the least significant difference test. Different letters on the histograms indicate statistically different at P < 0.05. * or ** above the lines indicate statistically different at P < 0.05 and P < 0.01, respectively.
Root growth inhibition is a typical symptom for plants under Al stress. Hence, root growth responses in Chinese fir were analyzed. Decreased root growth was observed for both genotypes as the Al treatment time increased (Figure 1A). When the exposure time exceeded 8 days, a significant difference in root elongation was detected between YX01 and YX02, and this difference became more evident as the exposure time increased to 16 days. However, Al exposure time longer 16 days did not lead to a further increase in the difference between YX01 and YX02. Similarly, the inhibitory effects of Al stress on root growth worsened as the Al concentration increased, with root growth inhibition reaching approximately 50% at 1000 μM Al (Figure 1B). Therefore, 1000 μM Al and 16 days of Al treatment period was employed in the following experiments.
Figure 1. Effects of different aluminum (Al) exposure time (A) and concentration (B) on relative root elongation of Chinese fir seedlings. Two-month-old Chinese fir seedlings were exposed to 1/2 strength complete nutrition solution without Al (CK), or with 1 mM AlCl3 (Al) for 24 d, or exposed to CK or different concentration Al for 16 d. Bars are means of four replicates ± SD. * or ** above lines represent significant differences at P < 0.05 and P < 0.01, respectively.
The Al treatment resulted in significantly increased of MDA and H2O2 contents in root tips of Chinese fir as compared with control (Figures 2A,B). Additionally, a more significantly higher accumulation of MDA and H2O2 contents was observed in Al-sensitive genotype YX02 when compared with Al-resistant genotype YX01. Moreover, the exposure to Al stress significantly decreased the root activity, with a greater decrease in YX02 than in YX01 (Figure 2C). Similar to the MDA and H2O2 contents, Al exposure resulted in a significantly increased Al accumulation in the roots of both genotypes, with a more significantly higher of Al content found in the roots of YX02 (Figure 2D).
Figure 2. Effects of Al treatment on malondialdehyde (MDA) content (A), hydrogen peroxide content (B), root activity (C) and Al content (D) in roots of Chinese fir seedlings. Two-month-old Chinese fir seedlings were exposed to 1/2 strength complete nutrition solution without Al (CK), or with 1 mM AlCl3 (Al) for 16 d. After treatment, root tips of Chinese fir were collected for analysis. Bars are means of four replicates ± SD. Different letters above histogram represent significant differences at P < 0.05. * or ** above lines represent significant differences between genotypes at P < 0.05 and P < 0.01, respectively. N.D. indicates not detected.
Plants commonly respond to Al stress by enhancing antioxidant enzyme activities to increase the scavenging of reactive oxygen species (ROS), thereby minimizing the effects of Al toxicity (Sun et al., 2015). In the current study, the catalase (CAT), superoxide dismutase (SOD), peroxidase (POD), and ascorbate peroxidase (APX) activities significantly increased in both genotypes following the Al treatment (Figure 3). Furthermore, the CAT, SOD, POD, and APX activities were substantially higher in the YX01 roots than in the YX02 ones (Figure 3). These results indicated that Chinese fir can initiate antioxidant-related defenses under Al stress to eliminate ROS. Moreover, the capacity to scavenge ROS is greater in YX01 than in YX02.
Figure 3. Effects of Al treatment on the activities catalase (CAT) enzyme (A), superoxide dismutase (SOD) enzyme (B), peroxidase (POD) enzyme (C), and ascorbate peroxidase (APX) enzyme (D) in roots of Chinese fir seedlings. Two-month-old Chinese fir seedlings were exposed to 1/2 strength complete nutrition solution without Al (CK), or with 1 mM AlCl3 (Al) for 16 d. After treatment, root tips of Chinese fir were collected for analysis. Bars are means of four replicates ± SD. Different letters above histogram represent significant differences at P < 0.05. * or ** above lines represent significant differences between genotypes at P < 0.05 and P < 0.01, respectively.
We further analyzed the Al content in the cell wall and its fractions because it is the major sites for Al accumulated in roots. Al was undetectable in the control. In contrast, the Al treatment significantly increased the cell wall Al content in both genotypes, but the Al content was significantly lower in YX01 than in YX02 (Figure 4A). A similar trend was observed when we analyzed the Al content in the cell wall polysaccharides. Consistent with the cell wall Al content, Al was not detected in the pectin, HC1, and HC2 of the control samples, whereas the Al treatment significantly increased the Al content in pectin, HC1 and HC2 (Figures 4B–D). More specifically, the Al content in pectin, HC1, and HC2 was, respectively, 38.12%, 49.09%, and 52.38% greater in YX02 than in YX01 (Figures 4B–D). We also found that Al was most abundant in pectin and HC1, implying these two cell wall components are the major Al-binding sites in Chinese fir roots.
Figure 4. Effects of Al treatment on Al content in cell wall (A), pectin (B), hemicellulose 1 (HC1) (C) and hemicellulose 2 (HC2) (D) of Chinese fir seedlings. Two-month-old Chinese fir seedlings were exposed to 1/2 strength complete nutrition solution without Al (CK), or with 1 mM AlCl3 (Al) for 16 d. After treatment, cell wall was extracted from root tips of Chinese fir and fraction into pectin, HC1, and HC2 for Al content determination. Bars are means of four replicates ± SD. Different letters above histogram represent significant differences at P < 0.05. * or ** above lines represent significant differences between genotypes at P < 0.05 and P < 0.01, respectively. N.D. indicates not detected.
Previous research revealed that the content cell wall polysaccharide determines the binding capacities of cell wall for metal ions. Accordingly, we analyzed the cell wall polysaccharide content. A significantly increased in pectin and HC1 contents was observed in roots of Chinese fir exposed to Al as compared with control. Additionally, the contents of pectin and HC1 in the roots of YX02 were significantly higher than that of YX01 (Figures 5A,B). Compared with the control samples, the exposure to Al stress also increased the HC2 content in the roots of both genotypes, although not significantly for YX01, However, the HC2 content was significantly higher in YX02 than in YX01 (Figure 5C).
Figure 5. Effects of Al treatment on uronic acid content in root tips of Chinese fir seedlings. Two-month-old Chinese fir seedlings were exposed to 1/2 strength complete nutrition solution without Al (CK), or with 1 mM AlCl3 (Al) for 16 d. After treatment, cell wall was extracted from root tips of Chinese fir and fraction into pectin (A), HC1 (B), and HC2 (C) for uronic acid measurement. Bars are means of four replicates ± SD. Different letters above histogram represent significant differences at P < 0.05. * or ** above lines represent significant differences between genotypes at P < 0.05 and P < 0.01, respectively.
The degree of pectin methyl-esterification (DM), due to PME activity is another important factor influencing metal ions accumulated in the cell wall. As shown in Figure 6A, the Al treatment leads to a significant decrease in the DM, relative to the control, especially in the Al-sensitive genotype YX02. The DM was 51.22% lower in the YX02 roots than in the YX01 roots following the Al treatment. Consistent with the decreased DM, the PME activity significantly increased in both genotypes treated with Al, with a greater increase in the Al-sensitive genotype (Figure 6B).
Figure 6. Effects of Al treatment on the degree of pectin methylesterification (A) and pectin methylesterase (B) in root tips of Chinese fir seedlings. Two-month-old Chinese fir seedlings were exposed to 1/2 strength complete nutrition solution without Al (CK), or with 1 mM AlCl3 (Al) for 16 d. After treatment, root tips of Chinese fir were collected for further analysis. Bars are means of four replicates ± SD. Different letters above histogram represent significant differences at P < 0.05. ** above lines represent significant differences between genotypes at P < 0.01, respectively.
Cell wall adsorption kinetics experiments were carried out by using cell wall from control and Al treatment of both genotypes to test whether the difference in cell wall Al accumulation both genotypes is associated with their difference in Al binding capacity. We found that the capacity of the cell wall to adsorb Al did not varied significantly between the two genotypes under control conditions. The Al treatment induced a considerable increase in the Al adsorption capacity of both genotypes. The cell wall of the Al-treated YX01 and YX02 roots adsorbed 324.85 μmol g–1 and 425.22 μmol g–1Al, respectively (Figure 7), suggesting Al-induced modifications in the cell wall enhanced the cell wall Al-binding capacity, resulting in enhanced Al accumulation in the cell wall.
Figure 7. Al adsorption kinetic of cell wall from Chinese fir roots. Two-month-old Chinese fir seedlings were exposed to 1/2 strength complete nutrition solution without Al (CK), or with 1 mM AlCl3 (Al) for 16 d. After treatment, root tips of Chinese fir were collected for cell wall extraction and analysis.
Root growth inhibition is recognized as one of the most typical symptoms of Al toxicity, which may resulted from inhibition of cell division and expansion, disruption of plasma membrane integrity (Silva et al., 2012; Liu et al., 2018). Therefore, Al-induced root growth inhibition is frequently used as an indicator of the Al tolerance of many plants, including wheat (Triticum aestivum L.) (Sun et al., 2020), Arabidopsis (Arabidopsis thaliana) (Lou et al., 2019), rice (Oryza sativa) (Zhu et al., 2017, 2019b), pea (Pisum sativum L.) (Li et al., 2016), trifoliate orange [Poncirus trifoliata (L.) Raf.] (Riaz et al., 2019), lettuce (Lactuca sativa) (Chen et al., 2020), and even coniferous trees (Hirano et al., 2012). In the present study, therefore, the variations in root growth under Al stress between two Chinese fir genotypes with contrasting Al tolerances were compared. Consistent with previous findings, in our time- and concentration-dependent Al-exposure experiments, Al treatment significantly restricted root elongation in both genotypes (Figure 1). Moreover, the genotypes responded differently to Al stress in terms of relative root elongation, with root elongation inhibited significantly more in the Al-sensitive genotype YX02 (Figure 1). There is accumulating evidence that Al-induced root growth inhibition is closely related with the Al accumulated in roots (Yu et al., 2015; Liu et al., 2018). For instance, Yu et al. (2015) proved that increasingly severe wheat root growth inhibition under Al stress conditions is accompanied by the accumulation of Al in root tips. Additionally, Liu et al. (2018) demonstrated that exacerbated root elogation inhibition under Al exposure mainly resulted from increased Al accumulation in root tips. Similar observations have also been reported for rice (Zhu et al., 2017) and Arabidopsis (Lou et al., 2019). Consistent with the greater inhibition of root growth in the Al-sensitive genotype YX02, the Al content was higher in YX02 than in the Al-resistant genotype YX01 (Figure 2D). Hence, the results of the current and previous studies suggest that in herbaceous plants as well as in coniferous tree species, such as Chinese fir, relative root elogation may be useful for distinguishing among genotypes with varying sensitivities to Al stress. Moreover, our results further confirmed previous findings and imply that Al-induced root growth inhibition is related to increased Al accumulation in root tips (Liu et al., 2018). The ROS-induced oxidative damage is a typical consequence of an exposure to excessive amounts of metals, including Al (Xu et al., 2012; Jin et al., 2013; Sun et al., 2015; Liu et al., 2018; Chen et al., 2020). Thus, oxidative damage is often used as a criterion for the occurrence of Al toxicity. Therefore, the H2O2 content (i.e., a typical ROS) and oxidative damage were analyzed in Chinese fir. In this study, the higher MDA content resulting from the greater accumulation of H2O2 in the YX02 roots than in the YX01 roots indicated the oxidative damage was more severe in of the Al-sensitive genotype than in the Al-resistant genotype (Figures 2A,B). It has been reported that antioxidant enzyme is vital for regulating oxidative damage in plants under Al stress (Xu et al., 2012). Increasing evidence showed that differences in response to oxidative damage responsible for plant difference in Al tolerance (Sun et al., 2014, 2015). For example, Yu et al. (2019) showed that enhancing antioxidant enzyme activities ameliorated Al resistance in both wheat cultivars, and a more prominent increased were observed in Al-tolerant one. Similar results also found in maize (Giannakoula et al., 2010). In this study, the severe oxidative damage observed in YX02 is associate with the significantly lower CAT, SOD, POD, and APX activities in YX02 than in YX01 under Al stress conditions (Figure 3), further confirmed previous results. Hence, above results imply that the more serious Al-induced oxidative damage observed in YX02 than in YX01 is due to a lower ROS scavenging capacity, which consequently increases H2O2 levels, thus leading to serous oxidative stress that restricts root growth (Liu et al., 2018).
In most cases, the Al accumulated in Al-stressed roots is associated with the binding of Al by the cell wall, which is the major Al-binding site in roots. Clarkson (1967) reported that 85%–90% of the Al accumulating in roots of barley (Hordeum vulgare L.) is located in the cell wall. Similarly, Liu et al. (2019) found that the Al accumulated in the cell walls of rice and wheat plants with different genotypes represents 64.9%–91.6% of the total Al content in the roots. Binding of Al to cell wall may destroy its structure, thus reduce cell wall extensibility. Indeed, previous study demonstrated that disorganized distribution of homogalacturonan (major component of cell wall pectin) epitopes is responsible for Al-induced root elongation inhibition (Li et al., 2009). Therefore, the cell wall is critical for regulating Al tolerance. Recent evidence suggests that pectin and HC are the two major fractions of cell wall responsible for binding of metal ions, including Al, iron and cadmium (Yang et al., 2011b; Zhu et al., 2014; Xu et al., 2015; Sun et al., 2016). In the current study, we found that pectin and HC1 make a great contribution to Al accumulation in cell wall as indicated by large portion of Al accumulated cell wall was found in these two components (Figure 4). Furthermore, the Al levels in the cell wall, pectin, HC1, and HC2 were greater in the roots of the Al-sensitive genotype YX02 than in the roots of the Al-resistant genotype YX01 (Figure 4). Accordingly, the Al accumulated in the pectin and HC fractions contribute greatly to the difference in the Al tolerance between Chinese fir genotypes. Generally, the binding capacity of cell wall for metal ions is related to the abundance of pectin and HC. For example, Eticha et al. (2005) reported that an Al treatment of maize (Zea mays L.) results in an increase in the root Al content of all cultivars, with a more prominent increased of Al were found in Al-sensitive cultivars due to higher accumulation of pectin in the roots. Similar results also reported for buckwheat, the results showed that the more significant accumulation of Al in roots of Al-sensitive cultivar “Liuku2” is associated with the higher increase and proportion of low-methylated pectin in its roots (Yang et al., 2011a). Additionally, Zhu et al. (2012b) proved that in the Arabidopsis mutant xth31, defects in xyloglucan synthesis substantially enhance plant Al-resistance because of a decrease in the cell wall HC content, which inhibits the ability of the roots to retain Al. Consistent with these earlier findings, we observed that an exposure to Al stress significantly increased the pectin and HC1 contents of both examined Chinese fir genotypes, although the increase was considerably more in the Al-sensitive genotype YX02 (Figure 5). This observation was in accordance with the increased Al content in the pectin and HC of YX02 (Figure 4). Therefore, these results are in agreement with previous findings, and imply that Al-induced increases in pectin and HC contents contribute greatly to the accumulation of Al in the cell wall and its genotypic difference in Al tolerance. In addition to the pectin and HC contents, the DM is another factor influencing the capacity of the cell wall to bind Al. Specifically, a lower DM can increase the number of carboxylic groups, which are potential serve as Al-binding sites. An earlier investigation revealed that the higher retention of Al in the cell wall of Al-sensitive rice cultivar is because of an increase in PME activity and a decrease in the DM, ultimately leading to enhanced plant sensitive to Al (Yang et al., 2008). Sun et al. (2016) also showed that in Al-sensitive wheat plants, eliminating the Al-induced accumulation of endogenous nitric oxide (NO) generation by a NO scavenger greatly decreased Al accumulation in cell wall via decreased PME activity and increased DM. Other studies have provided evidence of a negative correlation between the cell wall Al content and the DM (Eticha et al., 2005; Zhang et al., 2011; Yu et al., 2015; Sun et al., 2016). Therefore, the greater retention of Al in the YX02 cell wall than in the YX01 cell wall is likely associated with a decrease in the cell wall DM under Al stress conditions (Figure 6A). Pectin demethylation is reportedly regulated by PME activity (Xu et al., 2015). Consistent with decrease in the DM in both analyzed Chinese fir genotypes under Al stress, the Al treatment significantly increased the PME activity, especially in YX02 (Figure 6B). Considered together, these results suggest that the greater retention of Al in the cell wall of the Al-sensitive genotype YX02 is likely because of an increase in the pectin and HC contents, an enhancement of PME activity, and a decrease in the DM of the cell wall. Several reports have confirmed the metal ion-binding capacity of cell walls can be altered by modifying the cell wall under abiotic stress conditions. For instance, the Cd adsorption capacity of Arabidopsis plants increases in a phosphorus-deficient environment because of a decrease in the pectin and HC1 contents. Similarly, Ye et al. (2015) found that in tomato (Solanum lycopersicum), the application of exogenous NO enhances the ability of the cell wall to adsorb Fe by decreasing the cell wall DM via increased PME activity. In an earlier study, we proved that the Fe-binding capacity of the cell wall under Cd stress conditions increases as the pectin and HC contents increase and the DM decreases (Xu et al., 2015). In a recent study, Yang et al. (2019) observed that manganese (Mn) stress enhances the Mn-binding capacity of the cell wall by increasing the pectin content and PME and decreasing the DM in Mn-sensitive genotype of sugarcane (Saccharum officinarum L.). These results further support the importance of the regulatory effects of cell wall modifications on the capacity to bind metal ions. In line with these findings, we observed that an exposure to Al stress increasing the pectin and HC contents and the PME activity, while decreasing the DM in YX02, all of which enhance the ability of the cell wall to bind Al. These changes help to explain the greater adsorption of Al by the YX02 cell wall than by the YX01 cell wall (Figure 7).
In conclusion, the results of this study indicate that the differences in the Al tolerance of Chinese fir genotypes are associated with variations in Al-induced cell wall modifications and antioxidant enzyme activities. In response to toxic Al levels, the Al-sensitive genotype YX02 significantly increases its pectin and HC contents along with its PME activity, thereby substantially decreasing the cell wall DM, which enhances the ability of the cell wall to retain Al. Consequently, the Al-induced roots growth inhibition is exacerbated. Furthermore, the limited Al-induced stimulation of antioxidant enzymes in YX02 increases the ROS accumulation, resulting in severe oxidative stress.
The original contributions presented in the study are included in the article/supplementary material, further inquiries can be directed to the corresponding authors.
SX: data collection, methodology, analysis, and writing. LW, LL, MZ, and YT: data collection and analysis. GC and KL: writing original draft. YY: conceptualization, writing original draft, analysis, methodology, and funding acquisition. All authors contributed to the article and approved the submitted version.
This work was financially supported by the National Natural Science Foundation of China (31700535 and 32071754), National Natural Science Foundation of Fujian province (2021J01057), Forestry Peak Discipline Construction Project of Fujian Agriculture and Forestry University (FAFU) (71201800738).
The authors declare that the research was conducted in the absence of any commercial or financial relationships that could be construed as a potential conflict of interest.
All claims expressed in this article are solely those of the authors and do not necessarily represent those of their affiliated organizations, or those of the publisher, the editors and the reviewers. Any product that may be evaluated in this article, or claim that may be made by its manufacturer, is not guaranteed or endorsed by the publisher.
We thank Liwen Bianji, Edanz Editing China for editing the English text of this manuscript.
Blumenkrantz, N., and Asboe-Hansen, G. (1973). New method for quantitative determination of uronic acids. Anal. Biochem. 54, 484–489. doi: 10.1016/0003-2697(73)90377-1
Brunner, I., and Sperisen, C. (2013). Aluminum exclusion and aluminum tolerance in woody plants. Front. Plant Sci. 4:172. doi: 10.3389/fpls.2013.00172
Chen, Q., Wu, K.-H., Wang, P., Yi, J., Li, K.-Z., Yu, Y.-X., et al. (2012). Overexpression of MsALMT1, from the aluminum-sensitive Medicago sativa, enhances malate exudation and aluminum resistance in tobacco. Plant Mol. Biol. Rep. 31, 769–774. doi: 10.1007/s11105-012-0543-2
Chen, Y., Huang, L., Liang, X., Dai, P., Zhang, Y., Li, B., et al. (2020). Enhancement of polyphenolic metabolism as an adaptive response of lettuce (Lactuca sativa) roots to aluminum stress. Environ. Pollut. 261:114230. doi: 10.1016/j.envpol.2020.114230
Clarkson, D. T. (1967). Interactions between aluminium and phosphorus on root surfaces and cell wall material. Plant Soil 27, 347–356. doi: 10.1007/BF01376328
de Wit, H. A., Eldhuset, T. D., and Mulder, J. (2010). Dissolved Al reduces Mg uptake in Norway spruce forest: results from a long-term field manipulation experiment in Norway. For. Ecol. Manag. 259, 2072–2082. doi: 10.1016/j.foreco.2010.02.018
Duan, L., Yu, Q., Zhang, Q., Wang, Z., Pan, Y., Larssen, T., et al. (2016). Acid deposition in Asia: emissions, deposition, and ecosystem effects. Atmos. Environ. 146, 55–69. doi: 10.1016/j.atmosenv.2016.07.018
Eticha, D., Stass, A., and Horst, W. J. (2005). Cell-wall pectin and its degree of methylation in the maize root-apex significance for genotypic differences in aluminium resistance. Plant Cell Environ. 28, 1410–1420. doi: 10.1111/j.1365-3040.2005.01375.x
Fang, X. Z., Tian, W. H., Liu, X. X., Lin, X. Y., Jin, C. W., and Zheng, S. J. (2016). Alleviation of proton toxicity by nitrate uptake specifically depends on nitrate transporter 1.1 in Arabidopsis. New Phytol. 211, 149–158. doi: 10.1111/nph.13892
Giannakoula, A., Moustakas, M., Syros, T., and Yupsanis, T. (2010). Aluminum stress induces up-regulation of an efficient antioxidant system in the Al-tolerant maize line but not in the Al-sensitive line. Environ. Exp. Bot. 67, 487–494. doi: 10.1016/j.envexpbot.2009.07.010
Hirano, Y., Frey, B., and Brunner, I. (2012). Contrasting reactions of roots of two coniferous tree species to aluminum stress. Environ. Exp. Bot. 77, 12–18. doi: 10.1016/j.envexpbot.2011.10.007
Horst, W. J., Wang, Y., and Eticha, D. (2010). The role of the root apoplast in aluminium-induced inhibition of root elongation and in aluminium resistance of plants: a review. Ann. Bot. 106, 185–197. doi: 10.1093/aob/mcq053
Jin, C. W., Mao, Q. Q., Luo, B. F., Lin, X. Y., and Du, S. T. (2013). Mutation of mpk6 enhances cadmium tolerance in Arabidopsis plants by alleviating oxidative stress. Plant Soil 371, 387–396. doi: 10.1007/s11104-013-1699-8
Kochian, L. V., Piñeros, M. A., and Hoekenga, O. A. (2005). The physiology, genetics and molecular biology of plant aluminum resistance and toxicity. Plant Soil 274, 175–195. doi: 10.1007/s11104-004-1158-7
Kochian, L. V., PiñEros, M. A., Liu, J., and Magalhaes, J. V. (2015). Plant adaptation to acid soils the molecular basis for crop aluminum resistance. Annu. Rev. Plant Biol. 66, 571–598. doi: 10.1146/annurev-arplant-043014-114822
Li, C. X., Yan, J. Y., Ren, J. Y., Sun, L., Xu, C., Li, G. X., et al. (2020). A WRKY transcription factor confers aluminum tolerance via regulation of cell wall modifying genes. J. Integr. Plant Biol. 62, 1176–1192. doi: 10.1111/jipb.12888
Li, X., Li, Y., Qu, M., Xiao, H., Feng, Y., Liu, J., et al. (2016). Cell wall pectin and its methyl-esterification in transition zone determine Al resistance in cultivars of Pea (Pisum sativum). Front. Plant Sci. 7:39. doi: 10.3389/fpls.2016.00039
Li, Y. Y., Yang, J. L., Zhang, Y. J., and Zheng, S. J. (2009). Disorganized distribution of homogalacturonan epitopes in cell walls as one possible mechanism for aluminium-induced root growth inhibition in maize. Ann. Bot. 104, 235–241. doi: 10.1093/aob/mcp123
Liu, Q., Wang, Z. Y., Wu, K., Xing, C. H., Chang, H., Rao, Y. C., et al. (2019). Comparison of aluminum accumulation ability of cell wall polysaccharides in Oryza sativa and Triticum aestivum root tips under aluminum toxicity. Plant Sci. J. 37, 513–520.
Liu, W., Xu, F., Lv, T., Zhou, W., Chen, Y., Jin, C., et al. (2018). Spatial responses of antioxidative system to aluminum stress in roots of wheat (Triticum aestivum L.) plants. Sci. Total Environ. 627, 462–469. doi: 10.1016/j.scitotenv.2018.01.021
Lou, H. Q., Fan, W., Jin, J. F., Xu, J. M., Chen, W. W., Yang, J. L., et al. (2019). A NAC-type transcription factor confers aluminium resistance by regulating cell wall-associated receptor kinase 1 and cell wall pectin. Plant Cell Environ. 43, 463–478. doi: 10.1111/pce.13676
Luo, H., Chen, X., Shi, L., Cao, G., Lin, S., and Ye, Y. (2018a). Response of anti-oxidative system in leaves of different Al-tolerant Cunninghamia lanceolata to aluminum stress. Acta Bot. Bor. Occid. Sin. 38, 1306–1314.
Luo, H., Shi, L., Wang, F., Chen, X., Cao, G., Lin, S., et al. (2018b). Effects of low phosphorus and aluminum toxicity coupled stress on ascorbate-glutathione cycle in leaves of Cunninghamia lanceolata. Chin. J. Trop. Crops 39, 873–880.
Ma, X., Heal, K. V., Liu, A., and Jarvis, P. G. (2007). Nutrient cycling and distribution in different-aged plantations of Chinese fir in southern China. For. Ecol. Manag. 243, 61–74. doi: 10.1016/j.foreco.2007.02.018
Matsumoto, H. (2000). Cell biology of aluminum toxicity and tolerance in higher plants. Int. Rev.Cytol. 200, 1–46. doi: 10.1016/S0074-7696(00)00001-2
Prabagar, S., Hodson, M. J., and Evans, D. E. (2011). Silicon amelioration of aluminium toxicity and cell death in suspension cultures of Norway spruce (Picea abies (L.) Karst.). Environ. Exp. Bot. 70, 266–276. doi: 10.1016/j.envexpbot.2010.10.001
Rao, I. M., Miles, J. W., Beebe, S. E., and Horst, W. J. (2016). Root adaptations to soils with low fertility and aluminium toxicity. Ann. Bot. 118, 593–605. doi: 10.1093/aob/mcw073
Riaz, M., Yan, L., Wu, X., Hussain, S., Aziz, O., and Jiang, C. (2019). Boron supply maintains efficient antioxidant system, cell wall components and reduces aluminum concentration in roots of trifoliate orange. Plant Physiol. Biochem. 137, 93–101. doi: 10.1016/j.plaphy.2019.02.003
Ryder, M., Gérard, F., Evans, D. E., and Hodson, M. J. (2003). The use of root growth and modelling data to investigate amelioration of aluminium toxicity by silicon in Picea abies seedlings. J. Inorg. Biochem. 97, 52–58. doi: 10.1016/S0162-0134(03)00181-8
Silva, S., Rodriguez, E., Pinto-Carnide, O., Martins-Lopes, P., Matos, M., Guedes-Pinto, H., et al. (2012). Zonal responses of sensitive vs. tolerant wheat roots during Al exposure and recovery. J. Plant Physiol. 169, 760–769. doi: 10.1016/j.jplph.2012.01.011
Singh, S., Tripathi, D. K., Singh, S., Sharma, S., Dubey, N. K., Chauhan, D. K., et al. (2017). Toxicity of aluminium on various levels of plant cells and organism: a review. Environ. Exp. Bot. 137, 177–193. doi: 10.1016/j.envexpbot.2017.01.005
Sun, C., Liu, L., Yu, Y., Liu, W., Lu, L., Jin, C., et al. (2015). Nitric oxide alleviates aluminum-induced oxidative damage through regulating the ascorbate-glutathione cycle in roots of wheat. J. Integr. Plant Biol. 57, 550–561. doi: 10.1111/jipb.12298
Sun, C., Lu, L., Liu, L., Liu, W., Yu, Y., Liu, X., et al. (2014). Nitrate reductase-mediated early nitric oxide burst alleviates oxidative damage induced by aluminum through enhancement of antioxidant defenses in roots of wheat (Triticum aestivum). New Phytol. 201, 1240–1250. doi: 10.1111/nph.12597
Sun, C., Lu, L., Yu, Y., Liu, L., Hu, Y., Ye, Y., et al. (2016). Decreasing methylation of pectin caused by nitric oxide leads to higher aluminium binding in cell walls and greater aluminium sensitivity of wheat roots. J. Exp. Bot. 67, 979–989. doi: 10.1093/jxb/erv514
Sun, C., Lv, T., Huang, L., Liu, X., Jin, C., and Lin, X. (2020). Melatonin ameliorates aluminum toxicity through enhancing aluminum exclusion and reestablishing redox homeostasis in roots of wheat. J. Pineal Res. 68:e12642. doi: 10.1111/jpi.12642
von-Uexkull, H. R., and Mutert, E. (1995). Global extent, development and economic impact of acid soils. Plant Soil 171, 1–15. doi: 10.1007/s10535-012-0021-6
Wang, J., Hou, Q., Li, P., Yang, L., Sun, X., Benedito, V. A., et al. (2017). Diverse functions of multidrug and toxin extrusion (MATE) transporters in citric acid efflux and metal homeostasis in Medicago truncatula. Plant J. 90, 79–95. doi: 10.1111/tpj.13471
Wang, Q.-F., Zhao, Y., Yi, Q., Li, K. Z., Yu, Y. X., and Chen, L. M. (2010). Overexpression of malate dehydrogenase in transgenic tobacco leaves: enhanced malate synthesis and augmented Al-resistance. Acta Physiol. Plant. 32, 1209–1220. doi: 10.1007/s11738-010-0522-x
Xu, F. J., Li, G., Jin, C. W., Liu, W. J., Zhang, S. S., Zhang, Y. S., et al. (2012). Aluminum-induced changes in reactive oxygen species accumulation, lipid peroxidation and antioxidant capacity in wheat root tips. Biol. Plant. 56, 89–96. doi: 10.1007/s10535-012-0021-6
Xu, S. S., Lin, S. Z., and Lai, Z. X. (2015). Cadmium impairs iron homeostasis in Arabidopsis thaliana by increasing the polysaccharide contents and the iron-binding capacity of root cell walls. Plant Soil 392, 71–85. doi: 10.1007/s11104-015-2443-3
Yang, J. L., Li, Y. Y., Zhang, Y. J., Zhang, S. S., Wu, Y. R., Wu, P., et al. (2008). Cell wall polysaccharides are specifically involved in the exclusion of aluminum from the rice root apex. Plant Physiol. 146, 602–611. doi: 10.1104/pp.107.111989
Yang, J. L., Zhu, X. F., Peng, Y. X., Zheng, C., Li, G. X., Liu, Y., et al. (2011b). Cell wall hemicellulose contributes significantly to aluminum adsorption and root growth in Arabidopsis. Plant Physiol. 155, 1885–1892. doi: 10.1104/pp.111.172221
Yang, J. L., Zhu, X. F., Zheng, C., Zhang, Y. J., and Zheng, S. J. (2011a). Genotypic differences in Al resistance and the role of cell-wall pectin in Al exclusion from the root apex in Fagopyrum tataricum. Ann. Bot. 107, 371–378. doi: 10.1093/aob/mcq254
Yang, S., Yi, K., Chang, M. M., Ling, G. Z., Zhao, Z. K., and Li, X. F. (2019). Sequestration of Mn into the cell wall contributes to Mn tolerance in sugarcane (Saccharum officinarum L.). Plant Soil 436, 475–487. doi: 10.1007/s11104-019-03937-x
Ye, Y. Q., Jin, C. W., Fan, S. K., Mao, Q. Q., Sun, C. L., Yu, Y., et al. (2015). Elevation of NO production increases Fe immobilization in the Fe-deficiency roots apoplast by decreasing pectin methylation of cell wall. Sci. Rep. 5:10746. doi: 10.1038/srep10746
Ye, Y. Q., Luo, H. Y., Li, M., Zhang, J. J., Cao, G. Q., Lin, K. M., et al. (2019). Potassium ameliorates iron deficiency by facilitating the remobilization of iron from root cell walls and promoting its translocation from roots to shoots. Plant Soil 440, 507–521. doi: 10.1007/s11104-019-04111-z
Yu, Y., Jin, C., Sun, C., Wang, J., Ye, Y., Lu, L., et al. (2015). Elevation of arginine decarboxylase-dependent putrescine production enhances aluminum tolerance by decreasing aluminum retention in root cell walls of wheat. J. Hazard. Mater. 299, 280–288. doi: 10.1016/j.jhazmat.2015.06.038
Yu, Y., Zhou, W., Liang, X., Zhou, K., and Lin, X. (2019). Increased bound putrescine accumulation contributes to the maintenance of antioxidant enzymes and higher aluminum tolerance in wheat. Environ. Pollut. 252, 941–949. doi: 10.1016/j.envpol.2019.06.045
Zhang, X.-Q., Kirschbaum, M. U. F., Hou, Z., and Guo, Z. (2004). Carbon stock changes in successive rotations of Chinese fir (Cunninghamia lanceolata (lamb) hook) plantations. For. Ecol. Manag. 202, 131–147. doi: 10.1016/j.foreco.2004.07.032
Zhang, Z., Wang, H., Wang, X., and Bi, Y. (2011). Nitric oxide enhances aluminum tolerance by affecting cell wall polysaccharides in rice roots. Plant Cell Rep. 30, 1701–1711. doi: 10.1007/s00299-011-1078-y
Zheng, S. J. (2014). The role of cell wall in plant resistance to nutritional stresses and the underlying physiological and molecular mechanisms. Sci. Sin. 44, 334–341. doi: 10.1360/052014-49
Zheng, S. J., Lin, X., Yang, J., Liu, Q., and Tang, C. (2004). The kineticsof aluminum adsorption and desorption by root cell walls ofan aluminum resistant wheat (Triticum aestivum L.) cultivar. Plant Soil 261, 85–90. doi: 10.1023/B:PLSO.0000035576.71760.2b
Zhou, G., Pereira, J. F., Delhaize, E., Zhou, M., Magalhaes, J. V., and Ryan, P. R. (2014). Enhancing the aluminium tolerance of barley by expressing the citrate transporter genes SbMATE and FRD3. J. Exp. Bot. 65, 2381–2390. doi: 10.1093/jxb/eru121
Zhu, C. Q., Cao, X. C., Bai, Z. G., Zhu, L. F., Hu, W. J., Hu, A. Y., et al. (2019a). Putrescine alleviates aluminum toxicity in rice (Oryza sativa) by reducing cell wall Al contents in an ethylene-dependent manner. Physiol. Plant 167, 471–487. doi: 10.1111/ppl.12961
Zhu, C. Q., Cao, X. C., Zhu, L. F., Hu, W. J., Hu, A. Y., Abliz, B., et al. (2019b). Boron reduces cell wall aluminum content in rice (Oryza sativa) roots by decreasing H2O2 accumulation. Plant Physiol. Biochem. 138, 80–90. doi: 10.1016/j.plaphy.2019.02.022
Zhu, X. F., Shi, Y. Z., Lei, G. J., Fry, S. C., Zhang, B. C., Zhou, Y. H., et al. (2012b). XTH31, encoding an in vitro XEH/XET-active enzyme, regulates aluminum sensitivity by modulating in vivo XET action, cell wall xyloglucan content, and aluminum binding capacity in Arabidopsis. Plant Cell 24, 4731–4747. doi: 10.1105/tpc.112.106039
Zhu, X. F., Lei, G. J., Jiang, T., Liu, Y., Li, G. X., and Zheng, S. J. (2012a). Cell wall polysaccharides are involved in P-deficiency-induced Cd exclusion in Arabidopsis thaliana. Planta 236, 989–997. doi: 10.1007/s00425-012-1652-8
Zhu, X. F., Sun, Y., Zhang, B. C., Mansoori, N., Wan, J. X., Liu, Y., et al. (2014). TRICHOME BIREFRINGENCE-LIKE27 affects aluminum sensitivity by modulating the O-acetylation of xyloglucan and aluminum-binding capacity in Arabidopsis. Plant Physiol. 166, 181–189. doi: 10.1104/pp.114.243808
Keywords: aluminum, Chinese fir, cell wall, pectin methylation, pectin, hemicellulose
Citation: Xu S, Wu L, Li L, Zhong M, Tang Y, Cao G, Lin K and Ye Y (2022) Aluminum-Induced Alterations to the Cell Wall and Antioxidant Enzymes Involved in the Regulation of the Aluminum Tolerance of Chinese Fir (Cunninghamia lanceolata). Front. Plant Sci. 13:891117. doi: 10.3389/fpls.2022.891117
Received: 07 March 2022; Accepted: 11 April 2022;
Published: 28 April 2022.
Edited by:
Jian Li Yang, Zhejiang University, ChinaReviewed by:
Heqiang Lou, Zhejiang Agriculture and Forestry University, ChinaCopyright © 2022 Xu, Wu, Li, Zhong, Tang, Cao, Lin and Ye. This is an open-access article distributed under the terms of the Creative Commons Attribution License (CC BY). The use, distribution or reproduction in other forums is permitted, provided the original author(s) and the copyright owner(s) are credited and that the original publication in this journal is cited, in accordance with accepted academic practice. No use, distribution or reproduction is permitted which does not comply with these terms.
*Correspondence: Kaimin Lin, bGtteXhAMTYzLmNvbQ==; Yiquan Ye, eWV5aXF1YW4wMDhAMTYzLmNvbQ==
†These authors have contributed equally to this work
Disclaimer: All claims expressed in this article are solely those of the authors and do not necessarily represent those of their affiliated organizations, or those of the publisher, the editors and the reviewers. Any product that may be evaluated in this article or claim that may be made by its manufacturer is not guaranteed or endorsed by the publisher.
Research integrity at Frontiers
Learn more about the work of our research integrity team to safeguard the quality of each article we publish.