- 1Department of Agronomy and Plant Breeding, Faculty of Agriculture, Shahrood University of Technology, Semnan, Iran
- 2Department of Plant Production and Genetics, Faculty of Agriculture, Urmia University, Urmia, Iran
Salinity-induced stress is widely considered a main plant-growth-limiting factor. The positive effects of melatonin in modulating abiotic stresses have led this hormone to be referred to as a growth regulator in plants. This study aims to show how melatonin protects fenugreek against the negative effects of salt stress. Different amounts of melatonin (30, 60, and 90 ppm), salinity stress (150 mM and 300 mM), and the use of both salinity and melatonin were used as treatments. The results showed that applying different melatonin levels to salinity-treated fenugreek plants effectively prevented the degradation of chlorophyll a, chlorophyll b, total chlorophyll, and carotenoid contents compared with salinity treatment without melatonin application. Besides, melatonin increases the biosynthesis of enzymatic and non-enzymatic antioxidants, thereby adjusting the content of reactive oxygen species, free radicals, electrolyte leakage, and malondialdehyde content. It was observed that applying melatonin increased the activity of potassium-carrying channels leading to the maintenance of ionic homeostasis and increased intracellular water content under salinity stress. The results revealed that melatonin activates the defense signaling pathways in fenugreek through the nitric oxide, auxin, and abscisic acid-dependent pathways. Melatonin, in a similar vein, increased the expression of genes involved in the biosynthesis pathway of diosgenin, a highly important steroidal sapogenin in medical and food industries, and hence the diosgenin content. When 150 mM salinity stress and 60 ppm melatonin were coupled, the diosgenin concentration rose by more than 5.5 times compared to the control condition. In conclusion, our findings demonstrate the potential of melatonin to enhance the plant tolerance to salinity stress by stimulating biochemical and physiological changes.
Introduction
Plants normally face various biotic and abiotic stresses during their growth period, which may affect the quality and quantity of crops and prevent the plants from reaching their maximum genetic potential to achieve high yields (Parihar et al., 2015; Pandey et al., 2017). Salinity stress is one of the most major issues restricting plant production across the globe, and it has devastating environmental consequences (Kumar and Saddhe, 2018; Isayenkov and Maathuis, 2019). It affects plant growth, development, and productivity via ion toxicity, poor water absorption, and hormonal disorders (Ahanger et al., 2017; Kumar et al., 2020). Developing the plant species which are more tolerant to this environmental stress would be one of the most advantageous approaches to dealing with the unavoidable damages arisen out of salinity (Nxele et al., 2017; Kumar et al., 2020). Achieving such goal by applying methods based on classical plant breeding or biotechnology generally requires a deep understanding of plants’ physiological changes in response of plants to salinity stress (Parihar et al., 2015; Hanin et al., 2016).
Fenugreek (Trigonella foenum-graecum) is a dicotyledonous annual plant belonging to the Fabaceae family, the subfamily of Papilionaceae, is commonly used not only as an edible vegetable but also as a raw material for some kinds of medicine. In terms of medicinal use, fenugreek is of great importance as it contains diosgenin (McAnuff et al., 2005; Wani and Kumar, 2018). This valuable compound, with a molecular formula of C27H42O3, generally forms an amorphous powder and benefits from a unique chemical and thermal stability under a wide range of conditions (Blunden and Rhodes, 1968). Diosgenin, as a valuable component of steroid drugs, such as testosterone, progesterone and glucocorticoids, could be quite helpful in treating cholesterol, hyperlipidemia, diabetes, metabolic diseases, cancer and aging (Upadhyay et al., 2014; Wani and Kumar, 2018). Two different pathways were suggested by the literature review for diosgenin production, including lanosterol (LAN) to cholesterol and cycloartenol to cholesterol pathways. It has been known that some key genes direct phytosterols biosynthesis pathways (He et al., 2008; Vaidya et al., 2013; Gas-Pascual et al., 2014; Cheng et al., 2021). The squalene, produced via converting two molecules of farnesyl pyrophosphate by Squalene synthase (SQS), is oxidized to oxidosqualene by Squalene epoxidase (SEP). Next, cycloartenol is generated via oxidization of oxidosqualene by cycloartenol synthase (CAS). Then, cholesterol and phytosterols such as sitosterol are formed by the activities of Δ24-reductase sterol- methyltransferase (SMT1), respectively. Finally, furostanol 26-O-beta-glucoside is deglycosidased by furostanol glycoside 26-O-beta-glucosidase (BGL) and transformed to diosgenin (He et al., 2008; Vaidya et al., 2013; Gas-Pascual et al., 2014). Eventually, after a few steps, furostanol glycoside 26-O-beta-glucosidase catalyzes the final step of diosgenin formation, which deglycosidases the furostanol 26-O-beta-glucoside to diosgenin (Figure 1; He et al., 2008; Vaidya et al., 2013; Gas-Pascual et al., 2014; Mohammadi et al., 2020).
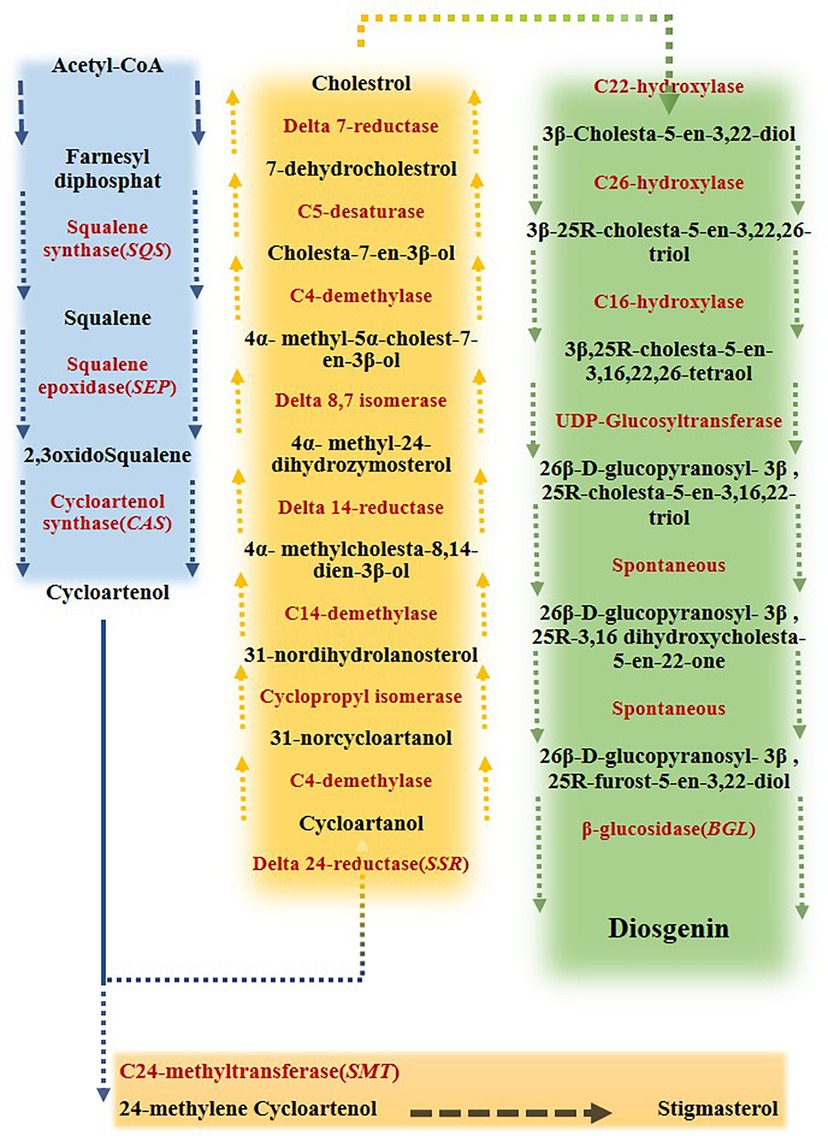
Figure 1. Biosynthesis pathway of diosgenin from farnesyl diphosphate in fenugreek. Acetyl-coenzyme A (acetyl-CoA) converts to cycloartenol via a series of multiple reactions. Cycloartenol converts to either cycloartenol, leading to cholesterol formation, or 24 methylene cycoartanol, leading to stigmasterol. This branch-point has a great influence on disogenin biosynthesis. Cholesterol then converts to 3ß -cholesta- 5-en- 3, 22-diol, which ultimately results in diosgenin production (Mohammadi et al., 2020).
Recent studies implied that the adverse impacts of abiotic stresses on plants might be largely modified through the exogenous application of various elicitors, like osmoprotectants (Wang et al., 2017; Estaji et al., 2019), phytohormones (Sytar et al., 2019; Rhaman et al., 2020), polyamines (Liu et al., 2016; El Amrani et al., 2019), and cold plasma (Ling et al., 2015; Ebrahimibasabi et al., 2020). Melatonin (N-acetyl-5-methoxytryptamine) is a natural antioxidant generated by all living species, including microbes, plants, and animals (Dubbels et al., 1995; Zhao et al., 2019). This chemical regulates flowering and seed germination, plant growth and development, photosynthesis, and enhancing stress tolerance in plants (Nawaz et al., 2016; Bose and Howlader, 2020).
Melatonin has been dubbed a “new growth regulator” in plants due to its favorable effects in moderating biotic and abiotic stresses (Arnao and Hernández-Ruiz, 2015; Al-Huqail et al., 2020; Bose and Howlader, 2020; Siddiqui et al., 2020a). Melatonin has a much higher antioxidant capacity than other antioxidants, which is probably related to its efficient transport via cell pores (Tan et al., 2002; Reiter et al., 2013). In addition to its antioxidant activity, the melatonin regulates the expression of many genes involved in physiological and biochemical processes (Siddiqui et al., 2019, 2020b; Bose and Howlader, 2020). Melatonin has been repeatedly mentioned as an important factor in improving plant response to drought (Ye et al., 2016; Li et al., 2018), salinity (Li et al., 2017a; Ren et al., 2020), cold stress (Yang et al., 2018b), herbicide protection (Park et al., 2012), ion toxicity (Yadu et al., 2018; Al-Huqail et al., 2020), and oxidative stress (Park et al., 2012).
In previous studies, the adverse effects of salinity stress on the quality and quantity of fenugreek were well established, although salinity has effectively increased the content of some important secondary metabolites, such as diosgenin and trigonelline in fenugreek (Sahari et al., 2016; Noohpisheh et al., 2021; Banakar et al., 2022). Environmental stresses on medicinal plants might vary from those on other plants, particularly agricultural crops. Although abiotic stress causes a decline in production in medicinal plants, the concentration of secondary metabolites, which are the most useful medicinal chemicals, eventually rises (Yang et al., 2018a; Li et al., 2020; Ogbe et al., 2020). In general, there are few reports on the melatonin effects on enhancing fenugreek tolerance under abiotic stresses and changing the secondary metabolites content, as well as the interaction effects of abiotic stresses and melatonin as two important elicitors on the secondary metabolite content in fenugreek (Xalxo and Keshavkant, 2019; Zamani et al., 2020). Current study aimed to assess the efficiency of melatonin in improving tolerance to salinity stress and its effect on the diosgenin content in fenugreek. Furthermore, identifying the optimal concentration of salinity and melatonin stress to increase the diosgenin content in fenugreek was another goal of this study.
Materials and methods
Plant materials and treatments
First, the seeds of fenugreek (Boshruyeh genotype) surface were sterilized in a 1% (v/v) sodium hypochlorite solution for 10 min, then the seeds were rinsed several times with sterile water and sown in the 25*35 cm pots containing a combination of peat moss, perlite, and sand in equal proportions in a growth chamber with a photoperiod of 16–8 h at a (day: night) temperature of 25–22°C with 60–70% humidity. In order to provide the plants with light, fluorescent lamps with a light intensity of the 400 μmol m−2 s−1 were used. The experimental treatments included control (the plants without melatonin and salinity) and the plants treated with different levels of melatonin (0, 30, 60, and 90 ppm), salinity (0, 150, and 300 mM), and the simultaneous use of salinity and melatonin. These concentrations were selected based on preliminary experiments. To prepare the melatonin solutions, the solute was dissolved in ethanol and then, distilled water was used to dilute the compound [ethanol/water (v/v) = 1/10000]. After establishing the establishment of seedlings at the three-leaf stage, various doses of melatonin (30, 60, and 90 ppm) were put to the irrigation water and added to the pots for 7 days. Then, a combination of ethanol and distilled water in a ratio of 1 in 10,000 was used to irrigate the control group (no melatonin). Next, 15 days of salinity treatments were administered. The plants were randomly divided into 12 groups: control, control plus 30, 60, and 90 ppm melatonin, salinity stress (150 mM), salinity stress (300 mM), salinity stress (150 mM) plus melatonin (30,60, and 90 ppm), and salinity stress (300 mM) plus melatonin (30,60, and 90 ppm). Salt and melatonin solution were added to each pot exactly equal. Finally, leaf samples (5 week old plants) were collected and stored in a freezer at −80°C. Melatonin and NaCl used in this study were purchased from Sigma-Merck Company (EC number: 200–797-7, CAS number: 73–31-4; EC number: 231–598-3, CAS number: 7647-14-5, respectively). According to the treatments applied, this study was conducted as a factorial in a completely randomized design with three replications.
Pigments measurement
First, 500 mg of leaf tissue was thoroughly ground with liquid nitrogen and mixed with 10 ml of dimethyl sulfoxide (Arnon, 1967). After heating the solutions in a water bath at 75°C for 4 h, the samples were centrifuged at 3000 rpm for 30 min at 4°C. Lastly, by using a spectrophotometer (UV-1800; Shimadzu Corporation, Kyoto, Japan), the amount of light absorption of supernatant was identified at wavelengths of 480, 649 and 665 nm and the traits were calculated based on the following formulas:
Relative water content
According to approach developed by Kirnak et al. (2001), with the aim of measuring relative water content, the fresh weight of five selected leaves from each plant were measured. After keeping the leaves immersed in distilled water for a whole night at 4°C with low light, the plant samples were transferred and kept in an oven for 24 h. By determining the weight of the entire dried plant leaves, their relative water content was calculated based on the following formula:
In this formula, Wf is fresh leaf weight, Wt is swollen leaf weight and Wd is leaf dry weight.
Soluble protein content and antioxidant activities
To extract the enzymatic solutions, 250 mg of finely crushed plant material (in a mortar with liquid nitrogen) was added to falcon tubes containing 2.5 ml of phosphate buffer (pH = 7.0, 50 mM) each. After vortexing the samples, they were centrifuged at 1500 rpm for 15 min at 4°C. The supernatant was transferred to separate tubes and was used to measure the activity of catalase (CAT), ascorbate peroxidase (APX), guaiacol peroxidase (GPX), superoxide dismutase (SOD), polyphenol oxidase (POD) and soluble protein. Total protein was measured by the Bradford (1976) method using a spectrophotometer (UV-1800; Shimadzu Corporation, Kyoto, Japan). Catalase activity was measured according to Scebba et al. (1998), which was based on the breakdown of hydrogen peroxide by the catalase enzyme. The activity of superoxide dismutase was measured spectrophotometrically (UV-1800; Shimadzu Corporation, Kyoto, Japan) and based on its inhibitory ability to photochemically reduce the nitrobluetetrazolium (NBT) at a wavelength of 560 nm (Kono, 1978). The activity of guaiacol peroxidase was measured at 470 nm (Drotar et al., 1985). The activity of ascorbate peroxidase and polyphenol oxidase was measured according to Ranieri et al. (2000), and Gauillard et al. (1993), respectively. Due to the reaction between ascorbate peroxidase and ascorbic acid and hydrogen peroxide, dehydro ascorbate is produced, which is read at a wavelength of 290 nm. The mixing compound contains 950 μl of 0.1 ml EDA, 1600 μl of 50 ml phosphate buffer (pH = 7), 400 μl of ascorbic acid 0.5 mM, 10 μl of hydrogen peroxide (30%) and 50 μl of antioxidant enzyme extract.
Electrolyte leakage
The unimpaired leaves were identified and separated from all treatments, and using the punching machines, the squared leaves (1*1 cm) were achieved and were subjected to 10 ml of distilled water in the falcon tubes. Then, the mixtures were well shaken for 30 min and by applying an EC meter (Weilheim, Germany) at a temperature fixed at 25°C, EC values (Ec1) were recorded. The falcon tubes and their contents were then kept in a water bath with a temperature of 90°C for 60 min prior to measuring their electrical conductivity (Ec2; Hepburn et al., 1986). Finally, using the mentioned method, the damage index was calculated based on the following formula:
Measurement of lipid peroxidation based on MDA
To analyze lipid peroxidation, 250 mg of ground leaf tissue (in a mortar with liquid nitrogen) was accurately weighted and then subjected to 5 ml of 50 mM potassium phosphate buffer (pH = 7.2). After vortexing, the solution was centrifuged at 3000 rpm for 15 min. Next, the supernatant and 0.5% thiobarbituric solution comprising 20% trichloroacetic acid (2 ml) was blended. The falcon tubes were exposed to a water bath at a temperature of 90°C for 30 min, immediately followed by an ice bath for the same time. The absorbance at 532 and 600 nm was measured using a spectrophotometer (UV-1800; Shimadzu Corporation, Kyoto, Japan; Stewart and Bewley, 1980) after 15 min of centrifugation at 3000 rpm. Using the following equation, the quantity of malondialdehyde was calculated:
In this formula, QD = Cuvette diameter (1 cm), QF = Extinction coefficient (155 mmol/cm) and DF = Dilution factor (20).
Total phenol and flavonoid content
By subjecting the plant samples to a temperature of 40°C prior to pulverization, they were thoroughly dried. After that, the samples were subjected to10 ml of 80% methanol per gram, and the achieved solution was shaken for a whole night. Then, the solutions were passed via a filter paper and the content of phenol and total flavonoid in the final extract was determined (McDonald et al., 2001). A mixture of 0.5 ml of folin–ciocalteu reagent (10%) and 4 ml of Na2CO3 solution (1 M) was blended with the 0.5 ml of plant extract, kept at room temperature for 15 min, and was measured using a spectrophotometer (UV-1800; Shimadzu Corporation, Kyoto, Japan) at 765 nm. Three replications were applied for all samples and standard curve of gallic acid (Merck, CAS Number: 149–91-7, EC Number: 205–749-9) at concentrations of 0, 20, 40, 60, 80, 100, 120 and 140 mg/l was used to determine the content of total phenol. Based on the aluminum chloride colorimetric approach, the total flavonoid content was measured. For this purpose, 400 μl of 2% aluminum chloride in liquid form was thoroughly mixed with 100 μl of sample solution. After introducing 1,200 μl of potassium acetate solution to the mixture and keeping it at 37°C for 40 min, the absorbance of the samples was recorded at 415 nm (Quettier-Deleu et al., 2000). The samples were analyzed for their flavonoid content by plotting the standard curve of rutin (Merck, CAS Number: 250249–75-3, EC Number: 205–814-1) at concentrations of 0, 20, 40, 60, 80, 100, 120, and 140 mg/l.
Total sugar content
The powdered leaf tissue was treated with 1.5 ml of 80% ethanol, vortexed for 10 min, and centrifuged at 3000 rpm for 15 min, and the supernatants were kept at 50°C to dry the existing alcohol. Prior to the second centrifugation, the dried samples were subjected to 10 ml of distilled water, 0.5 ml of normal barium hydroxide, and 0.5 ml of 5% zinc sulfate. 2 ml of the resultant supernatant was harvested and treated by 1 ml of 5% phenol and 5 ml of 98% sulfuric acid (V/V). After incubating the solutions at room temperature for 45 min, a spectrophotometer (UV-1800; Shimadzu Corporation, Kyoto, Japan) was applied to record the absorbance at 485 nm (Schlegel, 1956).
Nitric oxide content
The instructions suggested by Zhou et al. (2005) were followed in determining nitric oxide content. Moreover, 0.5 g of finely ground leaf tissue was subjected to 3 ml of 50 mM cold acetic acid containing 4% zinc acetate (pH = 4). After performing the centrifugation at 3000 rpm for 15 min, the supernatant was harvested and washed with 1 ml of extraction buffer and underwent another round of centrifugation under the mentioned conditions. After thoroughly combining the supernatant with 0.1 g of activated charcoal, it was filtered. In the last stage, 1 ml of grease reagent was added to 1 ml of the solution, followed by 30 min of incubation at room temperature. At 540 nm, the adsorption rate of the solution was measured. NO content was determined with the NaNO2 standard curve (Sigma, USA, CAS Number: 7632-00-0, EC Number: 231–555-9).
H2O2 content
First, 5 ml of 0.1% trichloroacetic acid (TCA) was well mixed with 0.5 g of the leaves, which were powdered in liquid nitrogen. The obtained mixture was subjected to centrifugation at 3500 rpm for 15 min. Then, the mixture was treated with 750 μl of supernatant, 750 μl of 10 mM potassium phosphate buffer (pH = 7.2), and 1.5 ml of potassium iodide (1 M), and the adsorption rate was recorded using a spectrophotometer (UV-1800; Shimadzu Corporation, Kyoto, Japan) at 390 nm. Finally, H2O2 content was plotted and measured using standard (Sigma, USA, CAS Number: 7722-84-1) curves (Loreto and Velikova, 2001).
Endogenous melatonin content
To measure melatonin, 2 g of powdered leaves were treated with 10 ml of methanol and then ultrasonicated at 25°C for 35 min. After performing centrifugation at 4000 rpm at 4°C for 15 min, the supernatant was harvested and dried using liquid nitrogen. Then, the obtained powder was subjected to 2 ml of 0.5% methanol, and then passed via an 18C solid phase extraction column which was previously activated with methanol and water. After that, the resulting solution was passed via a 0.2 μm filter (Z290823, Sigma-Aldrich, Taufkirchen, Germany) and underwent analysis using an HPLC column (Agilent Technologies Inc., USA, 1200 series, C18, 4.6 μm, 250 mm length, 5 mm diameter) at a wavelength of 280 nm to determine the melatonin content. The HPLC system consisted of a reversed-phase Nucleosil-100 C18 column with the integrated precolumn guard, an isocratic HPLC pump. Consideration was given to the mobile phase, which consisted of acetonitrile, Na2HPO4/H3PO4 buffer (15:85, pH = 4.5; Pothinuch and Tongchitpakdee, 2011), and a 1 ml/min flow rate. The volume injection and retention time were 20 μl and approximately 10 min, respectively. To determine the sample’s melatonin concentration, the sample peak area was compared to the melatonin standard curve (Sigma, USA, CAS Number: 73–31-4, EC Number: 200–797-7). The samples were dissolved in methanol and then placed in ultrasonic to prepare 50, 100, 150, 300, 500, 750, and 1,000 ppm of melatonin. The correlation coefficient (R2) was calculated 0.985 for the data sets.
Auxin content
For this purpose, 1.5 g of powdered leaves were thoroughly homogenized in 20 ml of an extract consisting of water and methanol in equal proportion. After 15 min of centrifugation at 3000 rpm, the solution was put in a freeze dryer to be chilled and evaporated, and 1 ml of 80% methanol was added. Prior to HPLC analysis (Agilent Technologies Inc., USA, 1200 series), the reconstituted eluate was filtered through a 0.45 m Whatman glass microfiber filter to remove impurities. The HPLC column was heated to 30°C with a 0.45% formic acid: acetonitrile gradient [0–5 min, 95:5% (v/v); 5–6 min, 95:5% to 0:100% (v/v); 6–16 min, 0:100% (v/v); Cao et al., 2006]. Eventually, the auxin concentration in leaf tissue was determined at the flow rate of 0.8 ml/min using an HPLC column (C18, 4.6 μm, 250 mm length, 5 mm diameter). The volume injection and retention were 20 μl and approximately 9 min, respectively. Peak spiking, retention time, and spectral properties were applied to identify the peak position. Furthermore, a linear regression equation of standard (Sigma, USA, CAS Number: 87–51-4, EC Number: 201–748-2) calibration curves was applied to determine the auxin concentration. The correlation coefficient (R2) was calculated 0.99 for the six different concentrations that were evaluated. The target component was quantified by the peak areas at the maximum wavelength of 260 nm.
Content of abscisic acid
First, 2.0 g of fresh leaves were well ground and subjected to 1 ml of a solution composed of methanol, ethyl acetate, and acetic acid at the rate of 1:50:49. Then, 20 mg of butylated hydroxytoluene (BTH) was introduced to this solution as antioxidant (Hubick and Reid, 1980). The solution was then passed via a 0.45 μm Whatman glass microfiber filter and by adding distilled water, the final volume was raised to 100 ml. Under vacuum and at a temperature of 35°C, a rotary apparatus was applied with the aim of evaporating the solvent (Hubick and Reid, 1980). Then, the samples were exposed to 10 ml of 50 mM hydrogen phosphate (pH = 7) followed by filtration. After adjusting the pH to 4.2 (Dobrev and Kaminek, 2002). 10 ml of ethyl acetate was added to the solution. The solution was then treated with 0.1 g of sodium sulfate to dehydrate the abscisic acid-containing ethyl acetate solution. After adding 5 ml of methyl chloride to the dried extract in order to evaporate it, the solutions were incubated at room temperature for 24 h. Finally, the extract was combined with 300 l of a 1% acetic acid-containing methanol solution and filtered. A HPLC column (Agilent Technologies Inc., USA, 1200 series, C18, 4.2 μm, 250 mm length, and 5 mm diameter) was applied with an interval of 4 to 5 min based on standard abscisic acid to determine the concentration of this important compound. In chromatographic separation stage, two mobile phases, including a medium comprised of water/acetonitrile/formic (A) acid in the volume ratio of 94.9:5:0.1, respectively, and another medium comprised of the same materials in the volume ratio of 10:89.9:0.1 (B), respectively, were applied. The elution program maintained 100% A for 5 min, followed by two consecutive linear gradients from 0 to 6% B in 10 min, and from 6 to 100% B in 5 min, and finally 100% B maintained for another 5 min (Dobrev and Kaminek, 2002). A flow rate of 0.8 ml/min was applied at a wavelength of 254 nm. The volume injection and retention were 20 μl and approximately 11 min, respectively. Finally, quantification was carried out given the specific area of the peak in relation to the retention time of the standard abscisic acid sample. ABA standard was obtained from Sigma, USA (CAS Number: 21293–29-8, EC Number: 244–319-5). The correlation coefficient (R2) was calculated 0.98 for the six different concentrations that were evaluated. The target component was quantified by the peak areas at the maximum wavelength of 260 nm.
Cl− content
A total of 6 ml of a mixture of HNO3: HCl (5:1 V/V) was added to 0.2 g of the finely ground plant samples and well homogenized. It aimed at evaporating the free acid in the solution, the digested materials were kept at 95°C for 5 h in a water bath. Using ion-free distilled water, the solutions were passed through a filter paper to a volume of 50 ml (Sagner et al., 1998). Finally, utilizing an atomic absorption spectrometer (105,924 GBC sensAA), Cl− concentration was determined.
Potassium and sodium content
The plant leaves were dried at 70°C for 48 h, and 0.1 g of the powdered leaves was accurately weighted. Then, 10 ml of glycolic acetic acid (0.1 N) was added to the plant materials, and the falcons were left at ambient temperature for 24 h. Prior to filtering the samples, they were kept at 70°C for 2 hours. Using a flame photometer (Shannon, Co. Clare, Ireland, PFP7 incl), the filtered extract was used to determine the concentration of the specified components in the investigated plant (Isaac and Kerber, 1971).
RNA extraction and cDNA synthesis
The RNA extraction process was carried out by following the manufacturer’s instructions accurately and utilizing an RNeasy plant mini kit (Qiagen). To remove DNA contamination, one microgram of RNA extract was treated with DNase I (Thermo Fisher Company). Furthermore, the RNA was assessed qualitatively and quantitatively applying 1% agarose gel and Nanodrop. Besides, 20 microliters of cDNA were synthesized using iScript cDNA synthesis kit (Bio-Rad, Hercules, CA, USA) according to the manufacturer’s instructions. The primers (Supplementary Table S1) were designed applying the Primer 3 Plus online program, and their accuracy was checked using Oligo Analyzer v.3.1.1
Real-time PCR reaction
For real-time PCR reaction, SYBR®Green PCR Master Mix 2X (Ampliqon) was applied. The reactions in question were conducted in a volume of 10 μl with three biological replications. Besides, a negative control (containing the all reaction components except for cDNA) was applied during the whole experiment to ensure that the samples were not contaminated. The mentioned reaction was conducted by applying 35 cycles of 95°C for 20 S, and 62°C for 40 S preceded by 95°C for 15 min.
Determining the diosgenin content
First, 1 g of powdered plant leaves was dissolved in 20 ml of 96% ethanol, and the mixture was thoroughly homogenized. Next, the solutions were ultrasonicated for 30 min and then, treated by 20 ml of sulfuric acid (2 N), and kept at 95°C for 2 h. Separating the solution ingredients by pure n-hexane was carried out three times. It aimed at neutralizing the acidic state, the solution was well washed with sodium hydroxide (1 M). The resulting solution was then washed with distilled water twice. Afterward, the n-hexane solution containing diosgenin was dried by subjecting it to a vacuum generated by a rotating equipment, and then dehydrated with 0.2 g of anhydrous sodium sulfate. After dissolving the obtained extract in acetonitrile, it was passed through a 0.22 μm Whatman glass microfiber filter (Zhou et al., 2010). The experimental conditions were isocratic binary system of acetonitrile: water (90:10). The process of determining the diosgenin content was carried out by utilizing a HPLC column (Agilent Technologies Inc., USA, 1200 series, C18, 4.2 μm, 250 mm length, 5 mm diameter) at the flow rate of 0.8 ml/min and the wavelength of 210 nm. Different concentrations of diosgenin (Sigma-Aldrich-Germany; CAS Number: 512049) including, 100, 150, 300, 500 and 1,000 ppm were produced by dissolving in acetonitrile prior to placing the samples in ultrasonic for 30 min. The volume injection and retention were 30 μl and approximately 23 min, respectively. Finally, the correlation coefficient (R2) was calculated 0.99 for the data sets.
Data analysis
Regarding the specific nature of the experimental data, the present study was carried out based on a factorial experiment in a completely randomized design. Using SPSS version 26 (IBM SPSS, Armonk, NY, USA),2 Duncan’s approach was used to do an analysis of variance with a comparison of means. At the 1% probability level, both the simple and combined effects of salinity and melatonin on all physiological and biochemical parameters were significant. Supplementary Table S2 displays the findings of a variance analysis of physiological and biochemical characteristics. Furthermore, melting and amplification curve analysis were accurately carried out to confirm the accuracy of the data before analyzing the expression data of the genes. To analyze the data obtained from real-time PCR, the relative expression of each gene was measured according to the relative standard curve method based on the formula 2−ΔΔCt (Livak and Schmittgen, 2001).
Results
The effects of melatonin on pigment and relative water contents under salt stress
The results showed that the contents of chlorophyll, carotenoids, and relative water significantly decreased (p < 0.01) under different salinity levels compared to the control. This reduction in chlorophyll a, chlorophyll b, total chlorophyll, carotenoids, and relative water contents at 300 mM salinity were 60, 56, 59, 55, and 53% respectively, compared to the control. Applying different levels of melatonin (especially, 30 and 60 ppm) in the absence of salinity stress led to a significant rise in these traits contents compared to the control (Table 1).
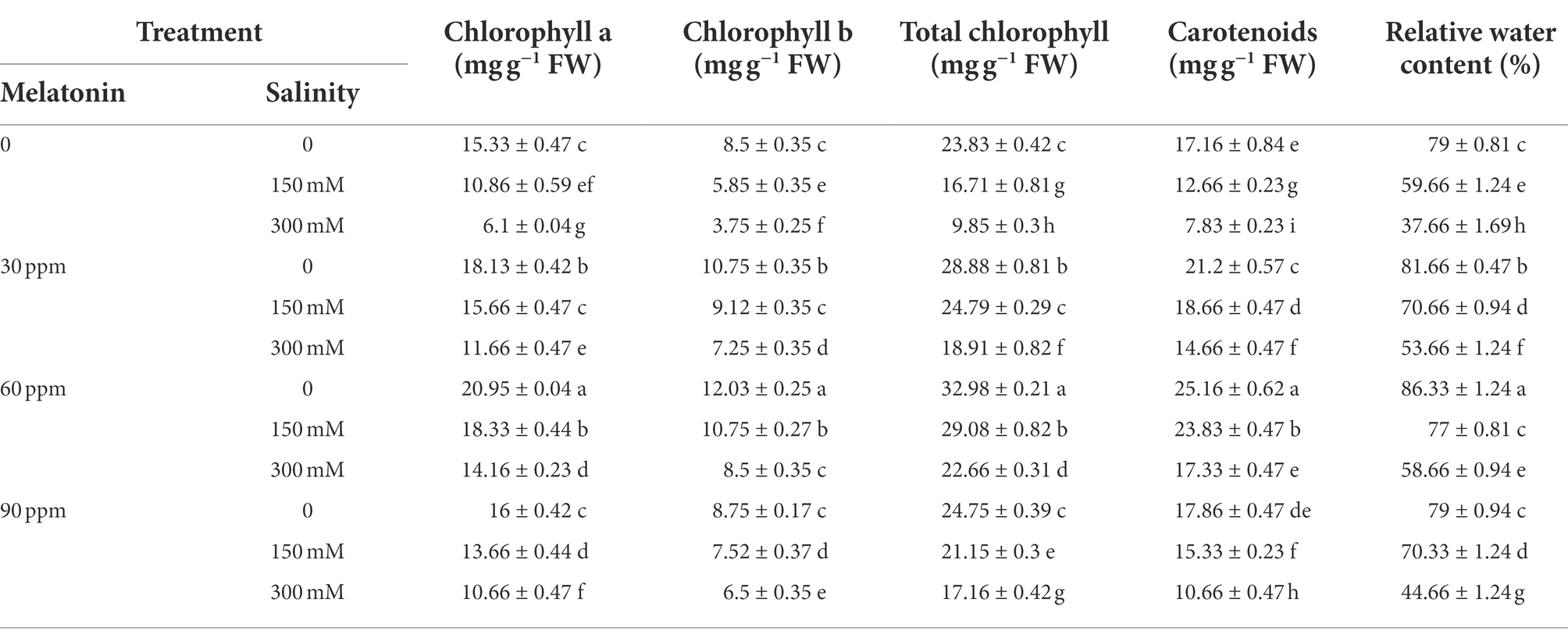
Table 1. The mean comparison effects of melatonin concentrations and different levels of NaCl on pigments and relative water content.
The highest chlorophyll a, chlorophyll b, total chlorophyll, carotenoid, and relative water contents were 20.94, 12.03, 32.97, 25.16 mg g−1 FW, and 86.33%, respectively under 60 ppm of melatonin without salinity. Besides, the application of different levels of melatonin along with different concentrations of salinity not only significantly prevented (p < 0.01) the reduction in the contents of these traits, but also enhanced their contents in relation to salinity treatment. The results showed that applying 60 ppm of melatonin to salinity-treated fenugreek plants (300 mM) effectively enhanced (p < 0.01) chlorophyll a (132%), chlorophyll b (126%), total chlorophyll (130%), carotenoid (121%), and relative water (56%) contents compared with salinity treatment without melatonin application (Table 1).
The effects of melatonin on antioxidant system under salt stress
Salinity stress, especially at the level of 300 mM, significantly raised (p < 0.01) the soluble protein content compared to the control. Application of 60 ppm melatonin in the absence of salinity substantially enhanced the soluble protein content by 40% compared to the control. Besides, the combination of varied salinity levels and melatonin led to a considerable increase (p < 0.01) in the amount of soluble protein compared to the salinity stress treatment. The greatest soluble protein content reached 518.33 mg ml−1 protein FW when 300 mM of salinity was paired with 60 ppm of melatonin, indicating a 298% increase in soluble protein content compared to the control (Table 2).
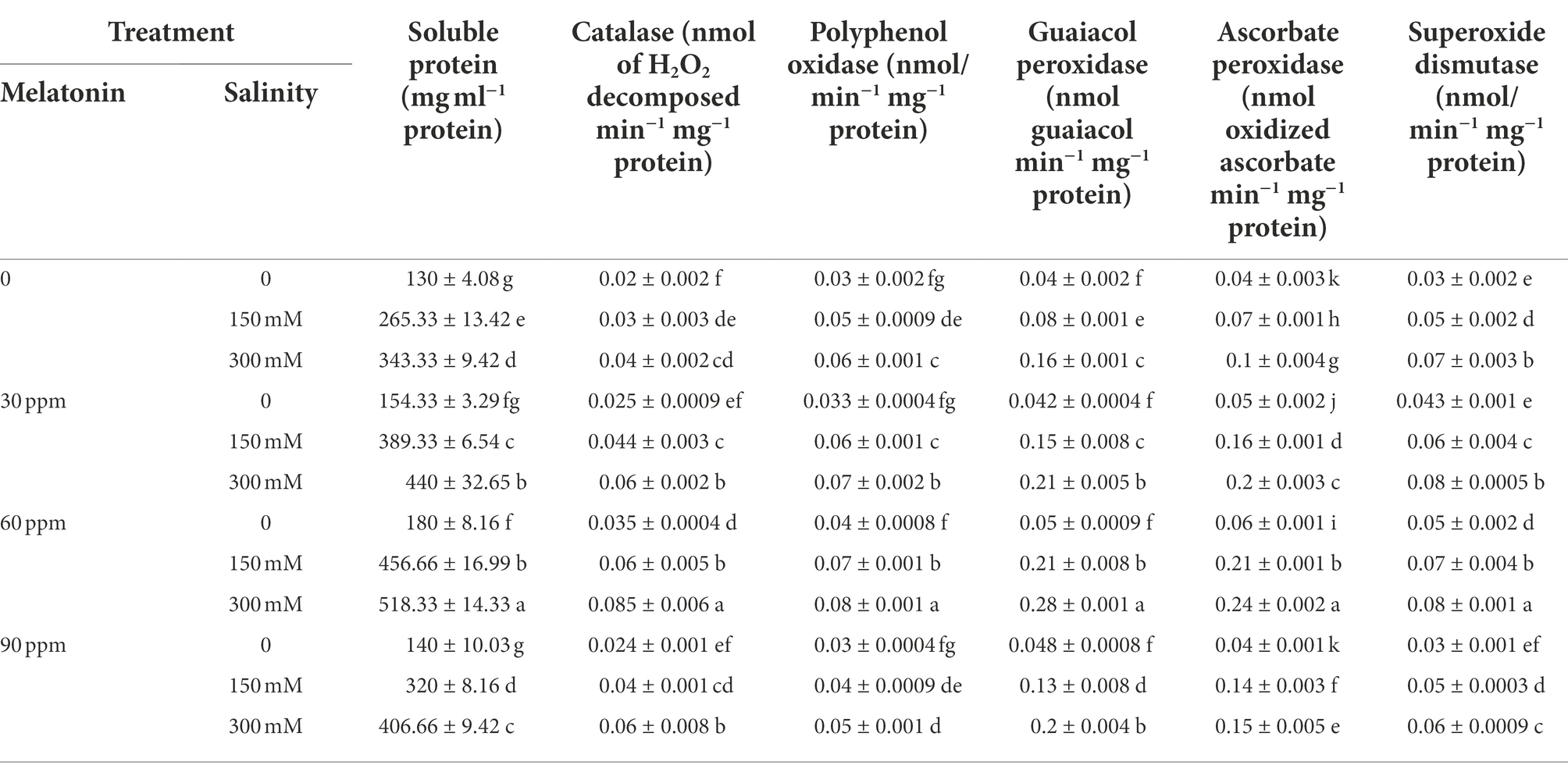
Table 2. The mean comparison effects of melatonin concentrations and different levels of NaCl on soluble protein content and antioxidant enzyme activities.
Compared to the control, catalase activity rose significantly (p < 0.01) as a function of the applied salinity level; whereas the application of different levels of melatonin had no significant effect on this parameter. Using different levels of melatonin along with different levels of salinity caused a very sharp rise in the activity of this enzyme. The highest activity of catalase reached 0.085 nmol of H2O2 decomposed min−1 mg−1protein by applying 60 ppm of melatonin and the salinity stress of 300 mM, which indicated a 325% increase in the activity of this enzyme compared to the control (Table 2).
The polyphenol oxidase activity under the salinity stress of 150 and 300 mM was enhanced (p < 0.01) by 66 and 100%, respectively, compared to the control; whereas the application of melatonin in the absence of salinity did not influence the activity of this enzyme. The highest polyphenol oxidase activity (0.08 nmol min−1 mg−1 protein); however, was observed in the treatment of 60 ppm melatonin and salinity of 300 mM (Table 1). Thus, using 30 and 60 ppm of melatonin with different salinity levels led to a substantial rise in polyphenol oxidase activity compared to the salinity treatment alone (Table 2).
Exposing the plants to salinity stress resulted in increased guaiacol peroxidase activity (p < 0.01). The application of different levels of melatonin; on the other hand, did not change the activity of this enzyme compared to the control. As far as, the addition of melatonin to the treatments came along with different salinity levels, a sharp rise in the activity of this enzyme was observed. The highest activity of guaiacol peroxidase (0.28 nmol guaiacol min−1 mg−1 protein) was recorded by applying 60 ppm of melatonin and the salinity stress of 300 mM (Table 2). The activity of this enzyme in the mentioned treatment increased by 600% compared to the control.
As a consequence of administering both salt stress and melatonin, ascorbate peroxidase activity significantly increased (p < 0.01) compared to the control. Additionally, simultaneous application of melatonin and salinity stress greatly increased the activity of this enzyme in comparison to salinity stress alone (without the use of melatonin). The highest activity of ascorbate peroxidase was 0.24 nmol oxidized ascorbate min−1 mg−1protein at salinity stress of 300 mM accompanied with 60 ppm of melatonin, which indicated a 500% increase in the activity of this enzyme compared to the control (Table 2).
Applying salinity stress notably raised (p < 0.01) the activity of the superoxide dismutase enzyme compared to the control. The activity of this enzyme under the salinity stress of 150 and 300 mM rose by 66 and 133%, respectively. Besides, a higher activity of superoxide dismutase (0.098 nmol min−1 mg−1 protein) was observed in the pots treated with 60 ppm of melatonin along with 300 mM of NaCl. Simultaneous application of 150 mM of NaCl and 30 and 60 ppm of melatonin as well as 300 mM of NaCl and 60 ppm of melatonin significantly increased the superoxide dismutase activity compared to the salinity stress alone (without the use of melatonin; Table 2).
The effects of melatonin on oxidative stress under salt stress
The electrolyte leakage index and malondialdehyde content after using 300 mM of salinity were enhanced by 77 and 160%, respectively. The highest and lowest amount of these two indicators were observed in salinity stress of 300 mM without the use of melatonin and in the treatment of 60 ppm of melatonin (without salinity stress), respectively (Table 3). Treating the plants grown under salinity stress (300 mM) with different levels of melatonin (especially 60 ppm) significantly reduced electrolyte leakage index and malondialdehyde content by 40 and 55% compared to the salinity stress condition (without the use of melatonin).
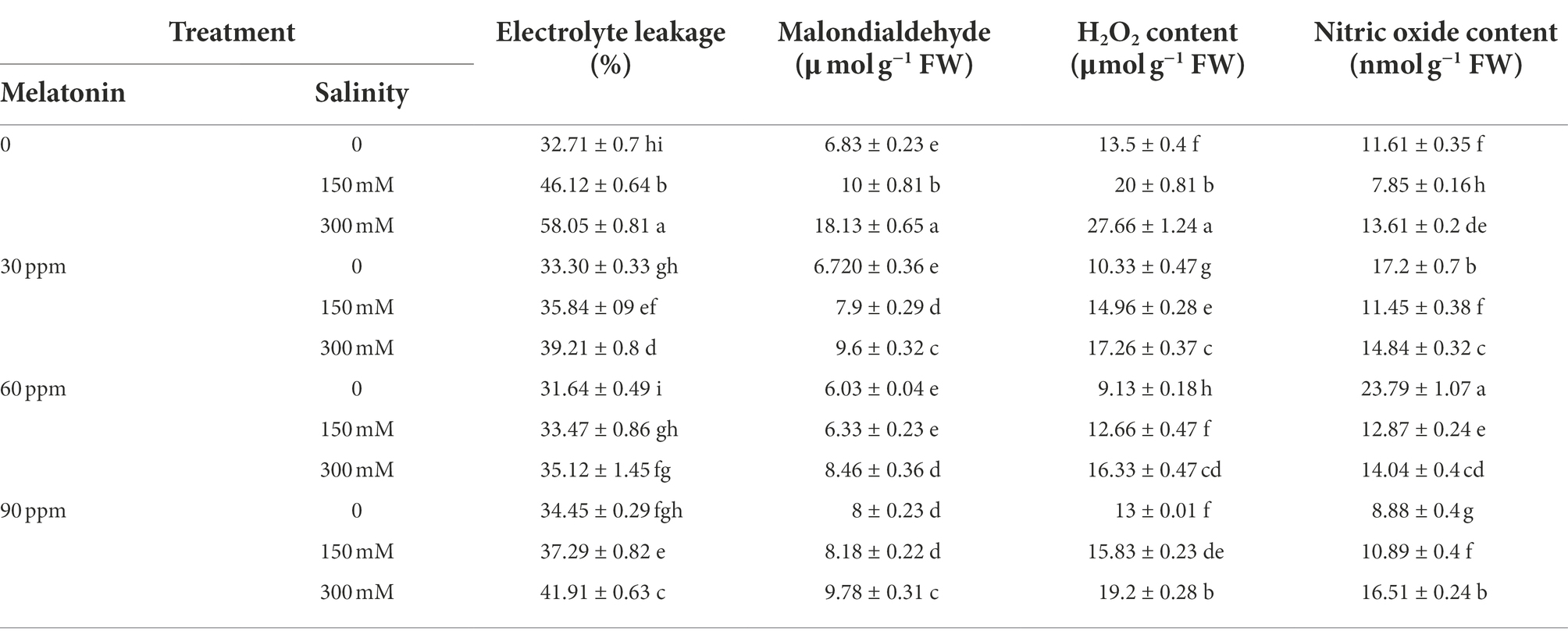
Table 3. The mean comparison effects of melatonin concentrations and different levels of NaCl on malondialdehyde content, electrolyte leakage index, H2O2, and nitric oxide content.
This study’s results showed a remarkable rise (17%; p < 0.01) in nitric oxide content under the influence of salinity stress (300 mM) compared to the control. Applying 30 and 60 ppm of melatonin led to 43 and 91% increases in the content of this trait compared to the control. The highest nitric oxide content (24 nmol g−1 FW) was obtained when applying 60 ppm of melatonin (Table 3). The combined use of different levels of melatonin and salinity stress (150 mM) considerably raised the nitric oxide content compared to the salinity treatment alone (150 mM without the use of melatonin).
The content of H2O2 rose (p < 0.01) remarkably following the application of different levels of salinity stress compared to the control. H2O2 content rose by 66 and 125% compared to the control after applying 150 and 300 mM of salinity, respectively. The use of different levels of melatonin significantly reduced the hydrogen peroxide content compared to the control. Moreover, using melatonin in plants treated with salinity significantly increased the content of this trait compared to plants treated with salinity alone. The highest amount of hydrogen peroxide was (28 μmol g−1 FW) recorded in 300 mM salinity treatment without the use of melatonin (Table 3).
The effects of melatonin on non-enzymatic antioxidant contents and sugar accumulation under salt stress
The flavonoid and total phenol content increased notably (p < 0.01) compared to the control as a function of the applied salinity levels. Moreover, applying different levels of melatonin without salinity enhanced these two traits compared to the control (Figure 2). Using 60 ppm of melatonin, the flavonoid and total phenol contents were enhanced by 133 and 75%, respectively. Different concentrations of melatonin applied to salt-stressed plants led to an increase in flavonoid and total phenol content compared to salinity stress conditions. The maximum levels of flavonoid and total phenol (40 and 46 mg g−1 FW) were seen when a salinity stress of 300 mM and 60 ppm of melatonin were simultaneously applied, however the levels of these compounds decreased dramatically when the melatonin dosage was increased to 90 ppm (Figure 2).
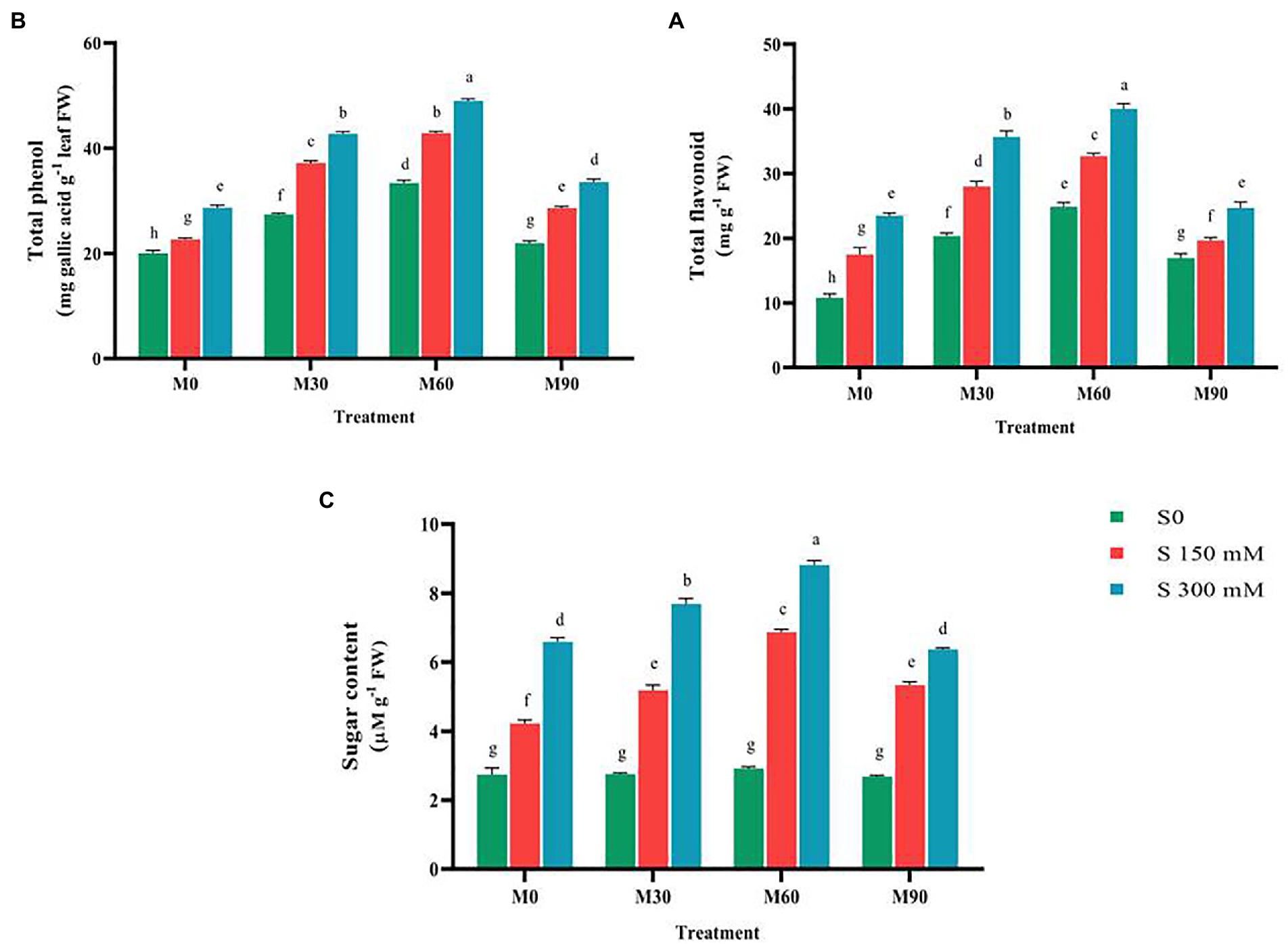
Figure 2. The mean comparison effects of melatonin concentrations and different levels of NaCl on (A) Total flavonoid, (B) Total phenol, (C) Sugar content. Duncan method at 1% probability level was applied to compare mean values. The columns having similar letters had no significant difference.
The salinity stress in a concentration-dependent manner caused a large increase (p < 0.01) in total sugar content compared to the control. This trait content, after using 150 and 300 mM of salinity, rose by 48 and 160%, respectively. Treating the plants with different levels of melatonin brought no significant change in total sugar content. Furthermore, the combined use of different levels of salinity and melatonin raised the total sugar content compared to the salinity treatments (without melatonin). The highest value (8.5 μM g−1 FW) of this trait was recorded under salinity treatment of 300 mM and melatonin level of 60 ppm, which indicated a 290% increase in the total sugar content compared to the control (Figure 2).
The effects of melatonin on hormonal profile contents under salt stress
Compared to the control, endogenous melatonin content rose (p < 0.01) significantly under the effect of various degrees of salinity stress, with the changes being regulated by the administered salinity levels. Endogenous melatonin concentration was increased by 250 and 500% at salinity levels of 150 and 300 mM, respectively. Treating the plants with melatonin enhanced the endogenous melatonin content in plants compared to the control. The endogenous melatonin content after the use of 30, 60 and 90 ppm of melatonin reached to 4, 7 and 6.5 nmol g−1 FW. Salinity stress accompanied with different levels of melatonin raised the content of endogenous melatonin compared to the salinity stress alone. The highest content of endogenous melatonin (28 nmol g−1 FW) was observed in salinity stress at 300 mM and melatonin at 60 ppm (Figure 3), which indicated a 760% increase in the total sugar content compared to the control.
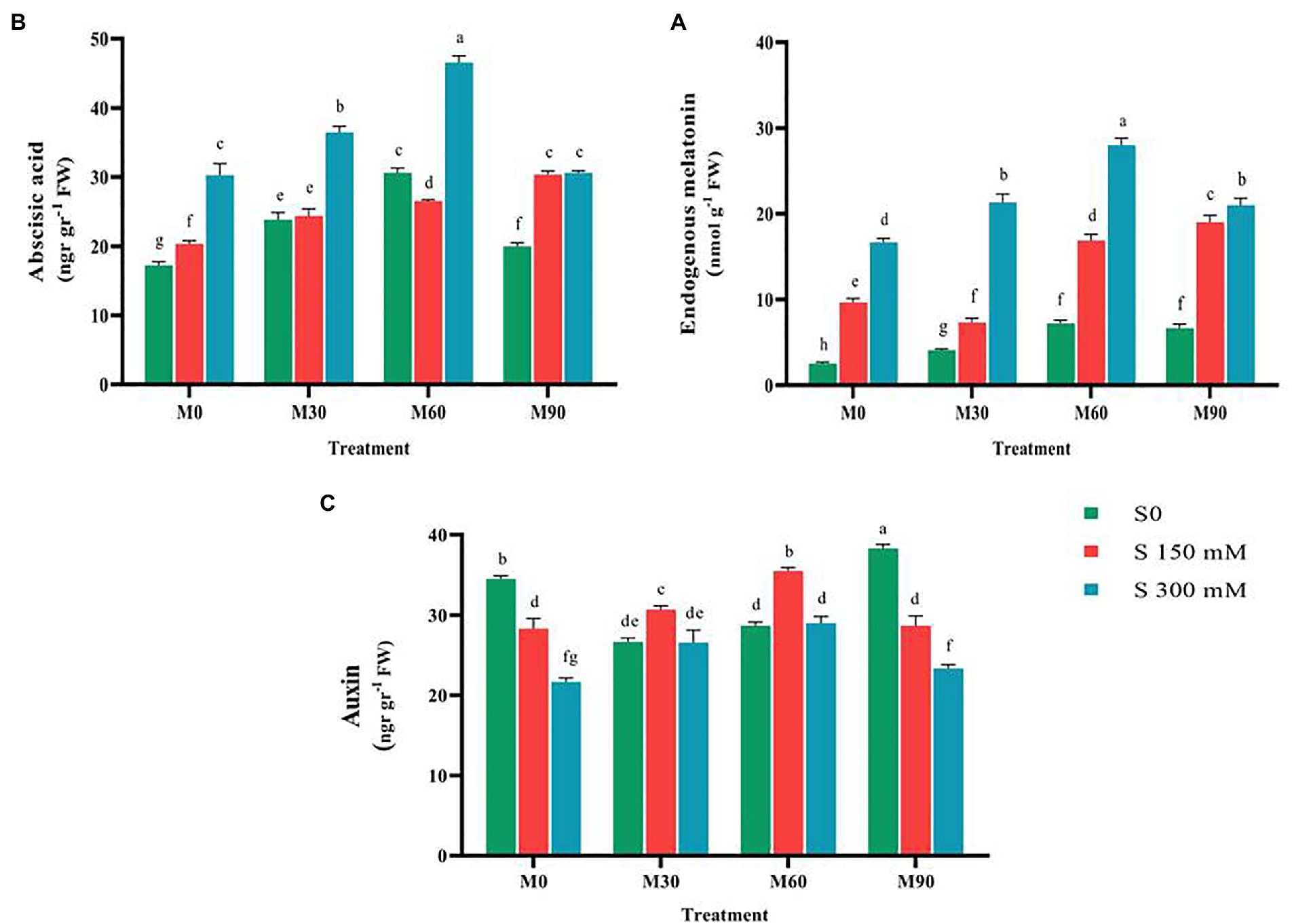
Figure 3. The mean comparison effects of melatonin concentrations and different levels of NaCl on (A) Endogenous melatonin content, (B) Abscisic acid content, (C) Auxin content. Duncan method at 1% probability level was applied to compare mean values. The columns having similar letters had no significant difference.
A review of the relevant researches showed that the effects of exogenous melatonin on the content of abscisic acid and auxin are quite different, as contradictory results have been reported (Arnao and Hernández-Ruiz, 2018, 2020; Zhang et al., 2019). Therefore, in this experiment, the relationship between melatonin and these two hormones under the control condition and salinity stress was investigated. The results of this study showed that salinity stress in a concentration-dependent manner has led to an increase (p < 0.01) in the content of abscisic acid (compared to the control). Compared to the control, the content of abscisic acid increased by 17 and 53% with the application of 150 and 300 mM of salinity, respectively. Thus, applying melatonin without salinity stress raised the content of this trait compared to the control. Simultaneous application of different levels of melatonin and salinity stress, especially at the salinity level of 300 mM and melatonin 60 ppm, significantly increased the content of abscisic acid (50%) in relation to salinity stress treatment (without the use of melatonin; Figure 3). In the mentioned treatment, the highest content of abscisic acid reached 45 ng g−1 FW.
When the average of various levels of melatonin and salinity stress were evaluated, the auxin content under the salinity stress was found to be significantly lower (p < 0.01) than in the control. When 150 and 300 mM of salt were applied, the auxin content fell by 25 and 66%, respectively, compared to the control. Indeed, the severity of applied salinity stress was a factor of influence in lowering the auxin content. The combined use of different levels of melatonin and salinity stress considerably raised the auxin content compared to the salinity treatment alone without the use of melatonin (Figure 3). Auxin content increased 30% by applying 60 ppm of melatonin and the salinity stress of 150 mM compared to the salinity treatment alone.
The effects of melatonin on mineral element contents under salt stress
The application of salinity stress significantly decreased (p < 0.01) the potassium content relative to the control. In contrast, exposing plants to varying concentrations of melatonin in the absence of salt stress led to a considerable increase in potassium content relative to the control. The potassium concentration increased by 20 and 50%, respectively, when treated with 30 and 60 ppm of melatonin. Simultaneous treatment of salinity stress and different levels of melatonin led to an increase in potassium content relative to salinity stress (no melatonin application). The highest potassium content (3% of dry matter) was achieved in the treatment of 60 ppm of melatonin and no salinity stress, and the lowest content (1.3% of dry matter) of this trait was recorded in the salinity stress of 300 mM (Figure 4).
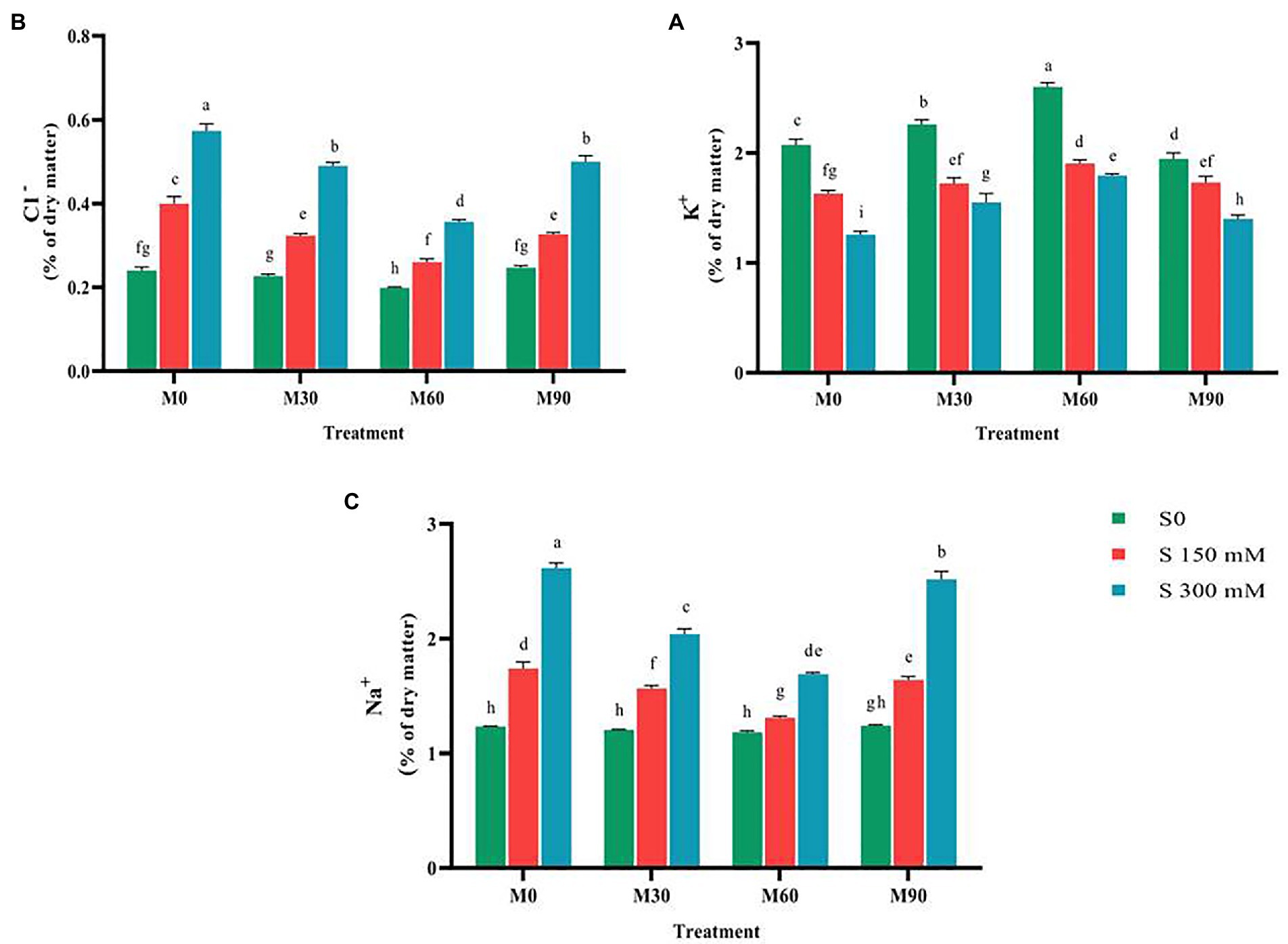
Figure 4. The mean comparison effects of melatonin concentrations and different levels of NaCl on (A) Potassium content, (B) Chlorine content, (C) Sodium content. Duncan method at 1% probability level was applied to compare mean values. The columns having similar letters had no significant difference.
After applying the salinity stress, performing a mean comparison of chlorine content revealed a rising trend (p < 0.01). Salinity stress of 150 and 300 mM resulted in 81 and 166% increase in chlorine content compared to the control. Application of 60 ppm melatonin without salinity stress remarkably reduced the chlorine content (15%) compared to the control; while other levels had no significant effect on this trait. Furthermore, simultaneous application of salinity stress at different levels of melatonin significantly lowered the chlorine (Cl−) content compared to the salinity stress treatment (without melatonin). It was observed that the application of 60 ppm of melatonin more effectively prevented the accumulation of chlorine in plant tissues under salinity stress (300 mM; Figure 4), so that by using this treatment, the chlorine content was reduced by 55% compared to the salinity treatment of 300 mM without the use of melatonin.
The salinity stress in a concentration-dependent manner led to a significant rise in intra-tissue sodium content (p < 0.01). Salinity stress of 150 and 300 mM resulted in a 50 and 100% increase in intra-tissue sodium content compared to the control. Treating the plants with different levels of melatonin (in absence of salinity stress) had negligible effects on sodium content (Na+) compared to the control. The combined use of melatonin and salinity considerably reduced the sodium content in plants compared to the salinity stress treatment (no melatonin application; Figure 4). The sodium content after using 60 ppm melatonin along with 300 mM of salinity decreased by 60% compared to the salinity treatment alone (no melatonin application).
The effects of melatonin on diosgenin biosynthesis pathway genes expression and diosgenin content
The expression of CAS (Cycloartenol synthase) gene under the influence of 150 mM salinity level (without melatonin) was not significantly different from the control, while it considerably increased (two-fold) at the level of 300 mM (Figures 1, 5). The expression of this gene sharply increased (p < 0.01) under the influence of different levels of melatonin (without salinity) so that it increased three-fold in the plants treated with 90 ppm of melatonin. Under salinity stress of 150 mM along with 60 ppm of melatonin, the expression of this gene increased seven times compared to the control. CAS gene expression was notably reduced when the plants were exposed to 300 mM salinity and treated with different levels of melatonin compared to the 150 mM NaCl. Furthermore, the expression of this gene was higher in 150 mM NaCl along with 60 and 30 ppm melatonin levels than in other treatments (Figure 5).
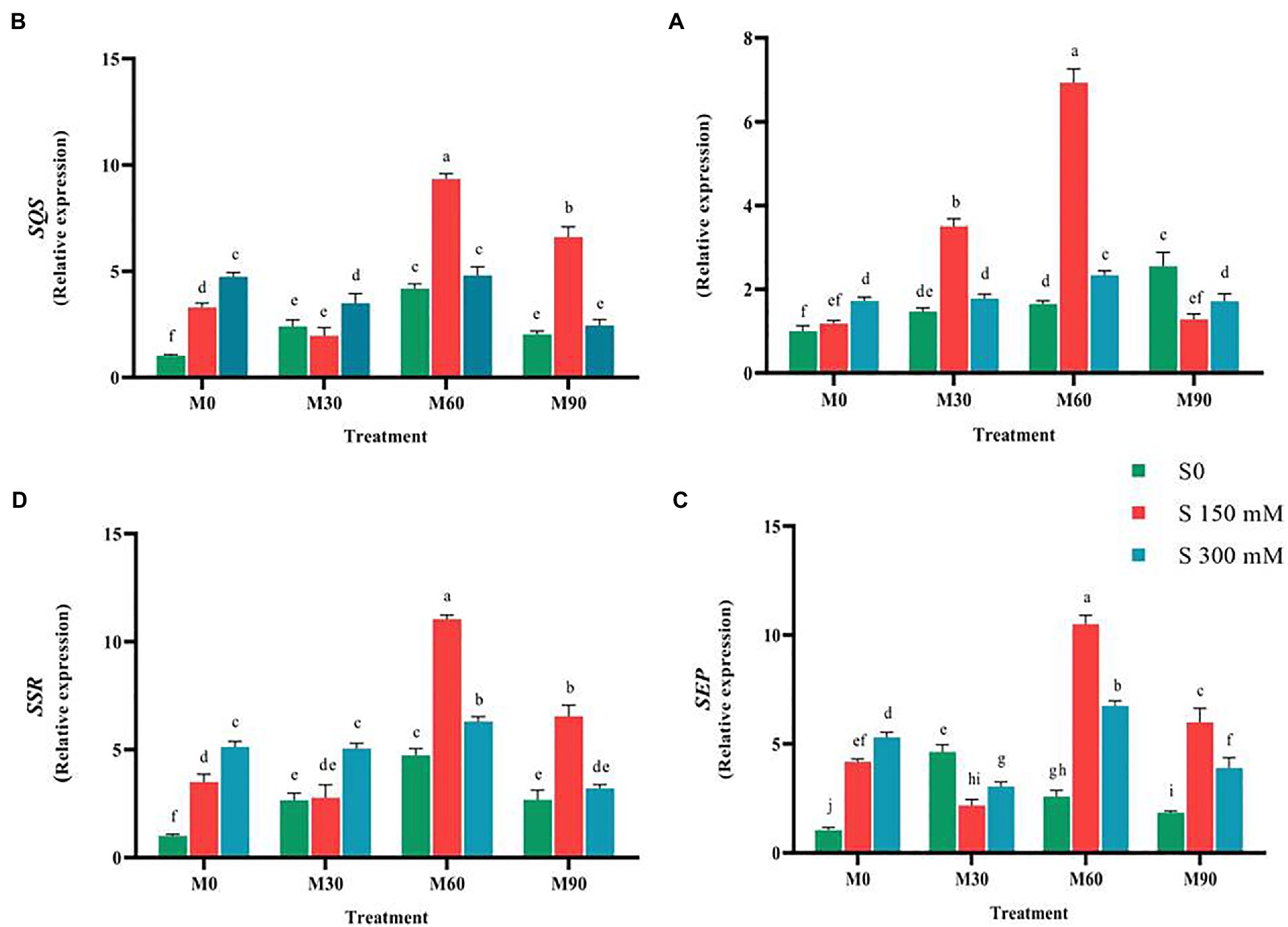
Figure 5. The mean comparison effects of melatonin concentrations and different levels of NaCl on the expression of (A) CAS, (B) SQS, (C) SEP, (D) SSR. Duncan method at 1% probability level was applied to compare mean values. The columns having similar letters had no significant difference.
Compared to the control, salt and melatonin treatment increased (p < 0.01) SQS gene (squalene synthase) expression. In compared to the salinity stress, simultaneous use of salinity (150 mM) and melatonin (60 and 90 ppm) greatly enhanced the expression of this gene (without melatonin), but simultaneous use of salinity (300 mM) and melatonin (60 ppm) had no significant influence on the expression of this gene. In the presence of 150 mM salinity and 60 ppm melatonin, the greatest expression of SQS (nine-fold) was observed (Figure 5).
Applying salinity stress (without the use of melatonin) in a concentration-dependent manner substantially enhanced the expression of SEP gene compared to the control. In comparison with the control, the expression of SEP rose significantly (4 and 5.3-fold increase, respectively, p < 0.01). Treating the fenugreek plants with low melatonin concentration (30 ppm) did not change the expression of this gene compared to the salinity stress (without the use of melatonin), while the expression of this gene underwent an increase under higher melatonin concentrations (60 and 90 ppm). The combined use of salinity stress (150 mM) and melatonin (60 and 90 ppm) enhanced the expression of this gene relative to the salinity stress (without melatonin use), while simultaneous application of salinity stress (150 mM) and melatonin (30 ppm) resulted in a significant decrease in the expression of this gene relative to the salinity stress. The simultaneous application of salinity stress (300 mM) and melatonin (30 and 90 ppm) and salinity stress (300 mM) and melatonin (60 ppm) decreased and increased the expression of this gene compared to salinity stress (without melatonin), respectively (Figure 5).
Exposing the plants to melatonin and salinity resulted in a significant rise (p < 0.01) in the expression of SSR compared to the control. Applying 150 and 300 mM of salinity caused a significant increase (3.5 and 5.5-fold increase, respectively, p < 0.01) in the expression of SSR compared to the control. The highest expression of this gene (12-fold) was recorded when 60 ppm of melatonin was combined with salinity stress of 150 mM. When a salinity of 150 mM was combined with 30 ppm of melatonin, the expression of this gene was reduced (without the use of melatonin), whereas when the same salinity level was combined with 60 and 90 ppm of melatonin, the expression of this gene increased significantly 3.14 and 1.85-fold, respectively, compared to the salinity stress without melatonin (Figure 5). The application of salinity and melatonin notably raised (p < 0.01) the expression of SMT gene (sterol methyltransferase) compared to the control, although no significant difference was observed among different levels of salinity stress. The expression of SMT experienced 3.1 and 3-fold increases with the application of 150 and 300 mM of salinity, respectively, compared to the control. Furthermore, the expression of this gene under 30, 60 and 90 ppm of melatonin without salinity stress rose by 2.5, 4.5 and 2.2-fold, respectively. The highest expression of SMT (six-fold), however, was recorded in 150 mM of salinity treatment along with 90 ppm of melatonin (Figure 6).
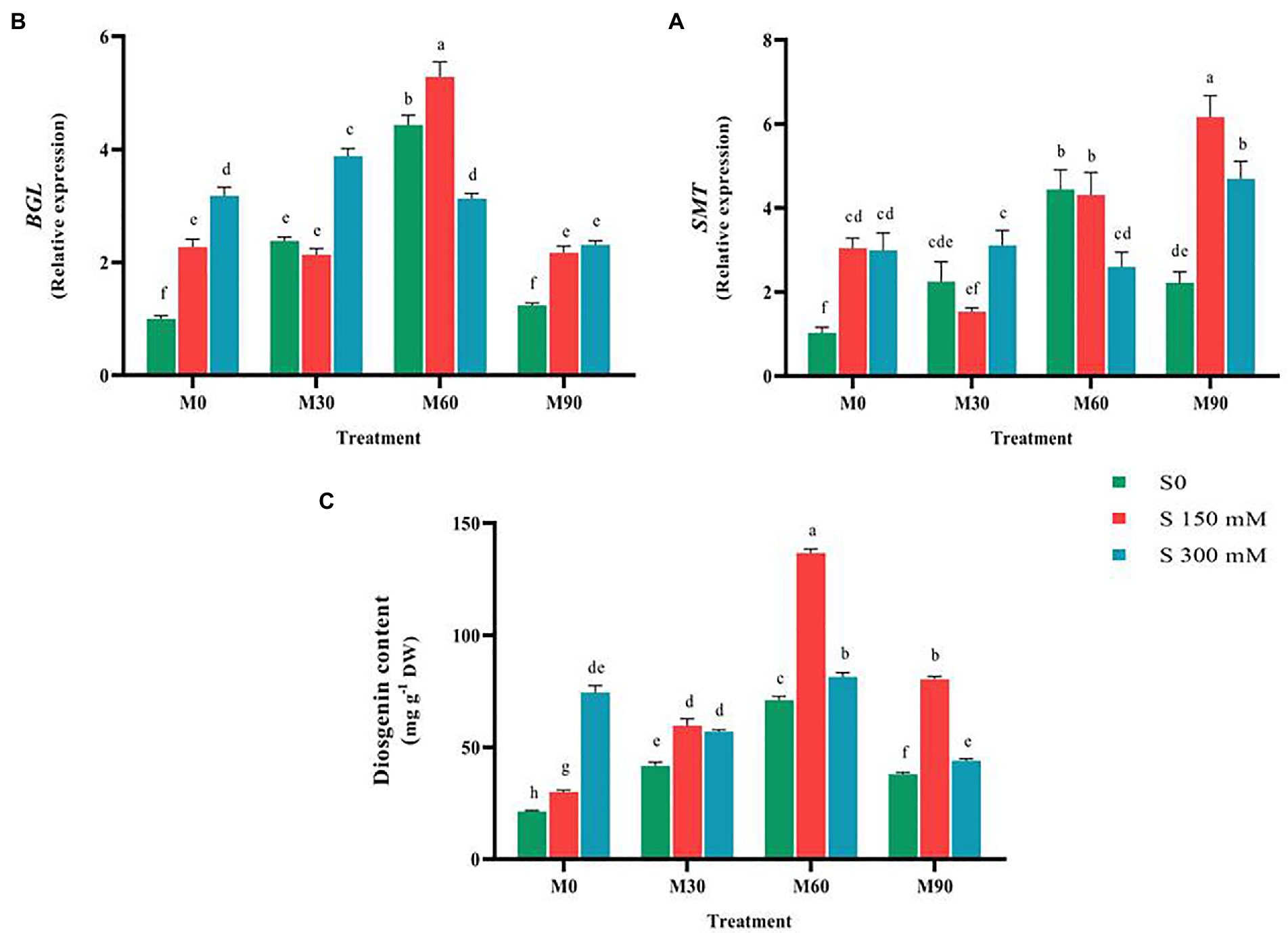
Figure 6. The mean comparison effects of melatonin concentrations and different levels of NaCl on the expression of (A) SMT, (B) BGL, and (C) Diosgenin content. Duncan method at 1% probability level was applied to compare mean values. The columns having similar letters had no significant difference.
BGL gene (26-O-Beta glucosidase) expression rose significantly (p < 0.01) under salinity stress without the use of melatonin compared to the control. Thus, the expression of this gene increased after using different levels of melatonin by 5 times (at the level of 60 ppm melatonin) compared to the control. The highest expression of this gene was recorded in the treatment of 150 mM NaCl combined with 60 ppm of melatonin by five-times compared to the control. Application of different levels of melatonin along with salinity stress of 300 mM significantly increased the expression of this gene compared to the control. The results showed that the interactions of salinity stress with different levels of melatonin raised the expression of this gene as a suitable stimulus. By increasing salinity stress from 150 to 300 mM (at the melatonin concentration of 60 ppm), the expression of this gene decreased (Figure 6).
Compared to the control, exposing the plants to salt and melatonin, particularly 60 ppm, dramatically increased (p < 0.01) the diosgenin level in fenugreek. When 150 and 300 mM of salt were applied, the diosgenin content increased significantly (50 and 275%, respectively) compared to the control. In addition, the diosgenin content rose by 100, 200 and 80% with the application of 30, 60 and 90 ppm of melatonin, respectively, compared to the control. The combined use of different levels of salinity (150 mM) and melatonin (all of the applied concentrations) substantially raised the diosgenin content compared to the 150 mM salinity treatment (without melatonin). Simultaneous application of different levels of salinity and melatonin (300 mM and 60 ppm) resulted in an increase in diosgenin content compared to the 300 mM salinity treatment (without melatonin). The highest content of diosgenin (131 mg g−1 DW) was recorded in the treatment of 150 mM salinity stress and 60 ppm of melatonin. By increasing salinity stress from 150 to 300 mM and melatonin concentration from 60 to 90 ppm, the diosgenin content considerably fell compared to NaCl of 150 mM (Figure 6).
Discussion
Exogenous melatonin alleviated NaCl-induced inhibition in biosynthesis of plant pigments and relative water content under salt stress
Given the fact that plant pigments such as chlorophylls and carotenoids, as the most important factors involved in photosynthesis, are considerably impaired by environmental stresses, monitoring these compounds may well highlight the impacts of any specific stresses on plants (Siddiqui et al., 2020a). The results of this study showed that applying the different levels of salinity stress led to a decrease in chlorophylls, carotenoids, and relative water contents in fenugreek. Our findings revealed that the application of melatonin (especially the level of 60 ppm) under the control (without salinity stress) and salinity condition enhanced (p < 0.01) the content of chlorophylls, carotenoids, and relative water (Table 1). Melatonin stimulates the synthesis of pigments by improving the genes involved in the biosynthesis pathway of chlorophylls and carotenoids (Sharif et al., 2018; Siddiqui et al., 2020a,b). The findings of this research back with earlier studies concerning melatonin’s beneficial effects on crops under the influence of abiotic stressors (Hossain et al., 2020; Ren et al., 2020). In fact, melatonin prevents the degradation of chlorophyll by enzymes such as chlorophyllase, phenophytinase, peroxidase, and regulates the enzyme pheophorbide a oxygenase (PaO; Sharif et al., 2018). The previous results suggest that the melatonin impact on photosynthetic pigments may be associated with increased capacity of the antioxidant system in plants (Li et al., 2017a; Xalxo and Keshavkant, 2019; Siddiqui et al., 2020a). In addition, the use of melatonin by increasing the concentration of magnesium ions, carotenoids, anthocyanins, and flavonoids, which are effective factors in photosynthesis, probably prevents the degradation of chlorophylls under stress (Li et al., 2017a; Bose and Howlader, 2020). In this study, melatonin increased the relative water content (RWC) of fenugreek plants subjected to salt stress to retain water (Table 1). It can be ascribed to the regulation of osmotic pressure by osmolytes or the improvement of water uptake by the roots (Keyvan, 2010). In fact, the exogenous application of melatonin enhances the photosynthetic activity and available plant water through osmotic regulation and reduction of H2O2 content in plants (Ren et al., 2020).
Exogenous melatonin improved cellular redox homeostasis under salt stress
Under stress conditions, the content of reactive oxygen species and free radicals normally increases and the plant is exposed to oxidative damage. In this scenario, a variety of enzymatic and non-enzymatic antioxidant defense mechanisms might form in plants (Siddiqui et al., 2020b). The treatment of plants with different levels of melatonin (particularly 60 ppm) enhanced the enzymatic and non-enzymatic antioxidant defense mechanisms (compared to the control; Tables 2 and 3; Figure 2). Melatonin, by regulating and enhancing the expression of genes involved in physiological and biochemical processes, leads to increased plant tolerance to abiotic stresses (Mukherjee, 2019). Our finding proved that exogenous application of melatonin could improve fenugreek tolerance to salinity stress by increasing antioxidant protection by improving the biosynthesis of antioxidant enzymes. Various studies have confirmed the role of melatonin as an important antioxidant in enhancing the activity of antioxidant enzymes and the direct scavenging of H2O2 content under salinity (Zhang et al., 2014; Huang et al., 2017; Zeng et al., 2018), oxidative (Li et al., 2016), drought (Ye et al., 2016; Li et al., 2018), and cold (Yang et al., 2018b) stress conditions. In a study by Lee et al. (2017), exogenous application of melatonin reduced salinity-induced damage in rice by improving the activity of superoxide dismutase, catalase, and reducing the H2O2 content.
The results of transcriptome analysis confirmed that melatonin is a powerful antioxidant, which could enhance the biosynthesis of other antioxidants, and thus lead to the activation of defense mechanisms in plants (Weeda et al., 2014). In general, the results of this study implied that plant tolerance to salinity stress is largely improved by using the exogenous melatonin through stimulating the antioxidant defense system and modulating reactive oxygen species as well as accumulating soluble protein. It is well documented in previous studies that applying exogenous melatonin is distributed throughout the plasma membrane and it causes a considerable rise in endogenous melatonin content through messaging processes (Nawaz et al., 2016; Bose and Howlader, 2020). The formation of this process may result in decreased reactive oxygen species and H2O2 levels, and probably equilibrium in the cell by stimulating the genes closely related to the biosynthesis processes of enzymatic and non-enzymatic antioxidants (Arnao and Hernández-Ruiz, 2015; Sharif et al., 2018). The action of melatonin on enhancing the antioxidant enzyme activates and removing reactive oxygen species and free radicals is likely related to the decrease of electrolyte leakage index and malondialdehyde content following the administration of varied amounts of melatonin in this research (Zhang et al., 2020). The similar results about the effects of melatonin on the reduction of these two traits under abiotic stress have been reported in other plants (Li et al., 2017a; Zhang et al., 2020). It seems that melatonin by stimulating the expression of the genes closely associated with key metabolic pathways such as phenylpropanoid, chlorophylls, and carotenoids biosynthesis, carbon stabilization, and glucose metabolism will be able to effectively reduce the oxidative stress caused by salinity stress and improves fenugreek tolerance to this destructive stress (Arnao and Hernández-Ruiz, 2018; Bose and Howlader, 2020). Since phenol and flavonoid molecules have the ability to donate oxygen atoms to free radicals, they may scavenge free radicals (Arnao and Hernández-Ruiz, 2018; Sharif et al., 2018; Bose and Howlader, 2020).
Melatonin treatment (especially 60 ppm) considerably boosted the nitric oxide level of the plants in this research as compared to the control (Table 3). Various publications have reported on the beneficial benefits of salinity and melatonin on boosting nitric oxide concentration (Mukherjee, 2019). Increasing the concentration of endogenous melatonin after the application of exogenous melatonin and subsequent stimulation of nitric oxide biosynthesis is one of the first plant defense responses in signaling pathways, which ultimately leads to increased plant tolerance (Lee et al., 2017). The results of this study emphasize the role of nitric oxide as an important gaseous molecule with physiological functions including salinity stress tolerance. Even though the interactions of melatonin and nitric oxide under stress condition in plants are somewhat controversial, the current findings enhanced our insight into the relationship between melatonin and nitric oxide content under salinity stress in fenugreek (Lee et al., 2017). Interestingly, the neutralizing effects of antioxidants by melatonin are dependent on the production of nitric oxide under stress conditions. Therefore, the endogenous nitric oxide content improves after increasing stimulation by melatonin caused by salinity stress (Kaya et al., 2020).
The production of reactive oxygen species contributes in messaging activities on one hand, and on the other hand, if the reactive of oxygen concentration is higher than the desired level; it can lead to serious damage to the plant. The findings of this investigation revealed that the application of different levels of melatonin (30 and 60 ppm) under followed by a significant reduction in H2O2 content. Besides, treating the plants grown under salinity stress with melatonin (especially 60 ppm) successfully increased the H2O2 content compared to salinity treatment (no melatonin application; Table 3). The results of various studies show that melatonin effectively increases the expression of genes involved in antioxidant biosynthesis and thus considerably reduces H2O2 content (Lee et al., 2017). In general, the results of previous studies emphasize the role of melatonin in increasing salinity tolerance owing to its antioxidant properties and prevention of overproduction of reactive oxygen species. Melatonin induces salinity tolerance in plants by reducing the production of reactive oxygen species, lipid peroxidation, and inducing gene expression of various antioxidants (Kaya et al., 2020). Interestingly, in this study, the changes in fenugreek H2O2 and NO contents following the applied treatments were very similar, suggesting that these compounds play a prominent role in increasing plant tolerance under stress conditions. Damage to cell membranes due to cold stress may be assigned to the increase in malondialdehyde content and electrolyte leakage index, which in turn, may be arisen out of the elevated free radicals and H2O2 levels (Li et al., 2017b; Cao et al., 2018). Malondialdehyde and H2O2 contents considerably decreased following the use of melatonin, possibly due to the stimulation of biosynthesis of enzymatic and non-enzymatic antioxidants (Zhang et al., 2014; Huang et al., 2017; Zeng et al., 2018).
Exogenous melatonin modulates endogenous hormone content
One of the valuable achievements of applying melatonin would be enhancement of the tolerance of plants to abiotic stresses via ABA-dependent pathway. It seems that abscisic acid is effective in increasing plant tolerance by regulating the content of reactive oxygen species (Arnao and Hernández-Ruiz, 2018, 2020; Sharif et al., 2018). The findings of this research showed that exogenous melatonin causes an increase in abscisic acid and endogenous melatonin content in In plants treated with salinity stress (Figure 3), which is likely regulated by melatonin’s direct and indirect effects on genes involved in intracellular chemical production (Arnao and Hernández-Ruiz, 2018, 2020; Sharif et al., 2018; Tiwari et al., 2020). Zhang et al. (2019) reported an increase in ABA biosynthesis and consequently a considerable improvement of plant tolerance to salinity stress. The results of various studies imply that the endogenous biosynthesis of melatonin increases under abiotic stress, which indicates the role of this hormone in plants responses to environmental stresses (Bose and Howlader, 2020). Accumulation of melatonin in plants is probably associated with enhanced expression of genes and activity of enzymes involved in melatonin biosynthesis. Exogenous melatonin infiltration enhances the plant’s endogenous melatonin content, which improves the plant’s resistance to salt stress (Bose and Howlader, 2020).
The role of auxin, as another regulator of plant growth, in abiotic stresses was proven (Arnao and Hernández-Ruiz, 2018; Sharif et al., 2018; Pérez-Llorca et al., 2019; Siddiqui et al., 2020a). The results of this study showed that the application of different levels of melatonin significantly enhanced the content of auxin, respectively (Figure 2). Changes in auxin content under salinity stress and melatonin treatment were reported in other studies (Pérez-Llorca et al., 2019). A positive and significant relationship between melatonin content and hormones, such as brassinosteroids (BRs), abscisic acid, and auxin was proven to regulate physiological processes in abiotic stress (Arnao and Hernández-Ruiz, 2018, 2020; Sharif et al., 2018; Pérez-Llorca et al., 2019; Siddiqui et al., 2020a). In this study, the increase of auxin content in plants treated with different levels of salinity and melatonin can be ascribed to increased expression of the main genes responsible for the biosynthesis of auxin and abscisic acid. Exogenous melatonin application possibly contributes in activating the H2O2, and NO-dependent pathway, enhancing the biosynthesis of abscisic acid and auxin, and regulating the signal transduction pathways, which raises fenugreek tolerance to abiotic stresses.
Exogenous melatonin maintains ionic homeostasis
Our finding implied that the sodium (Na+) and chlorine (Cl−) content in fenugreek plants under salinity stress rose substantially in relation to the normal (Figure 4). The increased intra-tissue sodium is most likely to blame for the decrease in potassium absorption (Zahedi et al., 2020). Furthermore, owing to nutritional imbalance and the presence of high sodium and calcium in salinity, potassium absorption is hampered (Wang et al., 2013). The results of this study indicated that treating the fenugreek plant with different levels of melatonin, particularly 60 ppm, under salinity stress successfully raised the potassium content compared to salinity stress (without melatonin application; Figure 3). The application of melatonin could control the expression of ion channel genes and maintain ionic equilibrium in plant under salt stress via altering the activity of transcription factors involved in this process and as a result, ion homeostasis and plant tolerance to salinity stress generally rise (Zhao et al., 2018). Yu et al. (2018) reported that exogenous melatonin stimulated triacylglycerol breakdown, fatty acids β-oxidation, and energy turnover under salinity conditions, thereby contributing to the maintenance of plasma membrane H+–ATPase activity and K+/Na+ homeostasis in sweet potato. Reduced sodium and chlorine content due to different levels of melatonin may be associated with elevated Ca2+-ATPase activity in the plasma membrane, which provides the energy needed to remove large amounts of sodium (Liu et al., 2020).
Exogenous melatonin effectively stimulates diosgenin biosynthesis pathway genes expression and diosgenin content
The effects of abiotic stresses on plants may result in negative changes in plant quality and quantity. Abiotic stresses effects in medicinal plants vary from those in other crops in that they may diminish biomass while increasing secondary metabolites. Therefore, the application of abiotic stresses in medicinal plants according to the purpose of their use (vegetative tissue or secondary metabolism) requires special expertise, particularly in identifying the intensity of this stress and the plant growth stage as well as using various stimulants (Parihar et al., 2015; Nxele et al., 2017). The concentration of stimulus and duration of exposure may highly contribute in increasing the performance of the desired stress and stimulus (Pavarini et al., 2012; Ebrahimibasabi et al., 2020). In this study, the combined effect of different levels of salinity stress and melatonin on the diosgenin content in fenugreek was evaluated to identify the best salinity and melatonin concentrations in order to achieve the highest diosgenin content.
The results of the present study indicated that salinity stress and melatonin application of 60 and 90 ppm have effectively enhanced the expression of the SQS gene, suggesting that this gene might be a pivotal point in the engineering of the diosgenin metabolite pathway (Figure 5). According to the results of the current study, similar to SQS gene salinity stress and the interaction of melatonin (60 ppm) and salinity stress resulted in the increased activity of SEP, and CAS genes compared to the control. Considering the favorable reaction of these two genes to melatonin, using of melatonin could be suggested as a suitable elicitor for the engineering of diosgenin. Since the activity of CAS gene is quite necessary in triggering the diosgenin biosynthetic pathway, its response to the interactions of salinity stress and melatonin promises identifying a suitable functional stimulus to increase the diosgenin content in fenugreek.
It is believed that there are some ambiguities in diosgenin biosynthetic pathway (Mohammadi et al., 2020). Moreover, exploring the complexities of this pathway and the functional elicitors with the aim of stimulating the increase of expression of these genes requires in-depth investigations. SMT and SSR genes expression increased under 150 mM and 300 mM of salinity compared to the control (Figures 5, 6). The two SSR and SMT genes compete for the biosynthesis pathway of diosgenin, with the SSR gene directing the pathway to diosgenin biosynthesis and the SMT gene directing the pathway to the biosynthesis of other plant sterols (Mohammadi et al., 2020; Zolfaghari et al., 2020). Therefore, this point is a very important point in the biosynthetic pathway of diosgenin.
The results of various studies show that stimulants such as salicylic acid, methyl jasmonates, brassinosteroids (BRs) alter and regulate the rate of biosynthesis, accumulation and exchange of substances stored in the vacuole, conversion or decomposition of plant metabolites, and eventually the amount of primary and secondary metabolites change (Odjakova and Hadjiivanova 2001). There are contradicting data about the application of various stimuli and the subsequent changes in the quantity of secondary metabolites, suggesting that different plants respond to stimuli in different ways. The length of stimulus exposure, development stage, and varying stimuli concentrations all influence plant responses (Angelova et al., 2006; Namdeo, 2007). Moreover, using melatonin in plants treated with salinity stress effectively enhanced the expression of the genes involved in the biosynthesis of diosgenin and ultimately the diosgenin content, while increasing the intensity of salinity stress (from 150 mM to 300 mM) along with melatonin (90 ppm) reduced the expression of all genes except for SMT gene. In fact, under conditions of severe (300 Mm of Nacl and 90 ppm of melatonin), increased expression of this gene leads the pathway of diosgenin biosynthesis to the production of other plant sterols, thereby increasing plant tolerance. Therefore, following the application of high melatonin concentration and severe salinity, which its destructive and toxic effects on plants were also proven in physiology section, the expression of SMT gene increased, which led to a decrease in the content of diosgenin. On the contrary, in lower doses of the applied treatments (150 Mm of Nacl and 30 and 60 ppm of melatonin), the expression of other genes involved in the pathway of diosgenin biosynthesis and finally the diosgenin content rose considerably compared to normal condition (Figure 6). Exogenous melatonin could effectively prevent the salt-induced accumulation of triacylglycerol in plant leaves (Yu et al., 2018). Furthermore, the genes responsible for fatty acid β-oxidation, triacylglycerol breakdown, and energy turnover are expressed more strongly following melatonin application which in turn leads to the accumulation of the main precursor of diosgenin biosynthesis, i.e., Acetyl-CoA. Accordingly, the higher diosgenin content in the plants treated by melatonin and encountered with salinity stress in the current study could be accounted for the same process. In general, the results of this study suggested that the combined use of salinity stress and melatonin effectively enhances the diosgenin content in fenugreek.
Conclusion
This study suggested that exogenous application of melatonin, as an important functional stimulant, at the specific concentrations of 30 and 60 ppm, would trigger physiological and biochemical changes in salt-treated fenugreek in order to increase the plant tolerance to salinity stress. Melatonin, in fact, protects fenugreek from the negative effects of salt stress by increasing the manufacture of enzymatic and non-enzymatic antioxidants, chlorophylls, and diosgenin, as well as preserving ionic homeostasis. The primary results of this research may add to our understanding of the benefits of administering melatonin to plants that are stressed by the environment. In conclusion, the results of this study would provide a deep understanding of the melatonin signaling pathway in response to salinity-stressed fenugreek, which could be effective in breeding or biotechnology-based programs.
Data availability statement
The raw data supporting the conclusions of this article will be made available by the authors, without undue reservation.
Author contributions
AE: conceptualization, data curation, formal analysis, project administration, supervision, investigation and methodology, validation, visualization, writing—original draft, and writing—review and editing. MME: investigation and methodology. MRA and HA: validation, formal analysis, and review and editing. All authors contributed to the article and approved the submitted version.
Acknowledgments
We thank the Shahrood University of Technology for financially supporting and providing the required facilities.
Conflict of interest
The authors declare that the research was conducted in the absence of any commercial or financial relationships that could be construed as a potential conflict of interest.
Publisher’s note
All claims expressed in this article are solely those of the authors and do not necessarily represent those of their affiliated organizations, or those of the publisher, the editors and the reviewers. Any product that may be evaluated in this article, or claim that may be made by its manufacturer, is not guaranteed or endorsed by the publisher.
Supplementary material
The supplementary material for this article can be found online at: https://www.frontiersin.org/articles/10.3389/fpls.2022.890613/full#supplementary-material
Footnotes
References
Ahanger, M. A., Akram, N. A., Ashraf, M., Alyemeni, M. N., Wijaya, L., and Ahmad, P. (2017). Plant responses to environmental stresses from gene to biotechnology. AoB. Plants. 9, 1–17. doi: 10.1093/aobpla/plx025
Al-Huqail, A. A., Khan, M. N., Ali, H. M., Siddiqui, M. H., Al-Huqail, A. A., AlZuaibr, F. M., et al. (2020). Exogenous melatonin mitigates boron toxicity in wheat. Ecotoxicol. Environ. Saf. 201:110822. doi: 10.1016/j.ecoenv.2020.110822
Angelova, Z., Georgiev, S., and Roos, W. (2006). Elicitation of plants. Biotechnol. Biotechnol. Equip. 20, 72–83. doi: 10.1080/13102818.2006.10817345
Arnao, M. B., and Hernández-Ruiz, J. (2015). Functions of melatonin in plants: a review. J. Pineal Res. 59, 133–150. doi: 10.1111/jpi.12253
Arnao, M. B., and Hernández-Ruiz, J. (2018). Melatonin and its relationship to plant hormones. Ann. Bot. 121, 195–207. doi: 10.1093/aob/mcx114
Arnao, M. B., and Hernández-Ruiz, J. (2020). Melatonin as a regulatory hub of plant hormone levels and action in stress situations. Plant Biol. 23, 7–19.
Banakar, M. H., Amiri, H., Ardakani, M. R. S., and Ranjbar, G. H. (2022). Susceptibility and tolerance of fenugreek (Trigonella foenum-graceum L.) to salt stress: physiological and biochemical inspections. Environ. Exp. Bot. 194:104748. doi: 10.1016/j.envexpbot.2021.104748
Blunden, G., and Rhodes, C. T. (1968). Stability of diosgenin. J. Pharm. Sci. 57, 602–604. doi: 10.1002/jps.2600570411
Bose, S. K., and Howlader, P. (2020). Melatonin plays multifunctional role in horticultural crops against environmental stresses: A review. Environ. Exp. Bot. 176:104063. doi: 10.1016/j.envexpbot.2020.104063
Bradford, M. M. (1976). A rapid and sensitive method for the quantitation of microgram quantities of protein utilizing the principle of protein-dye binding. Anal. Biochem. 72, 248–254. doi: 10.1016/0003-2697(76)90527-3
Cao, J., Murch, S. J., O’Brien, R., and Saxena, P. K. (2006). Rapid method for accurate analysis of melatonin, serotonin and auxin in plant samples using liquid chromatography–tandem mass spectrometry. J. Chromatogr. A 1134, 333–337. doi: 10.1016/j.chroma.2006.09.079
Cao, S., Shao, J., Shi, L., Xu, L., Shen, Z., Chen, W., et al. (2018). Melatonin increases chilling tolerance in postharvest peach fruit by alleviating oxidative damage. Sci. Rep. 8:806. doi: 10.1038/s41598-018-19363-5
Cheng, J., Chen, J., Liu, X., Li, X., Zhang, W., Dai, Z., et al. (2021). The origin and evolution of the diosgenin biosynthetic pathway in yam. Plant Commun. 2:100079. doi: 10.1016/j.xplc.2020.100079
Dobrev, P. I., and Kaminek, M. (2002). Fast and efficient separation of cytokinins from auxin and abscisic acid and their purification using mixed-mode solid-phase extraction. J. Chromatogr. 950, 21–29. doi: 10.1016/S0021-9673(02)00024-9
Drotar, A., Phelps, P., and Fall, R. (1985). Evidence for glutathione peroxidase activities in cultured plant cells. Plant Sci. 42, 35–40. doi: 10.1016/0168-9452(85)90025-1
Dubbels, R., Reiter, R. J., Klenke, E., Goebel, A., Schnakenberg, E., Ehlers, C., et al. (1995). Melatonin in edible plants identified by radioimmunoassay and by high performance liquid chromatography-mass spectrometry. J. Pineal Res. 18, 28–31. doi: 10.1111/j.1600-079X.1995.tb00136.x
Ebrahimibasabi, E., Ebrahimi, A., Momeni, M., and Amerian, M. R. (2020). Elevated expression of diosgenin-related genes and stimulation of the defense system in Trigonella foenum-graecum (fenugreek) by cold plasma treatment. Sci. Hortic. 271:109494. doi: 10.1016/j.scienta.2020.109494
El Amrani, A., Couée, I., Berthomé, R., Ramel, F., Gouesbet, G., and Sulmon, C. (2019). Involvement of polyamines in sucrose-induced tolerance to atrazine-mediated chemical stress in Arabidopsis thaliana. J. Plant Physiol. 238, 1–11. doi: 10.1016/j.jplph.2019.04.012
Estaji, A., Kalaji, H. M., Karimi, H. R., Roosta, H. R., and Moosavi-Nezhad, S. M. (2019). How glycine betaine induces tolerance of cucumber plants to salinity stress. Photosynthetica 57, 753–761. doi: 10.32615/ps.2019.053
Gas-Pascual, E., Berna, A., Bach, T. J., and Schaller, H. (2014). Plant oxidosqualene metabolism: cycloartenol synthase dependent sterol biosynthesis in Nicotiana benthamiana. PLoS One 9:e10915. doi: 10.1371/journal.pone.0109156
Gauillard, F., Richardforget, F., and Nicolas, J. (1993). New spectrophotometric assay for polyphenol oxidase activity. Anal. Biochem. 215, 59–65. doi: 10.1006/abio.1993.1554
Hanin, M., Ebel, C., Ngom, M., Laplaze, L., and Masmoudi, K. (2016). New insights on plant salt tolerance mechanisms and their potential use for breeding. Front. Plant Sci. 7:1787. doi: 10.3389/fpls.2016.01787
He, F., Zhu, Y., He, M., and Zhang, Y. S. (2008). Molecular cloning and characterization of the gene encoding squalene epoxidase in Panax notoginseng. DNA Seq. 19, 270–273. doi: 10.1080/10425170701575026
Hepburn, H. A., Naylor, R. E., and Stokes, D. T. (1986). Electrolyte leakage from winter barley tissue as an indicator of winter-hardiness. Ann. Appl. Biol. 108, 164–165. doi: 10.1111/aab.1986.108.s1.164
Hossain, M., Li, J., Sikdar, A., Hasanuzzaman, M., Uzizerimana, F., Muhammad, I., et al. (2020). Exogenous melatonin modulates the physiological and biochemical mechanisms of drought tolerance in Tartary buckwheat (Fagopyrum tataricum L.). Molecules 25:2828. doi: 10.3390/molecules25122828
Huang, Y. H., Liu, S. J., Yuan, S., Guan, C., Tian, D. Y., Cui, X., et al. (2017). Overexpression of ovine AANAT and HIOMT genes in switchgrass leads to improved performance and salt-tolerance. Sci. Rep. 7:12212. doi: 10.1038/s41598-017-12566-2
Hubick, K. T., and Reid, D. M. (1980). A rapid method for the extraction and analysis of abscisic acid from plant tissue. Plant Physiol. 65, 523–525. doi: 10.1104/pp.65.3.523
Isaac, R. A., and Kerber, J. D. (1971). “Atomic absorption and flame photometry: Techniques and uses in soil, plant, and water analysis,” in Instrumental Methods for Aanalysis of Soils and Plant Tissue. (Soil Science Society of America, Inc. Second Printing), 230.
Isayenkov, S. V., and Maathuis, F. J. (2019). Plant salinity stress: many unanswered questions remain. Front. Plant Sci. 10:80. doi: 10.3389/fpls.2019.00080
Kaya, C., Higgs, D., Ashraf, M., Alyemeni, M. N., and Ahmad, P. (2020). Integrative roles of nitric oxide and hydrogen sulfide in melatonin-induced tolerance of pepper (Capsicum annuum L.) plants to iron deficiency and salt stress alone or in combination. Physiol. Plant. 168, 256–277. doi: 10.1111/ppl.12976
Keyvan, S. (2010). The effects of drought stress on yield, relative water content, proline, soluble carbohydrates and chlorophyll of bread wheat cultivars. J. Animal Plant Sci. 8, 1051–1060.
Kirnak, H., Kaya, C., Tas, I., and Higgs, D. (2001). The influence of water deficit on vegetative growth, physiology, fruit yield and quality in eggplants. Bulgarian J. Plant Physiol. 27, 34–46.
Kono, Y. (1978). Generation of superoxide radical during autoxidation of hydroxylamine and an assay for superoxide dismutase. Arch. Biochem. Biophys. 186, 189–195. doi: 10.1016/0003-9861(78)90479-4
Kumar, A., Singh, S., Gaurav, A. K., Srivastava, S., and Verma, J. P. (2020). Plant growth-promoting bacteria: biological tools for the mitigation of salinity stress in plants. Front. Microbiol. 11:1216. doi: 10.3389/fmicb.2020.01216
Kumar, K., and Saddhe, A. A. (2018). “Targeting Aquaporins for conferring salinity tolerance in crops,” in Salinity Responses and Tolerance in Plants. Vol. 1. eds. V. Kumar, S. H. Wani, P. Suprasanna, and L. S. Tran (Cham: Springer), 65–84.
Lee, K., Choi, G. H., and Back, K. (2017). Cadmium-induced melatonin synthesis in rice requires light, hydrogen peroxide, and nitric oxide: key regulatory roles for tryptophan decarboxylase and caffeic acid O-methyltransferase. J. Pineal Res. 63:e12441. doi: 10.1111/jpi.12441
Li, H., Chang, J., Chen, H., Wang, Z., Gu, X., Wei, C., et al. (2017a). Exogenous melatonin confers salt stress tolerance to watermelon by improving photosynthesis and redox homeostasis. Front. Plant Sci. 8:295. doi: 10.3389/fpls.2017.00295
Li, H., He, J., Yang, X., Li, X., Luo, D., Wei, C., et al. (2016). Glutathione-dependent induction of local and systemic defense against oxidative stress by exogenous melatonin in cucumber (Cucumis sativus L.). J. Pineal Res. 60, 206–216. doi: 10.1111/jpi.12304
Li, J., Zeng, L., Cheng, Y., Lu, G., Fu, G., Ma, H., et al. (2018). Exogenous melatonin alleviates damage from drought stress in Brassica napus L. (rapeseed) seedlings. Acta Physiol. Plant. 40:43. doi: 10.1007/s11738-017-2601-8
Li, X., Yu, B., Cui, Y., and Yin, Y. (2017b). Melatonin application confers enhanced salt tolerance by regulating Na+ and cl - accumulation in rice. J. Plant Growth Regul. 83, 441–454. doi: 10.1007/s10725-017-0310-3
Li, Y., Kong, D., Fu, Y., Sussman, M. R., and Wu, H. (2020). The effect of developmental and environmental factors on secondary metabolites in medicinal plants. Plant Physiol. Biochem. 148, 80–89. doi: 10.1016/j.plaphy.2020.01.006
Ling, L., Jiangang, L., Minchong, S., Chunlei, Z., and Yuanhua, D. (2015). Cold plasma treatment enhances oilseed rape seed germination under drought stress. Sci. Rep. 5, 1–10. doi: 10.1038/srep13033
Liu, J., Shabala, S., Zhang, J., Ma, G., Chen, D., Shabala, L., et al. (2020). Melatonin improves rice salinity stress tolerance by NADPH oxidase-dependent control of the plasma membrane K+ transporters and K+ homeostasis. Plant Cell Environ. 43, 2591–2605. doi: 10.1111/pce.13759
Liu, Y., Liang, H., Lv, X., Liu, D., Wen, X., and Liao, Y. (2016). Effect of polyamines on the grain filling of wheat under drought stress. Plant Physiol. Biochem. 100, 113–129. doi: 10.1016/j.plaphy.2016.01.003
Livak, K. J., and Schmittgen, T. D. (2001). Analysis of relative gene expression data using real-time quantitative PCR and the 2− ΔΔCT method. Methods 25, 402–408. doi: 10.1006/meth.2001.1262
Loreto, F., and Velikova, V. (2001). Isoprene produced by leaves protects the photosynthetic apparatus against ozone damage, quenches ozone products, and reduces lipid peroxidation of cellular membranes. Plant Physiol. 127, 1781–1787. doi: 10.1104/pp.010497
McAnuff, M. A., Harding, W. W., Omoruyi, F. O., Jacobs, H., Morrison, E. Y., and Asemota, H. N. (2005). Hypoglycemic effects of steroidal sapogenins isolated from jamaican bitter yam, dioscorea polygonoides. Food Chem. Toxicol. 43, 1667–1672. doi: 10.1016/j.fct.2005.05.008
McDonald, S., Prenzler, P. D., Antolovich, M., and Robards, K. (2001). Phenolic content and antioxidant activity of olive extracts. Food Chem. 73, 73–84. doi: 10.1016/S0308-8146(00)00288-0
Mohammadi, M., Mashayekh, T., Rashidi-Monfared, S., Ebrahimi, A., and Abedini, D. (2020). New insights into diosgenin biosynthesis pathway and its regulation in (Trigonella foenum-graecum L.). Phytochem. Anal. 31, 229–241. doi: 10.1002/pca.2887
Mukherjee, S. (2019). Insights into nitric oxide–melatonin crosstalk and N-nitrosomelatonin functioning in plants. J. Exp. Bot. 70, 6035–6047. doi: 10.1093/jxb/erz375
Namdeo, A. (2007). Plant cell elicitation for production of secondary metabolites: a review. Pharmacogn. Rev. 1, 69–79.
Nawaz, M. A., Huang, Y., Bie, Z., Ahmed, W., Reiter, R. J., Niu, M., et al. (2016). Melatonin: current status and future perspectives in plant science. Front. Plant Sci. 6:1230. doi: 10.3389/fpls.2015.01230
Noohpisheh, Z., Amiri, H., Mohammadi, A., and Farhadi, S. (2021). Effect of the foliar application of zinc oxide nanoparticles on some biochemical and physiological parameters of Trigonella foenum-graecum under salinity stress. Plant Biosystems Int. J. Dealing Aspects Plant Biol. 155, 267–280. doi: 10.1080/11263504.2020.1739160
Nxele, X., Klein, A., and Ndimba, B. K. (2017). Drought and salinity stress alters ROS accumulation, water retention, and osmolyte content in sorghum plants. S. Afr. J. Bot. 108, 261–266. doi: 10.1016/j.sajb.2016.11.003
Odjakova, M., and Hadjiivanova, C. (2001). The complexity of pathogen defense in plants. Bulgarian J. Plant Physiol. 27, 101–109.
Ogbe, A. A., Finnie, J. F., and Van-Staden, J. (2020). The role of endophytes in secondary metabolites accumulation in medicinal plants under abiotic stress. S. Afr. J. Bot. 134, 126–134. doi: 10.1016/j.sajb.2020.06.023
Pandey, P., Irulappan, V., Bagavathiannan, M. V., and Senthil-Kumar, M. (2017). Impact of combined abiotic and biotic stresses on plant growth and avenues for crop improvement by exploiting physio-morphological traits. Front. Plant Sci. 8:537. doi: 10.3389/fpls.2017.00537
Parihar, P., Singh, S., Singh, R., Singh, V. P., and Prasad, S. M. (2015). Effect of salinity stress on plants and its tolerance strategies: a review. Environ. Sci. Pollut. Res. 22, 4056–4075. doi: 10.1007/s11356-014-3739-1
Park, S., Lee, D. E., Jang, H., Byeon, Y., Kim, Y. S., and Back, K. (2012). Melatonin-rich transgenic rice plants exhibit resistance to herbicide- induced oxidative stress. J. Pineal Res. 54, 258–263. doi: 10.1111/j.1600-079X.2012.01029.x
Pavarini, D. P., Pavarini, S. P., Niehues, M., and Lopes, N. P. (2012). Exogenous influences on plant secondary metabolite levels. Anim. Feed Sci. Technol. 176, 5–16. doi: 10.1016/j.anifeedsci.2012.07.002
Pérez-Llorca, M., Muñoz, P., Müller, M., and Munné-Bosch, S. (2019). Biosynthesis, metabolism and function of auxin, salicylic acid and melatonin in climacteric and non-climacteric fruits. Front. Plant Sci. 10:136. doi: 10.3389/fpls.2019.00136
Pothinuch, P., and Tongchitpakdee, S. (2011). Melatonin contents in mulberry (Morus spp.) leaves: effects of sample preparation, cultivar, leaf age and tea processing. Food Chem. 128, 415–419. doi: 10.1016/j.foodchem.2011.03.045
Quettier-Deleu, C., Gressier, B., Vasseur, J., Dine, T., Brunet, C., Luyckx, M., et al. (2000). Phenolic compounds and antioxidant activities of buckwheat (Fagopyrum esculentum Moench) hulls and flour. J. Ethnopharmacol. 72, 35–42. doi: 10.1016/S0378-8741(00)00196-3
Ranieri, A., Castagna, A., and Soldatini, G. F. (2000). Differential stimulation of ascorbate peroxidase isoforms by ozone exposure in sunflower plants. J. Plant Physiol. 156, 266–271. doi: 10.1016/S0176-1617(00)80316-8
Reiter, R., Tan, D. X., Rosales-Corral, S., and Manchester, C. L. (2013). The universal nature, unequal distribution and antioxidant functions of melatonin and its derivatives. Mini Rev. Med. Chem. 13, 373–384. doi: 10.2174/138955713804999810
Ren, J., Ye, J., Yin, L., Li, G., Deng, X., and Wang, S. (2020). Exogenous melatonin improves salt tolerance by mitigating osmotic, ion, and oxidative stresses in maize seedlings. Agronomy 10, 663. doi: 10.3390/agronomy10050663
Rhaman, M. S., Imran, S., Rauf, F., Khatun, M., Baskin, C. C., Murata, Y., et al. (2020). Seed priming with phytohormones: An effective approach for the mitigation of abiotic stress. Plan. Theory 10, 37. doi: 10.3390/plants10010037
Sagner, S., Kneer, R., Wanner, G., Cosson, J. P., Deus-Neumann, B., and Zenk, M. H. (1998). Hyperaccumulation, complexation and distribution of nickel in Sebertia acuminata. Phytochemistry 47, 339–347. doi: 10.1016/S0031-9422(97)00593-1
Sahari, M. A., Mehrafarin, A., and Naghdi, B. H. (2016). Changes of trigonelline, nicotinic acid and proline content in early growth stages of Trigonella foenum-graecum L. under saline condition. J. Medicinal Plants. 57, 47–55.
Scebba, F., Sebastiani, L., and Vitagliano, C. (1998). Changes in activity of antioxidative enzymes in wheat (Triticum aestivum) seedlings under cold acclimation. Physiol. Plant. 104, 747–752. doi: 10.1034/j.1399-3054.1998.1040433.x
Sharif, R., Xie, C., Zhang, H., Arnao, M. B., Ali, M., Ali, Q., et al. (2018). Melatonin and its effects on plant systems. Molecules 23:2352. doi: 10.3390/molecules23092352
Siddiqui, M. H., Alamri, S., Al-Khaishany, M. Y., Khan, M. N., Al-Amri, A., Ali, H. M., et al. (2019). Exogenous melatonin counteracts NaCl-induced damage by regulating the antioxidant system, proline and carbohydrates metabolism in tomato seedlings. Int. J. Mol. Sci. 20:353. doi: 10.3390/ijms20020353
Siddiqui, M. H., Alamri, S., Alsubaie, Q. D., and Ali, H. M. (2020a). Melatonin and gibberellic acid promote growth and chlorophyll biosynthesis by regulating antioxidant and methylglyoxal detoxification system in tomato seedlings under salinity. J. Plant Growth Regul. 39, 1488–1502. doi: 10.1007/s00344-020-10122-3
Siddiqui, M. H., Alamri, S., Khan, M. N., Corpas, F. J., Al-Amri, A. A., Alsubaie, Q. D., et al. (2020b). Melatonin and calcium function synergistically to promote the resilience through ROS metabolism under arsenic-induced stress. J. Hazard. Mater. 398:122882. doi: 10.1016/j.jhazmat.2020.122882
Stewart, R. R., and Bewley, J. D. (1980). Lipid peroxidation associated with accelerated aging of soybean axes. Plant Physiol. 65, 245–248. doi: 10.1104/pp.65.2.245
Sytar, O., Kumari, P., Yadav, S., Brestic, M., and Rastogi, A. (2019). Phytohormone priming: regulator for heavy metal stress in plants. J. Plant Growth Regul. 38, 739–752. doi: 10.1007/s00344-018-9886-8
Tan, D. X., Reiter, R. J., Manchester, L. C., Yan, M. T., El-Sawi, M., Sainz, R. M., et al. (2002). Chemical and physical properties and potential mechanisms: melatonin as a broad spectrum antioxidant and free radical scavenger. Curr. Top. Med. Chem. 2, 181–197. doi: 10.2174/1568026023394443
Tiwari, R. K., Lal, M. K., Naga, K. C., Kumar, R., Chourasia, K. N., Subhash, S., et al. (2020). Emerging roles of melatonin in mitigating abiotic and biotic stresses of horticultural crops. Sci. Hortic. 272:109592. doi: 10.1016/j.scienta.2020.109592
Upadhyay, S., Phukan, U. J., Mishra, S., and Shukla, R. K. (2014). De novo leaf and root transcriptome analysis identified novel genes involved in steroidal sapogenin biosynthesis in Asparagus racemosus. BMC Genomics 15, 746. doi: 10.1186/1471-2164-15-746
Vaidya, K., Ghosh, A., and Kumar, V. (2013). De novo transcriptome sequencing in Trigonella foenum-graecum L. to identify genes involved in the biosynthesis of diosgenin. Plant Genome 6, 1–11. doi: 10.3835/plantgenome2012.08.0021
Wang, M., Zheng, Q., Shen, Q., and Guo, S. (2013). The critical role of potassium in plant stress response. Int. J. Mol. Sci. 14, 7370–7390. doi: 10.3390/ijms14047370
Wang, Y., Gu, W., Meng, Y., Xie, T., Li, L., Li, J., et al. (2017). γ-Aminobutyric acid imparts partial protection from salt stress injury to maize seedlings by improving photosynthesis and upregulating osmoprotectants and antioxidants. Sci. Rep. 7, 1–13. doi: 10.1038/srep43609
Wani, S. A., and Kumar, P. (2018). Fenugreek: A review on its nutraceutical properties and utilization in various food products. J. Saudi Soc. Agric. Sci. 17, 97–106. doi: 10.1016/j.jssas.2016.01.007
Weeda, S., Zhang, N., Zhao, X., Ndip, G., Guo, Y., Buck, G. A., et al. (2014). Arabidopsis transcriptome analysis reveals key roles of melatonin in plant defense systems. PLoS One 9:e93462. doi: 10.1371/journal.pone.0093462
Xalxo, R., and Keshavkant, S. (2019). Melatonin, glutathione and thiourea attenuates lead and acid rain-induced deleterious responses by regulating gene expression of antioxidants in Trigonella foenum graecum L. Chemosphere 221, 1–10. doi: 10.1016/j.chemosphere.2019.01.029
Yadu, B., Chandrakar, V., Meena, R. K., Poddar, A., and Keshavkant, S. (2018). Spermidine and melatonin attenuate fluoride toxicity by regulating gene expression of antioxidants in Cajanus cajan L. J. Plant Growth Regul. 37, 1113–1126. doi: 10.1007/s00344-018-9786-y
Yang, L., Wen, K. S., Ruan, X., Zhao, Y. X., Wei, F., and Wang, Q. (2018a). Response of plant secondary metabolites to environmental factors. Molecules 23, 762. doi: 10.3390/molecules23040762
Yang, X. L., Xu, H., Li, D., Gao, X., Li, T. L., and Wang, R. (2018b). Effect of melatonin priming on photosynthetic capacity of tomato leaves under low-temperature stress. Photosynthetica 56, 884–892. doi: 10.1007/s11099-017-0748-6
Ye, J., Wang, S., Deng, X., Yin, L., Xiong, B., and Wang, X. (2016). Melatonin increased maize (Zea mays L.) seedling drought tolerance by alleviating drought-induced photosynthetic inhibition and oxidative damage. Acta Physiol. Plant. 38:48. doi: 10.1007/s11738-015-2045-y
Yu, Y., Wang, A., Li, X., Kou, M., Wang, W., Chen, X., et al. (2018). Melatonin-stimulated triacylglycerol breakdown and energy turnover under salinity stress contributes to the maintenance of plasma membrane H+–ATPase activity and K+/Na+ homeostasis in sweet potato. Front. Plant Sci. 9:256. doi: 10.3389/fpls.2018.00256
Zahedi, S., Hosseini, M., Abadía, M. S., and Marjani, M. (2020). Melatonin foliar sprays elicit salinity stress tolerance and enhance fruit yield and quality in strawberry (Fragaria× ananassa Duch.). Plant Physiol. Biochem. 149, 313–323. doi: 10.1016/j.plaphy.2020.02.021
Zamani, Z., Amiri, H., and Ismaili, A. (2020). Improving drought stress tolerance in fenugreek (Trigonella foenum-graecum) by exogenous melatonin. Plant Biosystems Int. J. Dealing Aspects Plant Biol. 154, 643–655. doi: 10.1080/11263504.2019.1674398
Zeng, L., Cai, J., Li, J., Lu, G., Li, C., Fu, G., et al. (2018). Exogenous application of a low concentration of melatonin enhances salt tolerance in rapeseed (Brassica napus L.) seedlings. Int. Agri. 17, 328–335. doi: 10.1016/S2095-3119(17)61757-X
Zhang, H., Zhang, N., Yang, R., Wang, L., Sun, Q., Li, D., et al. (2014). Melatonin promotes seed germination under high salinity by regulating antioxidant systems, ABA and GA4 interaction in cucumber (Cucumis sativus L.). J. Pineal Res. 57, 269–279. doi: 10.1111/jpi.12167
Zhang, M., He, S., Zhan, Y., Qin, B., Jin, X., Wang, M., et al. (2019). Exogenous melatonin reduces the inhibitory effect of osmotic stress on photosynthesis in soybean. PLoS One 14:e0226542. doi: 10.1371/journal.pone.0226542
Zhang, T., Shi, Z., Zhang, X., Zheng, S., Wang, J., and Mo, J. (2020). Alleviating effects of exogenous melatonin on salt stress in cucumber. Sci. Hortic. 262:109070. doi: 10.1016/j.scienta.2019.109070
Zhao, D., Yu, Y., Shen, Y., Liu, Q., Zhao, Z., Sharma, R., et al. (2019). Melatonin synthesis and function: evolutionary history in animals and plants. Front. Endocrinol. 10:249. doi: 10.3389/fendo.2019.00249
Zhao, G., Zhao, Y., Yu, X., Kiprotich, F., Han, H., Guan, R., et al. (2018). Nitric oxide is required for melatonin-enhanced tolerance against salinity stress in rapeseed (Brassica napus L.) seedlings. J. Mol. Sci. 19:1912. doi: 10.3390/ijms19071912
Zhou, B., Guo, Z., Xing, J., and Huang, B. (2005). Nitric oxide is involved in abscisic acid-induced antioxidant activities in Stylosanthes guianensis. J. Exp. Bot. 56, 3223–3228. doi: 10.1093/jxb/eri319
Zhou, N., Guo, J. F., Yang, L. Y., Xu, P., and Jiang, B. (2010). Determination of Diosgenin in Paris polyphylla var. yunnanensis at different collecting time by HPLC. Chin. J. Exp. Tradit. Med. Formulae 18, 54–56.
Keywords: salinity stress, melatonin, physiological changes, fenugreek and diosgenin
Citation: Mohamadi Esboei M, Ebrahimi A, Amerian MR and Alipour H (2022) Melatonin confers fenugreek tolerance to salinity stress by stimulating the biosynthesis processes of enzymatic, non-enzymatic antioxidants, and diosgenin content. Front. Plant Sci. 13:890613. doi: 10.3389/fpls.2022.890613
Edited by:
Lauren A. E. Erland, University of British Columbia, Okanagan Campus, CanadaReviewed by:
Manzer H. Siddiqui, King Saud University, Saudi ArabiaProtiva Rani Das, Virginia Tech, United States
Copyright © 2022 Mohamadi Esboei, Ebrahimi, Amerian and Alipour. This is an open-access article distributed under the terms of the Creative Commons Attribution License (CC BY). The use, distribution or reproduction in other forums is permitted, provided the original author(s) and the copyright owner(s) are credited and that the original publication in this journal is cited, in accordance with accepted academic practice. No use, distribution or reproduction is permitted which does not comply with these terms.
*Correspondence: Amin Ebrahimi, YW1pbmVicmFoaW1pQHNoYWhyb29kdXQuYWMuaXI=,